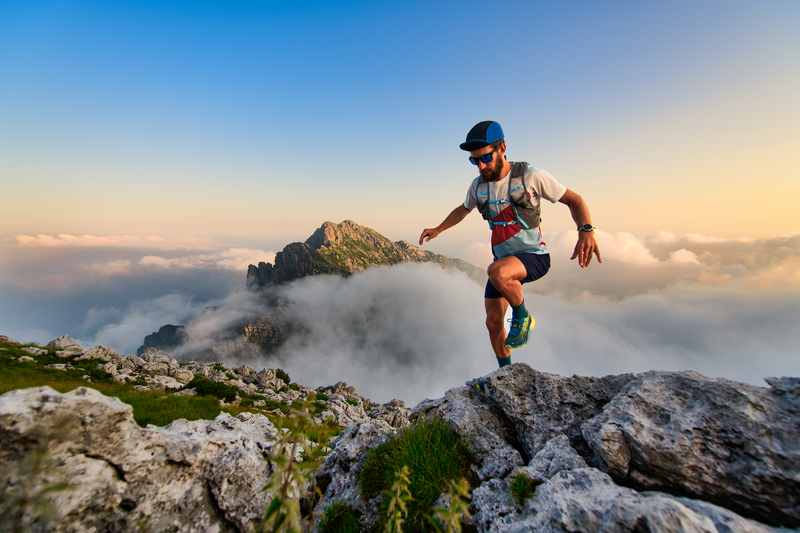
95% of researchers rate our articles as excellent or good
Learn more about the work of our research integrity team to safeguard the quality of each article we publish.
Find out more
REVIEW article
Front. Chem. , 18 December 2018
Sec. Inorganic Chemistry
Volume 6 - 2018 | https://doi.org/10.3389/fchem.2018.00634
This article is part of the Research Topic Realizing Multivalent Batteries: Recent Developments and Challenges View all 8 articles
Recent years have seen an intense and renewed interest in the Mg battery research, naming Mg-S the ≫Holy Grail≪ battery, and expectations that Mg battery system will be able to compete and surpass Li-ion batteries in a matter of years. Considerable progress has been achieved in the field of Mg electrolytes, where several new electrolytes with improved electrochemical performance and favorable chemical properties (non-corrosive, non-nucleophilic) were synthesized. Development in the field of cathodes remains a bit more elusive, with inorganic, sulfur, and organic cathodes all showing their upsides and downsides. This review highlights the recent progress in the field of Mg battery cathodes, paying a special attention to the performance and comparison of the different types of the cathodes. It also aims to define advantages and key challenges in the development of each type of cathodes and finally specific questions that should be addressed in the future research.
Electrical energy storage (EES) is a key technology of the imminent future, and one of the necessary requirements for the broader application of renewable energy resources. There is a plethora of EES technologies like different battery systems, fuel cells, supercapacitors, electrolyzers. However, the most mature and likely dominant EES technology in coming decades will be the Li-ion battery. Concerns about the availability of Li and other metals used in contemporary Li-ion batteries are inciting researchers to develop other technologies that would enable use of batteries on all application levels ranging from electromobility, stationary energy storage to robotics and of course portable electronics.
Magnesium is a promising material for the future post Li-ion batteries due to the high gravimetric (2,206 mAh/g) and volumetric capacity (3,834 mAh/cm3) of Mg anode. Non-dendritic deposition of Mg enables use of Mg metal anode in the practical Mg batteries. The higher redox potential of Mg, when compared with Li, decreases the overall voltage of the battery, but this is compensated by the high capacity of the anode, given the condition that capacity of the cathode does not decrease. Moreover, Mg is among ten most abundant elements in the Earth's crust, which should remove any concerns about sustainability of Mg batteries. Besides discussed benefits, there are several challenges connected with the use of Mg metal, mainly incompatibility of the Mg metal with the electrolytes commonly used in Li-ion batteries and lack of suitable cathodes. Mg deposition from solutions of Grignard reagent was reported already in early twentieth century (Gaddum and French, 1927). Unfortunately, the low conductivity and inadequate oxidative stability prevents their application as battery electrolytes. In 1990, Gregory et al. reported on the use of various Mg complexes and inorganic compounds that could function as electrolytes and cathodes, respectively (Gregory et al., 1990). A proof-of-concept Mg battery was demonstrated by Aurbach et al. who utilized a Mo6S8-Chevrel phase as a cathode and Mg(AlCl2BuEt)2 as an electrolyte (Aurbach et al., 2000). The battery displayed impressive long-term cycling, it could operate for more than 2,000 cycles with a capacity fade of < 15%. The voltage of this system was relatively low displaying two plateaus at 1.3 and 1.1 V. This seminal work of Aurbach et al. inspired others, and demonstrated the need to develop cathode materials with higher voltages. Along with development of new electrolytes, a lot of effort has been devoted into exploration of different magnesium insertion compounds based on oxide, polyanionic, or chalcogenide structures, with only limited success. On the other hand, the recent discoveries of non-nucleophilic electrolytes (Kim et al., 2011; Zhao-Karger et al., 2013, 2017; Doe et al., 2014) opened a path toward the use of electrophilic cathode materials like S and organic compounds, which are quickly catching up and in some characteristics also outperforming inorganic cathodes.
Due to thermodynamic instability of Mg and Li metals in electrolytes, a passive film formation is typically formed at the interphase. However, unlike passive film on Li which is good ionic conductor, Mg passive film typically tends to block the transport of the Mg2+ ions, which narrows the choice of salts and solvents for Mg electrolytes. Impurities in small amounts like H2O lead to the formation of compact passive films on Mg anode, which can significantly increase the overpotentials for Mg stripping/deposition (Connell et al., 2016; He et al., 2017). Thus, attention should be paid to minimization of water content in Mg electrolytes by using extra dry salts and solvents. Partially passivating Mg electrolytes [Mg(TFSI)2 without any additives] lead to large overpotentials for Mg stripping/deposition (Ha et al., 2014; Tutusaus et al., 2017). Practical cycling of 2-electrode Mg cells containing Mg anodes is impossible in electrolytes containing carbonates, nitriles, and Mg(ClO4)2 due to complete passivation of the Mg metal. Besides electrolyte components, the morphology and pretreatment procedures of Mg anode also affect the stripping/deposition process at the anode. This is illustrated in Figure 1 where the electrochemical behavior of Mg-organic battery is shown for two different types of anode. The cell with non-brushed Mg (unmodified native passive film) foil exhibits a higher overpotential in the initial cycles compared to the cell with synthesized Mg powder anode (Figure 1A—difference between doted and full line curves). The difference in the overpotential is particularly high at the start of the first cycle (Figure 1B) and lowers with continued cycling. Type of the Mg anode is especially important in chloride free electrolytes, which have limited capability for Mg surface etching.
