- 1The Center of New Energy Materials and Technology, School of Materials Science and Engineering, Southwest Petroleum University, Chengdu, China
- 2State Key Laboratory of Oil and Gas Reservoir Geology and Exploitation, Southwest Petroleum University, Chengdu, China
Powdery photocatalysts seriously restrict their practical application due to the difficult recycle and low photocatalytic activity. In this work, a monolithic g-C3N4/melamine sponge (g-C3N4/MS) was successfully fabricated by a cost-effective ultrasonic-coating route, which is easy to achieve the uniform dispersion and firm loading of g-C3N4 on MS skeleton. The monolithic g-C3N4/MS entirely inherits the porous structure of MS and results in a larger specific surface area (SSA) than its powdery counterpart. Benefit from this monolithic structure, g-C3N4/MS gains more exposed active sites, enhanced visible-light absorption and separation of photogenerated carriers, thus achieving noticeable photocatalytic activity on nitric oxide (NO) removal and CO2 reduction. Specifically, NO removal ratio is as high as 78.6% which is 4.5 times higher than that of the powdery g-C3N4, and yield rate of CO and CH4 attains 7.48 and 3.93 μmol g−1 h−1. Importantly, the features of low-density, high porosity, good elasticity, and firmness, not only endow g-C3N4/MS with flexibility in various environmental applications, but also make it easy to recycle and stable for long-time application. Our work provides a feasible approach to fabricate novel monolithic photocatalysts with large-scale production and application.
Introduction
Semiconductor photocatalysis is one of the promising strategies for pollutants abatement (Maggos et al., 2007; Huang et al., 2013, 2016; Zhao et al., 2015) and has attracted intense investigation in the past decades. Up to now, hundreds of semiconductors have been explored and applied in the field of environmental remediation (Liu et al., 2008; Hossain and Mukherjee, 2013; Huang et al., 2015a, 2017a; Zhou et al., 2016). However, only a few of them have been considered as potential candidates for practical application in view of their nontoxic, suitable band gap, band edge energy, good stability and earth-abundant source. g-C3N4 is one of those semiconductors, which possesses graphene-like structure and constituted mainly by carbon and nitrogen. Since g-C3N4 was first reported to photocatalytic water splitting by Wang et.al (Wang et al., 2009), it quickly becomes a hot material in photocatalysis. Afterwards, g-C3N4 has already been applied in various reactions such as CO2 photoreduction, NO removal, and dye degradation (Yan et al., 2009; Dong et al., 2014a; Sun H. et al., 2017). However, extensive studies revealed that g-C3N4 suffers from fast photo-generated carriers recombination, limited visible-light absorption, and low surface area. Various strategies have been carried out to overcome these intrinsic drawbacks of g-C3N4, such as elemental doping, composite with other materials and morphology control synthesis, etc. (Liu et al., 2010; Zhao et al., 2012; Hou et al., 2014; Cheng et al., 2015; Han Q. et al., 2015; Li et al., 2017; Yang et al., 2017). Besides above disadvantages, as a potential photocatalyst for practical application, g-C3N4 is also hindered by difficult recycle originating from particle heavy loss during its complicated recovery process, inefficient utilization of active sites and light energy resulting from particle aggregation. Comparison with the intrinsic drawbacks of g-C3N4, these problems are vital to achieve successful application of photocatalyst on actual environmental issues, but they are rarely studied and generally beyond the aforementioned strategies to overcome.
Recently, monolithic or integrated photocatalysts are found to be a plausible way to solve the practical application problem of photocatalyst (Cheng et al., 2016; Wan et al., 2018). The so-called monolithic photocatalyst usually consists of two parts, one is a macroscopical support with porous three-dimensional (3D) skeleton, and the other is the loaded photocatalyst particles. After integrating powdery catalysts on its support, their recycling becomes easy to achieve by a tweezer (Liu W. J. et al., 2015; Tang et al., 2017). Meanwhile, the 3D porous structure of support gives the powdery catalysts a high dispersion and exposes more active sites by avoiding particles agglomeration. Moreover, this structure also benefits light energy harvest and transportation of liquid or gas pollutants. Until now, graphene aerogels are the most studied monolithic photocatalyst supports because of their inherent large surface area, high porosity and low density. We have fabricated monolithic C3N4/graphene oxide aerogel (GOA) in our previous work and found obvious activity enhancement (Wan et al., 2016), which is in line with the results from other monolithic photocatalyst/graphene aerogels (Fan et al., 2015; Cui et al., 2017; Wang et al., 2017). However, the intrinsic brittleness and weak firmness of aerogels make it easy to break into pieces during mechanical deformation, which seriously restrict its potential in practical application. Other firm supports, such as carbon foam and Al2O3 ceramic foam with hard 3D framework, are also selected to fabricate monolithic photocatalyst (Dong et al., 2014b; Lin et al., 2016). Nevertheless, it is very difficult to achieve uniform loading by directly mixing photocatalyst with these hard supports. To overcome this problem, special strategies with high cost and energy consumption, like in-situ immobilizing approach and laser ablating deposition (Liang et al., 2015; Lin et al., 2016), are used to achieve the good catalyst dispersion. The sophisticated preparation method severely limits the hard supports to be extensively utilized in fabrication of monolithic photocatalyst. Based on the above considerations, the proper support remains an obstacle for the practical application of monolithic photocatalyst. Lately, melamine sponge (MS), a cheap commercial polymer foam which is widely used as kitchen and construction materials, is successfully used for oil-water separation by integrating with graphene (Liu T. T. et al., 2015; Zhao et al., 2016). The fabricated graphene/MS exhibits low-density, high porosity and high elasticity inherited from MS, which exactly match the support characteristics of the monolithic photocatalyst. Importantly, the good elasticity makes MS more ductile and avoids the drawbacks of brittle and hard materials. Therefore, MS is a potential alternative and selected as the support for monolithic photocatalyst fabrication.
Herein, we prepare a monolithic g-C3N4/MS by a facile ultrasonic-coating method at room temperature, which is very easy to achieve mass production. The monolithic structure endows g-C3N4/MS with enhanced light harvest and more exposed active sites, ensuring its good photocatalytic activity. Importantly, the as-prepared monolithic photocatalyst exhibits low-density, high porosity, high elasticity and good firmness, which not only make it flexible in various environmental applications including NO removal, and CO2 photoreduction, but also make it easy to recycle and suitable for practical application. Overall, our results provide a novel strategy to develop monolithic photocatlyst for practical application with large-scale production.
