- Hebei Key Laboratory of Organic Functional Molecules, College of Chemistry and Material Science, Hebei Normal University, Shijiazhuang, China
Reaction of Ru3(CO)12 with two equiv of 6-bromopyridine alcohols 6-bromopyCHROH [(R = C6H5 (L1); R = 4-CH3C6H4 (L2); R = 4-OMeC6H4 (L3); R = 4-ClC6H4 (L4); (R = 4-CF3C6H4 (L5); R = 2-OMeC6H4 (L6); R = 2-CF3C6H4 (L7)] and 6-bromopyC(Me)2OH (L8) in refluxing xylene afforded novel trinuclear ruthenium complexes [6-bromopyCHRO]2Ru3(CO)8 (1a-1g) and [6-bromopyC(Me)2O]2Ru3(CO)8 (1h). These complexes were characterized by FT-IR and NMR spectroscopy as well as elemental analysis. The structures of all the complexes were further confirmed by X-ray crystallographic analysis. In the presence of tert-butyl hydroperoxide (TBHP) as the source of oxidant, complexes 1a-1h displayed high catalytic activities for oxidation of primary and secondary alcohols and most of oxidation reactions could be completed within 1 h at room temperature.
Introduction
As a class of common starting materials, alcohols can beeasily converted into a variety of useful compounds via organic synthesis methods (Salvatore et al., 2001; Crabtree, 2017). Among all transfer strategies, oxidation of alcohols into their corresponding carbonyl compounds is one of the fundamental and important chemical reactions (Sheldon et al., 2000, 2002; Mallat and Baiker, 2004; Vazylyev et al., 2005; Parmeggiani and Cardona, 2012; Cao et al., 2014; Wang et al., 2017) and the oxidation products, including aldehydes, ketones and carboxylic acids, are important building blocks for synthesis of pharmaceuticals and fine chemicals (Caron et al., 2006; Bianchini and Shen, 2009; Simon and Li, 2012; Balaraman et al., 2013). Conventional oxidation methods to access these compounds usually require stoichiometric amounts of inorganic oxidants, such as chromium(VI) compounds (Canielli and Cardillo, 1984; Tojo and Fernández, 2007), hypervalent iodine reagents (Uyanik and Ishihara, 2009) or radical oxidants i.e., N-methylmorpholine-N-oxide (NMO) (Kumar et al., 2007; Gunasekaran et al., 2011; Saleem et al., 2013), 2,2,6,6-tetramethyl-1-piperidinyloxyl (TEMPO) (Dijksman et al., 2001; Wang et al., 2008; Allen et al., 2013). Such reactions often result in the generation of numerous wastes which caused serious environment problems. To ease this issue, great efforts have been devoted to the development of atom-economic and green methods. Molecular oxygen is one of the green oxidants and the H2O is the only by-product (Punniyamurthy et al., 2005). But in most aerobic alcohol oxidation systems, the additives e.g., TEMPO and large amounts of base are also needed, which makes the reaction system more complicated (Wang et al., 2005; Kumpulainen and Koskinen, 2009; Hoover et al., 2012). In addition, H2O2 is also used as an environmentally benign oxidant (Campestrini et al., 2004; Zhou et al., 2013; Ren et al., 2015; Vermaak et al., 2018). However, due to its limited oxidation capacity, the catalytic system should be assisted by carboxylic acid or H2SO4 as an additive to achieve a high efficiency (Dai et al., 2015; Miao et al., 2017). Compared with above oxidants, tert-butyl hydroperoxide (TBHP) is an alternative suitable oxidant and widely used in oxidation reactions, particularly in olefin epoxidation (Chen and Luck, 2016; Kashani et al., 2018) and C–H bond oxidation (Murahashi et al., 2000; Kudrik and Sorokin, 2017; Sarma et al., 2018). There are also some successful examples of using TBHP as an external oxidant in alcohol oxidation (Sarkar et al., 2014; Annunziata et al., 2018; Borah et al., 2018). In spite of this progress, less attention has been paid to this research area. Therefore, it is in an urgent demand to develop mild and efficient oxidation systems using TBHP as an oxidant.
It is well-known that transition metal complexes play a crucial role in oxidation process. Many transition metal catalysts including ruthenium (Shapley et al., 2000; Lybaert et al., 2017; Sarbajna et al., 2017; Moore et al., 2019), palladium (Stahl, 2004; Sigman and Jensen, 2006; Ho et al., 2018), copper (Velusamy et al., 2006; Jehdaramarn et al., 2018; Lagerspets et al., 2019), and iron (Coleman et al., 2010; Stanje et al., 2018) have been reported for promoting the oxidation of alcohols. Among them, ruthenium compounds are intensively studied because of their rich structures and various valence states. For example, Ramesh and co-workers reported that ruthenium(II) carbonyl 2-(arylazo)phenolate complexes could oxidize sensitive group-contained alcohols with moderate to high conversion (Kumar et al., 2007). The group of Zhang synthesized several ruthenium complexes containing 2-(biphenylazo)phenolate ligands that successfully achieved high catalytic activity in the presence of NMO without diminishing chemoselectivity (Tang et al., 2018). However, very few examples were focused on di-or tri-nuclear ruthenium complexes and their applications in organic reactions were limited. Recently, we have reported a series of triruthenium carbonyl complexes and their efficient oxidation behavior toward secondary alcohols, while these Ru compounds showed poor reactivity in oxidation of primary alcohols (Hao et al., 2018a,b, 2019). As part of our continuing efforts in developing novel ruthenium carbonyl complexes and their applications in alcohol oxidation, herein, we reported the synthesis and characterization of several ruthenium carbonyl complexes supported by pyridine-alkoxide ligands and their catalytic properties in the oxidation of primary and secondary alcohols using TBHP as an oxidant.
Experimental
Materials and Methods
All manipulations were performed under a nitrogen atmosphere using standard Schlenk techniques. Solvents for reaction were distilled from appropriate drying agents under N2 before use. All the chemical reagents were purchased from commercial sources. Ru3(CO)12 was prepared by literature methods (Fauré et al., 2003). NMR spectra were measured using a Bruker Avance III-500 NMR spectrometer at room temperature with TMS as internal standard. Melting points were determined using an SGW X-4A Digital Melting Point Apparatus. IR spectra were recorded as KBr disks on a Thermo Fisher iS 50 spectrometer in the range 4,000–600 cm−1 and elemental analyses were performed on a Vario EL III analyzer.
