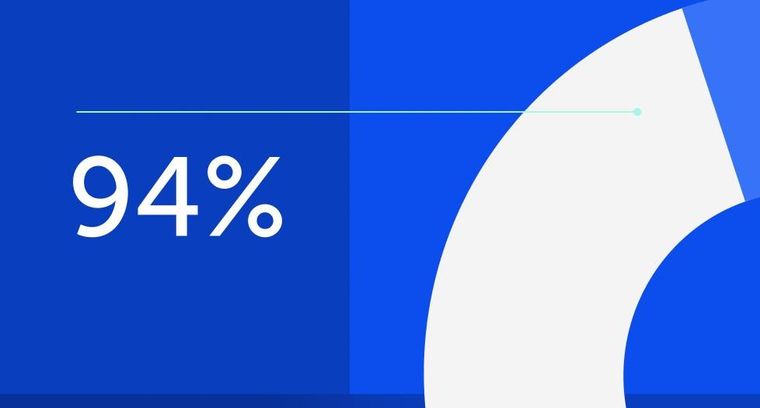
94% of researchers rate our articles as excellent or good
Learn more about the work of our research integrity team to safeguard the quality of each article we publish.
Find out more
REVIEW article
Front. Chem. Eng., 30 November 2023
Sec. Separation Processes
Volume 5 - 2023 | https://doi.org/10.3389/fceng.2023.1271045
This article is part of the Research TopicSolvent Extraction Pathways to Sustainable Industrial Processes: New Solvents, Modelling, and Design MethodsView all 7 articles
We summarize advances in lithium recognition receptors, focusing on their use as active reagents in circular processes such as liquid–liquid extraction, an established industrial process that is advantageous due to its large processing capacity and high selectivity. High-performance systems are required, given the increasing demand for effective separation processes for the recovery of valuable substances from spent lithium-ion batteries or the exploration of brines. Hence, the availability of powerful and highly selective receptors is particularly crucial. This mini-review summarizes the development of active receptors for lithium ion extraction and covers advances in receptors for both lithium ions and lithium salts. It discusses various receptor types, ranging from heteroditopic macrocyclic systems to simple β-diketones. The latter achieve particularly high lithium ion extraction yields in the presence of phosphine oxides such as trioctylphosphine oxide. Structural studies employing 4-phosphorylpyrazolone exhibited diverse coordination modes of lithium and represent clear evidence for the synergistic role of the co-ligand on a molecular level.
Lithium, an s-block element, has attracted extensive commercial interest in recent decades because it is indispensable in the production of lithium-ion batteries (LIBs) and as a component of many other commercial goods, such as glasses, ceramics, lubricants, and pharmaceuticals (Birch, 1999; Donald et al., 2008; Goodenough and Park, 2013; Mjos and Orvig, 2014; Parikh et al., 2019). LIBs are widely used to reduce reliance on fossil fuels in transport systems and to mitigate global warming by reducing CO2 emissions; this has led to a rapid increase in the consumption of the Earth’s lithium resources (Oliveira et al., 2015; Bae and Kim, 2021). Some projections suggest that global lithium demand will not be met by 2023 without recycling (Sonoc et al., 2015). This is particularly problematic at present, as the global lithium recovery rate is currently no more than 1% (Swain, 2017). Exploration of efficient strategies to detect and recover lithium from various sources, such as spent LIBs, brines, and seawater, will help increase available lithium resources and reduce environmental impacts, and thereby achieve carbon neutrality and sustainable development.
In recent years, various techniques have been used for lithium separation, including liquid–liquid extraction (He et al., 2018; Cui et al., 2019; Zhang et al., 2020; Chen et al., 2021; Hanada and Goto, 2021; Masmoudi et al., 2021), solid–liquid extraction (He et al., 2016; Gohil et al., 2019), adsorption (Wang et al., 2018), and membrane processes (Guo et al., 2016; Razmjou et al., 2019; Lu et al., 2020; Hou et al., 2021). This mini-review focuses on liquid–liquid extraction because of its advantages, such as large processing capacity, high selectivity and extraction efficiency, easy large-scale operation, and high potential for industrial lithium separation (Kumar et al., 2019; Bai et al., 2020). The selective binding of lithium ions by specific organic receptors has been challenging, and only a small selection of ligands, such as β-diketones, organophosphorus extractants, macrocyclic receptors, pyrazolone-based ligands, and ditopic receptors, has been developed and used in liquid–liquid or solid–liquid extraction (He et al., 2016; Swain, 2016; He et al., 2018; Gohil et al., 2019; Zhang et al., 2020; Hanada and Goto, 2021; Masmoudi et al., 2021). This review systematically summarizes the receptors applied for lithium recognition and separation. These can be divided into two categories according to their function: receptors for lithium cations and for lithium salts.
The most common receptors employed for lithium separation are β-diketones. Benzoyl-1,1,1-trifluoroacetone (HBTA, 1), 4,4,4-trifluoro-1-(2-thienyl)-1,3-butanedione (HTTA, 2), and α-acetyl-m-dodecylacetophenone as the major component of LIX 54 (3) are widely used for lithium extraction (Figure 1) (Healy, 1968; Nguyen and Lee, 2018; Zhang et al., 2018; Wang et al., 2019; Zhang et al., 2020; Zhang et al., 2021a; Zhang et al., 2021; Hanada and Goto, 2021; Masmoudi et al., 2021; Zhang et al., 2022). Neutral co-ligands, such as trioctylphosphine oxide (TOPO, 4), tributylphosphine oxide (TBPO, 5), tributylphosphate (TBP, 6), or mixtures of trialkylphosphine oxides (Cyanex 923, 7) are also required in the application of β-diketone extraction systems (Figure 1). These co-ligands can generally saturate the coordination sphere of lithium ions, and the resulting complexes are less hydrophilic, which promotes their transfer into the organic phase (Atanassova and Kurteva, 2016).
Healy (1968) studied the synergistic effect of using HTTA with various co-ligands (TOPO, TBP, etc.) for extracting alkali metal ions (Li+, Na+, K+, and Cs+). The results showed much higher extraction ability for Li+ than for Na+, K+, and Cs+. Slope analyses suggested that the extracted species can best be described as [M(TTA)S2] (M = Li+, Na+, K+, Cs+; S = co-ligands). Zhang et al. (2020) introduced deprotonated HBTA using a 2 M NaOH saponification reaction into a kerosene system in the presence of co-ligand TOPO and achieved 90% lithium extraction by a three-stage countercurrent. The loaded Li+ was stripped by 6 M HCl to obtain a lithium-rich solution. Pranolo et al. (2015) investigated a synergistic liquid–liquid extraction system containing LIX 54 and Cyanex 923 in ShellSol D70 for separating lithium from an aqueous solution containing a high concentration of sodium. More than 97% of the lithium was transferred to the organic phase, while less than 3% of the sodium present was co-extracted at pH 11 in a single extraction stage, demonstrating high lithium selectivity using the LIX 54 and Cyanex 923 synergistic system.
Ionic liquids (ILs) are considered to be effective reagents or “green solvents” for metal separation due to advantages such as negligible vapor pressure, high thermal stability, and tunable molecular structures (Sun et al., 2012). Various ILs have been extensively studied in recent years for the extraction of lithium (Shi et al., 2017a; Shi et al., 2017b; Cui et al., 2019; Wang et al., 2020; Bai et al., 2021; Cai et al., 2021; Zhang et al., 2021c; Hanada and Goto, 2021; Yu et al., 2021; Ole et al., 2022). Wang et al. (2020) investigated synergistic extraction systems containing diketonate-based ionic liquid extractants and a trialkylphosphine oxide—presumably Cyanex 923—for lithium separation in the presence of sodium. The ILs combined different deprotonated β-diketone anions, such as [BTA]− or [TTA]−, with trialkylmethylammonium ([A336]+) cation. Experiments showed that 83% of lithium can be extracted with the mixture of [A336][TTA] (8, Figure 2) and the phosphine oxide from a basic aqueous solution (pH = 10.2), which was higher than that of HTTA (72%) (Wang et al., 2020).
Hanada and Goto (2021) investigated a new synergistic deep eutectic solvent (DES) system containing a β-diketone (HBTA or HTTA) and a neutral extractant such as TOPO. Therefore, HBTA can act as a hydrogen bond donor (HBD) and TOPO as a hydrogen bond acceptor (HBA), and their mixture exhibited a high extraction power for lithium ions and good selectivity for lithium ions over sodium and potassium ions.
Overall, the various studies employing the β-diketones HTTA and HBTA showed a high binding affinity and selectivity for lithium ions over other alkali metal ions. However, there are still some issues that need to be addressed. For example, the β-diketones generally require saponification pretreatment that employs a strong base such as NaOH, or they need a very basic aqueous solution (pH > 11) for effective extraction of lithium ions. Consequently, a large amount of base is consumed, and it also results in the severe corrosion of equipment. Moreover, it is reported that β-diketones tend to have high dissolution in aqueous solutions under basic conditions, which may lead to loss of ligands (Xu et al., 2021). Nevertheless, high partition coefficients (D) of lithium and separation factors (ß) of lithium toward sodium, potassium, and cesium are obtained, as summarized and compared with other receptors in Table 1.
