- 1Division of Bioinformatics and Computational Biology, Department of Medical Informatics and Clinical Epidemiology, Oregon Health & Science University, Portland, OR, USA
- 2Department of Molecular and Medical Genetics, Oregon Health & Science University, Portland, OR, USA
- 3Division of Reproductive & Developmental Sciences, Oregon National Primate Research Center, Portland, OR, USA
- 4Department of Medicine, Knight Cardiovascular Institute, Oregon Health & Science University, Portland, OR, USA
- 5Departments of Obstetrics and Gynecology and Physiology and Pharmacology, Oregon Health & Science University School of Medicine, Portland, OR, USA
Mammalian genomes are scattered with thousands of copies of endogenous retroviruses (ERVs), mobile genetic elements that are relics of ancient retroviral infections. After inserting copies into the germ line of a host, most ERVs accumulate mutations that prevent the normal assembly of infectious viral particles, becoming trapped in host genomes and unable to leave to infect other cells. While most copies of ERVs are inactive, some are transcribed and encode the proteins needed to generate new insertions at novel loci. In some cases, old copies are removed via recombination and other mechanisms. This creates a shifting landscape of ERV copies within host genomes. New insertions can disrupt normal expression of nearby genes via directly inserting into key regulatory elements or by containing regulatory motifs within their sequences. Further, the transcriptional silencing of ERVs via epigenetic modification may result in changes to the epigenetic regulation of adjacent genes. In these ways, ERVs can be potent sources of regulatory disruption as well as genetic innovation. Here, we provide a brief review of the association between ERVs and gene expression, especially as observed in pre-implantation development and placentation. Moreover, we will describe how disruption of the regulated mechanisms of ERVs may impact somatic tissues, mostly in the context of human disease, including cancer, neurodegenerative disorders, and schizophrenia. Lastly, we discuss the recent discovery that some ERVs may have been pressed into the service of their host genomes to aid in the innate immune response to exogenous viral infections.
Background
A retroviral genome exists in different forms during its replication cycle. A viral particle, or virion, protects the RNA genome of the retrovirus during escape from the host cell and infection of new cells. A virion that enters a new host cell deploys its genomic payload, using its own reverse transcriptase to convert the RNA viral genome into a DNA copy which is integrated into the host genome, referred to as a provirus (Figure 1). Subsequently, a provirus can be transcribed into RNA again, and then translated by the host's ribosomal machinery to produce more virions. Ancient retroviral infections have occasionally resulted in such integrations into the germline of the host, becoming endogenous retroviruses (ERVs). While some ERVs have been shown to produce infectious particles (van der Laan et al., 2000), most ERV copies suffer mutations over evolutionary time that prevent the normal assembly of viral particles, preventing horizontal transmission of infections between individuals. However, while now trapped within the host genome, some of these provirus copies are still transcribed and can encode some if not all of the original viral proteins. Therefore, ERVs are classified as a family of autonomous retrotransposons. Further, offspring of the host can inherit any germline ERV insertions from their parents, resulting in a vertical transmission pattern with evolution (Figure 2). As much as 8% of the human genome consists of ERV sequences acquired through repeated endogenization events followed by subsequent retrotranspositional expansion of captured viral subfamilies.
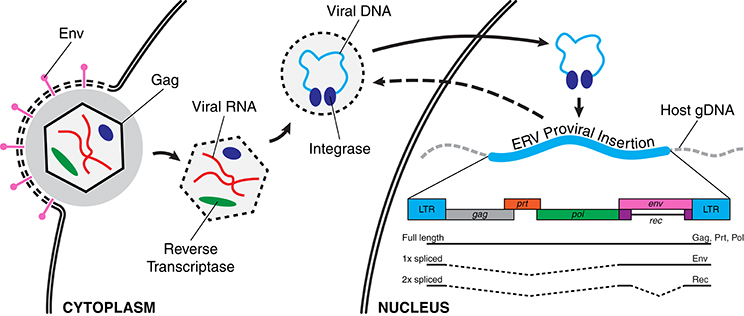
Figure 1. Retroviral infection and integration into host genome. Left to right: An infecting viral particle enters the host cell after its envelope, containing Env proteins (pink), fuses with the cell membrane. The viral capsid (hexagon), consisting largely of Gag proteins, contains the RNA form of the retroviral genome (red) as well as a reverse transcriptase (green). The viral genome is subsequently reverse transcribed into its DNA complement (light blue) and this viral genome then enters the nucleus with its associated integrase proteins (dark blue). A new viral integration is then inserted into the host genome, becoming a provirus. Lower right: A schematic of a retroviral genome with components indicated as colored boxes (gag, group-specific antigen; prt, protease; pol, polymerase; env, envelope protein; rec, accessory protein; LTR, long terminal repeat). Three splice variant transcripts are shown and their translated products given.
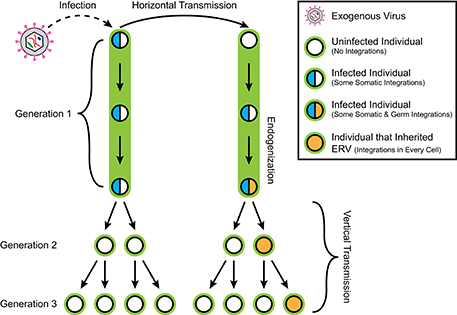
Figure 2. Retroviral infection, horizontal transmission, endogenization, and vertical transmission. An exogenous retrovirus infects an individual in generation 1, resulting in their accruing provirus integrations in some somatic cells. Horizontal transmission of the virus from the first individual to the second results in the second accruing somatic integrations as well. However, the second individual subsequently receives germline integrations. The descendants of the first individual do not inherit any retroviral integrations, while any germline integrations in the second individual are transmitted vertically to half of its descendants as endogenous retrovirus insertions present in every cell. Only half of the descendants of this second individual in Generation 2 inherit any given germline integration locus because any cell receiving a new integration does so on only one copy of the affected chromosome. This results in a heterozygous pattern of inheritance.
