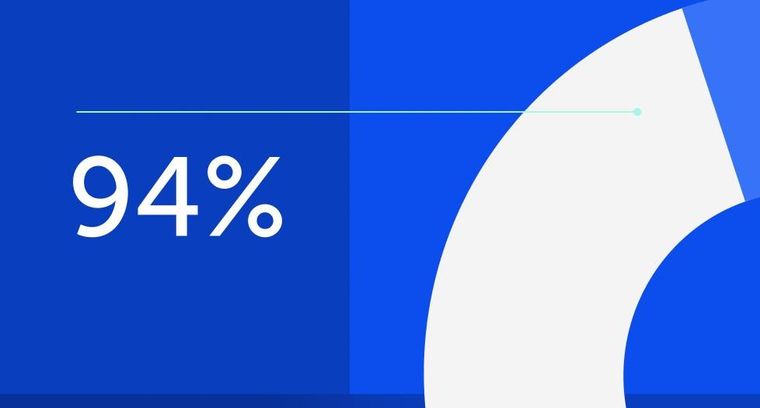
94% of researchers rate our articles as excellent or good
Learn more about the work of our research integrity team to safeguard the quality of each article we publish.
Find out more
ORIGINAL RESEARCH article
Front. Chem. Eng., 22 February 2024
Sec. Catalytic Engineering
Volume 6 - 2024 | https://doi.org/10.3389/fceng.2024.1334355
This study investigated the hydrodeoxygenation of palm oil by different oxide nanocatalysts of transition metals α -Fe2O3, NiO, and NiFe2O4, which were synthesized by hot injection. All nanomaterials were characterized by X-ray diffraction, Fourier transform infrared spectroscopy, and dynamic light dispersion. The catalytic evaluation was performed in a Parr-type reactor at 350°C, 3.5 MPa of H2 pressure, and 3 h of reaction. The liquid product obtained was analyzed by ultraviolet-visible light spectroscopy to identify the n-C16 generated during the reaction. The activity in the deoxygenation of fatty acids to produce n-C16 hydrocarbons has the following order: α-Fe2O3 < NiFe2O4 < NiO.
Deriving green diesel from the deoxygenation of vegetable oils has attracted attention due to the high increment of sulfur, nitrogen, oxygen, and metal heterocompounds in oil reserves, the high demand for fossil fuels, and the environmental problems they generate (Demirbas, 2004; Demirbas and Dincer, 2008). Therefore, it has become necessary to develop a green and renewable alternative that can be a substitute for petroleum-derived fuels. A wide variety of vegetable oils, such as soja, sunflower, jatropha, and palm (Guzman et al., 2010; Gong et al., 2012; Kim et al., 2013), have been used as feedstock for the production of this green diesel (Guzman et al., 2010; Gong et al., 2012; Kim et al., 2013). In particular, the molecular composition of palm oil contains mostly fatty acids such as C16 and C18, making it a promising feedstock (Srifa et al., 2014) because it contains relatively low concentrations of oxygen, which makes it very desirable for the production of hydrocarbons in the conventional diesel range. In addition, the fatty acid or triglyceride contents in certain oils (not edible) could be an alternative for producing clean n-C16. Green diesel has gained increased interest due to it being considered superior to fossil diesel and biodiesel. One of its most important attributes is a higher cetane index (ignition capacity in a certain period of time) because it does not contain oxygen in its molecules and has a high calorific value (Vonortas and Papayannakos, 2014). It is obtained by deoxygenation, hydrodeoxygenation (HDO), decarbonylation (DCO), and the decarboxylation (DCO2) of oil in the presence of hydrogen, high temperature (300°C–360 °C), H2 pressure (>3 MPa), and catalysts, which efficiently promote such reactions (Kovács et al., 2011; Morgan et al., 2012; Kubička et al., 2014; Srifa et al., 2015a; Srifa et al., 2015b). Therefore, the main objective of this research was to synthesize α-Fe2O3, NiFe2O4, and NiO nanocatalysts using the hot injection process, with only oleylamine as a surfactant, solvent, and reducing agent, as well as the catalytic evaluation of the deoxygenation of palm oil for the production of molecules in the diesel range.
