- 1Department of Chemistry, Tri-Chandra Multiple Campus, Tribhuvan University, Kathmandu, Nepal
- 2Central Department of Chemistry, Tribhuvan University, Kirtipur, Nepal
- 3Department of Chemistry, Prithvi Narayan Campus, Tribhuvan University, Pokhara, Nepal
In this study, we report on a combined approach to preparing an active electrode material for supercapattery application by making nanocomposites of Polyaniline/Cerium (PANI/Ce) with different weight percentages of magnetite (Fe3O4). Fourier-transform infrared spectroscopy (FTIR) and x-ray diffraction (XRD) analyses supported the interaction of PANI with Ce and the formation of the successful nanocomposite with magnetite nanoparticles. Scanning electron microscopy (SEM) and transmission electron microscopy (TEM) analyses showed the uniform and porous morphology of the composites. Cyclic voltammetry (CV) and galvanostatic charge–discharge (GCD) were used to test the supercapattery behavior of the nanocomposite electrodes in 1.0 M H2SO4. It was found that the supercapattery electrode of PANI/Ce+7 wt.% Fe3O4 exhibited a specific capacity of 171 mAhg−1 in the potential range of −0.2 to 1.0 V at the current density of 2.5 Ag−1. Moreover, PANI/Ce+7 wt.% Fe3O4 revealed a power density of 376.6 Wkg−1 along with a maximum energy density of 25.4 Whkg−1 at 2.5 Ag−1. Further, the cyclic stability of PANI/Ce+7 wt.% Fe3O4 was found to be 96.0% after 5,000 cycles. The obtained results suggested that the PANI/Ce+Fe3O4 nanocomposite could be a promising electrode material candidate for high-performance supercapattery applications.
Introduction
The study of energy storage devices is an important field of research aiming to develop the next generation of cost-effective, durable, energy-efficient, and environmentally friendly power sources for the feasible implantation of renewable energy (Patil et al., 2011). Electrochemical capacitors are a family of energy storage devices possessing excellent reversibility, high power delivery or uptake ability, and long operating lifetimes due to either the high reversible ion adsorption mechanism or the fast surface redox reaction (Simon and Gogotsi, 2008; Salanne et al., 2016; Wang et al., 2017). These remarkable features make electrochemical capacitors promising devices for power capture and supply, backup, safety, and low-maintenance applications (Hall et al., 2010). The low energy density (E) of electrochemical capacitors can be enhanced by hybridizing their merits with battery-grade materials together in a single device (Yu and Chen, 2020).
Supercapattery, a supercapacitor mixed with a battery, takes the merits of the high charge–discharge capability of supercapacitors and the large charge storage ability of batteries (Yu and Chen, 2016). In electrical double-layer capacitors (EDLCs), the capacitance arises by the accumulation of charges at the electrode/electrolyte interfaces on the application of voltage bias (Ji et al., 2014). In pseudocapacitors (PCs), the pseudocapacitance arises from superficial faradic reaction occurring at the electrode/electrolyte interfaces and possesses a much higher specific capacitance (Hu et al., 2015). Transition metal compounds (oxides/hydroxides/nitrides/sulfides) and electronically conducting polymers are extensively researched active materials for usage in PCs (Ballarin et al., 2019). The electrode materials in batteries store faradic charge but are non-capacitive in nature, which results in peak-shaped cyclic voltammetry (CV) and a non-linear galvanostatic charge–discharge (GCD) curve (Yu and Chen, 2016). The metal oxides and conducting polymer composites as redox materials can play an important role in the charge storage of a supercapattery (supercapacitor + battery) cells. These composites gained the merits of both capacitive and non-capacitive faradic charge storage mechanisms (Ma et al., 2013).
Among several electronically conducting polymers, polyaniline (PANI) has gained particular interest for its excellent redox reversibility, superior capacitive behavior with a high specific capacity, low cost, facile synthesis in the form of powder or thin-film by chemical or electrochemical oxidative polymerization, environmental-friendliness, and unique doping/dedoping behaviors (Li et al., 2009; Jiang et al., 2014). Despite all these peculiar features, one of the challenging issues that render PANI an active material for high-performance supercapattery is its low electronic conductivity and poor stability during the charge–discharge process (Patil et al., 2012; Tharani and Vinayagam, 2015). Extensive research work has been carried out to overcome this issue. An approach to tackle this issue is either metal doping, which enhances the electronic conduction of the electrode, or metal oxide nanocomposite formation, which facilitates the diffusion of ions due to the large accessible internal surface area. The latter provides an enhanced charge–discharge rate and, hence, supercapattery performance (Patil et al., 2012; Zeng et al., 2016).
In recent years, the doping of f-block Ce has gained more attention. It is due to the higher stability stemming from the accessibility of the shielded 4f orbitals for strong binding affinity with the nitrogen lone pair of PANI and the suitable redox activity (Tharani and Vinayagam, 2015; Li et al., 2018). Of the metal compounds, spinel metal oxides Fe3O4 nanoparticles have received more considerable attention. It has added merits such as improving the electrochemical activities, inexpensiveness, high theoretical specific capacity (926 mAhg−1), large potential window, and environmental compatibility (Umare et al., 2010; Xavier et al., 2014; Nithya and Arul, 2016; Prasankumar et al., 2018). Li et al. prepared PANI/Ce3+ and PANI/Ce4+ composites by in situ polymerization and found that the electrical conductivity of PANI/Ce(NO3)3 increased by 377% in comparison to pure PANI. The result was attributed to more charges and faster movements of Ce3+ (Li et al., 2018). Rantho et al. fabricated symmetric supercapacitors with supercapattery behavior based on carbonized iron cations adsorbed onto polyaniline, which exhibited a higher energy and power density of 41.3 Whkg−1 and 231.9 Wkg−1, respectively (Rantho et al., 2018). Prasankumar et al. investigated the pseudocapacitive performance of PANI wrapped over Fe3O4 nanoparticles and found that voids are built during the formation of microspheres from the nanosphere, which improved the charge storage (Prasankumar et al., 2018). A capacitance value of 572 Fg−1 at 0.5 Ag−1 was reported with capacitance retention of 82% after 5,000 charge–discharge cycles. Although the porous PANI/Fe3O4 nanocomposites used in the study enhanced the capacitive performance of the supercapacitors, the rate performance data implied limited ion transport and ion accessibility.
Herein, nanocomposites based on different weight percentages of Fe3O4 nanoparticles encapsulated in Ce-doped PANI were prepared by in situ chemical oxidative polymerization and used as active materials to prepare electrodes for high-performance supercapattery. The objective of the synthesis of PANI/Ce+7 wt.% Fe3O4 nanocomposite electrode for supercapattery is to improve the electronic conductivity by doping along with an increase in charge–discharge rate due to a decrease in the diffusion path length by the formation of nanocomposites. The Fe3O4 nanoparticle concentration was varied to determine its effect on the magnitude of different electrochemical performance metrics of PANI/Ce.
