- 1Institute of Bio- and Geosciences, IBG-2: Plant Sciences, Forschungszentrum Jülich GmbH, Jülich, Germany
- 2RWTH Aachen University, Aachen, Germany
Agricultural residues such as rapeseed straw can be a valuable source of cellulose, sugars, and aromatic molecules like lignin. Understanding its composition is crucial in order to develop suitable processing technology for the production of biofuel or biochemicals from rapeseed straw. Here, we developed a small-scale OrganoCat system to screen multiple technical conditions and different samples at higher throughput and utilize this system to analyze straw samples from a set of 14 genetically different Brassica lines on their processability. Correlation analysis was performed to investigate the effects of cell wall polymer features on rapeseed biomass disintegration. At comparably mild reaction conditions, the differences in recalcitrance towards OrganoCat fractionation within the set were especially associated with parameters such as pectic polysaccharide content, acetylation, and hemicellulose composition. These findings can subsequently be used to optimize and scale up the pretreatment and fractionation of lignocellulose derived from rapeseed straw.
1 Introduction
The use of agricultural residues as raw material to produce chemicals, materials, and fuels displays a promising alternative to conventional routes derived from non-renewable resources such as petroleum. Different agricultural wastes, such as wheat straw (Ruiz et al., 2013), rice straw (Satlewal et al., 2018), corn stover (Zhu et al., 2009), flax shives (Parsons et al., 2013), sugarcane bagasse (Vieira et al., 2020), empty fruit bunches (Grande et al., 2019), pineapple fibers (Banerjee et al., 2022) among others, have been investigated in the last decades as sources for fuels, chemical, and material production.
Rapeseed (Brassica napus) has traditionally been grown to produce animal feed and vegetable oil for human consumption. But in the last decades, an increasing fraction of rapeseed oil has been used as raw material for biodiesel production (Díaz et al., 2010). The production of rapeseed oil also generates significant amounts of rapeseed straw. These woody stems can have a diameter of 1–4 cm and a height of approximately 1.5 m. The straw is not suitable for cattle feed and therefore often left in the field to return nutrients to the soil (Svärd et al., 2015). Brassica species have very different cell wall structures compared to monocot plants (Yokoyama and Nishitani, 2004), and within these species, considerable genotypic variation exists affecting cell wall phenotype and therefore the lignocellulose composition (Wood et al., 2015). In the context of processing lignocellulose towards chemicals, materials, and fuels these variations will likely influence process efficiency and yields (Wood et al., 2015).
Pretreatment is an essential step in processes for the utilization of lignocellulose because the recalcitrant nature of the substrate forms a physicochemical barrier towards further conversion steps (Svärd et al., 2015). To improve the accessibility for subsequent conversion, different pretreatment methods have been proposed. Rapeseed straw has been processed by different technologies, such as steam explosion (Wood et al., 2015; Deng et al., 2020), hydrothermal (Díaz et al., 2010; Wang et al., 2016), alkali (Svärd et al., 2015), and acid treatments (Jeong and Oh, 2011).
Recently, efforts moved towards technologies that can enable a full valorization of lignocellulose thereby increasing the economic feasibility and reducing the ecological footprint (Chandel et al., 2018). Organosolv-like technologies are such examples. Among these, biphasic pretreatments using diluted acids are very promising, combining efficacy and low degradation due to their mild reaction conditions and in situ extraction of lignin from the reactive diluted acid phase (Sakdaronnarong et al., 2016; Sakdaronnarong et al., 2018). The OrganoCat capitalizes on such a biphasic system. In contrast to other biphasic approaches, the OrganoCat process uses a biogenic solvent and catalyst. The process conditions are selected to allow for a compromise between the quality and quantity of the fractionated components. This way, lignocellulose can be fractionated while sugar degradation to undesirable humins is kept to a minimum, lignin keeps more of its native structural features, and the process is still economically feasible (Grande et al., 2015). The mild reaction conditions (125°–160°C, 1–3 h) hydrolyze the amorphous non-cellulosic polysaccharides, and lignin is extracted into 2-methyltetrahydrofuran (2-MTHF). Water, solvent, and catalyst can be recycled comparably easily (Grande et al., 2015).
The aim of this study is, to establish a small-scale OrganoCat pretreatment and fractionation system which is capable of a medium throughput screening of different technical conditions and different samples of variable structure and composition. The established system then is further used to screen a set of different rapeseed accessions and evaluate their performance in the OrganoCat pre-treatment and fractionation.
2 Materials and methods
2.1 Materials
If not indicated otherwise, chemicals were purchased from Carl Roth and Sigma-Aldrich (Germany) and used without further purification.
The 14 Rapeseed accessions (Supplement) were selected from a collection of the Pre-Breed Yield project (Pflanzenforschung, de, 2022) and exhibit high genotypic and phenotypic diversity. Selected accessions were grown on a field site at 50°54′33.5″N 6°24′48.5″E near the Institute for Bio and Geosciences of the Forschungszentrum Jülich during the summer term of 2018. They were harvested at the fully ripened growth stage and 20 cm long stem segments directly below the branching segments were selected. The material was dried for 7 days at 85°C to constant weight.
For the establishment of the small-scale process system, beechwood material (RÄUCHERGOLD®, JRS, Germany) and straw from a commercial oilseed line was used, which was grown at two different locations near the Forschungszentrum Jülich and is referred to below as Rapeseed 1 or 2, depending on the location.
All biomass material was milled using a laboratory hammer mill M 400 (30 s−1, 2 min, Retsch, Germany) to a particle size smaller than 1 cm, homogenized in a single lot, and stored at room temperature until further use.