Figure 1. Galvanostatic cycling of Mg-organic battery with poly (anthraquinoyl sulfide) cathode and two different Mg anodes: non-brushed Mg foil (dashed) and synthesized Mg powder (full line). (A) Selected galvanostatic cycles at a current density of 50 mA/g in Mg2Cl3-HMDSAlCl3 in tetrahydrofuran (THF). (B) Inset into the first discharge at the start of the cycle. Reproduced with permission from Bitenc et al. (2015). Copyright Wiley-VCH.
Inorganic materials considered for Mg cathodes span from chalcogenides, oxides to polyanionic structures. While inorganic materials have already been reviewed in considerable detail (Canepa et al., 2017), we wish only to highlight the most important developments that represent advancement in understanding of Mg intercalation inside well-organized inorganic framework. From the early reports by Aurbach et al. on Mg intercalation into Mo6S8 (Aurbach et al., 2000), this material has served as a benchmarking material and is by far the most studied Mg cathode. Facile extraction of Mg ions from Mg2Mo6S8 at room temperature is possible only for the first Mg whereas it is more or less impossible for the second one due to trapping of Mg ions inside the structure (Levi et al., 2006). This effect can be mitigated by replacing S with Se, which allows a full capacity utilization of Mo6Se8 (Levi et al., 2005).
Other chalcogenides offer an opportunity of surpassing the electrochemical performance of Chevrel phases by using other transition metals, while maintaining low electrostatic interactions between the Mg ion and the anion lattice (Wang et al., 2015). Low electrostatic interactions lead to increased Mg ion mobility, but this comes at a cost of reduced cell voltage. One of the most studied materials are titanium sulfides which can be divided into three groups: bulk layered (TiS2), spinel (Ti2S4), or nanotubes (TiS2). Early results exemplified pronounced hysteresis (Amir et al., 2007) or showed questionable 2-electrode cycling in passivating Mg electrolytes (Tao et al., 2004). Recent reports have demonstrated that both thiospinel Ti2S4 (Sun et al., 2016a) and layered TiS2 (Sun et al., 2016b) can display good reversibility at elevated temperature of 60°C. Especially thiospinel, with its relatively good cycling stability represents considerable improvement over both capacity and voltage of the Chevrel phase cathode (Figure 2). The second most researched chalcogenide material is MoS2 (Li and Li, 2004; Liang et al., 2011, 2015). Among selenides TiSe2 (Gu et al., 2015) and WSe2 (Liu et al., 2013) have been examined. However, the latter was tested in an electrochemical window that exceeded the operating window of the electrolyte.
Figure 2. (A) First and second (inset) galvanostatic cycles of thiospinel Ti2S4 in all phenyl complex (APC) in THF electrolyte at 60°C. (B) Capacity and Coulombic efficiency of Ti2S4 at a 0.1C rate in APC in tetraglyme (TEG) electrolyte at 60°C. Reproduced with permission from Sun et al. (2016a). Copyright Royal Society of Chemistry.
Use of oxide materials opens a possibility to increase both the working voltage and capacity of the cathode materials. In practice most of the oxide materials suffer from poor Mg mobility (Rong et al., 2015; Sai Gautam et al., 2015), oxide conversion reactions (Zhang et al., 2015), pseudocapacitance and lack of suitable electrolytes that would enable reliable testing of their electrochemical activity. Certain layered materials like MoO3 (Spahr et al., 1995; Gershinsky et al., 2013), Mo2.48VO9.93 (Kaveevivitchai and Jacobson, 2016), and V2O5 (Gershinsky et al., 2013) have displayed reversible intercalation in electrolytes that cannot enable reversible Mg deposition/stripping. Solvent co-intercalation or intercalation of Mg2+ in hosts containing water have shown a beneficial effect on electrochemical performance for different MnO2 polymorphs [birnessite (Nam et al., 2015; Sun et al., 2016c) and spinel (Kim et al., 2015)] and V2O5 materials (Novak et al., 1999; Imamura and Miyayama, 2003; Tepavcevic et al., 2015).
Materials based on polyanionic structure are a very diverse group whose electrochemical performance is far from being fully explored. Early reports have shown a promising performance of different silicates (Mn, Fe, Co) (Feng et al., 2008; Li et al., 2011; NuLi et al., 2011). Although corrosion of Cu current collectors is the likely cause for identical redox potentials of silicates containing different transition metals and extremely large discrepancy between calculated and measured cathode potentials (Ling et al., 2012). Cycling of MgFeSiO4 prepared through the electrochemical delithiation of Li2FeSiO4 has demonstrated limited Mg insertion (Orikasa et al., 2014), which confirms contributions from side reactions in earlier reports. NASICON types of materials have been investigated with limited success (Makino et al., 2001; Huang et al., 2015) and have demonstrated magnesiation only on the surface of the particles (Huang et al., 2015). Intercalation of Mg into FePO4 caused amorphization of FePO4 due to thermodynamic instability of the intercalated product (Zhang and Ling, 2016). Among fluoro-polyanions, tavorite-FeSO4F should theoretically have good Mg mobility (Wu et al., 2014), while experimental results on MgFePO4F display limited electrochemical performance (Huang et al., 2014). An interesting group of materials are Prussian blue and its analogs that demonstrated the ability to intercalate several different multivalent ions both in aqueous and non-aqueous electrolytes (Gao et al., 2015; Lipson et al., 2016; Chen et al., 2017).
At the present state Chevrel phases and some other transition metal sulfides possess good reversibility of magnesium insertion while materials based on oxide and polyanionic structure cannot offer electrochemical performance required for practical cathodes due to poor mobility and structural instability. However, they might offer a prospect of developing high voltage Mg cathodes. Additional aspect, which has to be taken into consideration are interfaces between electrolyte and active cathode particles, particularly in chloride-containing electrolytes that are still used in many different experimental set-ups. A significant computational effort has been invested in the computer driven screening of the candidate inorganic hosts and could offer useful guidelines for future development (Emly and Van der Ven, 2015; Gautam et al., 2015; Rong et al., 2015; Sai Gautam et al., 2016; Kulish et al., 2017). Still, key requirements like good Mg2+ mobility inside intercalation host, long-term cycling stability and electrolyte compatibility will have to be demonstrated experimentally.
