- 1Facultad de Ciencias Biológicas, Universidad Autónoma de Coahuila, Torreón, Mexico
- 2Instituto Tecnológico de Santiago Papasquiaro, Academia en Ciencias de los Alimentos, Tecnológico Nacional de México, Santiago Papasquiaro, Mexico
- 3Tecnológico de Monterrey, School of Engineering and Sciences, Monterrey, Mexico
Astaxanthin (ASX) is a xanthophyll pigment considered as a nutraceutical with high antioxidant activity. Several clinical trials have shown the multiple health benefits of this molecule; therefore, it has various pharmaceutical industry applications. Commercial astaxanthin can be produced by chemical synthesis or through biosynthesis within different microorganisms. The molecule produced by the microorganisms is highly preferred due to its zero toxicity and superior therapeutic properties. However, the biotechnological production of the xanthophyll is not competitive against the chemical synthesis, since the downstream process may represent 70–80% of the process production cost. These operations denote then an opportunity to optimize the process and make this alternative more competitive. Since ASX is produced intracellularly by the microorganisms, high investment and high operational costs, like centrifugation and bead milling or high-pressure homogenization, are mainly used. In cell recovery, flocculation and flotation may represent low energy demanding techniques, whereas, after cell disruption, an efficient extraction technique is necessary to extract the highest percentage of ASX produced by the cell. Solvent extraction is the traditional method, but large-scale ASX production has adopted supercritical CO2 (SC-CO2), an efficient and environmentally friendly technology. On the other hand, assisted technologies are extensively reported since the cell disruption, and ASX extraction can be carried out in a single step. Because a high-purity product is required in pharmaceuticals and nutraceutical applications, the use of chromatography is necessary for the downstream process. Traditionally liquid-solid chromatography techniques are applied; however, the recent emergence of liquid-liquid chromatography like high-speed countercurrent chromatography (HSCCC) coupled with liquid-solid chromatography allows high productivity and purity up to 99% of ASX. Additionally, the use of SC-CO2, coupled with two-dimensional chromatography, is very promising. Finally, the purified ASX needs to be formulated to ensure its stability and bioavailability; thus, encapsulation is widely employed. In this review, we focus on the processes of cell recovery, cell disruption, drying, extraction, purification, and formulation of ASX mainly produced in Haematococcus pluvialis, Phaffia rhodozyma, and Paracoccus carotinifaciens. We discuss the current technologies that are being developed to make downstream operations more efficient and competitive in the biotechnological production process of this carotenoid.
Introduction
Astaxanthin (ASX) (3, 3′-dihydroxy-β,β-carotene-4, 4′- dione) is a natural red pigment that serves as a precursor of vitamin A (Singgih and Julianti, 2015). Due to its potent antioxidant activity (superior to α, β-carotene, lutein, lycopene, canthaxanthin, and vitamin E), astaxanthin has gained considerable popularity as a human dietary supplement (Cuellar-Bermudez et al., 2015). Various investigations have provided substantial evidence of the use of this compound for various health conditions like prevention and treatment of atherosclerosis, cancer chemoprevention, maintenance and treatment of eye and vision disorders, anti-inflammatory properties, dermatological conditions, among others (Table 1) (Williamson et al., 2020). The multiple health benefits that have been reported of ASX make this molecule a real alternative in the pharmaceutical industry for the prevention and treatment of several diseases.
Currently, industrial production of ASX is carried out mainly by chemical synthesis; however, the biosynthesis of this molecule, by the cultivation of microalgae, yeast, and bacteria, is gaining industrial importance. This shift occurs mainly due to safety concerns for human consumption and higher biological activities of the biosynthesized compound than the chemically prepared molecule (Diao et al., 2020). Unfortunately, the biotechnological process for ASX production is not economically competitive against chemical synthesis, so the improvement of ASX production, mainly the downstream processes, has become a significant scientific challenge, regardless of the microorganism used to produce this molecule. The downstream processes represent up to 80% of ASX’s production costs, so any improvement in these stages may have a considerable impact on the whole feasibility of the process.
Since ASX is produced intracellularly, cell recovery and cell disruption processes are the initial stages in the downstream process, being determinant in the process’s total yield. Most companies employ sedimentation and centrifugation to concentrate the biomass in ASX’s biotechnological production (Li et al., 2020b). However, new and more economical alternatives like flocculation and flotation have been proposed in the dewatering process of the biomass (Barros et al., 2015). Cell disruption is a necessary step in the obtention of the metabolite and can be carried out by classical physical, chemical, or enzymatic methods, with the use of ionic liquids in recent years, since they are considered green solvents and proved to be useful for cell disruption and ASX extraction (Choi et al., 2019). The use of a specific method may depend on the producing microorganism since bacteria, yeast, and microalgae differ in their cell wall and cellular membrane composition (Gomes et al., 2020).
After cell disruption and to avoid ASX degradation before the extraction process, spray drying or freeze-drying are generally used. The operational parameters must be carefully selected when using these technologies since ASX is a molecule with low stability and can be degraded during the drying process and storage of the powder (Ahmed et al., 2015). Similarly, the drying process may be used when formulating commercial ASX products, so the product stability must also be ensured (Feng et al., 2018; Zhao et al., 2019).
The ASX extraction from dried, cracked cells is carried out mainly using organic solvents due to the hydrophobic nature of ASX. However, there are other innovative approaches to improve the extraction process, such as combining cell disruption and ASX extraction into a single step and the use of compressed fluids technologies like supercritical fluid extraction (Molino et al., 2020) and pressurized liquid extraction (Gallego et al., 2019). Other assisted technologies like ultrasound-assisted, microwave-assisted, enzyme-assisted, and pulsed electric field-assisted are being extensively tested (Saini and Keum, 2018).
After extraction, the purification step is essential when preparing nutraceutical or pharmaceutical products since a high-purity product is required (99% or more). The liquid-solid chromatography is extensively used in ASX purification (Yang et al., 2017; Casella et al., 2020), whereas liquid-liquid chromatography may simplify and accelerate the process (Du et al., 2016; Fábryová et al., 2020). The parameters that influence the ASX purification in chromatography can be optimized to improve the process even though processing time is always the most difficult to reduce. On the other hand, the use of new techniques like comprehensive two-dimensional liquid chromatographies may considerably reduce sample processing time (Gallego et al., 2019).
The formulation is the final step in the downstream process of ASX. This molecule is relatively unstable due to its intrinsic reactivity; therefore, the formulation must ensure the purity, stability, and delivery of the compound in the best possible form. Encapsulation is the preferred method in ASX formulation, where different conventional techniques are extensively reported (Santos-Sánchez et al., 2020); however, modernization can be seen in the use of SC-CO2 to produce capsules of the carotenoid (Liu et al., 2019a).
Since ASX has shown great potential for the pharmaceutical industry, the need for a competitive biotechnological process is greatly desired. The present review focuses on the downstream process for the obtention of ASX, where we analyze the current technologies and recent advances that represent cheaper or more efficient alternatives. Because the microbial production of ASX can be carried out in different microorganisms, the cell disruption and extraction methods can vary from one organism to another. Also, the characteristics of the final product differ substantially, and the purification step may require pretreatment. Other reviews discussed the ASX production process in the microalga Haematococcus pluvialis, but we emphasize studies developed in microorganisms of industrial interest and accepted by the Food and Drug Administration (FDA) for ASX production. Specifically, we discuss H. pluvialis, Phaffia rhodozyma, and Paracoccus carotinifaciens in the biosynthesis and mainly the new insights in the downstream process for the production of ASX molecule.
The ASX Molecule
Carotenoids are terpenoid pigments of 40 carbon atoms, classified into carotenes and xanthophylls. Carotenes, like β-carotene and torulene, only have carbon and hydrogen in their chemical structure, while xanthophylls, like ASX and canthaxanthin, also contain oxygen (Mata-Gómez et al., 2014).
ASX has the molecular formula C40H5204, and its molar mass is 596.84 g/mol. It is a ketocarotenoid belonging to the chemical group of terpene compounds insoluble in water but soluble in most organic solvents. Its systematic IUPAC name is (6S)-6-hydroxy-[(1E,3E,5E,7E,9E,11E,13E,15E,17E)-18-[(4S)-4-hydroxy-2,6,6-trimethyl-3-oxocyclohexen-1-yl]-3,7,12,16-tetramethyloctadeca-1,3,5,7,9,11,13,15,17-nonaenyl]-2,4,4-trimethylcyclohex-2-en-1-one. It is known by astaxanthin, E-astaxanthin, (S,S)-astaxanthin, astaxanthin, and all-trans-astaxanthin (PubChem, 2020). This carotenoid contains eight successive condensed isoprene molecules and 11 conjugated double bonds (polyene system). The two ends of the ASX molecule have a β-ionone ring with hydroxy (OH) and keto (C=O) groups (Figure 1); these characteristics of the molecule can explain its higher antioxidant activity and its ability to be esterified (Ambati et al., 2014).
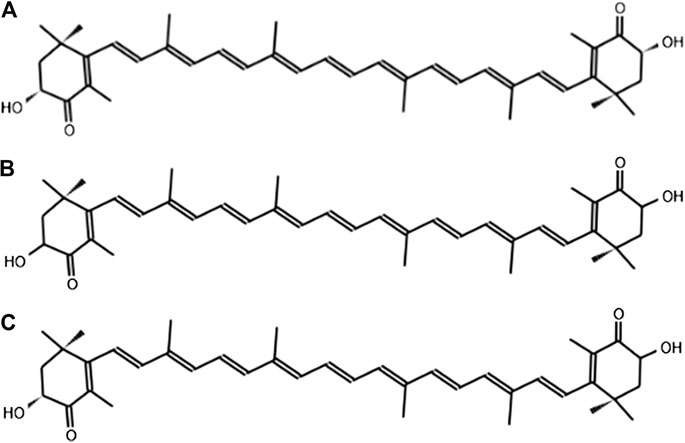
FIGURE 1. Stereoisomers of the chemical structure of astaxanthin: (A) 3 R,3′R; (B) 3S,3′S; (C) 3R, 3′S.
The double bonds present in ASX are critical in the antioxidant capacity of this carotenoid. They react and donate electrons to free radicals, neutralizing and converting them into stable molecules (Guerin et al., 2003). When one hydroxyl group reacts with a fatty acid, a monoester is formed, whereas when both hydroxyl groups react with fatty acids, a diester is formed (Ambati et al., 2014). Due to its chemical structure, ASX is sensitive to light, heat, and oxygen and therefore can quickly occur during extraction, isomerization, or degradation reactions (Fang et al., 2019). Like the esterification by one or two fatty acids, other reactions produce more stable structures than ASX free form (Hussein et al., 2006). ASX may also form a chemical complex with proteins (carotenoproteins) or lipoproteins (carotenolipoproteins) (Higuera-Ciapara et al., 2006).
The molecule of ASX displays geometric isomers cis or trans because each double bond from the polyene chain may exist in the two configurations (Higuera-Ciapara et al., 2006; Visioli and Artaria, 2017). We can find the isomers E-trans or Z-cis in ASX, the most common being all-E-trans ASX, 13-Z-cis ASX, and 9-Z-cis ASX (Honda et al., 2020b). Although cis-isomers are thermodynamically less stable than the trans-isomers, the cis-configurations have shown higher antioxidant power and bioactivity than that of the trans-configuration (Liu et al., 2018b; Yang et al., 2019).
All-E-trans ASX might exist as three stereoisomers since it contains chiral carbon atoms with R or S stereochemistry in their 3 and 3′ positions of the β-ionone rings. The three possible optical isomers of astaxanthin are the enantiomers 3R, 3′R and 3S, 3′S, and the meso form 3R, 3′S (Figure 1). Different antioxidant activities have been observed in the enantiomers of ASX, and it has been reported that 3S, 3′S-trans form exhibits higher bioactivity than the other stereoisomers (Liu et al., 2016; Sun et al., 2016).
Natural ASX and Synthetic ASX, Price Competitiveness
According to Zhang et al. (2020a), ASX consumption has increased dramatically in the last five years. Only natural astaxanthin (N-ASX) can be used as a human nutraceutical supplement due to several safety studies, extensive clinical trials, and regulations. This carotenoid is obtained from natural sources such as bacteria, protists, fungi, algae, plants, and crustacean by-products (Fang et al., 2019; Tran et al., 2019; Zhang et al., 2020a). Synthetic astaxanthin (S-ASX), on the other hand, has not been proven safe for human consumption, and its use is only limited as a feed ingredient to pigment the flesh of fish in aquaculture (Capelli et al., 2013). S-ASX is synthesized in a multistep process from petrochemicals. Further, N-ASX has shown no toxicity and has demonstrated superior therapeutic properties than S-ASX (Shah et al., 2016).
Commercial ASX is produced by the chemical method, S-ASX, or biosynthesis, N-ASX, in the alga Haematococcus pluvialis, the yeast Phaffia rhodozyma, and the bacteria Paracoccus carotinifaciens (Ambati et al., 2014; Katsumata et al., 2014). However, other microorganisms are promising for the biotechnological production of N-ASX (Sun et al., 2019; Tran et al., 2019). N-ASX has become a compound of great interest in the pharmaceutical industry, and many companies are producing this carotenoid (Table 2).
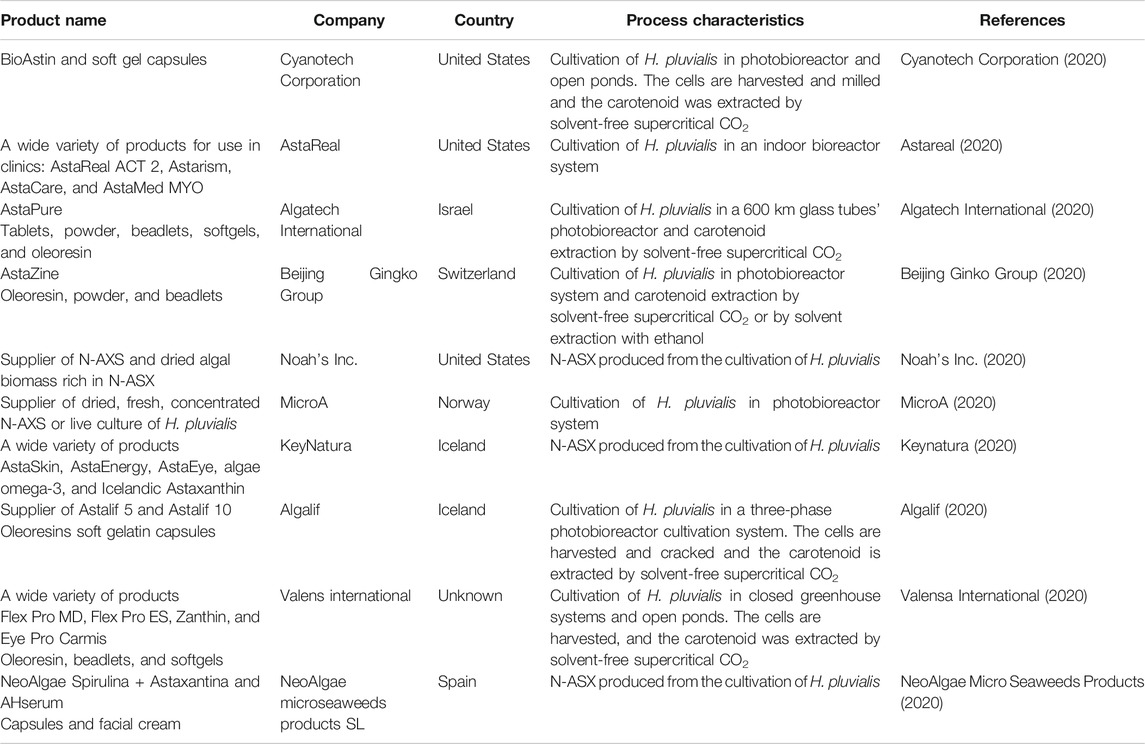
TABLE 2. Top ten ranked N-ASX-producing companies over the world according to Venture radar (2020).
Despite N-ASX’s advantages, about 99% of the ASX on the market is produced synthetically by big companies such as BASF and Hoffman-La Roche, while less than 1% is derived from natural sources (Molino et al., 2018b). In recent years, the market for N-ASX has been growing substantially. The market value of N-ASX depends on the purity of the product and varies from USD 2,500–7,000/kg to about USD 15,000/kg in the case of N-ASX for human applications such as cosmetics, food, and dietary supplements. S-ASX has a market value of about USD 1,000/kg (Koller et al., 2014; Shah et al., 2016; Molino et al., 2018b; Zhang et al., 2020a). Rodríguez-Sáiz et al. (2010) reported a price of USD 2,500/kg for pure N-ASX and suggested using improved strains of P. rhodozyma, low-cost raw materials, and the improvement of fermentation parameters may provide a cost-effective alternative method in preparing N-ASX. Panis and Carreon (2016) used a theoretical model to perform a technical and economic study for N-ASX production from H. pluvialis in two European cities: Livadeia (Greece) and Amsterdam (Netherlands). In that report, N-ASX production cost (not considering the downstream process) was calculated at €1,536/kg and 6,403/kg for Livadeia and Amsterdam, respectively, while the production cost for S-ASX was €880/kg. The authors concluded that N-ASX is yet unable to compete with S-ASX under the analyzed conditions.
