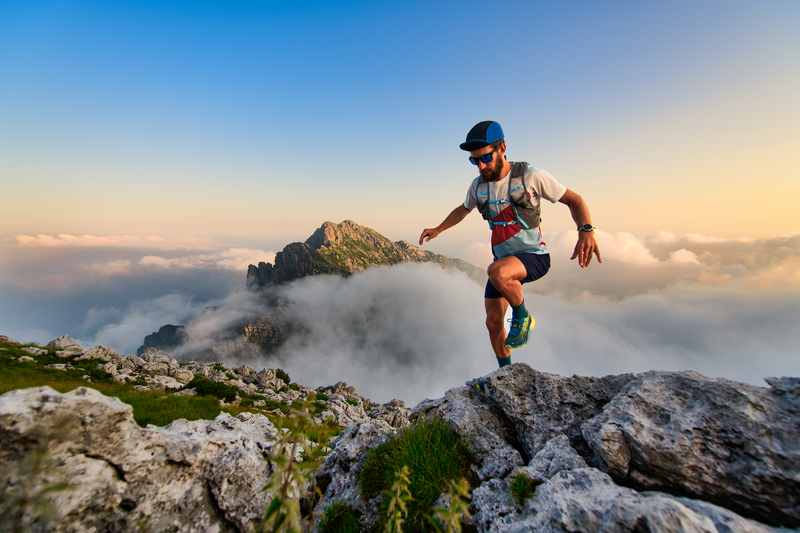
95% of researchers rate our articles as excellent or good
Learn more about the work of our research integrity team to safeguard the quality of each article we publish.
Find out more
REVIEW article
Front. Chem. , 03 April 2018
Sec. Supramolecular Chemistry
Volume 6 - 2018 | https://doi.org/10.3389/fchem.2018.00084
This article is part of the Research Topic Supramolecular Aspects in Catalysis View all 5 articles
In the last decades many efforts have been devoted to design supramolecular organocatalysts able to work in water as the reaction medium. The use of water as solvent provides promising benefits with respect to environmental impact. In this context, macrocyclic compounds played a role of primary importance thanks to their ease of synthesis and their molecular recognition abilities toward the reactants. The aim of this review is to give an overview of the recent advances in the field of supramolecular organocatalysis in water, focusing the attention on calixarene and cyclodextrins derivatives. Calixarenes and cyclodextrins, thanks to their hydrophobic cavities, are able to host selectively the substrates isolating they from the reaction environment. In addition, the synthetic versatilities of these macrocycles permits to introduce useful functional groups in close proximity of the hydrophobic binding sites. Regarding the cyclodextrins (CDs), we have here reviewed the their most recent uses as organocatalysts for the synthesis of heterocyclic compounds, in multi-component reactions and in carbon-carbon bond forming reactions. Examples have been reported in which CD catalysts are able to drive the regiochemistry of common organic reactions. In addition, cyclodextrins bearing catalytically active chiral groups, have shown excellent enantioselectivity in the catalysis of organic reactions. Recently reported results have shown that calixarene derivatives are able to accelerate organic reaction under “on-water” conditions with a significant selectivity toward the reactants. Under “on-water conditions” the hydrophobic effect, induced by insoluble calixarene derivatives, forces the reactants and the catalyst to aggregate and thus accelerating the reaction between them thanks to an amplification of weak secondary interactions. Regarding the use of water-soluble calixarene organocatalysts, we have here reviewed their role in the acceleration of common organic reactions.
In the last decades, a growing interest has been devoted to the development of new synthetic methodologies with the aim to emulate the high performance of biological processes (Dong et al., 2012; Raynal et al., 2014; Borsato and Scarso, 2016; Deraedt and Astruc, 2016; Kuah et al., 2016). In natural biosynthetic processes, the enzymatic machines work in water where they reach amazing levels of efficiency, selectivity, and specificity (Copeland, 2002; Bugg, 2012). Taking inspiration from the biological systems, several scientists have devoted many efforts to the design of artificial systems with the aim of mimicking the levels of performance of natural enzymes. At this regards, a particular emphasis has been placed to the study of organic reactions in water as the medium (Kobayashi and Li, 2012). Water, as a solvent, possesses many desirable characteristics for a reaction medium, because it is not only environmentally benign, but with its high heat capacity, high polarity, large cohesive energy, and hydrogen bonding abilities may have a significant impact on the reaction course influencing reaction rate and selectivity. In a pioneering work, Breslow highlighted the attractiveness of water as reaction medium and as reaction promoter of a Diels-Alder cycloaddition (Rideout and Breslow, 1980; Breslow, 1991; Simon and Li, 2012). Successively, Sharpless introduced for the first time the expression “on-water conditions” to indicate the rate acceleration observed in organic reactions when insoluble reactants are vigorously stirred in a water suspension (Narayan et al., 2005; Pirrung, 2006). As shown by Schreiner (Kleiner and Schreiner, 2006) and Rueping (Rueping and Theissmann, 2010), the polar nature and the hydrogen bonding abilities of water do not limit its use as a solvent in organocatalyzed reactions where the substrates are activated by covalent or secondary weak-interactions (Sakthivel et al., 2001; Pirrung and Sarma, 2004; Pirrung, 2006; Raj and Singh, 2009; Butler and Coyne, 2010; Mase and Barbas, 2010; Giacalone and Gruttadauria, 2013; De Rosa et al., 2016, 2017b; Jimeno, 2016). On the other hand, in fact, the natural enzymes stay and work in aqueous environment, where the enzyme-substrate complexes survive to the presence of water. The knowledge of the mechanisms underlying enzymatic catalysis has been an important source of inspiration for the design of supramolecular biomimetic organocatalysts (Steed and Atwood, 2009; Kirby and Hollfelder, 2010; Marchetti and Levine, 2011; Raynal et al., 2014). Thus, in the last years different types of supramolecular organocatalysts have been designed which have shown excellent catalytic efficiency and amazing regio-and stereoselectivity (Zhang and Tiefenbacher, 2015; Zhang et al., 2017; La Manna et al., 2018). The supramolecular organocatalysts so far known are constituted by macrocyclic scaffolds in which an internal cavity binds the substrate through secondary interactions with functional groups present in close proximity of the cavity. Inspired by the natural systems, in the macrocyclic-based supramolecular organocatalysts the internal cavity is able to accommodate the reactants isolating them from the bulk medium and provides a confined reaction environment. In this way, an enhancement of the local concentration of the reactants is obtained, thus promoting the reaction by proximity effects. In addition, the substrate selectivity is dictated by the size and shape of the internal cavity. More recently, many efforts have also been devoted to the design of self-assembled nanocapsules able to host different guests and to promote reactions inside their cavities. (Dong et al., 2011, 2012; Longstreet and McQuade, 2013; La Sorella et al., 2015; Deraedt and Astruc, 2016; Kuah et al., 2016).
In this review, we highlight some recent examples of macrocyclic-based supramolecular organocatalysts (Figure 1) able to catalyze organic reactions using water as a reaction medium. In details, we will focus our attention on examples concerning the use of calixarene and cyclodextrin macrocycles as scaffolds for supramolecular catalysts. Calixarenes and cyclodextrins are among the most popular macrocycles in supramolecular chemistry where they have shown amazing abilities in different fields. Calixarene and cyclodextrin macrocycles possess many desirable features for the design of supramolecular catalysts: (a) a hydrophobic internal cavity able to host the substrates in a selective way; (b) an excellent synthetic versatility which permits the introduction of functional groups in close proximity of their cavities. Thanks to these characteristics, calixarene, and cyclodextrin derivatives have found many applications in the field of supramolecular organocatalysis and we will highlight the most recent ones in this review.
Figure 1. Chemical structure of macrocyclic cavity-containing scaffolds: (A) cyclodextrins (CD); (B) calix[n]arenes.
In Tables 1, 2 the most recent catalytic performances of cyclodextrins and calixarenes evaluated in this work are summarized.
