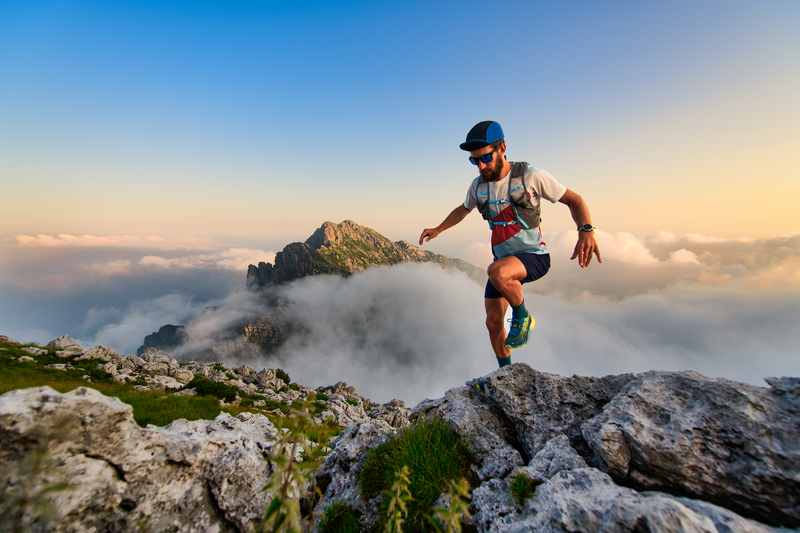
95% of researchers rate our articles as excellent or good
Learn more about the work of our research integrity team to safeguard the quality of each article we publish.
Find out more
MINI REVIEW article
Front. Chem. Eng. , 13 January 2022
Sec. Sustainable Process Engineering
Volume 3 - 2021 | https://doi.org/10.3389/fceng.2021.803513
This article is part of the Research Topic Optimization Methods for Biorefineries and Bio-based Supply Chains View all 7 articles
Recent techno-economic analysis (TEA) has underscored that for algal biofuels to be cost competitive with petroleum fuels, co-products are necessary to offset the cost of fuel production. The co-product suite must scale with fuel production while also maximizing value from the non-fuel precursor components. The co-product suite also depends on algal biomass composition, which is highly dynamic and depends on environmental conditions during cultivation. Intentional shifts in composition during cultivation are often associated with reduced biomass productivity, which can increase feedstock production costs for the algae-based biorefinery. The optimal algae-based biorefinery configuration is thus a function of many factors. We have found that comprehensive TEA, which requires the construction of process models with detailed mass and energy balances, along with a complete accounting of capital and operating expenditures for a commercial-scale production facility, provides invaluable insight into the viability of a proposed biorefinery configuration. This insight is reflected in improved viability for one biorefining approach that we have developed over the last 10 years, namely, the Combined Algal Processing (CAP) approach. This approach fractionates algal biomass into carbohydrate-, lipid-, and protein-rich fractions, and tailors upgrading chemistry to the composition of each fraction. In particular, transitioning from valorization of only the lipids to a co-product suite from multiple components of high-carbohydrate algal biomass can reduce the minimum fuel selling price (MFSP) from more than $8/gallon of gasoline equivalent (GGE) to $2.50/GGE. This paper summarizes that progress and discusses several surprising implications in this optimization approach.
The production of economically competitive algal biofuels has been a goal of the algae community for decades. Algae, as a nascent agricultural bioenergy crop, promise faster growth rates and higher fuel yields per unit land area than terrestrial crops and, because algae can grow in brackish or saltwater in locations not suitable for terrestrial agriculture, algal biomass can be produced in a way that does not compete with food crops. However, despite this potential, algal biofuels remain in a pre-commercial state, with modeled fuel selling prices indicating commercial production with current technology would be considerably more expensive than either petroleum-based fuels or terrestrial crop-based biofuels. Several factors contribute to this situation, including the cost of cultivation, the composition of the algae, and processing costs required to produce fuels and co-products from the algal biomass.
Since 2014, research efforts by national laboratories in the United States to quantify algal biofuel production costs have resulted in annual “State-of-Technology” (SOT) and design reports summarizing the current status of each of these factors based on published literature, experimental results, and industry consultation. These SOT reports describe detailed process models to quantify capital and operating costs, including mass and energy balances, for a model algae farm and biorefinery configuration (Davis et al., 2018). Modeling efforts are broadly divided into cultivation (covering all aspects of biomass production and dewatering) and conversion (extending from seasonal storage of dewatered biomass to finished fuels and co-products). All capital and operating expenditures for the combined cultivation/conversion facility are accounted for in a discounted cash flow rate of return analysis, allowing for the determination of the minimum biomass or fuel selling price (MBSP or MFSP) required to obtain a 10% internal rate of return for the facility. The scale of the conversion facility is consistent with a 5000-acre cultivation farm producing a single algae species, Scenedesmus acutus, with a composition high in fermentable carbohydrates. This cultivation facility corresponds to roughly 500 dry tons of algae biomass produced per day. Notably, the cultivation models have focused on open pond systems, as the higher culture densities and lower risk of contamination enabled by closed photobioreactor systems are at this time not enough to overcome the much higher capital costs for closed systems, at least with current technology (Clippinger and Davis, 2019).