Preparation of 6-bromopyCH(2-CF3C6H4)OH (L7)
To a dried Et2O (30 mL) of 2, 6-dibromopyridine (3.55 g, 15 mmol) at −78°C, n-BuLi (9.5 mL, 15 mmol) was added dropwise via a syringe in 10 min and the solution was stirred at −78°C for 1 h. After addition of 2-(trifluoromethy)benzaldehyde (2.61 g, 15 mmol), the mixture was allowed to warm to room temperature and stirred overnight. The reaction solution was neutralized with aqueous NH4Cl and the organic phase was separated. The aqueous layer was extracted with CH2Cl2 (3 × 10 mL), and combined organic fractions were dried over MgSO4 and the residue was placed in an Al2O3 column with ethyl acetate/petroleum ether as an eluent to give L7 as a off-white powders (2.04 g, 46%). 1H NMR (CDCl3, 500 MHz, 298 K): δ 7.69 (d, J = 7.9 Hz, 1 H, Py-H), 7.53 (t, J = 7.7 Hz, 1 H, Py-H), 7.45–7.49 (m, 2 H, C6H4), 7.41 (d, J = 8.2 Hz, 2 H, C6H4), 6.96 (d, J = 7.7 Hz, 1 H, Py-H), 6.15 (s, 1 H, CH), 4.93 (s, 1 H, OH). 13C NMR (CDCl3, 125 MHz, 298 K): δ 161.9, 141.1, 140.8, 139.5, 132.6, 129.9, 128.4, 128.2, 127.2, 125.5 (q, JC−F = 5.5 Hz), 123.1 (q, JC−F = 272 Hz), 120.6, 69.5 ppm.
Preparation of (6-bromopyCHC6H5O)2Ru3(CO)8 (1a)
A solution of ligand precursor L1 (0.248 g, 0.938 mmol) and Ru3(CO)12 (0.300 g, 0.469 mmol) in 30 mL of xylene was refluxed for 10 h. After evaporation of the solvent in vacuo, the residue was placed in an Al2O3 column. Elution with ethyl acetate/petroleum ether gave 1a as orange crystals (yield 0.307 g, 62%). Mp: 168–169°C. Anal. Calc. for C32H18Br2N2O10Ru3: C, 36.48; H, 1.72; N, 2.66. Found (%): C, 36.30; H, 1.85; N, 2.71. 1H NMR (CDCl3, 500 MHz, 298 K): δ 7.61 (d, J = 7.7 Hz, 2 H, Py-H), 7.25–7.29 (m, 8 H, Py-H, C6H4), 7.15 (d, J = 6.5 Hz, 4 H, C6H4), 6.62 (d, J = 7.7 Hz, 2 H, Py-H), 5.58 (s, 2 H, CH) ppm. 13C NMR (CDCl3, 125 MHz, 298 K): δ 205.9, 202.9, 200.8, 190.1, 170.8, 144.8, 143.4, 138.2, 128.7, 128.4, 128.3, 128.0, 120.0, 91.7 ppm. IR (υCO, KBr, cm−1): 2081(s), 2015(s), 1998(vs), 1909(s).
Preparation of [6-bromopyCH(4-MeC6H4O)]2Ru3(CO)8 (1b)
Complex 1b was prepared in a similar procedure to that described above for preparation of 1a. Reaction of L2 (0.261 g, 0.938 mmol) with Ru3(CO)12 (0.300 g, 0.469 mmol) in 30 mL of xylene generated complex 1b as orange crystals (yield 0.358 g, 70%). Mp: 173–175°C. Anal. Calc. for C34H22Br2N2O10Ru3: C, 37.76; H, 2.05 N, 2.59. Found (%): C, 37.91; H, 2.19, N, 2.45. 1H NMR (CDCl3, 500 MHz, 298 K): δ 7.60 (d, J = 7.7 Hz, 2 H, Py-H), 7.26 (t, J = 7.7 Hz, 2 H, Py-H), 7.09 (d, J = 7.9 Hz, 4 H, C6H4), 7.04 (d, J = 8.0 Hz, 4 H, C6H4), 6.60 (d, J = 7.7 Hz, 2 H, Py-H), 5.54 (s, 2 H, CH), 2.30 (s, 6 H, CH3) ppm. 13C NMR (CDCl3, 125 MHz, 298 K): δ 206.0, 203.0, 200.9, 190.2, 171.0, 144.7, 140.5, 138.1, 138.0, 129.3, 128.2, 127.9, 119.9, 91.5, 21.4 ppm. IR (υCO, KBr, cm−1): 2085(s), 2015(s), 2000(s), 1909(s).
Preparation of [6-bromopyCH(4-OMeC6H4O)]2Ru3(CO)8 (1c)
Complex 1c was prepared in a similar procedure to that described above for preparation of 1a. Reaction of L3 (0.276 g, 0.938 mmol) with Ru3(CO)12 (0.300 g, 0.469 mmol) in 30 ml of xylene generated complex 1c as orange crystals (yield 0.337 g, 65%). Mp: 176–177°C. Anal. Calc. for C34H22Br2N2O12Ru3: C, 36.67; H, 1.99, N, 2.52. Found (%): C, 36.53; H, 2.10, N, 2.44. 1H NMR (CDCl3, 500 MHz, 298 K): δ 7.59 (d, J = 7.7 Hz, 2 H, Py-H), 7.26 (t, J = 7.7 Hz, 2 H, Py-H), 7.07 (d, J = 8.6 Hz, 4 H, C6H4), 6.81 (d, J = 8.6 Hz, 4 H, C6H4), 6.60 (d, J = 7.7 Hz, 2 H, Py-H), 5.55 (s, 2 H, CH), 3.76 (s, 6H, OCH3) ppm. 13C NMR (CDCl3, 125 MHz, 298 K): δ 206.0, 203.0, 200.9, 190.2 171.1, 159.6, 144.7, 138.1, 135.9, 129.3, 128.2, 120.0, 114.1, 91.2, 55.4 ppm. IR (υCO, KBr, cm−1): 2078(s), 2004(s), 1993(vs), 1912(s).
Preparation of [6-bromopyCH(4-ClC6H4O)]2Ru3(CO)8 (1d)
Complex 1d was prepared in a similar procedure to that described above for preparation of 1a. Reaction of L4 (0.281 g, 0.938 mmol) with Ru3(CO)12 (0.300 g, 0.469 mmol) in 30 mL of xylene generated complex 1d as orange crystals (yield 0.267 g, 51%). Mp: 182–183°C. Anal. Calc. for C32H16Br2Cl2N2O10Ru3: C, 34.24; H, 1.44, N, 2.50. Found (%): C, 34.34; H, 1.52, N, 2.48. 1H NMR (CDCl3, 500 MHz, 298 K): δ 7.64 (d, J = 7.5 Hz, 2 H, Py-H), 7.31 (t, J = 7.7 Hz, 2 H, Py-H), 7.29 (d, J = 8.3 Hz, 4 H, C6H4), 7.08 (d, J = 8.3 Hz, 4 H, C6H4), 6.61 (d, J = 7.7 Hz, 2 H, Py-H), 5.54 (s, 2 H, CH) ppm. 13C NMR (CDCl3, 125 MHz, 298 K): δ 205.8, 202.8, 200.9,189.7, 170.1,144.9, 141.8, 138.3, 134.2, 129.3, 129.0, 128.6, 119.9, 90.9 ppm. IR (υCO, KBr, cm−1): 2084(s), 2012(s), 1998(s), 1915(s).