Cyclodextrin macrocycles (CD) are a family of naturally occurring molecules formed by α-1,4-linked D-glucose units associated head-to-tail to form a truncated conical structure. They have a hydrophobic inner core, made by glycosidic oxygen atoms and hydrocarbon CH-groups, which is surrounded by an outer hydrophilic surface formed by polar hydroxyl groups. The presence of hydroxyl groups ensures not only their water solubility but also the possibility of a direct interactions with substrates or the introduction of other functional groups. The number of the glucopyranoside units in the macrocycle modulates the cavity size being the most common cyclodextrins composed of 6 (α-CD), 7 (β-CD), and 8 (γ-CD) units (Figure 1).
The first evidences for the potential use of CDs in catalysis as enzyme models can be traced back to Bender's studies in the hydrolysis of phenyl acetate in the presence of CD where a remarkable substrate specificity was found (Van Etten et al., 1967a,b). Since then, a large number of works have been reported regarding the role of native and modified CDs in different types of reactions (Macaev and Boldescu, 2015; Srivastava et al., 2016; Bai et al., 2017). Their catalysis may be classified generally in two types: (a) covalent when an CD -reactant intermediate is formed through a covalent bond which then evolves in products, (b) non-covalent when the interactions between CD and reactants are non-covalent and CD hydrophobic cavity offers a microenvironment where the reaction takes place (Breslow and Dong, 1998; Takahashi, 1998; Komiyama and Monflier, 2006). Interestingly, complex of the CDs with transition metal have been used as water-soluble organometallic catalysts in several reactions, in addition CDs based material have been used as unconventional reaction media such as supramolecular hydrogels or low melting mixtures (LMMs) (Hapiot et al., 2014, 2017). Furthermore, when metals are not involved in the CD catalytic activity, the catalysis falls in the field of the supramolecular organocatalysis (Bogliotti and Dalko, 2007; Marinescu and Bols, 2010), and interesting works have been reported in recent years.
In 2011, Kumar et al. reported an efficient and eco-friendly procedure for the synthesis of tryptanthrin analogs 4 mediated by non-modified β-CD (Kumar et al., 2011). Tryptanthrin analogs are an interesting class of heterocycle compounds characterized by a broad spectrum of biologic activities against different pathogens such as antibacterial, antifungal, antitubercular activities and, furthermore a potential anticancer agent against some human cancer cell-lines (Dzierzbicka et al., 2003; Martinez-Viturro and Dominguez, 2007; Kshirsagar, 2015). The reaction did not proceed without the assistance of CD and, between the most common cyclodextrins, only β-CD proved to be the most efficient catalyst. These results pointed out the dual role played by the CD not only as catalyst but also as nanoreactor; the β-CD had the right cavity size to accommodate both reactants and thus induce the reactive events. The mechanistic hypothesis for the reaction proposed the imprisonment of the reactants in the CD cavity, the activation of carbonyl group at C-4 position of isatoic anhydride 1 through hydrogen bonding interaction with the hydroxyl groups of the CD, attack of the isatin 2 leading to cleavage of anhydride ring and formation of an intermediate 3 which, in turn, transforms into the final product 4. (Figure 2) 1H-NMR studies confirmed the formation of an inclusion complex between isatoic anhydride 1 and β-CD. The substrate scope proved general with different substituted isatins as well as substituted isatoic anhydrides affording the product in good to high yields not in a long time (5–9 h).
In 2012, Ramesh and coworkers reported an interesting example of a one-pot protocol mediated by CDs in water. (Figure 3) For the first time a cyclodextrin promoted via three-component reaction the synthesis of 2,3-dihydroquinazolin-4(1H)-one derivatives 8 and 9, molecules exhibiting several biological and pharmacological activities. The reaction between aniline 7, aldehyde 6 and isatoic anhydride 5 afforded the products in good yields and in short reaction times with only 10%mol β-CD. The recovery and reuse of the CD with little loss of efficiency, simple work-up and the use of water as reaction medium made it a powerful alternative method. (Ramesh et al., 2012).
In 2014, Kumar and Shukla used cyclodextrins as catalysts to perform a C(sp3)-H functionalization of 2-alkyl-azaarenes 10 with a series of homocyclic and heterocyclic diones 11 in water. (Figure 4) The reaction was interesting because it was a simple, green, and valid route to form carbon-carbon and carbon-heteroatom bonds, generally formed in the presence of transition metals. Different CDs were evaluated, β-CD turned out to be the best catalyst affording the product 12 in high yield in only 4 h at 80°C. The catalytic role of CD was pointed out by the absence of reactivity without CD. Studies by 1H-NMR spectra underlined up field shift of β-CD protons when mixed with the reactants thus confirming the formation of inclusion complexes. Under the optimal reaction conditions, the reaction was general for various isatins and 2-methylquinoline, different heterocyclic and homocyclic diones, regardless of the substitution pattern. Interestingly, the catalyst was recyclable up to 5 times without significant losses of efficiency (Kumar and Shukla, 2014).
Interesting and promising application of CD was proposed by Kumar et al. for the deprotection of hydroxyl groups. (Figure 5) They reported that THP/MOM/Ac/Ts ethers 13 could be deprotected under mild and eco-friendly conditions in the presence of β-CD in water. Particularly, the combined use of water and microwave irradiations greatly reduced the reaction times to minutes without loss of efficiency (Figure 5A). It is noteworthy that, under the reaction conditions, they observed not only deprotection of hydroxyl group but also a concomitant regioselective cyclization of chalcone epoxides 17 to 2-hydroxyindanones 18 and 2′-aminochalcones to aza-flavanones, respectively. Different evidence was recorded for the chalcones, the procedure stopped at the deprotection without evidence of cyclization. With 2′-aminochalcones the reaction efficiency depended on the nature of substituents. If the substituent on Ar2 ring of aminochalcones was electron-donating group, the cyclization was favored giving the product in higher yield, while an electron-withdrawing group slowed the cyclization and so decreased the reaction efficiency. In the reaction mechanism proposed by the authors, the CD played a dual role: (a) activation of the THP ether and epoxide by H-bond interactions and, at the same time (b) formation of inclusion complex controlled the regioselective ring opening of epoxide at β-carbon. (Kumar et al., 2015). Later, the authors extended this procedure (Kumar and Ahmed, 2016). The reaction of deprotection was carried out using β-CD and o-iodoxybenzoic acid (IBX) in water. Under these conditions a one-pot deprotection with oxidative cleavage of chalcone epoxides 23 and oxidative dehydrogenation of alcohols 21 was observed. The reaction afforded β-hydroxy-1, 2-diketones 24, α,β-unsaturated ketones 22 and 1,2,3-triketones 25 in moderate to high yields requiring low reaction time. Once again, the dual role played by β-CD was evident. In the THP ether deprotection and concurrent oxidative cleavage of chalcone epoxide, β-CD not only activated the ether and epoxide through hydrogen bonding interactions, but also controlled the regioselective opening of epoxide forming inclusion complex (Figure 5).
Figure 5. β-CD mediated deprotection of hydroxyl groups in water: (A) deprotection reaction and one-pot deprotection with subsequent cyclization of chalcone epoxides; (B) one-pot deprotection and concurrent oxidation reaction.
One-pot four-component procedure promoted by CD for the preparation of polysubstituted pyrroles 30 was reported for the first time by Konkala et al. (2016) The synthesis of substituted pyrroles is complicated mainly by the occurrence of undesired polymerization reactions or lack selectivity, this protocol provided efficient and selective. The poor results obtained by using sodium dodecyl sulfate (SDS) or PEG-400, pointed out that CD did not act as a transfer phase catalyst and so the high reaction performance in its presence could be attributed to the formation of inclusion complexes within its hydrophobic cavity thereby facilitating the sequence of the reaction. NMR studies confirmed the incorporation of the aldehyde 26 and the formation of β-CD-aldehyde inclusion complex. Once activated, the aldehyde reacted with nitromethane 29 to form nitrostyrene which, in turn, reacted with in situ generated β-ketoenamine and, a subsequent oxidative aromatization led to the final product. (Figure 6) The scope of the reaction is broad with respect to the nature of the aldehyde and amine affording many substituted pyrroles in good to high yields.
