- 1Uluu, Watermans Bay, WA, Australia
- 2Centre for Agricultural Engineering, University of Southern Queensland, Toowoomba, QLD, Australia
- 3School of Agriculture and Environment, The University of Western Australia, Perth, WA, Australia
- 4Institute of Agriculture, The University of Western Australia, Perth, WA, Australia
- 5Richgro Garden Products, Jandakot, WA, Australia
- 6Australian Centre for Water and Environmental Biotechnology, The University of Queensland, St Lucia, QLD, Australia
- 7Discipline of Engineering and Energy, Murdoch University, Murdoch, WA, Australia
Food waste (FW) costs the global economy $1 trillion annually and is associated with 8% of anthropogenic greenhouse gas emissions. Anaerobic digestion (AD) is an effective technology for recycling organic waste, including FW, for energy and nutrient recovery. Current major revenue streams for AD include the sale of biogas/power, gate fees, and digestate (fertiliser). However, subsidies provided by governments are a major profit driver for commercial facilities and are generally required for profitability, limiting its widespread adoption. Lactic acid (LA) is a high value intermediate of the AD process and literature evidence has indicated the recovery of LA can significantly boost the revenue generated from FW-AD. Moreover, FW fermentation naturally tends towards LA accumulation, promotion of LA producing bacteria, and inhibition of alternate competing microbes, making LA attractive for commercial production from FW. The integration of LA production and recovery into FW-AD could improve its economic performance and reduce the need for subsidy support, providing a platform for global adoption of the AD technology. However, challenges, such as 1) the low LA yield on FW, 2) seasonality of the FW composition, 3) unknown influence of LA recovery on downstream AD, and 4) impact of standard operational procedures for AD on upstream LA production, still exist making this focus area for future research. Even so, literature has shown the benefits of the LA-AD biorefinery, detailing improved process economics, increased FW utilisation, and elimination of subsidy support. Therefore, this review focuses on exploring the integrating LA production into AD by examining the current status of AD, LA integration strategies, challenges associated with LA production from FW, and identifies key challenges and considerations associated with downstream AD of fermented waste.
1 Introduction
Recent estimates have valued cost of managing the annual 2.5 billion tonnes of globally produced FW at nearly $230 billion (Safdie, 2023) and associated 8% of global anthropogenic greenhouse gas emissions with its production (WBA, 2018). FW encompasses a wide range of organic substrates, including dairy, fruit and vegetables, and distillery wastes produced along the whole supply chain (Kosseva, 2013), making its control a large scale complex issue. In an effort to reduce the FW production, the United Nations have set a goal to halve the production of FW per capita at the consumer and retail levels by 2030 (UN, 2015). Furthermore, the sustainable treatment of FW falls within multiple United Nations Sustainable Development Goals (UNSDGs), including the creation of sustainable production and consumption patterns, and taking action against climate change (UN, 2015). Consequently, the development of sustainable technologies for the recovery of value from this waste stream is becoming a focus point for modern research efforts.
Anaerobic digestion (AD) is a mature technology widely utilised for processing various organic wastes, including FW. In commercial applications, AD is commonly applied for processing large volumes of organic waste for the production of renewable energy (Comparetti et al., 2013). More recently, researchers have been investigating the applicability of AD on different waste streams, including lignocellulosic biomass, FW, sewage sludge, and animal manure (Carlsson et al., 2012; Mata-Alvarez et al., 2014; Sawatdeenarunat et al., 2015). In reference to the UNSDGs, the application of AD for FW recycling acts on numerous sustainability goals, including the promotion of sustainable agriculture (Goal 2), production of clean energy (Goal 6), development of sustainable cities (Goal 11), ensuring sustainable production and consumption patterns (Goal 12), and combats climate change (Goal 13). However, the low economic value of the AD outputs (i.e., biogas and digestate (the liquid effluent)) and high cost of investment (capital expenditure), management (operational expenditure), and operation limit (e.g., biogas production) limit the financial feasibility of AD to farm-scale applications unless financial incentives are provided by governments (Gebrezgabher et al., 2010; Massaro et al., 2015). Alternatively, facilities may charge gate-fees for incoming waste streams, which has been shown to improve the financial feasibility of AD (Bastidas-Oyanedel and Schmidt, 2018), however, it is unrealistic to target gate-fees as a primary revenue driver as, not only can they vary significantly (Rolewicz-Kalińska et al., 2016), but competition between biogas plants could significantly reduce or eliminate gate-fees as a revenue stream. Consequently, there has been a growing body of literature aiming to value-add to the AD technology with additional technologies to recover additional high-value products (Kim et al., 2016; Choi et al., 2017; Demichelis et al., 2017; Chen et al., 2018; Demichelis et al., 2018; López et al., 2018; Sawatdeenarunat et al., 2018).
Biorefineries, analogous to oil refineries, produce a range of fuels, chemicals, and materials, but use biomass as their source material instead of fossil fuels (Figure 1). AD has the potential to produce a variety of high value biomaterials, biochemicals, and biofuels (Moraes et al., 2014; Sawatdeenarunat et al., 2016; Bastidas-Oyanedel and Schmidt, 2018) with lactic acid (LA) being of particular interest in recent research (Kim et al., 2016; Demichelis et al., 2017; Demichelis et al., 2018; Bühlmann et al., 2021; Bühlmann et al., 2022a; Bühlmann et al., 2022b). LA is a high-value chemical utilised in a variety of industries, including the food and pharmaceutical industries, with modern applications aiming to produce biodegradable plastics (i.e., polylactic acid (PLA)) (Demichelis et al., 2017). Recent market estimates have forecast significant growth in the demand for LA over the coming years due to its applicability in multiple industries and the role it plays in the production of PLA (Biddy et al., 2016; Castro-Aguirre et al., 2016; Alves de Oliveira et al., 2018; Ranjan and Baghel, 2018).
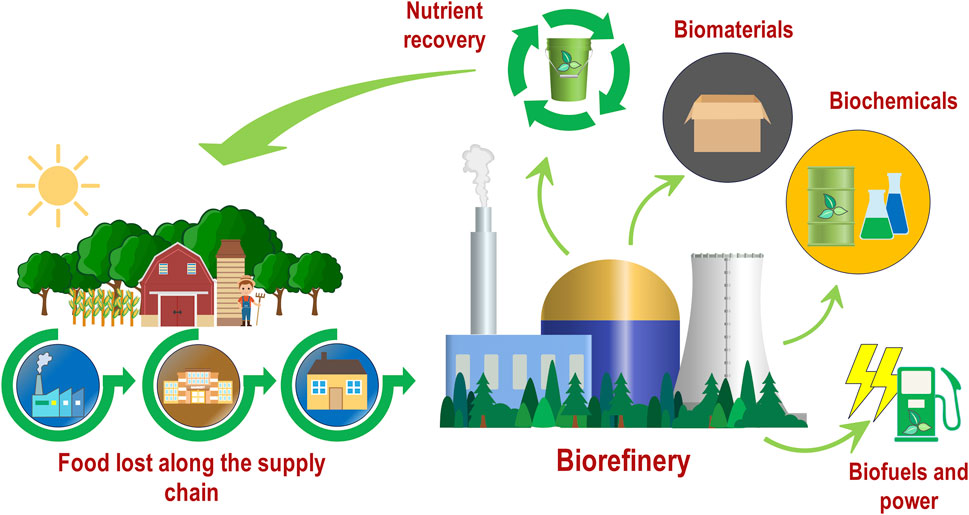
FIGURE 1. The food waste biorefinery concept utilising food waste produced throughout the supply chain for the production of valuable bio-products (Based on Mongkhonsiri et al., 2020).
A growing body of recent key literature has explored the upstream fermentation of FW for LA followed by the downstream utilisation of the fermentation residues in AD for biogas production, aiming to generate greater financial feasibility (Kim et al., 2016; Demichelis et al., 2017; Bastidas-Oyanedel and Schmidt, 2018; Bühlmann et al., 2021; Bühlmann et al., 2022a; Bühlmann et al., 2022b). The two processes can be closely integrated to synergistically improve their performance. For example, AD can provide essential power and heat required to meet the energy needs of LA fermentation and downstream separation and recovery, while the residues from LA recovery can be utilised within FW-AD for biogas production (Figure 2). Furthermore, LA fermentation can improve the economic performance of the AD technology by providing an additional revenue stream. While Bühlmann et al. (2022a) reported a reduction in biogas yields, due to a reduced loading rate to digestion when recovering LA, an overall increase in revenue was identified. In fact, literature has suggested LA can boost revenue to the point where subsidy support is not required (Bastidas-Oyanedel and Schmidt, 2018). Moreover, LA recovery provides an opportunity to increase the overall plant capacity to account for the reduced organic loading to downstream AD, potentially further increasing overall plant revenue. However, challenges including 1) low LA yield on FW, 2) seasonality of FW composition within the commercial FW context leading to variable LA yields, 3) impact of LA recovery methods on downstream AD, and 4) microbial contamination, still exist and hinder the development of future FW LA-AD biorefineries. While reviews have explored the LA fermentation (Rawoof Salma Aathika et al., 2021; Song et al., 2022) and AD separately (Srisowmeya et al., 2020), no reviews, to the authors knowledge, have explored the integration challenges between the two technologies. With this in mind, the following review explores the potential of integrating LA fermentation and recovery into FW-AD for improved profitability. The FW-AD context is examined from an industrial process perspective and the current status of LA fermentation and production is explored along with an examination of current key challenges in the LA-AD biorefinery context. Finally, important research prospects are outlined to identify critical areas that require investigation, which should lead to targeted research objectives.
2 Anaerobic digestion
AD is a complex multi-stage biological process which sequentially breaks down organic material, including a variety of high value intermediate metabolites, to its basic components (e.g., CO2 and CH4). AD has a long research history related to industrial applications, dating as far back as the 17th century when Van Helmont noted that decaying organic material produced flammable gases (Abbasi et al., 2012). Following over 300 years of development, and with the advancement of modern technology (including process control), it is possible to utilise AD for organic waste management (including FW) at large scale (Edwards et al., 2015). AD harnesses naturally occurring biological processes occurring in the absence of oxygen (i.e., anaerobic), which can be classified into four fundamental progressive stages, namely, hydrolysis, acidogenesis, acetogenesis, and methanogenesis. Each stage decomposes organic waste into progressively simpler metabolites (Melville et al., 2014; Sawatdeenarunat et al., 2015). Modern AD projects are generally developed to utilise one of two design modes (Figure 2); 1) Single-stage designs conduct all the above biological processes within a single vessel, and 2) two-stage designs aiming to split hydrolysis and acidogenesis (stage 1) from acetogenesis and methanogenesis (stage 2). In two-stage designs, stage 1 consists of a pre-fermentation vessel with a short hydraulic retention time (typically 2–3 days) prior to a much larger digester with much longer hydraulic retention time for the stage 2 digestion (typically 15–20 days) (Nabaterega et al., 2021). Several modern AD facilities incorporate two-stage designs which allow stage 1 biological processes to be separately optimised from the stage 2 biological processes, yielding improved performance and stability (Nasr et al., 2012; Aslanzadeh et al., 2014; Schievano et al., 2014).
2.1 Current status and challenges
The development of new AD projects within developed and developing countries will depend upon the local waste feedstock availability and utilisation or value of biogas energy. Small-scale AD units are the most common in developing countries such as Bangladesh, India, and Nepal, with the biogas primarily utilised for cooking purposes (Zaman and Reynolds, 2015). The residuals following digestion are typically used for the fertilisation of crop soil and as fish feed in aquaculture through pond fertilisation and increased growth of organisms (e.g., microalgae, zooplankton) which fish consume, reducing demand on fish feed (Nhu et al., 2015; Sawatdeenarunat et al., 2016). In contrast, developed countries utilise AD for processing large volumes of organic waste while utilising the biogas for the production of power or, increasingly, compressed biomethane (Zhao et al., 2010; Comparetti et al., 2013; Hakawati et al., 2017). The operation of FW AD includes many challenges, such as inhibitor accumulation (e.g., volatile fatty acids, ammonium, etc.) and process instability (Li et al., 2018). However, the cost of developing new AD facilities and utilisation of the liquid effluent from AD can be major barriers to its adoption.
2.1.1 Financial incentives
A number of significant challenges complicate development of AD projects within developed countries, such as authorisation to connect to the electrical grid (as well as monetary fees), feedstock type and availability, physical construction, plant reliability, and the removal, management and costs of the liquid by-product, digestate (Insight, 2016). These challenges are primarily related to technical feasibility or acquiring approval from regulatory authorities. However, as a single digester can cost US$ 953 per m3 of capacity (Chen et al., 2023), financing can also be a considerable challenge. AD also produces large volumes of effluent (e.g., digestate) which is low-value and can be a major cost burden to commercial facilities with transport (when used as an off-site fertiliser) and processing costs (primarily volume reduction) being substantial (O'Shea et al., 2022). Consequently, the success of commercial AD projects has heavily relied on subsidies (Gebrezgabher et al., 2010), limiting uptake of AD technology to date, because benefits and support provided by governmental policies can differ both internationally and nationally. For example, Europe is currently the pioneer of AD technology with 18,943 biogas plants in operation by the end of 2019 (EBA, 2021). However, the extensive implementation of AD around Europe is heavily driven by the financial subsidy policies provided by European governments to renewable energy producers and environmental regulations being strict but well developed, providing certainty to new projects (Linville et al., 2015; Vasco-Correa et al., 2018). In comparison, the Renewable Portfolio Standard in the US provides limited financial incentives for Anaerobic Digestion (AD). The subsidies tend to prioritize wind and solar energy over AD, which is biomass based. Moreover, these subsidies compete with the subsidies already given to fossil fuels (Edwards et al., 2015). Consequently, due to the limited financial support from governments, the financial viability of AD has been brought into question in the US, and as a result the adoption of AD technology has been significantly slower, with approximately 2,200 AD plants currently in operation (Simet and Fletcher, 2017).
Australia is an example of virtually no uptake of the AD technology, having 242 known AD projects in operation, predominantly consisting of municipal sewage sludge digesters and landfill gas facilities (Tait et al., 2021). While government programs have aided the development of some AD projects for FW and abattoir waste processing (Wong, 2017), the lack of incentives within Australia to promote the development of the technology limit its widespread adaption. However, several policies do aim to support the development of new AD installations such as the Emission Reduction Fund (ERF) and the Renewable Energy Target (RET) scheme which aim to provide an additional revenue stream for facilities by generating and selling Carbon Credit Units, and Small-Scale Technology Certificates (STC) or Large-Scale Generation Certificates (LGC), depending on the size of the AD installation (STC<100 kW < LGC) (Carlu et al., 2019). However, several barriers still harm the development of AD in Australia. ENEA, in collaboration with various Australian energy agencies and the Australian government, outlined several regulatory deficiencies which currently hinder the development of AD within Australia including; the absence of any national target for biogas production, financial uncertainties around power exports, and lack of uniform landfill levies among states (Carlu et al., 2019) which creates revenue uncertainty for new and existing AD projects.
2.1.2 Digestate
The liquid by-product produced from AD is commonly referred to as ‘digestate’ and, depending on the process and feedstock, can contain particulates in a wide range of 3.5%–13% (Plana and Noche, 2016). Generally, the solid fraction is composed of undigested material, such as lignin, while the liquid fraction contains mobile nutrients such as available nitrogen in the form of NH4-N (approx. 1,000–5,000 ppm NH4+-N) depending on the feedstock composition (Teglia et al., 2011; Akhiar, 2017; Coelho et al., 2018; Häfner et al., 2022). Depending on the feedstock composition, hydraulic retention time of the AD process, and process design (e.g., single- or two-stage), the characterisation of the solid and liquid fractions will vary (O'Connor et al., 2022).