Preparation of [6-bromopyCH(4-CF3C6H4O)]2Ru3(CO)8 (1e)
Complex 1e was prepared in a similar procedure to that described above for preparation of 1a. Reaction of L5 (0.339 g, 0.938 mmol) with Ru3(CO)12 (0.300 g, 0.469 mmol) in 30 mL of xylene generated complex 1e as orange crystals (yield 0.267 g, 48%). Mp: 177–179°C. Anal. Calc. for C34H16Br2F6N2O10Ru3: C, 34.33; H, 1.36, N, 2.36. Found (%): C, 34.44; H, 1.30, N, 2.43. 1H NMR (CDCl3, 500 MHz, 298 K): δ 7.67 (d, J = 7.7 Hz, 2 H, Py-H), 7.57 (d, J = 8.1 Hz, 4 H, C6H4), 7.35 (t, J = 7.8 Hz, 2 H, Py-H), 7.29 (d, J = 8.0 Hz, 4 H, C6H4), 6.64 (d, J = 7.6 Hz, 2 H, Py-H), 5.61 (s, 2 H, CH) ppm. 13C NMR (CDCl3, 125 MHz, 298 K): δ 205.6, 202.7, 200.9, 189.5, 169.7, 146.9, 145.0, 138.5, 130.7, 128.9, 128.2, 125.8 (q, JC−F = 2.7 Hz), 123.1 (q, JC−F = 270.5 Hz), 120.0, 91.1 ppm. IR (υCO, KBr, cm−1): 2083(s), 2017(s), 1993(s), 1912(s).
Preparation of [6-bromopyCH(2-OMeC6H4O)]2Ru3(CO)8 (1f)
Complex 1f was prepared in a similar procedure to that described above for preparation of 1a. Reaction of L6 (0.276 g, 0.938 mmol) with Ru3(CO)12 (0.300 g, 0.469 mmol) in 30 mL of xylene generated complex 1f as orange crystals (yield 0.298 g, 57%). Mp: 183–184°C. Anal. Calc. for C34H22Br2N2O12Ru3: C, 36.67; H, 1.99, N, 2.52. Found (%): C, 36.75; H, 2.12, N, 2.45. 1H NMR (CDCl3, 500 MHz, 298 K): δ 7.59 (d, J = 7.7 Hz, 2 H, Py-H), 7.24–7.19 (m, 4 H, C6H4, Py-H), 6.85 (d, J = 8.2 Hz, 2 H, C6H4), 6.76 (t, J = 7.4 Hz, 2 H, C6H4), 6.61 (d, J = 7.6 Hz, 2 H, Py-H), 6.50 (d, J = 7.5 Hz, 2 H, C6H4), 6.04 (s, 2 H, CH), 3.94 (s, 6 H, OCH3) ppm. 13C NMR (CDCl3, 125 MHz, 298 K): δ 206.2, 203.1, 201.1, 190.7, 171.2, 157.3, 144.9, 138.0, 131.7, 129.4, 128.3, 128.1, 120.0, 119.2, 110.3, 84.9, 55.2 ppm. IR (υCO, KBr, cm−1): 2086 (s), 2020 (vs), 1995 (s), 1960 (s), 1905 (s).
Preparation of [6-bromopyCH(2-CF3C6H4O)]2Ru3(CO)8 (1g)
Complex 1g was prepared in a similar procedure to that described above for preparation of 1a. Reaction of L7 (0.339 g, 0.938 mmol) with Ru3(CO)12 (0.300 g, 0.469 mmol) in 30 mL of xylene generated complex 1g as orange crystals (yield 0.212 g, 38%). Mp: 186–188°C. Anal. Calc. for C34H16Br2F6N2O10Ru3: C, 34.33; H, 1.36, N, 2.36. Found (%): C, 34.25; H, 1.41, N, 2.43. 1H NMR (CDCl3, 500 MHz, 298 K): δ 7.69 (d, J = 7.8 Hz, 2 H, Py-H), 7.62 (d, J = 7.9 Hz, 2 H, C6H4), 7.43 (t, J = 7.6 Hz, 2 H, Py-H), 7.35–7.30 (m, 6 H, C6H4), 6.51 (d, J = 7.7 Hz, 2 H, Py-H), 5.61 (s, 2 H, CH) ppm. 13C NMR (CDCl3, 125 MHz, 298 K): δ 204.9, 203.1, 200.8, 190.0, 169.9, 145.3, 141.9, 138.5, 132.3, 129.9, 128.7, 127.9, 126.4, 125.7 (q, JC−F = 4.6 Hz), 123.0 (q, JC−F = 273.8 Hz), 119.8, 85.5 ppm. IR (υCO, KBr, cm−1): 2089(m), 2022(s), 2001(s), 1969(s), 1914(s).
Preparation of [6-bromopyCH(Me)2O]2Ru3(CO)8 (1h)
Complex 1h was prepared in a similar procedure to that described above for preparation of 1a. Reaction of L8 (0.202 g, 0.938 mmol) with Ru3(CO)12 (0.300 g, 0.469 mmol) in 30 mL of xylene generated complex 1h as orange crystals (yield 0.305 g, 68%). Mp: 171–172°C. Anal. Calc. for C24H18Br2N2O10Ru3: C, 30.11; H, 1.89; N, 2.93. Found (%): C, 30.25; H, 1.99; N, 2.81. 1H NMR (CDCl3, 500 MHz, 298 K): δ 7.51 (d, J = 7.6 Hz, 2 H, Py-H), 7.41 (t, J = 7.7 Hz, 2 H, Py-H), 6.89 (d, J = 7.6 Hz, 2 H, Py-H), 1.37 (s, 6 H, CH3), 1.18 (s, 6 H, CH3) ppm. 13C NMR (CDCl3, 125 MHz, 298 K) δ 206.9, 202.9, 202.7, 191.0, 175.7, 145.6, 138.8, 128.2, 118.7, 87.7, 34.7, 31.1 ppm. IR (υCO, KBr, cm−1): 2080(s), 2007(s), 1966(s), 1906(s).
General Procedure for Catalytic Oxidation of Alcohols
An alcohol substrate (1.0 mmol), complex 1c (0.005 mmol) and TBHP (2.5 mmol) was placed in a 2-neck 25 mL round bottom flask and degassed 2 times. Two milliliter of dried CH3CN was then added and the resulting mixture was reacted at room temperature for 1 h under an N2 atmosphere. After the reaction was complete, the solvent was removed under reduced pressure and the residue was purified by Al2O3 column chromatography (eluent ethylacetate/petroleum ether v/v = 1/15) to afford the desired product, which was identified by comparison with the authentic sample through NMR and GC analyses.
Crystal Structural Determination
Single crystals of complexes 1a-1h suitable for X-ray crystal structural analysis were obtained from a CH2Cl2/n-hexane mixed solvent system. Data collection was performed on a Bruker SMART 1000 diffractometer, using graphite-monochromated Mo-K radiation (ω-φ scans, λ = 0.71073 Å). The structures were solved by direct methods and refined by full-matrix least squares. All calculations were using SHELXTL crystallographic software packages (Sheldrick, 1997). The crystal data and summary of X-ray data collection are presented in Tables S2, S3.