Materials and Methods
Materials
Aniline, obtained from Fisher Scientific, India, was purified using the double distillation method under reduced pressure. Ammonium persulfate ([NH4]2S2O8) and Cerium (III) nitrate hexahydrate (Ce[NO3]3.6H2O) were obtained from SD Fine Chemicals, India. Ammonium iron (II) sulfate hexahydrate ([NH4]2Fe[SO4]2.6H2O), iron(III) chloride hexahydrate (FeCl3.6H2O), sulfuric acid (H2SO4), sodium hydroxide (NaOH), hydrochloric acid (HCl), and Cetrimide purified were obtained from Merck Life Science Private Ltd., India. The binder polyvinylidene difluoride (PVDF), solvent N-Methyl-2-pyrrolidone (NMP), nickel foam (Ni-foam), and carbon black (nanopowder) were obtained from Xiamen Tob Energy Technology, China. All the mentioned chemicals were of analytical reagent grade and were used without any further chemical modification (except aniline). Double distilled water was used to prepare all solutions.
Preparation of Ce-Doped PANI/Fe3O4 Nanocomposites
Magnetite nanoparticles were prepared by using the co-precipitation method (Ghandoor et al., 2012). The solutions of (NH4)2Fe(SO4)2.6H2O and FeCl3.6H2O were mixed at a 1:2 ratio at 80°C, in an alkaline medium (0.8 M NaOH) to obtain Fe3O4 nanoparticles. Then, 1.82 ml of purified aniline was dispersed into 150 ml of 1.0 M HCl. Subsequently, 91.0 mg of Ce(NO3)3.6H2O was added to the solution and stirred at room temperature for 15 min to facilitate the proper dispersion of Ce3+ ions. An appropriate quantity of Fe3O4 nanoparticles (1, 3, 5, and 7 wt.%) and cetrimonium bromide (CTAB) in 20 ml of double distilled water was ultrasonicated for 20 min. The mixture was dispersed with aniline and cerium nitrate by further ultrasonication for 30 min. Then, 2.0 g of (NH4)2S2O8 in 30 ml of 1.0 M HCl was dropped into the above solution slowly under constant stirring for 7 h to complete the polycondenzation polymerization process. The final products were collected by filtration, washed with distilled water and ethanol, and dried for 16 h at 70°C. Also, for comparison, pure PANI and Ce-doped PANI were synthesized in the same manner without the addition of Fe3O4 nanoparticles and CTAB (Ghandoor et al., 2012).
Characterization of Synthesized Materials
The Fourier-transform infrared spectroscopy (FTIR) spectra of PANI and PANI/Ce with different wt.% of Fe3O4 were recorded using the IRPrestige-21 FTIR spectrometer (Shimadzu, Japan) in the range of 4,000–390 cm−1. The crystalline phase of the materials was investigated by powder X-ray diffraction (XRD) measurements on a Bruker diffractometer (Billerica, MA, United States) using Cu-Kα radiation (λ = 1.5406 Å) in the range of 2θ = 10°-70°. The UV-Vis spectra of materials dispersed in NMP were recorded using the Specord 200 Plus spectrophotometer (Analytik Jena, Jena, Germany). The morphology of the materials was observed by field-emission scanning electron microscopy (FE-SEM; S7400, Hitachi, Japan) equipped with an energy dispersive x-ray spectrometer (EDX), and the structure was characterized by transmission electron microscopy (TEM; JEM-2200, JEOL, Japan; 200 kV).
Electrochemical Measurements
All electrochemical properties were evaluated in a three-electrode setup comprising of a platinum wire as a counter electrode and a saturated calomel electrode (SCE) as a reference electrode. A Hokuto Denko (Japan) Ha-151 Potentiostat/Galvanostat controlled by a self-made LabVIEW-based program (Neupane, 2013) was used for the electrochemical measurements. The working electrodes were prepared by pressing a homogeneous slurry formed by grinding 80 wt.% active materials, 10 wt.% PVDF, and 10 wt.% carbon black with a few drops of the NMP solvent. The composition was further fixed onto a nickel foam current collector with a dimension of 1.0 cm × 0.5 cm and dried in a vacuum oven at 60°C for 12 h. The loading mass of active materials for all electrodes was 3.0 mg. CV and GCD measurements were carried out to estimate the capacities and cyclic stability of the materials in 1.0 M H2SO4. The specific capacity (Csp) was calculated from the integration of the resulting cyclic voltammogram, according to the equation (Zhang and Pan, 2015; Chen, 2017; Iqbal et al., 2020a; Numan et al., 2020):
The values of Csp, energy density (E), and power density (P) were calculated from GCD according to the equation (Zhang and Pan, 2015; Chen, 2017; Iqbal et al., 2020a; Numan et al., 2020):
A two-electrode symmetric cell system was also assembled to compare the performance metrics with the results obtained from the three-electrode cell in the 1.0 M H2SO4 electrolyte using a separator. The values of Csp, E, and P were calculated according to the equation (Zhang and Pan, 2015; Chen, 2017; Iqbal et al., 2020a; Numan et al., 2020):
Where the equivalent series resistance (ESR) calculated from the IRdrop of GCD curve. The current (I) with a discharge time (Δt) between potential limits excluding IRdrop. The tested potential window (ΔU) and mass (m) of active material within the electrode is also calculated with the above equation. It is well-known that the specific capacity of a three-electrode cell is four times larger than that of a two-electrode cell, so for comparison, Equation (3) is divided by 4 (Zhang and Pan, 2015; Chen, 2017).
Results and Discussion
Structure Characteristics and Morphology
The field-emission (FE-SEM) measurements were used to characterize the morphological features of different compositions. Figure 1A shows the aggregated coarse structure of the PANI nanorods. After doping Ce3+ on PANI, as shown in Figure 1B, well-distributed nanorods with nanopores are observed. The obtained morphology provides a large surface-area-to-volume ratio by averting the aggregation of PANI and may assist in influencing specific capacitance and charge–discharge rates. The SEM micrographs of Ce-doped PANI with different wt.% of Fe3O4 clearly show the short nanorod-like uniform morphological appearance (Figures 1C–F). Smooth Fe3O4 nanospheres are seen as adhered to the nanorods of PANI. The uniform morphology of the PANI/Ce+7 wt.% Fe3O4 composites exhibited extended interfaces with smaller particle size, as shown in Figure 1F. This feature might be due to the agglomerating tendency of the nanoparticles and the formation of a cluster or agglomerates.