To combat potential application of unsatisfactory digestate to land, standards, such as British standard “PAS110:2014”, which is seen as the baseline quality specification for digestate, aim to ensure digestate is safe and reliable for land application (WRAP, 2017). While there are potential for digestate to displace synthetic fertilisers, digestate is generally seen as a cost-burden due to its bulky nature, dilute nutrient content (as compared to chemical fertilisers), and costs associated with its storage, transport, and land application (Turnley et al., 2016; Mickan et al., 2022). Even so, the use of digestate within agriculture has shown its benefit, improving plant growth and producing crop yields similar to synthetic nitrogen (Ren et al., 2020), and also with field application following soil injection (Riva et al., 2016; Zilio et al., 2021). However, FW derived digestate in the agricultural context still requires significant transport costs. Recently Mickan et al. (2022) investigated digestate as a nutrient base for potting media with positive responses, in terms of plant growth, whilst reducing the aforementioned transport constraints (Mickan et al., 2022). Furthermore, researchers have explored alternative uses for digestate, such as growing microalgae (Ayre et al., 2017; Chuka-ogwude et al., 2022) and producing bioethanol (Sambusiti et al., 2016), with some researchers showing digestate could be a promising nutrient supplement and/or process water resource within the biotechnology industry to aid fermentation processes (Zhang et al., 2019; Ujor et al., 2020; Wang et al., 2021). Overall, while there are many potential use cases for digestate, no single solution is applicable in every commercial AD context, and sustained research efforts are required to continue exploring alternative end uses for AD digestate.
2.2 Anaerobic production of lactic acid
The sequential process by which AD breaks down biomass to biogas is complex and generates a variety of intermediate products, which if co-produced with biogas/power and digestate, could boost revenue from AD. LA is a promising high-value intermediate produced during the pre-fermentation stage of two-stage AD and has been the focus of a few recent studies (Kim et al., 2016; Bastidas-Oyanedel and Schmidt, 2018; Demichelis et al., 2018; Bühlmann et al., 2021; Bühlmann et al., 2022a; Bühlmann et al., 2022b; Chenebault et al., 2022). LA (C3H6O3) is a three-carbon organic acid which is generally sold as an 88 wt% solution which is expected to experience significant market growth to 1,960 kton by 2024/2025 valued at USD 9.8 billion (Alves de Oliveira et al., 2018; Presswire, 2018). The price of LA generally follows the price of starch and sugar feedstocks, and recently has reached around 3.0–4.0 USD·kg−1 (Alves de Oliveira et al., 2018). PLA (marketed as a compostable bioplastic) is anticipated to play a significant role in the expansion of the LA market with forecasts estimating, by 2025, 50% of all globally produced LA will be utilised for PLA production (Carcus, 2012; Djukić-Vuković et al., 2019), likely somewhat driven by the market for compostable plastics. The rapid growth of the LA market, high value of LA, and results of recent literature (Kim et al., 2016; Demichelis et al., 2017) suggest the development of the LA-AD biorefinery is a promising and potentially highly profitable method to improve the economic performance of AD.
Commercially, LA is primarily produced through fermentation with lactic acid bacteria (LAB) (Karp et al., 2011; Mazzoli et al., 2014; Juturu and Wu, 2016) utilising feedstocks consisting of pure sugars and nutrient-rich supplements (Hofvendahl and Hahn–Hägerdal, 2000; Abdel-Rahman et al., 2013). Both LA and feeds are commodity products, and the cost of the substrates heavily influences its market price (Reddy et al., 2016), resulting in the current production methods inflating the LA price and limiting its application for PLA production (Van Wouwe et al., 2016). Alternatively, waste feedstocks can reduce production costs by providing low-cost feedstock for LA production (Novik et al., 2017; Kwan et al., 2018). Furthermore, some waste streams, such as FW, contain nutrients that are essential for the growth of LAB and production of LA (Kim K. I. et al., 2003), reducing or potentially eliminating the need for nutrient supplements.
A large body of literature exists exploring LA fermentation and several reviews summarising the literature have been produced (Ghaffar et al., 2014; Alves de Oliveira et al., 2018; Eş et al., 2018). Fundamental research utilising waste streams for LA fermentation (Table 1) has identified that LA accumulation is promoted at low pH and short hydraulic retention time (HRT) with the separation of hydrolysis and fermentation leading to further improved productivity and yield (Demichelis et al., 2017). However, conditions that promote LA production are generally avoided in AD because LA has a much lower pKa value (3.8) compared to other organic acids like acetic (4.76), propionic (4.87), and butyric acids (4.82), and LA and its degradation product, propionic acid, can inhibit methanogenesis (Bo et al., 2007; Tang et al., 2016; Gu et al., 2018). Consequently, as research generally aims to optimise biogas production (Khan et al., 2016), it is typically identified as a metabolic by-product that needs to be avoided.
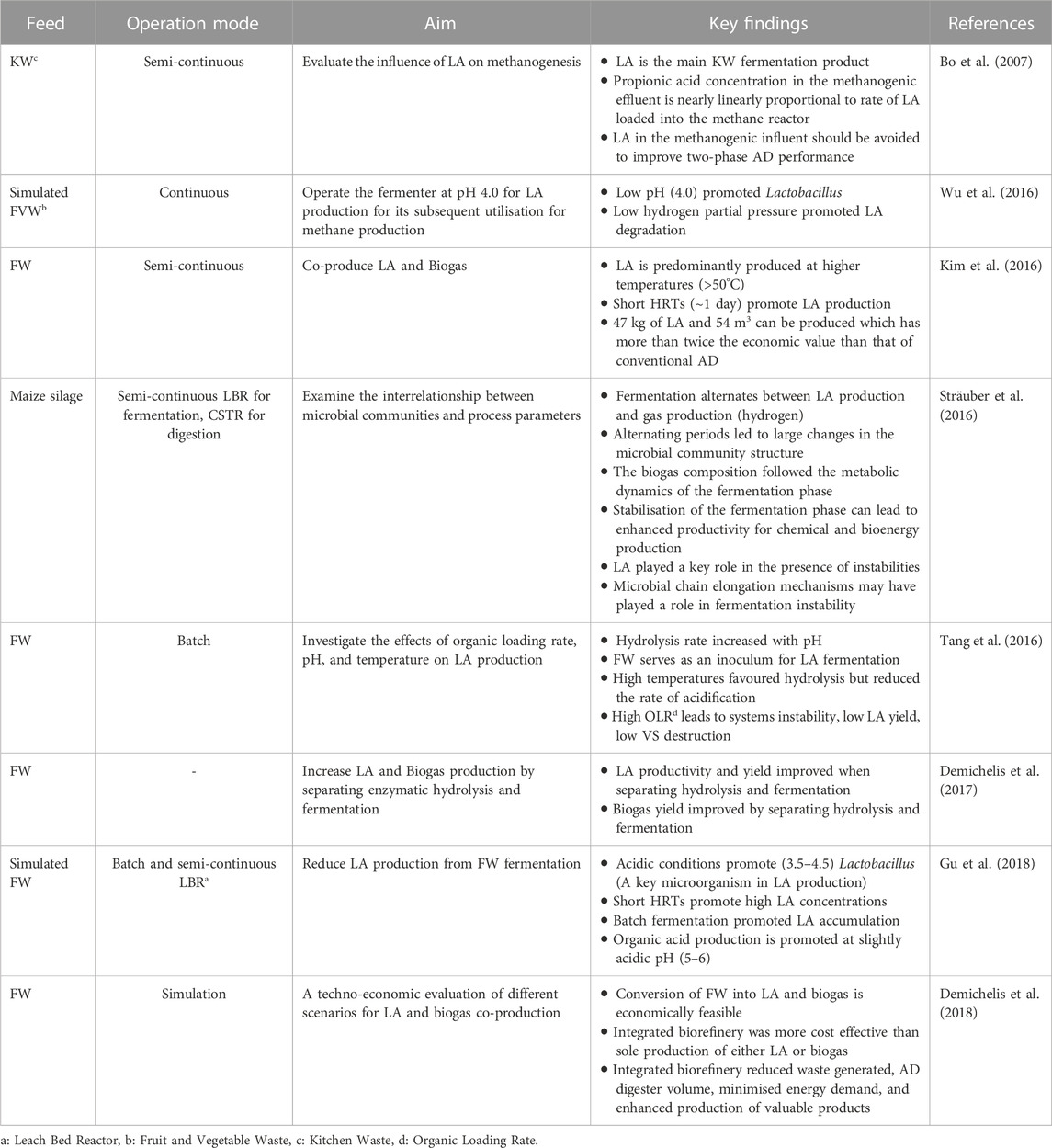
TABLE 1. Summary of key research achievements from some researchers regarding the production of LA from fermentation in two-stage AD.
While the conventional approach is to optimise AD for biogas production, recent literature has aimed to recover LA following fermentation (i.e., from the first-stage reactor) in two-stage AD processes. For example, Kim et al. (2016) and Demichelis et al. (2017) explored the recovery of LA from FW fermentation (simultaneous saccharification and fermentation) and its impacts on biogas production though subsequent AD of the solid fermentation residues. Both Kim et al. (2016) and Demichelis et al. (2017) reported a significant increase in value generated from FW processing (120%–180%), as compared to sole AD of FW, and noted the solid residues were suitable for methane production. A similar study by Bühlmann et al. (2022a) reported that, in addition to the solid fermentation residues, the liquid extraction residues following LA recovery (via ion exchange) were suitable for methane production and retained a significant (though 21% lower than raw feed) methane potential. While an overall net change in value generated from FW was not provided, the authors outlined a net 622 USD·day−1 increase in relative value (i.e., difference in estimated revenue from LA sales and loss due to reduced biogas production from LA recovery) when implementing LA recovery within a commercial FW-AD context. Overall, the findings from the available literature indicates coupling LA fermentation with subsequent extraction of LA with FW-AD can increase revenue generated from FW processing while having a minor impact on AD biogas production.
3 Integration of lactic acid fermentation into anaerobic digestion
Proper integration of biorefinery technologies for LA production and recovery with AD requires the understanding of not only the individual fermentation and biogasificiaiton processes, but also the interaction between the two processes. Control of LA fermentation and utilisation of certain separation technologies may lead to unwanted effects on AD, while operation of AD may unintentionally impact LA fermentation (depending on the integration strategy). Therefore, it is important to examine the standard operation of the two processes and develop strategies to ensure optimal performance of fermentation and AD within an LA-AD biorefinery context.
3.1 Process integration
LA fermentation is most commonly conducted with highly fermentable carbohydrates such as glucose and sucrose obtained from corn, sugarcane, and cassava commonly used commercial substrates (Olszewska-Widdrat et al., 2020). In contrast, the complex community which drives AD is capable of breaking down complex substrates for biogas production. In a FW context, FW contains many of the essential nutrients and carbon sources required for LA production (Kim et al., 2016; Tang et al., 2016; Pleissner et al., 2017), and thus is suitable for direct fermentation. Therefore, upstream fermentation for LA and downstream utilisation of fermentation residues for methane production is considered the most appropriate method to integrate the two processes. This integration approach is beneficial for two primary reasons, 1) the highly available sugars and nutrients within FW may be directly utilised for LA production while the more complex substrates are retained for AD, 2) modern AD designs incorporate a two-stage design, with an upstream fermenter installed to separate hydrolysis/acidogenesis with methane formation (Section 2.0). This provides an opportunity for LA fermentation and recovery by integrating into existing facilities through retrofitting an LA separation and recovery into the first stage of the widely adopted two stage AD design process (Figure 3).
While this is a promising integration method, it is important to understand that the commercial FW context is highly variable and has yet to be fully explored for LA production. A recent study has shown the availability of various feedstocks are dependent on complex market forces, which can impact LA yields (Bühlmann et al., 2021). However, Bühlmann et al. (2021) reported high yields for an unoptimized FW fermenter and noted a surprising stability in LA was observed, suggesting commercial LA production from complex highly variable FW is technically feasible.
3.2 Lactic acid fermentation
Many LA producing microorganisms exist, including bacteria, filamentous fungi, and yeast. However, lactic acid bacteria (LAB) are generally utilised as they are 1) Generally Regarded As Safe (GRAS), with the exception of some pathogenic streptococci strains, 2) robust organisms already adapted to the stress of industrial processes, 3) are capable of metabolising numerous mono- and di-saccharides, 4) fast growing, and 5) produce a variety of high value metabolites (Mazzoli et al., 2014; Juturu and Wu, 2016).
Many bacteria produce LA either as a primary or secondary product, but those labelled as “LAB” are exclusively grouped in the order Lactobacillales which include; Lactobacillus, Pediococcus, Carnobacterium, Aerococcus, Vagococcus, Enterococcus, Teragenococcus, Leuconostoc, Weissella, Streptococcus, Oenococcus, and Lactococcus (Juturu and Wu, 2016). Bacteria outside of this group have been utilised for LA production (Table 2), but most commercialised LAB fall into the genus Lactobacillus as they are tolerant to acidic conditions and can be easily engineered to selectively produce LA; however, other organisms applied include Streptococcus and Pediococcus (Lee, 2015; Biddy et al., 2016).