Experimental
Synthesis of g-C3N4
The polymeric g-C3N4 was prepared by pyrolysis of urea (Liu et al., 2011). In a typical process, 15 g urea was added into an alumina crucible with a cover and then heated to 550°C in a muffle furnace for 1 h with a heating rate of about 55°C min−1. After cool down to room temperature, the final yellow agglomerates were the pristine g-C3N4 and subsequently ground into powder for further use.
Preparation of Monolithic g-C3N4/MS
For the preparation of monolithic g-C3N4/MS, 2.5 g g-C3N4 powder was dispersed in 500 mL water and sonicated for 1 h to form a g-C3N4 suspension. MS was cut into suitable size and washed with deionized water and alcohol in order, then dried at room temperature. Next, the clean MS was immersed into g-C3N4 suspension for 30 min, and then squeezed out the excess solution. After that, the sample was transferred into a culture dish and freeze-dried (−70°C pre-freezing) for 48 h to obtain g-C3N4/MS. For comparison, powdery g-C3N4 without MS was processed through the same procedures and denoted as sonicated g-C3N4. To obtain the best photocatalytic activity of monolithic g-C3N4/MS, we finely investigated the effect of g-C3N4 suspension concentration (3 mg mL−1-40 mg mL−1) and MS thickness (0.5–2.5 cm). The sizes of monolithic g-C3N4/MS varied with different experiments and were stated at their first appearance in the text.
Characterization
Powder X-ray diffraction (PXRD) was performed on a PANalytical X'pert diffractometer with a Cu Ka radiation. Transmission electron microscopy (TEM) was performed on a FEI tecnai G2 F30 microscope operated at 200 kV. The morphology of g-C3N4/MS was observed through scanning electron microscopy (SEM) on a ZEISS EVO MA15 microscopy. The Fourier transform infrared (FT-IR) spectra were measured using a Nicolet 6700 spectrometer on samples embedded in KBr pellets. UV-vis diffuse reflectance spectrum (DRS) data were recorded on a Shimadzu UV-2600 spectrophotometer. Photoluminescence spectra were recorded on F-7000 FL spectrofluorometer with an excitation wavelength at 320 nm. X-ray photoelectron spectroscopy (XPS) was performed by using a Thermo Scientific Escalab 250Xi spectrometer. The specific surface area (SSA) was determined via using methylene blue (MB) adsorption method on a UV-vis spectrophotometer (UV-5100, Anhui Wanyi; Tran et al., 2015), the SSA of g-C3N4 and g-C3N4/MS were calculated by the following equation:
Where NA represents Avogadro's constant (6.02 × 1023 mol−1), AMB represents the covered area of per MB molecule (typically assumed to be 1.35 nm2), Co and Ce are the initial and equilibrium concentrations of MB, V is the volume of MB solution, MMB is the relative molecular mass of MB, and ms is the mass of the sample.
Evaluation of Photocatalytic Activity
The photocatalytic activity of g-C3N4/MS was evaluated in both gaseous systems. Photocatalytic removal of NO at ppb level was previously reported in details (Zhang et al., 2014). Typically, A 150 W metal halide lamp with a visible light filter (>420 nm) was employed to operate the experiment. A piece of g-C3N4/MS was put into the reactor for photocatalytic activity test. The light intensity is 35.88 mW cm−2 measured by a light intensity meter. The initial concentration of NO was diluted to 500 ppb by drying air. The flow rates of dry air and NO are set at 2 L min−1 and 9.5 mL min−1, respectively. The formula of degradation rate of NO was counted by the following equation:
Where C (NOx) represents the concentration of total nitric oxide (NO2 and NO), while C0 is the initial concentration of NO2 when reaching the adsorption-desorption equilibrium.
The photocatalytic reduction of CO2 was performed in a 380 mL home-made reactor at ambient temperature and pressure. A 300 W Xe lamp was used as a light source and positioned 8 cm above the photocatalytic reactor. In a typical test, a plastic beaker with 20 mL deionized water was deposited at the bottom of the reactor, and a culture dish with 100 mg g-C3N4 powder or g-C3N4/MS (38.5 cm2) was placed on the plastic beaker. Before irradiation, the reactor was sealed and vacuumed by a pump, then removed air by blowing argon for 15 min. Subsequently, 1 mL CO2 was injected into the reactor. After 4 h irradiation, 1 mL of gas was taken out from the reactor and analyzed by using a gas chromatograph (Techcomp GC7900) equipped with a flame ionized detector (FID) and a thermal conductivity detector (TCD). CO and CH4 were analyzed by the FID, and H2 was analyzed by TCD.
Results and Discussion
Fabrication and Physical Properties of g-C3N4/MS
The general preparation approach of g-C3N4/MS was illustrated in Figure 1A. Direct mixture of powdery g-C3N4 and MS is hard to gain a monolithic g-C3N4/MS with good particle dispersion and well contact between particle and MS skeleton. Therefore, the g-C3N4 powder was firstly added into water and sonicated for 30 min to form uniform suspension, which make the particle diffusion easy and fast in the porous structure of MS. Then, the pretreated MS was immersed into this suspension through dipping and squeezing procedures until MS was fully covered by g-C3N4 particles. Finally, followed by a conventional freezing-drying process, the monolithic g-C3N4/MS was obtained. Obviously, no large particles were observed on the cross-section photo in Figure 1A, indicating that the g-C3N4 powder was uniformly coated on MS skeleton. For comparison, we also dipped MS in a saturated solution of urea, which is the g-C3N4 precursor. After removing the water by freeze-drying, we attempted to fabricate the monolithic g-C3N4/MS by in-situ immobilizing approach at 550°C. However, the obtained monolithic composite became very fragile due to the carbonization of MS skeleton. Moreover, the g-C3N4 powder gathered on the composite surface with an inhomogeneous dispersion (Figure S1). The failed trial illustrated the mild ultrasonic-coating approach is superior to other methods for monolithic g-C3N4/MS fabrication. Importantly, the facile ultrasonic-coating approach developed here is also adapted to other porous supports, such as nickel foam, Al2O3 ceramic foam, glass fiber and polyester fiber, and the corresponding monolithic products of g-C3N4 are shown in Figure S2.
