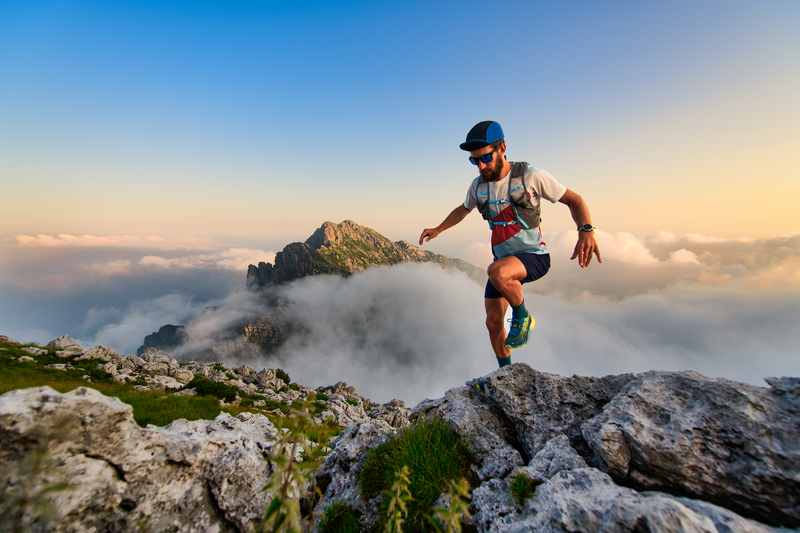
95% of researchers rate our articles as excellent or good
Learn more about the work of our research integrity team to safeguard the quality of each article we publish.
Find out more
BRIEF RESEARCH REPORT article
Front. Chem. Eng. , 23 April 2021
Sec. Environmental Chemical Engineering
Volume 3 - 2021 | https://doi.org/10.3389/fceng.2021.652445
Hydrothermal carbonization (HTC) is a promising technology for chemical and material synthesis. However, HTC produces not only valuable solid coal-materials but also yields process water (PW) with high chemical oxygen demand (COD) that requires extensive treatment. Anaerobic digestion (AD) has been used for initial treatment of HTC-PW, but the AD effluent is still high in COD and particles. Here, we show that microbial electrochemical technologies (MET) can be applied for COD removal from AD effluent of HTC-PW. Bioelectrochemical systems (BES) treating different shares of AD effluent from HTC-PW exhibited similar trends for current production. Thereby, maximum current densities of 0.24 mA cm−2 and COD removal of 65.4 ± 4.4% were reached (n = 3). Microbial community analysis showed that the genus Geobacter dominated anode biofilm and liquid phase of all reactors indicating its central role for COD oxidation and current generation.
Hydrothermal carbonization (HTC) is a thermochemical process that converts organic solid feedstock like biological wastes or biomass into carbonaceous products being coal-like materials (Funke and Ziegler, 2010; Hoekman et al., 2011). For HTC, the feedstock is heated in an oxygen lacking atmosphere at 180–350°C creating an autogenous pressure (Lu et al., 2012; Nizamuddin et al., 2017). During HTC a diverse set of chemical reactions takes place including hydrolysis, dehydration, decarboxylation, condensation, polymerization, and aromatization yielding a huge variety of gaseous, liquid, and solid products. Thereby, the latter is usually in focus. For instance, it has been demonstrated that a high diversity of feedstock can be converted by HTC into promising solid fuels (Xu and Jiang, 2017) or materials for energy technologies (Titirici and Antonietti, 2010). Therefore, HTC is considered as an environmentally friendly and energy-efficient sustainable technology for valorization of biomass and solid waste (Zhao et al., 2014).
