- 1Beijing National Laboratory for Molecular Sciences, CAS Key Laboratory of Colloid and Interface and Thermodynamics, CAS Research/Education Center for Excellence in Molecular Sciences, Institute of Chemistry, Chinese Academy of Sciences, Beijing, China
- 2School of Chemistry and Chemical Engineering, University of Chinese Academy of Sciences, Beijing, China
- 3Physical Science Laboratory, Huairou National Comprehensive Science Center, Beijing, China
The selective transformation of chitin into various renewable N-containing chemicals and medicines has attracted increasing attention. However, the N-acetyl groups in chitin construct strong hydrogen bond networks, which restricts its depolymerization and transformation. The selective conversion of robust chitin commonly requires considerable base catalysts to remove the N-acetyl group as a byproduct in advance, which is non-compliance with the principle of atomic economy. Herein, for the first time we demonstrate a novel approach to achieve the selective utilization of the N-acetyl group in chitin for transamidation of chitin with amines. A series of amine derivatives, mainly including aliphatic amine, cyclic amine and functionalized aromatic amine, could be selectively converted into the corresponding amide products frequently found in pharmaceuticals. Furthermore, the solid residue after removing the acetyl group (denoted as De-chitin) with the sufficient exposure of -NH2 groups as a solid base catalyst shows excellent performance in the aldol condensation reaction of furfural and acetone to produce fuel precursors. Our process provides a strategy that exploiting every functional group adequately in substrates to obtain value-added chemicals.
Introduction
Chitin, the second most abundant biopolymer on the earth after cellulose, consists of N-acetylglucosamine units with ß-1,4-glycosidic linkages. (Yabushita et al., 2015; Yan and Chen et al., 2015). Chitin is considered as the most promising biomass sources for the production of renewable N-containing chemicals and materials due to its high nitrogen content of around 7% by weight. (Omari et al., 2012; Chen et al., 2014; Chen et al., 2017; Kobayashi et al., 2017, 2019; Osada et al., 2019; Pham et al., 2019; Dai et al., 2020). In spite of the enormous economic and environmental interests, the existence of acetyl groups is the main challenge for the exploitation of chitin biomass, which seriously hampers its transformation into fuels and fine chemicals (Fang and Fan et al., 2019). It has been reported that deacetylation is commonly inevitable for the valorization of chitin. However, deacetylation is notorious due to the use of plenty of concentrated, corrosive basic solutions (Chen et al., 2017). Moreover, acetyl groups in chitin are removed as a byproduct, which results in low atomic utilization and is contrary to the theme of Green Chemistry.
Amides are enormously important building blocks in organic synthesis, and they serve as precursors for many value-added compounds, mainly including agrochemicals, pharmaceuticals, organic materials and polymers (Pattabiraman and Bode et al., 2011; Lundberg et al., 2014; Kniss, 2017). Hitherto, several synthetic measures have been achieved. Generally, the preparation of amide involves hydroamination of alkynes (Uenoyama et al., 2005; Valeur and Bradley et al., 2009), hydration of nitriles (Goto et al.,2008; Raman et al., 2009; Williams et al., 2011) and the coupling of carboxylic acids, aldehydes and alcohols with amines (Srinivas and Das, 2003; Hosseini-Sarvari and Sharghi et al., 2006; Gunanathan and Milstein, 2007, Milstein, 2011; Nordstrom et al., 2008; Arnold et al., 2008; Zweifel et al., 2009; Watson et al., 2009; Soule et al., 2011; Lundberg et al., 2012; Gosh et al., 2012; Yamaguchi et al., 2012). Additionally, transamidation is a convenient and straightforward method for exchanging the constituents of two different amide groups and some significant progress has been achieved in recent years (Eldred et al., 2003; Eldred et al., 2008; Nguyen et al., 2012, Allen et al., 2012; Zhang et al., 2012; Nageswara et al., 2014; Becerra-Figueroa et al. 2014; Garg et al. 2017; Yin et al., 2019; Ghosh et al., 2019). Chitin possesses enormous N-acetyl groups and can be recognized as a kind of renewable amide compounds. Selective deacetylation of N-acetyl groups with additional amine source through transamidation not only generated high-value amide products, but benefited to its further valorization. To the best of our knowledge, transamidation reaction regarding renewable chitin as amide source has never been reported.
Aldol condensation is known as an important C-C bond forming reaction and a cornerstone of synthetic chemistry (Yutthalekha et al., 2017; Xu et al., 2017; Ngo et al., 2019). Generally, aldol reaction proceeds in the presence of acid, base or acid-base bifunctional catalysts. Among those catalysts, base catalysts gave remarkable catalytic performance owing to its strong ability to abstract a-protons and active substrate (Ngo et al., 2019). Homogeneous base catalysts, such as NaOH and KOH, were widely employed in the aldol reaction, but showed some disadvantages, mainly involving equipment corrosion, non-recyclability and complex separation (West et al., 2008; Fakhfakh et al., 2008; Xing et al., 2010; Ramirez-Barria et al., 2016; Gu et al., 2017). To overcome these drawbacks, heterogeneous base catalysts (MgAl-hydrotalcite and MgO et al.) were considered as suitable alternatives, while the challenge of catalyst stability existed in the presence of water generated in the process of aldol condensation (Yang et al., 2013; Shen et al., 2016; Bing et al., 2017; Ngo et al., 2018). Therefore, exploring a solid base catalyst with outstanding water-resistance to enhance aldol reaction is very urgent. Chitosan is the partially deacetylated form of chitin. Chitosan with considerable -NH2 groups, as a promising renewable solid base catalyst, emerged to promote the aldol condensation reaction, due to its water resistance and recyclability (Sakthivel and Dhakshinamoorthy et al., 2017; Rani et al., 2018; Meninno, 2020; Anbu et al., 2020).