These ancient genomic residents represent a potent source of genomic and regulatory variability. The high degree of homology between these ERV copies, and the presence of the long terminal repeats (LTRs) at either end of each copy (Figure 1), provide an opportunity for non-allelic homologous recombination that can result in the excision of a given insertion, leaving behind only a single LTR copy. Recombination events between the different insertions of the same or similar ERV subfamilies can result in deletions, duplications, and other rearrangements of intervening genomic sequences. Additionally, the ERV sequences themselves can contain motifs that can disrupt or modulate nearby genes and regulatory regions. Not surprisingly, ERVs activity is associated with a number of human diseases and the target of epigenetic repression by the host genome. However, the consequences are not solely deleterious, as there is evidence that ERVs have been co-opted into important regulatory and developmental roles as well.
ERVs in Germ Cells and Pre-Implantation Embryos
Certain stages of mammalian pre-implantation embryo and germ cell development characterized by multiple waves of epigenetic reprogramming pose a unique challenge for the control of endogenous retroviral activity. During the two waves of epigenetic reprogramming that occur in primordial germ cells (PGCs) and fertilized oocytes, a considerable amount of DNA demethylation occurs. Examination of global DNA methylation at these stages have shown that levels within human and mouse pre-implantation embryos decrease beginning at the 1- to 2-cell stage, depending on the species, and up to or soon after the blastocyst stage (Kobayashi et al., 2012; Guo et al., 2014; Lee et al., 2014; Okae et al., 2014; Wang L. et al., 2014). Since DNA methylation is largely responsible for repression of many transposable elements, including ERVs (Walsh et al., 1998), the activity of ERVs and the alternative mechanisms repressing ERV activation during these periods of global hypomethylation have been the focus of a number of recent investigations.
Given that some ERV families have expanded substantially in the number of genomic integrations in animals (Tristem, 2000; Bénit et al., 2001), it has been hypothesized that widespread reactivation of ERVs during the waves of global reprogramming within germ cell and pre-implantation development are largely responsible for this expansion. On the other hand, it is also known that additional ERV repressive mechanisms must be in place in order to maintain genomic stability throughout epigenetic reprogramming and the highly choreographed molecular processes required for normal germ cell development, fertilization, and embryonic development. These ideas are not mutually exclusive, as there is substantial evidence supporting both reactivation (Fuchs et al., 2013; Wang J. et al., 2014; Grow et al., 2015) and alternative repression (Thomas and Schneider, 2011; Manghera and Douville, 2013; Leung et al., 2014; Liu et al., 2014; Schlesinger and Goff, 2015; Wolf et al., 2015; Thompson et al., 2016) across the vast number and variety of ERVs within the genome during germ cell development and embryogenesis.
Despite the existence of elaborate mechanisms that mediate ERV inactivation within the genome, there is extensive evidence that some ERVs are still active and play an important role during gametogenesis and pre-implantation development. Upregulation of ERV proviral transcription and protein expression has been well documented in early human embryos and embryonic stem cells (hESCs). For example, elevated expression of the ERV-H family has been observed within both naïve-like and primed hESC sub-populations (Wang J. et al., 2014; Theunissen et al., 2016; Supplementary Table 1). Additional transcripts from the ERV-K (HML-2) family are also observed at high levels within hESCs and rapidly decrease upon differentiation (Fuchs et al., 2013). Expression of ERV-K begins at the 8-cell stage, concurrent with embryonic genome activation (EGA), and continues throughout pre-implantation development into the blastocyst stage. A majority of actively transcribed ERV-K loci during this time are associated with LTR5HS, a specific subclass of LTR, which is confined to human and chimpanzee and contains an OCT4 binding motif. The LTR5HS subclass requires both hypomethylation and OCT4 binding for transcriptional activation, which synergistically facilitated ERV-K expression (Grow et al., 2015; Supplementary Table 1). Based on the elevated activity of these ERVs within hESCs and pre-implantation embryos, as well as their known interactions with other cellular factors during this time, it is thought that these ERVs have been functionally incorporated into roles important for defining and maintaining pluripotent specific states.
The role of LTRs as regulatory regions for proviral DNA represents an additional function that can be utilized by or incorporated into host genomes. In particular, LTRs are known to be co-opted as promoters or enhancer elements of nearby genes important during embryonic development and maintenance of pluripotency (Friedli and Trono, 2015). Nearly, ~33% of all transcripts in human embryonic tissues are associated with repetitive elements, suggesting a clear pattern of embryonic cell specificity for viral promoters (Fort et al., 2014). Many transcripts detected in the totipotent blastomeres of mouse 2-cell embryos are initiated from LTRs upon EGA as well, indicating that these repeat sequences may help drive cell-fate regulation in mammals (MacFarlan et al., 2012). Regulatory activities of certain LTRs have also been shown to provide important functions not only in embryonic cells, but also within germ cells during gametogenesis. For example, germline-specific transactivating p63 (GTAp63), a member of the p53 family and a transcript important for maintaining genetic fidelity in the human male germline, is under the transcriptional control of ERV9 LTR (Ling et al., 2002; Beyer et al., 2011; Liu and Eiden, 2011; Supplementary Table 1). Transcriptionally active GTAp63 suppresses proliferation and induces apoptosis upon DNA damage in healthy testis and is frequently lost in human testicular cancers. Restoration of GTAp63 expression levels in cancer cells was observed upon treatment with a histone deacetylase (HDAC) inhibitor, indicating possible epigenetic control of ERV9-mediated GTAp63 expression via activating histone acetylation marks. Thus, the ability of ERV9 regulatory regions to contribute to the maintenance of male germline stability is yet another example of how ERVs have evolved to serve an important function in their human hosts (Liu and Eiden, 2011).
ERVs in the Placenta
The placenta is a transient organ representing the maternal-fetal interface during pregnancy; it is derived from the outer trophectoderm (TE) layer of blastocysts, and plays a critical role in the gas, nutrient, and waste exchange required for normal embryonic growth. It is well established that both mouse and human placentas are hypomethylated compared to other somatic cells derived from either in vivo or in vitro sources (Ehrlich et al., 1982; Fuke et al., 2004; Cotton et al., 2009; Popp et al., 2010; Hon et al., 2013). As such, the DNA methylation levels of LTRs within human placentas more closely resemble that observed in oocytes than in somatic tissues, averaging ~60% methylation across the genome (Schroeder et al., 2015). Given this hypomethylation of LTRs in placentas, it is not surprising that numerous sub-families of ERV proviruses are expressed within human placental tissues. More specifically, there is evidence of proviral transcription from ERV-E (Yi and Kim, 2007), ERV3 (ERV-R; Boyd et al., 1993; Andersson et al., 2005), ERV-K (Kammerer et al., 2011), ERV-fb1 (Sugimoto et al., 2013), ERV-V1/2 (Esnault et al., 2013), ERV-W (Blond et al., 2000), and ERV-FRD (Blaise et al., 2003; Supplementary Tables 1, 2).