Results and Discussion
Synthesis of Ligands and Ruthenium Complexes
6-bromopyridine alcohol ligands L1-L6 and L8 were synthesized according to the literature procedure (Tsukahara et al., 1997; Song and Morris, 2004) and identified by NMR and elemental analysis prior to use. L7 was synthesized following similar methods. 2,6-Dibromopyridine reacted with n-BuLi and 1 equiv of o-substituted aldehydes was added to the reaction mixture, then hydrolysis led to the target ligand.
Ruthenium clusters were prepared in moderate to high yields from Ru3(CO)12 by treating with 2.0 equiv of ligands L1-L8 in refluxing xylene, respectively. The general synthetic route for these new compounds is depicted in Scheme 1. These trinuclear Ru complexes were identified by FI-IR, NMR spectroscopy and elemental analysis. The FT-IR spectra of all the complexes exhibit several absorption peaks around 1906–1950 cm−1, which can be assigned to the characteristic stretching vibration of the terminally coordinated CO. In the 1H NMR spectra of 1a-1g, the characteristic signal of –OH disappeared and the singlets resonance for the methyne adjacent to oxygen were observed at 5.5–6.1 ppm, which were shifted upfield when compared to those in the free ligands (L1-L7). In the 13C NMR spectra, the resonance signals around 85–92 ppm correspond to the methynes mentioned above, which are in good accordance with compound [pyC(Me)2O]2Ru3(CO)8 (δ 87.6 ppm) and [pyCHC6H5O]2Ru3(CO)8 (δ 89.3 ppm), which were previously reported in the literature (Hao et al., 2018b).
Crystal Structures of Complexes 1a-1h
Complexes 1a-1h were further characterized by X-ray crystallography. The molecular structures of 1a, 1c, and 1g are shown in Figures 1–3 with selected bond lengths and angles, respectively. Those of 1b, 1d-1f, and 1h are shown in Figures S1–S5, respectively. Details of the structural parameters are also given in Tables S2, S3. X-ray diffraction analysis shows that all the complexes 1a-1h are trinuclear ruthenium clusters accompanied by two pyridylalkoxo ligands simultaneously via their pyridyl N and hydroxy O atoms. Three Ru atoms adopt a pseudooctahedral coordinated mode. The unit cell of 1a contains two crystallographically-independent molecules which possess similar connectivity and only one molecular structure is depicted in Figure 1 for clarity. In complexes 1a-1h, the distances between the two Ru atoms directly connected to the ligands are in the range of 3.0236(10)-3.0516(10) Å, which are comparable to the Ru–Ru bond distances in complexes (μ-OC6H4OMe-2)2Ru3(CO)8 (3.012(1) Å) (Santini et al., 1987) and [PyCH = C(Ph)O]2Ru3(CO)8 (3.0693(6) Å) (Ma et al., 2017). The bond lengths of Ru(1)–N(1) varying from 2.252(7) Å to 2.330(14) Å observed in all complexes are slightly longer than those Ru–N bond lengths found in complexes {μ2-μ5:η1-(C5H4N)(C9H5)}Ru3(CO)9 [2.164(3) Å] (Chen et al., 2010) and (6-bromopyCMeC6H5O)Ru3(CO)9 (2.229(4) Å) (Hao et al., 2018b). The Ru(1)–O(1) bond lengths for 1a-1h are all similar and are in the range of 2.072(3)-2.093(5) Å, showing that the substitutions at 2- or 4- positions of benzene ring have no significant effect on bond lengths.
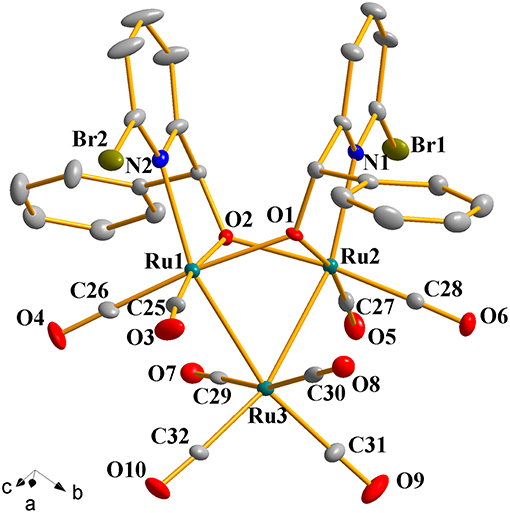
Figure 1. Perspective view of 1a with thermal ellipsoids are drawn at the 30% probability level. Hydrogens have been omitted for clarity. The selected bond lengths (Å) and angles (°): Ru(1)-Ru(3) 2.7743(10), Ru(2)-Ru(3) 2.7625(10), Ru(1)-O(1) 2.140(5), Ru(2)-O(1) 2.084(6), Ru(1)-N(2) 2.237(7), Ru(2)-N(1) 2.252(7); N(2)-Ru(1)-Ru(3) 157.9(2), N(2)-Ru(1)-Ru(2) 102.2(2), Ru(2)-O(1)-Ru(1) 91.4(2), Ru(1)-O(2)-Ru(2) 91.1(2), Ru(2)-Ru(3)-Ru(1) 66.20(3).
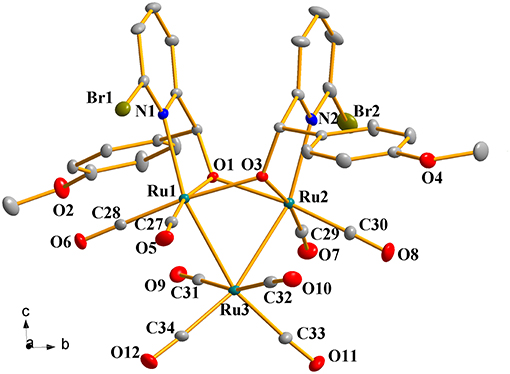
Figure 2. Perspective view of 1c with thermal ellipsoids are drawn at the 30% probability level. Hydrogens and solvent have been omitted for clarity. The selected bond lengths (Å) and angles (°): Ru(1)-O(1) 2.083(3), Ru(2)-O(1) 2.132(3), Ru(1)-Ru(3) 2.7649(5), Ru(2)-Ru(3) 2.7552(6), Ru(1)-N(1) 2.258(4), Ru(2)-N(2) 2.240(4); Ru(1)-O(1)-Ru(2) 92.33(12), Ru(2)-O(3)-Ru(1) 92.00(12), N(1)-Ru(1)-Ru(3) 157.10(10), N(2)-Ru(2)-Ru(3) 157.01(12), Ru(2)-Ru(3)-Ru(1) 66.848(15).
