- 1Department of Environmental Engineering, Institute of Science and Technology, São Paulo State University, Sorocaba, Brazil
- 2Department of Biochemistry and Tissue Biology, Institute of Biology, State University of Campinas, Campinas, Brazil
Chitosan, a polyaminosaccharide obtained by alkaline deacetylation of chitin, possesses useful properties including biodegradability, biocompatibility, low toxicity, and good miscibility with other polymers. It is extensively used in many applications in biology, medicine, agriculture, environmental protection, and the food and pharmaceutical industries. The amino and hydroxyl groups present in the chitosan backbone provide positions for modifications that are influenced by factors such as the molecular weight, viscosity, and type of chitosan, as well as the reaction conditions. The modification of chitosan by chemical methods is of interest because the basic chitosan skeleton is not modified and the process results in new or improved properties of the material. Among the chitosan derivatives, cyclodextrin-grafted chitosan and poly(ethylene glycol)-grafted chitosan are excellent candidates for a range of biomedical, environmental decontamination, and industrial purposes. This work discusses modifications including chitosan with attached cyclodextrin and poly(ethylene glycol), and the main applications of these chitosan derivatives in the biomedical field.
Introduction
Chitosan is a modified natural cationic polymer composed of β-(1→4)-linked D-glucosamine residues, obtained by partial N-deacetylation of chitin (Figure 1). This polyaminosaccharide polymer has polycationic characteristics, due to the presence of numerous amino groupings (Alves and Mano, 2008; Anitha et al., 2011), and possesses excellent chemical and biological properties, making it very attractive for applications in many areas including biology, chemistry, pharmaceuticals, medicine, agriculture, food, and environment (Yao et al., 2011; Kashyap et al., 2015). However, the amino groupings become positively charged at acid pH, so the polymer is only soluble in dilute acid solutions. Different strategies have been proposed in order to chemically modify chitosan in order to introduce new features to the polymer and extend its possible applications (Figure 2), one of which is copolymerization by grafting (Jayakumar et al., 2005; Alves and Mano, 2008; Thakur et al., 2014). Specific chemical modifications of chitosan that have been reported involve free amino groups on deacetylated units and hydroxyl groups on the C3 and C6 carbons (Filipović-Grcić et al., 2001; Prabaharan and Mano, 2006; Glasing et al., 2016).
Cyclodextrins are cyclic oligosaccharides composed of six to eight units of glucopyranosyl connected by α-(1→4) linkages (Davis and Brewster, 2004). This cyclic organization provides CDs with conical structures in which the central cavity is nonpolar, while the outer surface is polar. The characteristic cavities of cyclodextrins enable these substances to form inclusion complexes with many different kinds of molecules (Zhang et al., 2008; Nuchuchua et al., 2009; Mura, 2014). In pharmaceutical applications, CDs are extensively employed as solubilizers and stabilizers. A limiting factor is that they lack mucoadhesive properties, which can be resolved by grafting these oligosaccharides onto the structures of bioadhesive polymers such as chitosan.
Polyethylene glycol (PEG) is a hydrophilic polymer synthesized from ethylene oxide, consisting of polyether (linear or branched) terminated with hydroxyl groups (Roberts et al., 2012). PEG can be synthesized in a wide range of molecular sizes, with the different PEGs identified by a number indicating the average molecular weight (Schellekens et al., 2013). PEGs are listed by the FDA as generally safe compounds, due to their low toxicity, low immunogenicity and antigenicity, low flammability, biodegradability, and rapid excretion after administration to living organisms (CFR - Code of Federal Regulations Title 21, 2015). Due to these properties, PEGs are used in various fields, especially in the medical and pharmaceutical sectors, where conjugates of PEGs and proteins/drugs have been developed in order to extend circulation times in the blood (Garay et al., 2012). Another field of research that has emerged is the use of PEGs and their derivatives for grafting with polymers and other molecules. The high mobility of the chains, associated with conformational flexibility and water binding capability, enables the production of numerous bioconjugates (Cho et al., 2012).
Cyclodextrins grafted onto chitosan backbones have received considerable attention because carriers composed of this hybrid polymer possess the capability of CDs to form inclusion complexes with different molecules, together with the mucoadhesive properties of chitosan (Alves and Mano, 2008; Pillay et al., 2013). The grafting of PEGs onto chitosan has also been used as a strategy to improve the solubility and biocompatibility of chitosan. Generally, the terminal hydroxyl groups of PEGs are modified to generate derivatives capable of promoting nucleophilic displacements of the amino groups of chitosan. As a consequence, the grafting of PEG onto chitosan has been shown to decrease cytotoxicity and improve the stability of the resulting colloidal carrier system (Malhotra et al., 2011; Casettari et al., 2012).
In order to investigate the evolution of studies conducted during the last 20 years concerning chemical modification of chitosan, a search was performed with the ISI Web of Knowledge data base, using different combinations of keywords (cyclodextrin grafted chitosan; PEG grafted chitosan; PEGylated chitosan; modified chitosan; pharmaceutical applications). The data generated from this search are summarized in Figure 3. It can be seen that the numbers of publications in this field have increased over the years. When broad keywords were used (chemical modification and chitosan; chitosan and copolymerization), the publications increased gradually since the 1990s. For keywords with more specific terms (cyclodextrin grafted chitosan; PEG grafted chitosan; PEGylated chitosan; modified chitosan; pharmaceutical applications), increases started after the year 2000. This growth in publications reflects the interest in using hybrid polymers based on chitosan, which offer many advantages compared to pure chitosan, and indicates the relevance of research in this field due to the applicability of these polymers in many different fields. The aim of this review is to present the different types of chitosan modified with cyclodextrins or PEG (Figure 4), describing the grafting techniques and the use of these hybrid polymers in biotechnological and pharmaceutical applications.
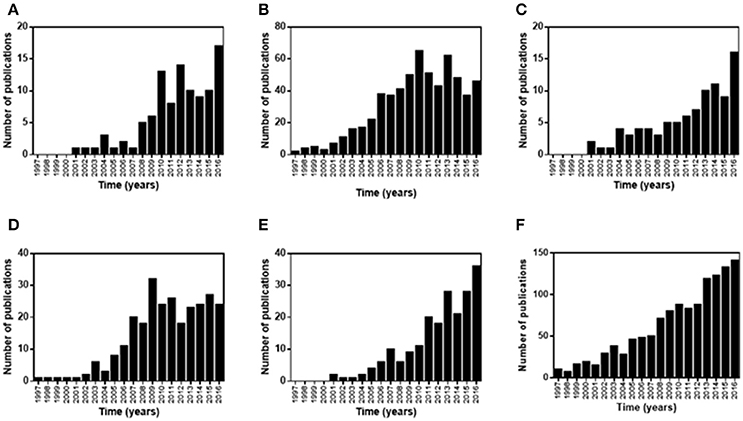
Figure 3. Numbers of publications during the last 20 years involving the chemical modification of chitosan. Each graph represents one combination of keywords: (A) Modified chitosan and pharmaceutical applications; (B) Chitosan and graft copolymerization; (C) Cyclodextrin grafted on chitosan; (D) Grafted poly(ethylene glycol) and chitosan; (E) PEGylated chitosan; (F) Chemical modification and chitosan.
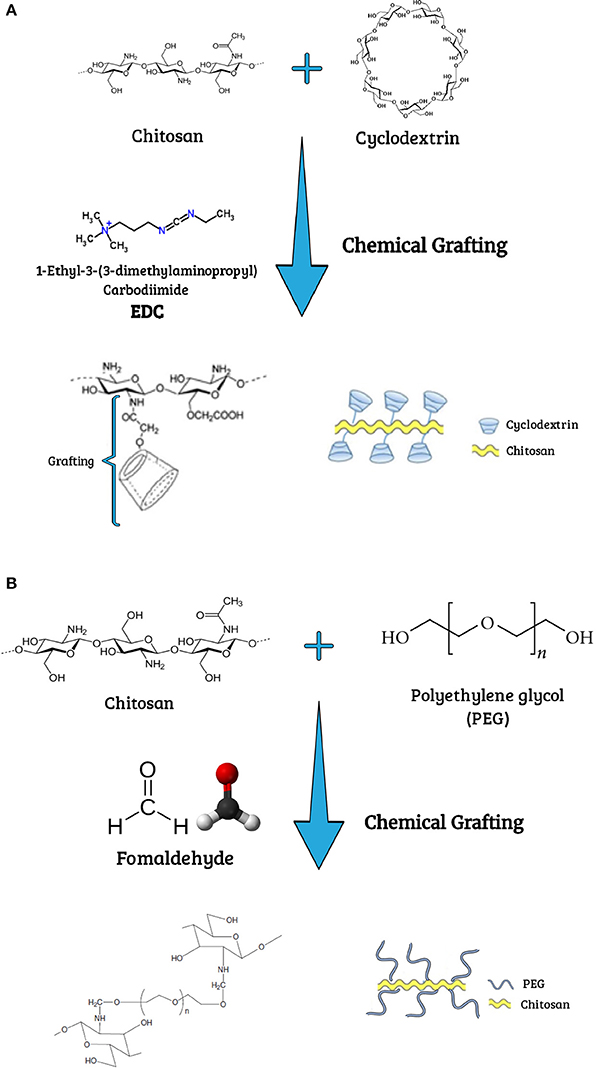
Figure 4. Schematic representations of (A) cyclodextrin chemically grafted onto chitosan, and (B) PEG chemically grafted onto chitosan.