Conversion pathway research and technology reports have generally followed one of two primary pathways. One is a fractionation approach termed Combined Algal Processing (CAP). The CAP approach uses pretreatment operations for cell disruption and carbohydrate hydrolysis, and lipid extraction operations to fractionate algal biomass into an organic lipid fraction, an aqueous hydrolysate, and a residual solid. The second approach is hydrothermal liquefaction (HTL), which also produces organic, aqueous, and solid phases, though yield to the latter two is typically minimized in favor of the organic phase, sometimes termed biocrude or bio-oil (Zhu et al., 2021). In the CAP approach, these aqueous and solid phases represent integral intermediates that can be further upgraded to either fuels or chemical co-products, as shown in Figure 1. Similarly, the organic lipid fraction can be upgraded to fuels, co-products, or both. This flexibility in the CAP approach affords multiple options to achieve MFSP targets through the possibility to valorize one or more constituents of the algal biomass to either fuels or co-products as best suited for the incoming biomass cost and composition. This manuscript focuses on the CAP approach as an example of using TEA to optimize process design and identify research gaps, though we note that a similar approach is also relevant to HTL and other algal conversion pathways.
FIGURE 1. Process flow diagram for upgrading algal biomass by the CAP approach. Adapted from Wiatrowski and Davis(Wiatrowski and Davis, 2021).
Within the CAP approach, we have found that adapting the valorization model to a proposed process configuration, coupled with experimental data to validate the model, provides invaluable insight into the relative feasibility of a given biorefinery configuration. Using this approach, we have found that sequential modification of the original 2014 CAP valorization model has decreased the projected MFSP from almost $8/gallon of gasoline equivalent (GGE) to less than $2.50/GGE, as shown in Figure 2. This approach has quantified the necessity of high-value, large-volume co-products to subsidize fuel production, as well as the surprisingly large influence of minimizing material losses. This mini-review summarizes the key findings of the annual SOT reports that have led to the decrease in MFSP for the CAP approach to algae biorefining.
FIGURE 2. Projected algal biofuel selling prices from CAP approach, adapted from Pienkos (Pienkos, 2019). Model details are available in various published reports (Davis et al., 2014; Davis et al., 2018; Davis and Wiatrowski, 2020; Wiatrowski and Davis, 2021), with process parameters harmonized to the basis reported in the 2019 SOT report (Davis et al., 2020a).
While early modeling efforts focused on projections for high-lipid algal biomass, typically referring to a total fatty acid content of the biomass of over 30% of the biomass, more recent work has indicated that a significant cost is associated with the production of such biomass (Dong et al., 2016a; Davis et al., 2018; Li et al., 2019; Wiatrowski and Davis, 2021). The targeted biomass costs of $480–500/ton were not realistic for a high-lipid composition due to the long nutrient-deplete cultivation time required for lipid accumulation without increasing biomass and the corresponding decrease in effective growth rate. Alternatively, genetic and metabolic engineering strategies are being developed to simultaneously increase carbon storage in the biomass as lipid, which may have potential to reduce the productivity cost burden in creating a bioenergy-relevant biomass composition (Ajjawi et al., 2017). Growth studies with highly productive wild-type species in an outdoor setting indicated that a high carbohydrate content may be feasible with potential cost advantages depending on the intrinsic value assigned to the carbohydrates (Laurens, 2021a; Davis, 2021), though currently the biomass harvested from outdoor open pond cultivation is more likely to be high in protein content and low in carbohydrates and lipids under typical nutrient-replete cultivation conditions (Davis and Klein, 2021). Notably, if a high carbohydrate content can be achieved, algae-derived hydrolysate fermentations akin to those explored for lignocellulosic hydrolysates become viable, and fuel or bioproduct production can occur through multiple pathways (Lim et al., 2021). Often, algal protein valorization invokes the production of animal feed or human food, though a hydrothermal liquefaction (HTL) process can convert proteins to biocrude oil, which can be catalytically upgraded to biofuel (Palardy et al., 2017). This pathway typically creates crude oils that contain high levels of heteroatoms, such as nitrogen, oxygen and sulfur, making the catalysis and upgrading more challenging and the overall process less flexible to fully valorize different biomass components as is the case for a fractionation process. Alternative pathways for converting protein to biofuels or bioproducts are much less developed but under investigation. Thus, there exist two primary technology gaps in algae biorefining: the technology to shift the biomass composition from high-protein to high-carbohydrate or high-lipid to maximize the storage of easily accessible, bioenergy-relevant components without losing productivity, and the technology to convert proteins to hydrocarbon fuels or other co-products while simultaneously supporting nutrient recycling strategies. Both areas are under investigation but fall outside of the scope of this review article.
