- 1Key Laboratory of Catalysis and Materials Science of the State Ethnic Affairs Commission & Ministry of Education, Hubei Province, College of Resources and Environmental Science, South-Central University for Nationalities, Wuhan, China
- 2College of Chemistry and Chemical Engineering, Wuhan University of Science and Technology, Wuhan, China
Anatase TiO2 (A-TiO2) usually exhibits superior photocatalytic activity than rutile TiO2 (R-TiO2). However, the phase transformation from A-TiO2 to R-TiO2 will inevitably happens when the calcination temperature is up to 600°C, which hampers the practical applications of TiO2 photocatalysis in hyperthermal situations. In this paper, high energy faceted TiO2 nanosheets (TiO2-NSs) with super thermal stability was prepared by calcination of TiOF2 cubes. With increase in the calcination temperature from 300 to 600°C, TiOF2 transforms into TiO2 hollow nanoboxes (TiO2-HNBs) assembly from TiO2-NSs via Ostwald Rippening process. Almost all of the TiO2-HNBs are disassembled into discrete TiO2-NSs when calcination temperature is higher than 700°C. Phase transformation from A-TiO2 to R-TiO2 begins at 1000°C. Only when the calcination temperature is higher than 1200°C can all the TiO2-NSs transforms into R-TiO2. The 500°C-calcined sample (T500) exhibits the highest photoreactivity toward acetone oxidation possibly because of the production of high energy TiO2-NSs with exposed high energy (001) facets and the surface adsorbed fluorine. Surface oxygen vacancy, due to the heat-induced removal of surface adsorbed fluoride ions, is responsible for the high thermal stability of TiO2-NSs which are prepared by calcination of TiOF2 cubes.
Introduction
Semiconductor photocatalysis has attracted much attention due to its potential applications such as water (Regmi et al., 2018; Xu et al., 2018) and air purification (Wen et al., 2015; Cui et al., 2017; Li et al., 2017a; Qi et al., 2017) and water splitting for clean H2 energy (Cheng et al., 2018) due to its peculiar chemical and physical properties. As a typical semiconductor photocatalyst, anatase TiO2 (A-TiO2) usually shows excellent photocatalytic activity. However, it usually transforms into poor photoreactive rutile TiO2 (R-TiO2) when calcination temperature is higher than about 600°C, which hampers the practical applications of A-TiO2 in hyperthermal situations (Lv et al., 2011; Liang et al., 2017). For example, the temperature of some industrial waste gases after burning can be as high as 900–1000°C, which makes the photocatalytic oxidation technology lose its power due to the phase transformation of A-TiO2 at such high temperature. There are many photocatalytically active stable TiO2-coated ceramic materials which are used for the control of organic contaminants, including sanitary wares, bathroom tiles and self-cleaning glass. They require high processing temperatures and therefore need excellent stability at high temperature (Periyat et al., 2009). Thus, exploration of thermally stable TiO2 photocatalyst is very important but this question remains unsolved.
As one of volatile organic compounds (VOCs), acetone is a widely used solvent especially in chemical plant. The emission of acetone can not only cause the problems to global environment, but also bring harms to human health (Zhu et al., 2016). When the concentration of acetone vapor is higher than 500 ppm, it can irritate eyes and discomfort respiratory system (Li et al., 2018). So, it is of great importance to explore an efficient method to decompose of acetone. Because semiconductor photocatalysis is considered as an environmentally benign way, it is no doubt very promising for VOCs removal under normal conditions.
It is generally accepted that the quantum efficiency of photocatalysis is highly related to the physical properties of TiO2 such as crystal structure, relative crystallinity, size of the particle, and specific surface area (Xu et al., 2007; Lan et al., 2015; Wang et al., 2015, 2016; Wen et al., 2015; Li et al., 2016; Sajan et al., 2016; Liang et al., 2017; Xia et al., 2017; Lin et al., 2018a,b). Recently, growing interesting has been devoted to the synthesis of TiO2 nanocrystals with exposed high energy {001} facets by surface fluorination of TiO2 to reduce the surface energy (Yang et al., 2008, 2009; Chen et al., 2010; Yu et al., 2014; Sajan et al., 2016; Liang et al., 2017). On considering that TiOF2 cubes are fluorine-containing materials, they prefer to transform into high energy TiO2 nanocrystals during calcination. After the removal of surface adsorbed fluorine ions at high temperature, the formed oxygen vacancy (OV) on the surface of the photocatalyst will prevent the fusion of neighboring A-TiO2 nanocrystals, which is believed to improve the thermal stability of TiO2 nanocrystals. Herein, we systematically studied the dependence of the structure and photocatalytic activity of TiOF2 cubes on the calcination temperature. Acetone was selected as the targeted VOCs to evaluate the photoreactivity of the as-prepared photocatalyst.
Experimental Section
Synthesis
Precursor TiOF2 was synthesized through the solvothermal reaction of Tetrabutyl titanium (TBT), acetic acid (HAc) and hydrofluoric acid (HF) (Huang et al., 2013). Typically, 20.0 g of TBT was dropwise added into the mixed solution containing 6.4 ml of HF (47 wt%) and 40.0 ml of HAc under magnetic stirring. The resulted white suspensions were transferred to an autoclave with volume of 100 ml, which was then kept at 200°C for 2 h. The white deposition was collected and washed with ethanol and distilled water until the filtrate is neutral (pH7). After oven dry at 80°C, we obtained the precursor TiOF2.
Precursor TiOF2 was then calcined at certain temperature (300-1200°C) for 2 h by keeping the same heating rate (5°C min−1). The obtained photocatalyst is denoted as Tx (Table 1), where x is the calcination temperature. For example, T500 is that the photocatalyst which was prepared by calcination of the precursor TiOF2 at 500°C for 2 h.