Organic materials are an attractive group of cathode materials, because they can be produced from low-cost and abundant resources, which in turn opens a path to more sustainable EES (Schon et al., 2016). The fact that they can be used with a variety of counter ions makes them especially interesting for the Mg system. Their downside is low volumetric energy density, which is compensated by high volumetric energy density of Mg anode. A rapid capacity fade due to dissolution of organic molecules into electrolyte remains a general issue. However, it can be effectively mitigated by grafting of organics onto a solid support (Genorio et al., 2010), preparation of insoluble polymers (Song et al., 2009), or use of selective separators (Senoh et al., 2011).
Organic materials often contain electrophilic centers inside their structures, which makes them incompatible with nucleophilic Mg electrolytes. However, there have been reports, where nucleophilic electrolytes are used with organic cathodes, but it is difficult to differentiate between electrochemical activity attributed to organic compounds and pseudocapacitance response from side reactions in this class of electrolytes (NuLi et al., 2007; Qiang et al., 2013). The first reversible electrochemical reaction of an organic compound with Mg2+ ions was demonstrated on dimethoxybenzoquinone (DMBQ) in 0.5 M Mg(ClO4)2 in γ-butyrolactone and confirmed through ex-situ XRD (Sano et al., 2012). Electrolyte used in this study passivates the Mg anode and reversible cycling of the cathode was achieved in a 3-electrode setup. Attempts of cycling organics in Mg passivating electrolytes in 2-electrode setups led to huge overpotentials for Mg stripping/deposition, which resulted in extremely poor cycling (Ha et al., 2014; Senoh et al., 2014, 2015).
A significant step forward was achieved by a combination of non-nucleophilic electrolytes and an organic polymer cathode—poly(anthraquinonyl sulfide)-PAQS (Bitenc et al., 2015). This combination allowed Mg stripping/deposition with reasonable overpotential and improved capacity retention of the cathode compared to previous results. Average discharge voltage of the cell was 1.5 V. The electrochemical mechanism of Mg-PAQS system was investigated through operando ATR-IR (attenuated total reflectance infrared spectroscopy) which confirmed the reduction of carbonyl bond upon discharge (Vizintin et al., 2018). This is evident from the difference ATR-IR spectra of PAQS cathode obtained by subtraction of two subsequent spectra at different state-of-charge (Figure 3A). A decrease of intensity for C = O band and appearance of new –C–O− band is observed during discharge, while in charge the intensity of C = O band increases and –C–O− band decreases. This observation confirms a reversible electrochemical mechanism through reduction of carbonyl bond. At a current density corresponding to 1C (Figure 3B) significantly lower capacities were obtained in Mg system compared to the Li counterpart by using same type of cathode composite, which points toward worse accessibility of electroactive groups in the former. Differences between obtained capacities in Li and Mg batteries are lower at lower current densities, but still remain relatively large for this polymer.
Figure 3. (A) ATR-IR spectroscopy of PAQS (red), operando ATR-IR of PAQS cathode in discharge (blue) and charge (green). (B) Capacity performance of PAQS cathode in Li and Mg battery system at different cycling rates in 0.4 M MgCl2-Mg(TFSI)2 in tetraglyme:dioxolane (1:1 vol%) (Vizintin et al., 2018). (Adapted from (Vizintin et al., 2018) under a Creative Commons attribution 4.0 International license. http://creativecommons.org/licenses/by/4.0/).
The use of non-nucleophilic electrolytes has been extended to DMBQ which in combination with Mg exhibited, for the first time, a discharge voltage of 2.0 V. However, dissolution of DMBQ, which is a small, relatively easily soluble molecule, into electrolyte caused a rapid capacity fade (Pan et al., 2016b). Due to high voltage and high specific capacity of the benzoquinone group, polymers based on benzoquinone could be considered promising cathode materials. Indeed, our study involving such polymer has demonstrated an average discharge voltage of 2.0 V as well as a good cycling stability (Bitenc et al., 2016). Here it has to be noted that for benzoquinone based polymers practical capacities close to theoretical ones (around 400 mAh/g) have still to be achieved not only for Mg, but also for Li systems.
Impressive results have been obtained using 1,4-polyanthraquinone (PAQ), which showed superior cycling properties, i.e., more than 1,000 cycles at elevated rates (1C and 2C) in an electrolyte containing Mg(HMDS)2-4MgCl2 (HMDS-hexamethyldisilazane) and THF (Pan et al., 2016a). Obtained capacity in this work represents only 50% of the theoretical value (260 mAh/g) and the authors did not report on capacities that could be obtained in a comparable Li system (Song et al., 2015), which could serve as a reference point providing additional information about the practical utilization of this material. Our results on PAQS show that there are significant differences between the capacities that can be obtained in different Mg electrolytes and a standard Li electrolyte (Figure 4).
Figure 4. Comparison of capacity retention of PAQS in Li 1 M LiTFSI in DME:DOL = 1:1 (vol%) and two Mg electrolytes [Mg(TFSI)2-2.5MgCl2, MgCl2-0.3AlCl3] at current density of 50 mA/g in voltage window from 0.5 to 2.5 V. Adapted with permission from Bitenc et al. (2015). Copyright Wiley-VCH.
All the Mg-based electrolytes displayed lower capacities than the Li reference electrolyte. However, the differences among the Mg electrolytes were very high, the Mg(TFSI)2 based electrolyte has a maximum capacity of 218 mAh/g, while maximum capacity in AlCl3 containing electrolyte is significantly lower, only 139 mAh/g. Although capacity fade is faster in Mg(TFSI)2 this electrolyte still has 50% bigger capacity after 20 cycles. Maximum attainable capacity is also relatively close to the theoretical capacity (225 mAh/g) of PAQS. All this facts illustrate that while the Mg(TFSI)2 salt can partially passivate the Mg anode (Tutusaus et al., 2017), it is probably the optimal salt for organic cathode at the present state of electrolyte development since the maximum capacity in Mg system can be achieved using a Mg(TFSI)2 based electrolyte. These results demonstrate that the active species in Mg electrolytes depend on the used salts and have a significant effect on the practical performance of organic cathodes. However, significant steps forward will be needed in the direction of improving the capacity retention. Recently, we investigated polyimide cathode materials, where we successfully demonstrated electrochemical activity of naphthalene-hydrazine diimide polymer. The polymer undergoes reversible reduction to enolate anion, which was confirmed using operando ATR-IR. This exemplifies that Mg-organic batteries are not limited to only quinone class of compounds, but can be easily applied to other type of compounds (Bančič et al., 2018). Polyimide based material has also been used as an anode in aqueos Mg battery, which utilized Prussian blue cathode (Chen et al., 2017). In aqueous Mg electrolyte polyimide cathode could be cycled for more than 2,000 cycles.
