- 1Postgraduate Program in Materials Science and Engineering (PPGMAT), University of Caxias do Sul, Caxias do Sul, Brazil
- 2Laboratory of Applied Virology, Department of Microbiology, Immunology and Parasitology, Federal University of Santa Catarina, Florianópolis, Brazil
The novel coronavirus designated as SARS-CoV-2 has risen the first pandemic caused by coronavirus and by November 26, 2020 is responsible for more than 1,410 million deaths. This scenario evidences that despite previous pandemics and epidemics in the world’s history, the current worldwide measures to contain and to mitigate viruses’ outbreaks are still disabled and insufficient. Therefore, this perspective reinforces the need for new and practical approaches for antiviral material developments and presents current technologies and its advances in this field of research focusing especially in surface materials since it is one of the most common interaction pathways. Furthermore, the roll that nanotechnology has been playing in the combat of viruses as well as the mechanisms that science has been discovering to inactivate these pathogenic microorganisms is presented. Finally, we suggest introducing new legislation and norms rather more specified on virucidal agents (materials and devices) than bactericidal ones in human environments such as hospitals, nursing homes, buses, and shopping centers to mitigate the current and future virus-based pandemics and epidemics.
Introduction
“Next time, we may not be so lucky.” These were the words of Dr Gro Harlem Brundtland, Director-General of the World Health Organization (WHO), when the first severe acute respiratory syndrome (SARS) global outbreak was contained in July 2003. The virus emerged in China and spread to more than 30 countries (mostly in Southeast Asia), resulting in 812 deaths. By that time, Dr Brundtland said that, although the epidemic was under control, the threat remained and researches into SARS must continue to improve world understanding and ability to handle another future outbreak (World Health Organization, 2003). This predicted situation has dramatically emerged in December 2019 with the rise of the new coronavirus designated as SARS-CoV-2 that led to a novel disease, COVID-19. On March 11, 2020, the WHO officially characterized the global COVID-19 outbreak as a pandemic, and by November 2020, the number of victims worldwide exceeded 1,4 million (World Health Organization, 2020a; World Health Organization, 2020b). This scenario impelled, once again, the world to look for practical and innovative solutions to stop the virus spread since respiratory viruses like SARS-CoV-2 cause a variety of pathologies from lung disorders (from mild upper respiratory tract infections to life-threatening pathologies like pneumonia and acute respiratory distress syndrome) to neurological disorders (lack of smell, taste, and memory) (Abdelrahman et al., 2020; Patel et al., 2020). Thus, despite the international commitment from many technological and scientific organizations in fighting such virus, the obstacles are many, including the fact that viruses in general take a couple of years to reemerge, which may discourage the researches (Stadler et al., 2003).
However, it is imperative to notice that viral respiratory infectious diseases have afflicted humans since ages. Three influenza pandemics were recorded only in the 20th century and the first one, the H1N1 “Spanish flu” pandemic of 1918, is estimated to have infected 50% of the world’s population with mortality between 40 and 50 million (Potter, 2001). In the meantime, the humanity has developed surprising technologies to go to the Moon, to put rovers on Mars, and genetically modified organisms to introduce resistance against plant diseases from insects or viruses or to increase tolerance toward herbicides that have radically changed our world. Unfortunately, despite recent advances in the control of epidemic outbreaks such as Ebola and the smallpox eradication, we have not learned as we should from previous viral epidemics and pandemics to develop a huge enough variety of materials with antiviral chemical activity to eliminate or reduce the viral charge in contact surfaces and environments, which would be of great value to fight against COVID-19 nowadays. The current pandemic has encountered the humanity with few tools to fight as some antiviral fabrics and some inorganic antiviral materials, which it is hard to be understood in terms of the size of scientific community and billionaire budgets for research and technology worldwide. Figure 1 shows the number of published articles for materials with antiviral and antibacterial surfaces in the last 25 years using the search engine “Web of Science–Clarivate Analytics”. One can see that a big difference is apparent. Although the trend for materials with antibacterial surfaces follows an exponential growth as a function of years, the trend for the antiviral surfaces does not follow a straightforward behavior. We will discuss below such behavior with more details. Moreover, there is a factor ∼29 in the last 25 years, where rather more articles related to antibacterial surfaces were published than antiviral surfaces, which means that for each article reporting an antiviral surface material (230 papers), another 29 are reporting antibacterial surface materials (6,642 articles). In another terms, regarding the last 5 years, of all the 4,366 publications of surface materials, the virucidal ones correspond only to 2.8%, and has been barely increased to 4.1% in 2020 alone. We understand that such big difference is due to the public legislation, which is focusing more on bacterial infections than viral ones. Thus, governmental institutions for health, food, and drugs guide the research, public calls, and budgets, which may have reinforced rather more bacterial issues than viral ones.
