- Department of Pharmacy, Health and Nutritional Sciences, University of Calabria, Rende, Italy
The human SLC37 gene family includes four proteins SLC37A1-4, localized in the endoplasmic reticulum (ER) membrane. They have been grouped into the SLC37 family due to their sequence homology to the bacterial organophosphate/phosphate (Pi) antiporter. SLC37A1-3 are the less characterized isoforms. SLC37A1 and SLC37A2 are Pi-linked glucose-6-phosphate (G6P) antiporters, catalyzing both homologous (Pi/Pi) and heterologous (G6P/Pi) exchanges, whereas SLC37A3 transport properties remain to be clarified. Furthermore, SLC37A1 is highly homologous to the bacterial glycerol 3-phosphate permeases, so it is supposed to transport also glycerol-3-phosphate. The physiological role of SLC37A1-3 is yet to be further investigated. SLC37A1 seems to be required for lipid biosynthesis in cancer cell lines, SLC37A2 has been proposed as a vitamin D and a phospho-progesterone receptor target gene, while mutations in the SLC37A3 gene appear to be associated with congenital hyperinsulinism of infancy. SLC37A4, also known as glucose-6-phosphate translocase (G6PT), transports G6P from the cytoplasm into the ER lumen, working in complex with either glucose-6-phosphatase-α (G6Pase-α) or G6Pase-β to hydrolyze intraluminal G6P to Pi and glucose. G6PT and G6Pase-β are ubiquitously expressed, whereas G6Pase-α is specifically expressed in the liver, kidney and intestine. G6PT/G6Pase-α complex activity regulates fasting blood glucose levels, whereas G6PT/G6Pase-β is required for neutrophil functions. G6PT deficiency is responsible for glycogen storage disease type Ib (GSD-Ib), an autosomal recessive disorder associated with both defective metabolic and myeloid phenotypes. Several kinds of mutations have been identified in the SLC37A4 gene, affecting G6PT function. An increased autoimmunity risk for GSD-Ib patients has also been reported, moreover, SLC37A4 seems to be involved in autophagy.
Introduction
The SLC37 family belongs to the largest human solute-carrier (SLC) superfamily, comprising more than 52 gene families, and over 400 membrane-bound proteins catalyzing the transport of metabolites across biological membranes (He et al., 2009; Perland and Fredriksson, 2017).
So far, four isoforms have been identified, named SLC37A1-4 (Bartoloni and Antonarakis, 2004; Chou and Mansfield, 2014). They are transmembrane proteins located in the endoplasmic reticulum (ER) membrane (Pan et al., 2011), and have been grouped into the SLC37 family due to their sequence homology to the bacterial organophosphate/phosphate (Pi) antiporter (Pao et al., 1998). Moreover, in the membrane transporter classification system included in the transport classification database, SLC37 carriers are reported to belong to the OPA family, classified as 2.A.1.4 (http://www.tcdb.org/). SLC37A1-4 translocases are also called sugar-phosphate exchangers SPX1-4 (Bartoloni et al., 2000; Takahashi et al., 2000; Bartoloni and Antonarakis, 2004), and are predicted to consist of 10–12 transmembrane domains (Chou and Mansfield, 2014).
SLC37A1, SLC37A2, and SLC37A4 are Pi-linked glucose-6-phosphate (G6P) antiporters, catalyzing both homologous (Pi/Pi) and heterologous (G6P/Pi) exchanges, and are inhibited to a very different extend by cholorogenic acid, while SLC37A3 transport activity is yet to be determinated (Chen et al., 2008; Pan et al., 2011).
SLC37A1, SLC37A2, and SLC37A3 are the less characterized SLC37 family members (Chou and Mansfield, 2014). SLC37A1 gene appears to be involved in breast (Iacopetta et al., 2010) and colorectal (Kikuchi et al., 2018) cancers. SLC37A2 has been recently proposed as a vitamin D (Wilfinger et al., 2014; Saksa et al., 2015) and a phospho-progesterone receptor (Knutson et al., 2017) target gene. Moreover, in obese murine models its expression seems to be related to chronic inflammation that supports metabolic syndrome (Kim et al., 2007). In dairy cattle, a SLC37A2 mutation appears to be responsible for increased female infertility due to embryonic death (Fritz et al., 2013; Reinartz and Dist, 2016). The SLC37A3 gene has been possibly related to congenital hyperinsulinemia (Proverbio et al., 2013). Furthermore, this gene seems to be involved in epigenetic modifications, because its methylation level depends on fasting glucose blood levels, at least after a significant weight loss (Benton et al., 2015). SLC37A4, also known as glucose-6-phosphate translocase (G6PT), is the more extensively studied isoform, and is a member of the multicomponent glucose-6-phosphatase system (G6Pase-system). In the liver and kidney, the activity of this complex is required to maintain blood glucose homeostasis (Bartoloni and Antonarakis, 2004). Additionally, it supports neutrophil and macrophage functions (Chou and Mansfield, 2014).
In the past, G6Pase-system was believed to consist of a glucose-6-phosphatase, with its active site facing the ER lumen, and three translocases (known as T1-3). In detail, T1 mediated G6P import through the ER membrane, whereas T2 and T3 catalyzed Pi and glucose efflux from the ER cavity, respectively (Gerin et al., 2001). Moreover, the presence of a regulatory 21 kDa hepatic microsomal glucose-6-phosphatase stabilizing protein (SP) was also hypothesized (Burchell et al., 1985). The existence of T2, T3, and SP has never been proven.
Based on recent scientific literature, T1 corresponds to G6PT, and it works in complex with either glucose-6-phosphatase-α (G6Pase-α, also called G6PC1) or glucose-6-phosphatase-β (G6Pase-β, known as G6PC3; Chou et al., 2002).
G6Pase-α is specifically expressed in the liver, kidney, and intestine, and it hydrolyzes intraluminal G6P to Pi and glucose, then this sugar exits the cell and enters the bloodstream to maintain interprandial blood glucose homeostasis (Chou and Mansfield, 2014).
G6PT deficiency is responsible for glycogen storage disease type Ib (GSD-Ib, OMIM232220), whereas G6Pase-α impairment causes GSD type Ia (GSD-Ia, OMIM232200) (Chou et al., 2010a,b). Both disorders prevent the final steps of gluconeogenesis and glycogenolysis; as a result, endogenous glucose production is severely compromised creating metabolic impairment, consisting of fasting hypoglycemia, hyperlipidemia, hyperuricemia, lactic acidemia, growth retardation, and amassing of glycogen and fat in the liver and kidneys, causing hepatomegaly and nephromegaly, respectively (Chou et al., 2002, 2010b).
In neutrophils, G6PT is functionally coupled to the ubiquitous G6Pase-β, in order to support neutrophil and macrophage functions (Chou et al., 2010a,b; Jun et al., 2010). G6Pase-β deficiency results in severe congenital neutropenia (Boztug et al., 2009). This condition has been considered as a glycogen storage disease I related syndrome (GSD-Irs, OMIM 612541).
Unlike GSD-Ia, both GSD-Irs (Cheung et al., 2007; Jun et al., 2010; McDermott et al., 2010) and GSD-Ib (Kim et al., 2008; Jun et al., 2014) can cause neutropenia and myeloid dysfunction.
In this review, we focus on the physiopathological role of the SLC37A family members, in particular on the best characterized G6PT, highlighting its role in autophagy, an increased autoimmunity risk for GSD-Ib patients, as well as new promising therapeutic strategies for GSD-Ib.
SLC37A1 Family Member
The human SLC37A1 protein, also knows as SPX1, is encoded by the SLC37A1 gene (NM_018964), mapped to chromosome 21q22.3, and containing 19 coding exons and 7 untranslated exons. Alternative splicing origins different transcripts, although the predicted protein sequence is identical, consisting of 533 amino acids, with a calculated molecular weight of 58 kDa (Bartoloni et al., 2000). This latter contains a mitochondrial cleavage site, as well as both N- and C-terminal ER signals for the ER retention (Bartoloni et al., 2000). This protein displays 59, 35, and 22% sequence identity with the human SLC37A2, SLC37A3 and SLC37A4 proteins, respectively (Chou et al., 2013), and it is 86% identical to its mouse homolog (Bartoloni and Antonarakis, 2004). SLC37A1 and SLC37A2 isoforms are the most related, while all the remaining pairwise sequence comparisons between the other SLC37 family members show lower sequence identity; hence, it is feasible that they might have had an independent evolution.
The human SLC37A1 protein shares 30 and 71% sequence identity to bacterial GlpT and Mus musculus SLC37A2, respectively (Takahashi et al., 2000); suggesting that mammalian SLC37A1 could be able to transport glycerol-3-phosphate (G3P), probably catalyzing an heterologous G3P/Pi exchange; therefore its gene was also called G3PP (Bartoloni et al., 2000).
A G3P transport activity has never been demonstrated, althought SLC37A1 association with glycolipid metabolism has been suggested (Bartoloni and Antonarakis, 2004; Dolce et al., 2011). The hypothetical role of SLC37A1 as a G3P exchanger has been postulated in cancer cells (Iacopetta et al., 2010). In details, in estrogen receptor (ESR) negative SkBr3 breast cancer cells (Lappano et al., 2017), as well as in ESR positive endometrial Ishikawa tumor cells, the expression of the SLC37A1 transcript was proven to be upregulated by the epidermal growth factor (EGF), through the EGF receptor/mitogen-activated protein kinase/Fos transduction pathway. Notably, in the same work the SLC37A1 protein localization in the ER was also demonstrated, supporting the hypothesis that this protein could import G3P into the ER lumen to sustain phospholipid biosynthesis, required to promote cancer progression (Iacopetta et al., 2010).
The functional role of SLC37A1 was also investigated in other human diseases. Analyses led in patients affected by glycerol kinase deficiency-like syndrome, with glyceroluria but lacking mutations in the human glycerol kinase gene, found only non-pathogenetic sequence variants in the human SLC37A1 gene, excluding its implication in this defect (Bartoloni et al., 2000).
Furthermore, this gene critically maps to autosomal recessive nonsyndromic deafness locus (DFNB10), on chromosome 21q22.3, but its involvement in the pathogenesis of this disease was also excluded by mutational analysis (Bartoloni et al., 2000).
More recently, SLC37A1 upregulation (at the mRNA and protein levels) was found in patients with colorectal cancer (CRC), and it was associated with positive venous invasion, liver metastasis, and poor patient outcomes (Kikuchi et al., 2018). Moreover, in a colon cancer cell line, LS180, SLC37A1 upregulation was positively correlated to Sialyl Lewis A and Sialyl Lewis X levels. These carbohydrate antigens are ligands for the adhesion molecule E selectin, and are responsible for the adhesion of cancer cells to the endothelium during metastasis, which is a typical process of cancer progression (Bartella et al., 2016; Iacopetta et al., 2017). Their positive modulation suggests that SLC37A1 might play a key role in the hematogenous metastasis of CRC, even if the underlying mechanisms remain unclear (Kikuchi et al., 2018).
Currently, it is proven that SLC37A1 can catalyze both heterologous G6P/Pi and homologous Pi/Pi exchanges, but it is poorly sensitive to chlorogenic acid and is not functionally coupled to G6Pases. On this basis, it is unlikely that it is involved in blood glucose homeostasis (Pan et al., 2011), so its physiological role remains to be clarified.
SLC37A1 mRNA is ubiquitously expressed, although mainly in adult kidney, spleen, liver, small intestine, bone marrow, as well as in fetal liver, brain, and spleen (Bartoloni et al., 2000). In the main gluconeogenetic organs, liver, and kidney, the relative SLC37A1 transcript levels are rather low with respect to the SLC37A4 levels, since they represent <2%, whereas in the intestine and pancreas they constitute 60 and 69%, respectively, of those observed for SLC37A4 (Pan et al., 2011). In macrophages, the SLC37A1 transcript level is 43% of that found for SLC37A4, while in neutrophils, it is markedly (about 280 %) higher, suggesting that SLC37A1 might have a key role in such cells (Chou and Mansfield, 2014).
SLC37A2 Family Member
SLC37A2, also knows as SPX2, was firstly identified in a work, conducted on mice and aimed to detect cAMP inducible genes playing a role in promoting cholesterol efflux from the macrophage cell line RAW264 via apoE and apoA1 (Takahashi et al., 2000). Two murine transcripts were identified, originated by the use of alternative polyadenylation sites. Both transcripts are highly expressed in bone marrow derived macrophages, and encode a 510 amino-acid protein with a predicted molecular weight of 55 kDa (Takahashi et al., 2000).
A further study showed that SLC37A2 is abundantly expressed in murine macrophages, spleen and thymus, as well as in white adipose tissue (WAT) of genetically obese mouse models, since WAT is subject to considerable macrophage infiltrations, and this promotes obesity-associated chronic inflammation underlying metabolic syndrome and other comorbidities of obesity (Kim et al., 2007). The murine SLC37A2 protein undergoes post-translational modifications by N-linked glycosylation, and it migrates as a heterogeneous species of 50–75 kDa (Kim et al., 2007).
The human SLC37A2 protein is encoded by the SLC37A2 gene (NM_198277), mapped to chromosome 11q24.2 and consisting of 18 coding exons. Alternative splicing originates four different transcripts. Only the longest isoform has been characterized (Pan et al., 2011). The corresponding human SLC37A2 transcript is expressed in murine liver, kidney, intestine, and pancreas, however the related expression levels are <4.5% of those found for SLC37A4 (Pan et al., 2011). Noticeably, the SLC37A2 transcript levels increase 46-fold during differentiation of human monocytic leukemia cells (THP-1) to macrophages (Kim et al., 2007).
The human SLC37A2 protein consists of 505 amino acids and displays 59, 36, and 23% sequence identity with the human SLC37A1, SLC37A3, and SLC37A4 proteins, respectively (Chou et al., 2013). Moreover, it is 90% identical to its mouse homolog (Bartoloni and Antonarakis, 2004). Like the murine protein, also the human protein is post-translationally modified by N-linked glycosylation.