TABLE 1. Distribution coefficients of lithium and separation factors of lithium over sodium, potassium, cesium, and magnesium for different receptors.
Acidic organophosphorus compounds such as di-(2-ethylhexyl) phosphoric acid (D2EHPA, 9) (Hano et al., 1992; Song et al., 2020; Meng et al., 2021; Mahmoudi et al., 2022), 2-ethylhexyl phosphonic acid mono-2-ethylhexyl ester (PC-88A, 10) (Hano et al., 1992; Zushi et al., 2000; Virolainen et al., 2017), di(2-ethylhexyl) phosphinic acid (P227, 11) (L et al., 2022), and bis(2,4,4-trimethylpentyl) phosphinic acid (Cyanex 272, 12) (Swain et al., 2010; Nguyen and Lee, 2018; Lu et al., 2021) were also probed for the extraction of lithium ions (Figure 3). Their application generally required the presence of neutral co-ligands such as TOPO, TBP, or Cyanex 923 (Hano et al., 1992; Lu et al., 2021; Meng et al., 2021). The synergistic effect provided by these co-ligands is the same as the mechanism mentioned earlier. Hano et al. (1992) studied the extraction of lithium ions using D2EHPA or PC-88A from alkali and alkaline earth metal ions (Li+, Na+, K+, Mg2+, and Ca2+). Both extractants exhibited higher selectivity for Li+ over Na+ and K+, but much higher extraction powers were observed for the divalent cations Mg2+ and Ca2+. An apparent synergistic effect was observed for lithium ion extraction after the addition of TBP as co-ligand. Shi et al. (2020) employed a saponified D2EHPA–kerosene system to remove Ca2+ and Mg2+ from Li+ concentrated solution. The process they proposed can remove 99% of Ca2+ and 98% of Mg2+ in a three-stage extraction, while the loss of Li+ was approximately 5% (Shi et al., 2020). Liu et al. (2022) found that 11 exhibited a superior separation ability of Ca2+ and Mg2+ from Li+. In a multi-element system, Ca2+ and Mg2+ were removed quantitatively, while only 0.7% Li+ was extracted. The purity of Li+ in aqueous solutions can reach more than 99% in the simulated system. Liu et al. (2022) investigated the efficiency of lithium ion extraction in the presence of Co2+ using the Cyanex272/TBP/kerosene system. The experiments showed that Co2+ was extracted almost quantitatively at a pH of 5.5, whereas at a lower pH no transfer of Li+ into the organic phase was observed. This pH was thus sufficient to separate the two metal ions. However, at a pH of 8, no more than 40% of the Li+ was extracted, and further increasing the pH did not result in higher extraction yields (Lu et al., 2021). These findings demonstrated that D2EHPA, PC-88A, P227, and Cyanex 272 have a much stronger coordination ability for divalent cations than monovalent lithium ions. Thus, organophosphorus compounds can be employed as strong chelating reagents to remove divalent cations from a solution containing mono- and divalent cations under acidic conditions.
D2EHPA-based ILs including tetrabutylammonium bis(2-ethylhexyl)-phosphate (13, [N4444][D2EHPA]) and tetraoctyl-ammonium bis(2-ethylhexyl)-phosphate (14, [N8888][D2EHPA]) (Figure 4) were synthesized by Shi et al. (2017b). Comparative studies with D2EHPA showed their suitability for extracting lithium ions in the absence of additional hydrochloric acid. While up to 90% of the Li+ present was transferred to the organic phase by 13, D2EHPA showed only negligible extraction activity. In addition, these experiments revealed that increasing the length of the alkyl chain of the used ammonium cation led to a decrease in their extraction ability. The authors attributed this to an increase in steric hindrance. Probing the lithium distribution ratio dependence on the IL concentration suggested the formation of 1:1 complexes, where one molecule of IL formed a complex with a single lithium ion during the extraction process. Competitive studies involving alkali metal ions showed a preferred extraction of Li+ over Na+, K+, Rb+, and Cs+ (Shi et al., 2017b).
FIGURE 4. Molecular structures of ionic liquids [N4444][D2EHPA] (13) and [N8888][D2EHPA] (14) for lithium extraction.
The TBP/FeCl3 extraction system is very commonly used for the separation of Li+ from a high concentration of Mg2+ (Zhou et al., 2012; Su et al., 2020a; Li and Binnemans, 2021a; Li and Binnemans, 2021b; Su et al., 2022; Sun et al., 2022). The typical extraction mechanism can be explained by Eqs 1−3 (Shi et al., 2019a):
These equations show that FeCl3 is a necessary source for forming an anionic species in the presence of a large excess of Cl−. Li+ can be transferred to the organic phase as a counterion to neutralize the complex of [FeCl4]- and TBP. A very high concentration of Cl− (> 6 M) is required to form [FeCl4]- in this system. In addition, a high HCl concentration is commonly required in the stripping process to prevent the loss of Fe3+ (Shi et al., 2019b; Su et al., 2020b). NaOH or Mg(OH)2 is usually utilized as a saponification agent to neutralize the proton in the organic phase after stripping (Zhou et al., 2020). The binding affinity of TBP toward cations followed the order H+ > Li+ > Na+ > Mg2+ ≈ K+ (Zhou et al., 2020); therefore, Na+ can be replaced by Li+ in the reuse cycle of extraction. Shi et al. (2019b) investigated the separation of Li+ from Mg2+ using a TBP/kerosene–FeCl3 system. With a TBP concentration of 75%, a molar ratio of 1.3/1 of Fe/Li, and an acid concentration of 0.01 mol/L in brine, 68% of lithium was extracted in a single stage. Subsequently, the loaded Li+ phase was stripped with 6 M HCl, and the organic phase was regenerated with 2 M NaOH (Shi et al., 2019b). Zhou et al. (2020) used HCl+MgCl2, HCl+NaCl, Mg(OH)2, or MgCO3 as washing, stripping, and regeneration reagents, respectively. Li+ extraction of about 65% was achieved, and the separation factor between Li+ and Mg2+ was 350 after a single-stage extraction (Zhou et al., 2020). Despite the high separation factor, there are two main problems to be solved in the TBP/kerosene–FeCl3 system: first, the easy formation of a third phase and, second, the difficult removal of loaded Li+ from the organic phase (Zhou et al., 2021).
To avoid the use of a high concentration of HCl in the stripping process, Su et al. (2020c) introduced PC-88A (10) into the TBP/kerosene organic phase. Slope analysis revealed that Li+ is extracted in the form of [Li·2TBP·FeCl4], indicating that 10 was not involved in the coordination during the Li+ extraction process. However, 10 can coordinate Fe3+ in the form of [FeCl2(10-H)·(10)·2TBP] once the loaded organic phase is washed by H2O. Consequently, Li+ was stripped from the extracted species by H2O instead of HCl solution, which dramatically increased the stripping efficiency more sustainably (Su et al., 2020c).
Based on the advantages of ILs, attempts have been made in recent years to replace FeCl3 with an IL to provide an anion for the extraction of lithium ions. Wang et al. (2019) introduced 1-butyl-3-methylimidazolium phosphotungstate ([Bmim]3PW12O40) as a co-extraction reagent in the TBP system. The experiments showed that selectivity under optimized conditions follows the order Li+ > Na+ > K+ ≈ Mg2+. Proton NMR spectroscopy analysis demonstrated that Li+ was extracted by a cation exchange mechanism: one [Bmim]+ cation was transferred to the aqueous phase for each Li+ extracted into the organic phase (Wang et al., 2019). Li et al. (2021) synthesized 1-butyl-3-methylimidazolium tetraphenylboron ([Bmin]BPh4) and prepared the organic phase in CH2BrCl solvent containing TBP and ([Bmin]BPh4). After a four-stage cross-flow extraction, 99% of Li+ was extracted. The loaded Li+ was completely removed using 2.0 mol/L Na2CO3 solution as the stripping agent, and the organic phase was reused directly without a regeneration process (Li et al., 2021). In order to avoid the loss of valuable IL cations such as [Bmim]+ during lithium ion extraction, Zhou et al. (2021) introduced sodium hexafluorophosphate (NaPF6) as a co-extraction reagent. The experiments showed that NaPF6 exhibits comparable efficiency as [Bmim][PF6] for lithium ion extraction (79%) from magnesium ion-rich solution by TBP. In addition, the studies revealed a more efficient stripping of Li+ from the loaded organic phase by HCl than by Na2CO3. However, Na2CO3 is also a potential stripping reagent since the Li+ can be stripped as Li2CO3 in the aqueous solution, and the organic phase can be directly reused for the next cycle (Zhou et al., 2021).
