- 1Department of Chemistry, University of Cyprus, Nicosia, Cyprus
- 2Department of Chemistry, University of Florida, Gainesville, FL, United States
- 3Department of Chemistry, University of Patras, Patras, Greece
We report the synthesis, crystal structures and magnetic properties of the giant heterometallic [Mn36Ni4]2−/0 (compounds 1, 2)/[Mn32Co8] (compound 3) “loops-of-loops-and-supertetrahedra” molecular aggregates and of a [Mn2Ni6]2+ compound (cation of 4) that is structurally related with the cation co-crystallizing with the anion of 1. In particular, after the initial preparation and characterization of compound [Mn2Ni6(μ4-O)2(μ3-OH)3(μ3-Cl)3(O2CCH3)6(py)8]2+[Mn36Ni4(μ4-O)8(μ3-O)4(μ3-Cl)8Cl4(O2CCH3)26(pd)24(py)4]2− (1) we targeted the isolation of (i) both the cationic and the anionic aggregates of 1 in a discrete form and (ii) the Mn/Co analog of [Mn36Ni4]2− aggregate. Our synthetic efforts toward these directions afforded the discrete [Mn36Ni4] “loops-of-loops-and-supertetrahedra” aggregate [Mn36Ni4(μ4-O)8(μ3-O)4(μ3-Cl)8Cl2(O2CCH3)26(pd)24(py)4(H2O)2] (2), the heterometallic Mn/Co analog [Mn32Co8(μ4-O)8(μ3-O)4(μ3-Cl)8Cl2(μ2-OCH2CH3)2(O2CCH3)28(pd)22(py)6] (3) and the discrete [Mn2Ni6]2+ cation [Mn2Ni6(μ4-O)2(μ3-OH)4(μ3-Cl)2(O2CCH3)6(py)8](ClO4)(OH) (4). The structure of 1 consists of a mixed valence [Mn28IIIMn8IINi4II]2− molecular aggregate that contains two Mn8IIINi2II loops separated by two Mn6IIIMn4II supertetrahedral units and a [Mn2IIINi6II]2+ cation based on two [MnIIINi3II(μ4-O)(μ3-OH)1.5(μ3-Cl)1.5]4+ cubane sub-units connected through both mono- and tri-atomic bridges provided by the μ4-O2− and carboxylate anions. The structures of 2–4 are related to those of the compounds co-crystallized in 1 exhibiting however some differences that shall be discussed in detail in the manuscript. Magnetism studies revealed the presence of dominant ferromagnetic interactions in 1–3 that lead to large ground state spin (ST) values for the “loops-of-loops-and-supertetrahedra” aggregates and antiferromagnetic exchange interactions in 4 that lead to a low (and possibly zero) ST value. In particular, dc and ac magnetic susceptibility studies revealed that the discrete [Mn36Ni4] aggregate exhibits a large ST value ~ 26 but is not a new SMM. The ac magnetic susceptibility studies of the [Mn32Co8] analog revealed an extremely weak beginning of an out-of-phase tail indicating the presence of a very small relaxation barrier assignable to the anisotropic Co2+ions and a resulting out-of-phase ac signal whose peak is at very low T.
Introduction
High nuclearity Mn carboxylate clusters continue to attract significant attention mainly because of their structural characteristics and physical properties (Bagai and Christou, 2009; Kostakis et al., 2010; Escuer et al., 2014). In particular, such compounds often exhibit interesting magnetic properties including high spin ground state values (Ako et al., 2006; Moushi et al., 2009) and single—molecule magnetism behavior (Sessoli et al., 1993; Bagai and Christou, 2009; Inglis et al., 2012; Milios and Winpenny, 2015). The latter appears in molecules exhibiting a large spin ground state (ST) value and significant easy axis magnetoanisotropy (Christou et al., 2000; Nakano and Oshio, 2011; Ferrando-Soria et al., 2017). In addition, Mn complexes have attracted significant attention since they are involved in the search for structural and functional analogs of the tetranuclear Mn complex that is present in the active site of photosystem II and is responsible for the photosynthetic oxidation of H2O to molecular O2 (Mukherjee et al., 2012; Yano and Yachandra, 2014; Gerey et al., 2016). Thus, Mn clusters have been proposed for various applications in diverse areas including magnetic refrigeration (Zheng et al., 2014), molecular spintronics (Bogani, 2015), quantum computation (Aromí et al., 2012), and catalysis (Maayan et al., 2018).
This interest has resulted in the development of several synthetic methods to Mn carboxylate clusters and the isolation of numerous high nuclearity complexes possessing a wide variety of shapes (wheels, disks, icosahedra, cuboctahedra, spheres, rods, etc.) and nuclearities (Kostakis et al., 2010). Some of these compounds exhibit very high nuclearities and dimensions with the list of giant, nanosized Mn clusters containing [Mn29] (Alexandropoulos et al., 2016), [Mn30] (Soler et al., 2004), [Mn31] (Abbasi et al., 2017), [Mn32] (Scott et al., 2005; Langley et al., 2011; Manoli et al., 2011), [Mn40Na4] (Moushi et al., 2007, 2010a), [Mn44] (Moushi et al., 2010a), [Mn49] (Manoli et al., 2016), [Mn70] (Vinslava et al., 2016), and [Mn84] (Tasiopoulos et al., 2004) aggregates. Although there are several homometallic nanosized Mn clusters reported, a [Mn28Cu17] aggregate is the only example of giant heterometallic Mn/M (M = any paramagnetic metal ion) compounds (Wang et al., 2007). Apart from their exciting crystal structures some of these nanosized aggregates exhibit interesting magnetic properties. For example, compounds [Mn30], [Mn31], [Mn32], [Mn70], and [Mn84] possess SMM behavior with an appreciable energy barrier to magnetization reorientation and represent a meeting of the bottom-up and top-down approaches to nanomagnetism (Papatriantafyllopoulou et al., 2016). In addition, compounds [Mn49] and [Mn28Cu17] display dominant ferromagnetic exchange interactions leading to giant ST values S = 61/2 and 51/2, respectively (Wang et al., 2007; Manoli et al., 2016). Note that the record ST values have appeared in other giant homometallic Fe42 (Kang et al., 2015) and heterometallic [Ni21Gd20] (Chen et al., 2018) clusters and are ST = 45 and 91, respectively. Interestingly although Mn cluster chemistry has proven to be the most fruitful source of giant metal clusters among other 3d metal ions there is only one heterometallic Mn/M (M = any metal ion) reported in contrast to the situation with other metal ions. For example, in Ni2+ or Cu2+ chemistry there are only a few giant homometallic clusters but there are several hetemetallic ones and especially Ni2+/4f and Cu2+/4f aggregates (Papatriantafyllopoulou et al., 2016).
Our group has been exploring reactions of diols with Mn—containing precursor compounds targeting to new high nuclearity Mn clusters and SMMs (Tasiopoulos and Perlepes, 2008). These investigations have afforded a series of giant Mn carboxylate clusters including [Mn25Na4] and [Mn49] aggregates consisting of eight and four decametallic supertetrahedral repeating sub-units (Manoli et al., 2016). Note that discrete metal clusters exhibiting a [(μ4-O)4]18+ supertetrahedral core analogous to that appeared in [Mn25Na4] and [Mn49] aggregates have been stabilized in several cases, especially with polyol-type ligands and in most cases these compounds exhibited entirely ferromagnetic exchange interactions and ST = 22 (Stamatatos et al., 2006; Manoli et al., 2007, 2008; Wu et al., 2011). The use of 1, 3-propanediol (pdH2) and its derivatives in Mn carboxylate chemistry afforded a family of [Mn44] and [Mn40Na4] loops consisting of four [Mn10M(μ3- O)2(O2CCH3)13(pd)6(py)2] ([Mn40M4]; M = Na+, x = 0; M = Mn2+, x = 1) loops linked through Na+ or Mn2+ ions (called “loops-of-loops”) and have a saddle-like topology. The [Mn44] analog of this family displays a spin ST = 6 ground state and SMM behavior (Moushi et al., 2007, 2010a).