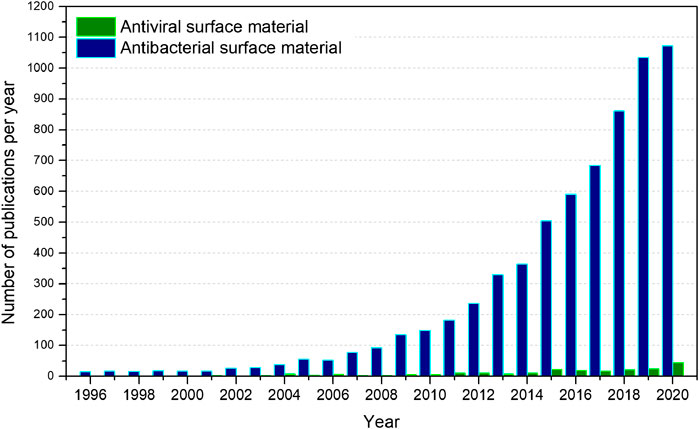
FIGURE 1. Global number of scientific publications over last 25 years obtained from the “Web of Science-Clarivate Analytics” database for “antibacterial surface material; bactericidal surface material; bactericide surface material” (antibacterial surface material) and “antiviral surface material; virucidal surface material; virucide surface material” (antiviral surface material). Search performed on November 26, 2020.
Nanotechnology as a Tool for Antiviral Surface Design
Current advances in nanotechnology have been providing promising alternatives to this area. Nanoparticles of copper (Cu), copper oxides (CuO and Cu2O), silver (Ag), and titanium oxide (TiO2), for example, are being widely studied as biocides. These nanomaterials and thin films have great potential as antiviral agents due to its intrinsic antiviral properties and/or its photodynamic and photothermal capabilities for reactive oxygen species (ROS) generation (Rajendran et al., 2020; Talebian et al., 2020). They have already proved its antiviral effect against several viruses, such as coronavirus 229 E (Warnes et al., 2015), hepatitis B virus (HBV) (Zan et al., 2007; Lu et al., 2008), herpes simplex virus types 1 (HSV-1) and 2 (HSV-2) and with human parainfluenza virus type 3 (HPIV-3) (Gaikwad et al., 2013), human immunodeficiency virus type 1 (HIV-1) (Borkow and Gabbay, 2004; Lara et al., 2010), influenza virus H1N1 (Borkow et al., 2010; Mori et al., 2013; Monmaturapoj et al., 2018), Norovirus (Warnes and Keevil, 2013; Park et al., 2014), and more recently, against the new coronavirus SARS-CoV-2 (Balagna et al., 2020; Behzadinasab et al., 2020). Gold (Au) and zinc oxide (ZnO) nanoparticles have also proved its biocidal activity, as well as silica compounds and carbon-based materials, such as graphene derivatives (Hasan et al., 2020b; Zhou et al., 2020).
These materials have also been used as hard nanomaterials (HNMs). According to Reina et al. (2020), HMNs represent a nonpolymeric organic or inorganic material group that shares a nanostructured hard core and a tunable surface chemistry. This family ranges from noble nanoparticles to glycofullerenes, for example, and their antiviral mechanisms are based in different actions, such as capsid denaturation, mimicking the cell surface, and mechanical disruption. Therefore, they can be applied not only to block the entry of viruses but also as drug delivery (Reina et al., 2020). Still, regarding the novel coronavirus, the inactivation of its distinct binding glycoprotein at the base of its spike, which has been discovered to be responsible to enter the human cells and to replicate, has become a target in the field of materials, especially by surface coatings due to its different possibilities of action using nanomaterials with direct or indirect antiviral activity or with receptor inactivation capability (Sun and Ostrikov, 2020).