Characterization
XRD patterns of the photocatalysts were performed on a X-ray diffractometer (D8- advance, Bruker Co., German), and the scan rate of Cu Ka radiation keeps 0.02° 2θ s−1, using an accelerated voltage and applied current of 15 kV and 20 mA, respectively. We observe the morphology of the prepared photocatalyst by an FESEM with an acceleration voltage of 20 kV (Hitach, Japan) and a TEM (Tecnai G20, USA) using an acceleration voltage of 200 kV, respectively. The optical property of the photocatalyst was measured by a spectrophotometer (UV-2550, Shimadzu, Japan) from 200 to 800 nm using BaSO4 as background. FTIR was obtained on a infrared spectrometer (NeXUS 470) using the KBr pellet technique. XPS was recorded using monochromatic Al-Ka radiation under vacuum at 2 × 10−6 Pa on a photoelectron spectrometer (VG Multilab 2000). The C1s peak at 284.8 eV originated from the surface adventitious carbon is used to reference all the binding energies. EPR signal of the photocatalyst was recorded in an EPR spectrometer (JES-FA 200, JEOL) at room temperature (frequency of 100 kHz and microwave power of 0.99 mW). Nitrogen sorption isotherm was measured on an ASAP 2020 nitrogen adsorption equipment (Micromeritics, USA). Before investigating the surface areas of the photocatalysts, all samples were degassed firstly at 200°C.
Photoelectrochemical Measurements
We use CHI760e as electrochemical workstation (Shanghai, China) to measure the transient photocurrent, EIS Nyquist plots and Mott-Schottky plots in a standard there-electrode system, where Pt wire was used as the counter electrode, and the prepared samples and Ag/AgCl in saturated KCl were used as the working and reference electrode, respectively. During the measurement, 0.4 M Na2SO4 was used as electrolyte solution. In the Mott-Schottky measurement, direct current potential polarization was kept at a fixed frequency, and the working electrode was prepared on a glassy carbon electrode. Before the test of photocurrent, 50 mg photocatalysts and 30 μL Nafion were dispersed into 1 mL water/absolute ethanol mixed solvent (v/v = 1/1), and then the mixed aqueous solution was dispersed uniformly through ultrasound to form a homogeneous catalyst colloid. The ITO/TiO2 electrode was prepared using the as-prepared photocatalyst colloid as precursor by a drip coating method. The light source is a 3W LED lamp (Shenzhen LAMPLIC, China) emitted mainly at 365 nm. The intensity for the lamp at working distance is measured to be 0.41 W/cm2.
Evaluation of the Photocatalytic Activity
Photocatalytic oxidation of gasous acetone was used to evaluate the photocatalytic activity of the photocatalyst, which was performed in a 15 L reactor at ambient temperature under UV light irradiation. 0.3 g of the powder was firstly dispersed in 30 mL of double distilled water by sonicating treatment for 5 min. The obtained suspensions were then evenly transferred into three glass dishes in diameters of about 7.0 cm. After drying at 80°C for about 2 h, the dishes that have been coated with a layer of the photocatalyst were placed in the reactor, and then 10 uL of acetone was injected into the reactor by a microsyringe. The vaporated acetone then begins to adsorb on the surface of the photocatalyst. Thirty minutes later, the adsorption–desorption equilibrium of acetone can be established. The photocatalytic oxidation of acetone begins after turning on the UV light, which is 5 cm above the dishes. The concentrations of acetone and the produced carbon dioxide in the reactor were determined online with a Photoacoustic IR Multigas Monitor (Model 1412, INNOVA). Before irradiation, the initial concentration of acetone after the adsorption equilibrium was about 300 ppm, which almost keeps unchanged for about 5 min before lighting a UV lamp (15W@365 nm).
Results and Discussion
Effect of Calcination Temperature on Phase Structure
Phase structure of the photocatalyst is important to the photoreactivity. Therefore, we used XRD to study the phase evolution of the photocatalyst during calcination. From Figure 1, a broad peak centering at 2θ = 23.4°, which corresponds to the (100) plane diffraction of TiOF2, was observed for the prepared precursor, and no any peak of TiO2 phases (A-TiO2 and R-TiO2) exists, indicating the successful synthesis of TiOF2(Huang et al., 2013). After calcination at 300°C for 2 h, the phase struccture of the sample (T300) almost keeps unchanged. With increase in the calcination temperature to 400°C, the peak intensity for TiOF2 decreases. Simultaneously, a small peak at 2θ = 25.3°, which corresponds to the (101) plane diffraction of A-TiO2, can be observed for T400 sample, indicating that phase transformation from TiOF2 to anatase begins. This phase transformation becomes obvious after calcination of TiOF2 at 500°C, and the prepared TiOF2 totally transforms into anatase TiO2 at 600°C.
It is proposed that the reaction for the heat-induced phase transformation from TiOF2 to TiO2 is as follows (Equation 1) (Zhao et al., 2018).
Further increase in the calcination from 600 to 900°C, the peak intensity of A-TiO2 increases, indicating the enhanced crystallization. Meanwhile, the narrowing of the width for the (101) plane diffraction peak indicates the increase of A-TiO2 crystallite size. The peak intensity for the (101) peak of anatase TiO2 begins to decrease at calcination temperature of 1000°C, which is a sign of anatase-to-rutile phase transfermation. The formation of rutile phase is confirmed from the XRD pattern of the T1100 sample due to the formation of a small peak at 2θ = 27.3°, which corresponds to the (110) plane diffraction of R-TiO2. Only when the calcination temperature reaches 1200°C can all of the A-TiO2 transform into R-TiO2.
Usually, A-TiO2 nanocrystals begin transform into R-TiO2 at about 600°C. However, in the present study, the temperature for anatase-to-rutile transformation is as high as 1100°C, which indicates that these samples are promising to be used in hyperthermal situations.
Evolution of the Morphology
The SEM images of the precursor TiOF2 is shown in Figure 2. It can be clearly observed that these TiOF2 nanoparticles are in cubic shape and relatively monodispersed (Figure 2a), which is consistent with the reported literatures (Chen et al., 2012; Wang et al., 2012). From the high resolution SEM image shown in Figure 2b, we can estimate that the sidelength of the TiOF2 cube is about 250 nm.