Further investigation of the reactions that afforded the [Mn40M4] loops-of-loops aggregates involved the use of various 3d paramagnetic metal ions in an attempt to isolate a series of heterometallic Mn/3d analogs and/or other large aggregates composed of smaller clusters. These investigations afforded compounds [Mn2Ni6(μ4-O)2(μ3-OH)3(μ3-Cl)3(O2CCH3)6(py)8]2+[Mn36Ni4(μ4-O)8(μ3-O)4(μ3-Cl)8Cl4(O2CCH3)26(pd)24(py)4]2− (1) [Mn36Ni4(μ4-O)8(μ3-O)4(μ3-Cl)8Cl2(O2CCH3)26(pd)24(py)4(H2O)2] (2) and [Mn32Co8(μ4-O)8(μ3-O)4(μ3-Cl)8Cl2(μ2-OCH2CH3)2(O2CCH3)28(pd)22(py)6] (3). The last compound discussed herein is the discrete [Mn2Ni6]2+ cation [Mn2Ni6(μ4-O)2(μ3-OH)4(μ3-Cl)2(O2CCH3)6(py)8](ClO4)(OH) (4), i.e., an analog of the complex co-crystallizing with the [Mn36Ni4]2− anion of 1. Compounds 1–3 are rare examples of giant heterometallic Mn/M clusters and possess an unprecedented “loop-of-loops-and-supertetrahedra” structural topology. Complexes 2 and 3 exhibit dominant ferromagnetic exchange interactions and large ST values which in the case of 2 is 26 ± 1. The [Mn36Ni4] aggregates (compounds 1 and 2) do not display SMM behavior as is also the case for their [(μ4-O)4]18+ supertetrahedral sub-unit, whereas for their [Mn32Co8] analog (complex 3) the existence of an out-of-phase tail is an indication of SMM behavior, however, further studies are required to confirm this conclusion. Part of this work, involving the synthesis and characterization of compound 2 has been communicated previously (Charalambous et al., 2012).
Materials and Methods
Materials and Physical Measurements
All manipulations were performed under aerobic conditions using chemicals and solvents as received, unless otherwise stated. [Mn3O(O2CCH3)6(py)3]·py was prepared as previously described (Vincent et al., 1987).
IR spectra were recorded in the solid state (KBr pellets) in the 4,000–400 cm−1 range using a Shimadzu Prestige−21 spectrometer. Elemental analysis (C, H, and N) were performed by the in-house facilities of the Chemistry Department at the University of Florida.
Variable-temperature dc magnetic susceptibility data down to 1.80 K were collected on a Quantum Design MPMS-XL SQUID magnetometer equipped with a 70 kG (7 T) dc magnet at the University of Florida. Diamagnetic corrections were applied to the observed paramagnetic susceptibilities using Pascal's constants. Samples were embedded in solid eicosane to prevent torquing. AC magnetic susceptibility data were collected on the same instrument employing a 3.5 G AC field oscillating at frequencies up to 1,500 Hz. Magnetization vs. field and temperature data were fit using the program MAGNET (Davidson, E. R.)1.
Experimental
[Mn2Ni6(μ4-O)2(μ3-OH)3(μ3-Cl)3(O2CCH3)6(py)8]2+ [Mn36 Ni4(μ4-O)8(μ3-O)4(μ3-Cl)8Cl4(O2CCH3)26 (pd)24(py)4]2−·6H2O, (1)·6H2O
To a stirred brown solution of [Mn3O(O2CCH3)6(py)3]·py (0.23 g, 0.27 mmol) in 15 ml CH3CN were added pdH2 (100 μL, 0.105 g, 1.38 mmol) and solid NiCl2·6H2O (0.066 g, 0.27 mmol). The reaction mixture was left under magnetic stirring for 10 min, filtered off and the filtrate was left undisturbed at room temperature. After 1 week brown X-ray quality crystals of 1 suitable for X-ray structural determination were formed. The crystals were isolated by filtration, washed with CH3CN and dried in vacuo; the yield was ~36%. The crystals for X-ray studies were maintained in contact with mother liquor to prevent solvent loss. % C H N Anal. for C196H315N12O135Cl15Mn38Ni10 [(1)·6H2O]: calcd: C 28.69, H 3.87, N 2.05; found: C 29.02, H 3.75, N 2.35. Metal analysis was performed via ICP-OES. Anal. Calc. for (1)·6H2O: Mn 25.44, Ni 7.15; found: Mn 25.64, Ni 7.38%. Selected IR data (cm−1, KBr pellet): 3,420 (s, br), 2,931 (m), 2,854 (m), 1,560 (s, br), 1,419 (s, br), 1,341 (w), 1,087 (s), 951 (m), 835 (w), 637 (s, br).
[Mn36Ni4(μ4-O)8(μ3-O)4(μ3-Cl)8Cl2(O2CCH3)26(pd)24 (py)4(H2O)2]·10H2O, (2)·10H2O
To a brown solution of [Mn3O(O2CCH3)6(py)3]·py (0.23 g, 0.27 mmol) in 15 mL CH3CN were added under magnetic stirring pdH2 (200 μL, 0.211 g, 2.77 mmol) and NiCl2·6H2O (0.066 g, 0.27 mmol). The reaction mixture was left under magnetic stirring at room temperature for ~1 h and then undisturbed for ~3 h. The resulting dark brown slurry was filtered off and the filtrate was left undisturbed at room temperature. After a few days X-ray quality crystals of 2·2CH3CN·12.30H2O were formed, isolated by filtration, washed with CH3CN and dried under vacuum. The crystals for X-ray studies were maintained in contact with mother liquor to prevent solvent loss. The yield was 35% based on total Mn content. % C H N Anal. for C144H266N4O124Cl10Mn36Ni4 [(2)·10H2O]: calcd: C 26.19, H 4.06, N 0.85; found: C 26.32, H 4.09, N 0.94. Metal analysis was performed via ICP-OES. Anal. Calc. for (2)·10H2O: Mn 29.94, Ni 3.55; found: Mn 30.09, Ni 3.68%. Selected IR data (cm−1, KBr pellet): 3,426 (s, br), 2,934 (m), 2,849 (m), 1,593 (s, br), 1,553 (w), 1,404 (m), 1,085 (s), 945 (w), 629 (s, br).
[Mn32Co8(μ4-O)8(μ3-O)4(μ3-Cl)8Cl2(μ2-OCH2CH3)2 (O2CCH3)28(pd)22(py)6]·20H2O, (3)·20H2O
To a stirred brown solution of [Mn3O(O2CCH3)6(py)3]·py (0.23 g, 0.27 mmol) in 12 ml of EtOH were added pdH2 (300 μL, 0.316 g, 4.15 mmol) and solid CoCl2·6H2O (0.066 g, 0.27 mmol) and the reaction mixture was left under magnetic stirring for 2 h. The resulting red-brown slurry was filtered off and the filtrate was layered with Et2O (1:3 v/v). After 2 weeks brown crystals of (3)·3.84 EtOH·6H2O were formed suitable for X-ray structural determination. The crystals were isolated by filtration, washed with EtOH and dried in vacuo; the yield was 29%. The crystals for X-ray studies were maintained in contact with mother liquor to prevent solvent loss. % C H N Anal. for C156H296N6O134Cl10Mn32Co8 [(3)·20H2O]: calcd: C 26.83, H 4.27, N 1.20; found: C 26.55, H 4.01, N 0.97. Metal analysis was performed via ICP-OES. Anal. Calc. for (3)·20H2O: Mn 25.17, Co 6.75; found: Mn 25.38, Co 6.88%. Selected IR data (cm−1, KBr pellet): 3,414 (s, br), 3,110 (s, br), 2,843 (w), 2,770 (w), 1,610 cm−1 (m), 1,556 (w), 1396 (s), 1,078 (m), 621(m).
