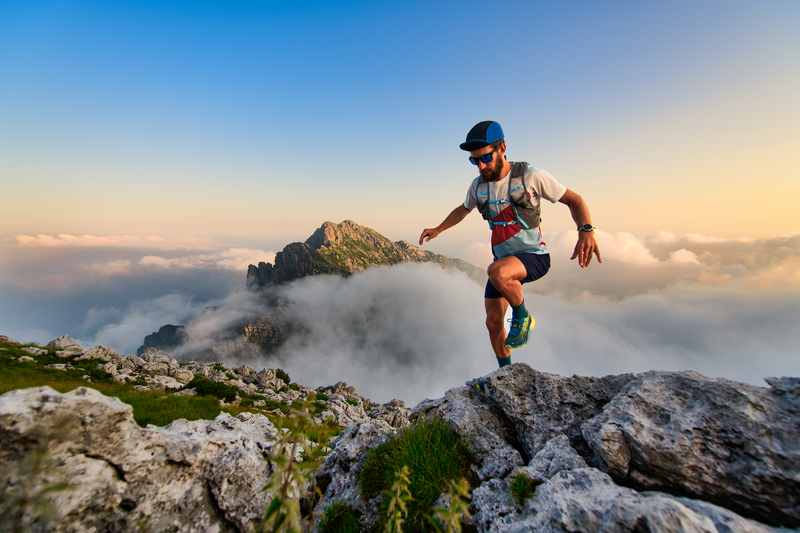
94% of researchers rate our articles as excellent or good
Learn more about the work of our research integrity team to safeguard the quality of each article we publish.
Find out more
PERSPECTIVE article
Front. Chem. Biol. , 10 January 2024
Sec. Bioinorganic Chemistry
Volume 2 - 2023 | https://doi.org/10.3389/fchbi.2023.1346465
This article is part of the Research Topic Linking Chemistry to Biology and Medicine via Metal ions: Developed from the 16th International Symposium on Applied Bioinorganic Chemistry View all 3 articles
Porphyrin complexes are present in many natural systems and have significant biological roles, such as light harvesting, oxygen transport, and catalysis. Owing to their intrinsic aromatic structure, porphyrin derivatives exhibit characteristic photophysical and electrochemical properties. Porphyrins and porphyrin-based derivatives have been extensively utilized in biomedical applications during the last decade. Specifically, porphyrinoids have been tested as agents in antimicrobial and photodynamic therapy, as well as in imaging applications (e.g., diagnosis of cancer cells). This perspective article summarizes the recent developments in our group concerning the application of porphyrin derivatives in biomedical applications. The current challenges and future prospects concerning the exploitation of porphyrin-based materials in biomedical applications are also discussed.
Porphyrinoids, frequently mentioned as “pigments of life” (Battersby, 2000), are tetrapyrrole derivatives with significant importance in natural occurring systems. Among the most well-known natural tetrapyrroles: i) heme is a porphyrin present in hemoglobin, being responsible for oxygen transport in blood, ii) chlorophyll (a chlorin) is the essential component in photosynthesis, by trapping sunlight, and iii) bacteriochlorophyll is a bacteriochlorin accountable for the photosynthesis in bacteria (Taniguchi and Lindsey, 2017). Ever since the seminal work of Battersby and co-workers regarding “pigments of life” (Battersby and McDonald, 1979; Battersby et al., 1980), porphyrin-based derivatives (and/or materials) were widely prepared for numerous applications due to their exceptional synthetic versatility, photo-, electro-, and thermal-stability, along with their unique photoelectrochemical features. The name of porphyrin derivatives originates from the Greek word “porphyra” which was used by ancient Greeks to describe the intense purple colour. From the structural viewpoint, porphyrin derivatives are composed of four pyrrolic units that are linked in a coplanar fashion by four methene bridges, resulting in a planar macrocyclic structure. It has an extended conjugated 18 π-electron system which is responsible for its aromatic nature, and its central cavity enables the accommodation of large number of metal cations.
On account of their substantial absorption in the visible region (400–450 nm Soret band, and 500–700 nm Q-bands) (Kim et al., 2008), as well as their ability to act as electron/energy donors/acceptors, porphyrins have been examined as artificial photosynthetic mimics (Bottari et al., 2012) and utilized in various solar cell technologies (Urbani et al., 2014; Zeng et al., 2020; Piradi et al., 2021; Molina et al., 2023). In addition, porphyrins (particularly metalloporphyrins), are considered as one of the most effective class of biomimetic catalysts (both in photo- and electro-catalysis), due to their significant catalytic activity in a great variety of chemical processes (Lin et al., 2021; Huang et al., 2022; Nikoloudakis et al., 2022; O'Neill et al., 2022; Domingo-Tafalla et al., 2023). Because of their tuneable optoelectronic properties, porphyrins are also used in molecular recognition and metal-ion sensing applications (Ogoshi and Mizutani, 1999; Ishizuka et al., 2022). Moreover, features of porphyrinoids such as biocompatibility, biological function mimicking, extended residence in cancer cells, are appealing properties for biomedical applications (Tian et al., 2023; Wang et al., 2023; Wu et al., 2023). For instance, photodynamic therapy (PDT) is a field dominated by the utilization of porphyrins (Mrinalini et al., 2021).
Researchers worldwide, have been utilizing porphyrinoinds for bio-imaging applications and drug delivery (Huang et al., 2015; Jenkins et al., 2016). This led to the development of porphyrin-based compounds as theragnostic agents in Magnetic Resonance Imaging (MRI), drug delivery and photodynamic cancer therapy (Stender et al., 2013; Cheng et al., 2014; Hammerer et al., 2014; Dong et al., 2017). Generally, porphyrins are used in the fields of disease treatment and in vivo diagnosis (Han et al., 2023). Regarding disease treatment, porphyrins can effectively treat inflammation and tumors since are able to produce reactive oxygen species (ROS) namely, singlet oxygen (1O2), hydroxyl and superoxide radicals, and hydrogen peroxide (Bustos et al., 2020; Wu et al., 2021). Apart from their exploitation in photodynamic therapy though, porphyrins can also have a photothermal therapy (PTT) effect against inflammation and tumors (Miao et al., 2021; Zhang et al., 2021). Concerning in vivo diagnosis, porphyrinoids can serve as organic ligands that coordinate paramagnetic ions (contrast agents for MRI) or as fluorescence chromophores, which both enable diagnosis in deep tissues (Egeblad and Werb, 2002; Zhang et al., 2007). Their intrinsic characteristics, such as: i) long resistance in tumors, ii) ability to mimic a great variety of biological processes, iii) biocompatibility, and iv) minimal side effects are the most essential advantages that established them as potential agents in biomedical applications. Nevertheless, porphyrins are subjected to significant limitations, namely, nonspecific targeting, instability, self-quenching under physiological conditions. Researchers typically overcome these issues by incorporating porphyrins with carriers such as liposomes, inorganic particles, polymers, micelles, etc (Huang et al., 2017; Managa et al., 2018; Wang et al., 2018; Zhang et al., 2018).
Our research group, explored the features and behaviour of porphyrins in various biomedical applications (Figure 1). More specifically, a water-soluble porphyrin was encapsulated in a hydrogel as healing agent to treat skin defects in rats (Dontas et al., 2023). In another recent report, βCD-triphenylporphyrin conjugates found to specifically target and accumulate in lysosomes effectively acting as drug release agents (Panagiotakis et al., 2023). Similarly to the latter example, water insoluble tetraphenylporphyrins bearing a single carboxyalkyl side-arm were synthesized, showing remarkable photostability and a nearly 90% photokilling efficiency toward cancer cells (Panagiotakis et al., 2022). We have also examined the ability of porphyrin-peptide hybrids as labelling agents, in order to potentially be tested as theragnostic agents (Glymenaki et al., 2022). Finally, peptide nucleic acids (PNAs) were connected to photosensitizers (bodipy and porphyrin) to develop compounds for photodynamic therapy (PDT) (Chang et al., 2020).
FIGURE 1. Scheme showing the different biomedical fields for application of porphyrin derivatives explored in our research group.
During the last decades, various approaches regarding the treatment and healing of skin defects have been explored (Takeo et al., 2015; Kamolz et al., 2022). The query though that frequently rises in clinical trials, is which treatment plan can be followed in each individual trauma. In many cases, a period of treatment with an effective agent is followed by the application of an alternative agent, since the wound healing process reaches a plateau (Seaman, 2002). Surgical procedures are arguably the most effective strategy to deal with widespread skin defects, namely, xenografts, autologous full- or split-thickness and allografts (Kamolz et al., 2022). However, apart from the aforementioned surgical techniques, hydrogels have also been extensively studied due to their unique features in healing skin defects (Loo et al., 2014). More specifically, hydrogels are biocompatible, uphold the desired hydration for the wound, are transparent which enables the observation of the healing process, provide gaseous permeability, and absorb the wound exudate (Amin and Abdel-Raheem, 2014; Li et al., 2021; Nuutila and Eriksson, 2021).
As a result, diverse hydrogels have been developed with the intention of creating a favourable environment for wound healing trials (Elangwe et al., 2022; Guamba et al., 2023). Self-assembling peptide hydrogels is one form of bioactive material tested in tissue regeneration and wound healing (Loo et al., 2014; Loo et al., 2015). This type of hydrogels is gaining considerable research attention as a result of their bioavailability and bioactivity. In addition, the encapsulation of a photosensitizer with antimicrobial properties within them is feasible. One of the most appropriate dipeptide, that act as a hydrogelator is Fmoc (9-fluorenylmethoxy-carbonyl) protected phenylalanine-phenylalanine (Fmoc-FF). Fmoc-FF hydrogels are able to encapsulate and release small molecules in regulating manner promoting adhesion and proliferation (Mahler et al., 2006; Fichman and Gazit, 2014). In addition, hydrogels of Fmoc-FF are being formed with an excellent stability and are also studied because of their chemical simplicity and versatility (Arakawa et al., 2020; Martinez-Serrano et al., 2022). Porphyrinoids have been used as encapsulated photosensitizers in hydrogels, since are able to produce ROS and possess great photostability (Li et al., 2021; Ding et al., 2021; Martinez-Serrano et al., 2022). Their good biocompatibility, oxygen transfer, and molecular recognition outline porphyrnoids as ideal candidates for killing various microorganisms (McKenzie et al., 2019; Dias et al., 2020). Remarkably, in nature peptides and porphyrins work together to arrange molecules in an orderly fashion which is crucial for biological functions. For example, in the light-harvesting complex of Rhodopseudomonas acidophila’s light-harvesting complex, the self-organization of porphyrinoids is being regulated by peptides (Frank and Cogdell, 2012).
Various porphyrin-based hydrogels have been investigated as antimicrobial agents in the last decades (Bonnett et al., 1993; Cabral et al., 2023; Thapa et al., 2023). Targeting the prevention of intraocular lens-associated infectious endophthalmitis, McCoy and co-workers developed hydrogels by combining anionic polymers of 2-(hydroxyethyl) methacrylate with the cationic tetrakis (4-N-methylpyridyl) porphyrin. The performed studies revealed high antimicrobial activity under intense light conditions due to 1O2 generation (Parsons et al., 2009). The same year, McCarron and co-workers evaluated poly (vinyl alcohol)-borate complexes as drug delivery platforms in photodynamic antimicrobial chemotherapy of wound infections. A similar cationic porphyrin was utilized in this study as well, namely, the tetrakis (4-N-methylpyridyl) porphyrin tetra tosylate (Donnelly et al., 2009). The porphyrin-based hydrogels adopted the shape of the wound, however by preserving at the same time their structural integrity. Overall, the porphyrin-hydrogels were phototoxic toward methicillin-resistant staphylococcus aureus. In another report, Lovell et al. developed optically active polyamide hydrogels using tetrakis (4-carboxyphenyl) porphyrin (TCP) (Lovell et al., 2011). The hydrogels were stable, without any observable degradation up to 2 months and also allowed a surgical resection in vivo using a fluorescence camera. The same TCP derivative, was also employed as a fluorescent tag in a biodegradable thermosensitive hydrogel, which demonstrated stable fluorescence for real time imaging (Lv et al., 2014).
