- 1Department of Oncology, The First Affiliated Hospital of Xinxiang Medical University, Weihui, Henan, China
- 2Department of Neurosurgery, Department of Urology, Medical Research Center, The Second Chengdu Hospital Affiliated to Chongqing Medical University, The Affiliated Hospital of Southwest Jiaotong University, The Third People’s Hospital of Chengdu, Chengdu, China
- 3College of Public Health (Shenzhen), Sun Yat-sen University, Shenzhen, China
- 4West China School of Basic Medical Sciences and Forensic Medicine, State Key Laboratory of Biotherapy and Cancer Center, West China Hospital, Collaborative Innovation Center for Biotherapy, Sichuan University, Chengdu, China
- 5Obesity and Metabolism Medicine-Engineering Integration Laboratory, Department of General Surgery, The Third People's Hospital of Chengdu, The Affiliated Hospital of Southwest Jiaotong University, Chengdu, China
- 6The Center of Gastrointestinal and Minimally Invasive Surgery, Department of General Surgery, The Third People's Hospital of Chengdu, The Affiliated Hospital of Southwest Jiaotong University, Chengdu, China
Tumor immunotherapy, modulating innate and adaptive immunity, has become an important therapeutic strategy. However, the tumor immune microenvironment’s (TIME) complexity and heterogeneity challenge tumor immunotherapy. Hydrogel is a hydrophilic three-dimensional (3D) mesh structure with good biocompatibility and drug release control, which is widely used in drug delivery, agriculture, industry, etc. Hydrogels loaded with immune cells, cytokines, immune checkpoint inhibitors, and anti-tumor drugs can achieve targeted delivery and ultimately activate the immune response in the TIME. In this review, we will summarize the components of the TIME and their immune effects, the emerging immunomodulatory agents, the characteristics and functions of hydrogels, and how hydrogels regulate innate and adaptive immune cells in the TIME.
1 Introduction
In recent years, the incidence and mortality of cancer have been rising all over the world. According to global statistics, there were 19.3 million new cases of cancer and nearly 10 million deaths due to cancer in 2020 (Sung et al., 2021). Tumor treatments mainly include surgery, chemotherapy, radiotherapy, immunotherapy, etc. Immunotherapy has presented an impressive anti-tumor effect in tumor therapy due to rewiring the tumor immune microenvironment (TIME) to attack cancer cells specifically (Tan et al., 2020). At present, a variety of cancer immunotherapies have been designed, including immune checkpoint blocking (ICB) therapies, cancer vaccines, agonistic antibodies, and chimeric antigen receptor T-Cell (CAR-T) immunotherapy (Tan et al., 2020; Wang and Mooney, 2018).
However, the TIME brings obstacles to the application of immunotherapy. In the TIME, there exist numerous immunosuppressive cell populations that promote tumor immune escape by directly inhibiting the function of immune cells or altering the tumor microenvironment. These immunosuppressive cells include Tregs, MDSCs, and tumor-associated macrophages (TAMs), among others. They collectively create an immunosuppressive environment by secreting immunosuppressive factors, depleting nutrients, or altering the pH of the tumor microenvironment. The extracellular matrix (ECM) of tumor tissue often exhibits excessive deposition and a “stiff” state of collagen, fibronectin, and other components, which not only restricts the infiltration of immune cells but also further inhibits their function through mechanisms such as inducing hypoxia. Additionally, abnormalities in tumor blood vessel structure constitute an important part of the physical barrier, impeding the effective delivery of immune cells and the supply of nutrients. Therefore, TMIE severely affects the efficacy of tumor immunotherapy (Tan et al., 2020; Xiao et al., 2023; Seo et al., 2022). How to precisely regulate the immune microenvironment, relieve immunosuppression, and enhance the efficacy of immunotherapy is crucial for the treatment of tumors.
The emergence of biomaterials has enabled researchers to achieve precise delivery of cytokines and targeted regulation of signaling pathways, making it possible to efficiently and low-toxically activate anti-tumor immunity (Wang and Mooney, 2018; Ruan et al., 2022; Hu et al., 2021). Hydrogel is a hydrophilic three-dimensional (3D) mesh structure of biological materials, and the water content can be as high as 90% (Xie et al., 2021; Ahmed, 2015; Cui et al., 2021). Due to the high degree of customizability and tunability, hydrogel can be designed to suit a variety of requirements. Therefore, hydrogel has a wide range of applications in many fields, including medicine (Ho et al., 2022; Khan et al., 2022). Due to biocompatibility, highly hydrophilic and porous structure, high drug-loading, low toxicity, stimuli-responsiveness, controlled release, hydrogels hold promise in overcoming immune barriers within the tumor immune microenvironment, enabling precise delivery of immunomodulatory drugs such as cytokines, as well as precise targeting of immunosuppressive cells. They have significant application prospects in the regulation of TMIE and immune activation.
Hydrogel can play a regulatory role in cell growth and differentiation, such as remodel the extracellular matrix (ECM), creating an environment conducive to cell growth and differentiation (Xiao et al., 2023; Yue et al., 2015). Hydrogel’s drug release rate can be controlled by adjusting its crosslinking degree, polymer chain length, and introducing functional groups (Teixeira et al., 2021; Ashfaq et al., 2020), which ensures its function of release immunomodulators in a controlled manner while protecting their bioactivity is highlighted (Cao et al., 2021; Almawash et al., 2022; Chin et al., 2020). What’s more, hydrogel can deliver immunomodulators or drugs for regulating tumor immunity. Hydrogel loaded with IL-4 and BMP-2 promotes macrophage differentiation into the M2 type, which can effectively regulate tumor immunity (Zou et al., 2021). GM-CSF released by an injectable heat-sensitive hydrogel mediates the recruitment, proliferation, and maturation of dendritic cells, promoting an effective immune response (Warren and Weiner, 2000; Liu et al., 2014). Another example is provided where the delivery of doxorubicin and cytosine-phospho-guanine self-crosslinking nanoparticles based on the hydrogel increases the number of CD8+ T cells and reduces the number of immunosuppressive cells in the TIME (Dong et al., 2020).
In this review, we initially examine the components of the tumor immune microenvironment (TIME) and their immune implications. Next, we summarize emerging immunomodulatory agents and provide an overview of hydrogels, including their classification, characteristics, and applications. Finally, we outline the primary mechanisms through which hydrogels regulate innate and adaptive immune cells, illustrating these with relevant examples to facilitate understanding of their functions.
2 Composition of the TIME
TIME consists of many components, including DCs, macrophages, natural killer (NK) cells, B cells, T cells, and ECM (Arneth, 2019). It is an immunosuppressive environment that benefits tumor progression. However, many components in the TIME play an active anti-tumor immune function. The components of the TIME and their immune effects are listed in Table 1 (Arneth, 2019; Bilotta et al., 2022).
2.1 DCs
DCs, originated from multipotent hematopoietic stem cells in the bone marrow, showing the remarkable ability to efficiently capture, process, and present antigens to T cells (Balan et al., 2019). After the activation of DCs, DCs showed increased costimulatory molecule expression, cytokine secretion, and antigen capture presentation ability (Veglia and Gabrilovich, 2017). Mature DCs can express major histocompatibility complex (MHC) and costimulatory molecule to effectively activate T cells, thus initiating the tumor-killing process of T cells (Banchereau et al., 2000; Gardner et al., 2020). Moreover, immunosuppressive factors on DCs are the key regulator of T cell immunity in the TIME (Oh et al., 2020). However, it was found that there were defects in the differentiation and activation of DCs in the TIME, which led to the inability of DCs to perform the immune surveillance function well (Veglia and Gabrilovich, 2017). Elevated lactate levels, hypoxia, adenosine accumulation, and decreased pH in the TIME affect the maturation and activation of DCs, which finally affect the antigen presentation ability of DCs (Veglia and Gabrilovich, 2017).
2.2 Macrophages
Macrophages can mediate phagocytosis and present antigens. Macrophages can differentiate into M1 and M2 macrophages, which exert an inverse influence on the progression of the tumor. M1 macrophages express Type I cytokines with an anti-tumor effect, such as tumor necrosis factor-α (TNF-α), interferon-γ (IFN-γ), IL-6; M2 macrophages express Type II cytokines, which inhibit T cell-mediated anti-tumor response, such as IL-10, IL-13, transforming growth factor-β (TGF-β) (Biswas and Mantovani, 2010). These cytokines can regulate TIME. For example, TNF-α can not only activate immune cells, but also promote tumor angiogenesis and immunosuppression in some cases (Cruceriu et al., 2020). M2 macrophages are the main type in the TIME, called TAMs, and help establish an immunosuppressive environment. And TAMs can promote the proliferation and metastasis of tumor cells by inhibiting the recognition and killing function of tumor cells by T cells and NK cells (Ngambenjawong et al., 2017; Qian and Pollard, 2010).
2.3 NK cells
NK cells, as the innate cytotoxic lymphocytes, are capable of recognizing and killing tumor cells without tumor antigens’ stimulation (Bald et al., 2020). Many tumor cells evade the immune recognition and killing of CD8+ T cells by down-regulating the expression of MHC-I. NK cells can recognize the “missing-self” phenotype of tumor cells (Myers and Miller, 2021; Kärre, 2002). Once NK cells are activated, NK cells can kill tumor cells by secreting perforin and granzyme. Moreover, NK cells can also exert anti-tumor immunity by mediating antibody-dependent cell-mediated cytotoxicity (ADCC) (Myers and Miller, 2021). For example, NKG2D serves as an activating receptor for NK cells, and its corresponding ligand is expressed in some tumor cells. When the NKG2D receptor binds to the ligand, it can trigger the killing function of NK cells in the TIME (Wang et al., 2020). Additionally, some cytokines and chemokines (IFN-γ, GM-CSF) can be secreted by NK cells, which have immunomodulatory and anti-tumor effects in the TIME.
2.4 B cells
B cells in the TIME are capable of regulating tumor cell survival and proliferation. Tertiary lymphoid structures (TLSs) can be formed in the TIME (Fridman et al., 2022). B cells in TLSs form germinal centers and secrete antibodies that can recognize tumor-associated antigens; B cells are also involved in antigen presentation in the TIME to activate immune response (N. et al., 2021; Chandnani et al., 2024). Further, B cells can also produce various immune-stimulating cytokines, including lymphotoxin and other cytokines (Tang et al., 2017).