2.2 Microscale OrganoCat system
The standard OrganoCat fractionation consists of a suspension of 500 mg biomass in 5 mL aqueous phase (.1 M oxalic acid) and 5 mL organic phase (2-MTHF), placed in a stainless-steel reactor. The reactor is pressurized with 10bar of argon and heated up to 140°C, maintaining this temperature for 3 h with a rotation speed of the stirring plate magnet set to 1,500 rpm. After cooling and depressurizing the reactor, the resulting phases are placed in a 50 mL Falcon tube (Sigma-Aldrich, Germany) and centrifuged for 5 min at 14,000 rpm for better phase separation. Then, the organic phase is separated using a syringe, and 2-MTHF is evaporated to recover the lignin fraction. The aqueous phase is filtered and stored at −4°C for further analysis. The remaining pulp is washed with distilled water until neutral pH is reached and air dried until constant weight (vom Stein et al., 2011).
Based on this procedure, the microscale was assessed for establishing a medium throughput system. Borosilicate glass vials (4 mL, 45.0 × 14.5 mm, CS-Chromatographie Service, Germany) were loaded with 100 mg of biomass. Then, 1 mL aqueous .1 M oxalic acid and 1 mL 2-MTHF and a micro-magnetic stirring bar (L 6 mm, Carl Roth, Germany) were added. The vials were tightly closed with a top-silicone septum screw polypropylene cap (CS-Chromatographie Service, Germany). A set of 10 vials per batch was placed into an oil bath at indicated conditions (125°C for 6 h/140°C for 3 h reaction time). The rotation speed of the stirring plate magnet used during the reaction time was set to 550 rpm.
Success rates were estimated for validation of the microscale system and were defined as the number of reactions completed after the indicated reaction time over the total reactions tested, in percentage.
Once established, microscale OrganoCat screening was conducted in rapeseed accessions in triplicates. Temperature and reaction time were varied to cover the range from non-optimal to optimal conditions. The mildest set of conditions applied was 125°C, 1 h reaction time, followed by 125°C for 3 h and 140°C for 1 h. The harshest set of conditions, previously established as optimal for OrganoCat processing, was 140°C for 3 h (Weidener et al., 2020).
2.3 Lignocellulose analysis
The analyses were carried out in triplicates. Wet chemical analysis was performed as previously described (Jablonowski et al., 2017; Grande et al., 2019). In brief, alcohol-insoluble residues were prepared (AIR) by extraction once with ethanol (70%, v/v), three times with chloroform: methanol solution (1:1, v/v), and once with acetone. Then, starch was removed from the remaining pellet by enzymatic digestion with amyloglucosidase and α-amylase (Megazyme, Ireland). The remaining de-starched AIR (dAIR) was washed with water and acetone, 3 times respectively, and left to dry at room temperature. All analyses were conducted with dAIR. Crystalline cellulose (CrC) content was determined by removing non-cellulosic material with Updegraff reagent and hydrolyzing the residue with sulphuric acid (72%, v/v). The remaining carbohydrate was measured by anthrone spectrophotometric essay. Total acetate content was determined by saponifying dAIR biomass with 0.5 M NaOH for 1 h and neutralizing it with 1M HCl. Total acetate content was then determined enzymatically using a kit (acetic acid kit K-ACETRM, Megazyme, Ireland). Lignin was determined using the Acetyl bromide (AcBr) soluble lignin method. First acetyl bromide solution (25%, v/v) was added and left shaking for 3 h at 50°C. Then, 2 M NaOH, 0.5 M hydroxylammonium chloride, and glacial acetic acid were added. AcBr-soluble lignin was determined spectrophotometrically using Kraft lignin as an external standard. Non-cellulosic polysaccharides were determined by hydrolysis with trifluoroacetic acid (TFA), and then high-performance anion-exchange chromatography with pulsed amperometric detection (HPAEC-PAD).
2.4 Analysis of OrganoCat product streams
After the reaction has been completed, the glass vials were cooled to room temperature. The phases were transferred to a 2 mL Eppendorf tube and centrifuge for 7 min at max. rpm for better differentiation. Given the small concentrations handled, only 500 µL of the organic phase (containing 2-MTHF and lignin) was taken, and lignin was isolated by rotating evaporation (Heidolph Instruments GmbH & CO. KG, Germany). The remaining supernatant was separated from the pulp by centrifuging twice for 5 min at 14,000 rpm. This aqueous phase was stored at −4°C for further analysis. The pulp was washed with distilled water and centrifuged for 5 min at max rpm until neutral pH. Subsequently, it was left to dry at 65°C until constant weight.
Pulp and lignin yields were obtained gravimetrically. Pulp yield was calculated from the ratio of dried pulp and initial biomass weight.
For total lignin yield, a dilution factor of 2-MTHF was estimated, as a small fraction of the organic solvent might be lost or dissolved in the aqueous phase and considering sample method with .5 mL taken from whole organic phase (Supplementary Table S1).
Monosaccharide composition in the hydrolysate was determined by high-performance anion-exchange chromatography with pulsed amperometric detection (HPAEC-PAD). Sugar yield was referred to as the difference between the total amount of monosaccharides in untreated rapeseed (TFA fraction) and the total composition in the hydrolysate of pretreated biomass.
Further pulp analysis was conducted as described in Section 2.4, starting with pulp material instead of dAIR. Cellulose enrichment (CE) of the Pulp was determined based on the difference in CrC content between untreated and pretreated biomass.
2.5 Statistical analysis
Pearson correlations (p > .05) between untreated and treated parameters were made. Normality was not assumed, and the analysis of variance for non-parametric data (Kruskal Wallis test, p > .05) was performed to know the effect of OrganoCat processing between rapeseed accessions, as well as among the several OrganoCat conditions. A heat map was built based on the normalized data of CE, sugar yield, and lignin yield (from the organic phase). A min-max normalization equation was used, where X is the mean value, Xmin is the minimum value and Xmax is the maximum value of a data set.
A Principal Component Analysis (PCA) was conducted using all obtained biochemical parameters (Supplementary Table S6). The statistical analyses were made by SPSS IBM SPSS Statistics v25.0 (2017).