Figure 6. One-pot four-component procedure for the synthesis of substituted pyrroles in water promoted by β-CD.
Recently, Floresta et al. employed γ-cyclodextrin as a catalyst in the 1, 3-dipolar cycloaddition between different nitrones 31 and styrenes or cynnamates 32 to give substituted isoxazolidines 33 and 34 in high yields and good to high diastereomeric excesses. The role of CD was ascribed to the formation of host-guest complexes between CD and the reactants mediated by noncovalent interactions (Figure 7). When the reactants were included together into the hydrophobic CD cavity, the catalytic event took place. In fact, the reaction did not proceed with α- and β-CD, their cavity size were too small to simultaneously accommodate both reactants. Evidences from HR FT-ICR MALDI-MS and NMR studies supported these conclusions. The recycle of catalyst without loss of activity, the use of water as reaction medium and high reaction efficiency made the protocol competitive with those carried out in organic solvent. Furthermore, in silico studies were used to rationalize the reaction stereoselectivity outcome and to improve it by the choice of proper substrates able to interact with the cyclodextrin through hydrogen bond interactions. In fact, the calculations supported an inclusion mode with the insertion of phenyl ring of styrene 32 within the cavity pointing to the down rim and with the phenyl of nitrone 31 protruding out the cavity at the height of secondary OH groups in the upper rim (Floresta et al., 2017).
Figure 7. γ-Cyclodextrin catalyzes 1, 3-dipolar cycloaddition between different nitrones and styrenes to afford substituted isoxazolidines.
Many modified cyclodextrins have been prepared through selective conversion of the hydroxyl groups to other functionalities with the aim to modify and improve their catalytic abilities. The introduction of one or more reactive binding sites in precise positions can offer multiple molecular recognition sites and allow a more effective and selective catalysis (Hattori and Ikeda, 2006).
In the field of supramolecular organocatalysis, the rationale of this approach is based on the possibility of combining the catalytic properties of an organocatalyst placed in an appropriate position on the CD scaffold and suitable for the target reaction and the ability of cyclodextrins to form inclusion complexes.
In 2012, Doyagüez and Fernández-Mayoralas reported the use of cyclodextrins functionalized with proline as efficient supramolecular organocatalysts for aldol reactions in water (Figure 8A). Several coniugates of L-proline and β-CD 38 were prepared changing the nature of the linker between proline and β-CD and tested as catalysts for the aldol reaction between p-nitrobenzaldehyde 35 and different ketones 36 and 37. A dependency on the nature of the linker was highlighted and the best results were obtained with more flexible succinamyl moiety. Studies were performed to understand the role played by β-CD. The reaction carried out with the proline arm alone, depleted of CD scaffold, did not take place even after prolonged reaction times, and the poor conversion observed by adding β-CD to the reaction suggested a role of β-CD in dissolving the reactants. The reaction efficiency decreased by adding sodium 2-naphthalenesulfonate, a known high affinity guest for β-CD (Inoue et al., 1993), pointing out the role of hydrophobic cavity by forming inclusion complexes with the aldehyde. Unfortunately, although the reaction efficiency ranged from moderate to high yields, the enantioselectivity was poor (Doyagüez and Fernández-Mayoralas, 2012).
Figure 8. Proline-cyclodextrin coniugates catalyze the aldol reaction: (A) Aldol reaction between p-nitrobenzaldehyde and different ketones in the presence of CD-derivatives 38; (B) Aldol reaction between different aldehydes and acetone in the presence of CD-derivative 43.
Higher enantioselectivities were obtained by Liu and Zhang for the aldol reaction between different aldehydes 41 and acetone 42 in the presence of a β-cyclodextrin 43 with a proline linked via a urea functional unit (Figure 8B). Aromatic and aliphatic aldehydes reacted with acetone in moderate to good yields and with good to high enantioselectivities. The reaction efficiency showed a substrate dependence that the authors attributed to the different binding abilities with the interior cavity of β-CD. In fact, the drop in the reaction enantioselectivity obtained in the order para-, meta- and orto-substituted benzaldehydes was consistent with the decrease of binding constants between the substituted aromatic aldehydes and β-CD. Moreover, an interesting advantage of the procedure was the recycle of the catalyst. After the product isolation with an organic solvent, the catalyst being soluble in water remained in the aqueous phase which could be used as reaction medium for another reaction. This process could be done several times without loss of the reactivity and enantioselectivity. (Liu and Zhang, 2015).
In 2013, β-cylodextrins peramino (per-6-ABCDs) functionalized on primary face were reported for the first time in an efficient one-pot procedure for the synthesis of enantiomerically enriched quinolone derivatives 47, privileged building blocks useful to access a wide array of biologically active compounds (Kanagaraj and Pitchumani, 2013). Per-6-ABCDs behaved simultaneously as a supramolecular host and a chiral multifunctional base catalyst giving the reaction adducts with high yields and enantiomeric excesses. The per-substitution of the CD with amino groups was demonstrated to be necessary for the reaction outcome. When the reaction was performed with native β-CD or β-CD and external base or per-amino-β-CD hydrochloride, the reaction efficiency fell down significantly in terms of conversion and enantioselectivity. The substrate scope of the reaction was explored using various aryl and heteroaryl substituted aldehydes 45. The reaction worked well showing independent on the electronic nature of aryl substituents, but their position on the ring and size exerted significant influence on the enantioselectivity suggesting the importance of a tight fitting of guests within the chiral cavity. The mechanism proposed involved in the first step the inclusion of o-aminoacetophenone 46 and aldehyde 45 within CD cavity. The primary amino groups of CD acted both as a base abstracting a proton by the acetyl group of o-aminoacetophenone 45 and hydrogen-bond donor toward the carbonyl group of aldehyde 46 (Figure 9A). The condensation between the activated substrates yielded the corresponding chalcone which subsequently underwent isomerization via aza-Michael reaction and then tautomerization to give the target compounds. The inclusion of o-aminoacetophenone 45 inside the CD cavity and its activation at Si face by amino groups of CD drove the stereochemical outcome of the aza-Michael addition. Excellent enantiomeric excesses were obtained with a host to guest ratio of 1:1. Molecular modeling studies, NMR and ESI-MS data provided further support to the proposed mechanism.
Figure 9. Synthesis of heterocycles promoted by CDs: (A) Peramino-CDs promote the synthesis of quinolone scaffolds; (B) Efficient synthesis of indole derivatives catalyzed by a sulfonated-β-cyclodextrin.
Another efficient application of modified CDs in the synthesis of heterocyclic scaffolds was reported by Tayade et al. A sulfonated-β-cyclodextrin was able to promote the reaction between different indoles 49 and isatins 48 to give the corresponding products 50 or 51 in good to excellent yields in very short reaction times (Figure 9B). The results obtained showed that the electronic effects of the substituents influenced only slightly the reaction outcome. The accelerated rate and high efficiency were ascribed to greater solubility of sulfonated-β-CD than native β-CD in water and more effective formation of inclusion complexes with isatins. Reaction optimization studies highlighted that 10 mol% of sulfonated-β-cyclodextrin and reflux temperature were the best conditions for this reaction resulting in excellent yields and extremely short reaction times (from 5 h at room temperature to 5 min at reflux). Additional advantage of the reaction was that the catalyst could be recovered and reused for three catalytic cycles without significant loss in catalytic activity (Tayade et al., 2015).
Amazing results were obtained using cyclodextrins in the Paal-Knorr condensation between 1,4-diketones and primary amines for the synthesis of pyrroles. (Menuel et al., 2014; Akelis et al., 2016) Partially methylated β-CDs (RAME-β-CD) have been used as mass transfer agents promoting the Paal-Knorr condensation of 1,4-diketones 53 and amines 52 for the synthesis of N-substituted pyrroles 54 under mild reaction conditions (Figure 10A). The activity of RAME-β-CDs resulted better than their native analogs and the results were explained on the basis of their higher water solubility and surface activity. The reaction was explored with different ketones and aromatic and aliphatic amines. In all cases, the corresponding products were obtained in good yields. The proposed mechanism involved the inclusion of amine inside the CD cavity, and an interaction between the amine and an hydroxyl group of the CD which promoted pyrrole formation. Particularly, when the reaction was performed with aliphatic and aromatic diamines 55, the amount of amine used affected the selective formation of monopyrrole 56 or unsymmetrical bis-pyrrole derivatives 57 (Figure 10B).
