- State Key Laboratory Breeding Base of Green Pesticide and Agricultural Bioengineering, Key Laboratory of Green Pesticide and Agricultural Bioengineering, State-Local Joint Laboratory for Comprehensive Utilization of Biomass, Center for R&D of Fine Chemicals, Ministry of Education, Guizhou University, Guiyang, China
Heterogeneous catalytic conversion of lignocellulosic components into valuable chemicals and biofuels is one of the promising ways for biomass valorization, which well meets green chemistry metrics, and can alleviate environmental and economic issues caused by the rapid depletion of fossil fuels. Among the identified biomass derivatives, furfural (FF) and 5-hydroxymethylfurfural (HMF) stand out as rich building blocks and can be directly produced from pentose and hexose sugars, respectively. In the past decades, much attention has been attracted to the selective hydrogenation of FF and 5-hydroxymethylfurfural using various heterogeneous catalysts. This review evaluates the recent progress of developing different heterogeneous catalytic materials, such as noble/non-noble metal particles, solid acids/bases, and alkali metal salts, for the efficient reduction of bio-based furanic aldehydes to alcohols. Emphasis is laid on the insights and challenges encountered in those biomass transformation processes, along with the focus on the understanding of reaction mechanisms to clarify the catalytic role of specific active species. Brief outlook is also made for further optimization of the catalytic systems and processes for the upgrading of biofuranic compounds.
Introduction
Selective conversion of reproducible bio-resources into fuels and valuable chemicals has caused great attention because of the rapid economic development of world accompanying with the ever-increasing demand for energy from fossil resources (Li et al., 2018a,b; He et al., 2019a). In this regard, lignocellulose, one of the most abundant and inexpensive carbon sources, is comprised of crucial polymers including cellulose, hemicellulose, and lignin. Among of them, cellulose and hemicellulose can be easily converted into biofuels and platform molecules by hydrolysis and subsequent reactions (Petrus and Noordermeer, 2006; Corma et al., 2007; Serrano-Ruiz et al., 2011; Sankar et al., 2012; Besson et al., 2013; Cai et al., 2014a; Climent et al., 2014; Liu and Zhang, 2015; De et al., 2016a,b; Li et al., 2016b; Zhou and Zhang, 2016).
5-Hydroxymethylfurfural (HMF) and furfural (FF), known as significant platform molecules, are obtained by hydrolysis/dehydration of cellulose and hemicellulose via the specific catalysis (Wang et al., 2018). FF can be converted into many useful chemicals via hydrogenation (Seo and Chon, 1981; Sitthisa and Resasco, 2011; Chen et al., 2012; Hronec et al., 2012), such as tetrahydrofurfuryl alcohol, 1,5-pentanediol, furan, 2-methylfuran, cyclopentanone, and furfuryl alcohol(FFA), as shown in Figure 1. FFA has received much attention due to its wide range of applications. For example, FFA can serve as low-cost raw material to synthesize lysine, ascorbic acid, plastics, common resins, and lubricants (Chen et al., 2002; Khan et al., 2011; Yan et al., 2014; Yuan et al., 2015a; Shirvani et al., 2018). In addition, FFA is widely used for the preparation of cast resins via cross-linked polymerization with itself and other compounds, such as FF, formaldehyde, phenolic compounds, and urea. Besides showing corrosion and solvent resistance, the resulting resins have excellent chemical, thermal and mechanical properties (Barr and Wallon, 1971).
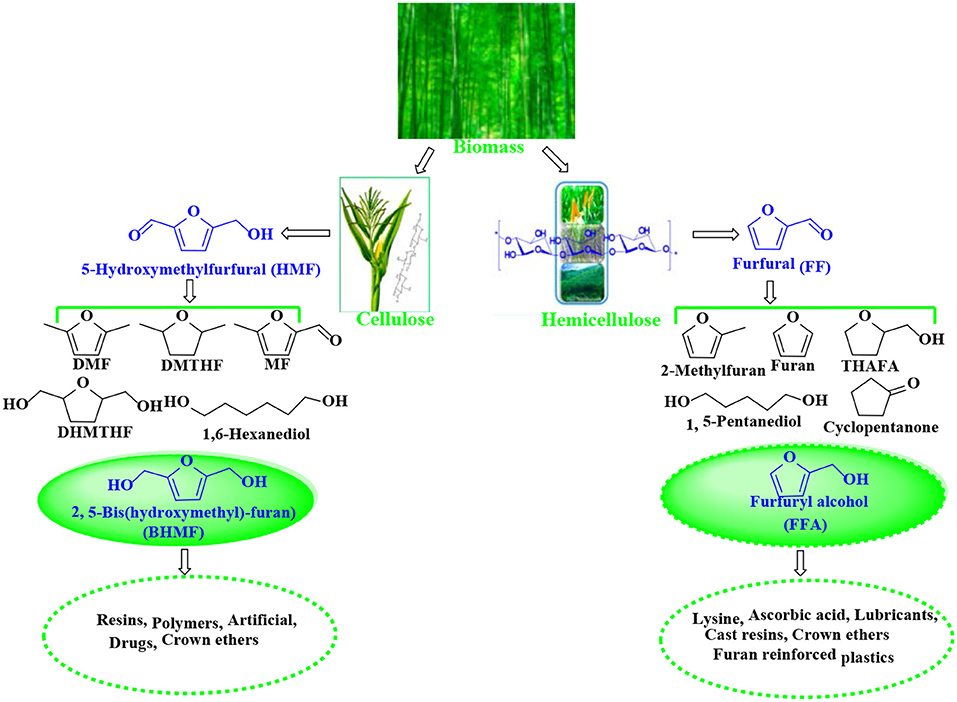
Figure 1. Catalytic upgrading of biomass-based HMF and FF into various value-added products. THAFA, tetrahydrofurfuryl alcohol; FFA, furfuryl alcohol; DMF, 2,5-dimethylfuran; DMTHF, 2,5-dimethoxytetrahydrofuran; MF, 5-methylfurfural; DHMTHF, 2,5-dihydroxymethyl-tetrahydrofuran; BHMF, 2,5-bis(hydroxymethyl)-furan.
Similar to FFA, 2,5-bis(hydroxymethyl)-furan (BHMF) is derived from the hydrogenation of 5-hydroxymethylfurfural (HMF) that can be originated from hexose sugars such fructose, glucose, and cellulose by hydrolysis/dehydration (Román-Leshkov et al., 2006; Zhao et al., 2007; Hu et al., 2009; Qi et al., 2009; Chen et al., 2014a) and is known as a key platform molecule for the production of various valuable products, such as 2,5-dimethylfuran (DMF), 2,5-dihydroxymethyl-tetrahydrofuran (DHMTHF), 2,5-dimethoxytetrahydrofuran (DMTHF), and 5-methylfurfural (MF) (Román-Leshkov et al., 2007; Kwon et al., 2013; Audemar et al., 2014; Hu et al., 2014; Revunova and Nikonov, 2015; Seemala et al., 2017, 2018; Wang T. et al., 2017). As one of the hopeful bio-based downstream products, BHMF can be used to prepare a variety of functional materials (e.g., polymers, resins, crown ethers, and rayon) and as universal brackets for the preparation of drugs and related bioactive compounds (Han et al., 2016).
Both homogeneous and heterogeneous catalysts can be used for biomass upgrading. The active site of the homogeneous catalyst is in the same phase as the reactant, and can effectively interact with the reaction substrate, usually resulting in a higher turnover frequency (TOF) rates than that of the heterogeneous catalyst. However, homogeneous catalysts are not widely used due to many disadvantages, such as high toxicity, corrosion, difficulty in separation and purification, and inefficient reusability (Sheldon, 2005). Heterogeneous catalysts are widely used in biomass upgrading reactions in view of their many advantages, as shown in Figure 2. For example, heterogeneous catalytic systems are less corrosive. Another advantage of heterogeneous catalysts is that they are highly stable to harsh reaction conditions (high temperature and pressure) (Kokel et al., 2017). What's more, heterogeneous solid catalysts can be easy to separate and highly reusable in continuous catalytic cycles, making the process cost-effective and more sustainable. Over the past several decades, different types of heterogeneous catalysts and advanced methods have been explored to catalyze the hydrogenation of bio-based furanic aldehydes to alcohols (Chen et al., 2014a). Several research groups are currently studying this reaction using various noble metals, such as Pd (Audemar et al., 2014; Li et al., 2018c), Pt (An et al., 2013; Shi et al., 2016), and Ru (Nishimura et al., 2014; Yuan et al., 2015b), non-noble metals, such as Cu (Lesiak et al., 2014; Upare et al., 2018) and ferrous metals (Fe, Ni, Co) (Yao et al., 2014; Yu et al., 2015; Halilu et al., 2016), solid acid-base catalysts, and alkali metal salt catalysts [e.g., K2CO3 (Long et al., 2018), Cs2CO3 (Long et al., 2019) and KF (Wu et al., 2018; Zhao et al., 2018)] for the catalytic upgrading of biomass-derived furanic aldehydes to alcohols.
Several important review articles have been reported on the development of heterogeneous catalysts for biomass upgrading reactions. For instance, Lin and Huber (2009) provided a review on the pivotal role of heterogeneous catalysis in biomass upgrading. In another review, Yan et al. (2014) have discussed heterogeneous catalysts for the hydrogenation of FF into fuel additives and value-added chemicals by advanced catalytic hydrogenation technologies. Gabriëls et al. (2015) reported the applications of homogeneous, heterogeneous, and combined homogeneous and heterogeneous catalytic systems in Guerbet chemistry. Gilkey and Xu (2016) provided a review on the upgrading of biomass-derived feedstocks through heterogeneous catalytic transfer hydrogenation (CTH), and discussed the challenges and opportunities of establishing CTH in the effective production of biofuels and renewable chemicals. Recently, Sudarsanam et al. (2018) provided a review on developing functional heterogeneous catalysts, such as carbon materials, metal-organic skeletons, solid-phase ionic liquids, and magnetic ferric oxide, to efficiently upgrade biomass. Herein, this review provides a critical review on catalytic upgrading of biomass-derived furanic aldehydes to alcohols by using various heterogeneous catalysts. The aim of this review is to provide a comprehensive overview of the production of BHMF or FFA from the hydrogenation of 5-hydroxymethylfurfural or FF. This review evaluates the recent progress of developing different heterogeneous catalytic materials, such as noble/non-noble metal particles, solid acids/bases, and alkali metal salts, for the efficient reduction of bio-based furanic aldehydes to alcohols. Emphasis is laid on the insights and challenges encountered in those biomass transformation processes.
Catalytic Strategies Toward Furanic Platforms
There have been many reports on the different reaction mechanisms for the production of FF from xylose (Marcotullio and De Jong, 2010; Yang et al., 2012), but most studies using heterogeneous catalysts are based on a cyclized dehydration mechanism that gradually releases three molecules of water (Marcotullio and De Jong, 2010). This mechanism typically uses Brønsted acid catalysts, while some modifications have been made to the Lewis acid catalysts to produce FF. As shown in Scheme 1, xylose is isomerized to xylulose in the presence of Lewis acid or base sites, which has been confirmed by several experimental studies (Takagaki et al., 2010; Choudhary et al., 2011; Tuteja et al., 2012). For example, Choudhary et al. (2011) reported that Lewis acid zeolite primarily converts xylose to xylulose with almost no FF formed, while in the presence of Brønsted acid catalysts, such as HCl and Amberlyst-15, the intermediate xylulose can be rapidly converted to FF. The properties of heterogeneous catalysts, such as acidity (acid type, strength, and amount), structure (pore size and surface area), hydrophobicity and stability, can significantly affect catalytic performance. For acidic species, the Lewis acid site can transfer the reaction pathway to the xylose-xylulose-FF route, which is faster than the direct reaction of xylose to the FF route by the Brønsted acid sites. In the presence of both Brønsted and Lewis acid sites, it is faster to catalyze the dehydration of xylulose to produce FF and acquire a higher yield of FF (Li et al., 2016d). Similar to the formation mechanism of FF, 5-hydroxymethylfurfural is obtained by dehydration of glucose or fructose or by hydrolysis of cellulose in the presence of both Lewis and Brønsted acid catalysts (Scheme 1) (Zakrzewska et al., 2011; Cai et al., 2014b; Svenningsen et al., 2018).
Catalytic Hydrogenation of Biofuranic Aldehydes to Alcohols
Non-precious Metal Particles
Cu-Based Catalysts
Hydrogenation of FF to FFA is usually carried out in a gas phase or a liquid phase process. Compared to gas phase hydrogenation, gas phase hydrogenation is generally preferred because it can be carried out under normal pressures. The gas phase catalytic process was first reported in 1929 by the use of copper supported as a catalyst in asbestos (Ricard and Guinot, 1929). Copper chromite catalysts have been widely used in various industrial processes, such as the decomposition or dehydration of the alcohol and the partial hydrogenation of vegetable oils and fatty acids (Rao et al., 1997). However, the main disadvantage of Cu-Cr catalysts is their high toxicity (Li et al., 2006). The final disposal of the catalyst causes serious environmental pollution (Huang et al., 2007). In order to decrease the chromium content of the Cr-containing catalyst while showing comparable catalytic activity, Huang et al. studied the application of TiO2 supported copper chromite catalyst in the selective hydrogenation of FFA, and the conversion of FF was 100% and the yield of FFA was 79% under the reaction conditions of 140°C for 2 h (Huang et al., 2007). The Cu-Cr catalyst is industrially used for the hydrogenation of FF at high temperature and pressure to produce FFA with moderate activity and selectivity (Baijun et al., 1998; Wu et al., 2005; Li et al., 2006). Burch et al. (1990) reported that ZnO had an important supporting role and could act as a reservoir of atomic hydrogen to promote hydrogen overflow in the reaction. It is known that the role of ZnO is to increase the dispersion of Cu, which can improve the performance of the catalyst (Fei et al., 2004; Pillai and Deevi, 2006). Therefore, in order to increase the conversion and selectivity of FF hydrogenation to FFA, Zn is added to the Cu(3):Cr(1) catalyst. Zirconium is known to enhance the acidity of the catalyst and also to increase the selectivity of the hydrogenation reaction (Pillai and Deevi, 2006; Liu et al., 2012). Zr was thus added into the Cu(3):Zn(2):Cr(1) catalyst to increase the selectivity of FFA formation. The results showed that Cu:Zn:Cr:Zr (3:2:1:3) was the optimal catalyst for the reduction of FF to FFA. Under the reaction conditions of 170°C and 3.5 h, the conversion of FF was 100% and selectivity reached 96% (Sharma et al., 2013).
The high toxicity of the chromium-containing catalysts promotes the development of new Cr-free catalytic systems. New restrictions now prevent the used chromite catalysts from being deposited in landfills, providing the impetus for the exploration of new alternative Cu catalysts free of chromium. Therefore, many non-chromium catalysts have been developed for the hydrogenation of FF and 5-hydroxymethylfurfural in the gas phase and liquid phase in the presence of different catalysts, as summarized in Table 1. To date, various single metal and bimetallic catalysts have been prepared, which are supported on relatively inert materials, such as silica and alumina. Among Cu-based catalysts, the main reason for using promoters and developing the multi-metallic catalysts was the utilization of a multi-component catalyst system to improve the catalyst performance, comparing to the single metal counterparts. Wang Y. et al. (2017) reported the conversion of FF was 98.7% and selectivity reached 96.4% by the catalyst CuCo0.4/C-873, but the selectivity of FFA and the conversion of FF were only 81.3 and 46.6%, respectively, with Cu/C-873 catalyst, indicating the promoting effect of cobalt. The increase in furfuryl alcohol selectivity may be due to the doping of cobalt resulting in better dispersion of the metal particles, which allows for good regulation of the structure of the active site. Selectively activating the C = O bond of the FF and the decomposition of hydrogen is a necessary condition for the hydrogenation of FF to furfuryl alcohol. In the catalytic system of Cu/C-873, metallic copper acts as an active site for activating the C = O bond and dissociating hydrogen molecules. However, copper with relatively large particle size is less active during hydrogen activation (Takagaki et al., 2010; Vargas-Hernández et al., 2014). By doping with cobalt, smaller and more easily dispersible copper and cobalt particle sizes can be found in the bimetallic CuCo/C-873 catalyst, which will contribute to generating more active reactive sites (Li et al., 2016d). Due to the presence of cationic species, in addition, the interaction of cobalt oxide (CoOx) with the C = O group of FF is more intense (Rao et al., 1997). Therefore, more CoOx sites can be obtained in a catalyst with high cobalt content and used to activate more C = O groups. In addition, metallic cobalt is more active than copper in terms of hydrogen dissociation. Although the metal cobalt alone exists, the C = O bond and the furan ring can be activated simultaneously. In contrast, the synergistic action of copper and cobalt prevents the activation of the furan ring, thus exhibiting the pronounced selectivity toward the partially hydrogenated product. Therefore, the catalytic performance of the bimetallic CuCo/C-873 catalyst is better than that of Cu/C-873 (Wang Y. et al., 2017). Unfortunately, the bimetallic CuCo/C-873 catalyst has a significant loss in catalytic activity over four consecutive cycles. Similarly, Chen et al. reported 76.8% BHMF selectivity and 70% 5-hydroxymethylfurfural conversion over catalyst Cu/C but only 82.9% BHMF selectivity and 63.9% conversion over CuZn/C in aqueous hydrogenation of 5-hydroxymethylfurfural to BHMF, illustrating the promoting effect of zinc (Chen et al., 2017). In addition to the above metals, the doping of metals, such as Ni, Fe, Mg, Al, etc. also improves the activity, selectivity, and the stability of copper-based catalysts for liquid-phase catalytic reactions (Villaverde et al., 2013; Yan et al., 2013b; Khromova et al., 2016).