The most notable ERV families producing functional proteins during placentation are ERV-W and ERV-FRD, corresponding to Syncytin-1 and Syncytin-2, respectively, which are critical for the cellular fusion underlying human placental syncytia formation and maintenance (Blond et al., 2000; Mi et al., 2000; Blaise et al., 2003, 2005; Dunk et al., 2012; Supplementary Table 2). Cellular fusion is a relatively unique function in normal healthy tissues, with muscle, bone and placenta being the major exceptions. Since regulation of this highly specified function is of much interest, the precise mechanisms underlying the transcriptional control of the Syncytin-1 gene have been the topic of several investigations. Both DNA and histone H3K9 methylation have been reported to be important for inactivating ERV-W and thus repressing Syncytin-1 expression, resulting in pathological conditions such as exogenous viral infections and preeclampsia when repression does not occur (Matousková et al., 2006; Gimenez et al., 2009; Li et al., 2014; Zhuang et al., 2014). It has been shown that transcriptional activation of the ERV-W locus and the promotion of cell fusion also requires the synergism of LTR promoter hypomethylation, along with the binding of several transcription factors such as GCM1, Sp1, and GATA family members (Yu et al., 2002; Cheng et al., 2004; Prudhomme et al., 2004; Cheng and Handwerger, 2005; Chang et al., 2011). Recently, another ERV-derived protein called suppressyn has been identified to alternatively regulate Syncytin-1, but not Syncytin-2-based cell fusion by inhibiting its interaction with the Syncytin-1 associated receptor, ASCT2 (Sugimoto et al., 2013; Supplementary Table 2). Suppressyn is a truncation product of the proviral env gene from the ERV-fb1 element and is transcribed within the placenta. Within normal human placentas, suppressyn is co-expressed with Syncytin-1 in the syncytiotrophoblast layer (Sugimoto et al., 2013), further supporting that these two factors are involved in cell-cell fusion regulation at the maternal-fetal interface in utero.
Notably, integration of ERV-W and ERV-FRD into the genome occurred prior to the divergence of Old World (Catarrhini; Cáceres et al., 2006) and New World (Platyrrhini) monkeys (Blaise et al., 2003), respectively, thus Syncytin-1 and Syncytin-2 are only present in higher-order primate (Haplorhini) species, although functionally similar yet distinct ERV proviral proteins have been discovered throughout most mammalian genomes, as reviewed in Imakawa et al. (2015). The ERV-V env gene present within Old World monkeys has also been implicated in trophoblast fusion activity, possibly alleviating the lack of functional Syncytin-1 within these species, while the ERV-V reiterations present within the human genome are not functional in this capacity (Esnault et al., 2013; Supplementary Table 2). Syncytin-A and Syncytin-B appear to function like human Syncytins within the mouse placenta and are known to have entered the murine (Muridae) lineages approximately 20 million years ago (Dupressoir et al., 2005). Similarly, Syncytin-Ory1 has been discovered in rabbits and hares (Leporidae; Heidmann et al., 2009), Syncytin-Car1 within 26 different species of carnivorans (Carnivora; Cornelis et al., 2012), Syncytin-Mar1 within the squirrel-related clade (either Scuridae or Marmotini; Redelsperger et al., 2014), Syncytin-Ten1 within tenrec (Tenrecidae; Cornelis et al., 2014), Syncytin-Rum1 in ruminants (Ruminantia; Cornelis et al., 2013), and Syncytin-Opo1 within the short-lived placenta of opossum and kangaroo marsupials (Marsupialia; Cornelis et al., 2015).
Several ERV captured env genes have been proposed to have an immunosuppressive role that is important for preventing maternal rejection of the semi-allogenic fetus during pregnancy. In addition to fusogenic properties derived from the env gene of ERV-FRD, Syncytin-2 contains a classical Env retroviral immunosuppressive domain that has been shown to have immunosuppressive activity via in vitro tumor-rejection assay (Mangeney et al., 2007). Given observed protein expression within cytotrophoblasts cells of the human placenta, Syncytin-2 has been suggested to facilitate fetal tolerance by suppressing the maternal immune system. Other ERV-derived env proteins from ERV-V and ERV-K have also been proposed to possess an immunosuppressive role in controlling the maternal immune system during pregnancy. This is based on findings that both families have one or more proviral loci in the genome with intact env open reading frames (ORFs) and a corresponding immunosuppressive domain. Additionally, both ERV-V and ERV-K expression has been observed within placental trophoblast cells at the maternal-fetal interface, although corresponding in vitro functional assays have not yet been completed to directly support in vivo findings (Kammerer et al., 2011; Subramanian et al., 2011; Supplementary Table 1). Until these studies are undertaken, the exact function of ERV-V and ERV-K and whether env protein expression from these ERVs induce maternal immunosuppression within the placenta, will remain unknown.
ERVs and Human Disease
Through insertional mutagenesis, recombination between homologous copies, and the regulatory disruption that epigenetic suppression of ERV insertions can cause to nearby gene loci, there are many mechanisms by which these elements might cause disease. In particular, their association with various cancers has been well demonstrated, as reviewed in Katoh and Kurata (2013). For instance, ERV activity has been strongly associated with many breast cancers (Golan et al., 2008; Wang-Johanning et al., 2008; Salmons et al., 2014). While in melanoma tissues, ERV-K expression of both RNA and protein has been shown (Büscher et al., 2005), and one recent study identified 24 ERV-K (HML-2) loci transcribed (Schmitt et al., 2013). In another study of Hodgkin's lymphoma, all cancer patient samples were found to have alternative transcripts of the CSF1R, an important locus associated with this cancer, that initiate at the LTR of an ERV located ~6.2 kb upstream of the normal promoter (Lamprecht et al., 2010).
