- 1Department of Chemical Science, University of Padova, Padova, Italy
- 2Institute of Biomolecular Chemistry of CNR, Padova Unit, Padova, Italy
- 3Key Laboratory for Modern Drug Delivery & High-Efficiency, School of Pharmaceutical Sciences and Technology, Tianjin University, Tianjin, China
- 4Laboratory of Experimental Pharmacology, IRCCS Istituto Tumori Giovanni Paolo II, Bari, Italy
- 5CNR Institute of Nanoscience (CNR-NANO), Modena, Italy
- 6Institute on Membrane Technology (ITM-CNR), UOS of Padova, Padova, Italy
The Anderson-Evans polyoxometalates (POM) display a promising anticancer activity. The conjugation with the GRP-receptor antagonist peptide Demobesin (fQWAVGHL-NHEt) was exploited to impart cell targeting capabilities and improve the selectivity of such polyanions. However, the POM interacts with the grafted peptides, inducing chains folding and self-assembly of the resulting hybrids, thus decreasing their recognition ability. Within this context, a tailored spacer, including two domains, i.e., a hydrophilic one (1,13-diamino-4,7,10-trioxatridecan-succinamic acid, Ttds) and a tetra-anionic one (Glu-Glu-Glu-Glu-βAla, EEEE-βA) was previously utilized to mitigate such interaction. In this work, hybrid POMs containing only Ttds or EEEE-βA were prepared and the contribution of the two spacers was separately studied by using 2D NMR, fluorimetry and circular dichroism (CD). Transmission electron microscopy (TEM) was also used to observe the impact of the different spacers on self-assembly. Owing to the relevant effects observed for EEEE-βA, MD calculations were finally performed to elucidate its behavior when incorporated in the hybrid POM. Our results show that, despite the stronger impact of EEEE-βA spacer, only when both spacer are present together it is possible to observe a significant effect on the retention of peptide's secondary structure and recognition capability.
1 Introduction
Polyoxometalates (POMs), a class of polynuclear oxo-bridged transition metal complexes (Blazevic and Rompel, 2016), have received extensive attention due to their rich topology and tunable chemical and physical properties. In addition to their application in multidisciplinary fields (Omwoma et al., 2015), such as material science (Yu et al., 2023) and catalysis (Wang and Yang, 2015), their biomedical activity (Čolović et al., 2020), as antitumor (Bijelic et al., 2019), antiviral (Dan et al., 2020), and antibacterial (Bijelic et al., 2018) agents, has been highlighted. The main advantage of POMs is that their properties (especially dimensions and charge) can be tailored to optimize the interaction with biological macromolecules (Arefian et al., 2017; Lentink et al., 2023). Moreover, they can be rationally synthesized on a multi-gram scale at a low cost.
POMs were generally proven to cross cell membranes, although with high toxicity and instability under physiological pH conditions (Wu and Liang, 2017). To overcome these drawbacks, different delivery systems were devised (Bijelic et al., 2019), while the POM architectures were successfully modified by introducing transition metals (such as Co, Ru or Mn) (Wang et al., 2014; Fabbian et al., 2022; Carvalho and Aureliano, 2023) or by grafting suitable organic pendants (Cameron et al., 2022). This last approach, consisting of the hybridization of inorganic POMs with organic or biological moieties, by exploiting weak interactions and/or covalent bonds, increases both stability and bioavailability (Dolbecq et al., 2010; Chang et al., 2022), and provides interesting opportunities for tracking (Modugno et al., 2018) and targeting (Zamolo et al., 2018; Lentink et al., 2023).
The Anderson-Evans polyoxometalates with the general formula [XM6O24]n- (n = 2–8) are constituted by six edge-sharing octahedra, MO6 (M=Mo or W), surrounding a central edge-sharing XO6 octahedron containing the heteroatom (X=Mn, Cr, I, etc.). The Mn-Anderson-Evans POM functionalized with TRIS (tris(hydroxymethyl)aminomethane), [(C4H9)4N]3[MnMo6O18{(OCH2)3CNH2}2] (POM-TRIS), and its derivatives have a proven cytotoxic effect on cancer cells (Mahvash et al., 2023). The functionalization of such POMs (Blazevic and Rompel, 2016) with biomolecules (Yang et al., 2013; Hosseini et al., 2021; Ramezani-Aliakbari et al., 2021) can thus be a convenient strategy to increase the bioactivity or to impart recognition ability. Among bio-conjugation opportunities, the interest in the use of peptides has recently increased (Albada and Metzler-Nolte, 2016), and there are already some examples dealing with the covalent associations of POMs with peptides (Yvon et al., 2014; Luo et al., 2023; Soria-Carrera et al., 2023). Within this scenario, the Anderson-Evans POM was decorated with Demobesin-1 (1, see Figure 1) (Ventura et al., 2018), a variant of bombesin peptide (QRLGNQWAVGHLM-NH2) isolated in amphibians, which is an antagonist of the gastrin-releasing peptide (GRP). The ability to target GRP-receptors (Aprikian et al., 1996; Rozengurt, 1998), overexpressed in different human cancers, including lung, stomach, prostate, ovarian and breast cancer (Gugger and Reubi, 1999; Markwalder and Reubi, 1999; Schally and Nagy, 1999; Pooja et al., 2019), makes bombesin derivatives suitable tools to drive cytotoxic drugs into tumor cells (Nock et al., 2003; Cescato et al., 2008). However, once conjugated to the POM core, peptide 1 did not impart any positive effect on the activity of the resulting bio-hybrid 1-POM, probably due to an unfavorable interaction between peptide side-chains and POM surface, that hampered the recognition of target receptors (Rubini et al., 2010). To improve the bioavailability of 1, a spacer composed of (i) an anionic region, containing four glutamic acids and a β-alanine residue (EEEE-βA) and (ii) a non-ionic Ttds tail, was conjugated to the N-terminal amino moiety of 1 (to produce peptide 4, see Supplementary Figure S1) (Tagliavini et al., 2021). The resulting POM (4-POM) showed better performance in terms of tumor cell recognition and is thus encouraging a further development of this approach.
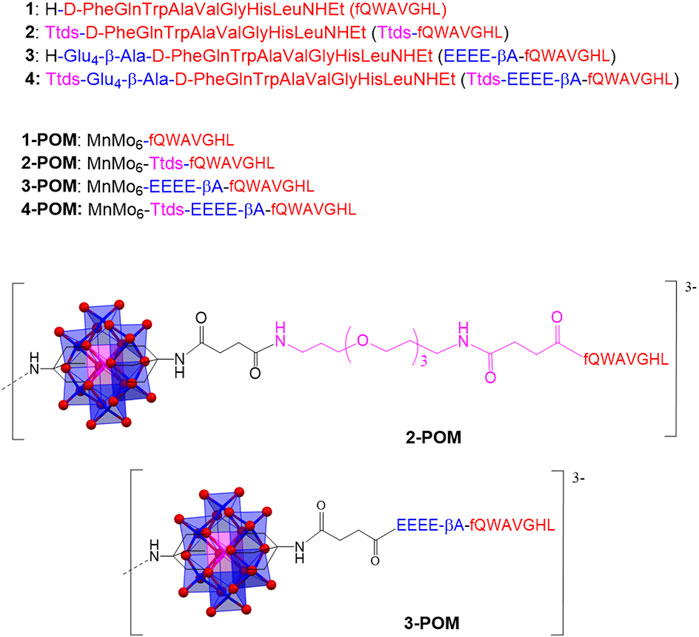
Figure 1. Aminoacidic sequence of the peptides 1-4, and structure of hybrids 2-POM and 3-POM (only one pendant is shown; tetrabutyl ammonium cations are also omitted).
In this work, the Mn-Anderson-Evans was functionalized with two 1 analogs containing one of the two portions of the previously described spacer. Peptide 2 contains only the Ttds group, while peptide 3 only the EEEE-βA anionic domain (Figure 1). The corresponding POM constructs (2-POM and 3-POM) were prepared (Figure 1 and Supplementary Figure S1) and compared with spacer-free, and Ttds-EEEE-βA containing POMs (1-POM and 4-POM, respectively), to investigate the contribution of different spacers. POM hybrids were characterized by various spectroscopic techniques, including ESI-MS, FT-IR, 2D-NMR, UV-Vis, CD and fluorimetry, DLS, TEM, and tested in vitro on cancer cells. Owing to its interesting properties, 3-POM was also investigated by MD calculations to explain the observed behavior.