In addition to solid products, process water (PW) is generated by HTC. Especially due to the different types of applicable feedstock, HTC-PW represents a waste stream containing a broad diversity of chemical compounds. These include insoluble compounds like carbon particles, moderately water soluble compounds (e.g., phenols), and water soluble compounds (e.g., furfurals, acids, and aldehydes) (Kruse and Gawlik, 2003). The organic compounds in the HTC-PW may contain more than 15% of the initial chemical energy of the feedstock with a comparable high chemical oxygen demand (COD) of up to 30 g L−1 (Kambo et al., 2017). Due to its high organic load and the presence of toxic compounds, like phenol or p-cresol, HTC-PW needs to be treated before being released into the environment (Weiner et al., 2014). As the treatment is very energy-demanding and cost-intensive, different approaches for turning HTC-PW into a resource are followed (Merzari et al., 2019). The most advanced approach is anaerobic digestion (AD) partially harvesting the chemical energy of HTC-PW as biogas (mainly CH4) (Kim et al., 2015; Passos and Ferrer, 2015). Currently, AD is used for treating various HTC-PW because of its low operational expenditures and capacity for treating high quantities of HTC-PW of different quality (Wirth and Mumme, 2014; Marin-Batista et al., 2020). However, AD removes only around 44–61% of the initial COD from HTC-PW (De La Rubia et al., 2018), and thus the AD effluent usually still exhibits a high COD of more than 10 g L−1 (Wirth et al., 2015). Moreover, AD effluents from HTC-PW also contain higher concentrations of ammonia, nitrogen, and phosphorus underlining the requirement of further treatment (Zhu et al., 2017; Marin-Batista et al., 2020). Several methods allowing COD removal from AD effluents have been reported, such as microalgae remediation (Cheng et al., 2015; Chuka-Ogwude et al., 2020), phytoremediation (Hu et al., 2019; Pincam et al., 2020), photosynthetic bacterial remediation (Wen et al., 2016), and use of abiotic electrochemical technologies (Goncalves et al., 2012).
Microbial electrochemical technologies (MET) are promising for treating different types of waste and recovering energy therefrom (Logan and Rabaey, 2012). The reactors of MET are termed bioelectrochemical systems (BES) and are based on the use of electroactive microorganisms (EAM) (Lovley, 2012). EAM are capable of extracellular electron transfer (EET) that allows EAM to couple their metabolism with electric current flow at electrodes. Apart from model substrates, different types of wastewater were treated with MET (Sun et al., 2016). Thereby, increasingly complex wastewaters that are comparable to AD effluent and that can only be treated with high efforts by conventional technologies attract increasing attention. For instance, Zhang and colleagues studied electricity generation from wheat straw hydrolysate with an initial COD of 1 g L−1 reaching a power density of 123 mW m−2 and associated coulombic efficiencies (CE) ranging from 15.5 to 37.1% in microbial fuel cells (MFC) (Zhang et al., 2009). Further, Wang and colleagues reported the use of rice straw hydrolysate in MFC reaching a maximum power density of 137.6 ± 15.5 mW m−2 at an initial COD concentration of 0.4 g L−1 (Wang et al., 2020). The treatment of AD effluent from HTC-PW in BES has yet not been demonstrated to our knowledge. Consequently, here we assessed its treatment with dual-chamber BES in potentiostatically controlled experiments and analyzed COD degradation (ΔCOD), electrochemical performance parameters [CE, maximum current density (jmax)], and the associated microbial communities.
All chemicals were of analytical or biochemical grade. If not stated otherwise, all provided potentials refer to the standard hydrogen electrode (SHE) by conversion from Ag/AgCl sat. KCl reference electrodes (+0.197 V vs. SHE).
AD effluent from treating HTC-PW that is denominated as raw waste water (WW) was used as sole feed for the BES experiments representing sole carbon and electron source as well as inoculum. In the HTC process, acidified sludge of a wastewater treatment plant with >100,000 population equivalents was carbonized. The produced PW was used as feed for a mesophilic AD. The AD effluent was stored in the dark at 4°C for 4 days for the first batch experiments (WW, SN1) and for 40 days for the second batch experiments (SN2) (Supplementary Figure 2)—see Table 1 for abbreviations of experiments. The AD effluent possessed a dissolved COD of 14.7 g L−1 and a total COD of 22.6 ± 4.7 g L−1 (n = 9) with a pH of 7.7 ± 0.1 and an ammonia concentration (N-) of 3.6 g L−1. The AD effluent was divided into two fractions: untreated AD effluent (that is WW) and AD effluent supernatant (obtained by physical precipitation for 48 h at 4°C, that is SN1 and SN2).
Table 1. List of performed potentiostatically controlled and open circuit potential (OCP) experiments with determined COD removal (ΔCOD), charge, and coulombic efficiency.
