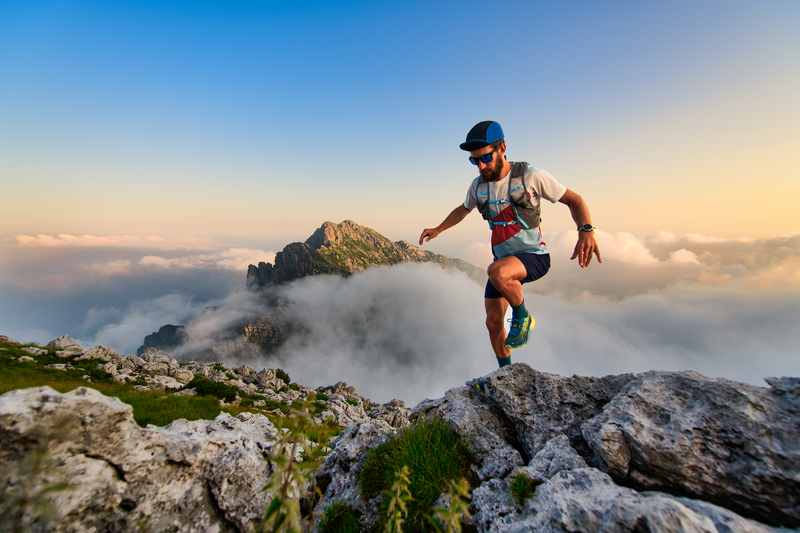
95% of researchers rate our articles as excellent or good
Learn more about the work of our research integrity team to safeguard the quality of each article we publish.
Find out more
ORIGINAL RESEARCH article
Front. Chem. Eng. , 16 December 2021
Sec. Materials Process Engineering
Volume 3 - 2021 | https://doi.org/10.3389/fceng.2021.787788
This article is part of the Research Topic Hierarchical and Multifunctional Materials in Chemical Engineering: Synthesis Strategies and Processing Challenges View all 7 articles
The sol–gel process accompanied by phase separation is one of the methods to prepare hierarchically porous monoliths, hierarchically porous monolith, which is applicable not only to oxides but also to various materials compositions such as metal phosphates, organic-polymers/carbons, metal-organic frameworks. It is not until recently, however, that progress has been made in the preparation of low-valence metal oxide HPMs, such as those of magnesium, manganese, cobalt, nickel, etc. Due to the difficulty of divalent metal precursors to form homogeneous gels, different approaches from those established for trivalent and tetravalent counterparts have been attempted. This short review introduces the methods and trials in the preparation of metal oxide HPMs from divalent metal salts.
Sol–gel process, in general, is a method for producing solid materials with controllable particles sizes at ambient temperature from small molecular precursors in a liquidus medium. Upon hydrolysis or any other activation, the precursor molecules polymerize, forming chemical bonds with each other to result dimers, trimers, oligomers, and then polymers. When such polymerized species (particle size: d = 1–1,000 nm) are dissolved/dispersed homogeneously in the system, the system is termed sol. When the polymerized species form an integrated network across the entire vessel, the system is termed gel, and the phenomenon is termed gelation. The important feature of gelation, the transition from sol to gel, is the sharp increase in viscosity, where the system loses macroscopic fluidity. Depending on the mobility of the system and the quickness of such spatial freezing, the gelation can capture the transition state of evolving phases when the phase separation occurs.
The overall reactions for tetraethoxysilane (TEOS), the most classical system in sol–gel research, are expressed in Figure 1. Since the isoelectric point (IEP) of silica/siloxane spices lies pH 2,3, the reaction is regarded to take place under acid-catalyzed conditions when pH < 2; and under base-catalyzed conditions when pH > 3. Under acid-catalyzed hydrolysis, the protonation of oxygen is a rate-determining step, followed by leaving of ethanol. Water- or alcohol-producing condensation is also catalyzed by acid so that the gelation time shortens as pH decreases below pH 2. Under base-catalyzed conditions, the nucleophilic attack silicon atom governs both hydrolysis and condensation. Due to relatively small partial charge values on O and Si (compared with other metals such as Ti and Zr), both electrophilic attack on O under acid-catalyzed condition and nucleophilic attack on Si under base-catalyzed condition is moderate, leading silicon alkoxides to exhibit slow hydrolysis and polycondensation kinetics.
Unless specially designed, gels prepared by sol–gel process generally possess only unimodal pores, which are the interstices of the structural unit of the gel network. The pore size ranges from a few to hundreds of nanometers that can be controlled by the conditions of sol–gel transition, aging, drying, heat-treatment, and so on. In order to obtain hierarchically porous structures, it is necessary to introduce other methods, such as (supra-)molecular template and phase separation, into the sol–gel process.
Spinodal decomposition is a mode of phase separation distinct from nucleation/growth, in which any small compositional fluctuation grows without an activation barrier. Compared with the nucleation/growth process that requires thermal activation, the spinodal decomposition generates transient phase domains spontaneously. One of the typical morphologies seen in isotropic spinodal decomposition with comparable volume fractions of two phases is co-continuous structure, where both separated conjugate phases are interconnected.
When the spinodal decomposition is combined with a sol–gel transition, a hierarchically porous monolith (HPM) can be obtained, in which larger pores are formed by phase separation and smaller pores by network formation via sol–gel process. However, it is not a simple mission to concertedly induce gelation and phase separation, since the chemical-bond formation governs the entire process. Therefore, it is necessary to design elaborately the starting composition for inducing the phase separation during the sol–gel transition and ensuring the gelation happen at the desired phase-separated state (Figure 2). About 3 decades ago, Nakanishi et al. prepared silica HPM via sol–gel process accompanied by phase separation for the first time (Nakanishi and Soga, 1991). Various factors on the control of the phase-separated morphology in the silica system, including precursor, solvent composition, additive component, temperature, and so on, have been investigated (Nakanishi and Soga, 1992a; Nakanishi and Soga, 1992b; Nakanishi and Soga, 1992c; Nakanishi and Soga, 1992d; Kaji et al., 1993; Nakanishi et al., 1994; Kaji et al., 1955; Takahashi et al., 1995; Nakanishi, 1997; Nakanishi and Soga, 1997; Nakanishi et al., 1998). The driving force of phase separation due to polymerization reaction can be explained by Flory–Huggins Eq. 1.
