- 1Department of Chemical Engineering, Herbert Wertheim College of Engineering, University of Florida, Gainesville, FL, United States
- 2College of Medicine, University of Florida, Gainesville, FL, United States
The fields of drug and gene delivery have been revolutionized by the discovery and characterization of polymer-based materials. Polymeric nanomaterials have emerged as a strategy for targeted delivery because of features such as their impressive biocompatibility and improved availability. Use of naturally derived polymers in these nanomaterials is advantageous due to their biodegradability and bioresorption. Natural biopolymer-based particles composed of silk fibroins and other silk fiber-inspired proteins have been the focus of research in drug delivery systems due to their simple synthesis, tunable characteristics, and ability to respond to stimuli. Several silk and silk-inspired polymers contain a high proportion of reactive side groups, allowing for functionalization and addition of targeting moieties. In this review, we discuss the main classes of silk and silk-inspired polymers that are being used in the creation of nanomaterials. We also focus on the fabrication techniques used in generating a tunable design space of silk-based polymeric nanomaterials and detail how that translates into use for drug delivery to several distinct microenvironments.
Introduction
The efficacy of treatment for numerous diseases and conditions including, but not limited to, cancers, autoimmune disorders, inflammation, and infection, is limited by how therapeutic agents and drugs are delivered. Systemically administered drugs suffer from low water solubility, rapid clearance, and can cause a variety of off-target side effects (Jain, 2020). Administering drugs via alternative routes is an area of interest, but overcoming the body’s natural barrier systems is a significant challenge. To address the limitations associated with systemic delivery, research focused on advanced drug delivery systems (DDS) has been a major area of interest (Hrkach and Langer, 2020). Nanomaterial-based DDS, such as nanoparticles, liposomes, and dendrimers, have been shown to enhance the bioavailability of various therapeutics (Blanco et al., 2015; Brannon et al., 2022). A major class of nanomaterial DDS are polymeric nanomaterials engineered to include features such as targeting moieties (Chen et al., 2021a). Using natural biopolymers for the creation of these DDS is further advantageous due to their impressive biocompatibility and biodegradability (George et al., 2019). The main classes of natural biopolymers used for the synthesis of nanoparticle-based systems are polysaccharides and proteins.
Silk fibroins and other silk fiber-inspired proteins have been shown to be biocompatible and easily digested by immune cells and proteolytic enzymes into products that can re-enter native metabolic processes on a tunable timeline (Guo et al., 2020; Jameson et al., 2021). Apart from their biocompatibility and tunable biodegradation, silk proteins are also advantageous polymers for the engineering of DDS due to the myriad of methods to induce increases in crystalline content. This translates into numerous methods for engineering nanomaterials of various sizes, depending on the end state application, that do not require the use of harsh solvents (Pham and Tiyaboonchai, 2020). Increased crystalline content also limits the tendency for burst release behavior (Wongpinyochit et al., 2019; Pham and Tiyaboonchai, 2020). The main silk proteins that have been explored for use in a variety of nanoparticle DDS are silk fibroins from the Bombyx mori silkworm. Bombyx mori silk fibroin is the most well-characterized silk protein source and has a variety of reactive amino acids to allow for functionalization (Murphy et al., 2008; Murphy and Kaplan, 2009; Asakura et al., 2015; Heichel and Burke, 2020; Bittencourt et al., 2022). Other silk proteins, including Bombyx mori sericin, genetically engineered silk fibroin proteins, silk fibers from other Antheraea species, and bioengineered spider fibroin silks (Bittencourt et al., 2022), are being explored due to their enhanced ability for functionalization, potential for a wider range of material and mechanical properties, and increased homogeneity of the starting polymer. In this review, we discuss the primary structure of these silk proteins and relate the structure to fabrication strategies for nanomaterials (Figure 1). We also highlight progress in the in vitro and in vivo evaluations of silk-based DDS.
Fabrication and evaluation of nanomaterial DDS based on silk proteins and silk fiber-inspired polymers
Bombyx mori fibroin
Bombyx mori silk is composed of two main protein groups, fibroin and sericin. Two fibroin fibers are surrounded by a sericin coating. When fibroin and sericin are present together, there is decreased biocompatibility (Ode Boni et al., 2022). Sericin is water soluble, allowing for fibroin to be readily extracted (Rockwood et al., 2011). Historically, sericin has been discarded as waste, but we discuss the potential for sericin nanomaterials in a later section.
Fibroin from Bombyx mori is biocompatible and the focus of biomedical research for applications including nanomaterials and both local and systemic DDS. Fibroin consists of heavy (∼325 kDa) and light (∼26 kDa) chains linked with a disulfide bond (Zhou et al., 2001). The light chain is elastic, hydrophilic, and characterized by nonrepeating sequences of amino acids. The heavy chain comprises highly hydrophobic and crystalline motifs (GAGAGS or GAGAGX, X = V or Y) separated by amorphous regions containing polar and charged amino acids (Zhou et al., 2001). The hydrophobic motifs can form layers of beta-sheet structures, thereby altering the crystallinity and mechanical properties of fibroin-based materials. The level of crystallinity varies with the material fabrication methods and can be further increased using post-processing techniques such as water annealing (Hu et al., 2011; Rockwood et al., 2011; Jameson et al., 2021). Fibroin nanoparticles have also been shown to have stimuli-responsive behavior, with increased degradation and drug release occurring as a function of stimuli such as pH and the presence of reactive oxygen species, making them advantageous for delivery of therapies like chemotherapeutics (Seib et al., 2013; Rezaei et al., 2020; Chen et al., 2021b).
To form nanomaterials with silk fibroin, researchers have developed an array of physical and chemical methods to induce the hydrophobic collapse of the hydrophobic motifs and encourage the formation of water insoluble nanoparticles (Murphy et al., 2008; Murphy and Kaplan, 2009; Wenk et al., 2010; Heichel and Burke, 2020; Hong et al., 2020). Recent studies have utilized techniques such as spray drying (Fuster et al., 2021), organic solvent desolvation (Hasanzadeh et al., 2020; Rezaei et al., 2020; Zahedi et al., 2021), salting out (Song et al., 2019), and microfluidic devices (Shimanovich et al., 2017; Matthew et al., 2020; Vargas Montoya et al., 2020; Matthew et al., 2022; Tomeh et al., 2022) (Table 1). Control of particle size and material properties to enhance cell material interaction (Xiao et al., 2022) and to optimize non-systemic delivery routes has been established (Takeuchi et al., 2019; Yang et al., 2019; Chung et al., 2022; Mitra et al., 2022).