Early TEA also indicated the necessity of load-leveling to store biomass from high-productivity months (spring and summer) for conversion to fuels and chemicals in low-productivity months. That is, the cost of extra capital investment for algae storage at a biorefinery and the operating cost of partial drying of some biomass was lower than the cost of sizing conversion equipment to handle spring and summer productivity and allowing some conversion capacity to sit idle in the fall and winter. Subsequently, preliminary studies indicated that ensiling the algal biomass (anaerobic storage of wet biomass, avoiding cost and energy penalties associated with drying, “Wet Storage (Peak Season)” block of Figure 1) was not detrimental to the conversion operations (Wendt et al., 2020). Thus, more recent modeling efforts have adopted the wet anaerobic storage approach for mitigating seasonal variability through conversion. Alternatively, algal biomass in low-productivity months may be supplemented with non-algae feedstocks of lower cost and nominally-similar composition such as brown grease and coffee grounds (Pereira et al., 2020; Spiller et al., 2020). These potential feedstocks are less seasonal, but developing a consistent supply chain (especially for spent coffee grounds) to balance the seasonal algae productivity variations is likely to be logistically challenging.
The pretreatment step for algal biomass serves two purposes. First, it disrupts cells so that intracellular lipids can be extracted. Second, it solubilizes carbohydrates and some proteins to produce a fermentable hydrolysate. Many technologies have been applied for algae cell lysis, though not always in a fuel production or whole biomass valorization context (Teymouri et al., 2018). Among those that have, acid pretreatment is among the most promising (Yu et al., 2015; Kruger et al., 2018). In particular, algal carbohydrates are readily hydrolyzed to fermentable monomeric sugars under acidic conditions, while the low pH assists in lipid extraction by keeping free fatty acids (occasionally observed in high quantities and likely due to action of lipases during harvesting and storage) protonated and denaturing emulsion-forming proteins that may inhibit lipid extraction (Dong et al., 2016b).
Lipid extraction was initially accomplished by simple hexane extraction as industrially practiced for extraction of lipids from dry terrestrial oilseed crops such as soybeans. However, with a wet feedstock such as algae, laboratory studies indicated that hexane alone was insufficient to completely recover lipids. In addition to forming emulsions, hexane was unable to completely access lipids trapped in hydrophilic flocs. Incorporating these results into TEA models indicated that the lost lipids imposed a severe economic penalty on the overall process, and thus alternative solvents were explored. The best lipid recovery was found with an ethanol-hexane mixture, with the ethanol assisting phase transfer of the lipids while reducing emulsion formation (Davis and Wiatrowski, 2020). The modest increase in lipid recovery more than offset the additional cost and energy demands required to recover ethanol through an additional distillation step beyond hexane alone.
Algal lipids contain fatty acids with chain lengths ranging from C8 to C24, with the majority consisting of C16 and C18 acids containing 1-3 double bonds. Additionally, lipids are readily extractable as triacylglycerides (TAGs) from some strains of algae, while others are extracted primarily as free fatty acids (FFAs). The different chain lengths and degrees of unsaturation allow for fractionation of the lipids into a portion that is highly suitable for fuel production and a portion that is more suited for co-products. In particular, long-chain, highly-unsaturated lipids fall outside the range of the most desirable fuels (jet fuel range of C9-C14 and diesel fuel range of C12-C20) and would require additional hydrogen for double bond saturation. Instead, these unsaturated fatty acids (or esters thereof) can be routed to high-value co-products such as conventional or nonisocyanate polyurethanes (Dong et al., 2021). As shown in Figure 2, routing some lipids to polyurethane co-products can reduce the MFSP by more than $5/GGE (Davis et al., 2020b; Dong et al., 2021; Wiatrowski and Davis, 2021).
