- 1Guangdong Provincial Key Laboratory of Research and Development of Natural Drugs, School of Pharmacy, Guangdong Medical University, Dongguan, China
- 2Dongguan Key Laboratory of Traditional Chinese Medicines for Prevention and Treatment of Digestive Diseases, Guangdong Medical University, Dongguan, China
- 3Dongguan Key Laboratory of Chronic Inflammatory Diseases, The First Dongguan Affiliated Hospital, Guangdong Medical University, Dongguan, China
- 4Cancer Center, The First Huizhou Affiliated Hospital, Guangdong Medical University, Huizhou, China
- 5Department of Acupuncture, The First Dongguan Affiliated Hospital, Guangdong Medical University, Dongguan, China
Chronic atrophic gastritis (CAG) is a prevalent digestive system disease characterized by atrophy of the gastric mucosa and the disappearance of inherent gastric glands. According to the theory of Correa’s cascade, CAG is an important pathological stage in the transformation from normal condition to gastric carcinoma. In recent years, the global incidence of CAG has been increasing due to pathogenic factors, including Helicobacter pylori infection, bile reflux, and the consumption of processed meats. In this review, we comprehensively described the etiology and clinical diagnosis of CAG. We focused on elucidating the regulatory mechanisms and promising therapeutic targets in CAG, with the expectation of providing insights and theoretical support for future research on CAG.
1 Introduction
Chronic atrophic gastritis (CAG) is one of the most prevalent gastrointestinal diseases, characterized by gastric mucosa atrophy, reduction of glandular structures, and mucosal nodules. CAG is one of the precancerous lesions of gastric cancer. Patients diagnosed with CAG usually suffer from nausea, loss of appetite, indigestion, vomiting, upper abdominal pain, and loss of weight (De Vries et al., 2008; Miceli et al., 2012).
Gastric cancer (GC) continues to rank as the fifth most common cancer and the fourth highest cause of cancer-related mortality globally. It is estimated that the global burden of GC will increase by 62% by 2040 (Thrift et al., 2023). The multifactorial progression of GC development, known as Correa’s cascade, comprises multiple sequential events (Piazuelo et al., 2021). Chronic inflammation constitutes the initial step in this cascade, leading to persistent irritation of the gastric glands and parietal cell atrophy (Kuligowski et al., 2016). With the aggravation of gastric mucosal inflammation, the second process is gastric precancerous lesions including chronic non-atrophic gastritis, intestinal metaplasia, and dysplasia (Si et al., 2024). The third phase of Correa’s cascade is gastric tumorigenesis. Therefore, timely and effective treatment of CAG is of great significance for the prevention of GC.
In this review, we will broadly delineate the etiology and contemporary diagnostic approaches of CAG. The research focus on targets and signaling pathways regulating CAG, which is beneficial for enhance our understanding of its pathogenesis and develop novel therapeutic interventions for CAG patients.
2 The etiology of CAG
The etiology of CAG is complicated and is associated with a variety of factors, which may act synergistically. The main causes for the development of CAG including Helicobacter pylori infection, bile reflux, N-nitroso compounds exposures, high salt intake, alcohol consumption, chronic persistent stress and immunologic factor.
2.1 Helicobacter pylori infection
CAG has several known inducers with the most common being Helicobacter pylori (Hp) infection. A recent cluster-randomized controlled trial shows that mass screening for Hp and its eradication can reduce the incidence of GC, thereby providing further evidence for the role of Hp in the pathogenesis of CAG and GC (Pan et al., 2024).
Hp-induced CAG is mainly mediated by the virulence factors VacA and CagA (Keates et al., 1997). The pathological roles of CagA involve regulating multiple signaling pathways in the host cells. Type IV secretory system (T4SS) is used to inject CagA into gastric epithelial cells, leading to the activation of downstream signaling pathways. Following injection, CagA undergoes tyrosine phosphorylation, contributing to DNA damage and the trigger of the Wnt/β-catenin pathway (Bauer et al., 2020). CagA has been observed to interact with E-cadherin, resulting in the translocation of β-catenin to the nucleus and the activation of Wnt target genes (Kurashima et al., 2008; Murata-Kamiya et al., 2007). Studies have observed that CagA enhances the degradation of the p53 tumor suppressor and reverses the p53-regulated apoptotic response (Buti et al., 2011). Moreover, the inactivation of the p53 tumor suppressor induces chromosomal or genomic instability and DNA double-strand breaks (Hanahan and Weinberg, 2011; Zamperone et al., 2019). VacA is the second most frequently studied Hp virulence factor. VacA can induce multiple pathological activities, including the inducement of vacuolation, release of cytochrome C from mitochondria, and trigger of inflammatory response (Camilo et al., 2017). In addition, VacA can also inhibit the activation and proliferation of immune cells (Cover and Blanke, 2005; Foegeding et al., 2016). Therefore, Hp infection plays a vital role in accelerating the pathology of CAG.
2.2 Bile reflux
Recently, accumulating evidence has demonstrated that bile reflux is also a key pathogenic factor in CAG and has been identified as an independent risk factor for GC according to an observational cross-sectional study (Zhang et al., 2021). Furthermore, another clinical study has provided histological evidence suggesting a close correlation between bile reflux and intestinal metaplasia (Dixon et al., 2002).
Bile reflux is characterized by the backflow of duodenal contents, such as bile and duodenal juice, into the gut. In physiological conditions, the majority of bile acid is secreted into the intestine lumen and circulates back to the liver. The gut microbiome facilitates the transformation of primary bile acids to secondary bile acids by dehydroxylation. Bile reflux into the stomach causes gastric mucosal dysfunction by inducing cell apoptosis, enhancing cell permeability with intercellular junctions damaged, which contributes to the aggravation of gastric inflammation (Goldman et al., 2010; Shi et al., 2016).
Farnesoid X receptor (FXR) is a key bile-acid binding receptor that plays an essential role in bile acid homeostasis. Research has demonstrated that FXR expression is significantly increased in intestinal metaplasia tissues (Qu and Shi, 2022). Numerous studies have shown that the NF-κB signaling pathway is associated with the regulation of FXR expression (Zhou et al., 2018). Recent studies further elucidated that bile reflux can alter the abundance and composition of gastric microbiota because of alkaline bile. Based on the 16S rRNA analysis, the pathogenic bacteria Comamonas, Halomonas, and Shewanella are found to be enriched in patients with bile reflux, which may contribute to the aggravation of CAG (Huang et al., 2022).
2.3 N-nitroso compounds exposure
Evidence has demonstrated that exposure to N-nitroso compounds (NOCs) is an essential factor contributing to CAG and the progression of GC. The common NOCs, including nitrosamines and nitrosamides, exhibit mutagenic, genotoxic, and carcinogenic effects in both experimental animals and humans (Kobayashi, 2018). People can be exposed to NOCs via the consumption of processed meats and cigarette smoking, both of which contribute to the endogenous formation of NOCs (Kuhnle et al., 2007; Tricker et al., 1991). It is widely recognized that NOCs in common dietary sources can be formed by bacterial nitrite reductases and increased pH in the stomach (Kobayashi, 2018).
It is widely proved that the underlying mechanisms by which exposure to NOCs promotes CAG and GC involve multiple targets and signaling pathways, including gene mutation and pro-inflammatory signaling pathways. Recent studies have shown that 1-methyl-3-nitro-1-nitrosoguanidine (MNNG) and N-methyl-N-nitrosourea (MNU) can facilitate N6-methyladenosine (m6A) modifications, thereby promoting the progression of GC (Song et al., 2024; Wang Q. et al., 2024). Additionally, a study on human epithelial cells has revealed that MNNG regulates the downstream pro-angiogenic factors of NF-κB by enhancing the phosphorylation of NF-κB p65, which may contribute to the increased incidence of CAG and GC (Chen Y. et al., 2018).
2.4 High salt intake
According to a retrospective review, a high-salt diet is closely associated with Hp infection and the progression of CAG and GC (Song et al., 2017). Significant advancements have been made in elucidating the mechanisms underlying the induction of CAG by high salt intake. An in vivo study shows that a high-salt diet induces malignant cell proliferation and lipid peroxidation (Vences-Mejía et al., 2005). Another study reveals that high salt intake increases the expression levels of cyclooxygenase-2 (COX-2) and inducible nitric oxide synthase (iNOS). Therefore, research has confirmed that the COX-2 inhibitor, celecoxib, can prevent the development of GC in the rodent model (Hu et al., 2004). In addition, evidence suggests a notable increase in inflammatory cytokines, including TNF-α, IL-1β, IL-6, and IFN-γ, in mice subjected to a high-salt diet (Leung et al., 2008).
2.5 Other etiological factors
Alcohol consumption is an essential independent risk factor for CAG and gastric ulcers. A clinical study has confirmed that the severity of gastric atrophy is closely correlated with alcohol consumption and frequency of drinking (Ozeki et al., 2024). Accumulating evidence indicates that alcohol can induce gastric injury through multiple mechanisms, mainly including the activation of oxidative stress and local inflammatory response (Liu F. et al., 2022). Other researchers have unveiled that excessive ethanol intake can damage gastric mucosal microcirculation and disrupt cell proliferation, resulting in activation of the NF-κB signaling cascade (Yu L. et al., 2020).
Chronic persistent stress is also an important inducer of CAG. Researchers usually use a mouse model subjected to water avoidance stress to stimulate the long-term psychological stress that contributes to the development of CAG (Bradesi et al., 2005). It is widely accepted that stress events can induce gastric acid secretion. Further studies have demonstrated that stress-induced parasympathetic overactivity and an increase in vagal outflow may contribute to excessive gastric acid secretion (Guo et al., 2012). A recent study has shown that the maintenance of gastric microbiota homeostasis is also an essential factor in stress-induced CAG (Han et al., 2020).
The immunologic factor is also a causative factor of CAG. Autoimmune atrophic gastritis is an autoimmune disease, characterized by the gastric corpus-fundus mucosa damaged (Massironi et al., 2019). The presence of anti-parietal cell antibodies can contribute to autoimmune atrophic gastritis (Goldenring, 2023). It is well-established that these anti-parietal cell antibodies demonstrate complement-dependent cytotoxic activity in vitro. However, the pathogenesis of autoimmune atrophic gastritis is still controversial. Accumulating evidence suggests that pro-inflammatory cytokines, including TGF-β, TNF-α, and IL-6, are involved in the development of autoimmune atrophic gastritis (Cascetta et al., 2024). Similarly, Hp infection has been found to promote the progress of autoimmune atrophic gastritis. The interaction between Hp antigens and gastric H+/K+ ATPase is considered a potential pathogenic mechanism underlying this process (Massironi et al., 2019).
3 Clinical diagnosis of CAG
Over the past decades, there are several diagnostic methods for CAG. In this section, we provide a summary of the main clinical diagnosis methods, including endoscopy, pathological diagnosis and gastric functioning markers. We also discuss some potential biomarkers on CAG diagnosis, which may provide novel solutions to early clinical diagnosis.
3.1 Endoscopy and pathological diagnosis
Given that the symptoms exhibited by patients are typically subtle, early diagnosis is crucial. The CAG diagnosis mainly relies on gastroscopy and pathological analysis of biopsies. Histopathological assessment of gastric biopsies of antrum and corpus mucosa is the gold standard for the diagnosis of CAG. The typical endoscopic features of CAG include the loss of gastric folds, a pallid appearance of the gastric mucosa, and the thinning of gastric mucosa (Shah et al., 2021). The OLGA/OLGIM classifications are used for the diagnosis and classification of CAG. However, gastroscopy cannot be performed frequently in a short time. Furthermore, gastroscopy causes damage to the esophagus, leading to patient discomfort and psychological pressure (Gai et al., 2023).
Recently, deep learning and artificial intelligence have been found to assist in the diagnosis of CAG. Studies have shown that deep learning models are superior to endoscopists and exhibit higher levels of accuracy, suggesting that they have better clinical diagnostic value (Turtoi et al., 2024; Zhao et al., 2022).
3.2 Gastric functioning markers
Serum biomarkers are beneficial for the diagnosis of CAG, including gastrin, pepsinogen (PG I, PG II), and gastric proton pump H+/K+ ATPase (Jia et al., 2024). Gastrin expression is upregulated in many gastric carcinomas of the stomach corpus (Smith et al., 2017), including endocrine tumors (Massironi et al., 2009). A large-scale retrospective cohort study shows that gastrin measurement is essential for the risk evaluation of GC (Nagasaki et al., 2023). Some study also indicate that gastrin level is an important marker for predicting the polyp risk in autoimmune gastritis patients (Massironi et al., 2024; Massironi et al., 2023). The low PG I level or low ratio between PG I and PG II usually indicate the presence of CAG. In addition, the pepsinogen level can help distinguish CAG and autoimmune atrophic gastritis (Chapelle et al., 2023). According to a prospective case-finding study, gastric proton pump H+/K+ ATPase is an effective biomarker for the identification of CAG in high-risk patients (Lahner et al., 2020).
3.3 Other potential biomarkers
In recent years, numerous novel noninvasive diagnostic methods have been discovered. Based on the 1H NMR-based metabonomics results, arginine, succinate, 3-hydroxybutyrate, valine, and α-ketoglutarate have potential as indicators of CAG risk (Cui et al., 2017). These metabolites have specificity and sensitivity, which have potential to act as early diagnosis biomarkers for CAG. In China, traditional Chinese medicine doctors usually observe the changes in patients’ tongues to diagnose diseases, because it is well-known that the changes in tongues are influenced by the physiological function of the stomach. In addition, in a study comparing gastritis patients with healthy individuals, researchers have found that Campylobacter concisus is detected in tongue coating, indicating tongue coating microbiota is a promising non-invasive biomarker for CAG (Cui et al., 2019). This study provides a non-invasive and individualized complement for CAG diagnosis. Numerous researches also show that gastric microbiome and serum proteins could be biomarkers for distinguishing CAG cases (Aziz et al., 2022; Dong et al., 2023) (Figure 1). Chromogranin A (CgA) is also identified as an effective biomarker to serve as indicating the histological degree of enterochromaffin-like (ECL) cell lesions, which reflect the severity of CAG. However, CgA is of limited clinical diagnosis utility because of a lack of sensitivity and specificity (Al-Toubah et al., 2019).
4 Programmed cell death in CAG
Programmed cell death is characterized by the orderly death of cells, regulated by genes for the stability of the internal environment, including apoptosis, autophagy, ferroptosis, pyroptosis, and necroptosis. It is well established that programmed cell death plays various roles in the pathogenesis of CAG (Figure 2).
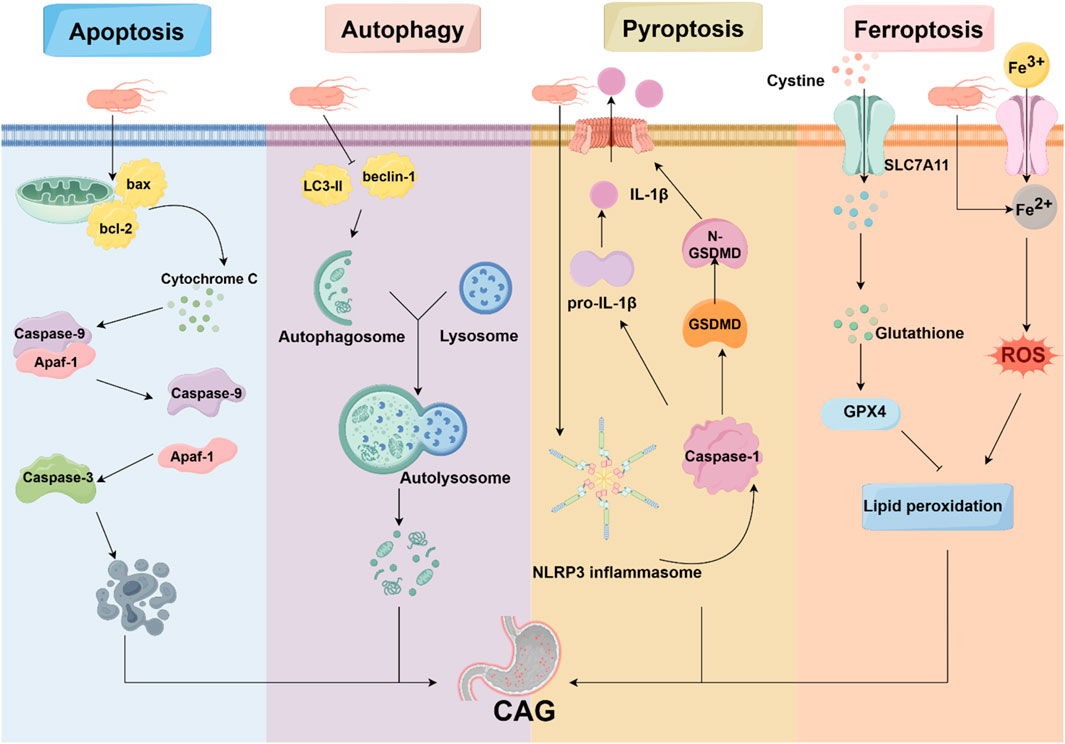
Figure 2. Programmed cell death in CAG. Programmed cell death is involved in the pathogenesis of CAG, including apoptosis, autophagy, pyroptosis, and ferroptosis. HP, acting as an avital pathogenic factor, can regulate programmed cell death and affect the development of CAG.
