- 1Institute of Multidisciplinary Research for Advanced Materials, Tohoku University, Sendai, Japan
- 2Department of Chemistry and Materials Technology, Graduate School of Science and Technology, Kyoto Institute of Technology, Kyoto, Japan
In this research, we have found that layered perovskite titanate Sr2TiO4 doped with Mn4+ exhibits photoluminescence even at room temperature despite no luminescence from Mn4+-doped SrTiO3 with a three-dimensional bulky perovskite structure. The relative position of t2g orbital of Mn to the valence band is a key factor for appearance of Mn4+-emission in Sr2TiO4:Mn. This result suggested usefulness of layered perovskite-type materials as hosts for Mn4+-activated phosphors than the bulky perovskite-type materials. Our investigation into photoluminescence of Mn4+-doped layered perovskite compounds has revealed that strontium scandium oxyfluoride Sr2ScO3F activated with Mn4+ exhibits Mn4+-emission with a peak at 697 nm under excitation at 300–600 nm and its emission intensity is much stronger than that of Sr2TiO4:Mn. The internal and external quantum yields of Sr2ScO3F:Mn were determined to be 50.5 and 43.5% under excitation at 345 nm, respectively.
Introduction
White light emitting diodes (W-LEDs) based on blue-LEDs are widely spreading to various fields as highly efficient solid lightings (Lin et al., 2016; Adachi, 2018; Wang et al., 2018). Artificial white light is basically obtained by combination of blue and yellow light emitted from a blue-LED chip and a yellow-emitting phosphor Y3Al5O12:Ce, respectively. Such white light is inevitably cool white with high color temperature due to poor emission strength of Y3Al5O12:Ce in red region. Efficient red-emitting phosphors are added to achieve artificial warm white light by tuning color temperature. Nitride phosphors activated with Eu2+ such as (Sr,Ca)AlSiN3:Eu2+ and M2Si5N8:Eu2+ (M = Ca, Sr, and Ba) are extensively studied and commercially used as the red-emitting phosphors (Li et al., 2006, 2009; Uheda et al., 2006; Watanabe and Kijima, 2009; Tsai et al., 2015; Wang et al., 2018). However, requirements of high temperature and high pressure in synthesis of nitrides are drawbacks of nitride phosphors rising the costs. Therefore, development of alternative yellow—to red-emitting phosphors activated with Eu2+, which can be synthesized milder conditions in comparison with nitrides, is also conducted for oxides, phosphates, and oxyhalides (Toda et al., 2006; Daicho et al., 2012, 2018; Kim et al., 2013; Sato et al., 2014; Wen et al., 2016). Besides, phosphors activated with Mn4+ have been recently paid attention due to capability of red emission using wide variety of host materials (Srivastava and Beers, 1996; Seki et al., 2013; Ye et al., 2013; Sasaki et al., 2014; Wang et al., 2014; Takeda et al., 2015, 2017; Zhou et al., 2016; Cai et al., 2017; Wu et al., 2017; Xi et al., 2017; Zhang et al., 2017; Adachi, 2018; Jansen et al., 2018). Octahedral 6-fold coordination sites are preferred for substitution of Mn4+ ions. Fluorides and aluminates are paid much attention as hosts of Mn4+-activated phosphors from the viewpoints of their insulating nature and octahedral sites. Besides, titanates having semiconducting nature are also available for hosts of Mn4+-activated phosphors (Srivastava and Beers, 1996; Seki et al., 2013; Ye et al., 2013; Sasaki et al., 2014; Takeda et al., 2015; Zhang et al., 2017). We have recently reported that double perovskite-type titanates La2MTiO6 (M: Mg and Zn) are available as host materials of Mn4+-activated phosphors although a representative perovskite-type titanate SrTiO3 doped with Mn4+ could not show any luminescence at room temperature due to significant thermal quenching at low temperature, ~100 K (Takeda et al., 2015). Low temperature photoluminescence measurements and theoretical band structure calculations have revealed the importance of relative position of Mn 3d orbitals to valence and conduction bands of host materials in order to avoid electron transfer from the valence band to empty t2g orbital of Mn and photoionization. The knowledge obtained from the previous researches encourages us to expand the research target for Mn4+-activated phosphors to Sr2TiO4 possessing a K2MgF4 type layered perovskite structure. Both SrTiO3 and Sr2TiO4 are members in a perovskite family composed of the same constituent elements. SrTiO3 of the representative perovskite-type compound is composed of TiO6 octahedra sharing corners infinitely, building three-dimensional bulky structure, while Sr2TiO4 has layers of the two-dimensional perovskite slab with a single TiO6 thickness separated by SrO layers as depicted in Figure 1. The decreases in structural dimension cause widening band gaps (Reyes-Lillo et al., 2016), which is thought to be a positive factor to suppress the electron transfer and/or the photoionization. Therefore, it is expected that comparison photoluminescence properties between SrTiO3:Mn and Sr2TiO4:Mn gives important information to understand the relationship between structural dimension and photoluminescence properties with Mn4+-activation.