2 Materials and methods
2.1 Reagents
Fmoc-amino acids, N-Fmoc-N-succinyl-4,7,10-trioxa-1,13-tridecanediamine (Fmoc-Ttds-OH), N,N-diisopropylethylamine (DIPEA), 2-(7-azabenzotriazol-1-yl)-N,N,N′,N′-tetramethyluronium hexafluorophosphate (HATU), 2-(1H-benzotriazole-1-yl)-1,1,3,3-tetramethyluronium hexafluorophosphate (HBTU), 1-hydroxybenzotriazole (HOBt), trifluoroacetic acid (TFA), piperidine, triisopropylsilane, were obtained from Iris Biotech. Tris(hydroxymethyl)aminomethane (TRIS), succinic anhydride, N,N′-dicyclohexylcarbodiimide (DCC), N-hydroxysuccinimide (NHS), DMF, diethyl ether, were purchased from Sigma-Aldrich and used without any further treatment.
2.2 Instruments
Nuclear Magnetic Resonance (NMR) Spectroscopy was performed on a Bruker DMX-400 instrument (Bruker Corporation, Billerica, MA, USA) operating at 399.92 MHz for 1H. Proton chemical shifts, in parts per million (ppm), are referred to the residual 1H-DMSO solvent signal in DMSO-d6 (δ = 2.49 ppm). About 4 mg of each sample were dissolved in 600 μL DMSO-d6 for analysis. All NMR experiments have been carried out at temperature of 298 K. One-dimensional (1D) 1H-NMR spectra were acquired using typically 32 scans with 32K data size. For the two-dimensional (2D) 1H NMR spectra, including COSY (Correlation Spectroscopy), TOCSY (Total Correlation Spectroscopy), and ROESY (Rotating Frame Overhauser Effect Spectroscopy), homonuclear pulse programs of the standard Bruker library were used (cosygpmfqf for COSY experiments, mlevetgp for TOCSY experiments, roesyetgp for ROESY experiments). Typically, 512 experiments of 50 scans each were performed: relaxation delay T2 equal to 1; size 4K; 5,597 Hz spectral width in F2. Spectral processing was performed using the software TopSpin.
Infrared Spectra were collected by preparing KBr pellets, on a Nicolet 5700 FI-IR instrument (Thermo Fisher Scientific, Waltham, MA; USA).
Electrospray Ionization Mass Spectroscopy (ESI-MS) was performed on a LC/MSD Trap SL Agilent instrument (Agilent Technologies, Santa Clara, CA, USA). The samples were dissolved in CH3CN and analyzed in negative mode.
High-performance liquid chromatography (HPLC) analyses were run on a Shimadzu system equipped with a binary pump (LC-10AD), SCL-10A controller, Knauer detector and Gastorr 154 degasser. The semi-preparative HPLC was performed on a Shimadzu system equipped with a binary pump (LC-8A), a SCL-8A controller, SPD-6A detector and ERMA (ERC-3562) degasser (Shimadzu Corporation, Kyoto, Japan).
Circular dichroism and UV-vis spectra were acquired on a Jasco J-1500 CD spectrometer combined with a Jasco PTC-423S temperature controller (JASCO Corporation, Tokyo, Japan). For Circular Dichroism (CD) analysis, 1 mg/mL stock solutions were prepared in TFE and diluted to 0.1 mg/mL in the investigated TFE/water mixtures (%TFE= 10, 20, 40, 60, 80, 100), which were prepared in 2 mL flasks. Far-UV CD spectra were collected (at least 16 scans per sample) in the 190–260 nm range in 0.1 cm pathlength quartz cuvettes (Hellma GmbH & Co., Müllheim, Germany) at 25°C at 50 nm/min scanning speed, 1 s response time, 1 nm bandwidth, 200 mdeg sensitivity, 0.5 nm datapitch. The secondary structure estimation (SSE) analysis of CD spectra was performed both using the Jasco Spectra manager application and the CDApps software developed at B23 beamline of the Diamond Light Source synchrotron (Didcot, UK) (Hussain et al., 2015), enabling signal deconvolution by means of the CONTINLL algorithm (encompassing α-helix, distorted α -helix, β-strand, distorted β-strand, turns and unordered reference structures).
Emission fluorescence experiments were collected on a Perkin Elmer LS50B spectrofluorimeter, equipped with FL-WinLab software (PerkinElmer, Inc., Waltham, MA, USA). Samples were measured in 10 mm pathlength quartz cuvettes at 25°C. Emission spectra were recorded in the range 300–550 nm, with excitation at λ=295 nm, 3.5 nm excitation and emission slits and 400 nm/min scan speed. The spectra were the average of 4 scans.
Dynamic Light Scattering (DLS) was monitored by a Zetasizer Nano ZS instrument (Malvern Panalytical Ltd, UK), collecting 3 series of measurements from 0.2 mM solutions in phosphate buffer (10 mM, pH 7) with 3% DMSO.
Transmission Electron Microscopy (TEM) was carried out using a FEI Tecnai G2 instrument (Thermo Fisher Scientific, Waltham, MA, USA), drop casting aqueous solutions (with 3% DMSO) onto copper grids.
2.3 Cytotoxicity tests
Cytotoxicity/viability tests were performed as 3-[4,5-dimethylthiazol-2-yl]-2,5-diphenyltetrazoliumbromide (MTT) assays. For the experiments, HeLa cervical cancer (LGC Standards S.r.l., Sesto San Giovanni, Italy), cultured according to the manufacturer’s instructions, were used. On day 1, 10,000 HeLa cervical cancer cells/well in a volume of 200 µL were plated in 96-wells plates, as previously reported (Tagliavini et al., 2021). The cells were exposed for 24, and 48 h to different concentrations of POM-TRIS, 2-POM, 3-POM (5, 25, 50, 100, and 150 μM). Stock solutions with 100 mM POM in water, containing 5% DMSO, were prepared by prior solubilization of POMs in DMSO, followed by dilution in water. The results were expressed as the mean ± SD percentage of living cells normalized to the control.
2.4 Molecular Dynamics (MD) investigations
The coordinates of the POM moiety, that was kept frozen during the simulations, were taken from the published crystal structure (Rosnes et al., 2012). For the peptide moieties, a fully extended starting structure was used. The peptide chains and the POM peptide complexes were build using standard molecular manipulation tools. For the peptides, the GROMOS 54a7 force field was used (Schmid et al., 2011). The parameters for non-standard residues were obtained by analogy with similar already parameterized chemical groups. As the POM structure is kept fixed during the MD simulations, only non-bonded parameters were derived, i.e., partial atomic charges and Lennard-Jones parameters. The partial atomic charges were evaluated from quantum chemical calculations on the isolated POM moiety bound on both sides to an amino group (see Figure 1). RESP charges (Bayly et al., 1993) were calculated at the density functional theory (DFT) level (Parr and Yang, 1995) with the B3LYP functional (Becke, 1993). The atomic basis sets were as follows: (i) for the Mn and Mo atoms, the LANL2DZ effective core potential for the inner electrons and a double Gaussian basis set of (5S,5P,5D)/[3S,3P,2D] quality for the valence electrons were used (Hay and Wadt, 1985); (ii) for the hydrogen, carbon, oxygen and nitrogen atoms, a standard 6-31+G(d) Gaussian basis set was used (Krishnan et al., 2008). Quantum chemical calculations were performed with the Gaussian09 package (M. Jea Frisch et al., 2009). The Lennard-Jones parameters for the POM were taken from the universal force field (UFF).
The POM-peptide hybrids were solvated in a dodecahedral box, large enough to contain the solute (with the peptides in the extended conformation) and at least 1.0 nm of solvent on all sides. Water was modeled by the simple point charge (SPC) model (Berendsen et al., 1987). The LINCS algorithm (Hess et al., 1997) was used to constrain bond lengths and a time step of 2 fs for numerical integration of the equations of motion was used. The particle mesh Ewald method (Darden et al., 1993) was used for the calculation of the long-range interactions and a cut-off of 1.1 nm was used. After a solute optimization and a subsequent solvent relaxation, each system was gradually heated from 50 to 300 K using short MD simulations. Then, a short (100 ps) equilibration simulation was performed for the two systems in the NPT ensemble with the velocity rescaling temperature coupling (Bussi et al., 2007) to keep the temperature constant at 300 K and the Berendsen pressure coupling (Berendsen et al., 1984) to keep the pressure constant at 1 bar. Three productive independent 100 ns-long MD simulations for each system were then carried out in the NVT ensemble at 300 K. For both compound 1-POM and compound 3-POM, the same starting structure was used for each of the three simulations, varying the initial velocities randomly generated conforming to a Maxwell velocity distribution at 300 K. The MD simulations were performed with the GROMACS (Van Der Spoel et al., 2005) software package. The secondary structure of the peptides was analyzed with the DSSP tool (Kabsch and Sander, 1983) of the GROMACS package.