We report here a novel catalytic system where the deacetylation of C2-acetamido groups to C2-amido groups selectively occurred through a transamidation process with amines. The amine sources mainly include aliphatic amine, cyclic amine and functionalized aromatic amine, generating the corresponding amide as the desired product with excellent yield. FT-IR spectra and XRD pattern demonstrated that the transamidation reaction proceeded mainly on the surface of chitin. Additionally, the solid residue after transamidation reaction (De-chitin), as a solid base catalyst with exposure of lots of -NH2 groups, exhibited outstanding catalytic activity on aldol condensation of furfural with acetone to produce fuel precursor. Our work offers a strategy to sufficiently use every functional groups in the substrate for the production of value-added chemicals from an atomic economy perspective.
Experimental section
Chemicals and Materials
Furfural (98%), acetone (99.9%), N, N-dimethylformamide (98%), chitin and chitosan were purchased from TCI. Aniline (99%), propylamine (99%), butylamine (99%), cyclopentamine (99%), cyclohexylamine (99%), benzylamine (99%), p-toluidine (99%), p-anisidine (99%), p-chloroaniline (98%), p-bromoaniline (99%) were purchased from Aladdin. NaOH (99%), Fe(OAc)2 (95%), Co(OAc)2 (98%), Ni(OAc)2 (97%), Cu(OAc)2 (99%), AlCl3 (99%), CoCl2 (97%), CuCl2 (98%) were purchased from Alfa Aesar.
Catalytic Reactions
The transamidation reaction was performed in a 50 ml flask at ambient pressure. Typically, chitin (0.10 g), catalyst (0.03 g), amine and DMF (3 ml) were placed into the flask. The reactor was purged with N2 three times. The reaction was then performed at 140°C under magnetic stirring for 12 h. The liquid solution was separated from the solid residual by centrifugation and analyzed qualitatively by GC-MS (Agilent 5977A) and quantitatively by GC equipped with a flame ionization detector (FID, Agilent 4890D) using dodecane as the internal standard. The experimental section of transamidation reaction involving other molecules is the same as the above process. Additionally, the solid residue (De-chitin) was collected, washed with ethanol and then dried in an oven for the next step.
The yield of amide product was calculated by using the equation: amide yield = (the actual moles of amide)/(the theoretical moles of amide). The theoretical moles of amide were obtained according to the degree of acetylation (DA) of chitin which was calculated based on elemental analysis (EA) (Chen et al., 2014).
C/N means the ratio of carbon to nitrogen (w/w).
The aldol condensation of furfural with acetone was conducted in a 10 ml Teflon-lined stainless-steel autoclave. Furfural (0.48 g, 5 mmol), acetone (1.16 g, 20 mmol) and De-chitin catalyst (0.10 g) were transferred into the autoclave. The reactor was charged to 1 MPa N2 pressure. The reaction was then performed at 140°C under magnetic stirring for 12 h. After the aldol condensation reaction, the autoclave was quenched in an ice-water bath to room temperature. The liquid solution was separated from the solid residual by centrifugation and analyzed qualitatively by GC-MS (Agilent, 5977A) and quantitatively by GC equipped with a flame ionization detector (FID, Agilent, 4890D) using dodecane as the internal standard. Conversion of furfural and yield of products were calculated by using the equation:
Characterization
Powder X-ray diffraction (XRD) patterns were obtained by Rigaku D/max-2500X-ray diffractometer (excitation source: Cu Kα radiation, λ = 0.15406 nm; tube voltage: 40 kV; tube current: 200 mA).
FT-IR spectra of commercially available chitin, chitosan and De-chitin were recorded with a TENSOR 27 spectrometer. The samples were blended with KBr for IR characterization.
Results and Discussion
Initially, aniline was employed as an amine source to investigate the transamidation reaction between chitin and aniline, and various catalysts were screened for the production of acetanilid at 140°C (Figure 1). The degree of acetylation (DA) in chitin is 95.4% based on the elemental analysis and the theoretical moles of acetanilid were calculated to 58.5 mg when the amount of chitin substrate is 100 mg (Supplementary Table S1) (Chen et al., 2014). The transamidation reaction did not occur without catalyst and homogeneous base catalyst (NaOH) showed poor performance with only 3.5% yield of acetanilid as the desired product. Lewis acid catalysts are known to be effective for this reaction. We evaluated lots of Lewis acid catalysts, mainly including Ni(OAc)2, AlCl3, Co(OAc)2, CoCl2, Fe(OAc)2, CuCl2 and Cu(OAc)2, and found that all those catalysts could produce target molecular. Among them, Cu(OAc)2 provided the best performance with 87.2% yield of acetanilid after 12 h at 140°C. Those results obviously indicated that metal core played a significant role in the transamidation reaction and the excellent catalytic activity of Cu catalyst may be attributed to the proper combination between acetyl groups in chitin and it.