In this research, we investigated photoluminescence properties of Mn4+-activated layered perovskite compounds. The differences in photoluminescence properties especially thermal quenching properties between SrTiO3:Mn and Sr2TiO4:Mn are discussed from features in crystal structures. In addition, we also investigated into photoluminescence properties of Sr2ScO3F:Mn possessing the K2MgF4 type structure as well as Sr2TiO4:Mn.
Experiments
Sample Preparation
All powder samples were synthesized by a solid state reaction method using SrCO3 (Kanto, 99.9%), SrF2 (Wako, 99.5%), rutile type TiO2 (Kojundo Chemical, 99.9%), Sc2O3 (Shin-Etsu Chemical, 99.99%), and Mn(NO3)2 6H2O (Wako, 98.0%) as raw materials. The stoichiometric mixtures of the raw materials were calcined at 1473 K for 5 h in air using alumina crucibles. Where, concentration of Mn substitution was fixed at 0.2 atom% to Ti or Sc. Non-doped samples were also synthesized by the same manner.
Characterization of Samples
Crystal phases of obtained samples were confirmed by powder X-ray diffraction (XRD) technique (Bruker, D2 Phaser). Photoluminescence measurements were performed using fluorescence spectrometers (Hitachi; F-4500 and Jasco; FP-6500). Photoluminescence spectra were also taken at low temperature (80–300 K with a step of 20 K) using a cryostat (Janis; VPF-475) under vacuum. Diffuse reflectance spectra of non-doped samples were taken by an absorption spectrometer equipping an integration sphere (Shimadzu; UV-3100). The band gaps of the non-doped samples with indirect transition were determined from (αhν)1/2–hν plot, where α, h, and ν represent Kubelka-Munk function, Planck constant, and frequency, respectively.
Band Structure Calculation
The band structures were calculated by the plane wave based density functional theory (DFT) using CASTEP program (Payne et al., 1992; Milman et al., 2000). The Perdew-Burke-Ernzerhof (PBE) functional was used together with the ultrasoft-core potentials (Vanderbilt, 1991; Perdew et al., 1996, 1997). The cutoff energies were set to 300 eV. The electron configurations of the atoms were O: 2s22p4, F: 2s22p5, Sc: 3s23p63d14s2, Ti: 3s23p63d24s2, Mn: 3d54s2, and Sr: 4s24p65s2. Super cells of Sr16Ti7MnO32 and Sr16Sc7MnO25F7 were employed for models of Sr2TiO4:Mn and Sr2ScO3F:Mn, respectively. Where, one F atom was also replaced with an O atom accompanied by the substitution of Mn for Sc to maintain the charge balance in the Sr2ScO3F:Mn system. From the experimental finding, the local electronic structure for the substituted Mn atom is known to be a 4+ cation, and the Mn ion is in the quintet state. Geometry optimization was carried out with respect to all atomic coordinates.
Results and Discussion
Luminescence of Sr2TiO4:Mn
Figure 2 shows photoluminescence spectra of SrTiO3:Mn and Sr2TiO4:Mn with corresponding excitation spectra at room temperature. Sr2TiO4:Mn showed deep-red emission with a peak at 725 nm attributed to2Eg→4A2g transition of Mn4+ under excitation at 300–580 nm. Although the emission intensity is not high, this is an interesting result taking into consideration of the fact that SrTiO3:Mn shows no emission at room temperature due to significant thermal quenching. Although both strontium titanates are composed of the same elements and are members of the perovskite family, a remarkable difference is present with regard to the structural dimension; Sr2TiO4 has a two-dimensional layered structure whereas SrTiO3 has a three-dimensional bulky one. Therefore, the appearance of Mn4+-emission in Sr2TiO4:Mn may reflect advantage of the layered perovskite structure in the band structure than bulky one. The further discussion about Sr2TiO4:Mn is described later.
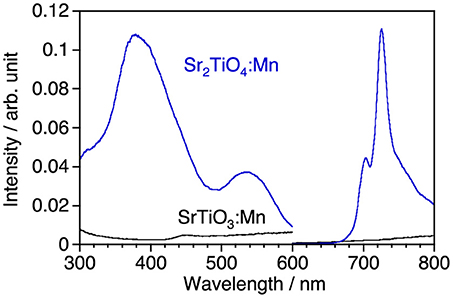
Figure 2. Luminescence spectra of SrTiO3:Mn and Sr2TiO4:Mn and corresponding excitation spectra at room temperature. Excitation and monitored wavelengths were 380 and 725 nm, respectively.