3 Results and discussion
3.1 Peptide and POM-peptide synthesis
Peptides 1-4 were prepared by solid phase synthesis adopting the Fmoc/HBTU strategy, according to the previously described procedure (Rubini et al., 2010; Ventura et al., 2018; Tagliavini et al., 2021). The N-ethylamide group was introduced ab initio using an ethyl-indole AM Resin. The peptides were purified by HPLC and characterized by ESI-MS, FT-IR and 2D-NMR (see Supplementary Figures S2–S11, showing the characterization of peptides 2 and 3).
[(C4H9)4N]3[MnMo6O18((OCH2)3CNH2)2] POM-TRIS was synthesized by addition of TRIS and manganese (III) acetate to [(C4H9)4N]4[Mo8O26] in refluxing acetonitrile for 24 h (58% yield). The POM-TRIS was then reacted with succinic anhydride in molar ratio 1:20 at 50°C in DMF for 24 h to obtain [(C4H9)4N]3[MnMo6O18{(OCH2)3CNHCO(CH2)2COOH}2] (POM-succ, 74% yield), which was activated by reaction with NHS/DCC in DMF at room temperature for 1 day, to obtain [(C4H9)4N]3[MnMo6O18{(OCH2)3CNHCO(CH2)2CO(C4H4NO3)}2] (POM-NHS, 68% yield) (Supplementary Figure S1) (Yvon et al., 2014).
The syntheses of the 1-4-POM hybrids were performed in air, at room temperature for 24 h, under continuous stirring (Supplementary Figure S1) (Ventura et al., 2018; Tagliavini et al., 2021). To this aim, POM-NHS and 2 equivs. of peptides (1-4) were dissolved in DMF, then DIPEA was added. The obtained solutions were placed in a closed vessel under diethyl ether vapors, for 24 h, to allow the precipitation of the products, which were finally washed with diethyl ether and dried under air, to achieve pale orange solids (yields >85%).
All POM-peptides conjugates were characterized by ESI-MS, FT-IR, 2D-NMR, CD, UV-vis (see Supplementary Figures S12–S27, showing the characterization of 2-POM and 3-POM).
FT-IR and ESI-MS are quick and useful tools to assess the successful conjugation. FTIR show the replacement of the C=O signals of NHS group, around 1740 cm−1, by a band at 1,650–1,685 cm−1 due to the amide C=O bond and by a peak ascribed to N-H bending at 1,535–1,543 cm−1. The integrity of the inorganic POM scaffold was confirmed by the retention of the bands at 942, 920–921, 900–902 and 663–670 cm−1 (Supplementary Figures S12, S20). ESI-MS (-) spectra show the bis-functionalized POMs, detected as di- or tri-anionic species (Supplementary Figures S13, S21).
3.2 NMR investigation
The resonance assignment of amino acid residues in peptides 2 and 3 and their corresponding POM derivatives were obtained by collecting COSY, TOCSY and ROESY spectra for all samples (Supplementary Tables S1–S4). Due to the limited solubility of POM hybrids in water, the analysis was performed in DMSO-d6. The latter is also useful for the study of peptides that interact with membrane receptors, having a dielectric constant (ε 47.5) very close to that of the membrane interface (ε 40).
Information on the secondary structure of peptides was obtained from the secondary chemical shift (Δδ) values of the Hα, calculated by subtracting representative random coil values from the observed Hα shift. In DMSO-d6, a minimum of four values of Δδ less than −0.16 ppm or greater than 0.22 ppm are indicative of the presence of α-helix or β-sheet secondary structures, respectively (Tremblay et al., 2010). According to these threshold values, it is possible to assess how the C-terminal regions of all peptides have a propensity to adopt a helical structure (see Val, Gly, His, Leu residues in Figure 2A), with the most negative Δδ values observed for peptide 2. Once conjugated on the POM, the peptides generally show even more negative Δδ values. The signals of peptide 1 show the biggest changes (see Δδ values for Gln and Trp residues before and after grafting, Figure 2), with a tendency to form the helical structure also at the N-terminus. 2-POM, instead, displays its most negative Δδ value at the His residue, towards the C-terminus.
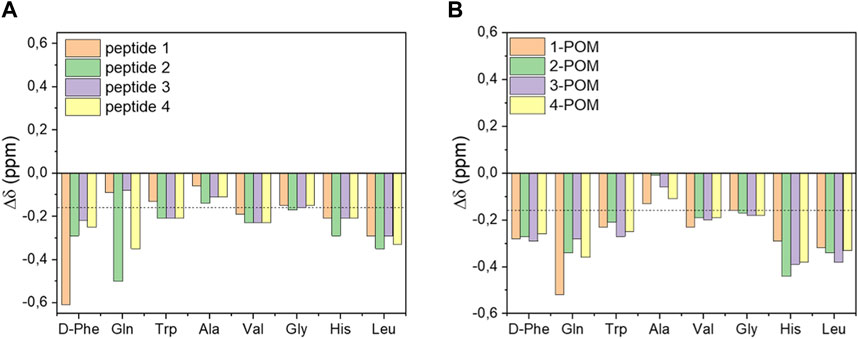
Figure 2. Secondary Hα chemical shift (Δδ) values of DB-1 derivatives (A) or of the corresponding POM-peptide hybrids (B). The dotted line indicates the threshold value for the presence of a helical secondary structure.
To evaluate the spacers’ contribution on the interactions between POM and different peptides, the NH and Hα secondary chemical shift values, determined by subtracting the NH and Hα resonance of each peptide to the resonance of corresponding POM construct were also compared (Figure 3). The presence of POM cluster had an evident impact in the resonance of the closer N-terminal peptide region in 1-POM and 2-POM, indicating that the introduction of the negatively charged Glu4-βAla sequence (in 3-POM and 4-POM) seems useful to attenuate the interaction between the peptide and the inorganic cluster.
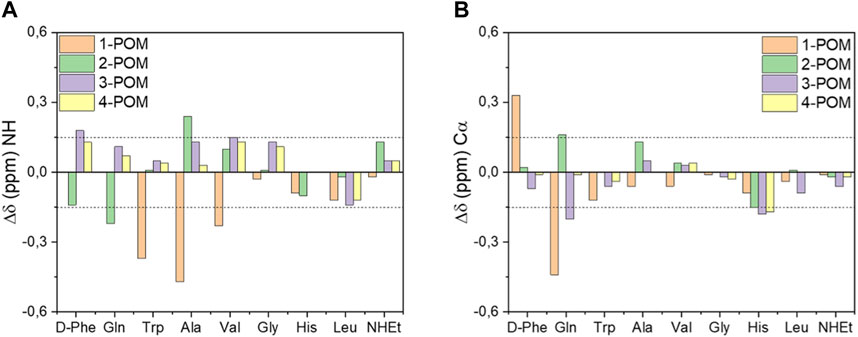
Figure 3. Influence of spacer on NH (A) and Hα (B) resonances of DB-1 in POM-peptide constructs. Secondary chemical shift values were determined by subtracting the NH or Hα chemical shift values of DB-1 analogues (peptide 1-4) to the resonance of corresponding POM constructs (POM 1-4). Values greater than |0.15| ppm (dotted lines) indicate a significant effect on resonances.
3.3 Circular dichroism spectroscopy
The far-UV (185–260 nm) CD spectrum of peptide is largely determined by the electronic transitions of the amide chromophores and provides useful information on its secondary structure. To overcome the high absorbance of DMSO in the far-UV region, that hinders its use as solvent in CD spectroscopy, 2,2,2-trifluoroethanol (TFE) was used as the solvent. TFE, in addition to being an excellent solvent for both peptide and the POM, is widely used to mimic the cell membrane environment (Ma et al., 2015). The influence of TFE on the secondary structure of peptides 2 and 3 was evaluated by recording CD spectra at increasing TFE percentages, so to monitor the behavior of the compounds in an environment with decreasing polarity (CD spectra in Figure 4; Supplementary Figures S28, S29, S32, S33), without the need of screening other solvents, where they display low solubility.
