- 1Department of Chemistry, National Chung Hsing University, Taichung, Taiwan
- 2Department of Chemistry, Graduate School of Science, The University of Tokyo, Tokyo, Japan
- 3Department of Medicinal and Applied Chemistry, Kaohsiung Medical University, Kaohsiung, Taiwan
- 4Department of Medical Research, Kaohsiung Medical University Hospital, Kaohsiung, Taiwan
A series of aminophenolate ligands with various pendant groups and associated ethyl Zn complexes were synthesized and studied as catalysts for the ring-opening polymerization (ROP) of lactides (LAs). The thiophenylmethyl group (L4ZnEt) increased the catalytic activity more than the benzyl group (L1ZnEt) did, and 2-fluorobenzyl (L3ZnEt) and 2-methoxybenzyl (L2ZnEt) groups had the opposite effect. In addition, the LA polymerization mechanism proved by Nuclear Magnetic Resonance and Density Function Theory was that LA was attracted by H···O bond of an α-hydrogen of the LA molecule and the phenoxyl oxygen of the catalyst. After the dissociation of amino group from the Zn atom, the benzyl alcohol initiated LA without replacing the ethyl group of Zn complex. It is the first case where the ethyl group is regarded as a ligand and cannot be replaced by benzyl alcohol, and this information is very important for the mechanism study of ROP.
Introduction
Because petrochemical polymers such as polystyrene, polypropylene, polyethylene, and poly(vinyl chloride) can be produced easily and cheaply, they are widely used as disposable packaging materials. Because these petrochemical polymers need more than a hundred years to degrade into innocuous soil manure (Rochman et al., 2013), polymer pollution has become a serious problem (Romer, 2010; Romer and Tamminen, 2014; Ladewig et al., 2015; Zhang et al., 2017). The replacement of non-biodegradable polymers with biodegradable materials is therefore a popular field of research (Levis and Barlaz, 2011). Poly(lactide) (PLA) is a biopolymer designed to ameliorate pollution by petrochemical plastics. Owing to its biodegradability, biocompatibility, and permeability, PLA is commonly used for various purposes, such as humidity detection (Sun et al., 2007), MRI contrast agents (Patel et al., 2008), cell/tissue anti-adhesion (Lih et al., 2015), nanocomposites (Raquez et al., 2013), drug delivery (Khemtong et al., 2009), blood circulation (Ma et al., 2008), bone replacement (Simpson et al., 2007), and tissue engineering (Place et al., 2009). Ring-opening polymerization (ROP) by using metal complexes (Bellemin and Dagorne, 2014; Guillaume et al., 2015; Sarazin and Carpentier, 2015; Huang et al., 2016; Fuoco and Pappalardo, 2017; Redshaw, 2017) as catalysts is a common method for the efficient synthesis of PLA. Because no cytotoxic metal residue is required in PLA for the biomaterials, the use of a non-cytotoxic metal such as zinc (Williams et al., 2003; Romain and Williams, 2014; Yang et al., 2015; Binda et al., 2016; Ebrahimi et al., 2016; Thevenon et al., 2016; Wang et al., 2016) in lactide polymerization has been investigated widely. Ligands are crucial for the catalyst design because their catalytic activity can be increased. In a study of Zn complexes (Williams et al., 2003) bearing tridentate aminophenolate ligands, a high catalytic activity was observed during rac-lactide (rac-LA) polymerization, as shown in Figure 1A. As in previous studies, the tetradentate aminophenol ligands reacted with Zn[N(SiMe3)2]2 and four coordinated Zn complexes with a non-coordinated fourth amino group were obtained, as shown in Figures 1B–D. According to the polymerization results of Figures 1B–E, the steric bulky substituents on the phenolate ring, the fourth coordinated amino groups, and chiral N-alkyl groups can increase the stereoselectivity of rac-LA polymerization, and maintain high catalytic activity. A survey of the coordination behavior of Zn complexes (Gao et al., 2013) revealed that five and six are the possible coordination numbers for these complexes, and even seven-coordinated Zn complexes were reported (Vaiana et al., 2007). It would be worthwhile to design the fourth coordinated group of tetradentate aminophenol ligands to interact with the Zn atom, and influence their catalytic activities. In this study, a series of aminophenol ligands and associated Zn complexes were synthesized, and their application in LAs polymerization was investigated.
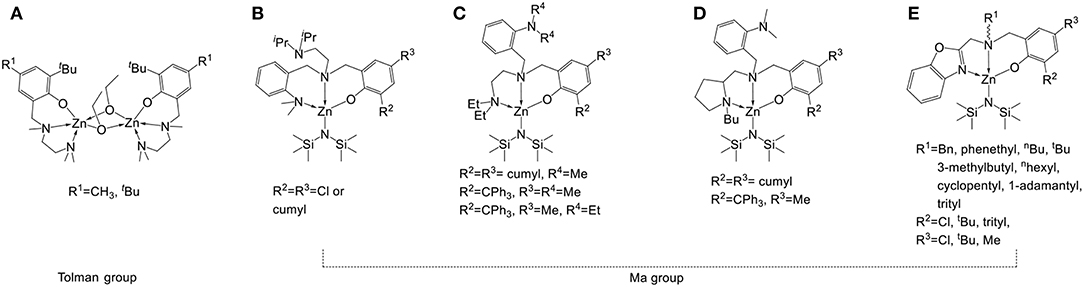
Figure 1. Zn complexes bearing tridentate (A; Williams et al., 2003), (E; Hu et al., 2018), and tetradentate (B–D; Yang et al., 2015) aminophenolate ligands.
Results and Discussion
Syntheses and Characterization
A series of N1-alkyl- N2,N2-dimethylethylene-1,2-diamines was synthesized by using NaBH4 to reduce 2-alkylideneamino-N,N-dimethylethylen-1-amines that were synthesized by condensing the aldehyde derivatives with dimethylethylenediamine in ethanol. All ligands L1-H–L4-H were prepared by refluxing a mixture of N1-alkyl- N2,N2-dimethylethylene-1,2-diamine, para-formaldehyde, and 2,4-bis(α,α-dimethylbenzyl)-phenol (Figure 2). All the ligands reacted with 1.1 equivalents of ZnEt2 in THF at 0°C to produce a moderate yield (74–83%) of Zn compounds after hexane washing. The Zn complex synthesis can be identified by the 1H NMR spectrum. The peaks of the methylene groups of HO[(tBu)2-Ph]CH2N and NCH2Ar from the 1H NMR spectrum are singlet in ligands and doublet of doublets in Zn complexes, and the proton of PhO-H (10.43–10.15 ppm) disappeared after ZnEt2 was added. The formulas and structures of the compounds were confirmed on the basis of 1H and 13C Nuclear Magnetic Resonance (NMR) spectra.
The X-ray structure of L1ZnEt (CCDC 1574177), Figure 3 revealed a four-coordinated, mononuclear Zn complex with one ethyl group and one aminophenolate ligand. In structural chemistry, the index for four-coordinated complexes (τ4) is the number that indicates the geometry of the coordination center (Yang et al., 2007). L1ZnEt showed an intermediate structure varying in geometry between trigonal pyramidal and seesaw (C2v, θ6 = 90°), with the corresponding τ4 value being 0.74. The distances between the Zn(1) atom and C(37) O(1), N(1), and N(2) atoms were 1.983(3), 1.9583(18), 2.159(2), and 2.121(2) Å, respectively.