Grafting Methods
The chemical modification of polysaccharides has received increasing attention in recent years, opening perspectives for applications of these modified macromolecules in different fields (Cumpstey, 2013; Pillay et al., 2013; Li et al., 2016). Among the modification methods, graft polymerization is a promising strategy that can be used to introduce a variety of functional groups to polymers (Bhattacharya, 2004; Jayakumar et al., 2005). In the case of chitosan, characteristics such as low water solubility, insolubility in organic solvents, and lack of thermal plasticity have limited its uses in some areas. Chemical modification can be used to overcome these problems (Sashiwa and Aiba, 2004; Olteanu, 2007; Li et al., 2016).
Grafting is a modification technique in which polymer monomers are covalently bonded to the backbone of a parent polymer (the substrate) (Bhattacharya, 2004). This process alters the surface properties, while the modified product still retains the bulk properties of the parent polymer (Yang et al., 2007). Use of grafting reduces desorption and conveys long-term chemical stability because of its covalent nature. Other techniques such as surface coating modifications do not provide temporal stability and the coatings are liable to desorption (Witono et al., 2012). There are various methods of graft copolymerization, employing a single monomer or mixtures of two or more monomers. Table 1 summarizes some of the characteristics and limitations of these different methodologies for grafting cyclodextrins onto chitosan.
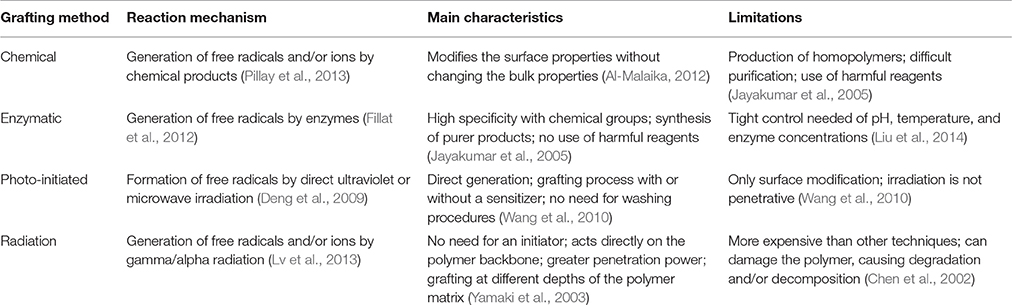
Table 1. Summary of the different grafting methods, reaction mechanisms, characteristics, and limitations.
Grafting by Schiff Base Formation and Reductive Amination
Functional groups containing a nitrogen-carbon double bond (C = N), where nitrogen forms a bond with aryl or alkyl groups, are known as Schiff bases. The Schiff base reaction (Scheme 1), first described by Schiff (1864), can proceed by a condensation reaction of an aldehyde or ketone with a primary amine (Schiff, 1864; da Silva et al., 2011; Ciaccia and Di Stefano, 2015). In order to favor the reaction, the solution must be sufficiently acid, resulting in protonation of the carbonyl compound and making the carbon more susceptible to nucleophilic attack (da Silva et al., 2011; Ciaccia and Di Stefano, 2015).
Substituent groups can be inserted into chitosan backbones by reductive amination (Scheme 2), which is a variation of Schiff base formation (Baxter and Reitz, 2002; Goszczynska et al., 2015). However, in this case, chitosan reacts with an aldehyde or ketone, resulting in an imine intermediate that is subsequently converted to an N-alkyl or N-aryl derivative by reduction employing sodium borohydride (NaBH4) or sodium cyanoborohydride (NaCNBH3) (Baxter and Reitz, 2002). The amines generated by these reactions are stable toward hydrolysis, and other advantages of this route are selective functionalization of the amino groups of chitosan and a homogeneous distribution of substituent groups in the chitosan backbone (Sajomsang, 2010; Badawy and Rabea, 2013).
Du and Hsieh (2007) performed PEGylation of chitosan by reductive amination and acylation, resulting in PEG-N-chitosan and PEG-N,O-chitosan, respectively. Chitosans with different molecular weights (137, 190, and 400 kDa) were used, together with different molecular weight PEGs (550, 2,000, and 5,000 Da). Both reductive amination and acylation resulted in the conversion of hydroxyl-terminal groups of PEG to more reactive groups. It was found that in both reactions, shorter chain lengths of either chitosan or PEG resulted in increased PEGylation. The degree of substitution of PEG-N-CS was between 0.12 and 0.44, while for PEG-N,O-CS, values of 1.50 and 0.60 were obtained for chitosan functionalized with 550 and 2,000 Da PEGs, respectively. All the PEGylated materials became water-soluble, due to the high content of PEG. The authors reported that DNA condensation was much more effective when mediated by pyrene or multi-walled carbon nanotubes.
Liu et al. (2008) prepared chitosan modified by β-cyclodextrin using a Schiff base reaction between 6-O-(4-formylphenyl)-β-cyclodextrin and chitosan. The modified chitosan was used to construct different supramolecular aggregations with pyrene, multi-walled carbon nanotubes, and a combination of pyrene and multi-walled carbon nanotubes. The effect of these supramolecular aggregates on DNA condensation was also evaluated.
Grafting by Amide Formation
Amide bonds are usually formed from pre-activated carboxylic acid derivatives such as acid chlorides and anhydrides, or by using diimide derivatives (Montalbetti and Falque, 2005; Pattabiraman and Bode, 2011). This reaction (Scheme 3) can proceed in different ways: (i) formation of an intermediate acylating agent, followed by aminolysis; (ii) formation of a reactive acylating agent from the acid, followed by treatment with amine; and (iii) addition of an activating or coupling agent, resulting in the in situ generation of an acylating agent from the acid, in the presence of imine (Montalbetti and Falque, 2005; Pattabiraman and Bode, 2011). Carbodiimides have shown great potential for the attachment of molecules onto the chitosan backbone, due to their allene functional groups (Mourya and Inamdar, 2008). The compound 1-ethyl-3-(3-(dimethylamino)propyl) carbodiimide (EDC) is the most widely used carbodiimide, because of its aqueous solubility (Hermanson, 2013). However, there are other carbodiimides that can be used, such as dicyclohexylcarbodiimide (DCC) and N,N′-diisopropylcarbodiimide (DIC), which require organic solvents since they are water-insoluble. The transformation of EDC into a non-toxic urea derivative results in low toxicity associated with the reactions performed with this compound (Hermanson, 2013).
Lin and Hsu (2015) prepared galactosylated chitosan grafted with methoxy poly(ethylene glycol) (mPEG) or short chain poly(ethylene glycol) diacid (PEGd), under aqueous conditions, by amide formation employing EDC, involving the amino groups of chitosan and the carboxylic groups of PEG. The degree of substitution of PEG on the chitosan was 3.7%. Evaluation was also made of the formation of a polyplex with DNA. Chitosan grafted with mPEG formed the most stable polyplex with DNA, followed by galactosylated chitosan grafted with PEGd. Compared to naked DNA, the polyplex enhanced DNA cellular transfection in HepG2 cells.
Tan et al. (2012) prepared and characterized chitosan grafted with carboxymethyl-β-cyclodextrin (CM-β-CD) for subsequent application in anticancer drug delivery. The grafting employed carbodiimide (EDC), which promoted amide formation between the carboxylic groups of CM-β-CD and the amine groups of chitosan. The degree of substitution was measured by a colorimetric method and showed that there was about one grafted CM-β-CD on every fifteenth monosaccharide unit of chitosan (223.05 μmol g−1). The carrier was able to release the anticancer drug at pH 5.0, but not at pH 7.4. This carrier could be used for anticancer drug release because it was pH-sensitive.
Grafting by “Click Reactions”
Although traditional organic syntheses are important tools for the production of new materials, they are inevitably time consuming and expensive (Nwe and Brechbiel, 2009). In order to overcome these limitations, Kolb et al. (2001) introduced the concept of click chemistry, establishing a set of criteria to encourage simplicity and robustness in chemical syntheses. They described a set of reactions that fulfilled these criteria, including chemoselective reactions with high yield, broad scope, removal of the product without use of chromatography, simplicity, and the ability to operate under different conditions. Any reaction fulfilling these prerequisites could be considered a click reaction. The term “click chemistry” refers to reactions where two functional groups react exclusively with each other and which can be performed at room and physiological temperatures. These reactions are divided into four major groups: (i) cycloadditions of unsaturated species; (ii) nucleophilic ring-openings; (iii) non-aldol type carbonyl chemistry; and (iv) additions to carbon-carbon multiple bonds (Hein et al., 2008). Among all the click reactions (Scheme 4), copper-catalyzed Huisgen 1,3-dipolar cycloadditions (CuAAC) of alkynes and azides are the most used (Wallyn et al., 2011; Kim and Kim, 2014).
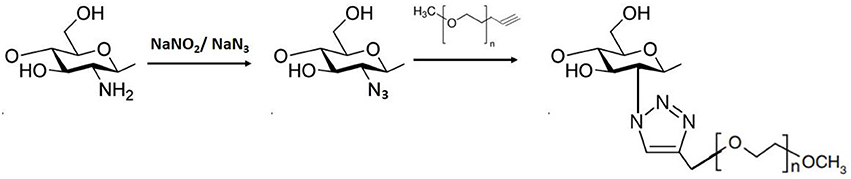
Scheme 4. 1,3-dipolar cycloaddition (adapted from Kulbokaite et al., 2009).
Kulbokaite et al. (2009) studied the preparation and characterization of methoxy PEGylated chitosan (chitosan-N-MPEG) copolymers using click reactions. The polymer was synthesized by the reaction of N-azidated (azide groups) chitosan with acetylene-terminated mPEG (alkyne groups), using 1,3-dipolar cycloaddition. The reaction was successful in a medium consisting of water and methylene chloride (1:1 v/v), but failed in a solution of 5% LiCl in N-methyl-2-pyrrolidone. The failure was attributed to the high concentration of LiI, which resulted in coordination competition with CuI and decreased its catalytic activity. It was shown that an equimolar ratio of CS and mPEG, or even low concentrations of mPEG, resulted in unreactivity of the alkyne groups of mPEG and consequently low degrees of substitution. Polymers synthesized with excess amounts of alkyne groups from mPEG showed degrees of substitution up to 40%, solubility in water, and residual amounts of Cu.