Acylpyrazolones, which are members of the β-diketone family, are widely used for the coordination of various metal ions (Figure 5) (Marchetti et al., 2005; Marchetti et al., 2015; Taydakov et al., 2020). Jensen et al. (1959) reported an improved method for synthesizing 1-phenyl-3-methyl-4-acyl-pyrazolones-5 and its use for the extraction of metal ions. Thereafter, acylpyrazolones have been used extensively as effective chelating reagents for various applications, such as the determination of metals in trace amounts; in coordination studies with various metal ions including transition metals, lanthanoids, and actinoids; and in the separation of metals (Marchetti et al., 2005; Marchetti et al., 2015; Taydakov et al., 2020). Bukowsky et al. (1992) synthesized two acylpyrazolone derivatives by the condensation reactions of 1-phenyl-3-methylpyrazol-5-one with the corresponding acid chlorides based on the method of Jensen et al. (1959). Liquid–liquid extraction studies employing 1-phenyl-3-methy1-4-stearoylpyrazol-5-one (15) or 1-phenyl-3-methy1-4-lauroylpyrazol-5-one (16) in a pH range of 4–7 showed high extraction of Mg2+ and Ca2+, whereas the determined distribution rate for Li+ was low. By adding TOPO, the authors observed a significant increase in the extraction of Li+. Therefore, the high separation factors for the alkaline earth metal ions were drastically diminished. However, other alkali metal ions were extracted to only a minor extent.
FIGURE 5. Molecular structures of acylpyrazolone derivatives used for lithium separation and their tautomeric forms.
In addition, much higher extraction of Li+ than of Na+, K+, and Cs+ was observed when 1-phenyl-3-methy1-4-benzoylpyrazol-5-one (17) and a phosphine oxide were used as an extractant and co-ligand, respectively (Bukowsky et al., 1992). Li et al. employed 17 and Cyanex 923 (7) to remove magnesium ions from lithium ion-rich brine. All Mg2+ from a synthetic solution containing 24 g/L Li+ and 0.24 g/L Mg2+ was removed, while only 0.6% Li+ was co-extracted. The slope analyses showed that Mg2+ was extracted as [Mg(17)2(7)2], while Li+ was extracted as a mixture of [Li(17)x(7)2] (x = 1, 2) (Li and Binnemans, 2020).
Based on the results obtained, it appears that higher binding affinity or extraction enhancement of acylpyrazolones toward metal cations can be obtained when phosphine oxides or phosphate esters are present in the extraction process. Matt et al. (1992) first introduced a phosphinoyl fragment into the pyrazolone moiety to synthesize 4-diphenylphosphinoyl pyrazolone (18) and investigated the binding properties for Fe3+ (Figure 6). However, very few follow-up studies on the synthesis and application of this type of P-containing pyrazolone ligands have since been reported.
Consequently, the Weigand group developed a series of new 4-phosphorylpyrazolone receptors by inserting a more tunable and versatile phosphoryl moiety into the pyrazolone backbone (Figure 7) (Zhang et al., 2022; Zhang et al., 2023). The lipophilicity of ligands depends on the properties of substituents in positions R1 and R2 (Figure 7). The bite size of the two O-donors of the ligands plays an important role in metal ion binding and selectivity and is highly tunable by the substituents at the R2 position. As shown in Figure 7, the bite size can be characterized by the distance between two O-donors (O∙∙∙O). Herein, we have listed the O∙∙∙O distance for each receptor in Table 2. Moreover, the electronic properties of the ligands are strongly influenced by the substituents at the R3 position (Figure 7). Zhang et al. (2022) synthesized different 4-phosphorylpyrazolone derivatives 19–21 by altering substituents at the R2 positions, while the substituents at the R1 and R3 positions were fixed with an isopropyl and a nitro group, respectively. X-ray crystallographic analysis of free ligands and their tetrabutylammonium salts revealed that the bite size between the chelating O-donor atoms of the phosphoryl and azole moieties is highly influenced by the R2 substituents, in which the smallest O···O distance is obtained in 21. Of the substituents studied, the phenyl group has a higher steric requirement and exhibits the smallest bite size between the chelating O-donor atoms. The ligands were successfully used to coordinate with lithium cation and various co-ligands (4, 5, and 6). The lithium complexes involved exhibited diverse coordination modes, such as dimer and trimer, which represent the first clear evidence for the synergistic role of these co-ligands on a molecular level (Figure 8). Sophisticated solution studies employing 1H, 31P, and 7Li NMR exhibit reversible interconversion between dimeric and trimeric species. Furthermore, mass spectrometry and liquid–liquid extraction studies also provide evidence for the presence of such multinuclear lithium complexes in solution. The highest Li+ extraction (78%) was obtained for 21 in a single element liquid–liquid extraction experiment at pH 8.2, which reflects the influence of bite size on lithium ion capture. Multi-element extraction studies confirm that 19–21 represent a new class of receptors that selectively extracted Li+ from high concentrations of Na+, K+, and Cs+ under mild conditions (pH = 8.3). Hence, up to 77% of Li+ was transferred into the organic phase, whereas 6% of Cs+ was extracted, while Na+ and K+ were extracted in trace amounts (< 1%).
TABLE 2. O
FIGURE 8. Molecular structures of (A) [Li2(21-H)2(5)2]
As an extension of this study, the Weigand group further modified the 4-phosphorylpyrazolone receptors and synthesized derivatives 22–25 by changing R3 substituents (Figure 7) (Zhang et al., 2023). Electron-neutral (R3 = H, 22), electron-donating (R3 = Me, 23), and electron-withdrawing (R3 = Cl, 24; R3 = CF3, 25) substituents were introduced to the R3 position, as the acid dissociation properties of this type of ligand are strongly influenced by the electronic nature of such groups (Zhang et al., 2023). Receptors 22–25 proved to be soluble in nonpolar solvents such as n-octane than 19–21, which is beneficial for real-world applications. Currently, kerosene (mainly composed of alkanes), as a nonpolar solvent, is the most common diluent for metal ion extraction in industry due to its low toxicity and cost. Therefore, the good solubility of receptors 22–25 in n-octane (C8H18) provides a good hint of their industrial application.
X-ray crystallographic analysis of magnesium and calcium complexes shed light on the coordination behavior of these metals, particularly for the co-ligands 4 and 5 involved complexes. Single-element liquid–liquid extraction experiments using an aqueous phase at a pH of 5 showed that the highest Li+ extraction (77%) was observed for 25, in which a strong electron-withdrawing group CF3 was introduced to the R3 position. Multi-element (including Li+, Na+, K+, Mg2+, and Ca2+) extraction experiments demonstrated that the phase transfer of Mg2+ and Ca2+ is much higher than Li+ at equilibrium pH 4.2. Mg2+ and Ca2+ were extracted quantitatively, while only 29% of Li+ was extracted and no Na+ and K+ were transferred into the organic phase using 25. Interestingly, a stepwise liquid–liquid extraction of Ca2+, Mg2+, and Li+ by pH regulation in an acidic pH range was achieved: the majority of Ca2+ (64%) was removed at pH 2 and most Mg2+ (93%) was extracted at pH 3, while all Ca2+ was already removed following several reiterations of the first step. The required pH (> 4.2) for the effective Li+ extraction (> 50%) is obviously higher than that observed for the extraction of Mg2+ and Ca2+. At equilibrium pH 6, 94% of Li+ was extracted and less than 4% of Na+ and K+ extractions were observed. The findings of the Weigand group demonstrated that 4-phosphorylpyrazolones can be employed as pH-regulated receptors for the stepwise separation of Ca2+, Mg2+, and Li+ from the alkali and alkaline earth metals (Zhang et al., 2023), which is probably most easily realized by using standard devices such as a mixer-settlers, in which the pH is adjusted before each mixer. The study indicated a way to separate lithium ion from acidic brine or spent LIB leaching solutions. This supports a more environmentally friendly future supply of lithium.