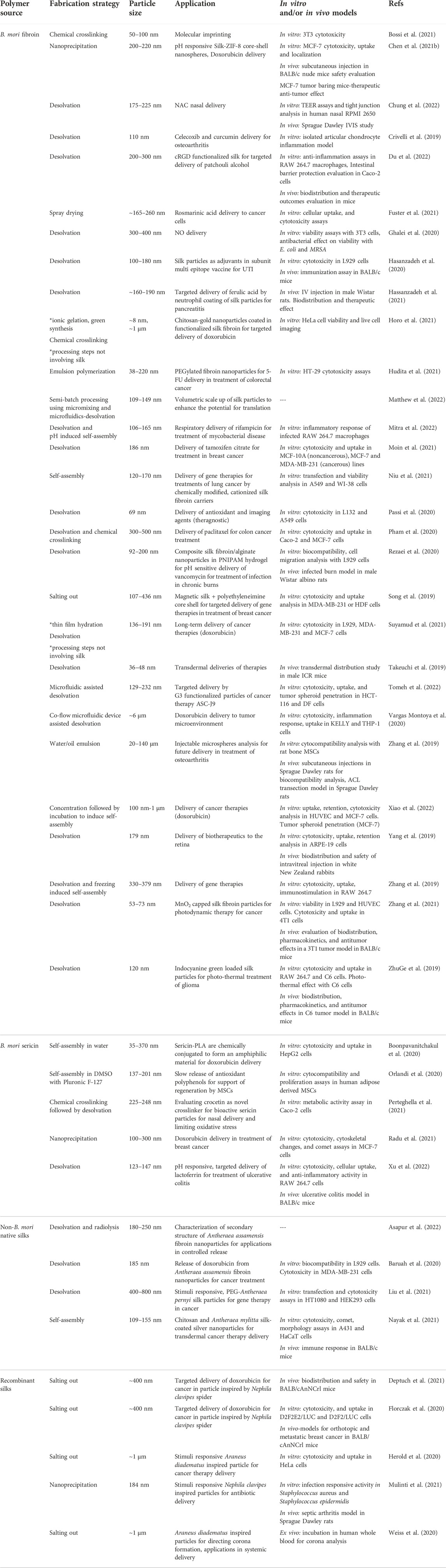
TABLE 1. Summary of current advances in silk and silk fiber-inspired nanomaterials for drug and gene delivery.
Recently, researchers have combined functionalization techniques with the use of composite materials to generate the next generation of silk-based nanomaterials that can function as targeted delivery systems (Song et al., 2019; Totten et al., 2019; Pham et al., 2020; Bossi et al., 2021; Hassanzadeh et al., 2021; Horo et al., 2021; Zahedi et al., 2021; Du et al., 2022) and as systems for both therapy and diagnostics (ZhuGe et al., 2019; Passi et al., 2020; Zhang et al., 2021). Pham et al. (2020) developed a fibroin nanoparticle system for delivery of therapeutics in the treatment of colon cancer. They encapsulated paclitaxel in fibroin particles (300–500 nm) formed by organic solvent desolvation and EDC crosslinking. They found their formulations were effective in colon and breast cell cancer lines, but interestingly observed that when the formulation was used in colon cancer lines, cytotoxic efficiency increased 10-fold relative to free drug (Pham et al., 2020). Du et al. (2022) also explored targeting sites in the colon for ulcerative colitis. They formed nanoparticles by desolvation and then surface functionalized them with cRGD. Safety and efficacy were evaluated in vitro and in vivo and it was found that the cRGD functionalized particles specifically interacted with integrin αv inflammation sites in the colon and contributed to an improved therapeutic outcome (Du et al., 2022). Horo et al. (2021) developed a composite material for targeted delivery of antitumor agents. They developed chitosan-stabilized gold nanoparticles (∼8 nm) loaded with doxorubicin and coated with fibroin conjugated with folic acid and fluorescein to promote the targeting of tumor cells. The silk coating contributed to an improved drug release profile and increased uptake and cytotoxicity in HeLa cultures. They also modified this formulation into larger particles (∼1,000 µm) using ionic gelation to address the potential for oral delivery (Horo et al., 2021). Song et al. (2019) also explored anti-cancer composite silk nanomaterials for targeted delivery for gene therapies. They utilized a salting out technique to fabricate magnetic silk + polyethyleneimine core shell nanoparticles with tunable size (∼100–500 nm). They demonstrated enhanced delivery of therapeutic antisense oligodeoxynucleotides by use of magnetofection in MDA-MB-231 breast cancer cells (Song et al., 2019). Silk-based nanomaterial DDS continue to be made of primarily Bombyx mori fibroin polymers, but recently researchers have also begun investigating other silk fiber biopolymers as well as alternative sources for silk fibroin biopolymers that have additional unique properties for use in biomedical applications.
Bombyx mori sericin
As mentioned previously, sericin proteins from Bombyx mori cocoons have traditionally been discarded as a waste product. However, recent work has highlighted how, when purified, sericin proteins are non-cytotoxic, have a high potential for reactive modification, and can facilitate cell-material interaction. A major limitation of sericin proteins is that they are highly water soluble, meaning the majority of nanomaterials made with sericin must be composite materials. Sericins from Bombyx mori are not as well characterized as their fibroin counterpart, but it has been shown that they are globular proteins in nature ranging from 20 to 310 kDa and mainly consist of reactive residues like serine and aspartic acid. This means sericin materials often have increased presence of random coil structures; however, crystallinity can still be induced in the material synthesis process by techniques such as desolvation. Recent studies utilizing sericin-based DDS have focused on applications including nasal delivery (Perteghella et al., 2021), pH responsive materials (Xu et al., 2022), antibacterial activity (Baker et al., 2020; Al Masud et al., 2021), cell therapy (Orlandi et al., 2020), and cancer therapies (Boonpavanitchakul et al., 2020; Baker et al., 2021; Radu et al., 2021) (Table 1).
To assemble sericin-based nanoparticles, researchers often have taken a two-step approach. First, taking advantage of the reactiveness of sericin, they chemically crosslink or modify the polymer. This is typically followed by a self-assembly or desolvation step. Boonpavanitchakul et al. (2020) conjugated a hydrophobic polymer, PLA, to sericin, forming an amphiphilic material. They were then able to self-assemble the PLA-silk sericin polymers into nanomaterials of tunable size (∼36–370 nm) depending on the pH and inclusion of additional crosslinkers, all taking place in aqueous conditions. They further demonstrated the potential of this system as a cancer therapy by showing effective loading of doxorubicin, enhanced release under acidic conditions, and effectiveness in vitro liver cancer cell models (Boonpavanitchakul et al., 2020). Perteghella et al. (2021) stabilized sericin by crosslinking with crocetin and formed ∼225 nm nanoparticles by desolvation in acetone. Perteghella et al. (2021) and colleagues are working toward optimizing these particles for nose-to-brain delivery and demonstrated cytocompatibility and protection from oxidative stress in Caco-2 epithelial cells. Xu et al. (2022) took a novel approach and optimized a transgenic silkworm to produce silk that is sericin-rich and has recombinant lactoferrin expressed in the sericin layer for treatment of ulcerative colitis. They were then able to synthesize ∼125 nm negatively charged sericin-lactoferrin nanospheres by acetone desolvation. The effectiveness of the system was evaluated by oral delivery in an ulcerative colitis mouse model. They demonstrated improved uptake efficiency (attributed to charge interactions) and inhibition of the NF-κβ inflammatory pathway associated with acute colitis (Xu et al., 2022). Overall, the recent progress in sericin DDS highlight the advantages of the increased bioactivity and reactivity of the protein. Researchers have also begun to evaluate the potential of silk from different species for this same purpose.