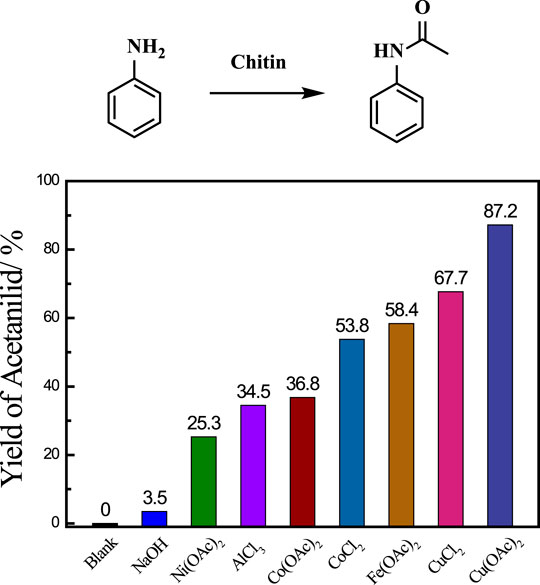
FiGURE 1. Catalysts screening for transamidation reaction. Reaction conditions: chitin 0.1 g, aniline 0.1 ml, DMF 2.9 ml, catalyst 30 mg, 140 C, 12 h.
With this promising result, the factors of reaction were further optimized, including solvents, reaction time, reaction temperature and the amount of Cu catalyst. The use of solvents involving tetrahydrofuran, acetonitrile, p-xylene, isopropanol and cyclohexane, did not provide the desired product, which demonstrates the critical role of DMF in promoting transamidation reaction (Figure 2A). Figure 2B illustrated the influence of reaction time on the transamidation reaction and the yield of acetanilide increased before 12 h. Prolonging the reaction time, the yield of the target product showed little change. In addition, the influence of reaction temperature on the yield of acetanilid was also investigated (Figure 2C) and the results showed that the reaction temperature played an important role in the transamidation reaction. The most suitable reaction temperature is 140°C and decreasing the reaction temperature proved to be unfavorable for the transamidation reaction. Moreover, the effect of the amounts of Cu catalyst on the yield of acetanilid was also studied. The yield of the target product increased continuously with the increasing amount of Cu(OAc)2 at the beginning and reached a plateau when the amount of Cu(OAc)2 was above 30 mg, revealing that Cu(OAc)2 catalyzed the reaction during the reaction process (Figure 2D). A moderate yield of 55.3% was still achieved when the amount of Cu catalyst was decreased to 15 mg.
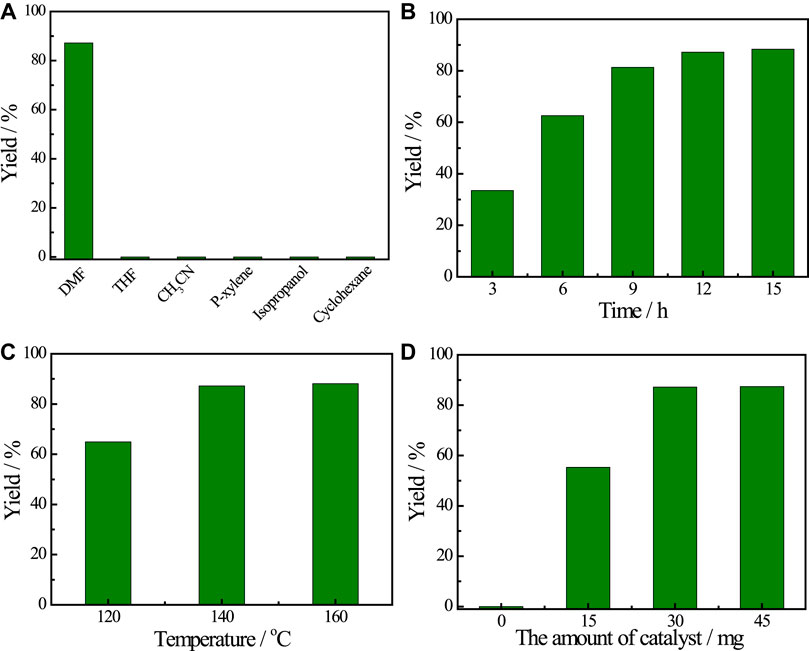
FIGURE 2. The optimization of reaction factors. (A) solvents screening. (B) reaction time. (C) reaction temperature. (D) the amount of catalyst. Reaction conditions: chitin 0.1 g, aniline 0.1 ml, DMF 2.9 ml, catalyst 30 mg, 140°C, 12 h.