ERVs have been demonstrated to be associated with a variety of neurologic diseases, as reviewed in Douville and Nath (2014). One such disease is amyotrophic lateral sclerosis (ALS). Elevated ERV-K (HML-2) activity has been observed in the brain tissue of ALS patients (Douville et al., 2011), while transgenic animals expressing the ERV-K env gene in cortical and spinal neurons developed motor dysfunction, suggesting that these elements may contribute to neurodegeneration (Li et al., 2015). Additionally, the expression of ERV-W env and gag has been observed in samples of muscle from ALS patients (Oluwole et al., 2007). While the ERV-W findings may be due to the inflammatory response (Alfahad and Nath, 2013), the support for the involvement of ERV-K in ALS is mounting, though causality has yet to be demonstrated. Multiple sclerosis (MS) is another neurological disease in which ERVs have been strongly implicated. MSRV (multiple sclerosis-associated retrovirus), a subtype of ERV-W, as well as ERV-W1 and W2 and ERV-H/F have all been linked to MS (reviewed in Christensen, 2016). One study showed significantly elevated Env antigen in serum of MS patients relative to controls, while qPCR of ERV-W in mononuclear cells from blood (PBMC) showed association with MS relative to controls (Perron et al., 2012a). This same study demonstrated Env expression in eight well-characterized MS brains that had lesions throughout the parenchyma and in perivascular infiltrates, as well as at the rim of chronic active lesions. ERV association with schizophrenia and bipolar disorder has been demonstrated through the presence of biomarkers for ERV-K and ERV-W found in blood, cerebrospinal fluid, and the pre-frontal cortex (Karlsson et al., 2001; Huang et al., 2006, 2011; Perron et al., 2012b). In one study of schizophrenia, hypermethylation of a specific ERV-W LTR insertion located in the regulatory region of the GABBR1 gene was associated with risk of schizophrenia (Hegyi, 2013). A nearly full-length ERV-K insertion near the PRODH gene, known to be associated with schizophrenia and other neuropsychiatric disorders, has been shown to work in concert with the internal PRODH CpG island to activate the gene. It is thought that aberrant DNA methylation of this locus may be a piece of the schizophrenia puzzle (Suntsova et al., 2013).
ERVs May Play a Role in the Innate Immune Response
While the majority of ERV proviruses have acquired mutations, thereby preventing translation into protein, certain families have been especially well preserved and contain functional ORFs for one or more of the classical proviral genes. Within primates, ERV-K (HML-2) represents the best-preserved and most recently active ERV, containing a substantial number of loci that have predicted coding potential throughout different primate genomes. It has also been observed that ERV-K encodes a small accessory protein, Rec, in naïve ES cells and human blastocysts. Overexpression of Rec protein within human pluripotent cells increases the innate antiviral response and can inhibit exogenous viral infections, suggesting an immunoprotective role of the ERV-K Rec protein during early embryonic development (Grow et al., 2015; Supplementary Table 1). An additional ERV-K proviral protein, gag, which makes up the core of viral particles in exogenous retroviruses, is also expressed within human blastocysts and pluripotent cells. Immunolabeling of ERV-K gag protein followed by confocal and transmission electron microscopy revealed ERV-K gag protein within structures of blastocysts resembling viral-like particle (VLPs). This suggested that some ERV proviral sequences within the human genome still retain the ability to code for viral proteins and form VLPs during normal human embryogenesis. Proteins produced from ERV env genes have also been demonstrated to function as restriction factors against exogenous retroviral infection (Malfavon-Borja and Feschotte, 2015).
Even ERV proviruses that do not contain functional ORFs can still harbor sequence motifs that serve to modulate the activity of nearby genes. For instance, interferon (IFN)-inducible enhancers have been dispersed via ERV insertions adjacent to IFN-inducible genes independently over mammalian evolution. This has resulted in regulatory networks of genes able to work in concert due to the presence of these ERV sequences. Further, CRISPR-Cas9 deletion of a MER41 insertion upstream of AIM2 in HeLa cells disrupted the endogenous IFNG-inducible regulation of this locus, demonstrating the utility that host genomes can obtain over time by harnessing ERV sequences (Chuong et al., 2016). In another example showing the variety of mechanisms by which ERVs are involved with innate immunity, Chiappinelli et al. (2015) demonstrated that induction of ERV expression, and especially bidirectional transcription of ERVs, activated a double-stranded RNA sensing pathway that triggers a type I interferon response and apoptosis.
Conclusions
The relationship between ERVs and the human genome is a diverse and complicated one, resulting from millions of years of co-evolution. ERVs are known to be involved in disease through insertional mutagenesis, as targets of epigenetic repression, and via recombination of sequences between the homologous copies of these elements scattered across the genome. Throughout mammalian evolution, the deleterious effects of ERVs have been balanced by the benefits gained from innovative co-option of their sequences and proteins by their host genomes. These innovations include the intimate relationship between ERV activity with embryonic and placental development, as well as a number of ERV-associated regulatory networks that have become important components of the normal function of our genome. An innate immune response to exogenous retroviral infection is likely only one of several ERV functional roles. Once thought to have been quiescent, dead residents of the human genome, we are only beginning to uncover the scope of how actively intertwined our biology is with these long-time genomic partners.
Author Contributions
TM and JR drafted the manuscript and figures. SC and LC edited the manuscript.
Funding
TM was supported by the National Library of Medicine of the National Institutes of Health under Award Number T15LM007088. JR was supported by the Collins Medical Trust Foundation and Glenn/AFAR Scholarship for Research in the Biology of Aging. LC and SC were supported by NIH/NICHD R01HD086073-A1, National Centers for Translational Research in Reproduction and Infertility (NCTRI). Additional funding for SC came from the Georgeanna Jones Foundation for Reproductive Medicine, Medical Research Foundation of Oregon, and the Collins Medical Trust. The content is solely the responsibility of the authors and does not necessarily represent the official views of the National Institutes of Health, the Collins Medical Trust Foundation, or Medical Research Foundation of Oregon.
Conflict of Interest Statement
The authors declare that the research was conducted in the absence of any commercial or financial relationships that could be construed as a potential conflict of interest.