Non-Bombyx mori native silk fibers
While most silk-based healthcare materials have traditionally been reverse engineered from Bombyx mori silk fibers, most insects in the Lepidopteran order produce silk fibers for a variety of applications (cocoon/web formation, egg coating, etc.,). Recent studies have found that some of these fibers expand the design space for silk-based materials by having more reactive residues and different mechanical properties. Recently, silk fibroin from Antheraea assamensis (Baruah et al., 2020; Asapur et al., 2022), Antheraea pernyi (Liu et al., 2021), and sericin from Antheraea mylitta (Nayak et al., 2021) have all been used in the development of nanoparticle DDS (Table 1).
Asapur et al. (2022) characterized the secondary structure of nanoparticles synthesized from Antheraea assamensis fibroin by desolvation and radiolysis methods. The fibroin from Antheraea assamensis is known for its ability to absorb high levels of UV radiation and its impressive tensile strength (Goswami et al., 2020). Like Bombyx mori fibroin, alanine-rich repeating motifs give this biopolymer the potential to form nanomaterials with high levels of β sheet crystallinity (Gupta et al., 2015). Asapur et al. (2022) found that the radiolysis method led to the formation of smaller ∼180 nm particles compared to ∼250 nm particles made with desolvation. Both particle types also had >30% crystalline content, showing potential for controlled release. Baruah et al. (2020) evaluated the release of doxorubicin from Antheraea assamensis fibroin particles made by desolvation and found efficient drug loading, and that the DDS increased the effectiveness relative to free doxorubicin of the cytotoxic effect on MDA-MB-231 cancer cells.
Liu et al. (2021) took advantage of the abundant reactive sites present in silk fibroin from Antheraea pernyi in the creation of their stimuli responsive DDS. They prepared particles from cationized Antheraea pernyi fibroin for gene delivery to cancer cells by desolvation in DMSO. Using the reactive capabilities of this fibroin, they grafted on an MMP-2 cleavable PEG to the particles surface. This allowed for limiting opsonization without sacrificing transfection when MMP-2 (highly expressed in the tumor microenvironment) cleaves the PEG in vitro. They demonstrated decreased protein adhesion and successful transfection to HEK293 cells (Liu et al., 2021). In addition to leveraging the biodiversity of silk fiber producing species to design novel polymeric nanomaterials for DDS, researchers have also recently been producing recombinant proteins inspired by silk fibers to create targeted DDS.
Silk fiber-inspired recombinant polymers
As an alternative to use of purified silk fibroin proteins, researchers have also designed recombinant silk proteins inspired by nature to make polymeric nanomaterials for drug and therapeutic delivery. One approach is to use bacteria, such as E. coli, to express silk-like peptides either alone or in combination with other useful protein sequences (Huang et al., 2015; Bowen et al., 2018; Yu et al., 2022). Another approach utilizes whole organism genetic modification to express engineered silk fibroins or other related therapeutic agents (e.g., sericins) (Xu et al., 2022; Tamura et al., 2000; Tomita et al., 2003; Xu and O'Brochta, 2015; Saviane et al., 2018; Baci et al., 2021a; Baci et al., 2021b; Zhong et al., 2011).
Much of the focus of genetic engineering of silk proteins is based on the silk fibroins produced by spiders. This is because, unlike Lepidopteran species, spiders’ cannibalistic and territorial tendencies make it so spiders cannot be farmed, meaning their fibers cannot be naturally sourced for biomedical research. Researchers have taken inspiration from the silk of Nephila clavipes and Araneus diadematus to design recombinant spider proteins. Silk produced from spiders (spidroin proteins) are ∼300 kDa and composed of a highly repetitive core, mainly consisting of alanine, glycine, and proline residues, flanked by small, nonrepetitive terminal domains, which are highly conserved across spider species (Babb et al., 2017). The development, expression, and purification of spider-inspired fibroin proteins (spidroin proteins) has been recently reviewed (Kiseleva et al., 2020; Bittencourt et al., 2022). For the purposes of this review, we focus on the nanoparticle fabrication techniques recently used in the progress toward targeted DDS, and recent publications are summarized in Table 1.
The Scheibel group designed nanoparticle systems based on Araneus diadematus with the goal of directing corona formation and stimuli responsive release (Herold et al., 2020; Weiss et al., 2020). They recombinantly produced polymers with either a net negative or net positive charge and used the salting out technique to form nanoparticles. They then performed ex vivo analysis of corona formation in whole blood (Weiss et al., 2020). It was observed that the negatively charged nanospheres primarily attracted complement proteins and immunoglobulins, while positively charged particles primarily had fibrinogen-based proteins adsorbed on their surface (Weiss et al., 2020). In a separate study, researchers utilized a polyanionic form of the silk from the aforementioned study and covalently attached a cytostatic drug to the particle surface and explored the response to pH and redox state (Herold et al., 2020). Ellman’s solution (a model to simulate sulfhydryl group containing drugs) was bound to cystine residues in the spider fibroin-inspired silk particle, which was then further modified by including a hydrazone linker. This system led to enhanced drug release under acidic conditions and oxidative stress in HeLa cultures (Herold et al., 2020). Researchers have also explored recombinantly producing proteins and peptides inspired by the spidroin proteins of other spider species (Ramezaniaghdam et al., 2022).
The Dam-Kozlowska group has been building toward a cancer therapeutic DDS based on a recombinant silk fiber inspired by Nephila clavipes (Florczak et al., 2014; Florczak et al., 2020; Deptuch et al., 2021). They were able to produce a polymer based on the MaSp1 sequence from Nephila clavipes and include a Her2 binding peptide. Particles were produced by salting out and loaded with doxorubicin. They demonstrated enhanced binding to Her2-overexpressing SKOV3 cells and showed the particles themselves were noncytotoxic and could deliver doxorubicin to kill cancer cells (Florczak et al., 2014). They recently tested this system in vivo and demonstrated safety (Deptuch et al., 2021) and targeted delivery in breast cancer models (Florczak et al., 2020). The development of DDS based on silk nanomaterials continues to grow and researchers are moving toward potential for clinical translation.