After calcination of the precursor TiOF2 at 300°C, the morphology of the resulted sample (T300) almost keeps unchanged (SEM image not shown here). However, some nanoboxes with hollow interiors can be clearly seen from the SEM image of T400 sample (Figure 3a). These anatase TiO2 hollow nanoboxes (TiO2-HNBs) assemble from TiO2 nanosheets (TiO2-NSs) with exposed high energy (001) facets (Wen et al., 2011). The thickness of the TiO2-NSs is about 30 nm. It has been proven that high energy TiO2 nanosheets with exposed (001) facets can be prepared by using fluoride ions as shape-directing reagent (Yang et al., 2008; Lv et al., 2012). Then, it is not hard to understand the formation of high energy TiO2-NSs during calcination of TiOF2, a kind of fluorine-containing materials.
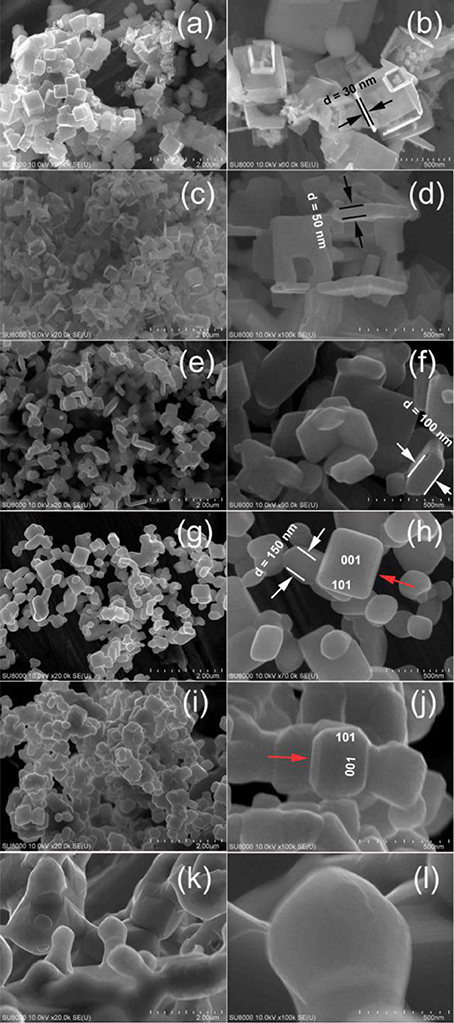
Figure 3. Comparison of the SEM images for T400 (a,b), T500 (c,d), T600 (e,f), T700 (g,h), T1100 (i,j), and T1200 (k,l) samples.
Since the sidelength of the obtained TiO2-HNBs is similar as these precursor TiOF2 cube, it is proposed that the formation of TiO2-HNBs assembly from TiO2-NSs is through a Ostwald Rippening process (Lou et al., 2008; Huang et al., 2013).
After calcination at 500°C, most of the TiOF2 cubes transform into TiO2-HNBs (Figure 3c). Figure 3d shows an enlarged SEM image of a broken TiO2 hollow nanobox, from which we can see that the thickness of the TiO2 nanosheet increases to about 50 nm, much thicker than that of T400 sample.
The structure of TiO2-HNBs was further confirmed by the corresponding TEM image of T500 sample (Figure 4a), from which we can observe the presence of some erected TiO2-NSs and a TiOF2 cube that has not totally transformed into anatase TiO2. This is consistent with the corresponding XRD characterization result (Figure 1d). From the side view HRTEM image of a discrete TiO2-NS (Figure 4b), we can clearly see the lattice spacing of 0.235 nm that parallels to the top and bottom facets, which corresponds to the (001) planes of A-TiO2 (Han et al., 2009). This confirms that the obtained TiO2-HNBs are assembly from high energy TiO2-NSs with exposed (001) facets (Wang et al., 2010).
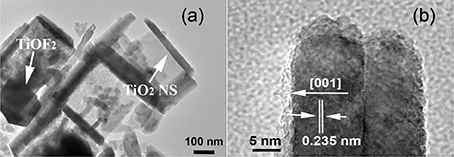
Figure 4. TEM image (a) and high-resolution TEM image (b) of T500 sample, arrows in (a) indicating the presence of TiOF2 cube and TiO2 nanosheet (TiO2-NS).
When the calcination temperature increases to 600°C, we can see that almost all of the TiO2-HNBs decompose into discrete TiO2-NSs (Figure 3e). From the truncated biypramidal shape of a TiO2 nanocrystals (Figure 3f), we can estimate that the thickness of the TiO2-NSs is about 100 nm (T600 sample), which increases to ca. 150 nm for T700 sample (Figures 3g,h). Even calcined at 1100°C, some TiO2 nanosheets still keep bipyramidal shape (decahedron) with exposed (001) and (101) facets (Figures 3i,j), further indicating the thermal stability of high energy TiO2-NSs.
The bipyramidal shapes of TiO2 nanostructures disappear (Figures 3k,l) when calcination of TiOF2 cubes at 1200°C. This can be explained by the sintering of the sample due to phase transformation from A-TiO2 to R-TiO2 (Figure 1).
By comparing the morphologies of TiO2-NSs from Figure 3, we can clearly see that heat treatment of TiOF2 cubes only results in the growth of TiO2 nanosheet along (001) direction. Scheme 1 illustrates the morphology evolution and phase transformation of TiOF2 cube during calcination.
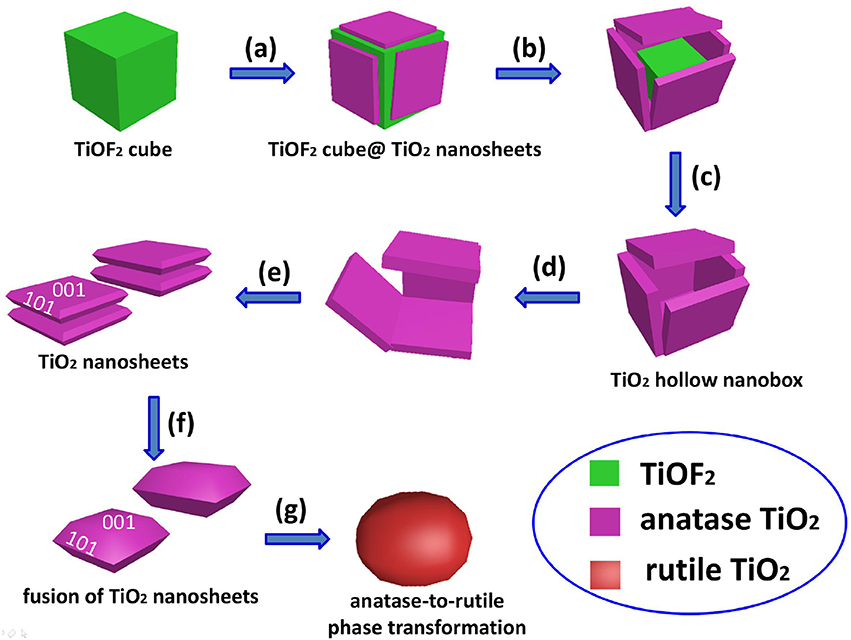
Scheme 1. Morphology evolution and phase transformation of TiOF2 cube during calcination at different temperatures: (a) 300°C, (b) 400°C, (c) 500°C, (d) 600°C, (e) 700°C, (f) 1100°C, and (g) 1200°C.