Comparison of Luminescence Properties Between Sr2ScO3F:Mn and Sr2TiO4:Mn
Although, as shown in Figure 3A, Sr2TiO4 has a wider band gap (3.46 eV) than SrTiO3 (3.21 eV) as reported in literature (Reyes-Lillo et al., 2016), other layered perovskite compounds possessing wider band gaps are preferred for efficient Mn4+-emission because of less probability of the electron transfer between Mn 3d and the valence and/or conduction band. Strontium scandium oxyfluoride Sr2ScO3F with a K2MgF4 type structure as well as Sr2TiO4, which has been recently discovered (Wang et al., 2015), was thought to be a good candidate because its octahedral building unit ScO5F based on the optically inert rare earth element was expected to give a wider energy gap in comparison with TiO6. XRD confirmed that Sr2ScO3F:Mn was obtained as the almost pure phase of Sr2ScO3F although it contained tiny amounts of SrF2 and SrSc2O4 as impurities whereas Sr2TiO4:Mn was obtained as a pure phase without any impurities (Figure 3B). Relative intensities of diffraction peaks of Sr2ScO3F:Mn at 14.1, 28.3, 43.0, and 58.5 degrees corresponding to reflections from (002), (004), (006), and (008), respectively, were remarkably strong in comparison with the standard ones due to orientation of crystals in (00l). The band gap of Sr2ScO3F has been discovered to be 5.38 eV, being wider than that of Sr2TiO4 (Figure 3A). Figure 4 shows emission and excitation spectra of Sr2ScO3F:Mn and Sr2TiO4:Mn at room temperature. Sr2ScO3F:Mn showed deep-red emission owing to transition of Mn4+ giving a peak at 697 nm. Obvious two excitation bands in 300–460 nm and in 480–580 nm are attributed to spin-allow4A2g→4T1g and4A2g→4T2g transition of Mn4+ ions, respectively, while a weak excitation band owing to spin-forbidden4A2g→2T2g transition is difficult to distinguish and it may be embedded in the tail of the4A2g→4T1g band as observed in other titanates and tantalates (Sasaki et al., 2014; Wang et al., 2014; Takeda et al., 2015, 2017). Interestingly, the emission from Sr2ScO3F:Mn was much stronger than that from Sr2TiO4:Mn; the internal and external quantum yields of Sr2ScO3F:Mn excited at 345 nm at room temperature (50.5 and 43.5%) were much higher than those of Sr2TiO4:Mn excited at 380 nm (3.4 and 2.5%). The Mn4+-emission from fluoride hosts consists of some very sharp lines while that from oxide hosts is broad (Zhou et al., 2016; Adachi, 2018). The emission from Sr2ScO3F:Mn is broad as well as Mn4+-activated oxide phosphors despite presence of the Sc-F bond. This means that influences of F upon the photoluminescence property of Sr2ScO3F:Mn are not significant. In Sr2ScO3F:Mn, it is preferred from the charge compensation that one fluorine is replaced with one oxygen when Mn4+ is substituted for Sc3+. Such co-substitution results in the formation of MnO6 octahedra which give broad Mn4+-emission. The spectra of CaSiAlN3:Eu, which is the representative red-emitting phosphor activated with Eu2+, are also shown in Figure 4. The Sr2ScO3F:Mn emission is sharper and stronger than the CaSiAlN3:Eu emission however the wavelength of the Sr2ScO3F:Mn emission is excessively long, that is, almost the half portion of emission is located in the invisible region (λ > 700 nm). CaAlSiN3:Eu can be excited by blue-LEDs (λ = 450–470 nm) more efficiently than Sr2ScO3F:Mn while Sr2ScO3F:Mn can be excited by near ultraviolet LEDs (λ = 350–400 nm) more efficiently than CaAlSiN3:Eu.
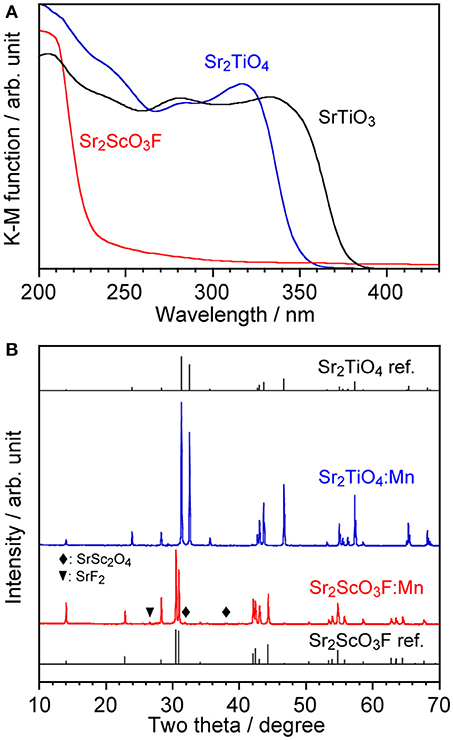
Figure 3. (A) Diffuse reflectance spectra of non-doped Sr2TiO4, Sr2ScO3F, and SrTiO3 and (B) XRD patterns of Sr2TiO4:Mn and Sr2ScO3F:Mn with reference patterns.
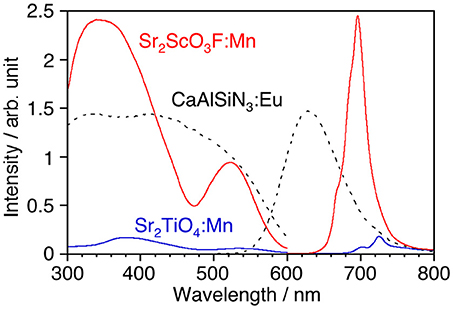
Figure 4. Luminescence spectra of Sr2TiO4:Mn, Sr2ScO3F:Mn, and CaAlSiN3:Eu with corresponding excitation spectra at room temperature. Excitation and monitored wavelengths were 380 and 725 nm for Sr2TiO4:Mn, 345 and 697 nm for Sr2ScO3F:Mn, and 340 and 630 nm for CaAlSiN3:Eu.