Macrocyclic receptors, particularly crown-4 derivatives, are another type of widely studied ligands for selective lithium ion recognition (Tagne kuate et al., 2010; TSE et al., 2021; Kim et al., 2022a; Gomez-Vega et al., 2022; Munasinghe et al., 2022; Yang et al., 2022). Charles J. Pedersen was the first to synthesize a series of crown ethers and study their coordination behavior with various metal salts (Pedersen, 1967). He shared the 1987 Nobel Prize in chemistry with Donald J. Cram and Jean-Marie Lehn for the development of supramolecular chemistry (Pedersen, 1967; Lehn, 1988). His detailed studies on the coordination behavior of crown ethers containing five to ten oxygen atoms with some alkali, alkaline earth, and lanthanoid metal ions such as Li+, Na+, K+, Rb+, Cs+, Ca2+, Sr2+, Ba2+, La3+, and Ce3+ provided very valuable clues for later extensive studies (Pedersen, 1967; Gokel et al., 2004a; Ge et al., 2009; Nisola et al., 2020; Yan et al., 2020; Docker et al., 2022). According to the “hard and soft acid and base” (HSAB) theory, the oxygen atoms of polyethers are hard bases that can form stable complexes with hard Lewis acids such as alkali metal ions Li+, Na+, and K+ through electrostatic interactions (Pearson, 1963; Gokel et al., 2004b; Sambe et al., 2021). The coordinated cations are generally located in the center of the crowns and, in some cases, sandwich structures are observed if the cation is too large to fit in the cavity (Sambe et al., 2021). Therefore, the size of the cavity of the crown ether is crucial for selective binding with different metal ions, and this parameter can be tuned by adjusting the oxygen atoms by introducing suitable substituents (Liu et al., 2019). It is known that 12- to 14-membered crown ether rings (cavity size in the range of 1.2–1.8 Å), such as 12-crown-4 (12C4, 26) (Figure 9), show the most selective preference for lithium ions (Pedersen, 1967; Olsher et al., 1991; Ali et al., 2018; Luo et al., 2018). There has been much effort to improve the selectivity of crown ethers toward coordination of the lithium cation by maintaining the overall cavity size; modification of those have also led to isotope separation of 6Li and 7Li (Cui et al., 2021). Kobiro and colleagues (Kazuya et al., 1988; Kobiro, 1996) and Buchanan et al. (1988) have already investigated the influence on extraction by modified 14-crown-4 ethers in the last century, using the dibenzo-derivative 27 and the dicyclohexyl derivative 28 (Figure 9). Furthermore, incorporation of bulky decalin units in 29 strongly enhanced the extraction power toward Li+ due to an increase in the lipophilicity of the corresponding lithium complexes in organic medium. The latter showed quantitative extraction of lithium picrate into chloroform at a receptor-to-cation ratio of 10:1, but 20% Na+ was also extracted under these conditions (Kobiro, 1996). In contrast, using the mono-substituted decalino-14-crown-4 ether 30, the Li+ extractions decreased to 81%, but the Li+/Na+ selectivity improved, as only 5% of Na+ was transferred into the organic phase. Furthermore, for the larger alkali metals K+, Rb+, and Cs+, extraction yields below 1% were observed, proving the receptor to be highly selective for Li+.
Gohil et al. (2019) synthesized the macrocyclic ionophore 31 (Figure 10), which selectively bound Li+ in the presence of other alkali and alkaline earth metal ions. Single crystals suitable for X-ray analysis confirmed that up to two metal centers are coordinated by the ligand. Competitive solid–liquid extraction experiments revealed that up to 69% of 31 was loaded by LiCl, whereas less than 5% of NaCl, KCl, MgCl2, and CaCl2 were loaded to the receptor in nitrobenzene if the salt mixtures were present in 50 times molar excess. Liquid–liquid extractions employing the same solvent showed a loading of the receptor of 26% when 1 M LiCl was used as the aqueous phase. The loading of Li+ marginally increased to 27% if NaCl, KCl, MgCl2, and CaCl2 were also present, while their loading was negligible (Gohil et al., 2019).
Calix[4]arene bearing different substituents at the lower rim (32–34; Figure 11) have been synthesized and employed for lithium ion extraction (Yaftian et al., 1997; Lukin and Vysotsky, 2001). Yaftian et al. (1997) studied the hybrid diamide-di(phosphine oxide) (32) functionalized calix[4]arenes for the recognition, extraction, and transport of alkali metal picrates. The authors found an extraction order of Li > Na > K > Rb > Cs, with no pronounced selectivity observed (65% Li+, 58% Na+, 45% K+, 20% Rb+, and 5% Cs+ extraction). Lukin and Vysotsky (2001) found that a calix[4]-arene derivative with four phosphoryl groups on the lower rim, such as diethoxyphosphoryl in 33, showed high lithium ion extraction and selectivity over other alkali metal ions.
Otho and colleagues studied p-t-octylcalix[4]arene derivatives for the extraction of lithium ions (Sadamatsu et al., 2016; Steven kurniawan et al., 2019; Kurniawan et al., 2021). A series of derivatives with propyl or acetic acid groups were therefore synthesized and investigated for the extraction of alkali metal ions (Sadamatsu et al., 2016). The authors observed that only monoacetic acid calix[4]arene 34 exhibited lithium ion selectivity over sodium ions. The same group studied 34 extensively in a microreactor system for the selective extraction of lithium ions from alkali and alkaline earth metal ions (Steven kurniawan et al., 2019). The kinetics of lithium extraction was significantly improved to 2.0 s compared to 24 h in a batch system. Recently, they developed a new concept regarding the fluid dynamics of lithium ion extraction employing 34 in T-type microreactor systems; they observed that the larger specific surface area, shorter diffusion distance, and the faster vortex velocity in the organic droplets dramatically enhanced the extraction kinetics (Kurniawan et al., 2021).
In the last few decades, a number of researchers have developed ion-pair receptors for the recognition of metal salts. Here, the receptor provides both a cation and an anion recognition site and offers the promise of strongly binding ion pairs or pairs of ions as the result of direct or indirect cooperative interactions between co-bound ions (Kim and Sessler, 2010). Mahoney et al. (2004a) studied the macro-bicyclic receptor 35 (Figure 12) for the recognition and extraction of LiCl and LiBr. While the chloride and bromide salts of sodium and potassium were isolated as contact ion pairs (Mahoney et al., 2004b), X-ray analysis of suitable single crystals of both lithium salts revealed their coordination as water-separated ion pairs. Competitive solid–liquid extraction studies showed a high selectivity of 35 for Li+ over Na+ and K+. When used as both chloride and bromide salts, more than 90% of the complex formed in the organic phase was identified as the respective Li+ complex, which was attributed by the authors to the unusually high solubility of the associated lithium ion pairs in organic solvents (Mahoney et al., 2004a).
FIGURE 12. Ion-pair receptors for lithium salt extraction, with a schematic drawing of their recognition of the metal ion and the anion in 35 and 36.
The Sessler group synthesized calix[4]pyrrole with hemisphere band (36) that functions as a lithium salt ion pair receptor; they demonstrated that this receptor can achieve high selectivity for lithium salts over the corresponding sodium and potassium salts under solid–liquid and liquid–liquid conditions (He et al., 2016). They also synthesized 37 and 38 and further enriched the library of ditopic ion-pair receptors that are capable of extracting LiCl from NaCl and KCl mixtures under solid–liquid and liquid–liquid extraction conditions (He et al., 2018). In solid–liquid extraction experiments, good selectivity of LiCl over NaCl and KCl was obtained for both receptors. In liquid–liquid extraction studies with 37, the reverse order was observed: KCl > NaCl > LiCl. In contrast, the authors observed for 38 no evidence of co-extraction of NaCl and KCl when LiCl was extracted; they concluded that there was pronounced selectivity toward LiCl. However, a drawback is that very high concentrations of LiCl (10 M LiCl) were required in the aqueous phase, and only 15% loading of the receptor was observed.
Further to this, Hong et al. (2020) synthesized triazole-bearing strapped calix[4]pyrrole 39 and studied its interaction with LiCl by 1H NMR and X-ray crystallography. The latter confirmed that the anion was bound to the calix[4]pyrrole unit through multiple hydrogen bonds. However, the crystal structure exhibited binding of Li+ to triazole nitrogen and the coordination of water. Nevertheless, a series of liquid–liquid and solid–liquid extraction studies demonstrated the successful transfer of LiCl into the organic phase. In addition, the authors did not detect any changes when comparative studies were performed with a mixture of LiCl, NaCl, and KCl; they concluded that NaCl and KCl were not co-extracted. This observation was confirmed in experiments with bromide salts. Again, the formation of host guest complexes was observed only for LiBr (Hong et al., 2020).
Based on lead work using calix[4]arenes as receptors for the extraction of lithium ions (Yaftian et al., 1997; Lukin and Vysotsky, 2001; Sadamatsu et al., 2016; Steven kurniawan et al., 2019; Kurniawan et al., 2021), the heteroditopic system 40 (Figure 13) was also developed (Kim et al., 2022b). The amidoindole groups on the upper rim provided H-bond donors for the interaction with the anion, while ether O-donor were provided in the lower rim for the coordination of the metal ion. Proton NMR studies provided evidence for the ion pair binding modes for LiCl and showed a high selectivity among the competitive alkali metal chloride salts NaCl, KCl, RbCl, and CsCl. Crystallographic analyses of the LiCl complex revealed that the lithium cation was coordinated with the carbonyl oxygen atoms of the amide groups, while the chloride anion was hydrogen-bonded to the indolyl NH protons. Thus, coordination of the metal salt within the calix[4]arene cavity was not observed, even when binding occurred as a contact ion pair complex. Solid–liquid extraction studies showed that 40 can extract LiCl or LiBr into chloroform.
The Beer group synthesized a series of heteroditopic macrocyclic receptors 41–43 (Figure 14) to investigate lithium halide recognition by employing halogen (41) and chalcogen bonding (42) (Tse et al., 2021). The calculated constants for 1:1 binding of I− using 1H NMR data obtained in CDCl3/CD3CN (1:1) are of Ka = 1236 M-1 (41), 622 M-1 (42), and 121 M-1 (43), illustrating a significantly stronger contribution of the iodo-triazole motifs compared to the chalcogen bonding motives as the hydrogen bonding host. Furthermore, the studies revealed that Li+ was coordinated by the phenanthroline moiety, while binding of the anion occurred in the vicinity of the halogen bonding site. Preliminary solid–liquid extraction studies showed that this type of receptor can be used as solid–liquid extractants for solubilizing solid lithium halide salts in organic media (Tse et al., 2021).