Uv-vis Absorption and FTIR Spectra
Light-harvesting ability plays a very important role on the photoreactivity of the photocatalyst (Li et al., 2017b). Therefore, we compared the UV-vis absorption spectra of the samples. It can be seen that from Figure 5 that, when calcination temperature is below 1100°C, all samples possess similar absorption spectra (Figure 5). The onset of the UV-vis absorption spectrum for T400 sample is at 389 nm, corresponding to a bangap of 3.19 eV. However, the absorption edge was obviously red-shifted for T1200 sample. The onset of the spectrum for T1200 sample begins at 424 nm, corresponding to a bandgap of 2.92 eV, which can be ascribed to the phase transformation (Figure 1).
Figure 6 compares the FTIR spectra of the photocatalysts treated at different calcination temperature. From which, it can be seen that all samples exhibit strong absorption peaks cenerting at about 3,427, 1,628, 1,403, and 554 cm−1. The peaks of 3,427 and 1,628 cm−1 originate from the -OH groups/H2O due to the adsorption of moisture from the air, while the peaks centering at about 1403 and 554 cm−1 originate from the vibration of Ti-O and O-Ti-O. Carefully view shows that there is a strong absorption peak at 978 cm−1, which decreases with increasing in the calcination temperature. This peak was attributed to the vibration of Ti-F bond of TiOF2. When calcination temperature increases to 600°C, the vibration of Ti-F disappears because of the complete phase transformation of TiOF2-to-anatase TiO2 (Figure 1; Zhao et al., 2018).
Analysis of XPS Spectrum
Figure 7A compares the XPS survey spectra of photocatalysts for T400, T500, and T600 photocatalysts. From which, it can be observed that the XPS spectra are similar. All these samples contain titanium (Ti), oxygen (O), fluorine (F), and carbon (C) elements. The C element originates from the adventitious hydrocarbon from the XPS instrument itself. We can also be clearly see that the peak intensity of F element tends to decrease with increasing the calcination temperature from 400 to 600°C. The atomic ratios of F:Ti were determined to be 1.19 for T400 sample, 0.50 for T500 sample, and 0.17 for T600 sample, respectively. The steady decrease in F content with increase in the calcination is because of the TiOF2 to A-TiO2 phase transformation (Figure 1 and Equation 1), and the removal of adsorbed fluoride ions on the surface of A-TiO2.
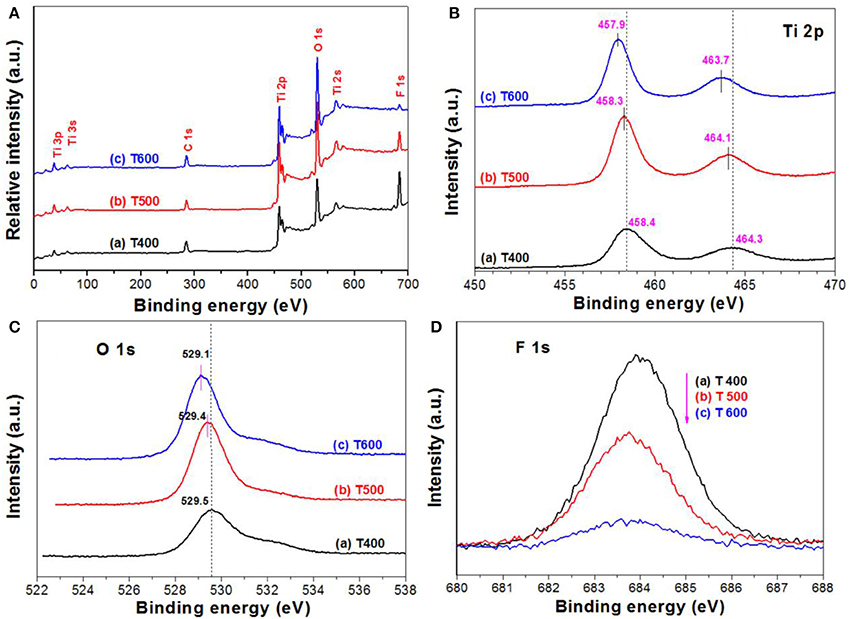
Figure 7. XPS survey spectra of the photocatalysts (A), and the corresponding high resolution XPS spectra in Ti 2p (B), O 1s (C), and F 1s (D) regions, respectively.
Figures 7B,C show the high resolution XPS spectra in Ti 2p and O 1s regions, respectively. It can be seen that, both the binding energies for Ti 2p and O 1s of the samples steady decrease with increasing the calcination temperature. This is attributed to the TiOF2-to-TiO2 phase transformation (Figure 1 and Equation 1), and the formation of oxygen vacancy due to the removal of surface adsorbed fluoride ions over TiO2 upon calcination (Lv et al., 2011). It has been accepted that almost all of the fluoride ions adsorbed on the surface of high energy TiO2 nanosheets can be removed after calcination at 500°C.
The F 1s binding energy peak centering at about 684 eV (Figure 7D) is designated to the surface fluoride (Ti-F). No peak with binding energy of about 688.5 eV, corresponding to the lattice F− of TiO2, is found in all the photocatalysts, indicating that calcination of TiOF2 cannot results in the doping of fluorine into anatase TiO2 (Yu et al., 2002). We can also clearly see that the peak intensity of F 1s steady decreases with increasing the calcination temperature, this can be attributed to the heat-induced phase transformation from TiOF2 to A-TiO2 and the desorption of surface adsorbed fluoride ions (Yang et al., 2008).