All experiments were carried out in dual-chamber BES composed of a 250 mL laboratory glass bottle (SCHOTT DURAN®, Labware SCHOTT AG, Germany) closed with a butyl rubber stopper with pierced holes for cathode chamber and reference electrode (Ag/AgCl sat. KCl, +0.197 V vs. standard hydrogen electrode, SE11, Xylem Analytics Germany Sales GmbH & Co. KG Sensortechnik Meinsberg, Germany) Both anode and cathode were graphite rods (length: 4 cm, diameter: 0.5 cm, surface area: 6.48 cm2, quality CP-2200, CP-Graphitprodukte GmbH, Germany) electrically connected via stainless steel wire (Goodfellow GmbH, Germany) covered by a polytetrafluoroethylene tube (Shrink-Kon PLG-HSB, elektroTECHNIK GmbH, Germany) and fixed with epoxy resin (HT2, R&G Faserverbundwerkstoffe, Germany). The anode chamber was filled with 250 mL mineral salt medium (MSM) as previously described by Dorer and colleagues leaving a headspace of about 30 mL (Dorer et al., 2016). The cathode chamber was a 15 mL round glass tube (1 cm diameter) ionically connected to the anode chamber via a cation exchange membrane (CEM, fumasep®FKE, FuMA-Tech GmbH, Germany) fixed by using an O-ring and aluminum cap and filled with 10 mL of MSM. Identical reactors that were not connected to the potentiostat served as biotic open circuit potential (OCP) controls (WW_OCP and SN_OCP). A potentiostatic controlled BES, but without addition of AD effluent served as a further control experiment (abiotic control). All BES were operated at 30°C and stirred at 400 rpm using a magnetic stirrer. Anodes were poised at +0.397 V and current was recorded every 600 s using a multipotentiostat (MPG-2, Bio-Logic Science Instruments, France). Cyclic voltammetry was performed with a scan rate of 1 mV s−1 from −0.303 to 0.497 V for three consecutive scans (the 3rd scan was used for analysis).
COD measurements were performed using cuvette tests (NANOCOLOR tube tests, COD 60–150 and COD 100–1,500, MACHEREY-NAGEL GmbH & Co. KG) according to the manufacturer's instruction. GC-MS analyses were performed with original AD effluent samples that is WW, which served as feed and inoculum. Details about GC-MS analysis are given in the Supplementary Section 1.2. Details of microbial analysis are given in the Supplementary Section 1.3.
The anode chambers of bioelectrochemical systems (BES) were fed with AD effluent from treatment of HTC-PW representing inoculum and substrate. Experiments were conducted by feeding 1 mL of WW per BES with 250 mL liquid volume leading to an initial COD of 247.4 ± 18.9 mg L−1. BES started to produce substantial current after a lag phase of about 4 days and reached jmax of 0.11 ± 0.01 mA cm−2 after around 1 week (Figure 1A). Subsequently, current production decreased, although COD removal (ΔCOD) amounted to only 11.7 ± 10.6% at the end of the experiment (Table 1). This suggests a fast removal of biodegradable compounds present in the AD effluent (e.g., acetic acid, propanoic acid, and n-butanoic acid; see Supplementary Table 1) by EAM and the persistence of a large fraction of material that is not bioavailable (e.g., enclosed in the solid particles) or not biodegradable. With only 4.2 ± 1.8%, ΔCOD of a non-polarized control reactor (WW_OCP) was apparently lower (Table 1). Noteworthy, solid particles that were presumably hardly biodegradable represent a main share of total COD in the WW. To study the contribution of solid particles to COD and its role for COD removal, particles were removed from the feed of BES by physical precipitation (Supplementary Figure 2). The reactors fed by supernatant of the raw waste water without solid particles (SN1) possessed a COD of 157.1 ± 16.9 mg L−1. The BES amended with SN1 (Figure 1B) performed similarly as the BES fed with WW. Current production increased after 4 days and decreased after 10 days resulting in a ΔCOD of 25.0 ± 11.4% (Table 1).The maximum current density was slightly lower (jmax = 0.08 ± 0.01 mA cm−2) compared to BES fed with WW and the total charge was produced in two consecutive peaks. Possible reasons are the commencing formation of food webs (e.g., including fermentation) (Koch et al., 2019) after first consumption of easy biodegradable substrates, the presence of different EAM metabolizing different substrates (Zhang et al., 2011) or even diauxie of EAM. This is in line with observations made by Zang and colleagues, who reported that two current peaks were present in a MFC using canna biomass that is a substrate with a similar complexity like AD effluent (Zang et al., 2010). Furthermore, Thygesen and colleagues demonstrated that lignocellulosic hydrolysate can be used for co-treatment with domestic wastewater for power generation by MFC and also reported more than one peak in the voltage output (Thygesen et al., 2011). Using WW and SN1 as feed yielded a CE of 145.4 ± 117.6% and 75.7 ± 56.3%, respectively (Table 1). The high standard deviations can thereby be attributed to the COD measurement being influenced by the presence of particles for WW and lower weight particles for SN1, as the latter was measured only 48 h after physical separation.