Figure 10. Paal-Knorr pyrrole synthesis assisted by partially methyled β-cyclodextrins: (A) Paal-Knorr condensation of 1,4-diketones and amines; (B) Paal-Knorr condensation of 1,4-diketones and diamines.
Calixarenes represent a fascinating class of macrocyclic compounds particularly investigated in supramolecular chemistry (Figure 1B). They are constituted by p-tert-butylphenol units bridged with methylene units. Calix[n]arenes have the general structure reported in Figure 1 in which the number n of phenolic units ranges from 4 to 20. However, the most studied members are those constituted by 4–8 aromatic units. The name “calixarene” was introduced by C. D. Gutsche who was inspired by the similarity of the cone-conformation of calix[4]arene 1a with a greek vase (calix crater) (Gutache et al., 1975; Gutsche and Muthukrishnan, 1978). Subsequently, this name was also extended to the other calixarene homologs. Calixarenes show a central hydrophobic cavity of controllable size, in addition to a wide upper rim and a narrow lower rim. The synthetic and structural versatility of these macrocycles make them suitable for different applications, (Böhmer, 1995; Gaeta et al., 2002; De Rosa et al., 2015; Neri et al., 2016; Talotta et al., 2017) including molecular recognition and sensing (Guo et al., 2011, 2012, 2013; Gaeta et al., 2012; Guo and Liu, 2014; Sun et al., 2016; Talotta et al., 2016; Yeon et al., 2016; Zheng et al., 2018), self-assembly processes (Rebek, 2000; Rudkevic, 2001; Díaz- Moscoso et al., 2016), synthesis of interpenetrated architectures (Gaeta et al., 2013, 2016; Arduini et al., 2016; De Rosa et al., 2017c; La Manna et al., 2017; Talotta et al., 2018), and catalysis (Cacciapaglia and Mandolini, 2000; Li et al., 2011; Deraedt and Astruc, 2016; Stoikov et al., 2016; Yilmaz and Sayin, 2016).
Calix[4]arenes bearing a chiral proline moiety at upper or lower rim have been successfully used by some research groups as organocatalysts for the direct aldol reaction between cyclohexanone 59 and various aromatic aldehydes 58 in water. (Figure 11) The catalytic activity of these calixarene derivatives highlighted the key role of their hydrophobic cavities for the reaction outcome, considering the poor activity of proline alone as promoter of the direct aldol reaction in water. For all catalysts the observed results suggested a mechanism based on general enamine catalysis where the reaction was accelerated by the formation of a hydrophilic and hydrophobic region through hydrogen bonding interactions between the free-OH groups of interfacial water molecules and the H-bond acceptor groups such as OH, NH, and CO groups of the calixarene scaffolds. Under optimal conditions, from good to high yields (>95%), high enantioselectivities (>90%) and from moderate to high diastereoselectivities (up to 65/35) were observed for all organocatalysts 60a-h. The recycle of the catalysts and their reuse without notable loss of activity and selectivity were characteristics common to all catalysts. Interestingly, catalyst 60e showed selectivity for cyclic ketones with different size thus providing further proof of the usefulness of its cavity as a selective nanoreactor for reactants (Li et al., 2009, 2010, 2017; Eymur et al., 2014; Uyanik et al., 2014; Aktas et al., 2016; Sahin et al., 2016).
Figure 11. Direct asymmetric aldol reaction catalyzed by calix[4]arene functionalized with a chiral proline.
A calix[4]arene bearing at lower rim imidazole moieties (64) was reported as an efficient phase-transfer catalyst for aromatic nucleophilic substitution reaction and benzyl nucleophilic substitution (Figure 12A). The high catalytic abilities compared to classical ionic liquids suggested that not only the good solubility in water due to the presence of ions groups but also the combination with complexation abilities of its cavity played an important role for the catalysis: the aromatic substrate solubility in water was improved by inclusion in the calixarene cavity and by its structural conformation. The catalytic activity of the calixarene derivative was higher with the calixarene in more stable cone conformation (Yang et al., 2012).
Figure 12. Water-soluble calixarenes as supramolecular organocatalysts in: (A) nucleophilic substitution reaction; (B) Mannich reaction; (C) cyclocondensation reaction; (D) Michael addition reaction.
In 2014, Sayin and Yilmaz investigated the feasibility to use calix[n]-arenes functionalized at the upper rim with quaternary ammonium salts 69 and 70 as catalyst for one-pot Mannich reaction between benzaldehyde 66, acetophenone 68 and aniline 67 in water. They found that the calixarene catalysts gave β-aminocarbonyl compounds 71 in high yields and very short reaction times, even with small amount of catalyst. (Figure 12B) Studies on the effect of cavity were carried out highlighting its involvement in the catalysis. In the presence of tetrabutylammonium bromide (TBAB) as competitive guest for the cavity, no conversion to reaction products was observed, even after prolonged reaction times, thus suggesting that the reaction occurred inside the cavity. Furthermore, comparing the results obtained with calix[4]- and calix[6]arene, it was highlighted that the calix[6]arene with a larger cavity size worked better than the other one in terms of yields and reaction times. Therefore, it could be concluded that the reaction was triggered by the host-guest complexation of benzaldehyde with calixarene, and the furoyl-piperazinium groups at the upper rim interacted with aniline and acetophenone by weak non-covalent interactions to bring closer to the benzaldehyde (Sayin and Yilmaz, 2014).
In 2015, Rahman et al. used p-sulfonic acid calixarenes 74 and 75 as organocatalysts for the synthesis of 2,3-dihydroquinazolyn-4(1H)-ones 76 in water (Figure 12C). The reaction of cyclocondensation between anthranilamide 72 and different carbonyl compounds 73 afforded the target compounds in high yields under mild reaction conditions. The reaction performed with p-hydroxy benzenesulfonic acid (p-HAS) as catalyst, resulted less efficient than the reaction with calixarene derivatives 74 underlining the role of calixarene framework combined with its ability to activate aldehyde via H-bonding interaction with the sulphonic groups on the reaction. The general scope, the scale-up of the reaction to gram-scale, easy work-up, low amount of catalyst, mild reaction condition, and short reaction time are considerable advantages of this protocol (Rahaman et al., 2015).
Another example of calixarene sulfonic acid derivatives as efficient organocatalysts has been described by Xie et al. Alkylation of indoles 77 with α,β-unsaturated ketones 78 by means of Michael addition was promoted by water-soluble calix[6]arene sulfonic acids under microwave irradiation in water (Figure 12D). Calix[6]arene sulfonic acids 79 acted as inverse phase transfer catalysts. Particularly, calixarene 79d bearing pendant aliphatic chains and thus offering the possibility to form micelles in water, proved to be the optimal catalyst. The scope of the reaction was broad with respect to different indoles 77 and α,β-unsaturated ketones 78, low amount of catalyst (5 mol%) are able to promote the reaction affording the Michael adducts in good to high yields (Xie et al., 2013).
Recently, calix[n]arene scaffolds adorned with a supramolecularly interacting group such as thiourea moiety have been used as organocatalysts for Vinylogous Mukaiyama Aldol reaction (VMAR) between 2-(trimethylsilyloxy)furan 82 and α-ketoesters 81 under “on-water” conditions (Figure 13A). The reaction is a useful protocol to obtain functionalized γ-butenolides, which are valuable building blocks for many biologically active compounds and, recently interesting for the engineering of new crystalline assemblies (De Rosa et al., 2009, 2017a). It was shown that the catalytic ability of the calixarenes 85 and 86 was closely related to their remarkable hydrophobicity and their recognition abilities toward the reactants through hydrogen—bonding interactions with a thioureido group at the upper rim. The catalysts were more active using water as reaction medium than other organic solvents, the reaction was completely regioselective affording only the γ-adducts 83 and, interestingly, a switch of stereoselectivity was observed going from organic solvent to water with a preference for anti diastereoisomer 83. It is noteworthy that, by increasing the dimension of the calixarene-catalyst (85 vs. 86), and consequently its hydrophobicity, a significant increase of the reaction rate occurred. Binding studies between catalyst and α-ketoesters by 1H-NMR spectroscopy and molecular mechanics calculations clearly indicated a supramolecular control of this catalysis associated with recognition abilities of calixarenes (De Rosa et al., 2016).