The SLC37A2 protein is able to catalyze both G6P/Pi and Pi/Pi exchanges (Pan et al., 2011). Similarly to SLC37A1, the SLC37A2 transport activity is poorly sensitive to chlorogenic acid and the protein is not functionally coupled to G6Pases, as well as it seems not to be involved in blood glucose homeostasis (Pan et al., 2011). Hence, the functional role of SLC37A2 is yet to be understood. Recently, SLC37A2 has been found as a vitamin D target gene (Wilfinger et al., 2014; Saksa et al., 2015). Vitamin D3 may affect gene regulation via the binding of its metabolite, 1α,25-dihydroxyvitamin D3 (1,25(OH)2D3), to the transcription factor vitamin D receptor (VDR). In monocytic and macrophage-like cells, the human SLC37A2 gene contains a conserved VDR-binding site allowing such modulation, although only in monocytic cells SLC37A2 is an early responding target gene, potentially useful as a biomarker of vitamin D3 status in the hematopoietic system (Wilfinger et al., 2014). In addition, in human peripheral blood mononuclear cells, changes in the expression of the SLC37A2 gene, together with those of other primary vitamin D target genes, are systematically associated with the alteration in the circulating form of vitamin D3. Remarkably, during vitamin D3 supplementation in pre-diabetic subjects those features allow a distinction into high and low responder patients (Saksa et al., 2015).
Remarkably, in dairy cattle a deleterious homozygous mutation (g.28879810C>T) was detected in an aborted fetus. This mutation was predicted to introduce a premature stop codon, strongly impairing protein structure, and it was believed to be responsible for embryonic lethality (Reinartz and Dist, 2016). The same mutation, leading to embryonic lethal defects with increased female infertility was also detected in another study (Fritz et al., 2013).
Considering that the SLC37A2 gene carries a VDR binding site, and that vitamin D3 may be involved in many biological pathways, such as calcium and phosphate homeostasis, cell growth, intracellular metabolism, as well as innate and adaptive immunity, embryonic death could depend on a deficit in such processes (Reinartz and Dist, 2016).
Recently, human SLC37A2 has also been proposed as a phospho-Ser294 progesterone receptor (phospho-Ser294 PR) target gene (Knutson et al., 2017). PR Ser294 phosphorylation is a common event in breast cancer progression, and its activity is significantly associated with invasive lobular carcinoma. The runt-related transcription factor 2 (RUNX2) is an osteoblast differentiation transcription factor expressed in developing breast epithelial cells; it appears to be required in the regulation of phospho-Ser294 PR target genes. In this regard, human SLC37A2 represents a good candidate as target gene, because it is expressed in monocytes, as well as in breast and cervical tissues, and it was found to contain multiple RUNX2 binding motifs immediately upstream and within the gene; moreover, its expression is proven to be upregulated by progestin in multiple cell line models (Knutson et al., 2017).
SLC37A3 Family Member
The human SLC37A3 protein, also knows as SPX3, is the less characterized SLC37 family member. It is encoded by the SLC37A3 gene (NM_207113), mapped to chromosome 7q34 and containing 17 coding exons. Alternative splicing originates three different transcripts (Bartoloni and Antonarakis, 2004).
One isoform, consisting of 494 amino acids, displays 35, 36, and 22% sequence identity with the human SLC37A1, SLC37A2, and SLC37A4 proteins, respectively (Chou et al., 2013), and it is 90% identical to its mouse and rat homologs (Bartoloni and Antonarakis, 2004). Even though SLC37A3 is an ER-associated protein, it fails to show an uptake activity (Pan et al., 2011), hence its functional properties remain to be clarified. Remarkably, the SLC37A3 transcript is extremely expressed in murine neutrophils, pancreas, and, to a lesser extent, in the liver, kidney, intestine, and macrophages (Pan et al., 2011; Chou et al., 2013), suggesting a possible functional role in the immune system and pancreas (Chou and Mansfield, 2014). In this latter regard, the human SLC37A3 gene could contribute to the pathogenesis of congenital hyperinsulinism of infancy (CHI). In detail, a mutation in this gene was found in one patient with CHI in which the molecular basis of the disease remained unknown, highligting that it could be responsible for the dysregulation of insulin secretion (Proverbio et al., 2013), even if the biological role of SLC37A3 in pancreatic insulin secretion has never been clarified.
More recently, epigenetic mechanisms were demonstrated to modify the human SLC37A3 gene, since a robust correlation between change in fasting glucose and DNA methylation level within the human SLC37A3 gene was found in subcutaneous adipose, after gastric bypass followed by a significant weight loss (Benton et al., 2015). This could suggest a possible involvement of SLC37A3 in obesity-related metabolic dysfunction.
SLC37A4 Family Member
SLC37A4 is the best functionally characterized SLC37 family member (Chen et al., 2000, 2002, 2008). The human protein is encoded by a single copy gene, SLC37A4 (NM_001467, OMIM 602671), mapped to chromosome 11q23 (Annabi et al., 1998), containing nine coding exons (Marcolongo et al., 1998; Gerin et al., 1999; Hiraiwa et al., 1999), and firstly isolated from a human bladder tumor cDNA library (Gerin et al., 1997).
This protein displays 20, 25, and 26% sequence identity with bacterial protein UhpT, GlpT, and UhpC, respectively (Gerin et al., 1997). UhpT and GlpT are OPAs (Maloney and Wilson, 1996), while UhpC is a putative G6P receptor controlling UhpT expression (Island et al., 1992).
SLC37A4 protein is scarcely related to the other SLC37 family members, as it shares 22% amino acid sequence homology with both SLC37A1 and SLC37A3, and it is 23% homologous to SLC37A2 (Chou et al., 2013). The human SLC37A4 protein is highly conserved in other species. Murine and rat homologous proteins share 98% sequence homology, as well as 95 and 93% sequence homology to the human protein, respectively (Lin et al., 1998). Two human tissue-specific splicing isoforms have been identified, because alternative splicing of exon 7 leads to the expression of two transcripts, G6PT and variant G6PT (vG6PT), differing by the inclusion of a 66-bp exon 7 sequence in vG6PT, and encoding proteins of 429 and 451 amino acids, respectively (Gerin et al., 1997; Hiraiwa et al., 1999; Lin et al., 2000). Human vG6PT contains 22 additional amino acids, and it is active in microsomal G6P transport; it has been detected in the brain, heart and skeletal muscle (Lin et al., 2000). G6PT mRNA is ubiquitously expressed, although at the highest levels in the liver, kidney, intestine (Lin et al., 1998; Pan et al., 2011), and in haematopoietic progenitor cells (Ihara et al., 2000). The physiological implications of those different expression patterns remain unclear. In this regard, inclusion of exon 7 sequence might increase vG6PT sensitivity for degradation, since in mouse models the turnover rate of vG6PT seems to be increased during myogenesis of muscle cells (Shieh et al., 2007). Both G6PT and vG6PT appear to be similarly active in G6P transport (Lin et al., 2000), although the majority of studies used G6PT.
Human G6PT is a hydrophobic protein whose transmembrane topology has been long debated. Hydropathy profile analysis predicted either 10 (Hoffman and Stoffel, 1993) or 12 transmembrane domains (Gerin et al., 1997). Protease protection and glycosylation scanning assays suggested a 10-transmembrane domains model, with both N- and C-termini protruding on the cytoplasmic side of the ER membrane (Pan et al., 1999). Conversely, homology modeling proposed a model containing 12 transmembrane α-helices (Almqvist et al., 2004). More recently, glycosylation scanning and protease sensitivity studies have indicated that the 10-domains model is more probable (Pan et al., 2009).
G6PT biological function is to translocate G6P from the cytoplasm into the ER lumen, where it is hydrolyzed to glucose and Pi either by G6Pase-α (Lei et al., 1996; Chou et al., 2010a,b) or by G6Pase-β (Shieh et al., 2003; Chou et al., 2010a,b).
In the past, only one G6Pase isoform was known, expressed exclusively in the liver, kidney and intestine (Lin et al., 1998). In 2003, a second isoform, ubiquitously expressed, was discovered and called G6Pase-β (Shieh et al., 2003). Consequently, the original isoform was renamed G6Pase-α. Both proteins are transmembrane phosphohydrolases essential for the last step of gluconeogenesis. They have similar topology (Pan et al., 1998; Shieh et al., 2003) and mechanism of action as regards G6P hydrolysis (Ghosh et al., 2002, 2004); their active sites are located inside the ER lumen, hence both enzymes require to be coupled with a functional G6PT to hydrolyze intraluminal G6P. On the other hand, G6Pase activity is required in turn for an efficient G6P transport (Lei et al., 1996).
Since G6PT is ubiquitous, tissue expression profiles of G6Pase-α or G6Pase-β, and the resulting G6PT/G6Pase complexes, reflect the different GSD-Ia, -Ib or -Irs phenotypes.
On this basis, when the G6PT/G6Pase-α complex is present in the main gluconeogenic organs (liver, kidney, and intestine), G6Pase-α mutations cause a defective glucose production with impaired blood glucose homeostasis between meals, that is the first biochemical hallmark of GSD-Ia (Chou et al., 2010a,b).
In neutrophils and macrophages, the G6PT/G6Pase-β complex preserves energy homeostasis and functionality, hence G6Pase-β mutations are responsible for GSD-Irs, an autosomal recessive disorder characterized by neutropenia and neutrophil dysfunction (Chou et al., 2010a,b), often associated with congenital cardiac and uro-genital anomalies (Boztug et al., 2009).
On the other hand, G6PT mutations underlie GSD-Ib, which implies either impaired metabolism as in GSD-Ia, or neutropenia and neutrophil dysfunction as in GSD-Irs (Chou et al., 2010a,b), even if in neutrophils and macrophages G6PT expression levels are rather low (Chou et al., 2013).
Physiopathological Role of SLC37A4
SLC37A4, known as G6PT or SPX4, is able to catalize both homologous Pi/Pi and heterologous G6P/Pi exchanges between the ER lumen and the cytoplasm (Chen et al., 2008). Early studies led on intact liver microsomes showed that the high specificity of G6PT for G6P is responsible for substrate specificity of the G6PT/G6Pase-α complex, since G6Pase-α is less specific for G6P (Arion et al., 1972, 1975).
G6PT transport activity is specifically and strongly inhibited by chlorogenic acid (Arion et al., 1997, 1998; Hemmerle et al., 1997; Hiraiwa et al., 1999; Chen et al., 2008), acting as a reversible, competitive inhibitor. Also some chlorogenic acid derivatives, S3483 (Arion et al., 1998; Leuzzi et al., 2003), and S4048 (Herling et al., 1999), competitively inhibit G6PT, even more potently than chlorogenic acid. They have been used in studies concerning metabolic impairment in GSD-1 animal models (Bandsma et al., 2001; Grefhorst et al., 2010).
Several studies have established that G6P uptake activity needs not only an active G6PT, but also a functional G6Pase (Lei et al., 1996; Shieh et al., 2003; Chen et al., 2008; Pan et al., 2011). In this regard, hepatic microsomes isolated from G6Pase-α-deficient (GSD-Ia) mice, maintaining a functional G6PT, showed decreased G6P uptake activity, when compared to wild type hepatic microsomes (Lei et al., 1996). Accordingly, in a GSD-Ia mouse model, G6P transport activity could be restored by gene therapy supporting G6Pase-α function (Zingone et al., 2000). On this basis, functional coupling between G6PT and G6Pase-α became evident. A first explanation was suggested considering that G6PT provided the enzyme's substrate by importing cytoplasmic G6P into the ER lumen. Secondly, the physical interaction between G6Pase-α and G6PT, probably mediated by allosteric mechanisms, could support transport activity. This functional coupling was verified achieving functional cell-based activity assays for recombinant G6PT proteins, in order to measure G6P transport activity (Hiraiwa et al., 1999; Chen et al., 2000, 2002, 2008; Pan et al., 2011). According to these studies, it was demonstrated that microsomes expressing a functional G6Pase-α, but lacking an active G6PT (G6Pase-α+/+/G6PT –/–) showed little or no G6P uptake activity. In the same way, microsomes expressing an active G6PT but having a defective G6Pase-α (G6Pase-α –/– /G6PT+/+) exhibited poor G6P uptake rates, and microsomes expressing functional G6Pase-α and G6PT (G6Pase-α+/+/G6PT+/+) had strikingly increased G6P uptake rates (Chou and Mansfield, 2014). Furthermore, using a reconstitution procedure into proteoliposomes (Della Rocca et al., 2015; Curcio et al., 2016) preloaded with Pi, G6PT was proven to be an antiporter able to efficiently exchange G6P/Pi, without needing for a G6Pase-α coexpression (Chen et al., 2008). Those evidences suggested that G6Pase-α coexpression might increase intraluminal Pi concentration, in order to create a driving Pi gradient, useful for supporting G6PT antiporter activity. Cell-based assays and functional reconstitution into proteoliposomes were also successfully employed to characterize 23 SLC37A4 mutations identified in GSD-Ib patients (Chen et al., 2008).
SLC37A4 and Autophagy
Recently, SLC37A4 was identified as a key activator of autophagy pathway, able to negatively regulate mammalian target of rapamycin complex 1 (mTORC1) activity (Ahn et al., 2015). Autophagy is activated under nutrient deficiency to preserve cell homeostasis, and its deficit is associated with several human diseases (Jiang and Mizushima, 2014). Amino acids deprivation induces autophagy by inhibiting mTORC1 (Sancak et al., 2010), conversely, mTORC1 inhibits autophagy by phosphorylating unc-51 like autophagy activating kinase 1 (ULK1) (Jung et al., 2009) and autophagy/beclin-1 regulator 1, in order to ubiquitinate ULK1 for degradation (Nazio et al., 2013). Autophagy can also be triggered by ER stresses (Kouroku et al., 2007) or hypoxia (Bellot et al., 2009). On the other hand, prolonged ER stress leads to the inhibition of autophagy flux (Lee H. et al., 2012). Among the several autophagy-related genes (ATGs), ULK1 plays a key role, since it encodes a serine/threonine kinase essential for the initiation step of autophagy, because it forms complexes that are mainly controlled by mTORC 1 (Ganley et al., 2009) and 5′ AMP-activated protein kinase (AMPK) (Kim et al., 2011).