[Mn2Ni6(μ4-O)2(μ3-OH)4(μ3-Cl)2(O2CCH3)6(py)8] (ClO4)(OH)·2H2O, (4)·2H2O
To a stirred brown solution of [Mn3O(O2CCH3)6(py)3]·py (0.20 g, 0.24 mmol) in 15 mL EtOH were added solids NiCl2·6H2O (0.06 g, 0.24 mmol) and NaClO4(0.03 g, 0.24 mmol) and the reaction mixture was left under magnetic stirring at room temperature for 2 h. The resulting brown slurry was filtered off and the dark brown filtrate was layered with Et2O (1:3 v/v) and left undisturbed at room temperature for a period of 1 week, upon which yellow-brown crystals of 4 suitable for X-ray structural determination were formed. The crystals were isolated by filtration, washed with EtOH and dried in vacuo; the yield was ~21%. C H N Anal. for C52H67N8O25Cl3Mn2Ni6 [(4)·2H2O]: calcd: C 35.24, H 3.81, N 6.32; found: C 35.54, H 3.55, N 6.74. Metal analysis was performed via ICP-OES. Anal. Calc. for (4)·2H2O: Mn 6.20, Ni 19.87; found: Mn 6.52, Ni 20.28 %. Selected IR data (cm−1, KBr pellet): 3,549 (w, br), 3,078 (w, br), 1,584 (s), 1,443 (s), 1,413 (s), 1,223 (m), 1,105 (s), 698 (s), 627 (m).
Single Crystal X-Ray Crystallography
Single crystal X-ray diffraction data for (1), (2)·2CH3CN·12.30H2O, (3)·3.84EtOH·6H2O, and (4) were collected on an Oxford-Diffraction Supernova diffractometer, equipped with a CCD detector utilizing Mo Ka (λ = 0.71073 Å) radiation. A suitable crystal was mounted on a Hampton cryoloop with Paratone-N oil and transferred to a goniostat where it was cooled for data collection. Empirical absorption corrections (multiscan based on symmetry-related measurements) were applied using CrysAlis RED software (Oxford Diffraction, 2008). The structures were solved by direct methods using SIR2004 (Burla et al., 2005) and refined on F2 using full-matrix least-squares with SHELXL-2014/7 (Sheldrick, 2014) Software packages used were as follows: CrysAlis CCD for data collection (Oxford Diffraction 2008). CrysAlis RED for cell refinement and data reduction (Oxford Diffraction 2008). WINGX for geometric calculations (Farrugia, 1999), and DIAMOND (Brandenburg, 2006) for molecular graphics. The non-H atoms were treated anisotropically, whereas the aromatic H atoms were placed in calculated, ideal positions and refined as riding on their respective carbon atoms. Electron density contributions from disordered guest molecules were handled using the SQUEEZE procedure from the PLATON software suit (Van der Sluis and Spek, 1990; Spek, 2003). Selected crystal data for (1), (2)·2CH3CN·12.30H2O, (3)·3.84 EtOH·6H2O, and (4) are summarized in Table S1, whereas selected bond lengths and angles are given in Tables S2–S5.
CCDC 1859793, CCDC 862029, CCDC 1859811, and CCDC 1859814 contain the supplementary crystallographic data for (1), (2)·2CH3CN·12.30H2O, (3)·3.84 EtOH·6H2O, and (4), respectively. These data can be obtained free of charge from The Cambridge Crystallographic Data Centre via www.ccdc.cam.ac.uk/data_request/cif.
Results
Syntheses
We have been systematically studying reactions of Mn salts and preformed clusters with diols as a route to new polynuclear clusters with novel structural characteristics and interesting magnetism (Tasiopoulos and Perlepes, 2008; Moushi et al., 2009, 2010b; Skordi et al., 2018). These studies have focused on the use of simple aliphatic diols such as pdH2 and its derivatives which due to their alkoxide arms exhibit a high bridging capability and a fruitful coordination chemistry. We recently reported a family of large molecular aggregates consisting of four smaller clusters linked through Na+ or Mn2+ ions (Moushi et al., 2010a). These large tetrameric {[Mn10M(μ3- O)2(O2CCH3)13(pd)6(py)2]4}x+ ([Mn40M4]; M = Na+, x = 0; M = Mn2+, x = 1), clusters contain four Mn10 loops linked through Na+ or Mn2+ ions and have a saddle-like topology. They were prepared from reactions of [Mn3O(O2CMe)6(py)3]·py (py = pyridine) with H2pd in the presence of NaN3 [Mn40Na4] or Mn(ClO4)2·6H2O [Mn44]. We were interested to extend this study by preparing analogous heterometallic MnxMy (M = a 3d metal ion) complexes. These studies involved the investigation of similar reactions to those led to the [Mn44] or [Mn40Na4] aggregates which however, in the place of Mn(ClO4)2·6H2O or NaN3 contained a 3d metal ion salt. The initial result of these studies was compound [Mn2Ni6(μ4-O)2(μ3-OH)3(μ3-Cl)3(O2CCH3)6(py)8]2+[Mn36Ni4(μ4-O)8(μ3-O)4(μ3-Cl)8Cl4(O2CCH3)26(pd)24(py)4]2− (1) that was obtained in ~36% yield from the reaction of [Mn3O(O2CCH3)6(py)3]·py with 1,3-propanediol (pdH2) in the presence of NiCl2·6H2O in 1:5:1 molar ratio in CH3CN. The formation of compound 1 is summarized in Equation (1):
Since compound 1 crystallized as a mixture of a cationic and an anionic complexes, we targeted the isolation of both of its components, and especially of [Mn36Ni4] aggregate, in a discrete form. From the molecular formula of 1 it was observed that in the [Mn2Ni6]2+ cation of 1 there are no pd2− ligands in contrast to the [Mn36Ni4]2− anion of 1 which contains 24 pd2− groups. We thus decided to increase the ratio of pd2− in the reaction mixture in order to facilitate the formation of the complex that contains pd2− groups and this modification resulted in the isolation of the discrete [Mn36Ni4] analog. In particular, the reaction of [Mn3O(O2CCH3)6(py)3]·py with pdH2 and NiCl2·6H2O in a 1:10:1 molar ratio in CH3CN afforded the discrete [Mn36Ni4] complex [Mn36Ni4O12Cl10(O2CCH3)26(pd)24(py)4(H2O)2]·10H2O in 35% yield. The formation of compound 2 is summarized in Equation (2):
After the isolation of compound 2 was realized, our efforts were focused on the synthesis of other Mn40−xMx (M = a 3d metal ion) heterometallic “loops-of-loops-and-supertetrahedra” molecular aggregates and also of the [Mn2Ni6] cation of 1 in a discrete form. The preparation of a Mn40−xCox analog was our initial target since the incorporation in this structure of the highly anisotropic Co2+ ions could result in the appearance of a different magnetic behavior in the resulting compound than those shown in 1 and 2. The formation of the Mn/Co species was achieved from the reaction of [Mn3O(O2CCH3)6(py)3]·py with pdH2 and CoCl2·6H2O in a 1:15:1 molar ratio in C2H5OH in ~29% yield. The formation of compound 3 is summarized in Equation (3):
The discrete [Mn2Ni6] cluster was also isolated by following a similar synthetic procedure to the one that led to the discrete [Mn36Ni4] aggregate which however, did not involve the use of pdH2 which is not present in this compound. Thus, the reaction of [Mn3O(O2CCH3)6(py)3]·py with NiCl2·6H2O in the presence of NaClO4 in a 1:1:1 molar ratio in C2H5OH afforded complex 4 in ~21% yield. The formation of compound 4 is summarized in Equation (4):
Description of the Structures
Complexes (1) and (3)·3.84 EtOH·6H2O crystallize in the triclinic space group P and (2)·2CH3CN·12.30H2O and (4) in the monoclinic I 2/a one. The [Mn36Ni4]2−, [Mn36Ni4], and [Mn32Co8] aggregates of 1, 2, and 3, respectively exhibit related structures. Similarly, the molecular structures of the [Mn2Ni6]2+ cation of 1 and 4 are also related. Thus, the structures of the [Mn36Ni4]2− anion and the [Mn2Ni6]2+ cation will be discussed in detail and compared to those of their related analogs 2–4. The molecular structures of the cation and the anion of 1, and the [Mn32Co8] aggregate and the sub-units of 3 are shown in Figures 1, 2, respectively. Structural figures and tables for 1–4 (bond lengths and angles, Mn/Ni/Co BVS calculations are reported in the Supplementary Material).