New strategies must be sought to make N-ASX production more competitive, and the downstream processes must be considered due to their high impact on the overall cost of the process as previously described. Zgheib et al. (2018) reported a theoretical economic study for extracting N-ASX from H. pluvialis. In this study, the authors assumed that the biomass suspension was supplied from the microalgae producing platform; therefore, they focused on the costs generated in the downstream process. Economic viability was achieved with an N-ASX price superior to USD 1,500/kg and biofertilizer's commercialization obtained from the residual H. pluvialis biomass, estimated at USD 40/kg, which added value to the process. Li et al. (2011) operated an N-ASX production pilot plant from which they scaled up to a hypothetical plant with a production capacity of about 900 kg N-ASX per year. According to their economic analysis, including the cultivation of H. pluvialis and the downstream process, the production cost of the carotenoid was USD 555/kg or USD 718/kg, considering a 10 years depreciation. In contrast, the production cost of S-ASX was estimated to be about USD 1,000/kg. The authors attributed N-ASX production’s low-cost to a low-cost photobioreactor employed, two-step cultivation of H. pluvialis, cheap labor, land, and utilities in China. Furthermore, this economic analysis was carried out based on biomass with 2.5% N-ASX and the authors mentioned that this concentration might be higher in the microalgae, so production costs would also decrease. Dursun et al. (2020) simulated two processes to obtain powdered N-ASX from wheat bran and oil mixture from olive pomace. The N-ASX was produced in solid-state fermentation by the cultivation of P. rhodozyma in the two agro-industrial wastes. The authors integrated the upstream and downstream processes in their economic analysis and calculated a production cost of USD 13,870/kg for the powder formulation (PF), whereas for the oil mixture formulation (OF), it was estimated at USD 7,809/kg. The two processes proved to be feasible, and the payback times of PF and OF processes were 4.92 and 7.19 years, respectively.
Replacing S-ASX for animal feed application with N-ASX is very attractive due to the superior properties of N-ASX. The main holdback is clearly the price of N-ASX, which may reach USD 5,000/kg whereas the S-ASX price is still only about USD 2,000/kg (Li et al., 2020b). On the other hand, although N-ASX generally has a higher production cost than S-ASX, its value may be overpriced since its market niche is directed to treating diseases.
Relevant Biotechnological Sources for N-ASX Production
Since N-ASX can be biotechnologically produced principally by H. pluvialis, P. rhodozyma, and P. carotinifaciens, it is of great interest to examine the characteristics of these microorganisms to understand the downstream processes carried out in each different cell type. Alternatively, many genetically modified organisms (GMO) have been generated and may represent a more efficient option to produce this red pigment.
Haematococcus pluvialis
H. pluvialis is the only authorized N-ASX-producing microorganism for human consumption. N-ASX obtained from this microalga has been approved in Europe, Japan, and the United States. The European Food Safety Authority (EFSA) has advised an acceptable daily intake of 0.034 mg/kg for humans, whereas the United State Food and Drug Administration (FDA) considers N-ASX from H. pluvialis as “GRAS” (generally recognized as safe) (Davinelli et al., 2018). H. pluvialis (class Chlorophyceae; order Volvocales; family Haematococcaceae) is a unicellular freshwater green microalga worldwide (Eom et al., 2006). It is a photosynthetic eukaryote cell with spherical, ellipsoidal, or pear-shape with two flagella when it grows under favorable cultural conditions. This type of morphology is called macrozooids (zoospore); the cells are green and between 8 and 20 µm long (Hagen et al., 2002). The macrozooids are fast-growing vegetative cells and may divide into 2–32 daughter cells by mitosis (Shah et al., 2016). When environmental stress occurs (i.e., nutrient deprivation, high salinity, nonoptimal temperature, or high light irradiance), the cells interrupt cell division, lose their flagella (they transform from motile to nonmotile cells), and increase their cell size. This morphology is known as hematocysts (aplanospores), and during that stage, the microalgae accumulate large amounts of astaxanthin and lipids, acquiring a characteristic bright red color (Hagen et al., 2002). Aplanospore cell walls are composed of an outer primary wall, a trilaminar sheath, a secondary wall, and a tertiary wall. The trilaminar sheath contains sporopollenin-like material (algaenan), while the secondary and tertiary walls contain mannose and cellulose. These structures give the cells high resistance against extreme environmental conditions and the ability to withstand mechanical and chemical attacks. Thus, the H. pluvialis structure makes it difficult to extract the N-ASX (Hagen et al., 2002; Safi et al., 2014; Liu et al., 2017). This microalga is considered the best source of N-ASX since it can accumulate 3.8–5.0% dry weight of this carotenoid, depending on the culture conditions, producing predominantly esterified N-ASX (95%) in the 3S, 3′S form (Chekanov et al., 2014; Khoo et al., 2019a).
Phaffia rhodozyma
P. rhodozyma was considered the only native N-ASX producing yeast until Rhodosporidium toruloides was recently reported (Tran et al., 2019). P. rhodozyma (class Tremellomycetes; order Cystofilobasidiales; family Cystofilobasidiaceae), also known as the teleomorphic state Xanthophyllomyces dendrorhous, is considered the second-best source of biotechnological production of N-ASX and produce mainly free and nonesterified 3R, 3′R N-ASX (Kucsera et al., 2000). This yeast is considered as GRAS microorganism and approved as a color stabilizer for fish feed supplementation by the FDA (Barredo et al., 2017). P. rhodozyma was initially isolated from slime fluxes from broad-leaved trees in Japan by Herman J. Phaff. Ten strains of this yeast were isolated in the late 60’s from Alnus, Betula, Cornus, Fagus, and Ulmus tree types. Later, it was also found in Russia, Chile, Finland, and the United States. The vegetative cells of P. rhodozyma are ellipsoidal, reproduce by budding, and occur singly, in pairs and sometimes in short chains. Sexual activity has been observed in this yeast, involving mating and sporulation between two yeast cells. The conjugation between the mother cell and its bud (pedogamy) or between two different cells has also been identified (Kucsera et al., 2000).
P. rhodozyma grows in different carbon substrates (Kanwugu et al., 2020) and can produce different carotenoids like N-ASX, phoenicoxanthin, hydroxyechinenone, echinenone, and β-carotene, with N-ASX being the main product (83–87% of total). The accumulation of these molecules gives the reddish color to the yeast colonies. The cell wall of the yeasts is rigid and remarkably thick, it represents 20–25% of the dry weight of the cell, and it is in contact with the extracellular medium. The primary structural components of that wall are polysaccharides, principally glucans and mannans, and a minor percentage of chitin and proteins. On the other hand, the plasma membrane of yeasts is a thin lipid bilayer with proteins that control the material exchange in the cell (Stewart and Stewart, 2017). N-ASX production by P. rhodozyma involves a growth phase, in which high cellular density is reached, and a maturation phase, where a stress condition may contribute to N-ASX formation (Schewe et al., 2017; Zhang et al., 2020b). It has been shown that stress triggers relevant structural changes in the yeast cell envelop system and reduces cell membrane fluidity (Qiu et al., 2019), which complicates obtaining the N-ASX produced by P. rhodozyma. Although, at this point, N-ASX produced by P. rhodozyma is destined for the aquaculture market, this yeast may eventually be able to compete with the H. pluvialis for N-ASX production for other applications.
Paracoccus carotinifaciens
The species carotinifaciens, haeundaensis, and marcusii from the genus Paracoccus have been reported in the production of N-ASX; especially, P. carotinifaciens is gaining significant relevance since its dry cell biomass has been approved by the United State FDA and the EFSA as a coloring agent in salmon and trout aquaculture (Katsumata et al., 2014). P. carotinifaciens (class Alphaproteobacteria; order Rhodobacterales; family Rhodobacteraceae) is a rod-shaped, nonspore-forming, motile by peritrichous flagella, Gram-negative, aerobic bacteria found in soil. The cells can be 0.30–0.75 µm in diameter and 1.0–5.0 µm in length and assemble round, smooth, orange colonies. The optimum growth of this bacteria is 28 °C and pH 6.5–7.5. It uses ammonium but not nitrate as nitrogen source and a wide variety of compounds like glucose, mannose, maltose, xylose, galactose, sucrose, lactose, and glycerol (Tsubokura et al., 1999). The cell envelope in Gram-negative bacteria consists of an outer membrane in contact with the external environment and an inner membrane, in contact with the intracellular material. The cell wall is located between these two membranes and is composed of peptidoglycan, a copolymer formed by N-acetyl-glucosamine and N-acetyl muramic acid. Unlike Gram-positive bacteria, the peptidoglycan content is lower in Gram-negative bacteria, making them less resistant to cell breakdown (Silhavy et al., 2010; Sutterlin et al., 2016; Starke et al., 2019). P. carotinifaciens produces a wide range of carotenoids, N-ASX being 55–60% of the total carotenoids of the cell. The N-ASX produced by this bacterium is nonesterified in the 3S, 3′S form, just like in wild Atlantic or Coho salmon (Katsumata et al., 2014). The N-ASX produced by this bacterium may be a potential candidate for human consumption since this molecule is the same as that produced by H. pluvialis (3S, 3′S form), which has been tested in many clinical trials (Davinelli et al., 2018; Giannaccare et al., 2020).
Nonnative Producing Microorganisms
Production of N-ASX in nonnative host microorganisms has been carried out through genetic engineering or metabolic engineering techniques. Although these strategies have allowed a considerable increase in the product concentration (Zhang et al., 2020a), N-ASX from these microorganisms is not currently allowed for human consumption or fish feed supplementation. There is still little consumer acceptance for products from a genetically modified organism (GMO); additionally, the regulatory approval can take a long time and may vary in different countries. On the other hand, N-ASX produced in GMOs has shown no toxic effects and abnormalities in animal models and has been effective in inhibiting metastasis of melanoma cells in a rodent model (Tseng et al., 2020). Simultaneously, many commercial pharmaceutical products are produced in GMOs, which means that this strategy could be essential in the future of the biotechnological production of N-ASX. The use of GMOs to produce N-ASX offers many advantages in biotechnological processes. These microorganisms are easy handed, characterized by single-stage N-ASX production, and considered GRAS and grow fast in single substrates, and the fermentation process is simple. Besides, they reach high N-ASX productivities, their genomes are easy to manipulate, and they have been used to produce commercial recombinant products, among them pharmaceutical products (Tripathi, 2016; Zhang et al., 2020a). Bacteria and yeasts have been genetically modified for N-ASX production; some of these microorganisms and their characteristics are shown in Table 3.
The Downstream Process in N-ASX Production
When selecting the recovery and purification processes, the aspects that must be considered are the location of the product (intracellular or extracellular), the concentration of the product and impurities in the fermentation broth, the physical and chemical properties of the molecule, the intended use of the product (purity), and the market price (Stanbury et al., 1995). An efficient downstream process in N-ASX production may increase productivity, decrease production costs, and make a competitive process against S-ASX production, even for aquaculture purposes. Many studies focus on optimization of N-ASX biosynthesis by the microorganism (strain selection, growth conditions, culture media, and genetic manipulation), while the downstream processes is an area that urgently needs improvements for N-ASX production to be competitive. A general downstream process workflow for N-ASX production may include: 1) cell recovery, 2) cell disruption, 3) drying, 4) extraction, 5) purification, and 6) formulation (Figure 2). As we discuss below, some strategies can be combined to avoid some stages of the workflow and reduce production costs.
Cell Recovery
Once the N-ASX has been produced by a biotechnological process in a bioreactor, the first step of the downstream process involves cell recovery. Because the product is intracellular, the recovered cells represent the total N-ASX synthesized by the microorganism. In this way, a suitable recovery method that ensures the most significant number of cells and reduces the associated costs with cell dewatering must be selected.
The microbial cells can be harvested mainly by gravity sedimentation, flocculation, flotation, membrane filtration, and centrifugation. Gravity sedimentation is an economical option, but this process strongly depends on the particle size (biomass), cellular concentration, differences between the cell and the liquid densities, and the cell’s ability to aggregate (Panis and Carreon, 2016; Liu et al., 2017). Flocculation refers to the aggregation of unstable and small particles through surface charge neutralization, electrostatic patching, or bridging after the addition of flocculants, which accelerate solids sedimentation (Matter et al., 2019). In the case of pharmaceutical products, flocculants are not recommended as they cause product contamination and make future purification difficult (Liu et al., 2017). The flocculation process is influenced by cell surface properties, cell concentration, pH, ionic strength, type and dosage of flocculants, and mixing process (Branyikova et al., 2018). Flotation is another operation that can be used for cell harvesting. In this process, gas bubbles are supplied to the broth, causing cells to transport to the liquid surface. The process mechanism depends on the formation of stable microorganism-bubble complexes, and the difference in density between these complexes and the liquid causes the flotation of biomass and foam development. This microorganism-enriched foam can then be removed with a skimmer (Gulden et al., 2020). In this technique, microbial removal depends on the recycling rate, air tank pressure, hydraulic retention time, and particle floating rate, while the concentration of the produced slurry depends on skimmer velocity and relative positions towards the surface of the liquid (Barros et al., 2015). Filtration is a size-exclusion method in which cells pass through a suitable membrane under high pressure to obtain a thick paste of biomass. This process is beneficial and scalable for cell harvesting when problems in membrane blocking can be minimized or prevented; however, it can be expensive (Matter et al., 2019). In tangential flow filtration, the medium flows tangentially across a membrane, and the retentate is recirculated. The cells are kept in suspension, thus avoiding blockage of the membrane (Barros et al., 2015). Centrifugation involves separating liquids from solids of different densities based on a mechanical, gravitational force, accelerating the sedimentation of the highest density components. However, due to the intensive energy requirement and high investment cost, this method is recommended only for high-value products like N-ASX (Stanbury et al., 1995; Matter et al., 2019).
In the case of H. pluvialis, cells are relatively small (10–60 µm in diameter), its cellular concentration only reaches about 0.5% dry weight, the densities of the cultivation medium and the microalgae cells are similar and are negatively charged, which results in a stable dispersed state of the algal suspension that makes sedimentation challenging (Panis and Carreon, 2016; Liu et al., 2017). On the other hand, Orona-Navar et al. (2017) reported that H. pluvialis synthesizes an increased number of polysaccharides and carbohydrates during the aplanospore phase (accumulation of N-ASX), which results in the formation of aggregates and rapid sedimentation. Furthermore, the N-ASX accumulation resulted in a growth in cell size and increasing the settling velocity of H. pluvialis (Baroni et al., 2019). The cultures of N-ASX producing bacteria and yeasts (native or nonnative) reach higher cell densities in much less time than algae cultures, increasing the settling rate of the cells and facilitating biomass harvesting (Zhang et al., 2020b). When using yeast for N-ASX production, it is highly recommended to select a flocculating yeast strain. Some yeast strains of S. cerevisiae, K. marxianus, and Y. lipolytica can adhere to other cells and flocculate, which simplifies the recovery of the cells and reduces the production costs by avoiding the use of costly operations like centrifugation or filtration (Dasgupta et al., 2017; Di Gianvito et al., 2017; Louhasakul et al., 2019).
Gravity sedimentation is a cost-effective and energy-efficient technique; nevertheless, it progresses at a slow rate, and typically a complementary operation must be used. For most applications, H. pluvialis harvesting generally comprises a two-step concentration involving gravity sedimentation and centrifugation (Barros et al., 2015; Zgheib et al., 2018; Li et al., 2020b). Centrifugation is a highly efficient method for biomass recovery (close to 100%); nonetheless, it represents a high investment cost and is high-energy consuming, which increases the production costs (Khoo et al., 2019b). The energy consumption of 1 kWh/m3 has been calculated in a disc-stack centrifuge to obtain 15–25% final slurry concentration of H. pluvialis (Panis and Carreon, 2016).