Figure 1. Chronoamperometric current densities of bioelectrochemical systems fed with different fractions of anaerobic digestion effluent treating hydrothermal carbonization process water polarized at +0.397 V vs. SHE (30°C and 150 rpm). With (A) untreated AD effluent (WW), (B) supernatant obtained from WW by physical precipitation for 48 h at 4°C (SN1), (C) supernatant obtained by physical precipitation from WW for 48 h at 4°C and after 40 days of storing (SN2). Green solid line: abiotic control. Black, blue, and red solid line: experimental replicates.
Interestingly, the second set of batch experiments using the same supernatant of raw waste water, but after storing it for 40 days (SN2) behaved differently. Here, current production started to increase around 4 days after incubation (similar to SN1) but declined after 8 days (Figure 1C). For feeding SN2, only one current peak with jmax of 0.18 ± 0.04 mA cm−2 was detected and 65.4 ± 4.4% COD was removed (Table 1). This amount is significantly higher than the ΔCOD of SN1 (see Table 1). We reason that the storage probably leads to increased availability of COD for EAM and hence higher current production, which is in accordance with Pant and colleagues (Pant et al., 2013). The achieved current densities of BES oxidizing SN2 are even comparable for glucose as substrate [e.g., of 0.12 mA cm−2, (Zhao et al., 2020)]. Further, the achieved CE of 35.5 ± 1.5% with SN2 is very decent, when being compared with the microbial electrochemical oxidation of wheat straw hydrolysate. For this substrate that possesses a similar complexity like AD effluent, CE ranging from 15.5 to 37.1% were reported (Zhang et al., 2009). Among all experiments, feeding BES with SN2 resulted in the highest average COD degradation rate with 11.6 ± 0.8 mg L−1 d−1 (see Supplementary Section 1.1). This average COD degradation rate was far lower than for BES treating equally complex feeds like straw hydrolysate (Wang et al., 2014), corn stover biomass (Nam et al., 2010), or real field chemical wastewater (Velvizhi et al., 2014) with maximum COD degradation rates of 144, 3,500, and 3,570 mg L−1 d−1, respectively. This might be due to the complex organic composition of the waste water, the low initial COD concentrations compared to these studies, but especially the fact that in this proof-of-concept study batch operation was used. Further, the here used reactors also limited the treatment rate as they were developed for fundamental studies, but not designed for high volume related performances as the anode surface area-to-volume ratio of 25.9 cm2 L−1 is comparable low when being compared with more application-oriented studies treating complex feeds, in which surface area-to-volume ratios such as 140 cm2 L−1 (Zhang et al., 2009) and 333.3 cm2 L−1 (Cerrillo et al., 2016) were applied.
The anode biofilms were characterized using cyclic voltammetry (CV, Supplementary Figure 1). Different redox systems were depicted for anodic biofilm, with formal potentials of −0.15 and −0.05 V. In line with microbial community analysis (vide infra) both redox systems could be clearly assigned to Geobacter spp. (Patil et al., 2012; Yang et al., 2017). Further smaller peaks were detected in the different experiments indicating the contribution of other EAM than Geobacteraceae to the electroactive food web. Deciphering these, however, is far beyond the scope of this Brief Research Report.
According to the TRFLP (terminal restriction fragment length polymorphism) analysis, both biofilm and planktonic cells of all BES reactors fed with raw waste water (WW) and supernatant (SN1 and SN2) were dominated by the genus Geobacter [TRF 213 base pairs (bp)] (Figure 2) (Korth et al., 2020). Noteworthy, no DNA could be extracted neither from biofilm nor liquid phase of the OCP control indicating no substantial biomass growth associated to COD removal by the use of alternative electron acceptors (e.g., nitrate and sulfate). The high abundance of Geobacter in liquid phase (40.3 ± 4.1% and 78.0 ± 4.0% for WW and SN1 combined with SN2, respectively), might be due to the presence of iron and humic substances in the WW, which could serve as electron shuttles or solid terminal electron acceptors (Roden et al., 2010). The high abundance of Geobacter in biofilm (64.7 ± 20.8% and 76.7 ± 20.7% for WW and SN1 combined with SN2, respectively) of all experiments is probably due to the occurrence of acetate in the AD effluent (Supplementary Table 1). It represents the favored substrate of Geobacter and leads to a high enrichment thereof especially in biofilm anodes as it was already shown numerous times (Chaudhuri and Lovley, 2003; Harnisch et al., 2011; Miceli et al., 2012; Logan et al., 2019). Yet, it is in contrast to one of our recent studies showing that also high performance anodes can be formed by microbiomes not including Geobacteraceae (Koch et al., 2019). The here obtained results support the phenomenon that Geobacter is widely observed and playing a central role in bioelectrochemical reactors as the main EET performer (Zhao et al., 2020).