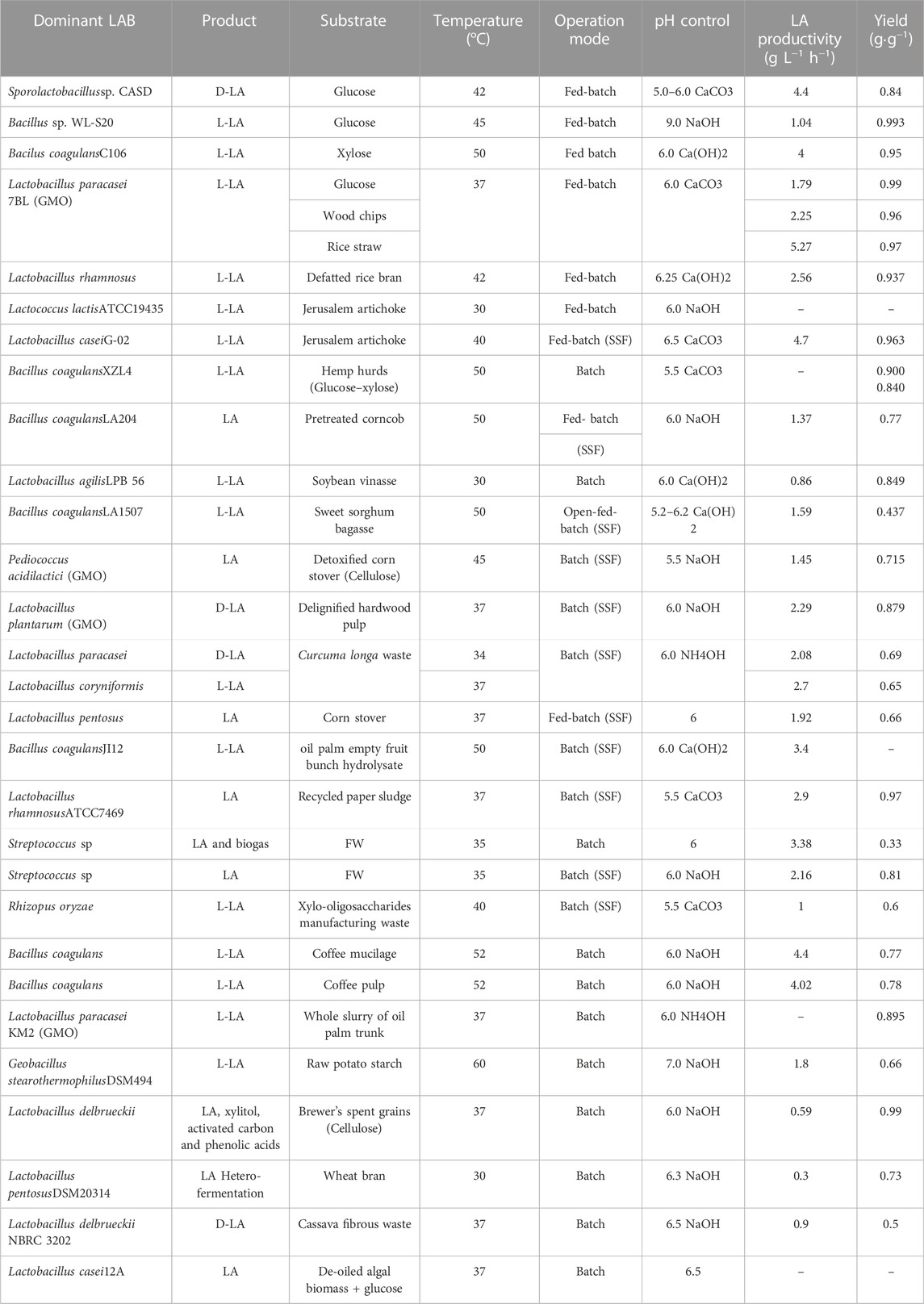
TABLE 2. Operating Conditions used for LA fermentation by some researchers (adopted from (Alves de Oliveira et al., 2018))
The optimal conditions for LA production vary depending on the microorganism utilised as LAB can grow in the pH range of 3.5–10 and temperature range of 5–45°C (Abdel-Rahman et al., 2013) and at even higher temperatures in some cases (Table 2). However, most LA fermentation processes are conducted at a pH ranging from 5.0–6.0 and at mesophilic temperatures (Table 2). For continuous processes, short hydraulic retention times (HRTs) are generally preferred as they tend to promote LA accumulation (Komemoto et al., 2009; Tang et al., 2016). However, a universal value for the optimal HRT is difficult to determine as it varies depending on various factors, especially on fermenter design and operation mode, FW composition, and operational parameters (Kim M. et al., 2003; Tang et al., 2016; Gu et al., 2018). Similarly, organic loading rates (OLRs) utilised for LA production vary (Kim et al., 2012; Tang et al., 2016; Luongo et al., 2019) depending on similar process factors, but high OLRs generally favour the production of LA and assist in limiting the conversion of LA to other organic acids (Bo & Pin-jing, 2014; Luongo et al., 2019).
The presence of a chiral carbon in LAs chemical structure can complicate fermentation as different bacteria can produce L- and D-LA (the two isomers of LA) in varying quantities depending on their production of L-lactate dehydrogenase (L-LDH) or D-LDH (Garvie, 1980; Liu, 2003; Eiteman and Ramalingam, 2015), or even depending on the use of alternative metabolic pathways such as the methylglyoxal bypass which produces a racemic LA mixture (Mazumdar et al., 2013). A mixture of L- and D-LA can be troublesome as the optical purity (OP; ratio of L-LA to the total LA present) affects its applicability, particularly if it is to be used for the synthesis of PLA (OP >98%) (Gandolfi et al., 2015) as several properties including crystallinity and thermal stability are impacted by the relative quantities of L- and D-LA within the polymer blend (Eiteman and Ramalingam, 2015). Furthermore, certain industries may require a specific isomeric form, such as the food and pharmaceutical industries which generally require L-LA as D-LA, in high dosages, can be harmful to humans (Alves de Oliveira et al., 2018). As the food industry demands the majority of the LA produced (∼85%) (Ahmad et al., 2020), L-LA is generally the target for fermentation. Studies have shown the OP can be controlled through the manipulation of environmental conditions (Gu et al., 2014; Zhang et al., 2017), supplementation of nutrients (Zhang et al., 2020b), co-fermentation (Ma et al., 2021), or utilisation of specific LAB strains (Yuan et al., 2018; Alexandri et al., 2019). However, the response of OP with changes in fermentation conditions is not consistent between studies and may be related to the differing microbial communities which form during mixed-culture fermentation, different pure bacteria cultures, or differences in dominant metabolic pathways utilised for LA.
3.2.1 Pre-treatment
Pre-treatment processes, which may be classified into biological, chemical and physical processes, are primarily designed to improve hydrolysis of complex biomass and increase the release of macromolecular substances (Yang et al., 2013; Wang et al., 2022). Many pre-treatment methods are available including extrusion, alkali pre-treatment, organosolv (an organic solvent-based treatment), and ammonia fibre expansion (AFEX) (Table 3). As the composition of wastes vary significantly, there can be no universal pre-treatment method for every feedstock. However, it has been suggested that the selected pre-treatment method should aim to, 1) avoid the need for size reduction of biomass particles, 2) preserve the hemicellulose fraction for lignocellulosic biomass, 3) reduce/remove inhibitory components and minimise their formation, 4) improve accessibility to difficult components within the biomass, 5) minimise power consumption, 6) improve the properties of the biomass surface for improved microbial interactions, 7) improve the hydrolysis rate of lipids and proteins, and 8) utilise a low cost catalyst/method for recycling of the catalyst and regeneration of lignin for co-product production (for lignocellulose) (Kumar and Sharma, 2017; Parthiba Karthikeyan et al., 2018). The majority of pre-treatment methods aim to improve the biodegradability of agricultural residues (Table 3) due to the presence of lignin, which is a major barrier to the enzymatic saccharification (Xu et al., 2016), or to aid in the breakdown of polysaccharides for microbes which may not produce the required enzymes for saccharification. However, most of the available pre-treatment methods are yet to be commercialised due to the high cost of biomass pre-treatment, and many do not meet the requirements for commercial application (Xu et al., 2016).
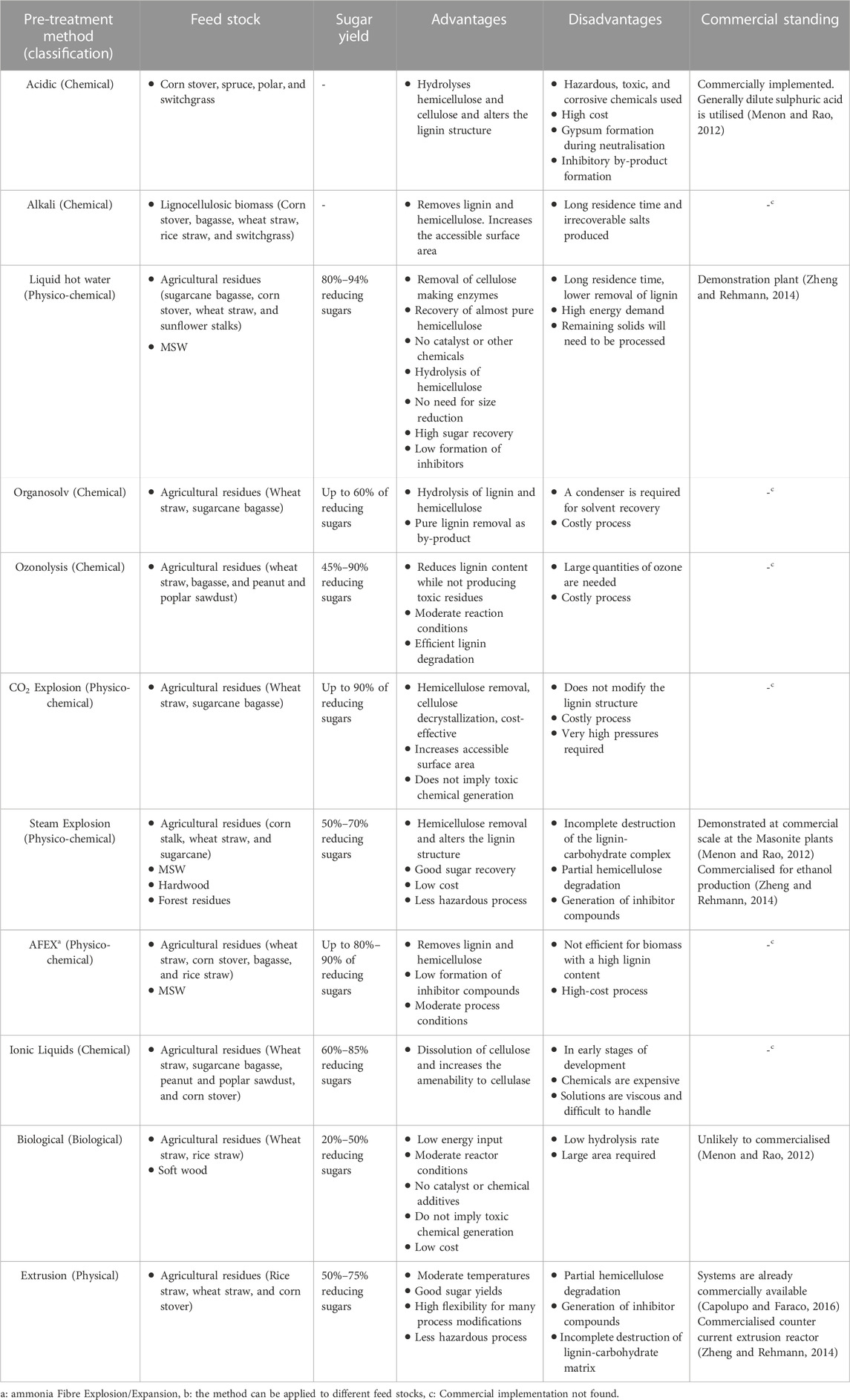
TABLE 3. Summary of some of pre-treatment methods for the treatment of various feedstocks (Modified from (Menon and Rao, 2012; Capolupo and Faraco, 2016))
As LAB struggle to ferment substrates composing of complex polymers, pre-treatment processes have shown their benefit, for example, with pulp mill residue (de Oliveira Moraes et al., 2016), corn stover (Ahring et al., 2016), and sugarcane bagasse (Wischral et al., 2019). Though FW is inherently relatively biodegradable, having been shown to produce substantial LA from direct fermentation (e.g., 0.46 gLA·gTS−1 (Tang et al., 2016), 0.18 gLA·gTS−1 (high TS content) (Yousuf et al., 2018), 0.55 gLA·gVS−1 (Bühlmann et al., 2022b), and 0.42 gLA·gVS−1 (Wang et al., 2021)), application of pre-treatment methods to FW have been shown to benefit LA fermentation by improving the final LA yield (Kim K. I. et al., 2003; Kwan et al., 2016; Pleissner et al., 2016; Demichelis et al., 2017; Ahmad et al., 2020).
3.2.2 Metabolic considerations
A number of different metabolic pathways can be utilised by bacteria for LA production, however, LAB are primarily described as utilising the glycolytic pathway (Embden–Meyerhof–Parnas pathway; Figure 4), or the phosphoketolase pathway for homo or hetero-fermentation, respectively (Wang et al., 2015). Homo-fermentative LAB produce LA as their primary end product (Papagianni, 2012; Wang et al., 2015), while hetero-fermentative LAB metabolise pentose producing equimolar amounts of LA, carbon dioxide, and ethanol or acetate (Wang et al., 2015). The specific pathway utilised by LAB is determined at the family level (Holzapfel and Wood, 2014), with crucial differences between these pathways provided by the presence of key enzymes utilised in each route; fructose 1,6-diphosphate (FDP) aldolase and phosphoketolase for the glycolytic and phosphoketolase pathways, respectively (Alves de Oliveira et al., 2018). Homo-fermentative LAB are generally preferred for LA production due to high production of LA with minimal by-products.
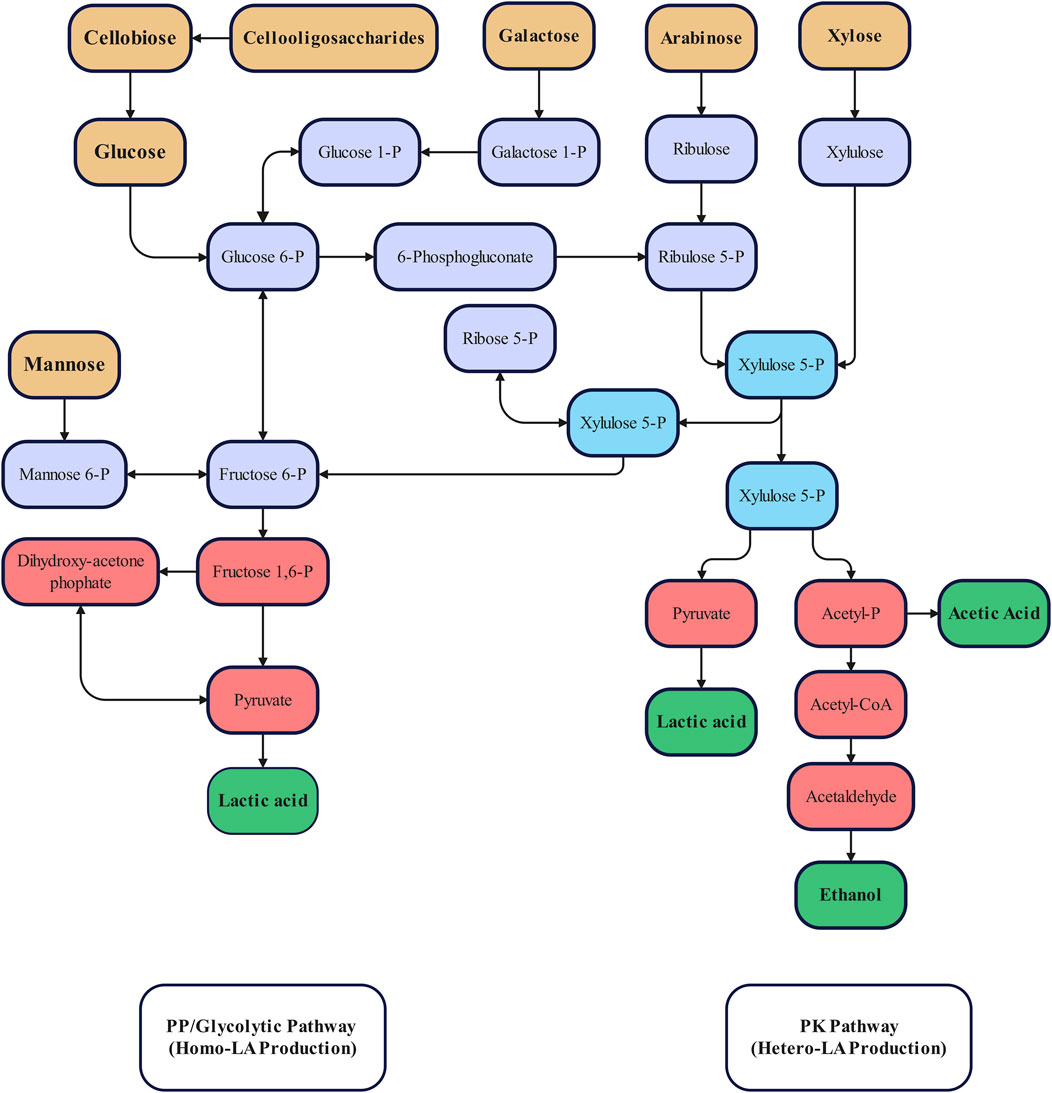
FIGURE 4. Simplified metabolic pathways for the production of lactic acid via homo- and hetero-fermentation (Modified from (Wang et al., 2015)). Colour has been used to improve readability and has not been intentionally used to separate stages within fermentation.