Future perspectives and conclusion
The wide range of material properties available from the reconstitution of silk fibers makes them an interesting class of materials for future translational research. Specifically, silk fibroins from Bombyx mori are able to generate a wide array of drug delivery systems (DDS) given the range of fabrication techniques, modification methods, and utility in composite materials. Furthermore, spider silk-inspired and sericin biopolymers represent additional classes of materials for DDS. Each class of materials offers its own unique features, such as biodegradation, controllable immunogenicity, and favorable mechanical properties.
While many systems have been proposed, grand challenges still exist in the application of these materials to clinical settings. As with any natural material or recombinantly produced protein, ensuring good manufacturing practices as well as methods to remove contaminating molecules or organisms, such and endotoxins or mycotoxins, is critical. Sourcing these natural materials can also be a challenge, as Bombyx mori cultivation and farming is subject to our changing climate and regional differences in farming practices (Gani and Ghosh, 2018; Shilpa et al., 2021), which can lead to variability in cocoons and subsequent silk properties. Recent advancements in genetic engineering technologies for the Bombyx mori silkworm suggest that improvements or modifications to protein structures within these systems is possible (Tamura et al., 2000; Xu and O'Brochta, 2015; Saviane et al., 2018; Baci et al., 2021a; Long et al., 2020), thus providing an avenue for addressing current challenges in protein structure highlighted throughout this review. However, any change is not without added complication or risk, as modification to silk fibroin protein sequences can pose new challenges in protein expression, spinning, or purification. Additionally, chemical modifications to existing natural materials can also alter chemistries for improvements in affinity binding and drug loading (Sato et al., 2012; Heichel and Burke, 2020). Continued efforts to further open the design space to include silk fibers or silk fiber-based proteins from other species as well as recombinantly produced polymers and peptides is another strategy for overcoming current challenges. These efforts demonstrate the enormous future potential for this class of materials and their scale-up, use in advanced manufacturing, generation of novel polymer solutions, and development of treatments for a myriad of diseases. While this review was limited to nanomaterial-based DDS, we expect that the use of novel silk proteins for the formation of materials such as scaffolds, films, foams, and hydrogels is an area of interest for local DDS, regenerative medicine, and tissue engineering. Similarly, clinical trials using materials from or synthesized to replicate components silk fibers are on-going, with a large focus on inert filler materials for treatment of conditions like vocal cord paralysis (National Library of Medicine (U.S.), 2019). These advances toward clinical application are positive first steps for the continued investigation and development of all-natural silk-inspired nanomaterials for drug delivery systems.
Author contributions
MP: Writing-original draft, review and editing, visualization, conceptualization LE: Visualization, writing-review and editing ND: Visualization, writing-review and editing JA: Writing-review and editing AC: Writing-review and editing IK: Writing-review and editing WS: Writing-review and editing, conceptualization, supervision. All authors have read and agreed to the final version of the manuscript.
Funding
All authors would like to acknowledge support from the Department of Defense Congressionally Directed Medical Research Fund (W81XWH2110199). LE and AC acknowledge support from the REU in Chemical Engineering REU Site at the University of Florida (NSF EEC-1852111). ND was supported by a grant from the National Institutes of Health (NIH T35HL007489). The content is solely the responsibility of the authors and does not necessarily represent the official views of the NIH. MP acknowledges support from the National Science Foundation Graduate Research Fellowship (DGE-1842473). Any opinions, findings, and conclusions or recommendations expressed in this material are those of the author(s) and do not necessarily reflect the views of the National Science Foundation or another funding agency. IK would like to acknowledge support from the UF Student Science Training Program (SSTP) and her summer research experience.
Acknowledgments
The Stoppel Lab would like to acknowledge the University of Florida (UF) Chemical Engineering Research Experience for Undergraduates program, the UF Herbert Wertheim College of Engineering Summer Undergraduate Research at Florida (SURF) Program, the UF Student Science Training Program, and the UF College of Medicine Medical Student Research Program for supporting development of trainees in the laboratory during Summer 2022. Figure 1 was created with a license from BioRender.com.
Conflict of interest
The authors declare that the research was conducted in the absence of any commercial or financial relationships that could be construed as a potential conflict of interest.
Publisher’s note
All claims expressed in this article are solely those of the authors and do not necessarily represent those of their affiliated organizations, or those of the publisher, the editors and the reviewers. Any product that may be evaluated in this article, or claim that may be made by its manufacturer, is not guaranteed or endorsed by the publisher.
References
Al Masud, M. A., Shaikh, H., Alam, M. S., Karim, M. M., Momin, M. A., Islam, M. A., et al. (2021). Green synthesis of silk sericin-embedded silver nanoparticles and their antibacterial application against multidrug-resistant pathogens. J. Genet. Eng. Biotechnol. 19 (1), 74. doi:10.1186/s43141-021-00176-5
Asakura, T., Okushita, K., and Williamson, M. P. (2015). Analysis of the structure of Bombyx mori silk fibroin by NMR. Macromolecules 48 (8), 2345–2357. doi:10.1021/acs.macromol.5b00160
Asapur, P., Mahapatra, S. K., and Banerjee, I. (2022). Secondary structural analysis of non-mulberry silk fibroin nanoparticles synthesized by using microwave and acetone method. J. Biomol. Struct. Dyn. 40 (9), 4100–4109. doi:10.1080/07391102.2020.1852970
Babb, P. L., Lahens, N. F., Correa-Garhwal, S. M., Nicholson, D. N., Kim, E. J., Hogenesch, J. B., et al. (2017). The Nephila clavipes genome highlights the diversity of spider silk genes and their complex expression. Nat. Genet. 49 (6), 895–903. doi:10.1038/ng.3852
Baci, G. M., Cucu, A. A., Giurgiu, A. I., Musca, A. S., Bagameri, L., Moise, A. R., et al. (2021). Advances in editing silkworms (Bombyx mori) genome by using the CRISPR-cas system. Insects 13 (1), 28. doi:10.3390/insects13010028
Baci, G. M., Moise, A. R., and Dezmirean, D. S. (2021). Utilization of transgenic Bombyx mori for biomaterials production. Sci. Pap. Animal Sci. Biotechnologies/Lucrari Stiintifice Zootehnie si Biotehnol. 54 (1).