Najjar and Abu-Shamleh (2020) mentioned that centrifugation alone is not suitable for microalgae harvesting. They suggested that flocculation, as a primary preconcentration step, can increase the energy output significantly, thus reducing the operation cost of the separation process. Putri et al. (2020) used aluminum sulfate as flocculant at a concentration range of 0.25–2.0 g/L in H. pluvialis cell harvesting. The highest flocculation efficiency of 94.5% was obtained at 1 g/L of aluminum sulfate with a pH value of 3.69. This efficiency is comparable with the 95% recovery obtained by Panis and Carreon (2016) using centrifugation in the separation of H. pluvialis cells. Although the addition of flocculants is not recommended in pharmaceutical applications, the authors stated that aluminum sulfate is nontoxic, and it is used in vaccine making and as a food additive. The flocculation-based process is a simple, efficient, scalable, and low-cost technique that may contribute to the one-step cell concentration process (Matter et al., 2019). Najjar and Abu-Shamleh (2020) mentioned that the energy cost for centrifugation and filtration is more than USD 2.00/kg oil in microalgae, while the cost for flocculation is USD 0.25/kg oil. However, flocculation has not yet been sufficiently explored in H. pluvialis cell harvesting to draw concrete conclusions about its superiority.
Electroflocculation is an up-and-coming technique for cell harvesting based on the in situ generation of flocculants. The use of aluminum electrode plates during electrolysis produces a metal flocculant that contributes to cell flocculation due to charge neutralization, which creates the sorption affinity for negatively charged cells. Electroflocculation has been reported as a low-cost, high-efficient, and uncomplicated method. Although this technique has not been explored in the N-ASX production, recent microalgae harvesting reports suggest its usefulness in this biotechnological process. For example, Shi et al. (2017) applied current densities of 22.2, 44.4, and 66.7 A/m2 in Chlorella vulgaris harvesting and reached a maximum harvesting efficiency of 98% for all treatments. Under higher current densities, shorter electrolysis time was needed to reach the maximum microalgae harvesting. Interestingly, the energy consumption for microalgae harvesting (higher than 80%) was 0.87 × 10–4, 2.22 × 10–4, and 2.94 × 10–4 kWh/g biomass for 22.2, 44.4, and 66.7 A/m2, respectively.
Hydrocyclone is a low capital cost centrifuge; it can handle large quantities of slurry and may be an option to substitute costly equipment like a disc-stack centrifuge and multichamber centrifuge (Najjar and Abu-Shamleh, 2020). Cilliers and Harrison, (2019) harvested cells of a flocculating strain of S. cerevisiae using a hydrocyclone. The principal variables considered in this study were yeast concentration in a range of 2.0–14 g dry cell weight/L and yeast floc size, where 90% of the flocs were smaller than approximately 20, 100, and 200 µm distributions. It was observed that recovery was maximized with an increase in floc size and decrease in yeast concentration; the highest recovery (85%) was obtained at 2.0 g dry cell weight/L and 200 µm floc size.
Flotation is another alternative for cell harvesting that has very promising energy-saving characteristics. Implementing this economic process in N-ASX recovery can be beneficial in the downstream processes (Sankaran et al., 2018; Alhattab et al., 2019). One of the various flotation techniques is dissolved air flotation, where at very high pressures, gas bubbles are produced in size range of 10–30 µm. This method is characterized by microbubbles and high turbulence that increase the probability of flotation, especially for hydrophobic substances and fine particles such as microorganisms. Thus, dissolved air flotation is an appropriate option in N-ASX downstream processes application. Gulden et al. (2020) compared the specific product related to the cost of flotation (F), centrifugation (C), and cross-flow filtration (CFF). Based on the energy demand, maintenance, installation capital, and additives, the expected product cost calculated for S. cerevisiae cell recovery was about 6, 10, and 12 €/t product for F, C, and CFF, respectively. The authors studied the effect of operational parameters and found that the flotation rate of the yeast was improved by increasing the recycle flow rate and saturation pressure, whereas increasing the gas flow rate improved the flotation rate and the energy efficiency of the process.
Some cultivation strategies can be adopted to decrease production costs in H. pluvialis cell recovery. Do et al. (2019) produced N-ASX from H. pluvialis in a Twin-Layer Porous Substrate Photobioreactor (TL-PSBR), where biomass harvest is less complicated. This system includes a substrate layer (paper) in which a suspension of the algae is immobilized and through which the substrate is recirculated. The algae grow over the paper, and at the end of the algae cultivation, the paper is removed and dried with no centrifugation or filtration to remove excess water. After cell cultivation, the substrate layer was harvested and dried for 2 h at 105 °C and the highest biomass productivity was 8.7 g/m2d.
Cell Disruption and N-ASX Extraction
Cell disruption and N-ASX extraction are near related operations since the amount of the extracted carotenoid depends on the efficiency of the selected cell disruption method. Many technologies can be carried out in parallel to make the downstream processes faster and more efficient. Table 4 shows recent reports about cell disruption and N-ASX extraction. These methodologies are discussed below.
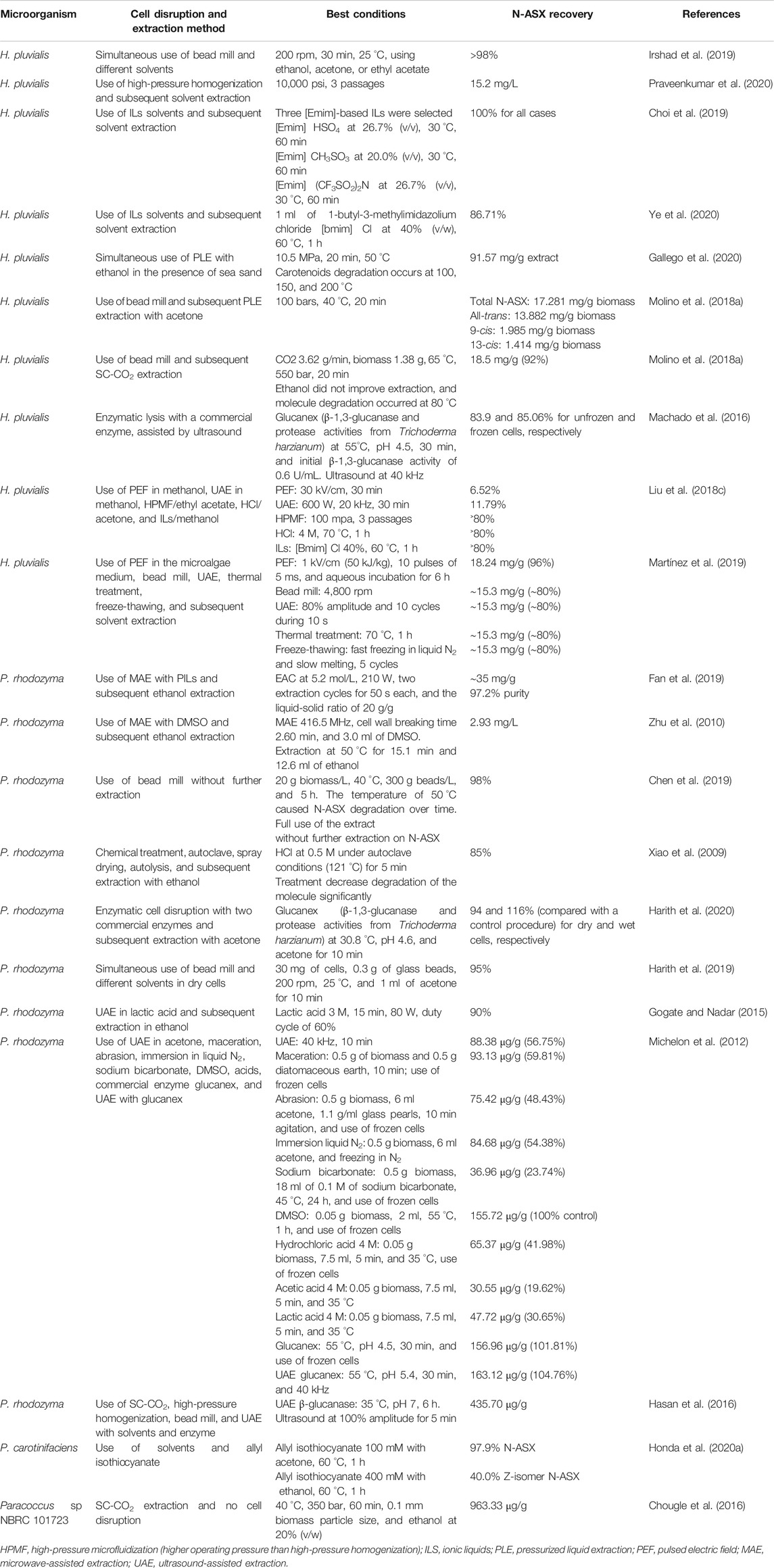
TABLE 4. Recent reports in N-ASX producing microorganisms cell disruption and N-ASX extraction methods.
Mechanical and Nonmechanical Cell Disruption Methods
Once the cells have been harvested, the next step is critical and encompasses the N-ASX release from producing microorganisms. Since N-ASX is an intracellular product, the breakdown of microalga, Gram-negative bacteria, and yeast cells needs to be carried out by different methods. The selected cell disruption method must be efficient and must break the largest number of cells possible since the more the cells are broken, the higher the amount of the product can be extracted, with a linear correlation between N-ASX recovery and the degree of cell disruption (Praveenkumar et al., 2020). The principal cell disruption techniques are bead milling, ultrasonication, osmotic shock, high-pressure homogenization, freeze-thawing, enzymatic lysis, and chemical lysis; they can be applied individually or in various combinations. Mechanical methods like bead milling and high-pressure homogenization may guarantee a high-yield N-ASX recovery; however, there is high-energy consumption associated with these techniques. These methods produce cell debris and have extended processing times, and the degradation may result in excessive heating.
It has been reported that bead milling and high-pressure homogenization are mostly used to disrupt large-scale processes for H. pluvialis cells (Panis and Carreon, 2016; Zgheib et al., 2018; Li et al., 2020b). In bead milling, the main operating parameters to take into account are rotational speed, time intervals, and the biomass/bead ratio (Irshad et al., 2019), while for high-pressure homogenization they are pressure and passage number (Praveenkumar et al., 2020). Chen et al. (2019) optimized the operating conditions of bead milling for the disruption of P. rhodozyma cells. The cell disruption was carried out at yeast concentrations of 10, 20, 30, and 40 g/L, the temperature of 22, 30, 40, and 50 °C, and bead amount of 100, 200, 250, and 300 g/L. The maximum extraction yield (98%) was achieved at the cell concentration of 20 g/L, 40 °C, and 300 g beads/L in 5 h. Molino et al. (2018b) reported that the recovery of N-ASX from H. pluvialis cells was 86.4% when using bead milling, while the recovery was less than 1% without cell breakdown. On the other hand, Praveenkumar et al. (2020) applied a high-pressure homogenization method to disrupt H. pluvialis cysts in one to three passages and three different pressures (10,000, 20,000, and 30,000 psi). The cell disruption efficiency did not improve when increasing the pressure intensity, but the number of passages significantly improved this parameter. Since the maximum percentage of disrupted cells was about 91% for 3 passages and the three pressures applied, the recommended pressure was 10,000 psi.
Although bead milling and high-pressure homogenization have shown high efficiency for cell disruption, nonmechanical methods are highly relevant since they may be more energy-efficient and represent a more economical alternative. Although enzymes are cost-intensive, they have been used in the cell disruption of H. pluvialis and P. rhodozyma due to their high specificity to the cell wall substrates of these microorganisms. A combination of various types of enzymes, instead of just one type, acts simultaneously and leads to a more effective N-ASX release. It has been reported that the presence of β-1,3-glucanase, protease, cellulose, and pectinase gives high cell disruption and high N-ASX extraction yields in H. pluvialis and P. rhodozyma (Harith et al., 2020; Ye et al., 2020).
Ionic liquids (ILs) are recently being used in N-ASX extraction, and they are considered as a real alternative to mechanical cell disruption methods (Choi et al., 2019; Liu et al., 2019b; Tan et al., 2020). ILs are salts comprised of relatively large asymmetric organic cations coupled with smaller inorganic or organic anions. Due to several properties such as high hydrolysis activity, remarkable solubilizing capacity for various organic and inorganic compounds, high conductivity, low volatility, low viscosity, and high thermal and chemical stabilities, ILs are considered “green” solvents. Furthermore, ILs can be specifically formulated to suit precise conditions and to solubilize particular compounds by altering their functional groups. They can be recycled for several cycles without any previous treatment and a reduction in efficiency. When using ILs, the concentration, temperature, processing time, and type of ILs are important parameters to consider. Choi et al. (2019) evaluated ten types of 1-ethyl-3-methylimidazolium [Emim]-based ILs at different temperatures, concentrations, and processing times to disrupt H. pluvialis cell walls [Emim] HSO4, [Emim] CH3SO3, and [Emim] (CF3SO2)2N which were selected and their optimized concentration for cell disruption and N-ASX extraction of about 100% were 26.7% (v/v), 20.0% (v/v), and 26.7% (v/v), respectively. The optimized temperature and processing times were 30 °C and 60 min for the three [Emim]-based ILs. At the same time, ILs have shown superior performance against other nonmechanical cell disruption methods. Ye et al. (2020) compared three types of ILs, cellulase, pectinase, and cellulase/pectinase in proportions 1:1, 1:2, and 2:1, and HCl 2, 4, and 6 M for the breakdown of H. pluvialis cells. The highest N-ASX extraction (86.71%) was achieved with 1-butyl-3-Methylimidazolium chloride [Bmim] Cl, while pectinase/cellulase at ratio 1:1 reached 71.0%, and HCl at 4 M reached 80.52%. Electron microscopy and transmission electron microscopy showed that all treatments hydrolyzed cellulase, hemicellulose, and lignin in the cell wall.
Extraction With Conventional Solvents
During N-ASX extraction, the largest amount of the carotenoid is obtained by using a two-step process. The first step involves cell disruption, whereas the second step involves organic solvent extraction. This strategy guarantees a high N-ASX recovery. Irshad et al. (2019) mention that if cyst cells of H. pluvialis are entirely disrupted, medium-to-high recovery of N-ASX is obtained 8–23 mg/g in the solvent extraction process. However, the two-step approaches are high-energy and time-consuming. Simultaneous cell disruption and extraction have been introduced as a new one-step approach that can be a cheaper alternative for N-ASX extraction. In solvent extraction, the solvent must be carefully chosen as it can be flammable, volatile, and often toxic. The use of green solvent, produced from renewable resources or environmentally benign, is strongly recommended (Saini and Keum, 2018). In the case of N-ASX extraction, ethanol, acetone, hexane, isopropyl alcohol, dichloromethane, and dimethyl sulfoxide (DMSO) are the most used, and the FDA classifies them as low risk to human health; they are approved, under certain limits, for their use in pharmaceuticals (FDA, 2020). The solvents’ effectiveness is related to their polarity and their capacity to permeate through the cell wall and cell membrane (Harith et al., 2019). Harith et al. (2019) compared ethanol, methanol, DMSO, and acetone, combined with simple glass beads disruption, in N-ASX extraction from P. rhodozyma. Although N-ASX was shown to have a lower solubility in acetone than in DMSO, the extraction efficiency was 95% and 40%, respectively. The authors mentioned that N-ASX exhibited superior solubility in some of the solvents; however, these solvents could not permeate through the cell wall and cell membrane and failed to extract the carotenoid attached to these structures. Irshad et al. (2019) carried out the N-ASX extraction from H. pluvialis using a ball mill grinding in the presence of different solvents at rotational speeds of 100–200 rpm, time intervals of 5–30 min, and temperature of 25°C. N-ASX recovery of ˃98% was achieved at 200 rpm, 30 min, with ethanol, acetone, and ethyl acetate. The extraction time for the one-step process (simultaneous wet grinding and solvent extraction) was 30 min, while the two-step process (dried biomass grinding and subsequent solvent extracting) took 60 min for maximum recovery of the carotenoid.
Since Gram-negative cells are more fragile than yeast and alga cells, the use of a solvent can be enough to disrupt E. coli and P. carotinifaciens and extract N-ASX in a single step. In the case of E. coli, the use of acetone at 55 °C (Lu et al., 2017; Li and Huang, 2018; Park et al., 2018) is sufficient for cell disruption and extraction, whereas ethanol at 60 °C is widely used for extraction from P. carotinifaciens cells (Honda et al., 2020a). Currently, there is little information about studies on N-ASX solvent extraction from bacteria, so further research should be generated in this area. It is of great interest to study different types of solvent, concentration, processing time, and simultaneous use of solvents with other techniques, and optimize these parameters. The use of conventional solvent extraction offers a simple approach; however, low efficiency (extraction yield), selectivity (purity), high solvent consumption, and long treatment times show the need to look for new extraction alternatives (Poojary et al., 2016).