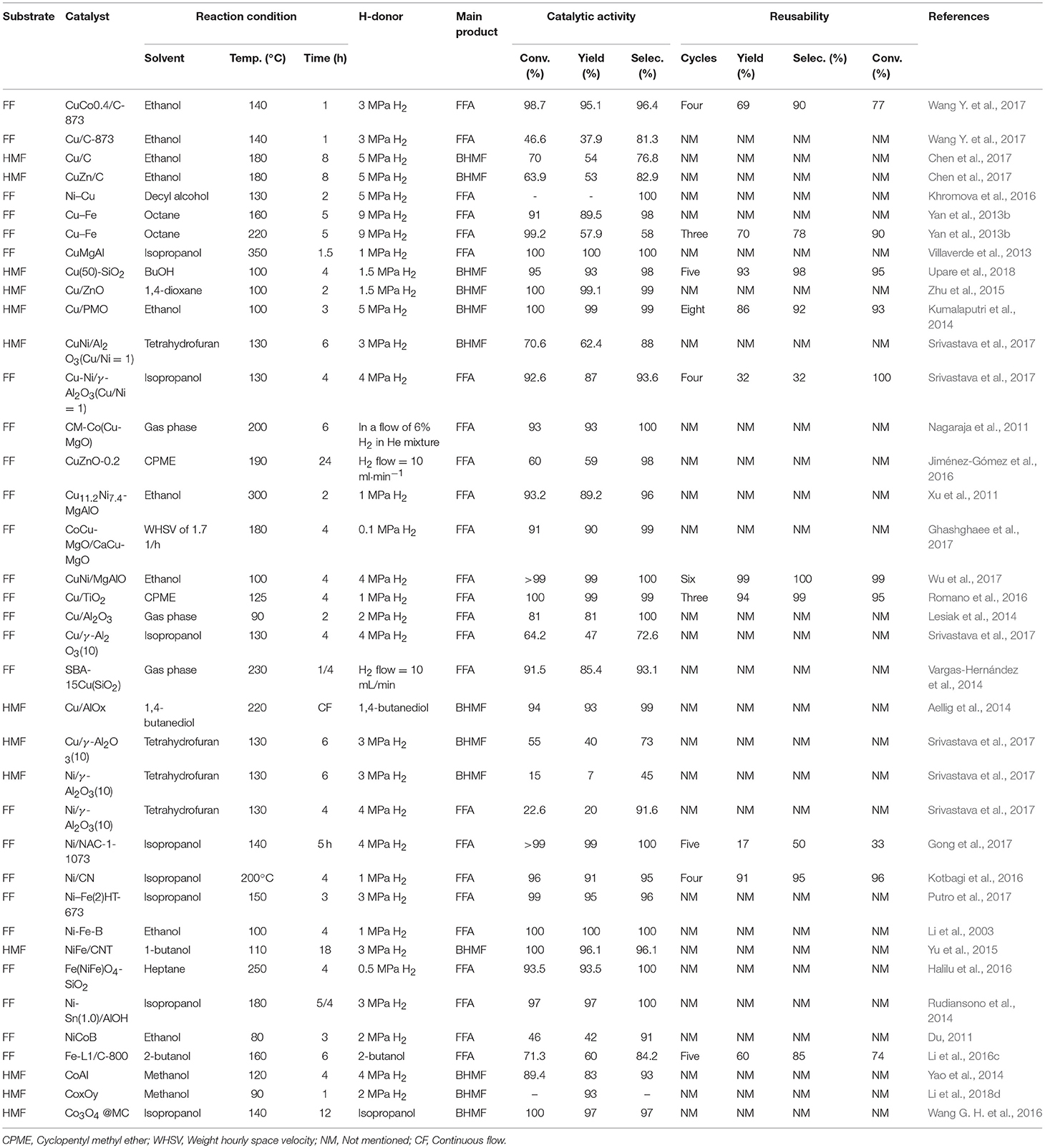
Table 1. Hydrogenation of FF and 5-hydroxymethylfurfural catalyzed by different non-precious metallic catalysts.
The addition of metal or non-metal oxides is also beneficial to increase the catalytic activity and stability of Cu-based catalysts. For example, Nagaraja et al. (2011) used the Cu-MgO-Co to catalyze the hydrogenation of FF, and the conversion and selectivity of FFA reached more than 90% at 225°C after 6 h. This may result from the higher surface and smaller crystallite size on the surface of the support. (Jiménez-Gómez et al., 2016) also reported that the use of amphoteric oxides, such as ZnO could change the electron density and dispersion of metallic copper, which can improve catalytic activity and resistance to deactivation (Dong et al., 2015). In the presence of Cu-ZnO, the conversion of FF and the selectivity of FFA were 93 and 82%, respectively (Jiménez-Gómez et al., 2016). Xu et al. reported that Cu was added to the hydrotalcite precursor layer by coprecipitation method, and the solid was calcined to prepare a Cr-free Cu-based catalyst, namely Cu11.2Ni4.7-MgAlO, in which the highly dispersed metal particles can well-contact with hydrogen to promote FF hydrogenation. Gratifyingly, this catalytic system gave FF conversion and FFA selectivity of 93.2 and 89.2% at 300°C, respectively (Xu et al., 2011). Ghashghaee et al. (2017) reported a novel method based on a combination of coprecipitation and hydrothermal methods for the preparation of a series of Cu-MgO catalysts containing various promoters (Co and Ca) and certain morphology. Ca is a basic promoter, and an appropriate amount of Ca can improve the thermal stability of the Cu-based catalyst in the hydrogenation reaction (Burch et al., 1990). According to previous reports, Co promoters can increase the selectivity of the reaction process by decreasing unwanted by-products (Reddy et al., 2007). Under the positive influence of two promoters (Co and Ca), the selectivity of FFA was over 99% (Ghashghaee et al., 2017). In addition to other metals, such as nickel, Cobalt, iron and magnesium species, the selectivity of FFA was relatively high (Villaverde et al., 2013; Yan et al., 2013b; Khromova et al., 2016; Wang Y. et al., 2017; Wu et al., 2017). The Cu supported on relatively inert materials, such as silica, ceria, zinc oxide, and alumina were capable of catalyzing the hydrogenation of FF to FFA, showing good performance with respect to the conversion of FF and the selectivity toward FFA (Sitthisa et al., 2011a; Lesiak et al., 2014; Vargas-Hernández et al., 2014; Srivastava et al., 2015; Romano et al., 2016; Du et al., 2018; Jiménez-Gómez et al., 2018; Yang et al., 2018).
Upare et al. (2018) reported the production of BHMF from 5-hydroxymethylfurfural using Cu(50)-SiO2, which were prepared with different Cu loading by precipitation-deposition method, achieving a BHMF yield of 93% and a 95% conversion of 5-hydroxymethylfurfural in BuOH at 100°C for 4 h under 1.5 MPa H2. The catalyst could be reused for five cycles with consistent activity. (Zhu et al., 2015) later utilized a copper-zinc oxide to catalyze the hydrogenation of 5-hydroxymethylfurfural to BHMF, achieving a 99.1% yield and a 100% 5-hydroxymethylfurfural conversion at 100°C for 2 h in presence of 1.5 MPa H2. For catalytic hydrogenation of 5-hydroxymethylfurfural, Cu-ZnO catalyst has high activity and selectivity, which can be attributed to high dispersion of Cu particles produced by strong interaction between copper and zinc oxide, and synergistic effect between surface sites of metal copper and weakly acidic sites from zinc oxide. (Kumalaputri et al., 2014) utilized a hydrotalcite-derived copper catalyst to catalyze the hydrogenation of 5-hydroxymethylfurfural, giving a BHMF yield of 99% and a 5-hydroxymethylfurfural conversion of 100%. Unfortunately, because the carbon species deposited on the catalyst surface, the catalyst activity was maintained for 3 cycles, and then the BHMF selectivity gradually decreased to 61, 53, 38, and 19% in the fourth to seventh cycles, respectively. Aellig et al. (2014) reported the AlOx supported Cu catalyst, which was synthesized by direct reduction of Cu-Al hydrotalcite precursor, for the hydrogenation of 5-hydroxymethylfurfural to BHMF. The AlOx supported copper showed better catalytic performance as compared with aluminum oxide supported one (Srivastava et al., 2017), achieving a 93% BHMF yield and 100% 5-hydroxymethylfurfural conversion at 100°C by using 1,4-butanediol as H-donor, demonstrating that the reduction temperature of the catalyst had an important effect on the catalytic activity, and a higher reduction temperature resulted in higher activity but the catalyst was less stable (Aellig et al., 2014).
Density functional theory (DFT) calculations and infrared (IR) spectroscopy techniques have been employed to provide explanations for the adsorbent patterns of different intermediates and reasonable reaction pathways. As one of the representative examples, Resasco's group proposed a Cu-based catalyst to catalyze the reduction of FF to FFA. They proposed that the adsorption of FF preferentially passes through the η1(O)-aldehyde binding mode, as shown in Scheme 2 (Sitthisa et al., 2011b). The FF molecule is perpendicular to the surface of the catalyst, and the aromatic ring undergoes a net repulsion due to the overlap of the three-dimensional band of the surface Cu atoms with the aromatic furan ring. Thus, the reaction can be carried out by an alkoxide (H attack on the C atom of the carbonyl group) or a hydroxyalkyl group (H addition to the O atom of the carbonyl group) intermediate. The latter mechanism is preferred because it has a lower activation energy barrier, which can be explained by the stabilizing effect of the aromatic furan ring on the hydroxyalkyl intermediate (Chen et al., 2014b).
Ferrous Metal (Fe, Ni, Co)-Based Catalysts
Among non-precious metals, metallic nickel is distinguished for its remarkable hydrogenation capacity. However, there are very few applications in the reduction of 5-hydroxymethylfurfural and FF to BHMF or furfuryl alcohol because of the strong interaction between the furan ring and the Ni site, resulting in the easy opening of the furan ring, which reduces the selectivity of BHMF and FFA. Merat et al. (1990) reported the use of a nickel catalyst to catalyze the hydrogenation of FF, and the main product was FFA at 130°C. The selective reduction ability of the single metal Ni catalysts in the reduction of FF to FFA is relatively poor because they have too high hydrogenation activity. Therefore, it is desirable to modify the nickel to increase the selective reduction of the aldehyde group to escape the occurrence of undesirable side reactions. The following three methods are a large number of previous studies: (i) using oxides, such as Al2O3 (Srivastava et al., 2017), SiO2 (Halilu et al., 2016) or N-doped activated carbon supports to interact strongly with nickel (Gong et al., 2017), (ii) adding oxyphilic metals, such as Fe (Li et al., 2003), Sn (Rudiansono et al., 2014), and Co (Du, 2011), and (iii) controlling the reaction conditions to restrain the opening reaction of the furan ring. For example, Srivastava et al. (2017) reported that Ni-loaded alumina was used to catalyze the hydrogenation of 5-hydroxymethylfurfural and FF with undesirable effect, resulting only into 15 and 22.6% conversion of 5-hydroxymethylfurfural and FF, respectively. Gong et al. (2017) used doped activated carbon supported metallic nickel (Ni/NAC), which was prepared by self-assembly in two-step calcination in nitrogen, to catalyze the hydrogenation of FF to FFA, giving excellent performance at 140°C for 2 h in the presence of 4 MPa H2. After consecutive five cycles, the catalytic activity of the catalyst was significantly reduced, and the conversion of FF was reduced from 99 to 33%. Kotbagi et al. (2016) reported that Ni/CN monolithic catalysts with different Ni content, which were prepared by using a one-pot co-gelation sol-gel technique, catalyzed the conversion of FF to FFA. 96% FF conversion and 95% selectivity FFA were obtained at 200°C for 4 h under 1 MPa H2. After the catalyst was reused four times, the reactivity did not decrease, indicating the preparation method of the catalyst has a great influence on the stability of the catalyst. Merging a third constituent, such as Fe, Sn, or Co, improves the catalytic activity. Iron is widely known for its abundant reserves, low price, environmental friendliness, and ease of recycling. Fe is mainly utilized as an oxophilic metal additive to enhance the reduction performance of other metals, such as copper (Yan et al., 2013b). Most importantly, the existence of oxophilic Fe as a doped additive improves the specific surface area of the catalyst, while the dosage of unsaturated Ni active sites and the distribution of Ni active sites facilitates the adsorption of C = O bonds of FF. Putro et al. (2017) adopted a simple hydrothermal method to prepare Ni-Fe alloy catalyst, and used to catalyze the reduction of FF to FFA, acquired excellent performance with a 99% FF conversion and a 95% FFA yield at 150°C for 3 h under a hydrogen pressure of 3 MPa. In order to increase the stability of the Ni-Fe catalyst, some researchers have added some supporters to the catalyst. For example, Li et al. (2003) reported the reduction of FF to FFA over the Fe-promoted Ni-B amorphous alloy catalysts (Ni-Fe-B), which was prepared by reducing mixed FeCl3 and NiCl2 with KBH4 in aqueous solution, in the liquid phase. The yield of FFA was 100% with complete conversion of FF, revealing that the strong interaction between boron and nickel accelerated the activation of the C = O bond in FF, and thereby promoted the selective formation of FFA. 5-Hydroxymethylfurfural could be selectively hydrogenated with carbon nanotube-supported bimetallic Ni-Fe (Ni-Fe/CNT) catalysts as shown by (Yu et al., 2015). A 96.1% BHMF yield and complete 5-hydroxymethylfurfural conversion were obtained under the conditions of 110°C and 3 MPa H2 for 18 h (Yu et al., 2015). Magnetic catalysts are well-known for facilitating catalyst separation with excellent catalytic activity, which is favored by many researchers. Halilu et al. (2016) reported magnetite Fe(NiFe)O4-SiO2 nanoparticles, which were synthesized by a facile co-precipitation method, catalyzed the hydrogenation of FF to FFA. Satisfactorily, this catalytic system gave FF conversion and FFA selectivity of 93.5% at 250°C for 2 h under hydrogen pressure of 0.5 MPa. Incorporating another third component, such as Sn (Rudiansono et al., 2014) or Co (Du, 2011) also increases the catalytic performances. Hydrogenation with hydrogen as a source of hydrogen has certain weaknesses, and high-pressure hydrogen often poses a safety hazard (He et al., 2018b; Long et al., 2019). Catalytic transfer hydrogenation (CTH) can be used as an alternative to reduce biomass-derived molecules, and a hydrogen donor (e.g., secondary alcohol) is used as a hydrogen source instead of molecular H2. Monometallic iron-based catalysts only exhibited low to moderate catalytic reactivity in the hydrogenation of FF. But several latest reports showed that this could be obviously promoted by C or N supporters (Li et al., 2003). Li et al. (2016c) utilized nitrogen-doped carbon-supported iron catalysts, which were prepared through simultaneous pyrolysis of metal complexes and carbon materials, for the hydrogenation of FF to furfuryl alcohol using 2-butanol as H-donor. A moderate FF conversion of 73.3% and FFA yield of 60% was obtained at 250°C. The catalyst was able to be reused for 5 times with no significant loss of catalytic activity. In addition to the above non-precious catalysts, cobalt-based catalysts were also utilized to catalyze the hydrogenation of FF and 5-hydroxymethylfurfural, giving FFA and BHMF in good yields (83–97%) (Yao et al., 2014; Wang G. H. et al., 2016; Li et al., 2018d).
Precious Metal Particles
Various noble metals and non-noble metals have been reported for the selective hydrogenation of FF and 5-hydroxymethylfurfural in the gas phase and the liquid phase. Liquid phase hydrogenation was investigated using different chromium and non-chromium catalysts (Mariscal et al., 2016). Generally, the Cu-based catalyst exhibits a remarkable selectivity for the hydrogenation of a carbonyl group, and the C-C double bond in the furan ring does not participate in the reaction. A second metal or promoter is sometimes added to improve activity or selectivity by increasing the surface area or acting as a Lewis acid site to polarize the C-O bond. However, the main drawback is that most of these catalysts cannot be reused (Wu et al., 2005). Therefore, other noble metals, such as Pd (Lesiak et al., 2014), Pt (Huang et al., 2016), Ru (Han et al., 2016), Ir (Nakagawa et al., 2014), and Au (Li et al., 2015), were added to increase their stability, where ethanol, water, ethanol, isopropyl alcohol, n-butyl alcohol, benzyl alcohol, heptane, tetrahydrofuran, or octane was used as the solvent, as shown in Table 2.
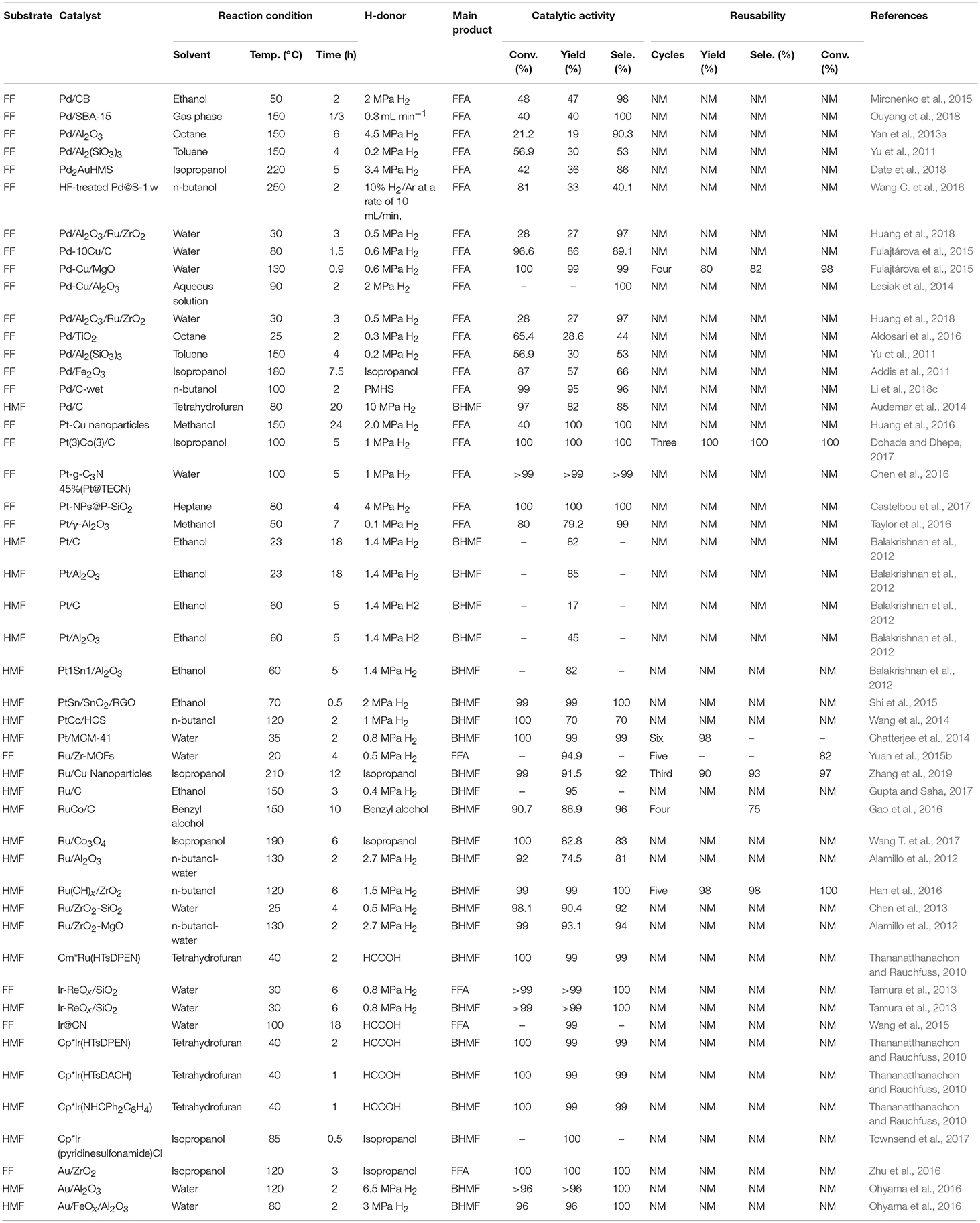
Table 2. Reduction of FF and 5-hydroxymethylfurfural catalyzed by different precious metallic catalysts.