where R denotes the gas constant, T denotes the temperature, Φ and P denote respectively the volume fractions and the polymerization degree of gel-rich phase (i = g) and solvent-rich phase (i = s), and χ the interaction parameter which measures the compatibility between the components. The entropic term,
Inspired by the success of silica HPM, the preparation of metal oxide HPM has been explored. Titanium alkoxides and zirconium alkoxides were used as precursors to prepare corresponding oxide HPMs (Konishi et al., 2006; Konishi et al., 2008; Hasegawa et al., 2010). The sol–gel process of metal alkoxides is identical fundamentally to that of silicon alkoxides, including hydrolysis and condensation. The important difference, however, is that the metal alkoxides possess higher reactivity than silicon alkoxides due to their lower electronegativities of the central metal atoms. As a result, only a few metal oxide HPMs, such as TiO2 (Konishi et al., 2006; Hasegawa et al., 2010) and ZrO2 (Konishi et al., 2008) had been prepared from metal alkoxides under more strict and complicated conditions, much fewer reports are found on other metal oxide HPMs prepared from alkoxides.
Sol-gel researchers have been trying to use inorganic salts to replace alkoxides. Thanks to the hydrolytic instability, many metal species can undergo condensation to form M–O–M network in favorable conditions. The sol–gel process of metal salt solution can also be divided into two steps: forced hydrolysis and condensation (Figure 3). In general, most Mz+ (Z < 4) exists in the form of aquo complex, thus an increase of pH is required for converting them into hydroxo complex. However, it had been a challenge to modify the pH of the system without generating precipitates (dispersed products as a result of uncontrolled gel formation) under a high concentration of metal precursor. Tokudome et al. referred to the epoxide-mediated sol–gel method, which was developed by Itoh et al. (Itoh et al., 1993) and Gash et al. (Gash et al., 2001a; Gash et al., 2001b), to obtain Al2O3 HPM using AlCl3 · 6H2O as the precursors (Tokudome et al., 2007). In a traditional way to increase the pH, alkaline reagents, such as sodium hydroxide, ammonia, are employed. When the alkaline reagent is mechanically mixed with the metal salt solution, a sudden and steep increase of pH in the limited part of the solution causes uncontrolled precipitation. In the epoxide-mediated sol–gel method, a neutral epoxide, e.g. propylene oxide, is added, and then the pH is increased by the irreversible ring-opening reaction Eq. 2.
(2)
Compared to the traditional way to raise the pH, epoxide can raise the pH homogeneously and gradually. Thus, it became a facile method to prepare monolithic aerogels and HPMs. Following the success of Al2O3 HPM, Guo et al. synthesized ZrO2 HPM from ZrOCl2 · 8H2O (Guo et al., 2015). Li et al. synthesized TiO2 HPM from TiOSO4 using a similar strategy, in which the pH was increased slowly by the generation of ammonia from the hydrolysis of formamide (Li et al., 2013). Although other transition metal oxide aerogels, such as iron oxide, chromium oxide, etc., had been prepared via the epoxide-mediated sol–gel method (Gash et al., 2001b), it was not easy for the preparation of HPM at that time, even if they were trivalent species. It is because precursor concentration for preparation of HPM is higher than that for the preparation of aerogels/xerogel with lower density, since a comparable volume fraction of gel-rich phase and solvent-rich phase is required for obtaining the 3D interconnected structures. Moreover, Gash et al. found that it was hard to prepare the metal oxide aerogels from M2+ ions (M2+ = Co2+, Ni2+, Cu2+, Zn2+) even by the epoxide-mediated sol–gel method, in which only precipitation was obtained (Gash et al., 2001b). Some divalent metal oxide aerogels have been prepared by the epoxide-mediated sol–gel method afterwards (Gash et al., 2004; Gao et al., 2007; Sick and Hope-Weeks, 2008; Wei et al., 2009; Baghi et al., 2013), but the preparation of HPMs still remained a challenge.
The difficulty of forming monolithic gel can be estimated through the electronegativity of the metal cation in its salt. Table 1 shows the electronegativity of some metal ions which was calculated by Li et al. in which the valence state and coordination number were taken into consideration (Li and Xue, 2006). The difficulty comes from two facts; 1) forced hydrolysis of metal ions with low electronegativity generally requires higher pH; 2) the condensation reaction between hydroxo complexes is more radical due to the greater polarization of the M–O bond. The oxygen atom holds higher electron density when it forms bonds with metal atoms with lower electronegativity. As a result, the deprotonation becomes harder and higher pH is required in the step of forced hydrolysis. Aquo complexes of less electronegative cations are more prone to olation/oxolation when pH is increased. Their quick and local condensation tend to form small dispersed condensates (precipitates) limiting the formation of infinitely grown network.
TABLE 1. Electronegativity (χi) of cations with coordination number (CN) of 6 (or 4)a.
Recently, some of HPMs have been prepared from divalent metal salts (Table 2), though each method has its own shortcomings and limitations. Nonetheless, we have summarized the methods and trials for the preparation of these metal oxide HPMs here, and hope this review will inspire other researchers to find better ways to achieve metal oxide HPMs from divalent metal salts.
The initial effort on the preparation of transition metal oxide HPM via epoxide-mediated sol–gel method combined with phase separation was in Fe-system using FeCl3 6H2O as a metal precursor by Kido et al. (2012). Since the pH range where Fe(III) starts to form precipitates was much lower than the case of Al2O3 HPM from AlCl3 · 6H2O by Tokudome et al., an effective method of inhibiting uncontrolled precipitation/crystallization of iron (oxy)hydroxide was required. Namely, poly (acrylamide) (PAAm) was utilized to suppress the undesired precipitation via the strong interaction between amide groups in PAAm and Fe-based species, resulting in Fe-PAAm-based HPM. Other polymer termed poly (acrylic acid) (HPAA or PAA) is also popular for the preparation of HPMs. This strategy is so versatile that it has been extended to many other metal-polymer-based systems, including the low-valence metal species, such as Mn(II), Ni(II), Cu(II) (Kido et al., 2013; Kido et al., 2014; Fukumoto et al., 2015; Liu et al., 2020a; Liu et al., 2020b). On the other hand, both PAAm and HPAA not only play a key role in gelation, but also act as a phase separation inducer. In the Cu-PAAm-based system, for example, the macroporous morphology can be controlled by the amount of PAAm (Figure 4). The phase separation took place to form gel-rich phase [metal (oxy)hydroxide and PAAm] and solvent-rich phase, of which compatibility was lowered due to the strong interaction between metal (oxy)hydroxide and PAAm. The phase separation tendency as well as volume fraction of gel-rich phase was affected by the added amount of PAAm (Figure 4).