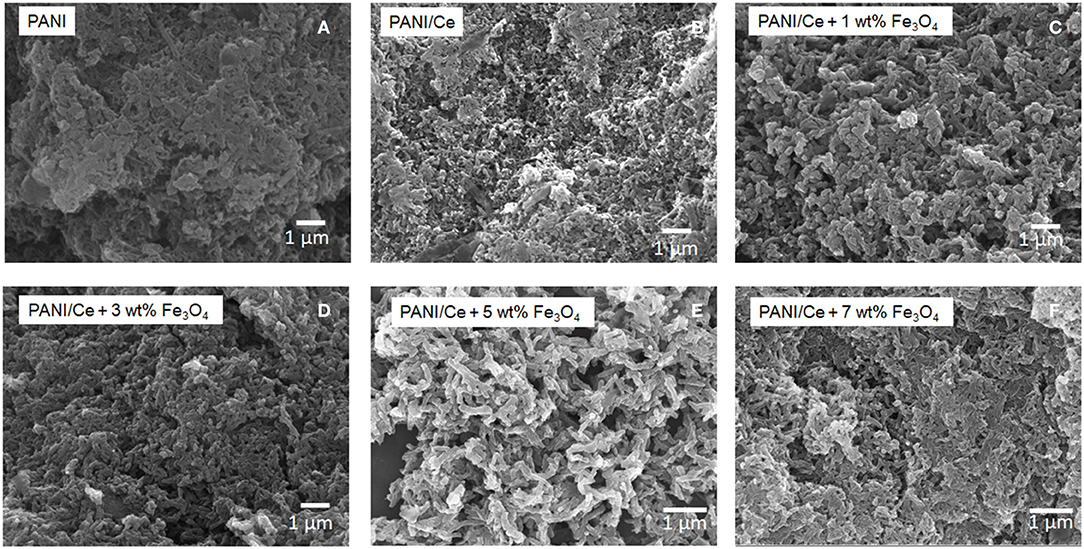
Figure 1. SEM images of (A) PANI, (B) PANI/Ce, PANI/Ce with (C) 1 wt.%, (D) 3 wt.%, (E) 5 wt.%, and (F) 7 wt.% Fe3O4.
From the SEM images, the effects of Ce doping and Fe3O4 addition on the morphological evolution of PANI nanocomposite helped to understand their role in developing a smaller particle size. For further structural information, PANI/Ce+5 wt.% Fe3O4 was chosen as the test subject to perform EDX mapping and TEM analysis. Figure 2 represents the EDX mapping of the PANI/Ce+5 wt.% Fe3O4 with its elemental composition of C, O, N, and Ce. The elements are homogeneously distributed within the nanocomposite (Figures 2A,B).
The morphology and size distribution of the PANI/Ce+5 wt.% Fe3O4 nanocomposites were further analyzed using TEM. Figure 3A displays a short nanorod-like morphology. The image reveals that the PANI/Ce chain was absorbed on the surface of the Fe3O4 nanoparticles. The nanoparticles are uniformly dispersed in the PANI/Ce matrix and form a regular polymeric chain-like nanostructure. The average width is 50–60 nm, which was calculated by using the ImageJ software as presented in the histogram and Lorentzian curve fitting in Figure 3B. Also, the length of the nanorod is in the range of 150–200 nm (Li et al., 2009).
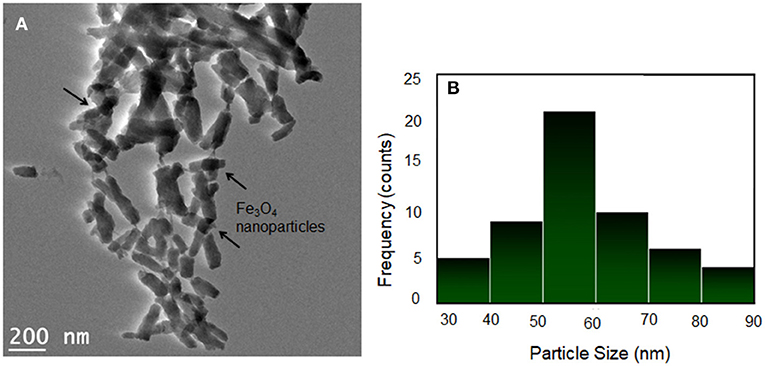
Figure 3. (A) TEM image of PANI/Ce+5 wt.% Fe3O4 and (B) the corresponding histogram and Lorentzian curve fitting. The arrows denote Fe3O4 nanoparticles.
Figure 4 shows the FTIR spectra of PANI, PANI/Ce, and Ce-doped PANI with different wt.% of Fe3O4. The characteristic peaks at 1,590, 1,488, 1,294, 1,158, and 836 cm−1 are attributed to the C=C stretching mode of a quinonoid ring, C=C stretching mode of a benzenoid ring, C-N stretching mode of a quinonoid ring, C-H in-plane and out of plane bending mode of PANI, respectively (Butoi et al., 2017). The broadband around 3,435 cm−1 are allocated to the N-H stretching mode of PANI (Tharani and Vinayagam, 2015). The peak corresponding to N-H stretching at 3,435 cm−1 is broadened and less intense due to the simultaneous occurrence of another new vibrational mode after Ce-doping. Sharp absorption peaks at 518 and 452 cm−1 are assigned to the presence of Fe3O4 in the PANI/Ce matrix; however, PANI also shows firm peaks in the same regions; therefore, the peak intensity decreases with the increase in the amount of Fe3O4 (Silva et al., 2020).
Similarly, Figure 5 represents the XRD patterns of Fe3O4, PANI, and PANI/Ce with different wt.% of Fe3O4. The diffraction peaks are associated with (111), (220), (311), (400), (511), and (440) planes, and all the Bragg planes are indexed to the cubic phase of Fe3O4 following the reported data (JCPDS file no. 79-0417) (Ghandoor et al., 2012). The average crystallite size of Fe3O4 nanoparticles is 8.45 nm, as calculated by using the Scherrer equation (Holzwarth and Gibson, 2011). Peaks that appeared at 2θ = 15.2°, 19.6°, and 26.1° revealed the semi-crystalline nature of PANI (Sampreeth et al., 2018). The degree of crystallinity of PANI/Ce with 3, 5, and 7 wt.% are found to be 2.16, 4.99, and 8.83%, respectively, as calculated using the (311) sharp peaks of Fe3O4 in the composites. In contrast, the XRD patterns of PANI/Ce with different wt.% of Fe3O4 displayed an increase in the degree of crystallinity with wt.% of Fe3O4 due to strong interaction between the vacant d-orbitals of Fe and the lone pair electrons of the nitrogen atom of PANI/Ce (Koysuren and Koysuren, 2019).
Figure 6A shows the UV-Vis spectra of PANI, PANI/Ce, and PANI/Ce with different wt.% Fe3O4. The observed absorption spectra revealed a significant absorption peak at 366 nm due to the Π-Π* transition of the benzenoid structure being in good agreement with the results mentioned in earlier work (Khan et al., 2015). A similar band is observed for PANI/Ce with the hypsochromic shift. And, the hyperchromic effect caused by a strong interaction between PANI and doped element, leading to an easy charge transfer process. A broad absorption band of Ce-doped PANI appears due to the superimposed electronic transition behavior on the other molecular energy state.