3 Results and discussion
3.1 Establishment of a small-scale OrganoCat system
To implement a system that allows higher throughput and handling smaller samples than the standard reactor system (Grande et al., 2015) a small-scale setup (named M-L) capable of processing multiple samples and less sample amount at the same time was established (Figure 1). The same parameters used for standard reactor OrganoCat were performed for this system (100 g/L biomass loading, 1:1 ratio of aqueous phase/organic phase, .1 M oxalic acid, 2-MTHF as organic phase). For the initial establishment of this system, milled beechwood material was used. To select the proper setup for further experiments, microscale system was tested at optimal OrganoCat conditions, as previously defined (Grande et al., 2015): 125°C for 6 h reaction time (Supplementary Table S2) and 140°C for 3 h. In the microscale system, 77.8% of reactions succeeded (100 mg biomass, 2 mL .1 M oxalic acid (aq.):2-MTHF (1:1, vol:vol)) at 125°C 6 h and 95.8% at 140°C 3 h. Given that the probability of failure in this system is less than 5%, the microscale system at 140°C 3 h was used for further analyses with rapeseed genotypes.
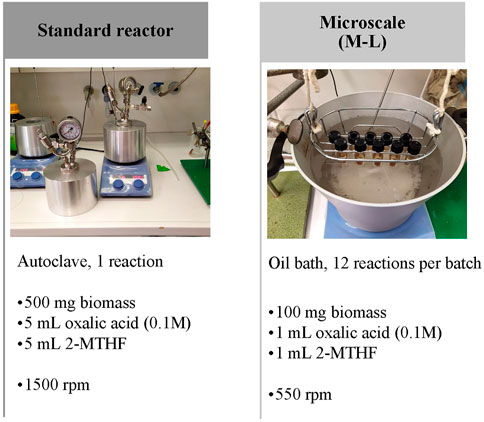
FIGURE 1. OrganoCat standard reactor system and and small systems design. Biomass loading 100 g/L, 1:1, vol:vol, oxalic acid:2-MTHF.
The M-L system then was evaluated concerning the yield (Pulp and lignin yield) and the obtained pulp characteristics (CrC, AcBr lignin, and acetyl groups) at the two before-mentioned conditions. These results were compared with those obtained by the standard reactor system at 140°C for 3 h (500 mg biomass loading, 5 mL aqueous phase) to validate it according to this setup. Similar pulp yields were found, varying from 54.1% to 55.2% of initial biomass in all systems (standard reactor and M-L at both conditions). A slightly lower lignin yield was exhibited in M-L at 125°C 6 h (Supplementary Table S2). An enrichment of cellulose in the pulp was achieved after OrganoCat processing at all processing conditions which was demonstrated by similar values for CrC, AcBr lignin, and acetyl groups in all three systems. For further experiments, 140°C for 3 h of reaction time was selected as the setting for M-L (Supplementary Table S2).
The established setup was then transferred to rapeseed material to test its robustness towards a different type of biomass. The M-L setup was used to process two different samples of rapeseed straw from a commercial oilseed line harvested from two different locations (referred to as Rapeseed 1 and 2) and again validated against the standard reactor mode (Figure 2). The pulp and lignin yields were similar between the different modalities. An enriched-cellulose pulp was produced after OrganoCat processing and slightly lower AcBr lignin content in M-L (Supplementary Table S3). These findings also demonstrated the effectiveness of the small system under the selected conditions. This setup was further used to investigate whether phenotypic differences in the cell walls of 14 rapeseed lines lead to differences in processing performance.
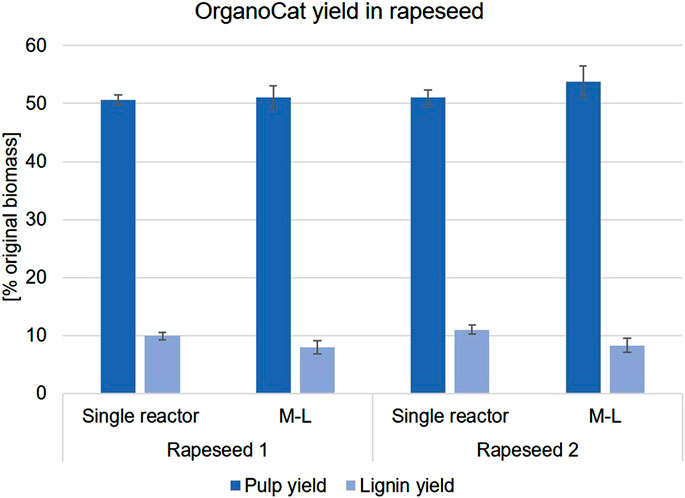
FIGURE 2. Pulp and lignin yield of Rapeseed 1 and 2 in a standard reactor and M-L modalities. Both systems were performed at 140°C for 3 h. For the standard reactor (n = 10), pulp yield was obtained by filter method, whereas pulp yield from the microscale system (n = 19) was obtained by drying method. Lignin yield for both systems was obtained by evaporating the organic phase and weighing the remaining solid.
3.2 Chemical characterization of cell wall features in the rapeseed accessions
Straw samples of 14 rapeseed lines were characterized by their biochemical cell wall features. The determined features were crystalline cellulose content (CrC), acetyl groups, acetyl-bromide lignin (AcBr lignin), and total sugars (from TFA fraction) in the percentage of mg/mg of de-starched Alcohol Insoluble Residue (dAIR) biomass. A full data set of the compositional details is given in the supplement (Supplementary Tables S4, S5).