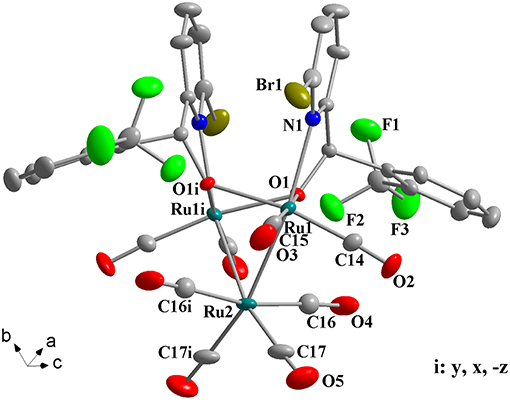
Figure 3. Perspective view of 1g with thermal ellipsoids are drawn at the 30% probability level. Hydrogens have been omitted for clarity. The selected bond lengths (Å) and angles (°): Ru(1)-O(1) 2.089(4), Ru(1)-O(1i) 2.147(4), Ru(1)-N(1) 2.313(5), Ru(2)-Ru(1) 2.7560(7), Ru(2)-Ru(1i) 2.7560(7), Ru(1)-O(1)-Ru(1i) 92.06(15), N(1)-Ru(1)-Ru(1i) 97.72(13), Ru(1)-Ru(2)-Ru(1i) 67.17(2).
Catalytic Activity on Oxidation of Alcohols
We commenced our study by using complex 1a as precatalyst and 1-phenylethanol as a simple substrate to obtain the appropriate conditions. Initially, various oxidants (NMO, H2O2, t-BuOOH, TEMPO) were tested to oxidize 1-phenylethanol. Among diffrerent oxidants applied in this studies, t-BuOOH was found to be the best oxidant and acetophenone can be obtained in a high yield of 75% (Table S1). The effect of solvent on this oxidation process was then evaluated (Table 1, entries 1–6). When the reaction was performed in CH2Cl2, THF or dioxide, the oxidation product was obtained in low yields (<55%). When using toluene or acetone as solvent, the the yield was enhanced slightly. To our delight, the yield of the desired ketone in CH3CN was 85%, which is higher than that in other solvents. Thus, acetonitrile was selected as the optimal solvent. The subsequent lowering the loading of 1a from 2.0 to 0.5 mol% did not significantly affect the yield, thus only 0.5 mol% catalyst 1a is sufficient for catalyzing the present reaction (Table 1, entries 3 and 7–9). Subsequently, the effect of TBHP on the reaction was examined. Upon increasing the amounts of TBHP from 1.0 to 3.0 mmol, the yield of acetophenone was gradually improved to 91%, and 2.5 mmol of TBHP was selected as the most suitable amount from the view of cost-saving (Table 1, entries 10–13). It was found that the reaction temperature had an obvious impact on the reaction efficiency. As shown in Table 1, when elevating the temperature from room temperature to 50 or 80°C, the yield of product decreased dramatically to 72 and 54%, respectively (Table 1, entries 14 and 15). Furthermore, the effect of reaction time on the rate of oxidation was investigated (Figure 4). When the reaction time was extended from 0 to 20 min, an almost linear increase of the yield was observed during the oxidation of 1-phenylethanol. After 40 min, the yield increased slightly, and further extension of the time after 60 min could hardly increase the yield. Finally, control experiments indicated that only traces (8%) of the desired product was formed without using complex 1a and almost no oxidation product was generated in the absence of TBHP (Table 1, entries 16 and 17).
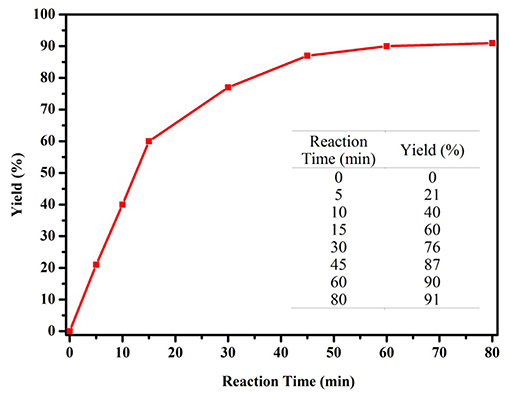
Figure 4. Influence of the reaction time on catalytic performance of complex 1a (1-phenylethanol 1.0 mmol, complex 1a 0.5 mol%, TBHP 2.5 mmol, CH3CN 2.0 mL).
The above interesting results encouraged us to continue the optimization study using different ruthenium complexes and the obtained results are summaried in Table 2. The complexes containing electronically rich ligands (1a-c, 1f, and 1h) exhibited higher catalytic activity than those bearing electronically poor ligands (1d, 1e, and 1g), suggesting that the catalytic behavior of Ru complexes was influenced by the electronic nature of the ligands. Catalysts 1f and 1g with ortho-substituents in the phenyl ring exhibited lower catalytic activity than their para-substituted analogs 1c and 1e (Table 2, entries 3–4 vs. 6–7). This difference is likely due to the fact that substitutions at 2-position of ligands caused more steric hindrance around the metal centers, thus influencing the coordination of metals with substrates. Besides, catalytic oxidation of 1-phenylethanol was also carried out in the presence of Ru3(CO)12 and the yield of the desired product was only 30% (Table 2, entry 9). Thus, the optimized reaction conditions are as follows: alcohol (1.0 mmol), TBHP (2.5 mmol), catalyst 1c (0.5 mol%), reaction time (1 h) at room temperature.
Under optimized conditions, we set out to test the catalytic performance of complex 1c in oxidation of different secondary alcohols. As listed in Table 3, a diverse array of functional groups including methyl-, chloro-, and trifluoromethyl- etc. on the phenyl ring of substituted 1-phenylethanol were tolerated (Table 3, entries 1–7). Additionally, several sterically encumbered substrates undergo oxidation in >90% yields (Table 3, entries 8–10). The fused-ring alcohols, that are 1,2,3,4-tetrahydro-1-naphthol and 1-indanol could be also converted to target product in 93 and 92% yields, respectively (Table 3, entries 11 and 12). As for secondary aliphatic alcohols, a prolonged reaction time (3h) was required to achieve high yields (Table 3, entries 13–16).
Moreover, the oxidation of primary alcohols was also tested in the standard conditions and the results were summarized in Table 4. The reaction of benzyl alcohols bearing electron-rich or electron-deficient substituents in the aromatic ring proceeded efficiently to furnish the corresponding benzaldehyde derivatives in excellent yields (Table 4, entries 1–7). Only trace amount of benzoic acids were detected, which demonstrated the superiority of the present catalytic system in terms of chemoselectivity. 2-Naphthalenemethanol showed satisfactory reactivity to provide 2-naphthaldehyde in >90% yield (Table 4, entry 8). The substrate having sensitive group (internal alkene) was also tolerated in this system, the carbon-carbon double was preserved in the final product (Table 4, entry 9). While the catalytic system displayed a diminished activity for oxidation of heterocyclic primary alcohols such as 2-furanmethanol and 2-thiophenemethanol, and <70% yields of the target products were obtained under optimized condition. This outcome can be explained by the strong coordination ability of heteroatoms with Ru centers, which led to the deactivation of the catalyst. Delightfully, decent yields of the heterocyclic products could be obtained by simply increasing the catalyst loading of 1c to 1.5 mol% (Table 4, entries 10–12).