Chen et al. (2013) synthesized chitosan 6-OH immobilized cyclodextrin by click reaction. Firstly, the amino group of chitosan was protected by Schiff base formation with benzaldehyde, followed by the generation of C6-OH p-toluenesulfonate. Subsequently, nucleophilic substitution of sulfonate with NaN3 resulted in Schiff base protected chitosan (BCTS-6-N3). Separately, alkynylic β-cyclodextrin (CD-OPg) was obtained. After the production of these substrates, the Cu (I)-catalyzed click reaction between BCTS-6-N3 and CD-OPg resulted in the 2-benzadehylde Schiff base chitosan 6-OH immobilized cyclodextrin derivative (BCTS-6-CD). The removal of the protecting group then resulted in CTS-6-CD. The loading of immobilized cyclodextrin in the final product was 223.17 μmol g−1.
Grafting by Nucleophilic Substitution Reaction
In addition to the two hydroxyl groups (primary and secondary), chitosan has a third reactive site that is attractive for chemical modification: the primary amine group (Kyzas and Bikiaris, 2015). The non-bonding electron pair on the primary unit acts as a proton acceptor, making chitosan a potent nucleophile (Scheme 5) (Buschmann et al., 2013). Tosyl groups are well known in organic chemistry because they are good electrophiles and leaving groups. Tosylation of the 6-OH group of chitosan has been employed in order to produce tosyl-chitosan as an intermediate, prior to further chitosan modification. Chen et al. (2012) prepared chitin with tosylated 6-OH groups, which were displaced by a monoamino β-cyclodextrin derivative via nucleophilic substitution. Subsequently, the cyclodextrin-grafted chitin was deacetylated, producing the modified chitosan. This method was effective for the production of 6-OH substituted cyclodextrin derivatives of chitosan with high levels of substitution, where the final polymer showed a substitution capacity of around 128.68 mol g−1.
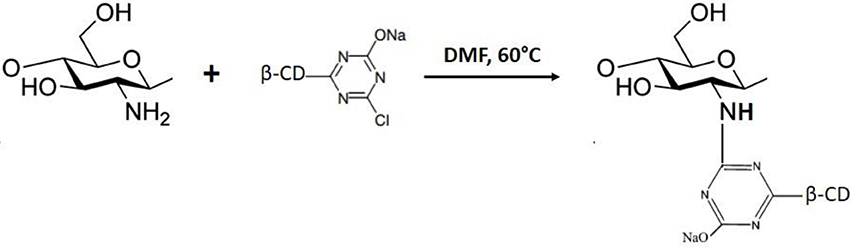
Scheme 5. Nucleophilic substitution reaction (adapted from Martel et al., 2001).
Monochlorotriazinyl groups are known in organic chemistry for their capacity to form covalent bonds with nucleophilic groups. Martel et al. (2001) prepared a chitosan derivative by attaching a monochlorotriazinyl derivative of β-CD onto the chitosan backbone. This reaction occurred by nucleophilic substitution of the chloride atoms of the β-CD by the amino groups of chitosan. The high degree of substitution (2.8) resulted in an insoluble product.
Grafting by Photo-Initiation
Photo-initiated grafting is a simple process based on the formation of radicals by the application of electromagnetic radiation (ultraviolet or microwave). The technique can follow two different routes, either with or without a sensitizer such as methyl methacrylate (Muftuoglu et al., 2004). When the polymer is non-photolabile, the use of a sensitizer is required, with the molecule absorbing light and attaining an excited/high energy state. The sensitizer then transfers this energy to the polymer, causing dissociation of the molecule and formation of the free radicals responsible for the grafting process (Deng et al., 2009). The main photosensitizers include benzoin ethyl ether, acrylated azo dyes, aromatic ketones (benzophenone and xanthone), and metal ions. However, there is still a need for photosensitizers that are more efficient (Deng et al., 2009; Wang et al., 2010).
Sharma and Rajesh (2017) reported a fast way to prepare β-cyclodextrin-grafted chitosan by a microwave assisted method. The capability of the resultant polymer to adsorb palladium (II) was evaluated. The method employing the microwave reactor required only 5 min at 140°C to complete the reaction, whereas the conventional method necessitated approximately 6 h of stirring at 70°C. The polymers showed high selectivity toward palladium (II), with around 96% being recovered from a contaminated solution.
Graft polymerization of chitosan can also be initiated by gamma radiation and enzymes (Wang et al., 2007; Karaki et al., 2016; Li et al., 2016; Yang et al., 2016). It can therefore be seen that this is a useful technique for improving the properties of chitosan and broadening the range of its possible applications. Selection of the graft copolymerization method to be used can be based on the intended use of the hybrid polymer, and each of the different methods has variables that can influence the grafting process (Sun et al., 2003; Thakur et al., 2014).
Characterization of Grafted Polymers
There has been rapid development in the field of grafting copolymerization of polysaccharides in the last decades, because grafted polysaccharides present new characteristics that strongly influence the properties of the material and open up new potential applications (Harish Prashanth and Tharanathan, 2007; Ji et al., 2014; Kyzas and Bikiaris, 2015). The characterization of grafted polysaccharides is essential in graft copolymerization research. There are several techniques employed in polymer characterization, which are selected according to the properties studied (Ratner, 1980; Sapsford et al., 2011; Meléndez-Ortiz and Bucio, 2015). Techniques used to characterize graft copolymerization (Table 2) can be divided into six main areas: (i) graft properties (such as the degree of substitution/graft); (ii) thermodynamic measurements; (iii) surface chemistry; (iv) surface topography; (v) crystalline structure; and (vi) mechanical properties (Ratner, 1980; Meléndez-Ortiz and Bucio, 2015).
Applications
This topic will be divided into two sections: (i) Examples of hybrid copolymers composed of cyclodextrin and chitosan (CD-CS) that have been produced in order to improve the solubility of the associated compound, and their main applications; (ii) discussion of changes in the solubility of copolymers composed of polyethylene glycol grafted onto chitosan (CS-PEG), and their applications in different fields.
Cyclodextrin-Grafted Chitosan
Asamoah-Asare et al. (2014) studied the preparation and characterization of nanoparticles composed of carboxymethyl chitosan (CMC) and carboxymethyl β-cyclodextrin (CM-β-CD), in order to produce a carrier system capable of carrying and releasing both hydrophilic and hydrophobic drugs. Investigation of the feasibility of producing the nanoparticles employed different concentrations of CMC (0.05–1.50%, w/v), while maintaining a constant CM-β-CD concentration (0.8 mg/mL). The results showed that use of a low concentration of CMC produced large nanoparticles, while a high concentration of CMC produced small nanoparticles. In addition, it was observed that use of the lowest and highest CMC concentrations did not result in production of sufficient nanoparticles to be characterized. All the nanoparticles showed negative surface charge, and the zeta potential of the nanoparticles increased as the CMC concentration decreased. The nanoparticles produced were very promising as carriers for both hydrophilic and hydrophobic drugs.
In another example involving drug delivery, Daimon et al. (2014) studied the preparation and physicochemical properties of β-CD grafted onto chitosan in order to develop a possible carrier system for drugs, employing insulin as a model compound. Investigation was made of the supramolecular aggregate formation mechanism and the morphological effects of the action of ionic species. The results revealed different morphologies of the macromolecular aggregates, depending on the media conditions employed. The macromolecular structure, nanoparticles, and large aggregates were formed in acetate, citrate, and phosphate buffers, respectively. In order to simulate the environment after oral administration, the network structure formed in buffer solution was diluted with phosphate buffer and it was observed that the network was maintained. Multivalent interactions consisting of host-guest and electrostatic interactions between β-CD-grafted chitosan and insulin enabled strong binding over a wide pH range.
In another study, Kono and Teshirogi (2015) reported the preparation of smart hydrogels for use as carrier systems for drugs, consisting of β-CD-grafted CMC hydrogels with different ratios of CMC and CD (CD-g-CMCs), produced using 1-ethyl-3-(3-dimethylaminopropyl) carbodiimide (EDC) and N-hydroxysuccinimide (NHS) as the coupling method. One hydrogel was prepared containing only carboxymethyl chitosan, without addition of the CD, and CD-g-CMC gels were prepared with different CD concentrations. Evaluation was made of the swelling at pH 4, 7, and 10, as well as drug adsorption/absorption and release. The drug model employed was acetylsalicylic acid (aspirin). The hydrogels showed low swelling at pH 4 and similar absorptions at pH 7 and 10, indicating that charged groups in the hydrogels participated in the swelling mechanism. The uptake of acetylsalicylic acid by the CD-g-CMC hydrogels was strongly dependent on the amount of CD grafted. After 24 h, the CD-g-CMC hydrogels adsorbed 23.9 μmol.g−1, while the CMC hydrogel only adsorbed 2.4 μmol.g−1 during the same period. The CD-g-CMC hydrogels showed fast release of acetylsalicylic acid in the first 2 h, after which the release became slower. The CMC hydrogels showed more rapid release, with the majority of the drug (86%) released within 2 h.
Other potential biomedical applications of systems based on cyclodextrins grafted onto chitosan that have been reported in the literature are summarized in Table 3.