Among the different accessions, crystalline cellulose varied from 38.32% to 46.70%, being the major component of the cell wall. The lowest CrC content was found in vivo I and the highest in Savannah. Acetyl groups varied between 2.01% and 4.29%, where the lowest content was found in Chousenshu and the highest in Savannah. AcBr lignin ranged between 28.84% (None) and 36.50% (Cobra). Interestingly over all lines, a high acetyl group content correlated with low lignin values (Table 1). As for the hemicellulose fraction, here defined as the sugars solubilized by TFA, the total sugar content ranged from 21.49% (Cobra) to 35.89% (Chousenshu). The glucose and xylose ratios were calculated based on the total content of polysaccharides in the TFA fraction. It was shown that pentose (C5) sugars were slightly more predominant than hexoses (C6), where xylose is the most prominent sugar, contributing by approximately half of the total sugar composition in the TFA hydrolysate, as already described in previous studies (Damm et al., 2017; Weidener et al., 2020). Followed by glucose and in only minor amounts the other sugars. Mannose could not be detected in our analytical setup since it was not possible to discriminate it from xylose in the ion chromatography. The glucose ratio negatively correlated with the xylose ratio (Pearson = −.92, p < .05). Abukama Natane had the highest C5: C6 content, whereas Rapid had the lowest. The biochemical characterization here reflects in general the results of previous studies that characterize Brassica species (Wood et al., 2015; Pei et al., 2016).
To sort the data and identify similarities between the lines based on their cell wall composition, a principal component analysis was performed (Figure 3) using a set of 12 normalized parameters (Supplementary Table S6). Figure 3A depicts the scores plot on the first three components explaining 61.2% of the variance in the panel. Figure 3B shows the loading plots of the parameters used with their share of each parameter in components C1, C2, and C3. For C1 and C2, the most relevant parameters were derived from polysaccharide matrix components. C1′s most important feature was glucose content in the TFA fraction followed by arabinose and fucose. In C2 the total content of sugars and xylose, as the most prominent sugar in this fraction were the dominant factors. The third component C3 is dominated by CrC, followed by acetyl groups and glucuronic acid as the most important factors. Interestingly, the lignin content represented by AcBr soluble lignin was not a prominent feature to discriminate between the lines. The full data set of the analysis is given in the supplement (Supplementary Table S5).
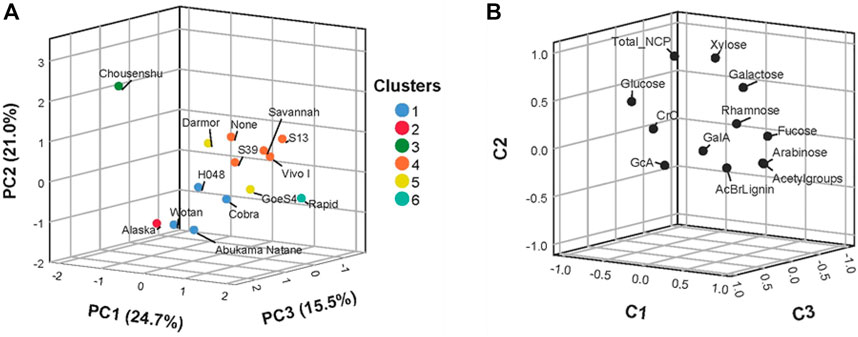
FIGURE 3. Discriminant analysis of the different rapeseed accessions. (A) Scores plot on the first three components. (B) Loadings plot on the first three components. Principle component analysis was performed with 12 variables (normalized data of mean values) measured in nine replicates for each accession.
To further group the rapeseed lines according to their cell wall characterization, hierarchical clustering was performed revealing three main clusters. The first one includes Abukama Natane, Cobra, H048, and Wotan. Whereas Vivo I, S13, Savannah, S39, and None comprised the second cluster. The third cluster is formed by Darmor and GoeS4. Three lines, namely Chousenshu, Alaska, and Rapid were not part of any cluster and appeared as individuals.
The overall detected variance within the set was not very high. The performed analyses gave an impression of the phenotypic differences with respect to the cell wall composition. In the following experiments, it was investigated whether these phenotypically different lines perform differently in a lignocellulose pretreatment and fractionation approach using the established small-scale OrganoCat system.
3.3 OrganoCat processing of rapeseed straw
Following the pretreatment reaction of the 14 rapeseed lines, the mean cellulose enrichment of the pulp, the sugar, and the lignin yield was determined for each line. In Figure 4 these results depict the susceptibility to the pretreatment for different reaction conditions. Cellulose Enrichment (CE) shows a relative increase in cellulose content in the pulp compared to the untreated biomass. Sugar yield indicates the amount of sugars that had been hydrolyzed during the reaction and lignin yield is referred to as the amount of solid isolated from the organic phase (Supplementary Table S7).
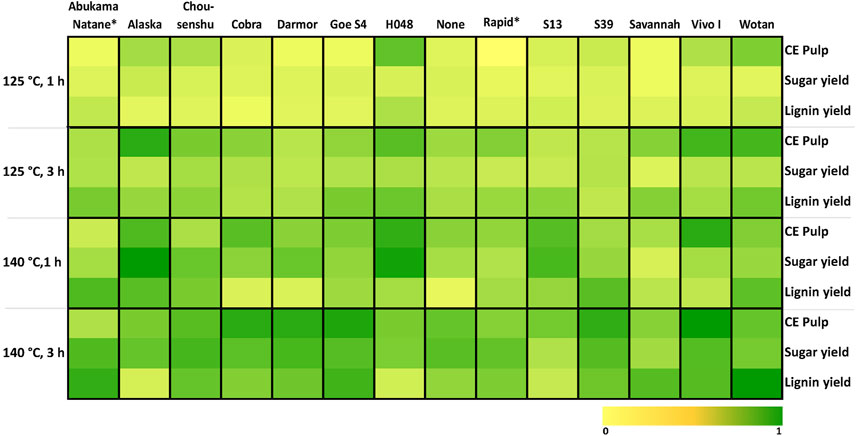
FIGURE 4. Heatmap of OrganoCat processability for 14 rapeseed lines under different conditions. Screening was conducted with the small-scale system design (M-L). Normalized data of cellulose enrichment in pulp, and sugar and lignin yield for color gradient. CE Pulp is the increase in the relative amount of cellulose in the pulp compared to the raw material. Sugar yield is defined as the amount of reducing sugars determined in the aqueous phase after pretreatment. Lignin yield is defined as the amount of lignin isolated from the organic phase after pretreatment. *The calculation of the values for CE Pulp at 125°C 1 h for Abukama Natane and Rapid resulted in negative numbers, thus these values were set to 0 for simplification.