Some LAB resemble obligatory homo-fermentative bacteria as they possess the capability to produce FDP aldolase, however, others are also capable of synthesising phosphoketolase, allowing hexose and pentose to be utilised via the above mentioned pathways (Salminen et al., 2004; Holzapfel and Wood, 2014). These LAB are known as facultative hetero-fermentative LAB. In this case, the behaviour of these LAB (i.e., homo- or hetero-fermentation) are determined by the available carbon source or from certain environmental factors such as nutritional, osmotic, and/or thermal stress (Alves de Oliveira et al., 2018). LA production through the Embden–Meyerhof–Parnas pathway is preferable as LA is the only product, maximising its yield on biomass. However, overall community metabolic pathways for LA production are influenced by environmental conditions, operating parameters, and feed composition (Papagianni, 2012; Holzapfel and Wood, 2014). Consequently, it may be useful to monitor metabolic pathways present to identify potential undesired shifts in the pathways. A variety of molecular methods are available for monitoring metabolic pathways during LA fermentation such as shotgun metabolomics, lipidomics, metagenomics, and predictive techniques based on DNA amplicon sequencing (e.g., PICRSUt, PICRUSt2, Tax4Fun, and FaproTax). Each differs in its approach, and associated advantages and disadvantages (Table 4). In-silico gene inference techniques stand out compared to the other three methods as, instead of directly measuring components within sample, taxonomic compositions, which are inferred from amplicon sequencing, are used to predict microbial functional genes (Sun et al., 2020). The major advantage of in silico techniques is their low cost compared to others which fully sequence the genome. For example, the cost of PICRUSt analyses can be 5–15 times lower than shotgun metagenomics (Mukherjee et al., 2017). However, these techniques are limited to the genomes listed in utilised databases, which are currently highly biased towards microorganisms associated with human health and associated biotechnology (Sun et al., 2020).
The method recommended for use in an industrial setting will primarily depend on its cost and complexity. For LA fermentation, it is expected that the microbial community will have a relatively low diversity (Kim et al., 2016; Tang et al., 2016), especially compared to communities within downstream digesters (Wu et al., 2016). Consequently, in silico gene inference analysis could be used as a low-cost method to monitor the metabolic activities of the biological system which could be intermittently confirmed with more complex sequencing techniques (Table 4) to maintain optimal LA production.
3.2.3 Nutritional requirements
LAB are complex, fastidious microorganisms which require rich and complex nutrients for growth (e.g., amino acids, vitamins, carbohydrates, and minerals) with some requiring specific growth factors, such as whey, and tomato juice (Holzapfel and Wood, 2014). A variety of amino acids have been identified as essential for the growth of LAB including glutamic acid, valine, isoleucine, and leucine, which are required by nearly all LAB, while many require methionine, tryptophan, and tyrosine (Garvie, 1967; Ledesma et al., 1977). A variety of vitamins such as pantothenate, niacin and biotin and the metals, Mg2+, Mn2+, and Zn2+, are also essential for many LAB (Holzapfel and Wood, 2014), albeit, metal ions are usually only required for enzymatic reactions (Archibald, 1986). While many nutrients have been identified as essential for many LAB, specific nutrient requirements are dependent on strain (Holzapfel and Wood, 2014).
During the fermentation of refined sugars, nutrient supplements are essential for fermentation as LAB lack many biosynthetic capabilities to synthesize nutrients for their own use (Hofvendahl and Hahn–Hägerdal, 2000; Abdel-Rahman et al., 2013). It has been hypothesised these requirements resulted from the bacteria evolving in nutrient-rich media, such as meat and milk, leading them to develop without the need for the bio-processes to synthesize these nutrients (Alves de Oliveira et al., 2018). Therefore, to make these nutrients available, yeast extract, peptone, meat extract, corn steep liquor, and malt extract are commonly utilised (Yang et al., 2013; Wang et al., 2015). As mentioned previously FWs contain many of the essential nutrients required by LAB for growth. Even so, literature has shown FWs may be deficient in some essential nutrients, such as nitrogen, which could limit LA production (Zhang et al., 2020a; Zhang et al., 2020b).
It is important to note the FW classification covers a vast range of different solid and liquid substrates. Generally, liquid wastes consist of process waters with elevated concentrations of suspended solids and soluble organics (e.g., carbohydrates, proteins, and lipids), while solid wastes are generally composed of the solid fraction of various foods, such as fruit and vegetable peels, bones, skins, and seeds (Thakur et al., 2021). However, specific waste streams, including meats, fruit and vegetables, and distillery wastes (Table 1 and 2), all of which may be further categorised into diverse waste streams, can vary substantially in composition (Kosseva, 2013). Consequently, selection of specific FW substrates to maximise LA yield and productivity can be challenging. Targeted selection of certain FW substrates for co-fermentation, such as dairy wastes, could be highly beneficial in this regard. Dairy wastes are known to be natural environments for LAB (Sar et al., 2022) and have been shown to have the potential to yield high LA concentrations (Abedi and Hashemi, 2020), suggesting dairy, or similar wastes, could form beneficial co-substrates for FW fermentation. This approach allows co-fermented substrates to provide essential nutrients which other co-substrates could be lacking, improving LA production.
Within an integrated LA-AD biorefinery, alternate nutrient supplementation approaches could be available. While literature has demonstrated the nutritional benefits of FW digestate in agriculture (Dutta et al., 2021; Mickan et al., 2022), recent research has identified LA fermentation may benefit from these nutrients present within digestate. Due to the nature of the FW substrate, FW digestate naturally contains elevated ammonium concentrations (Banks et al., 2011; Serna-Maza et al., 2015; Buhlmann et al., 2018), which may be suitable for LA fermentation. Limited available research has shown the benefits of digestate on FW fermentation, improving pH stability, increasing microbial diversity, and maintaining a low oxidation reduction potential (Wang et al., 2021), and has even been shown to be suitable as process water following pre-treatment (Zhang et al., 2019). Implementing partial digestate recirculation to LA fermentation within an LA-AD biorefinery may be an effective way to promote LA production and provide an alternative on-site use for the low-value digestate. However, additional research is required to understand the impact digestate may have on LA fermentation, the product spectrum, microbial community, and degradation pathways for LA production.
4 Integration challenges/considerations
While integrating LA production into AD has been suggested to improve the overall process economics (Section 2.2), care should be taken to ensure optimal operation of both processes. For this, specific feed requirements and operational parameters and chemical/nutrient supplements should be considered, emphasising their possible impact on downstream processes. Furthermore, special care should be taken when selecting the LA recovery method to ensure the usability of fermentation waste in AD.
4.1 Challenges using FW as a substrate for fermentation
While approximately 90% of worldwide LA production is achieved through fermentation (Karp et al., 2011), these processes primarily utilise food based feedstocks (Section 3.1) which are not only influenced by seasonal variability, but high cost coupled with the high cost of nutrient supplements increases the price of the LA production. In contrast, the versatile AD technology is capable of processing a variety of wastes including FW, cardboard, grease trap residues, and fat oils (Edwards et al., 2015). This capacity to receive various waste streams provides a buffer from feedstock supply uncertainties and seasonal changes in waste availability, though depending on location, surrounding industries, climate, season, population density and socio-demographics, and government policies such as landfill waste diversion, available feedstocks are expected to somewhat vary (Ghatak, 2011; Bühlmann et al., 2021). Utilisation of FW feedstocks for LA fermentation can reduces costs associated with acquiring feedstocks, however, fermentation of complex substrates, availability of mixed sugars, and introduction of alternate microbes from the complex FW feedstock may impact LA production.
4.1.1 Feedstock composition, and potential pre-treatment requirements
The maximum yield achieved and feedstock cost heavily influences the economics of fermentation (Manandhar and Shah, 2020). Even though waste streams can reduce operational costs associated with obtaining feedstocks, yields are still a challenge. Fermentation of waste to LA is primarily limited by the available carbohydrate fraction within the substrate. While carbohydrates make up a significant fraction of FW (Demichelis et al., 2017), LAB struggle to fully utilise the substrate. Pre-treatment of the FW via enzymatic, fungal, acidic, or alkali pre-treatments can effectively improve the LA yield on FWs (Kim K. I. et al., 2003; Kwan et al., 2016; Pleissner et al., 2016; Demichelis et al., 2017; Ahmad et al., 2020), but are costly and generally produce large quantities of solid and liquid wastes which require further treatment prior to disposal (Surendra et al., 2015). A diverse community adapted for LA accumulation could be beneficial for FW fermentation, allowing the increased utilisation of the diverse FW substrate from the presence of diverse hydrolytic bacteria. Moreover, utilisation of a functionally redundant community could improve process stability to changes in feedstock composition. However, while studies have explored the community structure during LA fermentation (Bühlmann et al., 2021; Wang et al., 2021) the functional stability of these cultures have not been fully explored.
Some wastes, such as lignocellulose (following pre-treatment), can release mixed sugars which may lead to carbon catabolite repression (CCR) (Abdel-Rahman and Sonomoto, 2016). The presence of a variety of different carbon sources may complicate fermentation as some bacteria limit the utilisation of secondary carbon sources when a primary source is present (i.e., CCR), which is a problem for most microbial producers (Görke and Stülke, 2008; Abdel-Rahman and Sonomoto, 2016). For example, Escherichia coli prefer glucose over lactose as a carbon source while Streptococcus thermophiles prefer lactose over glucose (Brückner and Titgemeyer, 2002). These bacteria will metabolise their preferred substrate before utilising the secondary source. Mixed sugar fermentation could result in increased costs associated with separation and purification stages (Wang et al., 2015) due to lower LA yields and increased by-product formation. This behaviour can be problematic when fermenting substrates containing various carbon sources as certain sugars may require the utilisation of hetero-fermentative pathways for LA production (Figure 4).
4.1.2 Microbial contamination
Microbial contamination is also a significant risk which can complicate microbial LA production, especially when utilising waste feedstocks, but is not widely explored for LA fermentation. AD facilities receive and utilise a variety of waste feed stocks which are primarily unsterilised. Though this does not pose a problem for AD, it can be a significant risk to the economics of LA fermentation. Many fermentation systems with optimised process conditions (e.g., pH, temperature, HRT, and OLR) tend to selectively promote LAB and LA production (Kim et al., 2016; Tang et al., 2016; Bühlmann et al., 2022b). However, FWs contain a variety of alternate bacteria, and are naturally enriched with many LAB (Kim et al., 2016), which may compete with target strains for substrate, reducing LA yields and selectivity. Pasteurisation is generally applied to eliminate the risk of microbial contamination, however, due to its high energy demands (Bolzonella et al., 2018; Liu et al., 2019), many studies aim to utilise FW without pasteurisation (Kim et al., 2016; Tang et al., 2016; Feng et al., 2018; Zhang et al., 2020a; Zhang et al., 2020b). An LA-AD biorefinery could hold a distinct advantage in this regard, producing large quantities of waste heat generated from biogas combustion which could be utilised for upstream pasteurisation. Future LA-AD biorefineries will likely utilise pasteurisation or finely tuned operational conditions to selectively promote the growth of target strains to ensure consistent LA production from fermentation.
4.2 Separation and recovery considerations
The viability of biologically derived products is heavily dictated by the cost of downstream processes required to isolate the target compound (Saboe et al., 2018). However, the separation and recovery of LA is difficult due to its low vapour pressure, high affinity to water, and tendency to undergo self-esterification (Sun et al., 2006). Consequently, separation and final purification can represent up to 50% of the production costs (Komesu et al., 2017a).
Traditionally, LA is recovered via gypsum precipitation and esterification, for the commercial production of high purity LA (Figure 5) (Lee, 2015; Komesu et al., 2017a). LA generally exists as a salt in the fermentation broth due to the addition of neutralising agents (CaCO3, Ca(OH)2, NaOH, NH3) to maintain optimal pH (5–7) (normally CaCO3, Ca(OH)2) (Lee, 2015; Komesu et al., 2017b; Alves de Oliveira et al., 2018). Following fermentation, the broth may be adjusted to a pH of 10 and heated to 80 oC in order to solubilise the calcium lactate and coagulate proteins within the broth to simplify filtration (Lee, 2015). The broth is then filtered and re-acidified with sulphuric acid to produce LA and precipitate gypsum. The resultant mixture is then filtered, producing a technical grade LA mixture (22%–44%) (Komesu et al., 2017a). This product can be further refined to produce high purity LA, through esterification with methanol, distillation, and hydrolysis (Figure 5).
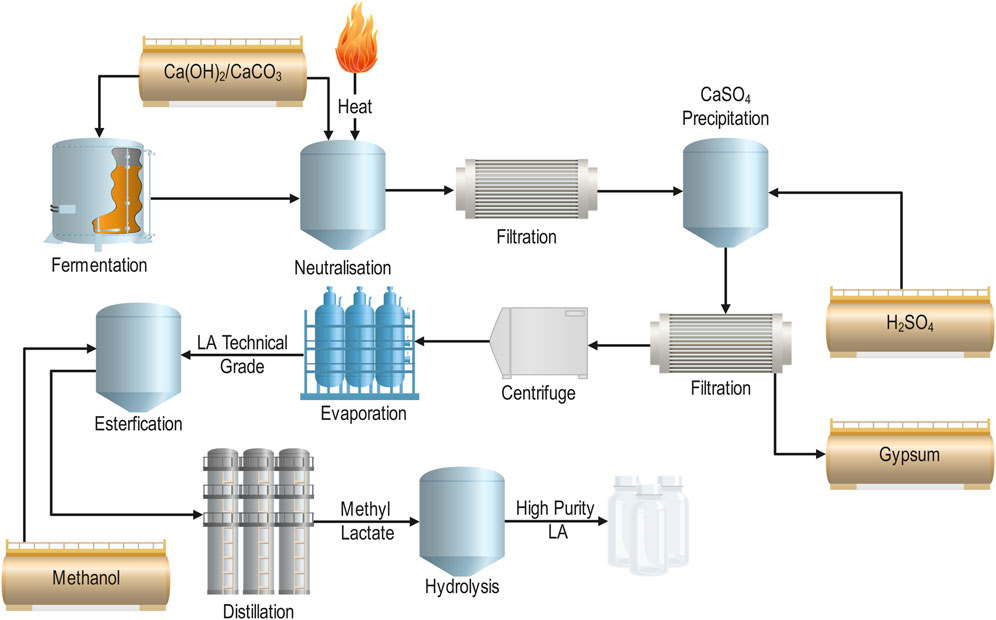
FIGURE 5. Conventional LA separation and purification processes (Modified from (Lee, 2015; Komesu et al., 2017a)).
While this recovery process is effective and a proven technology, it is costly, produces large quantities of gypsum waste, and requires large volumes of sulphuric acid (Komesu et al., 2017c; Jantasee et al., 2017; Alves de Oliveira et al., 2018; Singhvi et al., 2018). Consequently, research has been focused on developing and testing alternate separation and purification technologies for LA including; distillation, solvent extraction, adsorption, and membrane separation processes (reverse osmosis, ultrafiltration, and electrodialysis) (Komesu et al., 2017a). Ideally, the separation process selected for LA recovery should be based on the efficient and economical usage of these processes, along with a consideration of their individual advantages and disadvantages (Table 5) (Wasewar, 2005).