At the same time, the saturated lipid fraction may be converted to fuels using similar technology as that used for terrestrial plant oil hydroprocessing. By reaction and catalyst engineering, the product spectrum may be tuned toward renewable diesel or aviation fuel. In particular, the oils may be hydroprocessed to jet- and diesel-range hydrocarbons using a two-step hydroprocessing configuration over sequential Pd/C and Pt/SAPO-11 or Pt/USY catalysts (Robota et al., 2013; Kruger et al., 2017), though longer reaction time-on-stream experiments are needed to validate the catalyst stability. In contrast to terrestrial vegetable oil, extracted algal oils usually contain high levels of impurities, such as chlorophyll, nitrogen, and sulfur, though lower levels of these contaminants than the biocrude oils produced by HTL. Purification of the lipid stream may be necessary to increase the yield and catalyst life. Alternatively, purifying the lipids for polymer production, e.g., by distillation, may concomitantly provide a clean lipid stream for hydroprocessing to hydrocarbon fuels (Kruger et al., 2021). The removed impurities (e.g. pigments) might be used for value-added co-products to further reduce the cost.
Algal carbohydrates, proteins, and other materials solubilized during pretreatment have proven to be highly fermentable. Algal hydrolysates were successfully fermented to ethanol (Dong et al., 2016c; Knoshaug et al., 2018a), succinic acid (Knoshaug et al., 2018a; Knoshaug et al., 2018b), butyric acid (Wendt et al., 2020), muconic acid (Kruger, 2021), and 1,3-butanediol (BDO) (Laurens, 2021b) whether cultivated in freshwater or saltwater and with or without a nutrient-depletion step to enable accumulation of additional carbohydrates. Early TEA models investigated the economic potential to increase total fuel outputs via simple and low-cost fermentation to ethanol, though subsequent models suggested that fermentation to higher-value succinic acid had better potential to further decrease MFSP. As shown in Figure 2, the addition of ethanol as co-product resulted in a significant reduction in MFSP, and an even greater reduction was observed when the higher value compound, succinic acid, was evaluated. However, converting the carbohydrates to a co-product instead of a second fuel product significantly decreased the fuel yield per mass of algae. Fermentation instead to butyric acid or BDO, as precursors to hydrocarbon fuels, increased MFSP due to lower fermentation yields and more complex downstream operations along the fuel train than for ethanol, without the benefit of a high-value co-product such as succinic acid. However, incorporating the carboxylic acid or BDO fermentation pathways with a polyurethane co-product from lipids offered an optimal balance of fuel yield and co-product value, as shown in Figure 2, while also supporting the production of drop-in hydrocarbon fuels rather than ethanol. What is clear from this analysis is that the product stream from an algae biorefinery can be optimized for minimum MFSP or maximum fuel yield, but not both simultaneously.
Alternatively, the whole pretreated slurry can be fermented, either before or after lipid extraction. In this approach, water usage may be reduced because the residual solids do not need to be washed to recover fermentable hydrolysate, and microbes may be able to make use of oligomeric sugars and proteins that they would not otherwise have access to if solid-liquid separation and solid rinsing occurred prior to fermentation. While lipids survive the fermentation process, at least in the cases we have investigated, increased capital costs for larger fermenters that can handle high solids loadings are only justified in specific cases where solid-liquid separation would not otherwise be required (e.g., fermentation to a product amenable to distillation, such as ethanol). In other cases, the presence of the solids and lipids would complicate selective recovery of the fermentation product. Thus, TEA has found the process configuration shown in Figure 1 to be most advantageous, at least in a case where solid-liquid separation is required for downstream processing following fermentation (carboxylic acids, BDO).
The extracted solids contain significant amounts of ash, lipids, carbohydrates, proteins, and other organics, and as such are a suitable substrate for anaerobic digestion (AD) (Zhao et al., 2014; Ma et al., 2015). This process facilitates N and P recycle to the algae growth ponds (Kim et al., 2015; Kim et al., 2017), while also producing a biogas product capable of offsetting a significant portion of the algal biorefinery energy demand. Additionally, the CO2 (produced both during digestion and during combustion of the biogas) can be recycled to the pond to promote additional algae growth and reduce fresh CO2 cultivation demands. While the cost of CO2 to promote growth is relatively small compared to capital costs of the infrastructure required to grow and harvest the algae biomass, this is dependent on the CO2 retention efficiency in the cultivation system, and the cost of nutrients is somewhat larger. The circularity of both nutrients (N and P) and carbon in the process is one of the primary environmental drivers of overall process sustainability. Similarly, any excess biogas can be upgraded to renewable natural gas or can be utilized directly on-site to generate electricity. Alternatively, the high protein content of these solids may be leveraged in a number of applications (similar to whole high-protein algal biomass), including food and feed, fertilizer, and biomaterial production. While there may be significant market barriers for feed and agricultural operations, including suboptimal nutritional profiles and toxicity issues, biomaterials and polymers may find more ready acceptance. In particular, we have found that post extracted biomass can be used for bioplastic production to further reduce the cost of biofuel production and offset petroleum-based feedstocks in some polymer materials (Beckstrom et al., 2020).