Based on the previous literature report (Ma and Gong, 2018) and our results, a reasonable reaction mechanism has been proposed (Scheme 1). Initially, the carbonyl group of chitin is activated by Cu(OAc)2 catalyst through coordination. The activated chitin then undergoes nucleophilic attack by amine, which results in the formation of tetrahedral intermediate 1) With a proton transfer, the sterically congested intermediate 1 breaks down to generate intermediate 2) Finally, the target molecular, corresponding amide, is produced to finish the catalytic cycle.
Infrared spectroscopy is an important tool to acquire information on surface functional groups and all samples were analyzed by FT-IR (Supplementary Figure S1). The bands ranging from 2,800 to 3,500 cm−1 are assigned to the vibrations of -CH, -NH and -OH groups, and the peaks between 1500 and 1670 cm−1 represent amide bands. Commercial available chitosan and chitin gave remarkably different FT-IR spectra from 1,000−4,000 cm−1. Three obvious peaks at 1,115, 1,550, and 3,250 cm−1 in the chitin spectrum are absent in that of chitosan. Most importantly, the FT-IR spectrum of De-chitin highly resembles that of chitosan, indicating effective deacetylation of N-acetyl groups in the catalytic system.
The XRD patterns of the three samples are depicted in Supplementary Figure S2 to investigate their bulk phase structure. Chitin exhibits characteristic diffraction peaks centered at 9.6 and 19.6 of diffraction angle 2θ, representing the (020) and (110) planes of crystalline chitin, respectively. While chitosan has no obvious diffraction peaks, indicating its amorphous structure. Notably, the XRD pattern of De-chitin is similar with that of commercially available chitin. Namely, the original chitin structure is maintained during the Cu(OAc)2-catalyzed process. FT-IR and XRD characterizations indicated the deacetylation of C2-acetamido groups to C2-amido groups selectively occurred on the surface of chitin.
With the optimized conditions in hand, the amine scope of the Cu(OAc)2-catalyzed transamidation reaction was examined (Table 1). The results revealed broad applicability of this reaction to various amines, mainly including aliphatic amines, cyclic amines and functionalized aromatic amines, generating the corresponding amide products. Particularly noteworthy is that aliphatic amine, such as propylamine and butylamine, can undergo transamidation with chitin even without catalyst and the yields of corresponding amides are up to 93.5 and 92.1%, respectively. The promising result is mostly ascribed to the stronger nucleophilic capability of aliphatic amine than others. When cyclopentamine and cyclohexylamine were employed as amine sources, excellent yields of corresponding amide products were achieved (85.2 and 83.9%, respectively). For functionalized aromatic amines, different functional groups on the benzene ring were compatible with the reaction, including methyl, methoxy, bromide and chlorine groups. The reaction proceeded well with p-methyl or p-methoxy aniline as substrate. However, the chlorine and bromine groups were found to have a negative influence on the transamidation reaction, giving the corresponding amines 79.5 and 77.8% yield, respectively. Furthermore, to investigate the effect of steric hindrance on the reaction, o-toluidine and 2,6-dimethyaniline were employed to conduct the transamidation reaction. Notably, the yield of corresponding amide decreased to 83.7 and 51.4% with o-toluidine and 2,6-dimethyaniline as amine source as compared to that yield (87.2%) with aniline as amine source. The result demonstrated that the reaction was influenced by the steric effect of substrate, apart from nucleophilicity of the amine source.
After reaction, the solid residue (denoted as De-chitin) was separated by centrifugation, washed with ethanol and dried in an oven for the next step utilization. The initial Cu content is 9.54% based on the weight of chitin in catalytic system. We tested the Cu content in solid residue after reaction and found that only 0.07% Cu metal exist in the deacetylated chitin after centrifugation (Supplementary Table S2). The aldol condensation of furfural with acetone was employed as a model reaction to evaluate the catalytic performance of De-chitin. Figure 3 illustrated the influence of reaction time on the aldol reaction. The conversion of furfural increased continuously with the prolongation of reaction time in the beginning and reached a plateau at 12 h. De-chitin catalyst showed remarkable catalytic performance and furfural was completely converted over De-chitin catalyst after 12 h at 140°C, with 74.3% C8 yield and 7.9% C13 yield. Figure 4 revealed that the conversion of furfural was 12.1% even without catalyst under solvent-free condition. However, the conversion of furfural and yields of condensed products just increased slightly over chitin catalyst, mainly due to the existence of C2-acetamido groups and inadequate exposure of -NH2 groups. Notably, De-chitin catalyst exhibited excellent catalytic performance for aldol condensation reaction, with the furfural conversion up to 58.3%, which is much higher than that with chitin as catalyst. The deacetylation of C2-acetamido groups to C2-amido groups, namely the adequate exposure of surface -NH2 groups in the De-chitin sample, is responsible for the enhanced reaction activity.

FIGURE 3. Time conversion plot for the reaction of furfural with acetone over De-chitin as solid base catalyst. Reaction conditions: furfural 0.48 g, acetone 1.16 g, catalyst 0.1 g, 140°C.