Supplementary Material
The Supplementary Material for this article can be found online at: http://journal.frontiersin.org/article/10.3389/fchem.2017.00023/full#supplementary-material
References
Alfahad, T., and Nath, A. (2013). Retroviruses and amyotrophic lateral sclerosis. Antiviral Res. 99, 180–187. doi: 10.1016/j.antiviral.2013.05.006
Andersson, A. C., Yun, Z., Sperber, G. O., Larsson, E., and Blomberg, J. (2005). ERV3 and related sequences in humans: structure and RNA expression. J. Virol. 79, 9270–9284. doi: 10.1128/JVI.79.14.9270-9284.2005
Bénit, L., Dessen, P., and Heidmann, T. (2001). Identification, phylogeny, and evolution of retroviral elements based on their envelope genes. J. Virol. 75, 11709–11719. doi: 10.1128/JVI.75.23.11709-11719.2001
Beyer, U., Moll-Rocek, J., Moll, U. M., and Dobbelstein, M. (2011). Endogenous retrovirus drives hitherto unknown proapoptotic p63 isoforms in the male germ line of humans and great apes. Proc. Natl. Acad. Sci. U.S.A. 108, 3624–3629. doi: 10.1073/pnas.1016201108
Blaise, S., de Parseval, N., and Heidmann, T. (2005). Functional characterization of two newly identified human endogenous retrovirus coding envelope genes. Retrovirology 2:19. doi: 10.1186/1742-4690-2-19
Blaise, S., de Parseval, N., Bénit, L., and Heidmann, T. (2003). Genomewide screening for fusogenic human endogenous retrovirus envelopes identifies syncytin 2, a gene conserved on primate evolution. Proc. Natl. Acad. Sci. U.S.A. 100, 13013–13018. doi: 10.1073/pnas.2132646100
Blond, J. L., Lavillette, D., Cheynet, V., Bouton, O., Oriol, G., Chapel-Fernandes, S., et al. (2000). An envelope glycoprotein of the human endogenous retrovirus HERV-W is expressed in the human placenta and fuses cells expressing the type D mammalian retrovirus receptor. J. Virol. 74, 3321–3329. doi: 10.1128/JVI.74.7.3321-3329.2000
Boyd, M. T., Bax, C. M., Bax, B. E., Bloxam, D. L., and Weiss, R. A. (1993). The human endogenous retrovirus ERV-3 is upregulated in differentiating placental trophoblast cells. Virology 196, 905–909. doi: 10.1006/viro.1993.1556
Büscher, K., Trefzer, U., Hofmann, M., Sterry, W., Kurth, R., and Denner, J. (2005). Expression of human endogenous retrovirus K in melanomas and melanoma cell lines. Cancer Res. 65, 4172–4180. doi: 10.1158/0008-5472.CAN-04-2983
Cáceres, M., NISC Comparative Sequencing Program, and Thomas, J. W. (2006). The gene of retroviral origin Syncytin 1 is specific to hominoids and is inactive in old world monkeys. J. Hered. 97, 100–106. doi: 10.1093/jhered/esj011
Chang, C. W., Chang, G. D., and Chen, H. (2011). A novel cyclic AMP/Epac1/CaMKI signaling cascade promotes GCM1 desumoylation and placental cell fusion. Mol. Cell. Biol. 31, 3820–3831. doi: 10.1128/MCB.05582-11
Cheng, Y. H., and Handwerger, S. (2005). A placenta-specific enhancer of the human syncytin gene. Biol. Reprod. 73, 500–509. doi: 10.1095/biolreprod.105.039941
Cheng, Y. H., Richardson, B. D., Hubert, M. A., and Handwerger, S. (2004). Isolation and characterization of the human syncytin gene promoter. Biol. Reprod. 70, 694–701. doi: 10.1095/biolreprod.103.023473
Chiappinelli, K. B., Strissel, P. L., Desrichard, A., Li, H., Henke, C., Akman, B., et al. (2015). Inhibiting DNA methylation causes an interferon response in cancer via dsRNA including endogenous retroviruses. Cell 162, 974–986. doi: 10.1016/j.cell.2015.07.011
Christensen, T. (2016). Human endogenous retroviruses in neurologic disease. APMIS 124, 116–126. doi: 10.1111/apm.12486
Chuong, E. B., Elde, N. C., and Feschotte, C. (2016). Regulatory evolution of innate immunity through co-option of endogenous retroviruses. Science 351, 1083–1087. doi: 10.1126/science.aad5497
Cornelis, G., Heidmann, O., Bernard-Stoecklin, S., Reynaud, K., Véron, G., Mulot, B., et al. (2012). Ancestral capture of syncytin-Car1, a fusogenic endogenous retroviral envelope gene involved in placentation and conserved in Carnivora. Proc. Natl. Acad. Sci. U.S.A. 109, E432–E441. doi: 10.1073/pnas.1115346109
Cornelis, G., Heidmann, O., Degrelle, S. A., Vernochet, C., Lavialle, C., Letzelter, C., et al. (2013). Captured retroviral envelope syncytin gene associated with the unique placental structure of higher ruminants. Proc. Natl. Acad. Sci. U.S.A. 110, E828–E837. doi: 10.1073/pnas.1215787110
Cornelis, G., Vernochet, C., Carradec, Q., Souquere, S., Mulot, B., Catzeflis, F., et al. (2015). Retroviral envelope gene captures and syncytin exaptation for placentation in marsupials. Proc. Natl. Acad. Sci. U.S.A. 112, E487–E496. doi: 10.1073/pnas.1417000112
Cornelis, G., Vernochet, C., Malicorne, S., Souquere, S., Tzika, A. C., Goodman, S. M., et al. (2014). Retroviral envelope syncytin capture in an ancestrally diverged mammalian clade for placentation in the primitive Afrotherian tenrecs. Proc. Natl. Acad. Sci. U.S.A. 111, E4332–E4341. doi: 10.1073/pnas.1412268111
Cotton, A. M., Avila, L., Penaherrera, M. S., Affleck, J. G., Robinson, W. P., and Brown, C. J. (2009). Inactive X chromosome-specific reduction in placental DNA methylation. Hum. Mol. Genet. 18, 3544–3552. doi: 10.1093/hmg/ddp299
Douville, R. N., and Nath, A. (2014). Human endogenous retroviruses and the nervous system. Handb. Clin. Neurol. 123, 465–485. doi: 10.1016/B978-0-444-53488-0.00022-5