4.1 Apoptosis
Apoptosis is widely regarded as a caspase-mediated form of programmed cell death that plays a major role in the control of cell proliferation and tissue homeostasis (Chen Q. et al., 2018). The morphological characteristics of apoptosis include chromatin condensation, cell shrinkage, and nuclear fragmentation (Lockshin and Zakeri, 2001). The mitochondria-dependent apoptosis is activated by diverse signals such as oxidative stress and DNA damage, leading to the activation of pro-apoptotic factors. The death receptor-mediated apoptosis is triggered by the binding of Fas ligand, TNF-α, or TRAIL (Wang and El-Deiry, 2003).
It is generally recognized that the pathogenesis of CAG is strongly associated with apoptosis. Immunohistochemistry results of the clinical gastric resection specimens have shown that CAG and intestinal metaplasia are related to an increase in apoptotic gastric epithelial cells (Van Grieken et al., 2003). Increasing evidence suggests that Hp induces gastric epithelial cell apoptosis (Moss et al., 1996; Yamaguchi et al., 2000). According to electron microscopic observations, the virulence factor of Hp, VacA, induces apoptosis in GES-1 cells (Yuan et al., 2021). In vitro studies have provided the molecular mechanisms by which HP promotes apoptosis. AGS cells depleted of tumor necrosis factor receptor-associated protein 1 (TRAP1) increases apoptosis (Teng et al., 2019). However, the effect of Hp on apoptosis is not unidirectional, suggesting that the imbalance between proliferation and apoptosis contributes to Hp-induced CAG and GC. A study shows that Hp activates anti-apoptotic pathways to inhibit gastric epithelial self-renewal, leading to enhanced Hp colonization (Mimuro et al., 2007). Taken together, apoptosis is strongly related to CAG, and the medicines with anti-apoptotic activity can be used for future anti-CAG research.
4.2 Autophagy
Autophagy is a process involving the formation of autophagosomes to clear and degrade pathogens, damaged organelles, and unfolded proteins (Shu et al., 2023). The process of autophagy involves four predominant steps: autophagy initiation, vesicle nucleation, autophagosome formation, and hydrolyzation (Mizushima and Levine, 2020). Recent research has shown that several key autophagy-related genes, including ATG7, ATG16L1, and ATG12, are implicated in the progression of CAG and potentially serve as biomarkers, suggesting that autophagy is strongly associated with the pathogenesis of CAG (Yamaguchi et al., 2024; 2023).
However, autophagy plays a dual role in the development of CAG. On the one hand, the inhibition of autophagy exacerbates the apoptosis of GES-1 cells and gastric mucosa damage caused by aspirin (Hernández et al., 2016). Further studies show that the activation of autophagy by regulating mTOR can enhance apoptosis and oxidative stress in GES-1 cells (Chang et al., 2017). During the Hp infection, the inhibition of autophagy leads to DNA damage in Hp-infected cells and the development of gastric tumorigenesis via Rad51 ubiquitination (Xie et al., 2020). Several antioxidant agents can upregulate autophagy and downregulate apoptosis to prevent Hp-infected gastric injury (Yang and Chien, 2009).
On the other hand, uncontrolled autophagy leads to aberrant cell proliferation, which plays a key role in the development of CAG (Lu et al., 2021). The autophagy-related gene ATG16L1 has been revealed to increase the risk of Hp-induced inflammation. In addition, VacA is found to induce autophagy and cell death in gastric epithelial cells by regulating the endoplasmic reticulum stress pathway (Zhu et al., 2017). Therefore, researchers have found that clearance of autophagosomes offers a strategy to decrease Hp colonization (Zhang et al., 2018).
4.3 Pyroptosis
Pyroptosis is a new form of programmed cell death characterized by the activation of caspase family proteins. This activation leads to the cleavage of gasdermin D (GSDMD), ultimately leading to cell membrane disruption (Newton et al., 2024; Yu P. et al., 2021). In the classical pathway, inflammasomes are activated by pathogenic exposures, resulting in the recruitment and activation of caspase-1. Subsequently, GSDMD is cleaved, causing plasma membrane disruption and causing cytoplasmic efflux. Simultaneously, cleaved caspase-1 also activates inflammatory factors, including IL-1β and IL-18. The non-classical pathway is activated by lipopolysaccharide (LPS). Caspase-11, caspase-4, or caspase-5 can bind to LPS and hydrolyze GSDMD, triggering membrane perforation (Zhou et al., 2023).
A recent study has shown that pyroptosis is involved in the pathogenesis of gastritis and the progression of GC. Researchers constructed a cellular CAG model using GES-1 cells, discovering alterations in their morphology and plasma membrane (Zhou Z. et al., 2024). In addition, pyroptosis is also associated with Hp infection. The virulence factors of Hp, including CagA, VacA, FlaA, and UreB, regulate the inflammasome machinery and the release of pro-inflammatory cytokines (Kumar and Dhiman, 2008).
The NOD-like receptor protein 3 inflammasome (NLRP3) inflammasome is an important executor of pyroptosis. NLRP3 can combine with ASC and NEK7 to form the NLRP3 inflammasome, which activates caspase-1 that cleaves GSDMD into N-GSDMD (Sun et al., 2024). It has been recognized that the CagA protein of Hp activates the NLRP3 inflammasome, and silencing of NLRP3 can reverse the effect of Hp on cell migration (Zhang X. et al., 2022). Moreover, Hp has also been discovered to induce IL-18 and IL-1β production through activation of NLRP3 inflammasome (Li et al., 2015).
4.4 Ferroptosis
Ferroptosis is a distinct iron-dependent cell death characterized by the accumulation of lipid peroxides and redox imbalance (Zhou Q. et al., 2024). The morphological features of ferroptosis include mitochondria with condensed membranes and decreased cristae (Cao and Dixon, 2016; Dixon et al., 2012). Mechanistically, intracellular iron accumulation contributes to lipid peroxidation by the Fenton reaction and the production of reactive oxygen species (ROS) (Yan et al., 2021). Additionally, glutathione (GSH)-glutathione peroxidase 4 (GPX4) antioxidant system, as the key repressor of ferroptosis, plays a vital role in protecting cells from ferroptosis. It is observed that GSH serves as the electron donor for reducing toxic phospholipid hydroperoxides to non-toxic phospholipid alcohols (Seibt et al., 2019).
According to the multi-omics integration and molecular docking results, researchers confirm that the ferroptosis-related gene YWHAE is involved in the progression of CAG and GC (Liu D. et al., 2023). In addition, a recent study reveals that Hp infection increases the sensitivity of cells to RAS-selective-lethal 3 (RSL3)-induced ferroptosis by regulating phosphorylase kinase G2 (PHKG2) (Zhu et al., 2023). Hepcidin is an iron-regulatory hormone, that can regulate the cellular iron exporter (Nemeth and Ganz, 2023). Studies have revealed that hepcidin expression is elevated in CAG gastric tissue via the IL-6/STAT3 signaling pathway (Zhao et al., 2023). Furthermore, gastric hepcidin is highly expressed during Hp infection but normalized after eradication, suggesting that hepcidin may become a future target for CAG treatment by inhibiting ferroptosis (Schwarz et al., 2012).
5 Molecular pathways and key targets in the pathogenesis of CAG
Numbers of signaling pathways and the key targets were reported to be related with CAG development, including the NF-κB, Hedgehog pathway, TLRs pathway, PI3K/Akt pathway, MAPK pathway, Wnt/β-catenin pathway, p53 pathway, and Hippo pathway (Figure 3).
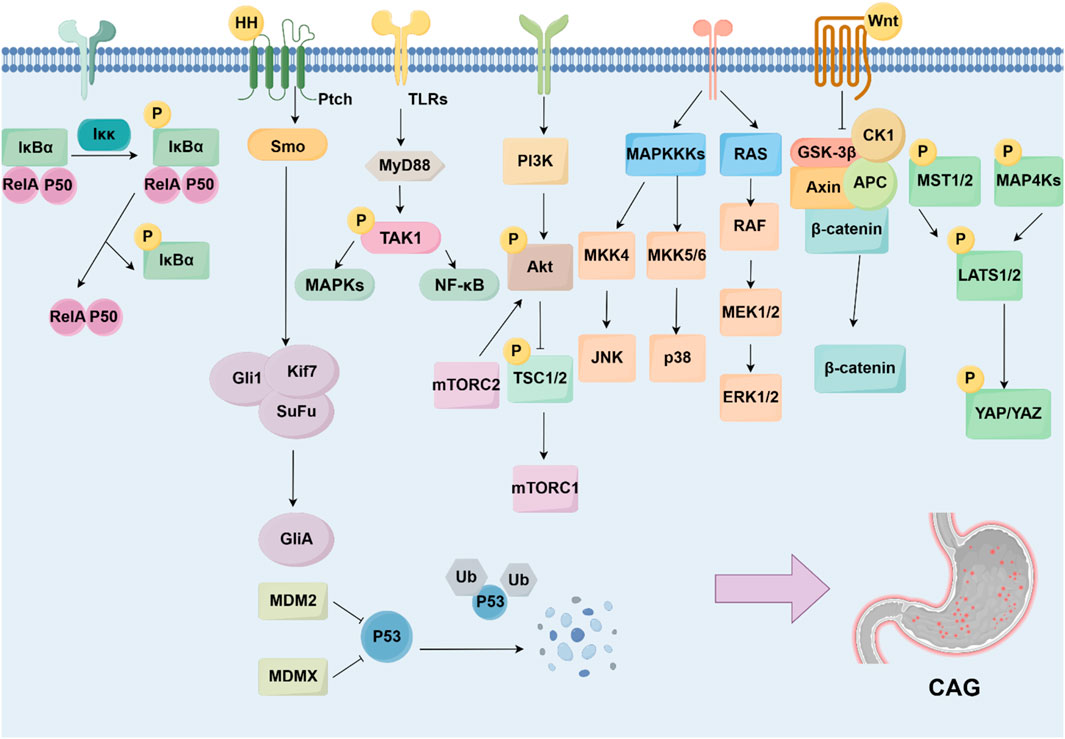
Figure 3. Signaling pathways and targets regulating CAG development. The development of CAG is achieved by numerous signaling pathways and targets, including NF-κB pathway, Hedgehog pathway, TLRs pathway, PI3K/Akt pathway, MAPK pathway, Wnt/β-catenin pathway, p53 pathway, and Hippo pathway.
5.1 NF-κB signaling pathway
NF-κB family includes five members, p65 (RelA), RelB, c-Rel, p105/p50, and p100/p52. The activation of NF-κB starts with the phosphorylation of IκB, induced by IKKs, leading to the release of NF-κB. The released NF-κB translocates to the nucleus and drives the transcription of targeted genes (Yu H. et al., 2020). It is widely recognized that NF-κB signaling pathway dysfunction has been associated with various human diseases, including inflammation, malignancies, and autoimmune disorders (Guo et al., 2024). Existing studies show that targeting the NF-κB signaling pathway can alleviate gastric damage and suppress inflammation, which is essential for the pathogenesis of CAG (Kim et al., 2021). For instance, the NF-κB inhibitor pyrrolidine dithiocarbamate (PDTC) has been found to inhibit excessive cell proliferation and reverse CAG in mice (Jiang et al., 2021).
Studies have demonstrated that activated NF-κB upregulates chemokines or adhesion molecules, thereby promoting inflammation in Hp-associated gastritis (Isomoto et al., 2000; Maubach et al., 2022). Similarly, conditional deletion of IκB promotes Hp-induced cell apoptosis and the development of dysplasia (Shibata et al., 2010). Meanwhile, Hp also induces the NF-κB signaling pathway by its effector ADP-heptose, leading to the establishment of a persistent effect. A diverse range of molecules regulate the activity of NF-κB in Hp-infected gastritis. For instance, the p53 upregulation modulator of apoptosis (PUMA), as a pro-apoptotic protein, has been discovered that NF-κB binds to PUMA’s promoter to contribute to the pathogenesis of Hp-infected gastritis (Dang et al., 2020).
5.2 Hedgehog signaling pathway
The Hedgehog signaling pathway is a pathway regulating embryonic development and tissue homeostasis. It includes three ligands, including Sonic hedgehog (Shh), Indian hedgehog (Ihh), and Desert hedgehog (Dhh), as well as three Gli proteins, Gli1, Gli2, and Gli3 (Jiang, 2022). It has become clear that gastric epithelial cells can secrete Shh, which contributes to the renewal of epithelial cells in response to gastric injury (Konstantinou et al., 2016). Therefore, accumulating evidence shows that the loss of the Hedgehog signaling pathway is an important indicator of Hp-associated CAG progressing to GC (Shiotani et al., 2005). The malfunction of the Hedgehog signaling pathway influences the progression of CAG, including the decrease in gastric acid secretion and the loss of parietal cells (Wessler et al., 2017).
Hp is reported to directly control the expression level of the Shh signal. A study shows that Hp-induced IL-1β inhibits the expression level of Shh, contributing to gastric atrophy (Waghray et al., 2010). However, in certain conditions, Hp-induced caudal-type homeobox 2 (Cdx2) can bind to the promoter of the Shh gene, leading to the downregulation of Shh (Shiotani et al., 2006). It has also been revealed that the Hedgehog signaling pathway acts as a macrophage chemoattractant and affects the immune response (Schumacher et al., 2012).
5.3 Toll-like receptors signaling pathway
Toll-like receptors (TLRs) can identify pathogen-associated molecular patterns (PAMPs), which mediate the immune response and activate intracellular signaling pathways, especially the MyD88-mediated pathway (Barton and Medzhitov, 2003). It is widely recognized that the TLR signaling pathway plays an essential role in immune response regulation and several inflammatory diseases.
Many studies on CAG have reported that the TLR signaling pathway is closely correlated with the progression of gastritis (Table 1). TLR4 gene polymorphisms have been found to mediate the inflammatory response in gastritis (Zhang Z. et al., 2024). Moreover, studies have shown that TLR9 promotes gastric tumorigenesis and facilitates Hp-infected gastritis (Tang et al., 2022). The most widely studied is the myeloid differentiation factor-88 (MyD88)-mediated signaling pathway. TLRs regulate inflammation through MyD88. It has been reported that TLRs interact with Hp by binding to MyD88, leading to the activation of the NF-κB signaling pathway and the release of inflammatory factors. Numerous studies show that the TLRs/MyD88 signaling pathway can increase Hp chemotaxis and promote cell migration through a diverse range of inflammatory and oncogenic pathways (Liu M. et al., 2023).
5.4 PI3K/Akt signaling pathway
The PI3K/Akt signaling pathway is a major signaling pathway that controls cell survival and metabolism (He et al., 2021). The PI3K/Akt signaling pathway belongs to the family of serine/threonine protein kinases and starts with the activation of receptor tyrosine kinases (RTKs) by growth factors. It is involved in the modulation of numerous downstream targets, which include NF-κB, mTOR, and MDM2 activation (Lei et al., 2022). Research shows that the PI3K/Akt signaling pathway affects neutrophils and lymphocytes, resulting in the promotion of inflammation.
Based on immunohistochemistry analysis of biopsy tissue, researchers discovered that the levels of p-PI3K and p-Akt in Hp-positive patients are higher than those in Hp-negative patients (Xie and Liu, 2018). A study demonstrates that Hp infection regulates eukaryotic protein translation by activating the PI3K/Akt signaling pathway, which can be reversed by the PI3K inhibitor, LY294002 (Sokolova et al., 2014). Hp also regulates downstream targets to affect the progression of CAG. Researchers have found that Hp regulates FoxO1/3a in gastric epithelial cells, which regulates the host immune response and cell apoptosis (Tabassam et al., 2012).
5.5 MAPK signaling pathway
The mitogen-activated protein kinases (MAPK) are a group of serine/threonine kinases and play a pivotal role in cell proliferation and differentiation (Bahar et al., 2023). The MAPK family consists of three major kinases: c-JUN N-terminal kinases (JNKs), extracellular signal-regulated kinases (ERKs), and p38 (Johnson and Lapadat, 2002). There is increasing evidence that the MAPK signaling pathway is an essential regulator of inflammatory diseases, including pancreatitis, acute colitis, and gastritis (Hong et al., 2024; Junyuan et al., 2018; Zhang J. et al., 2022). In addition, the activation of the MAPK signaling pathway is associated with the pathology of gastric mucosal injury (Arab et al., 2019; Fu et al., 2018). Immunostaining results reveal that Hp-induced changes in the gastric mucosa are associated with the activation of the MAPK signaling pathway (Kacar et al., 2007).
During Hp infection, the Hp structural compound CagL interacts with integrin α5β1 on gastric epithelial cells, leading to the activation of the MAPK signaling pathway (Gorrell et al., 2013). Moreover, it has been reported that oligomerization domain 1 (NOD1) is required for MAPK activation during Hp infection (Allison et al., 2009).
