- 1Department of Chemistry, University of Otago, Dunedin, New Zealand
- 2MacDiarmid Institute for Advanced Materials and Nanotechnology, Wellington, New Zealand
- 3Department of Pharmacology and Toxicology, University of Otago, Dunedin, New Zealand
New bis-quinoline (Lq) and bis-isoquinoline-based (Liq) ligands have been synthesized, along with their respective homoleptic [Pd2(Lq or Liq)4]4+ cages (Cq and Ciq). The ligands and cages were characterized by 1H, 13C and diffusion ordered (DOSY) NMR spectroscopies, high resolution electrospray ionization mass spectrometry (HR-ESIMS) and in the case of the bis-quinoline cage, X-ray crystallography. The crystal structure of the Cq architecture showed that the [Pd2(Lq)4]4+ cage formed a twisted meso isomer where the [Pd(quinoline)4]2+ units at either end of the cage architecture adopt the opposite twists (left and right handed). Conversely, Density Functional Theory (DFT) calculations on the Ciq cage architecture indicated that a lantern shaped conformation, similar to what has been observed before for related [Pd2(Ltripy)4]4+ systems (where Ltripy = 2,6-bis(pyridin-3-ylethynyl)pyridine), was generated. The different cage conformations manifest different properties for the isomeric cages. The Ciq cage is able to bind, weakly in acetonitrile, the anticancer drug cisplatin whereas the Cq architecture shows no interaction with the guest under the same conditions. The kinetic robustness of the two cages in the presence of Cl− nucleophiles was also different. The Ciq cage was completely decomposed into free Liq and [Pd(Cl)4]2− within 1 h. However, the Cq cage was more long lived and was only fully decomposed after 7 h. The new ligands (Liq and Lq) and the Pd(II) cage architectures (Ciq and Cq) were assessed for their cytotoxic properties against two cancerous cell lines (A549 lung cancer and MDA-MB-231 breast cancer) and one non-cancerous cell line (HDFa skin cells). It was found that Lq and Cq were both reasonably cytotoxic (IC50S ≈ 0.5 μM) against A549, while Ciq was slightly less active (IC50 = 7.4 μM). Liq was not soluble enough to allow the IC50 to be determined against either of the two cancerous cell lines. However, none of the molecules showed any selectivity for the cancer cells, as they were all found to have similar cytotoxicities against HDFa skin cells (IC50 values ranged from 2.6 to 3.0 μM).
Introduction
Metallosupramolecular architectures (MSAs) (Cook and Stang, 2015) have been attracting increasing attention over the past two decades due to their many potential applications including catalysis (Yoshizawa et al., 2009; Yoshizawa and Fujita, 2010; Martí-Centelles et al., 2018), storage (Mal et al., 2009), and sensing (Wang et al., 2011). Inspired by the success of cisplatin and other metallodrugs (Mjos and Orvig, 2014) there is emerging interest in exploiting MSAs for biomedical purposes (Cook et al., 2013; Therrien, 2015; Casini et al., 2017; Zhou et al., 2017). Several groups have examined MSAs as drug delivery vectors (Therrien et al., 2008; Schmitt et al., 2012; Yi et al., 2012; Zheng et al., 2015; Samanta et al., 2016, 2017; Bhat et al., 2017; Xu et al., 2017; Wang J.-F. et al., 2018; Yue et al., 2018). Additionally, MSAs have been shown to bind DNA (Oleksy et al., 2006; Garci et al., 2017; Zhao et al., 2017) and RNA (Phongtongpasuk et al., 2013; Malina et al., 2015), interact with proteins (Li et al., 2014; Mitchell et al., 2017) and have anticancer (Hotze et al., 2008; Faulkner et al., 2014; Grishagin et al., 2014; Dubey et al., 2015; Zheng et al., 2016; Allison et al., 2018) and antibacterial (Richards et al., 2009; Howson et al., 2012; Wang H. et al., 2018) properties.
Since the pioneering work of McMorran and Steel (McMorran and Steel, 1998) interest in [M2(L)4]n+ cage-type structures has burgeoned (Schmidt et al., 2014). Some time ago now we reported that a [Pd2(Ltripy)4]4+ cage (where Ltripy = 2,6-bis(pyridin-3-ylethynyl)pyridine) could host two molecules of the anticancer drug cisplatin within the cavity of the cage (Lewis et al., 2012), and thus had potential as a drug delivery vector. Disappointingly, the binding event, which was governed mainly by hydrogen bonding interactions, was weak (Preston et al., 2015). The host-guest complex formed in acetonitrile (CH3CN) and dimethylformamide (DMF) but unfortunately, in more hydrogen bond competitive solvents such as water and dimethyl sulfoxide (DMSO) no host-guest interaction was observed. Additionally, the parent Pd(II) based cage decomposed rapidly in the presence of nucleophiles (Lewis et al., 2012; McNeill et al., 2015). Thus, in order to exploit these [Pd2(L)4]4+ cages as cisplatin delivery vehicles these issues need to be addressed. We and others have examined a range of modifications to the parent [Pd2(L)4]4+ cage system in order to improve the solubility (Lewis and Crowley, 2014; Preston et al., 2015; Han et al., 2017) and other properties (Lewis et al., 2013, 2014; Kaiser et al., 2016; Schmidt et al., 2016a) of the cage. Efforts have also been made to enhance the strength of the host-guest interaction (Kim et al., 2015) and the stability of the cages in the presence of biologically revelant nucleophiles (Preston et al., 2016). However, while some improvements have been made these [Pd2(L)4]4+ cages still require further modifcations in order to be useful drug delivery vectors.
The [Pd2(L)4]4+ cages have also been examined for their cytotoxic properties. We showed that the parent [Pd2(Ltripy)4]4+ was modestly cytotoxic (IC50 values range from 40 to 70 μM) against a range of cancer cell lines but was less active than related bis-1,2,3-triazole [Pd2(L)4]4+ helicates (McNeill et al., 2015). We also examined the cytotoxicity of related amino substituted [Pd2(L)4]4+ cages against the same panel of cancer cells and found that they exhibited similar cytotoxic properties as the parent systems (Preston et al., 2016). Casini, Kühn and co-workers (Kaiser et al., 2016; Schmidt et al., 2016b) have measured the cytotoxicity of a series of related [Pd2(L)4]4+ cages and observed similar IC50 values (10–70 μM). Additionally, they have measured the cytotoxicity of mixtures of the cages and cisplatin and unsurprisingly have found that those mixtures are more cytotoxic than cage alone (IC50 = 2–13 μM). Yoshizawa, Ahmedova and co-workers have also found that [M2(L)4]4+ (M = Pd2+ or Pt2+) cages with similar, but more hydrophobic, dipyridyl anthracenyl ligands (Lanthracene) display high anticancer activity (IC50 values range from 0.9 to 37.4 μM against HL-60, HL-60/Dox, HT-29, T-24, SKW-3 cancer cell lines) (Ahmedova et al., 2016; Anife et al., 2016).