Douville, R., Liu, J., Rothstein, J., and Nath, A. (2011). Identification of active loci of a human endogenous retrovirus in neurons of patients with amyotrophic lateral sclerosis. Ann. Neurol. 69, 141–151. doi: 10.1002/ana.22149
Dunk, C. E., Gellhaus, A., Drewlo, S., Baczyk, D., Pötgens, A. J., Winterhager, E., et al. (2012). The molecular role of connexin 43 in human trophoblast cell fusion. Biol. Reprod. 86, 115. doi: 10.1095/biolreprod.111.096925
Dupressoir, A., Marceau, G., Vernochet, C., Bénit, L., Kanellopoulos, C., Sapin, V., et al. (2005). Syncytin-A and syncytin-B, two fusogenic placenta-specific murine envelope genes of retroviral origin conserved in Muridae. Proc. Natl. Acad. Sci. U.S.A. 102, 725–730. doi: 10.1073/pnas.0406509102
Ehrlich, M., Gama-Sosa, M. A., Huang, L. H., Midgett, R. M., Kuo, K. C., McCune, R. A., et al. (1982). Amount and distribution of 5-methylcytosine in human DNA from different types of tissues of cells. Nucleic Acids Res. 10, 2709–2721. doi: 10.1093/nar/10.8.2709
Esnault, C., Cornelis, G., Heidmann, O., and Heidmann, T. (2013). Differential evolutionary fate of an ancestral primate endogenous retrovirus envelope gene, the EnvV syncytin, captured for a function in placentation. PLoS Genet. 9:e1003400. doi: 10.1371/journal.pgen.1003400
Fort, A., Hashimoto, K., Yamada, D., Salimullah, M., Keya, C. A., Saxena, A., et al. (2014). Deep transcriptome profiling of mammalian stem cells supports a regulatory role for retrotransposons in pluripotency maintenance. Nat. Genet. 46, 558–566. doi: 10.1038/ng.2965
Friedli, M., and Trono, D. (2015). The developmental control of transposable elements and the evolution of higher species. Annu. Rev. Cell Dev. Biol. 31, 429–451. doi: 10.1146/annurev-cellbio-100814-125514
Fuchs, N. V., Loewer, S., Daley, G. Q., Izsvak, Z., Löwer, J., and Löwer, R. (2013). Human endogenous retrovirus K (HML-2) RNA and protein expression is a marker for human embryonic and induced pluripotent stem cells. Retrovirology 10:115. doi: 10.1186/1742-4690-10-115
Fuke, C., Shimabukuro, M., Petronis, A., Sugimoto, J., Oda, T., Miura, K., et al. (2004). Age related changes in 5-methylcytosine content in human peripheral leukocytes and placentas: an HPLC-based study. Ann. Hum. Genet. 68(Pt 3), 196–204. doi: 10.1046/j.1529-8817.2004.00081.x
Gimenez, J., Montgiraud, C., Oriol, G., Pichon, J. P., Ruel, K., Tsatsaris, V., et al. (2009). Comparative methylation of ERVWE1/syncytin-1 and other human endogenous retrovirus LTRs in placenta tissues. DNA Res. 16, 195–211. doi: 10.1093/dnares/dsp011
Golan, M., Hizi, A., Resau, J. H., Yaal-Hahoshen, N., Reichman, H., Keydar, I., et al. (2008). Human endogenous retrovirus (HERV-K) reverse transcriptase as a breast cancer prognostic marker. Neoplasia 10, 521–533. doi: 10.1593/neo.07986
Grow, E. J., Flynn, R. A., Chavez, S. L., Bayless, N. L., Wossidlo, M., Wesche, D. J., et al. (2015). Intrinsic retroviral reactivation in human preimplantation embryos and pluripotent cells. Nature 522, 221–225. doi: 10.1038/nature14308
Guo, H., Zhu, P., Yan, L., Li, R., Hu, B., Lian, Y., et al. (2014). The DNA methylation landscape of human early embryos. Nature 511, 606–610. doi: 10.1038/nature13544
Hegyi, H. (2013). GABBR1 has a HERV-W LTR in its regulatory region–a possible implication for schizophrenia. Biol. Direct 8:5. doi: 10.1186/1745-6150-8-5
Heidmann, O., Vernochet, C., Dupressoir, A., and Heidmann, T. (2009). Identification of an endogenous retroviral envelope gene with fusogenic activity and placenta-specific expression in the rabbit: a new “syncytin” in a third order of mammals. Retrovirology 6:107. doi: 10.1186/1742-4690-6-107
Hon, G. C., Rajagopal, N., Shen, Y., McCleary, D. F., Yue, F., Dang, M. D., et al. (2013). Epigenetic memory at embryonic enhancers identified in DNA methylation maps from adult mouse tissues. Nat. Genet. 45, 1198–1206. doi: 10.1038/ng.2746
Huang, W. J., Liu, Z. C., Wei, W., Wang, G. H., Wu, J. G., and Zhu, F. (2006). Human endogenous retroviral pol RNA and protein detected and identified in the blood of individuals with schizophrenia. Schizophr. Res. 83, 193–199. doi: 10.1016/j.schres.2006.01.007
Huang, W., Li, S., Hu, Y., Yu, H., Luo, F., Zhang, Q., et al. (2011). Implication of the env gene of the human endogenous retrovirus W family in the expression of BDNF and DRD3 and development of recent-onset schizophrenia. Schizophr. Bull. 37, 988–1000. doi: 10.1093/schbul/sbp166
Imakawa, K., Nakagawa, S., and Miyazawa, T. (2015). Baton pass hypothesis: successive incorporation of unconserved endogenous retroviral genes for placentation during mammalian evolution. Genes Cells 20, 771–788. doi: 10.1111/gtc.12278
Kammerer, U., Germeyer, A., Stengel, S., Kapp, M., and Denner, J. (2011). Human endogenous retrovirus K (HERV-K) is expressed in villous and extravillous cytotrophoblast cells of the human placenta. J. Reprod. Immunol. 91, 1–8. doi: 10.1016/j.jri.2011.06.102
Karlsson, H., Bachmann, S., Schröder, J., McArthur, J., Torrey, E. F., and Yolken, R. H. (2001). Retroviral RNA identified in the cerebrospinal fluids and brains of individuals with schizophrenia. Proc. Natl. Acad. Sci. U.S.A. 98, 4634–4639. doi: 10.1073/pnas.061021998
Katoh, I., and Kurata, S. (2013). Association of endogenous retroviruses and long terminal repeats with human disorders. Front. Oncol. 3:234. doi: 10.3389/fonc.2013.00234