Figure 2. Microbial community analysis of anode biofilms and liquid phase of bioelectrochemical systems sampled at the end of batch cultivation and of original waste water used as inoculum. 16S rRNA genes were analyzed via TRFLP using the restriction enzyme HaeIII. WW and SN indicate experiments using raw waste water and supernatant, respectively, as inoculum and feed. WW: n = 3, SN that is pooled samples of SN 1 and SN2: n = 5, Inoculum: n = 1, error bars represent standard deviations.
In this study, the proof-of-concept of using BES for the subsequent treatment of effluent from anaerobically digested HTC-PW resulting in further removal of COD and current production was demonstrated. The treatment technology was principally applicable for the three tested HTC-PW fractions (raw waste water, fresh supernatant, and stored supernatant). Thereby, reactors fed with stored and thus aged supernatant (SN2) reached the highest COD removal efficiency of 65.4 ± 4.4% and maximum current density of 0.24 mA cm−2. Starting from a diverse inoculum, electrochemically driven selection took place, with the genus Geobacter being highly enriched in biofilm and liquid phase probably due to the high presence of acetic acid in the raw waste water.
Based on the here achieved proof-of-concept, follow up studies addressing microbial structure-function relationships and process engineering [e.g., reactor engineering, different operational modes, pre-treatment of feed by, e.g., filtering as well as developing adaptation measures for biofilm electrodes (Chen et al., 2019)] are required to further assess the technological potential of BES for treatment of AD effluent from HTC-PW.
The original contributions presented in the study are included in the article/Supplementary Material, further inquiries can be directed to the corresponding author.
SD: methodology, investigation, data analysis, and writing—original draft. BK: conceptualization, supervision, methodology, data analysis, and writing—review and editing. CV: data analysis, supervision, and writing—review and editing. FH: conceptualization, methodology, supervision, project administration, data analysis, and writing—review and editing. All authors: contributed to the article and approved the submitted version.
This work was supported by the Helmholtz-Association within the Research Programme Renewable Energies.
The authors declare that the research was conducted in the absence of any commercial or financial relationships that could be construed as a potential conflict of interest.
We thank Marcel Pohl from DFBZ for providing AD effluent and respective analytical parameters. SD thanks China Scholarship Council (CSC201804910500) for 4-year granting study abroad.
The Supplementary Material for this article can be found online at: https://www.frontiersin.org/articles/10.3389/fceng.2021.652445/full#supplementary-material
Cerrillo, M., Oliveras, J., Vinas, M., and Bonmati, A. (2016). Comparative assessment of raw and digested pig slurry treatment in bioelectrochemical systems. Bioelectrochemistry 110, 69–78. doi: 10.1016/j.bioelechem.2016.03.004
Chaudhuri, S. K., and Lovley, D. R. (2003). Electricity generation by direct oxidation of glucose in mediatorless microbial fuel cells. Nat. Biotechnol. 21, 1229–1232. doi: 10.1038/nbt867
Chen, S., Patil, S. A., Brown, R. K., and Schröder, U. (2019). Strategies for optimizing the power output of microbial fuel cells: transitioning from fundamental studies to practical implementation. Appl. Energy 233–234, 15–28. doi: 10.1016/j.apenergy.2018.10.015
Cheng, J., Xu, J., Huang, Y., Li, Y., Zhou, J., and Cen, K. (2015). Growth optimisation of microalga mutant at high CO(2) concentration to purify undiluted anaerobic digestion effluent of swine manure. Bioresour. Technol. 177, 240–246. doi: 10.1016/j.biortech.2014.11.099
Chuka-Ogwude, D., Ogbonna, J., and Moheimani, N. R. (2020). A review on microalgal culture to treat anaerobic digestate food waste effluent. Algal. Res. 47, 101841–101857. doi: 10.1016/j.algal.2020.101841