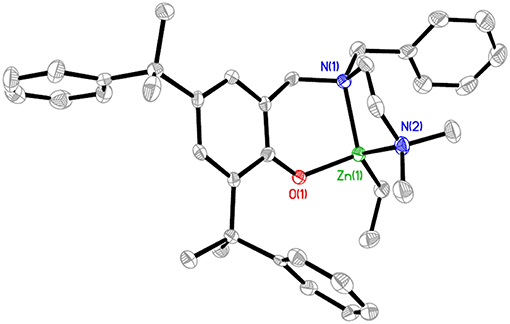
Figure 3. Molecular structure of L1ZnEt (ellipsoids drawn at the 50% probability, H atoms omitted for clarity). Selected bond lengths (Å) and angles (degrees): Zn(1)-O(1), 1.9583(18); Zn(1)-N(1), 2.159(2); Zn(1)-N(2), 2.121(2); Zn(1)-C(37), 1.983(3); O(1)-Zn(1)-N(1), 95.61(8); O(1)-Zn(1)-N(2), 102.08(9); O(1)-Zn(1)-C(37), 114.38(10); C(37)-Zn(1)-N(1), 134.15(11); C(37)-Zn(1)-N(2), 119.88(11); N(2)-Zn(1)-N(1), 83.55(9).
Ring Opening Polymerization of LAs
The catalytic activity of Zn complexes for L-LA and rac-LA polymerizations with benzyl alcohol (BnOH) as the initiator under a nitrogen atmosphere was investigated, and the results are given in Table 1. As shown by entries 1–4 in Table 1, all Zn complexes were active for L-LA polymerization at 25°C, producing polymers with narrow polydispersity indexes (Ð, 1.08–1.13). L4ZnEt had the most controllability of the polymer molar mass with similar values of Mncal, MnNMR, and MnGPC. However, the difference of catalytic activity of these Zn complexes was initially unclear. To determine the difference, the polymerization temperature was set at 100°C and polymerization was terminated after 2 h (entries 5–8 in Table 1). The catalytic activity of L-LA polymerization was in the following order: L4ZnEt > L1ZnEt > L3ZnEt > L2ZnEt. The results revealed that the thiophenylmethyl group of L4ZnEt increased the catalytic activity of Zn complexes more than the benzyl group of L1ZnEt did, and that 2-fluorobenzyl and 2-methoxybenzyl groups decreased the catalytic activity of the complexes. From the polymer data (entries 1–8 in Table 1), the values of MnNMR, and MnGPC were smaller than that of Mncal, and this phenomenon may be attributed to the transesterification. As shown by entries 9–12 in Table 1, the catalytic rates for rac-LA polymerization were the same as those for L-LA polymerization. The rac-PLA polymerized by L4ZnEt showed the highest selectivity. According to the literature, electronic donating substituents increased the catalytic activity of Zn catalysts (Chen et al., 2006, 2011, 2015; Huang et al., 2006; Chuang et al., 2011; Fliedel et al., 2014a,b). In our case, the pendant-chelating substituents do not coordinate with the Zn atom in the solid state, but they increase the catalytic activity. Crystal data imply that the fourth coordinated groups, such as thiophenylmethyl, 2-methoxybenzyl, and 2-fluorobenzyl groups, still do not coordinate to Zn atom, just like the behavior of the Zn complexes (1B-1D) shown in Figure 1; however, the low stereoselectivity of rac-LA polymerization was also observed in our case. These phenomena are ascribed, possibly, to the size of the pendant substituents. The reasons will be discussed with DFT results later. In addition, the Ð values of these rac-PLAs were higher than that of PLAs (Table 1), and it may be attributed to more transesterification at a higher temperature in longer polymerization time.
The controllability of polymer molar mass by using L4ZnEt as a catalyst was investigated, and the results are given in Table 2. The linear relationship between MnGPC and ([LA] × conv.)/[BnOH] exhibited in Figure 4 shows that the polymerization of LA by using L4ZnEt as a catalyst was highly controllable with acceptable Ð values.
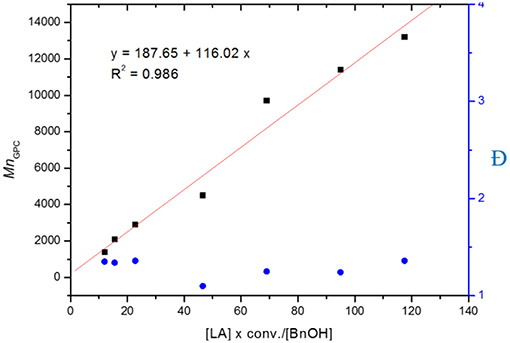
Figure 4. Linear plot of MnGPC vs. [LA] × conv. / [BnOH], with Ðindicated by blue dots (entries 1–7 in Table 2).
Kinetic Studies of Polymerization of L-LA by Using L3ZnEt
Kinetic studies of the polymerization of L-LA catalyzed by L3ZnEt in the presence of BnOH were performed to establish the reaction order with respect to [L-LA], [L3ZnEt], and [BnOH]. The experiments were performed at [L-LA]0/[L3ZnEt]/[BnOH] ratios of 50:1:1, 50:1:2, 50:1:3, 50:1:4, 50:2:1, 50:3:1, and 50:4:1 ([L-LA] = 0.5 M in 10 mL toluene at 25°C), respectively. The preliminary results indicated that the reaction rate was a first-order dependent on [L-LA] for all seven ratios (Figures 5, 7) according to Equation (1), where kobs = kprop[L3ZnEt]x[BnOH]y and kprop is the propagation rate constant. To determine the order (x) of [L3ZnEt], different [L3ZnEt] (10, 20, 30, and 40 mM) with the same [L-LA] (0.5 M) and [BnOH] (10 mM) were used (Figure 6). In addition, [BnOH] is regarded as a constant and incorporated into k1 (k1 = kprop[BnOH]y). The variable kobs is first assumed to be as described by Equation (2). When kobs is plotted against [L3ZnEt], a k1 values of 15.034 (M−1h−1) for x = 1 is obtained (Figure 6). The variable kobs is then assumed to be as described by Equation (3), where [L3ZnEt] is regarded as a constant and incorporated into k2. Furthermore, various concentrations of [BnOH] (10, 20, 30, and 40 mM) with the same [L-LA] (0.5 M) and [L3ZnEt] (10 mM) (Figure 7) are used. When kobs is plotted against [BnOH], a k2 values of 13.227 (M−1h−1) for y = 1 is obtained (Figure 8). Next, kprop is calculated to be 1,413 by averaging k1/[BnOH] and k2/[L3ZnEt]. L-LA polymerization using [L3ZnEt] and [BnOH] followed by an overall kinetic law given by Equation (4).
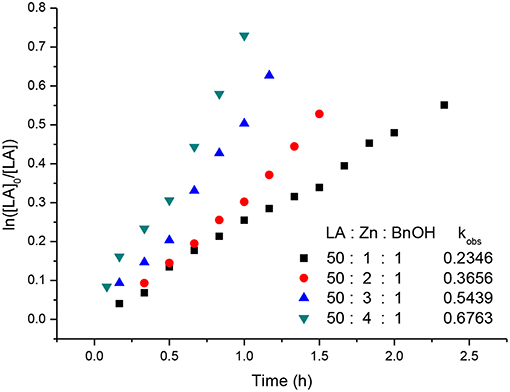
Figure 5. First-order kinetic plots for L-LA polymerizations vs. time in toluene (10 mL) at 25°C with various concentrations of complex [L3ZnEt].