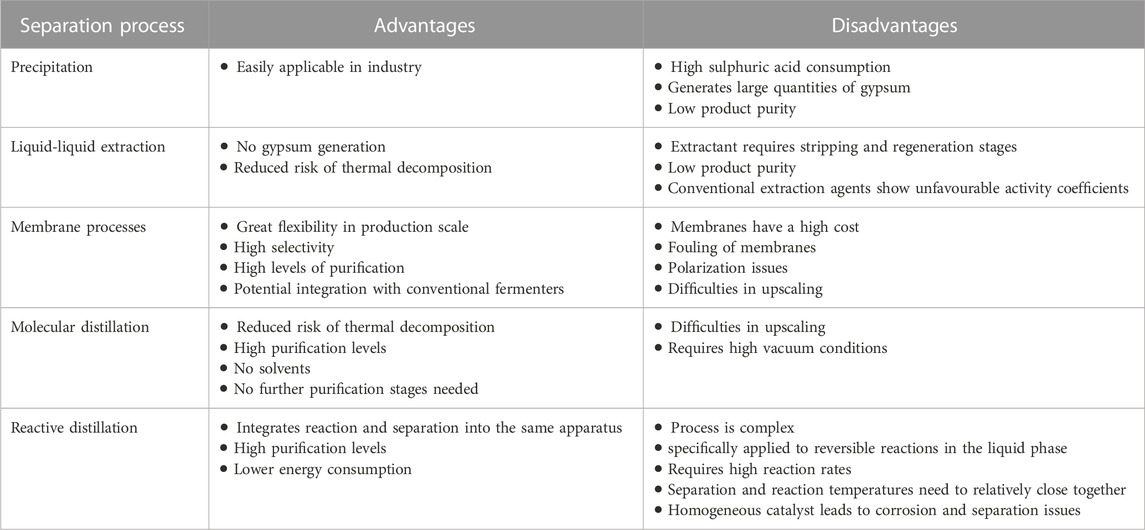
TABLE 5. Advantages and disadvantages of separation processes for the recovery of lactic acid (Adopted from (Komesu et al., 2017b)).
4.2.1 Advances in separation technologies
The current commercial method for LA separation and purification has many economic and environmental ramifications when obtaining pure LA. Consequently, alternative methods for the recovery and purification of LA have been the focus of recent literature (Table 5). Recovery techniques which can be utilised in situ show promise in reducing environmental impacts and reagent addition associated with fermentation and LA recovery. These technologies couple fermentation with separation for the continuous production and extraction of LA. A variety of technologies exist, each with its own associated advantages and disadvantages (Table 6). Two promising in situ techniques include liquid-liquid extraction and ion exchange due to their high efficiency and selectivity in recovering LA from fermentation broth.
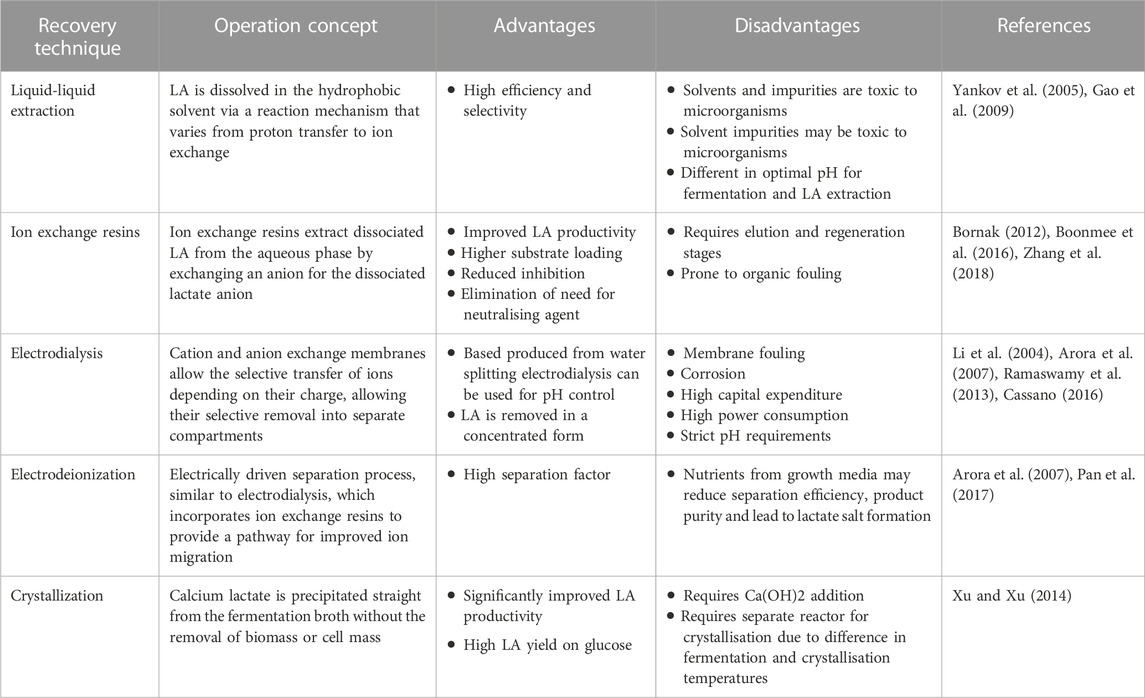
TABLE 6. Summary of advantages and disadvantages of different in situ recovery techniques (Modified from (Van Hecke et al., 2014))
4.2.2 Liquid–liquid extraction
In-situ liquid-liquid extraction is a promising method for LA recovery. A variety of solvents can be utilised including, water insoluble amines, quaternary ammonium salts, esters, or ketones. Due their high selectivity and efficiency of tertiary amines, as well as their poor solubility in the aqueous phase, they are also appropriate for LA extraction (Jantasee et al., 2017). A variety of tertiary amines have been tested utilising a number of different alcohols as diluents but trioctylamine in 1-octanol remains the extractant-diluent combination that provides the highest LA distribution (Krzyżaniak et al., 2013). However, it is important to note that these solvents are toxic to microorganisms (Singhvi et al., 2018), which is closely related to the hydrophobicity of the solvent, although the range of tolerable concentrations is depended by the type of microorganism (Matsumoto et al., 2004). Optimisation of the solvent concentration can reduce this toxic effect as shown by Gao et al. (2009).
4.2.3 Adsorption
Adsorption is another promising recovery technique and is widely used within industrial biotechnology as it is robust and relatively easy to operate (da Silva and Miranda, 2013). Various resins have been applied for LA recovery (Table 7) but weakly basic resins are generally preferred as they do not require powerful regeneration steps and have a much higher resistance to organic fouling compared to strongly basic resins (Tung and Judson King, 1994; Gluszcz et al., 2004). Several reports have explored anionic resins for LA recovery and notably Boonmee et al. (2016) and Ataei and Vasheghani-Farahani (2008) reported a 2.1–6.8 and 5 fold increase in LA productivity, respectively, when applying anion exchange to LA fermentation. Furthermore, Zhang et al. (2018) identified the cost to apply in situ anion exchange for LA recovery was similar to that of CaCO3 for pH control. Therefore, application of ion exchange for LA recovery can enhance the production rate of LA, allowing reduced process vessel volumes and capital costs, while simultaneously reducing the need for neutralising agents following fermentation.
4.2.4 Recovery method considerations
While a variety of LA recovery techniques have been explored in literature (Table 6), they are predominantly concerned with the recovery of LA and tend not to consider implications for downstream processes. Within an integrated LA-AD biorefinery, this would not only concern further purification stages, but also downstream AD which would likely utilise the extraction residues within AD for disposal and methane generation. This would likely include the solid and liquid fraction fractions. For this, the recovery method should be carefully considered to ensure LA is selectively extracted, not only to reduce downstream costs, but also to maximise the remaining organic fraction within the residues to maximise biogas production within AD. Furthermore, the extraction method should minimise the broths exposure to toxic or inhibitory compounds which may follow the extraction residues to downstream AD and inhibit methane formation or restrict digestate use within agriculture. For example, while liquid-liquid extraction processes can be effective for LA recovery (Section 4.2.2), a portion of the solvent will remain within the extracted broth and accumulate within AD. Depending on the microbial tolerances and the section of the community impacted, the retained solvent could impact AD process performance and stability, and impact the quality the digestate produced.
While only a handful of literature has examined the feasibility of utilising LA fermentation waste within AD (Dreschke et al., 2015; Kim et al., 2016; Demichelis et al., 2017; Bühlmann et al., 2022a), the results are promising. For example, Demichelis et al. (2017) outlined the solid fermentation residues were appropriate for methane formation, while, a similar study by Bühlmann et al. (2022a) reported the liquid extraction residues, following LA recovery via ion exchange, were also suitable for use within AD along with the solid fraction. Overall, AD can effectively utilise extraction residues from LA recovery, however, future work should explore the impacts of alternative recovery methods on the liquid fraction aiming to minimise downstream impacts to AD.
5 Concluding remarks and recommendations
Overall, the current literature review identified challenges with integrating LA production into commercial FW-AD; however, it was clear that LA production from waste streams could boost the economic performance of two-stage AD systems, providing a high-value by-product and better harnessing existing capital. FW, being composed of the primary carbohydrates and nutrients required for LA production, is a promising substrate for LA production with recent literature having shown solid FW fermentation residues and liquid extraction residues can be utilised within existing AD systems engineered for methane production. While these reports are promising for the LA-AD biorefinery, the commercial FW context is highly variable and complex, and has yet to be fully explored, particularly in areas related to waste availability, its diverse and varying composition, and its potential impact on LA fermentation and recovery. Furthermore, literature has yet to fully explore the integration into existing two-stage AD infrastructure. For this, the pre-fermenter would be converted to an LA fermenter which, to minimise start-up and operational costs, should be regulated through the control levers of pH and temperature. Moreover, different operational modes and reactor designs should be explored to elucidate their effects on LA-AD biorefinery concept. A pilot scale study would be highly beneficial in this regard, providing a platform for the study of LA production from diverse, complex, and variable FW, study stability of LA producing communities, how LA recovery impacts the BMP of the fermented FW, while also providing an opportunity for unknown process influences to be studied.
Limited literature has shown an industrial inoculum can be directed to target LA, however, further research is required to identify methods to redirect commercial fermentation to target and maximise LA. Furthermore, studies should explore community functional stability and explore methods to ensure consistent LA production. Such a study could be conducted on a continuous fermenter fermenting real diverse FW, while monitoring community composition, function, and LA production. Although literature has identified the solid and liquid residues are suitable for methane production, it is unclear how different recovery methods may impact the liquid fraction and resulting AD. Therefore, to continue the development of the LA-AD biorefinery, it is necessary to explore the above-mentioned research areas while aiming to maximise LA production and minimise the negative impacts imposed on downstream AD.
Author contributions
CB: Conceptualization, Writing–original draft, Writing–review and editing, Investigation, Project administration, Visualization. BM: Funding acquisition, Supervision, Writing–review and editing. ST: Supervision, Writing–review and editing. DB: Writing–review and editing. PB: Funding acquisition, Supervision, Writing–review and editing.
Funding
The author(s) declare financial support was received for the research, authorship, and/or publication of this article. Richgro Garden Products.
Acknowledgments
Tim and Geoff Richards from Richgro are greatly thanked for funding this research. A portion of this work was completed while author CB held a Research Training Program stipend and fee offset and top-up scholarship from Murdoch University, WA.
Conflict of interest
The authors declare that the research was conducted in the absence of any commercial or financial relationships that could be construed as a potential conflict of interest.
Publisher’s note
All claims expressed in this article are solely those of the authors and do not necessarily represent those of their affiliated organizations, or those of the publisher, the editors and the reviewers. Any product that may be evaluated in this article, or claim that may be made by its manufacturer, is not guaranteed or endorsed by the publisher.
Abbreviations
AD, anaerobic digestion; BMP, biochemical methane potential; CCR, carbon catabolite repression; FDP, fructose 1,6-DiPhosphate; FVW, fruit and vegetable waste; FW, food waste; GRAS, generally regarded as safe; KW, kitchen waste; LA, lactic acid; LAB, lactic acid bacteria; LDH, lactate DeHydrogenase; OLR, organic loading rate; OP, optical purity; PICRUSt, phylogenetic investigation of communities by reconstruction of unobserved states; PLA, poly-lactic Acid; TS, total solids; VS, volatile solids; wt, weight.
References
Abbasi, T., Tauseef, S. M., and Abbasi, S. A. (2012). Anaerobic digestion for global warming control and energy generation—an overview. Renew. Sustain. Energy Rev. 16 (5), 3228–3242. doi:10.1016/j.rser.2012.02.046
Abdel-Rahman, M. A., and Sonomoto, K. (2016). Opportunities to overcome the current limitations and challenges for efficient microbial production of optically pure lactic acid. J. Biotechnol. 236, 176–192. doi:10.1016/j.jbiotec.2016.08.008
Abdel-Rahman, M. A., Tashiro, Y., and Sonomoto, K. (2013). Recent advances in lactic acid production by microbial fermentation processes. Biotechnol. Adv. 31 (6), 877–902. doi:10.1016/j.biotechadv.2013.04.002
Abedi, E., and Hashemi, S. M. B. (2020). Lactic acid production - producing microorganisms and substrates sources-state of art. Heliyon 6 (10), e04974. doi:10.1016/j.heliyon.2020.e04974
Ahmad, A., Banat, F., and Taher, H. (2020). A review on the lactic acid fermentation from low-cost renewable materials: recent developments and challenges. Environ. Technol. Innovation 20, 101138. doi:10.1016/j.eti.2020.101138
Ahring, B. K., Traverso, J. J., Murali, N., and Srinivas, K. (2016). Continuous fermentation of clarified corn stover hydrolysate for the production of lactic acid at high yield and productivity. Biochem. Eng. J. 109, 162–169. doi:10.1016/j.bej.2016.01.012
Akhiar, A. (2017). Characterization of liquid fraction of digestates after solid-liquid separation from anaerobic co-digestion plants. Université Montpellier.
Alexandri, M., Neu, A. K., Schneider, R., López-Gómez, J. P., and Venus, J. (2019). Evaluation of various Bacillus coagulans isolates for the production of high purity L-lactic acid using defatted rice bran hydrolysates. Int. J. food Sci. Technol. 54 (4), 1321–1329. doi:10.1111/ijfs.14086
Alonso, A., Marsal, S., and Julià, A. (2015). Analytical methods in untargeted metabolomics: state of the art in 2015. Front. Bioeng. Biotechnol. 3, 23. doi:10.3389/fbioe.2015.00023
Alves de Oliveira, R., Komesu, A., Vaz Rossell, C. E., and Maciel Filho, R. (2018). Challenges and opportunities in lactic acid bioprocess design—from economic to production aspects. Biochem. Eng. J. 133, 219–239. doi:10.1016/j.bej.2018.03.003
Archibald, F. (1986). Manganese: its acquisition by and function in the lactic acid bacteria. CRC Crit. Rev. Microbiol. 13 (1), 63–109. doi:10.3109/10408418609108735
Arora, M. B., Hestekin, J. A., Snyder, S. W., Martin, E. J., Lin, Y. J., Donnelly, M. I., et al. (2007). The separative bioreactor: a continuous separation process for the simultaneous production and direct capture of organic acids. Sep. Sci. Technol. 42 (11), 2519–2538. doi:10.1080/01496390701477238
Aslanzadeh, S., Rajendran, K., and Taherzadeh, M. J. (2014). A comparative study between single- and two-stage anaerobic digestion processes: effects of organic loading rate and hydraulic retention time. Int. Biodeterior. Biodegrad. 95, 181–188. doi:10.1016/j.ibiod.2014.06.008
Ataei, S. A., and Vasheghani-Farahani, E. (2008). In situ separation of lactic acid from fermentation broth using ion exchange resins. J. Industrial Microbiol. Biotechnol. 35 (11), 1229–1233. doi:10.1007/s10295-008-0418-6
Ayre, J. M., Moheimani, N. R., and Borowitzka, M. A. (2017). Growth of microalgae on undiluted anaerobic digestate of piggery effluent with high ammonium concentrations. Algal Res. 24, 218–226. doi:10.1016/j.algal.2017.03.023
Banks, C. J., Chesshire, M., Heaven, S., and Arnold, R. (2011). Anaerobic digestion of source-segregated domestic food waste: performance assessment by mass and energy balance. Bioresour. Technol. 102 (2), 612–620. doi:10.1016/j.biortech.2010.08.005
Bastidas-Oyanedel, J.-R., and Schmidt, J. (2018). Increasing profits in food waste biorefinery—a techno-economic analysis. Energies 11 (6), 1551. doi:10.3390/en11061551
Biddy, M. J., Scarlata, C., and Kinchin, C. (2016). Chemicals from biomass: a market assessment of bioproducts with near-term potential. Golden, CO (United States): National Renewable Energy Lab. NREL/TP-5100-65509 United States 10.2172/1244312 NREL English.