Figure 13. Calixarenes as efficient organocatalyst for a vinylogous Mukaiyama aldol reaction (VMAR) under “on-water” conditions: (A) VMAR promoted by thioureido-calixarene derivatives 85 and 86; (B) VMAR catalyzed by tetraminocalix[4]arene 87.
In a later paper, the same authors proposed that the hydrophobic amplification observed under “on- water” conditions could enable even weaker H-bond donor moieties to catalyze the VMAR under “on-water” conditions (De Rosa et al., 2017b). In fact, simple tetraminocalix[4]arene 87 with weak H-bond donor NH2 groups was an efficient organocatalyst for the reaction of TMSFO 82 and α-ketoester 81 under “on-water” conditions and its catalytic activity was superior to that in organic solvents. Furthermore, its activity was better than previous catalysts 85 and 86 in terms both conversion, reaction times and selectivity and there was a switch of the stereoselectivity in favor of syn-adduct (Figure 13). The reaction was general with a variety of α-ketoesters with good to high yields and showed in all cases a preference for syn diastereomer syn 83. The reaction outcome was rationalized through a multipoint recognition model where the amino groups of the calixarene were engaged in H-bond interactions with both reactants favoring the stabilization of a ternary complex 81·82·87 that drove the attack at the activated carbonyl group of α-ketoesters on a favored side. The proposed model was in agreement with the differences in the conversion and stereoselectivity detected with different substrates.
In the last years, significant advances have been made in the field of supramolecular organocatalysis using water as a reaction medium and thus demonstrating its synthetic potential. Different types of reactions promoted by supramolecular organocatalysts in water have been reported, thus providing not only environmentally friendly and efficient procedures, but in some cases new reactivity and reaction pathways different from those in organic solvents. Two principal topics drive this type of catalysis: (1) the hydrophobic effect and (2) the molecular recognition. Here, we have focused our attention on selected recent developments in this field using cyclodextrins and calixarenes as supramolecular scaffolds. These macrocycles are interesting because they provide a hydrophobic cavity, with tunable size and shape, capable of selectively accommodating the substrates isolating them from the reaction environment. In addition, the easy functionalization of their scaffold allows to introduce additional catalytic groups or binding sites in specific positions. The reported examples highlight how the combined action of these two characteristics have a positive effect on the reaction outcome.
In analogy to natural enzymes which have evolved over time improving efficiency and selectivity, we think that supramolecular organocatalysis in water can be expected to grow in the coming years demonstrating a further broadening of its applications.
MD: Wrote manuscript and chose references; PL: Gave assistance for literature search; CT: Provided assistance for some revisions; AS: Contributed to the discussions and gave assistance for literature search; CG: Designed the main content; PN: Provided professional advice. All authors read and approved the final manuscript version to be submitted.
The authors declare that the research was conducted in the absence of any commercial or financial relationships that could be construed as a potential conflict of interest.
Akelis, L., Rousseau, J., Juskenas, R., Dodonova, J., Rousseau, C., Menuel, S., et al. (2016). Greener Paal–Knorr pyrrole synthesis by mechanical activation. Eur. J. Org. Chem. 2016, 31–35. doi: 10.1002/ejoc.201501223
Aktas, M., Uyanik, A., Eymur, S., and Yilmaz, M. (2016). L-proline derivatives based on a calix[4]arene scaffold as chiral organocatalysts for the direct asymmetric aldol reaction in water. Supramol. Chem. 28, 351–359. doi: 10.1080/10610278.2015.1073288
Arduini, A., Orlandini, G., Secchi, A., Credi, A., Silvi, S., and Venturi, M. (2016). “ Calixarene threading by viologen-based axles,” in Calixarenes and Beyond, eds P. Neri, J. L. Sessler, and M.-X Wang (Cham: Springer International Publishing), 761–781.
Bai, C. C., Tian, B. R., Zhao, T., Huang, Q., and Wang, Z. Z. (2017). Cyclodextrin-Catalyzed organic synthesis: reactions, mechanisms, and applications. Molecules 22:E1475. doi: 10.3390/molecules22091475
Bogliotti, N., and Dalko, P. I. (2007). “Shape- and site-selective asymmetric reactions”, in Enantioselective Organocatalysis: Reactions and Experimental Procedures, ed P. I. Dalko (Weinheim: Wiley-VCH Verlag GmbH & Co. KGaA), 425–449.
Böhmer, V. (1995). Calixarenes, macrocycles with (Almost) unlimited possibilities. Angew. Chem. Int. Ed. Engl. 34, 713–745. doi: 10.1002/anie.199507131
Borsato, G., and Scarso, A. (2016). “Catalysis within the self-assembled resorcin[4]arene hexamer,” in Organic Nanoreactors. From Molecular to Supramolecular Organic Compounds, ed S. Sadjadi (Boston, MA: Academic Press), 203–234.
Breslow, R. (1991). Hydrophobic effects on simple organic reactions in water. Acc. Chem. Res. 24, 159–164. doi: 10.1021/ar00006a001
Breslow, R., and Dong, S. D. (1998). Biomimetic reactions catalyzed by cyclodextrins and their derivatives. Chem. Rev. 98, 1997–2011.
Bugg, T. D. H. (2012). Introduction to Enzyme and Coenzyme Chemistry, 3rd Edn. Chichester: John Wiley & Sons, Ltd
Butler, R. N., and Coyne, A. G. (2010). Water: nature's reaction enforcer-comparative effects for organic synthesis “In-water” and “On-Water”. Chem. Rev. 110, 6302–6337. doi: 10.1021/cr100162c
Cacciapaglia, R., and Mandolini, L. (2000). “Calixarene based catalytic system,” in Calixarenes in Action, eds L. Mandolini and R. Ungaro (Singapore: Imperial College Press), 241–264.
Copeland, R. A. (2002). Enzymes: A Pratical Introduction to Structure, Mechanism, and Data Analysis, 2nd Edn. New York, NY: John Wiley & Sons, Inc.