Baker, A., Syed, A., Alyousef, A. A., Arshad, M., Alqasim, A., Khalid, M., et al. (2020). Sericin-functionalized GNPs potentiate the synergistic effect of levofloxacin and balofloxacin against MDR bacteria. Microb. Pathog. 148, 104467. doi:10.1016/j.micpath.2020.104467
Baker, A., Wahid, I., Hassan Baig, M., Alotaibi, S. S., Khalid, M., Uddin, I., et al. (2021). Silk cocoon-derived protein bioinspired gold nanoparticles as a formidable anticancer agent. J. Biomed. Nanotechnol. 17 (4), 615–626. doi:10.1166/jbn.2021.3053
Baruah, R. R., Chandra Kalita, M., and Devi, D. (2020). Novel non-mulberry silk fibroin nanoparticles with enhanced activity as potential candidate in nanocarrier mediated delivery system. RSC Adv. 10 (15), 9070–9078. doi:10.1039/c9ra08901b
Bittencourt, D. M. C., Oliveira, P., Michalczechen-Lacerda, V. A., Rosinha, G. M. S., Jones, J. A., and Rech, E. L. (2022). Bioengineering of spider silks for the production of biomedical materials. Front. Bioeng. Biotechnol. 10, 958486. doi:10.3389/fbioe.2022.958486
Blanco, E., Shen, H., and Ferrari, M. (2015). Principles of nanoparticle design for overcoming biological barriers to drug delivery. Nat. Biotechnol. 33 (9), 941–951. doi:10.1038/nbt.3330
Boonpavanitchakul, K., Bast, L. K., Bruns, N., and Magaraphan, R. (2020). Silk sericin-polylactide protein-polymer conjugates as biodegradable amphiphilic materials and their application in drug release systems. Bioconjug. Chem. 31 (10), 2312–2324. doi:10.1021/acs.bioconjchem.0c00399
Bossi, A. M., Bucciarelli, A., and Maniglio, D. (2021). Molecularly imprinted silk fibroin nanoparticles. ACS Appl. Mat. Interfaces 13 (27), 31431–31439. doi:10.1021/acsami.1c05405
Bowen, C. H., Dai, B., Sargent, C. J., Bai, W., Ladiwala, P., Feng, H., et al. (2018). Recombinant spidroins fully replicate primary mechanical properties of natural spider silk. Biomacromolecules 19 (9), 3853–3860. doi:10.1021/acs.biomac.8b00980
Brannon, E. R., Guevara, M. V., Pacifici, N. J., Lee, J. K., Lewis, J. S., and Eniola-Adefeso, O. (2022). Polymeric particle-based therapies for acute inflammatory diseases. Nat. Rev. Mat. 7 (10), 796–813. doi:10.1038/s41578-022-00458-5
Chen, L., Hong, W., Ren, W., Xu, T., Qian, Z., and He, Z. (2021). Recent progress in targeted delivery vectors based on biomimetic nanoparticles. Signal Transduct. Target. Ther. 6 (1), 225. doi:10.1038/s41392-021-00631-2
Chen, Y., Wu, H., Yang, T., Zhou, G., Chen, Y., Wang, J., et al. (2021). Biomimetic nucleation of metal-organic frameworks on silk fibroin nanoparticles for designing core-shell-structured pH-responsive anticancer drug carriers. ACS Appl. Mat. Interfaces 13 (40), 47371–47381. doi:10.1021/acsami.1c13405
Chung, T. W., Wu, T. Y., Siah, Z. Y., and Liu, D. Z. (2022). Antioxidative NAC-loaded silk nanoparticles with opening mucosal tight junctions for nasal drug delivery: An in vitro and in vivo study. Pharmaceutics 14 (6), 1288. doi:10.3390/pharmaceutics14061288
Crivelli, B., Bari, E., Perteghella, S., Catenacci, L., Sorrenti, M., Mocchi, M., et al. (2019). Silk fibroin nanoparticles for celecoxib and curcumin delivery: ROS-scavenging and anti-inflammatory activities in an in vitro model of osteoarthritis. Eur. J. Pharm. Biopharm. 137, 37–45. doi:10.1016/j.ejpb.2019.02.008
Deptuch, T., Florczak, A., Lewandowska, A., Leporowska, E., Penderecka, K., Marszalek, A., et al. (2021). MS1-type bioengineered spider silk nanoparticles do not exhibit toxicity in an in vivo mouse model. Nanomedicine Lond. Engl. 16 (18), 1553–1565. doi:10.2217/nnm-2021-0029
Du, Y., Shi, J., Duan, R., Tsim, K. W. K., Shen, L., Zhang, N., et al. (2022). cRGD peptide incorporated with patchouli alcohol loaded silk fibroin nanoparticles for enhanced targeting of inflammatory sites in colitis. Biomater. Adv. 140, 213069. doi:10.1016/j.bioadv.2022.213069
Florczak, A., Deptuch, T., Lewandowska, A., Penderecka, K., Kramer, E., Marszalek, A., et al. (2020). Functionalized silk spheres selectively and effectively deliver a cytotoxic drug to targeted cancer cells in vivo. J. Nanobiotechnology 18 (1), 177. doi:10.1186/s12951-020-00734-y
Florczak, A., Mackiewicz, A., and Dams-Kozlowska, H. (2014). Functionalized spider silk spheres as drug carriers for targeted cancer therapy. Biomacromolecules 15 (8), 2971–2981. doi:10.1021/bm500591p
Fuster, M. G., Carissimi, G., Montalban, M. G., and Villora, G. (2021). Antitumor activity of rosmarinic acid-loaded silk fibroin nanoparticles on HeLa and MCF-7 cells. Polym. (Basel) 13 (18), 3169. doi:10.3390/polym13183169
Gani, M. N. A., and Ghosh, M. K. (2018). Impact of climate change on agriculture and sericulture. J. ENTOMOLOGY ZOOLOGY Stud. 6 (5), 426–429.