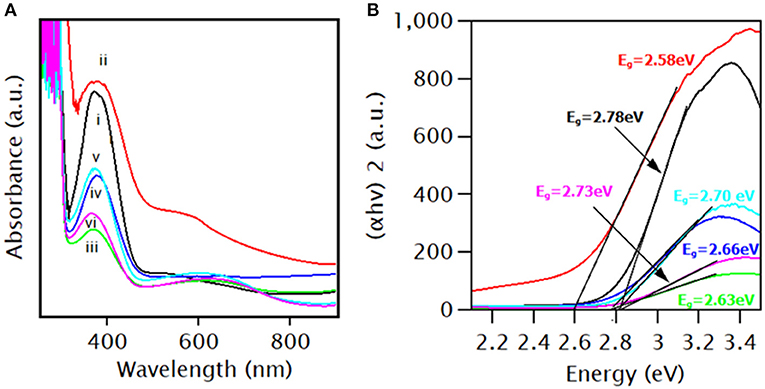
Figure 6. (A) UV-Vis spectra of (i) PANI, (ii) PANI/Ce, PANI/Ce with (iii) 1 wt.%, (iv) 3 wt.%, (v) 5 wt.%, and (vi) 7 wt.% Fe3O4, and (B) the corresponding Tauc plot.
The UV-Vis spectra of PANI/Ce with different wt.% of Fe3O4 showed the hypochromic effect of Ce-doped PANI due to the strong interaction between the Fe3O4 nanoparticles and the Ce-doped polymer chain (Umare et al., 2010). Also, the hyperchromic effects are observed up to 5 wt.% of Fe3O4, and then the hypochromic effect was observed for 7 wt.% of Fe3O4. The uniform dispersion of Fe3O4 nanoparticles within the PANI/Ce matrix leads to higher broadness and intensity of the PANI/Ce+5 wt.% Fe3O4 nanocomposites. In PANI/Ce+7 wt.% Fe3O4, the aggregation and the formation of the cluster in the PANI/Ce matrix diminished the intensity of the absorption peak. Therefore, it revealed a higher interfacial interaction for the 5 wt.% Fe3O4 composites (Sampreeth et al., 2018). Likewise, the bandgap energy of PANI, PANI/Ce, PANI/Ce+1, 3, 5, and 7 wt.% Fe3O4 were 2.78, 2.58, 2.63, 2.66, 2.70, and 2.73 eV, respectively (Figure 6B). The bandgap energy is decreased with Ce-doping, owing to allowed shallow states created in the bandgap (Bahmanrokh et al., 2020). Thus, the doping of cerium generated some energy levels that were close to the bandgap. It allowed a lower energy transition, resulting in a decrease in bandgap energy. Also, the bandgap energy increased with the wt.% of Fe3O4 in Ce-doped PANI due to the change in electron density and interfacial interactions (Sampreeth et al., 2018).
Electrochemical Characterizations
Electrochemical measurements were carried out to test the supercapattery performance of the prepared compositions. CVs of as-prepared PANI, PANI/Ce, and PANI/Ce with different wt.% of Fe3O4 used as the active electrode material in the three-electrode system were recorded at the sweep rate of 10 mVs−1. The fixed operating potential range of −0.2 to 1.0 V vs. SCE in 1.0 M H2SO4 electrolyte was used. CVs of active materials show oxidation and reduction peaks due to the faradic charge transfer process in the electrode materials, as shown in Figure 7A. The CV of PANI was composed of two redox couple reactions. The presence of an oxidization peak at about 0.23 V was due to the transformation of leucoemeraldine to conductive emeraldine salt form and the presence of another at 0.72 V was due to the formation of a fully oxidized pernigraniline state from the emeraldine oxidation state. The reduction peaks at 0.05 and 0.58 V represent the transformation of the leucoemeraldine and emeraldine base, respectively (Martyak et al., 2002). The Ce-doping led to a shift in oxidation peaks to 0.20 and 0.67 V, and the reduction peaks to 0.03 and 0.63 V, toward lower potential. As the wt.% of Fe3O4 increased in Ce-doped PANI, the peaks further shifted to the lower potential with an increase in the area of CV.
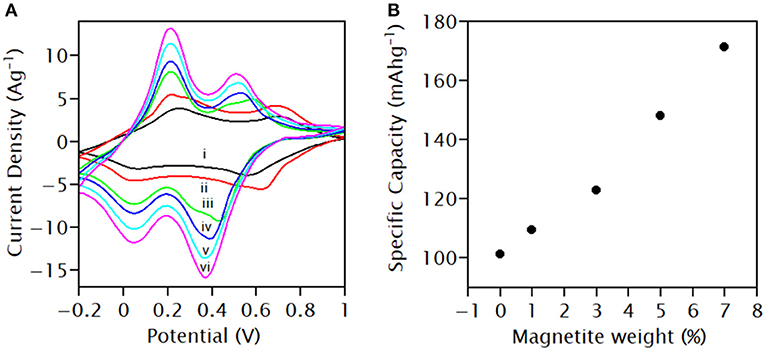
Figure 7. (A) Cyclic voltammetry of (i) PANI, (ii) PANI/Ce, PANI/Ce with (iii) 1 wt.%, (iv) 3 wt.%, (v) 5 wt.%, and (vi) 7 wt.% Fe3O4 in the three-electrode system at a scan rate of 10 mVs−1; (B) variation of specific capacity of PANI/Ce with wt.% of Fe3O4.
The formation of composites with Fe3O4 has enhanced the electrochemical performance of the Ce-doped PANI electrode due to three different redox mechanisms (Martyak et al., 2002). On the integration of the CV curves of PANI/Ce with 7 wt.% Fe3O4 from −0.2 to 0.0 V vs. SCE, a capacitance of 263.5 F/g was calculated due to capacitive charge storage. Also, the same calculation was performed on the same CV within the potential windows of −0.2 to 0.2 V, 0.2–0.4 V, and 0.4–0.8 V, and the capacitance values were calculated to be 1,071.5, 1,057.25, and 908.25 F/g, respectively, with a pair of redox peaks, which indicate that the capacitive charge storage mixed along with the faradic charge storage arises from a faradic mechanism involving peak-shaped CV. This calculation indicates that charge storage (Q) is some continuous function of the potential (U), and the derivative dQ/dU arises, which has properties of capacitive mentioned by Brousse et al. and non-capacitive faradic contribution (Brousse et al., 2015).
The specific capacity of PANI/Ce+7 wt.% Fe3O4 is 172 mAhg−1 (616.8 Cg−1), whereas that of pure PANI is 70 mAhg−1 (252 Cg−1). The specific capacity of Ce-doped PANI increased from 102 to 172 mAhg−1 with an increment in the wt.% of Fe3O4 from 1 to 7 wt.%, as depicted in Figure 7B. The results might be due to the increasing exposure of the electrode surface and increasing the number of active sites for surface redox reaction with the formation of composites (Augustyn et al., 2014).