The majority of the [Pd2(L)4]4+ cages examined to date feature pyridyl donors, as part of our efforts to improve the biological properties of these systems herein we describe the use of isoquinoline and quinoline-derived ligands for the assembly of two new [Pd2(L)4]4+ cages. While is well-known that isoquinoline and quinoline ligands can bind with palladium(II) and platinum(II) (Bondy et al., 2004) their use as donor systems in ligands for the generation MSAs has not been extensively explored (Bloch et al., 2016, 2017). These quinoline derived systems feature different electronic and steric properties compared to the parent pyridyl systems thus we also examine the effect these changes have on the host-guest chemistry with cisplatin, the stability of the cages in the presence of nucleophiles and the antiproliferative properties of the cages.
Results and Discussion
The synthesis of the new quinoline (Lq) and isoquinoline (Liq) based ligands was facile (Supplementary Material). Using sequential Sonogashira carbon-carbon cross coupling reactions from commercially available building blocks the ligands were generated in good yields (Lq = 86% and Liq = 78%). 1H and 13C NMR spectroscopic data were consistent with the formation of the ligands which was supported by high-resolution electrospray mass spectrometry (HR-ESIMS) (Figure 1 and Supplementary Material). Peaks corresponding to protonated and sodiated ligand were observed at m/z = 382.1320 and 404.1132, respectively, for Liq and similar peaks were observed for Lq (Supplementary Material).
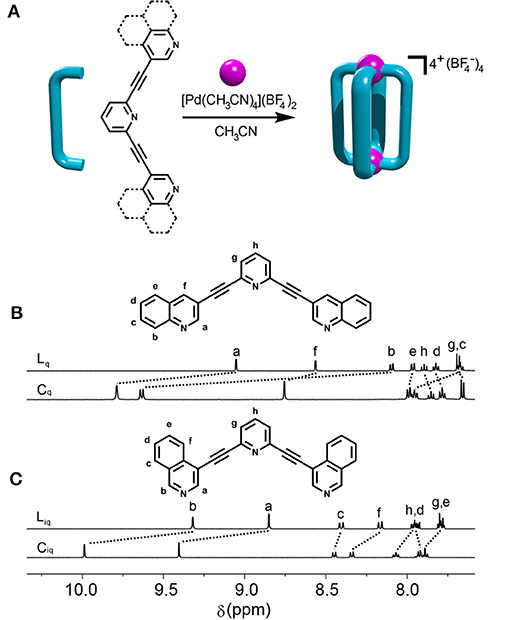
Figure 1. (A) General scheme for formation of [Pd2(L)4]4+ cages (Cq and Ciq) and partial 1H NMR spectra (400 MHz, CD3CN, 298 K) of (B) Lq and Cq, and (C) Liq and Ciq.
With the ligands in hand, the complexation with [Pd(CH3CN)4](BF4)2 in acetonitrile was examined (Figure 1). The cage formation was monitored using 1H NMR spectroscopy (CD3CN, 298 K) and showed that mixing [Pd(CH3CN)4](BF4)2 and the Liq ligand at room temperature (RT) in a 1:2 ratio led to the rapid (< 2 min) and quantitative formation of the expected Ciq cage (Figure 1), similar to what was observed with the parent Ltripy system (Lewis et al., 2012). Interestingly, the behavior of Lqwith [Pd(CH3CN)4](BF4)2 at room temperature was very different. After 5 min at RT the reaction mixture displayed multiple proton resonances, none of which were due to free ligand, consistent with the formation of a mixture of different cage isomers. The reaction was monitored using 1H NMR spectroscopy for 24 h at RT however little to no changes were observed after the first hour and the spectrum still displayed multiple proton resonances. A 1H DOSY experiment (CD3CN, 298 K) on the mixture showed that all the different proton resonances had the same diffusion co-efficient consistent with the postulate that the reaction mixture contains a series of cage isomers (Supplementary Material).
The assembly reaction between Lqwith [Pd(CH3CN)4](BF4)2 was then carried out at 65°C, in CD3CN and again monitored using 1H NMR spectroscopy (Figure 2). After 5 min the same complicated series of proton resonance were observed. However, with continued heating this slowly resolved into a single series of resonances (after 7 h), consistent with the formation of a single cage isomer (Figure 2). Pleasingly, both cages (Ciq and Cq) could be isolated by adding diethyl ether into the acetonitrile reaction mixtures providing the cages as colorless/tan precipitates in 88% (Ciq) or 92% (Cq) yield, respectively. 1H NMR spectroscopy (CD3CN, 298 K) exhibited the expected downfield shifts of the signals pertaining to protons Ha, Hb and Hf as well as the anticipated downfield shifts of the rest of the isoquinoline and quinoline protons resonances (Figure 1). HR-ESIMS data also supported the formation of the cages, showing ions corresponding to the loss of 2, 3 and 4 tetrafluoroborate () counterions (m/z = 956.1610 (2+), 608.4424 (3+), and 434.5832 (4+), Supplementary Material). 1H DOSY experiments (CD3CN, 298 K) on the ligands (Diffusion coefficients (D) = 13.1 (Lq) and 15.0 (Liq) x 10−10 m2 s−1) and cages (D = 4.1 (Cq) and 4.3 (Ciq) × 10−10 m2 s−1 were also consistent with the formation of the larger [Pd2(L)4]4+ cages (Supplementary Material).
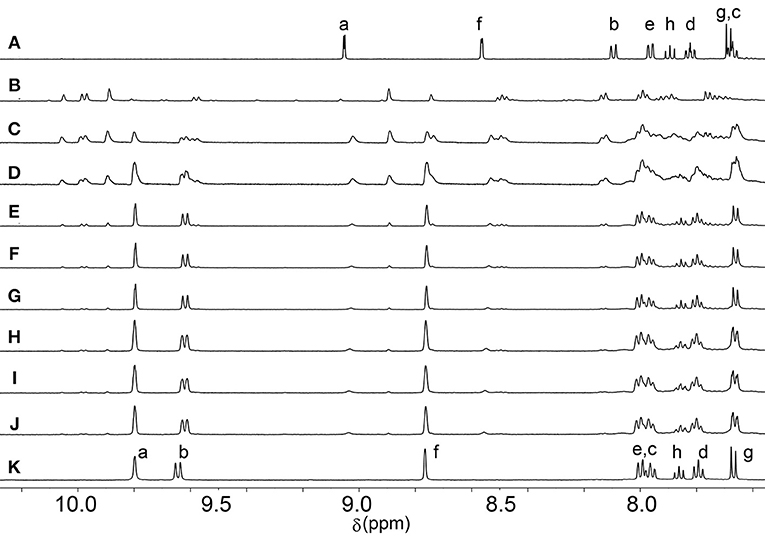
Figure 2. Partial 1H NMR (500 MHz, CD3CN, 338 K) spectra showing the formation of Cq over time at 65°C. (A) Lq, (B) initial complexation (t = 0), (C) t = 30 min, (D) t = 1 h, (E) t = 2 h, (F) t = 3 h, (G) t = 4 h, (H) t = 5 h, (I) t = 6 h, (J) t = 7 h, (K) isolated Cq.