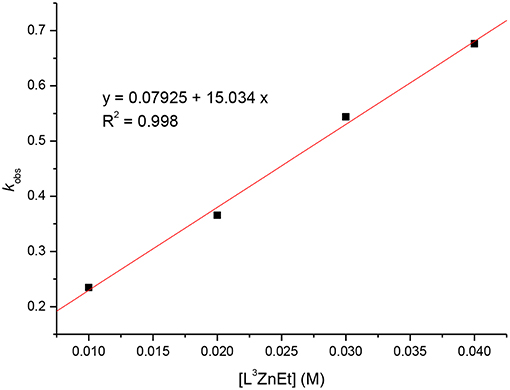
Figure 6. Linear plot of kobs vs. [L3ZnEt] for the polymerization of L-LA with [L-LA] = 0.5 M in toluene (10 mL) at 25°C.
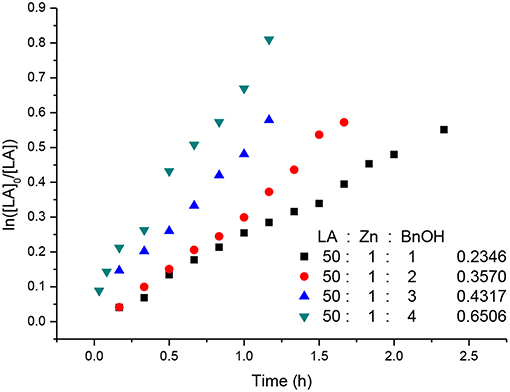
Figure 7. First-order kinetic plots for L-LA polymerizations vs. time in toluene (10 mL) at 25°C with various concentrations of [BnOH].
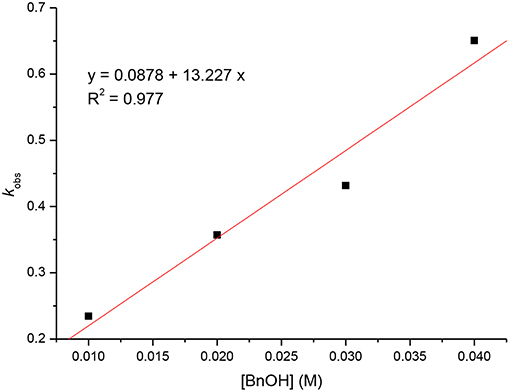
Figure 8. Linear plot of kobs vs. [BnOH] for the polymerization of L-LA with [L-LA] = 0.5 M in toluene (10 mL) at 25°C.
Proposed Mechanism
In order to realize the mechanism of LA polymerization by using Zn catalysts, the 1H NMR study of the reaction of L4ZnEt with one equivalent BnOH was investigated as shown in Figure S19. In Figure S19D, the 1H NMR spectrum revealed that L4ZnEt did not react with BnOH. This phenomenon was very surprising because most alkyl Zn complexes could react with alcohol to form Zn alkoxide complexes (Chuang et al., 2011; Fliedel et al., 2014a,b; Chen et al., 2015). To prove that the ethyl group of L4ZnEt could not be replaced by BnOH in polymerization process, the LA polymerization ([LA]:[Zn]:[BnOH] = 4:1:1, [LA] = 0.02 M in d8-toluene (0.5 mL) at 25°C) was monitored by 1H NMR as shown in Figure 9, Figure S20, and the ethyl group was always at 0.25 ppm from the beginning to the end of the polymerization (Figure S20). When these 1H NMR spectra revealed that the BnOH did not replace the ethyl group of the Zn complex in the LA polymerization process, we were curious about how BnOH initiated the monomer. To understand the polymerization mechanism, the interactions between the catalysts, the initiator, LA, and relative free energies of the intermediates of the catalytic reaction were studied using the DFT calculation.

Figure 9. 1H NMR spectra of the LA polymerization ([LA]:[Zn]:[BnOH]=4:1:1, [LA] = 0.02 M in d8-toluene (0.5 mL) at 25°C).
DFT Calculations: Mechanistic Study of LA Polymerization by Using Zn Complexes Bearing Aminophenolate Ligands as Catalysts
In our DFT calculations, the cumyl group at the 4 position of the phenol ring L2ZnEt was simplified to a hydrogen atom, since it is far from the zinc catalytic center. The initiator was replaced by a methanol molecule because different alcohol molecules often show similar activity (Chang et al., 2015). The mechanism is shown in Figure 10.
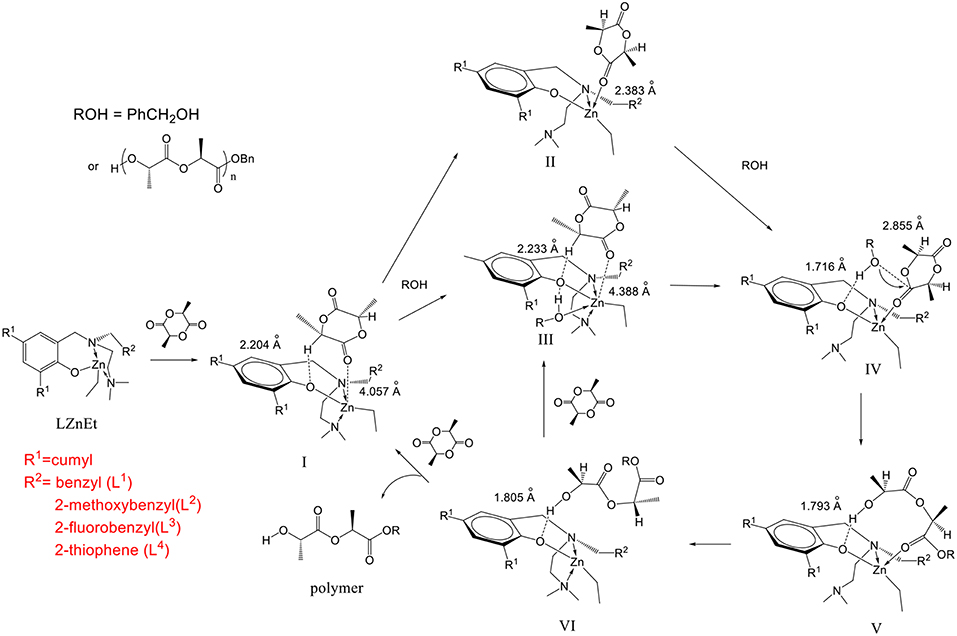
Figure 10. Mechanism of L-lactide polymerization with N,N,O-tridentate aminophenolate Zn complexes as catalysts and benzyl alcohol as an initiator.
When the catalyst reacted with LA, the LA molecule was initially stably bonded in an open pocket formed between the R1 group on the 2 position of the phenol ring and the pendant R2 group to form intermediate I as shown in Figure 11. The major interaction to stabilize this structure was found to be a unique hydrogen bond between an α-hydrogen of the LA molecule and the phenoxyl oxygen of the catalyst with a H···O distance of 2.204 Å. I then went through two possible pathways to form key catalytic intermediate IV. One of possible pathways is that the NMe2 group dissociated from the Zn center to produce an empty space to be coordinated by a carbonyl oxygen atom with a Zn-O bond (2.383 Å) to form intermediate II. After that, the initiator came into the complex to form intermediate IV. Another possible pathway is that the initiator (ROH) bonded to the Zn complex with a coordination bond between its oxygen atom and the Zn center (2.508 Å) and a hydrogen bond between the hydroxyl hydrogen atom of ROH and phenoxyl oxygen of catalyst to form intermediate III. The NMe2 group subsequently left the Zn atom to make a rearrangement to generate IV. In intermediate IV, the O atom of the initiator was close to the carbonyl group of LA with a distance of 2.855 Å to facilitate the ring opening reaction. Moreover, the hydrogen bond between the initiator and the phenoxyl O atom of Zn catalyst stabilized this transient intermediate structure (IV) and also activated the initiator. After the LA ring opening, a new hydroxyl group formed at one end of the polymer (or oligomer) and interacted with phenoxyl oxygen through a hydrogen bond with a coordination between the zinc center and the carbonyl group on the other end to form intermediate V. The NMe2 group then came back to re-coordinate on the zinc center to assist the leaving of the carbonyl group of the polymer to form intermediate VI and re-activated the catalyst. Finally, another LA monomer came in to reform intermediate III to continue the polymerization reaction or to expel the polymer from the catalyst to regenerate intermediate I to finish one catalytic cycle.