Figure 8A shows the GCD curves of all the samples which were carried out at a current density of 2.5 Ag−1. The GCD curves are non-linear and asymmetrical with charge–discharge plateaus, indicating that the capacity of PANI, PANI/Ce, and PANI/Ce with different wt.% of Fe3O4 mainly arose from both capacitive and non-capacitive contributions, as explained above (Iqbal et al., 2020a; Numan et al., 2020). A small IR drop in the GCD curve is observed (indicated by a linear drop). The specific capacity of PANI, Ce-doped PANI, and Ce-doped PANI with 1, 3, 5, and 7 wt.% Fe3O4 as a function of constant charge–discharge current density is found to be 70, 103, 108, 122, 149, and 171 mAhg−1, respectively. These values are similar to the CV plot (Figure 7A). The performance metrics were calculated after deducing the IR drop, as shown in Table 1. The increase in the number of delocalized ions of PANI by doping of Ce improved the conductivity and enhanced the specific capacity in Ce-doped PANI (Li et al., 2018). Also, the pseudocapacitive contribution of the Fe3O4 nanoparticles increased the specific capacity of composites. Umare et al. reported a discharge capacity value of 78.8 mAhg−1 at a current density of 0.5 mAcm−2 from the GCD for Zn-PANI/Fe3O4 (9:1) (Umare et al., 2010). Furthermore, Iqbal et al. observed a discharge capacity value of 162.5 Cg−1 at a current density of 0.4 Ag−1 in MOF/PANI (50/50%) with the lowest ESR resistance (Iqbal et al., 2020a). When compared to this MOF/PANI electrode, the electrode presented in this paper exhibits a higher specific capacity, which may be due to the favorable microstructure for ion diffusion and enhanced conductivity.
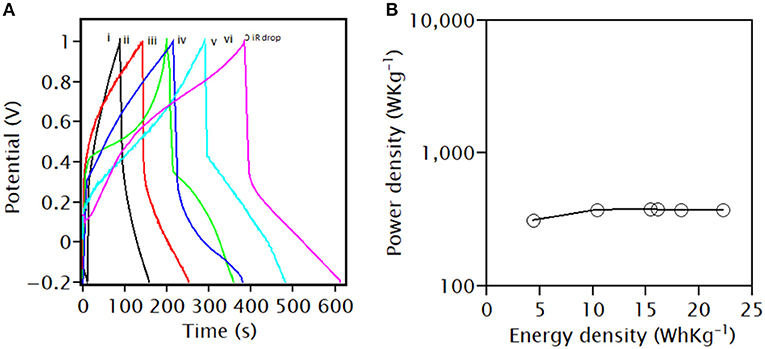
Figure 8. (A) GCD plots of (i) PANI, (ii) PANI/Ce, PANI/Ce with (iii) 1 wt.%, (iv) 3 wt.%, (v) 5 wt.%, and (vi) 7 wt.% Fe3O4 in the three-electrode system at a constant current density of 2.5 Ag−1 and (B) the corresponding Ragone plot.
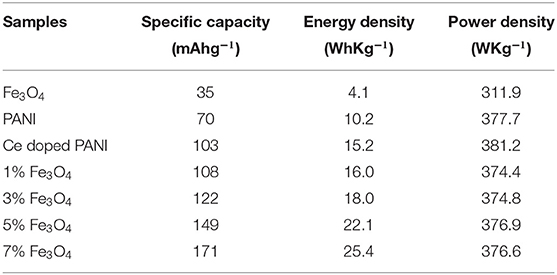
Table 1. Specific capacity, energy density, and power density of prepared sample electrode calculated from GCD.
However, the power density of the composite material value did not change significantly after 5 wt.% of Fe3O4 in the nanocomposites, as shown in Table 1. The result indicates that an increase in the wt.% of Fe3O4 nanoparticles above 7 wt.% would have enhanced the agglomerating tendency of nanoparticles. This would lead to the formation of clusters, which reduces the interfacial interaction between Fe3O4 and Ce-doped PANI, hence resulting in highly resistive electrode materials having lower power deliverance capacity.
As compared with the results from the other studies, the prepared PANI/Ce with 7 wt.% Fe3O4 offers a higher specific capacity of 171 mAhg−1 at a current density of 2.5 Ag−1. From the observation of the Ragone plot shown in Figure 8B, it can be seen that the PANI/Ce with 7 wt.% Fe3O4 composites display a maximum energy density of 25.4 WhKg−1 (Equation 3) without compromising the high power density of 376.6 WhKg−1 (Equation 4). Table 2 gives a comparison of the results obtained in this study with the values of some other supercapattery electrode composite materials based on pseudocapacitive charge storage in aqueous electrolytes from other studies, which again shows that the material proposed in this study gives superior specific capacity values.
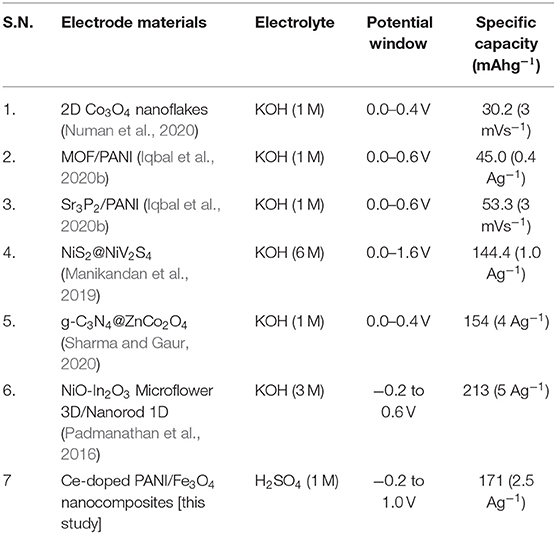
Table 2. Comparative specific capacity performance of supercapattery electrodes based on pseudocapacitive charge storage in aqueous electrolytes.
Figure 9 displays GCD profiles of PANI/Ce with 7 wt.% Fe3O4 at different current densities from 2 to 10 Ag−1. The specific capacity decreases from 188.7 to 97.3 mAhg−1 as the current density increases from 2 to 10 Ag−1. The capacity value decreases by 91%. Similarly, the initial value decreases to 52% when the discharge current increases from 2 to 2.5 Ag−1 and 2 to 10 Ag−1. The inner redox-active sites are not fully accessible for electroactive species at higher current densities, cause decreases in capacity value (Numan et al., 2020). The results indicate that the steady reversibility of the electrode material results from a lower IR drop (Muthulakshmi et al., 2006). The inset shows the linear relation between the IR drop and current densities. A lower ESR value of 0.02891 Ω is found for the PANI/Ce with 7 wt.% Fe3O4, imparting a high charge–discharge ability of electrode materials. This lower value of ESR could be attributed to enhanced electrical conductivity that leads to rapid electron transport even at high current densities during the charge–discharge process (Padmanathan et al., 2016).
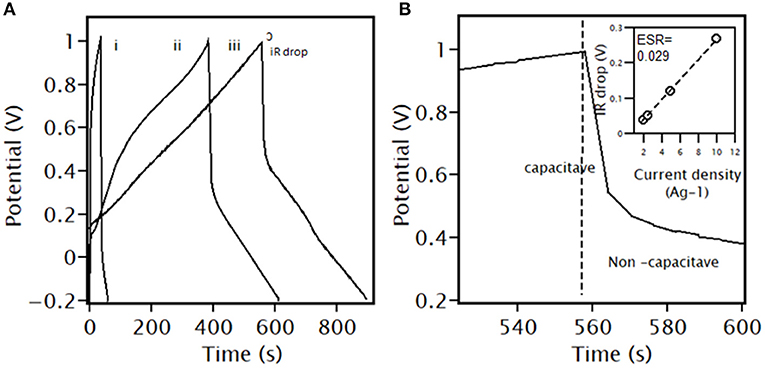
Figure 9. GCD plot of PANI/Ce+7 wt.% Fe3O4 at different current densities of (A) (i) 10 Ag−1, (ii) 2.5 Ag−1, and, (iii) 2 Ag−1 in the three-electrode system; (B) Zoom-in of 2.5 Ag−1. Inset: IR drop condition.