In general, the macrocyclic receptors bearing a rigid cavity for the complexation of metal ions or ion pairs have achieved a good selectivity of lithium salts over other alkali metal salts. However, the effective extraction of Li+ in the presence of Mg2+ with a similar ionic hydration radius is rarely reported and remains difficult (Xu et al., 2021).
The highly selective recognition and separation of lithium ions from alkali and alkaline earth metal ions are of great importance. In this review, we systematically summarized receptors that are employed in liquid–liquid and solid–liquid extractions. Cation receptors, such as β-diketones, organophosphorus extractants, macrocyclic receptors, and pyrazolone- and 4-phosphoryl-pyrazolone-based ligands, are widely employed for the recognition and extraction of lithium ions from aqueous solution. The β-diketone receptors have shown good selectivity for Li+ in alkali metal ion mixtures (Li+, Na+, K+, Rb+, and Cs+), but they preferentially extract alkaline earth metal ions (Mg2+ and Ca2+) when they are present. In addition, this type of extractant generally requires basic conditions, which not only increase the consumption of base but also can lead to extractant loss. Future studies may focus on the structural modulation of the molecules, such as the introduction of hydrophobic groups. Acidic organophosphorus receptors exhibited a stronger binding affinity toward alkaline earth metal ions than lithium ions and are therefore suitable for the removal of magnesium or calcium ions. The neutral organophosphorus TBP/FeCl3 extraction system showed high Li+/Mg2+ selectivity but relies on a high concentration of chloride ions, and TBP tends to be lost during the extraction process. In the future, it will be necessary to further develop more efficient co-extraction systems to reduce the dependence on chloride ions and extractant loss. Some macrocyclic molecules have both Li+/Na+ and Li+/Mg2+ selectivity, but the synthesis is complicated. As a new type of lithium receptor, 4-phosphorylpyrazolones have a high selectivity for lithium ions and have been applied to the recovery of lithium ions from simulated acidic brines at acid pH. Nevertheless, this mini-review provides an overview of active reagents used for lithium ion extraction in recent decades, which will help researchers form a clear understanding of functional groups for lithium ion binding. This will also be further incentive for developing more advanced receptors for lithium ion recovery from both primary sources, such as brines, and end-of-life products, such as spent lithium-ion batteries.
JZ: investigation, writing–original draft, and writing–review and editing. MW: investigation, project administration, writing–original draft, and writing–review and editing. LY: writing–review and editing. RL: writing–review and editing. JW: conceptualization, funding acquisition, methodology, project administration, resources, supervision, and writing–review and editing.
The authors declare that financial support was received for the research, authorship, and/or publication of this article from the Sino-German Collaboration of the German Science foundation (Project No. WE4621/4-1/392417756), the German Federal Ministry for Economic Affairs and Climate Action (SWELL project 03ETE042C), the Ministry of Science and Technology of China (No. 2021YFC2901500) and CAS Project for Young Scientists in Basic Research (Grant No. YSBR-038).
JZ thanks the China Scholarship Council (CSC No. 201804910750) for financial support.
The authors declare that the research was conducted in the absence of any commercial or financial relationships that could be construed as a potential conflict of interest.
All claims expressed in this article are solely those of the authors and do not necessarily represent those of their affiliated organizations, or those of the publisher, the editors, and the reviewers. Any product that may be evaluated in this article, or claim that may be made by its manufacturer, is not guaranteed or endorsed by the publisher.
Ali, M., Ahmed, I., Ramirez, P., Nasir, S., Mafe, S., Niemeyer, C. M., et al. (2018). Lithium ion recognition with nanofluidic diodes through host–guest complexation in confined geometries. Anal. Chem. 90 (11), 6820–6826. doi:10.1021/acs.analchem.8b00902
Atanassova, M., and Kurteva, V. (2016). Synergism as a phenomenon in solvent extraction of 4f-elements with calixarenes. RSC Adv. 6 (14), 11303–11324. doi:10.1039/c5ra22306g
Bae, H., and Kim, Y. (2021). Technologies of lithium recycling from waste lithium ion batteries: a review. Mater. Adv. 2 (10), 3234–3250. doi:10.1039/d1ma00216c
Bai, R., Wang, J., Wang, D., Zhang, Y., and Cui, J. (2021). Selective separation of lithium from the high magnesium brine by the extraction system containing phosphate-based ionic liquids. Sep. Purif. Technol. 274, 119051. doi:10.1016/j.seppur.2021.119051
Bai, Y., Muralidharan, N., Sun, Y.-K., Passerini, S., Stanley Whittingham, M., and Belharouak, I. (2020). Energy and environmental aspects in recycling lithium-ion batteries: concept of battery identity global passport. Mater. Today 41, 304–315. doi:10.1016/j.mattod.2020.09.001
Birch, N. J. (1999). Inorganic pharmacology of lithium. Chem. Rev. 99 (9), 2659–2682. doi:10.1021/cr9804240
Buchanan, G. W., Kirby, R. A., and Charland, J. P. (1988). Stereochemistry of crown ethers and their complexes in the solid state. Carbon-13 CPMAS NMR and x-ray crystallographic studies of configurationally isomeric dicyclohexano-14-crown-4 ethers, dibenzo-14-crown-4 ether, and some lithium thiocyanate salts. J. Am. Chem. Soc. 110 (8), 2477–2483. doi:10.1021/ja00216a022
Bukowsky, H., Uhlemann, E., Gloe, K., and Mühl, P. (1992). Liquid-liquid extraction of alkaline earth and alkali metal ions with acylpyrazolones. Anal. Chim. Acta 257 (1), 105–108. doi:10.1016/0003-2670(92)80156-2
Cai, C., Hanada, T., Fajar, A. T., and Goto, M. (2021). An ionic liquid extractant dissolved in an ionic liquid diluent for selective extraction of Li(I) from salt lakes. Desalination 509, 115073. doi:10.1016/j.desal.2021.115073
Chen, W., Li, X., Chen, L., Zhou, G., Lu, Q., Huang, Y., et al. (2021). Tailoring hydrophobic deep eutectic solvent for selective lithium recovery from the mother liquor of Li2CO3. Chem. Eng. J. 420, 127648. doi:10.1016/j.cej.2020.127648
Cui, L., Jiang, K., Wang, J., Dong, K., Zhang, X., and Cheng, F. (2019). Role of ionic liquids in the efficient transfer of lithium by Cyanex 923 in solvent extraction system. AlChE J. 65 (8), e16606. doi:10.1002/aic.16606
Cui, L., Li, S., Kang, J., Yin, C., Guo, Y., He, H., et al. (2021). A novel ion-pair strategy for efficient separation of lithium isotopes using crown ethers. Sep. Purif. Technol. 274, 118989. doi:10.1016/j.seppur.2021.118989
Docker, A., Marques, I., Kuhn, H., Zhang, Z., Félix, V., and Beer, P. D. (2022). Selective potassium chloride recognition, sensing, extraction, and transport using a chalcogen-bonding heteroditopic receptor. J. Am. Chem. Soc. 144 (32), 14778–14789. doi:10.1021/jacs.2c05333
Donald, I. W., Metcalfe, B. L., and Gerrard, L. A. (2008). Interfacial reactions in glass–ceramic-to-metal seals. J. Am. Ceram. Soc. 91 (3), 715–720. doi:10.1111/j.1551-2916.2007.02024.x
Ge, Z., Hu, J., Huang, F., and Liu, S. (2009). Responsive supramolecular gels constructed by crown ether based molecular recognition. Angew. Chem. Int. Ed. 48 (10), 1798–1802. doi:10.1002/anie.200805712
Lehn, J.-M. (1998). Supramolecular Chemistry—Scope and Perspectives Molecules, Supermolecules, and Molecular Devices (Nobel Lecture). Angew. Chem. Int. Ed. Engl. 27, 89–112. doi:10.1002/anie.198800891