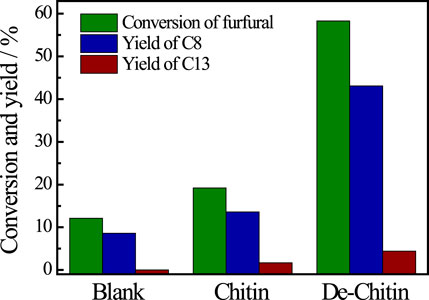
FIGURE 4. Aldol reaction catalyzed by various catalysts. Reaction conditions: furfural 0.48 g, acetone 1.16 g, catalyst 0.1g, 140°C, 4 h.
The recyclability of the De-chitin catalyst for aldol condensation of furfural with acetone was investigated by reusing the catalyst in consecutive catalytic run. The catalyst was separated by simple filtration, washed with ethanol and oven dried for five hours. It can be seen from Figure 5 that no obvious change was observed in the catalytic activity and product selectivity even after three runs. The recyclability test reveals the potential application of De-chitin as a heterogeneous catalyst for aldol condensation.
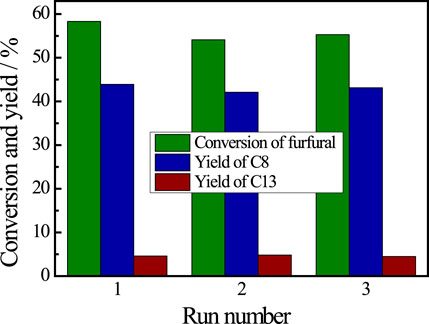
FIGURE 5. Recyclability test of De-chitin for aldol condensation. Reaction conditions: furfural 0.48 g, acetone 1.16 g, De-chitin 0.1 g, 140°C, 4 h.
Conclusion
In summary, N-acetyl groups in chitin were removed with additional amine sources through transamidation to generate corresponding amide products. The amine sources mainly involved aliphatic amines, cyclic amines and functionalized aromatic amines and the yields of corresponding amides commonly exceed 75%. Notably, aliphatic amines, such as propylamine and butylamine, can undergo transamidation with chitin even without catalyst and the yields of corresponding amides are up to 93.5 and 92.1%, respectively. The promising result is mostly ascribed to the stronger nucleophilic capability of aliphatic amine than others. Additionally, FT-IR and XRD results demonstrated that deacetylation reaction only occurred on the surface of chitin. Furthermore, the solid residue after transamidation reaction (De-chitin) was applied as a heterogeneous base catalyst for aldol condensation of furfural with acetone under mild reaction conditions and showed excellent catalytic activity. The catalyst was recovered and reused for three runs without any drop in its activity and selectivity. This work provides a measure to sufficiently use functional groups in the substrate to produce high-value chemicals from an atomic economy perspective.
Data Availability Statement
The original contributions presented in the study are included in the article/Supplementary Material, further inquiries can be directed to the corresponding author.
Author Contributions
YX, HL, and BH conceived the projects. YX carried out the experiments, analyzed data in the assist of XS and wrote the manuscript in the guidance of HL and BH.
Funding
The work was supported financially by National Natural Science Foundation of China (21871277) and Beijing Municipal Science & Technology Commission (Z191100007219009).
Conflict of Interest
The authors declare that the research was conducted in the absence of any commercial or financial relationships that could be construed as a potential conflict of interest.
Supplementary Material
The Supplementary Material for this article can be found online at: https://www.frontiersin.org/articles/10.3389/fceng.2020.634983/full#supplementary-material.
References
Al-Mourabit, C. L., Atkinson, B. N., and Williams, J. M. (2012). Transamidation of primary amides with amines using hydroxylamine hydrochloride as an inorganic catalyst. Angew Chem. Int. Ed. Engl. 51, 1383–1386. doi:10.1002/anie.201107348
Allen, C. L., and Williams, J. M. (2011). Metal-catalysed approaches to amide bond formation. Chem. Soc. Rev. 40, 3405–3415. doi:10.1039/c0cs00196a
Anbu, N., Maheswari, R., Elamathi, V., Varalakshmi, P., and Dhakshinamoorthy, A. (2020). Chitosan as a biodegradable heterogeneous catalyst for Knoevenagel condensation between benzaldehydes and cyanoacetamide. Catal. Commun. 138, 105954. doi:10.1016/j.catcom.2020.105954
Arnold, K., Davies, B., Hérault, D., and Whiting, A. (2008). Asymmetric direct amide synthesis by kinetic amine resolution: a chiral bifunctional aminoboronic acid catalyzed reaction between a racemic amine and an achiral carboxylic acid. Angew Chem. Int. Ed. Engl. 47, 2673–2676. doi:10.1002/anie.200705643
Becerra-Figueroa, L., Ojeda-Porras, A., and Gamba-Sánchez, D. (2014). Transamidation of carboxamides catalyzed by Fe(III) and water. J. Org. Chem. 79, 4544–4552. doi:10.1021/jo500562w