FIGURE 4. (A) Appearance of the Cu-PAAm-based as-dried gel. SEM images of samples prepared with varied amount of PAAm: (B) 0.5, (C) 0.6, (D) 0.7, (E) 0.8, and (F) 0.9 g. Ref. (Fukumoto et al., 2015).
This synthetic strategy is theoretically applicable to the preparation of HPM from any kind of metal precursor. The versatility has been proven by Liu et al. recently, in which metal-PAA-HPMs can be prepared from ZnCl2, CoCl2 6H2O, NiCl2 6H2O, MnCl2 4H2O, and FeCl2 4H2O, respectively, under the same conditions except for the kind of metal precursor (Figure 5) (Liu et al., 2020b). It is also shown that the feasibility in the preparation of the binary metal systems, such as Mn-Co, and Mn-Zn. It is reasonable to believe that more complex multi-metal HPMs can be achieved using HPAA. Compositional homogeneity of thus obtained HPMs have not been well examined yet.
FIGURE 5. (A) Appearance of the as-dried gels from left to right: Mn-PAA-based, Fe-PAA-based, Co-PAA-based, Ni-PAA-based, and Zn-PAA-based. SEM images of samples prepared with varied metal precursors: (B) MnCl2 4H2O, (C) FeCl2 4H2O, (D) CoCl2 6H2O, (E) NiCl2 6H2O, (F) ZnCl2. Ref. (Liu et al., 2020b).
Because of the strong interaction between the polymer and metal ions (and metal hydroxides), the polymers are stably remaining in the gel-rich phase. The compositional analysis in all the systems, such as Fourier transform infrared spectroscopy (FT-IR) and thermogravimeter-differential thermal analyzer (TG-DTA), has shown that the solid skeleton of the obtained HPMs contains a considerable mass fraction of the organic composition. Heat-treatment under an oxidative atmosphere is necessary if the crystallization of metal oxide and the removal of organic polymer are intended. However, the mechanical strength of the HPMs after heat-treatment in air is decreased significantly leading to the collapse of the monolith in many cases. Although SEM images shows that the macropores still remain in the heat-treated samples, their mechanical strength is limited as compared with ordinary porous ceramics. Thus, the term, organic supporters, should be used for PAAm and HPAA, and the HPMs prepared by this strategy should be expressed as metal-polymer-based HPMs (not metal oxide HPMs).
Lu et al. have tried to use small molecules with multiple carboxyl groups instead of polymer in order to produce the HPM. Citric acid, due to its high solubility, was chosen to prepare Zn-based HPMs in aqueous methanol (Lu et al., 2019a). No macroscopic gelation had been observed in the absence of citric acid. The Zn-citric acid-based HPM was obtained in the presence of citric acid, and the interconnected macropores were controlled by the volume fraction of propylene oxide in the starting solution. It was confirmed by FT-IR and TG-DTA that the citric acid played a role of structure supporter in gelation, which was very similar to PAAm and HPAA. Unfortunately, the monolithic gels became fragile after the removal of the most organic component by heat-treatment in air.
Although it is not easy to obtain metal oxide HPMs with negligible residual organic/carbon components, the carbon-containing supporter allows preparation of metallic or carbide HPMs when the samples are heat-treated under an inert atmosphere (Kido et al., 2012; Kido et al., 2013; Kido et al., 2014; Fukumoto et al., 2015; Liu et al., 2020a). Kido et al. reported that the metallic Ni/C HPM was obtained when the Ni-PAA-based gel was heat-treated under argon at above 400°C (Kido et al., 2013). Some micropores were generated after the heat-treatment, which may come from the carbonization of organic component. In Cu-PAAm-based system, the as-prepared HPM was reduced via a solvothermal treatment to obtain HPMs containing crystalline Cu2O or Cu (Fukumoto et al., 2015). The resultant HPMs possessed larger mesopore and boarder pore size distribution in contrast to the original one. The macroporous Cu/C monolith was obtained by heating the Cu-PAAm-based HPM under an argon flow above 400°C (Figure 6A), but only macropores were preserved while the micropores and mesopores could not be confirmed by nitrogen adsorption measurement. Moreover, the macroporous CuO monolith with negligible carbon could be obtained when the Cu/C HPM was further heat-treated in air at 400°C (Figure 6B).
FIGURE 6. SEM images of the Cu-PAAm samples: (A) calcined in argon at 800°C, (B) calcined in argon at 800°C and successively in air at 400°C. Insets show the appearance of each samples. Ref. (Fukumoto et al., 2015).
As shown above, organic supporters including polymers and small molecules, which have a strong interaction with metal species, can suppress the precipitation and promote the homogeneous gelation. However, the monolithic form cannot be preserved when the organic component is removed by heat-treatment in air. It is because the strong interaction between organic supporters and metal species suppresses the condensation reaction between metal–oxygen species themselves. An integrated network constructed by M–O–M bonding is hard to be formed in the entire gel product. It is, therefore, necessary to develop other synthetic strategies to prepare metal oxide HPMs.
How can we prepare homogeneous “oxide” HPM gels from divalent metal salts? It is well known that conditions including pH, solvent composition, coexisting anions and so on, are important factors influencing the hydrolysis and condensation reactions (Brinker and Scherer, 1990). The effects of solvent with varied polarity, dipole moment, viscosity, and protic or nonprotic behavior have been studied in the silicon alkoxide system (Artaki et al., 1986; Brinker and Scherer, 1990; Harris et al., 1990; Lee et al., 2015), but little is known on the effect of solvent in the inorganic metal salt system especially related to gel formation.