Differences in processability by the pretreatment were observed as the conditions gradually changed. Generally, milder OrganoCat conditions led to lower extraction yields, whereas more severe conditions increased their yields but depending on the rapeseed line differences in the effectiveness of the pretreatment could be observed. H048, Vivo I, Wotan, and Alaska seemed to be more prone to the OrganoCat treatment exhibiting higher product yields even at lower severities. Darmor, S39, and Savannah exhibited at the highest severity considerable product yields. Overall, hydrolyzed sugars noticeably incremented over increasing severity, indicating a greater pretreatment effect. Earlier studies already described that removing hemicellulose is one of the most important steps in rapeseed pretreatment and would result in improved further conversions (Wood et al., 2015; Pei et al., 2016). Therefore, conditions resulting in very high sugar yield suggest a complete valorization of rapeseed biomass.
To investigate in more detail the effect of the OrganoCat pretreatment on the residual pulp, six lines were chosen because of their contrasting performance at different reaction conditions. Abukama Natane, Cobra, and Savannah were chosen because they appeared to be more recalcitrant, Alaska, Rapid, and Vivo I to be more prone towards OrganoCat.
3.4 Biochemical characterization of OrganoCat pulps
The six selected lines were analyzed for CrC, AcBr lignin, acetyl groups, and total sugar content (TFA fraction). All features were determined for four different severities of OrganoCat pretreatment (Figure 5, Supplementary Table S8). In Figure 5A, there is a clear trend of increasing CrC content from untreated material to OrganoCat pretreatment. The largest cellulose enrichment is exhibited in vivo I, increasing from 38.3 wt. % in untreated material to 61.4 wt. % in the OrganoCat pulp material at the harshest condition, and in Cobra from 46.1 wt. % in untreated material to 65.5 wt. % in the pulp. Meanwhile, Abukama Natane exhibits the lowest cellulose enrichment, going from 42.3 wt. % in untreated material up to 49.1 wt. % in the pulp. However, after 125°C, 3 h the CrC content in the pulp remains nearly the same. A similar trend is observed for Rapid and Savannah after OrganoCat pretreatment at 125°C, 3 h. This may be explained by the presence of sugars derived from hemicellulose (Figure 5D) that might hinder the enrichment of cellulose. This circumstance has already been described for diluted acid or hydrothermal pretreatment of rapeseed material (Pei et al., 2016; Svärd, Brännvall and Edlund, 2017; Deng et al., 2020). Both lines present a higher hemicellulose content (TFA fraction) compared to the other lines. Alaska exhibited the highest CrC content already at medium severity being more or less constant thereafter.
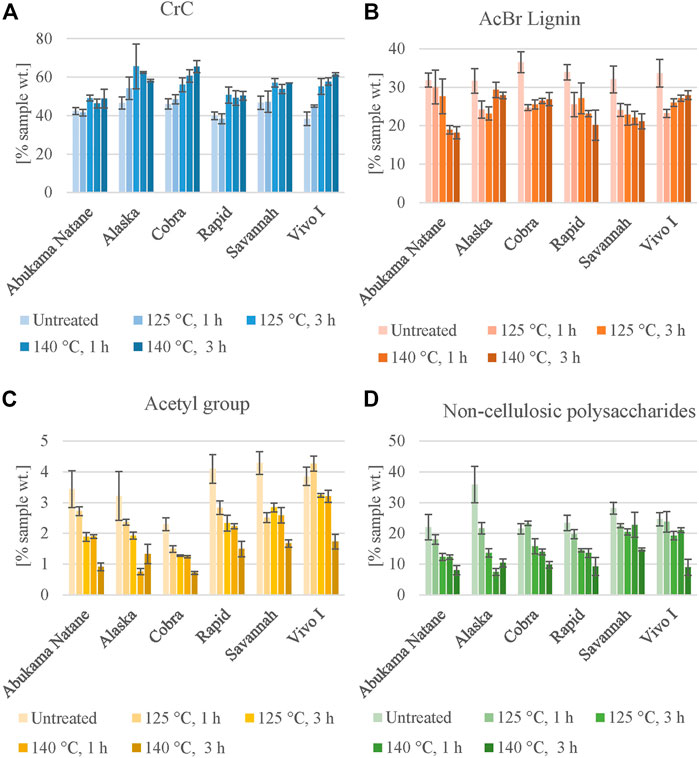
FIGURE 5. Changes in cell wall composition during OrganoCat pretreatment. Initial biomass is compared with pulps produced by different severities. Six selected lines were characterized biochemically (A) crystalline cellulose content (Seaman) (B) acetyl bromide lignin (C) acetyl groups o; (D) residual non-cellulosic polysaccharides of the pulp. Values are expressed as means ± standard deviation; n = 3.
The de-lignification process in the selected accessions is displayed in Figure 5B, where a decrease in AcBr lignin content for all lines after pretreatment is shown. Delignification in the pulp is not as pronounced as cellulose enrichment, especially between the different OrganoCat conditions, confirming that lignin removal is difficult from rapeseed biomass (Jeong and Oh, 2011; Pei et al., 2016; Deng et al., 2020). A progressive decline in AcBr lignin content in the pulp is observed in Rapid and Savannah, as well as in Abukama Natane. However, the latter presents almost no change at milder conditions compared to untreated material, but there is a considerable decrease at harsher conditions (140°C, 1 h, and 140°C, 3 h), underlining that at higher severities the removal of lignin from the pulp is more efficient. Cobra and Vivo I, which have the highest enrichment in cellulose, now exhibit almost no change in their lignin content at different severities, going from 24.7 to 23.2 wt% at mildest conditions to 26.9 and 27.9 wt% at harshest conditions, respectively. Alaska exhibits the lowest decrease in AcBr lignin content, displaying a more pronounced decrease at milder conditions but almost no change at harsher conditions. Therefore, Alaska may be an interesting candidate for OrganoCat-based processing at mild reaction conditions and to be studied in more detail in future experiments.