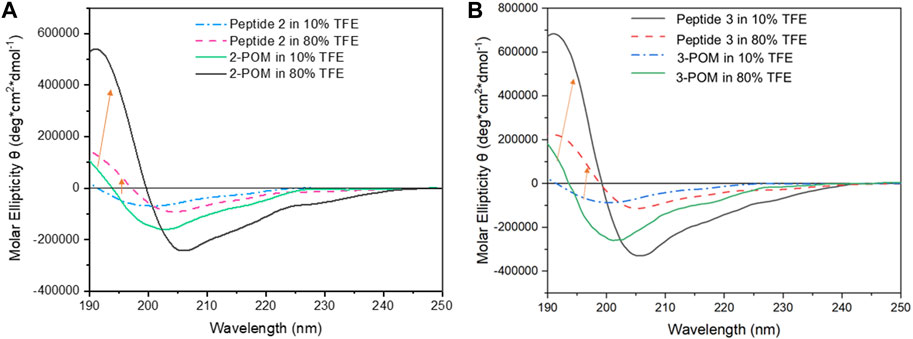
Figure 4. Far-UV CD spectra of 2 and 2-POM (A), and of and 3 and 3-POM (B), at 10 and 80% (v/v) TFE percentages (indicated). The spectra were recorded by a Jasco J-1500 spectropolarimeter using a 0.1 cm pathlength Suprasil quartz cuvette, 1 nm bandwidth, 50 nm/min scan speed, 1 nm data pitch, 1 s d.i.t.
At a low percentage of TFE (10% v/v), the CD spectra of peptides 2 and 3 show a negative band at 197–200 nm (n → π* electronic transition) and a positive band at λ<190 nm (π → π*), ascribed to an unordered conformation. In 80% TFE, the CD spectra of peptides 2 and 3 are characterized by a positive band at 192 nm and a negative band around 206 nm, generated by the presence of more ordered structures, involving an increased contribution of the α-helical structure.
Parallel experiments on POM-peptide constructs showed that, at low percentages of TFE (10% v/v, Figure 4), the introduction of the POM moiety influences the position of the dichroic bands, whose negative minima display a shift to λ = 202–204 nm. As far as the bands intensity is concerned, the double value of ellipticity is due to the presence of two peptide chains in the hybrids. Moreover, 3-POM shows a stronger signal than 2-POM, (with Θ = 2.7×105 deg cm2 dmol-1 at λ = 204 vs. 1.6×105 deg cm2 dmol−1 at λ = 202 nm, respectively, see Supplementary Figures S15, S23), likely due also to the presence of additional aminoacids in 3-POM. In such condition, β-strand and random coil are the main conformations (37%–38% and 32%–35%, respectively). At higher TFE percentage (≥80% v/v), the bands further increase their intensity, especially the positive one at 191 nm (Θ = 5.4 × 105 and 7.5 × 105 deg cm2 dmol-1 for 2-POM and 3-POM, respectively, see Supplementary Figures S29, S33), highlighting a strong tendency of the peptide to adopt to α-helix (up to 59%–68%) when exposed to the less hydrophilic medium and conjugated with the POM. The behavior observed in non-aqueous system is in agreement with the NMR analysis in DMSO-d6, and it is the result of a double effect: on one hand, TFE acts as secondary structure stabilizer, primarily inducing α-helical conformation (Vincenzi et al., 2019), on the other hand, the conjugation with POM may also assist the evolution to α-helix, as previously reported by (Yvon et al., 2014) for a different POM-peptide conjugate dissolved in CH3CN. Considering the estimated ratio between α-helix and β-strands in different TFE/H2O mixtures, hybrid POMs appear much more sensitive than free peptides to solvent change, being 2-POM the one showing the highest amount of α-helix (Supplementary Figures S30, S31, S34–S36). Nevertheless, the differences in the intensity of the bands level off when the compounds are situated in TFE ≥80% v/v.
For a sake of comparison, 1-POM and 4-POM showed the highest and the lowest impact on DB-1 folding, favoring, respectively, α-helix and β-strand conformations (Ventura et al., 2018; Tagliavini et al., 2021). In summary, the two spacers, Ttds and EEEEβA, have a relatively similar effect in terms of secondary structure evolution in H2O/TFE, and their behavior is intermediate with respect to the spacer-free and double-spacer POMs.
Interestingly, when POM and peptide are not conjugated (see Supplementary Figure S37, reporting the example for peptide 3 in the presence of 0.5 equiv. of POM-succ) no significant difference was observed in the CD spectra of peptide with or without POM at both the examined TFE percentages (10 and 40% v/v). This suggests that non-covalent interactions between POM and peptides are of intramolecular type and that the increase of dichroic signal observed for the hybrids can be due to an induction of chirality to the POM core in a less polar solvent, where intramolecular hydrogen bonds are promoted.
3.4 Fluorescence spectroscopy
Additional information on the interaction between peptides and POM was obtained analyzing the fluorescence emission of the Trp residue, being its emission dependent on the chemical surroundings. Comparing the emission wavelengths and the intensity of the Trp fluorescence in equimolar solutions of peptides alone or graphed to POM we did not observe a relevant shift of the maximum wavelength emission of Trp residue, suggesting that the POM did not alter the chemical surrounding of the Trp side-chain. Nevertheless, the emission intensity is always less intense in graphted peptides than in the corresponding free peptide, highlighting the occurrence of an energy transfer from the peptide to the POM (Zhang et al., 2007). Fluorescence quenching experiments were then performed adding cesium ions, to provide information on the exposure of the Trp side-chain. In Figure 5 the observed fluorescence (F), at λ= 295 nm, is reported, as F/F0, with respect to the initial one (F0). Instead of observing a further quenching of the fluorescence, however, Cs+ induced an increase in fluorescence emission, suggesting that the added ions disrupt the interaction between POM moiety and peptide, consequently making less efficient the energy transfer between the two domains (Tagliavini et al., 2021). The increase in fluorescent emission is more evident in 1-POM construct and decreases with increasing spacer length. 2-POM shows intermediate behavior, being also sensitive to the addition of Cs+ in terms of fluorescence recovery, while 3-POM and 2-POM appeared almost insensitive to Cs+. The behavior observed upon addition of KI has a trend in agreement with previous data, with fluorescence increasing for 1-POM>2-POM and fluorescence quenching being more effective for 4-POM than for 3-POM, that can only be quenched at higher KI concentrations (Supplementary Figure S38). In the same conditions, peptides 1-4 showed the expected quenching of the signal, with efficiency in the order 1>2>3=4, in agreement with the repulsion between negative charges of I− and Glu residues in 3 and 4.
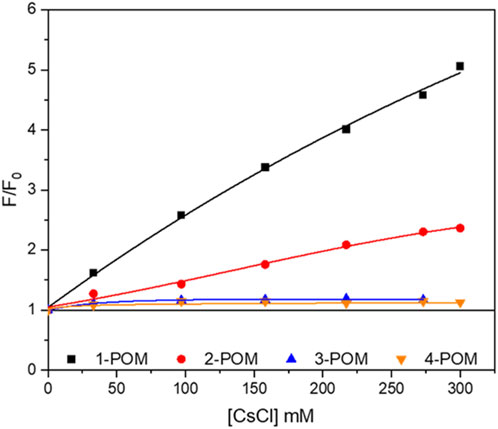
Figure 5. Fluorescence emission of POM-peptide hybrids (indicated) at increasing amounts of cesium ions (CsCl). Fluorescence is referred to as the ratio between the fluorescence at a given quencher concentration (F) and the fluorescence in its absence (F0).
These fluorescence experiments highlight a greater impact of the spacers in terms of peptide-POM interactions rather than POM-induced secondary structure evolution, demonstrating the need to attenuate them to increase peptide availability.
3.5 Transmission Electron Microscopy
The formation of aggregates may also have a strong impact on the accessibility of peptide chains. TEM was employed to achieve information about size and shape of the nanoparticles (Figure 6). In water with 3% DMSO, 1-POM forms particles with diameter up to 50 nm diameter (Tagliavini et al., 2021), 2-POM assembles into fibers of 200–300 nm in length, whereas 3-POM, in agreement with DLS data (showing particles with hydrodynamic diameter of 30–50 nm, see Supplementary Figure S39), forms amorphous particles with diameter smaller than 50 nm. Compound 4-POM shows entangled fibers in the 300–500 nm diameter range. To understand the role of POM, with respect to that of the peptides, in driving the morphology of the aggregates, we have also monitored the behavior of POM-TRIS, POM-succ and of a mixture of both. POM-TRIS (casted form acetonitrile solution) displays a tendency to align, forming fibers between 500 nm and 3 μm in length, as shown in Supplementary Figure S40, suggesting that the 1D morphology is likely dictated by POMs. On the contrary, POM-succ forms polyhedral crystals-like structures (Supplementary Figure S41). Moreover, fibers longer than 500 nm, differently from the ones formed by POM-TRIS, can be obtained by mixing equimolar amounts of POM-TRIS and POM-succ in acetonitrile, as represented in Supplementary Figure S42. Complementary interactions (amino groups/POM surface and amino groups/carboxylic groups) are thus also useful to establish directional interactions. Highly negatively charged POMs, as POM-succ and 3-POM, instead, only form nanoparticles.