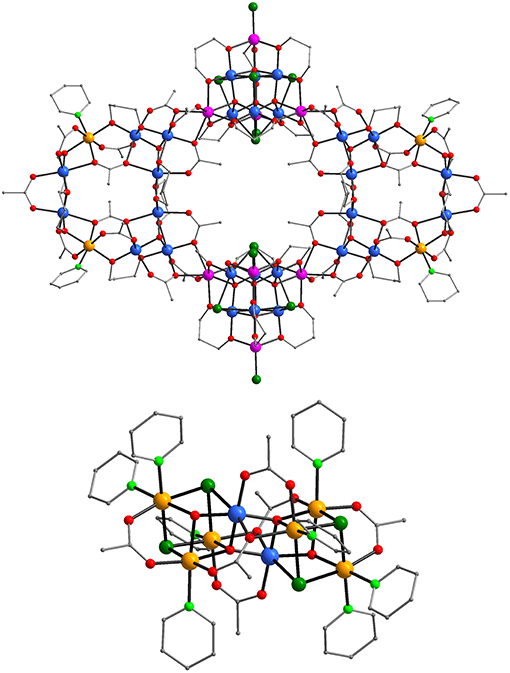
Figure 1. Representations of the molecular structures of the [Mn36Ni4]2− anion (top) and the [Mn2Ni6]2+ cation (bottom) of 1 Color code: MnII purple, MnIII blue, NiII orange, O red, N light green, Cl green, C gray. H atoms are omitted for clarity.
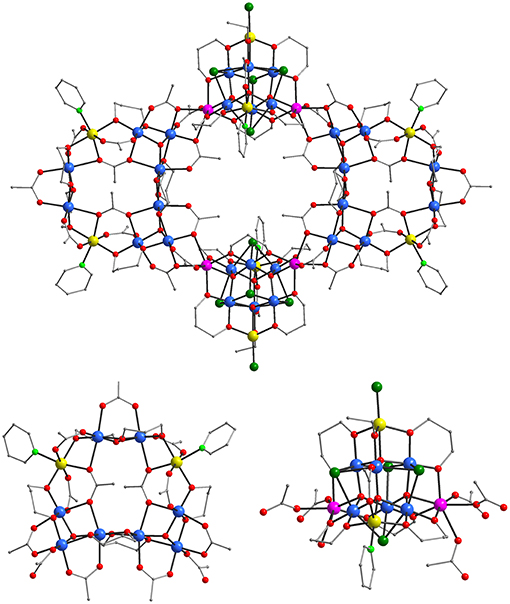
Figure 2. Representations of the molecular structure of 3 (top) and its [Co2] loop (bottom, left) and [] supertetrahedral (bottom, right) sub-units. Color code: MnII purple, MnIII blue, CoII yellow, O red; N light green, Cl green, C gray. H atoms are omitted for clarity.
The molecular structure of 1 consists of a [Mn36Ni4]2− anionic aggregate (Figure 1, top) the charge of which is balanced from a cationic [Mn2Ni6]2+ cluster (Figure 1, bottom). Note that the oxidation states of the Mn/Ni ions and the protonation levels of the ligands were determined by bond valence sum calculations (Brown and Altermatt, 1985; Liu and Thorp, 1993), charge considerations and inspection of metric parameters. The giant [Mn36Ni4]2− aggregate contains two [Ni2(μ3-O)2(O2CCH3)12(pd)6(py)2] loops (Figure S1, top) and two [(μ4-O)4(μ3-Cl)4(O2CCH3)Cl2(pd)6(H2O)] supertetrahedral sub-units (Figure S1, bottom) which are related to compounds either appeared as fragments in high nuclearity clusters or in a discrete form. In particular the [Ni2] loop exhibits analogous structure to the [] sub-unit of the tetrameric [Mn44] or [Mn40Na4] “loops-of-loops” aggregates (vide supra) with their main difference being the presence of two Ni2+ ions in the place of two Mn2+ ions (Moushi et al., 2010a). In addition, the [(μ4-O)4)]18+ supertetrahedral core that is present in 1 has appeared in several compounds either in discrete form (Stamatatos et al., 2006; Manoli et al., 2007, 2008; Wu et al., 2011) or as fragment of higher nuclearity clusters (Manoli et al., 2016). The MnIII and NiII ions are hexacoordinated exhibiting a distorted octahedral coordination geometry whereas the MnII ions adopt various coordination numbers and geometries. As expected, the MnIII ions display the expected Jahn-Teller (JT) elongations although the JT axes are not co-parallel.
Thus, each [Ni2] unit consists of two [(μ3-O)]7+ triangles and two dinuclear MnIIINiII moieties linked by pd2− RO− groups, and bridging CH3 ligands. The Mn and Ni ions are held together through 12 acetate and six pd2− bridging ligands. The acetate groups adopt syn, syn- η1:η1:μ (six CH3 ligands), η1:η2:μ3 (four CH3 ligands) and η2:η2:μ4 (two CH3 ligands) bridging modes whereas the pd2− ligands link metal ions in a η2:η2:μ3 fashion. The peripheral ligation of the [Ni2] loop is completed by two terminal py molecules. The [Ni2] loops are connected to the [] supertetrahedral sub-units through two μ3- and one μ- CH3 ligands bridging the Mn ions of each [O]7+ triangle to a MnII ion of a supertetrahedral sub-unit (Figure S3) constructing the nearly—planar [Ni4] “loop-of-loops-and-supertetrahedra” aggregate. The MnII ions of the [] sub-unit occupy the apex positions of a tetrahedron and the MnIII ions are located on its edges. The metal ions are connected by four μ4-O2− ions forming the [(μ4-O)4]18+ core which consists of four [MnII(μ4-O)]9+ vertex-sharing tetrahedra. The MnIII ions are bridged through four μ3- Cl− ions which occupy their JT axes. The two Mn2+ and one Mn3+ ions located in each edge of the tetrahedron are connected through six pd2− ligands bridging in a η2:η2:μ3 mode. The peripheral ligation of the [(μ4-O)4(μ3-Cl)4(pd)6(O2CCH3)Cl2] subunit (Figure S1) is completed by two terminal Cl− anions.
The molecular structure of the [] cation consists of two mixed metal [MnIII(μ4-O)(μ3-OH)1.5(μ3-Cl)1.5(O2CMe)3(py)4]+ cubanes linked through oxide and carboxylate ligands. In particular, the three NiII and one MnIII ions are connected through one μ4-O2−, one μ3-OH−, one μ3-Cl− and a mixed 0.5 Cl−/0.5 OH− site. The μ3-OH− and μ3-Cl− bridge two 2 NiII and one MnIII ions, the mixed 0.5 Cl−/0.5 OH− connects 3 NiII ions of the cubane whereas the μ4-O2− links 2 NiII and one MnIII ions of the cubane thus forming the [MnIII(μ4-O)(μ3-OH)1.5(μ3-Cl)1.5]4+ cubane core and one additional MnIII ion of the second cubane of the cation of 1. The Mn/Ni ions of each cubane are also bridged by three carboxylate ligands two of which bridge in the common syn, syn- η1:η1:μ fashion and the third one in a η1:η2:μ3 one. Their peripheral ligation is completed by four terminal pyridine molecules linked to the NiII ions. The two cubanes of the cation of 1 are linked apart from the μ4-O2− ions from two syn, syn- η1:η1:μ and two η1:η2:μ3 carboxylate ligands constructing the [Mn2Ni6(μ4-O)2(μ3-OH)3(μ3-Cl)3(O2CCH3)6(py)8]2+ cation of 1.
The molecular structure of the [Mn36Ni4] cluster of 2·2CH3CN·12.30H2O exhibits a striking similarity to the anion of 1. The main difference between the two complexes is their overall charge since compound 1 is anionic with a 2- charge and 2 is neutral. This difference in the charges appears because a terminal Cl− ligand of 1 linked to a MnII ion of the supertetrahedral sub-units has been replaced in 2 by a terminal H2O molecule. As a result, compound 2 contains two less Cl− anions and is neutral and thus, the [Mn36Ni4] aggregate is the only metal cluster appearing in the crystal structure.