Among the ATGs encoded proteins, ATG9 is a membrane polypeptide depending on ULK1 activity, that regulates autophagosomes biogenesis by delivering them membrane source derived from the trans Golgi network (Young et al., 2006). SLC37A4 seems to promote the initiation step of autophagy acting upstream of mTORC1. In detail, SLC37A4 overexpression increases the interaction between N-terminal Venus-tagged ULK1 (ULK1-VN) and C-terminal Venus-tagged ATG9 (ATG9-VC), improving autophagic flux independent of G6PT transport activity (Ahn et al., 2015).
Previous studies demonstrated that G6PT-mTORC1 signaling is essential in promoting autophagy in hepatic cell lines, in addition, mTORC1 failure is often related to metabolic diseases, including type 2 diabetes and cancer (Zoncu et al., 2011). In this regard, it was proposed that this translocase could affect mTORC1 function through calcium mobilization (Chen et al., 2003). Furthermore, it was also suggested that G6PT could modulate mTORC1 through AMPK, which in turn is an energy sensor activated in response to augmented cellular AMP, ADP or calcium levels (Mihaylova and Shaw, 2011). Since AMPK can regulate autophagy through either direct ULK1 phosphorylation or mTORC1 inhibition (Hawley et al., 2005), disruption of calcium mobilization due to SLC37A4 dysfunction might influence both AMPK and mTORC1, leading to autophagy inhibition (Ahn et al., 2015).
SLC37A4 Defect Leads to GSD-Ib
SLC37A4 is the G6PT shared by the G6PT/G6Pase-α or -β complexes and responsible for GSD-Ib (Chou et al., 2002, 2010b; Chou and Mansfield, 2014).
Early studies based on the activity of the G6PT/G6Pase-α complex suggested the existence of five GSD-I subtypes, referred to as Ia (affecting the G6Pase catalytic subunit), Ib (affecting G6PT), IaSP, Ic, and Id, believed to arise from T2, T3, and SP deficiency, respectively (Lei et al., 1995; Matern et al., 2002). Furthermore, G6Pase-β deficit was responsible for the onset of GSD-Irs (Boztug et al., 2009).
In the past, partial kinetic analysis demonstrated a deficit of Pi export from the microsomal lumen, suggesting the existence of a third form of GSD-I, called GSD-Ic (OMIM 232240), caused by the involvement of a third gene postulated in the pathogenesis of the disease (Nordlie et al., 1983). Subsequently, genotyping studies found out detrimental mutations in the human SLC37A4 gene (Veiga-da-Cunha et al., 1998; Galli et al., 1999; Janecke et al., 2000), therefore it was confirmed that either GSD-Ib or -Ic were caused by mutations occurring in the same gene (Veiga-da-Cunha et al., 1999). Additional defects, reported in patients, affected either microsomal glucose translocation (Lei et al., 1995), or SP, a hypothetical 21-kD protein, able to stabilize the G6Pase catalytic unit in vitro (Burchell et al., 1985). These conditions were initially classified as GSD-Id and GSD-IaSP (Burchell and Waddell, 1990), respectively. A patient diagnosed with GSD-IaSP was found to be homozygous for a G6Pase mutation, so GSD-IaSP was reclassified as GSD-Ia (Lei et al., 1995). In the same way, the diagnosis of GSD-Id was withdrawn, because this disorder was caused by a single mutation found in the human SLC37A4 gene (Veiga-da-Cunha et al., 1999, 2000). As a result, GSD-Ib was implicated in all the reported cases of non-GSD-Ia (Chou et al., 2010b).
G6PT deficiency causes GSD-Ib, an autosomal recessively inherited disease, involving ~20% of all GSD-I patients (Chou et al., 2002, 2010b). This disorder is not limited to any racial or ethnic group, although the prevalence of some mutations is higher in whites and Japanese ethnicity (Chou et al., 2010b).
Up to date, 110 separate mutations have been identified in the SLC37A4 gene of GSD-Ib and non-GSD-Ia studied patients, including 61 missense/nonsense 1 regulatory and 17 splicing mutations, 29 small insertion/deletions, and 2 gross deletions (http://www.hgmd.cf.ac.uk/ac/gene.php?gene=SLC37A4). They were distributed throughout the gene. The missense mutation c.443C>T (A148V) seems to be restricted to Korean population, since it has not been reported in other ethnic groups (Rihwa et al., 2017). Several residues critical for G6PT function reside in the consensus sequence shared by the SLC37 family members (Figure 1), as well as by other OPA family members (Chou and Mansfield, 2014). In the topological model proposed for G6PT according to glycosylation scanning and protease sensitivity studies (Pan et al., 2009), many mutations are located in the first ER luminal loop (Figure 2). Here, a conserved arginine (R28), corresponding to R46 in UhpT and essential for activity (Lloyd and Kadner, 1990), is believed to constitute part of the substrate binding site and is required for G6P transport (Pan et al., 2009). Furthermore, N-terminal residues and helix 1 play a key role in transport activity, because the N-terminal mutation called MIV, lacking the N-terminal domain (residues1-7) and the first part of helix 1 (residues 8-16), abolishes transport function (Chen et al., 2002), but it does not interfere with G6PT stability (Chou and Mansfield, 2014). Conversely, the C-terminal domain deeply affects protein stability, since the nonsense mutation R415X, eliminating the whole cytoplasmic tail, causes a more rapid G6PT degradation with respect to the wild-type (Chen et al., 2000). Moreover, integrity of helix 10 is structurally important, because nonsense mutations E401X and T408X reduce G6PT expression and affect its folding (Chou and Mansfield, 2014).
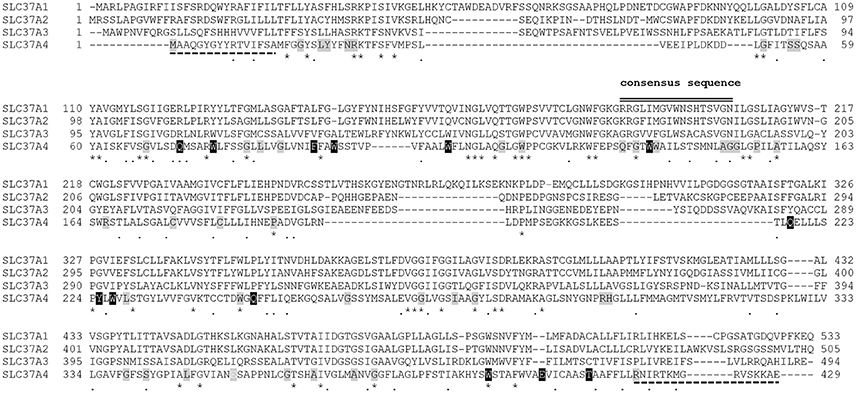
Figure 1. Alignment of the amino acid sequences of human SLC37A1, SLC37A2, SLC37A3, and SLC37A4 showing the location of nonsense and missense mutations identified in GSD-Ib patients. The aligned amino acid sequences are GENBANK accession numbers NP_061837.3 (SLC37A1), NP_938018.1 (SLC37A2), AAH46567.1 (SLC37A3), and CAG33014.1 (SLC37A4). Sequence conservation is indicated by an asterisk for identical residues, a dot for conserved substitutions, and a gap for non-conserved residues. The organo-phosphate/Pi antiporter family consensus sequence, ProSite PDOC00726, shared by the SLC37 family members is indicated by black double lines. Dashed black lines show lacking residues at the N- or C- terminal end in mutants MIV and R415X, respectively. Nonsense and missense mutations are highlighted in black or gray, respectively. Alignment has been performed by ClustalW.
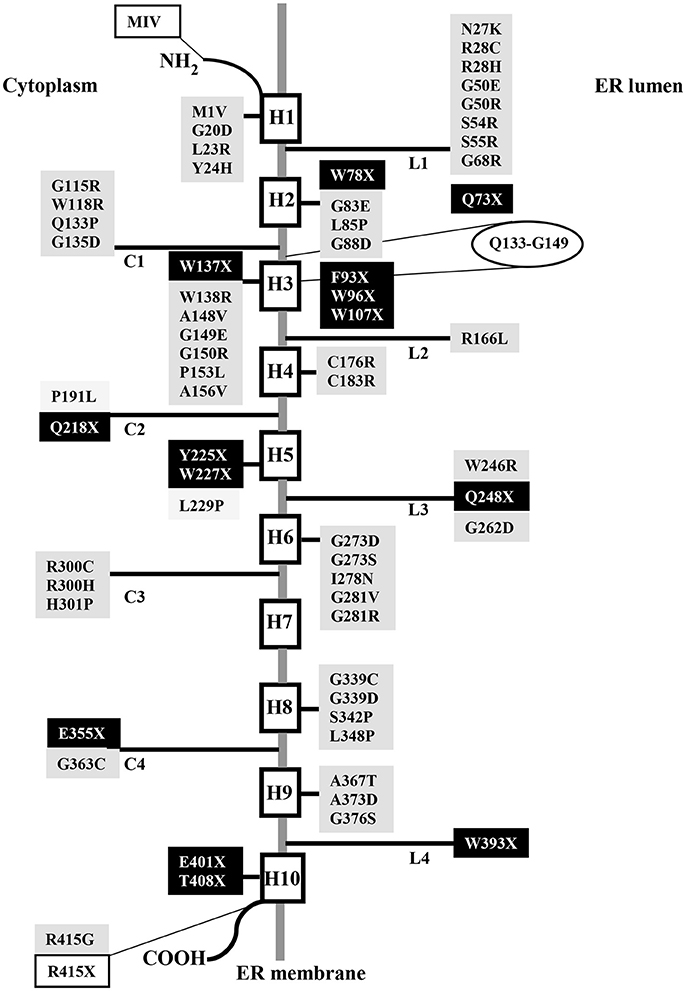
Figure 2. Schematic topological model of human G6PT displaying nonsense and missense mutations identified in GSD-Ib patients. Nonsense and missense mutations are highlighted in black or gray, respectively. The extension of the consensus sequence is reported in an ellipse. White boxes represent mutations that eliminate the N- or C- terminal domain.
SLC37A4 Defect: Biochemical Features and Clinical Phenotypes
GSD-Ib-related symptoms are associated with both defective metabolic and myeloid phenotypes (Chou et al., 2010a,b). GSD-Ib metabolic phenotype is shared with GSD-Ia. Interprandial blood glucose homeostasis is controlled by the liver, the principal gluconeogenic organ, and to a lesser extent, by the kidney and intestine. Between meals, G6P produced in these organs during gluconeogenesis and glycogenolysis is imported into the ER lumen by G6PT, where it is hydrolyzed by G6Pase-α to produce glucose, then exported back into the bloodstream (Figure 3A; Chen, 2001; Chou et al., 2002). Since G6PT, as well as G6Pase-α, are abundantly expressed in gluconeogenic organs, when G6PT is defective the G6PT/G6Pase-α complex activity is defective. As a result of an inadequate glucose production, patients suffer from fasting hypoglycemia. At the same time, G6P cytoplasmic elevation leads to an abnormal storage of glycogen, which causes progressive nephromegaly and hepatomegaly (favoring a protruding abdomen), along with hyperlipidaemia, hyperuricemia, and lactic acidemia, besides, hepatomegaly is worsened by liver fat accumulation (Chen, 2001; Chou et al., 2002; Figure 3B). In GSD-Ib patients, short stature, xanthomas, and diarrhea have also been reported; additionally, fasting hypoglycemia may cause seizure. Signs and symptoms of the disorder generally develop during the childhood, around the age of 3 or 4 months, when babies start to sleep through the night, not eating as frequently as newborns. Affected children have a typical aspect with puffy cheeks and doll-like facies (Bartram et al., 1981). Untreated GSD-Ib is childhood lethal (Chou and Mansfield, 2011). Long-term complications include growth retardation, delayed puberty, osteoporosis, pancreatitis, gout, pulmonary hypertension, polycystic ovaries, and increased risk of hepatocellula adenoma (Chou et al., 2002, 2010b; Rake et al., 2002).