Very recently, Hara et al. prepared Ni-based HPM via the classical epoxide-mediated method using NiCl2 · 6H2O as precursors (Hara et al., 2021). A uniform transparent gel was obtained when only methanol was employed as a solvent. Opaque gels or precipitates were obtained when the water ratio becomes high or the solvent with lower polarity was employed. The phase-separated morphology was not obtained even if polyethylene oxide (PEO) was added (Figure 7A), which is an effective phase separation inducer in other systems (Nakanishi et al., 1994; Tokudome et al., 2007; Konishi et al., 2008; Hasegawa et al., 2010). When a part of methanol was replaced by N,N-dimethylformamide (DMF), the phase separation occurred in the presence of PEO (Figures 7B–D). The macropore size was increased by increasing the volume ratio of DMF to methanol (Figure 7E). Hara et al. considered that the coordination between DMF and nickel hydroxide led to the incompatibility against PEO, thus increased the phase separation tendency. Besides, the macropore size could also be controlled by the amount of PEO in the DMF and methanol mixture solution (Figure 7F). The as-dried gel prepared with varied starting composition showed a similar structure at nanometer scale, where the specific surface area ranged from 400 to 474 m2 g−1 and the mesopore size were around 8 nm. TG-DTA results showed that the PEO was not distributed to the macroporous skeleton, which can be removed by the solvent exchange. Considering that PEO was not required to maintain the monolithicity of the gels in the system, the gel network should (dominantly) be constructed by M–O–M bonding. As a result, the crystalline NiO HPM and metallic Ni HPM without losing the monolithic form and macroporous structure were prepared after heat-treatment in varied atmospheres (Figure 8). Only a small amount of micropores and mesopores remained in the heat-treated samples due to the growth of crystallites, pores in sub-micrometer size were obtained instead.
FIGURE 7. SEM images and appearance of the as-dried nickel hydroxide-based monoliths with varied ratios of DMF and methanol (MeOH): VDMF, VMeOH = (A) 0, 6.0, (B) 2.6, 3.4, (C) 3.0, 3.0, and (D) 3.2, 2.8 ml wPEO = 40 mg. Cumulative pore size distributions of as-dried nickel hydroxide-based monoliths (E) with varied ration of DMF and MeOH, wPEO = 40 mg, and (F) with varied amounts of PEO, VDMF, VMeOH = 3.0, 3.0 ml. Ref. (Hara et al., 2021).
FIGURE 8. SEM images and appearance of the metallic nickel monoliths prepared with varied ratios of DMF and MeOH: VDMF, VMeOH = (A) 2.6, 3.4 (B) 3.0, 3.0 and (C) 3.2, 2.8 ml, wPEO = 40 mg. (D) FE-SEM image of the metallic nickel monolith: wPEO = 40 mg, VDMF, VMeOH = 3.0, 3.0 ml. (E) Cumulative and (F) differential pore size distributions of the metallic nickel monoliths prepared with varied ratio of DMF and MeOH, wPEO = 40 mg. The as-dried nickel hydroxide monoliths were heat treated in air at 600°C for 4 h, and then in N2 + H2 (9:1, volume ratio) at 400°C for 6 h. Ref. (Hara et al., 2021).
Another case of the preparation of HPM from divalent metal salts is MgO HPM, reported by Lu et al. (2019b). An aqueous methanol solution with a high concentration of MgCl2 (cMg > 2 M) was prepared at first. Then the propylene oxide was added for prompting the gelation. The monolithic gels were obtained when the ratio of methanol to water is larger than 4 with a total volume of 0.75 ml. Only soft “gel” (precipitating gel-like material) or precipitates was obtained if the volume fraction of water is too high. Due to the low solubility of magnesium hydroxide, the phase separation occurred in the absence of any additive. Poly (vinylpyrrolidone) (PVP) was employed to control the domain sizes by suppressing the phase separation tendency via the interaction between PVP and magnesium (oxy)hydroxide oligomer. Due to crystallization of Mg(OH)2, such crystalline Mg (OH)2 with small crystalline size, which cannot be detected by powder X-ray diffraction (XRD) though, tend to form dense flake-like structures. Consequently, the pores, resulting from the interstices of flake-like structures, are almost macropores and small fractions of mesopores. In order to organize the mesopores, some carboxylic acids, e.g. 1,3,5-benzenetricarboxylic (BTC), were employed to suppress the growth of Mg(OH)2 crystallites. Although the pore volume of the mesopore with a size larger than 30 nm was increased with the increasing amount of BTC, the pore size distribution was broad (Figure 9A). In addition, the presence of BTC retarded the gelation thus gave an effect on the macroporous morphology also, in which the domain sizes became large, and the interconnected structure changed to spherical aggregates (Figures 9B–I). Since the monolithic gel can be formed without any organic supporter, the MgO HPM was obtained after heat-treatment in air at above 400°C (Figure 10).
FIGURE 9. (A) Pore size distribution with varied amount of BTC. SEM images of Mg(OH)2 HPMs prepared with varied amount of BTC, (B) 0, (C) 10, (D) 20, (E) 30, (F) 40, (G) 50, (H) 60, (I) 70 μmol. Ref. (Lu et al., 2019b).
FIGURE 10. (A) Appearance and (B) SEM images of as-dried Mg(OH)2 gel. (C) Appearance and (D) SEM images of MgO HPM. Ref. (Lu et al., 2019b).
With respect to other divalent metal systems, such as Mn, Cu, and Zn, the preparation of HPM by the epoxide-mediated method has not been achieved yet. In the epoxide-mediated method, solution pH can be raised gradually, but it cannot further influence the hydrolysis and condensation reactions. The solvents thus play a key role in the control of the hydrolysis and condensation reactions and offer more possibilities for achieving gelation. Recently, Fe2O3 HPM was prepared by the epoxide-mediated method using DMF as a major solvent, while it had been considered difficult to form gels without organic supporters [(Kido et al., 2012; Hara et al., 2018)]. When the metal salt was dissolved in DMF, the anions in the metal salt will not be solvated completely. The complexing anion will give a decisive effect on the sol–gel behavior of the metal species, which will be discussed in the next section. Therefore, the polar aprotic solvent, such as DMF, may show us a new possibility to achieve metal oxide monolithic gel by the epoxide-mediated method.
(Warning: Abrupt exothermic reaction may occur in the epoxide-mediated method. Cares should be taken to avoid the boiling of the solvent mixture upon addition of epoxide reagent, by limiting the amount of solution small and arranging the reaction solution efficiently cooled. For example, the total solution volume less than 5 ml in an ice bath with intensive magnetic stirring can avoid most possible accidents).