In our recent report (Figure 2), we explored the treatment and healing of skin defects in rats using porphyrin-peptide hydrogels (Dontas et al., 2023). The developed hydrogels were transparent, in order to enable: i) the covering of the underlying tissue without adhesion, ii) the hydration during wound healing, and iii) the absorption of wound exudate. Two different families of rats were studied, namely, young and mature groups; interestingly, in both cases the application of the porphyrin-peptide hydrogel assisted in the healing process. Explicitly, concerning the mature animal group, the healing process was more pronounced due to the reduced healing rate of rats of advanced age. The porphyrinoid used in this work was a water-soluble 5,10,15,20-tetra-N-methyl-4-pyridyl) porphyrin tetra iodide ([TMPyP4+]I4−) which was synthesized according to our earlier work (Figure 2A) (Lazarides et al., 2014). For the successful development of the porphyrin-peptide hydrogel, a solvent-switch method was followed. In detail, ethanol was used as “good solvent” and water as the “bad solvent”. The Fmoc-FF peptide (Figure 2B) was dissolved in ethanol and the porphyrin in water, resulting in a final porphyrin/peptide ratio of 1:30. As illustrated in Figures 2C, D, the type of the vessel (either a glass vial or a plastic syringe), resulted in two different morphologies. It is worth noting that the hydrogel formed in a plastic syringe (Figure 2D), was the most suitable because it permitted its direct application onto the defect of the rat.
FIGURE 2. The molecular structures of: (A) (TMPyP4+)I4−, (B) Fmoc-FF, (C) hydrogel in a glass vial, (D) hydrogel upon syringe extrusion. Figure reproduced with permission from (Dontas et al., 2023). Copyright 2023, Springer Nature.
Three different parameters were evaluated in the case of young rats: i) the diameter, ii) the perimeter, and iii) the total area. Overall, the porphyrin hydrogel outperformed both the control as well as the hydrogel experiment (formed by Fmoc-FF in ethanol/water) concerning the healing process of all defect parameters. Noteworthy, the hydrogel was also beneficial compared to the control experiment most likely due to the hydration of the skin defect; however, not at the extend of the porphyrin hydrogel. Similar to the experiment of the young rats, in the respective measurements using the mature rats, the porphyrin hydrogel outperformed both the hydrogel and the control experiment in terms of healing effect (for all three different defect parameters). More importantly, beneficial effect of the porphyrin hydrogel utilization was more visible concerning the statistical significance and the time of wound healing. It was evident, that all the different parameters illustrated a similar healing pattern in both young and mature rats. We have summarized the most important results in the following Table 1. We list herein, the comparison of porphyrin hydrogel, hydrogel and control experiment concerning the 3rd day of our measurements regarding the three skin defect parameters (perimeter, diameter and area) for both the mature and young rats.
TABLE 1. Comparison of young and mature animals concerning the defect parameters (perimeter, diameter and area) for each experimental group (porphyrin-hydrogel, hydrogel, control).
Photodynamic therapy (PDT) is a widely used oncological intervention which has approved by various countries, bringing hope in patients diagnosed with cancer each year (Siegel et al., 2022). Compared to conventional methods, such as surgery, chemotherapy and radiation treatment, PDT presents several advantages. In specific, PDT is non-invasive, nonradiative and with spatial accuracy of the operation, leading to minimal side effects, cumulative radiation dose and recurrence (Lucky et al., 2015). PDT is a type of phototherapy that combines light irradiation, photosensitizers, and oxygen to selectively destroy cancer cells (Brown et al., 2004; Castano et al., 2006; Li et al., 2019). The photosensitizer (PS) is excited with light in order to convert molecular oxygen into cytotoxic ROS, such as 1O2 (Warszyńska et al., 2023). This procedure causes oxidative stress in cancer cells, which leads to cell death. PDT offers targeted treatment due to the specific activation of the PS by light, sparing normal tissues from unnecessary harm. Additionally, the targeted nature of PDT reduces side effects, establishing it as a more favourable choice compared to traditional cancer treatments. To that end, plenty research groups are exploring the utilization of PDT as a viable potential treatment for a variety of cancer types.
Even though PDT has been around for more than a century (Spikes, 1977; Szeimies et al., 2001), the vast interest in this kind of oncotherapy has grown as a result of recent progress in the fields of medicinal chemistry, cancer immunology, photochemistry, biochemistry, etc (Brown et al., 2004). Furthermore, PDT is employed in ophthalmology (age-related macular degeneration, AMD and treatment of localized infections) (Fine, 1999; Sułek et al., 2019), and dermatology (non-melanoma skin malignancies). The rising incidence of bacterial and fungal illnesses brought on by multidrug-resistant pathogens makes the latter use more crucial (Aroso et al., 2019). Many photosensitizers have been evaluated in PDT studies, both in vitro and in vivo; nonetheless, only a few of them demonstrated perfect abilities. As a result, contemporary research has been focussed on developing novel photosensitizers (PSs) (Dąbrowski et al., 2016; Kwiatkowski et al., 2018). Chemical purity, tumor cell selectivity, physico-chemical stability, brief period of time between dose and maximum accumulation in tumor tissues, activation at longer wavelengths for deeper penetration in the biological tissue, and quick bodily clearance are all necessary features that an ideal PS should possess (Castano et al., 2005). Porphyrinoids such as porphycenes, porphyrins, phthalocyanines and chlorins are the four primary families of PSs. Interestingly, each class possess unique photochemical and photophysical characteristics which lead to wide-range methods of action and light activation (De Rosa and Bentley, 2000).
The mechanisms of light absorption and energy transfer are the two key components of PDT (Figure 3). The singlet ground state of PS is a low energy molecular orbital containing two electrons with opposing spins. One of these electrons is promoted to a higher energy orbital after absorption of a photon retaining its spin state. The additional energy of the PS in this transient state can be dissipated either by internal conversion as heat or emission of a photon, as fluorescence. The PS in the singlet excited state may also go through a process called intersystem crossing, in which the spin of the excited electron is inverted, converting the molecule to an excited triplet state with two electrons with parallel spin (Ochsner, 1996; Juzeniene and Moan, 2007). Then the PS from the triplet excited state returns to the singlet ground state by emission of light (phosphorescence) or undergoes two kinds of reactions with surrounding molecules.
FIGURE 3. Illustration of mechanism of photodynamic therapy reaction. Figure reproduced with permission from (Kwiatkowski et al., 2018). Copyright 2018, Elsevier.
First, it can react with a biological substrate, for instance a cell membrane or a molecule, by transferring an electron or a proton to generate a radical cation or anion, respectively. ROS might be created by these radicals after their reaction with molecular oxygen. As an alternative, the triplet PS can transfer its energy directly to molecular oxygen, resulting in the formation of excited singlet oxygen (Figure 3). The ratio between both routes, which can happen concurrently, is determined by the nature of the PS, as well as the substrate and oxygen concentrations (Castano et al., 2004). The major advantage of PDT lies to the fact that due to the high reactivity and brief half-life of singlet oxygen and hydroxyl radicals, only molecules that are close to the PS (i.e., regions of PS localization) are immediately impacted and eventually destroyed.
To efficiently produce ROS in PDT, a well-chosen PS is necessary. The ideal combination of optical and photophysical properties should be present, including long triplet state lifetimes, high triplet quantum yield, and efficient absorption (Dąbrowski et al., 2016). To guarantee proper photon penetration deep into tissues and avoid endogenous chromophores, it is preferred to utilize molecules that absorb in the red or even near-infrared range (λ ∼ 630–850 nm). To minimize the damage of healthy tissues, the PS should be biocompatible, non-toxic, and quickly removed from the body after photodynamic therapy avoiding long-term accumulation. This explains why the bulk of PSs used today are naturally occurring porphyrinoids, which may be found in a variety of animal and plant species. Because of their availability, low toxicity, and beneficial optical properties, porphyrinoids are frequently used in PDT. In addition, they can conveniently bind a wide range of metal ions. The first medication used in PDT was created by extracting hematoporphyrin (HP) from dried blood by eliminating iron, and was given the brand name Photofrin®. Albeit the first results indicated promising cancer treatment results, especially regarding esophageal and lung cancer, the medical world did not widely acknowledge it as a significant breakthrough. Other porphyrin-based PSs that were examined by the Food and Drug Administration (FDA) and applied in clinical trials are Visudyne and Foscan. PDT alone is typically insufficient to elicit a suitable immune response because cancer cells may produce additional promoting chemicals or immunosuppressive cytokines via non-immunogenic mechanisms. Thus, it is considered crucial to start with the most comprehensive approach when designing PDT PSs with the desired qualities and to appropriately combine various therapeutic approaches later on. One way to improve PDT effectiveness is the utilization of nanotechnology. Therefore, it is possible to engineer nanoparticles to deliver PSs to cancer cells improving selectivity and minimizing side effects. Additional development could be achieved, by enhancing photosensitizers’ bioavailability and retention at the tumor site. Thus, many researchers designed novel nanomaterials with controllable structures via self-assembly approach (Charalambidis et al., 2011; Charalambidis et al., 2016; Karikis et al., 2016; Karikis et al., 2018; Li et al., 2018; Nikoloudakis et al., 2018; Xing et al., 2019).
Porphyrin chromophores present excellent biosafety and their absorption features are compatible with the biological window (650–900 nm), enabling deep tissue penetration. However, their low solubility in aqueous media significantly lowers singlet oxygen generation and limits their medical application. Therefore, various approaches were employed to improve the solubility of the porphyrin-based PSs in water, such as, encapsulation of PS in appropriate carriers (Yang et al., 2019) and conjugation of PS with hydrophilic moieties (Feng et al., 2016). In another approach, van Hest’s group prepared a peptide-porphyrin conjugate by coupling a pH-responsive dipeptide tryptophan-glycine (WG) to a hydrophobic porphyrin (P) core via amidation (Sun et al., 2020). This peptide-porphyrin hybrid (PWG) self-assembles into spherical nanoparticles under physiological conditions. However, when the nanoparticles were exposed to the acidic tumor microenvironment they transformed into nanofibers. The fibrillar nanostructures presented improved singlet oxygen generation ability and enabled high accumulation and long-term retention at tumor sites. In other report, Liang and co-workers prepared a porphyrin-oligopeptide conjugate (Ac-DEVDD-TPP) which demonstrated excellent solubility in water (Liu et al., 2023). Interestingly, after being taken up by oral cancer cells, Ac-DEVDD-TPP derivative was converted to D-TPP, which self-assembles into nanofibers around a mitochondrion. In vivo experiments demonstrated that upon laser irradiation, the porphyrin nanofibers induced efficient cell apoptosis and pyroptosis.
Another significant drawback of porphyrin-based PSs is their strong inclination to aggregate, which leads to the electronic excited state’s quenching and, therefore, reduces the quantum yield of singlet oxygen (Escudero et al., 2006; Helmich et al., 2010). Many approaches have been described to move past this difficulty, such as the use of metal-organic frameworks (MOFs) (Lu et al., 2014; Li et al., 2017), adding special groups onto porphyrin-based PSs (Haynes et al., 2017), and anchoring porphyrins onto nanoparticle drug carriers (Yu et al., 2016; Huang et al., 2017). To a certain extent, all of the aforementioned tactics may reduce aggregation-induced quenching of the PS and enhance singlet oxygen quantum yield. Nevertheless, hydrophobic porphyrinic PSs could be encapsulated into amphiphilic copolymers, and subsequently released properly. Zhang and co-workers reported an approach based on the preparation of novel porphyrin-polymeric nanoparticles (Jin et al., 2018). In this report, pollyhedral oligomeric silsesquioxane (POSS) and cage-shaped Si8O12-based NPs (Cordes et al., 2010), were used as polymers with increased mechanical strength and thermal stability. Therefore, a porphyrin and POSS copolymer were synthesized from 4-vinylbenzyl-terminated tetraphenylporphyrin and maleimide isobutyl polyhedral oligomeric silsesquioxane, via alternating reversible addition-fragmentation chain transfer polymerization. It was found that the aggregation induced quenching of the material and the PDT efficacy of this new porphyrin/POSS co-polymer was increased.