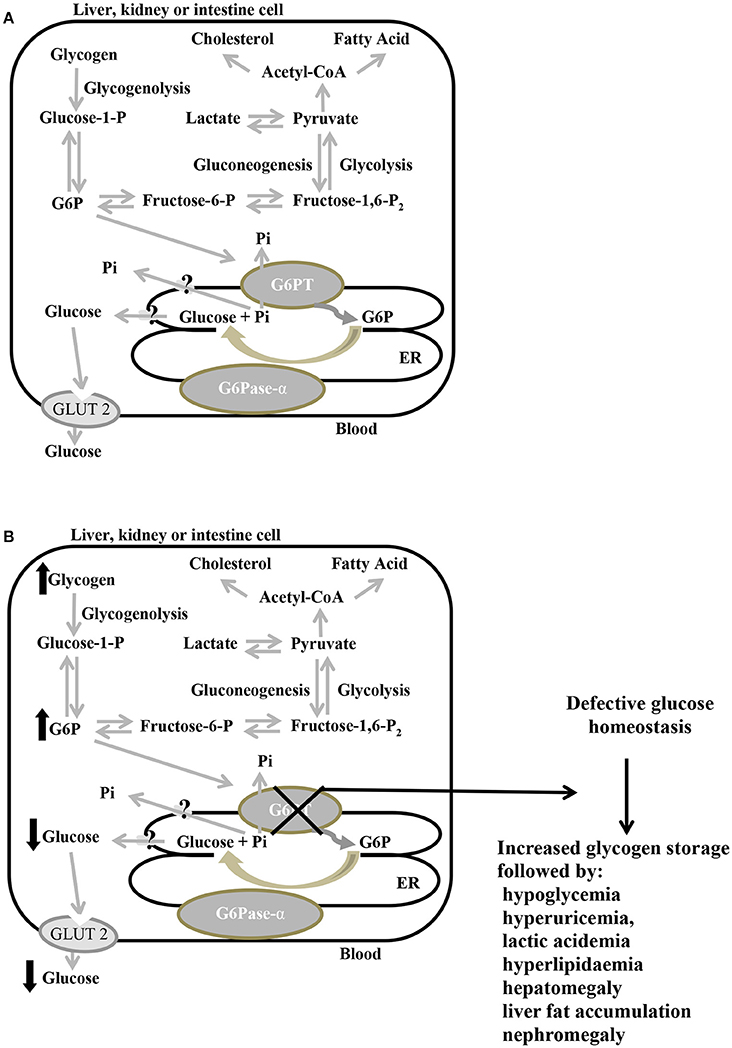
Figure 3. Primary metabolic pathways of G6P in the liver, kidney, and intestine, in normal (A) and defective G6PT (B) cells. Schematic cell harboring an extended endoplasmic reticulum (ER). G6Pase-α and G6PT are embedded in the ER membrane; the glucose transporter GLUT2 is embedded in the plasma membrane. Black arrows indicate metabolic changes due to defective SLC37A4. G6P, glucose-6-phosphate; G6Pase-α, glucose-6-phosphatase-α; G6PT, glucose-6-phosphate translocase; GLUT2, glucose transporter 2; P, phosphate; Pi, inorganic phosphate.
GSD-Ib myeloid phenotype is shared with GSD-Irs. A faulty G6PT/G6Pase-β complex activity causes neutrophil dysfunction and congenital neutropenia, therefore either GSD-Ib or GSD-Irs patients suffered from recurrent infections.
In neutrophils, glucose imported into the cytoplasm via GLUT1 is metabolized by hexokinase to G6P, which in turn enters the ER lumen through G6PT, where it can accumulate until it is hydrolyzed to glucose by G6Pase-β and transported back into the cytoplasm. Intracytoplasmic G6P/glucose ratio is affected by several pathways, such as glycolysis, pentose phosphate pathway, and recycling of G6P/glucose between the ER lumen and the cytoplasm (Jun et al., 2010; Figure 4A). The G6PT/G6Pase-β complex plays a key role in the third pathway, because glucose recycling decreases cytoplasmic G6P/glucose ratio, so regulating the previously mentioned cytoplasmic pathways for G6P metabolism. As a consequence, G6PT impairment arises a lack of glucose recycling that can cause impaired neutrophil, macrophage, and monocytes functionality, as well as energy homeostasis, leading to reduced intracellular levels of G6P, lactate, ATP and NADPH (McCawley et al., 1993; Jun et al., 2010). A defective G6PT can also cause reduced neutrophil respiratory burst, chemotaxis, calcium mobilization and phagocytic activities (Figure 4B; Kilpatrick et al., 1990; Chou et al., 2010a; Jun et al., 2014).
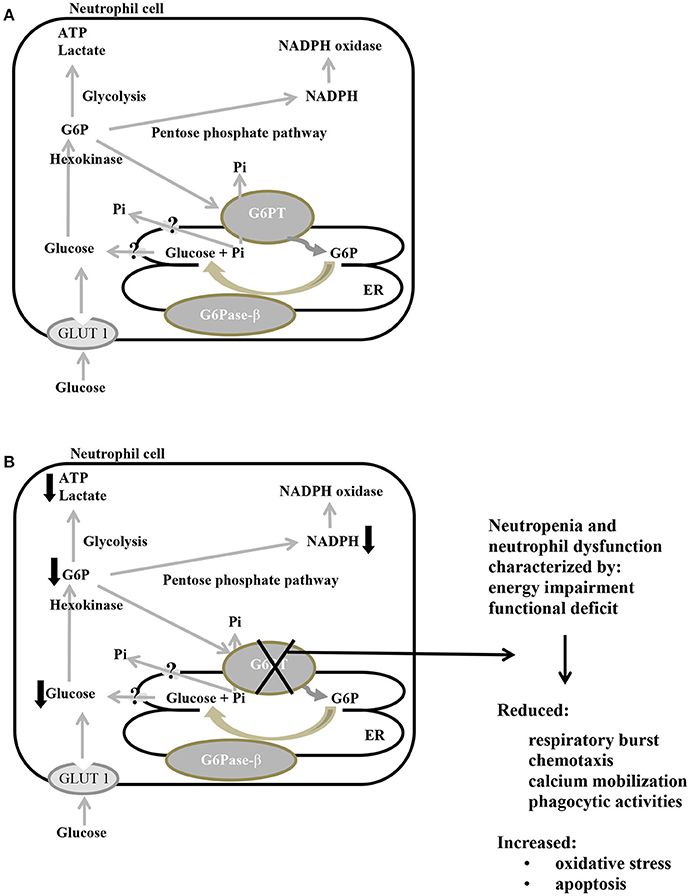
Figure 4. Main metabolic pathways of G6P in normal (A) and defective G6PT (B) neutrophils. Schematic cell showing an extended endoplasmic reticulum (ER) and the three major pathways (glycolysis, pentose phosphate pathway, and ER cycling) in which G6P is involved. G6Pase-β and G6PT are embedded in the ER membrane; GLUT 1 is embedded in the plasma membrane. Black arrows indicate metabolic changes due to defective SLC37A4.: G6P, glucose-6-phosphate; G6Pase-β, glucose-6-phosphatase-β; G6PT, glucose-6-phosphate translocase; GLUT 1, glucose transporter 1; P, phosphate; Pi, inorganic phosphate; ATP, adenosine triphosphate; NADPH, nicotinamide adenine dinucleotide phosphate.
Furthermore, in G6PT-deficient neutrophils, reduced respiratory burst was associated with an impaired activation of NADPH oxidase, a multicomponent enzyme promoting the production of reactive oxygen species (Jun et al., 2014). Neutrophil metabolism is dependent on anaerobic glycolysis for ATP production. Under hypoxia the protein levels of the hypoxia-inducible transcriptional factor-1α (HIF-1α) increase (Bárdos and Ashcroft, 2005), and neutrophils display defective respiratory burst activity (McGovern et al., 2011). HIF-1α is also an upstream activator of peroxisome proliferator-activated receptor-γ (PPAR-γ) (Krishnan et al., 2009), a nuclear receptor involved in the regulation of lipid and glucose metabolism, influencing inflammation and many other diseases (Kvandova et al., 2016). It was observed that in neutrophils PPAR-γ is constitutively expressed, and its activation leads to chemotaxis inhibition (Reddy et al., 2008). On this basis, it was supposed that the activation of the HIF-1α/PPAR-γ pathway in neutrophils of GSD-Ib patients could trigger neutrophil dysfunction, impairing chemotaxis and calcium mobilization activities (Jun et al., 2014).
GSD-Ib patients may also experience oral symptoms, consisting of dental caries, periodontal diseases, gingivitis, delayed dental maturation and eruption, oral bleeding diathesis and ulcers (Mortellaro et al., 2005). Remarkably, not all GSD-Ib patients manifest neutropenia or frequent infections (Kure et al., 2000; Melis et al., 2005; Angaroni et al., 2006; Martens et al., 2006). In this regard, in a multicentre study investigating the genotype/phenotype correlation on a cohort of 25 GSD-Ib patients, no correlation was found between individual mutations and the presence of neutropenia, bacterial infections or systemic complications. This evidence might suggest the existence of unknown factors able to influence immune phenotype, such as polymorphisms, proteins or genes, capable of modulating neutrophil differentiation, maturation, and apoptosis (Melis et al., 2005). Considering that neutrophils of GSD-Ib patients exhibited enhanced apoptosis, a causal relationship between apoptosis and neutropenia was hypothesized (Kuijpers et al., 2003; Jun et al., 2014). This theory was supported by further studies conducted on animal models, demonstrating that either neutrophils from G6Pase-β −/− mice or those from G6PT −/− mice exhibited enhanced ER stress and apoptosis (Cheung et al., 2007; Kim et al., 2008). So, neutrophil ER stress, higher oxidative stress and apoptosis might be underlying causes of neutropenia in GSD-Ib (Jun et al., 2010). In addition, neutrophil apoptosis in both G6Pase-β −/− (Jun et al., 2011) and G6PT −/− (Kim et al., 2008) mice was mediated by the intrinsic apoptosis pathway. In GSD-Ib, a maturation arrest seems not to be responsible for neutrophil dysfunction (Jun et al., 2014). Since neutrophils, as well as macrophages, have to import glucose from the blood by the glucose transporters (Pessin and Bell, 1992), these cells critically depend on the G6PT/G6Pase-β complex activity, especially when the need for glucose rises (Jun et al., 2014). This might provide a rationale for neutropenia caused by enhanced neutrophil ER stress, oxidative stress and apoptosis, when G6PT and/or G6Pase-β are defective (Kuijpers et al., 2003; Kim et al., 2008; Jun et al., 2010, 2014).
Autoimmunity Risk of SLC37A4 Defect
A strong association was found between GSD-Ib and inflammatory bowel disease (IBD), because many GSD-Ib patients with chronic gastrointestinal inflammation were diagnosed with IBD, which was clinically indistinguishable from idiopathic Crohn disease, assuming the involvement of an impaired mucosal innate immunity in the pathogenesis of IBD (Dieckgraefe et al., 2002). The association between neutropenia and IBD was supported by Visser et al., in a retrospective European study, showing that up to 77% of patients with GSD-Ib presented also neutropenia, as well as many GSD-Ib patients suffering from perioral or perianal infections (Visser et al., 2000).
In patients with GSD-Ib an increased risk for Crohn-like disease was reported (Melis et al., 2003), along with a severe deficit of hypothalamus-pituitary-thyroid axis, causing an increased prevalence of thyroid autoimmunity and hypothyroidism (Melis et al., 2007). In one patient, the occurrence of myasthenia gravis was also described (Melis et al., 2008). These observations paved the way to the hypothesis that GSD-Ib patients, but not those affected by GSD-Ia, were at increased risk for autoimmune disorders. The different risk degree between GSD-Ia and GSD-Ib patients might be related to the presence of neutropenia and neutrophil dysfunction only in GSD-Ib (Melis et al., 2007). Some studies have also suggested an association between lymphopenia and autoimmunity (Merayo-Chalico et al., 2016). Chronic lymphopenia might promote autoimmunity inducing the homeostatic expansion of T cell, which represents a normal compensatory reaction during lymphopenic conditions (King et al., 2004).
Recent studies have highlighted the molecular mechanisms underlying the increased frequency of autoimmunity disorders shown in GSD-Ib patients (Melis et al., 2017).
Regulatory T cells (Tregs) are a subset of CD4+ T cells involved in maintaining tolerance to self-antigens, and they play a critical role in human autoimmune diseases, because their imbalance seems to be responsible for the failure of local regulatory mechanisms (Dejaco et al., 2006). Those cells mediate their suppressive function by acting directly on conventional T cells (Tconvs) and antigen-presenting cells, such as dendritic cells (Rueda et al., 2016). Tregs express the transcription factor forkhead box P3 (FOXP3), which is very important for Tregs development and function, since defects in the FOXP3 gene cause a human lethal autoimmune disease (Bennett et al., 2001).
Treg responses can be modulated by several metabolic pathways (Buck et al., 2015), particularly by glycolysis, able to affect human Treg induction and function (De Rosa et al., 2015). In this regard, a defective engagement of glycolysis upon suboptimal T cell receptor (TCR) stimulation of conventional T cells (Tconvs) has been observed in several human autoimmune diseases, and it has been associated with a decreased suppressive function of Tregs (De Rosa et al., 2015).
In GSD-Ib, a defective G6PT causes a decreased capacity to mobilize glucose, leading to diminished glucose utilization. Consequently, a reduced engagement of glycolysis in T cells occurs upon suboptimal TCR stimulation; additionally, qualitative and quantitative alterations of peripheral regulatory T cells (pTregs) have been observed, along with impaired FOXP3 expression by Tconvs during low TCR activation (Melis et al., 2017).
Therapeutic Approaches for SLC37A4 Defect
Clinical therapies consist of dietary treatment, granulocyte colony-stimulating factor (G-CSF) therapy and transplantations.
Since recurrent hypoglycemia is responsible for lactic acidosis, hyperuricemia and hypertriglyceridemia, the primary goal of nutritional therapy is to achieve a stable normoglycemia. In addition, dietary treatment aims to reduce metabolic impairment, delaying some of the long-term GSD-Ib consequences. Unfortunately, elevated triglycerides and cholesterol blood levels may persist regardless of diet, along with their consequences (Cappello et al., 2016); moreover, even if nutritional therapy can improve long-term outcomes for many patients, several long-term complications remain. Recommended diet composition is 60–70% calories from carbohydrates, 10–15% from proteins and the remaining from fats (Goldberg and Slonim, 1993). Small frequent feedings rich in complex carbohydrates (particularly those higher in fiber) have to be regularly ingested over 24 h to achieve a good metabolic control (Wolfsdorf and Weinstein, 2003; Kishnani et al., 2014). GSD-Ib, as well as GSD-Ia patients, can use the same nutritional therapy to avoid hypoglycemia, especially nighttime (Chou et al., 2010b).
Cornstarch therapy in GSD-I is used since the early 1980s (Chen et al., 1984). Raw uncooked cornstarch is a slowly digested carbohydrate, which allows a slow release of glucose able to prolong the length of euglycemia between meals (Sidbury et al., 1986).