From the thermochemical data of our DFT studies as shown in Figure 12 and Table 3, it can be found that to generate IV from I, the pathway through II was a little more favored, since the relative free energy of II + MeOH was slightly lower than that of III (2.276 kcal mol−1 lower). This is because the molecular freedom (entropy) of II + methanol was much higher than that of III. After forming IV, the ring opening reaction to form V makes its relative free energy increase, due to the fact that the bulky polymer chain was restricted on the catalyst. However, after the carbonyl group dissociated from the zinc atom, the relative free energy of VI decreased dramatically. This large free energy decrease is a strong driving force to make the catalyst open the lactide ring.
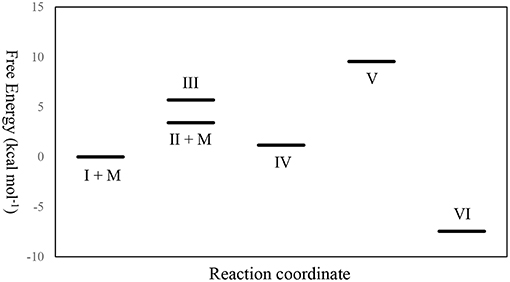
Figure 12. The relative free energy level diagram of all intermediates in the mechanism. M stands for methanol.
From our DFT studies, some important experimental phenomena can be well-explained. First, the stability of the basic Et group on the Zn atom of the catalyst can be explained by noting that the acidic proton of the initiator (RO-H) was retained on the phenoxyl oxygen of catalyst by hydrogen bond and the crowded structures of the catalysts (III, IV, and VI). These protected the Et group on the Zn atom from the acid proton. Second, the relative activities of the four catalysts can also be rationalized, possibly by the size of the pocket for accepting LA on the catalysts found in intermediate I, besides the electronic effects on the zinc center. The size of the pocket decides the easiness of LA entering the catalyst center, and the smaller pocket size also makes monomer coordination slightly different between D-LA and L-LA to reveal the higher stereselectivity in rac-LA polymerization. The pocket sizes of the four catalysts according to the sizes of the pendant groups and the percent buried volume (%Vbur) of the ligands with a sphere radius of 7 Å measured from the optimized L'ZnEt structures (Supporting Information) are in the following order: L4′ZnEt (32.6%) > L1′ZnEt (32.8%) > L3′ZnEt (33.4%) > L2′ZnEt (33.6%) (Falivene et al., 2016). This order is coincident with that of their catalytic activities and opposed to stereselectivity.
Conclusion
In this study, a series of aminophenolate ligands with various pendant groups and associated ethyl Zn complexes were synthesized to investigate the effect of pendant groups on the catalytic activity of the complexes during LA polymerization. The thiophenylmethyl group (L4ZnEt) increased the catalytic activity more than the benzyl group (L1ZnEt) did, and 2-fluorobenzyl (L3ZnEt) and 2-methoxybenzyl (L2ZnEt) groups showed the opposite effect. The new LA polymerization mechanism was proven by NMR study and DFT calculation, which showed that LA was attracted by the H···O bond of an α-hydrogen of the LA molecule and the phenoxyl oxygen of the catalyst. After the dissociation of the amino group from the Zn atom, the benzyl alcohol initiated LA without replacing the ethyl group of the Zn complex. It is the first case that the ethyl group is regarded as a ligand and cannot be replaced by benzyl alcohol. This information provides researchers with another possible mechanisms of ROP by using alkyl zinc complexes as catalysts.
Experiment Section
General
Standard Schlenk techniques and a N2-filled glove box were used throughout the isolation and handling of all the compounds. Solvents, L-LA, and deuterated solvents were purified prior to use. N,N-dimethylethylenediamine, 2,4-bis(α,α-dimethylbenzyl)phenol, sodium borohydride, benzaldehyde, 2-methoxybenzaldehyde, 2-fluorobenzaldehyde, 2-thiophenecarboxaldehyde, and diethyl zinc were purchased from Aldrich. 1H and 13C NMR spectra were recorded on a Varian Unity Inova-600 (600 MHz for 1H and 150 MHz for 13C) or a Varian Mercury-400 (400 MHz for 1H and 100 MHz for 13C) spectrometer with chemical shifts given in ppm from the internal tetramethylsilane or the central line of CDCl3. Gel permeation chromatography (GPC) measurements were performed on a Jasco PU-2080 plus system equipped with a RI-2031 detector using THF (high-performance liquid chromatography grade) as an eluent (flow rate 1.0 mL/min, at 40°C). The chromatographic column was Phenomenex Phenogel 5 μm 103 Å, and the calibration curve used to calculate Mn(GPC) was produced from polystyrene standards. The GPC results were calculated using the Scientific Information Service Corporation (SISC) chromatography data solution 3.1 edition.
Ligands and Associated Zn Complexes Synthesis
Synthesis of L1-H
N,N-Dimethylethylenediamine (0.88 g, 10 mmole) and benzaldehyde (1.06 g, 10 mmole) were refluxed in ethanol (20 mL) for 6 h. When the solution was cooled to 25°C, sodium borohydride (0.42 g, 11 mmole) was added and stirred overnight to form N1-benzyl-N2,N2-dimethylethane-1,2-diamine. After removing the precipitate from the solution, 2,4-bis(α,α-dimethylbenzyl)phenol (3.3 g, 10 mmole) and paraformaldehyde (0.45 g, 15 mmole) were transferred into the solution and refluxed for 12 h. Volatile materials were removed under vacuum to produce yellow mud. The mud was dissolved in CH2Cl2 (20 mL) and the solution was washed with water (3 × 40 mL), and the solvent removed at reduced pressure to produce the white powders. Yield: 3.08 g (59%). 1H NMR (CDCl3, 400 MHz): δ10.42 (1H, s, ArOH), 7.27 (5H, m, PhH), 7.23 (4H, m, PhH), 7.17 (5H, m, PhH), 6.91 (1H, m, PhH), 6.89 (1H, m, ArH), 6.75 (1H, d, ArH), 3.60 (2H, s, NCH2Ar), 3.37 (2H, s, NCH2Ph), 2.39 (2H, t, J = 4 Hz), NCH2CH2N), 2.27 (2H, t, J = 4 Hz, NCH2CH2N), 1.96 (6H, s, NCH3), 1.68 (12H, s, C(CH3)2Ph). 13C NMR (CDCl3, 100 MHz): δ153.46 (ArC-OH), 151.65, 151.46, 139.50, 137.52, 135.31, 129.69, 128.14, 127.80, 127.55, 127.07, 126.70, 126.37, 125.66, 125.30, 124.62, 124.54, 122.19 (ArC and PhC), 57.85 (NCPh), 57.37 (CN(CH3)2), 55.82 (ArCN), 49.84 (NCCN), 45.07 (CN(CH3)2), 42.40, 41.99 (ArC(CH3)2Ph), 31.10, 29.44 (ArC(CH3)2Ph). Anal. Calc. (found) for C36H44N2O: C 82.76% (83.03%), H 8.35% (8.52%), N 5.36% (5.38%).