Iron acetylacetonate (III) (Fe(C5H7O2)3, 97%), nickel acetylacetonate (II) (Ni(C5H7O2)2, 95%), oleylamine (C18H37N, technical grade, 70%) as reductor agent during the synthesis, ethanol (C2H6O, 99.9%) acquired in Sigma-Aldrich as the chemical solvent to remove organic residues from synthesis, heptane (n-C7, 99%) (Sigma-Aldrich), and toluene (C6H5CH3, 99.5%) acquired in Fermont were used as solvents in catalytic tests. Hexadecane (n-C16, 99%) (Sigma-Aldrich) was used as standard performance calibration for product analysis.
The nanomaterials were synthesized using hot injection. This method consisted of preheating the solvent oleylamine below its boiling point with a heating ramp of 20 °C/min and then adding the organometallic precursors. In general, for α -Fe2O3 catalysts, 2 mmol of the precursor Fe(acac)3 was added to the previously heated solvent, and the solution was kept under continuous agitation for 2 h. Next, for the NiO particles, 2 mmol of the precursor Ni(acac)2 was used in oleylamine with a reaction time of 2 h.
Finally, to synthesize the NiFe2O4 nanocatalysts, the same method was used slightly differently. The precursors were added in a 2:1 M ratio of Fe(acac)3 and Ni(acac)2 to the solvent to form a mixture and were kept under agitation for 3 h. The resulting solution from each reaction was removed from the heat source and allowed to cool to room temperature before any additional steps. Finally, the product was divided into three equal parts and deposited in 50-mL centrifuge tubes. Ethanol was added to each sample and centrifuged at 6,000 rpm for 25 min. At the end of centrifugation, the precipitate was collected and repeatedly washed with solvent to remove the residues from the reaction. Final samples were calcinated from room temperature to 673 K at 20 K/min over 2 h.
The crystal structure of the synthesized materials was obtained by X-ray diffraction (XRD) with a Bruker D8 Advance diffractometer under the following experimental conditions: CuKα radiation, 40 kV, and 30 mA, in a range of 2θ between 10° and 70°. The identification of the phase and crystal structure of each sample was determined using X’Highscore Plus software, version 3.0.5. Using the Debye–Scherrer equation, the crystal size for all synthesized samples was determined. Information was obtained on the functional groups present and the interactions between the bonds of the stabilizing molecules and the nanoparticles by FTIR spectroscopy in a Perkin Elmer spectrophotometer, Spectrum 100 model. The analyses were carried out in the mid-infrared region (4,000–500 cm−1). In addition, the distribution of the average size of the suspended particles was determined using the dynamic light dispersion technique with Anton Paar Litesizer 500 equipment and software. For this technique, the samples were prepared at a concentration of 0.1 mg/mL. JEOL, STEM J-7600 equipment was used to analyze the morphologic and particle size in the samples.
Catalytic deoxygenation tests were conducted in a batch reactor type Parr model 4,577. Each experiment was carried out with 26 mL of palm oil, 80 mL of n-heptane, and 1,000 ppm of the three different catalysts. The reactor was purged for 10 min with nitrogen to remove the air and then pressurized with hydrogen. The reaction conditions were 350 °C for 3 h and 3.5 MPa of H2 pressure. The agitation remained constant at 250 rpm to ensure the total dispersion of the nanocatalysts in the oil. A calibration curve was used to identify the n-C16 hydrocarbon generated during the reaction. A 1,000 mg·L−1 quantity of n-C16 stock solution had been previously prepared in toluene and dissolved in different concentrations. Changes in concentration were determined with the UV-vis spectrophotometer, Model Agilent Technologies-Carry-60. The initial concentrations for the calibration curve were solutions from 10 mg·L−1 to 60 mg·L−1. In brief, 1 μL of the sample was diluted in 100 mL of toluene, and the measurements were conducted to determine the n-alkane (n-C16) generated during the reaction.