2.5 T cells
Adaptive immunity mediated by CD8+ and CD4+ T cells is the leading force for killing tumor cells (Fridman et al., 2012). CD8+ T cells recognize MHC-I, while CD4+ T cells have an affinity for MHC-II (Raskov et al., 2021). CD8+ T cells can secrete particles containing granzymes, perforin, and cathepsin C to induce apoptosis of tumor cells (Farhood et al., 2019). In addition, activated CD8+ T cells can highly express Fas ligand (FasL), which can mediate Fas activation to induce target cell apoptosis binding to Fas (Fu et al., 2016). However, tumor cells can inhibit the killing effect of CD8+ T cells by reducing the level of MHC-I molecules on the cell membrane (Raskov et al., 2021). Another important type of T cells is CD4+ T cells. CD4+ T cells can secrete cytokines (IFN-γ, IL-4, IL-10, etc.) to regulate the immune response. Moreover, the subpopulations of CD4+ T cells have many functions and mechanisms in the TIME, which are playing the anti-tumor or pro-tumor role. T helper 1 (Th1) cells can promote recruitment of antigen-presenting cells, activate phagocytosis of macrophages, and inhibit angiogenesis; Th2 cells can inhibit antigen processing of DCs, promote polarization of M2 macrophages, activate immunosuppressive Tregs and Myeloid-derived suppressor cells (MDSCs), and activate the anti-tumor activity of NK cells; Th17 cells can promote angiogenesis (Miggelbrink et al., 2021; Ellyard et al., 2007; Basu et al., 2021). Tregs have the ability to inhibit anti-tumor immune response, thereby accelerating the process of tumor progression (Li C. et al., 2020). It is worth noting that there are other cells and molecules in the TIME that can inhibit effective anti-tumor immune responses, such as MDSCs, programmed cell death protein 1 (PD1), adenosine, and vascular endothelial growth factor (VEGF) (Cekic et al., 2014; Mannino et al., 2015; Keir et al., 2008; Pan et al., 2010).
2.6 MDSCs
Myeloid-Derived Suppressor Cells (MDSCs) are a type of immature myeloid cells with immunosuppressive functions, playing a crucial role in the tumor immune microenvironment (Veglia et al., 2021). They exert potent immunosuppressive effects by inhibiting T-cell function and promoting the development and maturation of regulatory T cells (Tregs). In the tumor immune microenvironment, MDSCs play a pivotal role in fostering tumor growth, progression, and metastasis by suppressing antitumor immune responses (Yaseen et al., 2020).
The immunosuppressive mechanisms are multifaceted, involving the production of immune-inhibitory cytokines such as interleukin-10 (IL-10), transforming growth factor-β (TGF-β), and arginase-1, which deplete essential amino acids required for T cell proliferation and function. Additionally, MDSCs express programmed death-ligand 1 (PD-L1), which binds to PD-1 on T cells, further inhibiting their activity. Moreover, MDSCs promote tumor angiogenesis by secreting vascular endothelial growth factor (VEGF), thereby providing nutritional support to the tumor. They also enhance tumor invasiveness by secreting matrix metalloproteinases (MMPs) and induce the polarization of macrophages towards the M2 phenotype, which further amplifies the immunosuppressive microenvironment (Gabrilovich and Nagaraj, 2009).
MDSCs play a crucial role in the tumor immune microenvironment by suppressing antitumor immune responses and promoting tumor progression. Understanding the complexities of MDSC biology and developing effective therapeutic strategies to target these cells hold great promise for improving cancer treatment outcomes (Ozbay Kurt et al., 2023).
3 Emerging immunomodulators of small molecule
After gaining a preliminary understanding of the composition of TMIE, which drugs might have a regulatory effect on it, thereby achieving activation of antitumor immunity. The past few years have witnessed an enormous interest in immunomodulatory agents to restrain tumors, especially drugs for treating other diseases, small molecules targeting drugs through chemical synthesis, and agonists of immune signaling screened from the compound library. Table 2 lists “Effective concentration” “Research model” “Administration” “Effect on immune cells” and “Mechanism” of emerging small molecular immunomodulators in tumors.
3.1 Tramadol
Tramadol (TRA) is a weak opioid anesthetic and is prescribed for pain in tumors where non-opioid agents do not provide effective pain relief. Intriguingly, TRA has presented the anti-tumor ability in multiple tumors, such as pancreatic ductal adenocarcinoma (Kuramochi et al., 2024), endometrial carcinoma (Liu L. C. et al., 2022), lung adenocarcinoma (Xia et al., 2016a), breast cancer (Xia et al., 2016b). Moreover, compared to other opioids, tramadol could preserve the immune function, counteracting the inhibition of NK cell activity and lymphocyte proliferation induced by surgery for rats (Gaspani et al., 2002) and patients (Bakr et al., 2016) in the lab and clinical, respectively. In addition, TRA could facilitate M1 macrophage polarization by activating nuclear factor kappa B (NF-κB)signaling, thereby promoting the proliferation and activation of T cells (Wang et al., 2024). In light of evidence that tramadol showed protective immune function against tumors, prescription of opioids, particularly tramadol, could be recommended.
3.2 Clotrimazole
Clotrimazole (CTZ), an antifungal drug, is also an anti-tumor agent owing to its multifactorial function, including a pro-immune effect. CTZ triggered DC-mediated antigen presentation by modulating lactate metabolic production, lysosome pathway, and CHOP expression in DCs, subsequently promoting T cell activation (Wang et al., 2021). Moreover, CTZ could reprogram TIME by attenuating the infiltration of immunosuppressive TAMs, leading to an anti-tumor effect (Ochioni et al., 2021).
3.3 Statins
Statins, specifically inhibiting HMG-CoA reductase, are widely prescribed to reduce cholesterol. The literature has demonstrated that statins, including mevastatin, simvastatin, and pitavastatin, could enhance the tumor-killing effect mediated by PBMC (Selvin et al., 2023). Furthermore, T cells pretreated with simvastatin and lovastatin presented an increased cytotoxic effect on tumor cells. Daily oral administration has enhanced tumors’ sensitivity to PD-1 therapy and promoted the shift to M1 macrophage (Kansal et al., 2023; Vesely et al., 2022).
3.4 Agonists of α2-adrenergic receptors
Agonists of α2-adrenergic receptors (α2-AR), including guanabenz (GBZ), clonidine (CLD), and guanfacine (GFC), have been identified with pro-immune function and anti-tumor effect. These agonists could activate macrophages, leading to the recruitment and activation of T cells in the TIME. The number of PMN-MDSC was reduced in both the MC38-OVA model and the TiRP model, while macrophages played a prominent role in the MC38-OVA model. Moreover, these agents enhance the anti-tumor effect of anti-PD-1 therapy (Zhu et al., 2023).
3.5 MSA-2
Systemic non-nucleotide STING agonist, MSA-2, which was screened from a compound library for oral administration, regressed tumor growth and presented durable anti-tumor immunity against reinoculation of tumor (Pan et al., 2020). Additionally, synergized with anti-PD-1 and osimertinib, MSA-2 could enhance the anti-tumor effect and reverse the resistance of this therapy (Pan et al., 2020; Lin et al., 2023; Li et al., 2024; Yi et al., 2022). Furthermore, MSA-2 stimulated pro-inflammatory cytokines secretion and activated the type I Interferon (IFN) signaling pathways in human monocytes (Kabelitz et al., 2022). IFN could promote DC maturation. MSA-2 triggered DC maturation and significantly enhanced mice BDMC activity, including increased cytokine secretion and antigen presentation capability. BMDCs treated with MSA-2 triggered the proliferation of naïve T cells. Besides, MSA-2 also could increase M1-like markers of BMDM rather than M2-like marker (Yi et al., 2022).
3.6 BPR1R024
Based on physicochemical property-driven optimizations from a multitargeting kinase inhibitor, BPR1R024 was designed for oral BPR1R024 administration and presented superior target selectivity to CSF1R. BPR1R024 dramatically inhibited M2 macrophage growth while maintaining minimal effect on M1 macrophage, leading to an anti-tumor effect in vivo (Lee et al., 2021).
Besides, a broad range of studies has demonstrated that compounds derived from natural products, such as Fucoidans (Kiselevskiy et al., 2022), Humulus lupulus (Vesaghhamedani et al., 2022), mushrooms (Balakrishnan et al., 2021), Huang qi (Balakrishnan et al., 2021), and honey (Masad et al., 2021), present potent anti-tumor immunity, indicating that dietary therapy is like to improve individual self-defense against tumors.
Generally, diverse immunomodulators have huge potential to inhibit tumor progression with canonical chemotherapy (Deng et al., 2022) and radiotherapy (Ni et al., 2022), function as adjuvant to tumor vaccines (Chen F. et al., 2023), reverse the resistance to anti-tumor therapy (Baumgartner et al., 2023), and preserve immune function after surgery.
4 Hydrogel in the regulation of TMIE
As an emerging biomaterial, What advantages do hydrogels possess that enable them to play a role in biomedicine, particularly in the regulation of TMIE? Hydrogels have different kinds and can be classified according to different classification methods. Hydrogels can be categorized into natural and synthetic hydrogels according to the component source. The former include fibrous proteins, alginates, etc. The latter include polyvinyl alcohol (PVA) and polyethylene glycol (PEG) (Cui et al., 2021). Moreover, physical and chemical hydrogels are classified according to binding and crosslinking methods (Ahmed, 2015). Physical hydrogels are formed by the combination of physical forces. Chemical hydrogels are formed by the crosslinking of chemical bonds. Classification based on response to external stimuli includes conventional and environmentally sensitive hydrogels. Traditional hydrogels are not sensitive to external stimuli, while environmentally sensitive hydrogels can respond to external stimuli (such as pH, temperature, pressure, and light) (Li and Su, 2018). In addition, hydrogels can also be classified according to the polymer composition (Ahmed, 2015). Figure 1 presents the classification of hydrogels.
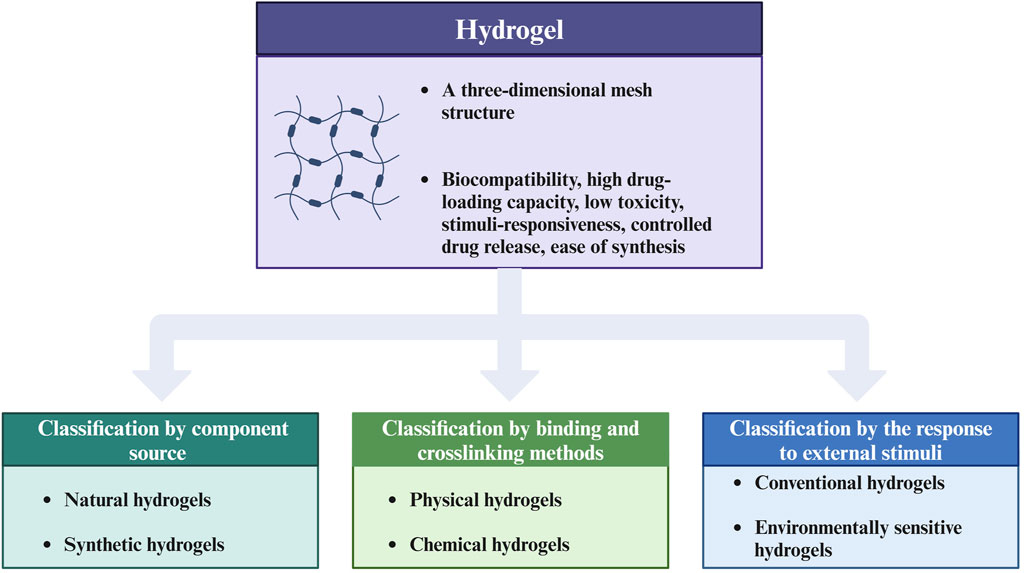
Figure 1. Classification chart of hydrogels. This figure introduces the advantages of hydrogels as biomaterials and summarizes three main classification methods of hydrogels, including classification by component source, by binding and crosslinking methods, and by the response to external stimuli. The figure is made with Biorender (https://biorender.com/).