Measurements of thermal quenching were performed at low (80–300 K) and high temperature ranges (298–473 K). Both samples suffered temperature quenching even in the low temperature range especially higher than 200 K as shown in Figures 5A–C. The maximum peak intensity decreased as measurement temperature rose while the emission of anti-Stokes sidebands, which were observed in regions shorter than 710 and 675 nm in Sr2TiO4:Mn and Sr2ScO3F:Mn, respectively, was enhanced due to transition of excited electrons to upper vibration states by thermal energy (Wu et al., 2017; Adachi, 2018). It results in the non-obvious decreases in the integrated emission intensity up to 200 K. Sr2ScO3F:Mn exhibited stronger emission than Sr2TiO4:Mn at all temperatures, moreover, the intensity of Sr2TiO4:Mn at 80 K was lower than that of Sr2ScO3F:Mn at 300 K. Thus, Sr2TiO4:Mn exhibited more remarkable thermal quenching in comparison with Sr2ScO3F:Mn. In the high temperature range (298–473 K), significant thermal quenching occurred in both samples as shown in Figure 5D, however Sr2ScO3F:Mn showed lesser thermal quenching than Sr2TiO4:Mn. At 373 K, Sr2ScO3F:Mn showed 20% of emission intensity in comparison with that at 298 K whereas emission from Sr2TiO4:Mn was completely quenched.
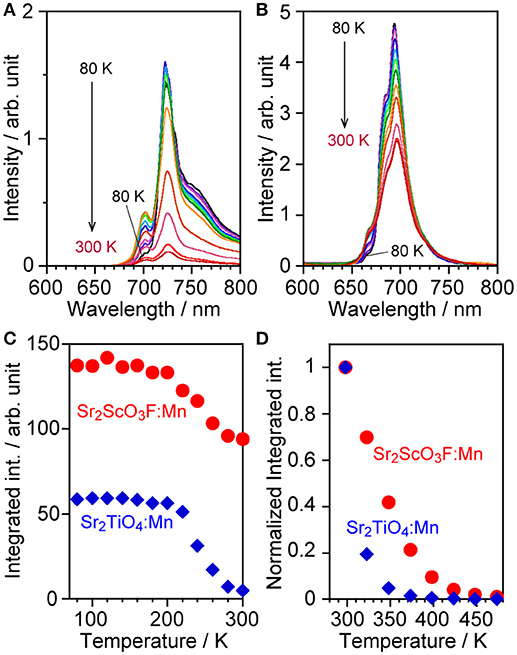
Figure 5. Emission spectra of (A) Sr2TiO4:Mn and (B) Sr2ScO3F:Mn at low temperature (80–300 K), (C) relative integrated emission intensity of them, and (D) normalized integrated emission intensity of Sr2TiO4:Mn and Sr2ScO3F:Mn at high temperature (298–473 K). Excitation wavelengths were 380 and 340–345 nm for Sr2TiO4:Mn and Sr2ScO3F:Mn, respectively.
Band Structures of Sr2TiO4:Mn and Sr2ScO3F:Mn
As described above, Sr2ScO3F:Mn showed superior characteristics, that is, higher emission intensity and lesser thermal quenching, to Sr2TiO4:Mn. Relative position of Mn 3d orbitals to the valence and conduction bands of host materials is an important factor for Mn4+-activated phosphors as we have reported previously (Takeda et al., 2015, 2017). Therefore, band structures of Sr2TiO4:Mn and Sr2ScO3F:Mn were investigated by the DFT method. Figure 6 depicts projected density of states (PDOS) near the band gap of Sr16Ti7MnO32 and Sr16Sc7MnO25F7 corresponding to Sr2TiO4:Mn and Sr2ScO3F:Mn. In Figure 6, positive and negative values in DOS represent DOS for up-spin (α) and down-spin (β) electrons, respectively, and 0 eV of energy represents the Fermi level. In Sr2TiO4:Mn, the valence and conduction bands of host are composed of O 2p and Ti 3d orbitals, respectively, like SrTiO3:Mn. In PDOS of Sr2TiO4:Mn, the t2g(α) orbitals of Mn look to be located slightly