The molecular structure of (3)·3.84 EtOH·6H2O (Figure 2, top) is also related to the anion of 1 and to 2·2CH3CN·12.30H2O with the main difference obviously being the presence in 3 of 32 Mn/8 Co ions instead of 36 Mn/4 Ni ions that appear in the anion of 1 and in 2. These eight CoII ions are located in the decametallic [] loops (Figure 2, bottom left) in the same positions that the NiII ions of the [Mn36Ni4] aggregates are found and also in the [] supertetrahedral sub-units (Figure 2, bottom right). In the latter the CoII ions occupy two apex positions in which MnII ions are located in the known [] supertetrahedra including the sub-units of the anion of 1 and in 2. In fact, this is a major difference between the [Mn36Ni4]0/2− and [Mn32Co8] since the former ones consist of one heterometallic [] and one homometallic [] sub-units, whereas the latter is based on two different types of heterometallic sub-units. Apart from these major differences in the structures of the [Mn36Ni4]0/2− and [Mn32Co8] compounds there are also some minor ones. These include the presence in the structure of [Mn32Co8] aggregate of two less pd2− ligands and two additional CH3 and C2H5O− groups compared to the structures of the [Mn36Ni4]0/2− complexes. This is because one pd2− group bridging 2 MnII/1MnIII ions of one edge of each supertetrahedron in [Mn36Ni4]0/2− complexes has been replaced by a syn, syn- η1:η1:μ- CH3 and a μ-EtO ligands in [Mn32Co8] aggregate. In addition, [Mn32Co8] aggregate contains a terminal py ligand bound to a CoII ion located in the [] supertetrahedra in the place of a Cl− ligand or a H2O molecule in the anion of 1 or 2, respectively.
The molecular structure of compound 4 is related to that of the cation of 1. In fact, there are only very minor differences between the two compounds. These include the replacement in 4 of the mixed 0.5 Cl−/0.5 OH− site that bridges 3 NiII ions of each cubane by a μ3-OH− anion. In addition, in complex 4 the positive charge of the []2+ cation is balanced by a Cl and a OH− lattice anions instead of the [Mn36Ni4]2− anionic aggregate.
Magnetic Properties
Solid-state dc magnetic susceptibility measurements were performed on polycrystalline samples of complexes 1·6 H2O, 2·10 H2O, 3·20 H2O, and 4·2 H2O under a magnetic field of 0.1 T in the temperature range 5-300 K. The obtained data are shown as χMT vs. T plot in Figure 3. For complexes 1·6 H2O, 2·10 H2O, and 3·20 H2O the χMT value increases continuously from 172.1, 118.6, and 130.5 cm3 mol−1 K at 300 K to a maximum value of 492.0 (at 20 K), 325.6 (at 15 K), and 273.7 cm3 mol−1 K (at 25 K) and then decreases at low T to 465.1, 304.3, and 226.1 cm3 mol−1 K at 5 K, respectively. For 4·2 H2O, the χMT value at 300 K is 18.03 cm3 mol−1 K and decreases continuously with decreasing temperature reaching a value of ~15.03 cm3 mol−1 K at 100 K and then rapidly to 2.94 cm3 mol−1 K at 5.0K (Figure 3 and Figure S6). The increase of the χMT values with decreasing T in 1·6 H2O—3·20 H2O indicates the presence of dominant ferromagnetic exchange interactions. The maximum χMT values of 2·10 H2O and 3·20 H2O suggest ST values of ~ 26 ± 1 and 22 ± 1, respectively. However, we note that in the case of 3·20 H2O it may not be safe to exclude any conclusions for the spin ground state using the spin-only formula due to the presence of CoII ions which is well known that exhibit strong spin-orbit coupling. The decrease in the χMT value at the lowest temperatures is attributed to zero-field splitting (ZFS), Zeeman effects from the applied field, and/or any weak intermolecular antiferromagnetic exchange interactions. In the case of 4·2 H2O, the continuous decrease of χMT with decreasing temperature, the small χMT value at 5 K and the fact that the curve heads to 0 at 0 K suggests the presence of antiferromagnetic exchange interactions possibly leading to a diamagnetic ground state. This may be rationalized assuming that the two [MnIII] are antiferromagnetically coupled leading to a diamagnetic ground state.
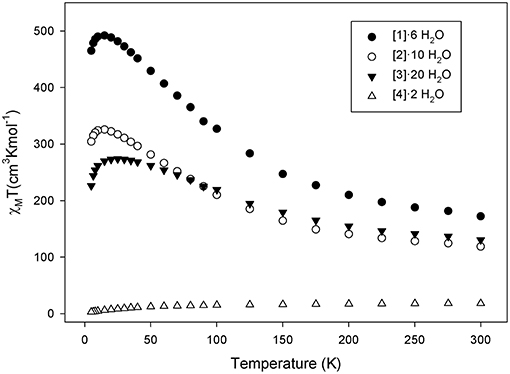
Figure 3. χMT vs. T plots for complexes (1)·6 H2O (•), (2)·10 H2O (◦), (3)·20 H2O (▾), and (4)·2 H2O (Δ) in the temperature range 5–300 K in a 0.1 T applied dc field.
Given the size and the complexity of the structures of 1–3, it is not possible to apply the Kambe method to determine the individual pairwise Mn-Mn, Mn-Ni, and Mn-Co exchange interaction parameters. In addition, the existence of two complexes co-crystallizing together in the structure of 1·6 H2O does not allow to obtain information for the D and ST values of these complexes. Furthermore, in 3·20 H2O, the presence of CoII ions exhibiting strong spin-orbit coupling and of many low lying excited states appearing due to the complexity of the giant [Mn32Co8] aggregate did not allow to obtain reliable S and D values from reduced magnetization fitting.
In the case of compound 2·10 H2O magnetization vs. dc field measurements at applied magnetic fields and temperatures in the 1–10 kG and 1.8–4.0 K ranges, respectively were performed. Low field data (≤ 1.0 T) were used, as we have previously done for many Mn clusters containing MnII atoms, to avoid problems from low-lying excited states. The data for complex 2·10 H2O are shown in Figure S7 as reduced magnetization (M/NμB) vs. H/T plot, where M is the magnetization, N is Avogadro's number, μB is the Bohr magneton, and H is the magnetic field.
The M/NμB vs. H/T data were fit by assuming that only the ground state is populated and by including axial zero-field splitting (D) and isotropic Zeeman interactions. The corresponding spin Hamiltonian is given by Equation (5),
where D is the axial ZFS parameter, Ŝz is the easy-axis spin operator, μ0 is the vacuum permeability, and H is the applied field. Equal quality fits, shown as the solid lines in Figure S7, were obtained for S = 25, 26, and 27 with parameters g = 2.03(1)/D = −0.007(1) cm−1, g = 1.96(1)/D = −0.004(1) cm−1, and g = 1.91(1)/D = −0.004(1) cm−1, respectively. Based on the obtained fits, we conclude that 2·10 H2O has a ground state of ST = 26 ± 1, and a very small D value.
Alternating current (ac) magnetic susceptibility data were collected for compounds 1–3 to obtain additional information about their ST values and the possibility to exhibit slow relaxation of the magnetization phenomena indicative of SMM behavior. The temperature dependence of the in-phase (), shown as T and out-of-phase () ac signals for 1–3 is shown in Figures S8–S10). These studies revealed that there are not any out-of-phase ac signals in 1 and 2 suggesting that these compounds are not new SMMs. In the case of 3, there is a barely visible beginning in the 1.8 K data of a frequency dependence in the in-phase plot and a concomitant very weak tail of an out-of-phase signal (/ ~ 1.5% at 1.8 K) representing the beginning of a signal whose peak is clearly far below the 1.8 K limit of our SQUID instrument, i.e., the anisotropy barrier is extremely small. In addition, ac data are in line with the conclusions obtained from dc studies concerning the ST values of 2 and 3. In particular, extrapolation of the χM'T signal of compounds 2 and 3 to 0 K from above ~8 K (to avoid the effects of intermolecular interactions at lower temperatures) gives values of ~340 and 255 cm3mol−1 K, respectively. These values are consistent with ST in the range 25–27 (ST = 25, g = 2.05; ST = 26, g = 1.97; ST = 27, g = 1.90) for 2 and 21–23 (ST = 21, g = 2.10; ST = 22, g = 2.01; ST = 23, g = 1.92) for 3.