Nowadays, hydrogels are widely used in biomedicine (including tissue engineering, drug delivery, 3D cell culture, wound dressing, etc.) (Ho et al., 2022), agriculture (Khan et al., 2022), and industrial materials (Flores-Valenzuela et al., 2023). Especially in medicine, hydrogels have presented specific feasibility and effectiveness for the treatment of anal fistulas (de la Portilla et al., 2021), gingival regeneration (Hutomo et al., 2023), wound dressing (Liang et al., 2021), and burn wound management (Goh et al., 2024), etc. Hydrogels can provide the loaded immune components with a niche similar to the environment in vivo due to their excellent biocompatibility, 3D network structure. Good biocompatibility means less toxicity for cells. The 3D network structure can make hydrogels have high permeability, allowing the transport of nutrients and the transfer of signaling molecules, ultimately promoting cell metabolism and proliferation (Yue et al., 2020). And highly hydrophilic and porous structure allows hydrogel to absorb and store large amounts of water, mimicking the ECM. Besides, this moist environment supports the wound healing (Zhang and Zhao, 2020). Moreover, hydrogels can promote wound healing via carrying growth factors and antibiotics, which are continuously released on the wound. In bone tissue engineering (BTE), hydrogels provide scaffolds for growth factor transport and cell adhesion (Yue et al., 2020). Hydrogels are injected or surgically implanted to fill complex bone defects (Xue et al., 2022; Buwalda et al., 2017).
Hydrogel’s 3D network mechanism can efficiently deliver immunomodulators to the tumor site and maintain a high concentration for a long time, while significantly reducing the incidence of systemic toxicity and the risk of adverse side effects during immunotherapy (Chao et al., 2020). Based on hydrogels, anti-tumor immune responses can be induced by targeting immune checkpoint molecules and factors. Among them, the typical are PD-1/PD-L1 (Shi et al., 2021), CTLA-4 (Chen W. et al., 2023), NKG2D (Wang et al., 2020), TLRs (Behzadi et al., 2021), TGF-β (Gulley et al., 2022), etc. For example, antiprogrammed cell death receptor ligand 1 (aPD-L1) released by the hydrogel inhibited the PD-1/PD-L1 pathway and restored cytotoxic T cell killing function at the tumor site (Shi et al., 2021). Hydrogels can also efficiently deliver DCs, NK cells, and T cells to the tumor site. Moreover, the stimulation response of the intelligent hydrogel enables the hydrogel to release these components at the tumor site accurately, improving the targeting and anti-tumor effect (Zhao et al., 2022). In summary, hydrogels have demonstrated significant potential in the tumor treatment.
4.1 Targeted modulation of DCs by hydrogels
DC vaccines have shown a positive effect on the strategy of tumor immunotherapy and have achieved good results in clinical therapy (Palucka and Banchereau, 2013; Timmerman et al., 1999). However, DCs have a high mortality rate and a high off-target risk during the process of reaching the site of the tumor (Palucka et al., 2007). So, it is often challenging to recruit sufficient dendritic cells (DCs) for uptake, processing, and presentation. By leveraging the properties of hydrogels, antigens and immunostimulants can be encapsulated, along with the addition of pro-inflammatory cytokines and chemokines. This approach enables the sustained release of antigens, immunostimulants, cytokines and chemokines, thereby enhancing antigen presentation, promoting inflammatory responses, increasing DC recruitment, and inducing a robust anti-tumor immune response (Lei et al., 2022). DCs work synergically with macrophages and NK cells to exert immune function and inhibit the function of Tregs, ultimately activating T cells to recognize and kill tumor cells. For example, a biomaterial system of alpha-cyclodextrin-polyethylene glycol hydrogel/CpG crosslinked nano-adjuvants was used to co-deliver tumor cells and DC vaccines (Yang et al., 2021), and subsequently recruited DCs to the tumor site (Figure 2).
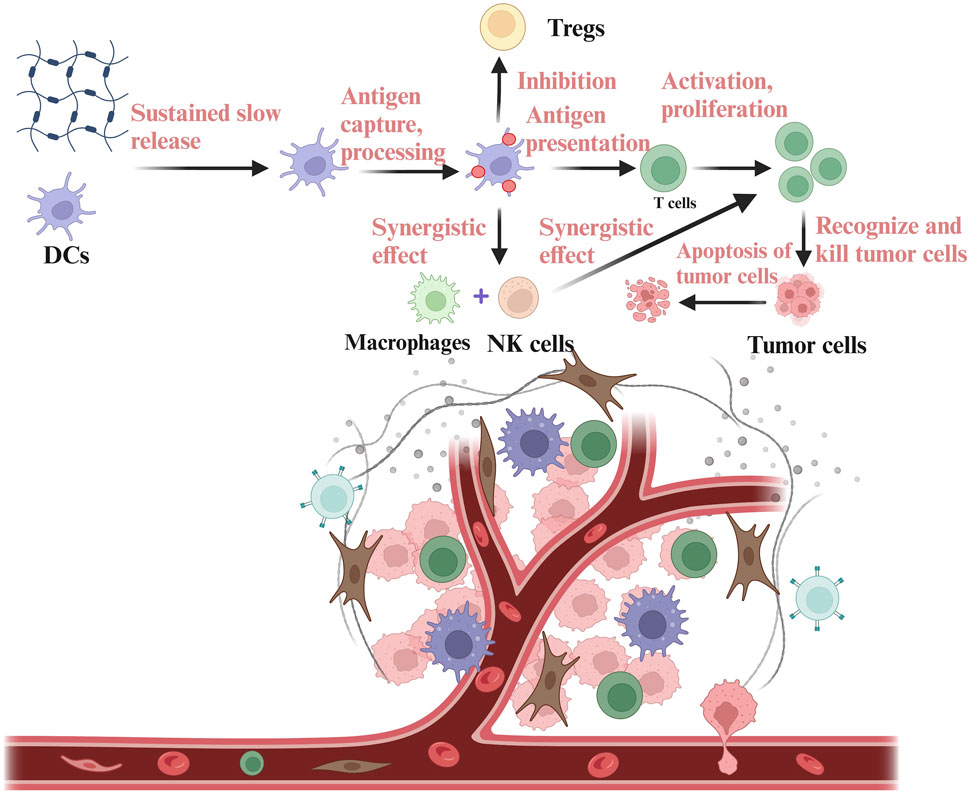
Figure 2. Delivery of DCs based on hydrogels in the TIME. DCs are encapsulated in the hydrogels and then released into the TIME. DCs released into the TIME can carry out antigen capture, processing and presentation, thus stimulating the anti-tumor immune function of T cells. DCs can also co-mediate immune response with NK cells and macrophages, while inhibiting immunosuppressive components in the TIME, such as Tregs. The figure is made with biorender (https://biorender.com/).
4.2 Targeted modulation of macrophages by hydrogels
TAMs are the main type of macrophages in the TIME. TAMs can support the growth and metastasis of tumor cells by inhibiting innate and adaptive immune cells and promoting angiogenesis. Tumor-associated macrophages (TAMs) can be targeted by depleting their numbers, repolarizing their function, or activating their ability to phagocytose tumor cells (Pathria et al., 2019). In tissue regeneration, a study found that the fibrin hydrogel may induce the anti-inflammatory phenotype of macrophages to eliminate the inflammatory response, leading to massive macrophage infiltration in the hydrogel scaffolds (Tanaka et al., 2019). This can also be applied to tumor therapy, but the direction of polarization needs to be transformed to M1 macrophages. Moreover, NF-κB signaling helps maintain the immunosuppressive phenotype of TAMs. Targeting NF-κB signaling in TAMs can inhibit tumor growth by enhancing macrophage tumoricidal activity and recruiting IL-12-dependent NK cells (Hagemann et al., 2008). Therefore, regulation of NF-κB based on hydrogels maybe a potential strategy.
4.3 Targeted modulation of NK cells by hydrogels
NK cells can inhibit tumor progression via anti-angiogenesis and proapoptotic effects (Myers and Miller, 2021). However, due to the complexity of the TIME, NK cell infiltration is less effective in immunotherapy for some solid tumors (Sun et al., 2015). Immunotherapy can increase NK cell infiltration to enhance immunosurveillance. It has been found that hydrogels loaded with immunomodulators can promote the infiltration of NK cells to induce the apoptosis of tumor cells more efficiently. A double pH-responsive hydrogel containing tumor acidity neutralizer and neutrophil extracellular traps (NETs) lyase in combination with NK cell infusion was used to prevent the recurrence of hepatocellular carcinoma (HCC) following resection (Cheng et al., 2022). Tumor acidity neutralizer effectively reduced the infiltration degree of immunosuppressive cells in the TIME while NETs lyase degraded NETs, which also enhanced NK cell infiltration. Hydrogels can also target NK cells by delivering and releasing anti-TIGIT monoclonal antibody (aTIGIT) to increase the amount of NK cells. A study exhibited that the MMP-2 degradable hydrogel promoted the formation of immunogenic TIME and reversed the exhaustion of NK cells and effector T cells by releasing DOX and aTIGIT (Tian et al., 2022), thus depressing tumor growth. In addition, targeting NKG2D based on hydrogels is an important way to activate the anti-tumor activity of NK cells in the TIME (Jiao et al., 2024).
4.4 Targeted modulation of MDSCs by hydrogels
MDSCs are a heterogeneous population, exerting immunosuppressive function in the TIME. MDSCs inhibit immunity in the TIME through reactive oxygen species (ROS), production of nitric oxide (NO), TGF-β, PD-L1 expression, and so on (Li et al., 2021). Multiple signal transduction pathways enhance the infiltration of MDSCs in the TIME, such as CXC chemokine ligand 13/CXC chemokine receptor type 5 (CXCL13/CXCR5) signaling, and chemokine ligand 15/chemokine receptor 1 (CCL15/CCR1) signaling (Li B.-H. et al., 2020). For example, CXCL13/CXCR5 can participate in MDSC recruitment and help tumor cells escape T cell immunity (Li B.-H. et al., 2020; Hussain et al., 2019). In addition, IL-6 is regarded as a major regulator of MDSC accumulation and activation, which is capable of promoting tumor cell proliferation and metastasis (Weber et al., 2021). Therefore, regulating the above signaling pathways or molecules maybe the important methods for targeting MDSCs.
4.5 Targeted modulation of T cells by hydrogels
The downregulation of MHC-I of tumor cells impedes the recognition for CD8+ T cells, leading to immune escape. Hydrogels loaded with immunomodulators can promote the recognition of tumor cells by CD8+ T cells by upregulating MHC-I (Zhao et al., 2023). A study found that in the hydrogels, 4-1BB antibody promoted T cell mitochondrial biogenesis and the axitinib-lowered hypoxia synergistically reversed T cell exhaustion (Zhang et al., 2022). At the same time, PF-06446846 amplified MHC-I expression to improve the recognition of tumor cells by T cells.