Crystals of Cq suitable for X-ray diffraction formed during the cooling of an acetonitrile solution of the cage from 65°C to room temperature. The structure was solved in the tetragonal space group P4/mnc with the asymmetric unit containing one eighth of the cage and one quarter of a counterion (Figure 3 and Supplementary Material). The other anions and some acetonitrile molecules could not be modeled sensibly thus the SQUEEZE routine was employed to account for the diffuse electron density (Supplementary Material). The data revealed the expected [Pd2(Lq)4]4+ cage structure. The Pd-N bond lengths (Pd1-N2 2.045 Å) were similar to what have been previously observed for the related [Pd2(Ltripy)4]4+ cages where the Pd-N bond lengths range from 2.016 to 2.027 Å (Lewis et al., 2012; Lewis and Crowley, 2014). The Lq ligands of the cage are twisted giving a V-shaped conformation where the terminal quinoline and central pyridyl heterocyclic units are not co-planar which is quite different to what was observed with the [Pd2(Ltripy)4]4+ cages. In X-ray structures of the parent [Pd2(Ltripy)4]4+ cages the Ltripy ligands were found in a linear conformation with the heterocyclic units coplanar. The twisting also alters the Pd1-Pd1′ distance within Cq related to the [Pd2(Ltripy)4]4+ cages. The Pd1-Pd1′ distances for the parent [Pd2(Ltripy)4]4+ cages range from 11.49 to 12.24 Å, whereas the Pd1-Pd1′ distance was found to be longer (12.506 Å) suggesting that the Cqcage has a larger cavity despite featuring the same 2,6-diethynylpyridine linker units. The [Pd(quinoline)4]2+ units at the top and bottom of Cq are twisted in opposite directions, the top cationic unit has a right handed twist while the bottom cationic unit has a left handed twist giving an overall meso structure (Figures 3B,C and Supplementary Material). Despite extensive efforts we were unable to obtain X-ray diffraction quality single crystals of Ciq. Thus, to gain further insight into the structure of Ciq we modeled the cage using Density Functional Theory (DFT) calculations (Figures 3D,E). Energy minimization of Ciq (DFT, BP86 def2-SVP, acetonitrile solvation, Supplementary Material) showed that the cage adopted a lantern shape similar to what was previously observed for [Pd2(Ltripy)4]4+ cages (Lewis et al., 2012; Lewis and Crowley, 2014). The calculated Pd – N bond distances (2.049 Å) and the Pd-Pd′ distance (11.758 Å) match well with those observed crystallographically for the related [Pd2(Ltripy)4]4+ cages. The Liq ligand adopts a linear conformation with all the heterocyclic units coplanar. The DFT calculations indicated that the Ciq is structurally very similar to the parent [Pd2(Ltripy)4]4+ cages whereas the Cq is more twisted and provided a cavity of different size and shape to the parent cages and the Ciq system.
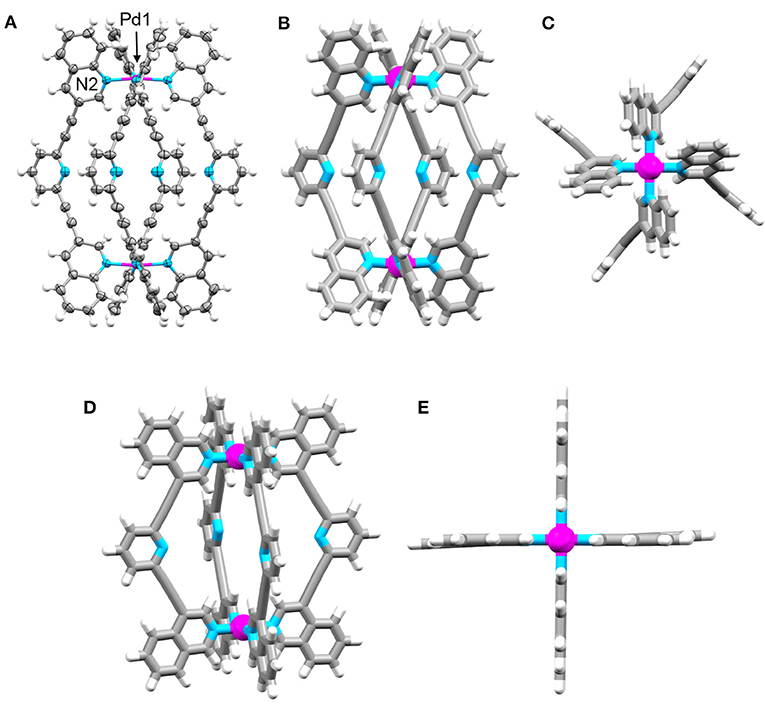
Figure 3. Molecular structures of Cq and Ciq. X-ray structure of Cq: (A) ellipsoid side view, (B) tube side view, and (C) tube top view showing paddle-like array of quinoline panels over palladium(II) center. Solvent molecules and counterions have been omitted for clarity. Ellipsoids are shown at 50% probability. DFT optimized (BP86 def2-SVP) model of Ciq; (D) side view and (E) top view showing lantern-shaped structure. Colors: carbon gray, nitrogen blue, palladium magenta, hydrogen white.
We and others have previously shown that other similar [Pd2(Ltripy)4]4+ cages can encapsulate cisplatin through hydrogen bonding interactions in CH3CN and DMF solvents (Lewis et al., 2012, 2013, 2014; Kaiser et al., 2016; Preston et al., 2016, 2017; Schmidt et al., 2016b). Therefore, we examined the ability of Ciq and Cq to interact with cisplatin in CH3CN using 1H NMR spectroscopy. Addition of an excess of cisplatin to a CD3CN solution of the Ciq cage resulted in a downfield shift and broadening (Δδ = 0.03 ppm) of the internally directed cage proton Ha (Figures 4A,B) indicative of cisplatin binding within the cage cavity, albeit weakly. A similar 1H NMR experiment was carried out with the Cq cage (Figures 4C,D). However, with the Cq cage no shifts were observed for any of the cage proton resonances in the presence of an excess of cisplatin suggesting that the more twisted Cq cage does not interact with the anticancer agent. The behavior was similar to what has been observed with a related twisted [Pd2(L2Atripy)4]4+ cage (where L2Atripy = 2,6-bis[2-(6-amino-3-pyridinyl)ethynyl]-4-pyridinemethanol) (Preston et al., 2016). The [Pd2(L2Atripy)4]4+ cage did not bind cisplatin in DMF solvent and the lack of binding was ascribed to the twisted cage cavity which was not as preorganised as those of the related lantern shaped [Pd2(Ltripy)4]4+ cages. Presumably the different sized cavity and different spatial arrangement of the hydrogen bond donors and acceptors caused by the twisting observed in the crystal structure of Cq impedes the cisplatin-Cq interaction in this case.