Bo, Z., and Pin-jing, H. (2014). Performance assessment of two-stage anaerobic digestion of kitchen wastes. Environ. Technol. 35 (10), 1277–1285. doi:10.1080/09593330.2013.866169
Bo, Z., Wei-min, C. A. I., and Pin-jing, H. E. (2007). Influence of lactic acid on the two-phase anaerobic digestion of kitchen wastes. J. Environ. Sci. 19 (2), 244–249. doi:10.1016/s1001-0742(07)60040-0
Bolzonella, D., Fatone, F., Gottardo, M., and Frison, N. (2018). Nutrients recovery from anaerobic digestate of agro-waste: techno-economic assessment of full scale applications. J. Environ. Manag. 216, 111–119. doi:10.1016/j.jenvman.2017.08.026
Boonmee, M., Cotano, O., Amnuaypanich, S., and Grisadanurak, N. (2016). Improved lactic acid production by in situ removal of lactic acid during fermentation and a proposed scheme for its recovery. Arabian J. Sci. Eng. 41 (6), 2067–2075. doi:10.1007/s13369-015-1824-5
Brückner, R., and Titgemeyer, F. (2002). Carbon catabolite repression in bacteria: choice of the carbon source and autoregulatory limitation of sugar utilization. FEMS Microbiol. Lett. 209 (2), 141–148. doi:10.1111/j.1574-6968.2002.tb11123.x
Buhlmann, C. H., Mickan, B. S., Jenkins, S. N., Tait, S., Kahandawala, T. K. A., and Bahri, P. A. (2018). Ammonia stress on a resilient mesophilic anaerobic inoculum: methane production, microbial community, and putative metabolic pathways. Bioresour. Technol. 275, 70–77. doi:10.1016/j.biortech.2018.12.012
Bühlmann, C. H., Mickan, B. S., Tait, S., and Bahri, P. A. (2022a). Developing a food waste biorefinery: lactic acid extraction using anionic resin and impacts on downstream biogas production. Chem. Eng. J. 431, 133243. doi:10.1016/j.cej.2021.133243
Bühlmann, C. H., Mickan, B. S., Tait, S., Batstone, D. J., Mercer, G. D., and Bahri, P. A. (2022b). Lactic acid from mixed food waste fermentation using an adapted inoculum: influence of pH and temperature regulation on yield and product spectrum. J. Clean. Prod. 373, 133716. doi:10.1016/j.jclepro.2022.133716
Bühlmann, C. H., Mickan, B. S., Tait, S., Renton, M., and Bahri, P. A. (2021). Lactic acid from mixed food wastes at a commercial biogas facility: effect of feedstock and process conditions. J. Clean. Prod. 284, 125243. doi:10.1016/j.jclepro.2020.125243
Capolupo, L., and Faraco, V. (2016). Green methods of lignocellulose pretreatment for biorefinery development. Appl. Microbiol. Biotechnol. 100 (22), 9451–9467. doi:10.1007/s00253-016-7884-y
Carcus, M. (2012). “Growth in PLA bioplastics: a production capacity of over 800,000 tonnes expected by 2020,” in Bio-based news.
Carlsson, M., Lagerkvist, A., and Morgan-Sagastume, F. (2012). The effects of substrate pre-treatment on anaerobic digestion systems: a review. Waste Manag. 32 (9), 1634–1650. doi:10.1016/j.wasman.2012.04.016
Cassano, A. (2016). “Integrated membrane processes in the food industry,” in Integrated membrane systems and processes.
Castro-Aguirre, E., Iñiguez-Franco, F., Samsudin, H., Fang, X., and Auras, R. (2016). Poly(lactic acid)—mass production, processing, industrial applications, and end of life. Adv. Drug Deliv. Rev. 107, 333–366. doi:10.1016/j.addr.2016.03.010
Chatham, J. C., and Blackband, S. J. (2001). Nuclear magnetic resonance spectroscopy and imaging in animal research. ILAR J. 42 (3), 189–208. doi:10.1093/ilar.42.3.189
Chen, Y., Pinegar, L., Immonen, J., and Powell, K. M. (2023). Conversion of food waste to renewable energy: a techno-economic and environmental assessment. J. Clean. Prod. 385, 135741. doi:10.1016/j.jclepro.2022.135741
Chen, Y.-d., Ho, S.-H., Nagarajan, D., Ren, N.-q., and Chang, J.-S. (2018). Waste biorefineries — integrating anaerobic digestion and microalgae cultivation for bioenergy production. Curr. Opin. Biotechnol. 50, 101–110. doi:10.1016/j.copbio.2017.11.017
Chenebault, C., Moscoviz, R., Trably, E., Escudié, R., and Percheron, B. (2022). Lactic acid production from food waste using a microbial consortium: focus on key parameters for process upscaling and fermentation residues valorization. Bioresour. Technol. 354, 127230. doi:10.1016/j.biortech.2022.127230
Choi, O. K., Lee, K., Park, K. Y., Kim, J.-K., and Lee, J. W. (2017). Pre-recovery of fatty acid methyl ester (FAME) and anaerobic digestion as a biorefinery route to valorizing waste activated sludge. Renew. Energy 108, 548–554. doi:10.1016/j.renene.2017.03.004
Chuka-ogwude, D., Mickan, B. S., Ogbonna, J. C., and Moheimani, N. R. (2022). Developing food waste biorefinery: using optimized inclined thin layer pond to overcome constraints of microalgal biomass production on food waste digestate. J. Appl. Phycol. 34 (6), 2917–2928. doi:10.1007/s10811-022-02829-5
Coelho, J. J., Prieto, M. L., Dowling, S., Hennessy, A., Casey, I., Woodcock, T., et al. (2018). Physical-chemical traits, phytotoxicity and pathogen detection in liquid anaerobic digestates. Waste Manag. 78, 8–15. doi:10.1016/j.wasman.2018.05.017
Comparetti, A., Febo, P., Greco, C., and Orlando, S. (2013). Current state and future of biogas and digestate production.
da Silva, A. H., and Miranda, E. A. (2013). Adsorption/desorption of organic acids onto different adsorbents for their recovery from fermentation broths. J. Chem. Eng. Data 58 (6), 1454–1463. doi:10.1021/je3008759
De Filippis, F., Parente, E., and Ercolini, D. (2017). Metagenomics insights into food fermentations. Microb. Biotechnol. 10 (1), 91–102. doi:10.1111/1751-7915.12421
Demichelis, F., Fiore, S., Pleissner, D., and Venus, J. (2018). Technical and economic assessment of food waste valorization through a biorefinery chain. Renew. Sustain. Energy Rev. 94, 38–48. doi:10.1016/j.rser.2018.05.064
Demichelis, F., Pleissner, D., Fiore, S., Mariano, S., Navarro Gutiérrez, I. M., Schneider, R., et al. (2017). Investigation of food waste valorization through sequential lactic acid fermentative production and anaerobic digestion of fermentation residues. Bioresour. Technol. 241, 508–516. doi:10.1016/j.biortech.2017.05.174
de Oliveira Moraes, A., Ramirez, N. I. B., and Pereira, N. (2016). Evaluation of the fermentation potential of pulp mill residue to produce d(−)-lactic acid by separate hydrolysis and fermentation using Lactobacillus coryniformis subsp. torquens. Appl. Biochem. Biotechnol. 180 (8), 1574–1585. doi:10.1007/s12010-016-2188-3
Djukić-Vuković, A., Mladenović, D., Ivanović, J., Pejin, J., and Mojović, L. (2019). Towards sustainability of lactic acid and poly-lactic acid polymers production. Renew. Sustain. Energy Rev. 108, 238–252. doi:10.1016/j.rser.2019.03.050
Dreschke, G., Probst, M., Walter, A., Pümpel, T., Walde, J., and Insam, H. (2015). Lactic acid and methane: improved exploitation of biowaste potential. Bioresour. Technol. 176, 47–55. doi:10.1016/j.biortech.2014.10.136
Dutta, S., He, M., Xiong, X., and Tsang, D. C. W. (2021). Sustainable management and recycling of food waste anaerobic digestate: a review. Bioresour. Technol. 341, 125915. doi:10.1016/j.biortech.2021.125915
EBA (2021). EBA Statistical Report 2020 shows significant growth and potential of biomethane to decarbonise the gas sector.
Edwards, J., Othman, M., and Burn, S. (2015). A review of policy drivers and barriers for the use of anaerobic digestion in Europe, the United States and Australia. Renew. Sustain. Energy Rev. 52, 815–828. doi:10.1016/j.rser.2015.07.112
Eiteman, M. A., and Ramalingam, S. (2015). Microbial production of lactic acid. Biotechnol. Lett. 37 (5), 955–972. doi:10.1007/s10529-015-1769-5
Eş, I., Mousavi Khaneghah, A., Barba, F. J., Saraiva, J. A., Sant'Ana, A. S., and Hashemi, S. M. B. (2018). Recent advancements in lactic acid production - a review. Food Res. Int. 107, 763–770. doi:10.1016/j.foodres.2018.01.001
Feng, K., Li, H., and Zheng, C. (2018). Shifting product spectrum by pH adjustment during long-term continuous anaerobic fermentation of food waste. Bioresour. Technol. 270, 180–188. doi:10.1016/j.biortech.2018.09.035
Gandolfi, S., Pistone, L., Ottolina, G., Xu, P., and Riva, S. (2015). Hemp hurds biorefining: a path to green l-(+)-lactic acid production. Bioresour. Technol. 191, 59–65. doi:10.1016/j.biortech.2015.04.118
Gao, M.-T., Shimamura, T., Ishida, N., Nagamori, E., Takahashi, H., Umemoto, S., et al. (2009). Extractive lactic acid fermentation with tri-n-decylamine as the extractant. Enzyme Microb. Technol. 44 (5), 350–354. doi:10.1016/j.enzmictec.2008.12.001
Garvie, E. I. (1967). The growth factor and amino acid requirements of species of the genus Leuconostoc, including Leuconostoc paramesenteroides (sp.nov.) and Leuconostoc oenos. J. Gen. Microbiol. 48 (3), 439–447. doi:10.1099/00221287-48-3-439
Garvie, E. I. (1980). Bacterial lactate dehydrogenases. Microbiol. Rev. 44 (1), 106–139. doi:10.1128/mr.44.1.106-139.1980
Gebrezgabher, S. A., Meuwissen, M. P. M., Prins, B. A. M., and Lansink, A. G. J. M. O. (2010). Economic analysis of anaerobic digestion—a case of Green power biogas plant in The Netherlands. NJAS - Wageningen J. Life Sci. 57 (2), 109–115. doi:10.1016/j.njas.2009.07.006
Ghaffar, T., Irshad, M., Anwar, Z., Aqil, T., Zulifqar, Z., Tariq, A., et al. (2014). Recent trends in lactic acid biotechnology: a brief review on production to purification. J. Radiat. Res. Appl. Sci. 7 (2), 222–229. doi:10.1016/j.jrras.2014.03.002
Ghatak, H. R. (2011). Biorefineries from the perspective of sustainability: feedstocks, products, and processes. Renew. Sustain. Energy Rev. 15 (8), 4042–4052. doi:10.1016/j.rser.2011.07.034
Gluszcz, P., Jamroz, T., Sencio, B., and Ledakowicz, S. (2004). Equilibrium and dynamic investigations of organic acids adsorption onto ion-exchange resins. Bioprocess Biosyst. Eng. 26 (3), 185–190. doi:10.1007/s00449-003-0348-7
Görke, B., and Stülke, J. (2008). Carbon catabolite repression in bacteria: many ways to make the most out of nutrients. Nat. Rev. Microbiol. 6, 613–624. doi:10.1038/nrmicro1932
Gu, S.-A., Jun, C., Joo, J. C., Kim, S., Lee, S. H., and Kim, Y. H. (2014). Higher thermostability of l-lactate dehydrogenases is a key factor in decreasing the optical purity of d-lactic acid produced from Lactobacillus coryniformis. Enzyme Microb. Technol. 58-59, 29–35. doi:10.1016/j.enzmictec.2014.02.008
Gu, X. Y., Liu, J. Z., and Wong, J. W. C. (2018). Control of lactic acid production during hydrolysis and acidogenesis of food waste. Bioresour. Technol. 247, 711–715. doi:10.1016/j.biortech.2017.09.166
Häfner, F., Hartung, J., and Möller, K. (2022). Digestate composition affecting N fertiliser value and C mineralisation. Waste and biomass valorization 13 (8), 3445–3462.
Hakawati, R., Smyth, B. M., McCullough, G., De Rosa, F., and Rooney, D. (2017). What is the most energy efficient route for biogas utilization: heat, electricity or transport? Appl. energy 206, 1076–1087. doi:10.1016/j.apenergy.2017.08.068
Hofvendahl, K., and Hahn–Hägerdal, B. (2000). Factors affecting the fermentative lactic acid production from renewable resources1. Enzyme Microb. Technol. 26 (2), 87–107. doi:10.1016/s0141-0229(99)00155-6
Holzapfel, W. H., and Wood, B. J. B. (2014). Lactic acid bacteria: biodiversity and taxonomy. Incorporated, Hoboken, UNITED KINGDOM: John Wiley and Sons.
Insight, B. (2016). Challenges of building anaerobic digestion plants. Bioenergy Insight 7. ad4Energy.
Jantasee, S., Kienberger, M., Mungma, N., and Siebenhofer, M. (2017). Potential and assessment of lactic acid production and isolation – a review. J. Chem. Technol. Biotechnol. 92 (12), 2885–2893. doi:10.1002/jctb.5237
John, R. P., Nampoothiri, K. M., and Pandey, A. (2008). L(+)-Lactic acid recovery from cassava bagasse based fermented medium using anion exchange resins. Braz. Archives Biol. Technol. 51, 1241–1248. doi:10.1590/s1516-89132008000600020
Jurowski, K., Kochan, K., Walczak, J., Barańska, M., Piekoszewski, W., and Buszewski, B. (2017). Analytical techniques in lipidomics: state of the art. Crit. Rev. Anal. Chem. 47 (5), 418–437. doi:10.1080/10408347.2017.1310613
Juturu, V., and Wu, J. C. (2016). Microbial production of lactic acid: the latest development. Crit. Rev. Biotechnol. 36 (6), 967–977. doi:10.3109/07388551.2015.1066305
Karp, S. G., Igashiyama, A. H., Siqueira, P. F., Carvalho, J. C., Vandenberghe, L. P. S., Thomaz-Soccol, V., et al. (2011). Application of the biorefinery concept to produce l-lactic acid from the soybean vinasse at laboratory and pilot scale. Bioresour. Technol. 102 (2), 1765–1772. doi:10.1016/j.biortech.2010.08.102
Khan, M. A., Ngo, H. H., Guo, W. S., Liu, Y. W., Zhou, J. L., Zhang, J., et al. (2016). Comparing the value of bioproducts from different stages of anaerobic membrane bioreactors. Bioresour. Technol. 214, 816–825. doi:10.1016/j.biortech.2016.05.013
Kim, D.-H., Lim, W.-T., Lee, M.-K., and Kim, M.-S. (2012). Effect of temperature on continuous fermentative lactic acid (LA) production and bacterial community, and development of LA-producing UASB reactor. Bioresour. Technol. 119, 355–361. doi:10.1016/j.biortech.2012.05.027
Kim, K. I., Kim, W. K., Seo, D. K., Yoo, I. S., Kim, E. K., and Yoon, H. H. (2003a). “Production of lactic acid from food wastes,” in Biotechnology for fuels and chemicals: the twenty-fourth symposium. Editors B. H. Davison, J. W. Lee, M. Finkelstein, and J. D. McMillan (Totowa, NJ: Humana Press), 637–647.