higher position than the valence band however a tail of t2g(α) is embedded in the valence band. The DFT calculation reveals that Sr2TiO4:Mn has absolutely different feature in the relative position of Mn 3d orbitals to the valence band from SrTiO3:Mn, in which the t2g(α) orbitals are deeply embedded in the valence band (Takeda et al., 2015). Although a part of t2g(α) orbitals is located in positive energy region, it doesn't indicate the presence of empty t2g(α) orbitals. The total numbers of electrons calculated for both Sr16Ti7MnO32 and Sr16Sc7MnO25F7 models were 443. In the quintet state, the numbers of occupied orbitals should be 223 and 220 for α- and β-electrons, respectively. If the top of t2g(α) orbital of Mn 3d is empty, the lowest unoccupied molecular orbital for α-electron (#224 α-orbital) should be Mn 3d located near 0 eV. However, #224 α-orbital is not Mn 3d located around 0 eV but Mn3d orbital below the conduction band [indicated as eg(α) in Figure 6] in both models. The small portion of the occupied orbitals beyond the Fermi level observed in PDOS is due to broadening of energy widths of orbitals by the smearing treatment in the process of PDOS creation. Thus, it has been confirmed that all Mn 3d orbitals around 0 eV are occupied ones. The PDOS of Sr2ScO3F:Mn shows that the t2g(α) orbitals of Mn is located slightly higher position than the valence band without tailing portion at a lower energy side. The electron density contour maps for top four occupied molecular orbitals including the highest occupied molecular orbital (HOMO) are compared to see details of the differences between Sr2TiO4:Mn and Sr2ScO3F:Mn (Figure 7). In Sr2ScO3F:Mn, contribution of the occupied Mn 3d orbitals is seen only in the top three occupied orbitals (from HOMO to HOMO−2) and the forth highest occupied orbital (HOMO−3) is composed of only O 2p orbital. On the other hand, small contribution of the Mn 3d orbital is also seen in HOMO−3 of Sr2TiO4:Mn although Mn 3d orbitals mainly contribute to top three occupied orbitals. If hybridization between O 2p and t2g(α) of Mn 3d is small, the occupied Mn 3d orbitals appear in only three orbitals. The appearance of Mn 3d in four orbitals in Sr2TiO4:Mn (from HOMO to HOMO−3) indicates stronger hybridization between O 2p and Mn 3d than Sr2ScO3F:Mn. It is also noticed in PDOS that energy gap between eg(α) of Mn 3d and the bottom of conduction band is larger in Sr2ScO3F:Mn than Sr2TiO4:Mn. It reflects the remarkably wider band gap of Sr2ScO3F than Sr2TiO4.
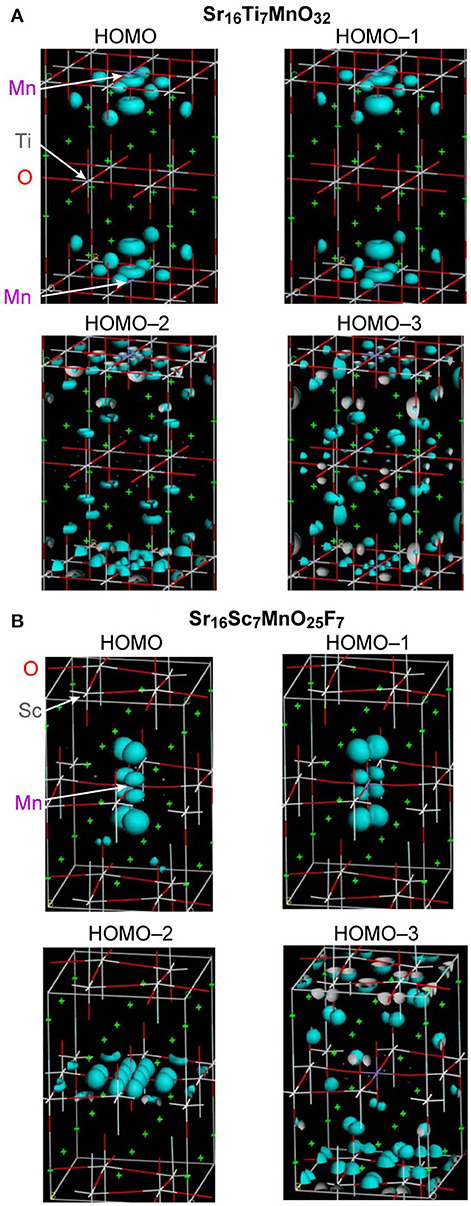
Figure 7. Electron density contour maps of top four occupied orbitals for (A) Sr16Ti7MnO32 and (B) Sr16Sc7MnO25F7.