As mentioned above, Figure 2A shows the number of published articles for materials with antiviral activity with more details where the time line also exhibits the last virus disease outbreaks and its correspondent mortality rate. One can see that the increase in the number of articles follows a step-by-step growth where each growth takes place after the starting of each outbreak. Moreover, Figure 2B presents a pie chart to highlight the most current materials that have been intensively studied for antiviral materials in the last 5 years. The publications covering these metallic and ceramic materials represent 50% of the data available from 2016 to 2020 since the other half concerns the use of plant extracts and natural compounds (such as chitosan), and also reviews and researches in the medical field regarding drugs and treatments. Silver-based compound/particle appears as the chemical element most used for antiviral activity so far. After that, golden-based, copper-based, and zinc-based compounds/particles follow the sequence. Finally, titanium-based compounds/particles and graphene finish the group of materials with antiviral activity.
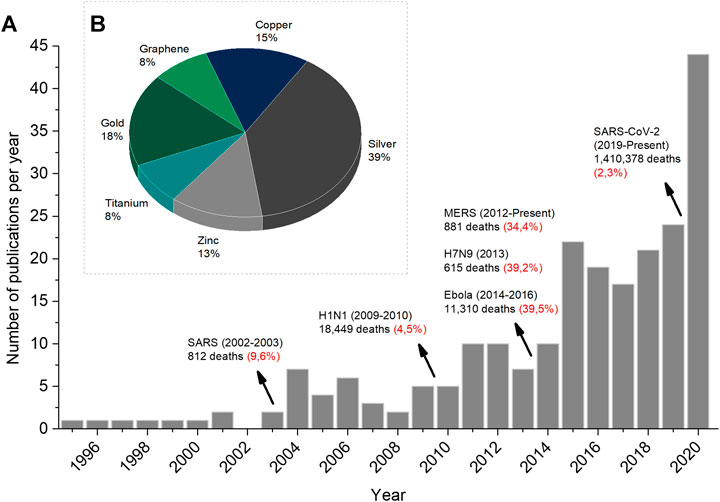
FIGURE 2. (A) Number of publications devoted to antiviral materials where the mortality rate (in red) of major virus disease outbreaks worldwide in the last 25 years is pointed out (B) Main antiviral materials under study in the last 5 years. The search is the same as described in Figure 1 but including the antiviral metallic and ceramic materials’ name. Search performed on November 26, 2020.
Surface and Coating Technologies
Before the scenario previously exposed, the studies in the last years have focused on two pathways: combining the advantages of two or more materials to improve its bactericidal and virucidal activities for hybrid coatings (Hodek et al., 2016) and incorporating biocidal agents in a variety of materials such as cotton fibers, latex, glass, and stainless steel (Borkow and Gabbay, 2004; Huang et al., 2020) since viruses, including the SARS-CoV-2 itself, can survive and remain infectious for days on inanimate surfaces and objects depending on the type of surface and the environmental conditions, (e.g., temperature and humidity) (Hasan et al., 2020b).
Regarding coatings, different technologies have been proposed with the aim of guaranteeing high biocide properties. The sol–gel method is an example of an extensively studied method for the preparation of organic–inorganic hybrid coatings with antimicrobial activity due to its advantages such as easy application to any kind of substrate, high purity, and homogeneity of the resulting coatings and low processing temperatures, and also enables to adjust the mechanical properties of the coating (Marini et al., 2007; Lee et al., 2010). Moongraksathum et al. (2019) developed Ag/TiO2 nanocomposite by the peroxo sol–gel method, which presented antibacterial and antiviral activity greater than 99.99%. The thermal spraying technique has also been reported as a promising method to provide antimicrobial coatings. Noda et al. (2009) presented a thermal sprayed antibacterial coating based on Ag-containing calcium phosphate that presented strong antibacterial activity to methicillin-resistant Staphylococcus aureus (MRSA), a bacteria resistant to many antibiotics, and because of that, may be considered as a promising tool to combat viruses since silver presents antiviral activity. As for cold spray coatings, Sousa and Cote. (2020) recently presented its application in providing SARS-CoV-2 surface inactivation. The antiviral activity of other metal spray techniques has also been reported by Champagne et al. (2019). Moreover, a relative new method for surface treatment is the plasma electrolytic oxidation (PEO), a procedure that among its advantages is the possibility to incorporate into the coating the particles suspended in the electrolyte, such as oxides, which also represents a promising technique to provide antiviral coating surfaces (Pezzato et al., 2019).