The immune activity of T cells can be activated by hydrogels loaded with cytokines (Liu et al., 2020; Del Río et al., 2020). Cytokines are soluble low molecular weight proteins secreted by T cells, B cells, NK cells, etc. IFN-α can regulate the proliferation and differentiation of T cells and increase the level of IFN-γ to promote apoptosis of tumor cells (Brinkmann et al., 1993). IFN-α can also promote the expression of MHC-I molecules and enhance antigen presentation to improve the recognition efficiency of tumor cells. IFN-α2b is an important subtype of IFN-α. A hydrogel encapsulated with the enhancer IFN-α2b in combination with T cell transfer and low-dose irradiation (LDI) maintained a high activity of IFN-α2b and enhanced the recruitment of T cells in the TIME (Liu et al., 2020). Finally, the tumor volume was reduced. The specific process is shown in Figure 3. Besides, the hydrogel loaded with CCL21 was found that it can recruit T cells for homing and infiltration (Del Río et al., 2020). Therefore, hydrogels can solve the challenges of low immune efficiency caused by insufficient expansion of T cells in tumor immunotherapy (Fesnak et al., 2016).
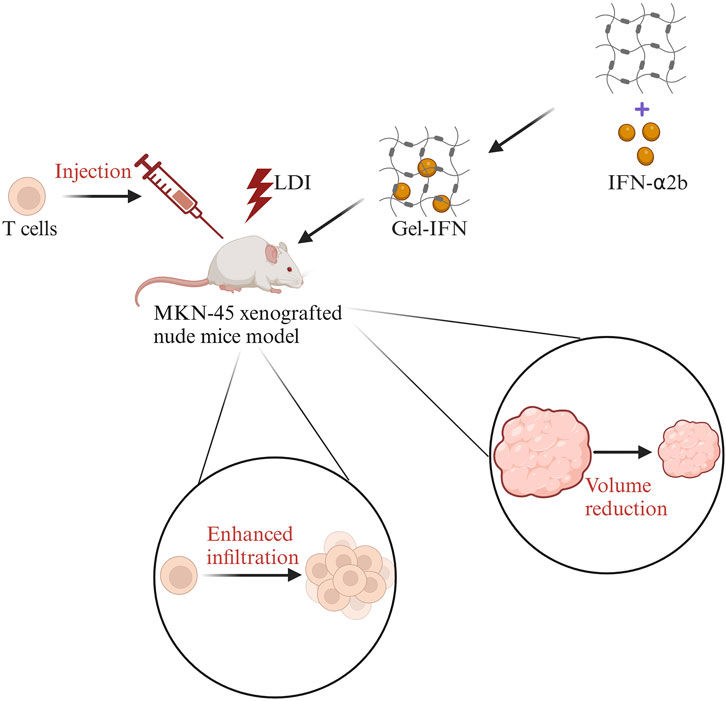
Figure 3. The sketch map of IFN-α2b hydrogels combined with T cell transfer and low-dose irradiation (LDI) in the treatment of gastric cancer. First, LDI was used in the MKN-45 xenografted nude mice model, then T cells were injected in the mice model, followed by IFN-α2b-loaded hydrogels (Gel-IFN). Finally, the enhanced infiltration of T cells and reduced tumor volume were observed. Modified from ref. Liu et al. (2020). The figure is made with biorender (https://biorender.com/).
Targeting immune checkpoints based on hydrogels is also a very important mechanism. Hydrogels can deliver immune checkpoint inhibitors to enhance the immune activation of T cells. Here, we mainly focus on the PD-1/PD-L1 checkpoint pathway. PD-1 is an important immunosuppressive molecule that can be expressed on the surface of activated T cells and B cells (Keir et al., 2008). When PD-1 binds to its ligand PD-L1, it produces inhibitory signals that inhibit the proliferation of T cells, cytokine release, and killing effect (Liu et al., 2021; Han et al., 2020). This binding can also initiate the programmed death of T cells (Liu et al., 2021; Han et al., 2020). Therefore, blocking the PD-1/PD-L1 pathway can restore immune surveillance and promote the recognition and killing of tumor cells by T cells. Moreover, T cell therapy combined with PD-1/PD-L1 checkpoint inhibitors can produce an obvious tumor cell-killing effect. For example, a hydrogel loaded with CAR-T cells, human platelets coupled with aPD-L1, IL-15, etc., can reshape the TIME and promote CAR-T cell infiltration (Hu et al., 2021).
Furthermore, L-arginine plays a crucial role in the proliferation, differentiation, and survival of T cells, enhancing their anti-tumor immune response (Geiger et al., 2016). Arginase1 (ARG1) exhibits immunosuppression by reducing the level of L-arginine (Geiger et al., 2016). Norvaline is an inhibitor of ARG1, and hydrogels can provide a powerful platform for norvaline to block the ARG1 pathway to regulate T cells. Hydrogels loaded with norvaline and doxorubicin hydrochloride achieved high drug loading and controlled release of norvaline to block the ARG1 pathway, showing promising efficacy in primary tumor ablation and inhibition of tumor metastasis (Ren et al., 2021).
As an important subtype of T cells, Tregs can mediate inhibition through regulation of DCs, cytolysis of target cells, and secretion of anti-inflammatory cytokines (Tanaka and Sakaguchi, 2017; Kim et al., 2020). Moreover, Tregs can not only interact directly with T cells and NK cells, but also indirectly inhibit the anti-tumor activity of T cells and NK cells through producing immunomodulatory cytokines such as IL-10 and TGF-β (Liu Y. et al., 2022). In the TIME, Tregs can express cytotoxic T-lymphocyte-associated antigen 4 (CTLA-4) molecules to promote immune escape (Watanabe et al., 2023). Immunosuppression function of Tregs can be reversed by the hydrogel loaded with CTLA-4 antibodies. In summary, targeting Tregs based on hydrogels maybe also an important method of enhancing anti-tumor immune responses (Pitt et al., 2016).
4.6 Targeted modulation of B cells by hydrogels
At present, there are few studies on how hydrogels target B cells, but some studies have proved that hydrogels have a positive regulatory effect on humoral immunity. The study found that sustained exposure to vaccine components based on polymer nanoscale (PNP) hydrogels enhanced the degree and time of germinal center responses in lymph nodes, promoting antigen-specific antibody affinity (Roth et al., 2020). Finally, the magnitude, duration, and quality of humoral immunity were enhanced. It provides an important reference for regulating B cells in the TIME by hydrogels to enhance anti-tumor immune response.
4.7 Hydrogel platforms or formulations in cancer immunotherapy
Among the various delivery systems and platforms, hydrogel platforms and hydrogel-based formulations have demonstrated considerable potential in enhancing the efficacy and safety of cancer immunotherapy (He et al., 2024). Recent advancements in hydrogel technology have led to the development of more sophisticated formulations, such as stimuli-responsive hydrogels that can release therapeutic agents in response to specific cues, such as pH changes or enzymatic activity (Solanki and Bhatia, 2024; Andrade et al., 2021). As research in this field continues to evolve, we anticipate the development of even more innovative and personalized hydrogel-based therapeutic strategies that will further improve immunotherapy outcomes.
4.7.1 TransCon hydrogel
TransCon hydrogel represents an innovative drug delivery system that merges the sustained-release properties and biocompatibility of hydrogels to achieve continuous and stable delivery of immunomodulators or other therapeutic agents directly to the tumor site (Lessmann et al., 2023). This system enhances drug bioavailability and minimizes systemic side effects by precisely regulating the rate and duration of drug release. In the context of cancer therapy, TransCon hydrogel is employed to administer a variety of immunomodulators, such as LR7/8 Agonist (Zúñiga et al., 2022) and IL-2 β/γ (Rosen et al., 2022), aiming to bolster the body’s immune response and facilitate the destruction of tumor cells. This targeted approach ensures that the therapeutic agents are delivered where they are most needed, optimizing their efficacy while reducing off-target effects.
4.7.2 STM-416
STM-416 is an injectable, biodegradable hydrogel formulation containing the TLR7/8 agonist resiquimod has been developed for intra-tumoral extended-release. The hydrogel serves as a carrier, offering a stable environment for drug release and providing a layer of protection to the immunomodulator against degradation by the body’s internal environment, thereby enhancing both its therapeutic effect and safety profile. It undergoing Phase 1/2a Study for bladder cancer, when administered during bladder tumor surgery, it locally and sustainably releases resiquimod, which binds to TLR7 and eight on immune cells like dendritic cells, macrophages, and B lymphocytes. This activates the TLR signaling pathway, leading to the induction of NF-kB and other transcription factors, enhanced Th1 immune responses, and increased cytokine production, particularly INF-a. Activation of dendritic cells further stimulates CTL and B-lymphocyte responses, potentially causing tumor cell lysis.
Collectively, the application of hydrogel platforms and hydrogel/immunomodulator formulations in cancer treatment introduces new avenues for precision medicine. By precisely controlling the release and distribution of drugs, these technologies can more effectively activate the immune system, enabling precise targeting of tumor cells. This results in improved treatment outcomes, reduced side effects, and ultimately, a better treatment experience and quality of life for patients. As research in this field continues to advance, the potential for these innovative approaches to revolutionize cancer treatment becomes increasingly evident.
5 Conclusion
TIME (Tumor Microenvironment) exhibits an immunosuppressive state that favors tumor progression. Hydrogels stand out as exceptional biomaterials in the realm of tumor immunotherapy due to their unique combination of biocompatibility and degradability. Furthermore, hydrogels have the capability to locally deliver immunomodulators, minimizing side effects, releasing them in a controlled manner directly to the tumor site, and preserving their bioactivity. The utilization of hydrogels can enhance the infiltration of immune cells into the TIME and activate the immune responses of dendritic cells (DCs), natural killer (NK) cells, T cells, and other immune cells. The regulatory mechanisms of hydrogels on innate and adaptive immunity within the TIME are intricate and diverse. Gaining a deeper understanding of how hydrogels regulate these immune cells in the TIME provides valuable insights for the optimal design of hydrogels and paves the way for the development of more efficient and safer tumor treatment strategies in the future.
Looking ahead, it is anticipated that the regulatory mechanisms of hydrogels in the TIME will be further explored with the advent of new drugs and technologies.
5.1 Precise drug delivery and controlled release
In the future, the primary application of hydrogels in TIME immune regulation will be as a precise drug delivery system. By adjusting the chemical composition, crosslinking strategies, and physical structure of hydrogels, researchers can design responsive hydrogels that release drugs in response to specific biological and pathological stimuli (such as pH, temperature, reactive oxygen species, etc.). This precise controlled release capability will significantly enhance the efficiency and safety of immunotherapy, reduce systemic adverse reactions, and broaden the therapeutic window.