Combined Algal Processing provides a flexible framework for valorization of algae with varying composition. In particular, by producing an organic lipid phase, an aqueous hydrolysate phase, and a residual solids phase, the conversion of each phase can be optimally tuned to their individual chemistries to produce both fuels and co-products. Because of this flexibility, process modeling and economic analysis are essential components to guide process development in terms of both the configuration of the unit operations and the suite of fuels and co-products.
In particular, economic analysis shows that the cost of the biomass remains the primary cost driver on overall integrated system economics for fuel and co-product production, especially for nutrient deplete (high-lipid or high-carbohydrate) biomass. Thus, the more deplete this biomass, the higher the value required from co-products to offset a higher biomass production cost. While somewhat species dependent, this analysis has indicated that high-lipid biomass, which typically requires longer cultivation times to achieve than high-carbohydrate biomass, will be particularly challenged to produce economically-viable biofuels unless significant strain engineering is able to mitigate the negative impacts on biomass productivity rate and maintain high productivities concomitantly with high lipid compositions. Similarly, while the carbohydrate-rich hydrolysate can be fermented to a variety of co-products and fuel precursors, the fermentation targets must balance the costs of the fermentation itself (aerobic vs anaerobic, rates, titers, yields), the required downstream operations for separations and/or fuel production, as well as the market size and value for co-products, to ensure that they scale with fuel production. It is perhaps worth noting that a completely different set of drivers exist to evaluate algal biorefinery concepts that do not include biofuels as an essential product.
Considering the techno-economic implications of our experimental results has produced several insightful findings in our research, including the applicability of wet algae storage to mitigate seasonality, the benefit of using a multicomponent solvent mix for lipid extraction, and the large impact of a polyurethane co-product from a relatively small fraction of the biomass. These findings invite further opportunities for cost tradeoffs when considering high-value co-product options from presently-underutilized fractions.
In addition to optimizing process configurations and target product suites, economic analysis has informed the most impactful research directions. In particular, it has been shown that two complementary research directions are urgently needed to enable economic viability (Davis et al., 2018): production of high lipid/carbohydrate biomass with satisfactory productivity and developing conversion technology for high-protein compositions which are cost-effective and produce sufficient yields of fuels and co-products. Additionally, the residual solids are somewhat underutilized, even with the environmentally-favorable circularity enabled by anaerobic digestion. If the carbon in this fraction could be routed to long-term sequestration as an additional co-product while still recycling the N and P as nutrients, the environmental benefits may remain while improving overall economics. Similarly, identification and valorization of other underutilized fractions (e.g., non-fermentable carbon in the hydrolysate, residues from lipid purification) represent opportunities to further improve economics, provided that the cost of valorization is more than offset by the achievable value.
Overall, techno-economic analysis has proven to be an extremely valuable tool in optimizing algal biorefineries in our lab over the last 10 years, and in informing promising research directions for the future.
JK wrote the original draft with support from RD and PP for Figure concepts. MW, RD, TD, EK, NN, LL and PP provided edits and input on intermediate drafts. JK compiled edits into the final version. PP and JK were the leads of projects that provided the basis of this work. Other authors were involved in those projects. All authors contributed to the article and approved the submitted version.
This work was authored in part by the National Renewable Energy Laboratory and financially supported by the U.S. Department of Energy under Contract No. DE-AC36-08GO28308 with the National Renewable Energy Laboratory, as part of the DOE Office of Energy Efficiency and Renewable Energy, Bioenergy Technologies Office. The views expressed in the article do not necessarily represent the views of the DOE or the U.S. Government. The U.S. Government retains and the publisher, by accepting the article for publication, acknowledges that the U.S. Government retains a nonexclusive, paid-up, irrevocable, world-wide license to publish or reproduce the published form of this work, or allow others to do so, for U.S. Government purposes.
Author PP is associated with Polaris Renewables, a company working to commercialize NIPU technology.
The remaining authors declare that the research was conducted in the absence of any commercial or financial relationships that could be construed as a potential conflict of interest.
All claims expressed in this article are solely those of the authors and do not necessarily represent those of their affiliated organizations, or those of the publisher, the editors and the reviewers. Any product that may be evaluated in this article, or claim that may be made by its manufacturer, is not guaranteed or endorsed by the publisher.