Synthesis of L2-H
We used a method similar to that for L1-H, except 2-methoxybenzaldehyde was used to replace benzaldehyde. Yield: 2.86 g (52%). 1H NMR (CDCl3, 400 MHz): δ10.22 (1H, s, ArOH), 7.24 (5H, m, PhH), 7.20 (4H, m, PhH), 7.16 (3H, m, PhH), 6.88 (1H, m, ArH), 6.78 (2H, m, PhH), 6.71 (1H, d, ArH), 3.64 (3H, s, PhOCH3), 3.59 (2H, s, NCH2Ar), 3.54 (2H, s, NCH2Ph), 2.41 (2H, t, J = 4 Hz, NCH2CH2N), 2.18 (2H, t, J = 4 Hz, NCH2CH2N), 2.01 (6H, s, NCH3), 1.67 (12H, s, C(CH3)2Ph). 13C NMR (CDCl3, 100 MHz): δ157.92 (PhCOCH3), 153.62 (ArC-OH), 151.64, 151.45, 139.42, 135.06, 131.63, 128.68, 127.76, 127.45, 126.66, 125.84, 125.65, 125.26, 125.23, 124.47, 124.36, 122.14, 120.26, 110.15 (ArC and PhC), 58.09 (NCPh), 56.08 [CN(CH3)2], 54.96 (ArCN), 53.05 (PhOCH3), 50.18 (NCCN), 45.23 [CN(CH3)2], 42.34, 41.86 [ArC(CH3)2Ph], 31.04, 29.35 [ArC(CH3)2Ph]. Anal. Calc. (found) for C37H46N2O2: C 79.79% (80.69%), H 8.30% (8.42%), N 5.04% (5.09%).
Synthesis of L3-H
We used a method similar to that for L1-H, except 2-florobenzaldehyde was used to replace benzaldehyde. Yield: 3.39 g (63%). 1H NMR (CDCl3, 400 MHz): δ10.32 (1H, s, ArOH), 7.25 (5H, m, PhH), 7.22 (4H, m, PhH), 7.15 (3H, m, PhH), 6.93 (1H, m, PhH), 6.91 (1H, m, ArH), 6.76 (1H, m, PhH), 6.73 (1H, d, ArH), 3.61 (2H, s, NCH2Ar), 3.47 (2H, s, NCH2Ph), 2.42 (2H, t, J = 4 Hz, NCH2CH2N), 2.28 (2H, t, J = 4 Hz, NCH2CH2N), 1.98 (6H, s, NCH3), 1.68 [12H, s, C(CH3)2Ph]. 13C NMR (CDCl3, 100 MHz): δ162.51 (PhCF), 160.07 (ArC-OH), 153.48, 151.64, 151.42, 139.56, 135.30, 132.36, 132.32, 128.85, 128.77, 127.80, 127.52, 126.69, 126.34, 125.66, 125.31, 124.67, 124.54, 124.15, 124.11, 124.03, 122.03 (ArC and PhC), 57.31 (NCPh), 55.84 [CN(CH3)2], 49.89 (ArCN), 43.59 (NCCN), 45.06 [CN(CH3)2], 42.39, 41.99 [ArC(CH3)2Ph], 31.07, 29.42 [ArC(CH3)2Ph]. Anal. Calc. (found) for C36H43N2OF: C 80.12% (80.26%), H 8.07% (8.04%), N 5.40% (5.20%).
Synthesis of L4-H
We used a method similar to that for L1-H, except 2-thiophenecarboxaldehyde was used to replace benzaldehyde.Yield: 3.16 g (60%). 1H NMR (CDCl3, 400 MHz): δ10.15 (1H, s, ArOH), 7.27 (4H, d, PhH), 7.22 (4H, d, PhH), 7.15 (2H, m, PhH), 7.10 (1H, m, PhH), 6.87 (1H, t, CHCHCHS), 6.72 (1H, d, CHCHCHS), 6.63 (1H, d, CHCHCHS), 3.69 (2H, s, NCH2Ar), 3.59 (2H, s, NCH2Ph), 2.51 (2H, t, J = 4 Hz, NCH2CH2N), 2.32 (2H, t, J = 4 Hz, NCH2CH2N), 2.05 (6H, s, NCH3), 1.68 [12H, s, C(CH3)2Ph]. 13C NMR (CDCl3, 100 MHz): δ153.60 (ArC-OH), 151.53, 151.43, 139.68, 138.53, 135.23, 127.81, 127.56, 126.70, 126.64, 126.48, 125.64, 125.31, 125.14, 124.69, 124.61, 121.76 (ArC, PhC, and thioC), 56.70 (NCPh), 56.27 [CN(CH3)2], 50.88 (ArCN), 49.71 (NCCN), 45.19 [CN(CH3)2], 42.38, 42.05 [ArC(CH3)2Ph], 31.03, 29.48 [ArC(CH3)2Ph]. Anal. Calc. (found) for C34H42N2OS: C 77.08% (77.52%), H 7.69% (8.04%), N 5.11% (5.32%).
Synthesis of L1ZnEt
ZnEt2 (0.617 g, 5.0 mmol) was added slowly to an ice cold (0°C) solution of L1-H (2.6 g, 5.0 mmol) in THF (15 mL), and the solution was stirred for 3 h. Volatile materials were removed under vacuum to yield a yellow mud. The mud was washed with hexane (20 mL) and a white powder was obtained after filtration. Yield: 2.54 g (83%). 1H NMR (CDCl3, 400 MHz): δ7.48 (2H, d, PhH), 7.41 (3H, t, PhH), 7.27 (7H, m, PhH), 7.18 (1H, m, PhH), 7.10 (1H, t, PhH), 4.01, 3.93 (2H, d, J = 6 Hz, NCH2Ph), 3.92, 3.23 (2H, d, J = 6 Hz, NCH2Ph), 2.67 (1H, m, NCH2CH2N), 2.45 (1H, m, NCH2CH2N), 2.33 (1H, m, NCH2CH2N), 2.16 (1H, m, NCH2CH2N), 2.11 (3H, s, NCH3), 1.98 [3H, s, C(CH3)2Ph], 1.71 [3H, s, C(CH3)2Ph], 1.68 [6H, s, C(CH3)2Ph], 1.33 (3H, t, ZnCH2CH3), 1.28 (3H, s, NCH3), 0.12 (2H, m, ZnCH2CH3). 13C NMR (CDCl3, 100 MHz): δ164.87 (ArC-OH), 152.52, 151.02, 136.60, 133.63, 132.30, 131.75, 131.59, 128.41, 128.41, 128.36, 128.15, 127.82, 127.69, 127.56, 127.28, 127.13, 126.98, 126.72, 125.88, 125.71, 124.98, 124.18, 124.84 (ArC and PhC), 59.96 (NCPh), 58.44 [CN(CH3)2], 57.25 (ArCN), 46.85 (NCCN), 45.15 [CN(CH3)2], 44.01, 42.14 [ArC(CH3)2Ph], 31.02, 26.78 [ArC(CH3)2Ph], 13.21 (ZnCH2CH3),−3.17 (ZnCH2CH3). Anal. Calc. (found) for C38H48N2OZn: C 74.66% (74.31%), H 7.97% (7.88%), N 4.86% (4.56%).