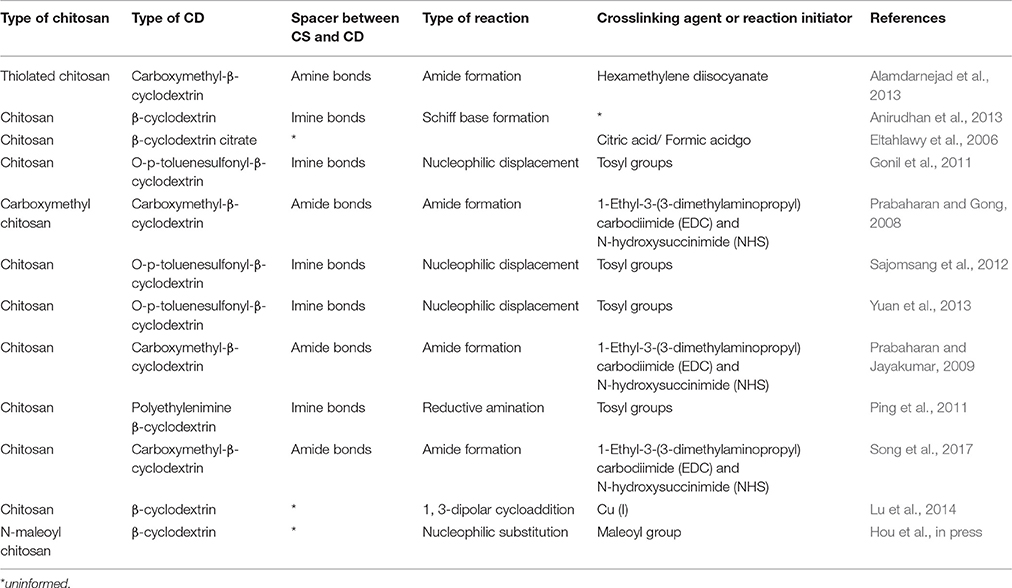
Table 3. Development of systems composed of cyclodextrin grafted onto a chitosan backbone for applications in different biomedical areas.
It can be seen from Table 2 that there are various potential applications of cyclodextrin-grafted chitosan systems. However, further advances are required in order to make it viable to manufacture these products at the commercial scale. Some of the synthesis procedures will need to be optimized in order to produce these grafted compounds for use in commercial products and industrial processes.
Chitosan-PEG
This section describes examples of the use of PEG grafted onto chitosan, considering the potential advantages of this strategy.
In a recent study by Anraku et al. (2014), chitosan was modified with PEG to increase the biocompatibility and water solubility of the polysaccharide. The formaldehyde linking method was used to modify chitosan, using different molecular weights of chitosan (22, 38, and 52 kDa) and a derivative of polyethylene glycol. Nanoparticle aggregates of PEG-CS were also prepared, and the functional and structural properties of the materials were investigated at neutral pH. The copolymer obtained possessed a cationic main chain composed of chitosan, and a non-ionic hydrophilic chain containing PEG. The degrees of substitution of the different copolymers synthesized were 25.6, 28.9, and 27.2 mol% for the mPEG-CS prepared with 22, 38, and 53 kDa chitosan, respectively. The mPEG-CS22, mPEG-CS38, and mPEG-CS53 particle sizes were 259, 413, and 452 nm, respectively, and all the copolymers exhibited almost identical zeta potentials (around +31 mV). The scavenging activities for 2,2-diphenyl-1-picrylhydrazyl (DPPH) revealed that the smallest nanoparticles had the greatest antioxidant activity.
Fu et al. (2014) reported the preparation and characterization of methoxypolyethylene glycol (mPEG) grafted onto chitosan (mPEG-g-CS) and the subsequent preparation of self-assembled polymeric micelles by the ultrasonic method. The integrity of the micelles was evaluated under different pH conditions, using 5-fluorouracil (5-FU) as a model drug. The solution pH affected the solubility of the copolymer, which increased slightly between pH 4 and 5. Micelles were not formed at solution pH greater than 7 or less than 4, and a higher pH favored larger particles. The micelles presented spherical morphology, without aggregation, and particle sizes ranged between 150 and 200 nm. The release profile showed a burst effect during the initial 0.5 h, followed by delayed release.
In another example, Hassani Najafabadi et al. (2014) prepared a PEGylated chitosan by conjugating methoxypolyethylene glycol to the hydroxyl group of chitosan. For this, the most reactive groups (NH2) were first protected with a surfactant. The resulting copolymer was used to prepare nanoparticles loaded with ibuprofen. Dynamic light scattering measurements showed that the loaded nanoparticles presented a mean size distribution of around 80.6 nm, while AFM, TEM, and SEM analyses revealed that the nanoparticles were spherical. The encapsulation efficiency was dependent on the amount of chitosan-PEG, with an increase in the chitosan-PEG amount from 0.1 to 1% (w/v) increasing the ibuprofen encapsulation efficiency from 41 to 99%. When only chitosan was employed in the drug release experiments, the drug was completely released in 12 h. When the copolymer (chitosan-PEG) was employed, release of ibuprofen from the nanoparticles remained incomplete after 48 h. Both chitosan and the copolymer showed near-first order drug release.
Other examples of applications of PEG grafted onto chitosan are listed in Table 4.
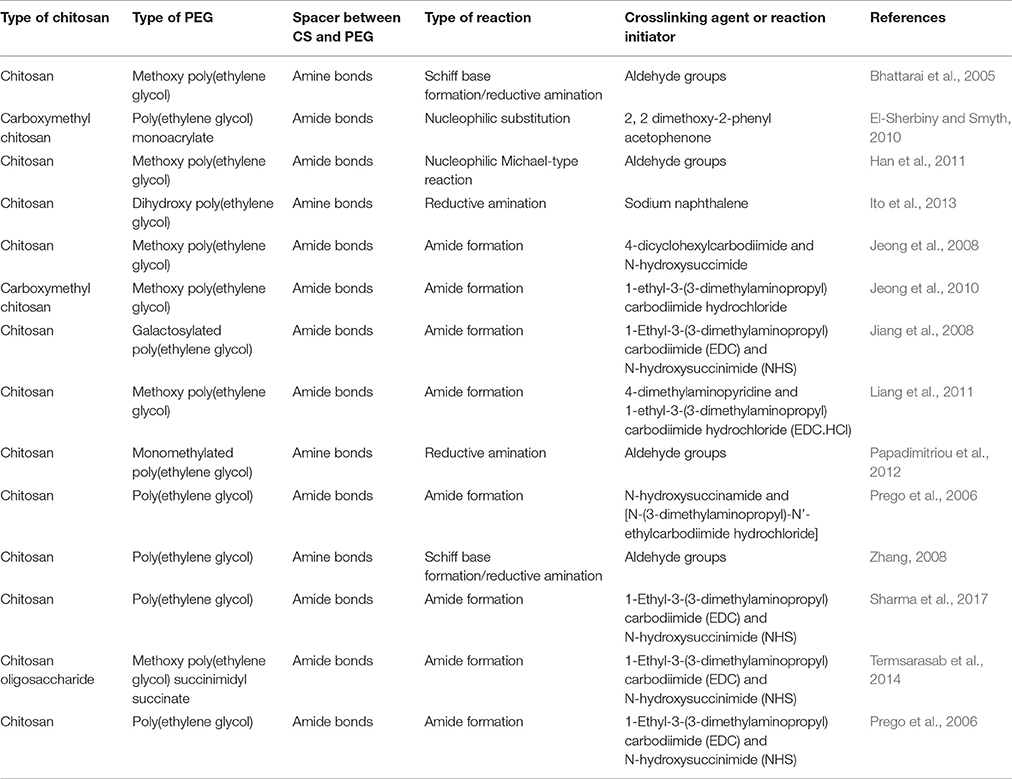
Table 4. Development of systems prepared with poly(ethylene glycol) grafted onto a chitosan backbone for applications in different biomedical areas.
The findings reported in the literature indicate that the strategy of grafting PEG onto chitosan is a good option for extending the applications of chitosan in different biomedical areas. However, synthesis procedures need to be developed at the industrial scale in order to enable the use of these materials in biotechnological and pharmaceutical applications, including commercial products and industrial processes.
Conclusions and Perspectives
Chitosans are aminopolysaccharides whose structures afford excellent possibilities for chemical alteration. Modifications of the chitosan skeleton are made in order to impart suitable properties and functionalities for application of these substances in different areas, especially the biomedical field. Although chitosan presents attractive properties for such uses, its aqueous insolubility is one of the main factors limiting its application on a large scale. Efforts to solve this problem have involved functionalization of the polymer with molecules that improve solubility in water.
This review presents recent advances in the use of chitosan derivatives functionalized with cyclodextrins or PEG. It is clear that many studies have achieved excellent results in terms of the solubilization of hydrophobic compounds (using cyclodextrins), as well as increased solubilization of chitosan (using PEG). There is good evidence that the use of these combined systems should bring benefits in biotechnological and pharmaceutical applications. Nonetheless, synthesis processes need to be improved to enable industrial-scale production of these materials, and emphasis should be given to synthesis routes based on the concepts of green chemistry. Considerable further progress in this area will be required in order to ensure that the promising results already reported in the literature lead to viable products and processes that are able to solve problems in the areas of health and biotechnology.
Author Contributions
EC and JO wrote the manuscript and LF contributed to the discussions and revised the manuscript. All authors approved the final manuscript.
Conflict of Interest Statement
The authors declare that the research was conducted in the absence of any commercial or financial relationships that could be construed as a potential conflict of interest.
Acknowledgments
The authors are grateful for the support provided by the São Paulo State Science Foundation (FAPESP, grants #2014/20273-4, #2014/20286-9, and #2015/15617-9) and CNPq.