The diffractogram of the materials synthesized from Fe(acac)3 is shown in Figure 1. The diffraction pattern indicates a rhombohedral structure (space group R-3, number 148). The peaks in 2θ = 24.125°, 33.115°, 35.612°, 40.836°, 49.417°, 54.005°, 62.385°, and 63.966° assigned to the planes (012), (104), (110), (113), (024), (116), (214), and (300) are characteristic of hematite phase α-Fe2O3 (PDF# 00-024-0072). By mixing the previous precursors of Fe(acac)3 and Ni(acac)2 in a 2:1 ratio, a cubic structure belonging to the space group Fd3m, number 227, was obtained. According to the diffraction peaks located in the plane, 2θ = 30.29°, 35.68°, 43.36°, 53.81°, 57.37°, and 63°, corresponding to the planes (220), (311), (400), (422), (511), and (440), are characteristic of the NiFe2O4 trevorite phase [PDF# 00-04, ISCD# 01-073-1523]. In both cases, the results were consistent with previous investigations (Belaïd et al., 2013; Kurtan et al., 2016; Babu and Reddy, 2020; Dippong et al., 2021). Figure 2 shows the diffractogram of the nanocatalysts synthesized from the organometallic precursor Ni(acac)2. The diffraction pattern indicates a cube structure centered on the faces (fcc) (space group Fm-3m, number 225). The peaks in 2θ = 37.22°, 43.25°, 62.83°, 75.35°, and 79.34° assigned to the planes (111), (200), (220), (311), and (222) correspond to NiO (ISCD# 01-073-1523). The same structure was reported in Gutierrez et al. (2013).
Using the Debye–Scherrer equation, the size of the crystal was determined for all the synthesized samples (Cullity and Stock, 2013). It was obtained from the maximum half-height width (FWHM) of the plane (104) α-Fe2O3, (200) NiO, and (311) NiFe2O4. For hematite and trevorite samples, the crystal size is equivalent to the particle size, suggesting a minimal form as a monocrystalline material. NiO catalysts are composed of 5.5 nm nanocrystals and have an average particle size of 146 nm, so it can be determined that it is a polycrystalline material. The summary of the DRX results is shown in Table 1.
The particle size distribution of the α-Fe2O3, NiO, and NiFe2O4 catalysts obtained by dynamic light dispersion is shown in Figure 3. The pink histogram shows particle sizes for hematite between 8 and 15 nm, with an average size of 11.74 nm. Trevorite catalysts have similar particle sizes of 8–17 nm and an average size of 12.28 nm, as can be seen on the blue histogram. NiO catalysts, unlike the previous two materials, have sizes between 100 and 280 nm caused by their possible agglomeration. This behavior was reported previously by Lam et al. (2020).
Figure 4 shows the micrographs of NiO, Fe2O3, and NiFe2O4 nanocatalysts. In general, the three micrographed materials exhibit particle sizes less than 50 nm; however, in two materials containing nickel, the presence of particles larger than 70–80 nm can be observed, attributed to agglomerations of smaller particles (Maity et al., 2009; Pan et al., 2014; Lam et al., 2020). Similar results were reported in DLS analyses, with smaller sizes and large particle size (>100 nm) attributable to the agglomeration of small particles. These differences were attributed to the precursors and reagents used in the synthesis. Furthermore, the micrographs for Fe2O3 and NiFe2O4 show the presence of nanoparticles less than 20 nm.