Ajjawi, I., Verruto, J., Aqui, M., Soriaga, L. B., Coppersmith, J., Kwok, K., et al. (2017). Lipid Production in Nannochloropsis Gaditana Is Doubled by Decreasing Expression of a Single Transcriptional Regulator. Nat. Biotechnol. 35 (7), 647–652. doi:10.1038/nbt.3865
Beckstrom, B. D., Wilson, M. H., Crocker, M., and Quinn, J. C. (2020). Bioplastic Feedstock Production from Microalgae with Fuel Co-products: A Techno-Economic and Life Cycle Impact Assessment. Algal Res. 46, 101769. doi:10.1016/j.algal.2019.101769
Clippinger, J. N., and Davis, R. E. (2019). Techno-economic Analysis for the Production of Algal Biomass via Closed Photobioreactors: Future Cost Potential Evaluated across a Range of Cultivation System Designs (Golden, CO (United States): National Renewable Energy Lab.(NREL)). NREL/TP-5100-72716. doi:10.2172/1566806
Davis, R., Bartling, A., and Tao, L. (2020). Biochemical Conversion of Lignocellulosic Biomass to Hydrocarbon Fuels and Products: 2019 State of Technology and Future Research (Golden, CO (United States): National Renewable Energy Lab (NREL)). No. NREL/TP-5100-76567. doi:10.2172/1659894
Davis, R. E. (2021). “Algal Biofuels Techno-Economic Analysis,” in DOE Bioenergy Technologies Office 2021 Project Peer Review (US Department of Energy).
Davis, R. E., Markham, J. N., Kinchin, C. M., Canter, C., Han, J., Li, Q., et al. (2018). 2017 Algae Harmonization Study: Evaluating the Potential for Future Algal Biofuel Costs, Sustainability, and Resource Assessment from Harmonized Modeling (Golden, CO (United States): National Renewable Energy Lab.(NREL)). doi:10.2172/1468333
Davis, R., Kinchin, C., Markham, J., Tan, E. C. D., Laurens, L. M. L., Sexton, D., et al. (2014). Process Design and Economics for the Conversion of Algal Biomass to Biofuels: Algal Biomass Fractionation to Lipid- and Carbohydrate-Derived Fuel Products (Golden, CO: National Renewable Energy Laboratory). NREL/TP-5100-62368. doi:10.2172/1271650
Davis, R., and Klein, B. (2021). Algal Biomass Production via Open Pond Algae Farm Cultivation: 2020 State of Technology and Future Research (United States. doi:10.2172/1784890
Davis, R., and Wiatrowski, M. (2020). Algal Biomass Conversion to Fuels via Combined Algae Processing (CAP): 2019 State of Technology and Future Research (Golden, CO (United States): National Renewable Energy Lab.(NREL)). NREL/TP-5100-76568. doi:10.2172/1659895
Davis, R., Wiatrowski, M., Kinchin, C., and Humbird, D. (2020). Conceptual Basis and Techno-Economic Modeling for Integrated Algal Biorefinery Conversion of Microalgae to Fuels and Products (2019 NREL TEA Update: Highlighting Paths to Future Cost Goals via a New Pathway for Combined Algal Processing) (Golden, CO (United States): National Renewable Energy Lab.(NREL)). doi:10.2172/1665822
Dong, T., Dheressa, E., Wiatrowski, M., Pereira, A. P., Zeller, A., Laurens, L. M. L., et al. (2021). Assessment of Plant and Microalgal Oil-Derived Nonisocyanate Polyurethane Products for Potential Commercialization. ACS Sustain. Chem. Eng. 9 (38), 12858–12869. doi:10.1021/acssuschemeng.1c03653
Dong, T., Knoshaug, E. P., Davis, R., Laurens, L. M. L., Van Wychen, S., Pienkos, P. T., et al. (2016). Combined Algal Processing: A Novel Integrated Biorefinery Process to Produce Algal Biofuels and Bioproducts. Algal Res. 19, 316–323. doi:10.1016/j.algal.2015.12.021
Dong, T., Knoshaug, E. P., Pienkos, P. T., and Laurens, L. M. L. (2016). Lipid Recovery from Wet Oleaginous Microbial Biomass for Biofuel Production: A Critical Review. Appl. Energ. 177, 879–895. doi:10.1016/j.apenergy.2016.06.002
Dong, T., Van Wychen, S., Nagle, N., Pienkos, P. T., and Laurens, L. M. L. (2016). Impact of Biochemical Composition on Susceptibility of Algal Biomass to Acid-Catalyzed Pretreatment for Sugar and Lipid Recovery. Algal Res. 18, 69–77. doi:10.1016/j.algal.2016.06.004