Glucose requirement normally decreases with age, so adults have a longer fasting tolerance with respect to children. Traditionally, in children older than 3 years, as well as in adults, normoglycemia during nighttime is maintained by the ingestion at bedtime of uncooked corn starch (Wolfsdorf and Crigler, 1997; Weinstein and Wolfsdorf, 2002; Shah and O'Dell, 2013) or of modified forms of corn starch (Bhattacharya et al., 2007; Correia et al., 2008). In children <3 years continuous tube feeding is usually needed (Greene et al., 1976), because the level of pancreatic amylase able to hydrolyze raw starch is low before 3 years of age (Chou et al., 2010b). A recent controlled crossover study enrolling adult GSD-Ia and GSD-Ib patients, highlighted that nighttime glucose control can be achieved also by the ingestion of a cooked pasta meal at bedtime, allowing a more palatable alternative to corn starch use (Hochuli et al., 2015).
G-CSF administration promotes the production of granulocytes (Mehta et al., 2015), increasing neutrophile numbers and reducing the frequency and severity of infections in GSD-Ib patients, but it can not improve neutrophile dysfunction (Visser et al., 2002). G-CSF also appears to increase neutrophil survival (Jun et al., 2011). In this regard, studies led in vivo, on murine G6Pase-β-deficient models, highlighted that G-CSF therapy was able to normalize neutrophil energy homeostasis and to improve functionality, as supported by improved neutrophil glucose uptake and increased G6P, ATP, and lactate intracellular concentrations (Jun et al., 2011).
A recent study has evidenced a partial improvement of neutrophil respiratory burst activity in a GSD-Ib patient by oral administration of galactose, even though the role of galactose in sugar metabolism of GSD-Ib neutrophils remains to be clarified (Letkemann et al., 2017).
Neutropenia associated with IBD can be treated by using a combination of G-CSF and 5-aminosalicylic acid (Visser et al., 2002; Koeberl et al., 2009; Chou et al., 2010b; Shah and O'Dell, 2013).
In some GSD-Ib patients, metabolic abnormalities can be corrected by liver or combined liver/kidney transplantation, since such operations have improved metabolic control, restoring normal fasting tolerance (Boers et al., 2014). Patients with impending renal failure are suggested to consider combined liver/kidney transplantation (Labrune, 2002). In GSD-Ib patients, myeloid dysfunctions can be treated by bone marrow transplantation, which appears to be a promising approach, even if it requires further validation (Pierre et al., 2008). According to the guidelines from the European study on GSD-I, liver transplantation is suggested in GSD-I patients with unresectable hepatocellular adenomas unresponsive to nutritional therapy, mainly if adenomas are associated with serious compression or hemorrhage, or in the case of enhanced risk of transformation into hepatocellular carcinomas (Rake et al., 2002). Although liver transplantation improves metabolic impairment (Reddy et al., 2009), its usefulness on renal disease and neutropenia or neutrophil dysfunction is yet to be settled.
Gene Therapy for SLC37A4 Defect
Gene therapy represents a promising strategy to correct metabolic abnormalities in all GSD-I patients (Chou and Mansfield, 2011), although currently it seems not to compensate for myeloid and renal dysfunction in GSD-Ib patients (Kwon et al., 2017).
Several gene transfer vectors, including recombinant adeno-associated virus (rAAV) vectors, have been developed and tested, either on GSD-Ia or on GSD-Ib animal models (Chou et al., 2015; Kwon et al., 2017).
G6PT knockout (G6PT−/−) mice are excellent G6PT-deficient murine models, since they exhibit all the metabolic and myeloid dysfunctions typical of human GSD-Ib (Chen et al., 2003). Some studies led on those mice showed that recombinant adenovirus-mediated G6PT gene transfer was able to deliver the transgene to the liver and bone marrow, improving metabolic and myeloid defects; however, this therapy was short-lived, owing to the fast loss of vector-mediated gene expression (Yiu et al., 2007).
Further studies led on such mice showed that a human G6PT expressing recombinant AAV serotypes 2/8 vector (rAAV8) directed by a hybrid chicken β-actin (CBA) promoter and a cytomegalovirus (CMV) enhancer, was able to deliver the transgene primarily to the liver, thus normalizing metabolic abnormalities. Nevertheless, two of the five treated mice survived for 51–72 weeks, developed multiple hepatocellular adenomas, and one experienced cancer transformation (Yiu et al., 2009). So, this therapeutic approach, while allowing normoglicemia, could not prevent long term complications of hepatocellular adenoma.
A further work, on G6Pase-α-deficient murine models (manifesting all the symptoms of human GSD-Ia), was led in order to compare two different gene transfer methods. In one case, murine G6Pase-α gene transfer in vivo was mediated by recombinant AAV serotypes 2/1 (rAAV1) or rAAV8 vectors, and expression was directed by hybrid CBA promoter/CMV enhancer. In the other case, a rAAV8 vector expressing human G6Pase-α directed by its promoter/enhancer (GPE) was employed (Yiu et al., 2010).
This work highlighted that the gluconeogenic tissue-specific human GPE was more effective in directing persistent in vivo hepatic transgene expression with respect to the CBA promoter/CMV enhancer, because human GPE utilization triggered a lesser humoral immune response, allowing complete normalization of hepatic G6Pase-α deficiency (Yiu et al., 2010). In addition, in murine GSD-Ia models, the use of a G6Pase-α-expressing rAAV vector under the GPE control was able to correct metabolic defects with no hepatocellular adenoma development (Lee Y. M. et al., 2012).
On this basis, recently two different liver directed gene therapy approaches were tested on G6PT−/− mice, using rAAV-GPE-G6PT and rAAV-miGT-G6PT, this latter consisted of a human G6PT expressing rAAV directed by human minimal G6PT promoter/enhancer (miGT) (Hiraiwa and Chou, 2001; Kwon et al., 2017).
Both vectors could deliver the hG6PT transgene to the liver, correcting metabolic anomalies, but rAAV-GPE-G6PT vector directed by the hG6Pase-α promoter/enhancer had greater efficacy. A full restoration of normal G6PT activity was not necessary to obtain significant therapeutic benefits, since a restoration of 3-62% seemed to confer protection against age-related obesity and insulin resistance, while restoration <6% exposed to hepatic cancer risk (Kwon et al., 2017).
Author Contributions
AC, RC and RL conducted literary review, wrote the article and prepared publication-ready figures, MM and VD designed and edited the article.
Conflict of Interest Statement
The authors declare that the research was conducted in the absence of any commercial or financial relationships that could be construed as a potential conflict of interest.
Acknowledgments
This work was supported by Associazione Italiana per la Ricerca sul Cancro (MM grant no. 16719/2015).
Abbreviations
AMPK, 5′ AMP-activated protein kinase; ATG, autophagy-related gene; CBA, chicken β-actin; CRC, colorectal cancer; WAT, white adipose tissue; CHI, congenital hyperinsulinism of infancy; Tconvs, conventional T cells; FOXP3, forkhead box P3; G6Pase-α, promoter/enhancer GPE; G6Pase-system, glucose-6-phosphatase system; G6PT, glucose-6-phosphate translocase; GSD-I, glycogen storage disease type I; G-CSF, granulocyte colony-stimulating factor; HIF-1α, hypoxia-inducible transcriptional factor-1α; IBD, inflammatory bowel disease; mTORC1, mammalian target of rapamycin complex 1; miGT, minimal G6PT promoter/enhancer; OPA, organophosphate/phosphate antiporter; PPAR-γ, peroxisome proliferator-activated receptor-γ; phospho-Ser294 PR, phospho-Ser294 progesterone receptor; CMV, cytomegalovirus; rAAV, recombinant adeno-associated virus; Tregs, regulatory T cells; RUNX2, runt-related transcription factor 2; SLC, solute-carrier; SP, stabilizing protein; SPX, sugar-phosphate exchangers; TCR, T cell receptor; ULK1, unc-51 like autophagy activating kinase 1; VDR, vitamin D receptor.
References
Ahn, H. H., Oh, Y., Lee, H., Lee, W., Chang, J. W., Pyo, H. K., et al. (2015). Identification of glucose-6-phosphate transporter as a key regulator functioning at the autophagy initiation step. FEBS Lett. 589, 2100–2109. doi: 10.1016/j.febslet.2015.05.018
Almqvist, J., Huang, Y., Hovmöller, S., and Wang, D. N. (2004). Homology modeling of the human microsomal glucose 6-phosphate transporter explains the mutations that cause the glycogen storage disease type Ib. Biochemistry 43, 9289–9297. doi: 10.1021/bi049334h
Angaroni, C. J., Labrune, P., Petit, F., Sastre, D., Capra, A. E., Dodelson de Kremer, R., et al. (2006). Glycogen storage disease type Ib without neutropenia generated by a novel splice-site mutation in the glucose-6-phosphate translocase gene. Mol. Genet. Metab. 88, 96–99. doi: 10.1016/j.ymgme.2005.12.011
Annabi, B., Hiraiwa, H., Mansfield, B. C., Lei, K. J., Ubagai, T., Polymeropoulos, M. H., et al. (1998). The gene for glycogen storage disease type 1b maps to chromosome 11q23. Am. J. Hum. Genet. 62, 400–405. doi: 10.1086/301727
Arion, W. J., Canfield, W. K., Ramos, F. C., Schindler, P. W., Burger, H. J., Hemmerle, H., et al. (1997). Chlorogenic acid and hydroxynitrobenzaldehyde: new inhibitors of hepatic glucose 6-phosphatase. Arch. Biochem. Biophys. 339, 315–322. doi: 10.1006/abbi.1996.9874
Arion, W. J., Canfield, W. K., Ramos, F. C., Su, M. L., Burger, H. J., Hemmerle, H., et al. (1998). Chlorogenic acid analogue S 3483: a potent competitive inhibitor of the hepatic and renal glucose-6-phosphatase systems. Arch. Biochem. Biophys. 351, 279–285. doi: 10.1006/abbi.1997.0563
Arion, W. J., Wallin, B. K., Carlson, P. W., and Lange, A. J. (1972). The specificity of glucose 6-phosphatase of intact liver microsomes. J. Biol. Chem. 247, 2558–2565.
Arion, W. J., Wallin, B. K., Lange, A. J., and Ballas, L. M. (1975). On the involvement of a glucose 6-phosphate transport system in the function of microsomal glucose 6-phosphatase. Mol. Cell. Biochem. 6, 75–83. doi: 10.1007/BF01732001
Bandsma, R. H., Wiegman, C. H., Herling, A. W., Burger, H. J., ter Harmsel, A., Meijer, A. J., et al. (2001). Acute inhibition of glucose-6-phosphate translocator activity leads to increased de novo lipogenesis and development of hepatic steatosis without affecting VLDL production in rats. Diabetes 50, 2591–2597. doi: 10.2337/diabetes.50.11.2591
Bárdos, J. I., and Ashcroft, M. (2005). Negative and positive regulation of HIF-1: a complex network. Biochim. Biophys. Acta 1755, 107–120. doi: 10.1016/j.bbcan.2005.05.001
Bartella, V., De Francesco, E. M., Perri, M. G., Curcio, R., Dolce, V., Maggiolini, M., et al. (2016). The G protein estrogen receptor (GPER) is regulated by endothelin-1 mediated signaling in cancer cells. Cell. Signal. 28, 61–71. doi: 10.1016/j.cellsig.2015.11.010
Bartoloni, L., and Antonarakis, S. E. (2004). The human sugar-phosphate/phosphate exchanger family SLC37. Pflugers Arch. 447, 780–783. doi: 10.1007/s00424-003-1105-0
Bartoloni, L., Wattenhofer, M., Kudoh, J., Berry, A., Shibuya, K., Kawasaki, K., et al. (2000). Cloning and characterization of a putative human glycerol 3-phosphate permease gene (SLC37A1 or G3PP) on 21q22.3: mutation analysis in two candidate phenotypes, DFNB10 and a glycerol kinase deficiency. Genomics 70, 190–200. doi: 10.1006/geno.2000.6395
Bartram, C. R., Przyrembel, H., Wendelz, U., Bremer, H. J., Schaub, J., and Haas, J. R. (1981). Glyeogenosis type I b complicated by severe granuloeytopenia resembling inherited Neutropenia. Eur. J. Pediatr. 137, 81–84. doi: 10.1007/BF00441175
Bellot, G., Garcia-Medina, R., Gounon, P., Chiche, J., Roux, D., Pouysségur, J., et al. (2009). Hypoxia-induced autophagy is mediated through hypoxia-inducible factor induction of BNIP3 and BNIP3L via their BH3 domains. Mol. Cell. Biol. 29, 2570–2581. doi: 10.1128/MCB.00166-09
Bennett, C. L., Christie, J., Ramsdell, F., Brunkow, M. E., Ferguson, P. J., Whitesell, L., et al. (2001). The immune dysregulation, polyendocrinopathy, enteropathy, X-linked syndrome (IPEX) is caused by mutations of FOXP3. Nat. Genet. 27, 20–21. doi: 10.1038/83713
Benton, M. C., Johnstone, A., Eccles, D., Harmon, B., Hayes, M. T., and Lea, R. A. (2015). An analysis of DNA methylation in human adipose tissue reveals differential modification of obesity genes before and after gastric bypass and weight loss. Genome Biol. 16:8. doi: 10.1186/s13059-014-0569-x
Bhattacharya, K., Orton, R. C., Qi, X., Mundy, H., Morley, D. W., Champion, M. P., et al. (2007). A novel starch for the treatment of glycogen storage diseases. J. Inherit. Metab. Dis. 30, 350–357. doi: 10.1007/s10545-007-0479-0
Boers, S. J., Visser, G., Smit, P. G., and Fuchs, S. A. (2014). Liver transplantation in glycogen storage disease type I. Orphanet J. Rare Dis. 9:47. doi: 10.1186/1750-1172-9-47
Boztug, K., Appaswamy, G., Ashikov, A., Schäffer, A. A., Salzer, U., Diestelhorst, J., et al. (2009). A syndrome with congenital neutropenia and mutations in G6PC3. N. Engl. J. Med. 360, 32–43. doi: 10.1056/NEJMoa0805051
Buck, M. D., O'Sullivan, D., and Pearce, E. L. (2015). T cell metabolism drives immunity. J. Exp. Med. 212, 1345–1360. doi: 10.1084/jem.20151159
Burchell, A., and Waddell, I. D. (1990). Diagnosis of a novel glycogen storage disease: type 1aSP. J. Inherit. Metab. Dis. 13, 247–249. doi: 10.1007/BF01799362
Burchell, A., Burchell, B., Monaco, M., Walls, H. E., and Arion, W. J. (1985). Stabilization of glucose-6-phosphatase activity by a 21000-dalton hepatic microsomal protein. Biochem. J. 230, 489–495. doi: 10.1042/bj2300489
Cappello, A. R., Dolce, V., Iacopetta, D., Martello, M., Fiorillo, M., Curcio, R., et al. (2016). Bergamot (Citrus bergamia Risso) flavonoids and their potential benefits in human hyperlipidemia and atherosclerosis: an overview. Mini Rev. Med. Chem. 16, 619–629. doi: 10.2174/1389557515666150709110222
Chen, L. Y., Lin, B., Pan, C. J., Hiraiwa, H., and Chou, J. Y. (2000). Structural requirements for the stability and microsomal transport activity of the human glucose-6-phosphate transporter. J. Biol. Chem. 275, 34280–34286. doi: 10.1074/jbc.M006439200
Chen, L. Y., Pan, C. J., Shieh, J. J., and Chou, J. Y. (2002). Structure-function analysis of the glucose-6-phosphate transporter deficient in glycogen storage disease type Ib. Hum. Mol. Genet. 11, 3199–3207. doi: 10.1093/hmg/11.25.3199
Chen, L. Y., Shieh, J. J., Lin, B., Pan, C. J., Gao, J. L., Murphy, P. M., et al. (2003). Impaired glucose homeostasis, neutrophil trafficking and function in mice lacking the glucose-6-phosphate transporter. Hum. Mol. Genet. 12, 2547–2558. doi: 10.1093/hmg/ddg263
Chen, S. Y., Pan, C. J., Nandigama, K., Mansfield, B. C., Ambudkar, S. V., and Chou, J. Y. (2008). The glucose-6-phosphate transporter is a phosphate-linked antiporter deficient in glycogen storage disease type Ib and Ic. FASEB J. 22, 2206–2213. doi: 10.1096/fj.07-104851
Chen, Y. T. (2001). “Glycogen storage diseases,” in The Metabolic and Molecular Bases of Inherited Disease, 8th Edn, eds C. R. Scriver, A. L. Beaudet, W. S. Sly, and D. Valle (New York, NY: McGraw-Hill), 1521–1551.