Figure 8 illustrates proposed mechanism based on photoluminescence measurements and band structure calculations for Mn4+-activated SrTiO3, Sr2TiO4, and Sr2ScO3F. The most significant difference in photoluminescence property between Sr2TiO4:Mn and SrTiO3:Mn is the appearance of Mn4+-emission in Sr2TiO4:Mn at room temperature. The difference in the relative position of t2g(α) orbitals of Mn between SrTiO3:Mn and Sr2TiO4:Mn is of importance in explanation for the appearance of Mn4+-emission in Sr2TiO4:Mn. In SrTiO3:Mn, the t2g(α) orbitals embedded in the valence band facilitate thermal quenching via the electron transfer from the valence band to the empty t2g in the excited state2Eg, resulting in low quenching temperature, ~100 K (Takeda et al., 2015). In contrast to SrTiO3:Mn, t2g(α) in Sr2TiO4:Mn is located slightly higher position than the valence band. Therefore, Sr2TiO4:Mn shows Mn4+-emission even at room temperature. Interaction between TiO6 octahedra may affect the relative position of t2g(α) orbitals of Mn. Each TiO6 octahedron connects to six TiO6 octahedra in SrTiO3 while TiO6 connects to four TiO6 octahedra in the perovskite slab in Sr2TiO4 as shown in Figure 1, indicating that degree of energy delocalization is higher in three-dimensional SrTiO3 than two-dimensional Sr2TiO4. The smaller interaction between TiO6 octahedra may cause less interaction between O 2p and occupied of Mn 3d orbitals, that is, t2g(α) orbitals. Thus, the advantage of two-dimensional layered perovskite structure for Mn4+-activated phosphors can be explained by the smaller interaction of MO6 octahedra. Such discussion can be applied to another Mn4+-activated titanate phosphor La2MgTiO6:Mn with a B-site ordered double perovskite structure, which is an efficient Mn4+-activated phosphor with 58.7% of an internal quantum yield (Takeda et al., 2015). In La2MgTiO6, each TiO6 octahedron is surrounded by six MgO6 octahedra, meaning that TiO6 is isolated from other TiO6 octahedra even though the perovskite-type structure (Lee et al., 2000). Thus, the structure of La2MgTiO6 can be regarded as a quasi-zero-dimensional structure with respect to the connection between TiO6 octahedra. The less interaction of TiO6 in La2MgTiO6:Mn leads the larger energy gap between the valence band and t2g(α) of Mn, resulting in the superior photoluminescence efficiency to Sr2TiO4:Mn. In Sr2ScO3F:Mn, the t2g(α) orbitals of Mn 3d are located above the valence band with a slightly larger energy gap than Sr2TiO4:Mn due to the small hybridization between O 2p and Mn 3d as described above. On the other hand, the larger energy gap between eg(α) and the bottom of conduction band in Sr2ScO3F:Mn suppresses quenching via photoionization, in which an electron in eg(α) orbitals in the excited state is transferred to the conduction band and then is relaxed without emission (Takeda et al., 2017). Thus, the two factors, the less interaction between t2g(α) and the valence band and the large energy gap between eg(α) and the bottom of conduction band, positively affect the smaller thermal quenching in Sr2ScO3F:Mn than Sr2TiO4:Mn, resulting in the stronger emission.
Conclusions
Photoluminescence properties of Mn4+-activated strontium titanates, SrTiO3:Mn with three-dimensional bulky perovskite structure and Sr2TiO4:Mn with two-dimensional layered perovskite structure, have been compared in this research. Sr2TiO4:Mn shows Mn4+-emission even at room temperature despite no emission from SrTiO3:Mn. In addition, the results in our systematic research suggest that the less interaction between MO6 octahedra of B-site cation in the perovskite family provides positive influences in Mn4+-emission. Comparison between Sr2TiO4:Mn and Sr2ScO3F:Mn indicates that ScO5F octahedra are preferable constituents to TiO6 ones for the Mn4+-activated phosphors. Thus, the present research demonstrates that scandium, which is one of optically inert rare earth elements, is a useful element as a major constituent for design of Mn4+-activated phosphors.
Author Contributions
HidK managed all experiments and wrote the manuscript. YT performed experiments on synthesis of samples and evaluation of photoluminescence properties of them. HisK performed band structure calculations. MakK and MasK planned experiments and made discussion about the results.
Funding
This research was partly supported by KAKENHI Grant Numbers JP16H06438 and JP16H06439 in Scientific Research on Innovative Area Mixed Anion and JP16H02391 in Scientific Research (A) and Dynamic Alliance for Open Innovation Bridging Human, Environment and Materials in Network Joint Research Center for Materials and Devices.
Conflict of Interest Statement
The authors declare that the research was conducted in the absence of any commercial or financial relationships that could be construed as a potential conflict of interest.
References