Acetyl groups after pretreatment are determined as the degree of O-acetylation, related to hemicellulose depolymerization (Weidener et al., 2020). In accordance, there is a clear diminishment of acetyl group content in all accessions after pretreatment (Figure 5C). The highest de-acetylation is found in Rapid, decreasing from 4.1 wt% in untreated material to 1.5 wt%, and in vivo I from 3.9 wt% to 1.7 wt%. Cobra has the lowest de-acetylation process.
Total sugars from hemicellulose (TFA fraction) of all accessions follow a similar pattern as de-acetylation (Figure 5D). A diminishment in total sugar content is exhibited. These findings are supported by previous studies (Grande et al., 2019; Weidener et al., 2020).
In total (Figure 6), there is a clear cellulose enrichment in the pulp throughout all conditions, but statistically significant differences (p < .05) between untreated biomass and OrganoCat pulp are only observed at higher severities. The de-lignification process seemed to be less prominent under the applied reaction conditions. This has also been described in other studies (Wood et al., 2015; Deng et al., 2020). Similarly, the de-acetylation process essentially happened under harsher conditions. There are only significant differences (p < .05) between untreated and the harshest conditions. The decrease in total sugar content (TFA fraction) and therefore hemicellulose depolymerization was the most prominent change but also mechanistically the most important one during the pretreatment. The removal of non-cellulosic sugars enables cellulose enrichment and to some extent lignin extraction. But the obtained results also demonstrate that further refinement of the pulp in terms of purity for subsequent conversion steps is needed (Orts et al., 2005; Jeong and Oh, 2011; Zhang, 2018; Sharma et al., 2019; Deng et al., 2020).
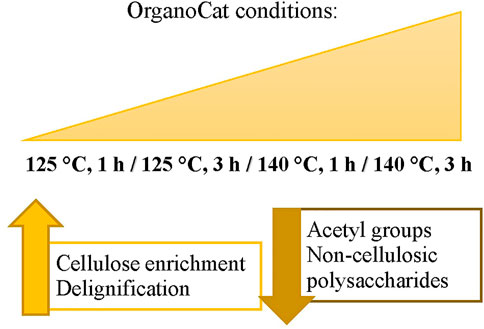
FIGURE 6. Diagram showing tendencies of pulp characterization features on selected rapeseed accessions when increasing OrganoCat severity.
3.5 Correlation analysis
Overall, we identified trends based on the pulp characterization of selected rapeseed accessions (Figure 7). Pearson correlations were built to test for any relation between chemical features in untreated biomass. Likewise, correlations were made for chemical features in the pulp, at the mildest and harshest conditions. Positive correlations are depicted in red circles, and negative in blue. The size of the circle reflects the value of the correlation factor R. However, most correlations exhibited p-values greater than .05.
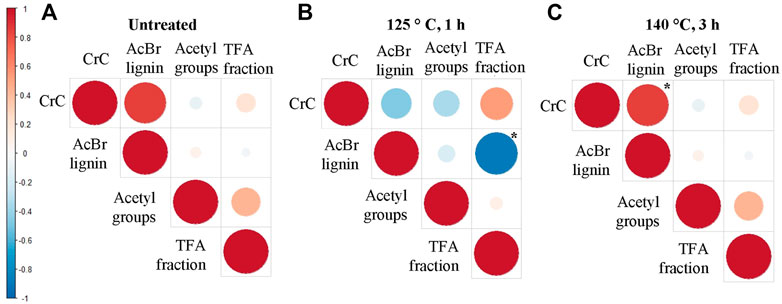
FIGURE 7. Correlation analysis of the biochemical features obtained from (A) initial and pretreated rapeseed straw at (B) low and (C) high severity. Pearson correlation factors were calculated for CrC, acetyl groups, AcBr-Lignin, and total sugar content of the initial biomass and pulp biomass yield at low (125°C, 1 h) and high severity (140°C, 3 h). Positive correlations are depicted in red circles, and negative in blue. The size of the circle reflects the value of the correlation factor r. * Statistically significant (p < .05).
In untreated biomass (Figure 7A), CrC and sugars in the Non-cellulosic polysaccharides (TFA fraction) exhibited a positive correlation. Given that at higher CrC content in the biomass, the higher sugar content of the hemicellulose fractions is found. Whereas acetyl groups negatively correlated with CrC and AcBr lignin. After biomass was subdued to OrganoCat pretreatment at the mildest conditions (Figure 7B), the most correlation between the measured feature showed the same tendency. A significant correlation (p < .05) was found between AcBr lignin and monosaccharide content (TFA fraction). In pulp obtained by the harshest pretreatment (Figure 7C), these correlations weakened. Only for CrC and AcBr lignin, a strong positive correlation was detected (p < .05).
From this overall perspective, we observed a shift in the correlations of the chemical features before and after pretreatment. Chemical features in the pulp at mildest conditions exhibit a similar pattern of correlations as untreated biomass. Whereas, at the harshest conditions, correlations become almost null except for CrC and AcBr lignin. This shift may indicate that at milder OrganoCat severity structural changes are more important and the removal of hemicellulose and lignin seems to be more even. On the contrary, at greater severity, the measured features of the pulp became less relevant and OrganoCat pretreatment could more effectively overcome the recalcitrance of the different substrates.
However, deeper analysis is needed to gain a detailed understanding of the chemical features affecting pulp production by OrganoCat. The multimodal analysis here demonstrated the importance of hemicellulosic factors during rapeseed pretreatment. OrganoCat processing extracts lignin into the organic phase. Therefore, pulp de-lignification is expected, and could be observed in all reactions, but chemical and correlation analysis pointed out it was a less prominent factor under the chosen conditions. It has to be stated that the variance of the lignin content in the examined rapeseed set was not high enough. It has also been described that lignin composition (Guaiacyl content) is more important for diluted acid pretreatment of rapeseed than simple lignin content (Pei et al., 2016).