Solvent properties (e.g., polarity) were also found to affect the interaction of the solvent with the reactants. Typically, with the increase of the polarity of the solvent, the interaction of FF with the solvent also increases. Accordingly, the yield of FFA would decrease as the adsorption of FF onto the catalyst was difficult. Among these solvents, water is the most polar solvent and should be the best medium for the reaction. Also, the product is easy to separate in this environment.
Pd-Based Catalysts
Palladium is well-known for its excellent catalytic performance in hydrogenation. Recently, different monometallic Pd-based materials were deeply studied for the hydrogenation of FF and 5-hydroxymethylfurfural. Palladium nanoparticles were deposited onto various supports, such as carbon (Audemar et al., 2014; Mironenko et al., 2015), SBA-15 silica (Ouyang et al., 2018), alumina (Yan et al., 2013a), titania (Aldosari et al., 2016), Al2(SiO3)3 (Yu et al., 2011), hexagonal mesoporous silica (Date et al., 2018), magnesium oxide (Fulajtárova et al., 2015), porous silicate (Biradar et al., 2013), and zeolite (Wang C. et al., 2016), showing good performance for the selective hydrogenation of FF and 5-hydroxymethylfurfural to FFA and BHMF, respectively. The improvement of C = O hydrogenation can be achieved by using a promoter, adjusting the particle size, changing the properties of the oxide support, and modifying the active metal with a second metal. The modification of the active metal with a second metal to form a bimetallic catalyst is an important factor in adjusting the catalytic selectivity of the C = O bond. The properties of bimetallic catalysts, such as electronic effects, synergistic effects, and alloy formation, significantly affect catalytic selectivity. In the Pd-based catalyst, the addition of a second metal, such as Cu (Fulajtárova et al., 2015) increased the hydrogenation selectivity of the various unsaturated carbonyl groups. In order to improve the performance of the catalyst, it is common to add a third metal. For example, Huang et al. (2018) added metal ruthenium to an alumina-supported palladium-based catalyst. Date et al. (2018) added metal Au to a palladium-based catalyst loaded with hexagonal mesoporous silica. In both cases, enhanced catalytic results were observed, as compared with the catalysts without doping additional metal species (Table 2, entry 5).
Although these catalytic systems have better catalytic effects, they all used hydrogen as a hydrogen source. To be noted, hydrogen is not easy to transport and store, making the experimental operation be cumbersome (Gandarias et al., 2012, 2013). In the presence of a catalyst, an alcohol is used as hydrogen donor, and FF is selectively converted to FFA by liquid-phase transfer hydrogenation (Yang et al., 2004; Nagaraja et al., 2007; Osatiashtiani et al., 2017), avoiding the use of inflammable hydrogen gas that involves complex storage and transportation process and thereby reducing the production cost of practical use. In this regard, it can solve the safety problems in the transportation process. In addition, non-corrosive alcohols can be obtained from sustainable biomass sources, and the dehydrogenation products (i.e., aldehydes or ketones) can be readily separated (Nagaraja et al., 2007; Bui et al., 2013; Jae et al., 2013). Therefore, the use of organic alcohols as hydrogen donors for the transfer hydrogenation of FF should be a more environmentally friendly alternative to conventional hydrogenation processes. Scholz et al. reported a Fe2O3-loaded Pd for the hydrogenation of FF to FFA with isopropanol as the hydrogen donor, with FFA yield and FF conversion in 57 and 87% at 180°C for 7.5 h, respectively. FFA cannot be selectively formed from FF in the presence of palladium, which on the other hand further undergoes hydrogenolysis to produce 2-methylfuran. Together with the hydrogenation reaction, FF undergoes decarbonylation to furan. As the conversion rate increased (the concentration of acetone increased accordingly), the aldol condensation product of FF and acetone 4-(furan-2-yl)but-3-en-2-one was detected. In addition, traces of furan-ring hydrogenated products, such as 2-methyltetrahydrofuran, (tetrahydrofuran-2-yl)methanol, and tetrahydrofuran were observed, indicating the high complexity of the reaction pathways (Addis et al., 2011). Unfortunately, the alcohol solvent can be further reacted with FFA by etherification to reduce its selectivity under the harsh conditions employed (Jae et al., 2013). Therefore, it is increasingly important to find an inexpensive and safe hydrogen donor. Polymethylhydrogensiloxane (PMHS), as a by-product of the silicon industry, is stable in water and air, and non-toxic and inexpensive (Addis et al., 2011). Li et al. (2018c) used PMHS as hydrogen source with Pd/C-wet to catalyze the hydrogenation of FF to FFA, giving FFA up to 95% yield at FF conversion of 99% under the reaction conditions of 15°C for 12 h. In addition to the formation of furfuryl alcohol, other substances, such as 2-methylfuran, 5-hydroxy-2-pentanone, and n-butyl levulinate were formed by appropriate control of the reaction parameters like reaction temperature and hydrosilane dosage.
Pt-Based Catalyst
Noble metal nanoparticles have proven to be effective catalysts in hydrogenation reactions (Durndell et al., 2015; Wei et al., 2015), which have been widely used in the industry. Among various noble metal nanoparticles, Pt catalyst is widely used in hydrogenation reactions due to its unique electrical and chemical properties (Chen et al., 2016). Due to complex side reactions (e.g., hydrogenolysis of C–O bonds, decarbonylation, over-hydrogenation and ring opening of furan), Pt-based catalysts are rarely used for the hydrogenation of FF and 5-hydroxymethylfurfural to FFA or BHMF (Chen et al., 2016). The physicochemical properties of the catalyst support significantly affect its liquid phase catalytic hydrogenation performance for unsaturated aldehydes and ketones (Mäki-Arvela et al., 2005). Pt-based catalysts have also been used in the FF or 5-hydroxymethylfurfural hydrogenation reaction by the addition of a suitable supporter. For example, Huang et al. (2016) using Pt-Cu nanoparticles catalyzed the hydrogenation of FF, giving the complete conversion of FF and 100% yield of FFA under the reaction conditions of 2 MPa hydrogen pressure and 150°C for 2 h. Platinum, in particular, has drawn recent attention for the hydrogenation of FF over SiO2 (Castelbou et al., 2017), γ-Al2O3 (Taylor et al., 2016), and TiO2 (Mäki-Arvela et al., 2005) oxide support. The TiO2-ZrO2 mixed oxide has a large surface area, strong surface acidity and alkalinity, high thermal stability and strong mechanical strength (Wang et al., 1983). Castelbou et al. (2017) utilized bimetallic Pt-Re supported on titanium dioxide and zirconium dioxide to catalyze the reduction of FF to FFA, with ethanol as solvent. 100% FF conversion with FFA yield of 95.7% was obtained under the reaction conditions of 130°C and 5.0 MPa hydrogen pressure (Castelbou et al., 2017). Balakrishnan et al. (2012) reported platinum, which was supported by carbon, aluminum oxide, or stannum, could catalyze the hydrogenation of 5-hydroxymethylfurfural to BHMF, and 82% yields of BHMF could be obtained with Pt1Sn1/Al2O3 at 60°C for 5 h, showing that the stannum dopant enhances its catalytic activity and selectivity for the formation of BHMF. Shi et al. (2015) prepared PtSn/SnO2/RGO was by first coating onto the surface of reduced graphene oxide (RGO) and SnO2 nanoparticles were then irradiated by microwave for a few minutes on the RGO plate. The resulting Pt3SnNPs efficiently catalyzed the hydrogenation of 5-hydroxymethylfurfural to BHMF, and a BHMF yield of 99% with a 99% conversion of 5-hydroxymethylfurfural was achieved at 2 MPa H2 under 70°C for 0.5 h, demonstrating that SnO2 can stabilize Pt nanoparticles and increase their catalytic activity.
The reused and regenerative properties of the catalyst played a crucial role in the choice of commercial applications. The catalyst was first reused without regeneration to determine if the activity of each reaction cycle was reduced. This indicated a slight decrease in the conversion of FF and 5-hydroxymethylfurfural per hydrogenation cycle. Similarly, it has recently been reported that other platinum-based bimetals, such as Pt-Fe/MWNT (Lesiak et al., 2014), Pt(3)Co(3)/C (Dohade and Dhepe, 2017), Pt-Sn/SiO2 (Liu et al., 2018), and PtCo/HCS (Wang et al., 2014) could catalyze the efficient hydrogenation of FF and 5-hydroxymethylfurfural to FFA and BHMF, respectively with activity being only slightly reduced after the repeated use. Based on this, Chatterjee et al. (2014) prepared a Pt/MCM-41 catalyst by hydrothermal method and used this catalyst to catalyze the reduction of 5-hydroxymethylfurfural to BHMF. A BHMF yield of 99% and a 100% conversion of 5-hydroxymethylfurfural was achieved at 2 MPa H2 under 35°C for 2 h. Recycling experiments showed that the catalyst could be recycled for at least six times, suggesting a moderate decrease in the activity possibly due to slight changes in the structure of the catalyst and Pt particle size.
Ru-Based Catalyst
Ruthenium-based catalysts are well-known for their excellent catalytic properties in the reduction of aromatic hydrocarbons (Cui et al., 2016), α,β-unsaturated aldehydes (Hájek et al., 2004), and furan compounds, particularly FF or 5-hydroxymethylfurfural (Nagpure et al., 2015). The monometallic Ru-based catalyst can efficiently reduce FF to furfuryl alcohol under mild conditions with temperature range and hydrogen pressure range of 20–180°C and 0.5–1.25 MPa, respectively (Table 2). The selection of the supporter becomes critical because of the particle size of ruthenium, the electron interaction of the ruthenium particles with the support, and the high reducibility of the RuOx substance, etc., which are the key factors affecting the activity of the catalysis. Many researchers have used support, such as silica (Chen et al., 2013), carbon (Gupta and Saha, 2017), metal organic framework (MOF) (Yuan et al., 2015b), aluminum oxyhydroxide (Han et al., 2016) and magnesium oxide (Alamillo et al., 2012), etc., with different compositions and properties, to prepared ruthenium-based catalysts that were used to catalyze the reduction of FF and 5-hydroxymethylfurfural to FFA or BHMF. Among them, a carrier capable of interacting effectively with Ru particles is an ideal choice. MOFs are well-known for their strong host-guest interaction between the framework and metal particles. Yuan et al. (2015b) utilized Ru/Zr-MOFs, which prepared by deposition-reduction of Ru on the Zr-MOFs, to catalyze the hydrogenation of FF to FFA, and an FFA yield of 94.3% was obtained at a low temperature of 20°C for 4 h under low hydrogen pressure of 0.5 MPa. After the sixth recycle, the catalyst showed no decrease in the activity, possibly due to the small Ru nanoparticles size and the good reducing ability which can be attributed to the medium interaction between Zr-MOFs and Ru particles.
Many studies have displayed that ruthenium-based catalytic systems, such as Ru/Cu Nanoparticles (Zhang et al., 2019), Ru/C (Gupta and Saha, 2017), RuCo/C (Gao et al., 2016), Ru/Co3O4 [147] (Wang T. et al., 2017), Ru/Al2O3 (Alamillo et al., 2012), Ru/C+AlCl3 (Panagiotopoulou et al., 2015), Ru/ZrO2-based (Han et al., 2016), and Cm*Ru(HTsDPEN) (Thananatthanachon and Rauchfuss, 2010), are highly efficient for the formation of BHMF. The Ru-based catalyst for the hydrogenation of 5-hydroxymethylfurfural to BHMF under mild conditions (temperature ranged from 25 to 210°C) in the presence of different H-donors, such as H2 (pressures changed from 0.4 to 2.7 MPa), alcohol, or formic acid. The best catalytic activity was obtained over Ru(OH)x/ZrO2, affording a BHMF yield of 99% and 5-hydroxymethylfurfural conversion of 100% at 120°C for 6 h with 1.5 MPa H2 pressure (Han et al., 2016). The stability of heterogeneous catalysts in a continuous catalytic process is very important. After the catalyst was reused for five times, no significant loss of catalytic activity was observed, and the conversion of 5-hydroxymethylfurfural and the yield of BHMF were the same as those with the fresh Ru(OH)x/ZrO2 catalyst. Also, researchers added solid support, such as silica and magnesium dioxide, by different methods onto the basis of Ru/ZrO2-based catalysts, trying to tune the structure of the catalyst to improve its catalytic activity and stability.
Mesoporous silica is ideal support because of its large pore size and is suitable for large-sized organic substrates and biomass derivatives (Huang et al., 2011). Metal-precursor ligands are typically used to immobilize clusters in mesopores at lower metal loading due to their relatively stable nature (Mihalcik and Lin, 2008). Because the pore size of mesoporous silica (>2 nm) is larger than the particle size of metal cluster, the impregnated support cannot achieve uniform mesoporous-silica supported noble metal cluster in metal saline solution. Metal clusters readily grow into large particles with a wide distribution of size (Yen et al., 2011). It has been developed to mix mixed elements (Zr, Ti, Al, etc.) into mesoporous silica to disperse metal species. Therefore, Chen et al. reported that ruthenium clusters immobilized in nanosized mesoporous zirconium silica (MSN-Zr) were synthesized by the immersion method starting from RuCl3 aqueous solution. Ruthenium cluster catalysts showed significant activity for 5-hydroxymethylfurfural hydrogenation in the water at room temperature under 0.5 MPa hydrogen pressure, giving 98.1% 5-hydroxymethylfurfural conversion, and 90.4% BHMF yield (Chen et al., 2013). Alamillo et al. (2012) reported that Ru/ZrO2-MgO could catalyze the reduction of 5-hydroxymethylfurfural to BHMF at 130°C for 2 h, with a 5-hydroxymethylfurfural conversion of 99% and a BHMF yield of 93.1%.
Ir-Based Catalysts
Iridium-based catalysts can catalyze the hydrogenation of FF or 5-hydroxymethylfurfural into FFA or BHMF at a temperature ranging from 30 to 100°C, respectively, as shown in Table 2. Tamura et al. used an Ir-ReOx/SiO2 to catalyze the reduction of FF and 5-hydroxymethylfurfural, giving FFA or BHMF yield of 100% under the reaction conditions of 30°C for 6 h under the hydrogenation pressure of 0.8 MPa H2 (Tamura et al., 2013). This high catalytic performance is possibly ascribed to the cooperative effect between the iridium and the inexpensive Re(III)Ox clusters, which partially covered the iridium surface. ReOx was believed to be high-efficiency in the reduction of polarized double bonds, promoting the adsorption of H2 onto the catalyst, and making it easier to dissociate the heterogeneous species of H2 into ionic hydrogen species (H+ and H−) (Guan et al., 2005).
Studies on the catalytic activity of Ir-based catalysts for the reduction of FF and 5-hydroxymethylfurfural by catalytic transfer hydrogenation have also been reported. Wang et al. (2015) reported that the Ir@CN catalyst, which were prepared by pyrolysis of 1,10-phenanthroline on activated carbon by pyrCl3 complexes, yielding small IrNPs (2–4 nm) supported on N-doped carbon, could catalyze the reduction of FF by catalytic transfer hydrogenation in the aqueous phase, affording a FFA yield of 99% at 100°C with formic acid which is an important and renewable by-product (also an H-donor) derived from the decomposition of biomass-derived carbohydrates into the levulinic acid reaction by acid catalysis (Weingarten et al., 2013).
In order to verify the feasibility and availability of catalytic transfer hydrogenation in other catalysts for the selective hydrogenation of 5-hydroxymethylfurfural, Thananatthanachon and Rauchfuss (2010) reported various amine–metal complexes, such as Cp*Ir(HTsDPEN), Cp*Ir(HTsDACH), and Cp*Ir(NHCPh2C6H4) (Cp* = 1,2,3,4,5-pentamethylcyclopenta-1,3-diene), to catalyze the hydrogenation of 5-hydroxymethylfurfural by adopted formic acid as a hydrogen donor. Notably, up to 99% BHMF yield was achieved at 40°C. The results showed that catalytic transfer hydrogenation can be used to selectively hydrogenate 5-hydroxymethylfurfural into BHMF. Unfortunately, homogeneous catalysts, peculiarly Cp*Ir (HTsDACH) and Cp*Ir (NHCPh2C6H4), have poor tolerance to formic acid, a significant loss of their initial catalytic activity within a few minutes. The mechanism of the reaction demonstrates the release of carbon dioxide (Scheme 3). In order to avoid the use of formic acid, Townsend et al. (2017) used Cp*Ir(pyridinesulfonamide)Cl (Cp* = pentamethylcyclopentadienyl) to catalyze the reduction of 5-hydroxymethylfurfural with isopropyl alcohol as hydrogen source, achieving a BHMF yield of 100% under the reaction conditions of 85°C for 0.5 h without alkali. The proposed catalytic mechanism is shown in Scheme 4. Preliminary mechanism study showed that the active catalyst for transfer hydrogenation is produced by dissociation of chloride. It is probably cooperative catalysis between the iridium center and the sulfonamide portion of the ligand.
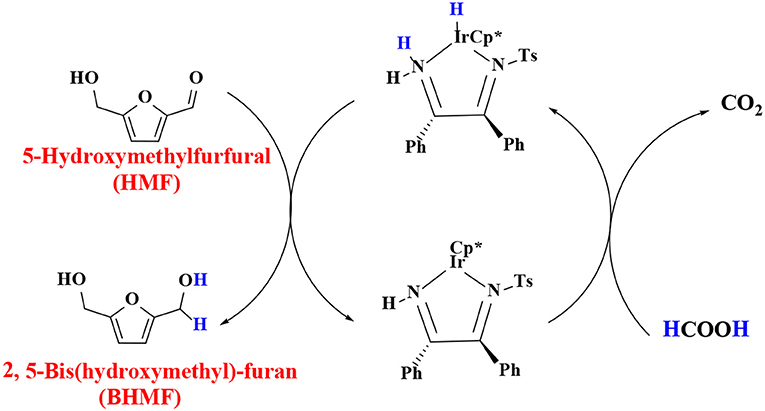
Scheme 3. The catalytic mechanism for the hydrogenation of 5-hydroxymethylfurfural (HMF) with isopropyl alcohol using Cp*Ir(TsDPEN).