Synthesis of L2ZnEt
We used a method similar to that for L2ZnEt, except L2-H was used to replace L1-H. L2ZnEt followed the procedure of L2ZnEt. Yield: 2.51g (78%). 1H NMR (CDCl3, 400 MHz): δ7.45 (2H, d, PhH), 7.33 (2H, m, PhH), 7.19 (7H, m, PhH), 7.07 (2H, m, PhH), 6.93 (2H, m, PhH), 6.51 (1H, s, PhH), 4.14, 4.06 (2H, d, J = 6 Hz, NCH2Ph), 3.91, 3.12 (2H, d, J = 6 Hz, NCH2Ph), 3.76 (3H, s, PhOCH3), 2.50 (2H, m, NCH2CH2N), 2.34 (2H, m, NCH2CH2N), 2.01 (3H, s, NCH3), 1.95 [3H, s, C(CH3)2Ph], 1.64 [3H, s, C(CH3)2Ph], 1.61 [6H, s, C(CH3)2Ph], 1.32 (3H, t, ZnCH2CH3), 1.06 (3H, s, NCH3), 0.09 (2H, m, ZnCH2CH3). 13C NMR (CDCl3, 100 MHz): δ165.03 (PhCOCH3), 164.14 (ArC-OH), 158.42, 152.59, 152.10, 150.76, 150.31, 133.85, 129.91, 128.23, 127.61, 127.48, 127.44, 126.89, 126.63, 125.69, 124.89, 124.12, 120.28, 111.00, 110.78 (ArC and PhC), 67.91 (PhOCH3), 58.91 (NCPh), 57.52 [CN(CH3)2], 55.29 (ArCN), 52.47 (NCCN), 45.34 [CN(CH3)2], 42.08, 31.10 [ArC(CH3)2Ph], 29.39, 26.21 [ArC(CH3)2Ph], (ZnCH2CH3) 13.21,−3.63 (ZnCH2CH3). Anal. Calc. (found) for C39H50N2O2Zn: C 72.27% (72.71%), H 7.28% (7.82%), N 4.52% (4.35%).
Synthesis of L3ZnEt
We used a method similar to that for L3ZnEt, except L3-H was used to replace L1-H.Yield: 2.58 g (82%). 1H NMR (CDCl3, 400 MHz): δ7.42 (2H, d, PhH), 7.33 (2H, m, PhH), 7.18 (7H, m, PhH), 7.11 (2H, m, PhH), 7.06 (2H, m, PhH), 6.48 (1H, s, PhH), 4.08, 3.94 (2H, d, J = 6 Hz NCH2Ph), 3.91, 3.14 (2H, d, J = 6 Hz, NCH2Ph), 2.47-2.44 (2H, m, NCH2CH2N), 2.08 (3H, s, NCH3), 2.00-1.94 (2H, m, NCH2CH2N), 1.92 [3H, s, C(CH3)2Ph], 1.63 [3H, s, C(CH3)2Ph], 1.61 [6H, s, C(CH3)2Ph], 1.28 (3H, t, ZnCH2CH3), 1.10 (3H, s, NCH3), 0.05 (2H, m, ZnCH2CH3). 13C NMR (CDCl3, 100 MHz): δ164.91 (PhCF), 163.01 (ArC-OH), 160.57, 152.55, 150.87, 136.62, 134.07, 133.66, 130.53, 128.23, 127.56, 127.39, 126.96, 126.70, 125.86, 124.98, 124.19, 121.63, 119.53, 119.37, 115.87, 115.64 (ArC and PhC), 58.36 (NCPh), 57.24 [CN(CH3)2], 51.92 (ArCN), 46.11 (NCCN), 45.15 [CN(CH3)2], 42.15, 41.96 [ArC(CH3)2Ph], 31.04, 26.62 [ArC(CH3)2Ph], (ZnCH2CH3) 13.18,−3.68 (ZnCH2CH3). Anal. Calc. (found) for C38H47N2OFZn: C 71.96% (72.20%), H 7.49% (7.49%), N 4.52% (4.43%).
Synthesis of L4ZnEt
We used a method similar to that for L4ZnEt, except L4-H was used to replace L1-H. Yield: 2.29 g (74%). 1H NMR (CDCl3, 400 MHz): δ 7.40 (2H, d, J = 4 Hz, PhH), 7.29 (1H, d, J = 2 Hz, thio-H), 7.21-7.10 (7H, m, PhH), 7.05-6.99 (1H, m, PhH), 6.88 (1H, s, PhH), 6.56 (1H, s, PhH), 4.18, 3.98 (2H, d, J = 6 Hz, NCH2Ph), 3.73, 3.31 (1H, d, J = 6 Hz, NCH2Ph), 2.66, 2.46 (2H, m, NCH2CH2N), 2.30, 2.23 (2H, m, NCH2CH2N), 2.11 (3H, s, NCH3), 1.89 [3H, s, C(CH3)2Ph], 1.65 [3H, s, C(CH3)2Ph], 1.62 [6H, s, C(CH3)2Ph], 1.35 (3H, s, NCH3), 1.22 (3H, t, ZnCH2CH3), 0.01 (2H, m, ZnCH2CH3). 13C NMR (CDCl3, 100 MHz): δ164.78 (ArC-OH), 152.49, 151.11, 136.79, 133.75, 133.65, 130.30, 128.10, 127.58, 127.21, 127.11,126.98, 126.73, 126.58, 125.97, 124.98, 124.17, 121.85 (ArC, PhC and thioC), 57.26 (NCPh), 57.10 [CN(CH3)2], 53.07 (ArCN), 47.49 (NCCN), 46.84 [CN(CH3)2], 44.28, 42.06 [ArC(CH3)2Ph], 30.99, 26.98 [ArC(CH3)2Ph], (ZnCH2CH3) 13.18,−3.84 (ZnCH2CH3). Anal. Calc. (found) for C36H46N2OSZn: C 69.65% (69.74%), H 7.43% (7.48%), N 4.68% (4.52%).
General Procedures for the Polymerization of L-LA and rac-LA
A typical polymerization procedure was exemplified by the synthesis of entry 1 of Table 1. The mixture of L-lactide (0.72 g, 5 mmol) and BnOH (5 mmol) in 5 mL toluene was added in the solution of zinc complex in toluene (5 mL) at 25°C. After the solution was stirred for 6.5 h, the reaction was then quenched by adding to a drop of ethanol, and the polymer was precipitated pouring into n-hexane (20.0 mL) to give white solids. The white solid was dissolved in CH2Cl2 (2.0 mL) and then n-hexane (20.0 mL) was added to give white crystalline solid. For rac-PLA, the Pr values for the selectivity of the heterotactic PLA were identified through 1H NMR spectra (5.0−5.2 ppm) of rac-PLA after decoupling at 1.57 ppm (methine group of rac-PLA) as shown in Figure S21.
X-Ray Crystallographic Study
X-ray diffraction data for a suitable crystal of L1ZnEt was collected on an Oxford diffraction limited Gemini S with graphite-monochromated Mo-Ka (λ = 0.71073 Å) radiation. All data were collected at 150 K with the ω-scan techniques. The structure was solved by direct methods and refined using Fourier techniques. An absorption correction based on SADABS was applied. All non-hydrogen atoms were refined by full-matrix least squares on F2 using the SHELXTL program package. Hydrogen atoms were located and refined by the geometry method. The cell refinement, data collection and reduction were done through the use of CrysAlisPro, Agilent Technologies, Version 1.171.37.31. The structure solution and refinement were performed by using SHELXS-97 and SHELXL-97, respectively. Molecular structure was generated by using the SHELXTL program.
DFT Calculation
The DFT calculation was carried out using the Gaussian09 program. The structures were built according to the X-ray structure of L1ZnEt. Their geometry optimizations were carried out using B3LPY (Becke, 1993). The LanL2DZ basis set (Hay and Wadt, 1985a,b) was used for Zn atom, and the 6-31G(d) basis set (Ditchfield et al., 1971) was used for other atoms. The minimum energy stationary point was confirmed by frequency analysis with the same calculation level.