Organic electrode materials offer good electrochemical performance in practical 2-electrode cells, which is summarized in Table 1. Currently, some of them exhibit long-term cycling performance, while others offer a possibility to achieve 2 V Mg batteries by application of the benzoquinone group (Bitenc et al., 2016). The next steps should focus on improving the capacity retention and achieving practical capacities close to theoretical ones. Results discussed above indicate that in certain combinations of active materials and electrolytes this goal is realistic. A practical realization of Mg-organic battery will also depend on the volumetric density, areal loadings of organic electrodes and the amount of electrolyte required for practical cells. Thus, future research efforts on the topic of organic materials should also address these questions.
Table 1. Summary of different organic compounds tested in 2-electrode setups with their theoretical capacity, average discharge voltages, capacity retention, and used electrolytes.
Sulfur is a promising cathode material for several battery systems such as Li-S, Na-S, and Mg-S. It offers a high gravimetric capacity of 1,672 mAh/g, which is ~10 times higher than the capacities of conventional insertion cathodes. It is considered as a cheap and sustainable cathode material. Its downsides are a lower voltage and a relatively low volumetric energy density. Thus, the Mg anode with its high volumetric capacity is an ideal anode to be coupled with the sulfur cathode. First Mg-S battery was reported jointly with first non-nucleophilic Mg electrolyte, Mg2Cl3-HMDSAlCl3 (Kim et al., 2011) with this electrolyte Mg-S battery demonstrated two reversible cycles, while electrochemical activity of sulfur cathode was confirmed with XPS (X-ray photoelectron spectroscopy). The use of THF solvent facilitated the dissolution of sulfur and polysulfides in the electrolyte causing a discharge voltage below 1 V, and a large polarization (Li et al., 2016). A significant step forward was achieved by preparation of electrolytes from a combination of salts, specifically MgCl2, Mg(HMDS)2, and AlCl3 in DEG and TEG. In such electrolytes the Mg-S system displayed two discharge plateaus, the first at around 1.6 V and the second sloped plateau below 1.2 V vs. Mg/Mg2+. Addition of ionic liquids slightly improved the discharge capacities, but the maximum capacities did not exceed 800 mAh/g due to kinetic limitations (Zhao-Karger et al., 2014). Increase of capacities in this system was achieved by impregnation of sulfur into reduced graphene oxide, but unfortunately no data is available about the capacitance and pseudocapacitance contribution of rGO (Vinayan et al., 2016). An additional approach toward improved capacity retention is coating of separators with carbon nanofibers, which effectively suppressed the initial capacity fade from the first to the second cycle (Yu and Manthiram, 2016).
Electrolyte in Mg-S batteries plays an important role and so far different non-nucleophilic Mg electrolytes have been proposed. A large family of electrolytes is based on different combinations using Mg(TFSI)2 salt. Electrolytes based only on Mg(TFSI)2 suffer from high over potential for Mg stripping and deposition (Ha et al., 2014) due to surface passivation (Tutusaus et al., 2017). The use of a 3-electrode cell is an option for fundamental studies (Bitenc et al., 2016), whereas addition of additives that form a beneficial interlayer on the surface of Mg anode could be a solution for more realistic 2-electrode systems, as exemplified by addition of I2 to electrolyte (Li et al., 2017). Mg(TFSI)2 salt can be used to facilitate the dissolution of MgCl2, which is by itself insoluble in ether type of solvents (Shterenberg et al., 2015). Due to commercial availability of both salts, this is indeed one of the most common Mg electrolytes. Our group has used these two salts in combination of TEG and DOL solvents. Such combinations led to a high initial capacity of 1,320 mAh/g. The cells displayed two discharge plateaus, a high voltage plateau at 1.4 V and a lower one at 1.2 V vs. Mg/Mg2+ (Robba et al., 2017).
By using XRD we were not able to detect any of crystalline forms of MgS, while diffraction peaks corresponding to sulfur disappeared during the first discharge plateau pointing toward electrochemical conversion of sulfur into polysulfides during the first plateau. Operando X-ray absorption near edge spectra (XANES) and resonant inelastic X-ray scattering (RIXS) (Figure 5A) confirmed this observation and showed that MgS precipitation starts at the beginning of the low voltage plateau. The comparison of synthesized and electrochemically prepared MgS revealed that the chemically prepared MgS crystallized in a rock-salt structure, while the electrochemically prepared analog adopted a wurtzite-like structure. The capacity fade was especially pronounced during starting cycles so that after 10 cycles the capacity value was < 400 mAh/g (Figure 5B). However, employing a highly concentrated electrolyte consisting of Mg(TFSI)2-2MgCl2 in DME improved significantly the cycling stability: more than 100 cycles with the final discharge capacity of above 600 mAh/g were achieved due to decreased solubility of polysulfides (Gao et al., 2017).
Figure 5. (A) First discharge of Mg-S battery in 0.4 M MgCl2-Mg(TFSI)2 in TEG:DOL (vol%) at C/60 and relative amounts of sulfur compounds as determined by operando RIXS. (B) Capacity and Coulombic efficiency of the same battery system tested at same electrochemical conditions. Adapted with permission from Robba et al. (2017). Copyright American Chemical Society.
Certain reports on Mg-S batteries in tris(hexafluoroisopropyl) borate [B(HFIP)3] based electrolytes exhibit a peculiar single discharge plateau at approximately 1.1 V with capacities above 1,000 mAh/g and low overpotential in charge (Du et al., 2017; Xu et al., 2017; Zhang et al., 2017). It has to be noted that these systems employed Cu current collectors, which might have a crucial role in cycling, given inferior cathode performance with Al current collector (Du et al., 2017). The significant differences in this report indicate that role of Cu current collector needs to be investigated in detail and the possibility of electrochemical cycling of copper sulfide species excluded. By contrast, cycling of S cathode in similar electrolyte, Mg(B(HFIP)4)2, led to a higher voltage plateau typical for other reports on Mg–S battery (Zhao-Karger et al., 2017, 2018).
The Mg-S battery is attractive from the point of energy density and sustainability of active materials, but there are many issues which need to be resolved. For that reason, a detailed investigation of both interfacial and bulk behavior is required. It would be highly beneficial to employ more bulk-sensitive analytical techniques such as XRD, NMR, XANES, EXAFS, and RIXS, for future investigation of Mg-S electrochemistry as opposed to the currently predominating use of XPS, which gives important information about surface phenomena, but might conceal certain phenomena occurring in the bulk. Additionally, research in the field of Mg-S batteries could benefit from standardization of the electrolyte amount, which is known to have a big influence on the mechanism and performance of Li-S batteries. Since the initial report on Mg-S battery system (Kim et al., 2011) a substantial progress has been made and the specific Mg-S systems demonstrate more than 100 discharge-charge cycles (Gao et al., 2017). Regardless of that, capacity retention remains a pressing issue in most of recent reports (Li et al., 2017; Robba et al., 2017; Zhao-Karger et al., 2017). Thus, more effort should be devoted to the investigation of Mg polysulfide mechanism, as only improved understanding will provide solid guidelines toward mitigation of this issue.