The symmetric supercapacitor with the supercapattery characteristics of PANI/Ce+5 wt.% Fe3O4 is further examined by GCD using a two-electrode system for device applications (Figure 10). The specific capacity, energy density, and power density are calculated to be 43 mAhg−1 (Equation 5), 21.8 WhKg−1 (Equation 6), and 374.9 WKg−1 (Equation 7), respectively. All the mentioned parameters are almost equal to the values calculated for the three-electrode system. It is worth noting that the specific capacity of the three-electrode system is almost four times the specific capacity of the two-electrode cell (Equation 2). This relates that the specific capacity of electrode materials is simply four-fold of the specific capacity of the cell in a symmetrical configuration (Chen, 2017). The results show a good capacity value shown by the nanocomposite of PANI/Ce+5 wt.% Fe3O4.
The cyclic stability test showed that PANI retained 40% of the initial capacity after 5,000 cycles of CV at a scan rate of 100 mVs−1 between −0.2 and 1.0 V, as shown in Figure 11A. The inferior stability of PANI resulted from the strain generated by the swelling and shrinkage during the doping–dedoping of charged ions during CV (Eftekhari et al., 2017). However, PANI/Ce and PANI/Ce+7 wt.% Fe3O4 maintained almost 66.0 and 96.0% of their initial capacity, respectively. The support of the Fe3O4 nanoparticles in the composites can adapt to the volume change that helps to suppress the structural alternation and chemical degradation of PANI in the composite electrodes (Chen, 2017). The stability of iron oxides in an acidic medium has been extensively studied by Salmimies et al., who used nitric acid and sulfuric acid and found dissolution of magnetite at high enough concentrations of these acids (Salmimies et al., 2011). We have also found a 52% degradation of magnetite by the 1.0 M H2SO4 acid after 1,000 cycles of CV at 10 mV/s when used independently. However, the composite materials of PANI/Ce+7 wt.% Fe3O4 showed degradation of only 4%, and therefore, the composite was not affected by the stability of the individual component.
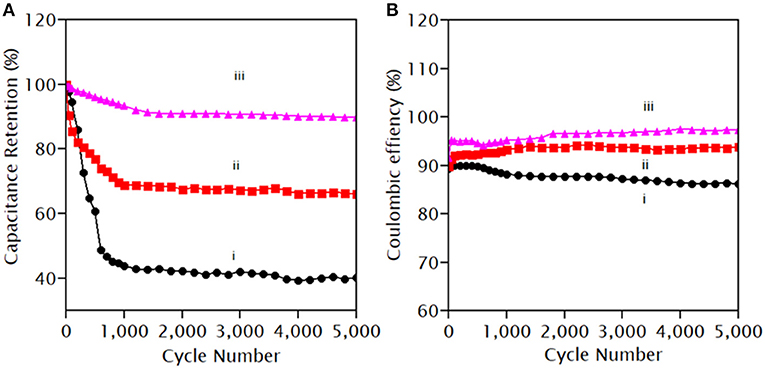
Figure 11. (A) Cycling performance and (B) coulombic efficiency of (i) PANI, (ii) PANI/Ce, and (iii) PANI/Ce with 7 wt% Fe3O4.
Also, the Coulombic efficiency of PANI, PANI/Ce, and PANI/Ce with 7 wt.% Fe3O4 was calculated to be 86.0, 93.8, and 97.3%, respectively, after 5,000 cycles, as shown in Figure 11B. The excellent stability over 5,000 cycles with retention of almost 96% of the initial capacity might be ascribed to the activation process which allows the trapped cations to diffuse out gradually or the insufficient contact of the nanocomposites with the H2SO4 aqueous solution during electrochemical processes (Gul et al., 2019). Thus, cyclic performance and Coulombic efficiency are enhanced due to the contribution of Ce and Fe3O4 on PANI and the synergistic interactions between them.
The enhanced electrochemical performance of PANI/Ce+7 wt.% Fe3O4-based supercapattery can be ascribed to (a) the multiple oxidation states of the redox-active electrode materials leading to the improved energy storage capacity of materials (Martyak et al., 2002); (b) the coordination effect between Ce and the N atom of PANI enhancing the conductivity of PANI, which gives fast accessibility for proton diffusion to electrochemically active sites (Li et al., 2018); (c) the composite formation of PANI/Ce with the Fe3O4 nanocomposites suppressing the mechanical stress, which leads to the enhancement in cyclability (Prasankumar et al., 2018); and (d) the high ionic conductivity of H+ electrolyte promoting better redox reaction of the composites (Yuan et al., 2008).
Conclusion
This study introduces a simple and effective method to synthesize cost-effective and efficient supercapattery electrode materials. The specific capacity and power performances are greatly enhanced by the doping of Ce, followed by the incorporation of Fe3O4 in PANI. PANI/Ce exhibits a maximum capacity of 171 mAhg−1 with an energy density of 25.4 WhKg−1 and a power density of 376.6 WKg−1 with 7 wt.% Fe3O4 at 2.5 Ag−1 of load. The capacity retention is 96.0% after 5,000 cycles of charge–discharge. The synthesized composite is a promising candidate for supercapattery with its attributes of high specific capacities, power density, energy density, and cycle life.
Data Availability Statement
The original contributions presented in the study are included in the article/supplementary material, further inquiries can be directed to the corresponding author/s.
Author Contributions
SP and DG performed the experiments. AD, NK, SS, and RY helped to analyze the data. SP and SN provided the first draft of the manuscript. AY conceived and designed the experiments. SN and AY finalized the manuscript. All authors analyzed the data, discussed the results, co-wrote the paper, and commented on the manuscript.
Funding
This project was funded by the University Grants Commission, Sanothimi, Nepal (University faculty research Grant No. FRG-73/74-S&T-08).
Conflict of Interest
The authors declare that the research was conducted in the absence of any commercial or financial relationships that could be construed as a potential conflict of interest.
Acknowledgments
The University Grants Commission, Sanothimi, Nepal was greatly acknowledged for providing the faculty research grant (Grant No. FRG-73/74-S&T-08). The Central Department of Chemistry and Tri-Chandra Multiple Campus, Tribhuvan University, Nepal, are thanked for providing all the necessary laboratory and instrumentation facilities (FTIR and UV-Vis). We would also like to thank the staff at the Center for Chonbuk University Research Facility (CURF), South Korea, for helping with the FE-SEM, EDS, and TEM analyses.