4 Conclusion
A Small scale OrganoCat system was successfully established. This system is capable to screen multiple small-sized biomass samples at different reaction conditions for efficient fractionation. Using this system, a set of genetically different rapeseed lines was processed. The lines Vivo I and Alaska were identified as very suitable lines for OrganoCat pretreatment, based on their yields and pulp characterization. Polysaccharide features are more important at milder conditions while OrganoCat conditions played a more important role at harsher severity.
Data availability statement
The original contributions presented in the study are included in the article/Supplementary Material, further inquiries can be directed to the corresponding author.
Author contributions
JMD: Methodology, Experiment conduction; data evaluation; Writing—original draft, Visualization. PG: Methodology, Writing—review and editing, Supervision, HK: Conceptualization, Writing—review and editing, Supervision, Funding acquisition.
Funding
This work was performed as part of the Bioeconomy Science Center (BioSC), supported by the project PREDIG. The scientific activities of the Bioeconomy Science Center were financially supported by the Ministry of Innovation, Science, and Research within the framework of the NRW Strategieprojekt BioSC (no. 313/323-400-00213).
Acknowledgments
The authors would like to thank Dr. Kerstin Nagel, Beate Uhlig, Lucy Harrison, Michelle Reutemann, and Vanessa Ploeger for contributing to the harvest and material preparation and the wet chemical analysis of the straw.
Conflict of interest
The authors declare that the research was conducted in the absence of any commercial or financial relationships that could be construed as a potential conflict of interest.
Publisher’s note
All claims expressed in this article are solely those of the authors and do not necessarily represent those of their affiliated organizations, or those of the publisher, the editors and the reviewers. Any product that may be evaluated in this article, or claim that may be made by its manufacturer, is not guaranteed or endorsed by the publisher.
Supplementary material
The Supplementary Material for this article can be found online at: https://www.frontiersin.org/articles/10.3389/fceng.2023.1098411/full#supplementary-material
References
Banerjee, S., Vijayaraghavan, R., Patti, A. F., and Arora, A. (2022). Integrated biorefinery strategy for valorization of pineapple processing waste into high-value products. Waste Biomass Valorization 13 (1), 631–643. doi:10.1007/s12649-021-01542-7
Chandel, A. K., Garlapati, V. K., Singh, A. K., Antunes, F. A. F., and da Silva, S. S. (2018). The path forward for lignocellulose biorefineries: Bottlenecks, solutions, and perspective on commercialization. Bioresour. Technol. 264, 370–381. doi:10.1016/j.biortech.2018.06.004
Damm, T., Grande, P. M., Jablonowski, N. D., Thiele, B., Disko, U., Mann, U., et al. (2017). OrganoCat pretreatment of perennial plants: Synergies between a biogenic fractionation and valuable feedstocks. Bioresour. Technol. 244, 889–896. doi:10.1016/j.biortech.2017.08.027
Pflanzenforschung, de (2022). PRE-BREED yield. https://www.pflanzenforschung.de/de/forschung-plant-2030/projekte/82/detail.
Deng, J., Zhu, X., Chen, P., He, B., Tang, S. w., Zhao, W., et al. (2020). Mechanism of lignocellulose modification and enzyme disadsorption for complete biomass saccharification to maximize bioethanol yield in rapeseed stalks’. Sustain. Energy Fuels 4 (2), 607–618. doi:10.1039/c9se00906j
Díaz, M. J., Cara, C., Ruiz, E., Romero, I., Moya, M., and Castro, E. (2010). Hydrothermal pre-treatment of rapeseed straw. Bioresour. Technol. 101 (7), 2428–2435. doi:10.1016/j.biortech.2009.10.085
Grande, P. M., Viell, J., Theyssen, N., Marquardt, W., Dominguez de Maria, P., and Leitner, W. (2015). Fractionation of lignocellulosic biomass using the OrganoCat process. R. Soc. Chem. 17 (6), 3533–3539. doi:10.1039/c4gc02534b
Grande, P. M., Weidener, D., Dietrich, S., Dama, M., Bellof, M., Maas, R., et al. (2019). OrganoCat fractionation of empty fruit bunches from palm trees into lignin, sugars, and cellulose-enriched pulp. ACS Omega. Am. Chem. Soc. 4, 14451–14457. doi:10.1021/acsomega.9b01371
Jablonowski, N. D., Kollmann, T., Nabel, M., Damm, T., Klose, H., Muller, M., et al. (2017). Valorization of Sida (Sida hermaphrodita) biomass for multiple energy purposes. GCB Bioenergy 9 (1), 202–214. doi:10.1111/gcbb.12346
Jeong, T. S., and Oh, K. K. (2011). Optimization of fermentable sugar production from rape straw through hydrothermal acid pretreatment. Bioresour. Technol. 102 (19), 9261–9266. doi:10.1016/j.biortech.2011.06.092
Orts, W. J., Shey, J., Imam, S. H., Glenn, G. M., Guttman, M. E., and Revol, J. F. (2005). Application of cellulose microfibrils in polymer nanocomposites. J. Polym. Environ. 13 (4), 301–306. doi:10.1007/s10924-005-5514-3
Parsons, R. V., Cenkowski, S., Sorensen, J. L., Beta, T., and Arntfield, S. D. (2013). Hemicellulose polysaccharide recovery from flax shive using alkaline solutions with sodium ethoxide pretreatment. Industrial Crops Prod. 44, 165–170. doi:10.1016/j.indcrop.2012.11.023