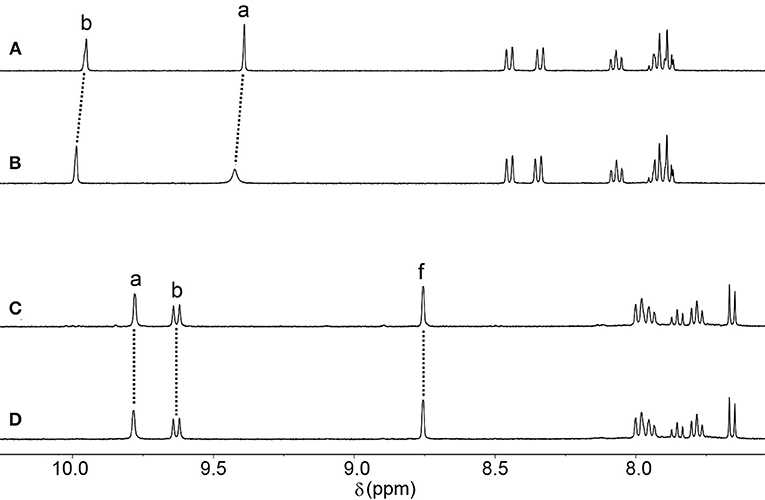
Figure 4. Partial 1H NMR (500 MHz, CD3CN, 298 K) stacked plot of (A) Ciq (B) Ciq + cisplatin (10 eq.) (C) Cq, and (D) Cq + cisplatin (10 eq.).
The kinetic robustness of the related [Pd2(Ltripy)4]4+ architectures in the presence of common biological nucleophiles (chloride (Cl−), histidine and cysteine) has been determined using 1H NMR competition experiments. When the parent [Pd2(Ltripy)4]4+ architectures were treated with 8 equivalents of tetrabutylammonium chloride the pyridyl substituted cages were rapidly and quantitatively decomposed (in < 5 min). To examine the effect of substituting the pyridyl donor units for quinoline heterocycles time-course 1H NMR competition experiments were carried out in d6-DMSO where 2 mM solutions of each cage (Cqor Ciq) were treated with 8 equivalents of tetrabutylammonium chloride at 298 K (Figure 5 and Supplementary Material). Within 30 s of adding Cl− to the Ciq cage, there were multiple species observed in the 1H NMR spectrum. These were attributed to the Ciq cage, [Cl⊂Ciq]3+, the [Pd2(Liq)2Cl4] macrocycle and free ligand based on our own previous results (Preston et al., 2015) and related literature. After 50 min, only uncoordinated ligand was visible in the 1H NMR spectrum (Supplementary Material).
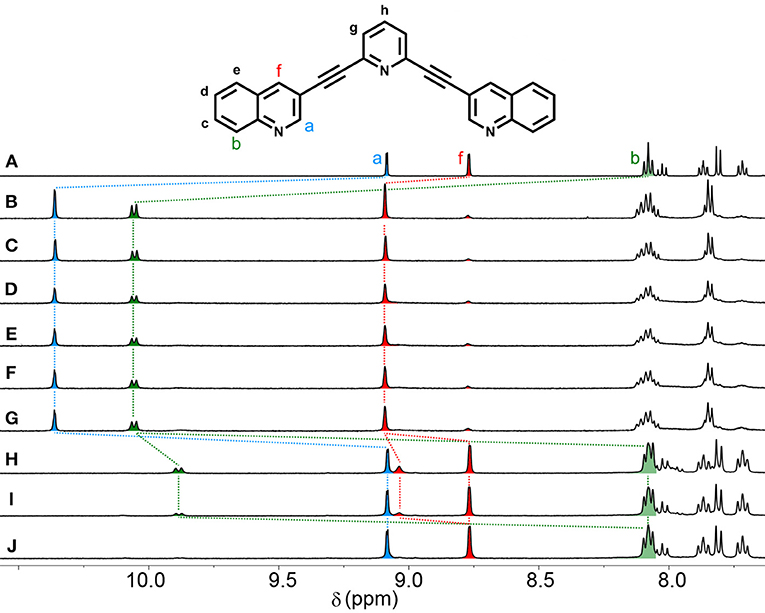
Figure 5. Partial 1H NMR (500 MHz, d6-DMSO, 298 K) spectra showing the stability of Cq in the presence of 8 eq. Cl− anions. (A) Lq, (B) Cq, (C) Cq with 8 eq. Cl− (t = 2 min), (D) t = 10 min, (E) t = 20 min, (F) t = 30 min, (G) t = 1 h, (H) t = 3 h, (I) t = 5 h, (J) t = 7 h.
Under the same conditions, Cq was stable for 1 h before showing signs of decomposition (Figure 5). After 3 h, there was no evidence of the Cq cage, and the 1H NMR spectrum displayed peaks corresponding to free ligand and a second metal-containing species, which based on the observed chemical shifts was most likely the neutral [Pd2(Lq)2Cl4] macrocycle (Figure 5H). This degradation behavior has been seen before with the [Pd2(Ltripy)4]4+ system in DMF (Preston et al., 2015). After 7 h, only free ligand could observed in the 1H NMR spectrum indicating that all the ligand containing metal complexes had been completely decomposed into [Pd(Cl)4]2−(Figure 5J).
In comparison to the previously reported [Pd2(Ltripy)4]4+ cage (τ1/2 = 2 min), the isoquinoline cage displayed an identical half-life (τ1/2 = 2 min), whereas the quinoline system was considerably more robust (τ1/2 = 2 h). Presumably the observed results reflect the different steric profiles of the two quinoline substituted cages (Cqor Ciq). The Cq cage has the quinoline moieties protecting the external face of the palladium, providing more impediment to nucleophilic attack from that face (Figure 6). The Ciq does not feature the same steric impediment as the benzene units of the isoquinoline heterocycles do not block the top face of the Ciq cage as much as they do in the quinoline Cq(Figure 6).
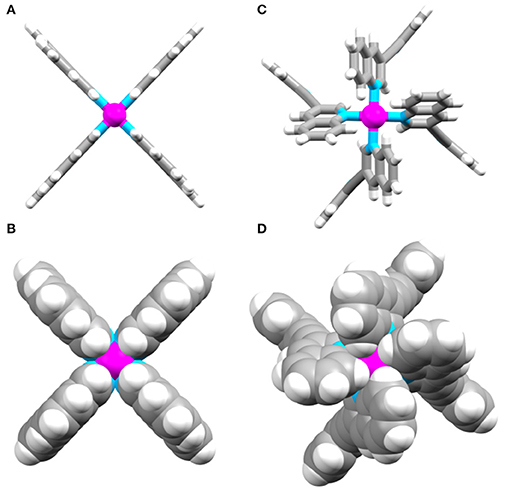
Figure 6. Top down views of (A) and (B) the DFT optimized model of Ciq, and (C) and (D) the X-ray structure of Cq.