Peptides are easily conjugated with other functional groups, including PSs, in many examples. The peptide-PS conjugates that are produced have the ability to operate like PS in addition to possess self-assembling capabilities. Consequently, phototherapy and catalysis can employ nanomaterials created by conjugate self-assembly (Li et al., 2015; Zhang et al., 2015; Carrión et al., 2017; Han et al., 2017). Additionally, DNA self-assembly relies on Watson-Crick base pairing, a specific interaction between base pairs. On the contrary, in the respective peptide self-assembly noncovalent interactions govern the process (Nikolova et al., 2011; Wu et al., 2017). A unique approach to create hybrid materials is to combine DNA nanoparticles with flexible and complicated peptide-based nanomaterials. The self-assembling properties of both peptides and DNA are combined in peptide nucleic acids (PNAs), synthetic structures having a peptide backbone and DNA bases (Berger et al., 2015). Because PNAs are very resistant to being broken down by nucleases or proteases, they are perfect building blocks for use in biomedical applications. The Watson-Crick base pairs, stacking interactions, and optical characteristics of PNAs were confirmed by Gazit and co-workers a few years ago (Berger et al., 2015). We recently developed two PNA-photosensitizer conjugates (PNA-BDP and PNA-TPP), in order to examine their catalytic potential and assembly behavior (Nikoloudakis et al., 2019). The assemblies that are produced have distinct architectures, suitable dimensions, and distinctive light-absorbing properties. In this work, Coutsolelos and co-workers explored their potency in antitumor phototherapy (Figure 4A) (Chang et al., 2020). The synthetic protocol for the preparation of both PNA-hybrids is illustrated in Figure 4B. The hybrid PNA-TPP was produced in high yield via an amide coupling between the TPP-NH2 and the Fmoc-PNA-G-(Boc)-OH. The coupling reagents for this reaction were dicyclohexylcarbodiimide (DCC) and N-hydroxybenzotriazole (HOBt), resulting in the final product (PNA-TPP) upon stirring at low temperature (8°C) for 48 h (yield: 58%). A similar process was used to prepare PNA-BDP; however, in this case room temperature was needed for the successful amide coupling reaction.
FIGURE 4. (A) Supramolecular nanoparticles constructed by self-assembly of PNA-photosensitizer conjugates for photodynamic therapy, (B) Synthetic procedure of PNA-TPP and PNA-BDP. Figure reproduced with permission from (Chang et al., 2020). Copyright 2020, ACS publications.
Both PNA-BDP and PNA-TPP nanomaterials were prepared via self-assembly in aqueous solution through π-π stacking and hydrophobic interactions, forming materials with uniform spherical morphology as demonstrated in SEM images (Figure 5). The diameter of the spheres was measured 102 ± 34 nm via dynamic light scattering (DLS). UV-Vis absorption measurements of the nanomaterials showed broadening of the peaks compared to their molecular counterparts. It is worth noting that in the case of PNA-TPP, a red-shift was observed, which is indicative for formation of J-aggregates. Similarly, quenching of their fluorescence spectra revealed the self-aggregation of the composites. Moreover, since stability of nanodrugs is quite important DLS measurements resulted that the average size of the particles was stable in various concentrations. Additionally, in cell culture media, namely, phosphate buffered saline (PBS) the size of the nanoparticles was not significantly altered. Upon light irradiation, the absorption band of PNA-TPP was slightly decreased, while the band of PNA-BDP was significantly decreased. The ability of both materials to produce singlet oxygen was perceived with the use of a trapping reagent, 9,10-anthracenediyl-bis(methylene) dimalonic acid. In vitro experiments PNA-BDP and PNA-TPP nanoparticles were incubated with MCF-7 and 3T3 cells and in both cases the cells could uptake the nanocomposites efficiently. Then, PDT evaluation was performed by scanning at 488 nm excitation (4 mW). Both nanoparticles showed efficient cytotoxicity of cells in a certain selected area, while in the dark no cytotoxicity was observed (Figure 5).
FIGURE 5. (A) Images from CLSM (right) and bright-field (left) demonstrating the PNA-BDP NPs’ selective PDT action on MCF-7 cells. Dead cells are shown by the red signal from PI. (B) Bright-field (left) and CLSM (right) pictures demonstrating the PNA-TPP NPs’ selective PDT action on MCF-7 cells; scale bars indicate 50 μm. The MCF-7 cells that were incubated with PNA-BDP NPs (C) and PNA-TPP NPs (D), treated with or without light irradiation, were tested for viability. Scale bars indicate 50 μm. Standard deviation (n = 5) is shown by error bars. Figure reproduced with permission from (Chang et al., 2020). Copyright 2020, ACS publications.
To date many porphyrin-based PDT studies have been reported, where porphyrins are in conjunction with a variety of materials, including amphiphiles, polymers, supramolecular polymers, and 3D nanoparticles. Porphyrin-based materials can lead to an increased PDT efficiency and a high loading concentration. However, in order to fully utilize these types of materials, a number of difficulties must be resolved in the near future. First, in order to reach deeper tissue, porphyrin-based materials’ emission and absorption must be shifted to longer wavelengths. Second, despite the variety of reported organic, inorganic, or hybrid porphyrin-based materials, it would be ideal to develop alternative therapies to rationally arrange the various functions against the particular tumor environment and get around PDT’s inherent limitations. Thirdly, up to now, the majority of published research papers on porphyrin-based materials have mostly concentrated on fundamental and basic scientific study. Therefore, porphyrin-based materials activated by NIR light that have superior biocompatibility and biodegradability, high photocytoxicity, outstanding distribution efficiency throughout the body, and efficient tumor targeting with minimum side effects are required for translational and clinical PDT practice. Consequently, further research and development are required to assure the effective use of these materials in clinical settings.
As discussed in the previous paragraph, the key component in PDT, i.e., the PS, should possess the following features: i) ability to produce 1O2 efficiently which is the cytotoxic agent responsible for the photokilling of tumor cells, ii) high absorbance in the long visible wavelengths (700–800 nm), iii) no toxicity in absence of light, iv) amphiphilic chemical structure as well as v) stability and solubility in injectable solvents (Ethirajan et al., 2011). Despite their great potential, typical porphyrins are insoluble in aqueous media; hence, demand a suitable molecular carrier which renders them water-soluble and assists their optimum delivery without changing their photosensitizing activity (Dąbrowski et al., 2016). Cyclodextrins (CDs) are promising candidates for this role since they include guest molecules in their hollow structures producing inclusion complexes. In addition, cyclodextrins can host porphyrin molecules and improve their solubility in water, physicochemical stability and bioavailability as well as controlling their release (Tian et al., 2020). Several reports have been published dealing with porphyrin PSs combined with cyclodextrins towards PDT activity (Liu et al., 2016).
In a recent report, Ikeda et al. (2017) prepared water-soluble complexes of cyclodextrin enclosing porphyrin derivatives with different substituents in the meso-positions (Figure 6A) and investigated their activity towards PDT (Ikeda et al., 2017). In particular, aniline- and phenol-substituted porphyrins demonstrated high photodynamic activity which was even greater than photofrin; i.e., a common drug in clinical used as photosensitizer. Covalent attachment of cyclodextrin with porphyrin is another approach towards cancer therapy. The porphyrin-cyclodextrin conjugates (Figure 6B) prepared by Král and co-workers presented combined PDT activity with chemotherapy (Králová et al., 2010). The versatile supramolecular system combined efficient enclosing of the drug in the cavity of the cyclodextrin moiety as well as the photosensitizing properties of the porphyrin moiety while the entire complex was accumulating in the cancer tissue. The combination of PDT and chemotherapy was explored also in a more recent work, where Zhao and co-workers synthesized the porphyrin-cyclodextrin PEG-Por-CD nanocarriers presented in Figure 6C (Lim et al., 2019). These conjugates self-assembled with oxaliplatin via host-guest interactions and demonstrated enhanced cytotoxicity and apoptosis compared to the corresponding monotherapy. Besides covalent and Van-der Waals interactions, cyclodextrins can be combined with porphyrins via electrostatic interactions towards 1O2 generation (Zagami et al., 2023).
FIGURE 6. (A) Structures of the water-soluble complexes of TMe-β-CyD cyclodextrin enclosing meso-substituted porphyrin derivatives. Figure reproduced with permission from (Ikeda et al., 2017). Copyright 2017, ACS publications; (B) schematic representation of the porphyrin-cyclodextrin supramolecular carrier-drug complex. Figure reproduced with permission from (Králová et al., 2010). Copyright 2010, ACS publications; (C) chemical structures of PEG-Por-CD carrier and oxliPt(IV)- ada drug. Figure reproduced with permission from (Lim et al., 2019). Copyright 2019, ACS publications.
In 2022, our research group contributed in this field through the preparation of porphyrin-cyclodextrin complexes towards PDT. In detail, we prepared and fully characterized unsymmetrical, mildly amphiphilic porphyrin derivatives, in addition to their complexes with pMβCD in water, and investigated their intracellular delivery, phototoxicity and dark toxicity against MCF-7 breast cancer cells (Panagiotakis et al., 2022). Scheme 1 illustrates the synthetic procedures for the preparation of the porphyrin derivatives bearing different peripheral substituents and a carboxylic side-chain of different length. In detail TPPOCOOHC2, TPPOCOOHC5, TPPOCOOHC11 and the meta-trihydroxy derivative mTHPPOCOOHC5, were synthesized in high yields starting from the corresponding ester followed by basic hydrolysis. The ester precursor porphyrins were prepared via nucleophilic substitution reactions between TPPOH and the appropriate ω-bromoalkyl ester (Brulé et al., 2004) (Scheme 1A). The meta-substituted ester precursor was synthesized from the suitable ester-protected aldehydes and pyrrole (Scheme 1B) using trifluoroacetic acid (TFA) according to Lindsey’s method (Lindsey et al., 1987). The symmetrical compounds TPP and mTHPP were also synthesized by the Adler and Longo method for comparison purposes (Adler et al., 1964). Analysis of the 1D and 2D NMR spectra proved that the stoichiometry of the complexes of pMβCD with the unsymmetrical amphiphilic porphyrins is 2:1, while there are variations in the complexation modes and in the number of supramolecular isomers.
SCHEME 1. Synthesis of carboxy-substituted porphyrins for the preparation of porphyrin-cyclodextrin complexes: (A) I) n = 1, n = 4: K2CO3, KI, DMF, 80°C, 2 h; n = 10: K2CO3, KI, DMF, r.t., 18 h, II) 1. KOH (aq), THF, MeOH, r.t., 24 h, 2. HCl 1 M, pH 4-5; (B) I) 1.TFA, r.t., 17 h, DCM, 2. DDQ, r.t., 2 h, DCM, 3. TEA, r.t., 15 min, DCM, II) 1. KOH (aq), THF, MeOH, r.t., 24 h, 2. HCl 1 M, pH 4-5. Figure reproduced with permission from (Panagiotakis et al., 2022). Copyright 2022, Elsevier.
Photogeneration of singlet oxygen was monitored using the water-soluble 1O2 sensor 9,10-anthracenediylbis(methylene)dimalonic acid (ABDA) and clearly verified that the rates of 1O2 production were larger in case of the porphyrin-CD complexes than for the free porphyrins, especially for TPPOCOOHC5, while no difference was detected for mTHPPOCOOHC5. After establishing the excellent stability of the pMβCD/porphyrin complexes under prolonged light irradiation in the presence of cell culture proteins for 24 h, the authors studied their cellular uptake by MCF-7 breast cancer cells using confocal microscopy. It was proven that pMβCD promotes enhanced cell uptake as well as endoplasmic reticulum (ER) distribution and high phototoxicity, while toxicity without light remains low. In Figure 7, the photodynamic activity against MCF-7 cells of the pMβCD/porphyrin complexes compared to the parent porphyrins is illustrated. The most effective monocarboxyporphyrins may be suggested as parts of more advanced photo and chemotherapy platforms, and their function can be facilitated by pMβCD.