5.2 Enhancement of immune cell infiltration and activation
The application of hydrogels is expected to significantly improve the infiltration and activation of immune cells in the TIME. By mimicking the properties of the extracellular matrix (ECM), hydrogels can provide a microenvironment conducive to the growth and activation of immune cells. Furthermore, hydrogels can load and slowly release cytokines and chemokines, attracting and activating immune cells such as dendritic cells (DCs), natural killer (NK) cells, T cells, etc., thereby enhancing the anti-tumor immune response.
5.3 Induction of immune tolerance and reduction of immune rejection
In cell therapy, the use of allogeneic cells faces the challenge of immune rejection. Hydrogels offer an innovative solution to mitigate this rejection. By encapsulating transplanted cells within a biocompatible hydrogel matrix, an immune-isolating physical barrier can be formed between the allogeneic cells and the host, effectively protecting the transplanted cells from cell-cell contact-mediated immune reactions. This strategy is expected to improve the success rate of cell therapy and promote its clinical application.
5.4 Synergistic combination immunotherapy
Future research directions also include exploring the application of hydrogels in synergistic combination immunotherapy. Through the hydrogel platform, multiple immunomodulators or cell therapies can be delivered simultaneously to achieve synergistic therapeutic effects. This combination strategy is expected to overcome the limitations of monotherapy and improve the overall efficacy of tumor immunotherapy.
5.5 Integration of new materials and technologies
With the continuous development of materials science and biotechnology, more new materials and technologies will be applied to the design and preparation of hydrogels in the future. For example, the emergence of novel hydrogel materials such as supramolecular hydrogels and smart hydrogels will provide more functions and broader application prospects for TIME immune regulation. At the same time, the integration of advanced biomanufacturing technologies such as gene editing and nanotechnology will further promote the development of hydrogels in the field of tumor immunotherapy.
5.6 Personalized medicine and precision medicine
The future prospects for hydrogels in TIME immune regulation also include their application in personalized medicine and precision medicine. By combining factors such as the patient’s genetic information, tumor type, stage, and immune status, customized hydrogel treatment plans can be designed for individual patients. This personalized medicine strategy will further improve the specificity and effectiveness of tumor immunotherapy.
In summary, the future prospects for hydrogels in TIME immune regulation are filled with hope and challenges. With the continuous progress of science and technology and the deepening of clinical applications, it is believed that hydrogels will play an increasingly important role in the field of tumor immunotherapy, bringing more blessings to cancer patients.
Author contributions
YL: Writing–original draft, Writing–review and editing. FL: Writing–original draft. YZ: Writing–original draft. LL: Writing–review and editing. HY: Writing–review and editing. SZ: Writing–review and editing. WY: Conceptualization, Data curation, Funding acquisition, Project administration, Supervision, Writing–original draft, Writing–review and editing.
Funding
The author(s) declare that financial support was received for the research, authorship, and/or publication of this article. This work was supported by the National Natural Science Foundation of China (82303238, 82373336, 82402108); Science and Technology Support Program of Sichuan Province (2024NSFSC1945, 2024NSFSC1761, 2023NSFSC0677, 2022YFQ0077, MZGC20240074), The Third People’s Hospital of Chengdu Scientific Research Project (2023PI18) and The Third People’s Hospital of Chengdu Clinical Research Program (CSY-YN-01-2023-013, CSY-YN-01-2023-025, CSY-YN-01-2023-005, CSY-YN-03-2024-003), Sichuan University “From 0 to 1” Innovative Research Project (2023SCUH0024), Sichuan Province Special Funding Project for Postdoctoral Fellows (TB2024041); Science and Technology Support Program of Chengdu (2024-YF05-01028-SN, 2024-YF05-00748-SN); Health Commission of Chengdu Medical Research Program (2024014, 2024063 and 2024291); Henan Province Postdoctoral Research Grant (202102095), and the 2023 Henan Province’s foreign professor introducing project (HNGD2023025).
Conflict of interest
The authors declare that the research was conducted in the absence of any commercial or financial relationships that could be construed as a potential conflict of interest.
Publisher’s note
All claims expressed in this article are solely those of the authors and do not necessarily represent those of their affiliated organizations, or those of the publisher, the editors and the reviewers. Any product that may be evaluated in this article, or claim that may be made by its manufacturer, is not guaranteed or endorsed by the publisher.
Generative AI statement
The author(s) declare that no Generative AI was used in the creation of this manuscript.
References
Ahmed, E. M. (2015). Hydrogel: preparation, characterization, and applications: a review. J. Adv. Res. 6, 105–121. doi:10.1016/j.jare.2013.07.006
Almawash, S., Osman, S. K., Mustafa, G., and El Hamd, M. A. (2022). Current and future prospective of injectable hydrogels—design challenges and limitations. Pharmaceuticals 15, 371. doi:10.3390/ph15030371
Andrade, F., Roca-Melendres, M. M., Durán-Lara, E. F., Rafael, D., and Schwartz, S. (2021). Stimuli-responsive hydrogels for cancer treatment: the role of pH, light, ionic strength and magnetic field. Cancers (Basel) 13, 1164. doi:10.3390/cancers13051164
Ashfaq, A., Clochard, M. C., Coqueret, X., Dispenza, C., Driscoll, M. S., Ulański, P., et al. (2020). Polymerization reactions and modifications of polymers by ionizing radiation. Polymers 12, 2877. doi:10.3390/polym12122877
Bakr, M. A., Amr, S. A., Mohamed, S. A., Hamed, H. B., Abd El-Rahman, A. M., Mostafa, M. A., et al. (2016). Comparison between the effects of intravenous morphine, tramadol, and ketorolac on stress and immune responses in patients undergoing modified radical mastectomy. Clin. J. Pain 32, 889–897. doi:10.1097/AJP.0000000000000338
Balakrishnan, B., Liang, Q., Fenix, K., Tamang, B., Hauben, E., Ma, L., et al. (2021). Combining the anticancer and immunomodulatory effects of Astragalus and shiitake as an integrated therapeutic approach. Nutrients 13, 2564. doi:10.3390/nu13082564
Balan, S., Saxena, M., and Bhardwaj, N. (2019). Dendritic cell subsets and locations. Int. Rev. Cell Mol. Biol. 348, 1–68. doi:10.1016/bs.ircmb.2019.07.004
Bald, T., Krummel, M. F., Smyth, M. J., and Barry, K. C. (2020). The NK cell–cancer cycle: advances and new challenges in NK cell–based immunotherapies. Nat. Immunol. 21, 835–847. doi:10.1038/s41590-020-0728-z
Banchereau, J., Briere, F., Caux, C., Davoust, J., Lebecque, S., Liu, Y.-J., et al. (2000). Immunobiology of dendritic cells. Annu. Rev. Immunol. 18, 767–811. doi:10.1146/annurev.immunol.18.1.767
Basu, A., Ramamoorthi, G., Albert, G., Gallen, C., Beyer, A., Snyder, C., et al. (2021). Differentiation and regulation of TH cells: a balancing act for cancer immunotherapy. Front. Immunol. 12, 669474. doi:10.3389/fimmu.2021.669474
Baumgartner, C. K., Ebrahimi-Nik, H., Iracheta-Vellve, A., Hamel, K. M., Olander, K. E., Davis, T. G. R., et al. (2023). The PTPN2/PTPN1 inhibitor ABBV-CLS-484 unleashes potent anti-tumour immunity. Nature 622, 850–862. doi:10.1038/s41586-023-06575-7
Behzadi, P., García-Perdomo, H. A., and Karpiński, T. M. (2021). Toll-like receptors: general molecular and structural biology. J. Immunol. Res. 2021, 9914854–9914921. doi:10.1155/2021/9914854
Bilotta, M. T., Antignani, A., and Fitzgerald, D. J. (2022). Managing the TME to improve the efficacy of cancer therapy. Front. Immunol. 13, 954992. doi:10.3389/fimmu.2022.954992
Biswas, S. K., and Mantovani, A. (2010). Macrophage plasticity and interaction with lymphocyte subsets: cancer as a paradigm. Nat. Immunol. 11, 889–896. doi:10.1038/ni.1937
Brinkmann, V., Geiger, T., Alkan, S., and Heusser, C. H. (1993). Interferon alpha increases the frequency of interferon gamma-producing human CD4+ T cells. J. Exp. Med. 178, 1655–1663. doi:10.1084/jem.178.5.1655
Buwalda, S. J., Vermonden, T., and Hennink, W. E. (2017). Hydrogels for therapeutic delivery: current developments and future directions. Biomacromolecules 18, 316–330. doi:10.1021/acs.biomac.6b01604
Cao, H., Duan, L., Zhang, Y., Cao, J., and Zhang, K. (2021). Current hydrogel advances in physicochemical and biological response-driven biomedical application diversity. Signal Transduct. Target. Ther. 6, 426. doi:10.1038/s41392-021-00830-x
Cekic, C., Day, Y.-J., Sag, D., and Linden, J. (2014). Myeloid expression of adenosine A2A receptor suppresses T and NK cell responses in the solid tumor microenvironment. Cancer Res. 74, 7250–7259. doi:10.1158/0008-5472.CAN-13-3583
Chandnani, N., Gupta, I., Mandal, A., and Sarkar, K. (2024). Participation of B cell in immunotherapy of cancer. Pathol. Res. Pract. 255, 155169. doi:10.1016/j.prp.2024.155169