Several steps forward have been made in development of cathodes, especially in the performance of organic and sulfur cathodes that can be cycled for more than hundred cycles with suitable non-nucleophilic electrolytes. The progress in the field of inorganic cathodes remains more gradual with some chalcogenides surpassing the performance of Chevrel phases at elevated temperatures, while the electrochemical performance of materials based on oxide and polyanionic structures still remains limited. Although Mg battery research is almost three decades old, no standard electrolytes and testing conditions have been set so far. This is a consequence of ongoing development of new electrolytes that goes side by side with cathode research. As we have shown here, the performance of cathode materials can be largely influenced by the type of electrolyte as well as by the properties of Mg anode. Thus, researchers should compare different electrolytes and anodes but also test composites without active materials to identify possible side reactions and compatibility of electrochemical cell (housing, current collectors…). Only then standardized systems will evolve and enable a better comparison of literature results. Most importantly, the electrochemical mechanisms should be investigated through use of bulk analytical techniques to improve our understanding of electrochemical reactions inside cathodes, which will lead to development of next generation Mg cathodes.
Both authors listed have made a direct and intellectual contribution to the work, and approved it for publication.
The authors declare that the research was conducted in the absence of any commercial or financial relationships that could be construed as a potential conflict of interest.
The research has received funding from the Slovenian Research Agency research program P2-0393 and from Honda R&D Europe. We would like to thank Dr. Alen Vižintin, used letter Ž is uppercase in current version. Ms. Ana Robba, and Prof. Dr. Miran Gaberšček, for discussion, all from National Institute of Chemistry in Ljubljana.
Amir, N., Vestfrid, Y., Chusid, O., Gofer, Y., and Aurbach, D. (2007). Progress in nonaqueous magnesium electrochemistry. J. Power Sources 174, 1234–1240. doi: 10.1016/j.jpowsour.2007.06.206
Aurbach, D., Lu, Z., Schechter, A., Gofer, Y., Gizbar, H., Turgeman, R., et al. (2000). Prototype systems for rechargeable magnesium batteries. Nature 407, 724–727. doi: 10.1038/35037553
Bančič, T., Bitenc, J., Pirnat, K., Kopač Lautar, A., Grdadolnik, J., Randon Vitanova, A., et al. (2018). Electrochemical performance and redox mechanism of naphthalene-hydrazine diimide polymer as a cathode in magnesium battery. J. Power Sources 395 , 25–30. doi: 10.1016/j.jpowsour.2018.05.051
Bitenc, J., Pirnat, K., Bančič, T., Gaberšček, M., Genorio, B., Randon-Vitanova, A., et al. (2015). Anthraquinone-based polymer as cathode in rechargeable magnesium batteries. ChemSusChem 8, 4128–4132. doi: 10.1002/cssc.201500910
Bitenc, J., Pirnat, K., Mali, G., Novosel, B., Randon Vitanova, A., and Dominko, R. (2016). Poly(Hydroquinoyl-Benzoquinonyl Sulfide) as an active material in Mg and Li organic batteries. Electrochem. commun. 69, 1–5. doi: 10.1016/j.elecom.2016.05.009
Canepa, P., Sai Gautam, G., Hannah, D. C., Malik, R., Liu, M., Gallagher, K. G., et al. (2017). Odyssey of multivalent cathode materials: open questions and future challenges. Chem. Rev. 117, 4287–4341. doi: 10.1021/acs.chemrev.6b00614
Chen, L., Bao, J. L., Dong, X., Truhlar, D. G., Wang, Y., Wang, C., et al. (2017). Aqueous Mg-ion battery based on polyimide anode and prussian blue cathode. ACS Energy Lett. 2, 1115–1121. doi: 10.1021/acsenergylett.7b00040
Connell, J. G., Genorio, B., Lopes, P. P., Strmcnik, D., Stamenkovic, V. R., and Markovic, N. M. (2016). Tuning the reversibility of Mg anodes via controlled surface passivation by H2O/Cl− in organic electrolytes. Chem. Mater. 28, 8268–8277. doi: 10.1021/acs.chemmater.6b03227
Doe, R. E., Han, R., Hwang, J., Gmitter, A. J., Shterenberg, I., Yoo, H. D., et al. (2014). Novel, electrolyte solutions comprising fully inorganic salts with high anodic stability for rechargeable magnesium batteries. Chem. Commun. 50, 243–245. doi: 10.1039/C3CC47896C
Du, A., Zhang, Z., Qu, H., Cui, Z., Qiao, L., Wang, L., et al. (2017). An efficient organic magnesium borate based electrolyte with non-nucleophilic characteristic for magnesium sulfur battery. Energy Environ. Sci. 10, 2616–2625. doi: 10.1039/C7EE02304A
Emly, A., and Van der Ven, A. (2015). Mg intercalation in layered and spinel host crystal structures for Mg batteries. Inorg. Chem. 54, 4394–4402. doi: 10.1021/acs.inorgchem.5b00188.