Kim, M., Gomec, C. Y., Ahn, Y., and Speece, R. E. (2003b). Hydrolysis and acidogenesis of particulate organic material in mesophilic and thermophilic anaerobic digestion. Environ. Technol. 24 (9), 1183–1190. doi:10.1080/09593330309385659
Kim, M.-S., Na, J.-G., Lee, M.-K., Ryu, H., Chang, Y.-K., Triolo, J. M., et al. (2016). More value from food waste: lactic acid and biogas recovery. Water Res. 96, 208–216. doi:10.1016/j.watres.2016.03.064
Komemoto, K., Lim, Y. G., Nagao, N., Onoue, Y., Niwa, C., and Toda, T. (2009). Effect of temperature on VFA’s and biogas production in anaerobic solubilization of food waste. Waste Manag. 29 (12), 2950–2955. doi:10.1016/j.wasman.2009.07.011
Komesu, A., Maciel, M., and Filho, R. (2017a). Separation and purification technologies for lactic acid – a brief review.
Komesu, A., Maciel, M. R. W., Rocha de Oliveira, J. A., da Silva Martins, L. H., and Maciel Filho, R. (2017b). Purification of lactic acid produced by fermentation: focus on non-traditional distillation processes. Sep. Purif. Rev. 46 (3), 241–254. doi:10.1080/15422119.2016.1260034
Komesu, A., Oliveira, J., Martins, L., Regina Wolf Maciel, M., and Filho, R. (2017c). Lactic acid production to purification: a review. BioResources 12 (2), 4364–4383. doi:10.15376/biores.12.2.4364-4383
Kosseva, M. R. (2013). “Sources, characterisation, and composition of food undustry wastes,” in Food industry wastes: assessment and recuperation of commodities. Editors M. R. Kosseva, and C. Webb (San Diego, UNITED STATES: Elsevier Science and Technology).
Krzyżaniak, A., Leeman, M., Vossebeld, F., Visser, T. J., Schuur, B., and de Haan, A. B. (2013). Novel extractants for the recovery of fermentation derived lactic acid. Sep. Purif. Technol. 111, 82–89. doi:10.1016/j.seppur.2013.03.031
Kumar, A. K., and Sharma, S. (2017). Recent updates on different methods of pretreatment of lignocellulosic feedstocks: a review. Bioresour. Bioprocess. 4 (1), 7. doi:10.1186/s40643-017-0137-9
Kwan, T. H., Hu, Y., and Lin, C. S. K. (2016). Valorisation of food waste via fungal hydrolysis and lactic acid fermentation with Lactobacillus casei Shirota. Bioresour. Technol. 217, 129–136. doi:10.1016/j.biortech.2016.01.134
Kwan, T. H., Hu, Y., and Lin, C. S. K. (2018). Techno-economic analysis of a food waste valorisation process for lactic acid, lactide and poly(lactic acid) production. J. Clean. Prod. 181, 72–87. doi:10.1016/j.jclepro.2018.01.179
Langille, M. G. I., Zaneveld, J., Caporaso, J. G., McDonald, D., Knights, D., Reyes, J. A., et al. (2013). Predictive functional profiling of microbial communities using 16S rRNA marker gene sequences. Nat. Biotechnol. 31 (9), 814–821. doi:10.1038/nbt.2676
Ledesma, O. V., De Ruiz Holgado, A. P., Oliver, G., Giori, G. S. D., Raibaud, P., and Galpin, J. V. (1977). A synthetic medium for comparative nutritional studies of lactobacilli. J. Appl. Bacteriol. 42 (1), 123–133. doi:10.1111/j.1365-2672.1977.tb00676.x
Lee, H. (2015). Development of lactic and SUCCINIC acid biorefinery configurations for integration into a thermomechanical pulp mill.
Li, H., Mustacchi, R., Knowles, C. J., Skibar, W., Sunderland, G., Dalrymple, I., et al. (2004). An electrokinetic bioreactor: using direct electric current for enhanced lactic acid fermentation and product recovery. Tetrahedron 60 (3), 655–661. doi:10.1016/j.tet.2003.10.110
Li, L., Peng, X., Wang, X., and Wu, D. (2018). Anaerobic digestion of food waste: a review focusing on process stability. Bioresour. Technol. 248, 20–28. doi:10.1016/j.biortech.2017.07.012
Linville, J. L., Shen, Y., Wu, M. M., and Urgun-Demirtas, M. (2015). Current state of anaerobic digestion of organic wastes in north America. Curr. Sustainable/Renewable Energy Rep. 2 (4), 136–144. doi:10.1007/s40518-015-0039-4
Liu, S. Q. (2003). Practical implications of lactate and pyruvate metabolism by lactic acid bacteria in food and beverage fermentations. Int. J. Food Microbiol. 83 (2), 115–131. doi:10.1016/s0168-1605(02)00366-5
Liu, X., Lendormi, T., and Lanoisellé, J.-L. (2019). Overview of hygienization pretreatment for pasteurization and methane potential enhancement of biowaste: challenges, state of the art and alternative technologies. J. Clean. Prod. 236, 117525. doi:10.1016/j.jclepro.2019.06.356
López, J. C., Arnáiz, E., Merchán, L., Lebrero, R., and Muñoz, R. (2018). Biogas-based polyhydroxyalkanoates production by Methylocystis hirsuta: a step further in anaerobic digestion biorefineries. Chem. Eng. J. 333, 529–536. doi:10.1016/j.cej.2017.09.185
Luongo, V., Policastro, G., Ghimire, A., Pirozzi, F., and Fabbricino, M. (2019). Repeated-Batch fermentation of cheese whey for semi-continuous lactic acid production using mixed cultures at uncontrolled pH. Sustainability 11 (12), 3330. doi:10.3390/su11123330
Ma, X., Gao, M., Li, C., Wang, N., Wang, Q., and Sun, X. (2021). Effects of different lignocellulosic wastes on alleviating acidification of L-lactic acid production from food waste fermentation. Bioresour. Technol. 342, 126043. doi:10.1016/j.biortech.2021.126043
Manandhar, A., and Shah, A. (2020). Techno-economic analysis of bio-based lactic acid production utilizing corn grain as feedstock. Processes 8 (2), 199. doi:10.3390/pr8020199
Massaro, V., Digiesi, S., Mossa, G., and Ranieri, L. (2015). The sustainability of anaerobic digestion plants: a win–win strategy for public and private bodies. J. Clean. Prod. 104, 445–459. doi:10.1016/j.jclepro.2015.05.021
Mata-Alvarez, J., Dosta, J., Romero-Güiza, M. S., Fonoll, X., Peces, M., and Astals, S. (2014). A critical review on anaerobic co-digestion achievements between 2010 and 2013. Renew. Sustain. Energy Rev. 36, 412–427. doi:10.1016/j.rser.2014.04.039
Matsumoto, M., Mochiduki, K., and Kondo, K. (2004). Toxicity of ionic liquids and organic solvents to lactic acid-producing bacteria. J. Biosci. Bioeng. 98 (5), 344–347. doi:10.1016/s1389-1723(04)00293-2
Mazumdar, S., Blankschien, M. D., Clomburg, J. M., and Gonzalez, R. (2013). Efficient synthesis of L-lactic acid from glycerol by metabolically engineered Escherichia coli Microb. Cell. factories 12 (1), 7. doi:10.1186/1475-2859-12-7
Mazzoli, R., Bosco, F., Mizrahi, I., Bayer, E. A., and Pessione, E. (2014). Towards lactic acid bacteria-based biorefineries. Biotechnol. Adv. 32 (7), 1216–1236. doi:10.1016/j.biotechadv.2014.07.005
Melville, L., Weger, A., Wiesgickl, S., and Franke, M. (2014). “Anaerobic digestion,” in Transformation of Biomass. Editors A. Hornung. doi:10.1002/9781118693643.ch2
Menon, V., and Rao, M. (2012). Trends in bioconversion of lignocellulose: biofuels, platform chemicals and biorefinery concept. Prog. Energy Combust. Sci. 38 (4), 522–550. doi:10.1016/j.pecs.2012.02.002
Mickan, B. S., Ren, A.-T., Buhlmann, C. H., Ghadouani, A., Solaiman, Z. M., Jenkins, S., et al. (2022). Closing the circle for urban food waste anaerobic digestion: the use of digestate and biochar on plant growth in potting soil. J. Clean. Prod. 347, 131071. doi:10.1016/j.jclepro.2022.131071
Mongkhonsiri, G., Charoensuppanimit, P., Anantpinijwatna, A., Gani, R., and Assabumrungrat, S. (2020). Process development of sustainable biorefinery system integrated into the existing pulping process. J. Clean. Prod. 255, 120278. doi:10.1016/j.jclepro.2020.120278
Moraes, B. S., Junqueira, T. L., Pavanello, L. G., Cavalett, O., Mantelatto, P. E., Bonomi, A., et al. (2014). Anaerobic digestion of vinasse from sugarcane biorefineries in Brazil from energy, environmental, and economic perspectives: profit or expense? Appl. Energy 113, 825–835. doi:10.1016/j.apenergy.2013.07.018
Mukherjee, A., Chettri, B., Langpoklakpam, J. S., Basak, P., Prasad, A., Mukherjee, A. K., et al. (2017). Bioinformatic approaches including predictive metagenomic profiling reveal characteristics of bacterial response to petroleum hydrocarbon contamination in diverse environments. Sci. Rep. 7 (1), 1108. doi:10.1038/s41598-017-01126-3
Nabaterega, R., Kumar, V., Khoei, S., and Eskicioglu, C. (2021). A review on two-stage anaerobic digestion options for optimizing municipal wastewater sludge treatment process. J. Environ. Chem. Eng. 9 (4), 105502. doi:10.1016/j.jece.2021.105502
Nagana Gowda, G. A., Zhang, S., Gu, H., Asiago, V., Shanaiah, N., and Raftery, D. (2008). Metabolomics-based methods for early disease diagnostics: a review. Expert Rev. Mol. diagnostics 8 (5), 617–633. doi:10.1586/14737159.8.5.617
Nasr, N., Elbeshbishy, E., Hafez, H., Nakhla, G., and Hesham El Naggar, M. (2012). Comparative assessment of single-stage and two-stage anaerobic digestion for the treatment of thin stillage. Bioresour. Technol. 111, 122–126. doi:10.1016/j.biortech.2012.02.019
Nhu, T. T., Dewulf, J., Serruys, P., Huysveld, S., Nguyen, C. V., Sorgeloos, P., et al. (2015). Resource usage of integrated Pig–Biogas–Fish system: partitioning and substitution within attributional life cycle assessment. Resour. Conservation Recycl. 102, 27–38. doi:10.1016/j.resconrec.2015.06.011
Novik, G., Meerovskaya, O., and Savich, V. (2017). Waste degradation and utilization by lactic acid bacteria: use of lactic acid bacteria in production of food additives, bioenergy and biogas.
O'Connor, J., Mickan, B. S., Rinklebe, J., Song, H., Siddique, K. H. M., Wang, H., et al. (2022). Environmental implications, potential value, and future of food-waste anaerobic digestate management: a review. J. Environ. Manag. 318, 115519. doi:10.1016/j.jenvman.2022.115519
Oldiges, M., Lütz, S., Pflug, S., Schroer, K., Stein, N., and Wiendahl, C. (2007). Metabolomics: current state and evolving methodologies and tools.
Olszewska-Widdrat, A., Alexandri, M., López-Gómez, J. P., Schneider, R., and Venus, J. (2020). Batch and continuous lactic acid fermentation based on A multi-substrate approach. Microorg. (Basel) 8 (7), 1084. doi:10.3390/microorganisms8071084
O'Shea, R., Lin, R., Wall, D. M., Browne, J. D., and Murphy, J. D. (2022). A comparison of digestate management options at a large anaerobic digestion plant. J. Environ. Manag. 317, 115312. doi:10.1016/j.jenvman.2022.115312
Pan, S.-Y., Snyder, S. W., Ma, H.-W., Lin, Y. J., and Chiang, P.-C. (2017). Development of a resin wafer electrodeionization process for impaired water desalination with high energy efficiency and productivity. ACS Sustain. Chem. Eng. 5 (4), 2942–2948. doi:10.1021/acssuschemeng.6b02455
Papagianni, M. (2012). Metabolic engineering of lactic acid bacteria for the production of industrially important compounds. Comput. Struct. Biotechnol. J. 3, e201210003. doi:10.5936/csbj.201210003
Parthiba Karthikeyan, O., Trably, E., Mehariya, S., Bernet, N., Wong, J. W. C., and Carrere, H. (2018). Pretreatment of food waste for methane and hydrogen recovery: a review. Bioresour. Technol. 249, 1025–1039. doi:10.1016/j.biortech.2017.09.105
Plana, P. V., and Noche, B. (2016). A review of the current digestate distribution models: storage and transport. WIT Trans. Ecol. Environ. 202, 345–357. doi:10.2495/wm160311
Pleissner, D., Demichelis, F., Mariano, S., Fiore, S., Navarro Gutiérrez, I. M., Schneider, R., et al. (2017). Direct production of lactic acid based on simultaneous saccharification and fermentation of mixed restaurant food waste. J. Clean. Prod. 143, 615–623. doi:10.1016/j.jclepro.2016.12.065
Pleissner, D., Neu, A.-K., Mehlmann, K., Schneider, R., Puerta-Quintero, G. I., and Venus, J. (2016). Fermentative lactic acid production from coffee pulp hydrolysate using Bacillus coagulans at laboratory and pilot scales. Bioresour. Technol. 218, 167–173. doi:10.1016/j.biortech.2016.06.078
Presswire, M. (2018). “Global lactic acid market is projected to post A cagr of 15.6% over the forecast period,” in M2 Presswire.
Ramaswamy, S., Huang, H.-J., and Ramarao, B. V. (2013). Separation and purification technologies in biorefineries. Somerset, UNITED KINGDOM: Wiley.
Ranjan, S., and Baghel, R. (2018). “Lactic acid market: global historical growth (2012–2016) and future outlook (2017–2024),” in Demand analysis and opportunity evaluation (Research Nester), 2018.