References
Alamdarnejad, G., Sharif, A., Taranejoo, S., Janmaleki, M., Kalaee, M. R., Dadgar, M., et al. (2013). Synthesis and characterization of thiolated carboxymethyl chitosan-graft-cyclodextrin nanoparticles as a drug delivery vehicle for albendazole. J. Mater. Sci. Mater. Med. 24, 1939–1949. doi: 10.1007/s10856-013-4947-9
Al-Malaika, S. (2012). Reactive Modifiers for Polymers. Dordrecht: Springer Science & Business Media.
Alves, N. M., and Mano, J. F. (2008). Chitosan derivatives obtained by chemical modifications for biomedical and environmental applications. Int. J. Biol. Macromol. 43, 401–414. doi: 10.1016/j.ijbiomac.2008.09.007
Anirudhan, T. S., Dilu, D., and Sandeep, S. (2013). Synthesis and characterisation of chitosan crosslinked-β-cyclodextrin grafted silylated magnetic nanoparticles for controlled release of Indomethacin. J. Magn. Magn. Mater. 343, 149–156. doi: 10.1016/j.jmmm.2013.04.007
Anitha, A., Maya, S., Deepa, N., Chennazhi, K. P., Nair, S. V., Tamura, H., et al. (2011). Efficient water soluble O-carboxymethyl chitosan nanocarrier for the delivery of curcumin to cancer cells. Carbohydr. Polym. 83, 452–461. doi: 10.1016/j.carbpol.2010.08.008
Anraku, M., Hiraga, A., Iohara, D., Uekama, K., Tomida, H., Otagiri, M., et al. (2014). Preparation and antioxidant activity of PEGylated chitosans with different particle sizes. Int. J. Biol. Macromol. 70, 64–69. doi: 10.1016/j.ijbiomac.2014.06.026
Asamoah-Asare, J., Zhang, Y., Chen, Y., Ren, C., and Fongming, M. (2014). Novel carboxymethyl chitosan-β-cyclodextrin nanoparticles as a drug delivery system; preparation and characterization. Int. J. Eng. Sci. Res. Technol. 3, 141–146.
Badawy, M. E., and Rabea, E. I. (2013). Synthesis and structure–activity relationship of N-(cinnamyl) chitosan analogs as antimicrobial agents. Int. J. Biol. Macromol. 57, 185–192. doi: 10.1016/j.ijbiomac.2013.03.028
Baxter, E. W., and Reitz, A. B. (2002). “Reductive aminations of carbonyl compounds with borohydride and borane reducing agents,” in Organic Reactions, ed John Wiley & Sons, Inc. (Hoboken, NJ: John Wiley & Sons, Inc.), 1–714. doi: 10.1002/0471264180.or059.01
Bhattacharya, A. (2004). Grafting: a versatile means to modify polymersTechniques, factors and applications. Prog. Polym. Sci. 29, 767–814. doi: 10.1016/j.progpolymsci.2004.05.002
Bhattarai, N., Ramay, H. R., Gunn, J., Matsen, F. A., and Zhang, M. (2005). PEG-grafted chitosan as an injectable thermosensitive hydrogel for sustained protein release. J. Controlled Release 103, 609–624. doi: 10.1016/j.jconrel.2004.12.019
Buschmann, M. D., Merzouki, A., Lavertu, M., Thibault, M., Jean, M., and Darras, V. (2013). Chitosans for delivery of nucleic acids. Adv. Drug Deliv. Rev. 65, 1234–1270. doi: 10.1016/j.addr.2013.07.005
Casettari, L., Vllasaliu, D., Castagnino, E., Stolnik, S., Howdle, S., and Illum, L. (2012). PEGylated chitosan derivatives: Synthesis, characterizations and pharmaceutical applications. Prog. Polym. Sci. 37, 659–685. doi: 10.1016/j.progpolymsci.2011.10.001
CFR - Code of Federal Regulations Title 21 (2015). Available online at: http://www.accessdata.fda.gov/scripts/cdrh/cfdocs/cfcfr/CFRSearch.cfm?fr=172.820 (Accessed September 3, 2015).
Chan, P., Kurisawa, M., Chung, J. E., and Yang, Y. Y. (2007). Synthesis and characterization of chitosan-g-poly(ethylene glycol)-folate as a non-viral carrier for tumor-targeted gene delivery. Biomaterials 28, 540–549. doi: 10.1016/j.biomaterials.2006.08.046
Chen, J., Iwata, H., Tsubokawa, N., Maekawa, Y., and Yoshida, M. (2002). Grafting of Polyethylene by γ-Ray Radiation. Grafting onto conductive carbon black and application as novel gas and solute sensors. Available online at: http://inis.iaea.org/Search/search.aspx?orig_q=RN:34057746 (Accessed September 3, 2015).
Chen, Y., Ye, Y., Li, R., Guo, Y., and Tan, H. (2013). Synthesis of chitosan 6-OH immobilized cyclodextrin derivates via click chemistry. Fibers Polym. 14, 1058–1065. doi: 10.1007/s12221-013-1058-7
Chen, Y., Ye, Y., Wang, L., Guo, Y., and Tan, H. (2012). Synthesis of chitosan C6-substituted cyclodextrin derivatives with tosyl-chitin as the intermediate precursor. J. Appl. Polym. Sci. 125, E378–E383. doi: 10.1002/app.36836
Cho, H. J., Yoon, I. S., Yoon, H. Y., Koo, H., Jin, Y. J., Ko, S. H., et al. (2012). Polyethylene glycol-conjugated hyaluronic acid-ceramide self-assembled nanoparticles for targeted delivery of doxorubicin. Biomaterials 33, 1190–1200. doi: 10.1016/j.biomaterials.2011.10.064
Ciaccia, M., and Di Stefano, S. (2015). Mechanisms of imine exchange reactions in organic solvents. Org. Biomol. Chem. 13, 646–654. doi: 10.1039/C4OB02110J
Cumpstey, I. (2013). Chemical modification of polysaccharides. ISRN Org. Chem. 2013, 1–27. doi: 10.1155/2013/417672
Daimon, Y., Izawa, H., Kawakami, K., Zywicki, P., Sakai, H., Abe, M., et al. (2014). Media-dependent morphology of supramolecular aggregates of β-cyclodextrin-grafted chitosan and insulin through multivalent interactions. J. Mater. Chem. B 2:1802. doi: 10.1039/c3tb21528h
Darabi, A., García-Valdez, O., Champagne, P., and Cunningham, M. F. (2016). PEGylation of chitosan via nitroxide-mediated polymerization in aqueous media. Macromol. React. Eng. 10, 82–89. doi: 10.1002/mren.201500024
da Silva, C. M., da Silva, D. L., Modolo, L. V., Alves, R. B., de Resende, M. A., Martins, C. V. B., et al. (2011). Schiff bases: a short review of their antimicrobial activities. J. Adv. Res. 2, 1–8. doi: 10.1016/j.jare.2010.05.004
Davidovich-Pinhas, M., Danin-Poleg, Y., Kashi, Y., and Bianco-Peled, H. (2014). Modified chitosan: A step toward improving the properties of antibacterial food packages. Food Packag. Shelf Life 1, 160–169. doi: 10.1016/j.fpsl.2014.01.007
Davis, M. E., and Brewster, M. E. (2004). Cyclodextrin-based pharmaceutics: past, present and future. Nat. Rev. Drug Discov. 3, 1023–1035. doi: 10.1038/nrd1576
Deng, J., Wang, L., Liu, L., and Yang, W. (2009). Developments and new applications of UV-induced surface graft polymerizations. Prog. Polym. Sci. 34, 156–193. doi: 10.1016/j.progpolymsci.2008.06.002
Deygen, I. M., and Kudryashova, E. V. (2016). New versatile approach for analysis of PEG content in conjugates and complexes with biomacromolecules based on FTIR spectroscopy. Colloids Surf. B Biointerfaces 141, 36–43. doi: 10.1016/j.colsurfb.2016.01.030
Du, J., and Hsieh, Y. L. (2007). PEGylation of chitosan for improved solubility and fiber formation via electrospinning. Cellulose 14, 543–552. doi: 10.1007/s10570-007-9122-3
Du, J., Zhou, Y., Wang, L., and Wang, Y. (2016). Effect of PEGylated chitosan as multifunctional stabilizer for deacetyl mycoepoxydience nanosuspension design and stability evaluation. Carbohydr. Polym. 153, 471–481. doi: 10.1016/j.carbpol.2016.08.002
Dubey, P., and Gopinath, P. (2016). PEGylated graphene oxide-based nanocomposite-grafted chitosan/polyvinyl alcohol nanofiber as an advanced antibacterial wound dressing. RSC Adv. 6, 69103–69116. doi: 10.1039/C6RA12192F
El-Sherbiny, I. M., and Smyth, H. D. (2010). Poly(ethylene glycol)–carboxymethyl chitosan-based pH-responsive hydrogels: photo-induced synthesis, characterization, swelling, and in vitro evaluation as potential drug carriers. Carbohydr. Res. 345, 2004–2012. doi: 10.1016/j.carres.2010.07.026
Eltahlawy, K., Gaffar, M., and Elrafie, S. (2006). Novel method for preparation of β-cyclodextrin/grafted chitosan and it's application. Carbohydr. Polym. 63, 385–392. doi: 10.1016/j.carbpol.2005.08.057
Esmaeili, A., and Ghobadianpour, S. (2016). Vancomycin loaded superparamagnetic MnFe2O4 nanoparticles coated with PEGylated chitosan to enhance antibacterial activity. Int. J. Pharm. 501, 326–330. doi: 10.1016/j.ijpharm.2016.02.013