Deraedt, C., and Astruc, D. (2016). Supramolecular nanoreactors for catalysis. Coord. Chem. Rev. 324, 106–122. doi: 10.1016/j.ccr.2016.07.007
De Rosa, M., La Manna, P., Soriente, A., Gaeta, C., Talotta, C., Hickey, N., et al. (2017a). Supramolecular synthons in the gamma-hydroxybutenolides. Cryst. Eng. Comm. 19, 5079–5088. doi: 10.1039/C7CE00953D
De Rosa, M., La Manna, P., Soriente, A., Gaeta, C., Talotta, C., Hickey, N., et al. (2017b). A simple tetraminocalix[4]arene as a highly efficient catalyst under “On-Water” conditions through hydrophobic amplification of weak hydrogen bonds. Chem. Eur. J. 23, 7142–7151. doi: 10.1002/chem.201701247
De Rosa, M., La Manna, P., Soriente, A., Gaeta, C., Talotta, C., and Neri, P. (2016). Exploiting the hydrophobicity of calixarene macrocycles for catalysis under “on-water” conditions. RSC Adv. 6, 91846–91851. doi: 10.1039/C6RA19270J
De Rosa, M., Soriente, A., Concilio, G., Talotta, C., Gaeta, C., and Neri, P. (2015). Nucleophilic functionalization of the Calix[6]arene Para- and Meta-p position via p-Bromodienone route. J. Org. Chem. 80, 7295–7300. doi: 10.1021/acs.joc.5b00978
De Rosa, M., Talotta, C., Gaeta, C., Soriente, A., Neri, P., Pappalardo, S., et al. (2017c). Calix[5]arene through-the-annulus threading of dialkylammonium guests weakly paired to the TFPB anion. J. Org. Chem. 82, 5162–5168. doi: 10.1021/acs.joc.7b00406
De Rosa, M., Talotta, C., and Soriente, A. (2009). A new organocatalytic approach to substituted unsaturated lactams. Lett. Org. Chem. 6, 301–305. doi: 10.2174/157017809788489936
Díaz- Moscoso, A., Arroyave, F. A., and Ballester, P. (2016). Moving systems of polar dimeric capsules out of thermal equilibrium by light irradiation. Chem. Comm. 52, 3046–3049. doi: 10.1039/C5CC10403C
Dong, Z., Luo, Q., and Liu, J. (2012). Artificial enzymes based on supramolecular scaffolds. Chem. Soc. Rev. 41, 7890–7908. doi: 10.1039/c2cs35207a
Dong, Z., Wang, Y., Yin, Y., and Liu, J. (2011). Supramolecular enzyme mimics by self-assembly. Curr. Opin. Colloid Interface Sci. 16, 451–458. doi: 10.1016/j.cocis.2011.08.006
Doyagüez, E. G., and Fernández-Mayoralas, A. (2012). Proline -cyclodextrin conjugates: synthesis and evaluation as catalysts for aldol reaction in water. Tetrahedron 68, 7345–7354. doi: 10.1016/j.tet.2012.06.089
Dzierzbicka, K., Trzonkowski, P., Sewerynek, P. L., and Mysliwski, A. (2003). Synthesis and cytotoxic activity of conjugates of muramyl and normuramyl dipeptides with batracylin derivatives. J. Med. Chem. 46, 978–986. doi: 10.1021/jm021067v
Eymur, S., Akceylan, E., Sahin, O., Uyanik, A., and Yilmaz, M. (2014). Direct enantioselective aldol reactions catalyzed by calix[4]arene-based L-proline derivatives in the water. Tetrahedron 70, 4471–4477. doi: 10.1016/j.tet.2014.05.034
Floresta, G., Talotta, C., Gaeta, C., De Rosa, M., Chiacchio, U., Neri, P., et al. (2017). γ-Cyclodextrin as a catalyst for the synthesis of 2-methyl-3,5-diarylisoxazolidines in water. J. Org. Chem. 82, 4631–4639. doi: 10.1021/acs.joc.7b00227
Gaeta, C., Martino, M., Gregoli, L., and Neri, P. (2002). Convenient regioselective functionalization at the upper-rim of p-tert-butylcalix[8]arene through a protection–deprotection procedure. Tetrahedron Lett. 43, 8875–8878. doi: 10.1016/S0040-4039(02)02204-9
Gaeta, C., Talotta, C., De Rosa, M., Soriente, A., and Neri, P. (2016). “Calixarene threading via superweak anion,” in Calixarenes and Beyond, eds P. Neri, J. L. Sessler, and M.-X. Wang (Cham: Springer International Publishing), 783–809.
Gaeta, C., Talotta, C., Farina, F., Teixeira, F. A., Marcos, P. A., Ascenso, J. R., et al. (2012). Alkylammonium cation complexation into the narrow cavity of Dihomooxacalix[4]arene macrocycle. J. Org. Chem. 77, 10285–10293. doi: 10.1021/jo3019945
Gaeta, C., Talotta, C., Mirra, S., Margarucci, L., Casapullo, A., and Neri, P. (2013). Catenation of calixarene annulus. Org. Lett. 15, 116–119. doi: 10.1021/ol303142c
Giacalone, F., and Gruttadauria, M. (2013). “Water in organocatalytic reactions,” in Comprehensive Enantioselective Organocatalysis: Catalysts, Reactions, and Applications, ed P. I. Dalko (Weinheim: Wiley-VCH Verlag GmbH & Co. KGaA), 673–717.
Guo, D.-S., and Liu, Y. (2014). Supramolecular chemistry of p-Sulfonatocalix[n]arenes and its biological applications. Acc. Chem. Res. 47, 1925–1934. doi: 10.1021/ar500009g
Guo, D.-S., Uzunova, V. D., Su, X., Liu, Y., and Nau, W. M. (2011). Operational calixarene-based fluorescent sensing systems for choline and acetylcholine and their application to enzymatic reactions. Chem. Sci. 2, 1722–1734. doi: 10.1039/C1SC00231G
Guo, D.-S., Wang, K., Wang, Y.-X., and Liu, Y. (2012). Cholinesterase-Responsive supramolecular vesicle. J. Am. Chem. Soc. 134, 10244–10250. doi: 10.1021/ja303280r
Guo, D.-S., Yang, J., and Liu, Y. (2013). Specifically monitoring butyrylcholinesterase by supramolecular tandem assay. Chem. Eur. J. 19, 8755–8759. doi: 10.1002/chem.201300980
Gutache, C. D., Kung, T. C., and Hsu, M-L. (1975). Abstracts of the 11th Midwest Regional Meeting of the American Chemical Society, Carbondale, IL.
Gutsche, C. D., and Muthukrishnan, R. (1978). Calixarenes. 1. analysis of the product mixtures produced by the base-catalyzed condensation of formaldehyde with para-substituted phenols. J. Org. Chem. 43, 4905–4906. doi: 10.1021/jo00419a052
Hapiot, F., Bricout, H., Menuel, S., Tilloy, S., and Monflier, E. (2014). Recent breakthroughs in aqueous cyclodextrin-assisted supramolecular catalysis. Catal. Sci. Technol. 4, 1899–1908. doi: 10.1039/c4cy00005f
Hapiot, F., Menuel, S., Ferreira, M., Léger, B., Bricout, H., Tilloy, S., et al. (2017). Catalysis in cyclodextrin-Based unconventional reaction media: recent developments and future opportunities. ACS Sustainable Chem. Eng. 5, 3598–3606. doi: 10.1021/acssuschemeng.6b02886
Hattori, K., and Ikeda, H. (2006). “Modification of cyclodextrins and the chemistry of modified cyclodextrins,” in Cyclodextrins and Their Complexes, ed H. Dodziuk (Weinheim: Wiley-VCH Verlag GmbH & Co. KGaA), 31–64.
Inoue, Y., Hakushi, T., Liu, Y., Tong, L., Shen, B., and Jin, D. (1993). Thermodynamics of molecular recognition by cyclodextrins. 1. calorimetric titration of inclusion complexation of naphthalenesulfonates with.alpha.-,.beta.-, and gamma.-cyclodextrins: enthalpy-entropy compensation. J. Am. Chem. Soc. 115, 475–481. doi: 10.1021/ja00055a017
Jimeno, C. (2016). Water in asymmetric organocatalytic systems: a global perspective. Org. Biomol. Chem. 14, 6147–6164. doi: 10.1039/C6OB00783J
Kanagaraj, K., and Pitchumani, K. (2013). Per-6-amino-β-cyclodextrin as a chiral base catalyst promoting one-pot asymmetric synthesis of 2-Aryl-2,3-dihydro-4-quinolones. J. Org. Chem. 78, 744–751. doi: 10.1021/jo302173a
Kirby, A. J., and Hollfelder, F. (2010). From Enzyme Models to Model Enzymes. Cambridge: John Wiley & Sons, Ltd.
Kleiner, C. M., and Schreiner, P. R. (2006). Hydrophobic amplification of noncovalent organocatalysis. Chem. Comm. 4315–4317. doi: 10.1039/b605850g
Kobayashi, S., and Li, C. -J. (2012). Science of Synthesis: Water in Organic Synthesis, eds S. Kobayashi, E. M. Carreira, C. P. Decicco, A. Fürstner, G. A. Molander, (Stuttgart: Thieme).
Komiyama, M., and Monflier, E. (2006). “Cyclodextrin catalysis,” in Cyclodextrin and Their Complexes, ed H. Dodziuk (Weinheim: Wiley-VCH Verlag GmbH & Co. KGaA), 93–105.