De La Rubia, M. A., Villamil, J. A., Rodriguez, J. J., and Mohedano, A. F. (2018). Effect of inoculum source and initial concentration on the anaerobic digestion of the liquid fraction from hydrothermal carbonisation of sewage sludge. Renew. Energy 127, 697–704. doi: 10.1016/j.renene.2018.05.002
Dorer, C., Vogt, C., Neu, T. R., Stryhanyuk, H., and Richnow, H. H. (2016). Characterization of toluene and ethylbenzene biodegradation under nitrate-, iron(III)- and manganese(IV)-reducing conditions by compound-specific isotope analysis. Environ. Pollut. 211, 271–281. doi: 10.1016/j.envpol.2015.12.029
Funke, A., and Ziegler, F. (2010). Hydrothermal carbonization of biomass: a summary and discussion of chemical mechanisms for process engineering. Biofuel Bioprod. Bior. 4, 160–177. doi: 10.1002/bbb.198
Goncalves, M. R., Marques, I. P., and Correia, J. P. (2012). Electrochemical mineralization of anaerobically digested olive mill wastewater. Water Res. 46, 4217–4225. doi: 10.1016/j.watres.2012.05.019
Harnisch, F., Koch, C., Patil, S. A., Hübschmann, T., Müller, S., and Schröder, U. (2011). Revealing the electrochemically driven selection in natural community derived microbial biofilms using flow-cytometry. Energy Environ. Sci. 4, 1265–1267. doi: 10.1039/c0ee00605j
Hoekman, S. K., Broch, A., and Robbins, C. (2011). Hydrothermal carbonization (HTC) of lignocellulosic biomass. Energ. Fuel 25, 1802–1810. doi: 10.1021/ef101745n
Hu, H., Zhou, Q., Li, X., Lou, W., Du, C., Teng, Q., et al. (2019). Phytoremediation of anaerobically digested swine wastewater contaminated by oxytetracycline via Lemna aequinoctialis: nutrient removal, growth characteristics and degradation pathways. Bioresour. Technol. 291, 121853–121860. doi: 10.1016/j.biortech.2019.121853
Kambo, H. S., Minaret, J., and Dutta, A. (2017). Process water from the hydrothermal carbonization of biomass: a waste or a valuable product? Waste Biomass Valori 9, 1181–1189. doi: 10.1007/s12649-017-9914-0
Kim, D., Lee, K., and Park, K. Y. (2015). Enhancement of biogas production from anaerobic digestion of waste activated sludge by hydrothermal pre-treatment. Int. Biodeterior Biodegr. 101, 42–46. doi: 10.1016/j.ibiod.2015.03.025
Koch, C., Huber, K. J., Bunk, B., Overmann, J., and Harnisch, F. (2019). Trophic networks improve the performance of microbial anodes treating wastewater. NPJ Biofilms Microbiomes 5, 27–35. doi: 10.1038/s41522-019-0100-y
Korth, B., Kretzschmar, J., Bartz, M., Kuchenbuch, A., and Harnisch, F. (2020). Determining incremental coulombic efficiency and physiological parameters of early stage Geobacter spp. enrichment biofilms. PLoS ONE 15:e0234077. doi: 10.1371/journal.pone.0234077
Kruse, A., and Gawlik, A. (2003). Biomass conversion in water at 330–410 °C and 30–50 MPa. Identification of key compounds for indicating different chemical reaction pathways. Ind. Eng. Chem. Res. 42, 267–279. doi: 10.1021/ie0202773
Logan, B. E., and Rabaey, K. (2012). Conversion of wastes into bioelectricity and chemicals by using microbial electrochemical technologies. Science 337, 686–690. doi: 10.1126/science.1217412
Logan, B. E., Rossi, R., Ragab, A., and Saikaly, P. E. (2019). Electroactive microorganisms in bioelectrochemical systems. Nat. Rev. Microbiol. 17, 307–319. doi: 10.1038/s41579-019-0173-x
Lovley, D. R. (2012). Electromicrobiology. Annu. Rev. Microbiol. 66, 391–409. doi: 10.1146/annurev-micro-092611-150104
Lu, X., Jordan, B., and Berge, N. D. (2012). Thermal conversion of municipal solid waste via hydrothermal carbonization: comparison of carbonization products to products from current waste management techniques. Waste Manag. 32, 1353–1365. doi: 10.1016/j.wasman.2012.02.012
Marin-Batista, J. D., Mohedano, A. F., Rodriguez, J. J., and De La Rubia, M. A. (2020). Energy and phosphorous recovery through hydrothermal carbonization of digested sewage sludge. Waste Manag. 105, 566–574. doi: 10.1016/j.wasman.2020.03.004