MAPK activation leads to numerous pathological processes. Hp induces overexpression of MMP-3 and MMP-9 via the MAPK signaling pathway, leading to the disturbance of host cellular signaling and cell adhesion (Karayiannis et al., 2023). Another study also shows that Hp increases the secretion of gastrin by MEK1, ERK2, and c-RAF in the MAPK signaling pathway (Gunawardhana et al., 2018).
5.6 Wnt/β-catenin signaling pathway
The Wnt/β-catenin signaling pathway is an essential pathway that plays roles in regulating cell proliferation, cell metabolism, cancer metastasis, and cancer immunity (Liu J. et al., 2022). The Wnt family includes Wnt3a, Wnt1, Wnt5a, and so on (Yu F. et al., 2021). When extracellular Wnt ligands bind to membrane receptors, the levels of β-catenin accumulate and are transferred to the nucleus, eventually becoming involved in the expression of downstream targeted genes, including c-myc and cyclin D1 (Reyes et al., 2020). It has been discovered that the Wnt/β-catenin signaling pathway functions as a molecular target for pathogenic bacteria, including Hp (Silva-García et al., 2019). In addition, it has been elucidated that the Wnt/β-catenin signaling pathway is involved in the progression of CAG, as it is recognized as a key regulator of EMT. Studies show that MNNG stimulation combined with Hp infection induces EMT by enhanced expression and activation of Wnt2 and β-catenin (Lin et al., 2019).
Aquaporin 5 (AQP5) is a member of the AQP family, which plays an essential role in modulating water transport (Tan et al., 2020). Hp infection has been reported to increase the expression level of AQP5 in gastric epithelial cells (Li et al., 2021). In addition, bioinformatics results suggest that achaete-scute complex-like 1 (ASCL1) binds to AQP5 (Wang et al., 2017). Therefore, researchers have proven that Hp infection activates the Wnt/β-catenin signaling pathway via upregulating ASCL1/AQP5 to induce CAG (Zuo et al., 2022).
5.7 p53 signaling pathway
TP53 is an important tumor suppressor gene that plays a vital role in regulating cell proliferation and apoptosis, and it has been considered a focus of cancer research (Kastenhuber and Lowe, 2017; Levine, 2020). Mutations in TP53 are strongly associated with the process of tumorigenesis. The p53 protein is promoted for degradation through ubiquitination by the negative regulators MDM2 and MDMX in the normal cellular environment (Bieging et al., 2014). When exposed to internal and external stresses, intracellular p53 protein is accumulated because of the cessation of p53 ubiquitination (Wang et al., 2023). Eventually, activated p53 binds to DNA and modulates gene transcription. In addition, numerous studies have shown that p53 also regulates other signaling pathways, including autophagy, ferroptosis, cellular senescence, and so on (Spike and Wahl, 2011; White, 2016).
The p53 signaling pathway is associated with Hp infection. It has been reported that Hp induces gastritis in p53-knockout mice compared to wild-type mice, suggesting that p53 plays an important role in Hp-associated CAG (Nagata et al., 2004). Similarly, the severity of CAG is closely related to p53 mutations (Taguchi et al., 2006). The underlying potential mechanisms have been discovered and reported. Hp has been shown to activate Akt1, resulting in the degradation of p53 (Wei et al., 2010). In addition, research shows that p53 and CXCL12 promote cellular senescence, leading to gastric mucosal atrophy (Cai et al., 2021).
5.8 Hippo signaling pathway
The Hippo signaling pathway plays a vital role in modulating cell proliferation, cell differentiation, and cell survival (Fu et al., 2022). The key components of the Hippo signaling pathway include mammalian STE20-like kinase1/2 (MST1/2), protein Salvador homolog 1 (SAV1), large tumor suppressor kinase 1/2 (LATS1/2), Yes-associated protein (YAP), and WW-domain-containing transcription regulator (TAZ) (Liu Y. et al., 2023). Dysregulation of the Hippo signaling pathway is associated with a variety of diseases.
Some findings underscore the fact that dysregulation of the Hippo signaling pathway is involved in the progression of Hp-induced CAG and GC. Researchers have shown that the expression of YAP in gastric tissue of Hp-positive patients is higher than that in Hp-negative patients (Li et al., 2018). It has been reported that Hp-related virulence factors, in combination with the Hippo signaling pathway, promote the processes of inflammation and carcinogenesis (Messina et al., 2023). Studies indicate that CagA promotes the delocalization of ZO-1 and ZO-2, thereby increasing the expression level of YAP. In turn, activation of YAP/TAZ also contributes to Hp infection and promotes the process of EMT (Tiffon et al., 2020). Additionally, Hp-induced YAP has been found to promote IL-1β expression, thereby mediating gastric carcinogenesis (Wu et al., 2019). However, a study has revealed that the Hippo signaling pathway protects gastric cells from Hp-induced EMT and metaplasia. The results show that LATS2 restricts Hp-induced EMT and metaplasia phenotype, thereby contributing to the control of the progression of CAG and GC (Molina-Castro et al., 2020). In conclusion, the Hippo signaling pathway exhibits dual effects in Hp-mediated CAG.
6 Novel mechanisms regulating CAG development
Numerous studies have shown the classic signaling pathways are associated with the development of CAG, but some novel mechanisms involved in the CAG has been discovered, which may provide a more comprehensive understanding of the pathogenesis of CAG. Recent studies have shown that angiogenesis, energy metabolism, gut microbiota and gastric microbiota, inflammatory microenvironment, oxidative stress, gastric stem cells defect, non-coding RNAs, exosomes are associated with CAG (Figure 4).
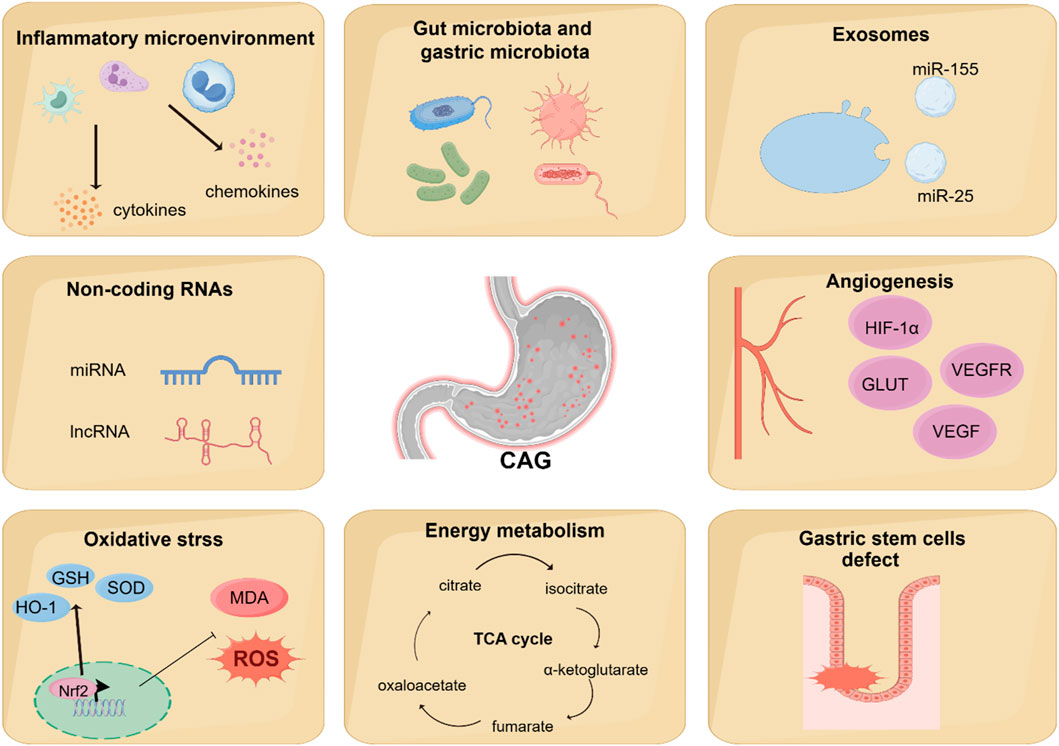
Figure 4. Novel mechanisms regulating CAG development. Recent studies have found that other novel mechanisms are associated with the regulation of CAG development, including angiogenesis, energy metabolism, gut microbiota and gastric microbiota, inflammatory microenvironment, oxidative stress, gastric stem cells defect, non-coding RNAs, and exosomes.
6.1 Angiogenesis
Angiogenesis is a physiological process involving the development of new blood vessels from existing ones. It is well-established that angiogenesis plays a vital role in maintaining homeostasis. However, because of the overexpression of pro-angiogenic factors and the inactivation of anti-angiogenic factors, angiogenesis dysregulation occurs in a variety of immune diseases. Furthermore, studies have shown that solid tumor cells, in response to a hypoxic microenvironment, secrete pro-angiogenic factors to promote the formation of new blood vessels. The vascular endothelial growth factor (VEGF) is the most important in the angiogenic system, as it mediates both vascular permeability and angiogenesis. Studies have shown that the imbalance of angiogenic factors and angiogenesis may play an important physiological role in the development of CAG (Kawano and Tsuji, 2000).
HIF-1α participates in regulating the relative expression of VEGF and facilitating the process of angiogenesis in hypoxic conditions (Kitajima and Miyazaki, 2013). Research has shown that the expression levels of HIF-1α, VEGF, VEGFR2, Pacilin, and SRC are upregulated in the CAG model group compared to control groups (Liu et al., 2024; Wen J.-X. et al., 2021).
Glucose transporters (GLUTs) are a kind of carrier of glucose for transporting and widely exist in cells (Ni et al., 2020). Numerous studies have shown that GLUTs are closely related to tumor angiogenesis (Airley and Mobasheri, 2007). Overexpression of GLUTs is frequently observed in tumors, with Glut1, 3, 4, 6, 10, and 12 being expressed in gastric carcinoma (Pujol-Gimenez et al., 2015; Schlößer et al., 2017). A previous study has shown that the expression levels of Glut4 and Glut1 significantly increase in precancerous lesions of gastric cancer (Zeng et al., 2022).
Recent research has demonstrated that the Notch signaling pathway also plays a pivotal role in angiogenesis. In mammals, there are three Notch receptors: Notch2, Notch3, and Notch4. In humans and mice, five Notch ligands have been identified, including the Serrate-like ligands Jagged1 (JAG1) and JAG2, as well as the delta-like ligands DLL1, DLL3, and DLL4 (Zhou et al., 2022). It is reported that overexpression of DLL4 is associated with poor prognosis in GC patients (Du et al., 2014). Furthermore, the upregulation of DLL4 has been confirmed to promote angiogenesis in the gastric tissue of gastric precancerous lesion groups (Gao et al., 2022).
6.2 Energy metabolism
In eukaryotes, a small amount of ATP is produced in the cytoplasm by glycolysis, but most of the ATP is produced in the mitochondria via oxidative phosphorylation. Mitochondrial function also plays a vital role in regulating lipid biosynthetic pathways and maintaining the levels of numerous metabolites. Research has elucidated that alterations in mitochondrial energy metabolism may represent an important mechanism underlying CAG, particularly in association with deficiencies in the respiratory complex I of mitochondria (Gruno et al., 2008). Similarly, according to the metabolomics-based network pharmacology, it has been reported that there exists a link between mitochondrial energy metabolism dysfunction and CAG (Cui et al., 2017; Liu et al., 2019).
More and more evidence has shown that Hp infection alters metabolites in host lesions, including the tricarboxylic acid (TCA) cycle, choline pathway, and urea cycle (Nishiumi et al., 2017). A urinary metabolomics and network pharmacology study revealed that CAG model rats show a reduction in α-ketoglutarate level, which may be attributed to dysfunction of the TCA cycle and disrupted energy metabolism (Liu et al., 2019). Further research has also shown that Hp infection damages mitochondrial activity, affecting a wide range of metabolites and metabolic pathways (Machado et al., 2013). Additionally, another study shows that Hp infection may result in alterations in energy metabolism accompanied by an increase in both glycolysis and oxidative phosphorylation (Zhu et al., 2024).
Three branched-chain amino acids (BCAAs), namely, leucine, isoleucine, and valine, play crucial roles in numerous physiological functions (Sivanand and Vander Heiden, 2020). Growing evidence indicates that BCAAs are implicated in a wide range of chronic human diseases, such as type 2 diabetes and cardiovascular diseases (White and Newgard, 2019). Meanwhile, two aromatic amino acids (AAAs), tyrosine and phenylalanine, are also essential amino acids that have been linked to the progression of chronic disease. An increase in BCAAs is related to the progression of CAG. According to the 1H NMR metabolomics results, it is evident that the levels of BCAAs and AAAs are significantly increased in the autoimmune CAG group (Xu et al., 2022).
6.3 Gut microbiota and gastric microbiota
Alterations in gut microbiota composition have been associated with various digestive disorders, including irritable bowel syndrome (IBS) and colorectal cancer (Thumann et al., 2019). Accumulating research has shown that changes in the diversity and abundance of gut microbiota play a crucial role during the progression from CAG to GC (Zhao and Yu, 2024). Based on 16s rRNA gene sequencing results, the progression from CAG to GC is characterized by a decrease in beneficial bacteria, such as Akkermansia, and enrichment of pathogens, such as Escherichia_Shigella (Yu C. et al., 2020).
It is generally recognized that microorganisms are unlikely to colonize the stomach because of its acidic conditions. However, recent research has found that Hp infection can lead to increased pH levels, which may allow other gastric microbes to colonize the stomach (Stewart et al., 2020). Meanwhile, an imbalance in the gastric microbiome is tightly associated with gastric diseases, including gastritis, gastric ulcer, and GC (Sohn et al., 2017; Yu et al., 2017). In vivo studies have also shown that gastric microbes contribute to the progression of GC after Hp eradication (Sung et al., 2020). Specifically, a bacterium called P. melaninogenica has been observed to be obviously increased in the gut of patients with bile acid reflux gastritis and GC. This bacterium produces LPS and interacts with TDCA, eventually promoting gastric carcinogenesis (Wang et al., 2022). In addition, a cross-sectional, monocentric study of patients with CAG revealed a higher colonization of Streptococcus, which is linked to a higher risk of GC (Conti et al., 2021). Evidence suggests that Streptococcus anginosus can cause acute inflammatory responses, parietal cell atrophy, and metaplasia (Fu et al., 2024).
Therefore, probiotic intervention is considered as an adjuvant therapy for CAG treatment. A open randomized clinical trial indicates that probiotics significantly improve the Hp eradication effect and limit the progression of CAG (Du et al., 2012). In GES-1 cell experiment, researchers have confirmed that L. acidophilus and L. bulgaricus obviously inhibit Hp adherence to GES-1 cells and regulate TLR4/IκBα/NF-κB signaling pathway (Song et al., 2019). Moreover, probiotics have been observed to regulate the gastric microbiota to alleviate Hp-induced gastric inflammation (He et al., 2022). A study shows that Lacticaseibacillus paracasei positively modulate the gastric microbiome and alleviate CAG in Hp-infected mice (Yu J. et al., 2024). Another experiment also confirms similar view. Lacticaseibacillus paracasei strain LPG-9 is identified as an anti-inflammatory, anti-Hp and gastroprotective probiotic, which is beneficial for CAG treatment (Xu et al., 2023).
6.4 Inflammatory microenvironment
It is well established that the inflammatory microenvironment is modulated by the development and progression of CAG. Inflammatory factors play a vital role in coordinating the inflammatory microenvironment (Table 2). Studies have confirmed that increased level of IL-1β is one of the feature of CAG, contributing to the regulation of mucosa atrophy (Ding et al., 2021). IL-13 acts directly on gastric epithelium cells, leading to metaplastic changes in epithelial cells during the progression from CAG to GC (Noto et al., 2022). Elevated levels of IL-17A induce apoptosis in gastric parietal cells and are related to the disease severity of CAG (Bockerstett et al., 2018). Interferon-γ (IFN-γ), a cytokine secreted by T helper cell 1(Th1 cells), contributes to the release of pro-inflammatory factors. It has been reported that IFN-γ treatment can cause mucous neck cell hyperplasia (Kang et al., 2005).
Peripheral blood monocytes are differentiated into M0 macrophages triggered by macrophage colony-stimulating factor (M-CSF). M0 macrophages can be polarized into M1 macrophages by LPS stimulation, or into M2 macrophages by M2 stimuli (Bosco, 2019). M1 macrophages are capable of producing pro-inflammatory factors including IL-1β, IL-6, IFN-γ, and TNF-α, thereby facilitating the pro-inflammatory response (Murray et al., 2014). In contrast, M2 macrophages can release anti-inflammatory factors such as TGF-β, IL-10, and CD206, contributing to the anti-inflammatory response (Ivashkiv, 2013). Macrophages exert significant effects on HP-induced gastritis. It has been reported that HP promotes M1 polarization by upregulating MAPK, NF-κB, and Notch signaling pathways (Tang et al., 2021; Wen J. et al., 2021). In addition, analysis of gastric specimens from humans and mice with Hp infection shows that the infiltration of M1 macrophages is increased compared to that of M2 macrophages, leading to the production of pro-inflammatory substances (Wei et al., 2024). Studies have revealed that quercetin reverses Hp-infected damage to gastric epithelial cells by regulating M1 macrophage polarization via the SP1/LCN2 axis (Wang Z. et al., 2024).