Kim, G.-Y., Yun, Y.-M., Shin, H.-S., and Han, J.-I. (2017). Cultivation of Four Microalgae Species in the Effluent of Anaerobic Digester for Biodiesel Production. Bioresour. Technol. 224, 738–742. doi:10.1016/j.biortech.2016.11.048
Kim, G.-Y., Yun, Y.-M., Shin, H.-S., Kim, H.-S., and Han, J.-I. (2015). Scenedesmus-based Treatment of Nitrogen and Phosphorus from Effluent of Anaerobic Digester and Bio-Oil Production. Bioresour. Technol. 196, 235–240. doi:10.1016/j.biortech.2015.07.091
Knoshaug, E. P., Dong, T., Spiller, R., Nagle, N., and Pienkos, P. T. (2018). Pretreatment and Fermentation of Salt-Water Grown Algal Biomass as a Feedstock for Biofuels and High-Value Biochemicals. Algal Res. 36, 239–248. doi:10.1016/j.algal.2018.10.024
Knoshaug, E. P., Mohagheghi, A., Nagle, N. J., Stickel, J. J., Dong, T., Karp, E. M., et al. (2018). Demonstration of Parallel Algal Processing: Production of Renewable Diesel Blendstock and a High-Value Chemical Intermediate. Green. Chem. 20 (2), 457–468. doi:10.1039/C7GC02295F
Kruger, J. S. (2021). “CAP Process Research,” in DOE Bioenergy Technologies Office 2021 Project Peer Review (US Department of Energy).
Kruger, J. S., Christensen, E. D., Dong, T., Van Wychen, S., Fioroni, G. M., Pienkos, P. T., et al. (2017). Bleaching and Hydroprocessing of Algal Biomass-Derived Lipids to Produce Renewable Diesel Fuel. Energy Fuels 31 (10), 10946–10953. doi:10.1021/acs.energyfuels.7b01867
Kruger, J. S., Cleveland, N. S., Yeap, R. Y., Dong, T., Ramirez, K. J., Nagle, N. J., et al. (2018). Recovery of Fuel-Precursor Lipids from Oleaginous Yeast. ACS Sustain. Chem. Eng. 6 (3), 2921–2931. doi:10.1021/acssuschemeng.7b01874
Kruger, J. S., Knoshaug, E. P., Dong, T., Hull, T. C., and Pienkos, P. T. (2021). Catalytic Hydroprocessing of Single-Cell Oils to Hydrocarbon Fuels : Converting Microbial Lipids to Fuels Is a Promising Approach to Replace Fossil Fuels. Johnson Matthey Technol. Rev. 65 (2), 227–246. doi:10.1595/205651321X16024905831259
Laurens, L. M. L. (2021). “Algae Biomass Composition,” in DOE Bioenergy Technologies Office 2021 Project Peer Review (US Department of Energy).
Laurens, L. M. L. (2021). “Rewiring Algal Carbon Energetics for Renewables,” in DOE Bioenergy Technologies Office 2021 Project Peer Review (US Department of Energy).
Li, Y., Leow, S., Dong, T., Nagle, N. J., Knoshaug, E. P., Laurens, L. M. L., et al. (2019). Demonstration and Evaluation of Hybrid Microalgae Aqueous Conversion Systems for Biofuel Production. ACS Sustain. Chem. Eng. 7 (6), 5835–5844. doi:10.1021/acssuschemeng.8b05741
Lim, J. H. K., Gan, Y. Y., Ong, H. C., Lau, B. F., Chen, W.-H., Chong, C. T., et al. (2021). Utilization of Microalgae for Bio-Jet Fuel Production in the Aviation Sector: Challenges and Perspective. Renew. Sustain. Energ. Rev. 149, 111396. doi:10.1016/j.rser.2021.111396
Ma, J., Zhao, Q.-B., Laurens, L. L. M., Jarvis, E. E., Nagle, N. J., Chen, S., et al. (2015). Mechanism, Kinetics and Microbiology of Inhibition Caused by Long-Chain Fatty Acids in Anaerobic Digestion of Algal Biomass. Biotechnol. Biofuels 8 (1), 141. doi:10.1186/s13068-015-0322-z
Palardy, O., Behnke, C., and Laurens, L. M. L. (2017). Fatty Amide Determination in Neutral Molecular Fractions of Green Crude Hydrothermal Liquefaction Oils from Algal Biomass. Energy Fuels 31 (8), 8275–8282. doi:10.1021/acs.energyfuels.7b01175
Pereira, A. P., Dong, T., Knoshaug, E. P., Nagle, N., Spiller, R., Panczak, B., et al. (2020). An Alternative Biorefinery Approach to Address Microalgal Seasonality: Blending with Spent Coffee Grounds. Sustain. Energ. Fuels 4 (7), 3400–3408. doi:10.1039/D0SE00164C
Pienkos, P. T. (2019). “Combined Algae Processing (CAP) Process Research,” in DOE Bioenergy Technologies Office 2019 Project Peer Review, Denver, CO, USA, November 2016 (US Department of Energy).