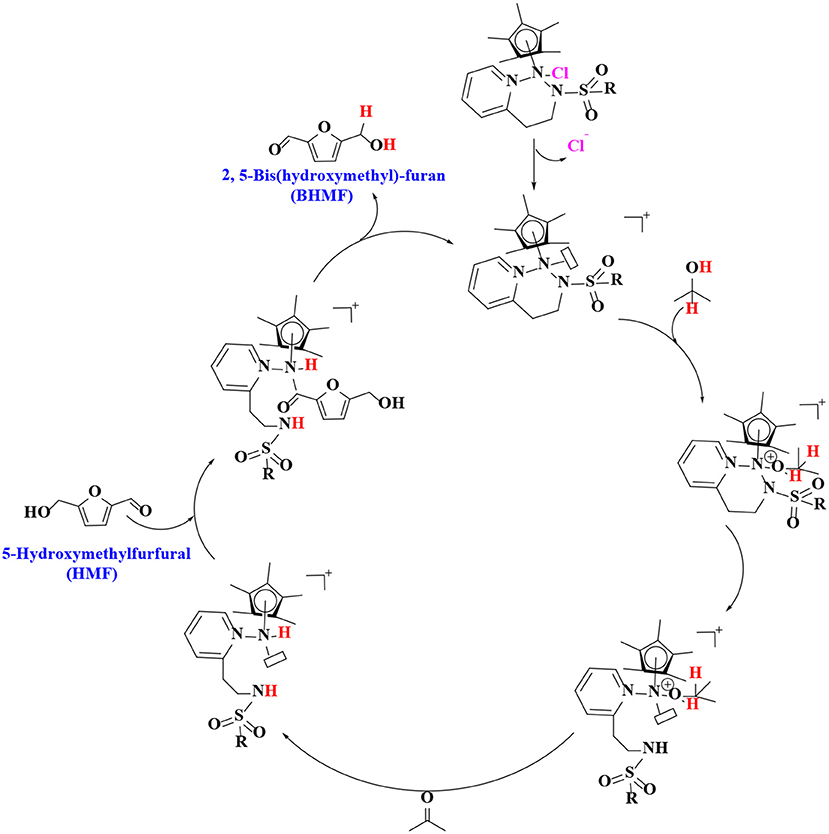
Scheme 4. The catalytic mechanism for the hydrogenation of 5-hydroxymethylfurfural (HMF) with formic acid using Cp*Ir(pyridinesulfonamide)Cl.
Au-Based Catalysts
As a result of the low activation/dissociation potency of a hydrogen source, the reactivity mediated by Au in H-donors (e.g., H2 or isopropanol) is usually lower than that of the platinum group metals discussed above (Cárdenas-Lizana and Keane, 2013). The surface properties of the support, such as the surface lattice structure, surface area or acidity and alkalinity, affect the structure and electronic state of the Au species. In order to adjust the catalytic effect, it is common to add another metal oxide to the Au-based catalyst (Akita et al., 2013). For example, Zhu et al. (2016) used Au/ZrO2, which was synthesized by an ultrasonic auxiliary deposition method, catalyzed the hydrogenation of FF to FFA. Precipitously, Au/ZrO2 revealed remarkable reduction reactivity, both the conversion of FF and the yield of furfuryl alcohol reached 100% in 3 h (Zhu et al., 2016). Although Au nanoparticles are widely used as active and highly selective catalysts in oxidation reactions (Yoskamtorn et al., 2014), their use in H2-mediated reduction reactions is rare because H2 is difficult to adsorb onto the Au surface for activation (Lu et al., 2014). However, in the catalytic transfer hydrogenation process, the active H* substance produced from isopropanol can promote the hydrogenation of FF at the Au surface site. In addition, it has been reported that the gold surface acted as a Lewis acid site and coupled with Lewis base to construct an effective inhibited Lewis activity on H * species by calculation and experiment (Lu et al., 2014). In the reaction system of Au/ZrO2, the carrier ZrO2 can be used as a Lewis base and formed an effective inhibited Lewis pair with the Lewis acid on the Au surface, thus enhancing the hydrogenation performance of FF. Alkaline metal oxide support can improve the selectivity of gold-based catalysts for hydrogenation of 5-hydroxymethylfurfural to BHMF (Ohyama et al., 2013).
Although the conversion of 5-hydroxymethylfurfural or FF is high, the selectivity of BHMF or FFA is very low over Pt (Balakrishnan et al., 2012), Pd (Date et al., 2017) and Cu (Srivastava et al., 2017) metal catalysts supported by Al2O3 in the reduction of 5-hydroxymethylfurfural. Therefore, Ohyama et al. (2013) utilized Au supported by trialumina to catalyze the selective reduction of 5-hydroxymethylfurfural to BHMF. Both the conversion of 5-hydroxymethylfurfural and the yield of BHMF were >96% under the reaction conditions of 120°C and 6.5 MPa H2 for 2 h. The high catalytic activity of Au/Al2O3 for catalytic reduction of 5-hydroxymethylfurfural to BHMF is attributed to the high hydrogenation activity of small Au clusters and the difficulty in converting BHMF to furan-ring opening products for its unique acid-base properties. However, the reaction conditions for the highly efficient and highly selective reduction of 5-hydroxymethylfurfural to BHMF by Au/Al2O3 are harsh. For example, both the reaction temperature and hydrogen pressure are high (120°C, 6.5 MPa). In order to make Au/Al2O3 hold excellent catalytic performance under mild reaction conditions, Ohyama et al. doped iron oxides with the support Al2O3, and the resulting catalyst (Au/FeOx/Al2O3) is highly selective for the reduction of 5-hydroxymethylfurfural to BHMF, giving 5-hydroxymethylfurfural conversion and BHMF yield both in 96% at 80°C and 3 MPa H2 for 2 h (Ohyama et al., 2016).
The Group VIII metal (Pd, Pt, Ir, Ru, or Au) does not have repulsion between the furan ring and the metal surface and favors a flat η2 (C–O) bonding mode. The preferred basic route for FFA is carried out in two steps (Scheme 5): (1) the hydrogenation reaction flat adsorbs the carbonyl group of η2 (C–O)-FF to form a hydroxyalkyl intermediate, followed by (2) reduction of the carbon with a hydroxyl group to form FFA.
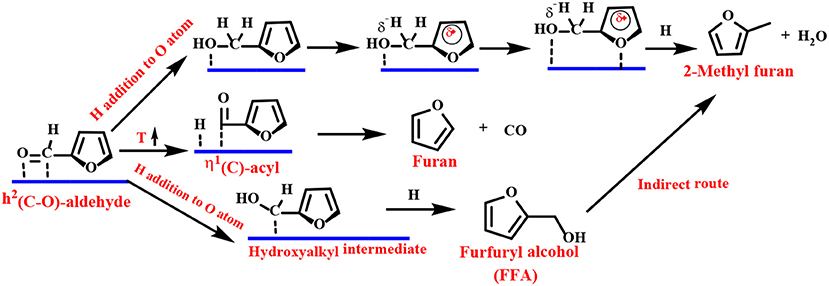
Scheme 5. Schematic illustration of FF hydrogenation to furfuryl alcohol (FFA) over group VIII metal-based catalysts.
Generally, various noble metals (e.g., Pd, Pt, Ru, Ir, and Au) and non-noble metals, including different chromium and non-chromium metals [such as Cu- and ferrous metals (Fe, Ni, or Co)-based], have been reported for the selective hydrogenation of FF and 5-hydroxymethylfurfural in the gas phase and the liquid phase. Chromium catalysts show good catalytic activity, but they are not widely available due to their high toxicity. The non-chromium metals, such as Cu-based catalysts exhibit a remarkable selectivity for the hydrogenation of a carbonyl group, and the C–C double bond in the furan ring does not participate in the reaction. A second metal or promoter is sometimes added to improve activity or selectivity by increasing the surface area or acting as a Lewis acid site to polarize the C–O bond. At the same time, the iron/cobalt/nickel-based catalysis also achieved better catalytic effects. However, the main drawback is that most of these catalysts cannot be reused. Therefore, other noble metals, such as Pd, Pt, Ru, Ir, and Au, were added to increase their stability, where ethanol, water, ethanol, isopropyl alcohol, n-butyl alcohol, benzyl alcohol, heptane, tetrahydrofuran, or octane was used as the solvent. The mechanism of precious and non-precious metal particles catalyzed the reduction of FF and 5-hydroxymethylfurfural to FFA and BHMF is also described.
Solid Acid-Base Catalysts
Solid acid-base catalysts, such as metal hydroxides and solid Lewis acid/base materials, are widely used in the reduction of FF and 5-hydroxymethylfurfural by catalytic transfer hydrogenation. Solid catalysts containing Lewis acids are often used for intermolecular and intramolecular hydrogen transfer, so many researchers use them to catalyze the upgrading reaction of biomass to produce valuable compounds (Li et al., 2014). The use of liquid hydrogen sources (e.g., alcohol and formic acid) instead of high-pressure H2 addresses the safety hazards posed by hydrogen (He et al., 2019b). In relation to this, it has recently been reported that Lewis acid zeolites, such as Hf- and Zr-, are used for the hydrogenation of biomass-derived carboxides, such as FF and 5-hydroxymethylfurfural, into corresponding alcohols through the reduction of Meerwein-Ponndorf-Verley (MPV), as shown in Table 3. For example, Hao et al. (2016) reported that ZrO(OH)2, which contained acidic and basic sites, catalyzed the hydrogenation of 5-hydroxymethylfurfural to BHMF, giving 83.7% yield of BHMF by the catalytic transfer hydrogenation reaction. This interesting work prompted more researchers to explore the complex containing zirconyl acid-base coupling site for catalytic transfer hydrogenation reaction, which could not only overcome the drawbacks encountered in the catalytic process (e.g., catalyst deactivation and harsh reaction conditions) but also improve the catalytic performance of the Zr-based materials. It is well-known that the magnetic catalysts can be easily separated from the reaction mixture by an external magnet for its distinct properties. Therefore, Hu et al. (2018) added magnetic components, such as iron, to zirconium hydroxide to prepare magnetic zirconium hydroxides MZH (Zr/Fe = 2), which was used to catalyze the reduction of 5-hydroxymethylfurfural to BHMF at 150°C for 5 h using 2-butanol as hydrogen source, and the obtained conversion of 5-hydroxymethylfurfural and the yield of BHMF were up to 98.4 and 89.6%, respectively. The catalyst could be recycled for continuous 5 times with no significant decrease in catalytic activity (Hu et al., 2018). Inorganic-organometallic phosphonates are well-known for their unique properties, such as excellent hydrolytic stability for silicas and polymers (de los Reyes et al., 2013). They are used in a wide range of applications for the adsorption and separation of toxic and contaminants, energy storage, and drug delivery (Ma and Yuan, 2011), and being used as a catalyst (Bhanja and Bhaumik, 2016) in the reduction of biomass-based aldehydes, especially FF and 5-hydroxymethylfurfural.
It is desirable that the pore size of the catalyst, which can be controlled by mesopores and macropores, ensures that the substrate readily enters the active site during the catalytic process. (Li et al., 2016a) reported that ZrPN (organotriphosphate-zirconium complex), a heterogeneous nitrogen-containing alkyltriphosphonate-metal hybrid (MPN), which can be used to enhance acid and base functionalization, and prepared by a simple method with no additional addition of a template. Proper adjustment of the alkali/acid site ratio in ZrPN can enhance the catalytic performance of the catalyst in the hydrogenation of FF and 5-hydroxymethylfurfural to FFA or BHMF, respectively. The yield of FFA and BHMF was significantly improved when the base/acid site is 1:0.7, FF conversion and FFA yield both in 98% were obtained at 140°C for 2 h by using isopropanol as H-donor (Li et al., 2016a). Under the same reaction conditions, the conversion of 5-hydroxymethylfurfural and the yield of BHMF reached 99 and 98%, respectively. Then, Li et al. (2017a) used the same method for synthesizing Zr-benzylphosphonate nanohybrids (BPPN), which can be prepared from o-, p-, or m-xylylenediphosphonates (o-, p-, or m-PhP) and zirconium salt via a simple and template-free assembly method. The conversion of 5-hydroxymethylfurfural and the yield of BHMF reached 99 and 93% over Zr-BPPN at 120°C for 2 h by using isopropanol as H-donor, respectively (Li et al., 2017a).
Metal-organic frameworks (MOFs), which were usually composed of metal substances and organic ligands, are known for the ability to produce porous and crystalline polymers, showing great potential in various fields, such as separation of materials, gas adsorption, sensing, storing of energy, and delivering of drug due to their unique properties (Zhou and Kitagawa, 2014). More and more researchers are keen to develop functional and structural materials for natural composites in crucial areas, such as material chemistry (Wegst et al., 2015). It is reported that substantial porous carbonaceous materials can be prepared by calcining and modifying lignocellulose with different functional groups, and showed very strong application prospects in many important fields, such as catalysis and drug delivery (Kai et al., 2016). The relatively strong stability of functional and structural materials can be obtained from natural organic molecular materials, such as phytic acid, polyphenols, and porphyrins, by self-assembly with a metal ion, such as Zr4+ (Song et al., 2015). 2,5-furandicarboxylic acid (FDCA), whose structure is similar to terephthalic acid, contains two –COOH groups at the 2,5-position of the ring. FDCA can also be used as an excellent organic ligand for the preparation of MOFs because it is beneficial to the production of polymers (Han et al., 2017). Inspired by these findings, Li et al. (2017a) prepared a mesoporous acid-based bifunctional nanocomposite Zr-FDCA by hydrothermal treatment of FDCA with zirconium ion, and the obtained Zr-FDCA was highly efficient for the reduction of biomass-based aldehydes, especially FF and 5-hydroxymethylfurfural, giving FF and 5-hydroxymethylfurfural conversion of 98 and 97% with FFA and BHMF yield of 96 and 87% at 140°C for 8 h by utilizing isopropanol as hydrogen source, respectively (Li et al., 2017a). Considering that the reusability of a solid catalyst is one of the most important features to evaluate its potential application in industrial production (Garcia-Olmo et al., 2016), Zr-FDCA was reused for six consecutive cycles and no significant loss in reactivity was observed, with 98% FF conversion and 96% FFA yield in the sixth cycle.
Besides FDCA, Li et al. (2019) also used para-xylylenediphosphonates as ligands to prepare Hf-based MOFs (PhP-Hf) by self-assembly with hafnium ion, and used for the hydrogenation of FF and 5-hydroxymethylfurfural. PhP-Hf (1:1.5) could catalyze the hydrogenation of FF to FFA, affording FF conversion of 100% with FFA yield of 99.2% at 120°C for 2 h using isopropanol as the hydrogen source. After the catalyst being reused for 5 times, only a minor decrease in catalytic activity was observed, indicating that the stability of the catalyst was high (Li et al., 2019). Similarly, Hf-FDCA prepared with the same method could also catalyze the reduction of 5-hydroxymethylfurfural to BHMF, achieving a high BHMF yield of 95% at 100°C for 5 h by using isopropanol as hydrogen source (Li et al., 2018a).
Regarding the reaction mechanism of PhP-Hf, the acid site (Hf4+) and the base site (O2−) of PhP-Hf (1:1.5) proved to synergistically catalyze the reduction of FF to FFA by MPV reaction. It was proposed that the critical transition state shown in Scheme 6 is in situ formed among FF, isopropanol, and acid-base coupling materials (Hf4+-O2−). Typically, a pair of acid-base sites (Hf4+-O2−) facilitates the adsorption of isopropanol onto PhP-Hf (1:1.5) and is capable of producing isopropanol and hydride by dissociation. The aldehyde groups in the FF may be activated by acidic Hf4+ species, thus resulting in the formation of a transition state with six linkages to achieve a hydrogen transfer pathway to produce FFA and acetone (Scheme 6). To be noted, some by-products, such as FF diisopropyl acetal, 2-isopropoxymethylfuran, isopropyl levulinate, and 2-decanoic acid may be formed in the presence of relatively strong acidic and basic sites.
Rojas-Buzo et al. (2018) used Hf-MOF-808 catalyst, which was prepared by self-assembly of 1,3,5-benzenetricarboxylic acid and HfCl4, to catalyze the reduction of FF and 5-hydroxymethylfurfural utilizing isopropanol as H-donor, giving FFA and BHMF both in yield of 97% at 100°C for 2 and 1.5 h, respectively. No significant loss in reactivity was found after the catalyst reused for 5 times, indicating that this catalyst was relatively stable. In the hydrogenation of FF and 5-hydroxymethylfurfural, the Zr-MOF or Hf-MOF catalyst not only exhibited excellent selectivity toward the formation of FFA and BHMF, but also showed relatively high catalytic stability during recycles. Zr-Beta or Sn-Beta zeolite catalyst also exhibits excellent catalytic performance during the hydrogenation of 5-hydroxymethylfurfural to BHMF via catalytic transfer hydrogenation using isopropanol as a hydrogen source. The strong Lewis acid sites on these catalysts can effectively catalyze the reduction of 5-hydroxymethylfurfural to BHMF, and also catalyzed the etherification of BHMF (Luo et al., 2014). The Lewis or Brønsted acid sites can be effectively adjusted by adding active metal oxide to the Zr-based catalyst. Wei et al. (2018) reported ZrBa(3)-SBA, prepared by loaded active metal oxides using the successive incipient-wetness impregnation method, showed high catalytic activity in the chemoselective reduction of 5-hydroxymethylfurfural to BHMF, with the 5-hydroxymethylfurfural conversion of 98.3% and BHMF yield of 91%. Exceptionally, ZrBa-SBA can be reused continuously for five times with no significant loss of catalytic performance, indicating that it was a stable catalyst for the tested reaction. ZrBa(3)-SBA exhibits high catalytic activity, which is attributable to the significant reduction in the total acid sites of ZrBa-SBA by the introduction of BaO, completely suppressing the etherification of 5-hydroxymethylfurfural or BHMF. However, the remaining acid sites of ZrBa-SBA (Lewis acid) are still sufficient for selective reduction of 5-hydroxymethylfurfural (Wei et al., 2018).
Typically, the preparation of Lewis acidic zeolites, such as Zr4+ catalysts is cumbersome. On the other hand, the presence of a strong Lewis acid causes some side reactions, such as etherification of FFA or BHMF with an alcohol, and ring opening of the furan ring. Therefore, it is still necessary to develop new catalytic pathways for the effective conversion of FF and 5-hydroxymethylfurfural to FFA and BHMF, respectively. It has been reported that base catalysts have great potential for catalyzing the carbonyl compounds to corresponding alcohols via catalytic transfer hydrogenation (Wang and Zhang, 2016). Biradar et al. (2016) showed that magnesium oxide could catalyze the hydrogenation of FF to FFA. Both the conversion of furfural and the yield of FFA were 99.2% at 170°C for 5 h utilizing isopropanol as the hydrogen source. MgO could be reused continuously for 5 cycles without significant loss in catalytic activity. Pasini et al. (2014) also used magnesium oxide as a catalyst for the hydrogenation of 5-hydroxymethylfurfural using methanol as a clean and efficient hydrogen source, affording 5-hydroxymethylfurfural conversion and BHMF yield both in 100% at 160°C for 3 h. Inexpensive mixed metal oxides are broadly used in catalytic fields due to their adjustable physicochemical properties, such as surface area, pore size distribution, and acid/base characteristics, which are closely related to catalytic activity. Solid materials with magnetic properties are the most interesting catalyst supporters due to their unique properties. For example, magnetic catalysts can be separated from the reaction mixture by external magnets due to their unique properties, which avoid weight loss of the catalyst during repeated use and make the operation of this catalytic system time-saving and energy efficient (Vu et al., 2014). Wang and Zhang (2016) supported γ-Fe2O3 onto hydroxyapatite to prepare γ-Fe2O3@HAP catalyst, which was used as a magnetic base catalyst to catalyze the reduction of FF into FFA. The conversion of FF and the yield of FFA were 90 and 65% at 180°C for 3 h by utilizing isopropanol as the hydrogen source, respectively. After recycling for 6 times under the optimized reaction conditions, the catalytic activity was not reduced. When the reaction time was extended to 10 h, the conversion of FF and the yield of FFA were up to 96.2 and 91.7%, respectively (Wang and Zhang, 2016). He et al. (2018a) also reported another magnetic catalyst Al7Zr3@Fe3O4, an acid/base bi-functional magnetic oxide catalyst, which can be prepared by a facile co-precipitation method. Al7Zr3@Fe3O4 could catalyze the hydrogenation of FF to FFA by using isopropanol as a hydrogen source, giving FF conversion of 99.1% and FFA yield of 90.5% at 180°C for 4 h. Al7Zr3@Fe3O4 (1/1) acted as a heterogeneous catalyst and could be recycled for 5 times with no remarkable loss of catalytic performance. Moreover, it was found that the weight of the catalyst was not lost after a straightforward recovery by using an external magnet. Remarkably, the catalyst also showed high-efficiency for reduction of other biomass-derived furanic aldehydes, such as 5-hydroxymethylfurfural. The conversion of 5-hydroxymethylfurfural and the yield of BHMF were 82.7 and 71% under the optimized reaction conditions, respectively (He et al., 2018a).