Konkala, K., Chorasia, R., Manjari, P. S., Domingues, N. L. C., and Katla, R. (2016). B-Cyclodextrin as a recyclable catalyst: aqueous phase one-pot four component synthesis of polyfunctionalized pyrroles. RSC Adv. 6, 43339–43344. doi: 10.1039/C6RA08335H
Kshirsagar, U. A. (2015). Recent developments in the chemistry of quinazolinone alkaloids. Org. Biomol. Chem. 13, 9336–9352. doi: 10.1039/C5OB01379H
Kuah, E., Toh, S., Yee, J., Ma, Q., and Gao, Z. (2016). Enzyme mimics: advances and applications. Chem. Eur. J. 22, 8404–8430. doi: 10.1002/chem.201504394
Kumar, A., and Shukla, R. D. (2014). β-Cyclodextrin catalysed C-C bond formation via C(sp3)-H functionalization of 2-Methyl azaarenes with diones in aqueous medium. Green Chem. 17, 848–851. doi: 10.1039/C4GC02287D
Kumar, A., Tripathi, V. D., and Kumar, P. (2011). β-Cyclodextrin catalysed synthesis of tryptanthrin in water. Green Chem. 13, 51–54. doi: 10.1039/C0GC00523A
Kumar, S., and Ahmed, N. (2016). β-Cyclodextrin/IBX in water: highly facile biomimetic one pot deprotection of THP/MOM/Ac/Ts ethers and concomitant oxidative cleavage of chalcone epoxides and oxidative dehydrogenation of alcohols. Green Chem. 18, 648–656. doi: 10.1039/C5GC01785H
Kumar, S., Verma, N., and Ahmed, N. (2015). β-Cyclodextrin in water: highly facile biomimetic one pot deprotection of phenolic THP/MOM/Ac/Ts ethers and concomitant regioselective cyclization of chalcone epoxides and 2'-aminochalcones. RSC Adv. 5, 85128–85138. doi: 10.1039/C5RA15996B
La Manna, P., De Rosa, M., Talotta, C., Gaeta, C., Soriente, A., Floresta, G., et al. (2018). The hexameric resorcinarene capsule as an artificial enzyme: ruling the regio and stereochemistry of a 1,3-dipolar cycloaddition between nitrones and unsaturated aldehydes. Org. Chem. Front. 5, 827–837. doi: 10.1039/C7QO00942A
La Manna, P., Talotta, C., Gaeta, C., Soriente, A., De Rosa, M., and Neri, P. (2017). Threading of an inherently directional calixarene wheel with oriented ammonium axles. J. Org. Chem. 82, 8973–8983. doi: 10.1021/acs.joc.7b01388
La Sorella, G., Strukul, G., and Scarso, A. (2015). Recent advances in catalysis in micellar media. Green Chem. 17, 644–683. doi: 10.1039/C4GC01368A
Li, Z.-Y., Chen, J.-W., Liu, Y., Xia, W., and Wang, L. (2011). The use of calixarenes in asymmetric catalysis. Curr. Org. Chem. 15, 39–61. doi: 10.2174/138527211793797837
Li, Z.-Y., Chen, J.-W., Wang, L., and Pan, Y. (2009). Highly enantioselective direct aldol reactions catalyzed by proline derivatives based on a calix[4]arene Scaffold in the presence of water. SynLett. 14, 2356–2360. doi: 10.1055/s-0029-1217710
Li, Z.-Y., Chen, J.-W., Zheng, C.-Q., Yin, Y., Wang, L., and Sun, X.-Q. (2017). Highly enantioselective aldol reactions catalysed by reusable upper rim-functionalized calix[4]arene-based L-proline organocatalyst in aqueous conditions. Tetrahedron 73, 78–85. doi: 10.1016/j.tet.2016.11.052
Li, Z.-Y., Lu, C.-X., Huang, G., Ma, J.-J., Sun, H., Wang, L., et al. (2010). Novel prolinamide organocatalysts based on calix[4]arene Scaffold for the enantioselective direct aldol reaction. Lett. Org. Chem. 7, 461–466. doi: 10.2174/157017810791824919
Liu, K., and Zhang, G. (2015). Direct asymmetric aldol reactions in aqueous media catalysed by a β-cyclodextrin–proline conjugate with a urea linker. Tetrahedron Lett. 56, 243–246. doi: 10.1016/j.tetlet.2014.11.084
Longstreet, A. R., and McQuade, D. T. (2013). Organic reaction systems: using microcapsules and microreactors to perform chemical synthesis. Acc. Chem. Res. 46, 327–338. doi: 10.1021/ar300144x
Macaev, F., and Boldescu, V. (2015). Cyclodextrins in asymmetric and stereospecific synthesis. Symmetry 7, 1699–1720. doi: 10.3390/sym7041699
Marchetti, L., and Levine, M. (2011). Biomimetic catalysis. ACS Catal. 1, 1090–1118. doi: 10.1021/cs200171u
Marinescu, L., and Bols, M. (2010). Cyclodextrins as supramolecular organo-catalysts. Curr. Org. Chem. 14, 1380–1398. doi: 10.2174/138527210791616885
Martinez-Viturro, C. M., and Dominguez, D. (2007). Synthesis of the antitumoural agent batracylin and related isoindolo[1,2-b]quinazolin-12(10H)-ones. Tetrahedron Lett. 48, 1023–1026. doi: 10.1016/j.tetlet.2006.11.168
Mase, N., and Barbas, C. F. III. (2010). In water, on water, and by water: mimicking nature's aldolases with organocatalysis and water. Org. Biomol. Chem. 8, 4043–4050. doi: 10.1039/C004970K
Menuel, S., Rousseau, J., Rousseau, C., Vaičiunaite Dodonova, J., Tumkevičius, S., and Monflier, E. (2014). Access to pyrrole derivatives in water with the assistance of methylated cyclodextrins. Eur. J. Org. Chem. 2014, 4356–4361. doi: 10.1002/ejoc.201402327
Narayan, S., Muldoon, J., Finn, M. G., Fokin, V. V., Kolb, H. C., and Sharpless, K. B. (2005). “On-water”: unique reactivity of organic compounds in aqueous suspension. Angew. Chem. Int. Ed. 44, 3275–3279. doi: 10.1002/anie.200462883
Neri, P., Sessler, J. L., and Wang, M.-X. (2016). Calixarenes and Beyond. Cham: Springer International Publishing.
Pirrung, M. C. (2006). Acceleration of organic reactions through aqueous solvent effects. Chem. Eur. J. 12, 1312–1317. doi: 10.1002/chem.200500959
Pirrung, M. C., and Sarma, K. D. (2004). Multicomponent reactions are accelerated in water. J. Am. Chem. Soc. 126, 444–445. doi: 10.1021/ja038583a
Rahaman, M., Ling, I., Abdullah, N., Hashim, R., and Hajra, A. (2015). Organocatalysis by p-sulfonic acid calix[4]arene: a convenient and efficient route to 2,3-dihydroquinazolin-4(1H)-ones in water. RSC Adv. 5, 7755–7760. doi: 10.1039/C4RA16374E
Raj, M., and Singh, V. K. (2009). Organocatalytic reactions in water. Chem. Commun. 6687–6703. doi: 10.1039/b910861k
Ramesh, K., Karnakar, K., Satish, G., Harsha Vardhan Reddy, K., and Nageswar, Y. V. D. (2012). Tandem supramolecular synthesis of substituted 2-aryl-2,3-dihydroquinazolin-4(1H)-ones in the presence of β-cyclodextrin in water. Tetrahedron Lett. 53, 6095–6099. doi: 10.1016/j.tetlet.2012.08.141
Raynal, M., Ballester, P., Vidal-Ferran, A., and van Leeuwen, P. W. (2014). Supramolecular catalysis. Part 2: artificial enzyme mimics. Chem. Soc. Rev. 43, 1734–1785. doi: 10.1039/C3CS60037H
Rebek, J. Jr. (2000). Host–guest chemistry of calixarene capsules. Chem. Commun. 637–643. doi: 10.1039/a910339m
Rideout, D. C., and Breslow, R. (1980). Hydrophobic acceleration of diels-alder reactions. J. Am. Chem. Soc. 102, 7816–7817. doi: 10.1021/ja00546a048
Rudkevic, D. M. (2001). “ Self-assembly in solution,” in Calixarenes 2001, eds Z. Asfari, V. Bohmer, J. Harrowfield, and J. Vicens (Dordrecht: Kluwer), 155–180.