As mentioned in the introduction, high reactivity of metal alkoxide is due the large electronegativity difference between the metal and oxygen atoms. The reactivity can be modified by employing alternative (partially) coordinating ligands. In controlling the colloidal particles formation, Matijević found that the morphology was affected by the co-existing anions [(Matijević, 1976; Matijevic, 1981)]. Thereafter, Livage et al. developed the partial charge model to explain this phenomenon and to demonstrate how the charge distribution is affected by the complexing anions [(Livage et al., 1988; Sanchez et al., 1988)]. Based on the electronegativity equalization principle, the electronegativity of an atom varies linearly with its partial charge and the electron density rearranges until all electronegativities reach a mean value. Thereby, the electron density can be withdrawn from oxygen to metal, when a strong complexing anion is associated with the metal atom (Figure 11). Alquier et al. prepared Nb2O5 gels using Nb(OEt)3Cl2 as a precursor, which was obtained by the reaction between NbCl5 and EtOH (Alquier et al., 1986). They found that Nb(OEt)3Cl2 was more stable than Nb(OEt)5 against water, thus the gelation from Nb(OEt)3Cl2 became more facile. It indicates that it may be a feasible strategy for the preparation of monolithic gel from divalent metal salts.
FIGURE 11. Scheme of halogenated alkoxide route. (M: metal atom, X: halogen atom, blue area: electron density).
However, the reactivity of low-valence metal salts toward alcohol is quite low. In order to obtain halogenated metal alkoxide, Lu et al. suggested a new route, in which metal bromides (MnBr2, CoBr2, and CuBr2) were reacted with epichlorohydrin in DMF by a ring-opening reaction (Lu et al., 2020b). The reactions were qualitatively confirmed by FT-IR (Figure 12A). In the control solutions containing epichlorohydrin and CuBr2 with a mole ratio of 2:1, the absorption of characteristic peaks of the epoxy ring (962, 928, 853, and 760 cm−1) decreased over time, but did not disappear completely within 30 min (Wang and Polavarapu, 2000). On the other hand, the presence of the new absorption band ranged from 628 to 532 cm−1 is attributed to the formation of Cu–OR bonding (Singh et al., 1981). Similar results were obtained in the Co- and Mn-systems. In the practical process, the mole ratio of epichlorohydrin to metal bromide was higher than 2, ice-bath was employed to slow down (or stop) the ring-opening reaction so that not all the metal bromides are converted to metal alkoxides instead of brominated metal alkoxides. The gelation was achieved after the addition of hydrochloric acid. Three kinds of as-dried samples possessed relatively narrow pore size distributions, indicating that the nanoparticles were quite uniform, which proved the hydrolysis and condensation reactions were mild (Figure 12B). The interconnected macropores were obtained using PVP and PEO simultaneously to control the phase separation behavior (Figures 12C–E). The gelation is a result of the polycondensation of metal species without any additive supporters, thus the crystalline metal oxide HPMs can be obtained with suppressed cracks, except for the Cu-system (Figures 12F–H). In the Cu-system, however, the remaining Br will lead to the crystallization of CuBr during the heat-treatment at around 200°C. The serious deformation and exothermic from the crystallization of CuBr may become the reason for the collapse.
FIGURE 12. (A) FT-IR spectra of the solution before (black curve) and after mixing with CuBr2. (B) Pore size distribution of the as-dried gels of Cu-, Co-, and Mn-systems. SEM images and appearances (inset) of the as-dried gel, (C) Cu-based, (D) Co-based, (E) Mn-based. SEM images and appearances (inset) of (F) CuO, (G) Co3O4, (H) Mn5O8. Ref. (Lu et al., 2020b).
To prove that the halogenated alkoxide route is also effective in the preparation of binary complex metal oxide, CoMn2O4 HPM has been prepared (Lu et al., 2021). The spinel CoMn2O4 HPMs were obtained after heat-treatment at above 300°C in air (Figure 13A). The nitrogen adsorption isotherms and the corresponding pore size distribution indicated the presence of mesopores before and after heat-treatment at varied temperatures (Figures 13B,C). The interconnected macroporous structure and monolithic form were preserved after heat-treatment at even 600 °C (Figures 13D,E). Element mapping results detected by energy-dispersive X-Ray spectroscopy (EDX) showed that Mn and Co. were uniformly distributed in the samples (Figures 13F–I). In addition, the MnCo2O4 and Cu-Mn complex HPMs can be also prepared by this strategy (partially unpublished).
FIGURE 13. (A) XRD patterns of CoMn2O4 HPMs after heat treatment at varied temperature in air. (B) Nitrogen adsorption–desorption isotherms and (C) mesopore size distributions of CoMn2O4 HPMs before and after heat treatment at varied temperature. SEM images of CoMn2O4 HPMs (D) before and (E) after heat treatment at 600°C (inset shows the appearance of the heat-treated sample). The EDX element mapping images of the CoMn2O4 HPMs heat-treated at 600°C: (F) C, (G) O, (H) Mn, (I) Co. Ref. (Lu et al., 2021).
Although the halogenated alkoxide route seems to be applicable for any kind of metal salts theoretically, more evidences are required to prove the concept. We have tried to prepare MgO and ZnO HPMs by this strategy, but either of them failed. In Mg-system, the MgBr2 cannot be dissolved in the epichlorohydrin–DMF solution. In Zn-system, only yellow and transparent gel-like materials with extremely high viscosity were obtained. The kinds of solvent and the epoxide need further exploration in these two systems.
For the metallic element with an atomic number less than 33, about 1/3 of their oxides are prepared from divalent metal salts. The investigation on the hydrolysis and condensation of divalent metal ions, therefore, is meaningful for the preparation of metal oxides by sol–gel method. It not only involves the preparation of HPM and aerogel/xerogel, but also the preparation of particles and films.
To date, utilizing organic supporter can effectively suppress the precipitation and thus produce HPMs from all of the divalent metal salt. However, the metal oxide HPMs with negligible impurity are hard to be obtained by heat-treatment in air, which is common way for the removal of the organic components and crystallization of metal oxides. Some of metal oxide HPMs, such as NiO and MgO, can be prepared by the classical epoxide-mediated method in a selected solvent composition. The halogenated alkoxide route shows its potential in the preparation of metal oxide HPMs from divalent metal salts.