In general, solid acid/base catalysts, such as ZrO(OH)2, Zr- or Hf-MOF, Zr- or Sn-Beta zeolite, MgO, γ-Fe2O3@HAP, and Al7Zr3@Fe3O4, were able to efficiently catalyze the hydrogenation of 5-hydroxymethylfurfural and FF to corresponding alcohols via MPV reduction. Zr- and Hf-MOF catalysts not only exhibited excellent selectivity for the synthesis of FFA or BHMF, but also showed relatively high catalytic stability during recycles. Zr- or Sn-Beta zeolite catalyst also exhibits excellent catalytic performance during the hydrogenation of 5-hydroxymethylfurfural to BHMF via catalytic transfer hydrogenation using isopropanol as the hydrogen source. Inexpensive mixed metal oxides, such as MgO and γ-Fe2O3@HAP, also achieved the good catalytic activity. Further, the mechanism mediated by the solid acid/base catalyst through the reduction of MPV was discussed.
Alkali Salt Catalysts
As discussed above, there have been many reports on different types of catalysts including noble metals (e.g., Pd, Pt, Ir, Ru, and Au) (Lesiak et al., 2014; Date et al., 2018; Shirvani et al., 2018), non-noble metals (e.g., Cu, Ni, Fe, Mg, and Co) (Villaverde et al., 2013; Yan et al., 2013b; Khromova et al., 2016; Osatiashtiani et al., 2017), and solid acid-base bifunctional catalysts (e.g., the Zr-and Hf-based) for the hydrogenation of FF or 5-hydroxymethylfurfural. However, they are either expensive or complicated to prepare. It is worth mentioning that H2 is the most common source of hydrogen, but usually requires a higher pressure (e.g., 8 MPa). In particular, when it comes to gas phase hydrogenation, a series of pressure-resistant instruments are required (Chen et al., 2002). For the purpose of safety and low cost, liquid phase transfer hydrogenation has received much attention by using formic acid or an alcohol, such as isopropanol as the H-donor (Addis et al., 2011; Li et al., 2016a). However, using formic acid as the hydrogen donor, there is some corrosion to the experimental equipment, and the alcohol solvent can be further reacted with FFA by etherification to reduce its selectivity under the harsh conditions employed (Zhang et al., 2016). Therefore, it is increasingly important to find an inexpensive and safe hydrogen donor and more desirable catalysts for the hydrogenation of FF and 5-hydroxymethylfurfural. Hydrosilylation is widely used in the catalytic reaction of carbonyl compounds to the corresponding alcohols (Oestreich and Hermeke, 2015). This catalytic process is very attractive using hydrosilanes as hydrogen source, which are very stable in water and air, and can be activated to produce silyl ethers that can be converted to alcohol and silanol via hydrolysis with an acid/base catalyst or activator under relatively mild conditions (Revunova and Nikonov, 2015; Muthukumar et al., 2016). Long et al. (2018) reported that potassium carbonate, an abundant, readily available, and cost-effective salt, could catalyze the hydrogenation of 5-hydroxymethylfurfural into BHMF at room temperature using Ph2SiH2 as H-donor in the presence of bio-based solvent 2-methyltetrahydrofuran, giving a good BHMF yield of 94% after 2 h (Table 4, entry 1). However, using Ph2SiH2 as hydrogen source not only tends to produce high molecular weight by-products, such as silanols that are detrimental to product separation, but also its reserve is not too much due to the relatively expensive price (Zhao et al., 2014). It is impending to find more ideal H-donor for the hydrogenation of biofuranic aldehyde. Polymethylhydrosiloxane (PMHS) is an attractive H-donor among commercially available hydrosilanes, which can be attributed to its unique character, which is inexpensive, commercially available, non-toxic, biodegradable, and stable in air and moisture (Volkov et al., 2015). In addition, PMHS is soluble in most organic solvents due to its low viscosity (Kumar et al., 2017). Based on these findings, Long et al. (2019) depicted a benign method to effectively catalyze the reduction of FF to FFA over commercially available Cs2CO3 utilizing non-toxic and cheap PMHS as a hydrogen source, giving an excellent FFA yield of 99% (Table 4, entry 2).
Regarding the reaction mechanism of Cs2CO3, Lewis base (carbonate 1) proved to efficiently catalyze the reduction of FF to furfuryl alcohol by hydrosilylation reaction (Scheme 7). After reacting with Lewis base (carbonate 1), PMHS is cleaved into small silane (H3SiMe 2), which forms pentavalent silicate with carbonate, and then reacts with carbonyl in FF to produce a hexavalent intermediate, which can activate the aldehyde group by hydride to obtain silyl ether 3, which is then hydrolyzed to gain FFA.
Although the above two alkali metal carbonates have excellent catalytic effects, the activity of the catalyst is greatly reduced after repeated use, which could be attributed to the interaction of carbonate with hydrosilane, facilitating the formation of silicon formate. The reusability of a solid catalyst is one of the most important features to evaluate its potential application in industrial production. Therefore, Zhao et al. (2018) utilized potassium fluoride that is a cheap alkali salt to catalyze the hydrogenation of FF and 5-hydroxymethylfurfural to FFA or BHMF, giving FFA or BHMF in a high yield of up to 97 and 98%, respectively (Wu et al., 2018; Zhao et al., 2018; Table 4, entries 3 and 4). Remarkably, this catalyst could be recycled for several consecutive cycles with no distinct loss of activity, demonstrating that the stability of potassium fluoride is high during the catalytic procedure.
Conclusions and Perspectives
This review illustrates the performance of heterogeneous catalysts, especially the metallic particles (supported on Cu, Mg, Fe, Ni, Co, Pd, Pt, Ru, Ir, and Au metals), solid acid/base (e.g., Zr-and Hf-based), and alkali metal salts (e.g., K2CO3, Cs2CO3, and KF) in the hydrogenation of biofuranic aldehydes to alcohols. Inferred from recent reaction relationships and reaction pathways, the conversion of FF and 5-hydroxymethylfurfural to useful chemicals and biofuels by different catalysts mainly involves several processes related to hydriding, destructive hydrogenation, decarburization, oxidization, and ring opening reactions. Therefore, the catalyst design is the principal element to acquire high-efficiency conversion of FF and 5-hydroxymethylfurfural to the desired products. Based on this direction, with hydrogen as the hydrogen source, the Cu-based catalysts exhibit a stronger avidity for the hydrogenation of FF and 5-hydroxymethylfurfural to FFA or BHMF, respectively, while the hydrogenation of the C = C-bonded furan ring on the Cu-based catalysts is not affected because of the exclusion between the furan nucleus and the Cu surface. Similarly, the Fe catalyst showed an excellent effect of selective hydrogenation of FF and 5-hydroxymethylfurfural to yield FFA or BHMF. Though the Ni catalysts exhibit high performance in the hydrogenation of biofuranic compounds, it is accompanied by side reactions, leading to the hydrogenation of the double bond of the furan ring. The Pd catalysts promote hydriding of C = O and C = C bonds and destructive hydrogenation of C-O bonds. In addition, under elevated conditions, the Pd catalyst also undergoes decarbonylation of FF or 5-hydroxymethylfurfural and furan ring opening due to the friable interactions between the carboxide carbon and palladium surfaces. Pt catalyst is widely used in the hydrogenation reactions due to its unique electrical and chemical properties. Obviously, the Au catalysts also exhibit relatively high catalytic performance in the furan ring opening reaction. In addition, Ru catalysts have been shown to have high catalytic activation for hydriding of C = O bonds and then destructive hydrogenation, gaining effective and useful production with fewer O atoms.
Metal hydroxides and solid Lewis acid/base materials are widely used in the hydrogenation of FF and 5-hydroxymethylfurfural by catalytic transfer hydrogenation with efficient catalytic performance. The utilization of alkali metal salts can not only solve the complicated preparation process of the catalyst, but also avoid the use of high-pressure hydrogen, alcohol, and formic acid by using hydrogen silane as a hydrogen source. The property of the catalyst' supporter (acidity, alkalinity and neutral) also plays a crucial character in these catalytic conversion reactions. Since the acidic carrier has a great influence on the hydrogenolysis and ring opening of the furan ring, which contributes to the cleavage of the C-O bond. However, the basic carrier results in either the oxidation or over hydrogenation of the furan-ring. Furthermore, the protic solvent promotes a higher solvability of the hydrogen than the aprotic solvent, and thus enhances the catalytic activation by increasing the interaction of hydrogen with the catalyst surface. In addition, the morphology and the particle size of the catalyst also have a significant influence on the catalytic capability. Furthermore, it is known that the incorporation of a second metal plays an essential role in the geometry and electron concentration of the active site of the conditioning catalyst, which results in the bimetallic catalyst to exhibit enhanced catalytic activation and stabilization. Although precious metal catalysts exhibit enhanced catalytic performance for furan conversion, several non-precious metals (Fe, Ni, Co, and Cu) supported heterogeneous catalysts have also been investigated. Hence, in order to satisfy global demand, the rich and cheaper metal catalytic system on the earth is gaining huge scientific and industrial concerns. Moreover, studies have shown that different other reaction parameters, such as reaction temperature, exhaust gas pressure (hydrogen), solvent properties (polar or non-polar), reducing agents (H2, ethanol, silane) also have a great influence on the catalytic reaction. This review not only discusses the mechanism by heterogeneous catalysts for the conversion of xylose and glucose to FF or 5-hydroxymethylfurfural, but also depicts representative examples on the conversion of FF or 5-hydroxymethylfurfural to FFA or BHMF in the presence of different catalysts, respectively, providing a theoretical support for the exploration of more environmentally friendly approaches to the hydrogenation of biomass-derived carbonyl compounds.
Although significant progress has been achieved for heterogeneous catalytic upgrading of biomass-derived furanic compounds, some advances are still needed in plenty of cases for the exploration of mercantile applications:
(a) Design of novel multi-functional catalysts to promote interaction with various substrates, so as to promote multi-step reactions in one-pot reactions, avoiding isolation and purification of intermediates.
(b) Control the acidity/alkalinity of the catalyst to improve the selectivity of the desirable product.
(c) In addition to precious metals, the establishment of non-precious metal-based catalysts is highly desirable for upgrading biomass-derived compounds.
(d) To comprehend mechanism insights and the catalyst structure-activity relationship, so as to guide the development of more efficient catalysts.
(e) Though it is safe and inexpensive to use hydrosilane as a hydrogen source, the products are not easy to separate. It is necessary to find a more favorable hydrogen source, greener solvent or improved catalytic system/process, which is better for separation of the products with good activity.
(f) The direct use of lignocelluloses, a cheaper and more readily available natural source, for biomass-derived alcohols would be of particular importance, thus decreasing the release of petroleum-derived pollutants.
(g) To meet the multiple conversion steps for biomass upgrading, it would be highly desirable to develop more economic and facile approaches for the preparation of multifunctional catalysts that can promote cascade or complex reaction processes.
Overall, this review comments recent examples on the catalytic hydrogenation of biofuranic aldehydes to alcohols over different heterogeneous catalysts. We believe that a comprehensive study on a wide range of catalytic biomass conversion processes may help to develop new and even more efficient ways for the upgrade of biomass-derived compounds to useful chemicals and biofuels.
Author Contributions
JL and HL: taking the lead in coordinating the review study and drafting the manuscript. JL, YX, and WZ: literature searching. SY: supervision. HL: conceptualization. SY and HL: funding acquisition and project administration. JL: writing-original draft. HL and SY: writing-review and editing.
Funding
This work was financially supported by the National Natural Science Foundation of China (21576059, 21666008), Fok Ying-Tong Education Foundation (161030), Key Technologies R&D Program of China (2014BAD23B01), and Guizhou Science and Technology Foundation ([2018]1037, [2017]5788).
Conflict of Interest Statement
The authors declare that the research was conducted in the absence of any commercial or financial relationships that could be construed as a potential conflict of interest.
References
Addis, D., Das, S., Junge, K., and Beller, M. (2011). Selective reduction of carboxylic acid derivatives by catalytic hydrosilylation. Angew. Chem. Int. Ed. 50, 6004–6011. doi: 10.1002/anie.201100145
Aellig, C., Jenny, F., Scholz, D., Wolf, P., Giovinazzo, I., Kollhoff, F., et al. (2014). Combined 1,4-butanediol lactonization and transfer hydrogenation/hydrogenolysis of furfural-derivatives under continuous flow conditions. Catal. Sci. Technol. 4, 2326–2331. doi: 10.1039/C4CY00213J
Akita, T., Kohyama, M., and Haruta, M. (2013). Electron microscopy study of gold nanoparticles deposited on transition metal oxides. Acc. Chem. Res. 46, 1773–1782. doi: 10.1021/ar300259n
Alamillo, R., Tucker, M., Chia, M., Pagán-Torres, Y., and Dumesic, J. A. (2012). The selective hydrogenation of biomass-derived 5-hydroxymethylfurfural using heterogeneous catalysts. Green Chem. 14, 1413–1419. doi: 10.1039/C2GC35039D
Aldosari, O. F., Iqbal, S., Miedziak, P. J., Brett, G. L., Jones, D. R., Liu, X., et al. (2016). Pd-Ru/TiO2 catalyst-an active and selective catalyst for furfural hydrogenation. Catal. Sci. Technol. 6, 234–242. doi: 10.1039/C5CY01650A
An, K., Musselwhite, N., Kennedy, G., Pushkarev, V. V., Baker, L. R., and Somorjai, G. A. (2013). Preparation of mesoporous oxides and their support effects on Pt nanoparticle catalysts in catalytic hydrogenation of furfural. J. Colloid Interf. Sci. 392, 122–128. doi: 10.1016/j.jcis.2012.10.029
Audemar, M., Vigier, K. D. O., Clacens, J. M., De Campo, F., and Jérôme, F. (2014). Combination of Pd/C and Amberlyst-15 in a single reactor for the acid/hydrogenating catalytic conversion of carbohydrates to 5-hydroxy-2, 5-hexanedione. Green Chem. 16, 4110–4114. doi: 10.1039/c4gc01158a