References
Augustyn, V., Simon, P., and Dunn, B. (2014). Pseudocapacitive oxide materials for high-rate electrochemical energy storage. Energy Environ. Sci. 7, 1597–1614. doi: 10.1039/C3EE44164D
Bahmanrokh, G., Cazorla, C., Mofarah, S. S., Shahmiri, R., Yao, Y., Ismail, I., et al. (2020). Band gap engineering of Ce-doped anatase TiO2 through solid solubility mechanisms and new defect equilibria formalism. Nanoscale 12, 4916–4934. doi: 10.1039/C9NR08604H
Ballarin, B., Boanini, E., Montalto, L., Mengucci, P., Nanni, D., Parise, C., et al. (2019). PANI/Au/Fe3O4 nanocomposite materials for high performance energy storage. Electrochim. Acta 322:134707. doi: 10.1016/j.electacta.2019.134707
Brousse, T., Bélanger, D., and Long, J. W. (2015). To be or not to be pseudocapacitive? J. Electrochem. Soc. 162:A5185. doi: 10.1149/2.0201505jes
Butoi, B., Groza, A., Dinca, P., Balan, A., and Barna, V. (2017). Morphological and structural analysis of polyaniline and poly(o-anisidine) layers generated in a DC glow discharge plasma by using an oblique angle electrode deposition configuration. Polymers 9:732. doi: 10.3390/polym9120732
Chen, G. Z. (2017). Supercapacitor and supercapattery as emerging electrochemical energy stores. Int. Mater. Rev. 62, 173–202. doi: 10.1080/09506608.2016.1240914
Eftekhari, A., Li, L., and Yang, Y. (2017). Polyaniline supercapacitors. J. Power Sources 347, 86–107. doi: 10.1016/j.jpowsour.2017.02.054
Ghandoor, H., El Zidan, H. M., Khalil, M. M. H., and Ismail, M. I. M. (2012). Synthesis and some physical properties of magnetite (Fe3O4) nanoparticles. Int. J. Electrochem. Sci. 7:12. Available online at: http://www.electrochemsci.org/papers/vol7/7065734.pdf
Gul, H., Shah, A.-H. A., and Bilal, S. (2019). Achieving ultrahigh cycling stability and extended potential window for supercapacitors through asymmetric combination of conductive polymer nanocomposite and activated carbon. Polymers 11:1678. doi: 10.3390/polym11101678
Hall, P. J., Mirzaeian, M., Fletcher, S. I., Sillars, F. B., Rennie, A. J. R., Shitta-Bey, G. O., et al. (2010). Energy storage in electrochemical capacitors: designing functional materials to improve performance. Energy Environ. Sci. 3, 1238–1251. doi: 10.1039/C0EE00004C
Holzwarth, U., and Gibson, N. (2011). The Scherrer equation versus the “Debye-Scherrer equation.” Nat. Nanotechnol. 6:534. doi: 10.1038/nnano.2011.145
Hu, C., He, S., Jiang, S., Chen, S., and Hou, H. (2015). Natural source derived carbon paper supported conducting polymer nanowire arrays for high performance supercapacitors. RSC Adv. 5, 14441–14447. doi: 10.1039/C4RA12220H
Iqbal, M. Z., Faisal, M. M., Ali, S. R., and Alzaid, M. (2020a). A facile approach to investigate the charge storage mechanism of MOF/PANI based supercapattery devices. Solid State Ionics 354:115411. doi: 10.1016/j.ssi.2020.115411
Iqbal, M. Z., Faisal, M. M., Sulman, M., Ali, S. R., Afzal, A. M., Kamran, M. A., et al. (2020b). Capacitive and diffusive contribution in strontium phosphide-polyaniline based supercapattery. J. Energy Storage 29:101324. doi: 10.1016/j.est.2020.101324
Ji, H., Zhao, X., Qiao, Z., Jung, J., Zhu, Y., Lu, Y., et al. (2014). Capacitance of carbon-based electrical double-layer capacitors. Nat. Commun. 5:3317. doi: 10.1038/ncomms4317
Jiang, X., Setodoi, S., Fukumoto, S., Imae, I., Komaguchi, K., Yano, J., et al. (2014). An easy one-step electrosynthesis of graphene/polyaniline composites and electrochemical capacitor. Carbon N. Y. 67, 662–672. doi: 10.1016/j.carbon.2013.10.055
Khan, M. D. A., Akhtar, A., and Nabi, S. A. (2015). Investigation of the electrical conductivity and optical property of polyaniline-based nanocomposite and its application as an ethanol vapor sensor. N. J. Chem. 39, 3728–3735. doi: 10.1039/C4NJ02260B
Koysuren, O., and Koysuren, H. N. (2019). Photocatalytic activity of polyaniline/Fe-doped TiO2 composites by in situ polymerization method. J. Macromol. Sci. Part A 56, 267–276. doi: 10.1080/10601325.2019.1565548
Li, H., Wang, J., Chu, Q., Wang, Z., Zhang, F., and Wang, S. (2009). Theoretical and experimental specific capacitance of polyaniline in sulfuric acid. J. Power Sources 190, 578–586. doi: 10.1016/j.jpowsour.2009.01.052
Li, Z., Shen, Y., Li, Y., Zheng, F., and Liu, L. (2018). Doping effects of cerium ion on structure and electrochemical properties of polyaniline. Polym. Int. 67, 121–126. doi: 10.1002/pi.5487
Ma, J., Liu, Y., Hu, Z., and Xu, Z. (2013). Electrochemical synthesis and performance of PANI electrode material for electrochemical capacitor. Ionics 19, 1405–1413. doi: 10.1007/s11581-013-0861-x
Manikandan, R., Raj, C. J., Yu, K. H., and Kim, B. C. (2019). Self-coupled nickel sulfide @ nickel vanadium sulfide nanostructure as a novel high capacity electrode material for supercapattery. Appl. Surf. Sci. 497, 143778. doi: 10.1016/j.apsusc.2019.143778
Martyak, N. M., McAndrew, P., McCaskie, J. E., and Dijon, J. (2002). Electrochemical polymerization of aniline from an oxalic acid medium. Progr. Organic Coatings 45, 23–32. doi: 10.1016/S0300-9440(02)00070-X
Muthulakshmi, B., Kalpana, D., Pitchumani, S., and Renganathan, N. G. (2006). Electrochemical deposition of polypyrrole for symmetric supercapacitors. J. Power Sources 158, 1533–1537. doi: 10.1016/j.jpowsour.2005.10.013
Neupane, S. (2013). Development of a Window Based Program to Control the Analogue Potentiostat in Combination with an ADA Convertor. Kathmandu: Journal of Nepal Chemical Society.