FIGURE 7. Viability of MCF-7 cancer cells after 24 h of incubation with pMβCD/porphyrin complexes (2 μM) compared to the parent porphyrins (2 μM). All compounds were irradiated at 6.28 J/cm2. The MTT assays were performed at 24 h post-irradiation. Figure reproduced with permission from (Panagiotakis et al., 2022). Copyright 2022, Elsevier.
In addition, our research group recently developed combinational PDT and chemotherapy systems based on cyclodextrins and porphyrins (Panagiotakis et al., 2023). The aim was to develop photoactive nanoparticle targeting cancer cell lysosomes for drug delivery and light-controlled release. In detail they covalently connected the best performing porphyrins of their previous work with mono-amino-substituted cyclodextrin and obtained new amphiphilic porphyrin-βCD conjugates. The successful synthesis of the porphyrin-βCD conjugates (Figure 8) was achieved via amide linkage between the mono(6-amino-6-deoxy)-βCD (Petter et al., 1990) and TPPOCOOHCx (Scheme 1) utilizing 4-(4,6-dimethoxy-1,3,5-triazin-2-yl)-4-methylmorpholinium chloride (DMTMM) (Kunishima et al., 1999) coupling reagent. Noteworthy the employment of common coupling reagents and conditions such as DCC and HOBt, or hexafluorophosphate azabenzotriazolyl-tetramethyluronium (HATU) or thionyl chloride (SOCl2) lead only to the recovery of the starting materials. Absorption and emission spectroscopy studies revealed that the length of the aliphatic chain linkers between the porphyrin and βCD moieties porphyrin-βCD molecules does not alter the photophysical properties both in DMF and in aqueous media. The conjugates self-assembled into nanoparticles of approximately ∼60 nm as demonstrated by scanning and transmission electron microscopy as well as DLS experiments. Importantly, the self-assembled nanoparticles (NPs) were photoactive and selectively targeted the lysosomes of MCF-7 cells.
FIGURE 8. Structures of porphyrin-βCD conjugates. Figure reproduced with permission from (Panagiotakis et al., 2023). Copyright 2023, Elsevier.
After establishing the above desired properties, we proceeded with the inclusion of anticancer drugs in the NPs. Two different drugs were investigated, the citrate salt of Tamoxifen (TamCit) and the N-adamantyl derivative of Gemcitabine (GEMADA). PBS solutions of the pre-assembled NPs were incubated with TamCit, GEM and a model guest 1-adamantylcarboxylic acid (ADACOOH) and according to DLS experiments the diameter of the nanoparticles was significantly increased. Confocal laser scanning microscopy was utilized once again for live imaging of human breast adenocarcinoma MCF-7 cells incubated with the conjugate NPs. Either empty or with the drug, the NPs present no toxicity in the dark for 48 h; thus, light irradiation induces the release of the drugs. After irradiation at 640 nm with a LED array lamp, the cells incubated with drug-loaded NPs displayed a major decrease of viability (>85%) for 48 h. The results showed strong synergy between the photodynamic and chemotherapeutic effects and therefore, photochemical internalization (PCI) is suggested to be the drug release mechanism. The above results are illustrated in Figure 9 which describes the viability of MCF-7 cells after 24 h of incubation with the NPs, with or without the investigated anticancer drugs. The authors suggest that the prepared porphyrin-βCD NPs, should be considered, along with other nano-assemblies (Xue et al., 2019), as efficient vehicles for the photo delivery of small drugs.
FIGURE 9. Toxicity against MCF-7 cells after 24 h of incubation with porphyrin-βCD NPs (10 μM) with or without anticancer drugs (7.5 μM), without (white and light grey bars) or with irradiation at 4.18 J/cm2, λ = 640 nm (dark grey and black bars). The MTT assays were performed at 24 h (dark grey bars) or 48 h (black bars) post-irradiation: (A) Control experiments with cells alone (control), TamCit or GEMADA; (B) TPPOCNHbmC2; (C) TPPOCNHbmC5; (D) TPPOCNHbmC11, alone and loaded with the drugs Figure reproduced with permission from (Panagiotakis et al., 2023). Copyright 2023, Elsevier.
Visualizing the trafficking and localization of proteins in living systems is the key to understand protein functions in their natural environment. Thus, labelling and tracking of biomolecules (peptides, antibodies, and proteins) inside alive cells provides a pathway for the determination of their interactions, mobility, dynamics, and functions (Schneider and Hackenberger, 2017). Several labelling methods such as radioactive tracers, photochromic compounds, electrochemical sensors, colorimetric biosensors, isotope markers, photo switchable biomaterials and fluorescent labels are available for this purpose (Ong and Mann, 2006; Chudakov et al., 2010; Rocha-Santos, 2014; Velikyan, 2014; Abdollahi et al., 2019; Rai and Ferreira, 2021). Among them, optical imaging by fluorescence microscopy is the most widely used and versatile visualization strategy in research and clinical practice mainly due to its non-destructive features and high sensitivity. Additionally, it fulfils the requirements of low concentration of fluorescent material and small measurement volume (Dean and Palmer, 2014). Recently, fluorescence microscopy and imaging have gained significant attention, owing to the growing availability of dyes, fluorescent proteins and probes alongside with an increasing number of fluorescent imaging methods.
Optical visualization by fluorescence microscopy requires the site-specific labelling of a protein of interest (POI) with a fluorescent derivative. The connection of the fluorophore with the desired biomolecule can be achieved biologically or chemically (Lotze et al., 2016). Peptide-based recognition tags provide an attractive strategy for protein labelling. In the tag-based method, the POI is genetically fused with an oligo-peptide that binds site-specifically to a desired synthetic fluorophore (Lai et al., 2015). The attachment of the fluorophore with the tag through metallochelate coupling is one of the most widely used strategies and has been employed in biotechnology and molecular biology by several research groups (Connell and Donnelly, 2018). It is based on the capability of nitrilotriacetic (NTA) complexes with transition metals (Zn2+, Ni2+, Co2+, or Cu2+) to coordinate specific amino acid residues, mostly oligo-histidine sequences, such as the hexa-histidine tag (His6-tag) (Leonhardt et al., 2023). The His6-tag is an oligo-histidine sequence in which six histidine residues have the ability to interact noncovalently and reversibly with a metallic complex of NTA. The main advantage of this approach is the high specificity, and additionally the tag can be expressed either at the N-termini or C-termini during the expression of the protein (You and Piehler, 2014).
Porphyrins and metallo-porphyrins are considered as promising labelling tools (Ethirajan et al., 2011; Rabiee et al., 2020; Sandland et al., 2021; Jin et al., 2023). Additionally, porphyrin derivatives were employed in PDT of tumours and as labels for the detection of cancer cells (Tian et al., 2020; Chen et al., 2021; Park et al., 2021; Shi et al., 2021). Dmitriev et al. (2016) reported the first example where porphyrin complexes bearing NTA moieties were applied in the labelling of various biomolecules that possess the His6-tag. More specifically, they succeeded to label through metallochelate coupling several peptides and proteins with phosphorescent Pt-porphyrin derivatives bearing NTA group. Their studies revealed that all synthesized metallochelate complexes display phosphorescence and sensitivity to O2, which is comparable with other (poly)peptide-based probes.
Coutsolelos and co-workers reported the synthesis and characterization of three porphyrin-based fluorescent probes (Figure 10), bearing modified lysine-NTA groups and could be applied in protein labelling through metallochelate coupling chemistry (Glymenaki et al., 2022). All hybrids were based on a free base porphyrin macrocycle which was covalently connected with an appropriately NTA-substituted ligand. In the first two probes (TPP-Lys-NTA and TPP-CC-Lys-NTA) the same phenyl-substituted porphyrin ring was employed, with the only difference being the group linking the two moieties. In TPP-Lys-NTA, the linkage was performed through an amide bond, while in TPP-CC-Lys-NTA, a triazine ring was employed as linker in order to increase the distance between the porphyrin and the NTA ligand. In the third dyad (Py3P-Lys-NTA) three pyridyl groups were introduced to the porphyrin periphery, targeting to increase the hydrophilicity of the probe; a desirable feature for labelling water-soluble peptides. The connection of the porphyrin with the NTA ligand was achieved through an amide bond using standard coupling reagents.
FIGURE 10. Molecular structures of the porphyrin-based probes described in this study Figure reproduced with permission from (Glymenaki et al., 2022). Copyright 2022, ACS publications.
Initially, absorption and emission spectroscopy studies were performed to explore if the three probes were able to coordinate with Ni2+ ions. These experiments demonstrated that the NTA ligand can bind Ni2+ and the characteristic absorption features of the dyes are preserved after the coordination. Noteworthy, emission studies revealed that the emission of these probes is not quenched after the coordination with Ni2+ ions. This observed feature is stimulating, since the majority of the reported fluorophores a significant emission quenching is observed upon the connection with the transition metal. This is the most common drawback of many fluorescent probes, since quenching of the emission decreases the detection limit of the probe after its connection with the desired POI (Dmitriev et al., 2016). Additionally, as a proof of concept, we studied two oligo-peptides (Fmoc-FH and RGDSGAITIGH, Figure 11) to examine whether the porphyrin-NTA dyads could be used as fluorescent probes and coordinate with them through the NTA ligand. Interestingly, these two peptides were able to self-assemble and form various nanostructures. Moreover, one histidine residue is present in their backbone; thus, they can be regarded as His6-tag models. Self-assembly based on oligo-peptides provides a novel approach for tumour nanotheranostics that possess functionality, biosafety, controllability and programmability, enabling the transition of supramolecular nanotheranostics from lab to bedside (Li et al., 2019).
FIGURE 11. Molecular structures of the oligopeptides in this work. Figure reproduced with permission from (Glymenaki et al., 2022). Copyright 2022, ACS publications.
Field-emission scanning electron microscopy (FESEM) experiments were performed to study the capability of porphyrin-NTA dyads to coordinate with histidine bearing peptides in the presence of Ni2+ ions. TPP-Lys-NTA hybrid self-assembles and forms flake-shaped nanostructures (Figure 12A). However, in the presence of Ni2+ ions the morphology of the porphyrin self-assembling nanostructures was altered, resulting in spherical architectures (Figure 12B). On the other hand, the Fmoc-FH peptide self-assembles forming a network of fibrils (Figure 12D). Noteworthy, in the case where both Fmoc-FH and TPP-Lys-NTA were present the two derivatives self-assembled independently and formed a fibrillar network covered with spherical nanostructures (Figure 12E). Finally, the sample which contained Fmoc-FH with TPP-Lys-NTA-Ni2+ gave rise to the formation of more well-defined nanostructures with spherical shape and increased diameter (Figure 12C). Similar results were observed for the other two probes as well. The differences that were observed before and after the addition of the oligo-peptides provide a strong indication for the successful metallochelate coupling. Solid state absorption and emission experiments also supported the connection of the probes with the oligopeptides through metallochelate coupling.
FIGURE 12. FESEM picture of: (A) TPP-Lys-NTA, (B) TPP-Lys-NTA and Ni2+, (C) Fmoc-FH and TPP-Lys-NTA with Ni2+, (D) Fmoc-FH, and (E) Fmoc-FH and TPP-Lys-NTA. All samples were prepared in HFIP:HEPES 50 mM pH 7.4 (3:7, v/v) solvent mixture. Figure reproduced with permission from (Glymenaki et al., 2022). Copyright 2022, ACS publications.
Moving one step forward, the authors examined the capability of the porphyrin-peptide hybrids to penetrate and accumulate in mammalian HeLa cancer cells, studying their potential application as anticancer agents. Confocal microscopy studies revealed that the porphyrin-NTA hybrids alone and their corresponding complexes with the oligopeptides can enter the HeLa cancer cells and accumulate into the cytoplasm. Thus, peptides with histidine residues in their backbone are especially suitable for intracellular deliver of labelling fluorophores or different type of molecules. The pKa of histidine’s imidazole group is around 6 and in can be protonated in the intracellular environment, especially in the acidic conditions within endosomes. This can result in endosomal lysis and release of the fluorophore or another type of molecule inside the cytoplasm.