Feng, Z., Yang, J., NuLi, Y., Wang, J., Wang, X., and Wang, Z. (2008). Preparation and electrochemical study of a new magnesium intercalation material Mg1.03Mn0.97SiO4. Electrochem. Commun. 10, 1291–1294. doi: 10.1016/j.elecom.2008.06.021
Gaddum, L. W., and French, H. E. (1927). The electrolysis of grignard solutions. J. Am. Chem. Soc. 49, 1295–1299. doi: 10.1021/ja01404a020
Gao, T., Hou, S., Wang, F., Ma, Z., Li, X., Xu, K., et al. (2017). Reversible S0/MgSxRedox chemistry in a MgTFSI2/MgCl2/DME electrolyte for rechargeable Mg/S batteries. Angew. Chem. Int. Ed. 56, 13526–13530. doi: 10.1002/anie.201708241
Gao, T., Noked, M., Pearse, A. J., Gillette, E., Fan, X., Zhu, Y., et al. (2015). Enhancing the reversibility of Mg/S battery chemistry through Li+ mediation. J. Am. Chem. Soc. 137, 12388–12393. doi: 10.1021/jacs.5b07820
Gautam, G. S., Canepa, P., Malik, R., Liu, M., Persson, K., and Ceder, G. (2015). First-Principles evaluation of multi-valent cation insertion into orthorhombic V2O5. Chem. Commun. 51, 13619–13622. doi: 10.1039/C5CC04947D
Genorio, B., Pirnat, K., Cerc-Korosec, R., Dominko, R., and Gaberscek, M. (2010). Electroactive organic molecules immobilized onto solid nanoparticles as a cathode material for lithium-ion batteries. Angew. Chemie Int. Ed. 49, 7222–7224. doi: 10.1002/anie.201001539
Gershinsky, G., Yoo, H. D., Gofer, Y., and Aurbach, D. (2013). Electrochemical and spectroscopic analysis of Mg2+ intercalation into thin film electrodes of layered oxides : V2O5 and MoO3. Langmuir 29, 10964–10972. doi: 10.1021/la402391f
Gregory, T. D., Hoffman, R. J., and Winterton, R. C. (1990). Nonaqueous electrochemistry of magnesium : applications to energy storage. J. Electrochem. Soc. 137, 775–780. doi: 10.1149/1.2086553
Gu, Y., Katsura, Y., Yoshino, T., Takagi, H., and Taniguchi, K. (2015). Rechargeable magnesium-ion battery based on a TiSe2-cathode with d-p orbital hybridized electronic structure. Sci. Rep. 5:12486. doi: 10.1038/srep12486
Ha, S. Y., Lee, Y. W., Woo, S. W., Koo, B., Kim, J. S., Cho, J., et al. (2014). Magnesium(II) Bis(Trifluoromethane Sulfonyl) imide-based electrolytes with wide electrochemical Windows for rechargeable magnesium batteries. ACS Appl. Mater. Interfaces 6, 4063–4073. doi: 10.1021/am405619v
He, S., Luo, J., and Liu, T. L. (2017). MgCl2/AlCl3 electrolytes for reversible Mg deposition/stripping: electrochemical conditioning or not? J. Mater. Chem. A 5, 12718–12722. doi: 10.1039/C7TA01769C
Huang, Z., Masese, T., Orikasa, Y., Mori, T., Minato, T., Tassel, C., et al. (2014). MgFePO4F as a feasible cathode material for magnesium batteries. J. Mater. Chem. A 2, 11578–11582. doi: 10.1039/c4ta01779j
Huang, Z.-D., Masese, T., Orikasa, Y., Mori, T., and Yamamoto, K. (2015). Vanadium phosphate as a promising high-voltage magnesium ion (de)-intercalation cathode host. RSC Adv. 5, 8598–8603. doi: 10.1039/C4RA14416C
Imamura, D., and Miyayama, M. (2003). Characterization of magnesium-intercalated V2O5/carbon composites. Solid State Ionics 161, 173–180. doi: 10.1016/S0167-2738(03)00267-4
Kaveevivitchai, W., and Jacobson, A. J. (2016). High capacity rechargeable magnesium-ion batteries based on a microporous molybdenum-vanadium oxide cathode. Chem. Mater. 28, 4593–4601. doi: 10.1021/acs.chemmater.6b01245
Kim, C., Phillips, P. J., Key, B., Yi, T., Nordlund, D., Yu, Y., et al. (2015). Direct observation of reversible magnesium ion intercalation into a spinel oxide host. Adv. Mater. 27, 3377–3384. doi: 10.1002/adma.201500083
Kim, H. S., Arthur, T. S., Allred, G. D., Zajicek, J., Newman, J. G., Rodnyansky, A. E., et al. (2011). Structure and compatibility of a magnesium electrolyte with a sulphur cathode. Nat. Commun. 2:427. doi: 10.1038/ncomms1435
Kulish, V. V., Koch, D., and Manzhos, S. (2017). Aluminium and magnesium insertion in sulfur-based spinels: a first-principles study. Phys. Chem. Chem. Phys. 19, 6076–6081. doi: 10.1039/C6CP08284J
Levi, E., Lancry, E., Mitelman, A., Aurbach, D., Ceder, G., Morgan, D., et al. (2006). Phase diagram of Mg insertion into chevrel phases, MgxMo6T8(T = S, Se). 1. Crystal structure of the sulfides. Chem. Mater. 18, 5492–5503. doi: 10.1021/cm061656f
Levi, M. D., Lancri, E., Levi, E., Gizbar, H., Gofer, Y., and Aurbach, D. (2005). The effect of the anionic framework of Mo6X8 chevrel phase (X = S, Se) on the Thermodynamics and the kinetics of the electrochemical insertion of Mg2+ ions. Solid State Ionics 176, 1695–1699. doi: 10.1016/j.ssi.2005.04.019
Li, W., Cheng, S., Wang, J., Qiu, Y., Zheng, Z., Lin, H., et al. (2016). Synthesis, crystal structure, and electrochemical properties of a simple magnesium electrolyte for magnesium/sulfur batteries. Angew. Chem. Int. Ed. 55, 6406–6410. doi: 10.1002/anie.201600256
Li, X., Gao, T., Han, F., Ma, Z., Fan, X., Hou, S., et al. (2017). Reducing Mg anode overpotential via ion conductive surface layer formation by iodine additive. Adv. Energy Mater. 8:1701728. doi: 10.1002/aenm.201701728
Li, X. L., and Li, Y. D. (2004). MoS2 nanostructures: synthesis and electrochemical Mg2+ intercalation. J. Phys. Chem. B 108, 13893–13900. doi: 10.1021/jp0367575
Li, Y., Nuli, Y., Yang, J., Yilinuer, T., and Wang, J. (2011). MgFeSiO4 prepared via a molten salt method as a new cathode material for rechargeable magnesium batteries. Chinese Sci. Bull. 56, 386–390. doi: 10.1007/s11434-010-4247-4
Liang, Y., Feng, R., Yang, S., Ma, H., Liang, J., and Chen, J. (2011). Rechargeable Mg batteries with graphene-like MoS2 cathode and ultrasmall Mg nanoparticle anode. Adv. Mater. 23, 640–643. doi: 10.1002/adma.201003560