To assess biological activity, the cytotoxic effect (as half-maximal inhibitory concentrations (IC50)) of the ligands and cages were determined against three different cell lines: cisplatin resistant MDA-MB-231 (breast cancer) (Lehmann et al., 2011), A549 (lung cancer) and non-cancerous primary cells: adult human dermal fibroblasts (HDFa) (Table 1 and Supplementary Material). The ligands Lq and Liq exhibited limited solubility, and so data above the concentration of 1 μM was unattainable. Below this threshold, Liq displayed minimal cytotoxic activity against both cell lines, while Lq was shown to be cytotoxic against A549 (IC50 = 0.5 μM). Both cages were observed to be cytotoxic against the malignant cell lines, with Cq showing the same level of toxicity as its ligand against lung cancer cells (IC50 = 0.5 μM). Cq was slightly less cytotoxic against MDA-MB-231 (IC50 = 1.7 μM), whereas Ciq was less cytotoxic than the quinoline analog, with the IC50 values ranging from 4.0 to 7.4 μM against the cancer cells. Both quinoline cages were found to be considerably more active than the related parent [Pd2(Ltripy)4]4+ cage system (IC50 = 41.4 and 56.7 μM against A549 and MDA-MB-231, respectively) (McNeill et al., 2015). The quinoline cages were also more active than cisplatin against the two cancer lines examined (cisplatin IC50 values = 41.2 and 9.4 μM, against MDA-MB-231 and A549, respectively) (Lo et al., 2015; McNeill et al., 2015). The quinoline cages Cq and Ciq were more cytotoxic than all the [Pd2(Ltripy)4]4+ cage systems reported in the literature (IC50 values for the Ltripy based systems ranged from 10 to 100 μM) (McNeill et al., 2015; Kaiser et al., 2016; Schmidt et al., 2016b). Additionally, Cq was also more active, albeit against different cancer cell lines (HL-60, HL-60/Dox, HT-29, T-24, SKW-3), than the hydrophobic [Pd2(Lanthracene)4]4+ cages of Yoshizawa and Ahmedova (IC50 values ranged from 0.9 to 37.4 μM) (Ahmedova et al., 2016; Anife et al., 2016). Cq was also more cytotoxic than a hydrophobic bis-hexyl-1,2,3-triazole substituted [Pd2(Lhextrz)4]4+ helicate, Chextrz, we developed previously (IC50 values = 6.9 and 6.0 μM against A549 and MDA-MB-231, respectively) (McNeill et al., 2015). We presume that the favorable combination of high hydrophobicity and the kinetic robustness against biological nucleophiles leads to the higher observed activity of Cq relative to the other [Pd2(L)4]4+ architectures. Disappointingly, neither of the cages (Cq and Ciq) showed any selectivity for the cancer cells, they were all found to have similar cytotoxicity against HDFa skin cells (IC50 values ranged from 2.6 to 3.0 μM).
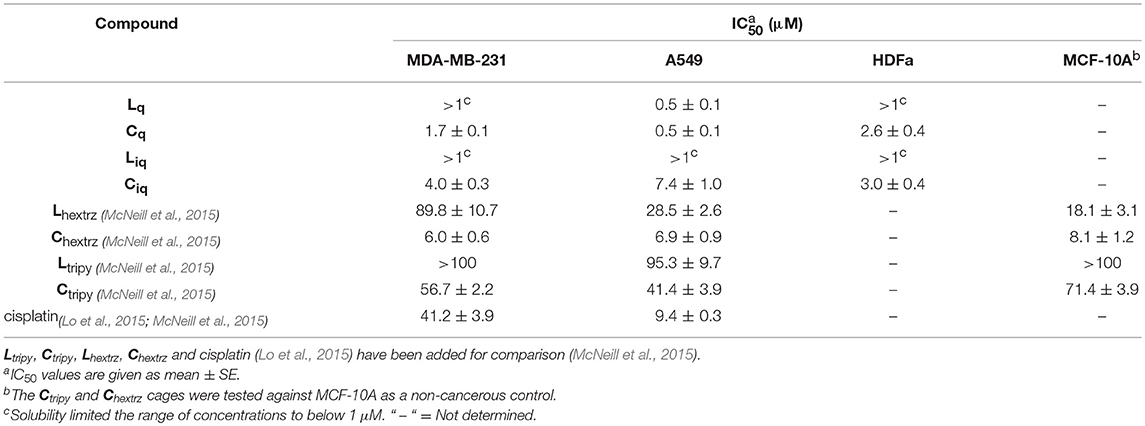
Table 1. Half-maximal inhibitory concentrations (IC50) of ligands Lq and Liq, and cages Cq and Ciq architectures at 24 h.
Conclusion
We have herein reported the synthesis, characterization, cisplatin binding, kinetic robustness and cytotoxicity of two new bis-isoquinoline and bis-quinoline derived [Pd2(L)4]4+ cage complexes. The crystal structure of Cq architecture showed that the [Pd2(L)4]4+ cage formed a twisted meso isomer where the [Pd(quinoline)4]2+ units at either end of the cage architecture adopt the opposite twists (left and right handed). Conversely, Density Functional Theory (DFT) calculations on the Ciq cage architecture indicated that a lantern shaped conformation similar to what has been observed before for related [Pd2(Ltripy)4]4+ systems was generated. The different cage conformations resulted in different properties for the isomeric cages. The Ciq cage is able to bind, weakly in acetonitrile, the anticancer drug cisplatin whereas the Cq architecture shows no interaction with the guest under the same conditions. The kinetic robustness of the two cages in the presence of Cl− nucleophiles was also different. The Ciq cage was completely decomposed into free Liq and [Pd(Cl)4]2−within 1 h. However, the Cq cage was more long lived and was only fully decomposed after 7 h. The ligands (Liq and Lq) and cages (Ciq and Cq) were assessed for their cytotoxic properties against two cancerous cell lines (A549 lung cancer cells and MDA-MB-231 breast cancer cells) and one non-cancerous cell line (HDFa skin cells). It was found that Lq and Cq were both reasonably cytotoxic against A549, while Ciq was slightly less active. The higher observed cytotoxicity of Cq relative to the other [Pd2(L)4]4+ architectures was presumed to be due the favorable combination of high hydrophobicity and the kinetic robustness against biological nucleophiles. However, none of the new molecules showed any selectivity for cancer cells, they were all found to have similar cytotoxicity against HDFa skin cells. A range of [Pd2(L)4]4+ cage systems have now been shown to be cytotoxic. However, in order to advance this class of MSA as anticancer agents more in depth mode of action/mechanistic studies on the origins of the cytotoxic activity are required. Studies to this effect are now underway.