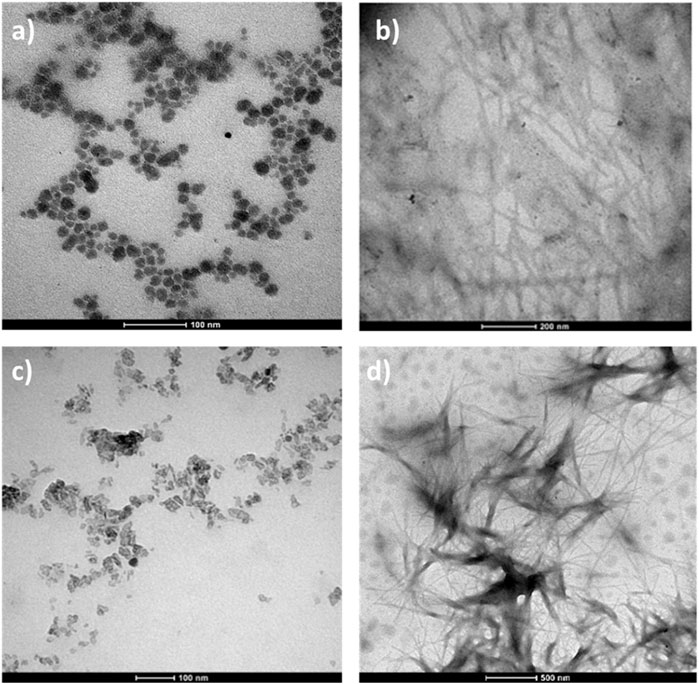
Figure 6. TEM measurements, 10−4 M solutions of a (A) 1-POM, scale bar =100 nm (B) 2-POM in 3% DMSO/water, scale bar =200 nm; (C) 3-POM in H2O, scale bar 100 nm; (D) 4-POM in 3% DMSO/water, scale bar 500 nm.
Noteworthy, in DMF as solvent, 2-POM showed a gelator effect, likely mediated by the polar Ttds chains (Supplementary Figure S43), as previously observed also for 4-POM (Tagliavini et al., 2021). These results underly how hybrid POMs may represent versatile building blocks, capable of exploiting both the polyanionic surface and the organic pendants to control the interactions.
3.6 MD simulations
From the data collected, it is evident that the spacer based on the tetra glutamic acid has a bigger impact than Ttds on improving the accessibility of the peptide chains. Indeed, POM-3 showed better behavior in terms of:
• retention of POM-free peptide’s secondary structure in DMSO-d6,
• higher accessibility of the peptide chain with respect to quenching by ions,
• lower tendency to aggregate.
MD simulations were thus performed to elucidate the structural features of POM-3, comparing the outcomes with those of 1-POM, in order to focus only on the effects of the addition of the anionic spacer. The structure and dynamics were studied by means of three independent 100 ns-long MD simulations for each compound, starting from an extended conformation for the peptide moiety. Such a starting conformation allows the secondary structure of both peptides to evolve in an unbiased way, highlighting possible differences. The analysis of the secondary structure sampled in the MD simulations is reported in Figure 7, where the time evolution of the fraction of β-structure, helical structure and unstructured peptide is shown for both compounds. It must be noted that a relevant amount of unstructured peptide is present in the MD simulations (≈50% on average), while in the experimental CD data a lower percentage of unstructured peptides was observed (32–35%). This can be ascribed to the relatively short simulation time (100 ns for each MD) that does not allow the peptides to assume a stable folded conformation. However, relevant structural differences between the two compounds can be already observed: in both compounds the peptides assume a β-structure, but in compound 1-POM a non-negligible fraction of helical structure is also present. The formation of helical structure is observed in two of the three simulations of 1-POM: in the third simulation, a particularly high unstructured fraction is present, and no helical structure is observed. 3-POM shows a bigger amount of β-structures. Similarly to the previous case, in the first simulation of compound 3-POM a more relevant percentage of helical structure is present with respect to the other two simulations of the same compound. The statistical sampling of different secondary structures is not surprising in MD simulations starting from an extended conformation. However, the difference in the average secondary structure content between the two compounds appears to be meaningful.
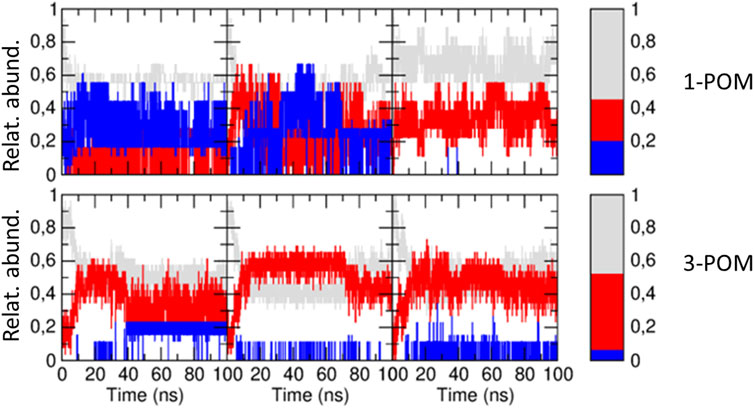
Figure 7. Time evolution of the relative abundance of β-structure (red), helical structure (blue) and unstructured peptide (gray) along the three independent MD simulations of compound 1-POM (upper panels) and compound 3-POM (lower panels). In the right panels, the same fractions, averaged along the three simulations, are reported for both compounds. To calculate such average values, the first 10 ns of each MD simulation have been neglected.
During the MD simulations, the peptide bent on the POM. In some cases, the POM-peptide interaction was stabilized by the formation of hydrogen bonds between the peptide and the oxygen atoms of the POM. An example of such bending is reported in Figure 8. The bending of the peptide on the POM moiety, monitored along the six MD simulations by plotting the distance between the peptide terminal residue and the POM center of mass, does not seem to be related to the structural differences. In fact, a similar distribution for the terminal residue to POM distance is obtained for both compounds (Supplementary Figure S44). This fact is important to explain the presence of interactions despite the presence of electrostatic repulsions and deserves attention, in terms or length of the peptide and functionality at the terminal end, for further structural optimization.
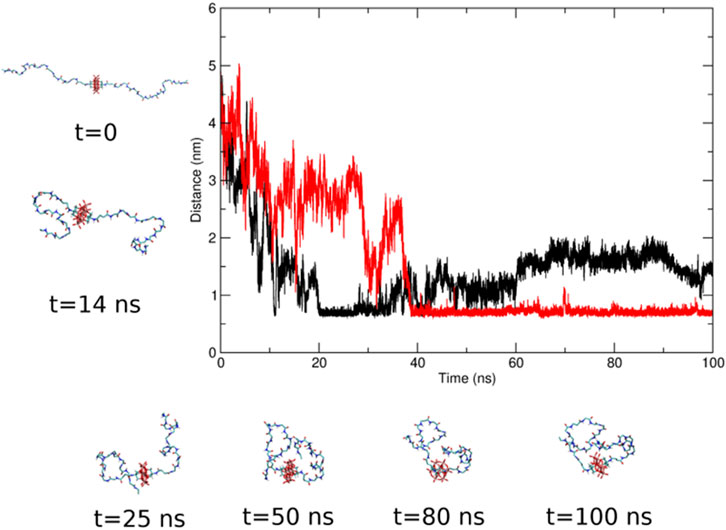
Figure 8. Time evolution of the distance between the terminal residue of the two peptides and the center of mass of the POM moiety in one of the MD simulations of compound 3-POM. The black and red lines refer to the two pendants. Some representative snapshots of the POM-peptides conformation are also shown.
The interactions between the two peptide chains, belonging to the same POM, were finally investigated for the two compounds, revealing that, in compound 1-POM, more interactions between the two peptide chains are present compared to compound 3-POM. This can be observed from Figure 9, where the time fraction of interchain contacts along the simulations of the two compounds is reported. Such a fraction is defined as follows. Being Ri and Rj the ith and jth residues of the two peptide chains, respectively, a contact between Ri and Rj is present at each MD frame in which the minimum distance d between Ri and Rj is below 0.5 nm. Figure 10 clearly shows that the two peptide chains are more frequently interacting during the MD simulations of compound 1-POM. The increased interchain interactions between the two peptides disfavor the formation of intrachain interactions. As a matter of fact, it can be observed in Supplementary Figure S45 that the number of intrachain hydrogen bonds (HB) in 3-POM is higher with respect to that in 1-POM. Nonetheless, the same Figure also shows that the number of interchain HBs is similar for 1-POM and 3-POM, suggesting that hydrogen bonding is not the main interchain interaction. Inspection of the trajectories reveals that interchain contacts in 1-POM are stabilized by aromatic interactions among His, Phe and Trp residues, that are often arranged in a sort of aromatic cleft (Supplementary Figure S46).