Author Contributions
Theoretical calculation was performed by K-HW and the rest of work was performed by W-YL under the supervision of C-CL and H-YC.
Conflict of Interest Statement
The authors declare that the research was conducted in the absence of any commercial or financial relationships that could be construed as a potential conflict of interest.
Acknowledgments
This study was supported by the Ministry of Science and Technology of Taiwan (Grant MOST 107-2113-M-037-001 and 104-2113-M-005-015-MY3) and Kaohsiung Medical University NSYSU-KMU JOINT RESEARCH PROJECT (NSYSUKMU 107-P010). We thank the Center for Research Resources and Development at Kaohsiung Medical University for instrumentation and equipment support.
Supplementary Material
The Supplementary Material for this article can be found online at: https://www.frontiersin.org/articles/10.3389/fchem.2019.00189/full#supplementary-material
References
Becke, A. D. (1993). Density-functional thermochemistry. III. The role of exact exchange. J. Chem. Phys. 98, 5648–5652. doi: 10.1063/1.464913
Bellemin, S., and Dagorne, S. (2014). Group 1 and 2 and early transition metal complexes bearing N-heterocyclic carbene ligands: coordination chemistry, reactivity, and applications. Chem. Rev. 114, 8747–8774. doi: 10.1021/cr500227y
Binda, P., Rivers, K., and Padgett, C. (2016). Zinc complexes of new chiral aminophenolate ligands: synthesis, characterization and reactivity toward lactide. Open J. Inorg. Chem. 6, 205–218. doi: 10.4236/ojic.2016.63016
Chang, M.-C., Lu, W.-Y., Chang, H.-Y., Lai, Y.-C., Chiang, M. Y., Chen, H.-Y., et al. (2015). Comparative study of aluminum complexes bearing N,O- and N,S- Schiff base in ring-opening polymerization of ε-caprolactone and L-lactide. Inorg. Chem. 54, 11292–11298. doi: 10.1021/acs.inorgchem.5b01858
Chen, C.-T., Wang, M.-C., and Huang, T.-L. (2015). Zinc complexes containing coumarin-derived anilido-aldimine ligands as catalysts for ring opening polymerization of L-lactide. Molecules 20, 5313–5328. doi: 10.3390/molecules20045313
Chen, H.-Y., Peng, Y.-L., Huang, T.-H., Sutar, A. K., Miller, S. A., and Lin, C.-C. (2011). Comparative study of lactide polymerization by zinc alkoxide complexes with a β-diketiminato ligand bearing different substituents J. Mol. Catal. A-Chem. 339, 61–71. doi: 10.1016/j.molcata.2011.02.013
Chen, H.-Y., Tang, H.-Y., and Lin, C.-C. (2006). Ring-opening polymerization of lactides initiated by zinc alkoxides derived from NNO-tridentate ligands. Macromolecules 39, 3745–3752. doi: 10.1021/ma060471r
Chuang, H.-J., Weng, S.-F., Chang, C.-C., Lin, C.-C., and Chen, H.-Y. (2011). Synthesis, characterization and catalytic activity of magnesium and zinc aminophenoxide complexes: catalysts for ring-opening polymerization of L-lactide. Dalton Trans. 40, 9601–9607. doi: 10.1039/c1dt11080b
Ditchfield, R., Hehre, W. J., and Pople, J. A. (1971). Self-consistent molecular-orbital methods. IX. an extended gaussian-type basis for molecular-orbital studies of organic molecules. J. Chem. Phys. 54, 724–728. doi: 10.1063/1.1674902
Ebrahimi, T., Mamleeva, E., Yu, I., Hatzikiriakos, S. G., and Mehrkhodavandi, P. (2016). The role of nitrogen donors in zinc catalysts for lactide ring-opening polymerization. Inorg. Chem. 55, 9445–9453. doi: 10.1021/acs.inorgchem.6b01722
Falivene, L., Credendino, R., Poater, A., Petta, A., Serra, L., Oliva, R., et al. (2016). SambVca 2. a web tool for analyzing catalytic pockets with topographic steric maps. Organometallics 35, 2286–2293. doi: 10.1021/acs.organomet.6b00371
Fliedel, C., Mameri, S., Dagorne, S., and Avilés, T. (2014a). Controlled ring-opening polymerization of trimethylene carbonate and access to PTMC-PLA block copolymers mediated by well-defined N-heterocyclic carbene zinc alkoxides. Appl. Organometal. Chem. 28, 504–511. doi: 10.1002/aoc.3154
Fliedel, C., Vila-Viçosa, D., Calhorda, M. J., Dagorne, S., and Avilés, T. (2014b). Dinuclear zinc–N-heterocyclic carbene complexes for either the controlled ring-opening polymerization of lactide or the controlled degradation of polylactide under mild conditions. ChemCatChem 6, 1357–1367. doi: 10.1002/cctc.201301015
Fuoco, T., and Pappalardo, D. (2017). Aluminum alkyl complexes bearing salicylaldiminato ligands: versatile initiators in the ring-opening polymerization of cyclic esters. Catalysts 7, 64–80. doi: 10.3390/catal7020064
Gao, B., Duan, R., Pang, X., Li, X., Qu, Z., Shao, H., et al. (2013). Zinc complexes containing asymmetrical N,N,O-tridentate ligands and their application in lactide polymerization. Dalton Trans. 42, 16334–16342. doi: 10.1039/c3dt52016a
Guillaume, S. M., Kirillov, E., Sarazin, Y., and Carpentier, J.-F. (2015). Beyond stereoselectivity, switchable catalysis: some of the last frontier challenges in ring-opening polymerization of cyclic esters. Chem. Eur. J. 21, 7988–8003. doi: 10.1002/chem.201500613
Hay, P. J., and Wadt, W. R. (1985a). Ab initio effective core potentials for molecular calculations. Potentials for the transition metal atoms Sc to Hg. J. Chem. Phys. 82, 270–283. doi: 10.1063/1.448799
Hay, P. J., and Wadt, W. R. (1985b). Ab initio effective core potentials for molecular calculations. Potentials for K to Au including the outermost core orbitals. J. Chem. Phys. 82, 299–310. doi: 10.1063/1.448975
Hu, J., Kan, C., and Ma, H. (2018). Exploring steric effects of zinc complexes bearing achiral benzoxazolyl aminophenolate ligands in isoselective polymerization of rac-lactide. Inorg. Chem. 57, 11240–11251. doi: 10.1021/acs.inorgchem.8b01839
Huang, B.-H., Lin, C.-N., Hsueh, M.-L., Athar, T., and Lin, C.-C. (2006). Well-defined sterically hindered zinc aryloxides: excellent catalysts for ring-opening polymerization of ε-caprolactone and L-lactide. Polymer 47, 6622–6629. doi: 10.1016/j.polymer.2006.07.015
Huang, B.-H., Tsai, C.-Y., Chen, C.-T., and Ko, B.-T. (2016). Metal complexes containing nitrogen-heterocycle based aryloxide or arylamido derivatives as discrete catalysts for ring-opening polymerization of cyclic esters. Dalton Trans. 45, 17557–17580. doi: 10.1039/c6dt03384a
Khemtong, C., Kessinger, C. W., and Gao, J. (2009). Polymeric nanomedicine for cancer MR imaging and drug delivery. Chem. Commun. 24, 3497–3510. doi: 10.1039/B821865J