Filipović-Grcić, J., Skalko-Basnet, N., and Jalsienjak, I. (2001). Mucoadhesive chitosan-coated liposomes: characteristics and stability. J. Microencapsul. 18, 3–12. doi: 10.1080/026520401750038557
Fillat, A., Gallardo, O., Vidal, T., Pastor, F. I. J., Díaz, P., and Roncero, M. B. (2012). Enzymatic grafting of natural phenols to flax fibres: Development of antimicrobial properties. Carbohydr. Polym. 87, 146–152. doi: 10.1016/j.carbpol.2011.07.030
Fu, D. J., Jin, Y., Xie, M. Q., Ye, Y. J., Qin, D. D., Lou, K. Y., et al. (2014). Preparation and characterization of mPEG grafted chitosan micelles as 5-fluorouracil carriers for effective anti-tumor activity. Chin. Chem. Lett. 25, 1435–1440. doi: 10.1016/j.cclet.2014.06.027
Garay, R. P., El-Gewely, R., Armstrong, J. K., Garratty, G., and Richette, P. (2012). Antibodies against polyethylene glycol in healthy subjects and in patients treated with PEG-conjugated agents. Expert Opin. Drug Deliv. 9, 1319–1323. doi: 10.1517/17425247.2012.720969
Garcia-Valdez, O., Darabi, A., Champagne, P., and Cunningham, M. (2015). PEGylation of chitosan via nitroxide chemistry in aqueous media. Polym. React. Eng. IX. Available online at: http://dc.engconfintl.org/polymer_rx_eng_IX/14
Glasing, J., Champagne, P., and Cunningham, M. F. (2016). Graft modification of chitosan, cellulose and alginate using reversible deactivation radical polymerization (RDRP). Curr. Opin. Green Sustain. Chem. 2, 15–21. doi: 10.1016/j.cogsc.2016.09.002
Gonil, P., Sajomsang, W., Ruktanonchai, U. R., Pimpha, N., Sramala, I., Nuchuchua, O., et al. (2011). Novel quaternized chitosan containing β-cyclodextrin moiety: Synthesis, characterization and antimicrobial activity. Carbohydr. Polym. 83, 905–913. doi: 10.1016/j.carbpol.2010.08.080
Goszczynska, A., Kwiecien, H., and Fijałkowski, K. (2015). Synthesis and antibacterial activity of Schiff bases and amines derived from alkyl 2-(2-formyl-4-nitrophenoxy)alkanoates. Med. Chem. Res. 24, 3561–3577. doi: 10.1007/s00044-015-1397-6
Han, J., Zhang, J., Yin, R., Ma, G., Yang, D., and Nie, J. (2011). Electrospinning of methoxy poly(ethylene glycol)-grafted chitosan and poly(ethylene oxide) blend aqueous solution. Carbohydr. Polym. 83, 270–276. doi: 10.1016/j.carbpol.2010.07.057
Harish Prashanth, K. V., and Tharanathan, R. N. (2007). Chitin/chitosan: modifications and their unlimited application potential—an overview. Trends Food Sci. Technol. 18, 117–131. doi: 10.1016/j.tifs.2006.10.022
Hassani Najafabadi, A., Abdouss, M., and Faghihi, S. (2014). Synthesis and evaluation of PEG-O-chitosan nanoparticles for delivery of poor water soluble drugs: Ibuprofen. Mater. Sci. Eng. C 41, 91–99. doi: 10.1016/j.msec.2014.04.035
Hauptstein, S., Bonengel, S., Griessinger, J., and Bernkop-Schnürch, A. (2014). Synthesis and characterization of ph tolerant and mucoadhesive (Thiol–Polyethylene Glycol) chitosan graft polymer for drug delivery. J. Pharm. Sci. 103, 594–601. doi: 10.1002/jps.23832
Hein, C. D., Liu, X. M., and Wang, D. (2008). Click chemistry, a powerful tool for pharmaceutical sciences. Pharm. Res. 25, 2216–2230. doi: 10.1007/s11095-008-9616-1
Hermanson, G. T. (ed.). (2013). “Zero-length crosslinkers,” in Bioconjugate Techniques (Elsevier), 259–273. doi: 10.1016/B978-0-12-382239-0.00004-2
Ho, T. H., Thanh Le, T. N., Nguyen, T. A., and Dang, M. C. (2015). Poly(ethylene glycol) grafted chitosan as new copolymer material for oral delivery of insulin. Adv. Nat. Sci. Nanosci. Nanotechnol. 6:035004. doi: 10.1088/2043-6262/6/3/035004
Hou, X., Zhang, W., He, M., Lu, Y., Lou, K., and Gao, F. (in press). Preparation characterization of β -cyclodextrin grafted N -maleoyl chitosan nanoparticles for drug delivery. Asian J. Pharm. Sci. doi: 10.1016/j.ajps.2017.07.007
Huang, S., Wang, L., Liu, L., Hou, Y., and Li, L. (2015). Nanotechnology in agriculture, livestock, and aquaculture in China. A review. Agron. Sustain. Dev. 35, 369–400. doi: 10.1007/s13593-014-0274-x
Ito, T., Yoshida, C., and Murakami, Y. (2013). Design of novel sheet-shaped chitosan hydrogel for wound healing: A hybrid biomaterial consisting of both PEG-grafted chitosan and crosslinkable polymeric micelles acting as drug containers. Mater. Sci. Eng. C 33, 3697–3703. doi: 10.1016/j.msec.2013.04.056
Jayakumar, R., Prabaharan, M., Reis, R. L., and Mano, J. F. (2005). Graft copolymerized chitosan—present status and applications. Carbohydr. Polym. 62, 142–158. doi: 10.1016/j.carbpol.2005.07.017
Jeong, Y. I., Jin, S. G., Kim, I. Y., Pei, J., Wen, M., Jung, T. Y., et al. (2010). Doxorubicin-incorporated nanoparticles composed of poly(ethylene glycol)-grafted carboxymethyl chitosan and antitumor activity against glioma cells in vitro. Colloids Surf. B Biointerfaces 79, 149–155. doi: 10.1016/j.colsurfb.2010.03.037
Jeong, Y. I., Kim, D. G., Jang, M. K., and Nah, J. W. (2008). Preparation and spectroscopic characterization of methoxy poly(ethylene glycol)-grafted water-soluble chitosan. Carbohydr. Res. 343, 282–289. doi: 10.1016/j.carres.2007.10.025
Ji, J., Wang, L., Yu, H., Chen, Y., Zhao, Y., Zhang, H., et al. (2014). Chemical modifications of chitosan and its applications. Polym. Plast. Technol. Eng. 53, 1494–1505. doi: 10.1080/03602559.2014.909486
Jiang, H. L., Kwon, J. T., Kim, E. M., Kim, Y. K., Arote, R., Jere, D., et al. (2008). Galactosylated poly(ethylene glycol)-chitosan-graft-polyethylenimine as a gene carrier for hepatocyte-targeting. J. Controlled Release 131, 150–157. doi: 10.1016/j.jconrel.2008.07.029
Jing, Z. W., Ma, Z. W., Li, C., Jia, Y. Y., Luo, M., Ma, X. X., et al. (2017). Chitosan cross-linked with poly(ethylene glycol)dialdehyde via reductive amination as effective controlled release carriers for oral protein drug delivery. Bioorg. Med. Chem. Lett. 27, 1003–1006. doi: 10.1016/j.bmcl.2016.12.072
Jokerst, J. V., Lobovkina, T., Zare, R. N., and Gambhir, S. S. (2011). Nanoparticle PEGylation for imaging and therapy. Nanomed. 6, 715–728. doi: 10.2217/nnm.11.19
Karaki, N., Aljawish, A., Humeau, C., Muniglia, L., and Jasniewski, J. (2016). Enzymatic modification of polysaccharides: mechanisms, properties, and potential applications: a review. Enzyme Microb. Technol. 90, 1–18. doi: 10.1016/j.enzmictec.2016.04.004
Kashyap, P. L., Xiang, X., and Heiden, P. (2015). Chitosan nanoparticle based delivery systems for sustainable agriculture. Int. J. Biol. Macromol. 77, 36–51. doi: 10.1016/j.ijbiomac.2015.02.039
Kim, J. H., and Kim, S. (2014). Phase transfer agent assisted biphasic CuAAC reaction. RSC Adv. 4, 26516–26523. doi: 10.1039/c4ra03356f
Kolb, H. C., Finn, M. G., and Sharpless, K. B. (2001). Click chemistry: diverse chemical function from a few good reactions. Angew. Chem. Int. Ed Engl. 40, 2004–2021. doi: 10.1002/1521-3773(20010601)40:11<2004::AID-ANIE2004>3.0.CO;2-5
Kolhe, P., and Kannan, R. M. (2003). Improvement in ductility of chitosan through blending and copolymerization with PEG: FTIR investigation of molecular interactions. Biomacromolecules 4, 173–180. doi: 10.1021/bm025689
Kono, H., and Teshirogi, T. (2015). Cyclodextrin-grafted chitosan hydrogels for controlled drug delivery. Int. J. Biol. Macromol. 72, 299–308. doi: 10.1016/j.ijbiomac.2014.08.030
Kulbokaite, R., Ciuta, G., Netopilik, M., and Makuska, R. (2009). N-PEG'ylation of chitosan via “click chemistry” reactions. React. Funct. Polym. 69, 771–778. doi: 10.1016/j.reactfunctpolym.2009.06.010
Kyzas, G. Z., and Bikiaris, D. (2015). Recent modifications of chitosan for adsorption applications: a critical and systematic review. Mar. Drugs 13, 312–337. doi: 10.3390/md13010312
Li, S., Xiong, Q., Lai, X., Li, X., Wan, M., Zhang, J., et al. (2016). Molecular modification of polysaccharides and resulting bioactivities: molecular modification of polysaccharides? Compr. Rev. Food Sci. Food Saf. 15, 237–250. doi: 10.1111/1541-4337.12161