Nonconventional Extraction Methods
Alternatively to conventional solvent extraction, pressurized liquid extraction (PLE) is a technique where the solvents are applied at high temperatures and high pressures. The solvents are maintained in liquid states and penetrate easier into the cellular matrix, with that the solubility and extraction of N-ASX significantly increased. High temperatures may affect the thermolabile carotenoids’ stability, so an increase in temperature must be done with great care (Gallego et al., 2019). Gallego et al. (2020) applied PLE in the presence of sea sand and pure ethanol at different temperatures, 10 MPa, and 20 min. The maximum concentration of total carotenoids 91.57 mg/g extract was achieved at 50 °C, while at temperatures higher than 100 °C, degradation of carotenoids was observed. Xiao et al. (2009) compared the use of HCl at 0.5 M under autoclave conditions (121 °C) for 5 min with HCl at 5M, 30 °C for 30 min in P. rhodozyma cells. The use of autoclave conditions led to a superior N-ASX extractability (84.8%) in much less time compared to high acid concentration treatment (only 72.5% extractability). Besides, N-ASX degradation was decreased by the short-time exposure to autoclave high temperatures.
In supercritical fluid extraction (SFE), the gas and liquid states of fluid cannot be differentiated under specific pressure and temperature conditions (critic points). A supercritical fluid presents high permeability, diffusion, and penetrates rapidly into the pores of complex matrices. SC-CO2 is a relatively new technology applied in N-ASX extraction, and it has become the most used technique in the large-scale production of this carotenoid (Panis and Carreon, 2016; Li et al., 2020b). SC-CO2 diffuses more efficiently through the cells than conventional organic solvents. It operates under mild temperatures (40–60 °C), which avoids the degradation of the compound and results in a solvent-free final product, easily separated from the N-ASX in the depressurizing process. SC-CO2 is not flammable and is regarded as a safe solvent (Molino et al., 2020). Since SC-CO2 is a nonpolar solvent, generally, a cosolvent is used to increase extraction efficiency due to the low solubility of N-ASX in nonpolar solvents. Ethanol is mostly used for this purpose in SC-CO2. This alcohol is considered GRAS without any concentration limits (Molino et al., 2018a). When using SFE, the main operational conditions that affect the extract composition are temperature, pressure, type and concentration of cosolvent, solvent flow rate, and extraction time. The main limitation of SFE is the high cost of the equipment. Also, the high operational pressure (50 MPa or more) increases the operational cost (Reyes et al., 2014; Saini and Keum, 2018; Molino et al., 2020; Li et al., 2020b). Molino et al. (2018a) evaluated the use of SC-CO2 in H. pluvialis extraction under different parameters, namely, the extraction time, temperature (50, 65, and 80 °C), pressure (100, 400, and 550 bars), and ethanol as a cosolvent. Temperature and pressure significantly affected the carotenoid extraction, while ethanol concentration did not influence the N-ASX extraction. The highest N-ASX recovery 18.5 mg/g (92%) was reported at 65 °C, 550 bars for 20 min. Although molecule degradation occurred at 80 °C, the highest purity of 34% was found at this temperature at 440 bars, 80 min, without cosolvent. The use of SC-CO2 was recently reported in P. rhodozyma by Harith et al. (2020). The authors studied the effect of the temperature, pressure, and ethanol concentration as a cosolvent in enzymatic pretreated cells and found that both pressure and cosolvent concentration significantly influenced N-ASX extraction. Although a prediction model for the extraction process was generated, the extraction efficiency only reached 22% (w/w) under the optimized conditions (76.8 °C, 360 bars, and 18% v/v ethanol concentration). The authors argued that enzymatic pretreatment with the commercial enzyme accellerase 1,500 (cellulase, hemicellulase, and β-glucanase activities) was not enough to disrupt the yeast cells since N-ASX extraction of ∼90% has been reported in SC-CO2 using a bead mill to disrupt P. rhodozyma cells before extraction (Lim et al., 2002). SC-CO2 has also been reported in N-ASX producing bacteria by Chougle et al. (2016). These authors formulated a mathematical model, based on experimental data, to make a complete optimization of N-ASX extraction from Paracoccus sp. The parameters analyzed were pressure, temperature, particle size of biomass, time, type of cosolvent, and cosolvent concentration. Pressure and biomass particle size had a significant effect, and maximum recovery of 963.33 μg/g was obtained under optimal conditions, while recovery of 317 μg/g was obtained with conventional acetone extraction.
In ultrasound-assisted extraction (UAE), ultrasonication is combined with solvents or enzymes to enhance mass transfer and consequent disruption of the cell wall. This technique has increased N-ASX recovery in less time since the acoustic cavitation leads to cell rupture enhancing the mass transfer of extractants. It has been reported that the use of a probe (horn) sonicator instead of a bath sonicator generates a more intense and uniform vibration zone, increasing the effectiveness of the treatment. The main parameters to control when using UAE are the ultrasonic power, intensity, temperature, and sample-to-solvent ratio (Haque et al., 2016; Saini and Keum, 2018). Machado et al. (2016) extracted N-ASX from H. pluvialis cells using three commercial enzymes assisted by ultrasound. The enzymatic reaction conditions of Gucanex (β-1,3-glucanase and protease activities from Trichoderma harzianum), Driselase (β-1,3-glucanase and xylanase activities from Basidiomycetes sp.), and Lyticase (β-1,3-glucanase and protease activities from Arthrobacter luteus) were optimized. The highest extractability was 83.9 and 85.06% for unfrozen and frozen cells, respectively, using Glucanex lysis assisted with ultrasound, while 79.34 and 81.4% were achieved in unfrozen and frozen cells, respectively, without ultrasound. Urnau et al. (2018) reported a 99% (680 μg/g) and 89.32% (614.25 μg/g) carotenoid recovery in P. rhodozyma using only DMSO and UAE acetone/methanol mixture, respectively. Although extraction with DMSO was superior, the processing time was considerably reduced by 45% with ultrasound.
Pulsed electric field (PEF)-assisted extraction is a promising technique that consists of the application of repetitive short high voltages pulses to induce permeabilization (electroporation) of biological material (plant, animal, or microbial cells). This technique requires low energy expenditure, easily scalable and nonthermal, and facilitates the purification of extracted compounds since it minimizes the release of cell debris. The pulse duration and electric field strength intensity are parameters that need to be optimized for each sample since they may change with a change in the electrical conductivity and texture of the sample (Saini and Keum, 2018; Aguilar-Machado et al. (2020). It has been suggested that PEF also triggers some enzyme activity, which in turn accelerates the autolysis and the subsequent release of N-ASX. Aguilar-Machado et al. (2020) evaluated PEF treatment with electric field strength between 10 and 25 kV/cm at 0.5 Hz frequency in a wild-type and mutant P. rhodozyma. The PEF pretreatment was ineffective for N-ASX extraction with ethanol in the two yeast strains. However, 2.43 mg/g (dw) (70% extractability) of carotenoids was extracted after aqueous incubation for 12 h of PEF-treated mutant strain cells at 25 kV/cm. The extraction of N-ASX was mediated by esterase activity triggered by PEF during incubation since this enzyme activity was higher in PEF-treated mutant cells compared with no treated mutant cells.
In microwave-assisted extraction (MAE), high-frequency waves (300 MHz–300 GHz) are generated and cause dielectric heating in the sample, primarily by absorption of water and other energy polar compounds present in wet biomass, resulting in temperature increases in the intracellular liquids, subsequently causing the water to evaporate and exert pressure on the cell wall leading to cell disruption (Kapoore et al., 2018). MAE’s main advantages are significantly decreased extraction time and a single-step process that combines cell disruption and compound extraction (Zhao et al., 2016; Fan et al., 2019). The irradiation power, irradiation time, and the number of cycles are the most important parameters when using this technology. Fan et al. (2019) used MAE with three protic ILs (PILs) to extract N-ASX from H. pluvialis. Ethanolammonium caproate (EAC) was selected, and the extraction efficiency of ∼35 mg/g was obtained at 210 W, 5.2 mol/L EAC concentration, two extraction cycles (50 s each), and liquid-solid ratio of 20 g/g. The short extraction time made this strategy very relevant for large-scale implementation. Furthermore, the authors listed other PIL advantages: low cost, easy synthesis, and low toxicity. Zhu et al. (2010) selected the frequency of 416.5 MHz MAE in the presence of DMSO to break the cells of P. rhodozyma and extract N-ASX in ethanol. They reported a 22% yield increase upon optimization of cell breaking time, ethanol volume, and extraction time.
It has been reported that N-ASX Z-isomers exhibit higher bioavailability and antioxidant activity than the all-E-isomers. Thus, the obtention of Z-isomers is of great interest for pharmaceutical use. The conversion of all-E-isomers to Z-isomers can be achieved by thermal treatment and different lighting and solvents (Yang et al., 2017). Besides, N-ASX Z-isomers are superbly soluble in ethanol than all-E-isomers (∼700 times more), which further facilitate the extraction (Honda et al., 2018). Honda et al. (2020a) compared different electrophilic compounds to enhance the extraction and thermal Z-isomerization of all-E-carotenoids from dried cell powder of P. carotinifaciens. The N-ASX extraction increased 27% compared to the control when allyl isothiocyanate (isomerization catalyst), from the Brassicaceae plant family, was used at 100 mM along with ethanol at 60 °C for 1 h. Also, the presence of the catalyst increased the content of N-ASX Z-isomer by 125%. The use of allyl isothiocyanate represented an efficient and environmentally friendly method for the extraction and Z-isomerization of the carotenoid.
New fermentation strategies are being explored to obtain N-ASX without any pretreatment of the cell (cell recovery, cell disruption, and cell drying). In extractive fermentation, some compounds allow a selective and continuous extraction of the product from the live cells, which preserve their integrity and productivity (Samorì et al., 2019). Li et al. (2020a) reported the use of Yarrowia lipolytica in a fermentation process with 70% of sunflower oil as a sole carbon source. The yeast produced N-ASX at a 167 mg/L concentration and secreted continuously up to 48% of the total product to the medium. In a similar strategy, cells are “milked” and reused. Samorì et al. (2019) reported almond oil and cyclohexane for N-ASX extraction from H. pluvialis. The oil and the solvent were compatible with the alga and kept it alive; the cells maintain their photosynthetic activity and produced the carotenoid again when the fresh medium was added. Praveenkumar et al. (2015) reported using a gold nanoscalpel to make an incision in a cell of H. pluvialis and extract the N-ASX. The cell healed the wound and reaccumulated the carotenoid, even twice as fast as the control. In another approach, germination of the cyst cells of H. pluvialis was induced, and the zoospores containing N-ASX were released. The product was extracted directly from the broth using ethyl acetate (Bauer and Minceva, 2019).
Drying
Once the cells have been disrupted, a drying process is necessary to prevent unstable N-ASX degradation before extraction and extend the shelf life. An ideal strategy would be to extract the carotenoid from the wet material obtained after cell disruption, avoiding the drying operation. However, in large-scale production, this strategy may be challenging to achieve. On the other hand, the drying operation may be used in the formulation process, as will be seen later.
Despite high operation and investment costs, spray drying is the most used technique in large-scale N-ASX production (Li et al., 2011, Li et al. 2020b; Panis and Carreon, 2016; Zgheib et al., 2018; Dursun et al., 2020). In this process, a solution, suspension, or emulsion is converted to a dried powder in a single step by passing an atomized spray through a high-temperature gas (Ziaee et al., 2019). The inlet temperature and the drying chamber’s outlet temperature are critical parameters in a spray drier equipment and must be carefully selected since degradation of the N-ASX molecule may occur due to high temperatures. Raposo et al. (2012) dried a cell suspension of H. pluvialis by spray drying. The inlet/outlet temperatures tested were 220/120 °C, 220/80 °C, 180/110 °C, and 180/80 °C. The cells resisted the drying process, and N-ASX degradation occurred at different storage conditions. The authors found that powders with higher water content were more stable, and N-ASX degradation was slower. The drying process at 180 °C (inlet) and 110 °C (outlet) produced the most stable N-ASX powder, and less than 10% of the carotenoid was degraded after 9 weeks stored at −21 °C under nitrogen. Up to 80% of the molecule was degraded in nine weeks when the powder (obtained at 180 °C (inlet) and 80 °C (outlet)) was stored at 21 °C under N2. Young et al. (2017) obtained powder of disrupted cells of H. pluvialis when operating a spray drier at temperatures 220 °C (inlet) and 140 °C (outlet), airflow 62 m3/h, pump flow 175 ml/h, and compressor pressure of 0.4–0.7 bars. Astatine, which is an indicator of oxidative damage in N-ASX, was not detected after drying. The stability of the products was measured at −20 and 4 °C in the presence or absence of N2, and up to ∼90% of N-ASX was retained over 16 weeks at −20 °C under N2. Xiao et al. (2009) used spray drying in P. rhodozyma at temperatures of 170 °C (inlet) and 68 °C (outlet). When calculating the relative residual N-ASX percentage, 39% of the molecule was degraded by the drying process. An and Choi, (2003) studied the N-ASX degradation after P. rhodozyma cell disruption with 0.2 M HCl at 90 °C for 8 h and spray drying at temperatures of 150 °C (inlet) and 85 °C (outlet). The percentage of degradation was 11.2% when the cell disrupted suspension was adjusted to pH 3 with NaOH, while being 17% when the suspension was at pH 7. The authors suggested that a higher NaOH degrades the carotenoid to astacin when neutralizing the suspension to pH 7. The use of spray drying in N-ASX produced in E. coli has also been reported by Lin et al. (2017). In this study, the cells were spray-dried at 120–150 °C (inlet temperature); the carotenoid was extracted with ethanol and crystallized. The crystals contained 79.5% of N-ASX and showed no toxicity on a rodent model.
Alternatively, freeze-drying, also known as lyophilization, is a widely used technique in N-ASX-producing cells. It involves freezing the material at −20 °C for dehydration, so it is milder than the high temperature in spray drying (180–220 °C). However, freeze-drying is more expensive in large-scale industrial processes (Khoo et al., 2019b). Ahmed et al. (2015) compared freeze-drying with spray drying in H. pluvialis cells. The authors reported that, after the microalgae’s drying process, the total N-ASX extracted with acetone was 6,454.9 μg/g in freeze-dried powder, while in spray-dried powder, it was only 4,578.6 μg/g. The powders obtained were stored under vacuum or in air at −20, 4, 20, and 37 °C, and it was showed that higher temperature and air storage accelerate N-ASX degradation. The freeze-dried N-ASX powder stored at −20 °C under vacuum showed higher stability, with only 12.3% degradation of the total carotenoid in 20 weeks of storage. Although freeze-drying is more expensive than spray drying, a cost-benefit analysis showed that freeze-drying was a better option. The freeze-drying process was used in N-ASX drying in GMO S. cerevisiae, but unfortunately, details of the process and its effect on N-ASX stability were not reported (Ukibe et al., 2009). Freeze-drying has also been applied in P. rhodozyma by Gervasi et al. (2018) at −50 °C for 24 h and by Harith et al. (2020) at −20 °C for 24 h to preserve the cells before N-ASX extraction, but the effects of the process over the carotenoid stability were no explored.
Purification
N-ASX’s bioactivity has been extensively tested in clinical trials, and its effectiveness makes it a compound with great potential as a nutraceutical or pharmaceutical product. When preparing this kind of product, the purity of the compound must be ensured. Once the extraction of N-ASX has been completed, purification is necessary due to impurities like other carotenoids, proteins, salts, esters, cell debris, antifoams, and solvents and other contaminants. In this section, we focused on the obtention of free N-ASX and the separation of this molecule and its esters. Table 5 shows the details of recent studies about the purification processes in N-ASX.
The presence of esters in N-ASX is undesirable since they may interfere with the absorption of the compound. Mimoun-Benarroch et al. (2016) compared the bioavailability of S-ASX and N-ASX from the microalgae H. pluvialis, the bacteria P. carotinifaciens, and the yeast P. rhodozyma on Caco-2/TC7 colonic cells. In this study, S-ASX and N-ASX’s absorption from bacteria and yeast was superior to N-ASX from microalgae. The authors mentioned that esterified N-ASX, produced only in the microalgae, needs to be hydrolyzed in the intestinal lumen before absorption, which causes a decrease in the process. Also, esters' presence also interferes with the chromatographic analysis/separation since esterified isomers cannot be distinguished unless the fatty acid chains of the N-ASX are removed. When removing ester groups, the carotenoid can be separated by chromatographic technics, and free N-ASX can be linked to water-soluble groups to form antioxidant drugs useful in pharmaceuticals (Su et al., 2018). The ester groups are generally removed by saponification using NaOH solution. However, this reaction may degrade the carotenoid molecules since the hydroxyl group in N-ASX can be dehydrogenated to form astacin. Incubation time, temperature, alkali concentration, and volume for partition and washing must be controlled when using saponification (Inbaraj et al., 2008; Mei et al., 2020). N-ASX esters can be hydrolyzed by the enzymolysis with lipase. The use of cholesterol esterase (EC 3.1.1.13) from Pseudomonas fluorescens is widely used by many H. pluvialis-producing companies to cleave carotenoid esters (Su et al., 2018). Su et al. (2018) reported complete enzymolysis with cholesterol esterase and saponification with NaOH of N-ASX. The maximum free N-ASX recovery was 89.5% when using the enzyme, and no semiastacin or astacin was observed, while the recovery was only 48.40% in the presence of alkali and semiastacin presence. The isomers all-trans, 9-cis, and 13-cis from the free carotenoid were present in 84, 12, and 4%, respectively.