Rueping, M., and Theissmann, T. (2010). Asymmetric brønsted acid catalysis in aqueous solution. Chem. Sci. 1, 473–476. doi: 10.1039/c0sc00206b
Sahin, O., Eymur, S., Uyanik, A., Akceyla, E., and Yilmaz, M. (2016). Chiral calix[4]arenes-bearing prolinamide functionality as organocatalyst for asymmetric direct aldol reactions in water. Polycyclic Aromatic Compounds 38, 1–12. doi: 10.1080/10406638.2016.1176058
Sakthivel, K., Notz, W., Bui, T., and Barbas, C. F. (2001). Amino acid catalyzed direct asymmetric aldol reactions: a bioorganic approach to catalytic asymmetric carbon-carbon bond-forming reactions. J. Am. Chem. Soc. 123, 5260–5267. doi: 10.1021/ja010037z
Sayin, S., and Yilmaz, M. (2014). The synthesis of new calix[n]arene quaternary ammonium salts and investigation of their catalytic affinities for three component Mannich-type reactions in water. RSC Adv. 4, 2219–2225. doi: 10.1039/C3RA43881C
Simon, M.-O., and Li, C.-J. (2012). Green chemistry oriented organic synthesis in water. Chem. Soc. Rev. 41, 1415–1427. doi: 10.1039/C1CS15222J
Srivastava, M., Rai, P., Singh, J., Yadav, S., Tripathi, B. P., and Singh, J. (2016). Role of β-CD in water as supramolecular catalysis. Curr. Organocatalysis 3, 32–38. doi: 10.2174/2213337202666150709164207
Steed, J. W., and Atwood, J. L. (2009). “Biological mimics and supramolecular catalysis,” in Supramolecular Chemistry, 2nd Edn. ed. Wiley (Chichester: John Wiley & Sons, Ltd), 778–827.
Stoikov, I. I., Yakimova, L. S., Puplampu, J. B., and Vavilova, A. A. (2016). “System based on calixarenes as the basis for the creation of catalysts and nanocontainers,” in Organic Nanoreactor. From Molecular to Supramolecular Organic Compounds, ed S. Sadjadi (Boston, MA: Academic Press), 85–110.
Sun, Y., Mei, Y., Quan, J., Xiao, X., Zhang, L., Tian, D., et al. (2016). The macroscopic wettable surface: fabricated by calix[4]arene-based host–guest interaction and chiral discrimination of glucose. Chem. Comm. 52, 14416–14418. doi: 10.1039/C6CC07956C
Talotta, C., Caruso, T., De Rosa, M., Gaeta, C., Soriente, A., and Neri, P. (2018). Threading fluorescent calixarene-wheels with ammonium axles. Supramol. Chem. 30, 1–15. doi: 10.1080/10610278.2018.1424852
Talotta, C., Gaeta, C., De Rosa, M., Ascenso, J. R., Marcos, P. M., and Neri, P. (2016). Alkylammonium guest induced-fit recognition by a flexible dihomooxacalix[4]arene derivative. Eur. J. Org. Chem. 2016, 158–167. doi: 10.1002/ejoc.201501319
Talotta, C., Gaeta, C., De Rosa, M., Soriente, A., and Neri, P. (2017). “Calixarenes,” in Comprehensive Supramolecular Chemistry, II., 2nd Edn, eds W. G. Gokel, L. Barbour, J. L. Atwood (The Boulevard, Langford Lane, Kidlington Oxford, UK: Elsevier), 49–74.
Tayade, Y. A., Patil, D. R., Wagh, Y. B., Jangle, A. D., and Dalal, D. S. (2015). An efficient synthesis of 3-indolyl-3-hydroxy oxindoles and 3,3-di(indolyl)indolin-2-ones catalyzed by sulfonated β-CD as a supramolecular catalyst in water. Tetrahedron Lett. 56, 666–673. doi: 10.1016/j.tetlet.2014.12.012
Uyanik, A., Bayrakci, M., Eymur, S., and Yilmaz, M. (2014). Upper rim-functionalized calix[4]arene-based l-proline as organocatalyst for direct asymmetric aldol reactions in water and organic media. Tetrahedron 70, 9307–9313. doi: 10.1016/j.tet.2014.10.063
Van Etten, R., Clowes, G. A., Sebastian, J.:F., and Bender, M. L. (1967a). The mechanism of the cycloamylose-accelerated cleavage of phenyl ester. J. Am. Chem. Soc. 89, 3253. doi: 10.1021/ja00989a028
Van Etten, R., Sebastian, J.:F., Clowes, G. A., and Bender, M. L. (1967b). Acceleration of phenyl ester cleavage by cycloamyloses. A model for enzymatic specificity. J. Am. Chem. Soc. 89, 3242. doi: 10.1021/ja00989a027
Xie, Y., Mao, L., and Li, L. (2013). Calix[6]arene sulfonic acids catalysed Michael reactions of indoles with α,β-unsaturated ketones in water assisted by microwave radiation. J. Chem. Res. 37, 476–479. doi: 10.3184/174751913X13734725288804
Yang, F., Guo, H., and Jiao, Z. (2012). Calixarene ionic liquids: excellent phase transfer catalysts for nucleophilic substitution reaction in water. J. Iran. Chem. Soc. 9, 327–332. doi: 10.1007/s13738-011-0027-6
Yeon, Y., Leem, S., Wagen, C., Lynch, V. M., Kim, S. K., and Sessler, J. L. (2016). 3-(Dicyanomethylidene)indan-1-one-Functionalized Calix[4]arene–Calix[4]pyrrole hybrid: an ion-pair sensor for cesium salts. Org. Lett. 18, 4396–4399. doi: 10.1021/acs.orglett.6b02155
Yilmaz, M., and Sayin, S. (2016). “Calixarenes in organo and biomimetic catalysis,” in Calixarenes and Beyond, eds P. Neri, J. L. Sessler, and M. X. Wang (Cham: Springer International Publishing), 719–742.
Zhang, Q., Catti, L., Pleiss, J., and Tiefenbacher, K. (2017). Terpene cyclizations inside a supramolecular catalyst: leaving-group-controlled product selectivity and mechanistic studies. J. Am. Chem. Soc. 139, 11482–11492. doi: 10.1021/jacs.7b04480
Zhang, Q., and Tiefenbacher, K. (2015). Terpene cyclization catalysed inside a self-assembled cavity. Nat. Chem. 7, 197–202. doi: 10.1038/nchem.2181
Keywords: supramolecular organocatalysis, water, hydrophobic effect, molecular recognition, calixarenes, cyclodextrins
Citation: De Rosa M, La Manna P, Talotta C, Soriente A, Gaeta C and Neri P (2018) Supramolecular Organocatalysis in Water Mediated by Macrocyclic Compounds. Front. Chem. 6:84. doi: 10.3389/fchem.2018.00084
Received: 10 January 2018; Accepted: 12 March 2018;
Published: 03 April 2018.
Edited by:
Alessandro Scarso, Università Ca' Foscari, ItalyCopyright © 2018 De Rosa, La Manna, Talotta, Soriente, Gaeta and Neri. This is an open-access article distributed under the terms of the Creative Commons Attribution License (CC BY). The use, distribution or reproduction in other forums is permitted, provided the original author(s) and the copyright owner are credited and that the original publication in this journal is cited, in accordance with accepted academic practice. No use, distribution or reproduction is permitted which does not comply with these terms.
*Correspondence: Margherita De Rosa, bWFkZXJvc2FAdW5pc2EuaXQ=
Disclaimer: All claims expressed in this article are solely those of the authors and do not necessarily represent those of their affiliated organizations, or those of the publisher, the editors and the reviewers. Any product that may be evaluated in this article or claim that may be made by its manufacturer is not guaranteed or endorsed by the publisher.
Research integrity at Frontiers
Learn more about the work of our research integrity team to safeguard the quality of each article we publish.