Liang, Y., Deng, L., Chen, C., Zhang, J., Zhou, R., Li, X., et al. (2011). Preparation and properties of thermoreversible hydrogels based on methoxy poly(ethylene glycol)-grafted chitosan nanoparticles for drug delivery systems. Carbohydr. Polym. 83, 1828–1833. doi: 10.1016/j.carbpol.2010.10.048
Lin, W. J., and Hsu, W. Y. (2015). Pegylation effect of chitosan based polyplex on DNA transfection. Carbohydr. Polym. 120, 7–14. doi: 10.1016/j.carbpol.2014.11.046
Liu, G., Li, K., Luo, Q., Wang, H., and Zhang, Z. (2017). PEGylated chitosan protected silver nanoparticles as water-borne coating for leather with antibacterial property. J. Colloid Interface Sci. 490, 642–651. doi: 10.1016/j.jcis.2016.11.103
Liu, J., Liu, G., and Liu, W. (2014). Preparation of water-soluble β-cyclodextrin/poly(acrylic acid)/graphene oxide nanocomposites as new adsorbents to remove cationic dyes from aqueous solutions. Chem. Eng. J. 257, 299–308. doi: 10.1016/j.cej.2014.07.021
Liu, Y., Yu, Z. L., Zhang, Y. M., Guo, D. S., and Liu, Y. P. (2008). Supramolecular architectures of beta-cyclodextrin-modified chitosan and pyrene derivatives mediated by carbon nanotubes and their DNA condensation. J. Am. Chem. Soc. 130, 10431–10439. doi: 10.1021/ja802465g
Lu, L., Shao, X., Jiao, Y., and Zhou, C. (2014). Synthesis of chitosan-graft-β-cyclodextrin for improving the loading and release of doxorubicin in the nanopaticles. J. Appl. Polym. Sci. 131:41034. doi: 10.1002/app.41034
Luo, Q., Gao, H., Peng, L., Liu, G., and Zhang, Z. (2016). Synthesis of PEGylated chitosan copolymers as efficiently antimicrobial coatings for leather. J. Appl. Polym. Sci. 133:43465. doi: 10.1002/app.43465
Lv, X., Song, W., Ti, Y., Qu, L., Zhao, Z., and Zheng, H. (2013). Gamma radiation-induced grafting of acrylamide and dimethyl diallyl ammonium chloride onto starch. Carbohydr. Polym. 92, 388–393. doi: 10.1016/j.carbpol.2012.10.002
Malhotra, M., Lane, C., Tomaro-Duchesneau, C., Saha, S., and Prakash, S. (2011). A novel method for synthesizing PEGylated chitosan nanoparticles: strategy, preparation, and in vitro analysis. Int. J. Nanomedicine 6, 485–494. doi: 10.2147/IJN.S17190
Malhotra, M., Tomaro-Duchesneau, C., Saha, S., Kahouli, I., and Prakash, S. (2013). Development and characterization of chitosan-PEG-TAT nanoparticles for the intracellular delivery of siRNA. Int. J. Nanomedicine 8, 2041–2052. doi: 10.2147/IJN.S43683
Mao, S., Shuai, X., Unger, F., Wittmar, M., Xie, X., and Kissel, T. (2005). Synthesis, characterization and cytotoxicity of poly(ethylene glycol)-graft-trimethyl chitosan block copolymers. Biomaterials 26, 6343–6356. doi: 10.1016/j.biomaterials.2005.03.036
Martel, B., Devassine, M., Crini, G., Weltrowski, M., Bourdonneau, M., and Morcellet, M. (2001). Preparation and sorption properties of a β-cyclodextrin-linked chitosan derivative. J. Polym. Sci. Part Polym. Chem. 39, 169–176. doi: 10.1002/1099-0518(20010101)39:1<169::AID-POLA190>3.0.CO;2-G
Meléndez-Ortiz, H. I., and Bucio, E. (2015). “Synthesis, characterization, and uses of novel-architecture copolymers through gamma radiation technique,” in Advanced Functional Materials, eds A. Tiwari and L. Uzun (Hoboken, NJ: John Wiley & Sons, Inc.), 433–462.
Montalbetti, C. A. G. N., and Falque, V. (2005). Amide bond formation and peptide coupling. Tetrahedron 61, 10827–10852. doi: 10.1016/j.tet.2005.08.031
Mourya, V. K., and Inamdar, N. N. (2008). Chitosan-modifications and applications: opportunities galore. React. Funct. Polym. 68, 1013–1051. doi: 10.1016/j.reactfunctpolym.2008.03.002
Muftuoglu, A. E., Yagci, Y., and Kazunori, S. E. (2004). Photoinitiated crosslinking and grafting of methylmethacrylate using N,N-dimethyl amino functional polystyrene block copolymers. Turk. J. Chem. 28, 469–476.
Mura, P. (2014). Analytical techniques for characterization of cyclodextrin complexes in aqueous solution: a review. J. Pharm. Biomed. Anal. 101, 238–250. doi: 10.1016/j.jpba.2014.02.022
Najafabadi, A. H., Abdouss, M., and Faghihi, S. (2014). Preparation and characterization of PEGylated chitosan nanocapsules as a carrier for pharmaceutical application. J. Nanoparticle Res. 16:2312. doi: 10.1007/s11051-014-2312-7
Novoa-Carballal, R., Riguera, R., and Fernandez-Megia, E. (2013). Disclosing an NMR-invisible fraction in chitosan and PEGylated copolymers and its role on the determination of degrees of substitution. Mol. Pharm. 10, 3225–3231. doi: 10.1021/mp400267m
Nuchuchua, O., Saesoo, S., Sramala, I., Puttipipatkhachorn, S., Soottitantawat, A., and Ruktanonchai, U. (2009). Physicochemical investigation and molecular modeling of cyclodextrin complexation mechanism with eugenol. Food Res. Int. 42, 1178–1185. doi: 10.1016/j.foodres.2009.06.006
Nwe, K., and Brechbiel, M. W. (2009). Growing applications of “click chemistry” for bioconjugation in contemporary biomedical research. Cancer Biother. Radiopharm. 24, 289–302. doi: 10.1089/cbr.2008.0626
Olteanu, C. E. (2007). Applications of functionalized chitosan. Sci. Study Res. VIII 3. Available online at: http://www.pubs.ub.ro/dwnl.php?id=CSCC6200708V03S01A0001 (Accessed August 23, 2015).
Papadimitriou, S. A., Achilias, D. S., and Bikiaris, D. N. (2012). Chitosan-g-PEG nanoparticles ionically crosslinked with poly(glutamic acid) and tripolyphosphate as protein delivery systems. Int. J. Pharm. 430, 318–327. doi: 10.1016/j.ijpharm.2012.04.004
Pattabiraman, V. R., and Bode, J. W. (2011). Rethinking amide bond synthesis. Nature 480, 471–479. doi: 10.1038/nature10702
Pillay, V., Seedat, A., Choonara, Y. E., du Toit, L. C., Kumar, P., and Ndesendo, V. M. (2013). A review of polymeric refabrication techniques to modify polymer properties for biomedical and drug delivery applications. AAPS PharmSciTech 14, 692–711. doi: 10.1208/s12249-013-9955-z
Ping, Y., Liu, C., Zhang, Z., Liu, K. L., Chen, J., and Li, J. (2011). Chitosan-graft-(PEI-β-cyclodextrin) copolymers and their supramolecular PEGylation for DNA and siRNA delivery. Biomaterials 32, 8328–8341. doi: 10.1016/j.biomaterials.2011.07.038
Prabaharan, M., and Gong, S. (2008). Novel thiolated carboxymethyl chitosan-g-β-cyclodextrin as mucoadhesive hydrophobic drug delivery carriers. Carbohydr. Polym. 73, 117–125. doi: 10.1016/j.carbpol.2007.11.005
Prabaharan, M., and Jayakumar, R. (2009). Chitosan-graft-β-cyclodextrin scaffolds with controlled drug release capability for tissue engineering applications. Int. J. Biol. Macromol. 44, 320–325. doi: 10.1016/j.ijbiomac.2009.01.005
Prabaharan, M., and Mano, J. F. (2006). Chitosan derivatives bearing cyclodextrin cavitiesas novel adsorbent matrices. Carbohydr. Polym. 63, 153–166. doi: 10.1016/j.carbpol.2005.08.051
Prego, C., Torres, D., Fernandez-Megia, E., Novoa-Carballal, R., Quiñoá, E., and Alonso, M. J. (2006). Chitosan–PEG nanocapsules as new carriers for oral peptide delivery. J. Controlled Release 111, 299–308. doi: 10.1016/j.jconrel.2005.12.015
Ratner, B. D. (1980). Characterization of graft polymers for biomedical applications. J. Biomed. Mater. Res. 14, 665–687. doi: 10.1002/jbm.820140512
Roberts, M. J., Bentley, M. D., and Harris, J. M. (2012). Chemistry for peptide and protein PEGylation. Adv. Drug Deliv. Rev. 64(Suppl.), 116–127. doi: 10.1016/j.addr.2012.09.025
Sahariah, P., Árnadóttir, B., and Másson, M. (2016). Synthetic strategy for selective N-modified and O-modified PEGylated chitosan derivatives. Eur. Polym. J. 81, 53–63. doi: 10.1016/j.eurpolymj.2016.05.020
Sajomsang, W. (2010). Synthetic methods and applications of chitosan containing pyridylmethyl moiety and its quaternized derivatives: a review. Carbohydr. Polym. 80, 631–647. doi: 10.1016/j.carbpol.2009.12.037