However, it is still a challenge to prepare some metal oxide (e.g. CuO, ZnO) HPMs without impurities and cracks. On the other hand, although the HPM has been obtained, the control of mesoporous structure is not easy (e.g. MgO). With respect to the successful cases (e.g. NiO, CoOx, MnOy), a lot more works are required for the scaling-up (first to device size) and practical application in the future. For these 4,5 decades, numerous experimental facts have been accumulated in sol-gel synthesis. The exploration of a novel composition with designated structure still relies largely on the trial and error. With better and deeper understanding of solution processing and structure controls, the dispersed knowledge should be organized into general tools/shortcuts with the aid of evolving machine-learning and artificial intelligence technology.
The original contributions presented in the study are included in the article/Supplementary Material, further inquiries can be directed to the corresponding author.
XL planned and executed the synthesis, characterization and data analysis of all the materials. KN proposed a grand plan to explore low-valence metal oxide monoliths and supervised all the aspects of the research. Both authors contributed to manuscript preparation equally.
This work was financially supported by Grant-in-Aid for Scientific Research 18H02056, MEXT, Japan.
The authors declare that the research was conducted in the absence of any commercial or financial relationships that could be construed as a potential conflict of interest.
All claims expressed in this article are solely those of the authors and do not necessarily represent those of their affiliated organizations, or those of the publisher, the editors and the reviewers. Any product that may be evaluated in this article, or claim that may be made by its manufacturer, is not guaranteed or endorsed by the publisher.
Alquier, C., Vandenborre, M. T., and Henry, M. (1986). Synthesis of Niobium Pentoxide Gels. J. Non-Crystalline Sol. 79, 383–395. doi:10.1016/0022-3093(86)90235-8
Artaki, I., Zerda, T. W., and Jonas, J. (1986). Solvent Effects on the Condensation Stage of the Sol-Gel Process. J. Non-Crystalline Sol. 81, 381–395. doi:10.1016/0022-3093(86)90504-1
Baghi, R., Peterson, G. R., and Hope-Weeks, L. J. (2013). Thermal Tuning of Advanced Cu Sol-Gels for Mixed Oxidation State Cu/CuxOy Materials. J. Mater. Chem. A. 1, 10898–10902. doi:10.1039/c3ta11957b
Brinker, C., and Scherer, G. (1990). Sol-Gel Science: The Physics and Chemistry of Sol-Gel Processing. San Diego, CA: Academic Press.
Dong, S., Zhu, W., Gao, X., Wang, Z., Wang, L., Wang, X., et al. (2016). Preparation of Tubular Hierarchically Porous Silicate Cement Compacts via a Tert-Butyl Alcohol (TBA)-based Freeze Casting Method. Chem. Eng. J. 295, 530–541. doi:10.1016/j.cej.2016.03.023
Fukumoto, S., Nakanishi, K., and Kanamori, K. (2015). Direct Preparation and Conversion of Copper Hydroxide-Based Monolithic Xerogels with Hierarchical Pores. New J. Chem. 39, 6771–6777. doi:10.1039/c5nj00479a
Gao, Y. P., Sisk, C. N., and Hope-Weeks, L. J. (2007). A Sol-Gel Route to Synthesize Monolithic Zinc Oxide Aerogels. Chem. Mater. 19, 6007–6011. doi:10.1021/cm0718419
Gash, A. E., Satcher, J. H., and Simpson, R. L. (2004). Monolithic Nickel(II)-based Aerogels Using an Organic Epoxide: the Importance of the Counterion. J. Non-Crystalline Sol. 350, 145–151. doi:10.1016/j.jnoncrysol.2004.06.030
Gash, A. E., Tillotson, T. M., Satcher, J. H., Poco, J. F., Hrubesh, L. W., and Simpson, R. L. (2001). Use of Epoxides in the Sol−Gel Synthesis of Porous Iron(III) Oxide Monoliths from Fe(III) Salts. Chem. Mater. 13, 999–1007. doi:10.1021/cm0007611
Gash, A. E., Tillotson, T. M., Satcher Jr, J. H., Hrubesh, L. W., and Simpson, R. L. (2001). New Sol-Gel Synthetic Route to Transition and Main-Group Metal Oxide Aerogels Using Inorganic Salt Precursors. J. Non-Crystalline Sol. 285, 22–28. doi:10.1016/s0022-3093(01)00427-6
Guo, X., Song, J., Lvlin, Y., Nakanishi, K., Kanamori, K., and Yang, H. (2015). Preparation of Macroporous Zirconia Monoliths from Ionic Precursors via an Epoxide-Mediated Sol-Gel Process Accompanied by Phase Separation. Sci. Tech. Adv. Mater. 16, 025003. doi:10.1088/1468-6996/16/2/025003
Hara, Y., Kanamori, K., Morisato, K., Miyamoto, R., and Nakanishi, K. (2018). Iron(III) Oxyhydroxide and Oxide Monoliths with Controlled Multiscale Porosity: Synthesis and Their Adsorption Performance. J. Mater. Chem. A. 6, 9041–9048. doi:10.1039/c8ta01691g
Hara, Y., Ono, M., Matsuda, S., Nakanishi, K., Kanamori, K., and Sakaushi, K. (2021). Tunable and Well-Defined Bimodal Porous Model Electrodes for Revealing Multiscale Structural Effects in the Nonaqueous Li-O2 Electrode Process. J. Phys. Chem. C 125, 1403–1413. doi:10.1021/acs.jpcc.0c10446
Harris, M. T., Brunson, R. R., and Byers, C. H. (1990). The Base-Catalyzed Hydrolysis and Condensation Reactions of Dilute and Concentrated TEOS Solutions. J. Non-Crystalline Sol. 121, 397–403. doi:10.1016/0022-3093(90)90165-i
Hasegawa, G., Kanamori, K., Nakanishi, K., and Hanada, T. (2010). Facile Preparation of Hierarchically Porous TiO2 Monoliths. J. Am. Ceram. Soc. 93, 3110–3115. doi:10.1111/j.1551-2916.2010.03831.x
Itoh, H., Tabata, T., Kokitsu, M., Okazaki, N., Imizu, Y., and Tada, A. (1993). Preparation of SiO2-Al2O3 Gels from Tetraethoxysilane and Aluminum Chloride. J. Ceram. Soc. Jpn. 101, 1081–1083. doi:10.2109/jcersj.101.1081
Kaji, H., Nakanishi, K., and Soga, N. (1955). Formation of Porous Gel Morphology by Phase Separation in Gelling Alkoxy-Derived Silica. Affinity between Silica Polymers and Solvent. J. Non-cryst. Sol. 181, 16–26.