6.5 Oxidative stress
During the progression of CAG, excessive amounts of ROS and reactive nitrogen species (RNS) are released in the inflammatory tissues of the stomach (Mousavi et al., 2020). The accumulation of ROS is well-recognized for its pivotal role in the pathogenesis of chronic inflammatory disorders. Researchers use the biomarkers of oxidative stress to investigate the effects of Hp eradication. The results show that the expression of iNOS and the production of nitric oxide (NO) are decreased after Hp eradication treatment (Pignatelli et al., 1998). As another evidence of oxidative stress related to CAG, researchers have observed that the level of malondialdehyde (MDA) is higher in the gastric tissue of Hp-infected patients (Wang et al., 2018).
The virulence factors of Hp contribute significantly to the oxidative stress observed in Hp-infected CAG. Studies have shown that higher hydrogen peroxide levels are measured with CagA-positive strains. Furthermore, VacA is capable of the activation of NF-κB signal and the generation of ROS. In addition, the virulence factors of Hp contribute to the accumulation of TNF-α and IL-8, which are important oxidative stress markers.
Hp-induced oxidative stress may contribute to DNA damage (Han et al., 2022). Studies have reported an increase in the level of γH2AX during Hp infection, serving as a marker for double-strand DNA breaks (Pignatelli et al., 2001). Further study shows that Hp-infected AGS cells exhibit a higher expression level of apurinic/apyrimidinic endonuclease 1 (APE1), potentially making it more difficult to repair DNA mutations (Manoel-Caetano et al., 2019).
6.6 Gastric stem cells defect
Gastric stem cells have the potential for self-renewal and differentiation, which contribute to maintaining the homeostasis of the stomach (Hata et al., 2018). In the corpus gland, gastric stem cells are marked by SOX2, LRIG1, and TFF2 (Liu Meng et al., 2023). In the antral gland, gastric stem cells are marked by LGR5, AXIN2, and AQP5 (Xiao and Zhou, 2020). Recent data supports the notion that gastric stem cell defect is an essential pathogenic factor of CAG and GC. By analyzing MNNG-induced CAG model mice and gastric organoids, a recent study shows that gastric stem cell defect is an essential CAG pathogenic factor associated with the decreasing of the EGF signaling pathway (Li et al., 2024). Additionally, another recent study has proved that gastric isthmus stem cells, marked by Mist1, promote gastric injury and inflammation by Wnt5a (Nienhüser et al., 2021).
In addition, accumulating evidence has proved that there is an interaction between gastric stem cells and Hp in the pathogenesis of CAG. Studies have shown that Hp targets Lrig1+ gastric stem cells, which contributes to increased inflammation and malignant proliferation in gastric (Wroblewski et al., 2019). Hp promotes the activation of the R-spondin 3 (Rspo3) signal, which drives the expansion of gastric stem cells. It is well recognized that Lgr4 is a target gene of Rspo3. Therefore, studies have revealed that gastric stem cells promote Hp-induced gastritis via the Rspo3-Lgr4 axis (Wizenty et al., 2022).
6.7 Non-coding RNAs
It is well known that non-coding RNAs (ncRNAs) are major RNA transcripts characterized by the ability to regulate gene expression by epigenetic mechanisms (Chen et al., 2022). The most studied types of ncRNAs include microRNAs (miRNAs), circularRNAs (circRNAs), and long-non-coding RNAs (lncRNAs). An increasing number of studies have identified that ncRNAs play vital roles in many diseases.
MiRNAs are a family of endogenous non-coding RNAs that modulate a diverse range of biological processes. It has become clear that miRNA expression is dysregulated in many human diseases, including cancers of the upper gastrointestinal tract (Peng and Croce, 2016). A single-center cross-sectional study in CAG patients has identified that the expression of miR-146a and miR-370 is substantially downregulated in these patients, suggesting that the dysregulation of miR-146a and miR-370 may be an indicator of early gastric neoplasia (Pita et al., 2021). Another study found that miR-196a-5p is related to the progression of CAG to GC and targets ACER2 for inducing malignant cell proliferation (Zheng et al., 2023). In addition, miRNA dysregulation has been involved in Hp-mediated gastritis and gastric carcinoma. MiRNAs have been reported to be mediators of host immune response by regulating TLRs signaling pathway and NF-κB signaling pathway (Săsăran et al., 2021). Moreover, compelling evidence has demonstrated that miRNAs and Hp regulate cell cycle, cell invasion, and metastasis (Noto and Peek, 2012).
LncRNAs are defined as RNAs over 200 nucleotides long that are not translated into proteins. It is widely believed that lncRNAs play an essential role in physiological and pathological processes and are tightly related to regulating cell cycle, cell apoptosis, and cell proliferation. Studies have suggested that lncRNAs polymorphisms are associated with CAG and GC, indicating that lncRNAs might be biomarkers for the diagnosis of GC (Liu X.-Y. et al., 2022; Petkevicius et al., 2020). Additionally, METTL3-regulated lncRNA SNHG7 has been reported to drive MNNG-induced gastric precancerous lesions and the EMT process (Jian et al., 2024).
6.8 Exosomes
Exosomes are a subset of extracellular vesicles that are secreted by eukaryotic cells and play a key role in intercellular communication (Dai et al., 2020). Exosomes participate in a diverse range of physiological and pathological processes, such as cell differentiation, cell migration, and cancer development. In addition, increasing studies have proven that exosomes can be used as biomarkers for disease diagnosis (Tan et al., 2024).
Many studies have shown that exosomes play an important role in CAG. Evidence has reported that Hp infection can induce the upregulation of miR-155 in exosomes secreted by macrophages, leading to the accumulation of pro-inflammatory cytokines such as IL-6, TNF-α, and IL-23 (Yao et al., 2015). Similarly, another research found that miR-25 levels are elevated in exosomes derived from GES-1 cells after Hp infection, resulting in upregulating NF-κB signaling pathway and the release of pro-inflammatory factors (Li et al., 2019).
7 Recommended treatment and management options for CAG
7.1 Lifestyle intervention
The development of CAG is tightly associated with living habits. Smoking, a high-salt diet, and the consumption of processed meat are linked to an increased prevalence of CAG. Dietary intervention is important for the prevention and treatment of CAG. For instance, studies have found that green tea extract can inhibit the process of CAG and gastric tumorigenesis in mouse models (Jeong et al., 2016). A study also shows that daily intake of sulforaphane-rich broccoli sprouts can prevent Hp and high-salt-induced gastric atrophy (Yanaka et al., 2009). In addition, a buckwheat diet is found to alleviate CAG in C57BL/6 mice, and an oat β-glucans diet can reverse CAG in human patients (Gudej et al., 2021; Li et al., 2020). Early symptoms of CAG can be reversed by improvements in lifestyle interventions and the side effects of non-pharmacological interventions are minimal. However, lifestyle interventions will not inhibit the development of disease in the later stages of CAG. Therefore, drug intervention is still essential.
7.2 Gastric acid secretion regulation medicines
The decreased gastric acid secretion is a major characteristic of CAG. Excessive gastric acid secretion causes histamine to bind to histamine 2 receptors, leading to the release of gastric acid into the gastric lumen (Engevik et al., 2020). H2 receptor antagonists and proton pump inhibitors are the most used medications to regulate gastric acid secretion. The representative drugs are ranitidine, nizatidine, omeprazole, and rabeprazole. In a clinical trial, a combination of ranitidine and omeprazole was found to improve the regulation of gastric acid secretion (Abdul-Hussein et al., 2015).
However, compelling evidence has demonstrated that long-term use of proton pump inhibitors is associated with complications such as GC, gastric neuroendocrine tumors, chronic kidney diseases, and heart failure (Abrahami et al., 2022; Cavalcoli et al., 2015; Hart et al., 2019; Ohori et al., 2023). A study has also revealed that exposure to proton pump inhibitors accelerates the senescence of endothelial cells by reducing telomere length (Yepuri et al., 2016). Given the severity of side effects, it is essential to reduce the unnecessary use of proton pump inhibitors (Farrell et al., 2022; Lee and McDonald, 2020).
7.3 Hp eradication medicines
Hp is a common worldwide pathogen that causes CAG, which can progress to severe gastrointestinal diseases. It is now well known that gastric carcinoma has become a preventable disease through the eradication of Hp. Conventional treatment regimens for Hp eradication include triple therapy and quadruple therapy (Chey et al., 2017). A standard triple therapy consists of a proton pump inhibitor and two antibiotics, such as clarithromycin and amoxicillin. The quadruple therapy is composed of a proton pump inhibitor, bismuth, and two antibiotics (Liu Y. et al., 2018).
However, the eradication rate of Hp is decreasing because of antibiotic resistance (Larsson and Flach, 2022). The underlying mechanisms driving this resistance include impaired regulation of drug uptake and chromosomally encoded mutations (Tshibangu-Kabamba and Yamaoka, 2021). In addition, the recurrence rate of Hp has shown an increasing trend over the past 10 years after eradication (Zhao et al., 2021). Studies have also revealed that Hp eradication therapy may lead to an increase in the level of pathogenic antibiotic-resistant bacteria in the gut (Hsu et al., 2019; Liou et al., 2020).
7.4 Gastric mucosal protective agents
The protection of the structure and physiological functions of the gastric mucosa plays an important role in CAG treatment. The gastric mucosal integrity is maintained by a variety of mechanisms, including pre-epithelial factors, the generation of PGs, surface epithelial cells connected with mucus, trefoil peptides, and so on (Laine et al., 2008). The most commonly used gastric mucosal protective agents include sucralfate, bismuth potassium citrate, and teprenone, which are reported to enhance mucus synthesis and secretion.
7.5 Traditional Chinese medicines treatment
Traditional Chinese medicines (TCM) play a vital role in the treatment of CAG and have gotten more attention for clinical applications. It is well known that TCM includes bioactive ingredients, proprietary Chinese medicines, and classical prescription medicines. Studies have shown great potential for TCM in the field of CAG treatment due to its multi-target regulatory abilities and low toxicity.
In a randomized double-blind clinical trial, treatment with Weierkang Pills was found to regulate the levels of trefoil peptides 3 (TFF3) and endothelial growth factor in biopsy specimens (Chen et al., 2023). In addition, numerous randomized clinical trials have confirmed that Moluodan protects gastric mucosa and reverses CAG without drug-induced side effects (Zou et al., 2024). Numerous classical prescriptions have also been confirmed to alleviate CAG, including Sijunzi Decoction, Huangqijianzhong Decoction, and Huazhuojiedu Decoction (Hao et al., 2022; Liu W. Z. et al., 2018; Zhu et al., 2024).
Many bioactive ingredients have also been proven to treat CAG. Astragaloside IV, a saponin derived from Astragalus membranaceous Bge, has been found to modulate the Ambra1/Beclin1 complex and inhibit autophagy, which contributes to the treatment of MNNG-induced gastric precancerous lesions (Cai et al., 2018). Kaempferol, a natural ingredient in Chaishaoliujun Decoction, can significantly decrease the expression of Shh, Ptch1, and Gli at both protein and mRNA levels, suggesting that it can inactivate the Hedgehog signaling pathway (Tu et al., 2022). Curcumin has also been found to inhibit the expression of TLRs and MyD88 in the Hp-infected mouse model (Santos et al., 2015).
TCM treatment is suitable for long-term use in CAG due to its lower toxicity and minimal side effects. However, there are some limitations in the development of TCM as a treatment for CAG. Toxicological and pharmacokinetic studies should be conducted to validate the safety of TCM. Furthermore, novel experimental methods should be developed to investigate the mechanisms of action of TCM. In addition, double-blind clinical trials are a crucial process for the future development of TCM.
8 Conclusion
Chronic atrophic gastritis (CAG) is a slowly progressing and intricate inflammatory disorder of the digestive system that imposes considerable economic and social consequences on individuals and society. Correa’s cascade indicates that CAG is a pivotal stage in the transition from gastritis to gastric cancer. Helicobacter pylori (Hp) infection significantly contributes to the onset of chronic non-atrophic gastritis, which evolves into CAG over decades, finally resulting in intestinal metaplasia and invasive cancer. In addition to its association with stomach cancer progression, CAG has been connected to compromised bone health, depression, and iron deficiency anemia (Aasarød et al., 2016; Nahon et al., 2003; Zhao et al., 2018). The prevention, early detection, and treatment of CAG necessitate considerable focus due to its extensive health ramifications. This review methodically analyzes the pathogenetic mechanisms of CAG, emphasizing the significance of programmed cell death and critical signaling pathways, including NF-κB, Hedgehog, PI3K/Akt, MAPKs, Wnt/β-catenin, p53, and Hippo. Furthermore, novel pathways including angiogenesis, energy metabolism, gut and stomach microbiome, inflammatory microenvironments, oxidative stress, gastric stem cell abnormalities, non-coding RNAs, and exosomes are examined, providing significant insights into prospective treatment targets. Although these pathways provide significant potential for enhancing CAG management, several, including non-coding RNAs and exosomes, are still in the preliminary phases of investigation. Obstacles, including the translation of molecular discoveries into clinical application and the management of patient variability, must be surmounted to optimize their potential. Future research must concentrate on discovering novel biomarkers for early detection, formulating targeted therapeutics to prevent the progression of CAG to gastric cancer, and utilizing modern technical approaches, such as proteomics and metabolomics, to elucidate regulatory mechanisms. By prioritizing these issues, researchers can facilitate the development of safer and more effective therapy strategies, hence enhancing outcomes for patients with CAG.
Author contributions
WK: Conceptualization, Data curation, Funding acquisition, Resources, Supervision, Writing–review and editing. JX: Formal Analysis, Investigation, Methodology, Writing–original draft. FX: Data curation, Formal Analysis, Investigation, Visualization, Writing–original draft. WH: Data curation, Formal Analysis, Investigation, Visualization, Writing–original draft. MM: Data curation, Formal Analysis, Investigation, Visualization, Writing–original draft. HS: Data curation, Formal Analysis, Investigation, Visualization, Writing–original draft. XY: Data curation, Formal Analysis, Funding acquisition, Investigation, Methodology, Writing–original draft. YR: Data curation, Formal Analysis, Funding acquisition, Investigation, Methodology, Writing–original draft. XH: Conceptualization, Data curation, Funding acquisition, Supervision, Writing–review and editing.
Funding
The author(s) declare that financial support was received for the research, authorship, and/or publication of this article. This research was funded by Guangdong Medical University Research Foundation (4SG24205G, 4SG24257G), Discipline Construction Project of Guangdong Medical University (4SG24002G, 4SG21229GDGFY01), Dongguan Science and Technology of Social Development Program (20211800905542, 20231800939832), Guangdong Basic and Applied Basic Research Foundation-Yifang Pharmaceutical Joint Fund (2023A1515220233), Natural Science Foundation of Guangdong Province (2022A1515011302, 2023A1515011116) and Student Innovation Research and Entrepreneurship Training of Guangdong Medical University (GDMU2023144, GDMU2023109).
Acknowledgments
All these figures are created with Figdraw.com.
Conflict of interest
The authors declare that the research was conducted in the absence of any commercial or financial relationships that could be construed as a potential conflict of interest.
Generative AI statement
The author(s) declare that no Generative AI was used in the creation of this manuscript.
Publisher’s note
All claims expressed in this article are solely those of the authors and do not necessarily represent those of their affiliated organizations, or those of the publisher, the editors and the reviewers. Any product that may be evaluated in this article, or claim that may be made by its manufacturer, is not guaranteed or endorsed by the publisher.
References
Aasarød, K. M., Mosti, M. P., Stunes, A. K., Reseland, J. E., Basso, T., Syversen, U., et al. (2016). Impaired skeletal health in patients with chronic atrophic gastritis. Scand. J. Gastroentero. 51, 774–781. doi:10.3109/00365521.2016.1141317
Abdul-Hussein, M., Freeman, J., and Castell, D. (2015). Concomitant administration of a histamine2 receptor antagonist and proton pump inhibitor enhances gastric acid suppression. Pharmacotherapy 35, 1124–1129. doi:10.1002/phar.1665
Abrahami, D., McDonald, E. G., Schnitzer, M. E., Barkun, A. N., Suissa, S., and Azoulay, L. (2022). Proton pump inhibitors and risk of gastric cancer: population-based cohort study. Gut 71, 16–24. doi:10.1136/gutjnl-2021-325097
Airley, R. E., and Mobasheri, A. (2007). Hypoxic regulation of glucose transport, anaerobic metabolism and angiogenesis in cancer: novel pathways and targets for anticancer therapeutics. Chemotherapy 53, 233–256. doi:10.1159/000104457
Al-Balushi, M. S., Said, E. A., Hasson, S. S., Al-Busaidi, J. Z., Al-Reesi, I., Idris, M., et al. (2016). High levels of IgA antibodies to Helicobacter Pylori among Omani women during pregnancy and after delivery. Iran. J. Immunol. 13, 114–123. ijiv13i2a4.