Once the ester groups have been eliminated, free N-ASX and its isomers must be separated and purified. Several studies have reported using normal-phase chromatography and reverse-phase chromatography (C18 column) for this purpose (Wang et al., 2008; Lafountain et al., 2013). Honda et al. (2020a) determined the conditions for separating N-ASX isomers from P. carotinifaciens by normal-phase HPLC. The best peaks separation was obtained in columns at 40 °C with silica gel and a mobile phase of hexane/ethyl acetate/acetone (70:20:10, v/v/v) at 1.2 ml/min. Yang et al. (2017) obtained N-ASX Z-isomers (9 and 13) from N-ASX all-E-isomers by using iodine-doped titanium dioxide (I-TiO2) as the catalyst. The isomers were purified by HPLC on a Kinetex XB-C18 column (reverse-phase chromatography), and 22.7% and 16.9% of 9Z and 13Z isomers were obtained, respectively. The purity of 9Z isomer was 95.3%, while the purity of 13Z isomer was 91.8%. Besides, Z-isomers were stable between pH 3.5 and 11.6 and in the presence of metal ions.
The purification process by chromatography is a scalable and straightforward operation. Sun et al. (2015) reported a general scheme for N-ASX purification from H. pluvialis. The crude extract of the carotenoid, obtained from the extraction process, was treated with 0.02 M NaOH at low temperatures. Liquid-liquid extraction (water/n-hexane) to remove alkali and chlorophyll impurities was carried out before the purification. The free N-ASX extract was passed through low-pressure silica gel (300–400 mesh) filled column chromatography (liquid-solid chromatography) for about 90 min, the carotenoid solution was crystallized, and the HPLC analysis showed a ˃96.5% purity. The author mentioned that low-pressure column chromatography (LPCC) is a low-cost option and highly scalable in comparison to other chromatography techniques like high-speed countercurrent chromatography (HSCCC) and reverse-phase HPLC (RP-HPLC) used in this carotenoid purification. When using liquid-solid chromatography, a large load capacity of the solid phase needs to be ensured. Casella et al. (2020) evaluated the commercial activated carbon Darco G-60 (100–325 mesh) in N-ASX’s purification from H. pluvialis. After accelerated solvent extraction at 100 bar, 67 °C with ethanol, the sample was subjected to chromatography in a column filled with 50, 100, and 200 mg of the commercial product at a flow rate of ∼1.0 ml/min. The adsorption capacity of the activated carbon was high with a value of 22.6 mg/g, and the method represented an economical option for carotenoid purification.
HSCCC is a liquid-liquid method based on the partitioning of compounds between two immiscible liquid phases without solid support. The advantages of this technique are reversible adsorption, low risk of sample denaturation, large load capacity, and excellent sample recovery (Zhao et al., 2015; Du et al., 2016). Du et al. (2016) selected the two-phase solvent system used in HSCCC based on a partition coefficient value (k) for N-ASX in the range of 0.5–2.0. The system n-hexane-acetone-ethanol-water (1:1:1:1, v/v/v/v) was selected since it presented a k value of 1.408 and the best peak resolution for N-ASX. The crude extract, obtained by solvent extraction, was subjected to HSCC and the upper phase (n-hexane and a spot of acetone, ethanol, and water) was used as the mobile phase and the lower phase (acetone, ethanol, water, and a spot n-hexane) as the stationary phase. The separation yield was 20.6 mg/L at 92.0% purity, and by further one-step silica gel chromatography, the purity on N-ASX from P. rhodozyma was 99.0%. Some reports claim that esterified N-ASX has better or equal pharmacological properties than free N-ASX, which is also unstable and susceptible to oxidation (Rao et al., 2015; Régnier et al., 2015). Due to this, some studies have focused on the purification of the esterified N-ASX. Fábryová et al. (2020) purified five esterified N-ASX molecules by high-performance counterchromatography (HPCCC), where n-heptane and acetonitrile (ratio 5:5) were used as the mobile phase in the lower phase of a biphasic solvent system, and a final purification step was carried out using semipreparative HPLC. The yields of the purified compounds were between 1 and 12 mg, exhibiting purities over 98%. Moreover, the purified esterified N-ASX oleic acid monoester had a cytotoxic effect against human gastric cancer cells.
In supercritical antisolvent fractionation (SAF), the SC-CO2 is in continuous contact with a polar liquid mixture (solvent + compounds extracted from a natural matrix) in a pressurized precipitation vessel. The supercritical fluid dissolves the polar solvent and the compounds soluble in the mixture SC-CO2/solvent, selectively precipitating heavier or more polar compounds that will not be soluble in the fractionation medium (Gonzalez-Coloma et al., 2012). Gallego et al. (2020) reported this technique, coupled with two-dimensional liquid chromatography for H. pluvial in N-ASX purification. The authors extracted N-ASX with pressurized ethanol, and after optimization of SAF parameters, an enriched extract with a value of 103.3 mg/g N-ASX was obtained by using 30 MPa CO2 pressure, 0.05 PLE extract/SC-CO2 mass flow rate ratio, and 20% (v/v) of water in the feed solution. The use of normal-phase chromatography in the first dimension and reverse-phase chromatography in the second dimension allowed the separation of N-ASX monoester, diesters, and free N-ASX in just 25 min.
Formulation
The formulation of N-ASX is the final step in the obtention of the nutraceutical or pharmaceutical products. Significant efforts have been carried out to obtain stable formulations containing bioactive molecules of the carotenoid. Since N-ASX quickly decomposes when exposed to light, oxygen, and/or heat and is poorly soluble in water, there is a need to obtain a formulation that minimizes these problems. Many reports have focused on the encapsulation process to enhance N-ASX stability and bioavailability. This procedure may protect the carotenoid from the acidity of gastric juice while allowing its subsequent release in the intestinal fluids (Liu et al., 2018a). Encapsulation is a process for trapping solids, liquids, or gaseous materials within a continuous film coating from another substance, producing particles with different diameters (Ozkan et al., 2019). According to their size, the capsules can be classified into macrocapsules (˃5,000 µm), microcapsules (1–5,000 µm), and nanocapsules (<1 µm); the last two are of interest for medical therapy. On the other hand, capsules can be divided into microcapsules and microspheres according to their shape and construction. Microcapsules are formed by an inner core (nuclei) that contains the active substance, covered with a layer of polymer, while in microspheres, the nucleus is dispersed and dissolved uniformly inside a polymer matrix (Santos-Sánchez et al., 2020).
Conventional encapsulating techniques used in N-ASX formulation include spray drying, lyophilization, solvent evaporation, coacervation, liposomes, and ionic gelation (Dorđević et al., 2014; Ozkan et al., 2019). In spray drying, the feed liquid may be a solution and emulsion or a suspension. Typical wall materials are polysaccharides and proteins. The characteristics of spray-dried powders are related to the factors discussed in Drying, as well as the sort of carrier agent and its concentration (Fatnassi et al., 2013). Freeze-drying has been used in many heat-sensitive antioxidants due to its low operating temperature; however, this technique characterizes by long process time (20 h or more), and the powders must be grounded after the drying process (Anwar and Kunz, 2011). In solvent evaporation, an emulsion of a polymer, active compound, organic solvent, and aqueous phase is prepared, and the solvent is removed by evaporation or liquid extraction. The particles are recovered and dried (Tiwari and Verma, 2011). Coacervation is a colloidal phenomenon that involves liquid-liquid phase separation of a single polymer (simple coacervation) or a mixture of two oppositely charged polymers (complex coacervation) in an aqueous solution triggered by physical forces, chemical interactions, or enzymatic cross-linker agents (Xiao et al., 2014). Liposomes are vesicles with single or multiple bilayers (principally phospholipids) with a hydrophilic head and hydrophobic tail groups. They are obtained by the dispersion of phospholipids in an aqueous solution using sufficient energy which can be supplied by ultrasonication, high-pressure homogenization, membrane extrusion, solvent evaporation, electro formation, thin-film dehydration/rehydration, dialysis, and liposome (Mozafari et al., 2008). Ionic gelation is based on the ability to crosslink polyelectrolytes in the presence of multivalent ions such as Ca+2, Ba+2, and Al+3. In this technique, spherical gel particles are prepared by dripping an aqueous polymer solution into a CaCl2 solution or preparing an emulsion of the hydrophobic active compound in a polymer solution and dripping it into a calcium solution (Lupo et al., 2014).
According to Adschiri and Yoko (2018), conventional encapsulation techniques have some limitations, such as difficulties in controlling the particle size of the product and the necessity to include some purification steps due to the use of solvents. Supercritical fluids precipitation is a methodology recently reported for the encapsulation of N-ASX. Tirado et al. (2019) used the supercritical emulsions extraction (SEE) technology to encapsulate N-ASX in ethyl cellulose (EC). In this study, the SC-CO2 was in contact with an emulsion prepared with ethyl acetate (EA), N-ASX, EC (oily phase), and EA-saturated water with tween 80 (water phase). The oily phase solvent and CO2 were recovered at the top of the column, and nanoparticle suspension was collected at the bottom of the tank. Different emulsions were prepared by varying EC concentrations in the oily phase and surfactant concentration in the water phase, and under the best conditions, the encapsulation efficiency was 84%, and the carotenoid preserved its antioxidant activity. The authors designed a continuous operation that allowed a high production capacity. Liu et al. (2019a) also reported using this technology when preparing microspheres of N-ASX. They optimized the process altering solvent ratio, temperature, pressure, carrier concentration, and flow rate. The N-ASX was dissolved in dichloromethane, acetone, and poly (l-lactic acid) (PLLA), and the solvents were evaporated into the bulk SC-CO2. The encapsulation efficiency was 91.5%, and the mean particle size was 954.6 nm. The SC-CO2 is a new and promising technology in N-ASX encapsulation, and since CO2 is not used to solubilize the carotenoid, it can be called supercritical antisolvent technology. Table 6 shows relevant and recent reports of conventional and nonconventional N-ASX encapsulation techniques.
Future Perspectives and Conclusions
Naturally synthesized astaxanthin, N-ASX, has shown superior bioactivity than the synthetically obtained S-ASX. Furthermore, several clinical trials confirm the safety of the natural compound and its effectiveness for the prevention and treatment of several diseases. Due to this, there is considerable interest in exploiting this compound at the pharmaceutical level. The market value of N-ASX raises to USD15,00.00/kg, while S-ASX costs about USD1,000.00/kg. A comprehensive view, which includes the upstream and the downstream process, must be considered in economic feasibility analysis to establish the N-ASX biotechnological production process. Many efforts need to be made in cell recovery stages, cell disruption, drying, product extraction, purification, and formulation, to make N-ASX production competitive.
Cell harvesting techniques should focus on strategies to avoid the use of centrifugation and filtration. The use of flocculants should be extensively studied in H. pluvialis as well as its influence on the molecule purification. On the other hand, the flotation technique has shown very promising results, mostly when used with flocculation. The use of yeasts and bacteria to produce N-ASX may represent an advantage in cell recovery because many yeasts, like S. cerevisiae, Y. lipolytica, and K. marxianus strains, can flocculate. These strains should be selected when generating nonnative N-ASX producing microorganisms. Also, flocculating E. coli strains can be generated by overexpressing the native bcsB gene (involved in the synthesis of extracellular cellulose) (Ojima et al., 2016).
During the downstream process, the amount of N-ASX obtained is positively related to cell disruption and the extraction method applied since it is an intracellular metabolite. The bead milling and high-pressure homogenization have shown high cell disruption efficiency. However, high equipment investment and high operational costs are the principal disadvantages of these methods. The drying process should not be carried out after cell disruption to reduce production costs and avoid carotenoid degradation. Although SC-CO2 technology is also expensive, it is being used in the large-scale N-ASX extraction process due to its high extraction efficiency and lack of degradation of the molecule (mild operating conditions). Alternatively, assisted technologies allow cell disruption and N-ASX extraction in a single operation; they operate under mild conditions, and the processing time can be concise. These technologies have been reported along with enzymes and conventional solvents. However, their use, along with ILs, which are considered “green solvents” and have shown high cell disruption efficiency, is scarcely reported. The bioreactor’s cell culture may be treated immediately by assisted technologies for N-ASX extraction without previous cell disruption and drying. On the other hand, there is a lack of information N-ASX’s extraction from native or nonnative N-ASX-producing bacteria; therefore, specific studies must be carried out in this area.
In pharmaceutical applications, a high-purity product is required. The use of liquid-liquid chromatography combined with liquid-solid chromatography may be useful, since the processes are relatively fast, and 99% purity has been obtained. On the other hand, the stability of the molecule must be guaranteed in pharmaceuticals. Many encapsulation techniques have shown high efficiency, high bioavailability, and stability. The use of SC-CO2 was recently reported as a high-efficiency encapsulation technique; due to its high cost, this method can be replaced by conventional encapsulation methods, which are efficient and less expensive. The purification and individual formulation of free N-ASX, its esters, or isomers is an opportunity to investigate each molecule’s individual activity and stability.
Author Contribution
All authors have made a substantial, direct, and intellectual contribution to the work and approved it for publication.
Conflict of Interest
The authors declare that the research was conducted in the absence of any commercial or financial relationships that could be construed as a potential conflict of interest.
Acknowledgments
The authors thank Consejo Nacional de Ciencia y Tecnología (CONACYT) for the scholarship 002823 and Tecnológico de Monterrey School of Engineering and Sciences for its support.
References
Adschiri, T., and Yoko, A. (2018). The journal of supercritical fluids supercritical fl uids for nanotechnology. J. Supercrit. Fluids 134, 167–175. doi:10.1016/j.supflu.2017.12.033
Aguilar-Machado, D., Delso, C., Martinez, J. M., Morales-Oyervides, L., Montañez, J., and Raso, J. (2020). Enzymatic processes triggered by PEF for astaxanthin extraction from Xanthophyllomyces dendrorhous. Front. Bioeng. Biotechnol 8, 857. doi:10.3389/fbioe.2020.00857
Ahmed, F., Li, Y., Fanning, K., Netzel, M., and Schenk, P. M. (2015). Effect of drying, storage temperature and air exposure on astaxanthin stability from Haematococcus pluvialis. Food Res. Int 74, 231–236. doi:10.1016/j.foodres.2015.05.021
Algalif (2020). Algalif. Available at: https://algalif.com/ (Accessed September 25 2020).
Algatech International (2020). Algatech international. Available at: https://www.algatech.com/ (Accessed September 25 2020).
Alhattab, M., Kermanshahi-pour, A., and Su-Ling Brooks, M. (2019). Dispersed air flotation of Chlorella saccharophila and subsequent extraction of lipids—effect of supercritical CO2 extraction parameters and surfactant pretreatment. Biomass Bioenergy 127, 105297. doi:10.1016/j.biombioe.2019.105297
Ambati, R. R., Phang, S. M., Ravi, S., and Aswathanarayana, R. G. (2014). Astaxanthin: sources, extraction, stability, biological activities and its commercial applications—a review. Mar. Drugs 12, 128–152. doi:10.3390/md12010128
An, G. H, and Choi, E. S. (2003). Preparation of the red yeast, Xanthophyllomyces dendrorhous, as feed additive with increased availability of astaxanthin. Biotechnol. Lett 25, 767–771. doi:10.1023/A:1023568319114
Anwar, S. H., and Kunz, B. (2011). The influence of drying methods on the stabilization of fish oil microcapsules: comparison of spray granulation, spray drying, and freeze drying. J. Food Eng 105, 367–378. doi:10.1016/j.jfoodeng.2011.02.047
Astareal (2020). Astareal. Available at: https://astareal.com/en/ (Accessed September 25 2020).
Baroni, É. G., Yap, K. Y., Webley, P. A., Scales, P. J., and Martin, G. J. O. (2019). The effect of nitrogen depletion on the cell size, shape, density and gravitational settling of Nannochloropsis salina, Chlorella sp. (marine) and Haematococcus pluvialis. Algal Res 39, 101454. doi:10.1016/j.algal.2019.101454
Barredo, J. L., García-Estrada, C., Kosalkova, K., and Barreiro, C. (2017). Biosynthesis of astaxanthin as a main carotenoid in the heterobasidiomycetous yeast Xanthophyllomyces dendrorhous. J. Fungi 3, 44. doi:10.3390/jof3030044
Barros, A. I., Gonçalves, A. L., Simões, M., and Pires, J. C. M. (2015). Harvesting techniques applied to microalgae: a review. Renew. Sustain. Energy Rev 41, 1489–1500. doi:10.1016/j.rser.2014.09.037
Bauer, A., and Minceva, M. (2019). Direct extraction of astaxanthin from the microalgae: Haematococcus pluvialis using liquid-liquid chromatography. RSC Adv 9, 22779–22789. doi:10.1039/c9ra03263k
Beijing Ginko Group (2020). Beijing ginko group. Available at: http://bggworld.com/ (Accessed September 25 2020).