Rawoof Salma Aathika, A., Senthil, K. P., Vo, D.-V. N., Devaraj, K., Mani, Y., Devaraj, T., et al. (2021). Production of optically pure lactic acid by microbial fermentation: a review. Environ. Chem. Lett. 19 (1), 539–556. doi:10.1007/s10311-020-01083-w
Reddy, L. V., Kim, Y.-M., Yun, J.-S., Ryu, H.-W., and Wee, Y.-J. (2016). l-Lactic acid production by combined utilization of agricultural bioresources as renewable and economical substrates through batch and repeated-batch fermentation of Enterococcus faecalis RKY1. Bioresour. Technol. 209, 187–194. doi:10.1016/j.biortech.2016.02.115
Ren, A.-T., Abbott, L. K., Chen, Y., Xiong, Y.-C., and Mickan, B. S. (2020). Nutrient recovery from anaerobic digestion of food waste: impacts of digestate on plant growth and rhizosphere bacterial community composition and potential function in ryegrass. Biol. Fertil. Soils 56 (7), 973–989. doi:10.1007/s00374-020-01477-6
Riva, C., Orzi, V., Carozzi, M., Acutis, M., Boccasile, G., Lonati, S., et al. (2016). Short-term experiments in using digestate products as substitutes for mineral (N) fertilizer: agronomic performance, odours, and ammonia emission impacts. Sci. total Environ. 547, 206–214. doi:10.1016/j.scitotenv.2015.12.156
Rolewicz-Kaliska, A., Oniszk-Popawska, A., Wesolowska, J., and Ryska, E. (2016). Conditions for the development of anaerobic digestion technologies using the organic fraction of municipal solid waste: perspectives for Poland. Environ. Dev. Sustain., 18 (5), 1279–1296.
Saboe, P. O., Manker, L. P., Michener, W. E., Peterson, D. J., Brandner, D. G., Deutch, S. P., et al. (2018). In situ recovery of bio-based carboxylic acids. Green Chem. 20 (8), 1791–1804. doi:10.1039/c7gc03747c
Safdie, S. (2023). Global food waste in 2023, 2023. Greenly. Available at: https://greenly.earth/en-gb.
Salminen, A., Wright, A. v., and Ouwehand, A. (2004). Lactic acid bacteria: microbiological and functional aspects. 3rd ed. New York: Marcel Dekker.
Sambusiti, C., Monlau, F., and Barakat, A. (2016). Bioethanol fermentation as alternative valorization route of agricultural digestate according to a biorefinery approach. Bioresour. Technol. 212, 289–295. doi:10.1016/j.biortech.2016.04.056
Sar, T., Harirchi, S., Ramezani, M., Bulkan, G., Akbas, M. Y., Pandey, A., et al. (2022). Potential utilization of dairy industries by-products and wastes through microbial processes: a critical review. Sci. Total Environ. 810, 152253. doi:10.1016/j.scitotenv.2021.152253
Sawatdeenarunat, C., Nam, H., Adhikari, S., Sung, S., and Khanal, S. K. (2018). Decentralized biorefinery for lignocellulosic biomass: integrating anaerobic digestion with thermochemical conversion. Bioresour. Technol. 250, 140–147. doi:10.1016/j.biortech.2017.11.020
Sawatdeenarunat, C., Nguyen, D., Surendra, K. C., Shrestha, S., Rajendran, K., Oechsner, H., et al. (2016). Anaerobic biorefinery: current status, challenges, and opportunities. Bioresour. Technol. 215, 304–313. doi:10.1016/j.biortech.2016.03.074
Sawatdeenarunat, C., Surendra, K. C., Takara, D., Oechsner, H., and Khanal, S. K. (2015). Anaerobic digestion of lignocellulosic biomass: challenges and opportunities. Bioresour. Technol. 178, 178–186. doi:10.1016/j.biortech.2014.09.103
Scalbert, A., Brennan, L., Fiehn, O., Hankemeier, T., Kristal, B. S., van Ommen, B., et al. (2009). Mass-spectrometry-based metabolomics: limitations and recommendations for future progress with particular focus on nutrition research. Metabolomics 5 (4), 435–458. doi:10.1007/s11306-009-0168-0
Schievano, A., Tenca, A., Lonati, S., Manzini, E., and Adani, F. (2014). Can two-stage instead of one-stage anaerobic digestion really increase energy recovery from biomass? Appl. Energy 124, 335–342. doi:10.1016/j.apenergy.2014.03.024
Serna-Maza, A., Heaven, S., and Banks, C. J. (2015). Biogas stripping of ammonia from fresh digestate from a food waste digester. Bioresour. Technol. 190, 66–75. doi:10.1016/j.biortech.2015.04.041
Simet, A., and Fletcher, K. (2017). “Biogas advances in the US,” in Biomass magazine, BBI international.
Singhvi, M., Zendo, T., and Sonomoto, K. (2018). Free lactic acid production under acidic conditions by lactic acid bacteria strains: challenges and future prospects. Appl. Microbiol. Biotechnol. 102 (14), 5911–5924. doi:10.1007/s00253-018-9092-4
Song, L., Yang, D., Liu, R., Liu, S., Dai, L., and Dai, X. (2022). Microbial production of lactic acid from food waste: latest advances, limits, and perspectives. Bioresour. Technol. 345, 126052. doi:10.1016/j.biortech.2021.126052
Srisowmeya, G., Chakravarthy, M., and Nandhini Devi, G. (2020). Critical considerations in two-stage anaerobic digestion of food waste – a review. Renew. Sustain. Energy Rev. 119, 109587. doi:10.1016/j.rser.2019.109587
Sträuber, H., Lucas, R., and Kleinsteuber, S. (2016). Metabolic and microbial community dynamics during the anaerobic digestion of maize silage in a two-phase process. Appl. Microbiol. Biotechnol. 100 (1), 479–491. doi:10.1007/s00253-015-6996-0
Sun, S., Jones, R. B., and Fodor, A. A. (2020). Inference-based accuracy of metagenome prediction tools varies across sample types and functional categories. Microbiome 8 (1), 46. doi:10.1186/s40168-020-00815-y
Sun, X., Wang, Q., Zhao, W., Ma, H., and Sakata, K. (2006). Extraction and purification of lactic acid from fermentation broth by esterification and hydrolysis method. Sep. Purif. Technol. 49 (1), 43–48. doi:10.1016/j.seppur.2005.08.005
Surendra, K. C., Sawatdeenarunat, C., Shrestha, S., Sung, S., and Khanal, S. (2015). Anaerobic digestion-based biorefinery for bioenergy and biobased products. Ind. Biotechnol. 11 (2), 103–112. doi:10.1089/ind.2015.0001
Tait, S., Harris, P. W., and McCabe, B. K. (2021). Biogas recovery by anaerobic digestion of Australian agro-industry waste: a review. J. Clean. Prod. 299, 126876. doi:10.1016/j.jclepro.2021.126876
Tang, J., Wang, X., Hu, Y., Zhang, Y., and Li, Y. (2016). Lactic acid fermentation from food waste with indigenous microbiota: effects of pH, temperature and high OLR. Waste Manag. 52, 278–285. doi:10.1016/j.wasman.2016.03.034
Teglia, C., Tremier, A., and Martel, J.-L. (2011). Characterization of solid digestates: Part 1, review of existing indicators to assess solid digestates agricultural use. Waste Biomass Valorization 2 (1), 43–58. doi:10.1007/s12649-010-9051-5
Thakur, M., Modi, V. K., Khedkar, R., and Singh, K. (2021). Characterization and treatment of waste from food processing industries. Singapore: Springer, 41–58.
Thang, V. H., and Novalin, S. (2008). Green Biorefinery: separation of lactic acid from grass silage juice by chromatography using neutral polymeric resin. Bioresour. Technol. 99 (10), 4368–4379. doi:10.1016/j.biortech.2007.08.045
Tung, A. L., and Judson King, C. (1994). Sorption and extraction of lactic and succinic acids at pH > pKa1. I. Factors governing equilibria. Industrial Eng. Chem. Res. 33, 3217–3223. doi:10.1021/ie00036a041
Turnley, D., Hopwood, L., Burns, C., and Maio, D. D. (2016). Assessment of digestate drying as an eligible heat use in the Renewable Heat Incentive.
Ujor, V. C., Okonkwo, C. C., Rush, B. B., McCrea, G. E., and Ezeji, T. C. (2020). Harnessing the residual nutrients in anaerobic digestate for ethanol fermentation and digestate remediation using Saccharomyces cerevisiae. Fermentation 6 (2), 52. doi:10.3390/fermentation6020052
Van Hecke, W., Kaur, G., and De Wever, H. (2014). Advances in in-situ product recovery (ISPR) in whole cell biotechnology during the last decade. Biotechnol. Adv. 32 (7), 1245–1255. doi:10.1016/j.biotechadv.2014.07.003
Van Wouwe, P., Dusselier, M., Vanleeuw, E., and Sels, B. (2016). Lactide synthesis and chirality control for polylactic acid production. ChemSusChem 9 (9), 907–921. doi:10.1002/cssc.201501695
Vasco-Correa, J., Khanal, S., Manandhar, A., and Shah, A. (2018). Anaerobic digestion for bioenergy production: global status, environmental and techno-economic implications, and government policies. Bioresour. Technol. 247, 1015–1026. doi:10.1016/j.biortech.2017.09.004
Wang, Q., Yang, L., Feng, K., Li, H., Deng, Z., and Liu, J. (2021). Promote lactic acid production from food waste fermentation using biogas slurry recirculation. Bioresour. Technol. 337, 125393. doi:10.1016/j.biortech.2021.125393
Wang, S., Xu, C., Song, L., and Zhang, J. (2022). Anaerobic digestion of food waste and its microbial consortia: a historical review and future perspectives. Int. J. Environ. Res. Public Health 19 (15), 9519. doi:10.3390/ijerph19159519
Wang, Y., Tashiro, Y., and Sonomoto, K. (2015). Fermentative production of lactic acid from renewable materials: recent achievements, prospects, and limits. J. Biosci. Bioeng. 119 (1), 10–18. doi:10.1016/j.jbiosc.2014.06.003
Wischral, D., Arias, J. M., Modesto, L. F., de França Passos, D., and Pereira, N. (2019). Lactic acid production from sugarcane bagasse hydrolysates by Lactobacillus pentosus: integrating xylose and glucose fermentation. Biotechnol. Prog. 35 (1), e2718. doi:10.1002/btpr.2718
Wong, G. (2017). “Market analysis of opportunities,” in Australia for Anaerobic Digestion Deployment. (SM01-CC-REP-0001).
WRAP, (2017). BSI PAS 110: Producing Quality Anaerobic Digestate. BSI Standards Limited: The Waste and Resources Action Programme, 2022.
Wu, Y., Wang, C., Liu, X., Ma, H., Wu, J., Zuo, J., et al. (2016). A new method of two-phase anaerobic digestion for fruit and vegetable waste treatment. Bioresour. Technol. 211, 16–23. doi:10.1016/j.biortech.2016.03.050
Xu, H., Li, B., and Mu, X. (2016). Review of alkali-based pretreatment to enhance enzymatic saccharification for lignocellulosic biomass conversion. Industrial Eng. Chem. Res. 55 (32), 8691–8705. doi:10.1021/acs.iecr.6b01907
Xu, K., and Xu, P. (2014). Efficient calcium lactate production by fermentation coupled with crystallization-based in situ product removal. Bioresour. Technol. 163, 33–39. doi:10.1016/j.biortech.2014.04.002
Yang, S.-T., El-Ensashy, H., and Thongchul, N. (2013). “Bioprocessing technologies in biorefinery for sustainable production of fuels, chemicals, and polymers,” in Biorefinery for sustainable production of fuels, chemicals, and polymers (Somerset, UNITED STATES: American Institute of Chemical Engineers).
Yankov, D., Jj, M., Gg, K., Albet, J., and Gg, M. (2005). Improvement of the lactic acid extraction. Extraction from aqueous solutions and simulated fermentation broth by means of mixed extractant and TOA, partially loaded with HCl. Chem. Biochem. Eng. Q. 19.
Yousuf, A., Bastidas-Oyanedel, J.-R., and Schmidt, J. E. (2018). Effect of total solid content and pretreatment on the production of lactic acid from mixed culture dark fermentation of food waste. Waste Manag. 77, 516–521. doi:10.1016/j.wasman.2018.04.035
Yuan, S.-F., Hsu, T.-C., Wang, C.-A., Jang, M.-F., Kuo, Y.-C., Alper, H. S., et al. (2018). Production of optically pure l(+)-lactic acid from waste plywood chips using an isolated thermotolerant Enterococcus faecalis SI at a pilot scale. J. industrial Microbiol. Biotechnol. 45 (11), 961–970. doi:10.1007/s10295-018-2078-5
Zaman, A., and Reynolds, C. (2015). The economic and bio-energy production potential of South Australian food waste using Anaerobic digestion. Adelaide, South Australia: Unmaking Waste.
Zhang, C., Yang, H.-Q., and Wu, D.-J. (2019). Study on the reuse of anaerobic digestion effluent in lactic acid production. J. Clean. Prod. 239, 118028. doi:10.1016/j.jclepro.2019.118028
Zhang, W., Li, X., He, Y., Xu, X., Chen, H., Zhang, A., et al. (2020a). Ammonia amendment promotes high rate lactate production and recovery from semi-continuous food waste fermentation. Bioresour. Technol. 302, 122881. doi:10.1016/j.biortech.2020.122881
Zhang, W., Li, X., Zhang, T., Li, J., Lai, S., Chen, H., et al. (2017). High-rate lactic acid production from food waste and waste activated sludge via interactive control of pH adjustment and fermentation temperature. Chem. Eng. J. 328, 197–206. doi:10.1016/j.cej.2017.06.174
Zhang, W., Xu, X., Yu, P., Zuo, P., He, Y., Chen, H., et al. (2020b). Ammonium enhances food waste fermentation to high-value optically active l-lactic acid. ACS Sustain. Chem. Eng. 8 (1), 669–677. doi:10.1021/acssuschemeng.9b06532
Zhang, Y., Qian, Z., Liu, P., Liu, L., Zheng, Z., and Ouyang, J. (2018). Efficient in situ separation and production of l-lactic acid by Bacillus coagulans using weak basic anion-exchange resin. Bioprocess Biosyst. Eng. 41 (2), 205–212. doi:10.1007/s00449-017-1858-z
Zhao, Q., Leonhardt, E., MacConnell, C., Frear, C., and Chen, S. (2010). Purification technologies for biogas generated by anaerobic digestion.
Zheng, J., and Rehmann, L. (2014). Extrusion pretreatment of lignocellulosic biomass: a review. Int. J. Mol. Sci. 15 (10), 18967–18984. doi:10.3390/ijms151018967
Keywords: lactic acid, fermentation, anaerobic digestion, biorefinery, integration
Citation: Bühlmann CH, Mickan BS, Tait S, Batstone DJ and Bahri PA (2023) Waste to Wealth: The power of food-waste anaerobic digestion integrated with lactic acid fermentation. Front. Chem. Eng. 5:1285002. doi: 10.3389/fceng.2023.1285002
Received: 29 August 2023; Accepted: 22 November 2023;
Published: 14 December 2023.
Edited by:
Antoni Sánchez, Autonomous University of Barcelona, SpainReviewed by:
Jialing Tang, Chengdu University, ChinaIoannis Vyrides, Cyprus University of Technology, Cyprus
Maria Kosseva, Consultant, Bulgaria
Copyright © 2023 Bühlmann, Mickan, Tait, Batstone and Bahri. This is an open-access article distributed under the terms of the Creative Commons Attribution License (CC BY). The use, distribution or reproduction in other forums is permitted, provided the original author(s) and the copyright owner(s) are credited and that the original publication in this journal is cited, in accordance with accepted academic practice. No use, distribution or reproduction is permitted which does not comply with these terms.
*Correspondence: Christopher H. Bühlmann, Q2hyaXMuYnVobG1hbm5AdXNxLmVkdS5hdQ==