Allen, L.-A. H. (2007). Phagocytosis and persistence of Helicobacter pylori. Cell. Microbiol. 9, 817–828. doi:10.1111/j.1462-5822.2007.00906.x
Allison, C. C., Kufer, T. A., Kremmer, E., Kaparakis, M., and Ferrero, R. L. (2009). Helicobacter Pylori induces MAPK phosphorylation and AP-1 activation via a NOD1-dependent mechanism. J. Immunol. 183, 8099–8109. doi:10.4049/jimmunol.0900664
Al-Toubah, T. E., Cives, M., Valone, T., Blue, K., and Strosberg, J. R. (2019). Sensitivity and specificity of the NETest: a validation study. JCO 37, 222. doi:10.1200/JCO.2019.37.4_suppl.222
Arab, H. H., Salama, S. A., Eid, A. H., Kabel, A. M., and Shahin, N. N. (2019). Targeting MAPKs, NF-κB, and PI3K/AKT pathways by Methyl Palmitate ameliorates ethanol-induced gastric mucosal injury in rats. J. Cell. Physiol. 234, 22424–22438. doi:10.1002/jcp.28807
Aziz, S., Rasheed, F., Zahra, R., and König, S. (2022). Gastric cancer pre-stage detection and early diagnosis of gastritis using serum protein signatures. Molecules 27, 2857. doi:10.3390/molecules27092857
Bahar, M. E., Kim, H. J., and Kim, D. R. (2023). Targeting the RAS/RAF/MAPK pathway for cancer therapy: from mechanism to clinical studies. Sig. Transduct. Target Ther. 8, 455. doi:10.1038/s41392-023-01705-z
Barton, G. M., and Medzhitov, R. (2003). Toll-Like receptor signaling pathways. Science 300, 1524–1525. doi:10.1126/science.1085536
Bauer, M., Nascakova, Z., Mihai, A.-I., Cheng, P. F., Levesque, M. P., Lampart, S., et al. (2020). The ALPK1/TIFA/NF-κB axis links a bacterial carcinogen to R-Loop-induced replication stress. Nat. Commun. 11, 5117. doi:10.1038/s41467-020-18857-z
Bieging, K. T., Mello, S. S., and Attardi, L. D. (2014). Unravelling mechanisms of P53-mediated tumour suppression. Nat. Rev. Cancer 14, 359–370. doi:10.1038/nrc3711
Bockerstett, K. A., Osaki, L. H., Petersen, C. P., Cai, C. W., Wong, C. F., Nguyen, T.-L. M., et al. (2018). Interleukin-17A promotes parietal cell atrophy by inducing apoptosis. Cell Mol. gastroenter. 5, 678–690.e1. doi:10.1016/j.jcmgh.2017.12.012
Bosco, M. C. (2019). Macrophage polarization: reaching across the aisle? J. Allergy Clin. Immun. 143, 1348–1350. doi:10.1016/j.jaci.2018.12.995
Bradesi, S., Schwetz, I., Ennes, H. S., Lamy, C. M. R., Ohning, G., Fanselow, M., et al. (2005). Repeated exposure to water avoidance stress inrats: a new model for sustained visceral hyperalgesia. Am. J. Physiol-Gastr. L. 289, G42–G53. doi:10.1152/ajpgi.00500.2004
Buti, L., Spooner, E., Van Der Veen, A. G., Rappuoli, R., Covacci, A., and Ploegh, H. L. (2011). Helicobacter pylori cytotoxin-associated gene A (CagA) subverts the apoptosis-stimulating protein of p53 (ASPP2) tumor suppressor pathway of the host. Proc. Natl. Acad. Sci. U.S.A. 108, 9238–9243. doi:10.1073/pnas.1106200108
Cai, Q., Shi, P., Yuan, Y., Peng, J., Ou, X., Zhou, W., et al. (2021). Inflammation-associated senescence promotes Helicobacter Pylori–induced atrophic gastritis. Cell. Mol. Gastroenter. 11, 857–880. doi:10.1016/j.jcmgh.2020.10.015
Cai, T., Zhang, C., Zhao, Z., Li, S., Cai, H., Chen, X., et al. (2018). The gastric mucosal protective effects of Astragaloside IV in MNNG-induced GPL Rats. Biomed. Pharmacother. 104, 291–299. doi:10.1016/j.biopha.2018.04.013
Camilo, V., Sugiyama, T., and Touati, E. (2017). Pathogenesis of Helicobacter pylori infection. Helicobacter 22, e12405. doi:10.1111/hel.12405
Cao, J. Y., and Dixon, S. J. (2016). Mechanisms of ferroptosis. Cell. Mol. Life Sci. 73, 2195–2209. doi:10.1007/s00018-016-2194-1
Capitani, N., Codolo, G., Vallese, F., Minervini, G., Grassi, A., Cianchi, F., et al. (2019). The lipoprotein HP1454 of Helicobacter pylori regulates T -cell response by shaping T -cell receptor signalling. Cell. Microbiol. 21, e13006. doi:10.1111/cmi.13006
Cascetta, G., Colombo, G., Eremita, G., Garcia, J. G. N., Lenti, M. V., Di Sabatino, A., et al. (2024). Pro- and anti-inflammatory cytokines: the hidden keys to autoimmune gastritis therapy. Front. Pharmacol. 15, 1450558. doi:10.3389/fphar.2024.1450558
Cavalcoli, F., Zilli, A., Conte, D., Ciafardini, C., and Massironi, S. (2015). Gastric neuroendocrine neoplasms and proton pump inhibitors: fact or coincidence? Scand. J. Gastroentero. 50, 1397–1403. doi:10.3109/00365521.2015.1054426
Chang, W., Bai, J., Tian, S., Ma, M., Li, W., Yin, Y., et al. (2017). Autophagy protects gastric mucosal epithelial cells from ethanol-induced oxidative damage via mTOR signaling pathway. Exp. Biol. Med. (Maywood). 242, 1025–1033. doi:10.1177/1535370216686221
Chapelle, N., Martin, J., Osmola, M., Hémont, C., Leroy, M., Vibet, M.-A., et al. (2023). Serum pepsinogens can help to discriminate between H. pylori-induced and auto-immune atrophic gastritis: results from a prospective multicenter study. Dig. Liver. Dis. 55, 1345–1351. doi:10.1016/j.dld.2023.03.015
Chen, B., Dragomir, M. P., Yang, C., Li, Q., Horst, D., and Calin, G. A. (2022). Targeting non-coding RNAs to overcome cancer therapy resistance. Sig. Transduct. Target. Ther. 7, 121. doi:10.1038/s41392-022-00975-3
Chen, Q., Kang, J., and Fu, C. (2018). The independence of and associations among apoptosis, autophagy, and necrosis. Sig. Transduct. Target. Ther. 3, 18. doi:10.1038/s41392-018-0018-5
Chen, X., Shen, K., Deng, Y., Mo, J., Ni, J., Hendi, M., et al. (2023). A randomized double-blind clinical trial of Weierkang Pills for the treatment of chronic atrophic gastritis. J. Ethnopharmacol. 57, 165–171. doi:10.1097/MCG.0000000000001663
Chen Y., Y., Fu, R., Xu, M., Huang, Y., Sun, G., and Xu, L. (2018). N-Methyl-N-Nitro-N-Nitrosoguanidine-mediated ING4 downregulation contributed to the angiogenesis of transformed human gastric epithelial cells. Life Sci. 199, 179–187. doi:10.1016/j.lfs.2018.02.034
Chey, W. D., Leontiadis, G. I., Howden, C. W., and Moss, S. F. (2017). ACG clinical guideline: treatment of Helicobacter pylori infection. Am. J. Gastroenterol. 112, 212–239. doi:10.1038/ajg.2016.563
Conti, L., Borro, M., Milani, C., Simmaco, M., Esposito, G., Canali, G., et al. (2021). Gastric microbiota composition in patients with corpus atrophic gastritis. Dig. Liver Dis. 53, 1580–1587. doi:10.1016/j.dld.2021.05.005
Cover, T. L., and Blanke, S. R. (2005). Helicobacter Pylori VacA, a paradigm for toxin multifunctionality. Nat. Rev. Microbiol. 3, 320–332. doi:10.1038/nrmicro1095
Cui, J., Cui, H., Yang, M., Du, S., Li, J., Li, Y., et al. (2019). Tongue coating microbiome as a potential biomarker for gastritis including precancerous cascade. Protein Cell 10, 496–509. doi:10.1007/s13238-018-0596-6
Cui, J., Liu, Y., Hu, Y., Tong, J., Li, A., Qu, T., et al. (2017). NMR-based metabonomics and correlation analysis reveal potential biomarkers associated with chronic atrophic gastritis. J. Pharm. Biomed. Anal. 132, 77–86. doi:10.1016/j.jpba.2016.09.044
Dai, J., Su, Y., Zhong, S., Cong, L., Liu, B., Yang, J., et al. (2020). Exosomes: key players in cancer and potential therapeutic strategy. Sig. Transduct. Target. Ther. 5, 145. doi:10.1038/s41392-020-00261-0
Dang, Y., Zhang, Y., Xu, L., Zhou, X., Gu, Y., Yu, J., et al. (2020). PUMA-mediated epithelial cell apoptosis promotes Helicobacter Pylori infection-mediated gastritis. Cell Death Dis. 11, 139. doi:10.1038/s41419-020-2339-x
De Vries, A. C., Van Grieken, N. C. T., Looman, C. W. N., Casparie, M. K., De Vries, E., Meijer, G. A., et al. (2008). Gastric cancer risk in patients with premalignant gastric lesions: a nationwide cohort study in The Netherlands. Gastroenterology 134, 945–952. doi:10.1053/j.gastro.2008.01.071
Ding, L., Chakrabarti, J., Sheriff, S., Li, Q., Thi Hong, H. N., Sontz, R. A., et al. (2022). Toll-like receptor 9 pathway mediates schlafen+-MDSC polarization during helicobacter-induced ggastric metaplasias. Gastroenterology 163, 411–425.e4. doi:10.1053/j.gastro.2022.04.031
Ding, L., Sontz, E. A., Saqui-Salces, M., and Merchant, J. L. (2021). Interleukin-1β suppresses gastrin via primary cilia and induces antral hyperplasia. Cell Mol. Gastroenter 11, 1251–1266. doi:10.1016/j.jcmgh.2020.12.008
Dixon, M. F., Mapstone, N. P., Neville, P. M., Moayyedi, P., and Axon, A. T. R. (2002). Bile reflux gastritis and intestinal metaplasia at the cardia. Gut 51, 351–355. doi:10.1136/gut.51.3.351
Dixon, S. J., Lemberg, K. M., Lamprecht, M. R., Skouta, R., Zaitsev, E. M., Gleason, C. E., et al. (2012). Ferroptosis: an iron-dependent form of nonapoptotic cell death. Cell 149, 1060–1072. doi:10.1016/j.cell.2012.03.042
Dong, T., Lan, X., Fan, B., Jia, S., Liu, F., Feng, Q., et al. (2023). Gastric bacteria as potential biomarkers for the diagnosis of atrophic gastritis. Mol. Biol. Rep. 50, 655–664. doi:10.1007/s11033-022-08001-z
Du, X., Cheng, Z., Wang, Y.-H., Guo, Z.-H., Zhang, S.-Q., Hu, J.-K., et al. (2014). Role of Notch signaling pathway in gastric cancer: a Meta-Analysis of the literature. World J. Gastroenterol. 20, 9191–9199. doi:10.3748/wjg.v20.i27.9191
Du, Y.-Q., Su, T., Fan, J. G., Lu, Y. X., Zheng, P., Li, X. H., et al. (2012). Adjuvant probiotics improve the eradication effect of triple therapy for Helicobacter pylori infection. WJG 18, 6302–6307. doi:10.3748/wjg.v18.i43.6302
Engevik, A. C., Kaji, I., and Goldenring, J. R. (2020). The physiology of the gastric parietal cell. Physiol. Rev. 100, 573–602. doi:10.1152/physrev.00016.2019
Farrell, B., Lass, E., Moayyedi, P., Ward, D., and Thompson, W. (2022). Reduce unnecessary use of proton pump inhibitors. BMJ e069211, e069211. doi:10.1136/bmj-2021-069211
Finch, D. K., Ettinger, R., Karnell, J. L., Herbst, R., and Sleeman, M. A. (2013). Effects of CXCL 13 inhibition on lymphoid follicles in models of autoimmune disease. Eur. J. Clin. Invest. 43, 501–509. doi:10.1111/eci.12063
Foegeding, N., Caston, R., McClain, M., Ohi, M., and Cover, T. (2016). An overview of Helicobacter pylori VacA toxin biology. Toxins 8, 173. doi:10.3390/toxins8060173
Fu, K., Cheung, A. H. K., Wong, C. C., Liu, W., Zhou, Y., Wang, F., et al. (2024). Streptococcus anginosus promotes gastric inflammation, atrophy, and tumorigenesis in mice. Cell 187, 882–896.e17. doi:10.1016/j.cell.2024.01.004
Fu, M., Hu, Y., Lan, T., Guan, K.-L., Luo, T., and Luo, M. (2022). The Hippo signalling pathway and its implications in human health and diseases. Sig. Transduct. Target. Ther. 7, 376. doi:10.1038/s41392-022-01191-9
Fu, Y., Wu, H., Cui, H., Li, Y., and Li, C. (2018). Gastroprotective and Anti-ulcer Effects of Oxymatrine against several gastric ulcer models in rats: possible roles of antioxidant, antiinflammatory, and prosurvival mechanisms. Phytother. Res. 32, 2047–2058. doi:10.1002/ptr.6148
Gai, X., Qian, P., Guo, B., Zheng, Y., Fu, Z., Yang, D., et al. (2023). Heptadecanoic acid and pentadecanoic acid crosstalk with fecal-derived gut microbiota are potential non-invasive biomarkers for chronic atrophic gastritis. Front. Cell. Infect. Microbiol. 12, 1064737. doi:10.3389/fcimb.2022.1064737
Gao, Y., Wang, J., Zhao, M., Xia, T., Liu, Q., Chen, N., et al. (2022). Atractylenolide III attenuates angiogenesis in gastric precancerous lesions through the downregulation of Delta-Like Ligand 4. Front. Pharmacol. 13, 797805. doi:10.3389/fphar.2022.797805
Goldenring, J. (2023). No H. pylori, no adenocarcinoma for patients with autoimmune gastritis. Gut 72, 1–2. doi:10.1136/gutjnl-2022-328068
Goldman, A., Shahidullah, M., Goldman, D., Khailova, L., Watts, G., Delamere, N., et al. (2010). A novel mechanism of acid and bile acid-induced DNA damage involving Na +/H + exchanger: implication for Barrett’s oesophagus. Gut 59, 1606–1616. doi:10.1136/gut.2010.213686
Gorrell, R. J., Guan, J., Xin, Y., Tafreshi, M. A., Hutton, M. L., McGuckin, M. A., et al. (2013). A novel NOD1-and CagA-independent pathway of Interleukin-8 induction mediated by the Helicobacter Pylori Type IV secretion system: the role of H. Pylori CagL in IL-8 secretion. Cell Microbiol. 15, 554–570. doi:10.1111/cmi.12055
Gruno, M., Peet, N., Tein, A., Salupere, R., Sirotkina, M., Valle, J., et al. (2008). Atrophic gastritis: deficient Complex I of the respiratory chain in the mitochondria of corpus mucosal cells. J. Gastroenterol. 43, 780–788. doi:10.1007/s00535-008-2231-4
Gudej, S., Filip, R., Harasym, J., Wilczak, J., Dziendzikowska, K., Oczkowski, M., et al. (2021). Clinical outcomes after oat Beta-Glucans dietary treatment in gastritis patients. Nutrients 13, 2791. doi:10.3390/nu13082791
Gunawardhana, N., Jang, S., Choi, Y. H., Hong, Y. A., Jeon, Y.-E., Kim, A., et al. (2018). Helicobacter pylori-induced HB-EGF upregulates gastrin expression via the EGF Receptor, C-Raf, Mek1, and Erk2 in the MAPK pathway. Front. Cell. Infect. Microbiol. 7, 541. doi:10.3389/fcimb.2017.00541
Guo, Q., Jin, Y., Chen, X., Ye, X., Shen, X., Lin, M., et al. (2024). NF-κB in biology and targeted therapy: new insights and translational implications. Sig. Transduct. Target. Ther. 9, 53. doi:10.1038/s41392-024-01757-9
Guo, S., Gao, Q., Jiao, Q., Hao, W., Gao, X., and Cao, J. M. (2012). Gastric mucosal damage in water immersion stress: mechanism and prevention with GHRP-6. WJG 18, 3145–3155. doi:10.3748/wjg.v18.i24.3145
Han, L., Shu, X., and Wang, J. (2022). Helicobacter pylori-mediated oxidative stress and gastric diseases: a review. Front. Microbiol. 13, 811258. doi:10.3389/fmicb.2022.811258
Han, Y., Li, Ya, Hu, Z., Wang, X., Liu, J., Ren, X., et al. (2020). Hydrogen sulfide-mediated resistance against water avoidance stress-induced gastritis by maintenance of gastric microbial homeostasis. MicrobiologyOpen 9, e00951. doi:10.1002/mbo3.951