Merzari, F., Langone, M., Andreottola, G., and Fiori, L. (2019). Methane production from process water of sewage sludge hydrothermal carbonization. A review. Valorising sludge through hydrothermal carbonization. Crit. Rev. Environ. Sci. Technol. 49, 947–988. doi: 10.1080/10643389.2018.1561104
Miceli, J. F. 3rd, Parameswaran, P., Kang, D. W., Krajmalnik-Brown, R., and Torres, C. I. (2012). Enrichment and analysis of anode-respiring bacteria from diverse anaerobic inocula. Environ. Sci. Technol. 46, 10349–10355. doi: 10.1021/es301902h
Nam, J. Y., Kim, H. W., Lim, K. H., and Shin, H. S. (2010). Effects of organic loading rates on the continuous electricity generation from fermented wastewater using a single-chamber microbial fuel cell. Bioresour. Technol. 101, S33–S37. doi: 10.1016/j.biortech.2009.03.062
Nizamuddin, S., Baloch, H. A., Griffin, G. J., Mubarak, N. M., Bhutto, A. W., Abro, R., et al. (2017). An overview of effect of process parameters on hydrothermal carbonization of biomass. Renew. Sustain. Ener. Rev. 73, 1289–1299. doi: 10.1016/j.rser.2016.12.122
Pant, D., Arslan, D., Van Bogaert, G., Gallego, Y. A., De Wever, H., Diels, L., et al. (2013). Integrated conversion of food waste diluted with sewage into volatile fatty acids through fermentation and electricity through a fuel cell. Environ. Technol. 34, 1935–1945. doi: 10.1080/09593330.2013.828763
Passos, F., and Ferrer, I. (2015). Influence of hydrothermal pretreatment on microalgal biomass anaerobic digestion and bioenergy production. Water Res. 68, 364–373. doi: 10.1016/j.watres.2014.10.015
Patil, S. A., Hägerhäll, C., and Gorton, L. (2012). Electron transfer mechanisms between microorganisms and electrodes in bioelectrochemical systems. Bioanal. Rev. 4, 159–192. doi: 10.1007/s12566-012-0033-x
Pincam, T., Brix, H., and Jampeetong, A. (2020). Growth performance of tropical wetland species (Cyperus involucratus Rottb. and Thalia geniculata L.) in anaerobic digester effluent and their water treatment efficiency. Ecol. Eng. 143, 105667–105676. doi: 10.1016/j.ecoleng.2019.105667
Roden, E. E., Kappler, A., Bauer, I., Jiang, J., Paul, A., Stoesser, R., et al. (2010). Extracellular electron transfer through microbial reduction of solid-phase humic substances. Nat. Geosci. 3, 417–421. doi: 10.1038/ngeo870
Sun, M., Zhai, L. F., Li, W. W., and Yu, H. Q. (2016). Harvest and utilization of chemical energy in wastes by microbial fuel cells. Chem. Soc. Rev. 45, 2847–2870. doi: 10.1039/C5CS00903K
Thygesen, A., Poulsen, F. W., Angelidaki, I., Min, B., and Bjerre, A.-B. (2011). Electricity generation by microbial fuel cells fuelled with wheat straw hydrolysate. Biomass Bioenerg. 35, 4732–4739. doi: 10.1016/j.biombioe.2011.09.026
Titirici, M. M., and Antonietti, M. (2010). Chemistry and materials options of sustainable carbon materials made by hydrothermal carbonization. Chem. Soc. Rev. 39, 103–116. doi: 10.1039/B819318P
Velvizhi, G., Goud, R. K., and Venkata Mohan, S. (2014). Anoxic bio-electrochemical system for treatment of complex chemical wastewater with simultaneous bioelectricity generation. Bioresour. Technol. 151, 214–220. doi: 10.1016/j.biortech.2013.10.028
Wang, X., Liu, Y., Cui, X., Xiao, J., Lin, G., Chen, Y., et al. (2020). Production of furfural and levoglucosan from typical agricultural wastes via pyrolysis coupled with hydrothermal conversion: Influence of temperature and raw materials. Waste Manag. 114, 43–52. doi: 10.1016/j.wasman.2020.06.045
Wang, Z., Lee, T., Lim, B., Choi, C., and Park, J. (2014). Microbial community structures differentiated in a single-chamber air-cathode microbial fuel cell fueled with rice straw hydrolysate. Biotechnol. Biofuel 7, 9–18. doi: 10.1186/1754-6834-7-9
Weiner, B., Poerschmann, J., Wedwitschka, H., Koehler, R., and Kopinke, F.-D. (2014). Influence of process water reuse on the hydrothermal carbonization of paper. ACS Sustain. Chem. Eng. 2, 2165–2171. doi: 10.1021/sc500348v