Kaji, H., Nakanishi, K., and Soga, N. (1993). Polymerization-induced Phase Separation in Silica Sol-Gel Systems Containing Formamide. J. Sol-gel Sci. Technol. 1, 35–46. doi:10.1007/bf00486427
Kido, Y., Hasegawa, G., Kanamori, K., and Nakanishi, K. (2014). Porous Chromium-Based Ceramic Monoliths: Oxides (Cr2O3), Nitrides (CrN), and Carbides (Cr3C2). J. Mater. Chem. A. 2, 745–752. doi:10.1039/c3ta13725b
Kido, Y., Nakanishi, K., Miyasaka, A., and Kanamori, K. (2012). Synthesis of Monolithic Hierarchically Porous Iron-Based Xerogels from Iron(III) Salts via an Epoxide-Mediated Sol-Gel Process. Chem. Mater. 24, 2071–2077. doi:10.1021/cm300495j
Kido, Y., Nakanishi, K., Okumura, N., and Kanamori, K. (2013). Hierarchically Porous Nickel/carbon Composite Monoliths Prepared by Sol-Gel Method from an Ionic Precursor. Microporous Mesoporous Mater. 176, 64–70. doi:10.1016/j.micromeso.2013.03.042
Konishi, J., Fujita, K., Nakanishi, K., and Hirao, K. (2006). Monolithic TiO2 with Controlled Multiscale Porosity via a Template-free Sol−Gel Process Accompanied by Phase Separation. Chem. Mater. 18, 6069–6074. doi:10.1021/cm0617485
Konishi, J., Fujita, K., Oiwa, S., Nakanishi, K., and Hirao, K. (2008). Crystalline ZrO2 Monoliths with Well-Defined Macropores and Mesostructured Skeletons Prepared by Combining the Alkoxy-Derived Sol-Gel Process Accompanied by Phase Separation and the Solvothermal Process. Chem. Mater. 20, 2165–2173. doi:10.1021/cm703351d
Lee, D. H., Han, S. W., and Kang, D. P. (2015). Size Change of Silica Nanoparticles Induced by Non-alcoholic Solvent Addition during Sol-Gel Reaction. J. Sol-gel Sci. Technol. 74, 78–83. doi:10.1007/s10971-014-3579-y
Lee, J., and Chang, J. Y. (2018). Pickering Emulsion Stabilized by Microporous Organic Polymer Particles for the Fabrication of a Hierarchically Porous Monolith. Langmuir 34, 11843–11849. doi:10.1021/acs.langmuir.8b02576
Li, K., and Xue, D. (2006). Estimation of Electronegativity Values of Elements in Different Valence States. J. Phys. Chem. A. 110, 11332–11337. doi:10.1021/jp062886k
Li, W., Guo, X., Zhu, Y., Hui, Y., Kanamori, K., and Nakanishi, K. (2013). Sol-gel Synthesis of Macroporous TiO2 from Ionic Precursors via Phase Separation Route. J. Sol-gel Sci. Technol. 67, 639–645. doi:10.1007/s10971-013-3123-5
Liu, F., Feng, D., Yang, H., and Guo, X. (2020). Preparation of Macroporous Transition Metal Hydroxide Monoliths via a Sol-Gel Process Accompanied by Phase Separation. Sci. Rep. 10, 4331. doi:10.1038/s41598-020-61195-9
Liu, W., Feng, D., Yang, H., and Guo, X. (2020). Synthesis of Hierarchically Porous MnO/C Composites via a Sol-Gel Process Followed by Two-step Combustion for Lithium-Ion Batteries. New J. Chem. 44, 12307–12316. doi:10.1039/d0nj01538e
Livage, J., Henry, M., and Sanchez, C. (1988). Sol-gel Chemistry of Transition Metal Oxides. Prog. Solid State. Chem. 18, 259–341. doi:10.1016/0079-6786(88)90005-2
Lu, X., Hasegawa, G., Kanamori, K., and Nakanishi, K. (2020). Hierarchically Porous Monoliths Prepared via Sol-Gel Process Accompanied by Spinodal Decomposition. J. Sol-gel Sci. Technol. 95, 530–550. doi:10.1007/s10971-020-05370-4
Lu, X., Kanamori, K., Hasegawa, G., and Nakanishi, K. (2021). Preparation of Hierarchically Porous Spinel CoMn 2 O 4 Monoliths via Sol-Gel Process Accompanied by Phase Separation. J. Am. Ceram. Soc. 104, 2449–2459. doi:10.1111/jace.17662
Lu, X., Kanamori, K., and Nakanishi, K. (2020). Hierarchically Porous Monoliths Based on Low-Valence Transition Metal (Cu, Co, Mn) Oxides: Gelation and Phase Separation. Natl. Sci. Rev. 7, 1656–1666. doi:10.1093/nsr/nwaa103
Lu, X., Kanamori, K., and Nakanishi, K. (2019). Preparation of Zinc Oxide with a Three-Dimensionally Interconnected Macroporous Structure via a Sol-Gel Method Accompanied by Phase Separation. New J. Chem. 43, 11720–11726. doi:10.1039/c9nj02373a
Lu, X., Kanamori, K., and Nakanishi, K. (2019). Synthesis of Hierarchically Porous MgO Monoliths with Continuous Structure via Sol-Gel Process Accompanied by Phase Separation. J. Sol-gel Sci. Technol. 89, 29–36. doi:10.1007/s10971-018-4682-2
Matijevic, E. (1981). Monodispersed Metal (Hydrous) Oxides - a Fascinating Field of Colloid Science. Acc. Chem. Res. 14, 22–29. doi:10.1021/ar00061a004
Matijević, E. (1976). The Role of Chemical Complexing in the Formation and Stability of Colloidal Dispersions. J. Colloid Interf. Sci 58, 374–389.