To summarize, other methods have also proved themselves efficient in providing antiviral coatings, such as the vapor deposition method (Krumdieck et al., 2019) and the physical absorption (painting) (Behzadinasab et al., 2020). Even more techniques can be found in the review of Imani et al. (2020). A recent coating developed to reduce the longevity of the novel SARS-CoV-2 on solids was reported by Behzadinasab et al. (2020). The authors presented a Cu2O/polyurethane coating that inactivated the virus in 1 h on coated glass and stainless steel retaining its activity after many exposures to virus for 13 days even after immersion in water. Hospital trials have also taken place in studying surface coatings and proved its importance. In 2013, a study carried out in intensive care units (ICUs) of three United States hospitals found out the reduction of more than 50% in the rate of bacterial infection when six items fabricated from copper alloys replaced constant touch surfaces, such as bed rails and call buttons, a promising trend for virucidal tests (Salgado et al., 2013).
As for the impregnation of bactericidal and virucidal particles, it enables not only the production of self-sanitizing surfaces to mitigate fomite transmissions (Hasan et al., 2020b) but also biocidal personal protection equipment (PPE), such as masks and gloves, which is a potential mean to limit the transmission of viruses because the current lack of virucidal activity of such PPE makes them a source of infection when discarded (Huang et al., 2020) developed a rechargeable respiratory mask containing graphene oxide, and Aasi et al. (2020) developed metal decorated single-wall carbon nanotubes that proved themselves promising aspirants for design of antiviral surfaces.
Talebian et al. (2020) also propose two more applications for the nanotechnology field: the development of nano-based bactericidal and virucidal disinfectants for air, surfaces, and to reinforce PPE such as facial respirators, by incorporating nanoparticles with biocidal activity, and the development of sensitive nano-based sensors for viral detection for early diagnosis of viral diseases. This last one tendency is also mentioned by Hasan et al. (2020b) that exposes that material’s surface with tuned electrical and optical properties provided from nanotechnology can be able to detect coronavirus and act as virus sensors.
Another trend regards to photoactive composite materials. The development of photo-driven biocidal materials requires an optimization of its functions because the biocidal effect usually drops under dim light or dark conditions as the ROS generated in light declines with time (Zhou et al., 2020). A wide range of examples concerning the use of phototherapy against viruses is reported by Wiehe et al. (2019), which may be an alternative weapon against SARS-CoV-2.
One more current virucidal strategy is related to the advances in the field of topography-mediated antibacterial surfaces. Hasan et al. (2020a), inspired by insect wing architecture, presented for the first time the antibacterial and antiviral property of an etched aluminum (Al) 6063 alloy surface. The width of individual nanostructures of 23 nm resulted in a surface able to lyse Gram-positive and Gram-negative bacterial cells and also to reduce the amount of viable common respiratory viruses. More details about natural and artificial surfaces with biocidal topography can be found in the review of Wu et al. (2018).
Although different inorganic compounds are showing effective antiviral activity, the mechanisms of the material surface for antiviral activity are not clear and fully understood. It seems to be rather more a trial and error method than systematic studies to identify, for example, in the case of enveloped virus, if the material surface is destroying or blocking the spikes and/or lipid membranes and/or nucleic acids, which may bring rich information about the inactivation mechanism. Thus, new and more detailed experiments are mandatory to understand better the antiviral activity of material surfaces to optimize and tune its antiviral action.