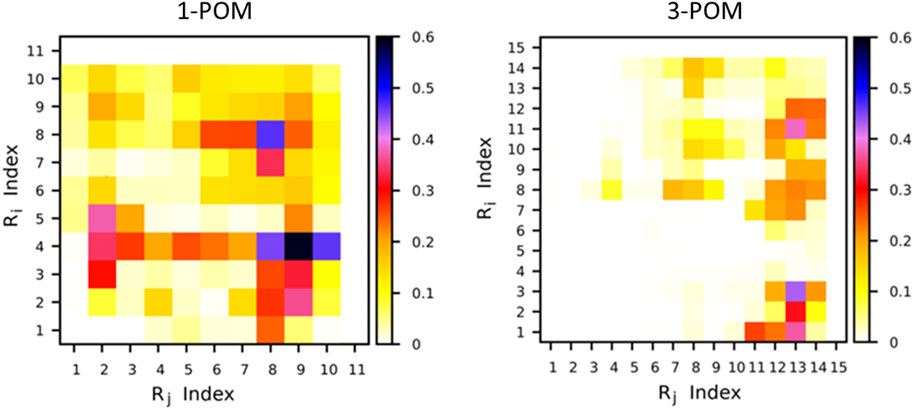
Figure 9. Fraction of interchain contacts in compounds 1-POM (left) and 3-POM (right). The color code represents the fraction of MD frames at which an Ri Rj contact is present.
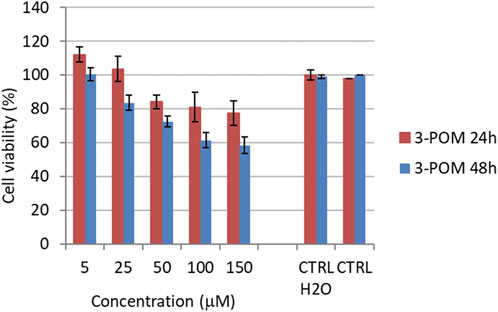
Figure 10. Cytotoxicity performances of 3-POM on HeLa cells at increasing concentration. Results are presented after 24 and 48 h.
The amount of such transient interactions is likely related to the different conformations observed; in particular, they can make the chains less available for longer range interactions required for β-structures as well as for targeting purposes.
3.7 Cytotoxicity tests
The toxicity assessments were performed on HeLa cervical cancer cells, which show a moderate overexpression of the bombesin receptor. While the peptides 2 and 3, as well as 2-POM exhibit only a minor anticancer activity, (IC50>200 μM), 3-POM led to 58% residual viability at 150 μM after 48 h (IC50 180 μM at 48 h, Figure 10). With respect to POM-TRIS (IC50=134 μM, with 46% residual viability at 150 μM after 48 h) and 4-POM (IC50=95 μM, with 33% residual viability at 150 μM after 48 h), the 3-POM is, thus, less active. However, with respect to 1-POM, which was also less active than POM-TRIS (Ventura et al., 2018), it shows a better activity. Moreover, the stronger effect observed at 25 μM of 3-POM, with respect to POM-TRIS, speaks in favor of a higher selectivity, at least, in the low concentration range (Table 1). These outcomes are consistent with the data herein collected, confirming, on one hand, the significant impact of tetra glutamic acid domain, on the other hand, the need of combining the two spacers to achieve a stronger biological activity.
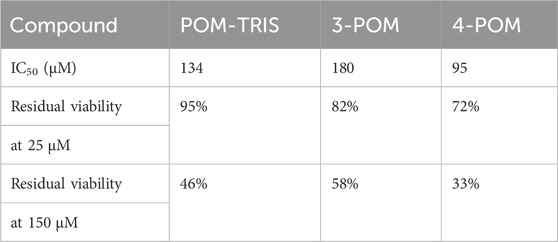
Table 1. IC50 values ad residual viability, observed for HeLa cells treated with POMs, after 48 h incubation.
4 Conclusion
This study delves into the asymmetric environment of novel Mn-Anderson POM-hybrids with potential anticancer activity. Specifically, distinct spacers, EEEEβA and Ttds, were introduced between the POM core and the pendant peptides, with the aim to maintain the peptide chains easily accessible even after grafting, so to facilitate the targeting of cancer cells. Peptide folding is, indeed, a common phenomenon in the presence of salts or other chaotropic agents, including POMs, and could lead to a decrease of biological activity (Soria-Carrera et al., 2023).
The incorporation of spacers could mitigate the influence of the POM cluster. According to 2D NMR, among the two spacers, EEEEβA and Ttds, the first proved to be more useful in minimizing molecular interactions. The negatively charged spacer, indeed, was confirmed to have a positive effect, as 3-POM and 4-POM showed lower CHα shifts compared to the free peptide. In contrast, compounds 1-POM and 2-POM displayed more differences in the secondary structure compared to bare Demobesin-1, favouring the formation of α-helix in DMSO-d6. However, the impact of the two spacers on secondary structure seemed not relevant in TFA/H2O solutions: CD spectroscopy did not highlight a meaningful difference between 2-POM and 3-POM, which both displayed intermediate behavior with respect to 1-POM and 4-POM. Quenching of fluorescence, instead, underscored the positive effect conferred by the charged spacer, with compounds 1-POM and 2-POM exhibiting no dynamic quenching upon the addition of both KI and CsCl, while 3-POM and 4-POM showed better responsivity of the side chain Trp.
MD calculation showed that 3-POM secondary structure is mostly composed by β-sheet and random coil conformations, with minor interchain interactions. Despite the high accessibility of the chains, however, they still tend to fold on the POM structure, exploiting the terminal residues for the interactions. For this reason, the biological activity of 3-POM towards HeLa cells appears still low, and justifies the need for a longer spacer, provided by the addition of Ttds, as for 4-POM. This observation may be crucial to overcome the general problem of undesired POM-triggered peptide folding, and will be useful to drive the design of a next generation of POM-based drugs, with possibility to control targeting and delivery, while enabling mechanistic studies for the still unclear mechanism of action of anticancer POMs. Further studied will evaluate the nature of POM scaffold.
Data availability statement
The original contributions presented in the study are included in the article/Supplementary Material, further inquiries can be directed to the corresponding authors.
Author contributions
HY: Data curation, Writing–original draft, Formal Analysis, Investigation, Software. CH: Methodology, Validation, Writing–original draft, Formal Analysis, Investigation, Software. MF: Formal Analysis, Investigation, Software, Writing–original draft. NB: Formal Analysis, Investigation, Software, Writing–original draft. VT: Formal Analysis, Investigation, Writing–original draft. XZ: Formal Analysis, Investigation, Writing–original draft. ES: Formal Analysis, Investigation, Writing–original draft. AC: Writing–original draft, Data curation. SS: Writing–original draft, Investigation, Formal Analysis, Methodology. AA: Formal Analysis, Writing–original draft, Data curation, Methodology. LP: Writing–original draft, Formal Analysis, Methodology. LZ: Writing–original draft, Methodology, Software, Validation. SC: Writing–original draft, Software, Validation, Methodology. PR: Conceptualization, Data curation, Methodology, Resources, Supervision, Validation, Writing–original draft, Writing–review and editing. MC: Methodology, Writing–original draft, Conceptualization, Data curation, Project administration, Resources, Supervision, Validation, Writing–review and editing.
Funding
The author(s) declare financial support was received for the research, authorship, and/or publication of this article. This work was supported by the Department of Chemical Sciences of the University of Padova (grant number P-DiSC#11NExuS_BIRD2019-UNIPD) and by MUR (project PRIN2022CAS9ZT).
Acknowledgments
HY thanks the China Scholarship Council for funding her PhD in Molecular Science at Padova University.
Conflict of interest
The authors declare that the research was conducted in the absence of any commercial or financial relationships that could be construed as a potential conflict of interest.
Publisher’s note
All claims expressed in this article are solely those of the authors and do not necessarily represent those of their affiliated organizations, or those of the publisher, the editors and the reviewers. Any product that may be evaluated in this article, or claim that may be made by its manufacturer, is not guaranteed or endorsed by the publisher.