George, A., Shah, P. A., and Shrivastav, P. S. (2019). Natural biodegradable polymers based nano-formulations for drug delivery: A review. Int. J. Pharm. X. 561, 244–264. doi:10.1016/j.ijpharm.2019.03.011
Ghalei, S., Mondal, A., Hopkins, S., Singha, P., Devine, R., and Handa, H. (2020). Silk nanoparticles: A natural polymeric platform for nitric oxide delivery in biomedical applications. ACS Appl. Mat. Interfaces 12 (48), 53615–53623. doi:10.1021/acsami.0c13813
Goswami, A., Goswami, N., Bhattacharya, A., Borah, P., and Devi, D. (2020). Composition and in silico structural analysis of fibroin from liquid silk of non-mulberry silkworm Antheraea assamensis. Int. J. Biol. Macromol. 163, 1947–1958. doi:10.1016/j.ijbiomac.2020.08.232
Guo, C., Li, C., and Kaplan, D. L. (2020). Enzymatic degradation of Bombyx mori silk materials: A review. Biomacromolecules 21 (5), 1678–1686. doi:10.1021/acs.biomac.0c00090
Gupta, A. K., Mita, K., Arunkumar, K. P., and Nagaraju, J. (2015). Molecular architecture of silk fibroin of Indian golden silkmoth, Antheraea assama. Sci. Rep. 5 (1), 12706. doi:10.1038/srep12706
Hasanzadeh, S., Farokhi, M., Habibi, M., Shokrgozar, M. A., Ahangari Cohan, R., Rezaei, F., et al. (2020). Silk fibroin nanoadjuvant as a promising vaccine carrier to deliver the FimH-IutA antigen for urinary tract infection. ACS Biomater. Sci. Eng. 6 (8), 4573–4582. doi:10.1021/acsbiomaterials.0c00736
Hassanzadeh, P., Arbabi, E., and Rostami, F. (2021). Coating of ferulic acid-loaded silk fibroin nanoparticles with neutrophil membranes: A promising strategy against the acute pancreatitis. Life Sci. 270, 119128. doi:10.1016/j.lfs.2021.119128
Heichel, D. L., and Burke, K. A. (2020). Enhancing the carboxylation efficiency of silk fibroin through the disruption of noncovalent interactions. Bioconjug. Chem. 31 (5), 1307–1312. doi:10.1021/acs.bioconjchem.0c00168
Herold, H. M., Dobl, A., Wohlrab, S., Humenik, M., and Scheibel, T. (2020). Designed spider silk-based drug carrier for redox- or pH-triggered drug release. Biomacromolecules 21 (12), 4904–4912. doi:10.1021/acs.biomac.0c01138
Hong, S., Choi, D. W., Kim, H. N., Park, C. G., Lee, W., and Park, H. H. (2020). Protein-based nanoparticles as drug delivery systems. Pharmaceutics 12 (7), 604. doi:10.3390/pharmaceutics12070604
Horo, H., Bhattacharyya, S., Mandal, B., and Kundu, L. M. (2021). Synthesis of functionalized silk-coated chitosan-gold nanoparticles and microparticles for target-directed delivery of antitumor agents. Carbohydr. Polym. 258, 117659. doi:10.1016/j.carbpol.2021.117659
Hrkach, J., and Langer, R. (2020). From micro to nano: Evolution and impact of drug delivery in treating disease. Drug Deliv. Transl. Res. 10 (3), 567–570. doi:10.1007/s13346-020-00769-6
Hu, X., Shmelev, K., Sun, L., Gil, E. S., Park, S. H., Cebe, P., et al. (2011). Regulation of silk material structure by temperature-controlled water vapor annealing. Biomacromolecules 12 (5), 1686–1696. doi:10.1021/bm200062a
Huang, W., Rollett, A., and Kaplan, D. L. (2015). Silk-elastin-like protein biomaterials for the controlled delivery of therapeutics. Expert Opin. Drug Deliv. 12 (5), 779–791. doi:10.1517/17425247.2015.989830
Hudita, A., Radu, I. C., Zaharia, C., Ion, A. C., Ginghina, O., Galateanu, B., et al. (2021). Bio- and hemo-compatible silk fibroin PEGylated nanocarriers for 5-fluorouracil chemotherapy in colorectal cancer: In vitro studies. Pharmaceutics 13 (5), 755. doi:10.3390/pharmaceutics13050755
Jain, K. K. (2020). An overview of drug delivery systems. Methods Mol. Biol. 2059, 1–54. doi:10.1007/978-1-4939-9798-5_1
Jameson, J. F., Pacheco, M. O., Butler, J. E., and Stoppel, W. L. (2021). Estimating kinetic rate parameters for enzymatic degradation of lyophilized silk fibroin sponges. Front. Bioeng. Biotechnol. 9 (537), 664306. doi:10.3389/fbioe.2021.664306
Kiseleva, A. P., Krivoshapkin, P. V., and Krivoshapkina, E. F. (2020). Recent advances in development of functional spider silk-based hybrid materials. Front. Chem. 8, 554. doi:10.3389/fchem.2020.00554
Liu, X., Luo, H., Niu, L., Feng, Y., Pan, P., Yang, J., et al. (2021). Cleavable poly(ethylene glycol) branched chain-modified Antheraea pernyi silk fibroin as a gene delivery carrier. Nanomedicine Lond. Engl. 16 (10), 839–853. doi:10.2217/nnm-2020-0481
Long, D., Xiao, B., and Merlin, D. (2020). Genetically modified silk fibroin nanoparticles for drug delivery: Preparation strategies and application prospects. Nanomedicine Lond. Engl. 15 (18), 1739–1742. doi:10.2217/nnm-2020-0182
Matthew, S. A. L., Rezwan, R., Perrie, Y., and Seib, F. P. (2022). Volumetric scalability of microfluidic and semi-batch silk nanoprecipitation methods. Molecules 27 (7), 2368. doi:10.3390/molecules27072368
Matthew, S. A. L., Totten, J. D., Phuagkhaopong, S., Egan, G., Witte, K., Perrie, Y., et al. (2020). Silk nanoparticle manufacture in semi-batch format. ACS Biomater. Sci. Eng. 6 (12), 6748–6759. doi:10.1021/acsbiomaterials.0c01028
Mitra, K., Chadha, A., Muthuvijayan, V., and Doble, M. (2022). Self-assembled inhalable immunomodulatory silk fibroin nanocarriers for enhanced drug loading and intracellular antibacterial activity. ACS Biomater. Sci. Eng. 8 (2), 708–721. doi:10.1021/acsbiomaterials.1c01357
Moin, A., Wani, S. U. D., Osmani, R. A., Abu Lila, A. S., Khafagy, E. S., Arab, H. H., et al. (2021). Formulation, characterization, and cellular toxicity assessment of tamoxifen-loaded silk fibroin nanoparticles in breast cancer. Drug Deliv. 28 (1), 1626–1636. doi:10.1080/10717544.2021.1958106
Mulinti, P., Shreffler, J., Hasan, R., Dea, M., and Brooks, A. E. (2021). Infection responsive smart delivery of antibiotics using recombinant spider silk nanospheres. Pharmaceutics 13 (9), 1358. doi:10.3390/pharmaceutics13091358
Murphy, A. R., and Kaplan, D. L. (2009). Biomedical applications of chemically-modified silk fibroin. J. Mat. Chem. 19 (36), 6443–6450. doi:10.1039/b905802h
Murphy, A. R., St John, P., and Kaplan, D. L. (2008). Modification of silk fibroin using diazonium coupling chemistry and the effects on hMSC proliferation and differentiation. Biomaterials 29 (19), 2829–2838. doi:10.1016/j.biomaterials.2008.03.039
National Library of Medicine (U.S.) (2019). Silk protein microparticle-based filler for injection augmentation. Identifier NCT03790956, https://ClinicalTrials.gov/show/NCT03790956.