What has been widely presented in the last 2 decades are comparative works that aim to present the advantages of the most currently used materials. Sunada et al. (2012) studied the inactivation of bacteriophages by solid-state copper(I) compounds (Cu2O, Cu2S, and CuI) by direct contact, and Minoshima et al. (2016) proved that, against silver-based compounds, Cu2O shows superior activity against enveloped and non-enveloped viruses, also proposing the direct contact mechanism. However, other mechanisms have been suggested, such as the generation of reactive oxygen species (ROS) which attack the viruses leading to its death, and inactivation by the ions released as well (Borkow and Gabbay, 2005; Miyauchi et al., 2020). As for silver-based compounds, some works present that the antiviral activity occurs due to the silver ions released from the coatings or the nanoparticles themselves (Sun and Ostrikov, 2020) while others present that a physical inhibition of binding between the virus and host cell occurs (Gaikwad et al., 2013; Mori et al., 2013). As for the TiO2, it is capable of inactivating microorganisms by the photocatalytic production of ROS. Therefore, many studies have focused on doping TiO2 with transition metal ions to enhance its antiviral properties (Park et al., 2014; Liu et al., 2015; Monmaturapoj et al., 2018). Thus, in view of the large number of possibilities of combinations and by knowing that the morphology of materials also influences their physical–chemical properties, studies in this area continue to require updates and improvements, especially regarding the durability and shelf life of such materials.
Final Remarks
New materials to control viruses in environments are extremely important to eliminate or reduce viral load, this for antiviral masks, fabrics, and filters for hospital, nursing homes, and vehicles for public transportation. Moreover, the virus inactivation mechanisms should be elucidated to improve the virucidal action of materials and devices. Finally, novel and more accurate public legislation and norms for antiviral materials should be created to eliminate or reduce the viral charge in closed environments and transportation that may slow down the transmission of viruses for current SARS-CoV-2 and future pandemics/epidemics.
Data Availability Statement
The original contributions presented in the study are included in the article/Supplementary Material; further inquiries can be directed to the corresponding author.
Author Contributions
ALS made the literature review and wrote the first version of the article; AFM revised the final text emphasizing the material surface activity; GF revised the biological issues of the article; CAF leaded the work, and gave the idea to explore bactericidal vs. virucidal activities in previous articles and integrate the information.
Conflict of Interest
The authors declare that the research was conducted in the absence of any commercial or financial relationships that could be construed as a potential conflict of interest.
Acknowledgments
The authors would like to thank the Epipolé Research Group-Antiviral Division. This work was financially possible due to the following project and company: Human Resources in Strategic Areas (CNPq #400912/2019-8) and Plasmar Tecnologia Ltd. ALS and CAF are CNPq fellows.
References
Aasi, A., Aghaei, S. M., Moore, M. D., and Panchapakesan, B. (2020). Pt-, Rh-, Ru-, and Cu-Single-Wall carbon nanotubes are exceptional candidates for design of anti-viral surfaces: a theoretical study. Int. J. Mol. Sci. 21, 1–23. doi:10.3390/ijms21155211
Abdelrahman, Z., Li, M., and Wang, X. (2020). Comparative review of SARS-CoV-2, SARS-CoV, MERS-CoV, and influenza A respiratory viruses. Front. Immunol. 11, 552909. doi:10.3389/fimmu.2020.552909