Hanahan, D., and Weinberg, R. A. (2011). Hallmarks of cancer: the next generation. Cell 144, 646–674. doi:10.1016/j.cell.2011.02.013
Hansson, M., Hermansson, M., Svensson, H., Elfvin, A., Hansson, L.-E., Johnsson, E., et al. (2008). CCL28 is increased in human Helicobacter pylori -induced gastritis and mediates recruitment of gastric immunoglobulin A-secreting Cells. Infect. Immun. 76, 3304–3311. doi:10.1128/IAI.00041-08
Hao, X., Zhou, P., Yang, Z., Yang, T., and Wang, Y. (2022). The therapeutic effect of Huazhuojiedu Decoction on precancerous lesions in a gastric cancer model via the regulation of Lnc 517368. J. Ethnopharmacol. 283, 114635. doi:10.1016/j.jep.2021.114635
Hart, E., Dunn, T. E., Feuerstein, S., and Jacobs, D. M. (2019). Proton pump inhibitors and risk of acute and chronic kidney disease: a retrospective cohort study. Pharmacotherapy 39, 443–453. doi:10.1002/phar.2235
Hata, M., Hayakawa, Y., and Koike, K. (2018). Gastric stem cell and cellular origin of cancer. Biomedicines 6, 100. doi:10.3390/biomedicines6040100
He, C., Peng, C., Xu, X., Li, N., Ouyang, Y., Zhu, Y., et al. (2022). Probiotics mitigate Helicobacter pylori-induced gastric inflammation and premalignant lesions in INS-GAS mice with the modulation of gastrointestinal microbiota. Helicobacter 27, e12898. doi:10.1111/hel.12898
He, Y., Sun, M. M., Zhang, G. G., Yang, J., Chen, K. S., Xu, W. W., et al. (2021). Targeting PI3K/Akt signal transduction for cancer therapy. Sig. Transduct. Target. Ther. 6, 425. doi:10.1038/s41392-021-00828-5
Hernández, C., Barrachina, M. D., Vallecillo-Hernández, J., Álvarez, Á., Ortiz-Masiá, D., Cosín-Roger, J., et al. (2016). Aspirin-induced gastrointestinal damage is associated with an inhibition of epithelial cell autophagy. J. Gastroenterol. 51, 691–701. doi:10.1007/s00535-015-1137-1
Hong, M., Moon, S.-K., Kim, H., and Hwang, D. (2024). Elucidating Korean meadowsweet (filipendula glaberrima nakai)-derived arabinogalactan protein-induced macrophage activation and its associated mechanism of action. Int. J. Biol. Macromol. 273, 132999. doi:10.1016/j.ijbiomac.2024.132999
Hsu, P., Pan, C., Kao, J. Y., Tsay, F., Peng, N., Kao, S., et al. (2019). Short-term and long-term Iimpacts of Helicobacter Pylori eradication with reverse hybrid therapy on the gut microbiota. J. Gastroen. Hepatol. 34, 1968–1976. doi:10.1111/jgh.14736
Hu, P. J., Yu, J., Zeng, Z. R., Leung, W. K., Lin, H. L., Tang, B. D., et al. (2004). Chemoprevention of gastric cancer by celecoxib in rats. Gut 53, 195–200. doi:10.1136/gut.2003.021477
Huang, G., Wang, S., Wang, J., Tian, L., Yu, Y., Zuo, X., et al. (2022). Bile reflux alters the profile of the gastric mucosa microbiota. Front. Cell. Infect. Microbiol. 12, 940687. doi:10.3389/fcimb.2022.940687
Isomoto, H., Mizuta, Y., Miyazaki, M., Takeshima, F., Omagari, K., Murase, K., et al. (2000). Implication of nf-κb in Helicobacter pylori -associated gastritis. Am. J. Gastroenterol. 95, 2768–2776. doi:10.1111/j.1572-0241.2000.02304.x
Ivashkiv, L. B. (2013). Epigenetic regulation of macrophage polarization and function. Trends Immunol. 34, 216–223. doi:10.1016/j.it.2012.11.001
Jeong, M., Park, J., Han, Y., Kangwan, N., Kwon, S., Kim, B., et al. (2016). Dietary intervention of Artemisia and green tea extracts to rejuvenate Helicobacter pylori -associated chronic atrophic gastritis and to prevent tumorigenesis. Helicobacter 21, 40–59. doi:10.1111/hel.12229
Jia, J., Zhao, H., Li, F., Zheng, Q., Wang, G., Li, D., et al. (2024). Research on drug treatment and the novel signaling pathway of chronic atrophic gastritis. Biomed. Pharmacother. 176, 116912. doi:10.1016/j.biopha.2024.116912
Jian, J., Feng, Y., Wang, R., Li, C., Zhang, L., Ruan, Y., et al. (2024). METTL3-regulated lncRNA SNHG7 drives MNNG-induced epithelial–mesenchymal transition in gastric precancerous lesions. Toxics 12, 573. doi:10.3390/toxics12080573
Jiang, J. (2022). Hedgehog signaling mechanism and role in cancer. Semin. Cancer Biol. 85, 107–122. doi:10.1016/j.semcancer.2021.04.003
Jiang, J.-Y., Liu, D.-J., and Liu, M.-X. (2021). The protective effect of NF-κB signaling pathway inhibitor PDTC on mice with chronic atrophic gastritis. Scand. J. Gastroentero. 56, 1131–1139. doi:10.1080/00365521.2021.1953130
Johnson, G. L., and Lapadat, R. (2002). Mitogen-activated protein kinase pathways mediated by ERK, JNK, and p38 protein kinases. Science 298, 1911–1912. doi:10.1126/science.1072682
Junyuan, Z., Hui, X., Chunlan, H., Junjie, F., Qixiang, M., Yingying, L., et al. (2018). Quercetin protects against intestinal barrier disruption and inflammation in acute necrotizing pancreatitis through TLR4/MyD88/p38 MAPK and ERS inhibition. Pancreatology 18, 742–752. doi:10.1016/j.pan.2018.08.001
Kacar, F., Meteoğlu, I., Yasa, H., and Levi, E. (2007). Helicobacter Pylori-induced changes in the gastric mucosa are associated with Mitogen-Activated Protein Kinase (MAPK) activation. Appl. Immunohisto M. M. 15, 224–228. doi:10.1097/01.pai.0000209863.35828.dd
Kang, W., Rathinavelu, S., Samuelson, L. C., and Merchant, J. L. (2005). Interferon gamma induction of gastric mucous neck cell hypertrophy. Lab. Invest. 85, 702–715. doi:10.1038/labinvest.3700260
Karayiannis, I., Martinez-Gonzalez, B., Kontizas, E., Kokkota, A. V., Petraki, K., Mentis, A., et al. (2023). Induction of MMP -3 and MMP -9 expression during Helicobacter pylori infection via MAPK signaling pathways. Helicobacter 28, e12987. doi:10.1111/hel.12987
Kastenhuber, E. R., and Lowe, S. W. (2017). Putting p53 in context. Cell 170, 1062–1078. doi:10.1016/j.cell.2017.08.028
Kawano, S., and Tsuji, S. (2000). Role of mucosal blood flow: a conceptional review in gastric mucosal injury and protection. J. Gastro. Hepatol. 15, 1–6. doi:10.1046/j.1440-1746.2000.02142.x
Keates, S., Hitti, Y., Upton, M., and Kelly, C. (1997). Helicobacter pylori infection activates NF-kappa B in gastric epithelial cells. Gastroenterology 113, 1099–1109. doi:10.1053/gast.1997.v113.pm9322504
Kikuchi, T., Kato, K., Ohara, S., Sekine, H., Arikawa, T., Suzuki, T., et al. (2000). The relationship between persistent secretion of RANTES and residual infiltration of eosinophils and memory T lymphocytes after Helicobacter pylori eradication. J. Pathol. 192, 243–250. doi:10.1002/1096-9896(2000)9999:9999<::AID-PATH688>3.0.CO;2-D
Kim, S. A., Oh, J., Choi, S. R., Lee, C. H., Lee, B.-H., Lee, M.-N., et al. (2021). Anti-gastritis and anti-lung injury effects of pine tree ethanol extract targeting both NF-κB and AP-1 pathways. Molecules 26, 6275. doi:10.3390/molecules26206275
Kitajima, Y., and Miyazaki, K. (2013). The critical impact of HIF-1a on gastric cancer biology. Cancers 5, 15–26. doi:10.3390/cancers5010015
Kobayashi, J. (2018). Effect of diet and gut environment on the gastrointestinal formation of N -nitroso compounds: a review. Nitric Oxide 73, 66–73. doi:10.1016/j.niox.2017.06.001
Konstantinou, D., Bertaux-Skeirik, N., and Zavros, Y. (2016). Hedgehog signaling in the stomach. Curr. Opin. Pharmacol. 31, 76–82. doi:10.1016/j.coph.2016.09.003
Kuhnle, G., Story, G., Reda, T., Mani, A., Moore, K., Lunn, J., et al. (2007). Diet-induced endogenous formation of nitroso compounds in the GI tract. Free Radic. Biol.Med. 43, 1040–1047. doi:10.1016/j.freeradbiomed.2007.03.011
Kuligowski, J., Sanjuan-Herráez, D., Vázquez-Sánchez, M. A., Brunet-Vega, A., Pericay, C., Ramírez-Lázaro, M. J., et al. (2016). Metabolomic analysis of gastric cancer progression within the Correa’s Cascade using ultraperformance liquid chromatography–mass spectrometry. J. Proteome Res. 15, 2729–2738. doi:10.1021/acs.jproteome.6b00281
Kumar, S., and Dhiman, M. (2008). Inflammasome activation and regulation during Helicobacter pylori pathogenesis. Microb. Pathog. 125, 468–474. doi:10.1016/j.micpath.2018.10.012
Kurashima, Y., Murata-Kamiya, N., Kikuchi, K., Higashi, H., Azuma, T., Kondo, S., et al. (2008). Deregulation of β-catenin signal by Helicobacter Pylori CagA requires the CagA-multimerization sequence. Int. J.Cancer. 122, 823–831. doi:10.1002/ijc.23190
Lahner, E., Marzinotto, I., Lampasona, V., Dottori, L., Bazzigaluppi, E., Brigatti, C., et al. (2020). Autoantibodies toward ATP4A and ATP4B subunits of gastric proton pump H+,K+-ATPase are reliable serological pre-Endoscopic markers of corpus atrophic gastritis. Clin. Transl. Gastroenterol. 11, e00240. doi:10.14309/ctg.0000000000000240
Laine, L., Takeuchi, K., and Tarnawski, A. (2008). Gastric mucosal defense and cytoprotection: bench to bedside. Gastroenterology 135, 41–60. doi:10.1053/j.gastro.2008.05.030
Laing, K., and Secombes, C. J. (2004). Chemokines. Dev. Com. Immunol. 28, 443–460. doi:10.1016/j.dci.2003.09.006
Larsson, D. G. J., and Flach, C.-F. (2022). Antibiotic resistance in the environment. Nat. Rev. Microbiol. 20, 257–269. doi:10.1038/s41579-021-00649-x
Lee, C. Y. Q., Chan, Y. T., Cheok, Y. Y., Tan, G. M. Y., Tang, T. F., Cheong, H. C., et al. (2022). Helicobacter pylori infection elicits type I interferon response in human monocytes via toll-like receptor 8 signaling. J. Immunol. Res. 2022, 3861518. doi:10.1155/2022/3861518
Lee, T. C., and McDonald, E. G. (2020). Deprescribing proton pump inhibitors: overcoming resistance. JAMA Int. Med. 180, 571–573. doi:10.1001/jamainternmed.2020.0040
Lei, Z.-N., Teng, Q.-X., Tian, Q., Chen, W., Xie, Y., Wu, K., et al. (2022). Signaling pathways and therapeutic interventions in gastric cancer. Sig. Transduct. Target. Ther. 7, 358. doi:10.1038/s41392-022-01190-w
Leung, W. K., Wu, K., Wong, C. Y. P., Cheng, A. S. L., Ching, A. K. K., Chan, A. W. H., et al. (2008). Transgenic cyclooxygenase-2 expression and high salt enhanced susceptibility to chemical-induced gastric cancer development in mice. Carcinogenesis 29, 1648–1654. doi:10.1093/carcin/bgn156
Levine, A. J. (2020). P53: 800 million years of evolution and 40 Years of discovery. Nat. Rev. Cancer 20, 471–480. doi:10.1038/s41568-020-0262-1
Li, K., Ma, X., Li, Z., Liu, Y., Shen, G., Luo, Z., et al. (2024). A natural peptide from a traditional Chinese Medicine has the potential to treat chronic atrophic gastritis by activating gastric stem cells. Adv. Sci. 11, 2304326. doi:10.1002/advs.202304326
Li, N., Feng, Y., Hu, Y., He, C., Xie, C., Ouyang, Y., et al. (2018). Helicobacter Pylori CagA promotes epithelial mesenchymal transition in gastric carcinogenesis via triggering oncogenic YAP Pathway. J. Exp. Clin. Cancer Res. 37, 280. doi:10.1186/s13046-018-0962-5
Li, N., Liu, S., Dong, K., Zhang, G., Huang, J., Wang, Z., et al. (2019). Exosome-transmitted miR-25 induced by H. pylori promotes vascular endothelial cell injury by targeting KLF2. Front. Cell. Infect. Microbiol. 9, 366. doi:10.3389/fcimb.2019.00366
Li, N., Xu, X., Yang, H., Wang, H., Ouyang, Y., Zhou, Y., et al. (2021). Activation of Aquaporin 5 by carcinogenic Helicobacter pylori infection promotes epithelial-mesenchymal transition via the MEK/ERK pathway. Helicobacter 26, e12842. doi:10.1111/hel.12842
Li, X., Liu, S., Luo, J., Liu, A., Tang, S., Liu, S., et al. (2015). Helicobacter pylori induces IL-1β and IL-18 production in human monocytic cell line through activation of NLRP3 inflammasome via ROS signaling pathway. Pathog. Dis. 73, ftu024. doi:10.1093/femspd/ftu024
Li, Y., Li, W., Wang, X., Ding, C., Liu, J., Li, Y., et al. (2020). High-salt diet-induced gastritis in C57BL/6 mice Is associated with microbial dysbiosis and alleviated by a buckwheat Diet. Mol. Nutr. Food Res. 64, 1900965. doi:10.1002/mnfr.201900965
Lin, L., Wei, H., Yi, J., Xie, B., Chen, J., Zhou, C., et al. (2019). Chronic CagA-positive Helicobacter Pylori infection with MNNG stimulation synergistically induces mesenchymal and cancer stem cell-like properties in gastric mucosal epithelial cells. J.Cell. Biochem. 120, 17635–17649. doi:10.1002/jcb.29031
Liou, J., Lee, Y., and Wu, M. (2020). Treatment of Helicobacter Pylori infection and its long-term impacts on gut microbiota. J.Gastroen. Hepatol. 35, 1107–1116. doi:10.1111/jgh.14992
Liu, D., Peng, J., Xie, J., and Xie, Y. (2023a). Comprehensive analysis of the function of Helicobacter-associated ferroptosis gene YWHAE in gastric cancer through multi-omics integration, molecular docking, and machine learning. Apoptosis 29, 439–456. doi:10.1007/s10495-023-01916-3
Liu, F., Gao, X., Li, Z., Zhang, X., Fan, H., Yu, G., et al. (2022). Protective effects of Scutellarin on acute alcohol intestinal injury. Chem. Biodivers. 19, e202100856. doi:10.1002/cbdv.202100856
Liu, J., Li, M., Chen, G., Yang, J., Jiang, Y., Li, F., et al. (2024). Jianwei Xiaoyan Granule ameliorates chronic atrophic gastritis by regulating HIF-1α-VEGF pathway. J. Ethnopharmacol. 334, 118591. doi:10.1016/j.jep.2024.118591
Liu, J., Xiao, Q., Xiao, J., Niu, C., Li, Y., Zhang, X., et al. (2022). Wnt/β-catenin signalling: function, biological mechanisms, and therapeutic opportunities. Sig. Transduct. Target. Ther. 7, 3. doi:10.1038/s41392-021-00762-6
Liu, M., Hu, Z., Wang, C., and Zhang, Y. (2023b). The TLR/MyD88 signalling cascade in inflammation and gastric cancer: the immune regulatory network of Helicobacter Pylori. J.Mol. Med. 101, 767–781. doi:10.1007/s00109-023-02332-5
Liu, M., Liu, Q., Zou, Q., Li, J., Chu, Z., Xiang, J., et al. (2023c). The composition and roles of gastric stem cells in epithelial homeostasis, regeneration, and tumorigenesis. Cell Oncol. 46, 867–883. doi:10.1007/s13402-023-00802-z
Liu, W. Z., Xie, Y., Lu, H., Cheng, H., Zeng, Z. R., Zhou, L. Y., et al. (2018). Chinese society of gastroenterology, Chinese study group on Helicobacter pylori and peptic ulcer. Fifth Chinese national consensus report on the management of Helicobacter pylori infection. Helicobacter 23, e12475. doi:10.1111/hel.12475