Overall, in this perspective article we illustrated the development of properly functionalized porphyrinoids as agents in several biomedical applications, namely, wound healing, PDT, photokilling cancer cells, drug release, and peptide labelling. The wound healing results using porphyrin-hydrogels in young and mature rats based on our work, illustrated that further studies would be of great interest. More precisely, as future directions we suggest the utilization of metallated porphyrinoids with higher 1O2 production rates, as well as diverse peptides which can still form transparent hydrogels though. These two approaches could potentially improve the stability and the efficiency of hydrogels targeting defect parameters similar to our study. We have also introduced a method for producing PDT nanodrugs by mixing PNAs with suitably functionalized photosensitizers. We were able to develop nanoparticles with distinct structures through the self-assembly of PNA-BDP and PNA-TPP hybrids, which were effectively internalized by cells and exhibited stability in biological fluids. In the absence of irradiation negligible cytotoxicity was observed; however, by utilizing laser irradiation selective cytotoxicity was detected rendering these self-assembled NPs as effective nanoagents. Moving one step forward, additional PNA derivatives connected with appropriate decorated porphyrin-based PSs should be developed, targeting to further improve both the identification of tumour cells as well as their treatment. Overall, this approach could advance the preclinical assessment and the clinical use of bioinspired biomaterials via the incorporation of such novel supramolecular therapeutic nanoagents. In addition, we have utilized cyclodextrin moieties in an effort to develop water-soluble porphyrin complexes with PDT properties. This strategy was proved very efficient; thus, other chromophores with alike photophysical features such as corroles, phthalocyanines and BODIPY dyes could also be used as photosensitizers in PDT studies. The porphyrin-cyclodextrin conjugates that we developed, targeting cancer cells for light-controlled drug release, demonstrated a facile way to develop photoactive nanoparticles; expanding the field of combinational PDT and chemotherapy into different small molecule chemotherapeutics. Regarding the protein labelling applications, we covalently connected appropriately modified NTA ligands with porphyrins in an effort to form fluorophores. One of the possible future direction in this field could be the preparation of similar hybrids by taking advantage of the metallochelate coupling chemistry, and eventually penetrate into cancer cells. Porphyrinoids have merited a distinct spot in biomedicine, owing to: i) their broad and intense absorption, ii) their facile structural modification, and iii) their ability to chelate various metals. As a consequence, porphyrin-based nanohybrids have attractive photoredox, photochemical, and photophysical properties and are considered as ideal theragnostic agents with vast potentials. However, in order to find a practical application, we should improve their stability against demetallation, biocompatibility, selectivity, off-target phototoxicity, 1O2 quantum yield, and absorption in the visible-NIR region. Undoubtedly, the successful preparation of porphyrin macrocycles with the above-mentioned characteristics will result in useful theragnostic agents in multiple disease areas.
The original contributions presented in the study are included in the article/Supplementary Material, further inquiries can be directed to the corresponding authors.
VN: Conceptualization, Writing–original draft, Writing–review and editing. EN: Writing–original draft, Writing–review and editing. KL: Investigation, Writing–original draft, Writing–review and editing. GC: Investigation, Writing–original draft, Writing–review and editing. AC: Investigation, Supervision, Writing–review and editing.
The author(s) declare financial support was received for the research, authorship, and/or publication of this article. This research was financed by the European Union and Greek national funds through the Operational Program Competitiveness, Entrepreneurship, and Innovation, under the call RESEARCH-CREATE-INNOVATE (project code: T1EDK-01504). In addition, this research has been co-financed by the European Union and Greek national funds through the Regional Operational Program ‘‘Crete 2014-2020,’’ project code OPS:5029187. Moreover, the European Commission’s Seventh Framework Program (FP7/2007-2013) under grant agreement no. 229927 (FP7- REGPOT-2008-1, Project BIO-SOLENUTI) and the Special Research Account of the University of Crete are gratefully acknowledged for the financial support of this research.
The authors declare that the research was conducted in the absence of any commercial or financial relationships that could be construed as a potential conflict of interest.
All claims expressed in this article are solely those of the authors and do not necessarily represent those of their affiliated organizations, or those of the publisher, the editors and the reviewers. Any product that may be evaluated in this article, or claim that may be made by its manufacturer, is not guaranteed or endorsed by the publisher.
Abdollahi, A., Roghani-Mamaqani, H., Razavi, B., and Salami-Kalajahi, M. (2019). The light-controlling of temperature-responsivity in stimuli-responsive polymers. Polym. Chem. 10, 5686–5720. doi:10.1039/c9py00890j
Adler, A. D., Longo, F. R., and Shergalis, W. (1964). Mechanistic investigations of porphyrin syntheses. I. Preliminary studies on ms-tetraphenylporphin. J. Am. Chem. Soc. 86, 3145–3149. doi:10.1021/ja01069a035
Amin, M. A., and Abdel-Raheem, I. T. (2014). Accelerated wound healing and anti-inflammatory effects of physically cross linked polyvinyl alcohol-chitosan hydrogel containing honey bee venom in diabetic rats. Archives Pharmacal Res. 37, 1016–1031. doi:10.1007/s12272-013-0308-y
Arakawa, H., Takeda, K., Higashi, S. L., Shibata, A., Kitamura, Y., and Ikeda, M. (2020). Self-assembly and hydrogel formation ability of Fmoc-dipeptides comprising α-methyl-L-phenylalanine. Polym. J. 52, 923–930. doi:10.1038/s41428-019-0301-5
Aroso, R. T., Calvete, M. J. F., Pucelik, B., Dubin, G., Arnaut, L. G., Pereira, M. M., et al. (2019). Photoinactivation of microorganisms with sub-micromolar concentrations of imidazolium metallophthalocyanine salts. Eur. J. Med. Chem. 184, 111740. doi:10.1016/j.ejmech.2019.111740
Battersby, A. R. (2000). Tetrapyrroles: the pigments of life. Nat. Product. Rep. 17, 507–526. doi:10.1039/b002635m
Battersby, A. R., Fookes, C. J. R., Matcham, G. W. J., and Mcdonald, E. (1980). Biosynthesis of the pigments of life: formation of the macrocycle. Nature 285, 17–21. doi:10.1038/285017a0
Battersby, A. R., and Mcdonald, E. (1979). Origin of the pigments of life: the type-III problem in porphyrin biosynthesis. Accounts Chem. Res. 12, 14–22. doi:10.1021/ar50133a003
Berger, O., Adler-Abramovich, L., Levy-Sakin, M., Grunwald, A., Liebes-Peer, Y., Bachar, M., et al. (2015). Light-emitting self-assembled peptide nucleic acids exhibit both stacking interactions and Watson–Crick base pairing. Nat. Nanotechnol. 10, 353–360. doi:10.1038/nnano.2015.27
Bonnett, R., Buckley, D. G., Burrow, T., Galia, A. B. B., Saville, B., and Songca, S. P. (1993). Photobactericidal materials based on porphyrins and phthalocyanines. J. Mater. Chem. 3, 323–324. doi:10.1039/jm9930300323
Bottari, G., Trukhina, O., Ince, M., and Torres, T. (2012). Towards artificial photosynthesis: supramolecular, donor–acceptor, porphyrin- and phthalocyanine/carbon nanostructure ensembles. Coord. Chem. Rev. 256, 2453–2477. doi:10.1016/j.ccr.2012.03.011
Brown, S. B., Brown, E. A., and Walker, I. (2004). The present and future role of photodynamic therapy in cancer treatment. Lancet Oncol. 5, 497–508. doi:10.1016/s1470-2045(04)01529-3
Brulé, E., De Miguel, Y. R., and Hii, K. K. (2004). Chemoselective epoxidation of dienes using polymer-supported manganese porphyrin catalysts. Tetrahedron 60, 5913–5918. doi:10.1016/j.tet.2004.05.026
Bustos, J., Vargas, L., and Quintero, R. (2020). Porfiria intermitente aguda: reporte de caso. Biomedica 40, 14–19. doi:10.7705/biomedica.4767
Cabral, F. V., Dos Santos Souza, T. H., Sellera, F. P., Fontes, A., and Ribeiro, M. S. (2023). Strengthening collaborations at the Biology-Physics interface: trends in antimicrobial photodynamic therapy. Biophys. Rev. 15, 685–697. doi:10.1007/s12551-023-01066-5
Carrión, E. N., Santiago, J., Sabatino, D., and Gorun, S. M. (2017). Synthesis and photophysical and photocatalytic properties of a highly fluorinated and durable phthalocyanine–peptide bioconjugate for potential theranostic applications. Inorg. Chem. 56, 7210–7216. doi:10.1021/acs.inorgchem.7b00847
Castano, A. P., Demidova, T. N., and Hamblin, M. R. (2004). Mechanisms in photodynamic therapy: part one-photosensitizers, photochemistry and cellular localization. Photodiagnosis Photodyn. Ther. 1, 279–293. doi:10.1016/s1572-1000(05)00007-4
Castano, A. P., Demidova, T. N., and Hamblin, M. R. (2005). Mechanisms in photodynamic therapy: part two-cellular signaling, cell metabolism and modes of cell death. Photodiagnosis Photodyn. Ther. 2, 1–23. doi:10.1016/s1572-1000(05)00030-x
Castano, A. P., Mroz, P., and Hamblin, M. R. (2006). Photodynamic therapy and anti-tumour immunity. Nat. Rev. Cancer 6, 535–545. doi:10.1038/nrc1894
Chang, R., Nikoloudakis, E., Zou, Q., Mitraki, A., Coutsolelos, A. G., and Yan, X. (2020). Supramolecular nanodrugs constructed by self-assembly of peptide nucleic acid–photosensitizer conjugates for photodynamic therapy. ACS Appl. Bio Mater. 3, 2–9. doi:10.1021/acsabm.9b00558
Charalambidis, G., Georgilis, E., Panda, M. K., Anson, C. E., Powell, A. K., Doyle, S., et al. (2016). A switchable self-assembling and disassembling chiral system based on a porphyrin-substituted phenylalanine–phenylalanine motif. Nat. Commun. 7, 12657. doi:10.1038/ncomms12657
Charalambidis, G., Kasotakis, E., Lazarides, T., Mitraki, A., and Coutsolelos, A. G. (2011). Self-assembly into spheres of a hybrid diphenylalanine–porphyrin: increased fluorescence lifetime and conserved electronic properties. Chem. A Eur. J. 17, 7213–7219. doi:10.1002/chem.201100362
Chen, J., Zhu, Y., and Kaskel, S. (2021). Porphyrin-based metal–organic frameworks for biomedical applications. Angew. Chem. Int. Ed. 60, 5010–5035. doi:10.1002/anie.201909880
Cheng, W. R., Haedicke, I. E., Nofiele, J., Martinez, F., Beera, K., Scholl, T. J., et al. (2014). Complementary strategies for developing Gd-free high-field T1 MRI contrast agents based on MnIII porphyrins. J. Med. Chem. 57, 516–520. doi:10.1021/jm401124b
Chudakov, D. M., Matz, M. V., Lukyanov, S., and Lukyanov, K. A. (2010). Fluorescent proteins and their applications in imaging living cells and tissues. Physiol. Rev. 90, 1103–1163. doi:10.1152/physrev.00038.2009
Connell, T. U., and Donnelly, P. S. (2018). Labelling proteins and peptides with phosphorescent d6 transition metal complexes. Coord. Chem. Rev. 375, 267–284. doi:10.1016/j.ccr.2017.12.001