Sajomsang, W., Nuchuchua, O., Gonil, P., Saesoo, S., Sramala, I., Soottitantawat, A., et al. (2012). Water-soluble β-cyclodextrin grafted with chitosan and its inclusion complex as a mucoadhesive eugenol carrier. Carbohydr. Polym. 89, 623–631. doi: 10.1016/j.carbpol.2012.03.060
Sapsford, K. E., Tyner, K. M., Dair, B. J., Deschamps, J. R., and Medintz, I. L. (2011). Analyzing nanomaterial bioconjugates: a review of current and emerging purification and characterization techniques. Anal. Chem. 83, 4453–4488. doi: 10.1021/ac200853a
Sashiwa, H., and Aiba, S. (2004). Chemically modified chitin and chitosan as biomaterials. Prog. Polym. Sci. 29, 887–908. doi: 10.1016/j.progpolymsci.2004.04.001
Schellekens, H., Hennink, W. E., and Brinks, V. (2013). The immunogenicity of polyethylene glycol: facts and fiction. Pharm. Res. 30, 1729–1734. doi: 10.1007/s11095-013-1067-7
Schiff, H. (1864). Mittheilungen aus dem Universitätslaboratorium in Pisa: Eine neue Reihe organischer Basen. Ann. Chem. Pharm. 131, 118–119. doi: 10.1002/jlac.18641310113
Sharma, A., Jyoti, K., Bansal, V., Jain, U. K., Bhushan, B., and Madan, J. (2017). Soluble telmisartan bearing poly (ethylene glycol) conjugated chitosan nanoparticles augmented drug delivery, cytotoxicity, apoptosis and cellular uptake in human cervical cancer cells. Mater. Sci. Eng. C 72, 69–76. doi: 10.1016/j.msec.2016.11.048
Sharma, S., and Rajesh, N. (2017). Expeditious preparation of β-cyclodextrin grafted chitosan using microwave radiation for the enhanced palladium adsorption from aqueous waste and an industrial catalyst. J. Environ. Chem. Eng. 5, 1927–1935. doi: 10.1016/j.jece.2017.03.015
Song, M., Li, L., Zhang, Y., Chen, K., Wang, H., and Gong, R. (2017). Carboxymethyl-β-cyclodextrin grafted chitosan nanoparticles as oral delivery carrier of protein drugs. React. Funct. Polym. 117, 10–15. doi: 10.1016/j.reactfunctpolym.2017.05.008
Sun, T., Xu, P., Liu, Q., Xue, J., and Xie, W. (2003). Graft copolymerization of methacrylic acid onto carboxymethyl chitosan. Eur. Polym. J. 39, 189–192. doi: 10.1016/S0014-3057(02)00174-X
Tan, H., Xue, Y., Luan, Q., and Yao, X. (2012). Evaluation of glycol chitosan-graft-carboxymethyl β-cyclodextrin as potential pH-sensitive anticancer drug carrier by surface plasmon resonance. Anal. Methods 4, 2784. doi: 10.1039/c2ay25295c
Termsarasab, U., Yoon, I. S., Park, J. H., Moon, H. T., Cho, H. J., and Kim, D. D. (2014). Polyethylene glycol-modified arachidyl chitosan-based nanoparticles for prolonged blood circulation of doxorubicin. Int. J. Pharm. 464, 127–134. doi: 10.1016/j.ijpharm.2014.01.015
Thakur, V. K., Thakur, M. K., and Gupta, R. K. (2014). Graft copolymers of natural fibers for green composites. Carbohydr. Polym. 104, 87–93. doi: 10.1016/j.carbpol.2014.01.016
Tsao, C. T., Hsiao, M. H., Zhang, M. Y., Levengood, S. L., and Zhang, M. (2015). Chitosan-PEG Hydrogel with Sol–Gel transition triggerable by multiple external stimuli. Macromol. Rapid Commun. 36, 332–338. doi: 10.1002/marc.201400586
Wallyn, S., Lammens, M., O'reilly, R. K., and Prez, F. D. (2011). Highly active, thermo-responsive polymeric catalytic system for reuse in aqueous and organic CuAAC reactions. J. Polym. Sci. Part Polym. Chem. 49, 2878–2885. doi: 10.1002/pola.24723
Wang, J. P., Chen, Y. Z., Ge, X. W., and Yu, H. Q. (2007). Gamma radiation-induced grafting of a cationic monomer onto chitosan as a flocculant. Chemosphere 66, 1752–1757. doi: 10.1016/j.chemosphere.2006.06.072
Wang, X., Colavita, P. E., Streifer, J. A., Butler, J. E., and Hamers, R. J. (2010). Photochemical grafting of alkenes onto carbon surfaces: identifying the roles of electrons and holes. J. Phys. Chem. C 114, 4067–4074. doi: 10.1021/jp911264n
Weidner, S. M., and Trimpin, S. (2010). Mass spectrometry of synthetic polymers. Anal. Chem. 82, 4811–4829. doi: 10.1021/ac101080n
Witono, J. R., Noordergraaf, I. W., Heeres, H. J., and Janssen, L. P. (2012). Graft copolymerization of acrylic acid to cassava starch—Evaluation of the influences of process parameters by an experimental design method. Carbohydr. Polym. 90, 1522–1529. doi: 10.1016/j.carbpol.2012.07.024
Xie, Y., Qiao, H., Su, Z., Chen, M., Ping, Q., and Sun, M. (2014). PEGylated carboxymethyl chitosan/calcium phosphate hybrid anionic nanoparticles mediated hTERT siRNA delivery for anticancer therapy. Biomaterials 35, 7978–7991. doi: 10.1016/j.biomaterials.2014.05.068
Yamaki, T., Asano, M., Maekawa, Y., Morita, Y., Suwa, T., Chen, J., et al. (2003). Radiation grafting of styrene into crosslinked PTEE films and subsequent sulfonation for fuel cell applications. Radiat. Phys. Chem. 67, 403–407. doi: 10.1016/S0969-806X(03)00075-6
Yang, C., Zhou, Y., Zheng, Y., Li, C., Sheng, S., Wang, J., et al. (2016). Enzymatic modification of chitosan by cinnamic acids: Antibacterial activity against Ralstonia solanacearum. Int. J. Biol. Macromol. 87, 577–585. doi: 10.1016/j.ijbiomac.2016.03.023
Yang, Q., Wang, L., Xiang, W., Zhou, J., and Li, J. (2007). Grafting polymers onto carbon black surface by trapping polymer radicals. Polymer 48, 2866–2873. doi: 10.1016/j.polymer.2007.01.074
Yao, K., Li, J., Yao, F., and Yin, Y. (2011). Chitosan-Based Hydrogels: Functions and Applications. London: CRC Press.
Yuan, Z., Ye, Y., Gao, F., Yuan, H., Lan, M., Lou, K., et al. (2013). Chitosan-graft-β-cyclodextrin nanoparticles as a carrier for controlled drug release. Int. J. Pharm. 446, 191–198. doi: 10.1016/j.ijpharm.2013.02.024
Zajac, A., Hanuza, J., Wandas, M., and Dyminska, L. (2015). Determination of N-acetylation degree in chitosan using Raman spectroscopy. Spectrochim. Acta. A. Mol. Biomol. Spectrosc. 134, 114–120. doi: 10.1016/j.saa.2014.06.071
Zhang, L., Zhao, Z. L., Wei, X. H., and Liu, J. H. (2013). Preparation and in vitro and in vivo characterization of cyclosporin A-loaded, PEGylated chitosan-modified, lipid-based nanoparticles. Int. J. Nanomedicine 8, 601–610. doi: 10.2147/IJN.S39685
Zhang, X. (2008). Nasal absorption enhancement of insulin using PEG-grafted chitosan nanoparticles. Eur. J. Pharm. Biopharm. 68, 526–534. doi: 10.1016/j.ejpb.2007.08.009
Zhang, X., Yao, J., Zhang, L., Fang, J., and Bian, F. (2014). Synthesis and characterization of PEG-conjugated quaternized chitosan and its application as a gene vector. Carbohydr. Polym. 103, 566–572. doi: 10.1016/j.carbpol.2013.12.072
Zhang, Y., Guo, Z., Ye, J., Xu, Q., Liang, X., and Lei, A. (2008). Preparation of novel beta-cyclodextrin chiral stationary phase based on click chemistry. J. Chromatogr. A 1191, 188–192. doi: 10.1016/j.chroma.2007.11.018
Keywords: chitosan, poly(ethylene glycol), grafting, copolymerization
Citation: Campos EVR, Oliveira JL and Fraceto LF (2017) Poly(ethylene glycol) and Cyclodextrin-Grafted Chitosan: From Methodologies to Preparation and Potential Biotechnological Applications. Front. Chem. 5:93. doi: 10.3389/fchem.2017.00093
Received: 27 June 2017; Accepted: 24 October 2017;
Published: 07 November 2017.
Edited by:
Giuseppe Mensitieri, University of Naples Federico II, ItalyReviewed by:
Daisuke Takeuchi, Tokyo Institute of Technology, JapanMessina Maria Grazia, Università degli Studi di Catania, Italy
Copyright © 2017 Campos, Oliveira and Fraceto. This is an open-access article distributed under the terms of the Creative Commons Attribution License (CC BY). The use, distribution or reproduction in other forums is permitted, provided the original author(s) or licensor are credited and that the original publication in this journal is cited, in accordance with accepted academic practice. No use, distribution or reproduction is permitted which does not comply with these terms.
*Correspondence: Leonardo F. Fraceto, bGVvbmFyZG9Ac29yb2NhYmEudW5lc3AuYnI=