The nanomaterials were analyzed by IR spectroscopy to determine the functional groups present on the surface. Figure 5 shows the spectra of the nanocatalysts of α-Fe2O3, NiO, and NiFe2O4 in the range of 4,000 to 400 cm-1. All the samples analyzed exhibit bands between 2,922 cm−1 and 2,854 cm−1 that correspond to the symmetrical (vs.) and asymmetric (vas) stretching vibrations of the groups (C–H) (Mourdikoudis and Liz-Marzán, 2013). These assignments are attributed to the oleylamine used in the synthesis of the material, indicating the presence of amino groups on the surface of the catalysts, which provide stability and prevent them from agglomerating (Zhai et al., 2012). The bands between 500 and 400 cm−1 correspond to the strong vibrations of the metal–oxygen bonds (Maity et al., 2009; Pan et al., 2014; Lam et al., 2020).
The conversion of palm oil consists of several steps. First, the double bonds in the triglycerides of palm oil are hydrogenated by a catalyst to form saturated triglycerides. Then, the C–O bond in saturated triglycerides is disengaged by hydrogenolysis to generate fatty acids and propane (Ruangudomsakul et al., 2022). The catalytic deoxygenation of palmitic acid can be performed in three ways (Figure 6). Hydrodeoxygenation can mainly occur, eliminating oxygen in the form of H2O through the cleavage of the C–O bond in the presence of hydrogen. Similarly, oxygen can be eliminated by the formation of CO and/or CO2 through the disengagement of the C–C bond (Choi et al., 2018). In all three cases, reactions are promoted in the presence of a catalyst; to improve the performance of the reaction, a solvent is used with a lower boiling point than oil to promote better activity (Di Vito Nolfi et al., 2021). In addition, the presence of a solvent also affects the performance of the reaction, allowing a high diffusivity that reduces resistance to the mass transfer of the reagents by facilitating the contact of the H2 and reagents with the catalyst (Lucantonio et al., 2023). The solvent content considerably increased the pressure due to isothermal expansion during the reaction. The used condition reaction (350 °C for 3 h and 3.5 MPa of H2 pressure) promoted the selectivity to n-C16 detected during the reaction.
The catalytic evaluation showed that the most active material in the formation of hydrocarbons in the n-C16 range was NiO (the highest yield nearly 56%) due to high activation of hydrogenation and the fragmentation of the side chains of triglycerides. Small nickel particles with particularly active sites such as steps, corners, and defects tend to deoxygenate C–O bonds (Chen et al., 2016; Mortensen et al., 2016; Shafaghat et al., 2016; Wang et al., 2018; KretzschmarOliverSeifert, 2024). The NiFe2O4 nanocatalysts obtain 35% yield, thus promoting action attributed to the synergy of the nickel and iron sites but lower than NiO nanoparticles. Finally, the α-Fe2O3 nanocatalysts showed a decrease compared to the NiO and NiFe2O4 catalysts because iron without a hydrogen activation site does not completely promote hydrogenation. Therefore, the efficiency of the deoxygenation of fatty acids in producing n-C16 hydrocarbons has the following order: α-Fe2O3 < NiFe2O4 < NiO (Figure 7).
FIGURE 7. Yields to “green diesel” of the n-alkane (n-C16) generated by all the nanocatalysts in the deoxygenation of palm oil. (350 C, 3.5 MPa initial H2 pressure, and 3 h reaction).
These results demonstrate a viable, fast, economical, and promising route for the synthesis of α-Fe2O3, NiFe2O4, and NiO catalysts at the nanometer scale. Iron can considerably decrease the particle size of Ni, forming a ferrite-type structure; However, this hot injection method promotes the formation of nickel nanoparticles (less than 50 nm) and aggregated particle sizes from 100 to 160 nm. Catalytic evaluation confirmed that a catalyst promoted by Ni facilitates a greater degree of deoxygenation. The reaction conditions are considered appropriate (350 C, 3 h, and 3.5 MPa initial H2 pressure) for generating n-alkanes (n-C16) in the diesel range. In all experimental processes, n-C16 was obtained as the main product, with the presence of other products with a greater added value than the oil source.
The original contributions presented in the study are included in the article/Supplementary material; further inquiries can be directed to the corresponding author.