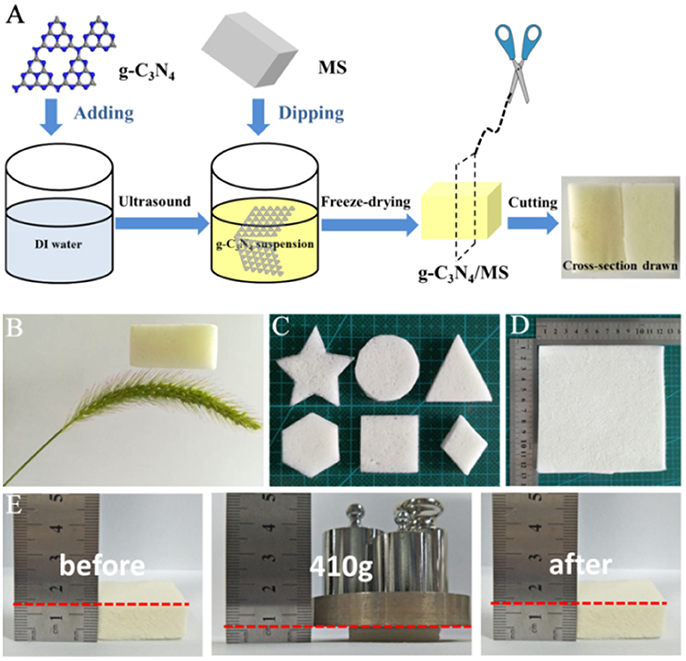
Figure 1. (A) Preparation process of g-C3N4/MS photocatalyst; (B) Ultra-light g-C3N4/MS resting on dog tail grass; (C) g-C3N4/MS with various shapes; (D) g-C3N4/MS with large area (12.0 × 12.0 × 0.5 cm3); (E) Mechanical property of g-C3N4/MS.
Physical properties of materials are pivotal to their practical application. As shown in Figure 1B, the monolithic g-C3N4/MS has an ultra-low density (11.5 mg cm−3), which can stay on dog tail grass and remarkably lighter than that of reported BiOBr/reduced GOA (50 mg cm−3) (Liu W. J. et al., 2015), TiO2/graphene aerogel (19 mg cm−3) (Qiu et al., 2014), and MoS2/reduced GOA (56.1 mg cm−3) (Zhang R. Y. et al., 2017). Moreover, SSA of g-C3N4/MS (7.6 m2 g−1) is much more larger than that of the pristine g-C3N4 (0.9 m2 g−1), which not only offer more active sites but also have larger absorption capacity than its powdery counterpart. In addition, facile modification of shapes and sizes endows g-C3N4/MS with a good flexibility to handle different situations in practical application (Figures 1C,D). More importantly, the g-C3N4/MS presents excellent elasticity. As revealed in Figure 1E, the g-C3N4/MS can instantaneously recover and maintain its integrity after removing of the heavy loading (410 g counterweight), suggesting that g-C3N4/MS possesses enough mechanical strength to deal with intricate operation in environmental abatement. To further ensure the firmness of g-C3N4 on MS skeletons, a test is carried out by blowing the g-C3N4/MS with a strong airflow for 12 h, the detailed simulation device diagram is shown in Figure S3a. The dropping g-C3N4 powder from g-C3N4/MS is collected and weighted up. The final weight loss is less than 7.60 mg, which account for 1.15% of g-C3N4 loaded on MS skeletons (Figure S3b). The above result demonstrates that g-C3N4 is firmly distributed on MS even under extreme work condition, which is pivotal to the recycle in practical application.
Photocatalytic Activity
Benefit from the characteristics of low-density, high porosity and good elasticity, the monolithic g-C3N4/MS can be used to removal gaseous pollutants. Therefore, two different photocatalysis applications including NO removal, dye degradation, and CO2 photoreduction, are selected to test the photocatalytic activity of the as-prepared monolithic g-C3N4/MS. NO, a typical air contaminants, mostly produced from the combustion of fossil fuels and the emission of vehicle exhaust, can cause a series of atmosphere pollution problems such as acid rain, photochemical smog and haze (Wang et al., 2016). Photo-oxidation technique is considered an alternative to remove NO at low concentration (Zhou et al., 2014). Therefore, the g-C3N4/MS samples are firstly investigated by NO removal at the indoor ppb level. Figure 2A shows the effect of g-C3N4 concentration on NO photo-removal ratio occurring on g-C3N4/MS. Notably, the MS coated with 5 mg mL−1 suspension achieves the highest removal ratio of 45% within 30 min. As the concentration of g-C3N4 suspension less than 5 mg mL−1, the photocatalytic activity of g-C3N4/MS gradually enhanced with the increased concentration of g-C3N4 suspension. With a concentration higher than 5 mg mL−1, the photocatalytic activity of the g-C3N4/MS slightly decreased, attributing to agglomeration of the excessive g-C3N4 which not only hinder the NO transport by blocking pore channels in MS, but also cause a reduced light transmittance. Moreover, the thicknesses of MS have also been investigated, which is closely associated with the light utilization. Benefit from the good transparence of MS, the activity of g-C3N4/MS enhanced along with increased MS thickness in Figure 2B, but the corresponding best unit mass rate constant (0.868 min−1 g−1) of NO removal is belong to g-C3N4/MS with 0.5 cm thickness (As shown in Figure S4). Based on above results, the optimal concentration and thickness were fixed at 5 mg mL−1 and 0.5 cm, respectively.
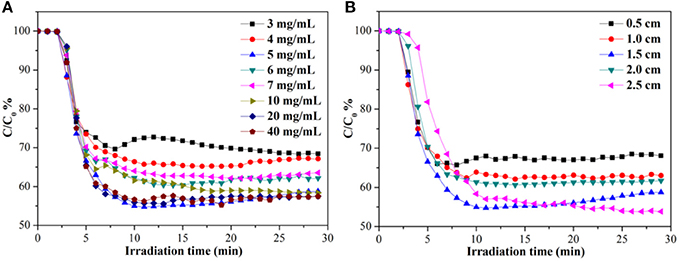
Figure 2. Photocatalytic NO removal ratios in presence of g-C3N4/MS fabricated with (A) different concentrations and (B) different thicknesses under visible light irradiation.