Cordes, D. B., Lickiss, P. D., and Rataboul, F. (2010). Recent developments in the chemistry of cubic polyhedral oligosilsesquioxanes. Chem. Rev. 110, 2081–2173. doi:10.1021/cr900201r
Dąbrowski, J. M., Pucelik, B., Regiel-Futyra, A., Brindell, M., Mazuryk, O., Kyzioł, A., et al. (2016). Engineering of relevant photodynamic processes through structural modifications of metallotetrapyrrolic photosensitizers. Coord. Chem. Rev. 325, 67–101. doi:10.1016/j.ccr.2016.06.007
Dean, K. M., and Palmer, A. E. (2014). Advances in fluorescence labeling strategies for dynamic cellular imaging. Nat. Chem. Biol. 10, 512–523. doi:10.1038/nchembio.1556
De Rosa, F. S., and Bentley, M. V. L. B. (2000). Photodynamic therapy of skin cancers: sensitizers, clinical studies and future directives. Pharm. Res. 17, 1447–1455. doi:10.1023/a:1007612905378
Dias, C. J., Sardo, I., Moura, N. M. M., Felgueiras, J., Neves, M. G. P. M. S., Fardilha, M., et al. (2020). An efficient synthetic access to new uracil-alditols bearing a porphyrin unit and biological assessment in prostate cancer cells. Dyes Pigments 173, 107996. doi:10.1016/j.dyepig.2019.107996
Ding, L. G., Wang, S., Yao, B. J., Li, F., Li, Y. A., Zhao, G. Y., et al. (2021). Synergistic antibacterial and anti-inflammatory effects of a drug-loaded self-standing porphyrin-COF membrane for efficient skin wound healing. Adv. Healthc. Mater. 10, e2001821. doi:10.1002/adhm.202001821
Dmitriev, R. I., O'donnell, N., and Papkovsky, D. B. (2016). Metallochelate coupling of phosphorescent Pt-porphyrins to peptides, proteins, and self-assembling protein nanoparticles. Bioconjugate Chem. 27, 439–445. doi:10.1021/acs.bioconjchem.5b00535
Domingo-Tafalla, B., Chatterjee, T., and Palomares, E. (2023). Recent advances in the rational designing of metalloporphyrinoid-based CO2 reduction catalysts: from molecular structural tuning to the application in catalysis. J. Porphyr. Phthalocyanines 27, 23–46. doi:10.1142/s1088424623300033
Dong, X., Chen, H. L., Qin, J. W., Wei, C., Liang, J., Liu, T. J., et al. (2017). Thermosensitive porphyrin-incorporated hydrogel with four-arm PEG-PCL copolymer (II): doxorubicin loaded hydrogel as a dual fluorescent drug delivery system for simultaneous imaging tracking in vivo. Drug Deliv. 24, 641–650. doi:10.1080/10717544.2017.1289570
Donnelly, R. F., Cassidy, C. M., Loughlin, R. G., Brown, A., Tunney, M. M., Jenkins, M. G., et al. (2009). Delivery of Methylene Blue and meso-tetra (N-methyl-4-pyridyl) porphine tetra tosylate from cross-linked poly(vinyl alcohol) hydrogels: a potential means of photodynamic therapy of infected wounds. J. Photochem. Photobiol. B Biol. 96, 223–231. doi:10.1016/j.jphotobiol.2009.06.010
Dontas, I. A., Lelovas, P., Parara, S., Galanos, A., Agrogiannis, G., Goutas, D., et al. (2023). Delivery of porphyrins through self-assembling peptide hydrogels for accelerated healing of experimental skin defects in vivo. Cureus 15, e39120. doi:10.7759/cureus.39120
Egeblad, M., and Werb, Z. (2002). New functions for the matrix metalloproteinases in cancer progression. Nat. Rev. Cancer 2, 161–174. doi:10.1038/nrc745
Elangwe, C. N., Morozkina, S. N., Olekhnovich, R. O., Krasichkov, A., Polyakova, V. O., and Uspenskaya, M. V. (2022). A review on chitosan and cellulose hydrogels for wound dressings. Polymers 14, 5163. doi:10.3390/polym14235163
Escudero, C., Crusats, J., Díez-Pérez, I., El-Hachemi, Z., and Ribó, J. M. (2006). Folding and hydrodynamic forces in J-aggregates of 5-Phenyl-10,15,20-tris(4-sulfophenyl)porphyrin. Angew. Chem. Int. Ed. 45, 8032–8035. doi:10.1002/anie.200603182
Ethirajan, M., Chen, Y., Joshi, P., and Pandey, R. K. (2011). The role of porphyrin chemistry in tumor imaging and photodynamic therapy. Chem. Soc. Rev. 40, 340–362. doi:10.1039/b915149b
Feng, X., Jiang, D., Kang, T., Yao, J., Jing, Y., Jiang, T., et al. (2016). Tumor-homing and penetrating peptide-functionalized photosensitizer-conjugated PEG-PLA nanoparticles for chemo-photodynamic combination therapy of drug-resistant cancer. ACS Appl. Mater. Interfaces 8, 17817–17832. doi:10.1021/acsami.6b04442
Fichman, G., and Gazit, E. (2014). Self-assembly of short peptides to form hydrogels: design of building blocks, physical properties and technological applications. Acta Biomater. 10, 1671–1682. doi:10.1016/j.actbio.2013.08.013
Fine, S. L. (1999). Photodynamic therapy with verteporfin is effective for selected patients with neovascular age-related macular degeneration. Archives Ophthalmol. 117, 1400–1402. doi:10.1001/archopht.117.10.1400
Frank, H. A., and Cogdell, R. J. (2012). “8.6 light capture in photosynthesis,” in Comprehensive biophysics. Editor E. H. Egelman (Amsterdam: Elsevier), 94–114.
Glymenaki, E., Kandyli, M., Apostolidou, C. P., Kokotidou, C., Charalambidis, G., Nikoloudakis, E., et al. (2022). Design and synthesis of porphyrin–nitrilotriacetic acid dyads with potential applications in peptide labeling through metallochelate coupling. ACS Omega 7, 1803–1818. doi:10.1021/acsomega.1c05013
Guamba, E., Vispo, N. S., Whitehead, D. C., Singh, A. K., Santos-Oliveira, R., Niebieskikwiat, D., et al. (2023). Cellulose-based hydrogels towards an antibacterial wound dressing. Biomaterials Sci. 11, 3461–3468. doi:10.1039/d2bm01369j
Hammerer, F., Garcia, G., Chen, S., Poyer, F., Achelle, S., Fiorini-Debuisschert, C., et al. (2014). Synthesis and characterization of glycoconjugated porphyrin triphenylamine hybrids for targeted two-photon photodynamic therapy. J. Org. Chem. 79, 1406–1417. doi:10.1021/jo402658h
Han, J., Liu, Y., Peng, D., Liu, J., and Wu, D. (2023). Biomedical application of porphyrin-based amphiphiles and their self-assembled nanomaterials. Bioconjugate Chem. 34, 2155–2180. doi:10.1021/acs.bioconjchem.3c00432
Han, K., Zhang, J., Zhang, W., Wang, S., Xu, L., Zhang, C., et al. (2017). Tumor-triggered geometrical shape switch of chimeric peptide for enhanced in vivo tumor internalization and photodynamic therapy. ACS Nano 11, 3178–3188. doi:10.1021/acsnano.7b00216
Haynes, D. A., Van Laeren, L. J., and Munro, O. Q. (2017). Cobalt porphyrin–thiazyl radical coordination polymers: toward metal–organic electronics. J. Am. Chem. Soc. 139, 14620–14637. doi:10.1021/jacs.7b07803
Helmich, F., Lee, C. C., Nieuwenhuizen, M. M. L., Gielen, J. C., Christianen, P. C. M., Larsen, A., et al. (2010). Dilution-induced self-assembly of porphyrin aggregates: a consequence of coupled equilibria. Angew. Chemie-International Ed. 49, 3939–3942. doi:10.1002/anie.201000162
Huang, H. Y., Song, W. T., Rieffel, J., and Lovell, J. F. (2015). Emerging applications of porphyrins in photomedicine. Front. Phys. 3, 23. doi:10.3389/fphy.2015.00023
Huang, P., Qian, X., Chen, Y., Yu, L., Lin, H., Wang, L., et al. (2017). Metalloporphyrin-encapsulated biodegradable nanosystems for highly efficient magnetic resonance imaging-guided sonodynamic cancer therapy. J. Am. Chem. Soc. 139, 1275–1284. doi:10.1021/jacs.6b11846
Huang, S., Chen, K., and Li, T.-T. (2022). Porphyrin and phthalocyanine based covalent organic frameworks for electrocatalysis. Coord. Chem. Rev. 464, 214563. doi:10.1016/j.ccr.2022.214563
Ikeda, A., Satake, S., Mae, T., Ueda, M., Sugikawa, K., Shigeto, H., et al. (2017). Photodynamic activities of porphyrin derivative–cyclodextrin complexes by photoirradiation. ACS Med. Chem. Lett. 8, 555–559. doi:10.1021/acsmedchemlett.7b00098
Ishizuka, T., Grover, N., Kingsbury, C. J., Kotani, H., Senge, M. O., and Kojima, T. (2022). Nonplanar porphyrins: synthesis, properties, and unique functionalities. Chem. Soc. Rev. 51, 7560–7630. doi:10.1039/d2cs00391k
Jenkins, S. V., Srivatsan, A., Reynolds, K. Y., Gao, F., Zhang, Y. B., Heyes, C. D., et al. (2016). Understanding the interactions between porphyrin-containing photosensitizers and polymer-coated nanoparticles in model biological environments. J. Colloid Interface Sci. 461, 225–231. doi:10.1016/j.jcis.2015.09.037
Jin, G.-Q., Wang, J.-X., Lu, J., Zhang, H., Yao, Y., Ning, Y., et al. (2023). Two birds one stone: β-fluoropyrrolyl-cysteine SNAr chemistry enabling functional porphyrin bioconjugation. Chem. Sci. 14, 2070–2081. doi:10.1039/d2sc06209g
Jin, J., Zhu, Y., Zhang, Z., and Zhang, W. (2018). Enhancing the efficacy of photodynamic therapy through a porphyrin/POSS alternating copolymer. Angew. Chem. Int. Ed. 57, 16354–16358. doi:10.1002/anie.201808811
Juzeniene, A., and Moan, J. (2007). The history of PDT in Norway Part one: identification of basic mechanisms of general PDT. Photodiagnosis Photodyn. Ther. 4, 3–11. doi:10.1016/j.pdpdt.2006.11.002
Kamolz, L. P., Kotzbeck, P., Schintler, M., and Spendel, S. (2022). Skin regeneration, repair, and reconstruction: present and future. Eur. Surg. 54, 163–169. doi:10.1007/s10353-022-00757-9
Karikis, K., Butkiewicz, A., Folias, F., Charalambidis, G., Kokotidou, C., Charisiadis, A., et al. (2018). Self-assembly of (boron-dipyrromethane)-diphenylalanine conjugates forming chiral supramolecular materials. Nanoscale 10, 1735–1741. doi:10.1039/c7nr08667a
Karikis, K., Georgilis, E., Charalambidis, G., Petrou, A., Vakuliuk, O., Chatziioannou, T., et al. (2016). Corrole and porphyrin amino acid conjugates: synthesis and physicochemical properties. Chem. A Eur. J. 22, 11245–11252. doi:10.1002/chem.201601026
Kim, K. S., Lim, J. M., Osuka, A., and Kim, D. (2008). Various strategies for highly-efficient two-photon absorption in porphyrin arrays. J. Photochem. Photobiol. C Photochem. Rev. 9, 13–28. doi:10.1016/j.jphotochemrev.2008.01.001
Králová, J., Kejík, Z., Bříza, T., Poučková, P., Král, A., Martásek, P., et al. (2010). Porphyrin−Cyclodextrin conjugates as a nanosystem for versatile drug delivery and multimodal cancer therapy. J. Med. Chem. 53, 128–138. doi:10.1021/jm9007278
Kunishima, M., Kawachi, C., Monta, J., Terao, K., Iwasaki, F., and Tani, S. (1999). 4-(4,6-dimethoxy-1,3,5-triazin-2-yl)-4-methyl-morpholinium chloride: an efficient condensing agent leading to the formation of amides and esters. Tetrahedron 55, 13159–13170. doi:10.1016/s0040-4020(99)00809-1
Kwiatkowski, S., Knap, B., Przystupski, D., Saczko, J., Kędzierska, E., Knap-Czop, K., et al. (2018). Photodynamic therapy – mechanisms, photosensitizers and combinations. Biomed. Pharmacother. 106, 1098–1107. doi:10.1016/j.biopha.2018.07.049
Lai, Y. T., Chang, Y. Y., Hu, L. G., Yang, Y., Chao, A. L., Du, Z. Y., et al. (2015). Rapid labeling of intracellular His-tagged proteins in living cells. Proc. Natl. Acad. Sci. 112, 2948–2953. doi:10.1073/pnas.1419598112
Lazarides, T., Delor, M., Sazanovich, I. V., Mccormick, T. M., Georgakaki, I., Charalambidis, G., et al. (2014). Photocatalytic hydrogen production from a noble metal free system based on a water soluble porphyrin derivative and a cobaloxime catalyst. Chem. Commun. 50, 521–523. doi:10.1039/c3cc45025b
Leonhardt, F., Gennari, A., Paludo, G. B., Schmitz, C., Da Silveira, F. X., Moura, D. C. D. A., et al. (2023). A systematic review about affinity tags for one-step purification and immobilization of recombinant proteins: integrated bioprocesses aiming both economic and environmental sustainability. 3 Biotech. 13, 186. doi:10.1007/s13205-023-03616-w