Chao, Y., Chen, Q., and Liu, Z. (2020). Smart injectable hydrogels for cancer immunotherapy. Adv. Funct. Mater. 30, 1902785. doi:10.1002/adfm.201902785
Chen, F., Li, T., Zhang, H., Saeed, M., Liu, X., Huang, L., et al. (2023). Acid-ionizable iron nanoadjuvant augments STING activation for personalized vaccination immunotherapy of cancer. Adv. Mater. 35, e2209910. doi:10.1002/adma.202209910
Chen, W., Wang, C., Liu, W., Zhao, B., Zeng, Z., Long, F., et al. (2023). A matrix-metalloproteinase-responsive hydrogel system for modulating the immune microenvironment in myocardial infarction. Adv. Mater. 35, 2209041. doi:10.1002/adma.202209041
Cheng, Y., Gong, Y., Chen, X., Zhang, Q., Zhang, X., He, Y., et al. (2022). Injectable adhesive hemostatic gel with tumor acidity neutralizer and neutrophil extracellular traps lyase for enhancing adoptive NK cell therapy prevents post-resection recurrence of hepatocellular carcinoma. Biomaterials 284, 121506. doi:10.1016/j.biomaterials.2022.121506
Chin, M. H., Norman, M. D., Gentleman, E., Coppens, M.-O., and Day, R. M. (2020). A hydrogel-integrated culture device to interrogate T cell activation with physicochemical cues. ACS Appl. Mater. Interfaces 12, 47355–47367. doi:10.1021/acsami.0c16478
Cruceriu, D., Baldasici, O., Balacescu, O., and Berindan-Neagoe, I. (2020). The dual role of tumor necrosis factor-alpha (TNF-α) in breast cancer: molecular insights and therapeutic approaches. Cell. Oncol. 43, 1–18. doi:10.1007/s13402-019-00489-1
Cui, R., Wu, Q., Wang, J., Zheng, X., Ou, R., Xu, Y., et al. (2021). Hydrogel-by-design: smart delivery system for cancer immunotherapy. Front. Bioeng. Biotech. 9, 723490. doi:10.3389/fbioe.2021.723490
de la Portilla, F., Dios-Barbeito, S., Maestre-Sánchez, M. V., Vázquez-Monchul, J. M., García-Cabrera, A. M., Ramallo, I., et al. (2021). Feasibility and safety of calcium alginate hydrogel sealant for the treatment of cryptoglandular fistula-in-ano: phase I/IIa clinical trial. Colorectal Dis. 23, 1499–1506. doi:10.1111/codi.15608
Del Río, E. P., Santos, F., Rodriguez, X. R., Martínez-Miguel, M., Roca-Pinilla, R., Arís, A., et al. (2020). CCL21-loaded 3D hydrogels for T cell expansion and differentiation. Biomaterials 259, 120313. doi:10.1016/j.biomaterials.2020.120313
Deng, J., Liu, L., Sun, J., Ma, Y., and Li, L. (2022). Effectiveness and safety of chemotherapy combined with immunomodulatory therapies for multiple myeloma: a protocol for systematic review and meta-analysis. Medicine 101, e29093. doi:10.1097/MD.0000000000029093
Dong, X., Yang, A., Bai, Y., Kong, D., and Lv, F. (2020). Dual fluorescence imaging-guided programmed delivery of doxorubicin and CpG nanoparticles to modulate tumor microenvironment for effective chemo-immunotherapy. Biomaterials 230, 119659. doi:10.1016/j.biomaterials.2019.119659
Ellyard, J. I., Simson, L., and Parish, C. R. (2007). Th2-mediated anti-tumour immunity: friend or foe? Tissue antigens 70, 1–11. doi:10.1111/j.1399-0039.2007.00869.x
Farhood, B., Najafi, M., and Mortezaee, K. (2019). CD8+ cytotoxic T lymphocytes in cancer immunotherapy: a review. J. Cell. Physiol. 234, 8509–8521. doi:10.1002/jcp.27782
Fesnak, A. D., June, C. H., and Levine, B. L. (2016). Engineered T cells: the promise and challenges of cancer immunotherapy. Nat. Rev. Cancer 16, 566–581. doi:10.1038/nrc.2016.97
Flores-Valenzuela, L. E., González-Fernández, J. V., and Carranza-Oropeza, M. V. (2023). Hydrogel applicability for the industrial effluent treatment: a systematic review and bibliometric analysis. Polymers 15, 2417. doi:10.3390/polym15112417
Fridman, W. H., Meylan, M., Petitprez, F., Sun, C. M., Italiano, A., and Sautès-Fridman, C. (2022). B cells and tertiary lymphoid structures as determinants of tumour immune contexture and clinical outcome. Nat. Rev. Clin. Oncol. 19, 441–457. doi:10.1038/s41571-022-00619-z
Fridman, W. H., Pagès, F., Sautès-Fridman, C., and Galon, J. (2012). The immune contexture in human tumours: impact on clinical outcome. Nat. Rev. Cancer 12, 298–306. doi:10.1038/nrc3245
Fu, Q., Fu, T.-M., Cruz, A. C., Sengupta, P., Thomas, S. K., Wang, S., et al. (2016). Structural basis and functional role of intramembrane trimerization of the Fas/CD95 death receptor. Mol. Cell 61, 602–613. doi:10.1016/j.molcel.2016.01.009
Gabrilovich, D. I., and Nagaraj, S. (2009). Myeloid-derived suppressor cells as regulators of the immune system. Nat. Rev. Immunol. 9, 162–174. doi:10.1038/nri2506
Gardner, A., de Mingo Pulido, Á., and Ruffell, B. (2020). Dendritic cells and their role in immunotherapy. Front. Immunol. 11, 531484. doi:10.3389/fimmu.2020.00924
Gaspani, L., Bianchi, M., Limiroli, E., Panerai, A. E., and Sacerdote, P. (2002). The analgesic drug tramadol prevents the effect of surgery on natural killer cell activity and metastatic colonization in rats. J. Neuroimmunol. 129, 18–24. doi:10.1016/s0165-5728(02)00165-0
Geiger, R., Rieckmann, J. C., Wolf, T., Basso, C., Feng, Y., Fuhrer, T., et al. (2016). L-arginine modulates T cell metabolism and enhances survival and anti-tumor activity. Cell 167, 829–842. doi:10.1016/j.cell.2016.09.031
Goh, M., Du, M., Peng, W. R., Saw, P. E., and Chen, Z. (2024). Advancing burn wound treatment: exploring hydrogel as a transdermal drug delivery system. Drug Deliv. 31, 2300945. doi:10.1080/10717544.2023.2300945
Gulley, J. L., Schlom, J., Barcellos-Hoff, M. H., Wang, X. J., Seoane, J., Audhuy, F., et al. (2022). Dual inhibition of TGF-β and PD-L1: a novel approach to cancer treatment. Mol. Oncol. 16, 2117–2134. doi:10.1002/1878-0261.13146
Hagemann, T., Lawrence, T., McNeish, I., Charles, K. A., Kulbe, H., Thompson, R. G., et al. (2008). “Re-educating” tumor-associated macrophages by targeting NF-kappaB. J. Exp. Med. 205, 1261–1268. doi:10.1084/jem.20080108
Han, Y., Liu, D., and Li, L. (2020). PD-1/PD-L1 pathway: current researches in cancer. Am. J. Cancer Res. 10, 727–742.
He, W., Zhang, Y., Qu, Y., Liu, M., Li, G., Pan, L., et al. (2024). Research progress on hydrogel-based drug therapy in melanoma immunotherapy. BMB Rep. 57, 71–78. doi:10.5483/BMBRep.2023-0160
Ho, T.-C., Chang, C.-C., Chan, H.-P., Chung, T.-W., Shu, C.-W., Chuang, K.-P., et al. (2022). Hydrogels: properties and applications in biomedicine. Molecules 27, 2902. doi:10.3390/molecules27092902
Hu, Q., Li, H., Archibong, E., Chen, Q., Ruan, H., Ahn, S., et al. (2021). Inhibition of post-surgery tumour recurrence via a hydrogel releasing CAR-T cells and anti-PDL1-conjugated platelets. Nat. Biomed. Eng. 5, 1038–1047. doi:10.1038/s41551-021-00712-1
Hussain, M., Adah, D., Tariq, M., Lu, Y., Zhang, J., and Liu, J. (2019). CXCL13/CXCR5 signaling axis in cancer. Life Sci. 227, 175–186. doi:10.1016/j.lfs.2019.04.053
Hutomo, D. I., Amir, L., Suniarti, D. F., Bachtiar, E. W., and Soeroso, Y. (2023). Hydrogel-based biomaterial as a scaffold for gingival regeneration: a systematic review of in vitro studies. Polymers 15, 2591. doi:10.3390/polym15122591
Jiao, D., Hao, M., Sun, R., Ren, X., Wei, Y., Ding, M., et al. (2024). Dynamic hybrid module-driven NK cell stimulation and release for tumor immunotherapy. Nano Lett. 24, 5481–5489. doi:10.1021/acs.nanolett.4c00425
Kabelitz, D., Zarobkiewicz, M., Heib, M., Serrano, R., Kunz, M., Chitadze, G., et al. (2022). Signal strength of STING activation determines cytokine plasticity and cell death in human monocytes. Sci. Rep. 12, 17827. doi:10.1038/s41598-022-20519-7
Kansal, V., Burnham, A. J., Kinney, B. L. C., Saba, N. F., Paulos, C., Lesinski, G. B., et al. (2023). Statin drugs enhance responses to immune checkpoint blockade in head and neck cancer models. J. Immunother. Cancer 11, e005940. doi:10.1136/jitc-2022-005940
Kärre, K. (2002). NK cells, MHC class I molecules and the missing self. Scand. J. Immunol. 55, 221–228. doi:10.1046/j.1365-3083.2002.01053.x
Keir, M. E., Butte, M. J., Freeman, G. J., and Sharpe, A. H. (2008). PD-1 and its ligands in tolerance and immunity. Annu. Rev. Immunol. 26, 677–704. doi:10.1146/annurev.immunol.26.021607.090331
Khan, F., Atif, M., Haseen, M., Kamal, S., Khan, M. S., Shahid, S., et al. (2022). Synthesis, classification and properties of hydrogels: their applications in drug delivery and agriculture. J. Mater. Chem. B 10, 170–203. doi:10.1039/d1tb01345a
Kim, J., Hope, C. M., Gantumur, N., Perkins, G. B., Stead, S. O., Yue, Z., et al. (2020). Encapsulation of human natural and induced regulatory T-cells in IL-2 and CCL1 supplemented alginate-GelMA hydrogel for 3D bioprinting. Adv. Funct. Mater. 30, 2000544. doi:10.1002/adfm.202000544
Kiselevskiy, M. V., Anisimova, N. Y., Ustyuzhanina, N. E., Vinnitskiy, D. Z., Tokatly, A. I., Reshetnikova, V. V., et al. (2022). Perspectives for the use of Fucoidans in clinical oncology. Int. J. Mol. Sci. 23, 11821. doi:10.3390/ijms231911821
Kuramochi, T., Sano, M., Kajiwara, I., Oshima, Y., Itaya, T., Kim, J., et al. (2024). Effects of tramadol via a µ-opioid receptor on pancreatic ductal adenocarcinoma in vitro and in vivo. Reg. Anesth. Pain Med. 49, 200–208. doi:10.1136/rapm-2023-104511
Lee, K. H., Yen, W. C., Lin, W. H., Wang, P. C., Lai, Y. L., Su, Y. C., et al. (2021). Discovery of BPR1R024, an orally active and selective CSF1R inhibitor that exhibits antitumor and immunomodulatory activity in a murine colon tumor model. J. Med. Chem. 64, 14477–14497. doi:10.1021/acs.jmedchem.1c01006