The fabrication and utilization of monolithic photocatalysts with large area on macro-scale is significant to their practical application. Therefore, a monolithic g-C3N4/MS with area of 12.0 × 12.0 cm2 is prepared for further test of NO removal under visible-light illumination (Figure 3A). For comparison, the NO removal on powdery g-C3N4 and pristine MS are also performed under the same conditions, respectively. Surprisingly, monolithic g-C3N4/MS presents the highest NO removal ratio of 78.6% in initial 5 min which is about 4.5 times higher than that of powdery g-C3N4 (17.6%), while no NO removal occurs on the pristine MS. After initial 5 min, NO removal ratio of g-C3N4/MS tends to be steady, which could be interpreted as partial active sites replaced by adsorptive or NO (Ai et al., 2009; Huang et al., 2010; Liu et al., 2017) and finally reached an adsorption and desorption equilibrium of these oxynitrides. Moreover, comparison with other photocatalysts in our previous work, large area g-C3N4/MS exhibits optimum activity (78.6%), which is approximate 4.25, 2.25 and 2.32 times higher than that of Bi2WO6/graphene (Zhou et al., 2014), C3N4/GOA (GOA) (Wan et al., 2016), and N-Bi2O2CO3/graphene quantum dots (Liu et al., 2017), respectively. Notably, the fraction of generated NO2 is lower than 5.4% over all our samples (as shown in Figure S5a), indicating those samples selectively oxidize NO to rather than NO2. Based on above results, the g-C3N4/MS with large area shows obviously enhanced photocatalytic activity than its powdery counterpart, indicating the monolithic photocatalyst is an effective strategy to solve the problems encountered by powdery photocatalysts in large-scale application.
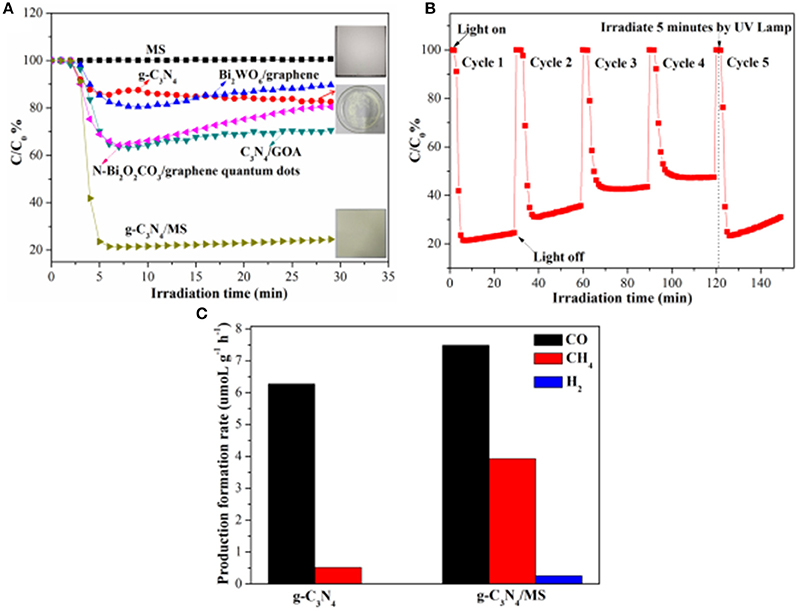
Figure 3. Photocatalytic activity of g-C3N4/MS under visible-light irradiation: (A) NO removal ratios of different samples; (B) Photocatalytic recycling test on large area g-C3N4/MS; (C) Production rate of CO, CH4 and H2 on g-C3N4, g-C3N4/MS respectively in photocatalytic CO2 reduction under UV-Vis light irradiation.
The stability and recyclability of photocatalysts are also important to their practical applications (Hu et al., 2017). Here, we carried out cycle and long-time tests to further evaluate the performance of the monolithic g-C3N4/MS. The result of cycle test is listed in Figure 3B. It can be seen that the NO removal ratio of g-C3N4/MS dropped quickly during the first two cycles. After that, the NO removal ratio approached to stabilization within third cycle and slightly decreased with incremental cycle-index, which attributed to temporary absorption equilibrium of oxynitrides (NO, ) in third cycle and their continuous accumulation on g-C3N4/MS. Fortunately, after UV lamp irradiation for 5 min, the adsorbed oxynitrides are desorbed and results in an immediate recovery of sample activity (Figure 3B). Furthermore, result of long-time test is displayed in Figure S5b. The g-C3N4/MS can achieve a stable catalytic performance after 1 h and keep a relatively high activity in the 2 h test interval. All above results confirm that the large area g-C3N4/MS has a superior recyclability and stability for NO removal, confirming its potential in practical application.
Apart from the photo-oxidation availability, the monolithic g-C3N4/MS also exhibits good ability of CO2 photoreduction. CO2 is the main greenhouse gas that generation from human activity and the combustion of fossil fuels which is responsible for global warming (Norby and Luo, 2004). As displayed in Figure 3C, the g-C3N4/MS shows higher photocatalytic activity (7.48 μmol g−1 h−1 CO, 3.93 μmol g−1 h−1 CH4 and 0.26 μmol g−1 h−1 H2) than that of powdery g-C3N4 (6.27 μmol g−1 h−1 CO, 0.52 μmol g−1 h−1 CH4 and 0 μmol g−1 h−1 H2). Notably, yield of CO is higher than that of CH4 on both two samples, because the conversion from CO2 to CO is 4-electron process, whereas that from CO2 to CH4 is 8-electrons process, obviously, the former is easier than the latter which can account for higher yield of CO. No H2 generated on g-C3N4 and traced H2 appeared on g-C3N4/MS indicate that all samples have high selectivity for CO2 reduction rather than H2 reduction.
In view of above results of NO removal, and CO2 photoreduction, the monolithic g-C3N4/MS does show practical potential in various applications and enhanced photocatalytic activity than its powdery counterpart. The large SAA must be responsible for this activity enhancement. However, to get a deep insight into the reasons of the improved performance, more investigations further carried out on the monolithic g-C3N4/MS.
Structure and Morphology
The PXRD patterns of MS, g-C3N4/MS, sonicated g-C3N4 and g-C3N4 are displayed in Figure 4. The peaks at ca. 13.1 and 27.3° can be assigned to (100) and (002) crystal planes of g-C3N4, respectively (Gholipour et al., 2016). Obviously, the PXRD patterns of g-C3N4 are consistent before and after ultrasonic process, confirming the crystal structure of g-C3N4 is very stable. Moreover, no difference is found in the PXRD patterns of g-C3N4/MS and MS, indicating the g-C3N4 particles are uniformly dispersed on the porous framework of MS rather than aggregated on its surface.
To reveal the microstructure and morphology of samples, TEM and SEM were conducted as shown in Figure 5. The TEM images of g-C3N4 and sonicated g-C3N4 show similar thin nano-flake structure with some mesopores (Figures 5A,B), which derived from gases releasing such as NH3 and CO2 during the pyrolysis of urea (Mao et al., 2013). Combination with PXRD results, it is sure no noticeable change appeared on the structure and morphology of g-C3N4 before and after the ultrasonic. In Figure 5C, the SEM image of MS reveals an interconnected 3D network structure with abundant open-cell pores, which not only offer sufficient channels for reactant transport, but also offer enough locations for photocatalyst particle dispersion. It is notable that the g-C3N4 was successfully coated on the smooth skeleton of MS according to the g-C3N4/MS image in Figure 5D. Based on the analysis of structure and morphology, the uniform dispersion of g-C3N4 achieves in monolithic g-C3N4/MS, confirming the feasibility of our coating strategy.