Liang, Y., Yoo, H. D., Li, Y., Shuai, J., Calderon, H. A., Robles Hernandez, F. C., et al. (2015). Interlayer-expanded molybdenum disulfide nanocomposites for electrochemical magnesium storage. Nano Lett. 15, 2194–2202. doi: 10.1021/acs.nanolett.5b00388
Ling, C., Banerjee, D., Song, W., Zhang, M., and Matsui, M. (2012). First-principles study of the magnesiation of olivines: redox reaction mechanism, electrochemical and thermodynamic properties. J. Mater. Chem. 22:13517. doi: 10.1039/c2jm31122d
Lipson, A. L., Han, S.-D., Kim, S., Pan, B., Sa, N., Liao, C., et al. (2016). Nickel hexacyanoferrate, a versatile intercalation host for divalent ions from nonaqueous electrolytes. J. Power Sources 325, 646–652. doi: 10.1016/j.jpowsour.2016.06.019
Liu, B., Luo, T., Mu, G., Wang, X., Chen, D., and Shen, G. (2013). Rechargeable Mg-ion batteries based on WSe2 nanowire cathodes. ACS Nano 7, 8051–8058. doi: 10.1021/nn4032454
Makino, K., Katayama, Y., Miura, T., and Kishi, T. (2001). Electrochemical insertion of magnesium to Mg0.5Ti2(PO4)3. J. Power Sources 99, 66–69. doi: 10.1016/S0378-7753(01)00480-3
Nam, K. W., Kim, S., Lee, S., Salama, M., Shterenberg, I., Gofer, Y., et al. (2015). The high performance of crystal water containing manganese birnessite cathodes for magnesium batteries. Nano Lett. 15, 4071–4079. doi: 10.1021/acs.nanolett.5b01109
Novak, P., Imhof, R., and Haas, O. (1999). Magnesium insertion electrodes for rechargeable nonaqueous batteries — a competitive alternative to lithium? Electrochim. Acta 45, 351–367. doi: 10.1016/S0013-4686(99)00216-9
NuLi, Y., Guo, Z., Liu, H., and Yang, J. (2007). A new class of cathode materials for rechargeable magnesium batteries: organosulfur compounds based on sulfur–sulfur bonds. Electrochem. Commun. 9, 1913–1917. doi: 10.1016/j.elecom.2007.05.009
NuLi, Y., Zheng, Y., Wang, Y., Yang, J., and Wang, J. (2011). Electrochemical intercalation of Mg2+ in 3D hierarchically porous magnesium cobalt silicate and its application as an advanced cathode material in rechargeable magnesium batteries. J. Mater. Chem. 21, 12437–12443. doi: 10.1039/c1jm10485c
Orikasa, Y., Masese, T., Koyama, Y., Mori, T., Hattori, M., Yamamoto, K., et al. (2014). High energy density rechargeable magnesium battery using earth-abundant and non-toxic elements. Sci. Rep. 4:5622. doi: 10.1038/srep05622
Pan, B., Huang, J., Feng, Z., Zeng, L., He, M., Zhang, L., et al. (2016a). Polyanthraquinone-based organic cathode for high-performance rechargeable magnesium-ion batteries. Adv. Energy Mater. 6:1600140. doi: 10.1002/aenm.201600140
Pan, B., Zhou, D., Huang, J., Zhang, L., Burrell, A. K., Vaughey, J. T., et al. (2016b). 2,5-Dimethoxy-1,4-Benzoquinone (DMBQ) as organic cathode for rechargeable magnesium-ion batteries. J. Electrochem. Soc. 163, A580–A583. doi: 10.1149/2.0021605jes
Qiang, C., Nuli, Y.-N., Guo, W., Yang, J., Wang, J.-L., and Guo, Y.-G. (2013). PTMA/graphene as a novel cathode material for rechargeable magnesium batteries. Acta Phys. Chim. Sin. 29, 2295–2299. doi: 10.3866/PKU.WHXB201309241
Robba, A., Vizintin, A., Bitenc, J., Mali, G., Arčon, I., Kavčič, M., et al. (2017). Mechanistic study of magnesium-sulfur batteries. Chem. Mater. 29, 9555–9564. doi: 10.1021/acs.chemmater.7b03956
Rong, Z., Malik, R., Canepa, P., Sai Gautam, G., Liu, M., Jain, A., et al. (2015). Materials design rules for multivalent ion mobility in intercalation structures. Chem. Mater. 27, 6016–6021. doi: 10.1021/acs.chemmater.5b02342
Sai Gautam, G., Canepa, P., Abdellahi, A., Urban, A., Malik, R., and Ceder, G. (2015). The intercalation phase diagram of Mg in V2O5 from first-principles. Chem. Mater. 27, 3733–3742. doi: 10.1021/acs.chemmater.5b00957
Sai Gautam, G., Canepa, P., Richards, W. D., Malik, R., and Ceder, G. (2016). Role of Structural H2O in intercalation electrodes: the case of Mg in nanocrystalline xerogel-V2O5. Nano Lett. 16, 2426–2431. doi: 10.1021/acs.nanolett.5b05273
Sano, H., Senoh, H., Yao, M., Sakaebe, H., and Kiyobayashi, T. (2012). Mg2+ storage in organic positive-electrode active material based on 2,5-dimethoxy-1,4-benzoquinone. Chem. Lett. 41, 1594–1596. doi: 10.1246/cl.2012.1594
Schon, T. B., McAllister, B. T., Li, P.-F., and Seferos, D. S. (2016). The rise of organic electrode materials for energy storage. Chem. Soc. Rev. 45, 6345–6404. doi: 10.1039/C6CS00173D
Senoh, H., Sakaebe, H., Sano, H., Yao, M., Kuratani, K., Takeichi, N., et al. (2014). Sulfone-based electrolyte solutions for rechargeable magnesium batteries using 2,5-Dimethoxy-1,4-benzoquinone positive electrode. J. Electrochem. Soc. 161, A1315–A1320. doi: 10.1149/2.0531409jes
Senoh, H., Sakaebe, H., Tokiwa, H., Uchida, M., Sano, H., Yao, M., et al. (2015). Charge-discharge performance of rechargeable organic-magnesium batteries using glyme-based electrolytes. ECS Trans. 69, 33–39. doi: 10.1149/06919.0033ecst
Senoh, H., Yao, M., Sakaebe, H., Yasuda, K., and Siroma, Z. (2011). A two-compartment cell for using soluble benzoquinone derivatives as active materials in lithium secondary batteries. Electrochim. Acta 56, 10145–10150. doi: 10.1016/j.electacta.2011.08.115
Shterenberg, I., Salama, M., Yoo, H. D., Gofer, Y., Park, J.-B., Sun, Y.-K., et al. (2015). Evaluation of (CF3SO2)2N− (TFSI) based electrolyte solutions for Mg batteries. J. Electrochem. Soc. 162, 7118–7128. doi: 10.1149/2.0161513jes
Song, Z., Qian, Y., Gordin, M. L., Tang, D., Xu, T., Otani, M., et al. (2015). Polyanthraquinone as a reliable organic electrode for stable and fast lithium storage. Angew. Chem. Int. Ed. 54, 13947–13951. doi: 10.1002/anie.201506673
Song, Z., Zhan, H., and Zhou, Y. (2009). Anthraquinone based polymer as high performance cathode material for rechargeable lithium batteries. Chem. Commun. 4, 448–450. doi: 10.1039/B814515F