Nayak, D., Thathapudi, N. C., Ashe, S., and Nayak, B. (2021). Bioengineered ethosomes encapsulating AgNPs and Tasar silk sericin proteins for non melanoma skin carcinoma (NMSC) as an alternative therapeutics. Int. J. Pharm. X. 596, 120265. doi:10.1016/j.ijpharm.2021.120265
Niu, L., Chen, G., Feng, Y., Liu, X., Pan, P., Huang, L., et al. (2021). Polyethylenimine-modified Bombyx mori silk fibroin as a delivery carrier of the ING4-IL-24 coexpression plasmid. Polym. (Basel) 13 (20), 3592. doi:10.3390/polym13203592
Ode Boni, B. O., Bakadia, B. M., Osi, A. R., Shi, Z., Chen, H., Gauthier, M., et al. (2022). Immune response to silk sericin-fibroin composites: Potential immunogenic elements and alternatives for immunomodulation. Macromol. Biosci. 22 (1), e2100292. doi:10.1002/mabi.202100292
Orlandi, G., Bari, E., Catenacci, L., Sorrenti, M., Segale, L., Farago, S., et al. (2020). Polyphenols-loaded sericin self-assembling nanoparticles: A slow-release for regeneration by tissue-resident mesenchymal stem/stromal cells. Pharmaceutics 12 (4), 381. doi:10.3390/pharmaceutics12040381
Passi, M., Kumar, V., and Packirisamy, G. (2020). Theranostic nanozyme: Silk fibroin based multifunctional nanocomposites to combat oxidative stress. Mater. Sci. Eng. C 107, 110255. doi:10.1016/j.msec.2019.110255
Perteghella, S., Rassu, G., Gavini, E., Obinu, A., Bari, E., Mandracchia, D., et al. (2021). Crocetin as new cross-linker for bioactive sericin nanoparticles. Pharmaceutics 13 (5), 680. doi:10.3390/pharmaceutics13050680
Pham, D. T., Saelim, N., and Tiyaboonchai, W. (2020). Paclitaxel loaded EDC-crosslinked fibroin nanoparticles: A potential approach for colon cancer treatment. Drug Deliv. Transl. Res. 10 (2), 413–424. doi:10.1007/s13346-019-00682-7
Pham, D. T., and Tiyaboonchai, W. (2020). Fibroin nanoparticles: A promising drug delivery system. Drug Deliv. 27 (1), 431PMC7144220–48. doi:10.1080/10717544.2020.1736208
Radu, I. C., Zaharia, C., Hudita, A., Tanasa, E., Ginghina, O., Marin, M., et al. (2021). In vitro interaction of doxorubicin-loaded silk sericin nanocarriers with MCF-7 breast cancer cells leads to DNA damage. Polym. (Basel) 13 (13), 2047. doi:10.3390/polym13132047
Ramezaniaghdam, M., Nahdi, N. D., and Reski, R. (2022). Recombinant spider silk: Promises and bottlenecks. Front. Bioeng. Biotechnol. 10, 835637. doi:10.3389/fbioe.2022.835637
Rezaei, F., Damoogh, S., Reis, R. L., Kundu, S. C., Mottaghitalab, F., and Farokhi, M. (2020). Dual drug delivery system based on pH-sensitive silk fibroin/alginate nanoparticles entrapped in PNIPAM hydrogel for treating severe infected burn wound. Biofabrication 13 (1), 015005. doi:10.1088/1758-5090/abbb82
Rockwood, D. N., Preda, R. C., Yucel, T., Wang, X., Lovett, M. L., and Kaplan, D. L. (2011). Materials fabrication from Bombyx mori silk fibroin. Nat. Protoc. 6 (10), 1612–1631. doi:10.1038/nprot.2011.379
Sato, M., Kojima, K., Sakuma, C., Murakami, M., Aratani, E., Takenouchi, T., et al. (2012). Production of scFv-conjugated affinity silk powder by transgenic silkworm technology. PLoS One 7 (4), e34632. doi:10.1371/journal.pone.0034632
Saviane, A., Romoli, O., Bozzato, A., Freddi, G., Cappelletti, C., Rosini, E., et al. (2018). Intrinsic antimicrobial properties of silk spun by genetically modified silkworm strains. Transgenic Res. 27 (1), 87. doi:10.1007/s11248-018-0059-0
Seib, F. P., Jones, G. T., Rnjak-Kovacina, J., Lin, Y., and Kaplan, D. L. (2013). pH-dependent anticancer drug release from silk nanoparticles. Adv. Healthc. Mat. 2 (12), 1606–1611. doi:10.1002/adhm.201300034
Shilpa, M., Gowda, D. M., Ramesh, B. S., and Avinash, G. (2021). Assessment of the impact of climatic parameters on silk cocoon production2021. Pharma Innovation J. SP-10 (12), 885–888.