Photocatalytic Oxidation of Acetone
Photocatalytic oxidation of acetone was used to monitor the photoreactivity of the prepared photocatalyst. The reaction for the photocatalytic oxidation of acetone is as follows (Equation 2).
Figure 8A compares the relative photoreactivity of the photocatalyst by calculation the decomposed acetone within 2 h. It was found that the photocatalytic activity of the precursor TiOF2 can be neglected (only 4.0 ppm acetone was decomposed). The poor photocatalytic activity of TiOF2 is possibly due to its easy recombination of photo-generated carriers.
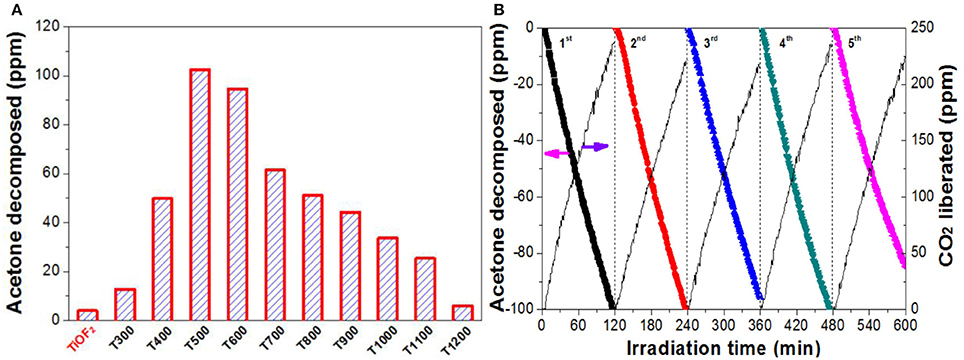
Figure 8. Comparison of the photoreactivity of the photocatalyst (A), and the recycling use of T500 sample (B) in photocatalytic oxidation of acetone.
The photocatalytic activity of the photocatalyst increases with increase in the calcination temperature from 300°C (12.8 ppm) to 500°C (102.5 ppm), which is due to the production of A-TiO2. When further increase in the heat temperature from 600°C (94.6 ppm) to 1100°C (25.4 ppm), the photocatalytic activity of the photocatalyst steady decreases, possibly due to the decreased specific surface area (Table 1). Only 5.9 ppm of acetone was found to decompose when T1200 sample is used as photocatalyst. This is because of the complete A-TiO2 to R-TiO2 phase transformation (Figure 1) and smallest BET surface area (0.4 m2g−1).
Reusability of the photocatalyst is also important from the viewpoint of the practical applications (Liu et al., 2010). Therefore, we monitored the recycling use of T500 sample for 5 times in acetone oxidation (Figure 8B). It can be seen that no obvious reactivity decrease was observed for T500 sample even continual use for 5 times, indicating that T500 sample is potential to be used in practical applications.
Mechanism
The value of photocurrent is usually used to evaluate the ability to generate and transfer of charge carriers for illuminated semiconductor photocatalyst (Cheng et al., 2018; Huang et al., 2018). The photocurrent of the prepared photocatalyst was therefore tested for several on-off cycles (Figure 9). It can be seen that, when irradiation of the TiO2 film electrodes, obvious prompt photocurrent signals are produced, which exhibit good reproducibility. When the lamp is turned off, the photocurrent value for all the TiO2 film electrodes are instantaneously close to zero. We can also see, with increasing the calcination temperature from 400 to 600°C, the photocurrent of the photocatalyst increases first and then decreases. The photocurrent of T500 sample is the as high as 2.8 uAcm−2, which is much higher than that of T400 (1.1 uAcm−2) and T600 (2.3 uAcm−2) samples. The photoreactivity of semiconductor photocatalyst is closely related to the efficiency of the separation of the photo-generated electron-hole pairs (Fu et al., 2008). So it is safe to predict that the photoreactivity of T500 is higher than that of T400 and T600 samples, which keeps in line with the experimental results (Figure 8).
It has been accepted that fluoride ions show strong affinity to titanium (Equation 3), and the photoreactivity of TiO2 nanocrystals can be obviously improved after the introduction of surface fluorination (Minero et al., 2000; Xu et al., 2007; Cheng et al., 2008). According to the study of Yu et al., the difference in surface energy makes the photo-generated electrons and holes migrate to (101) and (001) facets, respectively (Yu et al., 2014). The CB electrons aggregated in (101) facets are captured by surface adsorbed oxygen to produce super oxygen radicals (), while the VB holes aggregated on the (001)facets are transfer into hydroxyl radicals (·OH). Both and ·OH are important reactive oxygen species (ROSs) for the degradation of organics. When compared with pristine TiO2, surface fluorination of TiO2 changes the state of the formed hydroxyl radicals from surface bounded ·OH radicals (Equation 6) to mobile ·OH radicals (·OHfree). This is because the displacement of fluoride to surface OH− groups (Equation 3) induces the direct oxidization solvent water by holes (Equation 7).
As free ·OH radical are more active than surface bounded ·OH radicals, the oxidation of acetone into CO2 and H2O is greatly enhanced due to the attacks of super oxygen radicals and free ·OH radicals (Equation 8). The proposed mechanism for the enhanced photoreactivity of surface fluorinated TiO2 nanosheet toward acetone oxidation is shown in Scheme 2.
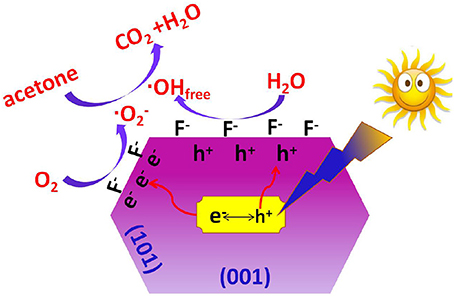
Scheme 2. Proposed mechanism for the enhanced photocatalytic activity of surface fluorinated TiO2 nanosheets with exposed high energy (001) facets toward acetone oxidation.