Liu, X., Cao, K., Xu, C., Hu, T., Zhou, L., Cao, D., et al. (2015). GATA-3 augmentation down-regulates Connexin43 in Helicobacter pylori associated gastric carcinogenesis. Cancer Biol. Ther. 16, 987–996. doi:10.1080/15384047.2015.1030552
Liu, X.-Y., Zhang, T.-Q., Zhang, Q., Guo, J., Zhang, P., Mao, T., et al. (2022). Differential long non-coding RNA expression analysis in chronic non-atrophic gastritis, gastric mucosal intraepithelial neoplasia, and gastric cancer tissues. Front. Genet. 13, 833857. doi:10.3389/fgene.2022.833857
Liu, Y., Xu, W., and Qin, X. (2019). Deciphering the mechanical network of chronic atrophic gastritis: a urinary time-dependent metabonomics-based network pharmacology study. Front. Physiol. 10, 1004. doi:10.3389/fphys.2019.01004
Liu, Y., Xu, W., Wang, G., and Qin, X. (2018). Material basis research for Huangqi Jianzhong Tang against chronic atrophic gastritis rats through integration of urinary metabonomics and SystemsDock. J. Ethnopharmacol. 223, 1–9. doi:10.1016/j.jep.2018.05.015
Liu, Y., Zhang, B., Zhou, Y., Xing, Y., Wang, Y., Jia, Y., et al. (2023d). Targeting Hippo pathway: a novel strategy for Helicobacter Pylori-induced gastric cancer treatment. Biomed. Pharmacother. 161, 114549. doi:10.1016/j.biopha.2023.114549
Lockshin, R. A., and Zakeri, Z. (2001). Programmed cell death and apoptosis: origins of the theory. Nat. Rev. Mol. Cell. Biol. 2, 545–550. doi:10.1038/35080097
Lu, S.-Y., Guo, S., Chai, S.-B., Yang, J.-Q., Yue, Y., Li, H., et al. (2021). Autophagy in gastric mucosa: the dual role and potential therapeutic target. Biomed. Res. Int. 2021, 2648065–2648068. doi:10.1155/2021/2648065
Lv, Y., Cheng, P., Zhang, J., Mao, F., Teng, Y., Liu, Y., et al. (2019). Helicobacter pylori –induced matrix metallopeptidase-10 promotes gastric bacterial colonization and gastritis. Sci. Adv. 5, eaau6547. doi:10.1126/sciadv.aau6547
Machado, A. M. D., Desler, C., Bøggild, S., Strickertsson, J. A. B., Friis-Hansen, L., Figueiredo, C., et al. (2013). Helicobacter Pylori infection affects mitochondrial function and DNA repair, thus, mediating geneticinstability in gastric cells. Mech. Ageing Dev. 134, 460–466. doi:10.1016/j.mad.2013.08.004
Manoel-Caetano, F. S., Rossi, A. F. T., Calvet De Morais, G., Severino, F. E., and Silva, A. E. (2019). Upregulation of the APE1 and H2AX genes and miRNAs involved in DNA damage response and repair in gastric cancer. Genes. Dis. 6, 176–184. doi:10.1016/j.gendis.2019.03.007
Massironi, S., Elvevi, A., Gallo, C., Laffusa, A., Tortorella, A., and Invernizzi, P. (2023). Exploring the spectrum of incidental gastric polyps in autoimmune gastritis. Digest.Liver Dis. 55, 1201–1207. doi:10.1016/j.dld.2023.02.008
Massironi, S., Gallo, C., Lahner, E., Sciola, V., Cavalcoli, F., Lenti, M. V., et al. (2024). Occurrence and characteristics of endoscopic gastric polyps in patients with autoimmune gastritis (AGAPE study): a multicentric cross-sectional study. Dig. Liver Dis., S1590865824008879. doi:10.1016/j.dld.2024.07.024
Massironi, S., Sciola, V., Spampatti, M.-P., Peracchi, M., and Conte, D. (2009). Gastric carcinoids: between underestimation and overtreatment. World J. Gastroenterol. 15, 2177–2183. doi:10.3748/wjg.15.2177
Massironi, S., Zilli, A., Elvevi, A., and Invernizzi, P. (2019). The changing face of chronic autoimmune atrophic gastritis: an updated comprehensive perspective. Autoimmun. Rev. 18, 215–222. doi:10.1016/j.autrev.2018.08.011
Maubach, G., Vieth, M., Boccellato, F., and Naumann, M. (2022). Helicobacter Pylori-induced NF-κB: trailblazer for gastric pathophysiology. Trends Mol. Med. 28, 210–222. doi:10.1016/j.molmed.2021.12.005
Messina, B., Lo Sardo, F., Scalera, S., Memeo, L., Colarossi, C., Mare, M., et al. (2023). Hippo pathway dysregulation in gastric cancer: from Helicobacter Pylori infection to tumor promotion and progression. Cell Death Dis. 14, 21. doi:10.1038/s41419-023-05568-8
Miceli, E., Lenti, M. V., Padula, D., Luinetti, O., Vattiato, C., Monti, C. M., et al. (2012). Common features of patients with autoimmune atrophic gastritis. Clin. Gastroenterol. H. 10, 812–814. doi:10.1016/j.cgh.2012.02.018
Mimuro, H., Suzuki, T., Nagai, S., Rieder, G., Suzuki, M., Nagai, T., et al. (2007). Helicobacter Pylori dampens gut epithelial self-renewal by inhibiting apoptosis, a bacterial strategy to enhance colonization of the stomach. Cell Host Microbe 2, 250–263. doi:10.1016/j.chom.2007.09.005
Minaga, K., Watanabe, T., Kamata, K., Asano, N., and Kudo, M. (2018). Nucleotide-binding oligomerization domain 1 and Helicobacter pylori infection: a review. WJG 24, 1725–1733. doi:10.3748/wjg.v24.i16.1725
Mizukami, Y., Kono, K., Kawaguchi, Y., Akaike, H., Kamimura, K., Sugai, H., et al. (2008). CCL17 and CCL22 chemokines within tumor microenvironment are related to accumulation of Foxp3 + regulatory T cells in gastric cancer. Int. J. Cancer 122, 2286–2293. doi:10.1002/ijc.23392
Mizushima, N., and Levine, B. (2020). Autophagy in human diseases. N. Engl. J. Med. 383, 1564–1576. doi:10.1056/NEJMra2022774
Molina-Castro, S. E., Tiffon, C., Giraud, J., Boeuf, H., Sifre, E., Giese, A., et al. (2020). The Hippo Kinase LATS2 controls Helicobacter Pylori-Induced epithelial-mesenchymal transition and intestinal metaplasia in gastric mucosa. Cell. Mol. gastroenter. 9, 257–276. doi:10.1016/j.jcmgh.2019.10.007
Moss, S. F., Calam, J., Agarwal, B., Wang, S., and Holt, P. R. (1996). Induction of gastric epithelial apoptosis by Helicobacter pylori. Gut 38, 498–501. doi:10.1136/gut.38.4.498
Mousavi, T., Hadizadeh, N., Nikfar, S., and Abdollahi, M. (2020). Drug discovery strategies for modulating oxidative stress in gastrointestinal disorders. Expert Opin. Drug Discov. 15, 1309–1341. doi:10.1080/17460441.2020.1791077
Murata-Kamiya, N., Kurashima, Y., Teishikata, Y., Yamahashi, Y., Saito, Y., Higashi, H., et al. (2007). Helicobacter pylori CagA interacts with E-cadherin and deregulates the beta-catenin signal that promotes intestinal transdifferentiation in gastric epithelial cells. Oncogene 26, 4617–4626. doi:10.1038/sj.onc.1210251
Murray, P. J., Allen, J. E., Biswas, S. K., Fisher, E. A., Gilroy, D. W., Goerdt, S., et al. (2014). Macrophage activation and polarization: nomenclature and experimental guidelines. Immunity 41, 14–20. doi:10.1016/j.immuni.2014.06.008
Nagasaki, N., Takigawa, H., Ito, M., Boda, T., Kotachi, T., Hayashi, R., et al. (2023). Diagnostic performance of the normal range of gastrin calculated using strict criteria based on a combination of serum markers and pathological evaluation for detecting gastritis: a retrospective study. BMC Gastroenterol. 23, 167. doi:10.1186/s12876-023-02816-1
Nagashima, H., Iwatani, S., Cruz, M., Jiménez Abreu, J. A., Uchida, T., Mahachai, V., et al. (2015). Toll-like receptor 10 in Helicobacter pylori infection. J. Infect. Dis. 212, 1666–1676. doi:10.1093/infdis/jiv270
Nagata, J., Kijima, H., Takagi, A., Ito, M., Goto, K., Yamazaki, H., et al. (2004). Helicobacter Pylori induces chronic active gastritis in P53-knockout mice. Int.J. Mo.l Med. doi:10.3892/ijmm.13.6.773
Nahon, S., Lahmek, P., Massard, J., Lesgourgues, B., De Serre, N. M., Traissac, L., et al. (2003). Helicobacter pylori -associated chronic gastritis and unexplained iron deficiency anemia: a reliable association? Helicobacter 8, 573–577. doi:10.1111/j.1523-5378.2003.00184.x
Nemati, M., Larussa, T., Khorramdelazad, H., Mahmoodi, M., and Jafarzadeh, A. (2017). Toll-like receptor 2: an important immunomodulatory molecule during Helicobacter pylori infection. Life Sci. 178, 17–29. doi:10.1016/j.lfs.2017.04.006
Nemeth, E., and Ganz, T. (2023). Hepcidin and iron in health and disease. Annu. Rev. Med. 74, 261–277. doi:10.1146/annurev-med-043021-032816
Newton, K., Strasser, A., Kayagaki, N., and Dixit, V. M. (2024). Cell death. Cell 187, 235–256. doi:10.1016/j.cell.2023.11.044
Ni, D., Ai, Z., Munoz-Sandoval, D., Suresh, R., Ellis, P. R., Yuqiong, C., et al. (2020). Inhibition of the facilitative sugar transporters (GLUTs) by tea extracts and catechins. FASEB J. 34, 9995–10010. doi:10.1096/fj.202000057RR
Nienhüser, H., Kim, W., Malagola, E., Ruan, T., Valenti, G., Middelhoff, M., et al. (2021). Mist1+ gastric isthmus stem cells are regulated by Wnt5a and expand in response to injury and inflammation in mice. Gut 70, 654–665. doi:10.1136/gutjnl-2020-320742
Nishiumi, S., Yoshida, M., and Azuma, T. (2017). Alterations in metabolic pathways in stomach of mice infected with Helicobacter Pylori. Microb. Pathog. 109, 78–85. doi:10.1016/j.micpath.2017.05.027
Nishiura, H., Iwamoto, S., Kido, M., Aoki, N., Maruoka, R., Ikeda, A., et al. (2013). Interleukin-21 and tumor necrosis factor-α are critical for the development of autoimmune gastritis in mice. J.Gastro. Hepatol. 28, 982–991. doi:10.1111/jgh.12144
Noto, C. N., Hoft, S. G., Bockerstett, K. A., Jackson, N. M., Ford, E. L., Vest, L. S., et al. (2022). IL13 acts directly on gastric epithelial cells to promote metaplasia development during chronic gastritis. Cell Mol. gastroenter. 13, 623–642. doi:10.1016/j.jcmgh.2021.09.012
Noto, J. M., and Peek, R. M. (2012). The role of microRNAs in Helicobacter pylori pathogenesis and gastric carcinogenesis. Front. Cell. Inf. Microbio. 1, 21. doi:10.3389/fcimb.2011.00021
Ohori, K., Yano, T., Katano, S., Nagaoka, R., Numazawa, R., Yamano, K., et al. (2023). Independent association between use of proton pump inhibitors and muscle wasting in patients with heart failure: a single-center, ambispective, observational study. Drugs Aging 40, 731–739. doi:10.1007/s40266-023-01035-3
Otani, K., Tanigawa, T., Watanabe, T., Nadatani, Y., Sogawa, M., Yamagami, H., et al. (2012). Toll-like receptor 9 signaling has anti-inflammatory effects on the early phase of Helicobacter pylori-induced gastritis. Biochem. Biophys. Res.Commun. 426, 342–349. doi:10.1016/j.bbrc.2012.08.080
Ozeki, K., Hada, K., and Wakiya, Y. (2024). Factors influencing the degree of gastric atrophy in Helicobacter pylori eradication patients with drinking habits. Microorganisms 12, 1398. doi:10.3390/microorganisms12071398
Pachathundikandi, S. K., Tegtmeyer, N., Arnold, I. C., Lind, J., Neddermann, M., Falkeis-Veits, C., et al. (2019). T4SS-dependent TLR5 activation by Helicobacter pylori infection. Nat. Commun. 10, 5717. doi:10.1038/s41467-019-13506-6
Pan, K.-F., Li, W.-Q., Zhang, L., Liu, W.-D., Ma, J.-L., Zhang, Y., et al. (2024). Gastric cancer prevention by community eradication of Helicobacter Pylori: a cluster-randomized controlled trial. Nat. Med. 30, 3250–3260. doi:10.1038/s41591-024-03153-w
Peng, Y., and Croce, C. M. (2016). The role of microRNAs in human cancer. Sig. Transduct. Target.Ther. 1, 15004. doi:10.1038/sigtrans.2015.4
Petkevicius, V., Streleckiene, G., Balciute, K., Link, A., Leja, M., Malfertheiner, P., et al. (2020). Association of long non-coding RNA polymorphisms with gastric cancer and atrophic gastritis. Genes 11, 1505. doi:10.3390/genes11121505
Piazuelo, M. B., Bravo, L. E., Mera, R. M., Camargo, M. C., Bravo, J. C., Delgado, A. G., et al. (2021). The Colombian chemoprevention trial: 20-Year follow-up of a cohort of patients with gastric precancerous lesions. Gastroenterology 160, 1106–1117.e3. doi:10.1053/j.gastro.2020.11.017
Pignatelli, B., Bancel, B., Estève, J., Malaveille, C., Calmels, S., Correa, P., et al. (1998). Inducible nitric oxide synthase, anti-oxidant enzymes and Helicobacter Pylori infection in gastritis and gastric precancerous lesions in humans. Eur. J. Cancer Prev. 7, 439–447. doi:10.1097/00008469-199812000-00003
Pignatelli, B., Bancel, B., Plummer, M., Toyokuni, S., Patricot, L.-M., and Ohshima, H. (2001). Helicobacter Pylori eradication attenuates oxidative stress in human gastric mucosa. Am. J. Gastroenterol. 96, 1758–1766. doi:10.1111/j.1572-0241.2001.03869.x
Pita, I., Libânio, D., Dias, F., Teixeira, A. L., Nogueira, I., Medeiros, R., et al. (2021). Original Article: MicroRNA dysregulation in the gastric carcinogenesis cascade: can we anticipate its role in individualized care? Pathobiology 88, 338–350. doi:10.1159/000515548
Pujol-Gimenez, J., De Heredia, F. P., Idoate, M. A., Airley, R., Lostao, M. P., and Evans, A. R. (2015). Could GLUT12 be a potential therapeutic target in cancer treatment? A preliminary report. J. Cancer 6, 139–143. doi:10.7150/jca.10429
Qu, X., and Shi, Y. (2022). Bile reflux and bile acids in the progression of gastric intestinal metaplasia. Chin. Med. J. 135, 1664–1672. doi:10.1097/CM9.0000000000002290
Reyes, M., Flores, T., Betancur, D., Peña-Oyarzún, D., and Torres, V. A. (2020). Wnt/β-Catenin signaling in oral carcinogenesis. Int. J. Mol. Sci. 21, 4682. doi:10.3390/ijms21134682
Santos, A., Lopes, T., Oleastro, M., Gato, I., Floch, P., Benejat, L., et al. (2015). Curcumin inhibits gastric inflammation induced by Helicobacter Pylori infection in a mouse model. Nutrients 7, 306–320. doi:10.3390/nu7010306
Săsăran, M. O., Meliț, L. E., and Dobru, E. D. (2021). MicroRNA modulation of host Immune response and inflammation triggered by Helicobacter pylori. Int. J. Mol. Sci. 22, 1406. doi:10.3390/ijms22031406
Schlößer, H. A., Drebber, U., Urbanski, A., Haase, S., Baltin, C., Berlth, F., et al. (2017). Glucose transporters 1, 3, 6, and 10 are expressed in gastric cancer and glucose transporter 3 is associated with UICC stage and survival. Gastric Cancer 20, 83–91. doi:10.1007/s10120-015-0577-x