Branyikova, I., Prochazkova, G., Potocar, T., Jezkova, Z., and Branyik, T. (2018). Harvesting of microalgae by flocculation. Fermentatio 4, 1–12. doi:10.3390/fermentation4040093
Capelli, B., Bagchi, D., and Cysewski, G. R. (2013). Synthetic astaxanthin is significantly inferior to algal-based astaxanthin as an antioxidant and may not be suitable as a human nutraceutical supplement. Nutrafoods 12, 145–152. doi:10.1007/s13749-013-0051-5
Casella, P., Musmarra, D., Dimatteo, S., Chianese, S., Karatza, D., Mehariya, S., et al. (2020). Purification of astaxanthin from microalgae by using commercial activated carbon. Chem. Eng. Trans 79, 295–300. doi:10.3303/CET2079050
Chekanov, K., Lobakova, E., Selyakh, I., Semenova, L., Sidorov, R., and Solovchenko, A. (2014). Accumulation of astaxanthin by a new Haematococcus pluvialis strain BM1 from the white sea coastal rocks (Russia). Mar. Drugs 12, 4504–4520. doi:10.3390/md12084504
Chen, L., Wang, J. L., Ni, H., and Zhu, M. J. (2019). Disruption of Phaffia rhodozyma cells and preparation of microencapsulated astaxanthin with high water solubility. Food Sci. Biotechnol 28, 111–120. doi:10.1007/s10068-018-0443-9
Choi, S. A., Oh, Y. K., Lee, J., Sim, S. J., Hong, M. E., Park, J. Y., et al. (2019). High-efficiency cell disruption and astaxanthin recovery from Haematococcus pluvialis cyst cells using room-temperature imidazolium-based ionic liquid/water mixtures. Bioresour. Technol 274, 120–126. doi:10.1016/j.biortech.2018.11.082
Chougle, J. A., Bankar, S. B., Chavan, P. V., Patravale, V. B., and Singhal, R. S. (2016). Supercritical carbon dioxide extraction of astaxanthin from Paracoccus NBRC 101723: mathematical modelling study. Separ. Sci. Technol 51, 2164–2173. doi:10.1080/01496395.2016.1178288
Cilliers, J. J., and Harrison, S. T. L. (2019). Yeast flocculation aids the performance of yeast dewatering using mini-hydrocyclones. Separ. Purif. Technol 209, 159–163. doi:10.1016/j.seppur.2018.06.019
Cuellar-Bermudez, S. P., Aguilar-Hernandez, I., Cardenas-Chavez, D. L., Ornelas-Soto, N., Romero-Ogawa, M. A., and Parra-Saldivar, R. (2015). Extraction and purification of high-value metabolites from microalgae: essential lipids, astaxanthin and phycobiliproteins. Microb. Biotechnol 8, 190–209. doi:10.1111/1751-7915.12167
Cyanotech Corporation (2020). Cyanotech corporation. Available at: https://www.cyanotech.com/ (Accessed September 25 2020).
Dasgupta, D., Ghosh, D., Bandhu, S., and Adhikari, D. K. (2017). Lignocellulosic sugar management for xylitol and ethanol fermentation with multiple cell recycling by Kluyveromyces marxianus IIPE453. Microbiol. Res 200, 64–72. doi:10.1016/j.micres.2017.04.002
Davinelli, S., Nielsen, M. E., and Scapagnini, G. (2018). Astaxanthin in skin health, repair, and disease: a comprehensive review. Nutrients 10, 522. doi:10.3390/nu10040522
Di Gianvito, P., Tesnière, C., Suzzi, G., Blondin, B., and Tofalo, R. (2017). FLO5 gene controls flocculation phenotype and adhesive properties in a Saccharomyces cerevisiae sparkling wine strain. Sci. Rep 7, 10786. doi:10.1038/s41598-017-09990-9
Diao, J., Song, X., Zhang, L., Cui, J., Chen, L., and Zhang, W. (2020). Tailoring cyanobacteria as a new platform for highly efficient synthesis of astaxanthin. Metab. Eng 61, 275–287. doi:10.1016/j.ymben.2020.07.003
Do, T. T., Ong, B. N., Nguyen Tran, M. L., Nguyen, D., Melkonian, M., and Tran, H. D. (2019). Biomass and astaxanthin productivities of Haematococcus pluvialis in an angled twin-layer porous substrate photobioreactor: effect of inoculum density and storage time. Biology 8. doi:10.3390/biology8030068
Dorđević, V., Balanč, B., Belščak-Cvitanović, A., Lević, S., Trifković, K., Kalušević, A., et al. (2014). Trends in encapsulation technologies for delivery of food bioactive compounds. Food Eng. Rev 7, 452–490. doi:10.1007/s12393-014-9106-7
Du, X., Dong, C., Wang, K., Jiang, Z., Chen, Y., Yang, Y., et al. (2016). Separation and purification of astaxanthin from Phaffia rhodozyma by preparative high-speed counter-current chromatography. J. Chromatogr. B Analyt. Technol. Biomed. Life Sci 1029-1030, 191–197. doi:10.1016/j.jchromb.2016.06.042
Dursun, D., Koulouris, A., and Dalgıç, A. C. (2020). Process simulation and techno economic analysis of astaxanthin production from agro-industrial wastes. Waste Biomass Valorization 11, 943–954. doi:10.1007/s12649-018-0439-y
Edelman, R., Engelberg, S., Fahoum, L., Meyron-Holtz, E. G., and Livney, Y. D. (2019). Potato protein- based carriers for enhancing bioavailability of astaxanthin. Food Hydrocolloids 96, 72–80. doi:10.1016/j.foodhyd.2019.04.058
Eom, H., Lee, C. G., and Jin, E. (2006). Gene expression profile analysis in astaxanthin-induced Haematococcus pluvialis using a cDNA microarray. Planta 223, 1231–1242. doi:10.1007/s00425-005-0171-2
Fábryová, T., Tůmová, L., da Silva, D. C., Pereira, D. M., Andrade, P. B., Valentão, P., et al. (2020). Isolation of astaxanthin monoesters from the microalgae Haematococcus pluvialis by high performance countercurrent chromatography (HPCCC) combined with high performance liquid chromatography (HPLC). Algal. Res 49, 101947. doi:10.1016/j.algal.2020.101947
Fan, Y., Niu, Z., Xu, C., Yang, L., Chen, F., and Zhang, H. (2019). Biocompatible protic ionic liquids-based microwave-assisted liquid-solid extraction of astaxanthin from Haematococcus pluvialis. Ind. Crop. Prod 141, 111809. doi:10.1016/j.indcrop.2019.111809
Fang, N., Wang, C., Liu, X., Zhao, X., Liu, Y., Liu, X., et al. (2019). De novo synthesis of astaxanthin: from organisms to genes. Trends Food Sci. Technol 92, 162–171. doi:10.1016/j.tifs.2019.08.016
Farruggia, C., Kim, M. B., Bae, M., Lee, Y., Pham, T. X., Yang, Y., et al. (2018). Astaxanthin exerts anti-inflammatory and antioxidant effects in macrophages in NRF2-dependent and independent manners. J. Nutr. Biochem 62, 202–209. doi:10.1016/j.jnutbio.2018.09.005
Fatnassi, M., Tourné-Péteilh, C., Peralta, P., Cacciaguerra, T., Dieudonné, P., Devoisselle, J. M., et al. (2013). Encapsulation of complementary model drugs in spray-dried nanostructured materials. J. Sol. Gel Sci. Technol 68, 307–316. doi:10.1007/s10971-013-3170-y
FDA (2020). Solvents. Available at: https://www.fda.gov/media/71737/download (Accessed October 15 2020).
Feng, Z. Z., Li, M. Y., Wang, Y. T., and Zhu, M. J. (2018). Astaxanthin from Phaffia rhodozyma: microencapsulation with carboxymethyl cellulose sodium and microcrystalline cellulose and effects of microencapsulated astaxanthin on yogurt properties. LWT (Lebensm.-Wiss. & Technol.) 96, 152–160. doi:10.1016/j.lwt.2018.04.084
Gallego, R., Arena, K., Dugo, P., Mondello, L., Ibáñez, E., and Herrero, M. (2020). Application of compressed fluid-based extraction and purification procedures to obtain astaxanthin-enriched extracts from Haematococcus pluvialis and characterization by comprehensive two-dimensional liquid chromatography coupled to mass spectrometry. Anal. Bioanal. Chem 412, 589–599. doi:10.1007/s00216-019-02287-y
Gallego, R., Bueno, M., and Herrero, M. (2019). Sub- and supercritical fluid extraction of bioactive compounds from plants, food-by-products, seaweeds and microalgae—an update. TrAC Trends Anal. Chem. (Reference Ed.) 116, 198–213. doi:10.1016/j.trac.2019.04.030
Gervasi, T., Pellizzeri, V., Benameur, Q., Gervasi, C., Santini, A., Cicero, N., et al. (2018). Valorization of raw materials from agricultural industry for astaxanthin and β-carotene production by Xanthophyllomyces dendrorhous. Nat. Prod. Res 32, 1554–1561. doi:10.1080/14786419.2017.1385024
Giannaccare, G., Pellegrini, M., Senni, C., Bernabei, F., Scorcia, V., and Cicero, A. F. G. (2020). Clinical applications of astaxanthin in the treatment of ocular diseases: emerging insights. Mar. Drugs 18, 1–13. doi:10.3390/md18050239
Gogate, P. R., and Nadar, S. G. (2015). Ultrasound-assisted intensification of extraction of astaxanthin from Phaffia rhodozyma. Indian Chem. Eng 57, 240–255. doi:10.1080/00194506.2015.1026947
Gomes, T. A., Zanette, C. M., and Spier, M. R. (2020). An overview of cell disruption methods for intracellular biomolecules recovery. Prep. Biochem. Biotechnol 50, 635–654. doi:10.1080/10826068.2020.1728696
Gonzalez-Coloma, A., Martín, L., Mainar, A. M., Urieta, J. S., Fraga, B. M., Rodríguez-Vallejo, V., et al. (2012). Supercritical extraction and supercritical antisolvent fractionation of natural products from plant material: comparative results on Persea indica. Phytochemistry Rev 11, 433–446. doi:10.1007/s11101-012-9267-z
Guerin, M., Huntley, M. E., and Olaizola, M. (2003). Haematococcus astaxanthin: applications for human health and nutrition. Trends Biotechnol 21, 210–216. doi:10.1016/S0167-7799(03)00078-7
Gulden, S. J., Riedele, C., Kopf, M. H., and Nirschl, H. (2020). Potential of flotation as alternative separation process in biotechnology with focus on cost and energy efficiency. Chem. Eng. Sci 218. doi:10.1016/j.ces.2019.07.035
Ha, H. K., Rankin, S. A., Lee, M. R., and Lee, W. J. (2019). Development and Characterization of Whey protein-based nano-delivery systems: a review. Molecules 24, 3254. doi:10.3390/molecules24183254
Hagen, C., Siegmund, S., and Braune, W. (2002). Ultrastructural and chemical changes in the cell wall of Haematococcus pluvialis (Volvocales, Chlorophyta) during aplanospore formation. Eur. J. Phycol 37, 217–226. doi:10.1017/S0967026202003669
Haque, F., Dutta, A., Thimmanagari, M., and Chiang, Y. W. (2016). Intensified green production of astaxanthin from Haematococcus pluvialis. Food Bioprod. Process 99, 1–11. doi:10.1016/j.fbp.2016.03.002
Harith, Z. T., de Andrade Lima, M., Charalampopoulos, D., and Chatzifragkou, A. (2020). Optimised production and extraction of astaxanthin from the yeast Xanthophyllomyces dendrorhous. Microorganisms 8, 430. doi:10.3390/microorganisms8030430
Harith, Z. T., Charalampopoulos, D., and Chatzifragkou, A. (2019). Rapeseed meal hydrolysate as substrate for microbial astaxanthin production. Biochem. Eng. J 151, 107330. doi:10.1016/j.bej.2019.107330
Hasan, M., Azhar, M., Nangia, H., Bhatt, P. C., and Panda, B. P. (2016). Influence of high-pressure homogenization, ultrasonication, and supercritical fluid on free astaxanthin extraction from β-glucanase-treated Phaffia rhodozyma cells. Prep. Biochem. Biotechnol 46, 116–122. doi:10.1080/10826068.2014.995807
Hasunuma, T., Takaki, A., Matsuda, M., Kato, Y., Vavricka, C. J., and Kondo, A. (2019). Single-stage astaxanthin production enhances the nonmevalonate pathway and photosynthetic central metabolism in Synechococcus sp. PCC 7002. ACS Synth. Biol 8, 2701–2709. doi:10.1021/acssynbio.9b00280
Henke, N. A., Heider, S. A., Peters-Wendisch, P., and Wendisch, V. F. (2016). Production of the marine carotenoid astaxanthin by metabolically engineered Corynebacterium glutamicum. Mar. Drugs 14. doi:10.3390/md14070124
Henke, N. A., and Wendisch, V. F. (2019). Improved astaxanthin production with Corynebacterium glutamicum by application of a membrane fusion protein. Mar. Drugs 17. doi:10.3390/md17110621
Higuera-Ciapara, I., Félix-Valenzuela, L., and Goycoolea, F. M. (2006). Astaxanthin: a review of its chemistry and applications. Crit. Rev. Food Sci. Nutr 46, 185–196. doi:10.1080/10408690590957188
Honda, M., Kageyama, H., Hibino, T., Sowa, T., and Kawashima, Y. (2020a). Efficient and environmentally friendly method for carotenoid extraction from Paracoccus carotinifaciens utilizing naturally occurring Z-isomerization-accelerating catalysts. Process Biochem 89, 146–154. doi:10.1016/j.procbio.2019.10.005
Honda, M., Kodama, T., Kageyama, H., Hibino, T., Wahyudiono, H., et al. (2018). Enhanced solubility and reduced crystallinity of carotenoids, β-carotene and astaxanthin, by Z-isomerization. Eur. J. Lipid Sci. Technol 120, 1800191. doi:10.1002/ejlt.201800191
Honda, M., Sowa, T., and Kawashima, Y. (2020b). Thermal- and photo-induced isomerization of all-E- and Z-isomer-rich xanthophylls: astaxanthin and its structurally-related xanthophylls, adonirubin, and adonixanthin. Eur. J. Lipid Sci. Technol 122, 1–9. doi:10.1002/ejlt.201900462
Hussein, G., Sankawa, U., Goto, H., Matsumoto, K., and Watanabe, H. (2006). Astaxanthin, a carotenoid with potential in human health and nutrition. J. Nat. Prod 69, 443–449. doi:10.1021/np050354
Inbaraj, B. S., Lu, H., Hung, C. F., Wu, W. B., Lin, C. L., and Chen, B. H. (2008). Determination of carotenoids and their esters in fruits of Lycium barbarum Linnaeus by HPLC-DAD-APCI-MS. J. Pharmaceut. Biomed. Anal 47, 812–818. doi:10.1016/j.jpba.2008.04.001
Irshad, M., Myint, A. A., Hong, M. E., Kim, J., and Sim, S. J. (2019). One-Pot, Simultaneous cell wall disruption and complete extraction of astaxanthin from Haematococcus pluvialis at room temperature. ACS Sustain. Chem. Eng 7, 13898–13910. doi:10.1021/acssuschemeng.9b02089
Jin, J., Wang, Y., Yao, M., Gu, X., Li, B., Liu, H., et al. (2018). Astaxanthin overproduction in yeast by strain engineering and new gene target uncovering. Biotechnol. Biofuels 11, 230. doi:10.1186/s13068-018-1227-4
Kanwugu, O. N., Shatunova, S. A., Glukhareva, T. V., and Kovaleva, E. G. (2020). Effect of different sugar sources on P. rhodozyma Y1654 growth and astaxanthin production. Agron. Res 18, 1700–1716. doi:10.15159/AR.20.117
Kapoore, R., Butler, T., Pandhal, J., and Vaidyanathan, S. (2018). Microwave-assisted extraction for microalgae: from biofuels to biorefinery. Biology 7, 18. doi:10.3390/biology7010018
Katsumata, T., Ishibashi, T., and Kyle, D. (2014). A sub-chronic toxicity evaluation of a natural astaxanthin-rich carotenoid extract of Paracoccus carotinifaciens in rats. Toxicol. Reports 1, 582–588. doi:10.1016/j.toxrep.2014.08.008
Keynatura, (2020). Keynatua. Available at: http://keynatura.com (Accessed September 25 2020).