Chen, Y. T., Cornblath, M., and Sidbury, J. B. (1984). Cornstarch therapy in type I glycogen-storage disease. N. Engl. J. Med. 310, 171–175. doi: 10.1056/NEJM198401193100306
Cheung, Y. Y., Kim, S. Y., Yiu, W. H., Pan, C. J., Jun, H. S., Ruef, R. A., et al. (2007). Impaired neutrophil activity and increased susceptibility to bacterial infection in mice lacking glucose-6-phosphatase-beta. J. Clin. Invest. 117, 784–793. doi: 10.1172/JCI30443
Chou, J. Y., and Mansfield, B. C. (2011). Recombinant AAV-directed gene therapy for type I glycogen storage diseases. Expert Opin. Biol. Ther. 11, 1011–1024. doi: 10.1517/14712598.2011.578067
Chou, J. Y., and Mansfield, B. C. (2014). The SLC37 family of sugar-phosphate/phosphate exchangers. Curr. Top. Membr. 73, 357–382. doi: 10.1016/B978-0-12-800223-0.00010-4
Chou, J. Y., Jun, H. S., and Mansfield, B. C. (2010a). Neutropenia in type Ib glycogen storage disease. Curr. Opin. Hematol. 17, 36–42. doi: 10.1097/MOH.0b013e328331df85
Chou, J. Y., Jun, H. S., and Mansfield, B. C. (2010b). Glycogen storage disease type I and G6Pase-b deficiency: etiology and therapy. Nat. Rev. Endocrinol. 6, 676–688. doi: 10.1038/nrendo.2010.189
Chou, J. Y., Sik Jun, H., and Mansfield, B. C. (2013). The SLC37 family of phosphate-linked sugar phosphate antiporters. Mol. Aspects Med. 34, 601–611. doi: 10.1016/j.mam.2012.05.010
Chou, J. Y., Jun, H. S., and Mansfield, B. C. (2015). Type I glycogen storage diseases: disorders of the glucose-6-phosphatase/glucose-6-phosphate transporter complexes. J. Inherit. Metab. Dis. 38, 511–519. doi: 10.1007/s10545-014-9772-x
Chou, J. Y., Matern, D., Mansfield, B. C., and Chen, Y. T. (2002). Type I glycogen storage diseases: disorders of the glucose-6-phosphatase complex. Curr. Mol. Med. 2, 121–143. doi: 10.2174/1566524024605798
Correia, C. E., Bhattacharya, K., Lee, P. J., Shuster, J. J., Theriaque, D. W., Shankar, M. N., et al. (2008). Use of modified cornstarch therapy to extend fasting in glycogen storage disease types Ia and Ib. Am. J. Clin. Nutr. 88, 1272–1276. doi: 10.3945/ajcn.2008.26352
Curcio, R., Muto, L., Pierri, C. L., Montalto, A., Lauria, G., Onofrio, A., et al. (2016). New insights about the structural rearrangements required for substrate translocation in the bovine mitochondrial oxoglutarate carrier. Biochim. Biophys. Acta 1864, 1473–1480. doi: 10.1016/j.bbapap.2016.07.009
De Rosa, V., Galgani, M., Porcellini, A., Colamatteo, A., Santopaolo, M., Zuchegna, C., et al. (2015). Glycolysis controls the induction of human regulatory T cells by modulating the expression of FOXP3 exon 2 splicing variants. Nat. Immunol. 16, 1174–1184. doi: 10.1038/ni.3269
Dejaco, C., Duftner, C., Grubeck-Loebenstein, B., and Schirmer, M. (2006). Imbalance of regulatory T cells in human autoimmune diseases. Immunology 117, 289–300. doi: 10.1111/j.1365-2567.2005.02317.x
Della Rocca, B. M., Miniero, D. V., Tasco, G., Dolce, V., Falconi, M., Ludovico, A., et al. (2015). Substrate-induced conformational changes of the mitochondrial oxoglutarate carrier: a spectroscopic and molecular modelling study. Mol. Membr. Biol. 22, 443–452. doi: 10.1080/09687860500269335
Dieckgraefe, B. K., Korzenik, J. R., Husain, A., and Dieruf, L. (2002). Association of glycogen storage disease 1b and Crohn disease: results of a North American survey. Eur. J. Pediatr. 161, S88–S92. doi: 10.1007/BF02680002
Dolce, V., Cappello, A. R., Lappano, R., and Maggiolini, M. (2011). Glycerophospholipid synthesis as a novel drug target against cancer. Curr. Mol. Pharmacol. 4, 167–175. doi: 10.2174/1874467211104030167
Fritz, S., Capitan, A., Djari, A., Rodriguez, S. C., Barbat, A., Baur, A., et al. (2013). Detection of haplotypes associated with prenatal death in dairy cattle and identification of deleterious mutations in GART, SHBG and SLC37A2. PLoS ONE 8:e65550. doi: 10.1371/journal.pone.0065550
Galli, L., Orrico, A., Marcolongo, P., Fulceri, R., Burchell, A., Melis, D., et al. (1999). Mutations in the glucose-6-phosphate transporter (G6PT) gene in patients with glycogen storage diseases type 1b and 1c. FEBS Lett. 459, 255–258.
Ganley, I. G., Lam du, H., Wang, J., Ding, X., Chen, S., and Jiang, X. (2009). ULK1.ATG13.FIP200 complex mediates mTOR signaling and is essential for autophagy. J. Biol. Chem. 284, 12297–12305. doi: 10.1074/jbc.M900573200
Gerin, I., Noel, G., and Van Schaftingen, E. (2001). Novel arguments in favor of the substrate-transport model of glucose-6-phosphatase. Diabetes 50, 1531–1538. doi: 10.2337/diabetes.50.7.1531
Gerin, I., Veiga-da-Cunha, M., Achouri, Y., Collet, J. F., and Van Schaftingen, E. (1997). Sequence of a putative glucose-6-phosphate translocase, mutated in glycogen storage disease type 1b. FEBS Lett. 419, 235–238. doi: 10.1016/S0014-5793(97)01463-4
Gerin, I., Veiga-da-Cunha, M., Noël, G., and Van Schaftingen, E. (1999). Structure of the gene mutated in glycogen storage disease type Ib. Gene 227, 189–195. doi: 10.1016/S0378-1119(98)00614-3
Ghosh, A., Shieh, J. J., Pan, C. J., and Chou, J. Y. (2004). Histidine 167 is the phosphate acceptor in glucose-6-phosphatase-beta forming a phosphohistidine enzyme intermediate during catalysis. J. Biol. Chem. 279, 12479–12483. doi: 10.1074/jbc.M313271200
Ghosh, A., Shieh, J. J., Pan, C. J., Sun, M. S., and Chou, J. Y. (2002). The catalytic center of glucose-6-phosphatase. HIS176 is the nucleophile forming the phosphohistidine-enzyme intermediate during catalysis. J. Biol. Chem. 277, 32837–32842. doi: 10.1074/jbc.M201853200
Goldberg, T., and Slonim, A. E. (1993). Nutrition therapy for hepatic glycogen storage diseases. J. Am. Diet. Assoc. 93, 1423–1430. doi: 10.1016/0002-8223(93)92246-T
Greene, H. L., Slonim, A. E., O'Neill, J. A. Jr., and Burr, I. M. (1976). Continuous nocturnal intragastric feeding for management of type 1 glycogen-storage disease. N. Engl. J. Med. 294, 423–425. doi: 10.1056/NEJM197602192940805
Grefhorst, A., Schreurs, M., Oosterveer, M. H., Cortés, V. A., Havinga, R., Herling, A. W., et al. (2010). Carbohydrate response-element-binding protein (ChREBP) and not the liver X receptor α (LXRα) mediates elevated hepatic lipogenic gene expression in a mouse model of glycogen storage disease type 1. Biochem. J. 432, 249–254. doi: 10.1042/BJ20101225
Hawley, S. A., Pan, D. A., Mustard, K. J., Ross, L., Bain, J., Edelman, A. M., et al. (2005). Calmodulin-dependent protein kinase kinase beta is an alternative upstream kinase for AMP-activated protein kinase. Cell Metab. 2, 9–19. doi: 10.1016/j.cmet.2005.05.009
He, L., Vasiliou, K., and Nebert, D. W. (2009). Analysis and update of the human solute carrier (SLC) gene superfamily. Hum. Genomics 3, 195–206. doi: 10.1186/1479-7364-3-2-195
Hemmerle, H., Burger, H. J., Below, P., Schubert, G., Rippel, R., Schindler, P. W., et al. (1997). Chlorogenic acid and synthetic chlorogenic acid derivatives: novel inhibitors of hepatic glucose-6-phosphate translocase. J. Med. Chem. 40, 137–145. doi: 10.1021/jm9607360
Herling, A. W., Burger, H., Schubert, G., Hemmerle, H., Schaefer, H., and Kramer, W. (1999). Alterations of carbohydrate and lipid intermediary metabolism during inhibition of glucose-6-phosphatase in rats. Eur. J. Pharmacol. 386, 75–82. doi: 10.1016/S0014-2999(99)00748-7
Hiraiwa, H., and Chou, J. Y. (2001). Glucocorticoids activate transcription of the gene for glucose-6-phosphate transporter, deficient in glycogen storage disease type 1b. DNA Cell Biol. 20, 447–453. doi: 10.1089/104454901316976073
Hiraiwa, H., Pan, C. J., Lin, B., Moses, S. W., and Chou, J. Y. (1999). Inactivation of the glucose-6-phosphate transporter causes glycogen storage disease type 1b. J. Biol. Chem. 274, 5532–5536. doi: 10.1074/jbc.274.9.5532
Hochuli, M., Christ, E., Meienberg, F., Lehmann, R., Krützfeldt, J., and Baumgartner, M. R. (2015). Alternative nighttime nutrition regimens in glycogen storage disease type I: a controlled crossover study. J. Inherit. Metab. Dis. 38, 1093–1098. doi: 10.1007/s10545-015-9864-2
Hoffman, K., and Stoffel, W. (1993). TMbase—A database of membrane spanning protein segments. Biol. Chem. 347, 166–170.