Discussion
We described herein the synthesis, crystal structures and magnetic properties of a series of high nuclearity mixed metal Mn/Ni and Mn/Co clusters. The first member of this family of complexes, compound 1, was prepared by targeted modifications in the reaction procedures that afforded the family of [Mn44] and [Mn40Na4] “loops-of-loops” aggregates reported by our group previously (Moushi et al., 2010a). Since complex 1 consists of a [Mn36Ni4]2− anion which co-crystallized together with a [Mn2Ni6]2+ cation, we targeted and achieved, following synthetic procedures containing elements of rational design, the isolation of the discrete [Mn36Ni4] (compound 2) and [Mn2Ni6]2+ (the cation of 4) clusters and also of another Mn/3d analog the [Mn32Co8] aggregate of 3. Arguably most of the known giant molecular aggregates have been afforded from serendipitous assembly synthetic procedures although recently there have been a few reports about elegant, rationally designed synthetic strategies that led to such high nuclearity clusters (Papatriantafyllopoulou et al., 2016 and references therein). The isolation of 2–4 thus, represents a rare example of targeted synthesis of high nuclearity metal—organic complexes from procedures that contain elements of rational design.
The heterometallic [Mn36Ni4]2−/0 and [Mn32Co8] “loops-of-loops-and-supertetrahedra” molecular aggregates are giant clusters exhibiting nanosized dimensions and large molecular weights approaching those of small proteins (for example the MW of compound 1 is ~8,095 g/mol and is comparable to those of small proteins which are ~10 kDa). In fact these are the second highest nuclearity heterometallic MnxMy (M = any metal ion) clusters and MxM'y (M, M′ = any 3d metal ion) with the highest nuclearity heterometallic Mn-containing complex and mixed 3d metal/3d metal cluster being a [Mn28Cu17] aggregate (Wang et al., 2007). Interestingly, the [Mn36Ni4]2−/0 and [Mn32Co8] aggregates are based on polynuclear sub-units that have appeared either as fragments and/or in a discrete form previously (Stamatatos et al., 2006; Manoli et al., 2007, 2008, 2016; Moushi et al., 2010a; Wu et al., 2011). This structural feature makes them a member of a very small family of large matal-organic clusters based on known in a discrete form polynuclear repeating units (Manoli et al., 2016; Papatriantafyllopoulou et al., 2016). Their [(μ4-O)4]18+ supertetrahedral repeating unit is very well-known in Mn cluster chemistry and has attracted significant interest due to its symmetric structure and the fact that most of the compounds containing this core exhibit entirely ferromagnetic exchange interactions and the maximum possible, for a [] complex, ST = 22 spin ground state value. The presence of this repeating unit in the [Mn36Ni4]2−/0 and [Mn32Co8] “loops-of-loops-and-supertetrahedra” aggregates ensures the appearance of dominant ferromagnetic exchange interactions between their metal ions and of a large ST value. Notably there are no NiII ions located in this sub-unit in the [Mn36Ni4]2−/0 clusters since all of them are found in the decametallic loops. However, in the [Mn32Co8] aggregate there are two CoII ions in each decametallic supertetrahedron located in the apex positions replacing two MnII ions. In fact, there have been reported some heterometallic Mn10−xMx (M = any metal ion) supertetrahedra where the MnII ions are partially or completely replaced, however, to the best of our knowledge there are no mixed metal Mn10−xCox analogs in the literature. Such compounds could be very attractive magnetically since they could possibly combine the ferromagnetic exchange interactions and large ST values appearing in the decametallic Mn-based supertetrahedra with a significant anisotropy due to the presence of CoII ions. The appearance of the CoII ions in the apex positions of the supertetrahedra is not surprising, not only because they replace a metal ion being in the same oxidation state (MnII) and as a result there are no charge variations in the new compound but also these positions have proven to be the most labile in this family of [] complexes. This is also supported from the isolation a series of heterometallic [[M4−x] (M = any metal ion) supertetrahedra, appearing in a discrete form and also as fragments of high nuclearity clusters, in which the MnII ions have been completely or partially replaced by other metal ions (Skordi et al., 2018 and references therein). The [Mn2Ni6]2+ cation appears also for the first time in heterometallic cluster chemistry although there are homometallic [] clusters reported exhibiting an analogous structural core (Boskovic et al., 2002).
Magnetism studies revealed that the [Mn36Ni4]2−/0/[Mn32Co8] aggregates exhibit dominant ferromamagnetic exchange interactions and large spin ground state values ST = 26 ± 1 ([Mn36Ni4]) and 22 ± 1 ([Mn32Co8]), although in the latter it is not safe to conclude for the ST value due to the presence of the anisotropic CoII ions. On the other hand, the [Mn2Ni6]2+ cation of 4 exhibits dominant antiferromagnetic exchange interactions leading to a diamagnetic ST value. In fact, a diamagnetic ground state has also been reported for the analogous homometallic [] cluster (Boskovic et al., 2002). This behavior could result from the presence of antiferromagnetic exchange interactions between the two [MnIII] (in 4) or [MnIII] (in the []) cubane sub-units leading to diamagnetic ground states. The reported ST values for 2 and 3 are among the larger ones for heterometallic aggregates with the ST ≈ 26 being the second highest value reported for a heterometallic cluster (Chen et al., 2018). Clearly the overall magnetic behavior of 2 exhibits remarkable analogies with that of its [] building block since they both display ferromagnetic exchange interactions, large ST and small D values and are not SMMs. On the other hand, in the case of 3 although it is not safe to conclude about the ST value, however, it is clear that the spin ground state value in 3 is smaller than that of 2. This can be attributed to the existence of more heterometal ions, since in 3 there are 8 CoII ions whereas in 2 only 4 NiII ions and also to the presence of stronger antiferromagnetic exchange interactions as expected for Mn/Co and Mn/Ni heterometallic compounds. In addition, the out-of-phase ac signals at low T in 3 appear due to the presence of the anisotropic CoII ions leading to the increase of the anisotropy and possibly to SMM behavior.
Summarizing, a series of heterometallic [Mn36Ni4]2−/0 and [Mn32Co8] “loops-of-loops-and-supertetrahedra” molecular aggregates and the cationic []2+ cluster were prepared by employing synthetic procedures containing elements of rational design. The “loops-of-loops-and-supertetrahedra” molecular aggregates of 1–3 are among the largest heterometallic Mn-containing clusters exhibiting dimensions and molecular weights comparable to those of small proteins. In addition, they exhibit dominant ferromagnetic exchange interactions and very large ST values. These compounds are new additions in the very small family of giant MnxMy (M = any metal ion) aggregates. Since this area is merely unexplored, further studies targeting to “loops-of-loops-and-supertetrahedra” analogs with various 3d and 4f metal ions and other high nuclearity heterometallic Mn/M clusters are in progress and the results will be reported in due course.
Author Contributions
MC was involved on the synthesis, crystallization, and characterization of the reported complexes. EM was involved on the synthesis, crystallization, and characterization of the reported complexes and on manuscript preparation. TN was involved on the investigation of the magnetic properties of compounds 3 and 4. CP was involved on the investigation of the magnetic properties of compounds 1 and 2 and on manuscript preparation. VN was involved on the refinement of the crystal structures of 1–4 and on manuscript preparation. GC was involved on the investigation of the magnetic properties of compounds 1–2 and on manuscript preparation. AT supervised the reported work and was involved on all parts of the project.
Funding
This work was supported by the Cyprus Research Promotion Foundation Grant PENEK/0311/04 which is co-funded by the Republic of Cyprus and the European Regional Development Fund. GC thanks the NSF for support (CHE-1565664). We also thank the European Union Seventh Framework Program (FP7/2007-2013) under Grant agreement number PIRSES-GA-2011-295190.
Conflict of Interest Statement
The authors declare that the research was conducted in the absence of any commercial or financial relationships that could be construed as a potential conflict of interest.