Khoo, K. S., Chew, K. W., Ooi, C. W., Ong, H. C., Ling, T. C., and Show, P. L. (2019a). Extraction of natural astaxanthin from Haematococcus pluvialis using liquid biphasic flotation system. Bioresour. Technol 290, 121794. doi:10.1016/j.biortech.2019.121794
Khoo, K. S., Lee, S. Y., Ooi, C. W., Fu, X., Miao, X., Ling, T. C., et al. (2019b). Recent advances in biorefinery of astaxanthin from Haematococcus pluvialis. Bioresour. Technol 288, 121606. doi:10.1016/j.biortech.2019.121606
Kim, J. H., Park, J. J., Lee, B. J., Joo, M. K., Chun, H. J., Lee, S. W., et al. (2016). Astaxanthin inhibits proliferation of human gastric cancer cell lines by interrupting cell cycle progression. Gut Liver 10, 369–374. doi:10.5009/gnl15208
Kim, S. H., Lim, J. W., and Kim, H. (2019). Astaxanthin prevents decreases in superoxide dismutase 2 level and superoxide dismutase activity in. J. Cancer Prev 24, 54–58. doi:10.15430/jcp.2019.24.1.54
Koller, M., Muhr, A., and Braunegg, G. (2014). Microalgae as versatile cellular factories for valued products. Algal Res 6, 52–63. doi:10.1016/j.algal.2014.09.002
Kucsera, J., Pfeiffer, I., and Takeo, K. (2000). Biology of the red yeast Xanthophyllomyces dendrorhous (Phaffia rhodozyma). Mycoscience 41, 195–199.
Lafountain, A. M., Frank, H. A., and Prum, R. O. (2013). Carotenoids from the crimson and maroon plumages of old world orioles (Oriolidae). Arch. Biochem. Biophys 539, 126–132. doi:10.1016/j.abb.2013.07.001
Lane, M. M., and Morrissey, J. P. (2010). Kluyveromyces marxianus: a yeast emerging from its sister’s shadow. Fungal Biol. Rev 24, 17–26. doi:10.1016/j.fbr.2010.01.001
Li, S., and Huang, J. C. (2018). Assessment of expression cassettes and culture media for different Escherichia coli strains to produce astaxanthin. Nat. Products Bioprospect 8, 397–403. doi:10.1007/s13659-018-0172-z
Li, J., Zhu, D., Niu, J., Shen, S., and Wang, G. (2011). An economic assessment of astaxanthin production by large scale cultivation of Haematococcus pluvialis. Biotechnol. Adv 29, 568–574. doi:10.1016/j.biotechadv.2011.04.001
Li, N., Han, Z., O’Donnell, T. J., Kurasaki, R., Kajihara, L., Williams, P. G., et al. (2020a). Production and excretion of astaxanthin by engineered Yarrowia lipolytica using plant oil as both the carbon source and the biocompatible extractant. Appl. Microbiol. Biotechnol 104, 6977–6989. doi:10.1007/s00253-020-10753-2
Li, X., Wang, X., Duan, C., Yi, S., Gao, Z., Xiao, C., et al. (2020b). Biotechnological production of astaxanthin from the microalga Haematococcus pluvialis. Biotechnol. Adv 43, 107602. doi:10.1016/j.biotechadv.2020.107602
Lim, G. B., Lee, S. Y., Lee, E. K., Haam, S. J., and Kim, W. S. (2002). Separation of astaxanthin from red yeast Phaffia rhodozyma by supercritical carbon dioxide extraction. Biochem. Eng. J 11, 181–187. doi:10.1016/S1369-703X(02)00023-2
Lin, Y. J., Lin, J. Y., Wang, D. S., Chen, C. H., and Chiou, M. H. (2017). Safety assessment of astaxanthin derived from engineered Escherichia coli K-12 using a 13-week repeated dose oral toxicity study and a prenatal developmental toxicity study in rats. Regul. Toxicol. Pharmacol 87, 95–105. doi:10.1016/j.yrtph.2017.05.003
Liu, B. J., van der Meer, J. P., Zhang, L., and Zhang, Y. (2017). “Cultivation of Haematococcus pluvialis for astaxanthin production,” in Microalgal production for biomass and high-value products. Editors S. P. Solocombe, and J. R. Benemann (Boca Raton, FL, United States: CRC Press), 267–294.
Liu, G., Hu, M., Zhao, Z., Lin, Q., Wei, D., and Jiang, Y. (2019a). Enhancing the stability of astaxanthin by encapsulation in poly (l-lactic acid) microspheres using a supercritical anti-solvent process. Particuology 44, 54–62. doi:10.1016/j.partic.2018.04.006
Liu, W., Wang, J., McClements, D. J., and Zou, L. (2018a). Encapsulation of β-carotene-loaded oil droplets in caseinate/alginate microparticles: enhancement of carotenoid stability and bioaccessibility. J. Funct. Foods 40, 527–535. doi:10.1016/j.jff.2017.11.046
Liu, X., Chen, X., Liu, H., and Cao, Y. (2018b). Antioxidation and anti-aging activities of astaxanthin geometrical isomers and molecular mechanism involved in Caenorhabditis elegans. J. Funct. Foods 44, 127–136. doi:10.1016/j.jff.2018.03.004
Liu, X., Luo, Q., Rakariyatham, K., Cao, Y., Goulette, T., Liu, X., et al. (2016). Antioxidation and anti-ageing activities of different stereoisomeric astaxanthin in vitro and in vivo. J. Funct. Foods 25, 50–61. doi:10.1016/j.jff.2016.05.009
Liu, Z. W., Yue, Z., Zeng, X. A., Cheng, J. H., and Aadil, R. M. (2019b). Ionic liquid as an effective solvent for cell wall deconstructing through astaxanthin extraction from Haematococcus pluvialis. Int. J. Food Sci. Technol 54, 583–590. doi:10.1111/ijfs.14030
Liu, Z. W., Zeng, X. A., Cheng, J. H., Liu, D. B., and Aadil, R. M. (2018c). The efficiency and comparison of novel techniques for cell wall disruption in astaxanthin extraction from Haematococcus pluvialis. Int. J. Food Sci. Technol 53, 2212–2219. doi:10.1111/ijfs.13810
Louhasakul, Y., Cheirsilp, B., Maneerat, S., and Prasertsan, P. (2019). Potential use of flocculating oleaginous yeasts for bioconversion of industrial wastes into biodiesel feedstocks. Renew. Energy 136, 1311–1319. doi:10.1016/j.renene.2018.10.002
Lu, Q., Bu, Y. F., and Liu, J. Z. (2017). Metabolic engineering of Escherichia coli for producing astaxanthin as the predominant carotenoid. Mar. Drugs 15. doi:10.3390/md15100296
Lupo, B., Maestro, A., Porras, M., Gutiérrez, J. M., and González, C. (2014). Preparation of alginate microspheres by emulsification/internal gelation to encapsulate cocoa polyphenols. Food Hydrocoll 38, 56–65. doi:10.1016/j.foodhyd.2013.11.003
Machado, F. R. S., Trevisol, T. C., Boschetto, D. L., Burkert, J. F. M., Ferreira, S. R. S., Oliveira, J. V., et al. (2016). Technological process for cell disruption, extraction and encapsulation of astaxanthin from Haematococcus pluvialis. J. Biotechnol 218, 108–114. doi:10.1016/j.jbiotec.2015.12.004
Martínez, J. M., Gojkovic, Z., Ferro, L., Maza, M., Álvarez, I., Raso, J., et al. (2019). Use of pulsed electric field permeabilization to extract astaxanthin from the Nordic microalga Haematococcus pluvialis. Bioresour. Technol 289, 121694. doi:10.1016/j.biortech.2019.121694
Mashhadi, N. S., Zakerkish, M., Mohammadiasl, J., Zarei, M., Mohammadshahi, M., and Haghighizadeh, M. H. (2018). Astaxanthin improves glucose metabolism and reduces blood pressure in patients with type 2 diabetes mellitus. Asia Pac. J. Clin. Nutr 27, 341–346. doi:10.6133/apjcn.052017.11
Mata-Gómez, L. C., Montañez, J. C., Méndez-Zavala, A., and Aguilar, C. N. (2014). Biotechnological production of carotenoids by yeasts: an overview. Microb. Cell Factories 13, 12. doi:10.1186/1475-2859-13-12
Matter, I. A., Hoang Bui, V. K., Jung, M., Seo, J. Y., Kim, Y. E., Lee, Y. C., et al. (2019). Flocculation harvesting techniques for microalgae: a review. Appl. Sci 9. doi:10.3390/app9153069
Mei, J., Zhao, X., Yi, Y., Zhang, Y., Wang, X., and Ying, G. (2020). Preparation of astaxanthin by lipase-catalyzed hydrolysis from its esters in a slug-flow microchannel reactor. Process Biochem 98, 241–246. doi:10.1016/j.procbio.2020.06.017
Michelon, M., de Matos de Borba, T., da Silva Rafael, R., Burkert, C. A. V., and de Medeiros Burkert, J. F. (2012). Extraction of carotenoids from Phaffia rhodozyma: a comparison between different techniques of cell disruption. Food Sci. Biotechnol 21, 1–8. doi:10.1007/s10068-012-0001-9
MicroA, (2020). MicroA. Available at: https://microa.no/ (Accessed September 25 2020).
Mimoun-Benarroch, M., Hogot, C., Rhazi, L., Niamba, C. N., and Depeint, F. (2016). The bioavailability of astaxanthin is dependent on both the source and the isomeric variants of the molecule. Bull. Univ. Agric. Sci. Vet. Med. Cluj-Napoca. Food Sci. Technol 73, 61. doi:10.15835/buasvmcn-fst:12350
Molino, A., Mehariya, S., Di Sanzo, G., Larocca, V., Martino, M., Leone, G. P., et al. (2020). Recent developments in supercritical fluid extraction of bioactive compounds from microalgae: role of key parameters, technological achievements and challenges. J. CO2 Util 36, 196–209. doi:10.1016/j.jcou.2019.11.014
Molino, A., Mehariya, S., Iovine, A., Larocca, V., Di Sanzo, G., Martino, M., et al. (2018a). Extraction of astaxanthin and lutein from microalga Haematococcus pluvialis in the red phase using CO₂ supercritical fluid extraction technology with ethanol as co-solvent. Mar. Drugs 16, 432. doi:10.3390/md16110432
Molino, A., Rimauro, J., Casella, P., Cerbone, A., Larocca, V., Chianese, S., et al. (2018b). Extraction of astaxanthin from microalga Haematococcus pluvialis in red phase by using generally recognized as safe solvents and accelerated extraction. J. Biotechnol 283, 51–61. doi:10.1016/j.jbiotec.2018.07.010
Mozafari, M. R., Johnson, C., and HatziantoniouDemetzos, S. C. (2008). Nanoliposomes and their applications in food nanotechnology. J. Liposome Res 18, 309–327. doi:10.1080/08982100802465941
Musa, Y., Alime, G., Cihangir, U., Özlem, Y. T., Levent, T., Ahmi, , et al. (2019). Effects of astaxanthin on antioxidant parameters in ARPE-19 cells on oxidative stress model. Int. J. Ophthalmol 12, 930–935. doi:10.18240/ijo.2019.06.08
Najjar, Y. S. H., and Abu-Shamleh, A. (2020). Harvesting of microalgae by centrifugation for biodiesel production: a review. Algal Res 51, 102046. doi:10.1016/j.algal.2020.102046
NeoAlgae Micro Seaweeds Products, (2020). NeoAlgae micro seaweeds products SL. Available at: http://neoalgae.es/ (Accessed September 25 2020).
Niizawa, I., Espinaco, B. Y., Zorrilla, S. E., and Sihufe, G. A. (2019). Natural astaxanthin encapsulation: use of response surface methodology for the design of alginate beads. Int. J. Biol. Macromol 121, 601–608. doi:10.1016/j.ijbiomac.2018.10.044
Noah’s Inc (2020). Noah’s inc. Available at: http://www.noahsinc.com (Accessed September 25 2020).
Ojima, Y., Takeda, S., and Taya, M. (2016). Floc formation of ethanol producing Escherichia coli KO11 cells and its application to repeated batch operation. J. Chem. Eng. Jpn 49, 793–798. doi:10.1252/jcej.16we040
Orona-Navar, A., Aguilar-Hernández, I., Cerdán-Pasarán, A., López-Luke, T., Rodríguez-Delgado, M., Cárdenas-Chávez, D. L., et al. (2017). Astaxanthin from Haematococcus pluvialis as a natural photosensitizer for dye-sensitized solar cell. Algal Res 26, 15–24. doi:10.1016/j.algal.2017.06.027
Ozkan, G., Franco, P., De Marco, I., Xiao, J., and Capanoglu, E. (2019). A review of microencapsulation methods for food antioxidants: principles, advantages, drawbacks and applications. Food Chem 272, 494–506. doi:10.1016/j.foodchem.2018.07.205
Pan, L., Zhang, S., Gu, K., and Zhang, N. (2018). Preparation of astaxanthin-loaded liposomes: characterization, storage stability and antioxidant activity. CyTA-J. Food 16, 607–618. doi:10.1080/19476337.2018.1437080
Panis, G., and Carreon, J. R. (2016). Commercial astaxanthin production derived by green alga Haematococcus pluvialis: a microalgae process model and a techno-economic assessment all through production line. Algal Res 18, 175–190. doi:10.1016/j.algal.2016.06.007
Park, S. Y., Binkley, R. M., Kim, W. J., Lee, M. H., and Lee, S. Y. (2018). Metabolic engineering of Escherichia coli for high-level astaxanthin production with high productivity. Metab. Eng 49, 105–115. doi:10.1016/j.ymben.2018.08.002
Poojary, M. M., Barba, F. J., Aliakbarian, B., Donsì, F., Pataro, G., Dias, D. A., et al. (2016). Innovative alternative technologies to extract carotenoids from microalgae and seaweeds. Mar. Drugs 14, 1–34. doi:10.3390/md14110214
Praveenkumar, R., Gwak, R., Kang, M., Shim, T. S., Cho, S., Lee, J., et al. (2015). Regenerative Astaxanthin extraction from a single Microalgal (Haematococcus pluvialis) cell using a gold nano-scalpel. ACS Appl. Mater. Interfaces 7, 22702–22708. doi:10.1021/acsami.5b07651
Praveenkumar, R., Lee, J., Vijayan, D., Lee, S. Y., Lee, K., Sim, S. J., et al. (2020). Morphological change and cell disruption of Haematococcus pluvialis cyst during high-pressure homogenization for astaxanthin recovery. Appl. Sci 10. doi:10.3390/app10020513
PubChem (2020). Astaxanthin. Available at: https://pubchem.ncbi.nlm.nih.gov/compound/Astaxanthin (Accessed October 5 2020).