Iacopetta, D., Carocci, A., Sinicropi, M. S., Catalano, A., Lentini, G., Ceramella, J., et al. (2017). Old drug scaffold, new activity: Thalidomide-correlated compounds exert different effects on breast cancer cell growth and progression. Chem. Med. Chem. 12, 381–389. doi: 10.1002/cmdc.201600629
Iacopetta, D., Lappano, R., Cappello, A. R., Madeo, M., De Francesco, E. M., Santoro, A., et al. (2010). SLC37A1 gene expression is up-regulated by epidermal growth factor in breast cancer cells. Breast Cancer Res. Treat. 122, 755–764. doi: 10.1007/s10549-009-0620-x
Ihara, K., Nomura, A., Hikino, S., Takada, H., and Hara, T. (2000). Quantitative analysis of glucose-6-phosphate translocase gene expression in various human tissues and haematopoietic progenitor cells. J. Inherit. Metab. Dis. 23, 583–592. doi: 10.1023/A:1005677912539
Island, M. D., Wei, B. Y., and Kadner, R. J. (1992). Structure and function of the uhp genes for the sugar phosphate transport system in Escherichia coli and Salmonella typhimurium. J. Bacteriol. 174, 2754–2762. doi: 10.1128/jb.174.9.2754-2762.1992
Janecke, A. R., Linder, M., Erdel, M., Mayatepek, E., Möslinger, D., Podskarbi, T., et al. (2000). Mutation analysis in glycogen storage disease type 1 non-a. Hum. Genet. 107, 285–289. doi: 10.1007/s004390000371
Jiang, P., and Mizushima, N. (2014). Autophagy and human diseases. Cell Res. 24, 69–79. doi: 10.1038/cr.2013.161
Jun, H. S., Lee, Y. M., McDermott, D. H., DeRavin, S. S., Murphy, P. M., Mansfield, B. C., et al. (2010). Lack of glucose recycling between endoplasmic reticulum and cytoplasm underlies cellular dysfunction in glucose-6-phosphatase-beta-deficient neutrophils in a congenital neutropenia syndrome. Blood 116, 2783–2792. doi: 10.1182/blood-2009-12-258491
Jun, H. S., Lee, Y. M., Song, K. D., Mansfield, B. C., and Chou, J. Y. (2011). G-CSF improves murine G6PC3-deficient neutrophil function by modulating apoptosis and energy homeostasis. Blood 117, 3881–3892. doi: 10.1182/blood-2010-08-302059
Jun, H. S., Weinstein, D. A., Lee, Y. M., Mansfield, B. C., and Chou, J. Y. (2014). Molecular mechanisms of neutrophil dysfunction in glycogen storage disease type Ib. Blood 123, 2843–2853. doi: 10.1182/blood-2013-05-502435
Jung, C. H., Jun, C. B., Ro, S. H., Kim, Y. M., Otto, N. M., Cao, J., et al. (2009). ULK-Atg13-FIP200 complexes mediate mTOR signaling to the autophagy machinery. Mol. Biol. Cell 20, 1992–2003. doi: 10.1091/mbc.E08-12-1249
Kikuchi, D., Saito, M., Saito, K., Watanabe, Y., Matsumoto, Y., Kanke, Y., et al. (2018). Upregulated solute carrier family 37 member 1 in colorectal cancer is associated with poor patient outcome and metastasis. Oncol. Lett. 15, 2065–2072. doi: 10.3892/ol.2017.7559
Kilpatrick, L., Garty, B. Z., Lundquist, K. F., Hunter, K., Stanley, C. A., Baker, L., et al. (1990). Impaired metabolic function and signaling defects in phagocytic cells in glycogen storage disease type 1b. J. Clin. Invest. 86, 196–202. doi: 10.1172/JCI114684
Kim, J. Y., Tillison, K., Zhou, S., Wu, Y., and Smas, C. M. (2007). The major facilitator superfamily member Slc37a2 is a novel macrophage-specific gene selectively expressed in obese white adipose tissue. Am. J. Physiol. Endoc. M. 293, E110–E120. doi: 10.1152/ajpendo.00404.2006
Kim, J., Kundu, M., Viollet, B., and Guan, K. L. (2011). AMPK and mTOR regulate autophagy through direct phosphorylation of Ulk1. Nat. Cell Biol. 13, 132–141. doi: 10.1038/ncb2152
Kim, S. Y., Jun, H. S., Mead, P. A., Mansfield, B. C., and Chou, J. Y. (2008). Neutrophil stress and apoptosis underlie myeloid dysfunction in glycogen storage disease type Ib. Blood 111, 5704–5711. doi: 10.1182/blood-2007-12-129114
King, C., Ilic, A., Koelsch, K., and Sarvetnick, N. (2004). Homeostatic expansion of T cells during immune insufficiency generates autoimmunity. Cell 117, 265–277. doi: 10.1016/S0092-8674(04)00335-6
Kishnani, P. S., Austin, S. L., Abdenur, J. E., Arn, P., Bali, D. S., Boney, A., et al. (2014). Diagnosis and management of glycogen storage disease type I: a practice guideline of the American College of Medical Genetics and Genomics. Genet. Med. 16:e1. doi: 10.1038/gim.2014.128
Knutson, T. P., Truong, T. H., Ma, S., Brady, N. J., Sullivan, M. E., Raj, G., et al. (2017). Posttranslationally modified progesterone receptors direct ligand-specific expression of breast cancer stem cell-associated gene programs. J. Hematol. Oncol. 10:89. doi: 10.1186/s13045-017-0462-7
Koeberl, D. D., Kishnani, P. S., Bali, D., and Chen, Y. T. (2009). Emerging therapies for glycogen storage disease type I. Trends Endocrinol. Metab. 20, 252–258. doi: 10.1016/j.tem.2009.02.003
Kouroku, Y., Fujita, E., Tanida, I., Ueno, T., Isoai, A., Kumagai, H., et al. (2007). ER stress (PERK/eIF2alpha phosphorylation) mediates the polyglutamine-induced LC3 conversion, an essential step for autophagy formation. Cell Death Differ. 14, 230–239. doi: 10.1038/sj.cdd.4401984
Krishnan, J., Suter, M., Windak, R., Krebs, T., Felley, A., Montessuit, C., et al. (2009). Activation of a HIF1α-PPARgamma axis underlies the integration of glycolytic and lipid anabolic pathways in pathologic cardiac hypertrophy. Cell Metab. 9, 512–524. doi: 10.1016/j.cmet.2009.05.005
Kuijpers, T. W., Maianski, N. A., Tool, A. T., Smit, G. P., Rake, J. P., Roos, D., et al. (2003). Apoptotic neutrophils in the circulation of patients with glycogen storage disease type 1b (GSD1b). Blood 101, 5021–5024. doi: 10.1182/blood-2002-10-3128
Kure, S., Hou, D. C., Suzuki, Y., Yamagishi, A., Hiratsuka, M., Fukuda, T., et al. (2000). Glycogen storage disease type Ib without neutropenia. J. Pediatr. 137, 253–256. doi: 10.1067/mpd.2000.107472
Kvandova, M., Majzúnová, M., and Dovinová, I. (2016). The role of PPARgamma in cardiovascular diseases. Physiol. Res. 65(Suppl. 3), S343–S363.
Kwon, J. H., Lee, Y. M., Cho, J. H., Kim, G. Y., Anduaga, J., Starost, M. F., et al. (2017). Liver-directed gene therapy for murine glycogen storage disease type Ib. Hum. Mol. Genet. 26, 4395–4405. doi: 10.1093/hmg/ddx325
Labrune, P. (2002). Glycogen storage disease type I: indications for liver and/or kidney transplantation. Eur. J. Pediatr. 161(Suppl. 1), S53–S55. doi: 10.1007/BF02679995
Lappano, R., Sebastiani, A., Cirillo, F., Rigiracciolo, D. C., Galli, G. R., Curcio, R., et al. (2017). The lauric acid-activated signaling prompts apoptosis in cancer cells. Cell Death Discov. 3:17063. doi: 10.1038/cddiscovery.2017.63. eCollection 2017
Lee, H., Noh, J. Y., Oh, Y., Kim, Y., Chang, J. W., Chung, C. W., et al. (2012). IRE1 plays an essential role in ER stress-mediated aggregation of mutant huntingtin via the inhibition of autophagy flux. Hum. Mol. Genet. 21, 101–114. doi: 10.1093/hmg/ddr445
Lee, Y. M., Jun, H. S., Pan, C. J., Lin, S. R., Wilson, L. H., Mansfield, B. C., et al. (2012). Prevention of hepatocellular adenoma and correction of metabolic abnormalities in murine glycogen storage disease type Ia by gene therapy. Hepatology 56, 1719–1729. doi: 10.1002/hep.25717
Lei, K. J., Chen, H., Pan, C. J., Ward, J. M., Mosinger, B., Lee, E. J., et al. (1996). Glucose-6-phosphatase dependent substrate transport in the glycogen storage disease type 1a mouse. Nat. Genet. 13, 203–209. doi: 10.1038/ng0696-203
Lei, K. J., Shelly, L. L., Lin, B., Sidbury, J. B., Chen, Y. T., Nordlie, R. C., et al. (1995). Mutations in the glucose-6-phosphatase gene are associated with glycogen storage disease types 1a and 1aSP but not 1b and 1c. J. Clin. Invest. 95, 234–240. doi: 10.1172/JCI117645
Letkemann, R., Wittkowski, H., Antonopoulos, A., Podskabi, T., Haslamc, S. M., Föll, D., et al. (2017). Partial correction of neutrophil dysfunction by oral galactose therapy in glycogen storage disease type Ib. Int. Immunopharmacol. 44, 216–225. doi: 10.1016/j.intimp.2017.01.020
Leuzzi, R., Bánhegyi, G., Kardon, T., Marcolongo, P., Capecchi, P. L., Burger, H. J., et al. (2003). Inhibition of microsomal glucose-6-phosphate transport in human neutrophils results in apoptosis: a potential explanation for neutrophil dysfunction in glycogen storage disease type 1b. Blood 101, 2381–2887. doi: 10.1182/blood-2002-08-2576
Lin, B., Annabi, B., Hiraiwa, H., Pan, C. J., and Chou, J. Y. (1998). Cloning and characterization of cDNAs encoding a candidate glycogen storage disease type 1b protein in rodents. J. Biol. Chem. 273, 31656–31670. doi: 10.1074/jbc.273.48.31656
Lin, B., Pan, C. J., and Chou, J. Y. (2000). Human variant glucose-6-phosphate transporter is active in microsomal transport. Hum. Genet. 107, 526–552. doi: 10.1007/s004390000404
Lloyd, A. D., and Kadner, R. J. (1990). Topology of the Escherichia coli UhpT sugar-phosphate transporter analyzed by using TnphoA fusions. J. Bacteriol. 172, 1688–1693. doi: 10.1128/jb.172.4.1688-1693.1990
Maloney, P. C., and Wilson, T. H. (1996) Escherichia coli Salmonella: Cellular Molecular Biology. Washington, DC: ASM Press. 1130–1148.
Marcolongo, P., Barone, V., Priori, G., Pirola, B., Giglio, S., Biasucci, G., et al. (1998). Structure and mutation analysis of the glycogen storage disease type 1b gene. FEBS Lett. 436, 247–250. doi: 10.1016/S0014-5793(98)01129-6
Martens, D. H., Kuijpers, T. W., Maianski, N. A., Rake, J. P., Smit, G. P., and Visser, G. (2006). A patient with common glycogen storage disease type Ib mutations without neutropenia or neutrophil dysfunction. J. Inherit. Metab. Dis. 29, 224–225. doi: 10.1007/s10545-006-0146-x
Matern, D., Seydewitz, H. H., Bali, D., Lang, C., and Chen, Y. T. (2002). Glycogen storage disease type I: diagnosis and phenotype/genotype correlation. Eur. J. Pediatr. 161, S10–S19. doi: 10.1007/s00431-002-0998-5
McCawley, L. J., Korchak, H. M., Cutilli, J. R., Stanley, C. A., Baker, L., Douglas, S. D., et al. (1993). Interferon-gamma corrects the respiratory burst defect in vitro in monocyte-derived macrophages from glycogen storage disease type 1b patients. Pediatr. Res. 34, 265–269. doi: 10.1203/00006450-199309000-00005
McDermott, D. H., De Ravin, S. S., Jun, H. S., Liu, Q., Priel, D. A., Noel, P., et al. (2010). Severe congenital neutropenia resulting from G6PC3 deficiency with increased neutrophil CXCR4 expression and myelokathexis. Blood 116, 2793–2802. doi: 10.1182/blood-2010-01-265942
McGovern, N. N., Cowburn, A. S., Porter, L., Walmsley, S. R., Summers, C., Thompson, A. A. R., et al. (2011). Hypoxia selectively inhibits respiratory burst activity and killing of Staphylococcus aureus in human neutrophils. J. Immunol. 186, 453–463. doi: 10.4049/jimmunol.1002213
Mehta, H. M., Malandra, M., and Corey, S. J. (2015). G-CSF and GM-CSF in Neutropenia. J. Immunol. 195, 1341–1349. doi: 10.4049/jimmunol.1500861
Melis, D., Balivo, R., Della Casa, R., Romano, A., Taurisano, R., Capaldo, B., et al. (2008). Myasthenia gravis in a patient affected by glycogen storage disease type Ib: a further manifestation of an increased risk for autoimmune disorders? J. Inherit. Metab. Dis. 31 (Suppl. 2), S227–S231. doi: 10.1007/s10545-008-0810-4
Melis, D., Carbone, F., Minopoli, G., La Rocca, C., Perna, F., De Rosa, V., et al. (2017). Cutting edge: increased autoimmunity risk in glycogen storage disease type 1b is associated with a reduced engagement of glycolysis in T Cells and an impaired regulatory T cell function. J. Immunol. 198, 3803–3808. doi: 10.4049/jimmunol.1601946
Melis, D., Fulceri, R., Parenti, G., Marcolongo, P., Gatti, R., Parini, R., et al. (2005). Genotype/phenotype correlation in glycogen storage disease type 1b: a multicentre study and review of the literature. Eur. J. Pediatr. 164, 501–508. doi: 10.1007/s00431-005-1657-4
Melis, D., Parenti, G., Della Casa, R., Sibilio, M., Berni Canani, R., Terrin, G., et al. (2003). Crohn's-like ileo-colitis in patients affected by glycogen storage disease Ib: two years_ follow-up of patients with a wide spectrum of gastrointestinal signs. Acta Paediatr. 92, 1415–1421. doi: 10.1111/j.1651-2227.2003.tb00825.x
Melis, D., Pivonello, R., Parenti, G., Della Casa, R., Salerno, M., Lombardi, G., et al. (2007). Increased prevalance of thyroid autoimmunity and hypothyroidism in patients with glycogen storage disease type 1. J. Pediatr. 150, 300–305. doi: 10.1016/j.jpeds.2006.11.056
Merayo-Chalico, J., Rajme-Lopez, S., Barrera-Vargas, A., Alcocer-Varela, J., Diaz-Zamudio, M., and Gomez-Martìn, D. (2016). Lymphopenia and autoimmunity: a double-edged sword. Hum. Immunol. 77, 921–929. doi: 10.1016/j.humimm.2016.06.016
Mihaylova, M. M., and Shaw, R. J. (2011). The AMPK signalling pathway coordinates cell growth, autophagy and metabolism. Nat. Cell Biol. 13, 1016–1023. doi: 10.1038/ncb2329
Mortellaro, C., Garagiola, U., Carbone, V., Cerutti, F., Marci, V., and Bonda, P. L. (2005). Unusual oral manifestations and evolution in glycogen storage disease type Ib. J. Craniofac. Surg.16, 45–52. doi: 10.1097/00001665-200501000-00010
Nazio, F., Strappazzon, F., Antonioli, M., Bielli, P., Cianfanelli, V., Bordi, M., et al. (2013). MTOR inhibits autophagy by controlling ULK1 ubiquitylation, self-association and function through AMBRA1 and TRAF6. Nat. Cell Biol. 15, 406–416. doi: 10.1038/ncb2708
Nordlie, R. C., Sukalski, K. A., Munoz, J. M., and Baldwin, J. J. (1983). Type 1c, a novel glycogenosis. J. Biol. Chem. 258, 9739–9744.