Supplementary material
The Supplementary Material for this article can be found online at: https://www.frontiersin.org/articles/10.3389/fchbi.2024.1377357/full#supplementary-material
References
Albada, B., and Metzler-Nolte, N. (2016). Organometallic-peptide bioconjugates: synthetic strategies and medicinal applications. Chem. Rev. 116 (19), 11797–11839. doi:10.1021/acs.chemrev.6b00166
Aprikian, A. G., Han, K., Chevalier, S., Bazinet, M., and Viallet, J. (1996). Bombesin specifically induces intracellular calcium mobilization via gastrin-releasing peptide receptors in human prostate cancer cells. J. Mol. Endocrinol. 16 (3), 297–306. doi:10.1677/jme.0.0160297
Arefian, M., Mirzaei, M., Eshtiagh-Hosseini, H., and Frontera, A. (2017). A survey of the different roles of polyoxometalates in their interaction with amino acids, peptides and proteins. Dalton Trans. 46, 6812–6829. doi:10.1039/C7DT00894E
Bayly, C. I., Cieplak, P., Cornell, W., and Kollman, P. A. (1993). A well-behaved electrostatic potential based method using charge restraints for deriving atomic charges: the RESP model. J. Phys. Chem. 97 (40), 10269–10280. doi:10.1021/j100142a004
Becke, A. D. (1993). Density-functional thermochemistry. III. The role of exact exchange. J. Chem. Phys. 98 (7), 5648–5652. doi:10.1063/1.464913
Berendsen, H. J. C., Grigera, J. R., and Straatsma, T. P. (1987). The missing term in effective pair potentials. J. Phys. Chem. 91 (24), 6269–6271. doi:10.1021/j100308a038
Berendsen, H. J. C., Postma, J. P. M., van Gunsteren, W. F., DiNola, A., and Haak, J. R. (1984). Molecular dynamics with coupling to an external bath. J. Chem. Phys. 81 (8), 3684–3690. doi:10.1063/1.448118
Bijelic, A., Aureliano, M., and Rompel, A. (2018). The antibacterial activity of polyoxometalates: structures, antibiotic effects and future perspectives. Chem. Commun. 54 (10), 1153–1169. doi:10.1039/C7CC07549A
Bijelic, A., Aureliano, M., and Rompel, A. (2019). Polyoxometalates as potential next-generation metallodrugs in the combat against cancer. Angew. Chem. Int. Ed. 58 (10), 2980–2999. doi:10.1002/anie.201803868
Blazevic, A., and Rompel, A. (2016). The Anderson–Evans polyoxometalate: from inorganic building blocks via hybrid organic–inorganic structures to tomorrows “Bio-POM”. Coord. Chem. Rev. 307, 42–64. doi:10.1016/j.ccr.2015.07.001
Bussi, G., Donadio, D., and Parrinello, M. (2007). Canonical sampling through velocity rescaling. J. Chem. Phys. 126 (1), 014101. doi:10.1063/1.2408420
Cameron, J. M., Guillemot, G., Galambos, T., Amin, S. S., Hampson, E., Mall Haidaraly, K., et al. (2022). Supramolecular assemblies of organo-functionalised hybrid polyoxometalates: from functional building blocks to hierarchical nanomaterials. Chem. Soc. Rev. 51 (1), 293–328. doi:10.1039/D1CS00832C
Carvalho, F., and Aureliano, M. (2023). Polyoxometalates impact as anticancer agents. Int. J. Mol. Sci. 24 (5), 5043. doi:10.3390/ijms24055043
Cescato, R., Maina, T., Nock, B., Nikolopoulou, A., Charalambidis, D., Piccand, V., et al. (2008). Bombesin receptor antagonists may Be preferable to agonists for tumor targeting. J. Nucl. Med. 49 (2), 318–326. doi:10.2967/jnumed.107.045054
Chang, D., Li, Y., Chen, Y., Wang, X., Zang, D., and Liu, T. (2022). Polyoxometalate-based nanocomposites for antitumor and antibacterial applications. Nanoscale Adv. 4 (18), 3689–3706. doi:10.1039/D2NA00391K
Čolović, M. B., Lacković, M., Lalatović, J., Mougharbel, A. S., Kortz, U., and Krstić, D. Z. (2020). Polyoxometalates in biomedicine: update and overview. Curr. Med. Chem. 27 (3), 362–379. doi:10.2174/0929867326666190827153532
Dan, K., Fujinami, K., Sumitomo, H., Ogiwara, Y., Suhara, S., Konno, Y., et al. (2020). Application of antiviral polyoxometalates to living environments—antiviral moist hand towels and stationery items. Appl. Sci. 10 (22), 8246. doi:10.3390/app10228246
Darden, T., York, D., and Pedersen, L. (1993). Particle mesh Ewald: an N⋅log(N) method for Ewald sums in large systems. J. Chem. Phys. 98 (12), 10089–10092. doi:10.1063/1.464397
Dolbecq, A., Dumas, E., Mayer, C. R., and Mialane, P. (2010). Hybrid Organic−Inorganic polyoxometalate compounds: from structural diversity to applications. Chem. Rev. 110 (10), 6009–6048. doi:10.1021/cr1000578
Fabbian, S., Giachin, G., Bellanda, M., Borgo, C., Ruzzene, M., Spuri, G., et al. (2022). Mechanism of CK2 inhibition by a ruthenium-based polyoxometalate. Front. Mol. Biosci. 9, 906390. doi:10.3389/fmolb.2022.906390
Gugger, M., and Reubi, J. C. (1999). Gastrin-releasing peptide receptors in non-neoplastic and neoplastic human breast. Am. J. Pathology 155 (6), 2067–2076. doi:10.1016/S0002-9440(10)65525-3
Hay, P. J., and Wadt, W. R. (1985). Ab initio effective core potentials for molecular calculations. Potentials for K to Au including the outermost core orbitals. J. Chem. Phys. 82 (1), 299–310. doi:10.1063/1.448975
Hess, B., Bekker, H., Berendsen, H. J. C., and Fraaije, JGEM (1997). LINCS: a linear constraint solver for molecular simulations. J. Comput. Chem. 18 (12), 1463–1472. doi:10.1002/(SICI)1096-987X(199709)18:12<1463::AID-JCC4>3.0.CO;2-H
Hosseini, M. S., Haghjooy Javanmard, S., Dana, N., Rafiee, L., and Rostami, M. (2021). Novel tocopherol succinate-polyoxomolybdate bioconjugate as potential anti-cancer agent. J. Inorg. Organomet. Polym. Mater. 31 (7), 3183–3195. doi:10.1007/s10904-021-01998-z
Hussain, R., Benning, K., Javorfi, T., Longo, E., Rudd, T. R., Pulford, B., et al. (2015). CDApps: integrated software for experimental planning and data processing at beamline B23, Diamond Light Source. J. Synchrotron Radiat. 22 (2), 465–468. doi:10.1107/S1600577514028161
Jea Frisch, M., Trucks, G. W., Schlegel, H. B., Scuseria, G. E., Robb, M. A., Cheeseman, J. R., et al. (2009). Gaussian 09, revision a. 1. Wallingford, CT: Gaussian Inc.
Kabsch, W., and Sander, C. (1983). Dictionary of protein secondary structure: pattern recognition of hydrogen-bonded and geometrical features. Biopolymers 22 (12), 2577–2637. doi:10.1002/bip.360221211
Krishnan, R., Binkley, J. S., Seeger, R., and Pople, J. A. (1980). Self-consistent molecular orbital methods. XX. A basis set for correlated wave functions. J. Chem. Phys. 72 (1), 650–654. doi:10.1063/1.438955
Lentink, S., Salazar Marcano, D. E., Moussawi, M. A., Vandebroek, L., Van Meervelt, L., and Parac-Vogt, T. (2023). Fine-tuning non-covalent interactions between hybrid metal-oxo clusters and proteins. Faraday Discuss. 244 (0), 21–38. doi:10.1039/D2FD00161F
Luo, J., Zhang, B., Yvon, C., Hutin, M., Gerislioglu, S., Wesdemiotis, C., et al. (2019). Self-assembly of polyoxometalate–peptide hybrids in solution: elucidating the contributions of multiple possible driving forces. Eur. J. Inorg. Chem. 2019, 380–386. doi:10.1002/ejic.201800158
Ma, Z., Wei, D., Yan, P., Zhu, X., Shan, A., and Bi, Z. (2015). Characterization of cell selectivity, physiological stability and endotoxin neutralization capabilities of α-helix-based peptide amphiphiles. Biomaterials 52, 517–530. doi:10.1016/j.biomaterials.2015.02.063
Mahvash, S., Zavareh, V. A., Taymouri, S., Mirian, M., Ramezani-Aliakbari, M., Dousti, F., et al. (2023). Anderson-type manganese polyoxomolybdate hybrid nanocomposite for boosting drug delivery against breast cancer. J. Drug Deliv. Sci. Technol. 87, 104778. doi:10.1016/j.jddst.2023.104778
Markwalder, R., and Reubi, J. C. (1999). Gastrin-releasing peptide receptors in the human prostate: relation to neoplastic transformation. Cancer Res. 59 (5), 1152–1159.