Putri, D. S., Sari, D. A., and Zuhdia, L. D. (2020). Flocculants optimization in harvesting freshwater microalgae Haematococcus pluvialis. JKR 5, 49–54.doi:10.20473/jkr.v5i1.20022
Qiu, X., Zhang, J., Zhou, J., Fang, Z., Zhu, Z., Li, J., et al. (2019). Stress tolerance phenotype of industrial yeast: industrial cases, cellular changes, and improvement strategies. Appl. Microbiol. Biotechnol 103, 6449–6462. doi:10.1007/s00253-019-09993-8
Rao, A. R., Sarada, R., Shylaja, M. D., and Ravishankar, G. A. (2015). Evaluation of hepatoprotective and antioxidant activity of astaxanthin and astaxanthin esters from microalga-Haematococcus pluvialis. J. Food Sci. Technol 52, 6703–6710. doi:10.1007/s13197-015-1775-6
Raposo, M. F. J., Morais, A. M. M. B., and Morais, R. M. S. C. (2012). Effects of spray-drying and storage on astaxanthin content of Haematococcus pluvialis biomass. World J. Microbiol. Biotechnol 28, 1253–1257. doi:10.1007/s11274-011-0929-6
Régnier, P., Bastias, J., Rodriguez-Ruiz, V., Caballero-Casero, N., Caballo, C., Sicilia, D., et al. (2015). Astaxanthin from Haematococcus pluvialis prevents oxidative stress on human endothelial cells without toxicity. Mar. Drugs 13, 2857–2874. doi:10.3390/md13052857
Reyes, F. A., Mendiola, J. A., Ibañez, E., and Del Valle, J. M. (2014). Astaxanthin extraction from Haematococcus pluvialis using CO2-expanded ethanol. J. Supercrit. Fluids 92, 75–83. doi:10.1016/j.supflu.2014.05.013
Rodríguez-Sáiz, M., De La Fuente, J. L., and Barredo, J. L. (2010). Xanthophyllomyces dendrorhous for the industrial production of astaxanthin. Appl. Microbiol. Biotechnol 88, 645–658. doi:10.1007/s00253-010-2814-x
Safi, C., Ursu, A. V., Laroche, C., Zebib, B., Merah, O., Pontalier, P. Y., et al. (2014). Aqueous extraction of proteins from microalgae: effect of different cell disruption methods. Algal. Res 3, 61–65. doi:10.1016/j.algal.2013.12.004
Saini, R. K., and Keum, Y. S. (2018). Carotenoid extraction methods: a review of recent developments. Food Chem 240, 90–103. doi:10.1016/j.foodchem.2017.07.099
Saleh, N. E., Wassef, E. A., and Shalaby, S. M. (2018). The role of dietary astaxanthin in European sea bass (Dicentrarchus labrax) growth, immunity, antioxidant competence and stress tolerance. Egypt. J. Aquat. Biol. Fish 22, 189–200. doi:10.21608/EJABF.2018.21044
Samorì, C., Pezzolesi, L., Galletti, P., Semeraro, M., and Tagliavini, E. (2019). Extraction and milking of astaxanthin from: Haematococcus pluvialis cultures. Green Chem 21, 3621–3628. doi:10.1039/c9gc01273g
Sankaran, R., Show, P. L., Lee, S. Y., Yap, Y. J., and Ling, T. C. (2018). Integration process of fermentation and liquid biphasic flotation for lipase separation from Burkholderia cepacia. Bioresour. Technol 250, 306–316. doi:10.1016/j.biortech.2017.11.050
Santos-Sánchez, N. F., Hernández-Carlos, B., Torres-Ariño, A., and Salas-Coronado, R. (2020). Astaxanthin and its formulations as potent oxidative stress inhibitors. Pharmacogn. Rev 14, 8–15. doi:10.3389/fphar.2018.01162
Schewe, H., Kreutzer, A., Schmidt, I., Schubert, C., and Schrader, J. (2017). High concentrations of biotechnologically produced astaxanthin by lowering pH in a Phaffia rhodozyma bioprocess. Biotechnol. Bioproc. Eng 22, 319–326. doi:10.1007/s12257-016-0349-4
Shah, M. M. R., Liang, Y., Cheng, J. J., and Daroch, M. (2016). Astaxanthin-producing green microalga Haematococcus pluvialis: from single cell to high value commercial products. Front. Plant Sci 7, 531. doi:10.3389/fpls.2016.00531
Shi, W., Zhu, L., Chen, Q., Lu, J., Pan, G., Hu, L., et al. (2017). Synergy of flocculation and flotation for microalgae harvesting using aluminium electrolysis. Bioresour. Technol 233, 127–133. doi:10.1016/j.biortech.2017.02.084
Silhavy, T. J., Kahne, D., and Walker, S. (2010). The bacterial cell envelope. Cold Spring Harb. Perspect. Biol 2, a000414. doi:10.1101/cshperspect.a000414
Singgih, M., and Julianti, E. (2015). “Food colorant from microorganisms,” in In Beneficial microorganisms in food and nutraceuticals. Editor Min-Tze. Liong (Cham, Switzerland: Springer), 265–284. doi:10.1007/978-3-319-23177-8_12
Stanbury, P. F., Whitaker, A., and Hall, S. J. (1995). “The recovery and purification of fermentation products,” in Principles of fermentation technology. Editors P. F. Stanbury, A. Whitaker, and S. J. Hall (Amsterdam, Netherlands: Elsevier), 277–312. doi:10.1016/b978-0-08-036131-4.50015-8
Starke, R., Jehmlich, N., Alfaro, T., Dohnalkova, A., Capek, P., Bell, S. L., et al. (2019). Incomplete cell disruption of resistant microbes. Sci. Rep 9, 5618. doi:10.1038/s41598-019-42188-9
Stewart, G. G., and Stewart, G. G. (2017). “The structure and function of the yeast cell wall, plasma membrane and periplasm,” in Brewing and distilling yeasts. Editor G. G. Stewart (Cham, Switzerland: Springer), 55–75. doi:10.1007/978-3-319-69126-8_5
Su, F., Xu, H., Yang, N., Liu, W., and Liu, J. (2018). Hydrolytic efficiency and isomerization during de-esterification of natural astaxanthin esters by saponification and enzymolysis. Electron. J. Biotechnol 34, 37–42. doi:10.1016/j.ejbt.2018.05.002
Sun, W., Lin, H., Zhai, Y., Cao, L., Leng, K., and Xing, L. (2015). Separation, purification, and identification of (3S,3′S)-trans-astaxanthin from Haematococcus pluvialis. Separ. Sci. Technol 50, 1377–1383. doi:10.1080/01496395.2014.976873
Sun, W., Xing, L., Lin, H., Leng, K., Zhai, Y., and Liu, X. (2016). Assessment and comparison of in vitro immunoregulatory activity of three astaxanthin stereoisomers. J. Ocean Univ. China 15, 283–287. doi:10.1007/s11802-016-2716-3
Sun, Z., Zhang, Y., Sun, L. P., and Liu, J. (2019). Light Elicits astaxanthin biosynthesis and accumulation in the fermented ultrahigh-density Chlorella zofinginesis. J. Agric. Food Chem 67, 5579–5586. doi:10.1021/acs.jafc.9b01176
Sutterlin, H. A., Shi, H., May, K. L., Miguel, A., Khare, S., Huang, K. C., et al. (2016). Disruption of lipid homeostasis in the Gram-negative cell envelope activates a novel cell death pathway. Proc. Natl. Acad. Sci. U. S. A 113, E1565–E1574. doi:10.1073/pnas.1601375113
Tan, J. S., Lee, S. Y., Chew, K. W., Lam, M. K., Lim, J. W., Ho, S. H., et al. (2020). A review on microalgae cultivation and harvesting, and their biomass extraction processing using ionic liquids. Bioengineered 11, 116–129. doi:10.1080/21655979.2020.1711626
Tirado, D. F., Palazzo, I., Scognamiglio, M., Calvo, L., Della Porta, G., and Reverchon, E. (2019). Astaxanthin encapsulation in ethyl cellulose carriers by continuous supercritical emulsions extraction: a study on particle size, encapsulation efficiency, release profile and antioxidant activity. J. Supercrit. Fluids 150, 128–136. doi:10.1016/j.supflu.2019.04.017
Tiwari, S., and Verma, P. (2011). Microencapsulation technique by solvent evaporation method (study of effect of process variables). Int. J. Pharm. Life Sci 2, 998–1005.
Tran, T. N., Tran, Q. V., Huynh, H. T., Hoang, N. S., Nguyen, H. C., and Ngo, D. N. (2019). Astaxanthin production by newly isolated Rhodosporidium toruloides: optimization of medium compositions by response surface methodology. Not. Bot. Horti Agrobot. Cluj-Napoca 47, 320–327. doi:10.15835/nbha47111361
Tripathi, N. K. (2016). Production and purification of recombinant proteins from Escherichia coli. Chem. Bio. Eng. Rev 3, 116–133. doi:10.1002/cben.201600002
Tseng, C. C., Lin, Y. J., Liu, W., Lin, H. Y., Chou, H. Y., Thia, C., et al. (2020). Metabolic engineering probiotic yeast produces 3S, 3′S-astaxanthin to inhibit B16F10 metastasis. Food Chem. Toxicol 135, 110993. doi:10.1016/j.fct.2019.110993
Tsubokura, A., Yoneda, H., and Mizuta, H. (1999). Paracoccus carotinifaciens sp. nov., a new aerobic Gram-negative astaxanthin-producing bacterium. Int. J. Syst. Evol. Microbiol 49, 277–282. doi:10.1099/00207713-49-1-277
Ukibe, K., Hashida, K., Yoshida, N., and Takagi, H. (2009). Metabolic engineering of Saccharomyces cerevisiae for astaxanthin production and oxidative stress tolerance. Appl. Environ. Microbiol 75, 7205–7211. doi:10.1128/AEM.01249-09
Urnau, L., Colet, R., Soares, V. F., Franceschi, E., Valduga, E., and Steffens, C. (2018). Extraction of carotenoids from Xanthophyllomyces dendrorhous using ultrasound-assisted and chemical cell disruption methods. Can. J. Chem. Eng 96, 1377–1381. doi:10.1002/cjce.23046
Valensa International (2020). Valens international. Available at: http://valensa.com (Accessed September 25 2020).
Veeruraj, A., Liu, L., Zheng, J., Wu, J., and Arumugam, M. (2019). Evaluation of astaxanthin incorporated collagen film developed from the outer skin waste of squid Doryteuthis singhalensis for wound healing and tissue regenerative applications. Mater. Sci. Eng. C 95, 29–42. doi:10.1016/j.msec.2018.10.055
Venture radar (2020). Astaxanthin. Available at: https://www.ventureradar.com/search/ranked/astaxanthin/ (Accessed October 10 2020).
Visioli, F., and Artaria, C. (2017). Astaxanthin in cardiovascular health and disease: mechanisms of action, therapeutic merits, and knowledge gaps. Food Funct 8, 39–63. doi:10.1039/c6fo01721e
Wang, C., Armstrong, D. W., and Chang, C. D. (2008). Rapid baseline separation of enantiomers and a mesoform of all-trans-astaxanthin, 13-cis-astaxanthin, adonirubin, and adonixantthin in standards and commercial supplements. J. Chromatogr 1194, 172–177. doi:10.1016/j.chroma.2008.04.063
Wang, J., Liu, S., Wang, H., Xiao, S., Li, C., Li, Y., et al. (2019). Xanthophyllomyces dendrorhous-derived astaxanthin regulates lipid metabolism and gut microbiota in obese mice induced by a high-fat diet. Mar. Drugs 17. 337. doi:10.3390/md17060337
Williamson, E. M., Liu, X., and Izzo, A. A. (2020). Trends in use, pharmacology, and clinical applications of emerging herbal nutraceuticals. Br. J. Pharmacol 177, 1227–1240. doi:10.1111/bph.v177.6/issuetoc
Xiao, A. F., Ni, H., Cai, H. N., Li, L. J., Su, W. J., and Yang, Q. M. (2009). An improved process for cell disruption and astaxanthin extraction from Phaffia rhodozyma. World J. Microbiol. Biotechnol 25, 2029–2034. doi:10.1007/s11274-009-0104-5
Xiao, Z., Liu, W., Zhu, G., Zhou, R., and Niu, Y. (2014). A review of the preparation and application of flavour and essential oils microcapsules based on complex coacervation technology. J. Sci. Food Agric 94, 1482–1494. doi:10.1002/jsfa.6491
Yang, C., Hassan, Y. I., Liu, R., Zhang, H., Chen, Y., Zhang, L., et al. (2019). Anti-inflammatory effects of different astaxanthin isomers and the roles of lipid transporters in the cellular transport of astaxanthin isomers in CaCo-2 cell monolayers. J. Agric. Food Chem 67, 6222–6231. doi:10.1021/acs.jafc.9b02102
Yang, C., Zhang, L., Zhang, H., Sun, Q., Liu, R., Li, J., et al. (2017). Rapid and efficient conversion of all-E-astaxanthin to 9Z- and 13Z-isomers and assessment of their stability and antioxidant activities. J. Agric. Food Chem 65, 818–826. doi:10.1021/acs.jafc.6b04962
Ye, Z., Tan, X. H., Liu, Z. W., Aadil, R. M., Tan, Y. C., and Inam-ur-Raheem, M. (2020). Mechanisms of breakdown of Haematococcus pluvialis cell wall by ionic liquids, hydrochloric acid and multi-enzyme treatment. Int. J. Food Sci. Technol 55, 3182–3189. doi:10.1111/ijfs.14582
Young, A. J., Pritchard, J., White, D., and Davies, S. (2017). Processing of astaxanthin-rich Haematococcus cells for dietary inclusion and optimal pigmentation in Rainbow trout, Oncorhynchus mykiss L. Aquacult. Nutr 23, 1304–1311. doi:10.1111/anu.12505
Zgheib, N., Saade, R., Khallouf, R., and Takache, H. (2018). Extraction of astaxanthin from microalgae: process design and economic feasibility study. IOP Conf. Ser. Mater. Sci. Eng 323, 012011. doi:10.1088/1757-899X/323/1/012011
Zhang, C., Chen, X., and Too, H. P. (2020a). Microbial astaxanthin biosynthesis: recent achievements, challenges, and commercialization outlook. Appl. Microbiol. Biotechnol 104, 5725–5737. doi:10.1007/s00253-020-10648-2
Zhang, C., Seow, V. Y., Chen, X., and Too, H. P. (2018). Multidimensional heuristic process for high-yield production of astaxanthin and fragrance molecules in Escherichia coli. Nat. Commun 9, 1858. doi:10.1038/s41467-018-04211-x
Zhang, J., Li, Q., Liu, J., Lu, Y., Wang, Y., and Wang, Y. (2020b). Astaxanthin overproduction and proteomic analysis of Phaffia rhodozyma under the oxidative stress induced by TiO2. Bioresour. Technol 311. doi:10.1016/j.biortech.2020.123525
Zhao, W., Wang, Y., Hao, W., Yang, H., Song, X., Zhao, M., et al. (2015). Preparative isolation and purification of urolithins from the intestinal metabolites of pomegranate ellagitannins by high-speed counter-current chromatography. J. Chromatogr. B Anal. Technol. Biomed. Life Sci 990, 111–117. doi:10.1016/j.jchromb.2015.03.024
Zhao, X., Liu, H., Zhang, X., Zhang, G., and Zhu, H. (2019). Astaxanthin from Haematococcus pluvialis microencapsulated by spray drying: characterization and antioxidant activity. JAOCS, J. Am. Oil Chem. Soc 96, 93–102. doi:10.1002/aocs.12170
Zhao, X., Zhang, X., Fu, L., Zhu, H., and Zhang, B. (2016). Effect of extraction and drying methods on antioxidant activity of astaxanthin from Haematococcus pluvialis. Food Bioprod. Process 99, 197–203. doi:10.1016/j.fbp.2016.05.007
Zhou, P., Xie, W., Li, A., Wang, F., Yao, Z., Bian, Q., et al. (2017). Alleviation of metabolic bottleneck by combinatorial engineering enhanced astaxanthin synthesis in Saccharomyces cerevisiae. Enzym. Microb. Technol 100, 28–36. doi:10.1016/j.enzmictec.2017.02.006
Zhu, C., Han, W., Chen, Z., and Han, Z. (2010). Statistical optimization of microwave-assisted astaxanthin extraction from Phaffia rhodozym. Proc. - 2010 3rd Int. Conf. Biomed. Eng. Informatics. BMEI 2010 5, 2104–2109. doi:10.1109/BMEI.2010.5639994
Ziaee, A., Albadarin, A. B., Padrela, L., Femmer, T., O’Reilly, E., and Walker, G. (2019). Spray drying of pharmaceuticals and biopharmaceuticals: critical parameters and experimental process optimization approaches. Eur. J. Pharmaceut. Sci 127, 300–318. doi:10.1016/j.ejps.2018.10.026
Keywords: Astaxanthin extraction, astaxanthin formulation, astaxanthin purification, cell disruption, cell recovery, Paracoccus carotinifaciens, Phaffia rhodozyma, Haematococcus pluvialis
Citation: Rodríguez-Sifuentes L, Marszalek JE, Hernández-Carbajal G and Chuck-Hernández C (2021) Importance of Downstream Processing of Natural Astaxanthin for Pharmaceutical Application. Front. Chem. Eng. 2:601483. doi: 10.3389/fceng.2020.601483
Received: 01 September 2020; Accepted: 09 December 2020;
Published: 19 January 2021.
Edited by:
Gyorgy Szekely, King Abdullah University of Science and Technology, Saudi ArabiaReviewed by:
Pau Loke Show, University of Nottingham Malaysia Campus, MalaysiaDino Musmarra, University of Campania Luigi Vanvitelli, Italy
Copyright © 2021 Rodríguez-Sifuentes, Marszalek, Hernández-Carbajal and Chuck-Hernández. This is an open-access article distributed under the terms of the Creative Commons Attribution License (CC BY). The use, distribution or reproduction in other forums is permitted, provided the original author(s) and the copyright owner(s) are credited and that the original publication in this journal is cited, in accordance with accepted academic practice. No use, distribution or reproduction is permitted which does not comply with these terms.
*Correspondence: Cristina Chuck-Hernández, Y3Jpc3RpbmEuY2h1Y2tAdGVjLm14