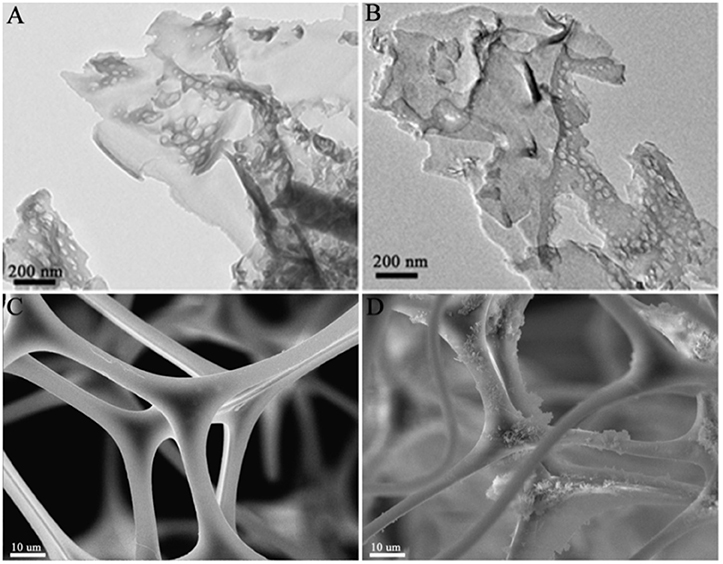
Figure 5. TEM images of samples: (A) g-C3N4; (B) sonicated g-C3N4. SEM images of samples: (C) MS; (D) g-C3N4/MS.
Figure 6 shows the FT-IR spectra of g-C3N4, g-C3N4/MS and MS. The spectrum of g-C3N4 displays typical peaks at 3000-3600 cm−1, 1200-1700 cm−1, and 811 cm−1, which are ascribed to the vibrational absorption of N-H and O-H, CN heterocycles and triazine unit (Kang et al., 2015; Wei et al., 2016; Sun Z. X. et al., 2017). Moreover, in the spectrum of MS, the prominent peaks located at 808, 1154, 1545, and 3422 cm−1, attributing to triazine ring bending, C-O stretching, C = N stretching and N-H stretching, while peaks centered at 988, 1329, and 1466 cm−1 corresponding to C-H bending vibrations (Pham and Dickerson, 2014; Zhang W. B. et al., 2017). Particularly, a new peak and an intensive peak appeared at 1334 cm−1 and 813 cm−1 in g-C3N4/MS spectrum, indicating a weak chemical interaction exists between g-C3N4 and MS skeletons. The above results reveal both van der waals and chemical interactions between g-C3N4 and MS, which explain the good firmness of monolithic g-C3N4/MS.
Band Structure and Photocatalytic Mechanism
The band gap of MS, g-C3N4 and g-C3N4/MS were determined by the results of UV-vis DRS in Figure 7A. Obviously, g-C3N4 absorption is located in visible region with a calculated band gap of 2.99 eV, while MS shows only UV light absorption with a wide band gap of 4.31 eV. Moreover, the DRS profile of the g-C3N4/MS exhibits a mechanical combination of the absorption features of the g-C3N4 and MS alone. Importantly, visible-light absorption of g-C3N4/MS gets a slight enhancement (2.79 eV), which may be attributed to the light multistage refraction and reflection on the MS framework (Dong et al., 2014b). In addition, the g-C3N4/MS not only enhances the light absorption, but also significantly suppresses the recombination of photo-generated carriers according to photoluminescence (PL) spectra in Figure 7B, which accounts for the enhancement of photocatalytic activity.
According to the above UV-vis DRS analysis (Figure 7A) and the XPS valence band spectrum (Figure S6), the band structure of g-C3N4/MS is proposed in Figure 8 with VB edge and CB edge located at 2.01 and −0.98 eV, respectively. As shown in Figure 8, the potential of VB holes (h+) is slight positive than OH−/OH (1.99 eV), while the potential of CB electron (e−) is much negative than that of O2/ (−0.28 eV). Therefore, the photogenerated holes can directly oxidize OH− to OH, and the photogenerated electrons can reduce easily O2 to . It is well known that OH and usually have strong oxidative ability and play the key role in photocatalytic oxidation reaction (Huang et al., 2015b,c, 2017b). Moreover, in consideration of the oxidation potentials of NO2/NO (1.03 eV), HNO2/NO (0.99 eV), HNO3/NO (0.94 eV) (Wan et al., 2016), all OH, and hole generated on g-C3N4/MS are able to remove NO. In addition, the reduction potentials of E (CO2/CH4), E (CO2/CO) and E (H2O/H2) were located at −0.24, −0.52, and −0.41 eV, respectively (Yu et al., 2014; Han B. et al., 2015). Comparison with CB potential (−0.98 eV), the photogenerated electron is capable of reducing CO2 on g-C3N4/MS. Based on above analyses and the results in photocatalytic activity part, the possible photocatalytic mechanism are simply illustrated in Figure 8. In short, there are three main pathways for NO removal, involving three different active oxidation species (OH, and h+), while there is one pathway for CO2 photoreduction, involving only one active species (e−).
Conclusions
In summary, a novel monolithic g-C3N4/MS was fabricated by a facile ultrasonic-coating method. This monolithic g-C3N4/MS possesses a uniform dispersion of g-C3N4 and large SSA, which not only facilitate exposing more active sites of g-C3N4 but also enhancing the visible-light absorption. Consequently, monolithic g-C3N4/MS shows obviously improved visible-light photocatalytic activity. The PL detection further reveals that enhanced separation of photogenerated carriers is also responsible for the activity enhancement. More importantly, the characteristics of low-density and high porosity allow the monolithic g-C3N4/MS to be applied in various environmental issues, while the high elasticity, good firmness, and mechanical strength give it noticeable recyclability and stability, confirming its feasibility for practical application. All in all, in this work, we fabricated a monolithic g-C3N4/MS with enhanced photocatalytic activity which can be easy to achieve large-scale production for practical photocatalysis application. Our results provide a low-cost and mild method for mass production of new monolithic photocatalysts.
Author Contributions
YY: Partly designed the experiments and wrote the manuscript; RZ, TR, and WW: Assisted in the analysis and interpretation of the data; YZ and QZ: Proposed the project, designed the experiment, and wrote the manuscript.