Recently, significantly growing interest has been devoted to studying of hierarchical nanostructures due to their unique properties and widespread potential applications (Lou et al., 2008). For example, when compared with solid spheres, TiO2 hollow spheres usually shows much higher photocatalytic activity mainly because they possess better light-harvesting ability (Li et al., 2009). In the present study, the hierarchically structured T500 sample, that is TiO2 hollow nanobox assembly from TiO2 nanosheets should also benefit the use of light (Figure 5), enhancing the photoreactivity.
However, the photoreactivity of TiO2-NSs steady decrease with increase in the calcination temperature from 500 to 1100°C due to the collapse of the hierarchical TiO2 hollow nanobox and the removal of surface adsorbed fluorine, leaving surface oxygen vacancy (Figure 8; Cheng et al., 2018).
The formation of surface oxygen vacancy was proved by electron paramagnetic resonance (EPR) technique. It was found that the signal intensity for oxygen vacancy of the photocatalyst increases with increase in the calcination temperature from 400 to 600°C (Figure 10) due to the heat-induced desorption of surface adsorbed fluoride ions (Equation 9).
It was believed that only when the crystalline size of the nanocrystal is larger than a critical size can phase transformation begin (Padmanabhan et al., 2007; Periyat et al., 2008, 2009). Therefore, the growth of TiO2 nanocrystal is a prerequisite before the phase transformation of A-TiO2 to R-TiO2. The growth of pristine TiO2 nanocrystal is relatively easy by formation of =Ti-O-Ti= chain between two neighboring TiO2 nanoparticles (Equation 10). However, the growth of the =Ti-O-Ti= chain is prevented due to the formation of surface oxygen vacancy (Equation 11). Only when the lattice oxygen is diffused from the bulk to the surface of TiO2 nanosheet with oxygen vacancy at high temperaure can the fusion of neighboring TiO2-NSs become possible (Lv et al., 2011). Therefore, it is not hard to understand the super thermal stability of TiO2-NSs prepared by calcination of TiOF2 cubes.
After the removal of surface adsorbed fluoride ions by calcination, these TiO2-NSs prefer to aggregate and grow along (001) direction to reduce the high surface energy (Lv et al., 2011). Then we can clearly observe the steady increase in the thickness of TiO2-NSs (Figure 3).
Conclusions
TiO2 nanosheets with high thermal stability were prepared by calcination of TiOF2 cubes. The anatase-to-rutile phase transformation temperature reaches as high as 1100°C. 500°C-calcined sample shows the highest photoreactivity toward acetone oxidation due to the surface fluorination. The high thermal stability of TiO2 nanosheets is ascribed to the introduction of surface oxygen vacancy after removal of the surface adsorbed fluoride ions, which prevents the growth of TiO2 nanosheets. The present study provide a novel way in design of thermally stable materials.
Author Contributions
TS and YD performed the experiments. KL planned the project, designed the experiments, and wrote the manuscript. ZH, QL and XL assisted in the analysis and interpretation of the data. ML revised the manuscript.
Conflict of Interest Statement
The authors declare that the research was conducted in the absence of any commercial or financial relationships that could be construed as a potential conflict of interest.
Acknowledgments
This work was supported by the National Natural Science Foundation of China (51672312, 21571192 and 21373275), the Science and Technology Program of Wuhan (2016010101010018) and Fundamental Research Funds for the Central Universities, South-Central University for Nationalities (CZT18016).
References
Chen, J. S., Tan, Y. L., Li, C. M., Cheah, Y. L., Luan, D., Madhavi, S., et al. (2010). Constructing hierarchical spheres from large ultrathin anatase TiO2 nanosheets with nearly 100% exposed (001) facets for fast reversible lithium storage. J. Am. Chem. Soc. 132, 6124–6130. doi: 10.1021/ja100102y
Chen, L., Shen, L. F., Nie, P., Zhang, X. G., and Li, H. S. (2012). Facile hydrothermal synthesis of single crystalline TiOF2 nanocubes and their phase transitions to TiO2 hollow nanocages as anode materials for lithium-ion battery. Electrochim. Acta 62, 408–415. doi: 10.1016/j.electacta.2011.12.058
Cheng, J. S., Hu, Z., Lv, K. L., Wu, X. F., Li, Q., Li, Y. H., et al. (2018). Drastic promoting the visible photoreactivity of layered carbon nitride by polymerization of dicyandiamide at high pressure. Appl. Catal. B 232, 330–339. doi: 10.1016/j.apcatb.2018.03.066
Cheng, X. F., Leng, W. H., Liu, D. P., Xu, Y. M., Zhang, J. Q., and Cao, C. N. (2008). Electrochemical preparation and characterization of surface-fluorinated TiO2 nanoporous film and its enhanced photoelectrochemical and photocatalytic properties. J. Phys. Chem. C 112, 8725–8734. doi: 10.1021/jp7097476
Cui, W., Li, J. Y., Cen, W. L., Sun, Y. J., Lee, S. C., and Dong, F. (2017). Steering the interlayer energy barrier and charge flow via bioriented transportation channels in g-C3N4: enhanced photocatalysis and reaction mechanism. J. Catal. 352, 351–360. doi: 10.1016/j.jcat.2017.05.017