Pei, Y., Li, Y., Zhang, Y., Yu, C., Fu, T., Zou, J., et al. (2016). G-lignin and hemicellulosic monosaccharides distinctively affect biomass digestibility in rapeseed. Bioresour. Technol. 203, 325–333. doi:10.1016/j.biortech.2015.12.072
Ruiz, H. A., Cerqueira, M. A., Silva, H. D., Rodriguez-Jasso, R. M., Vicente, A. A., and Teixeira, J. A. (2013). Biorefinery valorization of autohydrolysis wheat straw hemicellulose to be applied in a polymer-blend film. Carbohydr. Polym. 92 (2), 2154–2162. doi:10.1016/j.carbpol.2012.11.054
Sakdaronnarong, C., Pipathworapoom, W., Vichitsrikamol, T., Sema, T., Posoknistakul, P., Koo-amornpattana, W., et al. (2018). Integrative process for a sugarcane bagasse biorefinery to produce glucose, bio-oil and carbon microspheres. Process Saf. Environ. Prot. 116, 1–13. doi:10.1016/j.psep.2018.01.006
Sakdaronnarong, C., Saengsawang, A., Siriyutta, A., Jonglertjunya, W., Nasongkla, N., and Laosiripojana, N. (2016). An integrated system for fractionation and hydrolysis of sugarcane bagasse using heterogeneous catalysts in aqueous biphasic system. Chem. Eng. J. 285, 144–156. doi:10.1016/j.cej.2015.09.098
Satlewal, A., Agrawal, R., Bhagia, S., Das, P., and Ragauskas, A. J. (2018). Rice straw as a feedstock for biofuels: Availability, recalcitrance, and chemical properties. Biofuels, Bioprod. Biorefining 12 (1), 83–107. doi:10.1002/bbb.1818
Sharma, A., Thakur, M., Bhattacharya, M., Mandal, T., and Goswami, S. (2019). Commercial application of cellulose nano-composites – a review. Biotechnol. Rep. 21, e00316. doi:10.1016/j.btre.2019.e00316
Svärd, A., Brännvall, E., and Edlund, U. (2015). Rapeseed straw as a renewable source of hemicelluloses: Extraction, characterization and film formation. Carbohydr. Polym. 133, 179–186. doi:10.1016/j.carbpol.2015.07.023
Svärd, A., Brännvall, E., and Edlund, U. (2017). Rapeseed straw polymeric hemicelluloses obtained by extraction methods based on severity factor. Industrial Crops Prod. 95, 305–315. doi:10.1016/j.indcrop.2016.10.038
Vieira, S., Barros, M. V., Sydney, A. C. N., Piekarski, C. M., de Francisco, A. C., Vandenberghe, L. P. d. S., et al. (2020). Sustainability of sugarcane lignocellulosic biomass pretreatment for the production of bioethanol. Bioresour. Technol. 299, 122635. doi:10.1016/j.biortech.2019.122635
vomStein, T., Grande, P. M., Kayser, H., Sibilla, F., Leitner, W., and Dominguez de Maria, P. (2011). From biomass to feedstock: One-step fractionation of lignocellulose components by the selective organic acid-catalyzed depolymerization of hemicellulose in a biphasic system. R. Soc. Chem. 13 (7), 1772–1777. doi:10.1039/c1gc00002k
Wang, Z. W., Zhu, M. Q., Li, M. F., Wang, J. Q., Wei, Q., and Sun, R. C. (2016). Comprehensive evaluation of the liquid fraction during the hydrothermal treatment of rapeseed straw. Biotechnol. Biofuels 9 (1), 142. doi:10.1186/s13068-016-0552-8
Weidener, D., Dama, M., Dietrich, S. K., Ohrem, B., Pauly, M., Leitner, W., et al. (2020). Multiscale analysis of lignocellulose recalcitrance towards OrganoCat pretreatment and fractionation. Biotechnol. Biofuels 13 (1), 155. doi:10.1186/s13068-020-01796-8
Wood, I. P., Wellner, N., Elliston, A., Wilson, D. R., Bancroft, I., and Waldron, K. W. (2015). Effect of Brassica napus cultivar on cellulosic ethanol yield. Biotechnol. Biofuels 8 (1), 99. doi:10.1186/s13068-015-0278-z
Yokoyama, R., and Nishitani, K. (2004). Genomic basis for cell-wall diversity in plants. A comparative approach to gene families in rice and arabidopsis. Plant Cell. Physiology 45 (9), 1111–1121. doi:10.1093/pcp/pch151
Zhang, S., Chen, C., Duan, C., Hu, H., Li, H., Li, J., et al. (2018). Regenerated cellulose by the Lyocell process, a brief review of the process and properties. BioResources 13 (2), 4577–4592. doi:10.15376/biores.13.2.zhang
Zhu, Z., Sathitsuksanoh, N., Vinzant, T., Schell, D. J., McMillan, J. D., and Zhang, Y. H. P. (2009). Comparative study of corn stover pretreated by dilute acid and cellulose solvent-based lignocellulose fractionation: Enzymatic hydrolysis, supramolecular structure, and substrate accessibility. Biotechnol. Bioeng. 103 (4), 715–724. doi:10.1002/bit.22307
Keywords: lignocellulose, pretreatment, rapeseed, hemicellulose, cellulose
Citation: Martinez Diaz J, Grande PM and Klose H (2023) Small-scale OrganoCat processing to screen rapeseed straw for efficient lignocellulose fractionation. Front. Chem. Eng. 5:1098411. doi: 10.3389/fceng.2023.1098411
Received: 14 November 2022; Accepted: 04 January 2023;
Published: 16 January 2023.
Edited by:
Chang Geun Yoo, SUNY College of Environmental Science and Forestry, United StatesReviewed by:
Sasikumar Elumalai, Center of Innovative and Applied Bioprocessing (CIAB), IndiaYunxuan Wang, The University of Tennessee, United States
Copyright © 2023 Martinez Diaz, Grande and Klose. This is an open-access article distributed under the terms of the Creative Commons Attribution License (CC BY). The use, distribution or reproduction in other forums is permitted, provided the original author(s) and the copyright owner(s) are credited and that the original publication in this journal is cited, in accordance with accepted academic practice. No use, distribution or reproduction is permitted which does not comply with these terms.
*Correspondence: Holger Klose, aC5rbG9zZUBmei1qdWVsaWNoLmRl