Nakanishi, K. (1997). Pore Structure Control of Silica Gels Based on Phase Separation. J. Porous Mater. 4, 67–112. doi:10.1023/a:1009627216939
Nakanishi, K., Komura, H., Takahashi, R., and Soga, N. (1994). Phase Separation in Silica Sol-Gel System Containing Poly(ethylene Oxide). I. Phase Relation and Gel Morphology. Bcsj 67, 1327–1335. doi:10.1246/bcsj.67.1327
Nakanishi, K., Nagakane, T., and Soga, N. (1998). Designing Double Pore Structure in Alkoxy-Derived Silica Incorporated with Nonionic Surfactant. J. Porous Mater. 5, 103–110. doi:10.1023/a:1009633102016
Nakanishi, K., and Soga, N. (1991). Phase Separation in Gelling Silica-Organic Polymer Solution: Systems Containing Poly(sodium Styrenesulfonate). J. Am. Ceram. Soc. 74, 2518–2530. doi:10.1111/j.1151-2916.1991.tb06794.x
Nakanishi, K., and Soga, N. (1992). Phase Separation in Silica Sol-Gel System Containing Polyacrylic Acid I. Gel Formaation Behavior and Effect of Solvent Composition. J. Non-Crystalline Sol. 139, 1–13. doi:10.1016/s0022-3093(05)80800-2
Nakanishi, K., and Soga, N. (1992). Phase Separation in Silica Sol-Gel System Containing Polyacrylic Acid II. Effects of Molecular Weight and Temperature. J. Non-Crystalline Sol. 139, 14–24. doi:10.1016/s0022-3093(05)80801-4
Nakanishi, K., and Soga, N. (1992). Phase Separation in Silica Sol-Gel System Containing Polyacrylic Acid. III. Effect of Catalytic Condition. J. Non-Crystalline Sol. 142, 36–44. doi:10.1016/s0022-3093(05)80004-3
Nakanishi, K., and Soga, N. (1992). Phase Separation in Silica Sol-Gel System Containing Polyacrylic Acid. IV. Effect of Chemical Additives. J. Non-Crystalline Sol. 142, 45–54. doi:10.1016/s0022-3093(05)80005-5
Nakanishi, K., and Soga, N. (1997). Phase Separation in Silica Sol-Gel System Containing Poly(ethylene Oxide) II. Effects of Molecular Weight and Temperature. Bcsj 70, 587–592. doi:10.1246/bcsj.70.587
Sanchez, C., Livage, J., Henry, M., and Babonneau, F. (1988). Chemical Modification of Alkoxide Precursors. J. Non-Crystalline Sol. 100, 65–76. doi:10.1016/0022-3093(88)90007-5
Shen, K., Zhang, L., Chen, X., Liu, L., Zhang, D., Han, Y., et al. (2018). Ordered Macro-Microporous Metal-Organic Framework Single Crystals. Science 359, 206–210. doi:10.1126/science.aao3403
Sick, C. N., and Hope-Weeks, L. J. (2008). A Sol–Gel Route to Synthesize Monolithic Zinc Oxide Aerogels. J. Mater. Chem. 22, 2607–2610. doi:10.1021/cm0718419
Singh, J. V., Baranwal, B. P., and Mehrotra, R. C. (1981). Synthesis and Characterization of Some Alkoxide Derivatives of Copper(II). Z. Anorg. Allg. Chem. 477, 235–240. doi:10.1002/zaac.19814770633
Takahashi, R., Nakanishi, K., and Soga, N. (1995). Effects of Aging and Solvent Exchange on Pore Structure of Silica Gels with Interconnected Macropores. J. Non-Crystalline Sol. 189, 66–76. doi:10.1016/0022-3093(95)00203-0
Tokudome, Y., Fujita, K., Nakanishi, K., Miura, K., and Hirao, K. (2007). Synthesis of Monolithic Al2O3 with Well-Defined Macropores and Mesostructured Skeletons via the Sol−Gel Process Accompanied by Phase Separation. Chem. Mater. 19, 3393–3398. doi:10.1021/cm063051p
Wang, F., and Polavarapu, P. L. (2000). Conformational Stability of (+)-Epichlorohydrin. J. Phys. Chem. A. 104, 6189–6196. doi:10.1021/jp000757c
Wei, T.-Y., Chen, C.-H., Chang, K.-H., Lu, S.-Y., and Hu, C.-C. (2009). Cobalt Oxide Aerogels of Ideal Supercapacitive Properties Prepared with an Epoxide Synthetic Route. Chem. Mater. 21, 3228–3233. doi:10.1021/cm9007365
Keywords: sol-gel, phase separation, hierarchically porous monolith, low-valence metal oxide, divalent metal salt
Citation: Lu X and Nakanishi K (2021) Synthesis of Hierarchically Porous Metal Oxide Monoliths via Sol–Gel Process Accompanied by Phase Separation From Divalent Metal Salts: A Short Review. Front. Chem. Eng. 3:787788. doi: 10.3389/fceng.2021.787788
Received: 01 October 2021; Accepted: 29 November 2021;
Published: 16 December 2021.
Edited by:
Serena Esposito, Politecnico di Torino, ItalyReviewed by:
Apurba Sinhamahapatra, Indian Institute of Technology Dhanbad, IndiaCopyright © 2021 Lu and Nakanishi. This is an open-access article distributed under the terms of the Creative Commons Attribution License (CC BY). The use, distribution or reproduction in other forums is permitted, provided the original author(s) and the copyright owner(s) are credited and that the original publication in this journal is cited, in accordance with accepted academic practice. No use, distribution or reproduction is permitted which does not comply with these terms.
*Correspondence: Kazuki Nakanishi, ZGtuYWthbmlzaGlAaW1hc3MubmFnb3lhLXUuYWMuanA=
Disclaimer: All claims expressed in this article are solely those of the authors and do not necessarily represent those of their affiliated organizations, or those of the publisher, the editors and the reviewers. Any product that may be evaluated in this article or claim that may be made by its manufacturer is not guaranteed or endorsed by the publisher.
Research integrity at Frontiers
Learn more about the work of our research integrity team to safeguard the quality of each article we publish.