Based on our preliminary data and related Ru-catalyzed alcohol oxidation processes, a plausible inner-sphere mechanism for alcohol oxidation catalyzed by present ruthenium carbonyl complexes/TBHP system is proposed in Scheme 2. First, the catalyst A reacted with two molecules of TBHP to give Ru-oxide species I and tBuOH. Subsequent reaction of intermediate I with alcohol to form a five-membered ring transient state II, which then released water to give the alkoxide species III. Finally, the break of Ru-O bond in this intermediate occurred to regenerate catalyst A for next catalytic cycle and afforded the target carbonyl product.
Conclusions
In summary, a series of ruthenium carbonyl complexes bearing pyridine-alkoxide ligands were synthesized and exhibited excellent catalytic activity for the oxidation of both primary and secondary alcohol substrates, showing broad substrate scope. Of particular note was the effective oxidation of primary alcohols to desired aldehydes without over-oxidation, displaying good chemoselectivity. A striking advantage associated with this catalytic system is that the oxidation reaction can be completed within only 1 h at room temperature for most cases, which is far more efficient than previously reported Ru/TBHP or Ru/NMO systems.
Data Availability
The raw data supporting the conclusions of this manuscript will be made available by the authors, without undue reservation, to any qualified researcher.
Author Contributions
XYa and XYu were response for the synthesis and characterization of Ru complexes and catalytic experiment. KL conducted the synthesis of pyridine alcohol ligands. ZHan solved the single crystals and drew figures for the complexes. ZHao and JL supervised the whole work, drafted and revised the manuscript.
Funding
This work was supported by the National Natural Science Foundation of China (No. 21871076), the Hebei Natural Science Foundation of China (No. B2017205006), the Education Department Foundation of Hebei Province (Nos. ZD2018005 and QN2019036), and the Science Foundation of Hebei Normal University (Nos. L2017Z02 and L2018B08).
Conflict of Interest Statement
The authors declare that the research was conducted in the absence of any commercial or financial relationships that could be construed as a potential conflict of interest.
Supplementary Material
The Supplementary Material for this article can be found online at: https://www.frontiersin.org/articles/10.3389/fchem.2019.00394/full#supplementary-material
References
Allen, S. E., Walvoord, R. R., Padilla-Salinas, R., and Kozlowski, M. C. (2013). Aerobic copper-catalyzed organic reactions. Chem. Rev. 113, 6234–6458. doi: 10.1021/cr300527g
Annunziata, A., Esposito, R., Gatto, G., Cucciolito, M. E., Tuzi, A., Macchioni, A., et al. (2018). Iron (III) complexes with cross-bridged cyclams: synthesis and use in alcohol and water oxidation catalysis. Eur. J. Inorg. Chem. 2018, 3304–3311. doi: 10.1002/ejic.201800451
Balaraman, E., Khaskin, E., Leitus, G., and Milstein, D. (2013). Catalytic transformation of alcohols to carboxylic acid salts and H2 using water as the oxygen atom source. Nat. Chem. 5, 122–125. doi: 10.1038/nchem.1536
Bianchini, C., and Shen, P. K. (2009). Palladium-based electrocatalysts for alcohol oxidation in half cells and in direct alcohol fuel cells. Chem. Rev. 109, 4183–4206. doi: 10.1021/cr9000995
Borah, B. J., Mahanta, A., Mondal, M., Gogoi, H., Yamada, Y., and Bharali, P. (2018). Cobalt-copper nanoparticles catalyzed selective oxidation reactions: efficient catalysis at room temperature. Chem. Select 3, 9826–9832. doi: 10.1002/slct.201801140
Campestrini, S., Carraro, M., Ciriminna, R., Pagliaro, M., and Tonellato, U. (2004). Alcohols oxidation with hydrogen peroxide promoted by TPAP-doped ormosils. Tetrahedron Lett. 45, 7283–7286. doi: 10.1016/j.tetlet.2004.08.020
Canielli, G., and Cardillo, G. (1984). Chromium Oxidation in Organic Chemistry. Berlin: Springer Verlag.
Cao, Q., Dornan, L. M., Rogan, L., Hughes, N. L., and Muldoon, M. J. (2014). Aerobic oxidation catalysis with stable radicals. Chem. Commun. 50, 4524–4543. doi: 10.1039/C3CC47081D
Caron, S., Dugger, R. W., Ruggeri, S. G., Ragan, J. A., and Ripin, D. H. B. (2006). Large-scale oxidations in the pharmaceutical industry. Chem. Rev. 106, 2943–2989. doi: 10.1021/cr040679f
Chen, D., Zhang, X., Xu, S., Song, H., and Wang, B. (2010). Pyridyl-substituted indenyl ruthenium complexes: synthesis, structures, and reactivities. Organometallics 29, 3418–3430. doi: 10.1021/om100556y
Chen, Z., and Luck, R. L. (2016). Oxidation of olefins using atmospheric oxygen atoms initiated by tert-butylhydroperoxide or hydrogen peroxide with silver nanoparticles deposited on MCM-41 as catalysts. Green Chem. 18, 3354–3359. doi: 10.1039/C5GC03110A
Coleman, M. G., Brown, A. N., Bolton, B. A., and Guan, H. (2010). Iron-catalyzed oppenauer-type oxidation of alcohols. Adv. Synth. Catal. 352, 967–970. doi: 10.1002/adsc.200900896
Crabtree, R. H. (2017). Homogeneous transition metal catalysis of acceptorless dehydrogenative alcohol oxidation: applications in hydrogen storage and to heterocycle synthesis. Chem. Rev. 117, 9228–9246. doi: 10.1021/acs.chemrev.6b00556
Dai, W., Lv, Y., Wang, L., Shang, S., Chen, B., Li, G., et al. (2015). Highly efficient oxidation of alcohols catalyzed by a porphyrin-inspired manganese complex. Chem. Commun. 51, 11268–11271. doi: 10.1039/C5CC03657G
Dijksman, A., Marino-González, A., Mairata I Payeras, A., Arends, I. W. C. E., and Sheldon, R. A. (2001). Efficient and selective aerobic oxidation of alcohols into aldehydes and ketones using ruthenium/tempo as the catalytic system. J. Am. Chem. Soc. 123, 6826–6833. doi: 10.1021/ja0103804
Fauré, M., Saccavini, C., and Lavigne, G. (2003). New insight into a convenient base-promoted synthesis of Ru3(CO)12. Chem. Commun. 1578–1579. doi: 10.1039/B303884J
Gunasekaran, N., Remya, N., Radhakrishnan, S., and Karvembu, R. (2011). Ruthenium (II) carbonyl complexes with N-[di(alkyl/aryl) carbamothioyl] benzamide derivatives and triphenylphosphine as effective catalysts for oxidation of alcohols. J. Coord. Chem. 64, 491–501. doi: 10.1080/00958972.2010.548007
Hao, Z., Li, N., Yan, X., Li, Y., Zong, S., Liu, H., et al. (2018a). Ruthenium carbonyl complexes with pyridylalkanol ligands: synthesis, characterization and catalytic properties for aerobic oxidation of secondary alcohols. New J. Chem. 42, 6968–6975. doi: 10.1039/C8NJ00329G