Author Contributions
RV and JC conceived the idea, analyzed the data and wrote the manuscript. RV and LG conducted the synthesis. RV and DP conducted stability studies. RV, DP, and JJ conducted cytotoxicity studies. GG oversaw the cytotoxicity studies and analyzed the data. All authors provided feedback on the manuscript drafts and approved the submission.
Funding
JC thanks the University of Otago, Department of Chemistry and the MacDiarmid Institute for funding. RV was supported by a Claude McCarthy Fellowship. All the authors thank Charlotte Dobson, Pauline Lane and Oceanbridge for their kind donations which supported this work.
Conflict of Interest Statement
The authors declare that the research was conducted in the absence of any commercial or financial relationships that could be construed as a potential conflict of interest.
Acknowledgments
RV and JJ acknowledge the University of Otago for doctoral stipends. Dr. Anna Garden and Dr. David McMorran are thanked for useful discussions. Professor Sally McCormick, Department of Biochemistry, University of Otago is thanked for kindly providing a sample of the HDFa skin cells. The authors acknowledge the contribution of the NeSI high performance computing facilities to the results of this research. New Zealand's national facilities are provided by the New Zealand eScience Infrastructure and funded jointly by NeSI's collaborator institutions and through the Ministry of Business, Innovation and Employment's Research Infrastructure program. URL: https://www.nesi.org.
Supplementary Material
The Supplementary Material for this article can be found online at: https://www.frontiersin.org/articles/10.3389/fchem.2018.00563/full#supplementary-material
References
Ahmedova, A., Mihaylova, R., Momekova, D., Shestakova, P., Stoykova, S., Zaharieva, J., et al. (2016). M2L4 coordination capsules with tunable anticancer activity upon guest encapsulation. Dalton Trans. 45, 13214–13221. doi: 10.1039/C6DT01801G
Allison, S. J., Cooke, D., Davidson, F. S., Elliott, P. I. P., Faulkner, R. A., Griffiths, H. B. S., et al. (2018). Ruthenium-containing linear helicates and mesocates with tuneable p53-selective cytotoxicity in colorectal cancer cells. Angew. Chem. Int. Ed. 57, 9799–9804. doi: 10.1002/anie.201805510
Anife, A., Denitsa, M., Masahiro, Y., Pavletta, S., Georgi, M., Munetaka, A., et al. (2016). Anticancer potencies of PtII- and PdII-linked M2L4 coordination capsules with improved selectivity. Chem. Asian J. 11, 474–477. doi: 10.1002/asia.201501238
Bhat, I. A., Jain, R., Siddiqui, M. M., Saini, D. K., and Mukherjee, P. S. (2017). Water-soluble Pd8L4 self-assembled molecular barrel as an aqueous carrier for Hydrophobic Curcumin. Inorg. Chem. 56, 5352–5360. doi: 10.1021/acs.inorgchem.7b00449
Bloch, W. M., Abe, Y., Holstein, J. J., Wandtke, C. M., Dittrich, B., and Clever, G. H. (2016). Geometric complementarity in assembly and guest recognition of a bent Heteroleptic cis-[Pd2LA2LB2] coordination cage. J. Am. Chem. Soc. 138, 13750–13755. doi: 10.1021/jacs.6b08694
Bloch, W. M., Holstein, J. J., Hiller, W., and Clever, G. H. (2017). Morphological control of Heteroleptic cis- and trans- cages. Angew. Chem. Int. Ed. 56, 8285–8289. doi: 10.1002/anie.201702573
Bondy, C. R., Gale, P. A., and Loeb, S. J. (2004). Metal-organic anion receptors: arranging urea hydrogen-bond donors to encapsulate sulfate ions. J. Am. Chem. Soc. 126, 5030–5031. doi: 10.1021/ja039712q
Casini, A., Woods, B., and Wenzel, M. (2017). The Promise of Self-Assembled 3D Supramolecular coordination complexes for biomedical applications. Inorg. Chem. 56, 14715–14729. doi: 10.1021/acs.inorgchem.7b02599
Cook, T. R., and Stang, P. J. (2015). Recent developments in the preparation and chemistry of metallacycles and metallacages via coordination. Chem. Rev. 115, 7001–7045. doi: 10.1021/cr5005666
Cook, T. R., Vajpayee, V., Lee, M. H., Stang, P. J., and Chi, K.-W. (2013). Biomedical and biochemical applications of self-assembled metallacycles and metallacages. Acc. Chem. Res. 46, 2464–2474. doi: 10.1021/ar400010v
Dubey, A., Jeong, Y. J., Jo, J. H., Woo, S., Kim, D. H., Kim, H., et al. (2015). Anticancer activity and autophagy involvement of self-assembled arene-ruthenium metallacycles. Organometallics 34, 4507–4514. doi: 10.1021/acs.organomet.5b00512
Faulkner, A. D., Kaner, R. A., Abdallah, Q. M., Clarkson, G., Fox, D. J., Gurnani, P., et al. (2014). Asymmetric triplex metallohelices with high and selective activity against cancer cells. Nat. Chem. 6, 797–803. doi: 10.1038/nchem.2024
Garci, A., Castor, K. J., Fakhoury, J., Do, J.-L., Di Trani, J., Chidchob, P., et al. (2017). Efficient and rapid mechanochemical assembly of Platinum(II) squares for guanine quadruplex targeting. J. Am. Chem. Soc. 139, 16913–16922. doi: 10.1021/jacs.7b09819
Grishagin, I. V., Kushal, S., Olenyuk, B. Z., Pollock, J. B., Cook, T. R., and Stang, P. J. (2014). In vivo anticancer activity of rhomboidal Pt(II) metallacycles. Proc. Natl. Acad. Sci. U.S.A. 111, 18448–18453. doi: 10.1073/pnas.1418712111
Han, J., Schmidt, A., Zhang, T., Permentier, H., Groothuis, G. M., Bischoff, R., et al. (2017). Bioconjugation strategies to couple supramolecular exo-functionalized palladium cages to peptides for biomedical applications. Chem. Commun. 53, 1405–1408. doi: 10.1039/C6CC08937B
Hotze, A. C. G., Hodges, N. J., Hayden, R. E., Sanchez-Cano, C., Paines, C., Male, N., et al. (2008). Supramolecular iron cylinder with unprecedented DNA binding is a potent cytostatic and apoptotic agent without exhibiting genotoxicity. Chem. Biol. 15, 1258–1267. doi: 10.1016/j.chembiol.2008.10.016