Li, H. F., Xing, C. J., Huo, W. T., Zhao, J., Hao, Y. C., and Hu, Z. J. (2021a). Photoelectric conversion based on peptide-porphyrin conjugates assembled hydrogel. New J. Chem. 45, 7052–7055. doi:10.1039/d1nj00573a
Li, S., Zou, Q., Xing, R., Govindaraju, T., Fakhrullin, R., and Yan, X. (2019a). Peptide-modulated self-assembly as a versatile strategy for tumor supramolecular nanotheranostics. Theranostics 9, 3249–3261. doi:10.7150/thno.31814
Li, S.-Y., Cheng, H., Qiu, W.-X., Liu, L.-H., Chen, S., Hu, Y., et al. (2015). Protease-activable cell-penetrating peptide–protoporphyrin conjugate for targeted photodynamic therapy in vivo. ACS Appl. Mater. Interfaces 7, 28319–28329. doi:10.1021/acsami.5b08637
Li, S.-Y., Cheng, H., Xie, B.-R., Qiu, W.-X., Zeng, J.-Y., Li, C.-X., et al. (2017). Cancer cell membrane camouflaged cascade bioreactor for cancer targeted starvation and photodynamic therapy. ACS Nano 11, 7006–7018. doi:10.1021/acsnano.7b02533
Li, W. Q., Jian, X. L., Zou, Y. F., Wu, L., Huang, H. Y., Li, H., et al. (2021b). The fabrication of a gellan gum-based hydrogel loaded with magnesium ions for the synergistic promotion of skin wound healing. Front. Bioeng. Biotechnol. 9, 709679. doi:10.3389/fbioe.2021.709679
Li, X., Bai, H., Yang, Y., Yoon, J., Wang, S., and Zhang, X. (2019b). Supramolecular antibacterial materials for combatting antibiotic resistance. Adv. Mater. 31, 1805092. doi:10.1002/adma.201805092
Li, Y., Zou, Q., Yuan, C., Li, S., Xing, R., and Yan, X. (2018). Amino acid coordination driven self-assembly for enhancing both the biological stability and tumor accumulation of curcumin. Angew. Chem. Int. Ed. 57, 17084–17088. doi:10.1002/anie.201810087
Lim, W. Q., Yang, G., Phua, S. Z. F., Chen, H., and Zhao, Y. (2019). Self-assembled oxaliplatin(IV) prodrug–porphyrin conjugate for combinational photodynamic therapy and chemotherapy. ACS Appl. Mater. Interfaces 11, 16391–16401. doi:10.1021/acsami.9b04557
Lin, S., Huang, H., Ma, T., and Zhang, Y. (2020). Photocatalytic oxygen evolution from water splitting. Adv. Sci. 8, 2002458. doi:10.1002/advs.202002458
Lindsey, J. S., Schreiman, I. C., Hsu, H. C., Kearney, P. C., and Marguerettaz, A. M. (1987). Rothemund and Adler-Longo reactions revisited: synthesis of tetraphenylporphyrins under equilibrium conditions. J. Org. Chem. 52, 827–836. doi:10.1021/jo00381a022
Liu, G., Xu, X., Chen, Y., Wu, X., Wu, H., and Liu, Y. (2016). A highly efficient supramolecular photoswitch for singlet oxygen generation in water. Chem. Commun. 52, 7966–7969. doi:10.1039/c6cc02996e
Liu, X., Zhan, W., Gao, G., Jiang, Q., Zhang, X., Zhang, H., et al. (2023). Apoptosis-amplified assembly of porphyrin nanofiber enhances photodynamic therapy of oral tumor. J. Am. Chem. Soc. 145, 7918–7930. doi:10.1021/jacs.2c13189
Loo, Y., Goktas, M., Tekinay, A. B., Guler, M. O., Hauser, C. a.E., and Mitraki, A. (2015). Self-assembled proteins and peptides as scaffolds for tissue regeneration. Adv. Healthc. Mater. 4, 2557–2586. doi:10.1002/adhm.201500402
Loo, Y., Wong, Y. C., Cai, E. Z., Ang, C. H., Raju, A., Lakshmanan, A., et al. (2014). Ultrashort peptide nanofibrous hydrogels for the acceleration of healing of burn wounds. Biomaterials 35, 4805–4814. doi:10.1016/j.biomaterials.2014.02.047
Lotze, J., Reinhardt, U., Seitz, O., and Beck-Sickinger, A. G. (2016). Peptide-tags for site-specific protein labelling in vitro and in vivo. Mol. Biosyst. 12, 1731–1745. doi:10.1039/c6mb00023a
Lovell, J. F., Roxin, A., Ng, K. K., Qi, Q., Mcmullen, J. D., Dacosta, R. S., et al. (2011). Porphyrin-Cross-linked hydrogel for fluorescence-guided monitoring and surgical resection. Biomacromolecules 12, 3115–3118. doi:10.1021/bm200784s
Lu, K., He, C., and Lin, W. (2014). Nanoscale metal–organic framework for highly effective photodynamic therapy of resistant head and neck cancer. J. Am. Chem. Soc. 136, 16712–16715. doi:10.1021/ja508679h
Lucky, S. S., Soo, K. C., and Zhang, Y. (2015). Nanoparticles in photodynamic therapy. Chem. Rev. 115, 1990–2042. doi:10.1021/cr5004198
Lv, F., Mao, L., and Liu, T. (2014). Thermosensitive porphyrin-incorporated hydrogel with four-arm PEG–PCL copolymer: preparation, characterization and fluorescence imaging in vivo. Mater. Sci. Eng. C 43, 221–230. doi:10.1016/j.msec.2014.07.019
Mahler, A., Reches, M., Rechter, M., Cohen, S., and Gazit, E. (2006). Rigid, self-assembled hydrogel composed of a modified aromatic dipeptide. Adv. Mater. 18, 1365–1370. doi:10.1002/adma.200501765
Managa, M., Britton, J., Prinsloo, E., and Nyokong, T. (2018). Effects of Pluronic F127 micelles as delivering agents on the vitro dark toxicity and photodynamic therapy activity of carboxy and pyrene substituted porphyrins. Polyhedron 152, 102–107. doi:10.1016/j.poly.2018.06.031
Martinez-Serrano, R. D., Ugone, V., Porcu, P., Vonlanthen, M., Sorroza-Martinez, K., Cuetara-Guadarrama, F., et al. (2022). Novel porphyrin-containing hydrogels obtained by frontal polymerization: synthesis, characterization and optical properties. Polymer 247, 124785. doi:10.1016/j.polymer.2022.124785
Mckenzie, L. K., Bryant, H. E., and Weinstein, J. A. (2019). Transition metal complexes as photosensitisers in one- and two-photon photodynamic therapy. Coord. Chem. Rev. 379, 2–29. doi:10.1016/j.ccr.2018.03.020
Miao, Y. Y., Lv, S. B., Zheng, D. Y., Liu, Y. H., Liu, D. P., and Song, F. L. (2021). Porphyrin-based metal coordination polymers with self-assembly pathway-dependent properties for photodynamic and photothermal therapy. Biomaterials Sci. 9, 2533–2541. doi:10.1039/d0bm02112a
Molina, D., Follana-Berná, J., and Sastre-Santos, Á. (2023). Phthalocyanines, porphyrins and other porphyrinoids as components of perovskite solar cells. J. Mater. Chem. C 11, 7885–7919. doi:10.1039/d2tc04441b
Mrinalini, M., Naresh, M., Prasanthkumar, S., and Giribabu, L. (2021). Porphyrin-based supramolecular assemblies and their applications in NLO and PDT. J. Porphyr. Phthalocyanines 25, 382–395. doi:10.1142/s1088424621500243
Nikoloudakis, E., Karikis, K., Han, J., Kokotidou, C., Charisiadis, A., Folias, F., et al. (2019). A self-assembly study of PNA–porphyrin and PNA–BODIPY hybrids in mixed solvent systems. Nanoscale 11, 3557–3566. doi:10.1039/c8nr05667f
Nikoloudakis, E., Karikis, K., Laurans, M., Kokotidou, C., Solé-Daura, A., Carbó, J. J., et al. (2018). Self-assembly study of nanometric spheres from polyoxometalate-phenylalanine hybrids, an experimental and theoretical approach. Dalton Trans. 47, 6304–6313. doi:10.1039/c8dt00380g
Nikoloudakis, E., López-Duarte, I., Charalambidis, G., Ladomenou, K., Ince, M., and Coutsolelos, A. G. (2022). Porphyrins and phthalocyanines as biomimetic tools for photocatalytic H2 production and CO2 reduction. Chem. Soc. Rev. 51, 6965–7045. doi:10.1039/d2cs00183g
Nikolova, E. N., Kim, E., Wise, A. A., O’brien, P. J., Andricioaei, I., and Al-Hashimi, H. M. (2011). Transient Hoogsteen base pairs in canonical duplex DNA. Nature 470, 498–502. doi:10.1038/nature09775
Nuutila, K., and Eriksson, E. (2021). Moist wound healing with commonly available dressings. Adv. Wound Care 10, 685–698. doi:10.1089/wound.2020.1232
Ochsner, M. (1996). Light scattering of human skin: a comparison between zinc(II)— phthalocyanine and photofrin II®. J. Photochem. Photobiol. B Biol. 32, 3–9. doi:10.1016/1011-1344(95)07209-8
Ogoshi, H., and Mizutani, T. (1999). Novel approaches to molecular recognition using porphyrins. Curr. Opin. Chem. Biol. 3, 736–739. doi:10.1016/s1367-5931(99)00033-2
O'neill, J. S., Kearney, L., Brandon, M. P., and Pryce, M. T. (2022). Design components of porphyrin-based photocatalytic hydrogen evolution systems: a review. Coord. Chem. Rev. 467, 214599. doi:10.1016/j.ccr.2022.214599
Ong, S. E., and Mann, M. (2006). A practical recipe for stable isotope labeling by amino acids in cell culture (SILAC). Nat. Protoc. 1, 2650–2660. doi:10.1038/nprot.2006.427
Panagiotakis, S., Mavroidi, B., Athanasopoulos, A., Charalambidis, G., Coutsolelos, A. G., Paravatou-Petsotas, M., et al. (2022). Unsymmetrical, monocarboxyalkyl meso-arylporphyrins in the photokilling of breast cancer cells using permethyl-β-cyclodextrin as sequestrant and cell uptake modulator. Carbohydr. Polym. 275, 118666. doi:10.1016/j.carbpol.2021.118666
Panagiotakis, S., Mavroidi, B., Athanasopoulos, A., Gonçalves, A. R., Bugnicourt-Moreira, L., Regagnon, T., et al. (2023). Small anticancer drug release by light: photochemical internalization of porphyrin-β-cyclodextrin nanoparticles. Carbohydr. Polym. 306, 120579. doi:10.1016/j.carbpol.2023.120579
Park, J. M., Hong, K.-I., Lee, H., and Jang, W.-D. (2021). Bioinspired applications of porphyrin derivatives. Accounts Chem. Res. 54, 2249–2260. doi:10.1021/acs.accounts.1c00114
Parsons, C., Mccoy, C. P., Gorman, S. P., Jones, D. S., Bell, S. E. J., Brady, C., et al. (2009). Anti-infective photodynamic biomaterials for the prevention of intraocular lens-associated infectious endophthalmitis. Biomaterials 30, 597–602. doi:10.1016/j.biomaterials.2008.10.015
Petter, R. C., Salek, J. S., Sikorski, C. T., Kumaravel, G., and Lin, F. T. (1990). Cooperative binding by aggregated mono-6-(alkylamino)-.beta.-cyclodextrins. J. Am. Chem. Soc. 112, 3860–3868. doi:10.1021/ja00166a021
Piradi, V., Yan, F., Zhu, X., and Wong, W.-Y. (2021). A recent overview of porphyrin-based π-extended small molecules as donors and acceptors for high-performance organic solar cells. Mater. Chem. Front. 5, 7119–7133. doi:10.1039/d1qm00952d
Rabiee, N., Yaraki, M. T., Garakani, S. M., Garakani, S. M., Ahmadi, S., Lajevardi, A., et al. (2020). Recent advances in porphyrin-based nanocomposites for effective targeted imaging and therapy. Biomaterials 232, 119707. doi:10.1016/j.biomaterials.2019.119707
Rai, A., and Ferreira, L. (2021). Biomedical applications of the peptide decorated gold nanoparticles. Crit. Rev. Biotechnol. 41, 186–215. doi:10.1080/07388551.2020.1853031
Rocha-Santos, T. a.P. (2014). Sensors and biosensors based on magnetic nanoparticles. TrAC Trends Anal. Chem. 62, 28–36. doi:10.1016/j.trac.2014.06.016
Sandland, J., Rimmer, S. D., Savoie, H., and Boyle, R. W. (2021). Bio-orthogonal conjugation of a cationic metalloporphyrin to BSA and HSA via “click” chemistry. ChemBioChem 22, 2624–2631. doi:10.1002/cbic.202100176
Schneider, A. F. L., and Hackenberger, C. P. R. (2017). Fluorescent labelling in living cells. Curr. Opin. Biotechnol. 48, 61–68. doi:10.1016/j.copbio.2017.03.012
Seaman, S. (2002). Dressing selection in chronic wound management. J. Am. Podiatric Med. Assoc. 92, 24–33. doi:10.7547/87507315-92-1-24
Shi, Y., Zhang, F., and Linhardt, R. J. (2021). Porphyrin-based compounds and their applications in materials and medicine. Dyes Pigments 188, 109136. doi:10.1016/j.dyepig.2021.109136
Siegel, R. L., Miller, K. D., Fuchs, H. E., and Jemal, A. (2022). Cancer statistics, 2022. CA A Cancer J. Clin. 72, 7–33. doi:10.3322/caac.21708
Spikes, J. D. (1977). “Photosensitization in biological systems,” in Research in photobiology. Editor A. Castellani (Boston, MA: Springer US), 231–233.