Spahr, M. E., Novák, P., Haas, O., and Nesper, R. (1995). Electrochemical insertion of lithium, sodium, and magnesium in molybdenum(VI) oxide. J. Power Sources 54, 346–351. doi: 10.1016/0378-7753(94)02099-O
Sun, X., Bonnick, P., Duffort, V., Liu, M., Ceder, G., and Nazar, L. F. (2016a). A high capacity thiospinel cathode for Mg batteries. Energy Environ. Sci. 9, 2273–2277. doi: 10.1039/C6EE00724D
Sun, X., Bonnick, P., and Nazar, L. F. (2016b). Layered TiS2 positive electrode for Mg batteries. ACS Energy Lett. 1, 297–301. doi: 10.1021/acsenergylett.6b00145
Sun, X., Du, V., Mehdi, B. L., Browning, N. D., and Nazar, L. F. (2016c). Investigation of the mechanism of Mg insertion in birnessite in nonaqueous and aqueous rechargeable Mg-ion batteries. Chem. Mater. 28, 534–542. doi: 10.1021/acs.chemmater.5b03983
Tao, Z. L., Xu, L. N., Gou, X. L., and Yuan, H. T. (2004). TiS2 nanotubes as the cathode materials of Mg-ion batteries. Chem. Commun. 2080–2081. doi: 10.1039/b403855j
Tepavcevic, S., Liu, Y., Zhou, D., Lai, B., Maser, J., Zuo, X., et al. (2015). Nanostructured layered cathode for rechargeable Mg-ion Batteries. 9, 8194–8205. doi: 10.1021/acsnano.5b02450
Tutusaus, O., Mohtadi, R., Singh, N., Arthur, T. S., and Mizuno, F. (2017). Study of electrochemical phenomena observed at the Mg metal/electrolyte interface. ACS Energy Lett. 2, 224–229. doi: 10.1021/acsenergylett.6b00549
Vinayan, B. P., Zhao-Karger, Z., Diemant, T., Chakravadhanula, V. S. K., Schwarzburger, N. I., Cambaz, M. A., et al. (2016). Performance study of magnesium-sulfur battery using a graphene based sulfur composite cathode electrode and a non-nucleophilic Mg electrolyte. Nanoscale 8, 3296–3306. doi: 10.1039/C5NR04383B
Vizintin, A., Bitenc, J., Kopač Lautar, A., Pirnat, K., Grdadolnik, J., Stare, J., et al. (2018). Probing electrochemical reactions in organic cathode materials via in operando infrared spectroscopy. Nat. Commun. 9:661. doi: 10.1038/s41467-018-03114-1
Wang, Y., Richards, W. D., Ong, S. P., Miara, L. J., Kim, J. C., Mo, Y., et al. (2015). Design principles for solid-state lithium superionic conductors. Nat. Mater. 14, 1026–1031. doi: 10.1038/nmat4369
Wu, J., Gao, G., Wu, G., Liu, B., Yang, H., Zhou, X., et al. (2014). Tavorite-FeSO4 F as a potential cathode material for Mg ion batteries: a first principles calculation. Phys. Chem. Chem. Phys. 16, 22974–22978. doi: 10.1039/C4CP03176H
Xu, H., Zhang, Z., Cui, Z., Du, A., Lu, C., Dong, S., et al. (2017). Strong anion receptor-assisted boron-based Mg electrolyte with wide electrochemical window and non-nucleophilic characteristic. Electrochem. Commun. 83, 72–76. doi: 10.1016/j.elecom.2017.09.001
Yu, X., and Manthiram, A. (2016). Performance enhancement and mechanistic studies of magnesium-sulfur cells with an advanced cathode structure. ACS Energy Lett. 1, 431–437. doi: 10.1021/acsenergylett.6b00213
Zhang, R., and Ling, C. (2016). Unveil the chemistry of olivine FePO4 as magnesium battery cathode. ACS Appl. Mater. Interfaces 8, 18018–18026. doi: 10.1021/acsami.6b03297
Zhang, R. G., Mizuno, F., and Ling, C. (2015). Fullerenes: non-transition metal clusters as rechargeable magnesium battery cathodes. Chem. Commun. 51, 1108–1111. doi: 10.1039/C4CC08139K
Zhang, Z., Cui, Z., Qiao, L., Guan, J., Xu, H., Wang, X., et al. (2017). Novel design concepts of efficient Mg-ion electrolytes toward high-performance magnesium–selenium and magnesium–sulfur batteries. Adv. Energy Mater. 7:1602055. doi: 10.1002/aenm.201602055
Zhao-Karger, Z., Gil Bardaji, M. E., Fuhr, O., and Fichtner, M. (2017). A new class of non-corrosive, highly efficient electrolytes for rechargeable magnesium batteries. J. Mater. Chem. A 5, 10815–10820. doi: 10.1039/C7TA02237A
Zhao-Karger, Z., Liu, R., Dai, W., Li, Z., Diemant, T., Vinayan, B. P., et al. (2018). Toward highly reversible magnesium-sulfur batteries with efficient and practical Mg[B(Hfip)4]2Electrolyte. ACS Energy Lett. 3, 2005–2013. doi: 10.1021/acsenergylett.8b01061
Zhao-Karger, Z., Zhao, X., Fuhr, O., and Fichtner, M. (2013). Bisamide based non-nucleophilic electrolytes for rechargeable magnesium batteries. RSC Adv. 3:16330. doi: 10.1039/c3ra43206h
Keywords: batteries, magnesium, cathode, sulfur, organic redox compounds
Citation: Bitenc J and Dominko R (2018) Opportunities and Challenges in the Development of Cathode Materials for Rechargeable Mg Batteries. Front. Chem. 6:634. doi: 10.3389/fchem.2018.00634
Received: 05 October 2018; Accepted: 06 December 2018;
Published: 18 December 2018.
Edited by:
Pieremanuele Canepa, National University of Singapore, SingaporeReviewed by:
Masaki Matsui, Kobe University, JapanCopyright © 2018 Bitenc and Dominko. This is an open-access article distributed under the terms of the Creative Commons Attribution License (CC BY). The use, distribution or reproduction in other forums is permitted, provided the original author(s) and the copyright owner(s) are credited and that the original publication in this journal is cited, in accordance with accepted academic practice. No use, distribution or reproduction is permitted which does not comply with these terms.
*Correspondence: Robert Dominko, cm9iZXJ0LmRvbWlua29Aa2kuc2k=
Disclaimer: All claims expressed in this article are solely those of the authors and do not necessarily represent those of their affiliated organizations, or those of the publisher, the editors and the reviewers. Any product that may be evaluated in this article or claim that may be made by its manufacturer is not guaranteed or endorsed by the publisher.
Research integrity at Frontiers
Learn more about the work of our research integrity team to safeguard the quality of each article we publish.