Modugno, G., Fabbretti, E., Dalle Vedove, A., Da Ros, T., Maccato, C., Hosseini, H. S., et al. (2018). Tracking fluorescent polyoxometalates within cells. Eur. J. Inorg. Chem. 2018 (46), 4955–4961. doi:10.1002/ejic.201800802
Nock, B., Nikolopoulou, A., Chiotellis, E., Loudos, G., Maintas, D., Reubi, J., et al. (2003). [99mTc]Demobesin 1, a novel potent bombesin analogue for GRP receptor-targeted tumour imaging. Eur. J. Nucl. Med. Mol. Imaging 30 (2), 247–258. doi:10.1007/s00259-002-1040-x
Omwoma, S., Gore, C. T., Ji, Y., Hu, C., and Song, Y. F. (2015). Environmentally benign polyoxometalate materials. Coord. Chem. Rev. 286, 17–29. doi:10.1016/j.ccr.2014.11.013
Parr, R. G., and Yang, W. (1995). Density-functional theory of the electronic structure of molecules. Annu. Rev. Phys. Chem. 46 (1), 701–728. doi:10.1146/annurev.pc.46.100195.003413
Pooja, D., Gunukula, A., Gupta, N., Adams, D. J., and Kulhari, H. (2019). Bombesin receptors as potential targets for anticancer drug delivery and imaging. Int. J. Biochem. Cell Biol. 114, 105567. doi:10.1016/j.biocel.2019.105567
Ramezani-Aliakbari, M., Soltanabadi, A., Sadeghi-aliabadi, H., Varshosaz, J., Yadollahi, B., Hassanzadeh, F., et al. (2021). Eudesmic acid-polyoxomolybdate organo-conjugate as novel anticancer agent. J. Mol. Struct. 1240, 130612. doi:10.1016/j.molstruc.2021.130612
Rosnes, M. H., Yvon, C., Long, D. L., and Cronin, L. (2012). Mapping the synthesis of low nuclearity polyoxometalates from octamolybdates to Mn-Anderson clusters. Dalton Trans. 41 (33), 10071–10079. doi:10.1039/C2DT31008B
Rozengurt, E. (1998). Signal transduction pathways in the mitogenic response to G protein–coupled neuropeptide receptor agonists. J. Cell. Physiology 177 (4), 507–517. doi:10.1002/(SICI)1097-4652(199812)177:4<507::AID-JCP2>3.0.CO;2-K
Rubini, C., Ruzza, P., Spaller, M. R., Siligardi, G., Hussain, R., Udugamasooriya, D. G., et al. (2010). Recognition of lysine-rich peptide ligands by murine cortactin SH3 domain: CD, ITC, and NMR studies. Biopolymers 94 (3), 298–306. doi:10.1002/bip.21350
Schally, A. V., and Nagy, A. (1999). Cancer chemotherapy based on targeting of cytotoxic peptide conjugates to their receptors on tumors. Eur. J. Endocrinol. 141 (1), 1–14. doi:10.1530/eje.0.1410001
Schmid, N., Eichenberger, A. P., Choutko, A., Riniker, S., Winger, M., Mark, A. E., et al. (2011). Definition and testing of the GROMOS force-field versions 54A7 and 54B7. Eur. Biophysics J. 40 (7), 843–856. doi:10.1007/s00249-011-0700-9
Soria-Carrera, H., Atrián-Blasco, E., Martín-Rapún, R., and Mitchell, S. G. (2023). Polyoxometalate–peptide hybrid materials: from structure–property relationships to applications. Chem. Sci. 14 (1), 10–28. doi:10.1039/D2SC05105B
Tagliavini, V., Honisch, C., Serratì, S., Azzariti, A., Bonchio, M., Ruzza, P., et al. (2021). Enhancing the biological activity of polyoxometalate–peptide nano-fibrils by spacer design. RSC Adv. 11 (9), 4952–4957. doi:10.1039/D0RA10218K
Tremblay, M.-L., Banks, A. W., and Rainey, J. K. (2010). The predictive accuracy of secondary chemical shifts is more affected by protein secondary structure than solvent environment. J. Biomol. NMR 46 (4), 257–270. doi:10.1007/s10858-010-9400-5
Van Der Spoel, D., Lindahl, E., Hess, B., Groenhof, G., Mark, A. E., and Berendsen, H. J. C. (2005). GROMACS: fast, flexible, and free. J. Comput. Chem. 26 (16), 1701–1718. doi:10.1002/jcc.20291
Ventura, D., Calderan, A., Honisch, C., Krol, S., Serratì, S., Bonchio, M., et al. (2018). Synthesis and biological activity of an Anderson polyoxometalate bis-functionalized with a Bombesin-analog peptide. Peptide Sci. 110 (5), e24047. doi:10.1002/pep2.24047
Vincenzi, M., Mercurio, F. A., and Leone, M. (2019). About TFE: old and new findings. Curr. protein & peptide Sci. 20 (5), 425–451. doi:10.2174/1389203720666190214152439
Wang, L., Yu, K., Zhou, B. B., Su, Z. H., Gao, S., Chu, L. L., et al. (2014). The inhibitory effects of a new cobalt-based polyoxometalate on the growth of human cancer cells. Dalton Trans. 43 (16), 6070–6078. doi:10.1039/C3DT53030B
Wang, S.-S., and Yang, G.-Y. (2015). Recent advances in polyoxometalate-catalyzed reactions. Chem. Rev. 115 (11), 4893–4962. doi:10.1021/cr500390v
Wu, L., and Liang, J. (2017). “Polyoxometalates and their complexes toward biological application,” in Supramolecular Chemistry of biomimetic systems. Editor J. Li (Singapore: Springer), 311–354. doi:10.1007/978-981-10-6059-5_13
Yang, H.-K., Cheng, Y. X., Su, M. M., Xiao, Y., Hu, M. B., Wang, W., et al. (2013). Polyoxometalate–biomolecule conjugates: a new approach to create hybrid drugs for cancer therapeutics. Bioorg. Med. Chem. Lett. 23 (5), 1462–1466. doi:10.1016/j.bmcl.2012.12.081
Yu, B., Zhao, X., Ni, J., and Yang, F. (2023). Multiscale assembly of polyoxometalates: from clusters to materials. ChemPhysMater 2 (1), 20–29. doi:10.1016/j.chphma.2022.03.006
Yvon, C., Surman, A. J., Hutin, M., Alex, J., Smith, B. O., Long, D., et al. (2014). Polyoxometalate clusters integrated into peptide chains and as inorganic amino acids: solution- and solid-phase approaches. Angew. Chem. Int. Ed. 53 (13), 3336–3341. doi:10.1002/anie.201311135
Zamolo, V. A., Modugno, G., Lubian, E., Cazzolaro, A., Mancin, F., Giotta, L., et al. (2018). Selective targeting of proteins by hybrid polyoxometalates: interaction between a bis-biotinylated hybrid conjugate and avidin. Front. Chem. 6, 278. doi:10.3389/fchem.2018.00278
Keywords: peptides, polyoxometalates, targeting, 2D NMR, MD calculations
Citation: Yu H, Honisch C, Frigo M, Balice N, Tagliavini V, Zhao X, Stramiglio E, Campofelice A, Serratì S, Azzariti A, Porcelli L, Zanetti Polzi L, Corni S, Ruzza P and Carraro M (2024) Impact of different spacers on the conjugation between Anderson-Evans polyoxometalates and peptides. Front. Chem. Biol 3:1377357. doi: 10.3389/fchbi.2024.1377357
Received: 27 January 2024; Accepted: 12 March 2024;
Published: 25 March 2024.
Edited by:
Lukáš Krivosudský, Comenius University, SlovakiaReviewed by:
Nadiia I. Gumerova, University of Vienna, AustriaRami Oweini, University of La Verne, United States
Copyright © 2024 Yu, Honisch, Frigo, Balice, Tagliavini, Zhao, Stramiglio, Campofelice, Serratì, Azzariti, Porcelli, Zanetti Polzi, Corni, Ruzza and Carraro. This is an open-access article distributed under the terms of the Creative Commons Attribution License (CC BY). The use, distribution or reproduction in other forums is permitted, provided the original author(s) and the copyright owner(s) are credited and that the original publication in this journal is cited, in accordance with accepted academic practice. No use, distribution or reproduction is permitted which does not comply with these terms.
*Correspondence: Paolo Ruzza, cGFvbG8ucnV6emFAdW5pcGQuaXQ=; Mauro Carraro, bWF1cm8uY2FycmFyb0B1bmlwZC5pdA==