Howson, S. E., Bolhuis, A., Brabec, V., Clarkson, G. J., Malina, J., Rodger, A., et al. (2012). Optically pure, water-stable metallo-helical 'flexicate' assemblies with antibiotic activity. Nat. Chem. 4, 31–36. doi: 10.1038/nchem.1206
Kaiser, F., Schmidt, A., Heydenreuter, W., Altmann, P. J., Casini, A., Sieber, S. A., et al. (2016). Self-assembled palladium and platinum coordination cages: photophysical studies and anticancer activity. Eur. J. Inorg. Chem. 2016, 5189–5196. doi: 10.1002/ejic.201600811
Kim, T. Y., Lucas, N. T., and Crowley, J. D. (2015). A diaryl-linked [Pd2L4]4+ metallosupramolecular architecture: synthesis, structures and cisplatin binding studies. Supramol. Chem. 27, 734–745. doi: 10.1080/10610278.2015.1063633
Lehmann, B. D., Bauer, J. A., Chen, X., Sanders, M. E., Chakravarthy, A. B., Shyr, Y., et al. (2011). Identification of human triple-negative breast cancer subtypes and preclinical models for selection of targeted therapies. J. Clin. Invest. 121, 2750–2767. doi: 10.1172/JCI45014
Lewis, J. E., McAdam, C. J., Gardiner, M. G., and Crowley, J. D. (2013). A facile “click” approach to functionalised metallosupramolecular architectures. Chem. Commun. 49, 3398–3400. doi: 10.1039/c3cc41209a
Lewis, J. E. M., and Crowley, J. D. (2014). Exo- and endo-hedral interactions of counteranions with tetracationic Pd2L4 metallosupramolecular architectures. Supramol. Chem. 26, 173–181. doi: 10.1080/10610278.2013.842644
Lewis, J. E. M., Elliott, A. B. S., McAdam, C. J., Gordon, K. C., and Crowley, J. D. (2014). 'Click' to functionalise: synthesis, characterisation and enhancement of the physical properties of a series of exo- and endo-functionalised Pd2L4 nanocages. Chem. Sci. 5, 1833–1843. doi: 10.1039/c4sc00434e
Lewis, J. E. M., Gavey, E. L., Cameron, S. A., and Crowley, J. D. (2012). Stimuli-responsive Pd2L4 metallosupramolecular cages: towards targeted cisplatin drug delivery. Chem. Sci. 3, 778–784. doi: 10.1039/c2sc00899h
Li, M., Howson, S. E., Dong, K., Gao, N., Ren, J., Scott, P., et al. (2014). Chiral metallohelical complexes enantioselectively target Amyloid β for treating Alzheimer's disease. J. Am. Chem. Soc. 136, 11655–11663. doi: 10.1021/ja502789e
Lo, W. K., Huff, G. S., Preston, D., McMorran, D. A., Giles, G. I., Gordon, K. C., et al. (2015). A dinuclear Platinum(II) N4Py complex: an unexpected coordination mode for N4Py. Inorg. Chem. 54, 6671–6673. doi: 10.1021/acs.inorgchem.5b01032
Mal, P., Breiner, B., Rissanen, K., and Nitschke, J. R. (2009). White Phosphorus is air-stable within a self-assembled tetrahedral capsule. Science 324, 1697–1699. doi: 10.1126/science.1175313
Malina, J., Scott, P., and Brabec, V. (2015). Recognition of DNA/RNA bulges by antimicrobial and antitumor metallohelices. Dalton Trans. 44, 14656–14665. doi: 10.1039/C5DT02018B
Martí-Centelles, V., Lawrence, A. L., and Lusby, P. J. (2018). High activity and efficient turnover by a simple, self-assembled “Artificial Diels-Alderase”. J. Am. Chem. Soc. 140, 2862–2868. doi: 10.1021/jacs.7b12146
McMorran, D. A., and Steel, P. J. (1998). The first coordinatively saturated, quadruply stranded helicate and its encapsulation of a hexafluorophosphate anion. Angew Chem. Int. Ed. 37, 3295–3297.
McNeill, S. M., Preston, D., Lewis, J. E., Robert, A., Knerr-Rupp, K., Graham, D. O., et al. (2015). Biologically active [Pd2L4]4+ quadruply-stranded helicates: stability and cytotoxicity. Dalton Trans. 44, 11129–11136. doi: 10.1039/C5DT01259G
Mitchell, D. E., Clarkson, G., Fox, D. J., Vipond, R. A., Scott, P., and Gibson, M. I. (2017). Antifreeze protein mimetic metallohelices with potent ice recrystallization inhibition activity. J. Am. Chem. Soc. 139, 9835–9838. doi: 10.1021/jacs.7b05822
Mjos, K. D., and Orvig, C. (2014). Metallodrugs in medicinal inorganic chemistry. Chem. Rev. 114, 4540–4563. doi: 10.1021/cr400460s
Oleksy, A., Blanco, A. G., Boer, R., Usón, I., Aymam,í, J., Rodger, A., et al. (2006). Molecular recognition of a three-way DNA junction by a Metallosupramolecular helicate. Angew. Chem. Int. Ed. 45, 1227–1231. doi: 10.1002/anie.200503822
Phongtongpasuk, S., Paulus, S., Schnabl, J., Sigel, R. K., Spingler, B., Hannon, M. J., et al. (2013). Binding of a designed anti-cancer drug to the central cavity of an RNA three-way junction. Angew. Chem. Int. Ed. 52, 11513–11516. doi: 10.1002/anie.201305079
Preston, D., Fox-Charles, A., Lo, W. K. C., and Crowley, J. D. (2015). Chloride triggered reversible switching from a metallosupramolecular [Pd2L4]4+ cage to a [Pd2L2Cl4] metallo-macrocycle with release of endo- and exo-hedrally bound guests. Chem. Commun. 51, 9042–9045. doi: 10.1039/C5CC02226F
Preston, D., Lewis, J. E., and Crowley, J. D. (2017). Multicavity [PdnL4]2n+ cages with controlled segregated binding of different guests. J. Am. Chem. Soc. 139, 2379–2386. doi: 10.1021/jacs.6b11982
Preston, D., McNeill, S. M., Lewis, J. E., Giles, G. I., and Crowley, J. D. (2016). Enhanced kinetic stability of [Pd2L4]4+ cages through ligand substitution. Dalton Trans. 45, 8050–8060. doi: 10.1039/C6DT00133E
Richards, A. D., Rodger, A., Hannon, M. J., and Bolhuis, A. (2009). Antimicrobial activity of an iron triple helicate. Int. J. Antimicrob. Agents 33, 469–472. doi: 10.1016/j.ijantimicag.2008.10.031
Samanta, S. K., Moncelet, D., Briken, V., and Isaacs, L. (2016). Metal-organic Polyhedron capped with Cucurbit[8]uril delivers Doxorubicin to cancer cells. J. Am. Chem. Soc. 138, 14488–14496. doi: 10.1021/jacs.6b09504