Conflict of Interest Statement
The authors declare that the research was conducted in the absence of any commercial or financial relationships that could be construed as a potential conflict of interest.
Acknowledgments
We gratefully acknowledge financial support from the Sichuan Provincial International Cooperation Project (2017HH0030), National Natural Science Foundation of China (21403172) and the Innovative Research Team of Sichuan Province (2016TD0011).
Supplementary Material
The Supplementary Material for this article can be found online at: https://www.frontiersin.org/articles/10.3389/fchem.2018.00156/full#supplementary-material
References
Ai, Z. H., Ho, W. K., Lee, S. C., and Zhang, L. Z. (2009). Efficient photocatalytic removal of NO in indoor air with hierarchical bismuth oxybromide nanoplate microspheres under visible light. Environ. Sci. Technol. 43, 4143–4150. doi: 10.1021/es9004366
Cheng, F. X., Wang, H. N., and Dong, X. P. (2015). The amphoteric properties of g-C3N4 nanosheets and fabrication of their relevant heterostructure photocatalysts by an electrostatic re-assembly route. Chem. Commun. 51, 7176–7179. doi: 10.1039/C5CC01035G
Cheng, W., Rechberger, F., and Niederberger, M. (2016). From 1D to 3D–macroscopic nanowire aerogel monoliths. Nanoscale 8, 14074–14077. doi: 10.1039/C6NR04429H
Cui, C., Li, S., Qiu, Y. W., Hu, H. H., Li, X. Y., and Li, C. R. (2017). Fast assembly of Ag3PO4 nanoparticles within three-dimensional graphene aerogels for efficient photocatalytic oxygen evolution from water splitting under visible light. Appl. Catal. B Environ. 200, 666–672. doi: 10.1016/j.apcatb.2016.07.056
Dong, F., Ou, M. Y., Jiang, Y. K., Guo, S., and Wu, Z. B. (2014a). Efficient and durable visible light photocatalytic performance of porous carbon nitride nanosheets for air purification. Ind. Eng. Chem. Res. 53, 2318–2330. doi: 10.1021/ie4038104
Dong, F., Wang, Z. Y., Li, Y. H., Ho, W. K., and Lee, S. C. (2014b). Immobilization of polymeric g-C3N4 on structured ceramic foam for efficient visible light photocatalytic air purification with real indoor illumination. Environ. Sci. Technol. 48, 10345–10353. doi: 10.1021/es502290f
Fan, Y., Ma, W., Han, D., Gan, S., Dong, X., and Niu, L. (2015). Convenient recycling of 3D AgX/graphene aerogels (X = Br, Cl) for efficient photocatalytic degradation of water pollutants. Adv. Mater. 27, 3767–3773. doi: 10.1002/adma.201500391
Gholipour, M. R., Béland, F., and Do, T. O. (2016). Post-calcined carbon nitride nanosheets as an efficient photocatalyst for hydrogen production under visible light irradiation. ACS Sustain. Chem. Eng. 5, 213–220. doi: 10.1021/acssuschemeng.6b01282
Han, B., Wei, W., Chang, L., Cheng, P. F., and Hu, Y. H. (2015). Efficient visible light photocatalytic CO2 reforming of CH4. ACS Catal. 6, 494–497. doi: 10.1021/acscatal.5b02653
Han, Q., Wang, B., Zhao, Y., Hu, C., and Qu, L. (2015). A Graphitic-C3N4“Seaweed” architecture for enhanced hydrogen evolution. Angew. Chem. Int. Ed. 54, 11433–11437. doi: 10.1002/anie.201504985
Hossain, S. T., and Mukherjee, S. K. (2013). Toxicity of cadmium sulfide (CdS) nanoparticles against Escherichia coli and HeLa cells. J. Hazard. Mater. 260, 1073–1082. doi: 10.1016/j.jhazmat.2013.07.005
Hou, Y., Zuo, F., Dagg, A. P., Liu, J. K., and Feng, P. Y. (2014). Branched WO3 nanosheet array with layered C3N4 heterojunctions and CoOx nanoparticles asa flexible photoanode for efficient photoelectrochemical water oxidation. Adv. Mater. 26, 5043–5049. doi: 10.1002/adma.201401032
Hu, J. D., Chen, D. Y., Li, N. J., Xu, Q. F., Li, H., He, J. H., et al. (2017). In situ fabrication of Bi2O2CO3/MoS2 on carbon nanofibers for efficient photocatalytic removal of NO under visible-light irradiation. Appl. Catal. B Environ. 217, 224–231. doi: 10.1016/j.apcatb.2017.05.088
Huang, H. W., Han, X., Li, X., Wang, S., Chu, P. K., and Zhang, Y. (2015a). Fabrication of multiple heterojunctions with tunable visible-light-active photocatalytic reactivity in BiOBr–BiOI full-range composites based on microstructure modulation and band structures. ACS Appl. Mater. Interfaces 7, 482–492. doi: 10.1021/am5065409
Huang, H. W., He, Y., Lin, Z. S., Kang, L., and Zhang, Y. H. (2013). Two novel Bi-based borate photocatalysts: crystal structure, electronic structure, photoelectrochemical properties, and photocatalytic activity under simulated solar light irradiation. J. Phys. Chem. C 117, 22986–22994. doi: 10.1021/jp4084184
Huang, H. W., He, Y., Li, X. W., Li, M., Zeng, C., Dong, F., et al. (2015b). Bi2O2(OH)(NO3) as a desirable [Bi2O2]2+ layered photocatalyst: strong intrinsic polarity, rational band structure and {001} active facets co-beneficial for robust photooxidation capability. J. Mater. Chem. A 3, 24547–24556. doi: 10.1039/C5TA07655B
Huang, H. W., Li, X. W., Wang, J. J., Dong, F., Chu, P. K., Zhang, T. R., et al. (2015c). Anionic group self-doping as a promising strategy: band-gap engineering and multi-functional applications of high-performance doped Bi2O2CO3. ACS Catal. 5, 4094–4103. doi: 10.1021/acscatal.5b00444
Huang, H. W., Xiao, K., He, Y., Zhang, T. R., Dong, F., Du, X., et al. (2016). In situ assembly of BiOI@Bi12O17Cl2 p-n junction: charge induced unique front-lateral surfaces coupling heterostructure with high exposure of BiOI {001} active facets for robust and nonselective photocatalysis. Appl. Catal. B Environ. 199, 75–86. doi: 10.1016/j.apcatb.2016.06.020