Hao, Z., Li, Y., Li, C., Wu, R., Ma, Z., Li, S., et al. (2019). Synthesis of a series of new ruthenium organometallic complexes derived from pyridine-imine ligands and their catalytic activity in oxidation of secondary alcohols. Appl. Organomet. Chem. 33:e4750. doi: 10.1002/aoc.4750
Hao, Z., Yan, X., Liu, K., Yue, X., Han, Z., and Lin, J. (2018b). Ruthenium carbonyl complexes with pyridine-alkoxide ligands: synthesis, characterization and catalytic application in dehydrogenative oxidation of alcohols. New J. Chem. 42, 15472–15478. doi: 10.1039/C8NJ03706J
Ho, W. C., Chung, K., Ingram, A. J., and Waymouth, R. M. (2018). Pd-catalyzed aerobic oxidation reactions: strategies to increase catalyst lifetimes. J. Am. Chem. Soc. 140, 748–757. doi: 10.1021/jacs.7b11372
Hoover, J. M., Steves, J. E., and Stahl, S. S. (2012). Copper (I)/TEMPO-catalyzed aerobic oxidation of primary alcohols to aldehydes with ambient air. Nat. Protoc. 7, 1161–1166. doi: 10.1038/nprot.2012.057
Jehdaramarn, A., Pornsuwan, S., Chumsaeng, P., Phomphrai, K., and Sangtrirutnugul, P. (2018). Effects of appended hydroxyl groups and ligand chain length on copper coordination and oxidation activity. New J. Chem. 42, 654–661. doi: 10.1039/C7NJ03113K
Kashani, S. H., Moghadam, M., Tangestaninejad, S., Mirkhani, V., and Mohammadpoor-Baltork, I. (2018). Ruthenium nanoparticles immobilized on nano-silica functionalized with thiol-based dendrimer: a nanocomposite material for oxidation of alcohols and epoxidation of alkenes. Catal. Lett. 148, 1110–1123. doi: 10.1007/s10562-018-2313-8
Kudrik, E. V., and Sorokin, A. B. (2017). Oxidation of aliphatic and aromatic CH bonds by t-BuOOH catalyzed by μ-nitrido diiron phthalocyanine. J. Mol. Catal. A Chem. 426, 499–505. doi: 10.1016/j.molcata.2016.08.013
Kumar, K. N., Ramesh, R., and Liu, Y. (2007). Synthesis, structure and catalytic activity of cycloruthenated carbonyl complexes containing arylazo phenolate ligands. J. Mol. Catal. A Chem. 265, 218–226. doi: 10.1016/j.molcata.2006.10.015
Kumpulainen, E. T., and Koskinen, A. M. (2009). Catalytic activity dependency on catalyst components in aerobic copper-TEMPO oxidation. Chem. Eur. J. 15, 10901–10911. doi: 10.1002/chem.200901245
Lagerspets, E., Lagerblom, K., Heliövaara, E., Hiltunen, O.-M., Moslova, K., Nieger, M., et al. (2019). Schiff base Cu (I) catalyst for aerobic oxidation of primary alcohols. Mol. Catal. 468, 75–79. doi: 10.1016/j.mcat.2019.02.003
Lybaert, J., Trashin, S., Maes, B. U., De Wael, K., and Abbaspour Tehrani, K. (2017). Cooperative electrocatalytic and chemoselective alcohol oxidation by Shvo's catalyst. Adv. Synth. Catal. 359, 919–925. doi: 10.1002/adsc.201600783
Ma, Z.-H., Liu, Q., Qin, M., Han, Z.-G., and Lin, J. (2017). Synthesid, structure and reactivity of a trinuclear ruthenium cluster compound [PyCH = C(Ph)O]2Ru3(CO)8. Chin. J. Inorg. Chem. 2017, 1293–1298. doi: 10.11862/CJIC.2017.135
Mallat, T., and Baiker, A. (2004). Oxidation of alcohols with molecular oxygen on solid catalysts. Chem. Rev. 104, 3037–3058. doi: 10.1021/cr0200116
Miao, C., Li, X.-X., Lee, Y.-M., Xia, C., Wang, Y., Nam, W., et al. (2017). Manganese complex-catalyzed oxidation and oxidative kinetic resolution of secondary alcohols by hydrogen peroxide. Chem. Sci. 8, 7476–7482. doi: 10.1039/C7SC00891K
Moore, P. W., Zerk, T. J., Burns, J. M., Bernhardt, P. V., and Williams, C. M. (2019). Hydrogen-bonding interactions in the ley-griffith oxidation: practical considerations for the synthetic chemist. Eur. J. Org. Chem. 2019, 303–308. doi: 10.1002/ejoc.201800860
Murahashi, S.-I., Komiya, N., Oda, Y., Kuwabara, T., and Naota, T. (2000). Ruthenium-catalyzed oxidation of alkanes with tert-butyl hydroperoxide and peracetic acid. J. Org. Chem. 65, 9186–9193. doi: 10.1021/jo001348f
Parmeggiani, C., and Cardona, F. (2012). Transition metal based catalysts in the aerobic oxidation of alcohols. Green Chem. 14, 547–564. doi: 10.1039/c2gc16344f
Punniyamurthy, T., Velusamy, S., and Iqbal, J. (2005). Recent advances in transition metal catalyzed oxidation of organic substrates with molecular oxygen. Chem. Rev. 105, 2329–2364. doi: 10.1021/cr050523v
Ren, L., Wang, L., Lv, Y., Shang, S., Chen, B., and Gao, S. (2015). Synthesis of 6, 7-dihydro-5H-cyclopenta[b]pyridin-5-one analogues through manganese-catalyzed oxidation of the CH2 adjacent to pyridine moiety in water. Green Chem. 17, 2369–2372. doi: 10.1039/C4GC02471K
Saleem, F., Rao, G. K., Kumar, A., Mukherjee, G., and Singh, A. K. (2013). Half-sandwich ruthenium (II) complexes of click generated 1, 2, 3-triazole based organosulfur/-selenium ligands: structural and donor site dependent catalytic oxidation and transfer hydrogenation aspects. Organometallics 32, 3595–3603. doi: 10.1021/om400057e
Salvatore, R. N., Yoon, C. H., and Jung, K. W. (2001). Synthesis of secondary amines. Tetrahedron 57, 7785–7812. doi: 10.1016/S0040-4020(01)00722-0
Santini, C. C., Basset, J.-M., Fontal, B., Krause, J., Shore, S., and Charrier, C. (1987). Surface organometallic chemistry: synthesis and X-ray structure of Ru3(CO)8(μ-OC6H4OMe-2)2, an oxygen stabilized triruthenium cluster. J. Chem. Soc. Chem. Commun. 512–513. doi: 10.1039/C39870000512
Sarbajna, A., Dutta, I., Daw, P., Dinda, S., Rahaman, S. W., Sarkar, A., et al. (2017). Catalytic conversion of alcohols to carboxylic acid salts and hydrogen with alkaline water. ACS Catal. 7, 2786–2790. doi: 10.1021/acscatal.6b03259
Sarkar, S. K., Jana, M. S., Mondal, T. K., and Sinha, C. (2014). Alcohol oxidation reactions catalyzed by ruthenium-carbonyl complexes of thioarylazoimidazoles. Appl. Organomet. Chem. 28, 641–651. doi: 10.1002/aoc.3174
Sarma, D., Majumdar, B., and Sarma, T. K. (2018). Carboxyl-functionalized carbon dots as competent visible light photocatalysts for aerobic oxygenation of alkyl benzenes: role of surface functionality. ACS Sustain. Chem. Eng. 6, 16573–16585. doi: 10.1021/acssuschemeng.8b03811
Shapley, P. A., Zhang, N., Allen, J. L., Pool, D. H., and Liang, H.-C. (2000). Selective alcohol oxidation with molecular oxygen catalyzed by Os-Cr and Ru-Cr complexes. J. Am. Chem. Soc. 122, 1079–1091. doi: 10.1021/ja982171y
Sheldon, R., Arends, I., Ten Brink, G.-J., and Dijksman, A. (2002). Green, catalytic oxidations of alcohols. Acc. Chem. Res. 35, 774–781. doi: 10.1021/ar010075n
Sheldon, R. A., Arends, I., and Dijksman, A. (2000). New developments in catalytic alcohol oxidations for fine chemicals synthesis. Catal. Today 57, 157–166. doi: 10.1016/S0920-5861(99)00317-X
Sheldrick, G. H. (1997). SHELX 97, A Software Package for the Solution and Refinement of X-ray Data. Göttingen: University of Göttingen.