Lei, L., Huang, D., Gao, H., He, B., Cao, J., and Peppas, N. A. (2022). Hydrogel-guided strategies to stimulate an effective immune response for vaccine-based cancer immunotherapy. Sci. Adv. 8, eadc8738. doi:10.1126/sciadv.adc8738
Lessmann, T., Jones, S. A., Voigt, T., Weisbrod, S., Kracker, O., Winter, S., et al. (2023). Degradable hydrogel for sustained localized delivery of anti-tumor drugs. J. Pharm. Sci. 112, 2843–2852. doi:10.1016/j.xphs.2023.05.018
Li, B.-H., Garstka, M. A., and Li, Z.-F. (2020). Chemokines and their receptors promoting the recruitment of myeloid-derived suppressor cells into the tumor. Mol. Immunol. 117, 201–215. doi:10.1016/j.molimm.2019.11.014
Li, C., Jiang, P., Wei, S., Xu, X., and Wang, J. (2020). Regulatory T cells in tumor microenvironment: new mechanisms, potential therapeutic strategies and future prospects. Mol. Cancer 19, 116–123. doi:10.1186/s12943-020-01234-1
Li, T., Liu, T., Zhu, W., Xie, S., Zhao, Z., Feng, B., et al. (2021). Targeting MDSC for immune-checkpoint blockade in cancer immunotherapy: current progress and new prospects. Clin. Med. Insights Oncol. 15, 11795549211035540. doi:10.1177/11795549211035540
Li, T., Zhang, W., Niu, M., Wu, Y., Deng, X., and Zhou, J. (2024). STING agonist inflames the cervical cancer immune microenvironment and overcomes anti-PD-1 therapy resistance. Front. Immunol. 15, 1342647. doi:10.3389/fimmu.2024.1342647
Li, X., and Su, X. (2018). Multifunctional smart hydrogels: potential in tissue engineering and cancer therapy. J. Mater. Chem. B 6, 4714–4730. doi:10.1039/c8tb01078a
Liang, Y., He, J., and Guo, B. (2021). Functional hydrogels as wound dressing to enhance wound healing. ACS nano 15, 12687–12722. doi:10.1021/acsnano.1c04206
Lin, Z., Wang, Q., Jiang, T., Wang, W., and Zhao, J. J. (2023). Targeting tumor-associated macrophages with STING agonism improves the antitumor efficacy of osimertinib in a mouse model of EGFR-mutant lung cancer. Front. Immunol. 14, 1077203. doi:10.3389/fimmu.2023.1077203
Liu, J., Chen, Z., Li, Y., Zhao, W., Wu, J., and Zhang, Z. (2021). PD-1/PD-L1 checkpoint inhibitors in tumor immunotherapy. Front. Pharmacol. 12, 731798. doi:10.3389/fphar.2021.731798
Liu, L. C., Wu, Z. S., Chen, J. L., Wu, Z. F., Lai, H. C., and Huang, Y. H. (2022). Mitochondrial dysfunction involved in the cytotoxicity of tramadol in human endometrial carcinoma cells. Int. J. Mol. Sci. 24, 99. doi:10.3390/ijms24010099
Liu, Q., Zhang, D., Qian, H., Chu, Y., Yang, Y., Shao, J., et al. (2020). Superior antitumor efficacy of IFN-α2b-incorporated photo-cross-linked hydrogels combined with T cell transfer and low-dose irradiation against gastric cancer. Int. J. Nanomed. 15, 3669–3680. doi:10.2147/IJN.S249174
Liu, Y., Geng, Y., Yue, B., Lo, P.-C., Huang, J., and Jin, H. (2022). Injectable hydrogel as a unique platform for antitumor therapy targeting immunosuppressive tumor microenvironment. Front. Immunol. 12, 832942. doi:10.3389/fimmu.2021.832942
Liu, Y., Xiao, L., Joo, K.-I., Hu, B., Fang, J., and Wang, P. (2014). In situ modulation of dendritic cells by injectable thermosensitive hydrogels for cancer vaccines in mice. Biomacromolecules 15, 3836–3845. doi:10.1021/bm501166j
Mannino, M. H., Zhu, Z., Xiao, H., Bai, Q., Wakefield, M. R., and Fang, Y. (2015). The paradoxical role of IL-10 in immunity and cancer. Cancer Lett. 367, 103–107. doi:10.1016/j.canlet.2015.07.009
Masad, R. J., Haneefa, S. M., Mohamed, Y. A., Al-Sbiei, A., Bashir, G., Fernandez-Cabezudo, M. J., et al. (2021). The immunomodulatory effects of honey and associated flavonoids in cancer. Nutrients 13, 1269. doi:10.3390/nu13041269
Miggelbrink, A. M., Jackson, J. D., Lorrey, S. J., Srinivasan, E. S., Waibl-Polania, J., Wilkinson, D. S., et al. (2021). CD4 T-cell exhaustion: does it exist and what are its roles in cancer? Clin. Cancer Res. 27, 5742–5752. doi:10.1158/1078-0432.CCR-21-0206
Myers, J. A., and Miller, J. S. (2021). Exploring the NK cell platform for cancer immunotherapy. Nat. Rev. Clin. Oncol. 18, 85–100. doi:10.1038/s41571-020-0426-7
Ngambenjawong, C., Gustafson, H. H., and Pun, S. H. (2017). Progress in tumor-associated macrophage (TAM)-targeted therapeutics. Adv. Drug Deliv. Rev. 114, 206–221. doi:10.1016/j.addr.2017.04.010
Ni, K., Xu, Z., Culbert, A., Luo, T., Guo, N., Yang, K., et al. (2022). Author Correction: synergistic checkpoint-blockade and radiotherapy-radiodynamic therapy via an immunomodulatory nanoscale metal-organic framework. Nat. Biomed. Eng. 6, 1449–1450. doi:10.1038/s41551-022-00966-3
N, J., J, T., Sl, N., and Gt, B. (2021). Tertiary lymphoid structures and B lymphocytes in cancer prognosis and response to immunotherapies. Oncoimmunology 10, 1900508. doi:10.1080/2162402x.2021.1900508
Ochioni, A. C., Imbroisi Filho, R., Esteves, A. M., Leandro, J. G. B., Demaria, T. M., do Nascimento Júnior, J. X., et al. (2021). Clotrimazole presents anticancer properties against a mouse melanoma model acting as a PI3K inhibitor and inducing repolarization of tumor-associated macrophages. Biochim. Biophys. Acta Mol. Basis Dis. 1867, 166263. doi:10.1016/j.bbadis.2021.166263
Oh, S. A., Wu, D.-C., Cheung, J., Navarro, A., Xiong, H., Cubas, R., et al. (2020). PD-L1 expression by dendritic cells is a key regulator of T-cell immunity in cancer. Nat. Cancer 1, 681–691. doi:10.1038/s43018-020-0075-x
Ozbay Kurt, F. G., Lasser, S., Arkhypov, I., Utikal, J., and Umansky, V. (2023). Enhancing immunotherapy response in melanoma: myeloid-derived suppressor cells as a therapeutic target. J. Clin. Invest. 133, e170762. doi:10.1172/JCI170762
Palucka, A. K., Ueno, H., Fay, J. W., and Banchereau, J. (2007). Taming cancer by inducing immunity via dendritic cells. Immunol. Rev. 220, 129–150. doi:10.1111/j.1600-065X.2007.00575.x
Palucka, K., and Banchereau, J. (2013). Dendritic-cell-based therapeutic cancer vaccines. Immunity 39, 38–48. doi:10.1016/j.immuni.2013.07.004
Pan, B. S., Perera, S. A., Piesvaux, J. A., Presland, J. P., Schroeder, G. K., Cumming, J. N., et al. (2020). An orally available non-nucleotide STING agonist with antitumor activity. Science 369, eaba6098. doi:10.1126/science.aba6098
Pan, P.-Y., Ma, G., Weber, K. J., Ozao-Choy, J., Wang, G., Yin, B., et al. (2010). Immune stimulatory receptor CD40 is required for T-cell suppression and T regulatory cell activation mediated by myeloid-derived suppressor cells in cancer. Cancer Res. 70, 99–108. doi:10.1158/0008-5472.CAN-09-1882
Pathria, P., Louis, T. L., and Varner, J. A. (2019). Targeting tumor-associated macrophages in cancer. Trends Immunol. 40, 310–327. doi:10.1016/j.it.2019.02.003
Pitt, J., Marabelle, A., Eggermont, A., Soria, J.-C., Kroemer, G., and Zitvogel, L. (2016). Targeting the tumor microenvironment: removing obstruction to anticancer immune responses and immunotherapy. Ann. Oncol. 27, 1482–1492. doi:10.1093/annonc/mdw168
Qian, B.-Z., and Pollard, J. W. (2010). Macrophage diversity enhances tumor progression and metastasis. Cell 141, 39–51. doi:10.1016/j.cell.2010.03.014
Raskov, H., Orhan, A., Christensen, J. P., and Gögenur, I. (2021). Cytotoxic CD8+ T cells in cancer and cancer immunotherapy. Br. J. Cancer 124, 359–367. doi:10.1038/s41416-020-01048-4
Ren, X., Wang, N., Zhou, Y., Song, A., Jin, G., Li, Z., et al. (2021). An injectable hydrogel using an immunomodulating gelator for amplified tumor immunotherapy by blocking the arginase pathway. Acta Biomater. 124, 179–190. doi:10.1016/j.actbio.2021.01.041
Rosen, D. B., Kvarnhammar, A. M., Laufer, B., Knappe, T., Karlsson, J. J., Hong, E., et al. (2022). TransCon IL-2 β/γ: a novel long-acting prodrug with sustained release of an IL-2Rβ/γ-selective IL-2 variant with improved pharmacokinetics and potent activation of cytotoxic immune cells for the treatment of cancer. J. Immunother. Cancer 10, e004991. doi:10.1136/jitc-2022-004991
Roth, G. A., Gale, E. C., Alcántara-Hernández, M., Luo, W., Axpe, E., Verma, R., et al. (2020). Injectable hydrogels for sustained codelivery of subunit vaccines enhance humoral immunity. ACS Cent. Sci. 6, 1800–1812. doi:10.1021/acscentsci.0c00732
Ruan, S., Huang, Y., He, M., and Gao, H. (2022). Advanced biomaterials for cell-specific modulation and restore of cancer immunotherapy. Adv. Sci. 9, 2200027. doi:10.1002/advs.202200027
Selvin, T., Berglund, M., Lenhammar, L., Jarvius, M., Nygren, P., Fryknäs, M., et al. (2023). Phenotypic screening platform identifies statins as enhancers of immune cell-induced cancer cell death. BMC cancer 23, 164. doi:10.1186/s12885-023-10645-4
Seo, H. S., Wang, C.-P. J., Park, W., and Park, C. G. (2022). Short review on advances in hydrogel-based drug delivery strategies for cancer immunotherapy. Tissue Eng. Regen. Med. 19, 263–280. doi:10.1007/s13770-021-00369-6
Shi, Y., Li, D., He, C., and Chen, X. (2021). Design of an injectable polypeptide hydrogel depot containing the immune checkpoint blocker anti-PD-L1 and doxorubicin to enhance antitumor combination therapy. Macromol. Biosci. 21, 2100049. doi:10.1002/mabi.202100049
Solanki, R., and Bhatia, D. (2024). Stimulus-responsive hydrogels for targeted cancer therapy. Gels 10, 440. doi:10.3390/gels10070440
Sun, C., Sun, H.-y., Xiao, W.-h., Zhang, C., and Tian, Z.-g. (2015). Natural killer cell dysfunction in hepatocellular carcinoma and NK cell-based immunotherapy. Acta Pharmacol. Sin. 36, 1191–1199. doi:10.1038/aps.2015.41