Gohil, H., Chatterjee, S., Yadav, S., Suresh, E., and Paital, A. R. (2019). An ionophore for high lithium loading and selective capture from brine. Inorg. Chem. 58 (11), 7209–7219. doi:10.1021/acs.inorgchem.9b00135
Gokel, G. W., Leevy, W. M., and Weber, M. E. (2004). Crown ethers: sensors for ions and molecular scaffolds for materials and biological models. Chem. Rev. 104 (5), 2723–2750. doi:10.1021/cr020080k
Gokel, G. W., Leevy, W. M., and Weber, M. E. (2004). Crown ethers: sensors for ions and molecular scaffolds for materials and biological models. Chem. Rev. 104 (5), 2723–2750. doi:10.1021/cr020080k
Gomez-Vega, J., Soto-Cruz, J. M., JuáREZ-SáNCHEZ, O., Santacruz-Ortega, H., Gálvez-Ruiz, J. C., Corona-Martínez, D. O., et al. (2022). Tritopic bis-urea receptors for anion and ion-pair recognition. ACS Omega 7 (26), 22244–22255. doi:10.1021/acsomega.2c00935
Goodenough, J. B., and Park, K.-S. (2013). The Li-ion rechargeable battery: a perspective. J. Am. Chem. Soc. 135 (4), 1167–1176. doi:10.1021/ja3091438
Guo, Y., Ying, Y. L., Mao, Y. Y., Peng, X., and Chen, B. (2016). Polystyrene sulfonate threaded through a metal-organic framework membrane for fast and selective lithium-ion separation. Angew. Chem. Int. Ed. 55 (48), 15120–15124. doi:10.1002/anie.201607329
Hanada, T., and Goto, M. (2021). Synergistic deep eutectic solvents for lithium extraction. ACS Sustain. Chem. Eng. 9 (5), 2152–2160. doi:10.1021/acssuschemeng.0c07606
Hano, T., Matsumoto, M., Ohtake, T., Egashir, N., and Hori, F. (1992). Recovery of lithium from geothermal water by solvent extraction technique. Solvent Extr. Ion. Exch. 10 (2), 195–206. doi:10.1080/07366299208918100
He, Q., Williams, N. J., Oh, J. H., Lynch, V. M., Kim, S. K., Moyer, B. A., et al. (2018). Selective solid–liquid and liquid–liquid extraction of lithium chloride using strapped calix[4]pyrroles. Angew. Chem. Int. Ed. 57 (37), 11924–11928. doi:10.1002/anie.201805127
He, Q., Zhang, Z., Brewster, J. T., Lynch, V. M., Kim, S. K., and Sessler, J. L. (2016). Hemispherand-strapped calix[4]pyrrole: an ion-pair receptor for the recognition and extraction of lithium nitrite. J. Am. Chem. Soc. 138 (31), 9779–9782. doi:10.1021/jacs.6b05713
Healy, T. V. (1968). Synergism in the solvent extraction of alkali metal ions by thenoyl trifluoracetone. J. Inorg. Nucl. Chem. 30 (4), 1025–1036. doi:10.1016/0022-1902(68)80322-7
Hong, K.-I., Kim, H., Kim, Y., Choi, M. G., and Jang, W. D. (2020). Strapped calix[4]pyrrole as a lithium salts selective receptor through separated ion-pair binding. Chem. Commun. 56 (72), 10541–10544. doi:10.1039/d0cc04809g
Hou, J., Zhang, H., Thornton, A. W., Hill, A. J., Wang, H., and Konstas, K. (2021). Lithium extraction by emerging metal–organic framework-based membranes. Adv. Funct. Mater. 31 (46), 2105991. doi:10.1002/adfm.202105991
Jensen, B. S., Meier, H., Lundquist, K., and Refn, S. (1959). The synthesis of 1-phenyl-3-methyl-4-acyl-pyrazolones-5. Acta Chem. Scand. 13 (8), 1668–1670. doi:10.3891/acta.chem.scand.13-1668
Kazuya, K., Toshitaka, H., Toshihiro, M., Kakiuchi, K., Tobe, Y., and Odaira, Y. (1988). Decalino-14-crown-4. New type of lithium ion selective ionophore. Bull. Chem. Soc. Jpn. 61 (11), 4164–4166. doi:10.1246/bcsj.61.4164
Kim, S. H., Yeon, Y., Lee, A., Lynch, V. M., He, Q., Sessler, J. L., et al. (2022). Tetraamidoindolyl calix[4]arene as a selective ion pair receptor for LiCl. Org. Chem. Front. 9, 6888–6893. doi:10.1039/d2qo01519f
Kim, S. H., Yeon, Y., Lee, A., Lynch, V. M., He, Q., Sessler, J. L., et al. (2022). Tetraamidoindolyl calix[4]arene as a selective ion pair receptor for LiCl. Org. Chem. Front. 9 (24), 6888–6893. doi:10.1039/d2qo01519f
Kim, S. K., and Sessler, J. L. (2010). Ion pair receptors. Chem. Soc. Rev. 39 (10), 3784–3809. doi:10.1039/c002694h
Kobiro, K. (1996). New class of lithium ion selective crown ethers with bulky decalin subunits. Coord. Chem. Rev. 148, 135–149. doi:10.1016/0010-8545(96)01209-x
Kumar, A., Fukuda, H., Hatton, T. A., and Lienhard, J. H. (2019). Lithium recovery from oil and gas produced water: a need for a growing energy industry. ACS Energy Lett. 4 (6), 1471–1474. doi:10.1021/acsenergylett.9b00779
Kurniawan, Y. S., Sathuluri, R. R., Ohto, K., Iwasaki, W., Kawakita, H., Morisada, S., et al. (2021). New concept for the study of the fluid dynamics of lithium extraction using calix[4]arene derivatives in T-type microreactor systems. Separations 8 (5), 70. doi:10.3390/separations8050070
Liu, T., Chen, J., Li, H., Han, Y., and Liu, M. (2022). High-efficiency removal of calcium and magnesium from lithium-concentrated solution via counter-current extraction using di-(2-ethylhexyl)phosphinic acid. ACS Sustain. Chem. Eng. 10 (2), 967–974. doi:10.1021/acssuschemeng.1c06964
Li, R., Wang, W., Wang, Y., Wei, X., Cai, Z., and Zhou, Z. (2021). Novel ionic liquid as co-extractant for selective extraction of lithium ions from salt lake brines with high Mg/Li ratio. Sep. Purif. Technol. 277, 119471. doi:10.1016/j.seppur.2021.119471
Li, Z., and Binnemans, K. (2021). Mechanism of ferric chloride facilitating efficient lithium extraction from magnesium-rich brine with tri-n-butyl phosphate. Ind. Eng. Chem. Res. 60 (23), 8538–8547. doi:10.1021/acs.iecr.1c01003
Li, Z., and Binnemans, K. (2021). Opposite selectivities of tri-n-butyl phosphate and Cyanex 923 in solvent extraction of lithium and magnesium. AlChE J. 67 (7), e17219. doi:10.1002/aic.17219
Li, Z., and Binnemans, K. (2020). Selective removal of magnesium from lithium-rich brine for lithium purification by synergic solvent extraction using β-diketones and Cyanex 923. AlChE J. 66 (7), e16246. doi:10.1002/aic.16246
Liu, G., Zhao, Z., and Ghahreman, A. (2019). Novel approaches for lithium extraction from salt-lake brines: a review. Hydrometallurgy 187, 81–100. doi:10.1016/j.hydromet.2019.05.005
Lu, J., Stevens, G. W., and Mumford, K. A. (2021). Development of heterogeneous equilibrium model for lithium solvent extraction using organophosphinic acid. Sep. Purif. Technol. 276, 119307. doi:10.1016/j.seppur.2021.119307
Lu, J., Zhang, H., Hou, J., Li, X., Hu, X., Hu, Y., et al. (2020). Efficient metal ion sieving in rectifying subnanochannels enabled by metal–organic frameworks. Nat. Mater 19 (7), 767–774. doi:10.1038/s41563-020-0634-7
Lukin, OLEG, and Vysotsky, M. O. (2001). O-Phosphorylated calix[4]arenes as Li+-selectivereceptors. J. Phys. Org. Chem. 14 (7), 468–473. doi:10.1002/poc.382
Luo, Y., Marets, N., and Kato, T. (2018). Selective lithium ion recognition in self-assembled columnar liquid crystals based on a lithium receptor. Chem. Sci. 9 (3), 608–616. doi:10.1039/c7sc03652c
Mahmoudi, S., Babakhani, A., Noori, M., Azizitorghabeh, A., and Rashchi, F. (2022). Synergistic extraction and separation of cobalt and lithium using D2EHPA and CYANEX 272. Min. Metall. Explor. 39 (2), 777–792. doi:10.1007/s42461-021-00531-7
Mahoney, J. M., Beatty, A. M., and Smith, B. D. (2004). Selective Solid−Liquid extraction of lithium halide salts using a ditopic macrobicyclic receptor. Inorg. Chem. 43 (24), 7617–7621. doi:10.1021/ic049066b
Mahoney, J. M., Nawaratna, G. U., Beatty, A. M., Duggan, P. J., and Smith, B. D. (2004). Transport of alkali halides through a liquid organic membrane containing a ditopic salt-binding receptor. Inorg. Chem. 43 (19), 5902–5907. doi:10.1021/ic0494859
Marchetti, F., Pettinari, C., and Pettinari, R. (2005). Acylpyrazolone ligands: synthesis, structures, metal coordination chemistry and applications. Coord. Chem. Rev. 249 (24), 2909–2945. doi:10.1016/j.ccr.2005.03.013