Baijun, L., Lianhai, L., Bingchun, W., Tianxi, C., and Iwatani, K. (1998). Liquid phase selective hydrogenation of furfural on Raney nickel modified by impregnation of salts of heteropolyacids. Appl. Catal. A Gen. 171, 117–122. doi: 10.1016/S0926-860X(98)00081-7
Balakrishnan, M., Sacia, E. R., and Bell, A. T. (2012). Etherification and reductive etherification of 5-(hydroxymethyl) furfural: 5-(alkoxymethyl) furfurals and 2, 5-bis (alkoxymethyl) furans as potential bio-diesel candidates. Green Chem. 14, 1626–1634. doi: 10.1039/C2GC35102A
Barr, J. B., and Wallon, S. B. (1971). The chemistry of furfuryl alcohol resins. J. Appl. Polym. Sci. 15, 1079–1090. doi: 10.1002/app.1971.070150504
Besson, M., and Gallezot, P., Pinel, C. (2013). Conversion of biomass into chemicals over metal catalysts. Chem. Rev., 114, 1827–1870. doi: 10.1021/cr4002269
Bhanja, P., and Bhaumik, A. (2016). Organic-inorganic hybrid metal phosphonates as recyclable heterogeneous catalysts. ChemCatChem, 8, 1607–1616. doi: 10.1002/cctc.201501303
Biradar, N. S., Hengne, A. M., Birajdar, S. N., Niphadkar, P. S., Joshi, P. N., and Rode, C. V. (2013). Single-pot formation of THFAL via catalytic hydrogenation of FFR over Pd/MFI catalyst. ACS Sustain. Chem. Eng. 2, 272–281. doi: 10.1021/sc400302b
Biradar, N. S., Hengne, A. M., Sakate, S. S., Swami, R. K., and Rode, C. V. (2016). Single pot transfer hydrogenation and aldolization of furfural over metal oxide catalysts. Catal. Lett. 146, 1611–1619. doi: 10.1007/s10562-016-1786-6
Bui, L., Luo, H., Gunther, W. R., and Román-Leshkov, Y. (2013). Domino reaction catalyzed by zeolites with Brønsted and Lewis acid sites for the production of γ-valerolactone from furfural. Angew. Chem. Int. Ed. Engl. 125, 8180–8183. doi: 10.1002/anie.201302575
Burch, R., Golunski, S. E., and Spencer, M. S. (1990). The role of copper and zinc oxide in methanol synthesis catalysts. J. Chem. Soc. Farad. Trans. 86, 2683–2691. doi: 10.1039/FT9908602683
Cai, C. M., Nagane, N., Kumar, R., and Wyman, C. E. (2014b). Coupling metal halides with a co-solvent to produce furfural and 5-HMF at high yields directly from lignocellulosic biomass as an integrated biofuels strategy. Green Chem. 16, 3819–3829. doi: 10.1039/C4GC00747F
Cai, C. M., Zhang, T., Kumar, R., and Wyman, C. E. (2014a). Integrated furfural production as a renewable fuel and chemical platform from lignocellulosic biomass. J. Chem. Technol. Biot. 89, 2–10. doi: 10.1002/jctb.4168
Cárdenas-Lizana, F., and Keane, M. A. (2013). The development of gold catalysts for use in hydrogenation reactions. J. Mater. Sci. 48, 543–564. doi: 10.1007/s10853-012-6766-7
Castelbou, J. L., Szeto, K. C., Barakat, W., Merle, N., Godard, C., Taoufik, M., et al. (2017). A new approach for the preparation of well-defined Rh and Pt nanoparticles stabilized by phosphine-functionalized silica for selective hydrogenation reactions. Chem. Commun. 53, 3261–3264. doi: 10.1039/C6CC10338C
Chatterjee, M., Ishizaka, T., and Kawanami, H. (2014). Selective hydrogenation of 5-hydroxymethylfurfural to 2,5-bis-(hydroxymethyl)furan using Pt/MCM-41 in an aqueous medium: a simple approach. Green Chem. 16, 4734–4739. doi: 10.1039/C4GC01127A
Chen, B., Li, F., Huang, Z., and Yuan, G. (2017). Carbon-coated Cu-Co bimetallic nanoparticles as selective and recyclable catalysts for production of biofuel 2,5-dimethylfuran. Appl. Catal. B Environ. 200, 192–199. doi: 10.1016/j.apcatb.2016.07.004
Chen, J., Li, K., Chen, L., Liu, R., Huang, X., and Ye, D. (2014a). Conversion of fructose into 5-hydroxymethylfurfural catalyzed by recyclable sulfonic acid-functionalized metal–organic frameworks. Green Chem. 16, 2490–2499. doi: 10.1039/C3GC42414F
Chen, J., Liu, R., Guo, Y., Chen, L., and Gao, H. (2014b). Selective hydrogenation of biomass-based 5-hydroxymethylfurfural over catalyst of palladium immobilized on amine-functionalized metal–organic frameworks. ACS Catal. 5, 722–733. doi: 10.1021/cs5012926
Chen, J. Z., Lu, F., Zhang, J. J., Yu, W. Q., Wang, F., Gao, J., et al. (2013). Immobilized Ru clusters in nanosized mesoporous zirconium silica for the aqueous hydrogenation of furan derivatives at room temperature. ChemCatChem 5, 2822–2826. doi: 10.1002/cctc.201300316
Chen, K., Mori, K., Watanabe, H., Nakagawa, Y., and Tomishige, K. (2012). C–O bond hydrogenolysis of cyclic ethers with OH groups over rhenium-modified supported iridium catalysts. J. Catal. 294, 171–183. doi: 10.1016/j.jcat.2012.07.015
Chen, X., Li, H., Luo, H., and Qiao, M. (2002). Liquid phase hydrogenation of furfural to furfuryl alcohol over Mo-doped Co-B amorphous alloy catalysts. Appl. Catal. A Gen. 233, 13–20. doi: 10.1016/S0926-860X(02)00127-8
Chen, X., Zhang, L., Zhang, B., Guo, X., and Mu, X. (2016). Highly selective hydrogenation of furfural to furfuryl alcohol over Pt nanoparticles supported on g-C3N4 nanosheets catalysts in water. Sci. Rep. 6:28558. doi: 10.1038/srep28558
Choudhary, V., Pinar, A. B., Sandler, S. I., Vlachos, D. G., and Lobo, R. F. (2011). Xylose isomerization to xylulose and its dehydration to furfural in aqueous media. ACS Catal. 1, 1724–1728. doi: 10.1021/cs200461t
Climent, M. J., Corma, A., and Iborra, S. (2014). Conversion of biomass platform molecules into fuel additives and liquid hydrocarbon fuels. Green Chem. 16, 516–547. doi: 10.1039/C3GC41492B
Corma, A., Iborra, S., and Velty, A. (2007). Chemical routes for the transformation of biomass into chemicals. Chem. Rev. 107, 2411–2502. doi: 10.1021/cr050989d
Cui, X., Surkus, A.-E., Junge, K., Topf, C., Radnik, J., Kreyenschulte, C., et al. (2016). Highly selective hydrogenation of arenes using nanostructured ruthenium catalysts modified with a carbon-nitrogen matrix. Nat. Commun. 7:11326. doi: 10.1038/ncomms11326
Date, N. S., Biradar, N. S., Chikate, R. C., and Rode, C. V. (2017). Effect of reduction protocol of Pd catalysts on product distribution in furfural hydrogenation. ChemistrySelect 2, 24–32. doi: 10.1002/slct.201601790
Date, N. S., Parola, V. L., Rode, C. V., and Testa, M. L. (2018). Ti-doped Pd-Au catalysts for one-pot hydrogenation and ring opening of furfural. Catalysts 8:252. doi: 10.3390/catal8060252
de los Reyes, M., Majewski, P. J., Scales, N., and Luca, V. (2013). Hydrolytic stability of mesoporous zirconium titanate frameworks containing coordinating organic functionalities. ACS Appl. Mater. Interfaces 5, 4120–4128. doi: 10.1021/am3031695
De, S., Dutta, S., and Saha, B. (2016a). Critical design of heterogeneous catalysts for biomass valorization: current thrust and emerging prospects. Catal. Sci. Technol. 6, 7364–7385. doi: 10.1039/C6CY01370H
De, S., Zhang, J., Luque, R., and Yan, N. (2016b). Ni-based bimetallic heterogeneous catalysts for energy and environmental applications. Energy Environ. Sci. 9, 3314–3347. doi: 10.1039/C6EE02002J
Dohade, M. G., and Dhepe, P. L. (2017). Efficient hydrogenation of concentrated aqueous furfural solutions into furfuryl alcohol under ambient conditions in presence of PtCo bimetallic catalyst. Green Chem. 19, 1144–1154. doi: 10.1039/C6GC03143A
Dong, F., Zhu, Y., Zheng, H., Zhu, Y., Li, X., and Li, Y. (2015). Cr-free Cu-catalysts for the selective hydrogenation of biomass-derived furfural to 2-methylfuran: the synergistic effect of metal and acid sites. J. Mol. Catal. A: Chem. 398, 140–148. doi: 10.1016/j.molcata.2014.12.001
Du, C. H. (2011). Preparation of NiCoB/SiO2 nanoalloy catalysts and their catalytic hydrogenation performance. Adv. Mater. Res. 194–196, 652–655. doi: 10.4028/www.scientific.net/AMR
Du, J., Zhang, J., Sun, Y., Jia, W., Si, Z., Gao, H., et al. (2018). Catalytic transfer hydrogenation of biomass-derived furfural to furfuryl alcohol over in-situ prepared nano Cu-Pd/C catalyst using formic acid as hydrogen source. J. Catal. 368, 69–78. doi: 10.1016/j.jcat.2018.09.025
Durndell, L. J., Parlett, C. M., Hondow, N. S., Isaacs, M. A., Wilson, K., and Lee, A. F. (2015). Selectivity control in Pt-catalyzed cinnamaldehyde hydrogenation. Sci. Rep. 5:9425. doi: 10.1038/srep09425
Fei, J. H., Yang, M. X., Hou, Z. Y., and Zheng, X. M. (2004). Effect of the addition of manganese and zinc on the properties of copper-based catalyst for the synthesis of syngas to dimethyl ether. Energy Fuels 18, 1584–1587. doi: 10.1021/ef049961f
Fulajtárova, K., Soták, T., Hronec, M., Vávra, I., Dobročka, E., and Omastová, M. (2015). Aqueous phase hydrogenation of furfural to furfuryl alcohol over Pd-Cu catalysts. Appl. Catal. A Gen. 502, 78–85. doi: 10.1016/j.apcata.2015.05.031
Gabriëls, D., Hernández, W. Y., Sels, B., Van Der Voort, P., and Verberckmoes, A. (2015). Review of catalytic systems and thermodynamics for the Guerbet condensation reaction and challenges for biomass valorization. Catal. Sci. Technol. 5, 3876–3902. doi: 10.1039/C5CY00359H
Gandarias, I., Fernández, S. G., Doukkali, M., Requies, J., and Arias, P. L. (2013). Physicochemical study of glycerol hydrogenolysis over a Ni-Cu/Al2O3 catalyst using formic acid as the hydrogen source. Top. Catal. 56, 995–1007. doi: 10.1007/s11244-013-0063-9
Gandarias, I., Requies, J., Arias, P. L., Armbruster, U., and Martin, A. (2012). Liquid-phase glycerol hydrogenolysis by formic acid over Ni-Cu/Al2O3 catalysts. J. Catal. 290, 79–89. doi: 10.1016/j.jcat.2012.03.004
Gao, Z., Yang, L., Fan, G. L., and Li, F. (2016). Promotional role of surface defects on carbon-supported Ru-based catalysts in transfer hydrogenation of furfural. ChemCatChem 8, 3769–3779. doi: 10.1002/cctc.201601070
Garcia-Olmo, A. J., Yepez, A., Balu, A. M., Romero, A. A., Li, Y., and Luque, R. (2016). Insights into the activity, selectivity and stability of heterogeneous catalysts in the continuous flow hydroconversion of furfural. Catal. Sci. Technol. 6, 4705–4711. doi: 10.1039/C6CY00249H
Ghashghaee, M., Sadjadi, S., Shirvani, S., and Farzaneh, V. (2017). A novel consecutive approach for the preparation of Cu-MgO catalysts with high activity for hydrogenation of furfural to furfuryl alcohol. Catal. Lett. 147, 318–327. doi: 10.1007/s10562-016-1948-6
Gilkey, M. J., and Xu, B. (2016). Heterogeneous catalytic transfer hydrogenation as an effective pathway in biomass upgrading. ACS Catal. 6, 1420–1436. doi: 10.1021/acscatal.5b02171
Gong, W., Chen, C., Zhang, H., Zhang, Y., Zhang, Y., Wang, G., et al. (2017). Highly selective liquid-phase hydrogenation of furfural over N-doped carbon supported metallic nickel catalyst under mild conditions. Mol. Catal. 429, 51–59. doi: 10.1016/j.molcata.2016.12.004
Guan, H., Iimura, M., Magee, M. P., Norton, J. R., and Zhu, G. (2005). Ruthenium-catalyzed ionic hydrogenation of iminium cations. Scope and mechanism. J. Am. Chem. Soc. 127, 7805–7814. doi: 10.1021/ja0506861
Gupta, D., and Saha, B. (2017). Carbon nanosphere supported Ru catalyst for the synthesis of renewable herbicide and chemicals. Catal. Commun. 100, 206–209. doi: 10.1016/j.catcom.2017.07.005
Hájek, J., Kumar, N., Mäki-Arvela, P., Salmi, T, and Murzin, D. Y. (2004). Selective hydrogenation of cinnamaldehyde over Ru/Y zeolite. J. Mol. Catal. A Chem. 217, 145–154. doi: 10.1016/j.molcata.2004.03.015
Halilu, A., Ali, T. H., Atta, A. Y., Sudarsanam, P., Bhargava, S. K., and Abd Hamid, S. B. (2016). Highly selective hydrogenation of biomass-derived furfural into furfuryl alcohol using a novel magnetic nanoparticles catalyst. Energy Fuels 30, 2216–2226. doi: 10.1021/acs.energyfuels.5b02826
Han, J. S., Kim, Y. H., Jang, H. S., Hwang, S. Y., Jegal, J., Kim, J. W., et al. (2016). Heterogeneous zirconia-supported ruthenium catalyst for highly selective hydrogenation of 5-hydroxymethyl-2-furaldehyde to 2,5-bis(hydroxymethyl)furans in various n-alcohol solvents. RSC Adv. 6, 93394–93397. doi: 10.1039/C6RA18016G
Han, X., Li, C., Liu, X., Xia, Q., and Wang, Y. (2017). Selective oxidation of 5-hydroxymethylfurfural to 2, 5-furandicarboxylic acid over MnOx-CeO2 composite catalysts. Green Chem. 19, 996–1004. doi: 10.1039/C6GC03304K
Hao, W., Li, W., Tang, X., Zeng, X., Sun, Y., Liu, S., et al. (2016). Catalytic transfer hydrogenation of biomass-derived 5-hydroxymethyl furfural to the building block 2,5-bishydroxymethyl furan. Green Chem. 18, 1080–1088. doi: 10.1039/C5GC01221J
He, J., Li, H., Riisager, A., and Yang, S. (2018a). Catalytic transfer hydrogenation of furfural to furfuryl alcohol with recyclable Al–Zr@ Fe mixed oxides. ChemCatChem 10, 430–438. doi: 10.1002/cctc.201701266
He, J., Li, H., Saravanamurugan, S., and Yang, S. (2019a). Catalytic upgrading of biomass-derived sugars with acidic nanoporous materials: structural role in carbon-chain length variation. ChemSusChem 12, 347–378. doi: 10.1002/cssc.201802113
He, J., Nielsen, M. R., Hansen, T. W., Yang, S., and Riisager, A. (2019b). Hierarchically constructed NiO with improved performance for catalytic transfer hydrogenation of biomass-derived aldehydes. Catal. Sci. Technol. 9, 1289–1300. doi: 10.1039/C8CY02536C
He, J., Schill, L., Yang, S., and Riisager, A. (2018b). Catalytic transfer hydrogenation of bio-based furfural with NiO nanoparticles. ACS Sustain. Chem. Eng. 6, 17220–17229. doi: 10.1021/acssuschemeng.8b04579
Hronec, M., Fulajtarová, K., and Liptaj, T. (2012). Effect of catalyst and solvent on the furan ring rearrangement to cyclopentanone. Appl. Catal. A 437, 104–111. doi: 10.1016/j.apcata.2012.06.018
Hu, L., Lin, L., and Liu, S. (2014). Chemoselective hydrogenation of biomass-derived 5-hydroxymethylfurfural into the liquid biofuel 2,5-dimethylfuran. Ind. Eng. Chem. Res. 53, 9969–9978. doi: 10.1021/ie5013807
Hu, L., Yang, M., Xu, N., Xu, J. X., Zhou, S. Y., Chu, X. Z., et al. (2018). Selective transformation of biomass-derived 5-hydroxymethylfurfural into 2,5-dihydroxymethylfuran via catalytic transfer hydrogenation over magnetic zirconium hydroxides. Korean J. Chem. Eng. 35, 99–109. doi: 10.1007/s11814-017-0238-3
Hu, S., Zhang, Z., Song, J., Zhou, Y., and Han, B. (2009). Efficient conversion of glucose into 5-hydroxymethylfurfural catalyzed by a common Lewis acid SnCl4 in an ionic liquid. Green Chem. 11, 1746–1749. doi: 10.1039/B914601F
Huang, R., Cui, Q., Yuan, Q., Wu, H., Guan, Y., and Wu, P. (2018). Total hydrogenation of furfural over Pd/Al2O3 and Ru/ZrO2 mixture under mild conditions: essential role of tetrahydrofurfural as an intermediate and support effect. ACS Sustainable Chem. Eng. 6, 6957–6964. doi: 10.1021/acssuschemeng.8b00801
Huang, S., Yang, N., Wang, S., Sun, Y., and Zhu, Y. (2016). Tuning the synthesis of platinum–copper nanoparticles with a hollow core and porous shell for the selective hydrogenation of furfural to furfuryl alcohol. Nanoscale 8, 14104–14108. doi: 10.1039/C6NR03894H
Huang, W., Li, H., Zhu, B., Feng, Y., Wang, S., and Zhang, S. (2007). Selective hydrogenation of furfural to furfuryl alcohol over catalysts prepared via sonochemistry. Ultrason. Sonochem. 14, 67–74. doi: 10.1016/j.ultsonch.2006.03.002
Huang, Y. L., Xu, S., and Lin, V. S. Y. (2011). Bifunctionalized mesoporous materials with site-separated brønsted acids and bases: catalyst for a two-step reaction sequence. Angew. Chem. Int. Ed. Engl. 123, 687–690. doi: 10.1002/anie.201004572