Adachi, S. (2018). Photoluminescence properties of Mn4+-activated oxide phosphors for use in white-LED applications: a review. J. Lumin. 202, 263–281. doi: 10.1016/j.jlumin.2018.05.053
Cai, P., Qin, L., Chen, C., Wang, J., and Seo, H. J. (2017). Luminescence, energy transfer and optical thermometry of a novel narrow red emitting phosphor: Cs2WO2F4:Mn4+. Dalton Trans. 46, 14331–14340. doi: 10.1039/C7DT02751F
Daicho, H., Iwasaki, T., Enomoto, K., Sasaki, Y., Maeno, Y., Shinomiya, Y., et al. (2012). A novel phosphor for glareless white light-emitting diodes. Nat. Commun. 3:1132. doi: 10.1038/ncomms2138
Daicho, H., Shinomiya, Y., Enomoto, K., Nakano, A., Sawa, H., Matsuishi, S., et al. (2018). A novel red-emitting K2Ca(PO4)F:Eu2+ phosphor with a large Stokes shift. Chem. Commun. 54, 884–887. doi: 10.1039/C7CC08202A
Jansen, T., Jüstel, T., Kirm, M., Vielhauer, S., Khaidukov, N. M., and Makhovd, V. N. (2018). Composition dependent spectral shift of Mn4+ luminescence in silicate garnet hosts CaY2M2Al2SiO12 (M = Al, Ga, Sc). J. Lumin. 198, 314–319. doi: 10.1016/j.jlumin.2018.02.054
Kim, S. W., Hasegawa, T., Ishigaki, T., Uematsu, K., Toda, K., and Sato, M. (2013). Efficient red emission of blue-light excitable new structure type NaMgPO4:Mn4+ phosphor. ECS Solid State Lett. 2, R49–R51. doi: 10.1149/2.004312ssl
Lee, D.-Y., Yoon, S.-J., Yeo, J.-H., Nahm, S., Paik, J. H., Whang, K.-C., et al. (2000). Crystal structure and microwave dielectric properties of La(Mg1/2Ti1/2)O3 ceramics. J. Mater. Sci. Lett. 19, 131–134. doi: 10.1023/A:1006603615193
Li, H.-L., Xie, R.-J., Hirosaki, N., Takeda, T., and Zhou, G. H. (2009). Synthesis and luminescence properties of orange–red-emitting M2Si5N8:Eu2+ (M = Ca, Sr, Ba) light-emitting diode conversion phosphors by a simple nitridation of MSi2. Int. J. Appl. Ceram. Technol. 6, 459–464. doi: 10.1111/j.1744-7402.2009.02370.x
Li, Y. Q., Steen, J. E. J., Krevel, J. W. H., Botty, G., Delsing, A. C. A., DiSalvo, F. J., et al. (2006). Luminescence properties of red-emitting M2Si5N8:Eu2+ (M = Ca, Sr, Ba) LED conversion phosphors. J. Alloys Compd. 417, 273–279. doi: 10.1016/j.jallcom.2005.09.041
Lin, Y. C., Karlsson, M., and Bettinelli, M. (2016). Inorganic phosphor materials for lighting. Top. Curr. Chem. 374:21. doi: 10.1007/s41061-016-0023-5
Milman, V., Winkler, B., White, J. A., Pickard, C. J., Payne, M. C., Akhmatskaya, E. V., et al. (2000). Electronic structure, properties, and phase stability of inorganic crystals: a pseudopotential plane-wave study. Int. J. Quan. Chem. 77, 895–910. doi: 10.1002/(SICI)1097-461X(2000)77:5 < 895::AID-QUA10>3.0.CO;2-C
Payne, M. C., Teter, M. P., Allan, D. C., Arias, T. A., and Johnnopoulos, J. D. (1992). Iterative minimization techniques for ab initio total-energy calculations: molecular dynamics and conjugate gradients. Rev. Mod. Phys. 64, 1045–1097. doi: 10.1103/RevModPhys.64.1045
Perdew, J. P., Burke, K., and Ernzerhof, M. (1996). Generalized gradient approximation made simple. Phys. Rev. Lett. 77, 3865–3868. doi: 10.1103/PhysRevLett.77.3865
Perdew, J. P., Burke, K., and Ernzerhof, M. (1997). Generalized gradient approximation made simple. Phys. Rev. Lett. 77, 3865–3868. doi: 10.1103/PhysRevLett.78.1396
Reyes-Lillo, S. E., Rangel, T., Bruneval, F., and Neaton, J. B. (2016). Effects of quantum confinement on excited state properties of SrTiO3 from ab initio many-body perturbation theory. Phys. Rev. B 94:041107. doi: 10.1103/PhysRevB.94.041107
Sasaki, T., Fukushima, J., Hayashi, Y., and Takizawa, H. (2014). Synthesis of photoluminescence properties of Mn4+-doped BaMg6Ti6O19 phosphor. Chem. Lett. 43, 1061–1063. doi: 10.1246/cl.140282
Sato, Y., Kato, H., Kobayashi, M., Masaki, T., Yoon, D.-H., and Kakihana, M. (2014). Tailoring of deep-red luminescence in Ca2SiO4:Eu2+. Angew. Chem. Int. Ed. 53, 7756–7759. doi: 10.1002/anie.201402520
Seki, K., Kamei, S., Uematsu, K., Ishigaki, T., Toda, K., and Sato, M. (2013). Enhancement of the luminescence efficiency of Li2TiO3:Mn4+ red emitting phosphor for white LEDs. J. Ceram. Process. Res. 14, s67–s70.
Srivastava, A. M., and Beers, W. W. (1996). Luminescence of Mn4+ in the distorted perovskite Gd2MgTiO6. J. Electrochem. Soc. 143, L203–L205. doi: 10.1149/1.1837087
Takeda, Y., Kato, H., Kobayashi, M., Kobayashi, H., and Kakihana, M. (2015). Photoluminescence properties of Mn4+-activated perovskite-type titanates, La2MTiO6:Mn4+ (M = Mg and Zn). Chem. Lett. 44, 1541–1543. doi: 10.1246/cl.150748
Takeda, Y., Kato, H., Kobayashi, M., Nozawa, S., Kobayashi, H., and Kakihana, M. (2017). Photoluminescence properties of double perovskite tantalates activated with Mn4+, AE2LaTaO6:Mn4+ (AE = Ca, Sr, and Ba). J. Phys. Chem. C 121, 18837–18844. doi: 10.1021/acs.jpcc.7b06280
Toda, K., Kawakami, Y., Kousaka, S., Ito, Y., Komeno, A., Uematsu, K., et al. (2006). New silicate phosphors for a white LED. IEICE Trans. Electoron. E89–C, 1406–1412.