Huang, H. W., Tu, S. C., Zeng, C., Zhang, T. R., Reshak, A. H., and Zhang, Y. H. (2017a). Macroscopic polarization enhancement promoting photo− and piezoelectric− induced charge separation and molecular oxygen activation. Angew. Chem. 39, 12022–12026. doi: 10.1002/ange.201706549
Huang, H. W., Xiao, K., Zhang, T. R., Dong, F., and Zhang, Y. H. (2017b). Rational design on 3D hierarchical bismuth oxyiodides via in situ self-template phase transformation and phase-junction construction for optimizing photocatalysis against diverse contaminants. Appl. Catal. B Environ. 203, 879–888. doi: 10.1016/j.apcatb.2016.10.082
Huang, Y., Ai, Z. H., Ho, W. K., Chen, M. J., and Lee, S. C. (2010). Ultrasonic spray pyrolysis synthesis of porous Bi2WO6 microspheres and their visible-light-induced photocatalytic removal of NO. J. Phys. Chem. C 114, 6342–6349. doi: 10.1021/jp912201h
Kang, S. F., Fang, Y., Huang, Y. K., Cui, L. F., Wang, Y. Z., Qin, H. F., et al. (2015). Critical influence of g-C3N4 self-assembly coating on the photocatalytic activity and stability of Ag/AgCl microspheres under visible light. Appl. Catal. B Environ. 168, 472–482. doi: 10.1016/j.apcatb.2015.01.002
Li, P. H., Wang, F., Wei, S. Q., Li, X. Y., and Zhou, Y. (2017). Mechanistic insights into CO2 reduction on Cu/Mo-loaded two-dimensional g-C3N4 (001). Phys. Chem. Chem. Phys. 19, 4405–4410. doi: 10.1039/C6CP08409E
Liang, Q., Li, Z., Yu, X., Huang, Z. H., Kang, F., and Yang, Q. H. (2015). Macroscopic 3D porous graphitic carbon nitride monolith for enhanced photocatalytic hydrogen evolution. Adv. Mater. 27, 4634–4639. doi: 10.1002/adma.201502057
Lin, Z. Y., Li, J. L., Zheng, Z. Q., Li, L. H., Yu, L. L., Wang, C. X., et al. (2016). A floating sheet for efficient photocatalytic water splitting. Adv. Energy Mater. 6:1600510. doi: 10.1002/aenm.201600510
Liu, G., Niu, P., Sun, C. H., Smith, S. C., Chen, Z. G., Lu, G. Q., et al. (2010). Unique electronic structure induced high photoreactivity of sulfur-doped graphitic C3N4. J. Am. Chem. Soc. 132, 11642–11648. doi: 10.1021/ja103798k
Liu, G., Zhao, Y. N., Sun, C. H., Li, F., Lu, G. Q., and Cheng, H. M. (2008). Synergistic effects of B/N doping on the visible-light photocatalytic activity of mesoporous TiO2. Angew. Chem. Int. Ed. 47, 4516–4520. doi: 10.1002/anie.200705633
Liu, J. H., Zhang, T. K., Wang, Z. C., Dawson, G., and Chen, W. (2011). Simple pyrolysis of urea into graphitic carbon nitride with recyclable adsorption and photocatalytic activity. J. Mater. Chem. 21, 14398–14401. doi: 10.1039/c1jm12620b
Liu, T. T., Zhao, G. Z., Zhang, W. H., Chi, H. J., Hou, C. L., and Sun, Y. Y. (2015). The preparation of superhydrophobic graphene/melamine composite sponge applied in treatment of oil pollution. J. Porous Mater. 22, 1573–1580. doi: 10.1007/s10934-015-0040-8
Liu, W. J., Cai, J. Y., and Li, Z. H. (2015). Self-assembly of semiconductor nanoparticles/reduced graphene oxide (RGO) composite aerogels for enhanced photocatalytic performance and facile recycling in aqueous photocatalysis. ACS Sustain. Chem. Eng. 3, 277–282. doi: 10.1021/sc5006473
Liu, Y., Yu, S., Zhao, Z. Y., Dong, F., Dong, X. A., and Zhou, Y. (2017). N-Doped Bi2O2CO3/graphene quantum dot composite photocatalyst: enhanced visible-light photocatalytic no oxidation and in situ drifts studies. J. Phys. Chem. C 121, 12168–12177. doi: 10.1021/acs.jpcc.7b02285
Maggos, T., Bartzis, J. G., Leva, P., and Kotzias, D. (2007). Application of photocatalytic technology for NOx removal. Appl. Phys. A 89, 81–84. doi: 10.1007/s00339-007-4033-6
Mao, J., Peng, T. Y., Zhang, X. H., Li, K., Ye, L. Q., and Zan, L. (2013). Effect of graphitic carbon nitride microstructures on the activity and selectivity of photocatalytic CO2 reduction under visible light. Catal. Sci. Technol. 3, 1253–1260. doi: 10.1039/c3cy20822b
Norby, R. J., and Luo, Y. Q. (2004). Evaluating ecosystem responses to rising atmospheric CO2 and global warming in a multi-factor world. New Phytol. 162, 281–293. doi: 10.1111/j.1469-8137.2004.01047.x
Pham, V. H., and Dickerson, J. H. (2014). Superhydrophobic silanized melamine sponges as high efficiency oil absorbent materials. ACS Appl. Mater. Interfaces 6, 14181–14188. doi: 10.1021/am503503m
Qiu, B. C., Xing, M. Y., and Zhang, J. L. (2014). Mesoporous TiO2 nanocrystals grown in situ on graphene aerogels for high photocatalysis and lithium-ion batteries. J. Am. Chem. Soc. 136, 5852–5855. doi: 10.1021/ja500873u
Sun, H., Zhou, X. Z., Zhang, H. Z., and Tu, W. X. (2017). An efficient exfoliationmethod to obtain graphitic carbon nitride nanosheets with superior visible-light photocatalytic activity. Int. J. Hydrogen Energy 42, 7930–7937. doi: 10.1016/j.ijhydene.2016.12.080
Sun, Z. X., Fischer, J. M. T. A., Li, Q., Hu, J., Tang, Q. J., Wang, H. Q., et al. (2017). Enhanced CO2 photocatalytic reduction on alkali-decorated graphitic carbon nitride. Appl. Catal. B Environ. 216, 146–155. doi: 10.1016/j.apcatb.2017.05.064
Tang, L., Jia, C. T., Xue, Y. C., Li, L., Wang, A. Q., Xu, G., et al. (2017). Fabrication of compressible and recyclable macroscopic g-C3N4/GO aerogel hybrids for visible-light harvesting: a promising strategy for water remediation. Appl. Catal. B Environ. 219, 241–248. doi: 10.1016/j.apcatb.2017.07.053