Bing, W., Zheng, L., He, S., Rao, D., Xu, M., Zheng, L., et al. (2017). Insights on active sites of CaAl-hydrotalcite as a high-performance solid base catalyst toward aldol condensation. ACS Catal. 8, 656–664. doi:10.1021/acscatal.7b03022
Chen, X., Chew, S. L., Kerton, F. M., and Yan, N. (2014). Direct conversion of chitin into a N-containing furan derivative. Green Chem. 16, 2204–2212. doi:10.1039/c3gc42436g
Chen, X., Yang, H., Zhong, Z., and Yan, N. (2017). Base-catalysed, one-step mechanochemical conversion of chitin and shrimp shells into low molecular weight chitosan. Green Chem. 19, 2783–2792. doi:10.1039/c7gc00089h
Dai, J., Li, F., and Fu, X. (2020). Towards shell biorefinery: advances in chemical-catalytic conversion of chitin biomass to organonitrogen chemicals. ChemSusChem. 13, 6498-6508. doi:10.1002/cssc.202001955
Dander, J. E., Baker, E. L., and Garg, N. K. (2017). Nickel-catalyzed transamidation of aliphatic amide derivatives. Chem. Sci. 8, 6433–6438. doi:10.1039/c7sc01980g
Eldred, S. E., Stone, D. A., Gellman, S. H., and Stahl, S. S., 2003). Catalytic transamidation under moderate conditions. J. Am. Chem. Soc. 125, 3422–3423. doi:10.1021/ja028242h
Fakhfakh, N., Cognet, P., Cabassud, M., Lucchese, Y., and De Los Ríos, M. D. (2008). Stoichio-kinetic modeling and optimization of chemical synthesis: application to the aldolic condensation of furfural on acetone. Chem. Eng. Process 47, 349–362. doi:10.1016/j.cep.2007.01.015
Ghosh, S. C., Ngiam, J. S., Seayad, A. M., Tuan, D. T., Chai, C. L., and Chen, A. (2012). Copper-catalyzed oxidative amidation of aldehydes with amine salts: synthesis of primary, secondary, and tertiary amides. J. Org. Chem. 77, 8007–8015. doi:10.1021/jo301252c
Ghosh, T., Jana, S., and Dash, J. (2019). KOtBu-promoted transition-metal-free transamidation of primary and tertiary amides with amines. Org. Lett. 21, 6690–6694. doi:10.1021/acs.orglett.9b02306
Goto, A., Endo, K., and Saito, S. (2008). Rh (I)-catalyzed hydration of organonitriles under ambient conditions. Angew Chem. Int. Ed. Engl. 47, 3607–3609. doi:10.1002/anie.200800366
Gu, M., Xia, Q., Liu, X., Guo, Y., and Wang, Y., 2017). Synthesis of renewable lubricant alkanes from biomass-derived platform chemicals. ChemSusChem. 10, 4102–4108. doi:10.1002/cssc.201701200
Gunanathan, C., Ben-David, Y., and David, M., 2007). Direct synthesis of amides from alcohols and amines with liberation of H2. Science 317, 790–792. doi:10.1126/science.1145295
Hosseini-Sarvari, M., and Sharghi, H. (2006). ZnO as a new catalyst for N-formylation of amines under solvent-free conditions. J. Org. Chem. 71, 6652–6654. doi:10.1021/jo060847z
Hülsey, M. J., Yang, H., and Yan, N. (2018). Sustainable routes for the synthesis of renewable heteroatom-containing chemicals. ACS Sustain. Chem. Eng. 6, 5694–5707. doi:10.1021/acssuschemeng.8b00612
Jing, Y., Guo, Y., Xia, Q., Liu, X., and Wang, Y. (2019a). Catalytic production of value-added chemicals and liquid fuels from lignocellulosic biomass. Inside Chem. 5, 2520–2546. doi:10.1016/j.chempr.2019.05.022
Jing, Y., Xin, Y., Guo, Y., Liu, X., and Wang, Y. (2019b). Highly efficient Nb2O5 catalyst for aldol condensation of biomass-derived carbonyl molecules to fuel precursors. Chin. J. Catal. 40, 1168–1177. doi:10.1016/S1872-2067(19)63371-1
Kniss, A. R. (2017). Long-term trends in the intensity and relative toxicity of herbicide use. Nat. Commun. 8, 14865. doi:10.1038/ncomms14865
Kobayashi, H., Techikawara, K., and Fukuoka, A. (2017). Hydrolytic hydrogenation of chitin to amino sugar alcohol. Green Chem. 19, 3350–3356. doi:10.1039/c7gc01063j
Lundberg, H., Tinnis, F., and Adolfsson, H. (2012). Direct amide coupling of non-activated carboxylic acids and amines catalysed by zirconium(IV) chloride. Chemistry 18, 3822–3826. doi:10.1002/chem.201104055
Lundberg, H., Tinnis, F., Selander, N., and Adolfsson, H. (2014). Catalytic amide formation from non-activated carboxylic acids and amines. Chem. Soc. Rev. 43, 2714–2742. doi:10.1039/c3cs60345h