Fu, H. B., Xu, T. G., Zhu, S. B., and Zhu, Y. F. (2008). Photocorrosion inhibition and enhancement of photocatalytic activity for ZnO via hybridization with C60. Environ. Sci. Technol. 42, 8064–8069. doi: 10.1021/es801484x
Han, X. G., Kuang, Q., Jin, M. S., Xie, Z. X., and Zheng, L. S. (2009). Synthesis of titania nanosheets with a high percentage of exposed (001) facets and related photocatalytic properties. J. Am. Chem. Soc. 131, 3152–3153. doi: 10.1021/ja8092373
Huang, T. T., Li, Y. H., Wu, X. F., Lv, K. L., Li, Q., Li, M., et al. (2018). In-situ transformation of Bi2WO6 to highly photoreactive Bi2WO6@Bi2S3 nanoplate via ion exchange. Chin. J. Catal. 39, 718–727. doi: 10.1016/S1872-2067(17)62913-9
Huang, Z. A., Wang, Z. Y., Lv, K. L., Zheng, Y., and Deng, K. J. (2013). Transformation of TiOF2 cube to a hollow nanobox assembly from anatase TiO2 nanosheets with exposed {001} facets via solvothermal strategy. ACS Appl. Mater. Interfaces 5, 8663–8669. doi: 10.1021/am4023048
Lan, J. F., Wu, X. F., Lv, K. L., Si, L. L., and Deng, K. J. (2015). Fabrication of TiO2 hollow microspheres using K3PW12O40 as template. Chin. J. Catal. 36, 2237–2243. doi: 10.1016/S1872-2067(15)60987-1
Li, X. F., Lv, K. L., Deng, K. J., Tang, J. F., Su, R., Sun, J., et al. (2009). Synthesis and characterization of ZnO and TiO2 hollow spheres with enhanced photoreactivity. Mater. Sci. Eng. B 158, 40–47. doi: 10.1016/j.mseb.2008.12.036
Li, X., Yu, J. G., and Jaroniec, M. (2016). Hierarchical photocatalysts. Chem. Soc. Rev. 45, 2603–2636. doi: 10.1039/C5CS00838G
Li, Y. H., Lv, K. L., Ho, W. K., Dong, F., Wu, X. F., and Xia, Y. (2017a). Hybridization of rutile TiO2 (rTiO2) with g-C3N4 quantum dots (CN QDs): an efficient visible-light-driven Z-scheme hybridized photocatalyst. Appl. Catal. B 202, 611–619. doi: 10.1016/j.apcatb.2016.09.055
Li, Y. H., Lv, K. L., Ho, W. K., Zhao, Z. W., and Yu, H. (2017b). Enhanced visible-light photo-oxidation of nitric oxide using bismuth-coupled graphitic carbon nitride composite heterostructures. Chin. J. Catal. 38, 321–329. doi: 10.1016/S1872-2067(16)62573-1
Li, Y. H., Wu, X. F., Ho, W. K., Lv, K. L., Li, Q., Li, M., et al. (2018). Graphene-induced formation of visible-light-responsive SnO2-Zn2SnO4 Z-scheme photocatalyst with surface vacancy for the enhanced photoreactivity towards NO and acetone oxidation. Chem. Eng. J. 336, 200–210. doi: 10.1016/j.cej.2017.11.045
Liang, L., Li, K. N., Lv, K. L., Ho, W. K., and Duan, Y. Y. (2017). Highly photoreactive TiO2 hollow microspheres with super thermal stability for acetone oxidation. Chin. J. Catal. 38, 2085–2093. doi: 10.1016/S1872-2067(17)62952-8
Lin, S., Sun, S. Y., Shen, K. X., Tan, D. Y., Zhang, H. P., Dong, F. Q., et al. (2018a). Photocatalytic microreactors based on nano TiO2-containing clay colloidosomes. Appl. Clay Sci. doi: 10.1016/j.clay.2017.08.022. [Epub ahead of print].
Lin, S., Sun, S. Y., Wang, K., Shen, K. X., Ma, B. B., Ren, Y. Q., et al. (2018b). Bioinspired design of alcohol dehydrogenase@nano TiO2 microreactors for sustainable cycling of NAD+/NADH coenzyme. Nanomaterials 8, 1–9. doi: 10.3390/nano8020127
Liu, Y., Chen, L. F., Hu, J. C., Li, J. L., and Richards, R. (2010). TiO2 nanoflakes modified with gold nanoparticles as photocatalysts with high activity and durability under near UV irradiation. J. Phys. Chem. C 114, 1641–1645. doi: 10.1021/jp910500c
Lou, X. W., Archer, L. A., and Yang, Z. C. (2008). Hollow micro-/nanostructures: synthesis and applications. Adv. Mater. Weinheim. 20, 3987–4019. doi: 10.1002/adma.200800854
Lv, K. L., Cheng, B., Yu, J. G., and Liu, G. (2012). Fluorine ions-mediated morphology control of anatase TiO2 with enhanced photocatalytic activity. Phys. Chem. Chem. Phys. 14, 5349–5362. doi: 10.1039/c2cp23461k
Lv, K. L., Xiang, Q. J., and Yu, J. G. (2011). Effect of calcination temperature on morphology and photocatalytic activity of anatase TiO2 nanosheets with exposed {001} facets. Appl. Catal. B. 104, 275–281. doi: 10.1016/j.apcatb.2011.03.019
Minero, C., Mariella, G., Maurino, V., and Pelizzetti, E. (2000). Photocatalytic transformation of organic compounds in the presence of inorganic anions. 1. Hydroxyl-mediated and direct electron-transfer reactions of phenol on a titanium dioxide-fluoride system. Langmuir. 16, 2632–2641. doi: 10.1021/la9903301
Padmanabhan, S. C., Pillai, S. C., Colreavy, J., Balakrishnan, S., McCormack, D. E., Perova, T. S., et al. (2007). A simple sol-gel processing for the development of high-temperature stable photoactive anatase titania. Chem. Mater. 19, 4474–4481. doi: 10.1021/cm070980n
Periyat, P., McCormack, D. E., Hinder, S. J., and Pillai, S. C. (2009). One-pot synthesis of anionic (nitrogen) and cationic (sulfur) codoped high-temperature stable, visible light active, anatase photocatalysts. J. Phys. Chem. C 113, 3246–3253. doi: 10.1021/jp808444y
Periyat, P., Pillai, S. C., McCormack, D. E., Colreavy, J. C., and Hinder, S. J. (2008). Improved high-temperature stability and sun-light-driven photocatalytic activity of sulfur-doped anatase TiO2. J. Phys. Chem. C 112, 7644–7652. doi: 10.1021/jp0774847