Marchetti, F., Pettinari, R., and Pettinari, C. (2015). Recent advances in acylpyrazolone metal complexes and their potential applications. Coord. Chem. Rev. 303, 1–31. doi:10.1016/j.ccr.2015.05.003
Masmoudi, A., Zante, G., TréBOUET, D., Barillon, R., and Boltoeva, M. (2021). Solvent extraction of lithium ions using benzoyltrifluoroacetone in new solvents. Sep. Purif. Technol. 255, 117653. doi:10.1016/j.seppur.2020.117653
Matt, D., Lakkis, D., Grandjean, D., Balegroune, F., and Laidoudi, A. (1992). Structure of tris (4-diphenylphosphinoyl-3-methyl-1-phenylpyrazol-5-onato-O, O') iron(III). Acta Crystallogr. Sect. C. Cryst. Struct. Commun. 48 (8), 1408–1411. doi:10.1107/s0108270192000374
Meng, X., Long, Y., Tian, Y., Li, W., Liu, T., and Huo, S. (2021). Electro-membrane extraction of lithium with D2EHPA/TBP compound extractant. Hydrometallurgy 202, 105615. doi:10.1016/j.hydromet.2021.105615
Mjos, K. D., and Orvig, C. (2014). Metallodrugs in medicinal inorganic chemistry. Chem. Rev. 114 (8), 4540–4563. doi:10.1021/cr400460s
Munasinghe, V. K., Pancholi, J., Manawadu, D., Zhang, Z., and Beer, P. D. (2022). Mechanical bond enhanced lithium halide ion-pair binding by halogen bonding heteroditopic rotaxanes. Chem. Eur. J. 28, e202201209. doi:10.1002/chem.202201209
Nguyen, T. H., and Lee, M. S. (2018). A review on the separation of lithium ion from leach liquors of primary and secondary resources by solvent extraction with commercial extractants. Processes 6 (5), 55. doi:10.3390/pr6050055
Nisola, G. M., Parohinog, K. J., Cho, M. K., Burnea, F. K. B., Lee, J. Y., Seo, J. G., et al. (2020). Covalently decorated crown ethers on magnetic graphene oxides as bi-functional adsorbents with tailorable ion recognition properties for selective metal ion capture in water. Chem. Eng. J. 389, 123421. doi:10.1016/j.cej.2019.123421
Olea, F., DuráN, G., DíAZ, G., Villarroel, E., Araya-López, C., Cabezas, R., et al. (2022). Ionic liquids for the selective solvent extraction of lithium from aqueous solutions: a theoretical selection using COSMO-RS. Minerals 12 (2), 190. doi:10.3390/min12020190
Oliveira, L., Messagie, M., Rangaraju, S., Sanfelix, J., Hernandez Rivas, M., and Van Mierlo, J. (2015). Key issues of lithium-ion batteries – from resource depletion to environmental performance indicators. J. Clean. Prod. 108, 354–362. doi:10.1016/j.jclepro.2015.06.021
Olsher, U., Izatt, R. M., Bradshaw, J. S., and Dalley, N. K. (1991). Coordination chemistry of lithium ion: a crystal and molecular structure review. Chem. Rev. 91 (2), 137–164. doi:10.1021/cr00002a003
Parikh, V. P., Ahmadi, A., Parekh, M. H., Sadeghi, F., and Pol, V. G. (2019). Upcycling of spent lithium cobalt oxide cathodes from discarded lithium-ion batteries as solid lubricant additive. Environ. Sci. Technol. 53 (7), 3757–3763. doi:10.1021/acs.est.8b07016
Pearson, R. G. (1963). Hard and soft acids and bases. J. Am. Chem. Soc. 85 (22), 3533–3539. doi:10.1021/ja00905a001
Pedersen, C. J. (1967). Cyclic polyethers and their complexes with metal salts. J. Am. Chem. Soc. 89 (26), 2495–2496. doi:10.1021/ja00986a052
Pranolo, Y., Zhu, Z., and Cheng, C. Y. (2015). Separation of lithium from sodium in chloride solutions using SSX systems with LIX 54 and Cyanex 923. Hydrometallurgy 154, 33–39. doi:10.1016/j.hydromet.2015.01.009
Razmjou, A., Asadnia, M., Hosseini, E., Habibnejad Korayem, A., and Chen, V. (2019). Design principles of ion selective nanostructured membranes for the extraction of lithium ions. Nat. Commun. 10 (1), 5793. doi:10.1038/s41467-019-13648-7
Sadamatsu, H., Hanada, T., Morisada, S., Kawakita, H., and Ohto, K. (2016). Comprehensive comparison of alkali metal extraction with a series of calix[4]arene derivatives with propyl and/or acetic acid groups. J. Incl. Phenom. Macrocycl. Chem. 84 (1), 87–97. doi:10.1007/s10847-015-0586-8
Sambe, K., Hoshino, N., Takeda, T., Nakamura, T., and Akutagawa, T. (2021). Crystal structures and physical properties of (M+)(Crown Ethers)x(TCNQ)y salts with M+ = Li+, Na+, K+, Rb+, and Cs+. Cryst. Growth Des. 21 (10), 5928–5942. doi:10.1021/acs.cgd.1c00812
Shi, C., Jing, Y., Xiao, J., Wang, X., and Jia, Y. (2017). Liquid-liquid extraction of lithium using novel phosphonium ionic liquid as an extractant. Hydrometallurgy 169, 314–320. doi:10.1016/j.hydromet.2017.02.015
Shi, C., Jing, Y., Xiao, J., Wang, X., Yao, Y., and Jia, Y. (2017). Solvent extraction of lithium from aqueous solution using non-fluorinated functionalized ionic liquids as extraction agents. Sep. Purif. Technol. 172, 473–479. doi:10.1016/j.seppur.2016.08.034
Shi, D., Cui, B., Li, L., Peng, X., Zhang, L., and Zhang, Y. (2019). Lithium extraction from low-grade salt lake brine with ultrahigh Mg/Li ratio using TBP – kerosene – FeCl3 system. Sep. Purif. Technol. 211, 303–309. doi:10.1016/j.seppur.2018.09.087
Shi, D., Cui, B., Li, L., Peng, X., Zhang, L., and Zhang, Y. (2019). Lithium extraction from low-grade salt lake brine with ultrahigh Mg/Li ratio using TBP–kerosene–FeCl3 system. Sep. Purif. Technol. 211, 303–309. doi:10.1016/j.seppur.2018.09.087
Shi, D., Cui, B., Li, L., Xu, M., Zhang, Y., Peng, X., et al. (2020). Removal of calcium and magnesium from lithium concentrated solution by solvent extraction method using D2EHPA. Desalination 479, 114306. doi:10.1016/j.desal.2019.114306
Song, Y., Zhao, Z., and He, L. (2020). Lithium recovery from Li3PO4 leaching liquor: solvent extraction mechanism of saponified D2EHPA system. Sep. Purif. Technol. 249, 117161. doi:10.1016/j.seppur.2020.117161
Sonoc, A., Jeswiet, J., and Soo, V. K. (2015). Opportunities to improve recycling of automotive lithium ion batteries. Procedia CIRP 29, 752–757. doi:10.1016/j.procir.2015.02.039
Steven Kurniawan, Y., Rao Sathuluri, R., Ohto, K., Iwasaki, W., Kawakita, H., Morisada, S., et al. (2019). A rapid and efficient lithium-ion recovery from seawater with tripropyl-monoacetic acid calix[4]arene derivative employing droplet-based microreactor system. Sep. Purif. Technol. 211, 925–934. doi:10.1016/j.seppur.2018.10.049
Su, H., Li, Z., Zhang, J., Liu, W., Zhu, Z., Wang, L., et al. (2020). Combining selective extraction and easy stripping of lithium using a ternary synergistic solvent extraction system through regulation of Fe3+ coordination. ACS Sustain. Chem. Eng. 8 (4), 1971–1979. doi:10.1021/acssuschemeng.9b06432
Su, H., Li, Z., Zhang, J., Zhu, Z., Wang, L., and Qi, T. (2020). Recovery of lithium from salt lake brine using a mixed ternary solvent extraction system consisting of TBP, FeCl3 and P507. Hydrometallurgy 197, 105487. doi:10.1016/j.hydromet.2020.105487
Su, H., Li, Z., Zhu, Z., Wang, L., and Qi, T. (2020). Extraction relationship of Li+ and H+ using tributyl phosphate in the presence of Fe(III). Sep. Sci. Technol. 55 (9), 1677–1685. doi:10.1080/01496395.2019.1604759
Su, H., Tan, B., Zhang, J., Liu, W., Wang, L., Wang, Y., et al. (2022). Modelling of lithium extraction with TBP/P507–FeCl3 system from salt-lake brine. Sep. Purif. Technol. 282, 120110. doi:10.1016/j.seppur.2021.120110
Sun, Q., Chen, H., and Yu, J. (2022). Investigation on the lithium extraction process with the TBP–FeCl3 solvent system using experimental and DFT methods. Ind. Eng. Chem. Res. 61 (13), 4672–4682. doi:10.1021/acs.iecr.1c05072
Sun, X., Luo, H., and Dai, S. (2012). Ionic liquids-based extraction: a promising strategy for the advanced nuclear fuel cycle. Chem. Rev. 112 (4), 2100–2128. doi:10.1021/cr200193x
Swain, B., Jeong, J., Yoo, K., and Lee, J. c. (2010). Synergistic separation of Co(II)/Li(I) for the recycling of LIB industry wastes by supported liquid membrane using Cyanex 272 and DR-8R. Hydrometallurgy 101 (1-2), 20–27. doi:10.1016/j.hydromet.2009.11.012