JM-B: conceptualization, investigation, methodology, project administration, validation, formal analysis, funding acquisition, resources, and writing–review and editing. CG-C: formal analysis, investigation, methodology, and writing–original draft. ML-M: investigation, supervision, and writing–review and editing. ND-Z: investigation, validation, and writing–review and editing. LV-I: investigation, writing–review and editing, and supervision. MM-M: data curation, supervision, and writing–review and editing.
The author(s) declare financial support was received for the research, authorship, and/or publication of this article. The authors acknowledge project 19000.23-P from Tecnológico Nacional de México for financial support.
The authors declare that the research was conducted in the absence of any commercial or financial relationships that could be construed as a potential conflict of interest.
All claims expressed in this article are solely those of the authors and do not necessarily represent those of their affiliated organizations, or those of the publisher, the editors, and the reviewers. Any product that may be evaluated in this article, or claim that may be made by its manufacturer, is not guaranteed or endorsed by the publisher.
Babu, L. K., and Reddy, Y. V. R. (2020). A novel thermal decomposition approach for the synthesis and properties of superparamagnetic nanocrystalline NiFe2O4 and its antibacterial, electrocatalytic properties. J. Supercond. Nov. Magn. 33 (4), 1013–1021. doi:10.1007/s10948-019-05262-x
Belaïd, S., Laurent, S., Vermeech, M., Vander Elst, L., Perez-Morga, D., and Muller, R. N. (2013). A new approach to follow the formation of iron oxide nanoparticles synthesized by thermal decomposition. Nanotechnology 24 (5), 055705. doi:10.1088/0957-4484/24/5/055705
Chen, L., Li, H., Fu, J., Miao, C., Lv, P., and Yuan, Z. (2016). Catalytic hydroprocessing of fatty acid methyl esters to renewable alkane fuels over Ni/HZSM-5 catalyst. Today 259 (1), 266–276. Part 2. doi:10.1016/j.cattod.2015.08.023
Choi, I.-H., Lee, J.-S., Kim, C.-U., Kim, T.-W., Lee, K.-Y., and Hwang, K.-R. (2018). Production of bio-jet fuel range alkanes from catalytic deoxygenation of Jatropha fatty acids on a WOx/Pt/TiO2 catalyst. Fuel (Lond) 215, 675–685. doi:10.1016/j.fuel.2017.11.094
Cullity, B. D., and Stock, S. R. (2013). Elements of X-ray diffraction: pearson new international edition. 3. London, England: Pearson Education.
Demirbas, A. (2004). Bioenergy, global warming, and environmental impacts. Energy sources. 26 (3), 225–236. doi:10.1080/00908310490256581
Demirbas, A., and Dincer, K. (2008). Sustainable green diesel: a futuristic view. Energy Sources Recovery Util. Environ. Eff. 30 (13), 1233–1241. doi:10.1080/15567030601082829
Dippong, T., Levei, E. A., and Cadar, O. (2021). Recent advances in synthesis and applications of MFe2O4 (M = co, Cu, Mn, Ni, Zn) nanoparticles. Nanomater. (Basel) 11 (6), 1560. doi:10.3390/nano11061560
Di Vito Nolfi, G., Gallucci, K., and Rossi, L. (2021). Green diesel production by catalytic hydrodeoxygenation of vegetables oils. Int. J. Environ. Res. Public Health 18 (24), 13041. doi:10.3390/ijerph182413041
Gong, S., Shinozaki, A., Shi, M., and Qian, E. W. (2012). Hydrotreating of Jatropha oil over alumina based catalysts. Energy fuels. 26 (4), 2394–2399. doi:10.1021/ef300047a