Stender, A. S., Marchuk, K., Liu, C., Sander, S., Meyer, M. W., Smith, E. A., et al. (2013). Single cell optical imaging and spectroscopy. Chem. Rev. 113, 2469–2527. doi:10.1021/cr300336e
Sułek, A., Pucelik, B., Kobielusz, M., Łabuz, P., Dubin, G., and Dąbrowski, J. M. (2019). Surface modification of nanocrystalline TiO2 materials with sulfonated porphyrins for visible light antimicrobial therapy. Catalysts 9, 821. doi:10.3390/catal9100821
Sun, B., Chang, R., Cao, S., Yuan, C., Zhao, L., Yang, H., et al. (2020). Acid-activatable transmorphic peptide-based nanomaterials for photodynamic therapy. Angew. Chem. Int. Ed. 59, 20582–20588. doi:10.1002/anie.202008708
Szeimies, R.-M., Dräger, J., Abels, C., and Landthaler, M. (2001). Chapter 1 History of photodynamic therapy in dermatology. Compr. Ser. Photosciences 2, 3–15. doi:10.1016/S1568-461X(01)80105-8
Takeo, M., Lee, W., and Ito, M. (2015). Wound healing and skin regeneration. Cold Spring Harb. Perspect. Med. 5, a023267. doi:10.1101/cshperspect.a023267
Taniguchi, M., and Lindsey, J. S. (2017). Synthetic chlorins, possible surrogates for chlorophylls, prepared by derivatization of porphyrins. Chem. Rev. 117, 344–535. doi:10.1021/acs.chemrev.5b00696
Thapa, R. K., Kim, J. O., and Kim, J. (2023). Antimicrobial strategies for topical biofilm-based wound infections: past, present, and future. J. Pharm. Investigation 53, 627–641. doi:10.1007/s40005-023-00628-9
Tian, B., Liu, Y., and Liu, J. (2020a). Cyclodextrin as a magic switch in covalent and non-covalent anticancer drug release systems. Carbohydr. Polym. 242, 116401. doi:10.1016/j.carbpol.2020.116401
Tian, J., Huang, B., Nawaz, M. H., and Zhang, W. (2020b). Recent advances of multi-dimensional porphyrin-based functional materials in photodynamic therapy. Coord. Chem. Rev. 420, 213410. doi:10.1016/j.ccr.2020.213410
Tian, Z., Li, H., Liu, Z., Yang, L., Zhang, C., He, J., et al. (2023). Enhanced photodynamic therapy by improved light energy capture efficiency of porphyrin photosensitizers. Curr. Treat. Options Oncol. 24, 1274–1292. doi:10.1007/s11864-023-01120-0
Urbani, M., Grätzel, M., Nazeeruddin, M. K., and Torres, T. (2014). Meso-substituted porphyrins for dye-sensitized solar cells. Chem. Rev. 114, 12330–12396. doi:10.1021/cr5001964
Velikyan, I. (2014). Prospective of 68Ga-radiopharmaceutical development. Theranostics 4, 47–80. doi:10.7150/thno.7447
Wang, M., Huang, G., You, Z., Jia, R., Zhong, Y., and Bai, F. (2023). Progress of porphyrin-based nanoassemblies for cancer theranostics. Chem. Res. Chin. Univ. 39, 612–623. doi:10.1007/s40242-023-3127-9
Wang, X., Yan, F., Liu, X., Wang, P., Shao, S., Sun, Y., et al. (2018). Enhanced drug delivery using sonoactivatable liposomes with membrane-embedded porphyrins. J. Control. Release 286, 358–368. doi:10.1016/j.jconrel.2018.07.048
Warszyńska, M., Repetowski, P., and Dąbrowski, J. M. (2023). Photodynamic therapy combined with immunotherapy: recent advances and future research directions. Coord. Chem. Rev. 495, 215350. doi:10.1016/j.ccr.2023.215350
Wu, K., Zheng, Y., Chen, R., Zhou, Z., Liu, S., Shen, Y., et al. (2023). Advances in electrochemiluminescence luminophores based on small organic molecules for biosensing. Biosens. Bioelectron. 223, 115031. doi:10.1016/j.bios.2022.115031
Wu, W.-J., Yang, W., and Tsai, M.-D. (2017). How DNA polymerases catalyse replication and repair with contrasting fidelity. Nat. Rev. Chem. 1, 0068. doi:10.1038/s41570-017-0068
Wu, X. L., Yang, H., Chen, X. M., Gao, J. X., Duan, Y., Wei, D. H., et al. (2021). Nano-herb medicine and PDT induced synergistic immunotherapy for colon cancer treatment. Biomaterials 269, 120654. doi:10.1016/j.biomaterials.2021.120654
Xing, R., Zou, Q., Yuan, C., Zhao, L., Chang, R., and Yan, X. (2019). Self-assembling endogenous biliverdin as a versatile near-infrared photothermal nanoagent for cancer theranostics. Adv. Mater. 31, 1900822. doi:10.1002/adma.201900822
Xue, X., Lindstrom, A., and Li, Y. (2019). Porphyrin-based nanomedicines for cancer treatment. Bioconjugate Chem. 30, 1585–1603. doi:10.1021/acs.bioconjchem.9b00231
Yang, Y., Wang, L., Cao, H., Li, Q., Li, Y., Han, M., et al. (2019). Photodynamic therapy with liposomes encapsulating photosensitizers with aggregation-induced emission. Nano Lett. 19, 1821–1826. doi:10.1021/acs.nanolett.8b04875
You, C. J., and Piehler, J. (2014). Multivalent chelators for spatially and temporally controlled protein functionalization. Anal. Bioanal. Chem. 406, 3345–3357. doi:10.1007/s00216-014-7803-y
Yu, Z., Pan, W., Li, N., and Tang, B. (2016). A nuclear targeted dual-photosensitizer for drug-resistant cancer therapy with NIR activated multiple ROS. Chem. Sci. 7, 4237–4244. doi:10.1039/c6sc00737f
Zagami, R., Rubin Pedrazzo, A., Franco, D., Caldera, F., De Plano, L. M., Trapani, M., et al. (2023). Supramolecular assemblies based on polymeric cyclodextrin nanosponges and a cationic porphyrin with antimicrobial photodynamic therapy action. Int. J. Pharm. 637, 122883. doi:10.1016/j.ijpharm.2023.122883
Zeng, K., Tong, Z., Ma, L., Zhu, W.-H., Wu, W., and Xie, Y. (2020). Molecular engineering strategies for fabricating efficient porphyrin-based dye-sensitized solar cells. Energy & Environ. Sci. 13, 1617–1657. doi:10.1039/c9ee04200h
Zhang, D., Qi, G. B., Zhao, Y. X., Qiao, S. L., Yang, C., and Wang, H. (2015). In situ formation of nanofibers from purpurin18-peptide conjugates and the assembly induced retention effect in tumor sites. Adv. Mater. 27, 6125–6130. doi:10.1002/adma.201502598
Zhang, L. P., Geng, Y., Li, L. J., Tong, X. F., Liu, S., Liu, X. M., et al. (2021). Rational design of iridium-porphyrin conjugates for novel synergistic photodynamic and photothermal therapy anticancer agents. Chem. Sci. 12, 5918–5925. doi:10.1039/d1sc00126d
Zhang, W., Lu, J., Gao, X. N., Li, P., Zhang, W., Ma, Y., et al. (2018). Enhanced photodynamic therapy by reduced levels of intracellular glutathione obtained by employing a nano-MOF with CuII as the active center. Angew. Chemie-International Ed. 57, 4891–4896. doi:10.1002/anie.201710800
Keywords: porphyrins, biomedical applications, drug release, photodynamic therapy, wound healing
Citation: Nikolaou V, Nikoloudakis E, Ladomenou K, Charalambidis G and Coutsolelos AG (2024) Porphyrins—valuable pigments of life. Front. Chem. Biol 2:1346465. doi: 10.3389/fchbi.2023.1346465
Received: 29 November 2023; Accepted: 27 December 2023;
Published: 10 January 2024.
Edited by:
Ana Maria Da Costa Ferreira, University of São Paulo, BrazilReviewed by:
Koiti Araki, University of São Paulo, BrazilCopyright © 2024 Nikolaou, Nikoloudakis, Ladomenou, Charalambidis and Coutsolelos. This is an open-access article distributed under the terms of the Creative Commons Attribution License (CC BY). The use, distribution or reproduction in other forums is permitted, provided the original author(s) and the copyright owner(s) are credited and that the original publication in this journal is cited, in accordance with accepted academic practice. No use, distribution or reproduction is permitted which does not comply with these terms.
*Correspondence: Kalliopi Ladomenou, a2xhZG9tZW5vdUBjaGVtLmlodS5ncg==; Georgios Charalambidis, Z2NoYXJhbEBlaWUuZ3I=; Athanassios G. Coutsolelos, YWNvdXRzb2xAdW9jLmdy
Disclaimer: All claims expressed in this article are solely those of the authors and do not necessarily represent those of their affiliated organizations, or those of the publisher, the editors and the reviewers. Any product that may be evaluated in this article or claim that may be made by its manufacturer is not guaranteed or endorsed by the publisher.
Research integrity at Frontiers
Learn more about the work of our research integrity team to safeguard the quality of each article we publish.