Qi, K. Z., Cheng, B., Yu, J. G., and Ho, W. K. (2017). A review on TiO2-based Z-scheme photocatalysts. Chin. J. Catal. 38, 1936–1955. doi: 10.1016/S1872-2067(17)62962-0
Regmi, C., Joshi, B., Ray, S. K., Gyawali, G., and Pandey, R. P. (2018). Understanding mechanism of photocatalytic microbial decontamination of environmental wastewater. Front. Chem. 6:33. doi: 10.3389/fchem.2018.00033
Sajan, C. P., Wageh, S., Al-Ghamdi, A. A., Yu, J. G., and Cao, S. W. (2016). TiO2 nanosheets with exposed {001} facets for photocatalytic applications. Nano Res. 9, 3–27. doi: 10.1007/s12274-015-0919-3
Wang, Q., Qin, Z. N., Chen, J., Ren, B. S., Chen, Q. F., Guo, Y. C., et al. (2016). Green synthesis of nickel species in situ modified hollow microsphere TiO2 with enhanced photocatalytic activity. Appl. Surf. Sci. 364, 1–8. doi: 10.1016/j.apsusc.2015.12.033
Wang, X. F., Yu, R., Wang, K., Yang, G. Q., and Yu, H. G. (2015). Facile template-induced synthesis of Ag-modified TiO2 hollow octahedra with high photocatalytic activity. Chin. J. Catal. 36, 2211–2218. doi: 10.1016/S1872-2067(15)60978-0
Wang, Z. Y., Huang, B. B., Dai, Y., Zhang, X. Y., Qin, X. Y., Li, Z., et al. (2012). Topotactic transformation of single-crystalline TiOF2 nanocubes to ordered arranged 3D hierarchical TiO2 nanoboxes. CrystEngComm 14, 4578–4581. doi: 10.1039/C2CE25271F
Wang, Z. Y., Lv, K. L., Wang, G. H., Deng, K. J., and Tang, D. G. (2010). Study on the shape control and photocatalytic activity of high-energy anatase titania. Appl. Catal. B 100, 378–385. doi: 10.1016/j.apcatb.2010.08.014
Wen, C. Z., Hu, Q. H., Guo, Y. N., Gong, X. Q., Qiao, S. Z., and Yang, H. G. (2011). From titanium oxydifluoride (TiOF2) to titania (TiO2): phase transition and non-metal doping with enhanced photocatalytic hydrogen (H2) evolution properties. Chem. Commun. 47, 6138–6140. doi: 10.1039/c1cc10851d
Wen, J. Q., Li, X., Liu, W., Fang, Y. P., Xie, J., and Xu, Y. H. (2015). Photocatalysis fundamentals and surface modification of TiO2 nanomaterials. Chin. J. Catal. 36, 2049–2070. doi: 10.1016/S1872-2067(15)60999-8
Xia, Y., Li, Q., Lv, K. L., and Li, M. (2017). Heterojunction construction between TiO2 hollowsphere and ZnIn2S4 flower for photocatalysis application. Appl. Surf. Sci. 398, 81–88. doi: 10.1016/j.apsusc.2016.12.006
Xu, X., Sun, Y. F., Fan, Z. H., Zhao, D. Q., Xiong, S. M., Zhang, B. Y., et al. (2018). Mechanisms for· and·OH production on flowerlike BiVO4 photocatalysis based on electron spin resonance. Frontier in Chem. 6:64. doi: 10.3389/fchem.2018.00064
Xu, Y. M., Lv, K. L., Xiong, Z. G., Leng, W. H., Liu, W. P. D., and Xue, X. J. (2007). Rate enhancement and rate inhibition of phenol degradation over irradiated anatase and rutile TiO2 on the addition of NaF: new insight into the mechanism. J. Phys. Chem. C 111, 19024–19032. doi: 10.1021/jp076364w
Yang, H. G., Liu, G., Qiao, S. Z., Sun, C. H., Jin, Y. G., Smith, S. C., et al. (2009). Solvothermal synthesis and photoreactivity of anatase TiO2 nanosheets with dominant {001} facets. J. Am. Chem. Soc. 131 4078–4083. doi: 10.1021/ja808790p
Yang, H. G., Sun, C. H., Qiao, S. Z., Zou, J., Liu, G., Smith, S. C., et al. (2008). Anatase TiO2 single crystals with a large percentage of reactive facets. Nature 453, 638–641. doi: 10.1038/nature06964
Yu, J. C., Yu, J. G., Ho, W. K., Jiang, Z. T., and Zhang, L. Z. (2002). Effects of F− doping on the photocatalytic activity and microstructures of nanocrystalline TiO2 powders. Chem. Mater. 14, 3808–3816. doi: 10.1021/cm020027c
Yu, J. G., Low, J. X., Xiao, W., Zhou, P., and Jaroniec, M. (2014). Enhanced photocatalytic CO2-reduction activity of anatase TiO2 by coexposed {001} and {101} facets. J. Am. Chem. Soc. 136, 8839–8842. doi: 10.1021/ja5044787
Zhao, X., Du, Y. T., Zhang, C. J., Tian, L. J., Li, X. F., Deng, K. J., et al. (2018). Enhanced visible photocatalytic activity of TiO2 hollow boxes modified by methionine for RhB degradation and NO oxidation. Chin. J. Catal. 39, 736–746. doi: 10.1016/S1872-2067(18)63039-6
Keywords: TiO2, photocatalytic degradation, acetone, fluorine, oxygen vacancy
Citation: Shi T, Duan Y, Lv K, Hu Z, Li Q, Li M and Li X (2018) Photocatalytic Oxidation of Acetone Over High Thermally Stable TiO2 Nanosheets With Exposed (001) Facets. Front. Chem. 6:175. doi: 10.3389/fchem.2018.00175
Received: 07 April 2018; Accepted: 30 April 2018;
Published: 18 May 2018.
Edited by:
Fan Dong, Chongqing Technology and Business University, ChinaReviewed by:
Xin Li, South China Agricultural University, ChinaShiyong Sun, Southwest University of Science and Technology, China
Copyright © 2018 Shi, Duan, Lv, Hu, Li, Li and Li. This is an open-access article distributed under the terms of the Creative Commons Attribution License (CC BY). The use, distribution or reproduction in other forums is permitted, provided the original author(s) and the copyright owner are credited and that the original publication in this journal is cited, in accordance with accepted academic practice. No use, distribution or reproduction is permitted which does not comply with these terms.
*Correspondence: Kangle Lv, bHZrYW5nbGVAbWFpbC5zY3VlYy5lZHUuY24=
Mei Li, bGltZWlAbWFpbC5zY3VlYy5lZHUuY24=