Robota, H. J., Alger, J. C., and Shafer, L. (2013). Converting Algal Triglycerides to Diesel and HEFA Jet Fuel Fractions. Energy Fuels 27 (2), 985–996. doi:10.1021/ef301977b
Spiller, R., Knoshaug, E. P., Nagle, N., Dong, T., Milbrandt, A., Clippinger, J., et al. (2020). Upgrading Brown Grease for the Production of Biofuel Intermediates. Bioresour. Technol. Rep. 9, 100344. doi:10.1016/j.biteb.2019.100344
Teymouri, A., Adams, K. J., Dong, T., and Kumar, S. (2018). Evaluation of Lipid Extractability after Flash Hydrolysis of Algae. Fuel 224, 23–31. doi:10.1016/j.fuel.2018.03.044
Wendt, L. M., Wahlen, B. D., Knoshaug, E. P., Nagle, N. J., Dong, T., Spiller, R., et al. (2020). Anaerobic Storage and Conversion of Microalgal Biomass to Manage Seasonal Variation in Cultivation. ACS Sustain. Chem. Eng. 8 (35), 13310–13317. doi:10.1021/acssuschemeng.0c03790
Wiatrowski, M., and Davis, R. (2021). Algal Biomass Conversion to Fuels via Combined Algae Processing (CAP): 2020 State of Technology and Future Research (Golden, CO (United States): National Renewable Energy Lab.(NREL)). doi:10.2172/1784888
Yu, X., Dong, T., Zheng, Y., Miao, C., and Chen, S. (2015). Investigations on Cell Disruption of Oleaginous Microorganisms: Hydrochloric Acid Digestion Is an Effective Method for Lipid Extraction. Eur. J. Lipid Sci. Technol. 117 (5), 730–737. doi:10.1002/ejlt.201400195
Zhao, B., Ma, J., Zhao, Q., Laurens, L., Jarvis, E., Chen, S., et al. (2014). Efficient Anaerobic Digestion of Whole Microalgae and Lipid-Extracted Microalgae Residues for Methane Energy Production. Bioresour. Technol. 161, 423–430. doi:10.1016/j.biortech.2014.03.079
Zhu, Y., Jones, S. B., Schmidt, A. J., Job, H. M., Billing, J. M., Collett, J. R., et al. (2021). Microalgae Conversion to Biofuels and Biochemical via Sequential Hydrothermal Liquefaction (SEQHTL) and Bioprocessing: 2020 State of Technology (Richland, WA (United States): Pacific Northwest National Lab (PNNL)). PNNL-30124. doi:10.2172/1784347
Keywords: algae, biorefining, techno-economic analyisis, co-products, bio-based products, renewable diesel, sustainable aviation fuel
Citation: Kruger JS, Wiatrowski M, Davis RE, Dong T, Knoshaug EP, Nagle NJ, Laurens LML and Pienkos PT (2022) Enabling Production of Algal Biofuels by Techno-Economic Optimization of Co-Product Suites. Front. Chem. Eng. 3:803513. doi: 10.3389/fceng.2021.803513
Received: 28 October 2021; Accepted: 10 December 2021;
Published: 13 January 2022.
Edited by:
Aristide Giuliano, ENEA - Centro Ricerche Trisaia, ItalyReviewed by:
Cristina Cavinato, Ca’ Foscari University of Venice, ItalyCopyright © 2022 Kruger, Wiatrowski, Davis, Dong, Knoshaug, Nagle, Laurens and Pienkos. This is an open-access article distributed under the terms of the Creative Commons Attribution License (CC BY). The use, distribution or reproduction in other forums is permitted, provided the original author(s) and the copyright owner(s) are credited and that the original publication in this journal is cited, in accordance with accepted academic practice. No use, distribution or reproduction is permitted which does not comply with these terms.
*Correspondence: Jacob S. Kruger, SmFjb2IuS3J1Z2VyQG5yZWwuZ292
Disclaimer: All claims expressed in this article are solely those of the authors and do not necessarily represent those of their affiliated organizations, or those of the publisher, the editors and the reviewers. Any product that may be evaluated in this article or claim that may be made by its manufacturer is not guaranteed or endorsed by the publisher.
Research integrity at Frontiers
Learn more about the work of our research integrity team to safeguard the quality of each article we publish.