Gutierrez, A., Perpiñán, M. F., Sánchez, A. E., and Torralba, M. C. (2013). Solvotermal synthesis of NiO, Ni and NiS nanoparticles. J. Nanosci. Nanotechnol. 13 (1), 461–466. doi:10.1166/jnn.2013.6717
Guzman, A., Torres, J. E., Prada, L. P., and Nuñez, M. L. (2010). Hydroprocessing of crude palm oil at pilot plant scale. Catal. Today 156 (1–2), 38–43. doi:10.1016/j.cattod.2009.11.015
Kim, S. K., Brand, S., Lee, H.-S., Kim, Y., and Kim, J. (2013). Production of renewable diesel by hydrotreatment of soybean oil: effect of reaction parameters. Chem. Eng. J. 228, 114–123. doi:10.1016/j.cej.2013.04.095
Kovács, S., Kasza, T., Thernesz, A., Horváth, I. W., and Hancsók, J. (2011). Fuel production by hydrotreating of triglycerides on NiMo/Al2O3/Fcatalyst. Chem. Eng. J. 176–177, 237–243. doi:10.1016/j.cej.2011.05.110
KretzschmarOliverSeifert, N. B. M. (2024). Controlling the reaction network of Ni/silica derived conversion of bio-oil surrogate guaiacol. Carbon Resour. Convers. 7, 100187. doi:10.1016/j.crcon.2023.05.006
Kubička, D., Horáček, J., Setnička, M., Bulánek, R., Zukal, A., and Kubičková, I. (2014). Effect of support-active phase interactions on the catalyst activity and selectivity in deoxygenation of triglycerides. Appl. Catal. B 145, 101–107. doi:10.1016/j.apcatb.2013.01.012
Kurtan, U., Güngüneş, H., Sözeri, H., and Baykal, A. (2016). Synthesis and characterization of monodisperse NiFe2O4 nanoparticles. Ceram. Int. 42 (7), 7987–7992. doi:10.1016/j.ceramint.2016.01.200
Lam, M. M., Melo-Banda, J. A., Macias, F. D., Portales, B., Dominguez, J. M., Silva, R., et al. (2020). Transition metal nanocatalysts by modified inverse microemulsion for the heavy crude oil upgrading at reservoir. Catal. Today 349, 81–87. doi:10.1016/j.cattod.2018.05.052
Lucantonio, S., Di Giuliano, A., Rossi, L., and Gallucci, K. (2023). Green diesel production via deoxygenation process: a review. Energies 16 (2), 844. doi:10.3390/en16020844
Maity, D., Choo, S.-G., Yi, J., Ding, J., and Xue, J. M. (2009). Synthesis of magnetite nanoparticles via a solvent-free thermal decomposition route. J. Magn. Magn. Mater 321 (9), 1256–1259. doi:10.1016/j.jmmm.2008.11.013
Morgan, T., Santillan-Jimenez, E., Harman-Ware, A. E., Ji, Y., Grubb, D., and Crocker, M. (2012). Catalytic deoxygenation of triglycerides to hydrocarbons over supported nickel catalysts. Chem. Eng. J. 189–190, 346–355. doi:10.1016/j.cej.2012.02.027
Mortensen, P. M., Grunwaldt, J.-D., Jensen, P. A., and Jensen, A. D. (2016). Influence on nickel particle size on the hydrodeoxygenation of phenol over Ni/SiO2. Catal. Today 259 (1), 277–284. Part 2. doi:10.1016/j.cattod.2015.08.022
Mourdikoudis, S., and Liz-Marzán, L. M. (2013). Oleylamine in nanoparticle synthesis. Chem. Mater 25 (9), 1465–1476. doi:10.1021/cm4000476
Pan, Y., Jia, R., Zhao, J., Liang, J., Liu, Y., and Liu, C. (2014). Size-controlled synthesis of monodisperse nickel nanoparticles and investigation of their magnetic and catalytic properties. Appl. Surf. Sci. 316, 276–285. doi:10.1016/j.apsusc.2014.07.203
Ruangudomsakul, M., Osakoo, N., Wittayakun, J., Keawkumay, C., Butburee, T., Youngjan, S., et al. (2022). Hydrodeoxygenation of palm oil to green diesel products on mixed-phase nickel phosphides. Mol. Catal. 523 (111422), 111422. doi:10.1016/j.mcat.2021.111422