The handling editor declared a past co-authorship with several of the authors (CP, VN, GC, AT).
Supplementary Material
The Supplementary Material for this article can be found online at: https://www.frontiersin.org/articles/10.3389/fchem.2019.00096/full#supplementary-material
Footnotes
1. ^MAGNET, Indiana University.
References
Abbasi, P., Quinn, K., Alexandropoulos, D. I., Damjanović, M., Wernsdorfer, W., Escuer, A., et al. (2017). Transition metal single-molecule magnets: a {Mn31} nanosized cluster with a large energy barrier of ~60 K and magnetic hysteresis at ~5 K. J. Am. Chem. Soc. 139, 15644–15647. doi: 10.1021/jacs.7b10130
Ako, A. M., Hewitt, I. J., Mereacre, V., Clérac, R., Wernsdorfer, W., Anson, C. E., et al. (2006). Ferromagnetically coupled Mn19 aggregate with a record S = 83/2 ground spin state. Angew. Chem. Int. Ed. 45, 4926–4929. doi: 10.1002/anie.200601467
Alexandropoulos, D. I., Fournet, A., Cunha-Silva, L., Christou, G., and Stamatatos, T. C. (2016). “Molecular nanoclusters”: a 2 nm-sized {Mn29} cluster with a spherical structure. Inorg. Chem. 55, 12118–12121. doi: 10.1021/acs.inorgchem.6b02363
Aromí, G., Aguilà, D., Gamez, P., Luis, F., and Roubeau, O. (2012). Design of magnetic coordination complexes for quantum computing. Chem. Soc. Rev. 41, 537–546. doi: 10.1039/C1CS15115K
Bagai, R., and Christou, G. (2009). The Drosophila of single-molecule magnetism: [Mn12O12(O2CR)16(H2O)4]. Chem. Soc. Rev. 38, 1011–1026. doi: 10.1039/b811963e
Bogani, L. (2015). Experiments on molecular magnets for molecular spintronics. Struct. Bond. 164, 331–382. doi: 10.1007/430_2014_170
Boskovic, C., Wernsdorfer, W., Folting, K., Huffman, J. C., Hendrickson, D. N., and Christou, G. (2002). Single-molecule magnets: novel Mn8 and Mn9 carboxylate clusters containing an unusual pentadentate ligand derived from pyridine-2,6-dimethanol. Inorg. Chem. 41, 5107–5118. doi: 10.1021/ic020217p
Brown, I. D., and Altermatt, D. (1985). Bond-valence parameters obtained from a systematic analysis of the Inorganic Crystal Structure Database. Acta Cryst. B41, 244–247. doi: 10.1107/S0108768185002063
Burla, M. C., Caliandro, R., Camalli, M., Carrozzini, B., Cascarano, G. L., De Caro, L., et al. (2005). SIR2004: an improved tool for crystal structure determination and refinement. J. Appl. Cryst. 38, 381–388. doi: 10.1107/S002188980403225X
Charalambous, M., Moushi, E. E., Papatriantafyllopoulou, C., Wernsdorfer, W., Nastopoulos, V., Christou, G., et al. (2012). A Mn36Ni4 ‘loop-of-loops-and-supertetrahedra’ aggregate possessing a high ST = 26 ± 1 spin ground state. Chem. Commun. 48, 5410–5412. doi: 10.1039/c2cc30654a
Chen, W.-P., Singleton, J., Qin, L., Camón, A., Engelhardt, L., Luis, F., et al. (2018). Quantum Monte Carlo simulations of a giant {Ni21Gd20} cage with a S = 91 spin ground state. Nat. Commun. 9:2107. doi: 10.1038/s41467-018-04547-4
Christou, G., Gatteschi, D., Hendrickson, D. N., and Sessoli, R. (2000). Single-molecule magnets. MRS Bull. 25, 66–71. doi: 10.1557/mrs2000.22
Escuer, A., Esteban, J., Perlepes, S. P., and Stamatatos, T. C. (2014). The bridging azido ligand as a central “player” in high-nuclearity 3d-metal cluster chemistry. Coord. Chem. Rev. 275, 87–129. doi: 10.1016/j.ccr.2014.04.001
Farrugia, L. J. (1999). WinGX program features. J. Appl. Cryst. 32, 837–838. doi: 10.1107/S0021889899006020
Ferrando-Soria, J., Vallejo, J., Castellano, M., Martínez-Lillo, J., Pardo, E., Cano, J., et al. (2017). Molecular magnetism, quo vadis? A historical perspective from acoordination chemist viewpoint. Coord. Chem. Rev. 339, 17–103. doi: 10.1016/j.ccr.2017.03.004
Gerey, B., Goure, E., Fortage, J., Pecaut, J., and Collomb, M. N. (2016). Manganese-calcium/strontium heterometallic compounds and their relevance for the oxygen-evolving center of photosystem II. Coord. Chem. Rev. 319, 1–24. doi: 10.1016/j.ccr.2016.04.002
Inglis, R., Milios, C. J., Jones, L. F., Piligkos, S., and Brechin, E. K. (2012). Twisted molecular magnets. Chem. Commun. 48, 181–190. doi: 10.1039/C1CC13558A
Kang, S., Zheng, H., Liu, T., Hamachi, K., Kanegawa, S., Sugimoto, K., et al. (2015). A ferromagnetically coupled Fe42 cyanide-bridged nanocage. Nat. Commun. 6:5955. doi: 10.1038/ncomms6955
Kostakis, G. E., Ako, A. M., and Powell, A. K. (2010). Structural motifs and topological representation of Mn coordination clusters. Chem. Soc. Rev. 39, 2238–2271. doi: 10.1039/b918192j
Langley, S. K., Stott, R. A., Chilton, N. F., Moubaraki, B., and Murray, K. S. (2011). A high nuclearity mixed valence {Mn32} complex. Chem. Commun. 47, 6281–6283. doi: 10.1039/c1cc00035g
Liu, W., and Thorp, H. H. (1993). Bond valence sum analysis of metal-ligand bond lengths in metalloenzymes and model complexes. 2. Refined distances and other enzymes. Inorg. Chem. 32, 4102–4105. doi: 10.1021/ic00071a023
Maayan, G., Gluz, N., and Christou, G. (2018). A bioinspired soluble manganese cluster as a water oxidation electrocatalyst with low overpotential. Nat. Catalysis 1, 48–54. doi: 10.1038/s41929-017-0004-2
Manoli, M., Alexandrou, S., Pham, L., Lorusso, G., Wernsdorfer, W., Evangelisti, M., et al. (2016). Magnetic “Molecular Oligomers” based on decametallic supertetrahedra: a giant Mn49 cuboctahedron and its Mn25Na4 fragment. Angew. Chem. Int. Ed. Engl. 55, 679–684. doi: 10.1002/anie.201509461
Manoli, M., Collins, A., Parsons, S., Candini, A., Evangelisti, M., and Brechin, E. K. (2008). Mixed-valent Mn supertetrahedra and planar discs as enhanced magnetic coolers. J. Am. Chem. Soc. 130, 11129–11139. doi: 10.1021/ja802829d
Manoli, M., Inglis, R., Manos, M. J., Nastopoulos, V., Wernsdorfer, W., Brechin, E. K., et al. (2011). A [Mn32] double-decker wheel. Angew. Chem. Int. Ed. 50, 4441–4444. doi: 10.1002/anie.201100976
Manoli, M., Johnstone, R. D. L., Parsons, S., Murrie, M., Affronte, M., Evangelisti, M., et al. (2007). A ferromagnetic mixed-valent Mn supertetrahedron: towards low-temperature magnetic refrigeration with molecular clusters. Angew. Chem. Int. Ed. Engl. 46, 4456 −4460. doi: 10.1002/anie.200701027
Milios, C. J., and Winpenny, R. E. P. (2015). Cluster-based single-molecule magnets. Struct. Bond. 164, 1–110. doi: 10.1007/430_2014_149
Moushi, E. E., Lampropoulos, C., Wernsdorfer, W., Nastopoulos, V., Christou, G., and Tasiopoulos, A. J. (2007). A large [Mn10Na]4 loop of four linked Mn10 loops. Inorg. Chem. 46, 3795–3787. doi: 10.1021/ic062454o