Kobayashi, H., Sakurai, T., Imai, M., Takahashi, N., Fukuda, A., Yayoi, O., et al. (2012). Contribution of intragenic DNA methylation in mouse gametic DNA methylomes to establish oocyte-specific heritable marks. PLoS Genet. 8:e1002440. doi: 10.1371/journal.pgen.1002440
Lamprecht, B., Walter, K., Kreher, S., Kumar, R., Hummel, M., Lenze, D., et al. (2010). Derepression of an endogenous long terminal repeat activates the CSF1R proto-oncogene in human lymphoma. Nat. Med. 16, 571–579. doi: 10.1038/nm.2129
Lee, H. J., Hore, T. A., and Reik, W. (2014). Reprogramming the methylome: erasing memory and creating diversity. Cell Stem Cell 14, 710–719. doi: 10.1016/j.stem.2014.05.008
Leung, D., Du, T., Wagner, U., Xie, W., Lee, A. Y., Goyal, P., et al. (2014). Regulation of DNA methylation turnover at LTR retrotransposons and imprinted loci by the histone methyltransferase Setdb1. Proc. Natl. Acad. Sci. U.S.A. 111, 6690–6695. doi: 10.1073/pnas.1322273111
Li, F., Nellaker, C., Sabunciyan, S., Yolken, R. H., Jones-Brando, L., Johansson, A. S., et al. (2014). Transcriptional derepression of the ERVWE1 locus following influenza A virus infection. J. Virol. 88, 4328–4337. doi: 10.1128/JVI.03628-13
Li, W., Lee, M. H., Henderson, L., Tyagi, R., Bachani, M., Steiner, J., et al. (2015). Human endogenous retrovirus-K contributes to motor neuron disease. Sci. Transl. Med. 7:307ra153. doi: 10.1126/scitranslmed.aac8201
Ling, J., Pi, W., Bollag, R., Zeng, S., Keskintepe, M., Saliman, H., et al. (2002). The solitary long terminal repeats of ERV-9 endogenous retrovirus are conserved during primate evolution and possess enhancer activities in embryonic and hematopoietic cells. J. Virol. 76, 2410–2423. doi: 10.1128/jvi.76.5.2410-2423.2002
Liu, M., and Eiden, M. V. (2011). Role of human endogenous retroviral long terminal repeats (LTRs) in maintaining the integrity of the human germ line. Viruses 3, 901–905. doi: 10.3390/v3060901
Liu, S., Brind'Amour, J., Karimi, M. M., Shirane, K., Bogutz, A., Lefebvre, L., et al. (2014). Setdb1 is required for germline development and silencing of H3K9me3-marked endogenous retroviruses in primordial germ cells. Genes Dev. 28, 2041–2055. doi: 10.1101/gad.244848.114
MacFarlan, T. S., Gifford, W. D., Driscoll, S., Lettieri, K., Rowe, H. M., Bonanomi, D., et al. (2012). Embryonic stem cell potency fluctuates with endogenous retrovirus activity. Nature 487, 57–63. doi: 10.1038/nature11244
Malfavon-Borja, R., and Feschotte, C. (2015). Fighting fire with fire: endogenous retrovirus envelopes as restriction factors. J. Virol. 89, 4047–4050. doi: 10.1128/JVI.03653-14
Mangeney, M., Renard, M., Schlecht-Louf, G., Bouallaga, I., Heidmann, O., Letzelter, C., et al. (2007). Placental syncytins: genetic disjunction between the fusogenic and immunosuppressive activity of retroviral envelope proteins. Proc. Natl. Acad. Sci. U.S.A. 104, 20534–20539. doi: 10.1073/pnas.0707873105
Manghera, M., and Douville, R. N. (2013). Endogenous retrovirus-K promoter: a landing strip for inflammatory transcription factors? Retrovirology 10:16. doi: 10.1186/1742-4690-10-16
Matousková, M., Blazková, J., Pajer, P., Pavlícek, A., and Hejnar, J. (2006). CpG methylation suppresses transcriptional activity of human syncytin-1 in non-placental tissues. Exp. Cell Res. 312, 1011–1020. doi: 10.1016/j.yexcr.2005.12.010
Mi, S., Lee, X., Li, X., Veldman, G. M., Finnerty, H., Racie, L., et al. (2000). Syncytin is a captive retroviral envelope protein involved in human placental morphogenesis. Nature 403, 785–789. doi: 10.1038/35001608
Okae, H., Chiba, H., Hiura, H., Hamada, H., Sato, A., Utsunomiya, T., et al. (2014). Genome-wide analysis of DNA methylation dynamics during early human development. PLoS Genet. 10:e1004868. doi: 10.1371/journal.pgen.1004868
Oluwole, S. O., Yao, Y., Conradi, S., Kristensson, K., and Karlsson, H. (2007). Elevated levels of transcripts encoding a human retroviral envelope protein (syncytin) in muscles from patients with motor neuron disease. Amyotroph. Lateral Scler. 8, 67–72. doi: 10.1080/17482960600864207
Perron, H., Germi, R., Bernard, C., Garcia-Montojo, M., Deluen, C., Farinelli, L., et al. (2012a). Human endogenous retrovirus type W envelope expression in blood and brain cells provides new insights into multiple sclerosis disease. Mult. Scler. 18, 1721–1736. doi: 10.1177/1352458512441381
Perron, H., Hamdani, N., Faucard, R., Lajnef, M., Jamain, S., Daban-Huard, C., et al. (2012b). Molecular characteristics of human endogenous retrovirus type-W in schizophrenia and bipolar disorder. Transl. Psychiatry 2:e201. doi: 10.1038/tp.2012.125
Popp, C., Dean, W., Feng, S., Cokus, S. J., Andrews, S., Pellegrini, M., et al. (2010). Genome-wide erasure of DNA methylation in mouse primordial germ cells is affected by AID deficiency. Nature 463, 1101–1105. doi: 10.1038/nature08829
Prudhomme, S., Oriol, G., and Mallet, F. (2004). A retroviral promoter and a cellular enhancer define a bipartite element which controls env ERVWE1 placental expression. J. Virol. 78, 12157–12168. doi: 10.1128/JVI.78.22.12157-12168.2004
Redelsperger, F., Cornelis, G., Vernochet, C., Tennant, B. C., Catzeflis, F., Mulot, B., et al. (2014). Capture of syncytin-Mar1, a fusogenic endogenous retroviral envelope gene involved in placentation in the Rodentia squirrel-related clade. J. Virol. 88, 7915–7928. doi: 10.1128/JVI.00141-14