Nithya, V. D., and Arul, N. S. (2016). Progress and development of Fe3O4 electrodes for supercapacitors. J. Mater. Chem. A 4, 10767–10778. doi: 10.1039/C6TA02582J
Numan, A., Ramesh kumar, P., Khalid, M., Ramesh, S., Ramesh, K., Shamsudin, E. M., et al. (2020). Facile sonochemical synthesis of 2D porous Co3O4 nanoflake for supercapattery. J. Alloys Compd. 819:153019. doi: 10.1016/j.jallcom.2019.153019
Padmanathan, N., Shao, H., McNulty, D., O'Dwyer, C., and Razeeb, K. M. (2016). Hierarchical NiO-In2O3 microflower (3D)/ nanorod (1D) hetero-architecture as a high performance supercapacitor electrode with excellent cyclic stability. J. Mater. Chem. A 4, 4820–4830. doi: 10.1039/C5TA10407F
Patil, D. S., Shaikh, J. S., Dalavi, D. S., Karanjkar, M. M., Devan, R. S., Ma, Y. R., et al. (2011). An Mn doped polyaniline electrode for electrochemical supercapacitor. J. Electrochem. Soc. 158:A653. doi: 10.1149/1.3561428
Patil, D. S., Shaikh, J. S., Pawar, S. A., Devan, R. S., Ma, Y. R., Moholkar, A. V., et al. (2012). Investigations on silver/polyaniline electrodes for electrochemical supercapacitors. Phys. Chem. Chem. Phys. 14, 11886–11895. doi: 10.1039/C2CP41757J
Prasankumar, T., Wiston, B. R., Gautam, C. R., Ilangovan, R., and Jose, S. P. (2018). Synthesis and enhanced electrochemical performance of PANI/Fe3O4 nanocomposites as supercapacitor electrode. J. Alloys Compd. 757, 466–475. doi: 10.1016/j.jallcom.2018.05.108
Rantho, M. N., Madito, M. J., and Manyala, N. (2018). Symmetric supercapacitor with supercapattery behavior based on carbonized iron cations adsorbed onto polyaniline. Electrochim. Acta 262, 82–96. doi: 10.1016/j.electacta.2018.01.001
Salanne, M., Rotenberg, B., Naoi, K., Kaneko, K., Taberna, P.-L., Grey, C. P., et al. (2016). Efficient storage mechanisms for building better supercapacitors. Nat. Energy 1:16070. doi: 10.1038/nenergy.2016.70
Salmimies, R., Mannila, M., Kallas, J., and Häkkinen, A. (2011). Acidic dissolution of magnetite: experimental study on the effects of acid concentration and temperature. Clays Clay Miner. 59, 136–146. doi: 10.1346/CCMN.2011.0590203
Sampreeth, T., Al-Maghrabi, M. A., Bahuleyan, B. K., and Ramesan, M. T. (2018). Synthesis, characterization, thermal properties, conductivity and sensor application study of polyaniline/cerium-doped titanium dioxide nanocomposites. J. Mater. Sci. 53, 591–603. doi: 10.1007/s10853-017-1505-8
Sharma, M., and Gaur, A. (2020). Designing of carbon nitride supported ZnCo2O4 hybrid electrode for high-performance energy storage applications. Sci. Rep. 10:2035. doi: 10.1038/s41598-020-58925-4
Silva, C. A. S., Silva, R. L. S. E., Figueiredo, A. T., de Alves, V. N., Silva, C. A. S., Silva, R. L. S., et al. (2020). Magnetic solid-phase microextraction for lead detection in aqueous samples using magnetite nanoparticles. J. Braz. Chem. Soc. 31, 109–115. doi: 10.21577/0103-5053.20190134
Simon, P., and Gogotsi, Y. (2008). Materials for electrochemical capacitors. Nat. Mater. 7, 845–854. doi: 10.1038/nmat2297
Tharani, S., and Vinayagam, S. C. (2015). Synthesis and characterization of cerium oxide doped polyaniline/titanium oxide nanocomposites for supercapacitor applications. Int. J. Inno. Res. Sci. 4, 11213–11222. doi: 10.15680/IJIRSET.2015.0411066
Umare, S. S., Shambharkar, B. H., and Ningthoujam, R. S. (2010). Synthesis and characterization of polyaniline–Fe3O4 nanocomposite: electrical conductivity, magnetic, electrochemical studies. Synth. Met. 160, 1815–1821. doi: 10.1016/j.synthmet.2010.06.015
Wang, H., Zhenyu, G., Suwei, Y., Zixuan, L., and Weiguo, Z. (2017). Design and synthesis of ternary graphene/polyaniline/Co3O4 hierarchical nanocomposites for supercapacitors. Int. J. Electrochem. Sci. 12, 3721–3731. doi: 10.20964/2017.05.66
Xavier, C. S., Paskocimas, C. A., Motta, F. V., da Araújo, V. D., Aragón, M. J., Tirado, J. L., et al. (2014). Microwave-assisted hydrothermal synthesis of magnetite nanoparticles with potential use as anode in lithium ion batteries. Mater. Res. 17, 1065–1070. doi: 10.1590/1516-1439.264714
Yu, L., and Chen, G. Z. (2016). Redox electrode materials for supercapatteries. J. Power Sources 326, 604–612. doi: 10.1016/j.jpowsour.2016.04.095
Yu, L., and Chen, G. Z. (2020). Supercapatteries as high-performance electrochemical energy storage devices. Electrochem. Energy Rev. 3, 271–285. doi: 10.1007/s41918-020-00063-6
Yuan, C., Su, L., Gao, B., and Zhang, X. (2008). Enhanced electrochemical stability and charge storage of MnO2/carbon nanotubes composite modified by polyanilinne coating layer in acidic electrolytes. Electrochim. Acta 53, 7039–7047. doi: 10.1016/j.electacta.2008.05.037
Zeng, G., Chen, Y., Chen, L., Xiong, P., and Wei, M. (2016). Hierarchical cerium oxide derived from metal-organic frameworks for high performance supercapacitor electrodes. Electrochim. Acta 222, 773–780. doi: 10.1016/j.electacta.2016.11.035
Keywords: Ce-doping, magnetite nanocomposites, polyaniline, specific capacity, supercapattery
Citation: Pandey S, Neupane S, Gupta DK, Das AK, Karki N, Singh S, Yadav RJ and Yadav AP (2021) Ce-Doped PANI/Fe3O4 Nanocomposites: Electrode Materials for Supercapattery. Front. Chem. Eng. 3:650301. doi: 10.3389/fceng.2021.650301
Received: 06 January 2021; Accepted: 29 March 2021;
Published: 28 April 2021.
Edited by:
Richard G. A. Wills, University of Southampton, United KingdomReviewed by:
Ramesh Kasi, University of Malaya, MalaysiaLuis Fernando Arenas, University of Southampton, United Kingdom
Copyright © 2021 Pandey, Neupane, Gupta, Das, Karki, Singh, Yadav and Yadav. This is an open-access article distributed under the terms of the Creative Commons Attribution License (CC BY). The use, distribution or reproduction in other forums is permitted, provided the original author(s) and the copyright owner(s) are credited and that the original publication in this journal is cited, in accordance with accepted academic practice. No use, distribution or reproduction is permitted which does not comply with these terms.
*Correspondence: Shova Neupane, c2hvdmFfbiYjeDAwMDQwO3lhaG9vLmNvbQ==; Amar Prasad Yadav, YW1hcjJ5JiN4MDAwNDA7eWFob28uY29t