Balagna, C., Perero, S., Percivalle, E., Nepita, E. V., and Ferraris, M. (2020). Virucidal effect against Coronavirus SARS-CoV-2 of a silver nanocluster/silica composite sputtered coating. Open Ceram. 1, 100006. doi:10.1016/j.oceram.2020.100006
Behzadinasab, S., Chin, A., Hosseini, M., Poon, L., and Ducker, W. A. (2020). A surface coating that rapidly inactivates SARS-CoV-2. ACS Appl. Mater. Inter. 12, 34723–34727. doi:10.1021/acsami.0c11425
Borkow, G., and Gabbay, J. (2004). Putting copper into action: copper-impregnated products with potent biocidal activities. FASEB J. 18, 1728–1730. doi:10.1096/fj.04-2029fje
Borkow, G., and Gabbay, J. (2005). Copper as a biocidal tool. Curr. Med. Chem. 12, 2163–2175. doi:10.2174/0929867054637617
Borkow, G., Zhou, S. S., Page, T., and Gabbay, J. (2010). A novel anti-influenza copper oxide containing respiratory face mask. PLoS One 5, e11295. doi:10.1371/journal.pone.0011295
Champagne, V., Sundberg, K., and Helfritch, D. (2019). Kinetically deposited copper antimicrobial surfaces. Coatings 9, 1–9. doi:10.3390/coatings9040223
Gaikwad, S., Ingle, A., Gade, A., Rai, M., Falanga, A., Incoronato, N., et al. (2013). Antiviral activity of mycosynthesized silver nanoparticles against herpes simplex virus and human parainfluenza virus type 3. Int. J. Nanomedicine 8, 4303–4314. doi:10.2147/IJN.S50070
Hasan, J., Xu, Y., Yarlagadda, T., Schuetz, M., Spann, K., and Yarlagadda, P. K. (2020a). Antiviral and antibacterial nanostructured surfaces with excellent mechanical properties for hospital applications. ACS Biomater. Sci. Eng. 6, 3608–3618. doi:10.1021/acsbiomaterials.0c00348
Hasan, M. A., Esther, A. C. M., Dey, A., and Mukhopadhyay, A. K. (2020b). A review on coronavirus survivability on material’s surfaces: present research scenarios, technologies and future directions. Surf. Eng. 2020, 1226–1239. doi:10.1080/02670844.2020.1833277
Hodek, J., Zajícová, V., Lovětinská-Šlamborová, I., Stibor, I., Müllerová, J., and Weber, J. (2016). Protective hybrid coating containing silver, copper and zinc cations effective against human immunodeficiency virus and other enveloped viruses. BMC Microbiol. 16 (Suppl. 1), 56–12. doi:10.1186/s12866-016-0675-x
Huang, H., Fan, C., Li, M., Nie, H. L., Wang, F. B., Wang, H., et al. (2020). COVID-19: a call for physical scientists and engineers. ACS Nano. 14(4), 3747–3754. doi:10.1021/acsnano.0c02618
Imani, S. M., Ladouceur, L., Marshall, T., Maclachlan, R., Soleymani, L., and Didar, T. F. (2020). Antimicrobial nanomaterials and coatings: current mechanisms and future perspectives to control the spread of viruses including SARS-CoV-2. ACS Nano. 14, 12341–12369. doi:10.1021/acsnano.0c05937
Krumdieck, S. P., Boichot, R., Gorthy, R., Land, J. G., Lay, S., Gardecka, A. J., et al. (2019). Nanostructured TiO2 anatase-rutile-carbon solid coating with visible light antimicrobial activity. Sci. Rep. 9, 1883–1911. doi:10.1038/s41598-018-38291-y
Lara, H. H., Ayala-Nuñez, N. V., Ixtepan-Turrent, L., and Rodriguez-Padilla, C. (2010). Mode of antiviral action of silver nanoparticles against HIV-1. J. Nanobiotechnology 8, 1–10. doi:10.1186/1477-3155-8-1
Lee, S. M., Lee, B. S., Byun, T. G., and Song, K. C. (2010). Preparation and antibacterial activity of silver-doped organic-inorganic hybrid coatings on glass substrates. Colloids Surf. A Physicochem. Eng. Asp. 355, 167–171. doi:10.1016/j.colsurfa.2009.12.010
Liu, M., Sunada, K., Hashimoto, K., and Miyauchi, M. (2015). Visible-light sensitive Cu(II)-TiO2 with sustained anti-viral activity for efficient indoor environmental remediation. J. Mater. Chem. A. 3, 17312–17319. doi:10.1039/c5ta03756e
Lu, L., Sun, R. W., Chen, R., Hui, C., Ho, C., Luk, J. M., et al. (2008). Silver nanoparticles inhibit hepatitis B virus replication. Antivir. Ther. 13, 253–262.