Salmons, B., Lawson, J. S., and Günzburg, W. H. (2014). Recent developments linking retroviruses to human breast cancer: infectious agent, enemy within or both? J. Gen. Virol. 95(Pt 12), 2589–2593. doi: 10.1099/vir.0.070631-0
Schlesinger, S., and Goff, S. P. (2015). Retroviral transcriptional regulation and embryonic stem cells: war and peace. Mol. Cell. Biol. 35, 770–777. doi: 10.1128/MCB.01293-14
Schmitt, K., Reichrath, J., Roesch, A., Meese, E., and Mayer, J. (2013). Transcriptional profiling of human endogenous retrovirus group HERV-K(HML-2) loci in melanoma. Genome Biol. Evol. 5, 307–328. doi: 10.1093/gbe/evt010
Schroeder, D. I., Jayashankar, K., Douglas, K. C., Thirkill, T. L., York, D., Dickinson, P. J., et al. (2015). Early developmental and evolutionary origins of gene body DNA methylation patterns in mammalian placentas. PLoS Genet. 11:e1005442. doi: 10.1371/journal.pgen.1005442
Subramanian, R. P., Wildschutte, J. H., Russo, C., and Coffin, J. M. (2011). Identification, characterization, and comparative genomic distribution of the HERV-K (HML-2) group of human endogenous retroviruses. Retrovirology 8:90. doi: 10.1186/1742-4690-8-90
Sugimoto, J., Sugimoto, M., Bernstein, H., Jinno, Y., and Schust, D. (2013). A novel human endogenous retroviral protein inhibits cell-cell fusion. Sci. Rep. 3:1462. doi: 10.1038/srep01462
Suntsova, M., Gogvadze, E. V., Salozhin, S., Gaifullin, N., Eroshkin, F., Dmitriev, S. E., et al. (2013). Human-specific endogenous retroviral insert serves as an enhancer for the schizophrenia-linked gene PRODH. Proc. Natl. Acad. Sci. U.S.A. 110, 19472–19477. doi: 10.1073/pnas.1318172110
Theunissen, T. W., Friedli, M., He, Y., Planet, E., O'Neil, R. C., Markoulaki, S., et al. (2016). Molecular criteria for defining the naive human pluripotent state. Cell Stem Cell 19, 502–515. doi: 10.1016/j.stem.2016.06.011
Thomas, J. H., and Schneider, S. (2011). Coevolution of retroelements and tandem zinc finger genes. Genome Res. 21, 1800–1812. doi: 10.1101/gr.121749.111
Thompson, P. J., MacFarlan, T. S., and Lorincz, M. C. (2016). Long terminal repeats: from parasitic elements to building blocks of the transcriptional regulatory repertoire. Mol. Cell 62, 766–776. doi: 10.1016/j.molcel.2016.03.029
Tristem, M. (2000). Identification and characterization of novel human endogenous retrovirus families by phylogenetic screening of the human genome mapping project database. J. Virol. 74, 3715–3730. doi: 10.1128/JVI.74.8.3715-3730.2000
van der Laan, L. J., Lockey, C., Griffeth, B. C., Frasier, F. S., Wilson, C. A., Onions, D. E., et al. (2000). Infection by porcine endogenous retrovirus after islet xenotransplantation in SCID mice. Nature 407, 90–94. doi: 10.1038/35024089
Walsh, C. P., Chaillet, J. R., and Bestor, T. H. (1998). Transcription of IAP endogenous retroviruses is constrained by cytosine methylation. Nat. Genet. 20, 116–117. doi: 10.1038/2413
Wang, J., Xie, G., Singh, M., Ghanbarian, A. T., Rasko, T., Szvetnik, A., et al. (2014). Primate-specific endogenous retrovirus-driven transcription defines naive-like stem cells. Nature 516, 405–409. doi: 10.1038/nature13804
Wang, L., Zhang, J., Duan, J., Gao, X., Zhu, W., Lu, X., et al. (2014). Programming and inheritance of parental DNA methylomes in mammals. Cell 157, 979–991. doi: 10.1016/j.cell.2014.04.017
Wang-Johanning, F., Radvanyi, L., Rycaj, K., Plummer, J. B., Yan, P., Sastry, K. J., et al. (2008). Human endogenous retrovirus K triggers an antigen-specific immune response in breast cancer patients. Cancer Res. 68, 5869–5877. doi: 10.1158/0008-5472.CAN-07-6838
Wolf, G., Yang, P., Fuchtbauer, A. C., Fuchtbauer, E. M., Silva, A. M., Park, C., et al. (2015). The KRAB zinc finger protein ZFP809 is required to initiate epigenetic silencing of endogenous retroviruses. Genes Dev. 29, 538–554. doi: 10.1101/gad.252767.114
Yi, J. M., and Kim, H. S. (2007). Molecular phylogenetic analysis of the human endogenous retrovirus E (HERV-E) family in human tissues and human cancers. Genes Genet. Syst. 82, 89–98. doi: 10.1266/ggs.82.89
Yu, C., Shen, K., Lin, M., Chen, P., Lin, C., Chang, G. D., et al. (2002). GCMa regulates the syncytin-mediated trophoblastic fusion. J. Biol. Chem. 277, 50062–50068. doi: 10.1074/jbc.M209316200
Keywords: endogenous retrovirus, genome, human disease, pre-implantation embryo, stem cells, placenta, innate immunity
Citation: Meyer TJ, Rosenkrantz JL, Carbone L and Chavez SL (2017) Endogenous Retroviruses: With Us and against Us. Front. Chem. 5:23. doi: 10.3389/fchem.2017.00023
Received: 22 December 2016; Accepted: 20 March 2017;
Published: 07 April 2017.
Edited by:
Tammy A. Morrish, Independent Investigator, Ann Arbor, USAReviewed by:
Ahmet M. Denli, Salk Institute for Biological Studies, USAReiner Strick, Universitätsklinikum Erlangen, Germany
Copyright © 2017 Meyer, Rosenkrantz, Carbone and Chavez. This is an open-access article distributed under the terms of the Creative Commons Attribution License (CC BY). The use, distribution or reproduction in other forums is permitted, provided the original author(s) or licensor are credited and that the original publication in this journal is cited, in accordance with accepted academic practice. No use, distribution or reproduction is permitted which does not comply with these terms.
*Correspondence: Thomas J. Meyer, dGhvbWFzLmpvc2h1YS5tZXllckBnbWFpbC5jb20=