Ma, J., and Gong, H. (2018). Cobalt(II)-catalyzed N-acylation of amines through a transamidation reaction. Eur. J. Org Chem., 2018, 4940–4948. doi:10.1002/ejoc.201800253
Meninno, S. (2020). Valorization of waste: sustainable organocatalysts from renewable resources. ChemSusChem. 13, 439–468. doi:10.1002/cssc.201902500
Milstein, L. U., Vogt, H., and Madsen, R. (2008). Amide synthesis from alcohols and amines by the extrusion of dihydrogen. J. Am. Chem. Soc. 130, 17672–17673. doi:10.1021/ja808129p
Nageswara, R. S., Chandra, M. D., and Adimurthy, S. (2014). Chitosan: an efficient recyclable catalyst for transamidation of carboxamides with amines under neat conditions. Green Chem. 16, 4122–4126. doi:10.1039/c4gc01402b
Ngo, D. T., Sooknoi, T., and Resasco, D. E. (2018). Improving stability of cyclopentanone aldol condensation MgO-based catalysts by surface hydrophobization with organosilanes. Appl. Catal. B Environ. 237, 835–843. doi:10.1016/j.apcatb.2018.06.044
Ngo, D. T., Tan, Q., Wang, B., and Resasco, D. E. (2019). Aldol condensation of cyclopentanone on hydrophobized MgO. promotional role of water and changes in the rate-limiting step upon organosilane functionalization. ACS Catal. 9, 2831–2841. doi:10.1021/acscatal.8b05103
Omari, K. W., Dodot, L., and Kerton, F. M. (2012). A simple one-pot dehydration process to convert N-acetyl-D-glucosamine into a nitrogen-containing compound, 3-acetamido-5-acetylfuran. ChemSusChem. 5, 1767–1772. doi:10.1002/cssc.201200113
Osada, M., Shoji, S., Suenaga, S., and Ogata, M. (2019). Conversion of N-acetyl-d-glucosamine to nitrogen-containing chemicals in high-temperature water. Fuel Process. Technol. 195, 106154. doi:10.1016/j.fuproc.2019.106154
Pattabiraman, V. R., and Bode, J. W. (2011). Rethinking amide bond synthesis. Nature 480, 471–479. doi:10.1038/nature10702
Pham, T. T., Lindsay, A. C., Chen, X., Gözaydin, G., Yan, N., and Sperry, J. (2019). Transferring the biorenewable nitrogen present in chitin to several N-functional groups. Sustainable Chemistry and Pharmacy 13, 100143. doi:10.1016/j.scp.2019.100143
Ramirez-Barria, C., Guerrero-Ruiz, A., Castillejos-López, E., Rodríguez-Ramos, I., Durand, J., Volkman, J., et al. (2016). Surface properties of amphiphilic carbon nanotubes and study of their applicability as basic catalysts. RSC Adv. 6, 54293–54298. doi:10.1039/c6ra08032d
Ramón, R. S., Marion, N., and Nolan, S. P. (2009). Gold activation of nitriles: catalytic hydration to amides. Chemistry 15, 8695–8697. doi:10.1002/chem.200901231
Rani, D., Singla, P., and Agarwal, J. (2018). ‘Chitosan in water’ as an eco-friendly and efficient catalytic system for Knoevenagel condensation reaction. Carbohydr. Polym. 202, 355–364. doi:10.1016/j.carbpol.2018.09.008
Sagawa, T., Kobayashi, H., Murata, C., Shichibu, Y., Konishi, K., and Fukuoka, A. (2019). Catalytic conversion of a chitin-derived sugar alcohol to an amide-containing isosorbide analog. ACS Sustain. Chem. Eng. 7, 14883–14888. doi:10.1021/acssuschemeng.9b02985
Sakthivel, B., and Dhakshinamoorthy, A. (2017). Chitosan as a reusable solid base catalyst for knoevenagel condensation reaction. J. Colloid Interface Sci. 485, 75–80. doi:10.1016/j.jcis.2016.09.020
Sheng, X., Li, N., Li, G., Wang, W., Wang, A., Cong, Y., et al. (2016). Direct synthesis of gasoline and diesel range branched alkanes with acetone from lignocellulose. Green Chem. 18, 3707–3711. doi:10.1039/c6gc01127f
Soulé, J. F., Miyamura, H., and Kobayashi, S. (2011). Powerful amide synthesis from alcohols and amines under aerobic conditions catalyzed by gold or gold/iron, -nickel or -cobalt nanoparticles. J. Am. Chem. Soc. 133, 18550–18553. doi:10.1021/ja2080086
Srinivas, K. V. N. S., and Das, B. (2003). A highly convenient, efficient, and selective process for preparation of esters and amides from carboxylic acids using Fe (3+)-K-10 montmorillonite clay. J. Org. Chem. 68, 1165–1167. doi:10.1021/jo0204202
Hoerter, J. M., Otte, K. M., Gellman, S. H., Cui, Q., and Stahl, S. S., (2008). Discovery and mechanistic study of Al(III)-catalyzed transamidation of tertiary amides. J. Am. Chem. Soc. 130, 647–654. doi:10.1021/ja0762994