Pan, C. J., Chen, S. Y., Jun, H. S., Lin, S. R., Mansfield, B. C., and Chou, J. Y. (2011). SLC37A1 and SLC37A2 are phosphate-linked, glucose-6-phosphate antiporters. PLoS ONE 6:e23157. doi: 10.1371/journal.pone.0023157
Pan, C. J., Chen, S. Y., Lee, S., and Chou, J. Y. (2009). Structure-function study of glucose-6-phosphate transporter, an eukaryotic antiporter deficient in glycogen storage disease type Ib. Mol. Genet. Metab. 96, 32–37. doi: 10.1016/j.ymgme.2008.10.005
Pan, C. J., Lei, K. J., Annabi, B., Hemrika, W., and Chou, J. Y. (1998). Transmembrane topology of glucose-6-phosphatase. J. Biol. Chem. 273, 6144–6148. doi: 10.1074/jbc.273.11.6144
Pan, C. J., Lin, B., and Chou, J. Y. (1999). Transmembrane topology of human glucose-6-phosphate transporter. J. Biol. Chem. 274, 13865–13869. doi: 10.1074/jbc.274.20.13865
Pao, S. S., Paulsen, I. T., and Saier, M. H. Jr. (1998). Major facilitator superfamily. Microbiol. Mol. Biol. Rev. 62, 1–34.
Perland, E., and Fredriksson, R. (2017). Classification systems of secondary active transporters. Trends Pharmacol. Sci. 38, 305–315. doi: 10.1016/j.tips.2016.11.008
Pessin, J. E., and Bell, G. I. (1992). Mammalian facilitative glucose transporter family: structure and molecular regulation. Annu. Rev. Physiol. 54, 911–930. doi: 10.1146/annurev.ph.54.030192.004403
Pierre, G., Chakupurakal, G., McKiernan, P., Hendriksz, C., Lawson, S., and Chakrapani, A. (2008). Bone marrow transplantation in glycogen storage disease type 1b. J. Pediatr. 152, 286–288. doi: 10.1016/j.jpeds.2007.09.031
Proverbio, M. C., Mangano, E., Gessi, A., Bordoni, R., Spinelli, R., Asselta, R., et al. (2013). Whole genome SNP genotyping and exome sequencing reveal novel genetic variants and putative causative genes in congenital hyperinsulinism. PLoS ONE 8:e68740. doi: 10.1371/journal.pone.0068740
Rake, J. P., Visser, G., Labrune, P., Leonard, J. V., Ullrich, K., and Smit, G. P. (2002). Glycogen storage disease type I: diagnosis, management, clinical course and outcome. Results of the European Study on Glycogen Storage Disease Type I (ESGSDI). Eur. J. Pediatr. 161, S20–S34. doi: 10.1007/BF02679990
Reddy, R. C., Narala, V. R., Keshamouni, V. G., Milam, J. E., Newstead, M. W., and Standiford, T. J. (2008). Sepsis-induced inhibition of neutrophil chemotaxis is mediated by activation of peroxisome proliferator-activated receptor-{gamma}. Blood 112, 4250–4258. doi: 10.1182/blood-2007-12-128967
Reddy, S. K., Austin, S. L., Spencer-Manzon, M., Koeberl, D. D., Clary, B. M., Desai, D. M., et al. (2009). Liver transplantation for glycogen storage disease type Ia. J. Hepatol. 51, 483–490. doi: 10.1016/j.jhep.2009.05.026
Reinartz, S., and Dist, O. (2016). Validation of deleterious mutations in vorderwald cattle. PLoS ONE 11:e0160013. doi: 10.1371/journal.pone.0160013
Rihwa, C., Hyung-Doo, P., Jung Min, K., Jeongho, L., Dong Hwan, L., Suk Jin, H., et al. (2017). Novel SLC37A4 mutations in korean patients with glycogen storage disease Ib. Ann. Lab. Med. 37, 261–266. doi: 10.3343/alm.2017.37.3.261
Rueda, C. M., Jackson, C. M., and Chougnet, C. A. (2016). Regulatory T-Cell-mediated suppression of conventional T-Cells and dendritic cells by different cAMP intracellular pathways. Front. Immunol. 7:216. doi: 10.3389/fimmu.2016.00216
Saksa, N., Neme, A., Ryynänen, J., Uusitupa, M., de Mello, V. D., Voutilainen, S., et al. (2015). Dissecting high from lowresponders in a vitamin D3 intervention study. J. Steroid Biochem. Mol. Biol. 148, 275–282. doi: 10.1016/j.jsbmb.2014.11.012
Sancak, Y., Bar-Peled, L., Zoncu, R., Markhard, A. L., Nada, S., and Sabatini, D. M. (2010). Regulator-Rag complex targets mTORC1 to the lysosomal surface and is necessary for its activation by amino acids. Cell 141, 290–303. doi: 10.1016/j.cell.2010.02.024
Shah, K. K., and O'Dell, S. D. (2013). Effect of dietary interventions in the maintenance of normoglycaemia in glycogen storage disease type 1a: a systematic review and meta-analysis. J. Hum. Nutr. Diet. 26, 329–339. doi: 10.1111/jhn.12030
Shieh, J. J., Chen, C. T., and Huang, W. R. (2007). The variant glucose-6-phosphate transporter decreases protein stability and requires MyoD-dependent alternative splicing during myogenesis of muscle cells. FASEB J. 21:A243.
Shieh, J. J., Pan, C. J., Mansfield, B. C., and Chou, J. Y. (2003). Glucose-6-phosphate hydrolase, widely expressed outside the liver, can explain age-dependent resolution of hypoglycemia in glycogen storage disease type Ia. J. Biol. Chem. 278, 47098–47103. doi: 10.1074/jbc.M309472200
Sidbury, J. B., Chen, Y. T., and Roe, C. R. (1986). The role of raw starches in the treatment of type I glycogenosis. Arch. Intern. Med. 146:370–373. doi: 10.1001/archinte.1986.00360140200029
Takahashi, Y., Miyata, M., Zheng, P., Imazato, T., Horwitz, A., and Smith, J. D. (2000). Identification of cAMP analogue inducible genes in RAW264 macrophages. Biochim. Biophys. Acta 1492, 385–394. doi: 10.1016/S0167-4781(00)00133-0
Veiga-da-Cunha, M., Gerin, I., and Van Schaftingen, E. (2000). How many forms of glycogen storage disease type I? Eur. J. Pediatr. 159, 314–318. doi: 10.1007/s004310051279
Veiga-da-Cunha, M., Gerin, I., Chen, Y.-T., de Barsy, T., de Lonlay, P., Dionisi-Vici, C., et al. (1998). A gene on chromosome 11q23 coding for a putative glucose-6-phosphate translocase is mutated in glycogenstorage disease types Ib and Ic. Am. J. Hum. Genet. 63, 976–983. doi: 10.1086/302068
Veiga-da-Cunha, M., Gerin, I., Chen, Y. T., Lee, P. J., Leonard, J. V., Maire, I., et al. (1999). The putative glucose 6-phosphate translocase gene is mutated in essentially all cases of glycogen storage disease type I non-a. Eur. J. Hum. Genet. 7, 717–723. doi: 10.1038/sj.ejhg.5200366
Visser, G., Rake, J. P., Fernandes, J., Labrune, P., Leonard, J. V., Moses, S., et al. (2000). Neutropenia, neutrophil dysfunction, and inflammatory bowel disease in glycogen storage disease type Ib: results of the European Study on Glycogen storage disease type I. J. Pediatr. 137, 187–191. doi: 10.1067/mpd.2000.105232
Visser, G., Rake, J. P., Labrune, P., Leonard, J. V., Moses, S., Ullrich, K., et al. (2002). Granulocyte colony-stimulating factor in glycogen storage disease type 1b. results of the European Study on Glycogen Storage Disease Type 1. Eur. J. Pediatr. 161, S83–S87. doi: 10.1007/BF02680001
Weinstein, D. A., and Wolfsdorf, J. I. (2002). Effect of continuous glucose therapywith uncooked cornstarch on the long-term clinical course of type 1a glycogen storage disease. Eur. J. Pediatr. 161(Suppl. 1), S35–S39. doi: 10.1007/BF02679991
Wilfinger, J., Seuter, S., Tuomainen, T. P., Virtanen, J. K., Voutilainen, S., Nurmi, T., et al. (2014). Primary vitamin D receptor target genes as biomarkers for the vitamin D3 status in the hematopoietic system. J. Nutr. Biochem. 25, 875–884. doi: 10.1016/j.jnutbio.2014.04.002
Wolfsdorf, J. I., and Crigler, J. F. Jr. (1997). Cornstarch regimens for nocturnal treatment of young adults with type I glycogen storage disease. Am. J. Clin. Nutr. 65, 1507–1511 doi: 10.1093/ajcn/65.5.1507
Wolfsdorf, J. I., and Weinstein, D. A. (2003). Glycogen storage diseases. Rev. Endocr. Metab. Disord. 4, 95–102. doi: 10.1023/A:1021831621210
Yiu, W. H., Lee, Y. M., Peng, W. T., Pan, C. J., Mead, P. A., Mansfield, B. C., et al. (2010). Complete normalization of hepatic G6PC deficiency in murine glycogen storage disease type Ia using gene therapy. Mol. Ther. 18, 1076–1084. doi: 10.1038/mt.2010.64
Yiu, W. H., Pan, C. J., Allamarvdasht, A., Kim, S. Y., and Chou, J. Y. (2007). Glucose-6-phosphate transporter gene therapy corrects metabolic and myeloid abnormalities in glycogen storage disease type Ib mice. Gene Ther.14, 219–226. doi: 10.1038/sj.gt.3302869
Yiu, W. H., Pan, C. J., Mead, P. A., Starost, M. F., Mansfield, B. C., and Chou, J. Y. (2009). Normoglycemia alone is insufficient to prevent long term complications of hepatocellular adenoma in glycogen storage disease type Ib mice. J. Hepatol. 51, 909–917. doi: 10.1016/j.jhep.2008.11.026
Young, A. R., Chan, E. Y., Hu, X. W., Kochl, R., Crawshaw, S. G., High, S., et al. (2006). Starvation and ULK1-dependent cycling of mammalian Atg9 between the TGN and endosomes. J. Cell Sci. 119, 3888–3900. doi: 10.1242/jcs.03172
Zingone, A., Hiraiwa, H., Pan, C.-J., Lin, B., Chen, H., Ward, J. M., et al. (2000). Correction of glycogen storage disease type 1a in a mouse model by gene therapy. J. Biol. Chem. 275, 828–832. doi: 10.1074/jbc.275.2.828
Keywords: SLC37A1-4, endoplasmic reticulum, glucose-6-phosphate translocase, G6PT deficiency, glycogen storage disease type Ib
Citation: Cappello AR, Curcio R, Lappano R, Maggiolini M and Dolce V (2018) The Physiopathological Role of the Exchangers Belonging to the SLC37 Family. Front. Chem. 6:122. doi: 10.3389/fchem.2018.00122
Received: 27 January 2018; Accepted: 30 March 2018;
Published: 17 April 2018.
Edited by:
Graça Soveral, Universidade de Lisboa, PortugalReviewed by:
Antimo Migliaccio, Università degli Studi della Campania L. Vanvitelli Naples, ItalyAn-Ping Chen, Mayo Clinic, United States
Ilka Haferkamp, Technische Universität Kaiserslautern, Germany
Copyright © 2018 Cappello, Curcio, Lappano, Maggiolini and Dolce. This is an open-access article distributed under the terms of the Creative Commons Attribution License (CC BY). The use, distribution or reproduction in other forums is permitted, provided the original author(s) and the copyright owner are credited and that the original publication in this journal is cited, in accordance with accepted academic practice. No use, distribution or reproduction is permitted which does not comply with these terms.
*Correspondence: Marcello Maggiolini, bWFyY2VsbG9tYWdnaW9saW5pQHlhaG9vLml0; bWFyY2VsbG8ubWFnZ2lvbGluaUB1bmljYWwuaXQ=
†These authors have contributed equally to this work.