Shimanovich, U., Ruggeri, F. S., De Genst, E., Adamcik, J., Barros, T. P., Porter, D., et al. (2017). Silk micrococoons for protein stabilisation and molecular encapsulation. Nat. Commun. 8 (1), 15902. doi:10.1038/ncomms15902
Song, W., Gregory, D. A., Al-Janabi, H., Muthana, M., Cai, Z., and Zhao, X. (2019). Magnetic-silk/polyethyleneimine core-shell nanoparticles for targeted gene delivery into human breast cancer cells. Int. J. Pharm. X. 555, 322–336. doi:10.1016/j.ijpharm.2018.11.030
Suyamud, C., Phetdee, C., Jaimalai, T., and Prangkio, P. (2021). Silk fibroin-coated liposomes as biomimetic nanocarrier for long-term release delivery system in cancer therapy. Molecules 26 (16), 4936. doi:10.3390/molecules26164936
Takeuchi, I., Shimamura, Y., Kakami, Y., Kameda, T., Hattori, K., Miura, S., et al. (2019). Transdermal delivery of 40-nm silk fibroin nanoparticles. Colloids Surfaces B Biointerfaces 175, 564–568. doi:10.1016/j.colsurfb.2018.12.012
Tamura, T., Thibert, C., Royer, C., Kanda, T., Abraham, E., Kamba, M., et al. (2000). Germline transformation of the silkworm Bombyx mori L. using a piggyBac transposon-derived vector. Nat. Biotechnol. 18 (5), 81–84. doi:10.1038/71978
Tomeh, M. A., Hadianamrei, R., Xu, D., Brown, S., and Zhao, X. (2022). Peptide-functionalised magnetic silk nanoparticles produced by a swirl mixer for enhanced anticancer activity of ASC-J9. Colloids Surfaces B Biointerfaces 216, 112549. doi:10.1016/j.colsurfb.2022.112549
Tomita, M., Munetsuna, H., Sato, T., Adachi, T., Hino, R., Hayashi, M., et al. (2003). Transgenic silkworms produce recombinant human type III procollagen in cocoons. Nat. Biotechnol. 21 (1), 52–56. doi:10.1038/nbt771
Totten, J. D., Wongpinyochit, T., Carrola, J., Duarte, I. F., and Seib, F. P. (2019). PEGylation-Dependent metabolic rewiring of macrophages with silk fibroin nanoparticles. ACS Appl. Mat. Interfaces 11 (16), 14515–14525. doi:10.1021/acsami.8b18716
Vargas Montoya, N., Peterson, R., Ornell, K. J., Albrecht, D. R., and Coburn, J. M. (2020). Silk particle production based on silk/PVA phase separation using a microfabricated Co-flow device. Molecules 25 (4), 890. doi:10.3390/molecules25040890
Weiss, A. C. G., Herold, H. M., Lentz, S., Faria, M., Besford, Q. A., Ang, C. S., et al. (2020). Surface modification of spider silk particles to direct biomolecular corona formation. ACS Appl. Mat. Interfaces 12 (22), 24635–24643. doi:10.1021/acsami.0c06344
Wenk, E., Murphy, A. R., Kaplan, D. L., Meinel, L., Merkle, H. P., and Uebersax, L. (2010). The use of sulfonated silk fibroin derivatives to control binding, delivery and potency of FGF-2 in tissue regeneration. Biomaterials 31 (6), 1403–1413. doi:10.1016/j.biomaterials.2009.11.006
Wongpinyochit, T., Vassileiou, A. D., Gupta, S., Mushrif, S. H., Johnston, B. F., and Seib, F. P. (2019). Unraveling the impact of high-order silk structures on molecular drug binding and release behaviors. J. Phys. Chem. Lett. 10 (15), 4278–4284. doi:10.1021/acs.jpclett.9b01591
Xiao, L., Ding, Z., Zhang, X., Wang, X., Lu, Q., and Kaplan, D. L. (2022). Silk nanocarrier size optimization for enhanced tumor cell penetration and cytotoxicity in vitro. ACS Biomater. Sci. Eng. 8 (1), 140–150. doi:10.1021/acsbiomaterials.1c01122
Xu, H., and O'Brochta, D. A. (2015). Advanced technologies for genetically manipulating the silkworm Bombyx mori, a model Lepidopteran insect. Proc. R. Soc. B 282 (1810), 20150487–20150488. doi:10.1098/rspb.2015.0487
Xu, S., Yang, Q., Wang, R., Tian, C., Ji, Y., Tan, H., et al. (2022). Genetically engineered pH-responsive silk sericin nanospheres with efficient therapeutic effect on ulcerative colitis. Acta Biomater. 144, 81–95. doi:10.1016/j.actbio.2022.03.012
Yang, P., Dong, Y., Huang, D., Zhu, C., Liu, H., Pan, X., et al. (2019). Silk fibroin nanoparticles for enhanced bio-macromolecule delivery to the retina. Pharm. Dev. Technol. 24 (5), 575–583. doi:10.1080/10837450.2018.1545236
Yu, J., Guo, Y., Gu, Y., Fan, X., Li, F., Song, H., et al. (2022). A novel silk fibroin protein-based fusion system for enhancing the expression of nanobodies in Escherichia coli. Appl. Microbiol. Biotechnol. 106 (5-6), 1967–1977. doi:10.1007/s00253-022-11857-7
Zahedi, P., Besheli, N. H., Farokhi, M., Mottaghitalab, F., Sohrabi, A., and Ghorbanian, S. A. (2021). Silk fibroin nanoparticles functionalized with fibronectin for release of vascular endothelial growth factor to enhance angiogenesis. J. Nat. Fibers 19, 9223–9234. doi:10.1080/15440478.2021.1982814
Zhang, H., Lai, L., Wang, Y., Ye, B., Deng, S., Ding, A., et al. (2019). Silk fibroin for CpG oligodeoxynucleotide delivery. ACS Biomater. Sci. Eng. 5 (11), 6082–6088. doi:10.1021/acsbiomaterials.9b01413
Zhang, L., Yang, R., Yu, H., Xu, Z., Kang, Y., Cui, H., et al. (2021). MnO2-capped silk fibroin (SF) nanoparticles with chlorin e6 (Ce6) encapsulation for augmented photo-driven therapy by modulating the tumor microenvironment. J. Mat. Chem. B 9 (17), 3677–3688. doi:10.1039/d1tb00296a
Zhong, B., Wei, H., and Zhuang, L. (2011). Advances in research of silk gland bioreactor of piggyBac-mediated transgenic silkworm. Sci. Agric. Sin. 44 (21), 4488–4498.
Zhou, C. Z., Confalonieri, F., Jacquet, M., Perasso, R., Li, Z. G., and Janin, J. (2001). Silk fibroin: Structural implications of a remarkable amino acid sequence. Proteins. 44 (2), 119–122. doi:10.1002/prot.1078
ZhuGe, D. L., Wang, L. F., Chen, R., Li, X. Z., Huang, Z. W., Yao, Q., et al. (2019). Cross-linked nanoparticles of silk fibroin with proanthocyanidins as a promising vehicle of indocyanine green for photo-thermal therapy of glioma. Artif. Cells Nanomed. Biotechnol. 47 (1), 4293–4304. doi:10.1080/21691401.2019.1699819
Keywords: polymeric nanoparticles, natural biopolymers, silk, drug delivery, nanomaterials
Citation: Pacheco MO, Eccles LE, Davies NA, Armada J, Cakley AS, Kadambi IP and Stoppel WL (2022) Progress in silk and silk fiber-inspired polymeric nanomaterials for drug delivery. Front. Chem. Eng. 4:1044431. doi: 10.3389/fceng.2022.1044431
Received: 14 September 2022; Accepted: 23 November 2022;
Published: 19 December 2022.
Edited by:
Giulia Bozzano, Politecnico di Milano, ItalyCopyright © 2022 Pacheco, Eccles, Davies, Armada, Cakley, Kadambi and Stoppel. This is an open-access article distributed under the terms of the Creative Commons Attribution License (CC BY). The use, distribution or reproduction in other forums is permitted, provided the original author(s) and the copyright owner(s) are credited and that the original publication in this journal is cited, in accordance with accepted academic practice. No use, distribution or reproduction is permitted which does not comply with these terms.
*Correspondence: Whitney L. Stoppel, d2hpdG5leS5zdG9wcGVsQHVmbC5lZHU=