Samanta, S. K., Quigley, J., Vinciguerra, B., Briken, V., and Isaacs, L. (2017). Cucurbit[7]uril enables multi-stimuli-responsive release from the self-assembled Hydrophobic phase of a metal organic Polyhedron. J. Am. Chem. Soc. 139, 9066–9074. doi: 10.1021/jacs.7b05154
Schmidt, A., Casini, A., and Kuehn, F. E. (2014). Self-assembled M2L4 coordination cages: synthesis and potential applications. Coord. Chem. Rev. 275, 19–36. doi: 10.1016/j.ccr.2014.03.037
Schmidt, A., Hollering, M., Han, J., Casini, A., and Kühn, F. E. (2016a). Self-assembly of highly luminescent heteronuclear coordination cages. Dalton Trans. 45, 12297–12300. doi: 10.1039/C6DT02708C
Schmidt, A., Molano, V., Hollering, M., Pöthig, A., Casini, A., and Kühn, F. E. (2016b). Evaluation of new palladium cages as potential delivery systems for the anticancer drug Cisplatin. Chem. Eur. J. 22, 2253–2256. doi: 10.1002/chem.201504930
Schmitt, F., Freudenreich, J., Barry, N. P., Juillerat-Jeanneret, L., Süss-Fink, G., and Therrien, B. (2012). Organometallic cages as vehicles for intracellular release of Photosensitizers. J. Am. Chem. Soc. 134, 754–757. doi: 10.1021/ja207784t
Therrien, B. (2015). Biologically relevant arene ruthenium metalla-assemblies. CrystEngComm 17, 484–491. doi: 10.1039/C4CE02146K
Therrien, B., Suess-Fink, G., Govindaswamy, P., Renfrew, A. K., and Dyson, P. J. (2008). The “complex-in-a-complex” cations [(acac)2M⊂Ru6-(piPrC6H4Me)6(tpt)2(dhbq)3]6+: a trojan horse for cancer cells. Angew. Chem. Int. Ed. 47, 3773–3776. doi: 10.1002/anie.200800186
Wang, H., Qian, X., Wang, K., Su, M., Haoyang, W.-W., Jiang, X., et al. (2018). Supramolecular Kandinsky circles with high antibacterial activity. Nat. Commun. 9:1815. doi: 10.1038/s41467-018-04247-z
Wang, J.-F., Huang, L.-Y., Bu, J.-H., Li, S.-Y., Qin, S., Xu, Y.-W., et al. (2018). A fluorescent calixarene-based dimeric capsule constructed via a M(II)-terpyridine interaction: cage structure, inclusion properties and drug release. RSC Adv. 8, 22530–22535. doi: 10.1039/C8RA02146E
Wang, M., Vajpayee, V., Shanmugaraju, S., Zheng, Y.-R., Zhao, Z., Kim, H., et al. (2011). Coordination-driven self-assembly of M3L2 trigonal cages from preorganized metalloligands incorporating octahedral metal centers and fluorescent detection of nitroaromatics. Inorg. Chem. 50, 1506–1512. doi: 10.1021/ic1020719
Xu, W.-Q., Fan, Y.-Z., Wang, H.-P., Teng, J., Li, Y.-H., Chen, C.-X., et al. (2017). Investigation of binding behavior between drug molecule 5-Fluoracil and M4L4-type Tetrahedral cages: selectivity, capture, and release. Chem. Eur. J. 23, 3542–3547. doi: 10.1002/chem.201606060
Yi, J. W., Barry, N. P., Furrer, M. A., Zava, O., Dyson, P. J., Therrien, B., et al. (2012). Delivery of Floxuridine derivatives to cancer cells by water-soluble organometallic cages. Bioconjugate Chem. 23, 461–471. doi: 10.1021/bc200472n
Yoshizawa, M., and Fujita, M. (2010). Development of unique chemical phenomena within nanometer-sized, self-assembled coordination hosts. Bull. Chem. Soc. Jpn. 83, 609–618. doi: 10.1246/bcsj.20100035
Yoshizawa, M., Klosterman, J. K., and Fujita, M. (2009). Functional molecular flasks: new properties and reactions within discrete, self-assembled hosts. Angew. Chem. Int. Ed. 48, 3418–3438. doi: 10.1002/anie.200805340
Yue, Z., Wang, H., Li, Y., Qin, Y., Xu, L., Bowers, D. J., et al. (2018). Coordination-driven self-assembly of a Pt(IV) prodrug-conjugated supramolecular hexagon. Chem. Commun. 54, 731–734. doi: 10.1039/C7CC07622C
Zhao, A., Howson, S. E., Zhao, C., Ren, J., Scott, P., Wang, C., et al. (2017). Chiral metallohelices enantioselectively target hybrid human telomeric G-quadruplex DNA. Nucleic Acids Res. 45, 5026–5035. doi: 10.1093/nar/gkx244
Zheng, Y.-R., Suntharalingam, K., Bruno, P. M., Lin, W., Wang, W., Hemann, M. T., et al. (2016). Mechanistic studies of the anticancer activity of an octahedral hexanuclear Pt(II) cage. Inorg. Chim. Acta 452, 125–129. doi: 10.1016/j.ica.2016.03.021
Zheng, Y.-R., Suntharalingam, K., Johnstone, T. C., and Lippard, S. J. (2015). Encapsulation of Pt(IV) prodrugs within a Pt(II) cage for drug delivery. Chem. Sci. 6, 1189–1193. doi: 10.1039/C4SC01892C
Keywords: palladium(II), anticancer, self-assembly, metallosupramolecular, quinoline
Citation: Vasdev RAS, Gaudin LF, Preston D, Jogy JP, Giles GI and Crowley JD (2018) Anticancer Activity and Cisplatin Binding Ability of Bis-Quinoline and Bis-Isoquinoline Derived [Pd2L4]4+ Metallosupramolecular Cages. Front. Chem. 6:563. doi: 10.3389/fchem.2018.00563
Received: 29 September 2018; Accepted: 31 October 2018;
Published: 22 November 2018.
Edited by:
Jonathan Kitchen, Institute of Natural and Mathematical Sciences, Massey University, New ZealandReviewed by:
Robert Elmes, Maynooth University, IrelandNicholas White, Australian National University, Australia
Copyright © 2018 Vasdev, Gaudin, Preston, Jogy, Giles and Crowley. This is an open-access article distributed under the terms of the Creative Commons Attribution License (CC BY). The use, distribution or reproduction in other forums is permitted, provided the original author(s) and the copyright owner(s) are credited and that the original publication in this journal is cited, in accordance with accepted academic practice. No use, distribution or reproduction is permitted which does not comply with these terms.
*Correspondence: Roan A. S. Vasdev, cm9hbi52YXNkZXZAb3RhZ28uYWMubno=
James D. Crowley, amNyb3dsZXlAY2hlbWlzdHJ5Lm90YWdvLmFjLm56