Tsai, Y. T., Chiang, C. Y., Zhou, W., Lee, J. F., Sheu, H. S., and Liu, R. S. (2015). Structural ordering and charge variation induced by cation substitution in (Sr,Ca)AlSiN3:Eu phosphor. J. Am. Chem. Soc. 137, 8936–8939. doi: 10.1021/jacs.5b06080
Uheda, K., Hirosaki, N., Yamamoto, Y., Naito, A., Nakajima, T., and Yamamoto, H. (2006). Luminescence properties of a red phosphor, CaAlSiN3:Eu2+, for white light-emitting diodes, Electrochem. Solid State Lett. 9, H22–H25. doi: 10.1149/1.2173192
Vanderbilt, D. (1991). Implementation of ultrasoft pseudopotentials in ab initio molecular dynamics. Phys. Rev. B 43, 6796–6799. doi: 10.1103/PhysRevB.43.6796
Wang, L., Xie, R. J., Suehiro, T., Takeda, T., and Hirosaki, N. (2018). Down-conversion nitride materials for solid state lighting: recent advances and perspectives. Chem. Rev. 118, 1951–2009. doi: 10.1021/acs.chemrev.7b00284
Wang, L., Yuan, L., Xu, Y., Zhou, R., Qu, B., Ding, N., et al. (2014). Luminescent properties of LaLiTaO6:Mn4+ and its application as red emission LEDs phosphor. Appl. Phys. A: Mater. Sci. Process. 117, 1777–1783. doi: 10.1007/s00339-014-8827-z
Wang, Y., Tang, K., Zhu, B., Wang, D., Hao, Q., and Wang, Y. (2015). Synthesis and structure of a new layered oxyfluoride Sr2ScO3F with photocatalytic property. Mater. Res. Bull. 65, 42–46. doi: 10.1016/j.materresbull.2015.01.042
Watanabe, H., and Kijima, N. (2009). Crystal structure and luminescence properties of SrxCa1−−xAlSiN3:Eu2+ mixed nitride phosphors. J. Alloy Compd. 475, 434–439. doi: 10.1016/j.jallcom.2008.07.054
Wen, D., Kuwahara, H., Kato, H., Kobayashi, M., Sato, Y., Masaki, T., et al. (2016). Anomalous orange light-emitting (Sr,Ba)2SiO4:Eu2+ phosphors for warm white LEDs. ACS Appl. Mater. Interfaces 8, 11615–11620. doi: 10.1021/acsami.6b02237
Wu, W.-L., Fang, M.-H., Zhou, W., Lesniewski, T., Mahlik, S., Grinberg, M., et al. (2017). High color rendering index of Rb2GeF6:Mn4+ for light-emitting diodes. Chem. Mater. 29, 935–939. doi: 10.1021/acs.chemmater.6b05244
Xi, L., Pan, Y., Zhu, M., Lian, H., and Lin, J. (2017). Room-temperature synthesis and optimized photoluminescence of a novel red phosphor NaKSnF6:Mn4+ for application in warm WLEDs. J. Mater. Chem. C 5, 9255–9263. doi: 10.1039/C7TC02996A
Ye, T., Li, S., Wu, X., Xu, M., Wei, X., Wang, K., et al. (2013). Sol-gel preparation of efficient red phosphor Mg2TiO4:Mn4+ and XAFS investigation on the substitution of Mn4+ for Ti4+. J. Mater. Chem. C 1, 4327–4333. doi: 10.1039/C3TC30553H
Zhang, S., Hu, Y., Duan, H., Fu, Y., and He, M. (2017). An efficient, broad-band red-emitting Li2MgTi3O8:Mn4+ phosphor for blue-converted white LEDs. J. Alloys Compd. 693, 315–325. doi: 10.1016/j.jallcom.2016.09.203
Keywords: photoluminescence, scandium oxyfluoride, layered perovskite, tetravalent manganese, red emission
Citation: Kato H, Takeda Y, Kobayashi M, Kobayashi H and Kakihana M (2018) Photoluminescence Properties of Layered Perovskite-Type Strontium Scandium Oxyfluoride Activated With Mn4+. Front. Chem. 6:467. doi: 10.3389/fchem.2018.00467
Received: 20 July 2018; Accepted: 18 September 2018;
Published: 04 October 2018.
Edited by:
Nobuhito Imanaka, Osaka University, JapanReviewed by:
Tomokatsu Hayakawa, Nagoya Institute of Technology, JapanSaburo Hosokawa, Kyoto University, Japan
Copyright © 2018 Kato, Takeda, Kobayashi, Kobayashi and Kakihana. This is an open-access article distributed under the terms of the Creative Commons Attribution License (CC BY). The use, distribution or reproduction in other forums is permitted, provided the original author(s) and the copyright owner(s) are credited and that the original publication in this journal is cited, in accordance with accepted academic practice. No use, distribution or reproduction is permitted which does not comply with these terms.
*Correspondence: Hideki Kato, aGlkZWtpLmthdG8uZTJAdG9ob2t1LmFjLmpw