Marini, M., De Niederhausern, S., Iseppi, R., Bondi, M., Sabia, C., Toselli, M., et al. (2007). Antibacterial activity of plastics coated with silver-doped organic-inorganic hybrid coatings prepared by sol-gel processes. Biomacromolecules 8, 1246–1254. doi:10.1021/bm060721b
Minoshima, M., Lu, Y., Kimura, T., Nakano, R., Ishiguro, H., Kubota, Y., et al. (2016). Comparison of the antiviral effect of solid-state copper and silver compounds. J. Hazard. Mater. 312, 1–7. doi:10.1016/j.jhazmat.2016.03.023
Miyauchi, M., Sunada, K., and Hashimoto, K. (2020). Antiviral effect of visible light-sensitive CuxO/TiO2 photocatalyst. Catalysts 10, 1–19. doi:10.3390/catal10091093
Monmaturapoj, N., Sri-on, A., Klinsukhon, W., Boonnak, K., and Prahsarn, C. (2018). Antiviral activity of multifunctional composite based on TiO2-modified hydroxyapatite. Mater. Sci. Eng. C 92, 96–102. doi:10.1016/j.msec.2018.06.045
Moongraksathum, B., Chien, M.-Y., and Chen, Y.-W. (2019). Antiviral and antibacterial effects of silver-doped TiO 2 prepared by the peroxo sol-gel method. J. Nanosci. Nanotechnol. 19, 7356–7362. doi:10.1166/jnn.2019.16615
Mori, Y., Ono, T., Miyahira, Y., Nguyen, V. Q., Matsui, T., and Ishihara, M. (2013). Antiviral activity of silver nanoparticle/chitosan composites against H1N1 influenza A virus. Nanoscale Res. Lett. 8, 93. doi:10.1186/1556-276x-8-93
Noda, I., Miyaji, F., Ando, Y., Miyamoto, H., Shimazaki, T., Yonekura, Y., et al. (2009). Development of novel thermal sprayed antibacterial coating and evaluation of release properties of silver ions. J. Biomed. Mater. Res. Part. B Appl. Biomater. 89, 456–465. doi:10.1002/jbm.b.31235
Park, G. W., Cho, M., Cates, E. L., Lee, D., Oh, B. T., Vinjé, J., et al. (2014). Fluorinated TiO₂ as an ambient light-activated virucidal surface coating material for the control of human norovirus. J. Photochem. Photobiol. B, Biol. 140, 315–320. doi:10.1016/j.jphotobiol.2014.08.009
Patel, A., Charani, E., Ariyanayagam, D., Abdulaal, A., Denny, S. J., Mughal, N., et al. (2020). New-onset anosmia and ageusia in adult patients diagnosed with SARS-CoV-2 infection. Clin. Microbiol. Infect. 26, 1236–1241. doi:10.1016/j.cmi.2020.05.026
Pezzato, L., Cerchier, P., Brunelli, K., Bartolozzi, A., Bertani, R., and Dabalà, M. (2019). Plasma electrolytic oxidation coatings with fungicidal properties. Surf. Eng. 35, 325–333. doi:10.1080/02670844.2018.1441659
Potter, C. W. (2001). A history of influenza. J. Appl. Microbiol. 91, 572–579. doi:10.1046/j.1365-2672.2001.01492.x
Rajendran, S., Mukherjee, A., Nguyen, T. A., Godugu, C., and Shukla, R. K. (2020). in Nanotoxicity: prevention and antibacterial applications of nanomaterials. Editors S. Rajendran, A. Mukherjee, T. A. Nguyen, C. Godugu, and R. K. B. T.-N. Shukla. 1st ed. (Amsterdam, Netherlands: Elsevier) doi:10.1016/C2018-0-05517-6
Reina, G., Peng, S., Jacquemin, L., Andrade, A. F., and Bianco, A. (2020). Hard nanomaterials in time of viral pandemics. ACS Nano. 14, 9364–9388. doi:10.1021/acsnano.0c04117
Salgado, C. D., Sepkowitz, K. A., John, J. F., Cantey, J. R., Attaway, H. H., Freeman, K. D., et al. (2013). Copper surfaces reduce the rate of healthcare-acquired infections in the intensive care unit. Infect. Control. Hosp. Epidemiol. 34, 479–486. doi:10.1086/670207
Sousa, B. C., and Cote, D. L. (2020). Antimicrobial copper cold spray coatings and SARS-CoV-2 surface inactivation. MRS Adv. 5(56), 2873–2880. doi:10.1557/adv.2020.366