Wen, S., Liu, H., He, H., Luo, L., Li, X., Zeng, G., et al. (2016). Treatment of anaerobically digested swine wastewater by Rhodobacter blasticus and Rhodobacter capsulatus. Bioresour. Technol. 222, 33–38. doi: 10.1016/j.biortech.2016.09.102
Wirth, B., and Mumme, J. (2014). Anaerobic digestion of waste water from hydrothermal carbonization of corn silage. Appl. Bioener. 1, 1–10. doi: 10.2478/apbi-2013-0001
Wirth, B., Reza, T., and Mumme, J. (2015). Influence of digestion temperature and organic loading rate on the continuous anaerobic treatment of process liquor from hydrothermal carbonization of sewage sludge. Bioresour. Technol. 198, 215–222. doi: 10.1016/j.biortech.2015.09.022
Xu, X., and Jiang, E. (2017). Treatment of urban sludge by hydrothermal carbonization. Bioresour. Technol. 238, 182–187. doi: 10.1016/j.biortech.2017.03.174
Yang, G., Huang, L., You, L., Zhuang, L., and Zhou, S. (2017). Electrochemical and spectroscopic insights into the mechanisms of bidirectional microbe-electrode electron transfer in Geobacter soli biofilms. Electrochem. Commun. 77, 93–97. doi: 10.1016/j.elecom.2017.03.004
Zang, G. L., Sheng, G. P., Tong, Z. H., Liu, X. W., Teng, S. X., Li, W. W., et al. (2010). Direct electricity recovery from Canna indica by an air-cathode microbial fuel cell inoculated with rumen microorganisms. Environ. Sci. Technol. 44, 2715–2720. doi: 10.1021/es902956e
Zhang, Y., Min, B., Huang, L., and Angelidaki, I. (2009). Generation of electricity and analysis of microbial communities in wheat straw biomass-powered microbial fuel cells. Appl. Environ. Microbiol. 75, 3389–3395. doi: 10.1128/AEM.02240-08
Zhang, Y., Min, B., Huang, L., and Angelidaki, I. (2011). Electricity generation and microbial community response to substrate changes in microbial fuel cell. Bioresour. Technol. 102, 1166–1173. doi: 10.1016/j.biortech.2010.09.044
Zhao, F., Heidrich, E. S., Curtis, T. P., and Dolfing, J. (2020). The effect of anode potential on current production from complex substrates in bioelectrochemical systems: a case study with glucose. Appl. Microbiol. Biotechnol. 104, 5133–5143. doi: 10.1007/s00253-020-10547-6
Zhao, P., Shen, Y., Ge, S., and Yoshikawa, K. (2014). Energy recycling from sewage sludge by producing solid biofuel with hydrothermal carbonization. Energ. Convers. Manage. 78, 815–821. doi: 10.1016/j.enconman.2013.11.026
Keywords: microbial electrochemical technologies, wastewater treatment, electroactive microorganisms, microbial electrochemistry, microbial fuel cell, Geobacter biofilm anode
Citation: Dai S, Korth B, Vogt C and Harnisch F (2021) Microbial Electrochemical Oxidation of Anaerobic Digestion Effluent From Treating HTC Process Water. Front. Chem. Eng. 3:652445. doi: 10.3389/fceng.2021.652445
Received: 12 January 2021; Accepted: 19 March 2021;
Published: 23 April 2021.
Edited by:
Susana Rodriguez-Couto, Lappeenranta-Lahti University of Technology (LUT University), FinlandReviewed by:
Joan Dosta, University of Barcelona, SpainCopyright © 2021 Dai, Korth, Vogt and Harnisch. This is an open-access article distributed under the terms of the Creative Commons Attribution License (CC BY). The use, distribution or reproduction in other forums is permitted, provided the original author(s) and the copyright owner(s) are credited and that the original publication in this journal is cited, in accordance with accepted academic practice. No use, distribution or reproduction is permitted which does not comply with these terms.
*Correspondence: Falk Harnisch, ZmFsay5oYXJuaXNjaEB1ZnouZGU=; Benjamin Korth, YmVuamFtaW4ua29ydGhAdWZ6LmRl
Disclaimer: All claims expressed in this article are solely those of the authors and do not necessarily represent those of their affiliated organizations, or those of the publisher, the editors and the reviewers. Any product that may be evaluated in this article or claim that may be made by its manufacturer is not guaranteed or endorsed by the publisher.
Research integrity at Frontiers
Learn more about the work of our research integrity team to safeguard the quality of each article we publish.