Sigman, M. S., and Jensen, D. R. (2006). Ligand-modulated palladium-catalyzed aerobic alcohol oxidations. Acc. Chem. Res. 39, 221–229. doi: 10.1021/ar040243m
Simon, M.-O., and Li, C.-J. (2012). Green chemistry oriented organic synthesis in water. Chem. Soc. Rev. 41, 1415–1427. doi: 10.1039/C1CS15222J
Song, D., and Morris, R. H. (2004). Cyclometalated tridentate CNN ligands with an amine or amido donor in platinum (II) and palladium (II) complexes and a novel potassium alkoxide aggregate. Organometallics 23, 4406–4413. doi: 10.1021/om0496232
Stahl, S. S. (2004). Palladium oxidase catalysis: selective oxidation of organic chemicals by direct dioxygen-coupled turnover. Angew. Chem. Int. Ed. 43, 3400–3420. doi: 10.1002/anie.200300630
Stanje, B., Traar, P., Schachner, J., Belaj, F., and Mösch-Zanetti, N. (2018). Iron catalyzed oxidation of benzylic alcohols to benzoic acids. Dalton Trans. 47, 6412–6420. doi: 10.1039/C8DT00819A
Tang, L.-H., Wu, F., Lin, H., Jia, A.-Q., and Zhang, Q.-F. (2018). Synthesis, structure and catalytic alcohol oxidation by ruthenium (III) supported by schiff base and triphenylphosphine ligands. Inorg. Chim. Acta 477, 212–218. doi: 10.1016/j.ica.2018.03.017
Tojo, G., and Fernández, M. (2007). Oxidation of Primary Alcohols to Carboxylic Acids. A Guide to Current Common Practice (New York, NY: Springer).
Tsukahara, T., Swenson, D. C., and Jordan, R. F. (1997). Neutral and cationic zirconium benzyl complexes containing bidentate pyridine-alkoxide ligands. synthesis and olefin polymerization chemistry of (pyCR2O)2Zr(CH2Ph)2 and (pyCR2O)2Zr (CH2Ph) complexes. Organometallics 16, 3303–3313. doi: 10.1021/om9702439
Uyanik, M., and Ishihara, K. (2009). Hypervalent iodine-mediated oxidation of alcohols. Chem. Commun. 16, 2086–2099. doi: 10.1039/b823399c
Vazylyev, M., Sloboda-Rozner, D., Haimov, A., Maayan, G., and Neumann, R. (2005). Strategies for oxidation catalyzed by polyoxometalates at the interface of homogeneous and heterogeneous catalysis. Top. Catal. 34, 93–99. doi: 10.1007/s11244-005-3793-5
Velusamy, S., Srinivasan, A., and Punniyamurthy, T. (2006). Copper (II) catalyzed selective oxidation of primary alcohols to aldehydes with atmospheric oxygen. Tetrahedron Lett. 47, 923–926. doi: 10.1016/j.tetlet.2005.11.149
Vermaak, V., Young, D. A., and Swarts, A. J. (2018). Catalytic oxidation of alcohols with novel non-heme N 4-tetradentate manganese (ii) complexes. Dalton Trans. 47, 16534–16542. doi: 10.1039/C8DT03808B
Wang, N., Liu, R., Chen, J., and Liang, X. (2005). NaNO2-activated, iron–TEMPO catalyst system for aerobic alcohol oxidation under mild conditions. Chem. Commun. 42, 5322–5324. doi: 10.1039/b509167e
Wang, Q., Chai, H., and Yu, Z. (2017). Dimeric ruthenium (II)-NNN complex catalysts bearing a pyrazolyl-pyridylamino-pyridine ligand for transfer hydrogenation of ketones and acceptorless dehydrogenation of alcohols. Organometallics 36, 3638–3644. doi: 10.1021/acs.organomet.7b00587
Wang, X., Liu, R., Jin, Y., and Liang, X. (2008). TEMPO/HCl/NaNO2 catalyst: a transition-metal-free approach to efficient aerobic oxidation of alcohols to aldehydes and ketones under mild conditions. Chem. Eur. J. 14, 2679–2685. doi: 10.1002/chem.200701818
Keywords: ruthenium carbonyl complexes, alcohols oxidation, t-butyl hydroperoxide, pyridine alcohols, chemoselectivity
Citation: Yan X, Yue X, Liu K, Hao Z, Han Z and Lin J (2019) Synthesis and Structures of Ruthenium Carbonyl Complexes Bearing Pyridine-Alkoxide Ligands and Their Catalytic Activity in Alcohol Oxidation. Front. Chem. 7:394. doi: 10.3389/fchem.2019.00394
Received: 01 April 2019; Accepted: 16 May 2019;
Published: 04 June 2019.
Edited by:
Feng Luo, East China University of Science and Technology, ChinaReviewed by:
Baiquan Wang, Nankai University, ChinaMuhammad Hanif, The University of Auckland, New Zealand
Copyright © 2019 Yan, Yue, Liu, Hao, Han and Lin. This is an open-access article distributed under the terms of the Creative Commons Attribution License (CC BY). The use, distribution or reproduction in other forums is permitted, provided the original author(s) and the copyright owner(s) are credited and that the original publication in this journal is cited, in accordance with accepted academic practice. No use, distribution or reproduction is permitted which does not comply with these terms.
*Correspondence: Zhiqiang Hao, aGFvemhpcWlhbmcxMDAxQDE2My5jb20=; Jin Lin, bGluamluNjRAMTI2LmNvbQ==
†These authors have contributed equally to this work