Stadler, K., Masignani, V., Eickmann, M., Becker, S., Abrignani, S., Klenk, H. D., et al. (2003). SARS–beginning to understand a new virus. Nat. Rev. Microbiol. 1, 209–218. doi:10.1038/nrmicro775
Sun, Z., and Ostrikov, K. K. (2020). Future antiviral surfaces: lessons from COVID-19 pandemic. Sustain. Mater. Technol. 25, e00203. doi:10.1016/j.susmat.2020.e00203
Sunada, K., Minoshima, M., and Hashimoto, K. (2012). Highly efficient antiviral and antibacterial activities of solid-state cuprous compounds. J. Hazard. Mater. 235-236, 265–270. doi:10.1016/j.jhazmat.2012.07.052
Talebian, S., Wallace, G. G., Schroeder, A., Stellacci, F., and Conde, J. (2020). Nanotechnology-based disinfectants and sensors for SARS-CoV-2. Nat. Nanotechnol. 15, 618–621. doi:10.1038/s41565-020-0751-0
Warnes, S. L., and Keevil, C. W. (2013). Inactivation of norovirus on dry copper alloy surfaces. PLoS One 8, e75017. doi:10.1371/journal.pone.0075017
Warnes, S. L., Little, Z. R., and Keevil, C. W. (2015). Human coronavirus 229E remains infectious on common touch surface materials. MBio 6, e01697–15. doi:10.1128/mBio.01697-15
Wiehe, A., O’Brien, J. M., and Senge, M. O. (2019). Trends and targets in antiviral phototherapy. Photochem. Photobiol. Sci. 18, 2565–2612. doi:10.1039/c9pp00211a
World Health Organization (2003). SARS outbreak contained worldwide. Available at: https://www.who.int/mediacentre/news/releases/2003/pr56/en/ (Accessed October 7, 2020).
World Health Organization (2020a). Coronavirus disease 2019 (COVID-19). Situation report 209. Available at: https://www.who.int/emergencies/diseases/novel-coronavirus-2019/situation-reports/ (Accessed September 7, 2020).
World Health Organization (2020b). WHO coronavirus disease (COVID-19) dashboard. Available at: https://covid19.who.int/ (Accessed October 9, 2020).
Wu, S., Zhang, B., Liu, Y., Suo, X., and Li, H. (2018). Influence of surface topography on bacterial adhesion: a review (review). Biointerphases 13, 060801. doi:10.1116/1.5054057
Zan, L., Fa, W., Peng, T., and Gong, Z. K. (2007). Photocatalysis effect of nanometer TiO2 and TiO2-coated ceramic plate on Hepatitis B virus. J. Photochem. Photobiol. B. Biol. 86, 165–169. doi:10.1016/j.jphotobiol.2006.09.002
Keywords: antimicrobial materials, virus inactivation, surface chemical activity, health management nanotechnology, antiviral legislation
Citation: Schio AL, Michels AF, Fongaro G and Figueroa CA (2021) Trends in the Antiviral Chemical Activity of Material Surfaces Associated With the SARS-CoV-2 Outbreak. Front. Chem. Eng. 3:636075. doi: 10.3389/fceng.2021.636075
Received: 08 December 2020; Accepted: 25 January 2021;
Published: 05 March 2021.
Edited by:
Aditya Kumar, Indian Institute of Technology Dhanbad, IndiaReviewed by:
Luca Pezzato, University of Padua, ItalyAdriana Ispas, Technische Universität Ilmenau, Germany
Copyright © 2021 Schio, Michels, Fongaro and Figueroa. This is an open-access article distributed under the terms of the Creative Commons Attribution License (CC BY). The use, distribution or reproduction in other forums is permitted, provided the original author(s) and the copyright owner(s) are credited and that the original publication in this journal is cited, in accordance with accepted academic practice. No use, distribution or reproduction is permitted which does not comply with these terms.
*Correspondence: Carlos Alejandro Figueroa, Y2FmaWd1ZXJAdWNzLmJy; Gislaine Fongaro, Z2lzbGFpbmUuZm9uZ2Fyb0B1ZnNjLmJy