Sung, H., Ferlay, J., Siegel, R. L., Laversanne, M., Soerjomataram, I., Jemal, A., et al. (2021). Global cancer statistics 2020: GLOBOCAN estimates of incidence and mortality worldwide for 36 cancers in 185 countries. Ca Cancer J. Clin. 71, 209–249. doi:10.3322/caac.21660
Tan, S., Li, D., and Zhu, X. (2020). Cancer immunotherapy: pros, cons and beyond. Biomed. Pharmacother. 124, 109821. doi:10.1016/j.biopha.2020.109821
Tanaka, A., and Sakaguchi, S. (2017). Regulatory T cells in cancer immunotherapy. Cell Res. 27, 109–118. doi:10.1038/cr.2016.151
Tanaka, R., Saito, Y., Fujiwara, Y., Jo, J.-i., and Tabata, Y. (2019). Preparation of fibrin hydrogels to promote the recruitment of anti-inflammatory macrophages. Acta Biomater. 89, 152–165. doi:10.1016/j.actbio.2019.03.011
Tang, H., Zhu, M., Qiao, J., and Fu, Y. X. (2017). Lymphotoxin signalling in tertiary lymphoid structures and immunotherapy. Cell Mol. Immunol. 14, 809–818. doi:10.1038/cmi.2017.13
Teixeira, M. O., Antunes, J. C., and Felgueiras, H. P. (2021). Recent advances in fiber-hydrogel composites for wound healing and drug delivery systems. Antibiot. (Basel) 10, 248. doi:10.3390/antibiotics10030248
Tian, T., Wang, J., Yin, F., Wu, M., He, W., Wang, X., et al. (2022). Activation of cascade-like antitumor immune responses through in situ doxorubicin stimulation and blockade of checkpoint coinhibitory receptor TIGIT. Adv. Healthc. Mater. 11, 2102080. doi:10.1002/adhm.202102080
Timmerman, M., John, M., and Levy, M. (1999). Dendritic cell vaccines for cancer immunotherapy. Annu. Rev. Med. 50, 507–529. doi:10.1146/annurev.med.50.1.507
Veglia, F., and Gabrilovich, D. I. (2017). Dendritic cells in cancer: the role revisited. Curr. Opin. Immunol. 45, 43–51. doi:10.1016/j.coi.2017.01.002
Veglia, F., Sanseviero, E., and Gabrilovich, D. I. (2021). Myeloid-derived suppressor cells in the era of increasing myeloid cell diversity. Nat. Rev. Immunol. 21, 485–498. doi:10.1038/s41577-020-00490-y
Vesaghhamedani, S., Ebrahimzadeh, F., Najafi, E., Shabgah, O. G., Askari, E., Shabgah, A. G., et al. (2022). Xanthohumol: an underestimated, while potent and promising chemotherapeutic agent in cancer treatment. Prog. Biophys. Mol. Biol. 172, 3–14. doi:10.1016/j.pbiomolbio.2022.04.002
Vesely, M. D., Zhang, T., and Chen, L. (2022). Resistance mechanisms to anti-PD cancer immunotherapy. Annu. Rev. Immunol. 40, 45–74. doi:10.1146/annurev-immunol-070621-030155
Wang, H., and Mooney, D. J. (2018). Biomaterial-assisted targeted modulation of immune cells in cancer treatment. Nat. Mater. 17, 761–772. doi:10.1038/s41563-018-0147-9
Wang, J., Li, C.-D., and Sun, L. (2020). Recent advances in molecular mechanisms of the NKG2D pathway in hepatocellular carcinoma. Biomolecules 10, 301. doi:10.3390/biom10020301
Wang, L., Guo, W., Guan, H., Yan, N., Cai, X., and Zhu, L. (2024). Tramadol suppresses growth of orthotopic liver tumors via promoting M1 macrophage polarization in the tumor microenvironment. Naunyn Schmiedeb. Arch. Pharmacol. 397, 4205–4218. doi:10.1007/s00210-023-02871-1
Wang, Z., Xu, F., Hu, J., Zhang, H., Cui, L., Lu, W., et al. (2021). Modulation of lactate-lysosome axis in dendritic cells by clotrimazole potentiates antitumor immunity. J. Immunother. Cancer 9, e002155. doi:10.1136/jitc-2020-002155
Warren, T. L., and Weiner, G. J. (2000). Uses of granulocyte-macrophage colony-stimulating factor in vaccine development. Curr. Opin. Hematol. 7, 168–173. doi:10.1097/00062752-200005000-00007
Watanabe, T., Ishino, T., Ueda, Y., Nagasaki, J., Sadahira, T., Dansako, H., et al. (2023). Activated CTLA-4-independent immunosuppression of Treg cells disturbs CTLA-4 blockade-mediated antitumor immunity. Cancer Sci. 114, 1859–1870. doi:10.1111/cas.15756
Weber, R., Groth, C., Lasser, S., Arkhypov, I., Petrova, V., Altevogt, P., et al. (2021). IL-6 as a major regulator of MDSC activity and possible target for cancer immunotherapy. Cell. Immunol. 359, 104254. doi:10.1016/j.cellimm.2020.104254
Xia, M., Tong, J. H., Ji, N. N., Duan, M. L., Tan, Y. H., and Xu, J. G. (2016a). Tramadol regulates proliferation, migration and invasion via PTEN/PI3K/AKT signaling in lung adenocarcinoma cells. Eur. Rev. Med. Pharmacol. Sci. 20, 2573–2580.
Xia, M., Tong, J. H., Zhou, Z. Q., Duan, M. L., Xu, J. G., Zeng, H. J., et al. (2016b). Tramadol inhibits proliferation, migration and invasion via α2-adrenoceptor signaling in breast cancer cells. Eur. Rev. Med. Pharmacol. Sci. 20, 157–165.
Xiao, M., Tang, Q., Zeng, S., Yang, Q., Yang, X., Tong, X., et al. (2023). Emerging biomaterials for tumor immunotherapy. Biomater. Res. 27, 47. doi:10.1186/s40824-023-00369-8
Xie, Z., Shen, J., Sun, H., Li, J., and Wang, X. (2021). Polymer-based hydrogels with local drug release for cancer immunotherapy. Biomed. Pharmacother. 137, 111333. doi:10.1016/j.biopha.2021.111333
Xue, X., Hu, Y., Wang, S., Chen, X., Jiang, Y., and Su, J. (2022). Fabrication of physical and chemical crosslinked hydrogels for bone tissue engineering. Bioact. Mater. 12, 327–339. doi:10.1016/j.bioactmat.2021.10.029
Yang, A., Bai, Y., Dong, X., Ma, T., Zhu, D., Mei, L., et al. (2021). Hydrogel/nanoadjuvant-mediated combined cell vaccines for cancer immunotherapy. Acta Biomater. 133, 257–267. doi:10.1016/j.actbio.2021.08.014
Yaseen, M. M., Abuharfeil, N. M., Darmani, H., and Daoud, A. (2020). Mechanisms of immune suppression by myeloid-derived suppressor cells: the role of interleukin-10 as a key immunoregulatory cytokine. Open Biol. 10, 200111. doi:10.1098/rsob.200111
Yi, M., Niu, M., Wu, Y., Ge, H., Jiao, D., Zhu, S., et al. (2022). Combination of oral STING agonist MSA-2 and anti-TGF-β/PD-L1 bispecific antibody YM101: a novel immune cocktail therapy for non-inflamed tumors. J. Hematol. Oncol. 15, 142. doi:10.1186/s13045-022-01363-8
Yue, K., Trujillo-de Santiago, G., Alvarez, M. M., Tamayol, A., Annabi, N., and Khademhosseini, A. (2015). Synthesis, properties, and biomedical applications of gelatin methacryloyl (GelMA) hydrogels. Biomaterials 73, 254–271. doi:10.1016/j.biomaterials.2015.08.045
Yue, S., He, H., Li, B., and Hou, T. (2020). Hydrogel as a biomaterial for bone tissue engineering: a review. Nanomater. (Basel) 10, 1511. doi:10.3390/nano10081511
Zhang, D., Li, Q., Chen, X., Nie, X., Xue, F., Xu, W., et al. (2022). An injectable hydrogel to modulate T cells for cancer immunotherapy. Small 18, 2202663. doi:10.1002/smll.202202663
Zhang, M., and Zhao, X. (2020). Alginate hydrogel dressings for advanced wound management. Int. J. Biol. Macromol. 162, 1414–1428. doi:10.1016/j.ijbiomac.2020.07.311
Zhao, M., Zhu, S., Zhang, D., Zhou, C., Yang, Z., Wang, C., et al. (2023). Long-lasting postoperative analgesia with local anesthetic-loaded hydrogels prevent tumor recurrence via enhancing CD8+ T cell infiltration. J. Nanobiotechnol. 21, 50. doi:10.1186/s12951-023-01803-8
Zhao, Y., Ran, B., Xie, X., Gu, W., Ye, X., and Liao, J. (2022). Developments on the smart hydrogel-based drug delivery system for oral tumor therapy. Gels 8, 741. doi:10.3390/gels8110741
Zhu, J., Naulaerts, S., Boudhan, L., Martin, M., Gatto, L., and Van den Eynde, B. J. (2023). Tumour immune rejection triggered by activation of α2-adrenergic receptors. Nature 618, 607–615. doi:10.1038/s41586-023-06110-8
Zou, M., Sun, J., and Xiang, Z. (2021). Induction of M2-type macrophage differentiation for bone defect repair via an interpenetration network hydrogel with a GO-based controlled release system. Adv. Healthc. Mater. 10, 2001502. doi:10.1002/adhm.202001502
Zúñiga, L. A., Leßmann, T., Uppal, K., Bisek, N., Hong, E., Rasmussen, C. E., et al. (2022). Intratumoral delivery of TransCon(™) TLR7/8 Agonist promotes sustained anti-tumor activity and local immune cell activation while minimizing systemic cytokine induction. Cancer Cell Int. 22, 286. doi:10.1186/s12935-022-02708-6
Keywords: hydrogel, immunomodulator, tumor immune microenvironment, innate immunity, adaptive immunity
Citation: Liu Y, Liu F, Zeng Y, Lin L, Yu H, Zhang S and Yang W (2024) Hydrogel systems for spatiotemporal controlled delivery of immunomodulators: engineering the tumor immune microenvironment for enhanced cancer immunotherapy. Front. Cell Dev. Biol. 12:1514595. doi: 10.3389/fcell.2024.1514595
Received: 21 October 2024; Accepted: 28 November 2024;
Published: 13 December 2024.
Edited by:
Christopher Synatschke, Max Planck Institute for Polymer Research, GermanyReviewed by:
Junhua Mai, Houston Methodist Research Institute, United StatesShubao Liu, Hong Kong Institute of Innovation and Technology, Hong Kong SAR, China
Copyright © 2024 Liu, Liu, Zeng, Lin, Yu, Zhang and Yang. This is an open-access article distributed under the terms of the Creative Commons Attribution License (CC BY). The use, distribution or reproduction in other forums is permitted, provided the original author(s) and the copyright owner(s) are credited and that the original publication in this journal is cited, in accordance with accepted academic practice. No use, distribution or reproduction is permitted which does not comply with these terms.
*Correspondence: Wenyong Yang, eXdlbnlvbmdAaG90bWFpbC5jb20=; Sunfu Zhang, c3VuZnV6aEAxNjMuY29t; Hui Yu, aHVpeXU3MjRAMTYzLmNvbQ==
†Lead Contact
‡These authors have contributed equally to this work