Nguyen, T. B., Sorres, J., Tran, M. Q., Ermolenko, L., and Al-Mourabit, A., (2012). Boric acid: a highly efficient catalyst for transamidation of carboxamides with amines. Org. Lett. 14, 3202–3205. doi:10.1021/ol301308c
Uenoyama, Y., Fukuyama, T., Nobuta, O., Matsubara, H., and Ryu, I. (2005). Alkyne carbonylation by radicals: tin-radical-catalyzed synthesis of alpha-methylene amides from 1-alkynes, carbon monoxide, and amines. Angew Chem. Int. Ed. Engl. 44, 1075–1078. doi:10.1002/anie.200461954
Valeur, E., and Bradley, M. (2009). Amide bond formation: beyond the myth of coupling reagents. Chem. Soc. Rev. 38, 606–631. doi:10.1039/b701677h
Wang, J., Li, N., Li, G., Wang, W., Wang, A., Wang, X., et al. (2013). Solvent-free synthesis of C10 and C11 branched alkanes from furfural and methyl isobutyl ketone. ChemSusChem. 6, 1149–1152. doi:10.1002/cssc.201300318
Watson, A. J., Maxwell, A. C., and Williams, J. M. (2009). Ruthenium-catalyzed oxidation of alcohols into amides. Org. Lett. 11, 2667–2670. doi:10.1021/ol900723v
West, R. M., Liu, Z. Y., Peter, M., Gärtner, C. A., and Dumesic, J. A. (2008). Carbon-carbon bond formation for biomass-derived furfurals and ketones by aldol condensation in a biphasic system. J. Mol. Catal. Chem. 296, 18–27. doi:10.1016/j.molcata.2008.09.001
Xing, R., Subrahmanyam, A. V., Olcay, H., Qi, W., Pendse, H., and Huber, G. W. (2010). Production of jet and diesel fuel range alkanes from waste hemicellulose-derived aqueous solutions. Green Chem. 12, 1933–1946. doi:10.1039/c0gc00263a
Xu, J., Li, N., Yang, X., Li, G., Wang, A., Cong, Y., et al. (2017). Synthesis of diesel and jet fuel range alkanes with furfural and angelica lactone. ACS Catal. 7, 5880–5886. doi:10.1021/acscatal.7b01992
Yabushita, M., Kobayashi, H., Kuroki, K., Ito, S., and Fukuoka, A. (2015). Catalytic depolymerization of chitin with retention of N-acetyl group. ChemSusChem. 8, 3760–3763. doi:10.1002/cssc.201501224
Yamaguchi, K., Kobayashi, H., Oishi, T., and Mizuno, N. (2012). Heterogeneously catalyzed synthesis of primary amides directly from primary alcohols and aqueous ammonia. Angew Chem. Int. Ed. Engl. 51, 544–547. doi:10.1002/anie.201107110
Yan, N., and Chen, X. (2015). Sustainability: don’t waste seafood waste. Nature 524, 155–157. doi:10.1038/524155a
Ye, W., Ma, H., Liu, L., Yu, J., Lai, J., Fang, Y., et al. (2019). Biocatalyzed route for the preparation of surface-deacetylated chitin nanofibers. Green Chem. 21, 3143–3151. doi:10.1039/c9gc00857h
Yin, J., Zhang, J., Cai, C., Deng, G. J., and Gong, H. (2019). Catalyst-free transamidation of aromatic amines with formamide derivatives and tertiary amides with aliphatic amines. Org. Lett. 21, 387–392. doi:10.1021/acs.orglett.8b03542
Yutthalekha, T., Suttipat, D., Salakhum, S., Thivasasith, A., Nokbin, S., Limtrakul, J., et al. (2017). Aldol condensation of biomass-derived platform molecules over amine-grafted hierarchical FAU-type zeolite nanosheets (Zeolean) featuring basic sites. Chem Commun (Camb) 53, 12185–12188. doi:10.1039/c7cc06375j
Zhang, M., Imm, S., Bähn, S., Neubert, L., Neumann, H., and Beller, M. (2012). Efficient copper(II)-catalyzed transamidation of non-activated primary carboxamides and ureas with amines. Angew Chem. Int. Ed. Engl. 51, 3905–3909. doi:10.1002/anie.201108599
Keywords: chitin, N-acetyl group, transamidation, amine, amide
Citation: Xin Y, Shen X, Liu H and Han B (2021) Selective Utilization of N-acetyl Groups in Chitin for Transamidation of Amines. 2:634983. doi: 10.3389/fceng.2020.634983
Received: 29 November 2020; Accepted: 28 December 2020;
Published: 29 January 2021.
Edited by:
Yanqin Wang, East China University of Science and Technology, ChinaCopyright © 2021 Xin, Shen, Liu and Han. This is an open-access article distributed under the terms of the Creative Commons Attribution License (CC BY). The use, distribution or reproduction in other forums is permitted, provided the original author(s) and the copyright owner(s) are credited and that the original publication in this journal is cited, in accordance with accepted academic practice. No use, distribution or reproduction is permitted which does not comply with these terms.
*Correspondence: Huizhen Liu, liuhz@iccas.ac.cn