Jae, J., Zheng, W., Lobo, R. F., and Vlachos, D. G. (2013). Production of dimethylfuran from hydroxymethylfurfural through catalytic transfer hydrogenation with ruthenium supported on carbon. ChemSusChem 6, 1158–1162. doi: 10.1002/cssc.201300288
Jiménez-Gómez, C. P., Cecilia, J. A., Durán-Martín, D., Moreno-Tost, R., Santamaría-González, J., Mérida-Robles, J., et al. (2016). Gas-phase hydrogenation of furfural to furfuryl alcohol over Cu/ZnO catalysts. J. Catal. 336, 107–115. doi: 10.1016/j.jcat.2016.01.012
Jiménez-Gómez, C. P., Cecilia, J. A., Franco-Duro, F. I., Pozo, M., Moreno-Tost, R., and Maireles-Torres, P. (2018). Promotion effect of Ce or Zn oxides for improving furfuryl alcohol yield in the furfural hydrogenation using inexpensive Cu-based catalysts. Mol. Catal. 455, 121–131. doi: 10.1016/j.mcat.2018.06.001
Kai, D., Tan, M. J., Chee, P. L., Chua, Y. K., Yap, Y. L., and Loh, X. J. (2016). Towards lignin-based functional materials in a sustainable world. Green Chem. 18, 1175–1200. doi: 10.1039/C5GC02616D
Khan, F. A., Vallat, A., and Süss-Fink, G. (2011). Highly selective low-temperature hydrogenation of furfuryl alcohol to tetrahydrofurfuryl alcohol catalysed by hectorite-supported ruthenium nanoparticles. Catal. Commun. 12, 1428–1431. doi: 10.1016/j.catcom.2011.05.024
Khromova, S. A., Bykova, M. V., Bulavchenko, O. A., Ermakov, D. Y., Saraev, A. A., Kaichev, V. V., et al. (2016). Furfural hydrogenation to furfuryl alcohol over bimetallic Ni-Cu sol-gel catalyst: a model reaction for conversion of oxygenates in pyrolysis liquids. Top. Catal. 59, 1413–1423. doi: 10.1007/s11244-016-0649-0
Kokel, A., Schäfer, C., and Török, B. (2017). Application of microwave-assisted heterogeneous catalysis in sustainable synthesis design. Green Chem. 19, 3729–3751. doi: 10.1039/C7GC01393K
Kotbagi, T. V., Gurav, H. R., Nagpure, A. S., Chilukuri, S. V., and Bakker, M. G. (2016). Highly efficient nitrogen-doped hierarchically porous carbon supported Ni nanoparticles for the selective hydrogenation of furfural to furfuryl alcohol. RSC Adv. 6, 67662–67668. doi: 10.1039/C6RA14078E
Kumalaputri, A. J., Bottari, G., Erne, P. M., Heeres, H. J., and Barta, K. (2014). Tunable and selective conversion of 5-HMF to 2,5-furandimethanol and 2,5-dimethylfuran over copper-doped porous metal oxides. ChemSusChem 7, 2266–2275. doi: 10.1002/cssc.201402095
Kumar, G., Muthukumar, M. A., and Sekar, G. (2017). A mild and chemoselective hydrosilylation of α-keto amides by using a Cs2CO3/PMHS/2-MeTHF system. J. Org. Chem. 33, 4883–4890. doi: 10.1002/ejoc.201700374
Kwon, Y., de Jong, E., Raoufmoghaddam, S., and Koper, M. T. (2013). Electrocatalytic hydrogenation of 5-hydroxymethylfurfural in the absence and presence of glucose. ChemSusChem 6, 1659–1667. doi: 10.1002/cssc.201300443
Lesiak, M., Binczarski, M., Karski, S., Maniukiewicz, W., Rogowski, J., Szubiakiewicz, E., et al. (2014). Hydrogenation of furfural over Pd-Cu/Al2O3 catalysts. The role of interaction between palladium and copper on determining catalytic properties. J. Mol. Catal. A Chem. 395, 337–348. doi: 10.1016/j.molcata.2014.08.041
Li, H., Chai, W. M., Luo, H. S., and Li, H. X. (2006). Hydrogenation of furfural to furfuryl alcohol over Co-B amorphous catalysts prepared by chemical reduction in variable media. Chin. J. Chem. 24, 1704–1708. doi: 10.1002/cjoc.200690319
Li, H., Fang, Z., He, J., and Yang, S. (2017a). Orderly layered Zr-benzylphosphonate nanohybrids for efficient acid-base-mediated bifunctional/cascade catalysis. ChemSusChem, 10, 681–686. doi: 10.1002/cssc.201601570
Li, H., He, J., Riisager, A., Saravanamurugan, S., Song, B., and Yang, S. (2016a). Acid-base bifunctional zirconium N-alkyltriphosphate nanohybrid for hydrogen transfer of biomass-derived carboxides. ACS Catal. 6, 7722–7727. doi: 10.1021/acscatal.6b02431
Li, H., Li, Y., Fang, Z., and Smith, R. L. Jr. (2019). Efficient catalytic transfer hydrogenation of biomass-based furfural to furfuryl alcohol with recycable Hf-phenylphosphonate nanohybrids. Catal. Today 319, 84–92. doi: 10.1016/j.cattod.2018.04.056
Li, H., Liu, X., Yang, T., Zhao, W., and Saravanamurugan, S., Yang, S. (2017b). Porous zirconium-furandicarboxylate microspheres for efficient redox conversion of biofuranics. ChemSusChem 10, 1761–1770. doi: 10.1002/cssc.201601898
Li, H., Luo, H., Zhuang, L., Dai, W., and Qiao, M. (2003). Liquid phase hydrogenation of furfural to furfuryl alcohol over the Fe-promoted Ni-B amorphous alloy catalysts. J. Mol. Catal. A Chem. 203, 267–275. doi: 10.1016/S1381-1169(03)00368-6
Li, H., Yang, S., Riisager, A., Pandey, A., Sangwan, R. S., Saravanamurugan, S., et al. (2016b). Zeolite and zeotype-catalysed transformations of biofuranic compounds. Green Chem. 18, 5701–5735. doi: 10.1039/C6GC02415G
Li, H., Yang, T., and Fang, Z. (2018a). Biomass-derived mesoporous Hf-containing hybrid for efficient Meerwein-Ponndorf-Verley reduction at low temperatures. Appl. Catal. B Environ. 227, 79–89. doi: 10.1016/j.apcatb.2018.01.017
Li, H., Zhao, W., Dai, W., Long, J., Watanabe, M., Meier, S., et al. (2018b). Noble metal-free upgrading of multi-unsaturated biomass derivatives at room temperature: Silyl species enable reactivity. Green Chem. 20, 5327–5335. doi: 10.1039/C8GC02934B
Li, H., Zhao, W., Saravanamurugan, S., Dai, W., He, J., Meier, S., et al. (2018c). Control of selectivity in hydrosilane-promoted heterogeneous palladium-catalysed reduction of furfural and aromatic carboxides. Commun. Chem. 1, 32. doi: 10.1038/s42004-018-0033-z
Li, J., Liu, J. L., Zhou, H. J., and Fu, Y. (2016c). Catalytic transfer hydrogenation of furfural to furfuryl alcohol over nitrogen-doped carbon-supported iron catalysts. ChemSusChem 9, 1339–1347. doi: 10.1002/cssc.201600089
Li, M., Hao, Y., Cárdenas-Lizana, F., and Keane, M. A. (2015). Selective production of furfuryl alcohol via gas phase hydrogenation of furfural over Au/Al2O3. Catal. Commun. 69, 119–122. doi: 10.1016/j.catcom.2015.06.007
Li, X., Jia, P., and Wang, T. (2016d). Furfural: a promising platform compound for sustainable production of C4 and C5 chemicals. ACS Catal. 6, 7621–7640. doi: 10.1021/acscatal.6b01838
Li, X. L., Zhang, K., Chen, S. Y., Li, C., Li, F., Xu, H. J., et al. (2018d). A cobalt catalyst for reductive etherification of 5-hydroxymethyl-furfural to 2,5-bis(methoxymethyl)furan under mild conditions. Green Chem. 20, 1095. doi: 10.1039/C7GC03072J
Li, Y. P., Head-Gordon, M., and Bell, A. T. (2014). Analysis of the reaction mechanism and catalytic activity of metal-substituted beta zeolite for the isomerization of glucose to fructose. ACS Catal. 4, 1537–1545. doi: 10.1021/cs401054f
Lin, Y. C., and Huber, G. W. (2009). The critical role of heterogeneous catalysis in lignocellulosic biomass conversion. Energy Environ. Sci. 2, 68–80. doi: 10.1039/B814955K
Liu, B., and Zhang, Z. (2015). Catalytic conversion of biomass into chemicals and fuels over magnetic catalysts. ACS Catal. 6, 326–338. doi: 10.1021/acscatal.5b02094
Liu, E., Locke, A. J., Frost, R. L., and Martens, W. N. (2012). Sulfated fibrous ZrO2/Al2O3 core and shell nanocomposites: a novel strong acid catalyst with hierarchically macro-mesoporous nanostructure. J. Mol. Catal. A Chem. 353, 95–105. doi: 10.1016/j.molcata.2011.11.010
Liu, L., Lou, H., and Chen, M. (2018). Selective hydrogenation of furfural over Pt based and Pd based bimetallic catalysts supported on modified multiwalled carbon nanotubes (MWNT). Appl. Catal. A Gen. 550, 1–10. doi: 10.1016/j.apcata.2017.10.003
Long, J., Zhao, W., Xu, Y., Li, H., and Yang, S. (2018). Carbonate-catalyzed room-temperature selective reduction of biomass-derived 5-hydroxymethylfurfural into 2, 5-bis(hydroxymethyl) furan. Catalysts 8:633. doi: 10.3390/catal8120633
Long, J., Zhao, W., Xu, Y., Wu, W., Fang, C., Li, H., et al. (2019). Low-temperature catalytic hydrogenation of bio-based furfural and relevant aldehydes using cesium carbonate and hydrosiloxane. RSC Adv. 9, 3063–3071. doi: 10.1039/C8RA08616H
Lu, G., Zhang, P., Sun, D., Wang, L., Zhou, K., Wang, Z. X., et al. (2014). Gold catalyzed hydrogenations of small imines and nitriles: enhanced reactivity of Au surface toward H2 via collaboration with a Lewis base. Chem. Sci. 5, 1082–1090. doi: 10.1039/C3SC52851K
Luo, J., Yu, J., Gorte, R. J., Mahmoud, E., Vlachos, D. G., and Smith, M. A. (2014). The effect of oxide acidity on HMF etherification. Catal. Sci. Technol. 4, 3074–3081. doi: 10.1039/C4CY00563E
Ma, T. Y., and Yuan, Z. Y. (2011). Metal phosphonate hybrid mesostructures: environmentally friendly multifunctional materials for clean energy and other applications. ChemSusChem 4, 1407–1419. doi: 10.1002/cssc.201100050
Mäki-Arvela, P., Hájek, J., Salmi, T., and Murzin, D. Y. (2005). Chemoselective hydrogenation of carbonyl compounds over heterogeneous catalysts. Appl. Catal. A Gen. 292, 1–49. doi: 10.1016/j.apcata.2005.05.045
Marcotullio, G., and De Jong, W. (2010). Chloride ions enhance furfural formation from D-xylose in dilute aqueous acidic solutions. Green Chem. 12, 1739–1746. doi: 10.1039/B927424C
Mariscal, R., Maireles-Torres, P., Ojeda, M., Sádaba, I., and Granados, M. L. (2016). Furfural: a renewable and versatile platform molecule for the synthesis of chemicals and fuels. Energy Environ. Sci. 9, 1144–1189. doi: 10.1039/C5EE02666K
Merat, N., Godawa, C., and Gaset, A. (1990). High selective production of tetrahydrofurfuryl alcohol: catalytic hydrogenation of furfural and furfuryl alcohol. J. Chem. Technol. Biot. 48, 145–159. doi: 10.1002/jctb.280480205
Mihalcik, D. J., and Lin, W. B. (2008). Mesoporous silica nanosphere supported ruthenium catalysts for asymmetric hydrogenation. Angew. Chem. Int. Ed. Engl. 120, 6325–6328. doi: 10.1002/anie.200705656
Mironenko, R. M., Belskaya, O. B., Gulyaeva, T. I., Nizovskii, A. I., Kalinkin, A. V., Bukhtiyarov, V. I., et al. (2015). Effect of the nature of carbon support on the formation of active sites in Pd/C and Ru/C catalysts for hydrogenation of furfural. Catal. Today 249, 145–152. doi: 10.1016/j.cattod.2014.10.037
Muthukumar, A., Mamillapalli, N. C., and Sekar, G. (2016). Potassium phosphate-catalyzed chemoselective reduction of α-keto amides: route to synthesize passerini adducts and 3-phenyloxindoles. Adv. Synth. Catal. 358, 643–652. doi: 10.1002/adsc.201500815
Nagaraja, B. M., Padmasri, A. H., Raju, B. D., and Rao, K. S. R. (2011). Production of hydrogen through the coupling of dehydrogenation and hydrogenation for the synthesis of cyclohexanone and furfuryl alcohol over different promoters supported on Cu–MgO catalysts. Int. J. Hydrogen Energy 36, 3417–3425. doi: 10.1016/j.ijhydene.2010.12.013
Nagaraja, B. M., Padmasri, A. H., Seetharamulu, P., Reddy, K. H. P., Raju, B. D., and Rao, K. R. (2007). A highly active Cu-MgO-Cr2O3 catalyst for simultaneous synthesis of furfuryl alcohol and cyclohexanone by a novel coupling route-Combination of furfural hydrogenation and cyclohexanol dehydrogenation. J. Mol. Catal. A Chem. 278, 29–37. doi: 10.1016/j.molcata.2007.07.045
Nagpure, A. S., Venugopal, A. K., Lucas, N., Manikandan, M., Thirumalaiswamy, R., and Chilukuri, S. (2015). Renewable fuels from biomass-derived compounds: Ru-containing hydrotalcites as catalysts for conversion of HMF to 2,5-dimethylfuran. Catal. Sci. Technol. 5, 1463–1472. doi: 10.1039/C4CY01376J
Nakagawa, Y., Takada, K., Tamura, M., and Tomishige, K. (2014). Total hydrogenation of furfural and 5-hydroxymethylfurfural over supported Pd-Ir alloy catalyst. ACS Catal. 4, 2718–2726. doi: 10.1021/cs500620b
Nishimura, S., Ikeda, N., and Ebitani, K. (2014). Selective hydrogenation of biomass-derived 5-hydroxymethylfurfural (HMF) to 2,5-dimethylfuran (DMF) under atmospheric hydrogen pressure over carbon supported PdAu bimetallic catalyst. Catal. Today 232, 89–98. doi: 10.1016/j.cattod.2013.10.012
Oestreich, M., and Hermeke, J. (2015). A unified survey of Si-H and H-H bond activation catalysed by electron-deficient boranes. Mohr. J. Chem. Soc. Rev. 44, 2202–2220. doi: 10.1039/C4CS00451E
Ohyama, J., Esaki, A., Yamamoto, Y., Arai, S., and Satsuma, A. (2013). Selective hydrogenation of 2-hydroxymethyl-5-furfural to 2,5-bis(hydroxymethyl)furan over gold sub-nanoclusters. RSC Adv. 3, 1033–1036. doi: 10.1039/C2RA22190J
Ohyama, J., Hayashi, Y., Ueda, K., Yamamoto, Y., Arai, S., and Satsuma, A. (2016). Effect of FeOx modification of Al2O3 on its supported Au catalyst for hydrogenation of 5-hydroxymethylfurfural. J. Phys. Chem. C. 120, 15129–15136. doi: 10.1021/acs.jpcc.6b01542
Osatiashtiani, A., Lee, A. F., and Wilson, K. (2017). Recent advances in the production of γ-valerolactone from biomass-derived feedstocks via heterogeneous catalytic transfer hydrogenation. J. Chem. Technol. Biotechnol. 92, 1125–1135. doi: 10.1002/jctb.5213
Ouyang, W., Yepez, A., Romero, A. A., and Luque, R. (2018). Towards industrial furfural conversion: Selectivity and stability of palladium and platinum catalysts under continuous flow regime. Catal. Today 308, 32–37. doi: 10.1016/j.cattod.2017.07.011
Panagiotopoulou, P., Martin, N., and Vlachos, D. G. (2015). Liquid-phase catalytic transfer hydrogenation of furfural over homogeneous Lewis Acid-Ru/C catalysts. ChemSusChem 8, 2046–2054. doi: 10.1002/cssc.201500212
Pasini, T., Lolli, A., Albonetti, S., Cavani, F., and Mella, M. (2014). Methanol as a clean and efficient H-transfer reactant for carbonyl reduction: scope, limitations, and reaction mechanism. J. Catal. 317, 206–219. doi: 10.1016/j.jcat.2014.06.023
Petrus, L., and Noordermeer, M. A. (2006). Biomass to biofuels, a chemical perspective. Green Chem. 8, 861–867. doi: 10.1039/B605036K
Pillai, U. R., and Deevi, S. (2006). Copper-zinc oxide and ceria promoted copper-zinc oxide as highly active catalysts for low temperature oxidation of carbon monoxide. Appl. Catal. B Environ. 65, 110–117. doi: 10.1016/j.apcatb.2005.12.020
Putro, W. S., Kojima, T., Hara, T., Ichikuni, N., and Shimazu, S. (2017). Selective hydrogenation of unsaturated carbonyls by Ni-Fe-based alloy catalysts. Catal. Sci. Technol. 7, 3637–3646. doi: 10.1039/C7CY00945C
Qi, X., Watanabe, M., Aida, T. M., and Smith, R. L. Jr. (2009). Efficient process for conversion of fructose to 5-hydroxymethylfurfural with ionic liquids. Green Chem. 11, 1327–1331. doi: 10.1039/B905975J
Rao, R., Dandekar, A., Baker, R. T. K., and Vannice, M. A. (1997). Properties of copper chromite catalysts in hydrogenation reactions. J. Catal. 171, 406–419. doi: 10.1006/jcat.1997.1832
Reddy, B. M., Reddy, G. K., Rao, K. N., Khan, A., and Ganesh, I. (2007). Silica supported transition metal-based bimetallic catalysts for vapour phase selective hydrogenation of furfuraldehyde. J. Mol. Catal. A Chem. 265, 276–282. doi: 10.1016/j.molcata.2006.10.034
Revunova, K., and Nikonov, G. I. (2015). Main group catalysed reduction of unsaturated bonds. Dalton Trans. 44, 840–866. doi: 10.1039/C4DT02024C
Ricard, E., and Guinot, H. M. (1929). Process for the Manufacture of Furfuryl Alcohol and Methylfurane. US1739919A. Washington, DC: SA des distilleries des Deux-Sevres.