Swain, B. (2017). Recovery and recycling of lithium: a review. Sep. Purif. Technol. 172, 388–403. doi:10.1016/j.seppur.2016.08.031
Swain, B. (2016). Separation and purification of lithium by solvent extraction and supported liquid membrane, analysis of their mechanism: a review. J. Chem. Technol. Biotechnol. 91 (10), 2549–2562. doi:10.1002/jctb.4976
Tagne Kuate, A. C., Iovkova, L., Hiller, W., Schürmann, M., and Jurkschat, K. (2010). Organotin-substituted [13]-Crown-4 ethers: ditopic receptors for lithium and cesium halides. Organometallics 29 (21), 5456–5471. doi:10.1021/om100409v
Taydakov, I. V., Belousov, Y. A., Lyssenko, K. A., Varaksina, E., Drozdov, A. A., Marchetti, F., et al. (2020). Synthesis, phosphorescence and luminescence properties of novel europium and gadolinium tris-acylpyrazolonate complexes. Inorg. Chim. Acta 502, 119279. doi:10.1016/j.ica.2019.119279
Tse, Y. C., Docker, A., Zhang, Z., and Beer, P. D. (2021). Lithium halide ion-pair recognition with halogen bonding and chalcogen bonding heteroditopic macrocycles. Chem. Commun. 57 (40), 4950–4953. doi:10.1039/d1cc01287h
Virolainen, S., Fini, M. F., Laitinen, A., and Sainio, T. (2017). Solvent extraction fractionation of Li-ion battery leachate containing Li, Ni, and Co. Sep. Purif. Technol. 179, 274–282. doi:10.1016/j.seppur.2017.02.010
Wang, J., Yang, S., Bai, R., Chen, Y., and Zhang, S. (2019). Lithium recovery from the mother liquor obtained in the process of Li2CO3 production. Ind. Eng. Chem. Res. 58 (3), 1363–1372. doi:10.1021/acs.iecr.8b05495
Wang, J., Yang, S., Zhang, X., Wang, Y., Wang, D., Li, W., et al. (2020). Extraction mechanism of lithium from the alkali solution with diketonate-based ionic liquid extractants. Energy Fuels 34 (9), 11581–11589. doi:10.1021/acs.energyfuels.0c02346
Wang, S., Zheng, S., Wang, Z., Cui, W., Zhang, H., Yang, L., et al. (2018). Superior lithium adsorption and required magnetic separation behavior of iron-doped lithium ion-sieves. Chem. Eng. J. 332, 160–168. doi:10.1016/j.cej.2017.09.055
Wang, Y., Liu, H., Fan, J., Liu, X., Hu, Y., Hu, Y., et al. (2019). Recovery of lithium ions from salt lake brine with a high magnesium/lithium ratio using heteropolyacid ionic liquid. ACS Sustain. Chem. Eng. 7 (3), 3062–3072. doi:10.1021/acssuschemeng.8b04694
Xu, P., Hong, J., Qian, X., Xu, Z., Xia, H., Tao, X., et al. (2021). Materials for lithium recovery from salt lake brine. J. Mater. Sci. 56 (1), 16–63. doi:10.1007/s10853-020-05019-1
Yaftian, M. R., Burgard, M., Matt, D., Wieser, C., and Dieleman, C. (1997). Multifunctional calix[4]arenes containing pendant amide and phosphoryl groups: their use as extracting agents and carriers for alkali cations. J. Incl. Phenom. Mol. Recognit. Chem. 27 (2), 127–140. doi:10.1023/a:1007975928021
Yan, F., Chen, F., Wu, X.-H., Luo, J., Zhou, X. S., Horsley, J. R., et al. (2020). Unique metal cation recognition via crown ether-derivatized oligo(phenyleneethynylene) molecular junction. J. Phy. Chem. C 124 (16), 8496–8503. doi:10.1021/acs.jpcc.9b11908
Yang, J. H., Kim, J., Hay, B. P., Lee, K., and Kim, S. K. (2022). Tris (pyridin-2-ylmethyl) amine-based ion pair receptors for selective lithium salt recognition. Eur. J. Org. Chem. 2022 (30), e202200808. doi:10.1002/ejoc.202200808
Yu, X., Cui, J., Liu, C., Yuan, F., Guo, Y., and Deng, T. (2021). Separation of magnesium from high Mg/Li ratio brine by extraction with an organic system containing ionic liquid. Chem. Eng. Sci. 229, 116019. doi:10.1016/j.ces.2020.116019
Zhang, J., Liu, Y., Liu, W., Wang, L., Chen, J., Zhu, Z., et al. (2021). Mechanism study on the synergistic effect and emulsification formation of phosphine oxide with β-diketone for lithium extraction from alkaline systems. Sep. Purif. Technol. 279, 119648. doi:10.1016/j.seppur.2021.119648
Zhang, J., Tanjedrew, N., Wenzel, M., Royla, P., Du, H., Kiatisevi, S., et al. (2023). Selective separation of lithium, magnesium and calcium using 4-phosphoryl pyrazolones as pH-regulated receptors. Angew. Chem. Int. Ed. 62 (13), e202216011. doi:10.1002/anie.202216011
Zhang, J., Wenzel, M., Steup, J., Schaper, G., Hennersdorf, F., Du, H., et al. (2022). 4-Phosphoryl pyrazolones for highly selective lithium separation from alkali metal ions. Chem. Eur. J. 28 (1), e202103640. doi:10.1002/chem.202103640
Zhang, L., Ji, L., Li, L., Shi, D., Xu, T., Peng, X., et al. (2021). Recovery of Co, Ni, and Li from solutions by solvent extraction with β-diketone system. Hydrometallurgy 204, 105718. doi:10.1016/j.hydromet.2021.105718
Zhang, L., Li, J., Ji, L., et al. (2021). Separation of lithium from alkaline solutions with hydrophobic deep eutectic solvents based on β-diketone. J. Mol. Liq. 344, 117729. doi:10.1016/j.molliq.2021.117729
Zhang, L., Li, J., Liu, R., Zhou, Y., Zhang, Y., Ji, L., et al. (2022). Recovery of lithium from salt lake brine with high Na/Li ratio using solvent extraction. J. Mol. Liq. 362, 119667. doi:10.1016/j.molliq.2022.119667
Zhang, L., Li, L., Rui, H., Shi, D., Peng, X., Ji, L., et al. (2020). Lithium recovery from effluent of spent lithium battery recycling process using solvent extraction. J. Hazard. Mater. 398, 122840. doi:10.1016/j.jhazmat.2020.122840
Zhang, L., Li, L., Shi, D., Peng, X., Song, F., Nie, F., et al. (2018). Recovery of lithium from alkaline brine by solvent extraction with β-diketone. Hydrometallurgy 175, 35–42. doi:10.1016/j.hydromet.2017.10.029
Zhou, W., Li, Z., and Xu, S. (2021). Extraction of lithium from magnesium-rich solution using tri-n-butyl phosphate and sodium hexafluorophosphate. J. Sustain. Metall. 7 (3), 1368–1378. doi:10.1007/s40831-021-00430-7
Zhou, Z., Fan, J., Liu, X., Hu, Y., Wei, X., Hu, Y., et al. (2020). Recovery of lithium from salt-lake brines using solvent extraction with TBP as extractant and FeCl3 as co-extraction agent. Hydrometallurgy 191, 105244. doi:10.1016/j.hydromet.2019.105244
Zhou, Z., Qin, W., Liang, S., Tan, Y., and Fei, W. (2012). Recovery of lithium using tributyl phosphate in methyl isobutyl ketone and FeCl3. Ind. Eng. Chem. Res. 51 (39), 12926–12932. doi:10.1021/ie3015236
Keywords: liquid–liquid extraction, lithium, receptor, ionic liquids, separation
Citation: Zhang J, Wenzel M, Yang L, Luckay RC and Weigand JJ (2023) Receptors for the recognition and extraction of lithium. Front. Chem. Eng. 5:1271045. doi: 10.3389/fceng.2023.1271045
Received: 01 August 2023; Accepted: 13 October 2023;
Published: 30 November 2023.
Edited by:
Sophie Charton, Commissariat à l’Energie Atomique et aux Energies Alternatives (CEA), FranceReviewed by:
Leigh Martin, Oak Ridge National Laboratory (DOE), United StatesCopyright © 2023 Zhang, Wenzel, Yang, Luckay and Weigand. This is an open-access article distributed under the terms of the Creative Commons Attribution License (CC BY). The use, distribution or reproduction in other forums is permitted, provided the original author(s) and the copyright owner(s) are credited and that the original publication in this journal is cited, in accordance with accepted academic practice. No use, distribution or reproduction is permitted which does not comply with these terms.
*Correspondence: Jan J. Weigand, amFuLndlaWdhbmRAdHUtZHJlc2Rlbi5kZQ==
Disclaimer: All claims expressed in this article are solely those of the authors and do not necessarily represent those of their affiliated organizations, or those of the publisher, the editors and the reviewers. Any product that may be evaluated in this article or claim that may be made by its manufacturer is not guaranteed or endorsed by the publisher.
Research integrity at Frontiers
Learn more about the work of our research integrity team to safeguard the quality of each article we publish.