Moushi, E. E., Lampropoulos, C., Wernsdorfer, W., Nastopoulos, V., Christou, G., and Tasiopoulos, Aj.J (2010a). Inducing single-molecule magnetism in a family of loop-of-loops aggregates: heterometallic Mn40Na4 clusters and the homometallic Mn44 analogue. J. Am. Chem. Soc. 132, 16146–16155. doi: 10.1021/ja106666h
Moushi, E. E., Masello, A., Wernsdorfer, W., Nastopoulos, V., Christou, G., and Tasiopoulos, A. J. (2010b). A Mn15 single-molecule magnet consisting of a supertetrahedron incorporated in a loop. Dalton Trans. 39, 4978–4985. doi: 10.1039/b927205d
Moushi, E. E., Stamatatos, T. C., Wernsdorfer, W., Nastopoulos, V., Christou, G., and Tasiopoulos, A. J. (2009). A Mn17 octahedron with a giant ground-state spin: occurrence in discrete form and as multidimensional coordination polymers. Inorg. Chem. 48, 5049–5051. doi: 10.1021/ic801795x
Mukherjee, S., Stull, J. A., Yano, J., Stamatatos, T. C., Pringouri, K., Stich, T. A., et al. (2012). Synthetic model of the asymmetric [Mn3CaO4] cubane core of the oxygen-evolving complex of photosystem II. Proc. Natl. Acad. Sci. U.S.A. 109, 2257–2262. doi: 10.1073/pnas.1115290109
Nakano, M., and Oshio, H. (2011). Magnetic anisotropies in paramagnetic polynuclear metal complexes. Chem. Soc. Rev. 40, 3239–3248. doi: 10.1039/c0cs00223b
Papatriantafyllopoulou, C., Moushi, E. E., Christou, G., and Tasiopoulos, A. J. (2016). Filling the gap between the quantum and classical worlds of nanoscale magnetism: giant molecular aggregates based on paramagnetic 3d metal ions. Chem. Soc. Rev. 45, 1597–1628. doi: 10.1039/C5CS00590F
Scott, R. T. W., Parsons, S., Murugesu, M., Wernsdorfer, W., Christou, G., and Brechin, E. K. (2005). Linking centered manganese triangles into larger clusters: a {Mn32} Truncated Cube. Angew. Chem. Int. Ed. Engl. 44, 6540–6543. doi: 10.1002/anie.200501881
Sessoli, R., Tsai, H.-L., Schake, A. R., Wang, S., Vincent, J. B., Folting, K., et al. (1993). High-spin molecules: [Mn12O12(O2CR)16(H2O)]. J. Am. Chem. Soc. 115, 1804–1816. doi: 10.1021/ja00058a027
Sheldrick, G. M. (2014). SHELXL-2014/7, Program for Refinement of Crystal Structures. University of Göttingen.
Skordi, K., Papatriantafyllopoulou, C., Zartilas, S., Poole, K. M., Nastopoulos, V., Christou, G., et al. (2018). Homometallic {Mn10} and heterometallic {Mn6Ca4} supertetrahedra exhibiting an unprecedented { MnII} oxidation state level and heterometal ions distribution. Polyhedron 151, 433–440. doi: 10.1016/j.poly.2018.05.029
Soler, M., Wernsdorfer, W., Folting, K., Pink, M., and Christou, G. (2004). Single-molecule magnets: a large Mn30 Molecular Nanomagnet Exhibiting Quantum Tunneling of Magnetization. J. Am. Chem. Soc. 126, 2156–2165. doi: 10.1021/ja0297638
Stamatatos, T. C., Abboud, K. A., Wernsdorfer, W., and Christou, G. (2006). High-nuclearity, high-symmetry, high-spin molecules: a mixed-valence Mn10 cage possessing rare T symmetry and an S = 22 ground state. Angew. Chem. Int. Ed. 45, 4134 −4137. doi: 10.1002/anie.200600691
Tasiopoulos, A. J., and Perlepes, S. P. (2008). Diol-type ligands as central ‘players’ in the chemistry of high-spin molecules and single-molecule magnets. Dalton Trans. 37, 5537–5555. doi: 10.1039/B805014G
Tasiopoulos, A. J., Vinslava, A., Wernsdorfer, W., Abboud, K. A., and Christou, G. (2004). Giant single-molecule magnets: a {Mn84} torus and its supramolecular nanotubes. Angew. Chem. Int. Ed. 43, 2117–2121. doi: 10.1002/anie.200353352
Van der Sluis, P., and Spek, A.L. (1990). BYPASS: an effective method for the refinement of crystal structures containing disordered solvent regions. Acta Crystallogr. Sect. A 46, 194–201. doi: 10.1107/S0108767389011189
Vincent, J. B., Chang, H.-R., Folting, K., Huffman, J. C., Christou, G., and Hendrickson, D.N. (1987). Preparation and physical properties of trinuclear oxo-centered manganese complexes of general formulation [Mn3O(O2CR)6L3]0, + (R = methyl or phenyl; L = a neutral donor group) and the crystal structures of [Mn3O(O2CMe)6(pyr)3](pyr) and [Mn3O(O2CPh)6(pyr)2(H2O)]·0.5MeCN. J. Am. Chem. Soc. 109, 5703–5711. doi: 10.1021/ja00253a023
Vinslava, A., Tasiopoulos, A. J., Wernsdorfer, W., Abboud, K. A., and Christou, G. (2016). Molecules at the quantum–classical nanoparticle interface: giant Mn70 single-molecule magnets of ~4 nm diameter. Inorg. Chem. 55, 3419–3430. doi: 10.1021/acs.inorgchem.5b02790
Wang, W.-G., Zhou, A.-J., Zhang, W.-X., Tong, M.-L., Chen, X.-M., Nakano, M., et al. (2007). Giant heterometallic Cu17Mn28 cluster with Td symmetry and high-spin ground state. J. Am. Chem. Soc. 129, 1014–1015. doi: 10.1021/ja065707l
Wu, G., Huang, J., Sun, L., Bai, J., Li, G., Cremades, E., et al. (2011). ST = 22 [Mn10] Supertetrahedral building-block to design extended magnetic networks. Inorg. Chem. 50, 8580–8587. doi: 10.1021/ic201154n
Yano, J., and Yachandra, V. (2014). Mn4Ca cluster in photosynthesis: where and how water is oxidized to dioxygen. Chem. Rev. 114, 4175–4205. doi: 10.1021/cr4004874
Keywords: Mn, heterometallic clusters, diols, magnetic properties, crystal structures
Citation: Charalambous M, Moushi EE, Nguyen TN, Papatriantafyllopoulou C, Nastopoulos V, Christou G and Tasiopoulos AJ (2019) Giant Heterometallic [Mn36Ni4]0/2− and [Mn32Co8] “Loops-of-Loops-and-Supertetrahedra” Molecular Aggregates. Front. Chem. 7:96. doi: 10.3389/fchem.2019.00096
Received: 04 August 2018; Accepted: 04 February 2019;
Published: 05 March 2019.
Edited by:
Albert Escuer, University of Barcelona, SpainReviewed by:
Jose Ramon Galan-Mascaros, Institut Català d'Investigació Química, SpainBelen Albela, École Normale Supérieure de Lyon, France
Copyright © 2019 Charalambous, Moushi, Nguyen, Papatriantafyllopoulou, Nastopoulos, Christou and Tasiopoulos. This is an open-access article distributed under the terms of the Creative Commons Attribution License (CC BY). The use, distribution or reproduction in other forums is permitted, provided the original author(s) and the copyright owner(s) are credited and that the original publication in this journal is cited, in accordance with accepted academic practice. No use, distribution or reproduction is permitted which does not comply with these terms.
*Correspondence: Anastasios J. Tasiopoulos, YXRhc2lvQHVjeS5hYy5jeQ==
†Present Address: Eleni E. Moushi, Department of Life Sciences, School of Sciences, European University Cyprus, Nicosia, Cyprus Constantina Papatriantafyllopoulou, School of Chemistry, National University of Ireland Galway, Galway, Ireland