Rojas-Buzo, S., García-García, P., and Corma, A. (2018). Catalytic transfer hydrogenation of biomass-derived carbonyls over hafnium-based metal–organic frameworks. ChemSusChem 11, 432–438. doi: 10.1002/cssc.201701708
Román-Leshkov, Y., Barrett, C. J., Liu, Z. Y., and Dumesic, J. A. (2007). Production of dimethylfuran for liquid fuels from biomass-derived carbohydrates. Nature 447, 982–985. doi: 10.1038/nature05923
Román-Leshkov, Y., Chheda, J.N., and Dumesic, J. A. (2006). Phase modifiers promote efficient production of hydroxymethylfurfural from fructose. Science 312, 1933–1937. doi: 10.1126/science.1126337
Romano, P. N., de Almeida, J. M., Carvalho, Y., Priecel, P., Falabella Sousa-Aguiar, E., and Lopez-Sanchez, J. A. (2016). Microwave-assisted selective hydrogenation of furfural to furfuryl alcohol employing a green and noble metal-free copper catalyst. ChemSusChem 9, 3387–3392. doi: 10.1002/cssc.201601398
Rudiansono, R., Takayoshi, H., Nobuyuki, I., and Shogo, S. (2014). Development of nanoporous Ni-Sn alloy and application for chemoselective hydrogenation of furfural to furfuryl alcohol. Bull. Chem. Reac. Eng. Catal. 9, 53–59. doi: 10.9767/bcrec.9.1
Sankar, M., Dimitratos, N., Miedziak, P. J., Wells, P. P., Kiely, C. J., and Hutchings, G. J. (2012). Designing bimetallic catalysts for a green and sustainable future. Chem. Soc. Rev. 41, 8099–8139. doi: 10.1039/C2CS35296F
Seemala, B., Cai, C.M., Wyman, C.E., and Christopher, P. (2017). Support induced control of surface composition in Cu-Ni/TiO2 catalysts enables high yield co-conversion of HMF and furfural to methylated furans. ACS Catal. 7, 4070–4082. doi: 10.1021/acscatal.7b01095
Seemala, B., Meng, X., Parikh, A., Nagane, N., Kumar, R., Wyman, C.E., et al. (2018). Hybrid catalytic biorefining of hardwood biomass to methylated furans and depolymerized technical lignin. ACS Sustain. Chem. Eng. 6, 10587–10594. doi: 10.1021/acssuschemeng.8b01930
Seo, G., and Chon, H. (1981). Hydrogenation of furfural over copper-containing catalysts. J. Catal. 67, 424–429. doi: 10.1016/0021-9517(81)90302-X
Serrano-Ruiz, J. C., Luque, R., and Sepúlveda-Escribano, A. (2011). Transformations of biomass-derived platform molecules: from high added-value chemicals to fuels via aqueous-phase processing. Chem. Soc. Rev. 40, 5266–5281. doi: 10.1039/C1CS15131B
Sharma, R. V., Das, U., Sammynaiken, R., and Dalai, A. K. (2013). Liquid phase chemo-selective catalytic hydrogenation of furfural to furfuryl alcohol. Appl. Catal. A Gen. 454, 127–136. doi: 10.1016/j.apcata.2012.12.010
Sheldon, R. A. (2005). Green solvents for sustainable organic synthesis: state of the art. Green Chem. 7, 267–278. doi: 10.1039/B418069K
Shi, J., Wang, Y., Yu, X., Du, W., and Hou, Z. (2016). Production of 2, 5-dimethylfuran from 5-hydroxymethylfurfural over reduced graphene oxides supported Pt catalyst under mild conditions. Fuel 163, 74–79. doi: 10.1016/j.fuel.2015.09.047
Shi, J., Zhang, M., Du, W., Ning, W., and Hou, Z. (2015). SnO2-isolated Pt3Sn alloy on reduced graphene oxide: an efficient catalyst for selective hydrogenation of C = O in unsaturated aldehydes. Catal. Sci. Technol. 5, 3108–3112. doi: 10.1039/C5CY00393H
Shirvani, S., Ghashghaee, M., Farzaneh, V., and Sadjadi, S. (2018). Influence of catalyst additives on vapor-phase hydrogenation of furfural to furfuryl alcohol on impregnated copper/magnesia. Biomass Conv. Bioref. 8, 79–86. doi: 10.1007/s13399-017-0244-z
Sitthisa, S., An, W., and Resasco, D. E. (2011a). Selective conversion of furfural to methylfuran over silica-supported NiFe bimetallic catalysts. J. Catal. 284, 90–101. doi: 10.1016/j.jcat.2011.09.005
Sitthisa, S., and Resasco, D. E. (2011). Hydrodeoxygenation of furfural over supported metal catalysts: a comparative study of Cu, Pd and Ni. Catal. Lett. 141, 784–791. doi: 10.1007/s10562-011-0581-7
Sitthisa, S., Sooknoi, T., Ma, Y., Balbuena, P. B., and Resasco, D. E. (2011b). Kinetics and mechanism of hydrogenation of furfural on Cu/SiO2 catalysts. J. Catal. 277, 1–13. doi: 10.1016/j.jcat.2010.10.005
Song, J., Zhou, B., Zhou, H., Wu, L., Meng, Q., Liu, Z., et al. (2015). Porous zirconium–phytic acid hybrid: a highly efficient catalyst for Meerwein–Ponndorf–Verley reductions. Angew. Chem. Int. Ed. 54, 9399–9403. doi: 10.1002/anie.201504001
Srivastava, S., Jadeja, G. C., and Parikh, J. (2017). Synergism studies on alumina-supported copper-nickel catalysts towards furfural and 5-hydroxymethylfurfural hydrogenation. J. Mol. Catal. A Chem. 426, 244–256. doi: 10.1016/j.molcata.2016.11.023
Srivastava, S., Solanki, N., Mohanty, P., Shah, K. A., Parikh, J. K., and Dalai, A. K. (2015). Optimization and kinetic studies on hydrogenation of furfural to furfuryl alcohol over SBA-15 supported bimetallic copper-cobalt catalyst. Catal. Lett. 145, 816–823. doi: 10.1007/s10562-015-1488-5
Sudarsanam, P., Zhong, R., Van den Bosch, S., Coman, S. M., Parvulescu, V. I., and Sels, B. F. (2018). Functionalised heterogeneous catalysts for sustainable biomass valorisation. Chem. Soc. Rev. 47, 8349–8402. doi: 10.1039/C8CS00410B
Svenningsen, G. S., Kumar, R., Wyman, C. E., and Christopher, P. (2018). Unifying mechanistic analysis of factors controlling selectivity in fructose dehydration to 5-hydroxymethylfurfural by homogeneous acid catalysts in aprotic solvents. ACS Catal. 8, 5591–5600. doi: 10.1021/acscatal.8b01197
Takagaki, A., Ohara, M., Nishimura, S., and Ebitani, K. (2010). One-pot formation of furfural from xylose via isomerization and successive dehydration reactions over heterogeneous acid and base catalysts. Chem. Lett. 39, 838–840. doi: 10.1246/cl.2010.838
Tamura, M., Tokonami, K., Nakagawa, Y., and Tomishige, K. (2013). Rapid synthesis of unsaturated alcohols under mild conditions by highly selective hydrogenation. Chem. Commun. 49, 7034–7036. doi: 10.1039/C3CC41526K
Taylor, M. J., Durndell, L. J., Isaacs, M. A., Parlett, C. M., Wilson, K., Lee, A. F., et al. (2016). Highly selective hydrogenation of furfural over supported Pt nanoparticles under mild conditions. Appl. Catal. B Environ. 180, 580–585. doi: 10.1016/j.apcatb.2015.07.006
Thananatthanachon, T., and Rauchfuss, T. B. (2010). Efficient route to hydroxymethylfurans from sugars via transfer hydrogenation. ChemSusChem 3, 1139–1141. doi: 10.1002/cssc.201000209
Townsend, T. M., Kirby, C., Ruff, A., and O'Connor, A. R. (2017). Transfer hydrogenation of aromatic and linear aldehydes catalyzed using Cp*Ir(pyridinesulfonamide)Cl complexes under base-free conditions. J. Organomet. Chem. 843, 7–13. doi: 10.1016/j.jorganchem.2017.05.004
Tuteja, J., Nishimura, S., and Ebitani, K. (2012). One-pot synthesis of furans from various saccharides using a combination of solid acid and base catalysts. Bull. Chem. Soc. Jpn. 85, 275–281. doi: 10.1246/bcsj.20110287
Upare, P. P., Hwang, Y. K., and Hwang, D. W. (2018). An integrated process for the production of 2, 5-dihydroxymethylfuran and its polymer from fructose. Green Chem. 20, 879–885. doi: 10.1039/C7GC03597G
Vargas-Hernández, D., Rubio-Caballero, J. M., Santamaría-González, J., Moreno-Tost, R., Merida-Robles, J. M., Perez-Cruz, M. A., et al. (2014). Furfuryl alcohol from furfural hydrogenation over copper supported on SBA-15 silica catalysts. J. Mol. Catal. A Chem. 383, 106–113. doi: 10.1016/j.molcata.2013.11.034
Villaverde, M. M., Bertero, N. M., and Garetto, T. F., Marchi, A. J. (2013). Selective liquid-phase hydrogenation of furfural to furfuryl alcohol over Cu-based catalysts. Catal. Today 213, 87–92. doi: 10.1016/j.cattod.2013.02.031
Volkov, A., Gustafson, K. P., Tai, C. W., Verho, O., Backvall, J. E., and Adolfsson, H. (2015). Mild deoxygenation of aromatic ketones and aldehydes over Pd/C using polymethylhydrosiloxane as the reducing agent. Angew. Chem. Int. Ed. 54, 5122–5126. doi: 10.1002/anie.201411059
Vu, H. H. T., Atabaev, T. S., Nguyen, N. D., Hwang, Y., and Kim, H. (2014). Luminescent core-shell Fe3O4@Gd2O3:Er3+, Li+ composite particles with enhanced optical properties. J. Sol-Gel Sci. Technol. 71, 391–395. doi: 10.1007/s10971-014-3382-9
Wang, C., Wang, L., Zhang, J., Wang, H., Lewis, J. P., and Xiao, F. S. (2016). Product selectivity controlled by zeolite crystals in biomass hydrogenation over a palladium catalyst. J. Am. Chem. Soc. 138, 7880–7883. doi: 10.1021/jacs.6b04951
Wang, F., and Zhang, Z. (2016). Catalytic transfer hydrogenation of furfural into furfuryl alcohol over magnetic γ-Fe2O3@ HAP catalyst. ACS Sustain. Chem. Eng. 5, 942–947. doi: 10.1021/acssuschemeng.6b02272
Wang, G. H., Deng, X., Gu, D., Chen, K., Tüysüz, H., Spliethoff, B., et al. (2016). Co3O4 nanoparticles supported on mesoporous carbon for selective transfer hydrogenation of α,β-unsaturated aldehydes. Angew. Chem. Int. Ed. 55, 11101–11105. doi: 10.1002/anie.201604673
Wang, G. H., Hilgert, J., Richter, F. H., Wang, F., Bongard, H. J., Spliethoff, B., et al. (2014). Platinum-cobalt bimetallic nanoparticles in hollow carbon nanospheres for hydrogenolysis of 5-hydroxymethylfurfural. Nat. Mater. 13, 293–300. doi: 10.1038/nmat3872
Wang, H., Li, X., Lan, X., and Wang, T. (2018). Supported ultrafine NiCo bimetallic alloy nanoparticles derived from bimetal–organic frameworks: a highly active catalyst for furfuryl alcohol hydrogenation. ACS Catal. 8, 2121–2128. doi: 10.1021/acscatal.7b03795
Wang, I., Chang, W. F., Shiau, R. J., Wu, J. C., and Chung, C. S. (1983). Nonoxidative dehydrogenation of ethylbenzene over TiO2-ZrO2 catalysts: I. Effect of composition on surface properties and catalytic activities. J. Catal. 83, 428–436. doi: 10.1016/0021-9517(83)90067-2
Wang, T., Zhang, J., Xie, W., Tang, Y., Guo, D., and Ni, Y. (2017). Catalytic transfer hydrogenation of biobased HMF to 2,5-bis-(hydroxymethyl) furan over Ru/Co3O4. Catalysts 7:92. doi: 10.3390/catal7030092
Wang, Y., Miao, Y., Li, S., Gao, L., and Xiao, G. (2017). Metal-organic frameworks derived bimetallic Cu-Co catalyst for efficient and selective hydrogenation of biomass-derived furfural to furfuryl alcohol. Mol. Catal. 436, 128–137. doi: 10.1016/j.mcat.2017.04.018
Wang, Z., Huang, L., Geng, L., Chen, R., Xing, W., Wang, Y., et al. (2015). Chemoselective transfer hydrogenation of aldehydes and ketones with a heterogeneous iridium catalyst in water. Catal. Lett. 145, 1008–1013. doi: 10.1007/s10562-014-1473-4
Wegst, U. G. K., Bai, H., Saiz, E., Tomsia, A. P., and Ritchie, R. O. (2015). Bioinspired structural materials. Nat. Mater. 14, 23–36. doi: 10.1038/nmat4089
Wei, J., Cao, X., Wang, T., Liu, H., Tang, X., Zeng, X., et al. (2018). Catalytic transfer hydrogenation of biomass-derived 5-hydroxymethylfurfural into 2,5-bis(hydroxymethyl)furan over tunable Zr-based bimetallic catalysts. Catal. Sci. Technol. 8, 4474–4484. doi: 10.1039/C8CY00500A
Wei, Z., Pan, R., Hou, Y., Yang, Y., and Liu, Y. (2015). Graphene-supported Pd catalyst for highly selective hydrogenation of resorcinol to 1,3-cyclohexanedione through giant π-conjugate interactions. Sci. Rep. 5:15664. doi: 10.1038/srep15664
Weingarten, R., Kim, Y. T., Tompsett, G. A., Fernandez, A., Han, K. S., Hagaman, E. W., et al. (2013). Conversion of glucose into levulinic acid with solid metal(IV) phosphate catalysts. J. Catal. 304, 123–134. doi: 10.1016/j.jcat.2013.03.023
Wu, J., Gao, G., Li, J., Sun, P., Long, X., and Li, F. (2017). Efficient and versatile CuNi alloy nanocatalysts for the highly selective hydrogenation of furfural. Appl. Catal. B Environ. 203, 227–236. doi: 10.1016/j.apcatb.2016.10.038
Wu, J., Shen, Y., Liu, C., Wang, H., Geng, C., and Zhang, Z. (2005). Vapor phase hydrogenation of furfural to furfuryl alcohol over environmentally friendly Cu-Ca/SiO2 catalyst. Catal. Commun. 6, 633–637. doi: 10.1016/j.catcom.2005.06.009
Wu, W., Zhao, W., Fang, C., Wang, Z., Yang, T., Li, H., et al. (2018). Quantitative hydrogenation of furfural to furfuryl alcohol with recyclable KF and hydrosilane at room temperature in minutes. Catal. Commun. 105, 6–10. doi: 10.1016/j.catcom.2017.11.005
Xu, C., Zheng, L., Liu, J., and Huang, Z. (2011). Furfural hydrogenation on nickel-promoted Cu-containing catalysts prepared from hydrotalcite-like precursors. Chin. J. Chem. 29, 691–697. doi: 10.1002/cjoc.201190142
Yan, K., Jarvis, C., Lafleur, T., Qiao, Y., and Xie, X. (2013a). Novel synthesis of Pd nanoparticles for hydrogenation of biomass-derived platform chemicals showing enhanced catalytic performance. RSC Adv. 3, 25865–25871. doi: 10.1039/C3RA43619E
Yan, K., Liao, J., Wu, X., and Xie, X. (2013b). A noble-metal free Cu-catalyst derived from hydrotalcite for highly efficient hydrogenation of biomass-derived furfural and levulinic acid. RSC Adv. 3, 3853–3856. doi: 10.1039/C3RA22158J
Yan, K., Wu, G., Lafleur, T., and Jarvis, C. (2014). Production, properties and catalytic hydrogenation of furfural to fuel additives and value-added chemicals. Renew. Sust. Energy Rev. 38, 663–676. doi: 10.1016/j.rser.2014.07.003
Yang, J., Zheng, H. Y., Zhu, Y. L., Zhao, G. W., Zhang, C. H., Teng, B. T., et al. (2004). Effects of calcination temperature on performance of Cu-Zn-Al catalyst for synthesizing γ-butyrolactone and 2-methylfuran through the coupling of dehydrogenation and hydrogenation. Catal. Commun. 5, 505–510. doi: 10.1016/j.catcom.2004.06.005
Yang, X., Meng, Q., Ding, G., Wang, Y., Chen, H., lei Zhu, Y., et al. (2018). Construction of novel Cu/ZnO-Al2O3 composites for furfural hydrogenation: the role of Al components. Appl. Catal. A Gen. 561, 78–86. doi: 10.1016/j.apcata.2018.04.005
Yang, Y., Hu, C. W., and Abu-Omar, M. M. (2012). Synthesis of furfural from xylose, xylan, and biomass using AlCl3·6H2O in biphasic media via xylose isomerization to xylulose. ChemSusChem 5, 405–410. doi: 10.1002/cssc.201100688
Yao, S. X., Wang, X. C., Jiang, Y. J., Wu, F., Chen, X. G., and Mu, X. D. (2014). One-step conversion of biomass-derived 5-hydroxymethylfurfural to 1,2,6-hexanetriol over Ni-Co-Al mixed oxide catalysts under mild conditions. ACS Sustain. Chem. Eng. 2, 173–180. doi: 10.1021/sc4003714
Yen, C. H., Lin, H. W., and Tan, C. S. (2011). Hydrogenation of bisphenol A-Using a mesoporous silica based nano ruthenium catalyst Ru/MCM-41 and water as the solvent. Catal. Today 174, 121–126. doi: 10.1016/j.cattod.2011.01.050
Yoskamtorn, T., Yamazoe, S., Takahata, R., Nishigaki, J.-I., Thivasasith, A., Limtrakul, J., et al. (2014). Thiolate-mediated selectivity control in aerobic alcohol oxidation by porous carbon-supported Au25 clusters. ACS Catal. 4, 3696–3700. doi: 10.1021/cs501010x
Yu, L. L., He, L., Chen, J., Zheng, J. W., Ye, L. M., Lin, H. Q., et al. (2015). Robust and recyclable nonprecious bimetallic nanoparticles on carbon nanotubes for the hydrogenation and hydrogenolysis of 5-hydroxymethylfurfural. ChemCatChem 7, 1701–1707. doi: 10.1002/cctc.201500097
Yu, W., Tang, Y., Mo, L., Chen, P., Lou, H., and Zheng, X. (2011). One-step hydrogenation–esterification of furfural and acetic acid over bifunctional Pd catalysts for bio-oil upgrading. Bioresour. Technol. 102, 8241–8246. doi: 10.1016/j.biortech.2011.06.015
Yuan, Q., Ye, F., Xue, T., and Guan, Y. (2015a). Room temperature hydrogenation of furfuryl alcohol by Pd/titanate nanotube. Appl. Catal. A Gen. 507, 26–33. doi: 10.1016/j.apcata.2015.09.034
Yuan, Q., Zhang, D., van Haandel, L., Ye, F., Xue, T., Hensen, E. J., et al. (2015b). Selective liquid phase hydrogenation of furfural to furfuryl alcohol by Ru/Zr-MOFs. J. Mol. Catal. A Chem. 406, 58–64. doi: 10.1016/j.molcata.2015.05.015
Zakrzewska, M. E., Bogel-Łukasik, E., and Bogel-Łukasik, R. (2011). Ionic liquid-mediated formation of 5-hydroxymethylfurfural-a promising biomass-derived building block. Chem. Rev. 111, 397–417. doi: 10.1021/cr100171a
Zhang, J., Xie, W., Liang, Q., Peng, L., and He, L. (2019). Nano-fibrillated cellulose as a versatile carrier of Ru/Cu nanoparticles for the catalytic transfer hydrogenation of 5-hydroxymethyfural to 2, 5-bishydroxymethylfuran. ChemistrySelect 4, 2846–2850. doi: 10.1002/slct.201900285
Zhang, Q., Li, S. S., Zhu, M. M., Liu, Y. M., He, H. Y., and Cao, Y. (2016). Direct reductive amination of aldehydes with nitroarenes using bio-renewable formic acid as a hydrogen source. Green Chem. 18, 2507–2513. doi: 10.1039/C5GC02583D
Zhao, H., Holladay, J. E., Brown, H., and Zhang, Z. C. (2007). Metal chlorides in ionic liquid solvents convert sugars to 5-hydroxymethylfurfural. Science 316, 1597–1600. doi: 10.1126/science.1141199
Zhao, M., Xie, W., and Cui, C. (2014). Cesium carbonate catalyzed chemoselective hydrosilylation of aldehydes and ketones under solvent-free conditions. Chem. Eur. J. 20, 9259–9262. doi: 10.1002/chem.201403497
Zhao, W., Wu, W., Li, H., Fang, C., Yang, T., Wang, Z., et al. (2018). Quantitative synthesis of 2,5-bis (hydroxymethyl) furan from biomass-derived 5-hydroxymethylfurfural and sugars over reusable solid catalysts at low temperatures. Fuel 217, 365–369. doi: 10.1016/j.fuel.2017.12.069
Zhou, H. C. J., and Kitagawa, S. (2014). Metal-organic frameworks (MOFs). Chem. Soc. Rev. 43, 5415–5418. doi: 10.1039/c4cs90059f
Zhou, P., and Zhang, Z. (2016). One-pot catalytic conversion of carbohydrates into furfural and 5-hydroxymethylfurfural. Catal. Sci. Technol. 6, 3694–3712. doi: 10.1039/C6CY00384B
Zhu, S., Xue, Y., Guo, J., Cen, Y., Wang, J., and Fan, W. (2016). Integrated conversion of hemicellulose and furfural into γ-valerolactone over Au/ZrO2 catalyst combined with ZSM-5. ACS Catal. 6, 2035–2042. doi: 10.1021/acscatal.5b02882
Keywords: heterogeneous catalysis, biomass conversion, carbohydrates, furfural, 5-hydroxymethylfurfural
Citation: Long J, Xu Y, Zhao W, Li H and Yang S (2019) Heterogeneous Catalytic Upgrading of Biofuranic Aldehydes to Alcohols. Front. Chem. 7:529. doi: 10.3389/fchem.2019.00529
Received: 04 June 2019; Accepted: 11 July 2019;
Published: 26 July 2019.
Edited by:
Juan Antonio Cecilia, Universidad de Málaga, SpainReviewed by:
Rajeev Kumar, University of California, Riverside, United StatesXianhai Zeng, Xiamen University, China
Copyright © 2019 Long, Xu, Zhao, Li and Yang. This is an open-access article distributed under the terms of the Creative Commons Attribution License (CC BY). The use, distribution or reproduction in other forums is permitted, provided the original author(s) and the copyright owner(s) are credited and that the original publication in this journal is cited, in accordance with accepted academic practice. No use, distribution or reproduction is permitted which does not comply with these terms.
*Correspondence: Hu Li, aGxpMTNAZ3p1LmVkdS5jbg==; Song Yang, amh6eC5tc21AZ21haWwuY29t