Ladewig, S. M., Bao, S., and Chow, A. T. (2015). Natural fibers: a missing link to chemical pollution dispersion in aquatic environments. Environ. Sci. Technol. 49, 12609–12610. doi: 10.1021/acs.est3b04754
Levis, J. W., and Barlaz, M. A. (2011). Is biodegradability a desirable attribute for discarded solid waste? Perspectives from a national landfill greenhouse gas inventory model. Environ. Sci. Technol. 45, 5470–5476. doi: 10.1021/es200721s
Lih, E., Oh, S. H., Joung, Y. K., Leed, J. H., and Han, D. K. (2015). Polymers for cell/tissue anti-adhesion. Prog. Polym. Sci. 44, 28–61. doi: 10.1016/j.progpolymsci.2014.10.004
Ma, W.-J., Yuan, X.-B., Kang, C.-S., Su, T., Yuan, X.-Y., Pu, P.-Y., et al. (2008). Evaluation of blood circulation of polysaccharide surface-decorated PLA nanoparticles. Carbohydr. Polym. 72, 75–81. doi: 10.1016/j.carbpol.2007.07.033
Patel, D., Moon, J. Y., Chang, Y., Kim, T. J., and Lee, G. H. (2008). Polymeric nano-micelles using poly(ethylene glycol) and poly(trimethylene carbonate) diblock copolymers as a drug carrier. Colloid Surf. A-Physicochem. Eng. Asp. 313–314, 91–94. doi: 10.1016/j.colsurfa.2007.04.078
Place, E. S., George, J. H., Williams, C. K., and Stevens, M. M. (2009). Synthetic polymer scaffolds for tissue engineering. Chem. Soc. Rev. 38, 1139–1151. doi: 10.1039/b811392k
Raquez, J.-M., Habibi, Y., Murariu, M., and Dubois, P. (2013). Polylactide (PLA)-based nanocomposites. Prog. Polym. Sci. 38, 1504–1542. doi: 10.1016/j.progpolymsci.2013.05.014
Redshaw, C. (2017). Use of metal catalysts bearing Schiff base macrocycles for the ring opening polymerization (ROP) of cyclic esters. Catalysts 7, 165–176. doi: 10.3390/catal7050165
Rochman, C. M., Hoh, E., Hentschel, B. T., and Kaye, S. (2013). Long-term field measurement of sorption of organic contaminants to five types of plastic pellets: implications for plastic marine debris. Environ. Sci. Technol. 47, 1646–1654. doi: 10.1021/es303700s
Romain, D. C., and Williams, C. K. (2014). Chemoselective polymerization control: from mixed-monomer feedstock to copolymers. Angew. Chem. Int. Ed. 53, 1607–1610. doi: 10.1002/anie.201309575
Romer, J. R. (2010). The Evolution of SF's plastic bag ban. Golden Gate Univ. Environ. Law J. 439, 439–465. Available online at: https://digitalcommons.law.ggu.edu/gguelj/vol1/iss2/5/
Romer, J. R., and Tamminen, L. M. (2014). Plastic bag reduction ordinances: New York city's proposed charge on all carryout bags as a model for U.S. cities. Tulane Environ. Law J. 27, 237–275. Available online at: https://static1.squarespace.com/static/59bd5150e45a7caf6bee56f8/t/59bd52ae7e2a5fb4e246dfda/1514156600769/plastic-bag-reduction-ordinances.pdf
Sarazin, Y., and Carpentier, J.-F. (2015). Discrete cationic complexes for ring-opening polymerization catalysis of cyclic esters and epoxides. Chem. Rev. 115, 3564–3614. doi: 10.1021/acs.chemrev.5b00033
Simpson, R. L., Wiria, F. E., Amis, A. A., Chua, C. K., Leong, K. F., Hansen, U. N., et al. (2007). Development of a 95/5 poly(L-lactide-co-glycolide)/hydroxylapatite and β-tricalcium phosphate scaffold as bone replacement material via selective laser sintering. J. Biomed. Mater. Res. B 17, 17–25. doi: 10.1002/jbm.b.30839
Sun, Y.-L., Chen, Y.-Z., Wu, R.-J., Chavali, M., Huang, Y.-C., Su, P.-G., et al. (2007). Poly(l-lactide) stabilized gold nanoparticles based QCM sensor for low humidity detection. Sens. Actuator B-Chem. 126, 441–446. doi: 10.1016/j.snb.2007.03.029
Thevenon, A., Romain, C., Bennington, M. S., White, A. J. P., Davidson, H. J., Brooker, S., et al. (2016). Dizinc lactide polymerization catalysts: Hyperactivity by control of ligand conformation and metallic cooperativity. Angew. Chem. Int. Ed. 55, 8680–8685. doi: 10.1002/anie.201602930
Vaiana, L., Regueiro-Figueroa, M., Mato-Iglesias, M., Platas-Iglesias, C., Esteban-Gómez, D., de Blas, A., et al. (2007). Seven-coordination versus six-coordination in divalent first-row transition-metal complexes derived from 1,10-diaza-15-crown-5. Inorg. Chem. 46, 8271–8282. doi: 10.1021/ic7008946
Wang, H., Yang, Y., and Ma, H. (2016). Exploring steric effects in diastereoselective synthesis of chiral aminophenolate zinc complexes and stereoselective ring-opening polymerization of rac-lactide. Inorg. Chem. 55, 7356–7372. doi: 10.1021/acs.inorgchem.6b00378
Williams, C. K., Breyfogle, L. E., Choi, S. K., Nam, W., Young, V. G., Hillmyer, M. A., et al. (2003). A highly active zinc catalyst for the controlled polymerization of lactide. J. Am. Chem. Soc. 125, 11350–11359. doi: 10.1021/ja0359512
Yang, L., Powell, D. R., and Houser, R. P. (2007). Structural variation in copper(I) complexes with pyridylmethylamide ligands: structural analysis with a new four-coordinate geometry index, τ4. Dalton Trans. 955–964. doi: 10.1039/B617136B
Yang, Y., Wang, H., and Ma, H. (2015). Stereoselective polymerization of rac-lactide catalyzed by zinc complexes with tetradentate aminophenolate ligands in different coordination patterns: kinetics and mechanism. Inorg. Chem. 54, 5839–5854. doi: 10.1021/acs.inorgchem.5b00558
Keywords: zinc, polymerization, catalyst, lactide, kinetic
Citation: Lu W-Y, Wu K-H, Chen H-Y and Lin C-C (2019) Synthesis and Characterization of N,N,O-Tridentate Aminophenolate Zinc Complexes and Their Catalysis in the Ring-Opening Polymerization of Lactides. Front. Chem. 7:189. doi: 10.3389/fchem.2019.00189
Received: 24 October 2018; Accepted: 12 March 2019;
Published: 05 April 2019.
Edited by:
Clemens Kilian Weiss, Fachhochschule Bingen, GermanyReviewed by:
Cesar Liberato Petzhold, Federal University of Rio Grande do Sul, BrazilJincai Wu, Lanzhou University, China
Mina Mazzeo, University of Salerno, Italy
Marinos Pitsikalis, National and Kapodistrian University of Athens, Greece
Copyright © 2019 Lu, Wu, Chen and Lin. This is an open-access article distributed under the terms of the Creative Commons Attribution License (CC BY). The use, distribution or reproduction in other forums is permitted, provided the original author(s) and the copyright owner(s) are credited and that the original publication in this journal is cited, in accordance with accepted academic practice. No use, distribution or reproduction is permitted which does not comply with these terms.
*Correspondence: Kuo-Hui Wu, d3VrdW9odWlAY2hlbS5zLnUtdG9reW8uYWMuanA=
Hsuan-Ying Chen, aGNoZW5Aa211LmVkdS50dw==
Chu-Chieh Lin, Y2NobGluQG1haWwubmNodS5lZHUudHc=
orcid.org/0000-0003-1424-3540