Shafaghat, H., Sirous Rezaei, P., Ashri, W. M., and Wan DaudRezaei, P. S. (2016). Catalytichydrodeoxygenation of simulated phenolic bio-oil to cycloalkanes and aromatic hydrocarbons over bifunctional metal/acid catalysts of Ni/HBeta, Fe/HBeta and NiFe/HBeta. J. Industrial Eng. Chem. 35, 268–276. doi:10.1016/j.jiec.2016.01.001
Srifa, A., Faungnawakij, K., Itthibenchapong, V., and Assabumrungrat, S. (2015a). Roles of monometallic catalysts in hydrodeoxygenation of palm oil to green diesel. Chem. Eng. J. 278, 249–258. doi:10.1016/j.cej.2014.09.106
Srifa, A., Faungnawakij, K., Itthibenchapong, V., Viriya-Empikul, N., Charinpanitkul, T., and Assabumrungrat, S. (2014). Production of bio-hydrogenated diesel by catalytic hydrotreating of palm oil over NiMoS2/γ-Al2O3 catalyst. Bioresour. Technol. 158, 81–90. doi:10.1016/j.biortech.2014.01.100
Srifa, A., Viriya-empikul, N., Assabumrungrat, S., and Faungnawakij, K. (2015b). Catalytic behaviors of Ni/γ-Al2O3and Co/γ-Al2O3 during the hydrodeoxygenation of palm oil. Catal. Sci. Technol. 5 (7), 3693–3705. doi:10.1039/c5cy00425j
Vonortas, A., and Papayannakos, N. (2014). Comparative analysis of biodiesel versus green diesel: comparative analysis of biodiesel versus green diesel. Wiley Interdiscip. Rev. Energy Environ. 3 (1), 3–23. doi:10.1002/wene.78
Wang, X., Zhu, S., Wang, S., Wang, J., Fan, W., and Lv, Y. (2018). Ni nanoparticles entrapped in nickel phyllosilicate for selective hydrogenation of guaiacol to 2-methoxycyclohexanol. Appl. Catal. A General 568 (25), 231–241. doi:10.1016/j.apcata.2018.10.009
Keywords: nanocatalysts, transition metal oxide, palm oil, deoxygenation, green diesel
Citation: Guerrero-Corona CE, Melo-Banda JA, Lam-Maldonado M, Vega-Ibarra LA, Diaz-Zavala NP and Meraz-Melo MA (2024) Transition metal oxide nanocatalysts for the deoxygenation of palm oil to green diesel. Front. Chem. Eng. 6:1334355. doi: 10.3389/fceng.2024.1334355
Received: 07 November 2023; Accepted: 15 January 2024;
Published: 22 February 2024.
Edited by:
Jose Escobar, Mexican Institute of Petroleum, MexicoReviewed by:
María Barrera, Universidad Veracruzana, MexicoCopyright © 2024 Guerrero-Corona, Melo-Banda, Lam-Maldonado, Vega-Ibarra, Diaz-Zavala and Meraz-Melo. This is an open-access article distributed under the terms of the Creative Commons Attribution License (CC BY). The use, distribution or reproduction in other forums is permitted, provided the original author(s) and the copyright owner(s) are credited and that the original publication in this journal is cited, in accordance with accepted academic practice. No use, distribution or reproduction is permitted which does not comply with these terms.
*Correspondence: J. A. Melo-Banda, YWFyb24ubWJAY2RtYWRlcm8udGVjbm0ubXg=
Disclaimer: All claims expressed in this article are solely those of the authors and do not necessarily represent those of their affiliated organizations, or those of the publisher, the editors and the reviewers. Any product that may be evaluated in this article or claim that may be made by its manufacturer is not guaranteed or endorsed by the publisher.
Research integrity at Frontiers
Learn more about the work of our research integrity team to safeguard the quality of each article we publish.