Tran, D. N. H., Kabiri, S., Sim, T. R., and Losic, D. (2015). Selective adsorption of oil-water mixtures using polydimethylsiloxane (PDMS)-graphene sponges. Environ. Sci. Water Res. Technol. 1, 298–305. doi: 10.1039/C5EW00035A
Wan, W. C., Yu, S., Dong, F., Zhang, Q., and Zhou, Y. (2016). Efficient C3N4/graphene oxide macroscopic aerogel visible-light photocatalyst. J. Mater. Chem. A 4, 7823–7829. doi: 10.1039/C6TA01804A
Wan, W. C., Zhang, R. Y., Ma, M. Z., and Zhou, Y. (2018). Monolithic aerogel photocatalysts: a review. J. Mater. Chem. A 6, 754–775. doi: 10.1039/C7TA09227J
Wang, X., Maeda, K., Thomas, A., Takanabe, K., Xin, G., Carlsson, J. M., et al. (2009). A metal-free polymeric photocatalyst for hydrogen production from water under visible light. Nat. Mater. 8, 76–80. doi: 10.1038/nmat2317
Wang, X., Liang, Y. H., An, W. J., Hu, J. S., Zhu, Y. F., and Cui, W. Q. (2017). Removal of chromium (VI) by a self-regenerating and metal free g-C3N4/graphene hydrogel system via the synergy of adsorption and photo-catalysis under visible light. Appl. Catal. B Environ. 219, 53–62. doi: 10.1016/j.apcatb.2017.07.008
Wang, Y. L., Cui, X. B., Yang, Q. Y., Liu, J., Gao, Y., Sun, P., et al. (2016). Preparation of Ag-loaded mesoporous WO3 and its enhanced NO2 sensing performance. Sensors Actuat. B 225, 544–552. doi: 10.1016/j.snb.2015.11.065
Wei, H. T., Zhang, Q., Zhang, Y. C., Yang, Z. J., Zhu, A. P., and Dionysiou, D. D. (2016). Enhancement of the Cr (VI) adsorption and photocatalytic reduction activity of g-C3N4 by hydrothermal treatment in HNO3 aqueous solution[J]. Appl. Catal. A General 521, 9–18. doi: 10.1016/j.apcata.2015.11.005
Yan, S. C., Li, Z. S., and Zou, Z. G. (2009). Photodegradation performance of g-C3N4 fabricated by directly heating melamine. Langmuir 25, 10397–10401. doi: 10.1021/la900923z
Yang, L. Q., Huang, J. F., Shi, L., Gao, L. Y., Yu, Q., Jie, Y. N., et al. (2017). A surface modification resultant thermally oxidized porous g-C3N4 with enhanced photocatalytic hydrogen production. Appl. Catal. B Environ. 204, 335–345. doi: 10.1016/j.apcatb.2016.11.047
Yu, J. G., Jin, J., Cheng, B., and Jaroniec, M. (2014). A noble metal-free reduced graphene oxide-CdS nanorod composite for the enhanced visible-light photocatalytic reduction of CO2 to solar fuel. J. Mater. Chem. A 2, 3407–3416. doi: 10.1039/c3ta14493c
Zhang, Q., Zhou, Y., Wang, F., Dong, F., Li, W., Li, H. M., et al. (2014). From semiconductors to semimetals: bismuth as a photocatalyst for NO oxidation in air. J. Mater. Chem. A 2, 11065–11072. doi: 10.1039/C4TA01339E
Zhang, R. Y., Wan, W. C., Li, D. W., Dong, F., and Zhou, Y. (2017). Three-dimensional MoS2/reduced graphene oxide aerogel as a macroscopic visible-light photocatalyst. Chin. J. Catal. 38, 313–320. doi: 10.1016/S1872-2067(16)62568-8
Zhang, W. B., Zhai, X. L., Xiang, T. H., Zhou, M., Zang, D. L., Gao, Z. X., et al. (2017). Superhydrophobic melamine sponge with excellent surface selectivity and fire retardancy for oil absorption. J. Mater. Sci. 52, 73–85. doi: 10.1007/s10853-016-0235-7
Zhao, J., Guo, Q. J., Wang, X., Xie, H. L., and Chen, Y. Z. (2016). Recycle and reusable melamine sponge coated by graphene for highly efficient oil-absorption. Colloid Surface A 488, 93–99. doi: 10.1016/j.colsurfa.2015.09.048
Zhao, S. S., Chen, S., Yu, H. T., and Quan, X. (2012). g-C3N4/TiO2 hybrid photocatalyst with wide absorption wavelength range and effective photogenerated charge separation. Sep. Purif. Technol. 99, 50–54. doi: 10.1016/j.seppur.2012.08.024
Zhao, Z., Sun, Y., and Dong, F. (2015). Graphitic carbon nitride based nanocomposites: a review. Nanoscale 7, 15–37. doi: 10.1039/C4NR03008G
Zhou, Y., Zhang, X. J., Zhang, Q., Dong, F., Wang, F., and Xiong, Z. (2014). Role of graphene on the band structure and interfacial interaction of Bi2WO6/graphene composites with enhanced photocatalytic oxidation of NO. J. Mater. Chem. A 2, 16623–16631. doi: 10.1039/C4TA03762F
Keywords: g-C3N4/melamine sponge, photocatalysis, NO removal, monolith, visible light
Citation: Yang Y, Zhang Q, Zhang R, Ran T, Wan W and Zhou Y (2018) Compressible and Recyclable Monolithic g-C3N4/Melamine Sponge: A Facile Ultrasonic-Coating Approach and Enhanced Visible-Light Photocatalytic Activity. Front. Chem. 6:156. doi: 10.3389/fchem.2018.00156
Received: 02 March 2018; Accepted: 19 April 2018;
Published: 18 May 2018.
Edited by:
Fan Dong, Chongqing Technology and Business University, ChinaReviewed by:
Liwu Zhang, Fudan University, ChinaHongwei Huang, China University of Geosciences, China
Copyright © 2018 Yang, Zhang, Zhang, Ran, Wan and Zhou. This is an open-access article distributed under the terms of the Creative Commons Attribution License (CC BY). The use, distribution or reproduction in other forums is permitted, provided the original author(s) and the copyright owner are credited and that the original publication in this journal is cited, in accordance with accepted academic practice. No use, distribution or reproduction is permitted which does not comply with these terms.
*Correspondence: Qian Zhang, zhangqian@swpu.edu.cn
Ying Zhou, yzhou@swpu.edu.cn