Schmidinger, B., Petri, K., Lettl, C., Li, H., Namineni, S., Ishikawa-Ankerhold, H., et al. (2022). Helicobacter pylori binds human Annexins via Lipopolysaccharide to interfere with Toll-like Receptor 4 signaling. PLoS Pathog. 18, e1010326. doi:10.1371/journal.ppat.1010326
Schumacher, M. A., Donnelly, J. M., Engevik, A. C., Xiao, C., Yang, L., Kenny, S., et al. (2012). Gastric Sonic Hedgehog acts as a macrophage chemoattractant during the immune response to Helicobacter pylori. Gastroenterology 142, 1150–1159.e6. doi:10.1053/j.gastro.2012.01.029
Schwarz, P., Kübler, J. A. M., Strnad, P., Müller, K., Barth, T. F. E., Gerloff, A., et al. (2012). Hepcidin is localised in gastric parietal cells, regulates acid secretion and is induced by Helicobacter pylori infection. Gut 61, 193–201. doi:10.1136/gut.2011.241208
Seibt, T. M., Proneth, B., and Conrad, M. (2019). Role of GPX4 in ferroptosis and its pharmacological implication. Free Radic. Biol.Med. 133, 144–152. doi:10.1016/j.freeradbiomed.2018.09.014
Shah, S. C., Piazuelo, M. B., Kuipers, E. J., and Li, D. (2021). AGA clinicalpractice update on the diagnosis and management of atrophic gastritis: expert review. Gastroenterology 161, 1325–1332.e7. doi:10.1053/j.gastro.2021.06.078
Shi, Y., Wei, Y., Zhang, T., Zhang, J., Wang, Y., and Ding, S. (2016). Deoxycholic acid could induce apoptosis and trigger gastric carcinogenesis on gastric epithelial cells by quantitative proteomic analysis. Gastroent. Res. Pract. 2016, 9638963. doi:10.1155/2016/9638963
Shibata, W., Takaishi, S., Muthupalani, S., Pritchard, D. M., Whary, M. T., Rogers, A. B., et al. (2010). Conditional deletion of IκB-Kinase-β accelerates Helicobacter-dependent gastric apoptosis, proliferation, and preneoplasia. Gastroenterology 138, 1022–1034.e10. doi:10.1053/j.gastro.2009.11.054
Shiotani, A., Iishi, H., Uedo, N., Ishiguro, S., Tatsuta, M., Nakae, Y., et al. (2005). Evidence that loss of Sonic Hedgehog is an indicator of Helicobater Pylori-induced atrophic gastritis progressing to gastric cancer. Am. J. Gastroenterol. 100, 581–587. doi:10.1111/j.1572-0241.2005.41001.x
Shiotani, A., Iishi, H., Uedo, N., Ishihara, R., Ishiguro, S., Tatsuta, M., et al. (2006). Helicobacter Pylori -induced atrophic gastritis progressing to gastric cancer exhibits Sonic Hedgehog loss and aberrant CDX2 expression. Aliment. .Pharmacol. Ther. 24, 71–80. doi:10.1111/j.1365-2036.2006.00028.x
Shu, F., Xiao, H., Li, Q.-N., Ren, X.-S., Liu, Z.-G., Hu, B.-W., et al. (2023). Epigenetic and post-translational modifications in autophagy: biological functions and therapeutic targets. Sig. Transduct. Target. Ther. 8, 32. doi:10.1038/s41392-022-01300-8
Si, Y.-T., Xiong, X.-S., Wang, J.-T., Yuan, Q., Li, Y.-T., Tang, J.-W., et al. (2024). Identification of chronic non-atrophic gastritis and intestinal metaplasia stages in the Correa's cascade through machine learning analyses of SERS spectral signature of non-invasively-collected human gastric fluid samples. Biosens. Bioelectron. 262, 116530. doi:10.1016/j.bios.2024.116530
Silva-García, O., Valdez-Alarcón, J. J., and Baizabal-Aguirre, V. M. (2019). Wnt/β-Catenin signaling as a molecular target by pathogenic bacteria. Front. Immunol. 10, 2135. doi:10.3389/fimmu.2019.02135
Sivanand, S., and Vander Heiden, M. G. (2020). Emerging roles for branched-chain amino acid metabolism in cancer. Cancer Cell 37, 147–156. doi:10.1016/j.ccell.2019.12.011
Smith, J. P., Nadella, S., and Osborne, N. (2017). Gastrin and gastric cancer. Cell. Mol. gastroenter. 4, 75–83. doi:10.1016/j.jcmgh.2017.03.004
Sohn, S.-H., Kim, N., Jo, H. J., Kim, J., Park, J. H., Nam, R. H., et al. (2017). Analysis of gastric body microbiota by pyrosequencing: possible role of bacteria other than Helicobacter Pylori in the gastric carcinogenesis. J. Cancer Prev. 22, 115–125. doi:10.15430/JCP.2017.22.2.115
Sokolova, O., Vieth, M., Gnad, T., Bozko, P. M., and Naumann, M. (2014). Helicobacter Pylori promotes Eukaryotic Protein translation by activating phosphatidylinositol 3 Kinase/mTOR. Int. J. Biochem. Cell Biol. 55, 157–163. doi:10.1016/j.biocel.2014.08.023
Song, H., Zhou, L., Liu, D., Ge, L., and Li, Y. (2019). Probiotic effect on Helicobacter pylori attachment and inhibition of inflammation in human gastric epithelial cells. Exp. Ther. Med. 18, 1551–1562. doi:10.3892/etm.2019.7742
Song, J. H., Kim, Y. S., Heo, N. J., Lim, J. H., Yang, S. Y., Chung, G. E., et al. (2017). High salt intake is associated with atrophic gastritis with intestinal metaplasia. Cancer. epidem. biomar. 26, 1133–1138. doi:10.1158/1055-9965.EPI-16-1024
Song, P., Li, X., Chen, S., Gong, Y., Zhao, J., Jiao, Y., et al. (2024). YTHDF1 mediates N -METHYL- N -nitrosourea-induced gastric carcinogenesis by controlling HSPH1 translation. Cell Prolif. 57, e13619. doi:10.1111/cpr.13619
Spike, B. T., and Wahl, G. M. (2011). p53, stem cells, and reprogramming: tumor suppression beyond guarding the genome. Gene. Cancer. 2, 404–419. doi:10.1177/1947601911410224
Stewart, O. A., Wu, F., and Chen, Y. (2020). The role of gastric microbiota in gastric cancer. Gut Microbes 11, 1220–1230. doi:10.1080/19490976.2020.1762520
Sun, J., Wang, S., Zhao, Z., Lu, J., Zhang, Y., An, W., et al. (2024). Oxymatrine attenuates ulcerative colitis through inhibiting pyroptosis mediated by the NLRP3 inflammasome. Molecules 29, 2897. doi:10.3390/molecules29122897
Sung, J. J. Y., Coker, O. O., Chu, E., Szeto, C. H., Luk, S. T. Y., Lau, H. C. H., et al. (2020). Gastric microbes associated with gastric inflammation, atrophy and intestinal metaplasia 1 year after Helicobacter pylori eradication. Gut 69, 1572–1580. doi:10.1136/gutjnl-2019-319826
Tabassam, F. H., Graham, D. Y., and Yamaoka, Y. (2012). Helicobacter pylori -associated regulation of forkhead transcription factors FoxO1/3a in human gastric cells. Helicobacter 17, 193–202. doi:10.1111/j.1523-5378.2012.00939.x
Taguchi, A., Ohmiya, N., Itoh, A., Hirooka, Y., Niwa, Y., Mori, N., et al. (2006). Severity of atrophic gastritis related to antiparietal cell antibody and gastric carcinogenesis, including P53 mutations. J. Gastroen. Hepatol. 21, 545–551. doi:10.1111/j.1440-1746.2005.03983.x
Tan, F., Li, X., Wang, Z., Li, J., Shahzad, K., and Zheng, J. (2024). Clinical applications of stem cell-derived exosomes. Sig. Transduct. Target .Ther. 9, 17. doi:10.1038/s41392-023-01704-0
Tan, S. H., Swathi, Y., Tan, S., Goh, J., Seishima, R., Murakami, K., et al. (2020). AQP5 enriches for stem cells and cancer origins in the distal stomach. Nature 578, 437–443. doi:10.1038/s41586-020-1973-x
Tang, K., McLeod, L., Livis, T., West, A. C., Dawson, R., Yu, L., et al. (2022). Toll-like Receptor 9 promotes initiation of gastric tumorigenesis by augmenting inflammation and cellular proliferation. Cell. Mol. Gastroenter 14, 567–586. doi:10.1016/j.jcmgh.2022.06.002
Tang, L., Tang, B., Lei, Y., Yang, M., Wang, S., Hu, S., et al. (2021). Helicobacter pylori-induced heparanase promotes H. pylori colonization and gastritis. Front. Immunol. 12, 675747. doi:10.3389/fimmu.2021.675747
Teng, Y., Liu, X., Han, B., Ma, Q., Liu, Y., Kong, H., et al. (2019). Helicobacter Pylori -downregulated Tumor Necrosis Factor Receptor-associated Protein 1 mediates apoptosis of human gastric epithelial cells. J. Cell. Physiol. 234, 15698–15707. doi:10.1002/jcp.28223
Thrift, A. P., Wenker, T. N., and El-Serag, H. B. (2023). Global burden of gastric cancer: epidemiological trends, risk factors, screening and prevention. Nat. Rev. Clin. Oncol. 20, 338–349. doi:10.1038/s41571-023-00747-0
Thumann, T. A., Pferschy-Wenzig, E.-M., Moissl-Eichinger, C., and Bauer, R. (2019). The role of gut microbiota for the activity of medicinal plants traditionally used in the European Union for gastrointestinal disorders. J. Ethnopharmacol. 245, 112153. doi:10.1016/j.jep.2019.112153
Tiffon, C., Giraud, J., Molina-Castro, S. E., Peru, S., Seeneevassen, L., Sifré, E., et al. (2020). TAZ Controls Helicobacter pylori-induced epithelial–mesenchymal transition and cancer stem cell-Like invasive and tumorigenic properties. Cells 9, 1462. doi:10.3390/cells9061462
Tricker, A. R., Ditrich, C., and Preussmann, R. (1991). N -Nitroso compounds in cigarette tobacco and their occurrence in mainstream tobacco smoke. Carcinogenesis 12, 257–261. doi:10.1093/carcin/12.2.257
Tsai, H.-F., and Hsu, P.-N. (2017). Modulation of tumor necrosis factor-related apoptosis-inducing ligand (TRAIL)-mediated apoptosis by Helicobacter pylori in immune pathogenesis of gastric mucosal damage. J. Microbiol.Immunol 50, 4–9. doi:10.1016/j.jmii.2016.01.002
Tshibangu-Kabamba, E., and Yamaoka, Y. (2021). Helicobacter Pylori infection and antibiotic resistance — from biology to clinical implications. Nat. Rev. Gastroenterol. Hepatol. 18, 613–629. doi:10.1038/s41575-021-00449-x
Tu, E., Ang, D. K. Y., Bellingham, S. A., Hogan, T. V., Teng, M. W. L., Smyth, M. J., et al. (2012). Both IFN -γ and IL -17 are required for the development of severe autoimmune gastritis. Eur. J. Immunol. 42, 2574–2583. doi:10.1002/eji.201142341
Tu, W., Hong, Y., Huang, M., Chen, M., and Gan, H. (2022). Effect of Kaempferol on Hedgehog Signaling pathway in rats with --chronic atrophic gastritis – based on network pharmacological screening and experimental verification. Biomed. Pharmacother. 145, 112451. doi:10.1016/j.biopha.2021.112451
Turtoi, D. C., Brata, V. D., Incze, V., Ismaiel, A., Dumitrascu, D. I., Militaru, V., et al. (2024). Artificial intelligence for theautomatic diagnosis of gastritis: a systematic review. JCM 13, 4818. doi:10.3390/jcm13164818
Van Grieken, N. C. T., Meijer, G. A., zur Hausen, A., Meuwissen, S. G. M., Baak, J. P. A., and Kuipers, E. J. (2003). Increased apoptosis in gastric mucosa adjacent to intestinal metaplasia. J. Clin. Pathol. 56, 358–361. doi:10.1136/jcp.56.5.358
Vences-Mejía, A., Caballero-Ortega, H., Dorado-González, V., Gamboa-Domínguez, A., Gómez-Ruiz, C., Camacho-Carranza, R., et al. (2005). Cytochrome P450 expression in rat Gastric epithelium with intestinal metaplasia induced by high dietary NaCl levels. Environ. Toxicol. Pharmacol. 20, 57–64. doi:10.1016/j.etap.2004.10.010
Waghray, M., Zavros, Y., Saqui–Salces, M., El–Zaatari, M., Alamelumangapuram, C. B., Todisco, A., et al. (2010). Interleukin-1beta promotes gastric atrophy through suppression of Sonic Hedgehog. Gastroenterology 138, 562–572.e5722. doi:10.1053/j.gastro.2009.10.043
Wang, C.-Y., Shahi, P., Huang, J. T. W., Phan, N. N., Sun, Z., Lin, Y.-C., et al. (2017). Systematic analysis of the achaete-scute complex-like gene signature in clinical cancer patients. Mol. Clin. Oncol. 6, 7–18. doi:10.3892/mco.2016.1094
Wang, H., Guo, M., Wei, H., and Chen, Y. (2023). Targeting p53 pathways: mechanisms, structures, and advances in therapy. Sig. Transduct. Target. Ther. 8, 92. doi:10.1038/s41392-023-01347-1
Wang, Q., Huang, Y., Jiang, M., Tang, Y., Wang, Q., Bai, L., et al. (2024). The demethylase ALKBH5 mediates ZKSCAN3 expression through the m6A modification to activate VEGFA transcription and thus participates in MNNG-induced gastric cancer progression. J. Hazard. Mater. 473, 134690. doi:10.1016/j.jhazmat.2024.134690
Wang, S., and El-Deiry, W. S. (2003). TRAIL and apoptosis induction by TNF-family death receptors. Oncogene 22, 8628–8633. doi:10.1038/sj.onc.1207232
Wang, S., Kuang, J., Zhang, H., Chen, W., Zheng, X., Wang, J., et al. (2022). Bile Acid–microbiome interaction promotes gastric carcinogenesis. Adv. Sci. 9, 2200263. doi:10.1002/advs.202200263
Wang, Y., Chiang, W., Kuo, F., Wu, M., Shih, H., Wang, S. S. W., et al. (2018). Levels of malondialdehyde in the gastric juice: its association with Helicobacter pylori infection and stomach diseases. Helicobacter 23, e12460. doi:10.1111/hel.12460
Wang, Z., Zhou, X., Hu, X., and Zheng, C. (2024). Quercetin ameliorates Helicobacter pylori -induced gastric epithelial cell injury by regulating specificity Protein 1/Lipocalin 2 axis in Gastritis. J. Appl.Toxicol. 44, 641–650. doi:10.1002/jat.4566
Wei, J., Nagy, T. A., Vilgelm, A., Zaika, E., Ogden, S. R., Romero–Gallo, J., et al. (2010). Regulation of p53 tumor suppressor by Helicobacter pylori in gastric epithelial cells. Gastroenterology 139, 1333–1343. doi:10.1053/j.gastro.2010.06.018
Wei, Y.-F., Xie, S.-A., and Zhang, S.-T. (2024). Current research on the interaction between Helicobacter Pylori and macrophages. Mol. Biol. Rep. 51, 497. doi:10.1007/s11033-024-09395-8
Wen, J., Chen, C., Luo, M., Liu, X., Guo, J., Wei, T., et al. (2021). Notch signaling ligand Jagged1 enhances macrophage-mediated response to Helicobacter pylori. Front. Microbiol. 12, 692832. doi:10.3389/fmicb.2021.692832
Wen, J.-X., Tong, Y., Ma, X., Wang, R., Li, R., Song, H., et al. (2021). Therapeutic effects and potential mechanism of dehydroevodiamine on N-methyl-N′-nitro-N-nitrosoguanidine-induced chronic atrophic gastritis. Phytomedicine 91, 153619. doi:10.1016/j.phymed.2021.153619
Wessler, S., Krisch, L. M., Elmer, D. P., and Aberger, F. (2017). From inflammation to gastric cancer – the importance of Hedgehog/GLI signaling in Helicobacter Pylori-induced chronic inflammatory and neoplastic diseases. Cell Commun. Signal 15, 15. doi:10.1186/s12964-017-0171-4
White, E. (2016). Autophagy and P53. Cold Spring Harb. Perspect. Med. 6, a026120. doi:10.1101/cshperspect.a026120
White, P. J., and Newgard, C. B. (2019). Branched-chain amino acids in disease. Science 363, 582–583. doi:10.1126/science.aav0558
Wizenty, J., Müllerke, S., Kolesnichenko, M., Heuberger, J., Lin, M., Fischer, A., et al. (2022). Gastric stem cells promote inflammation and gland remodeling in response to Helicobacter pylori via Rspo3-Lgr4 axis. EMBO J. 41, e109996. doi:10.15252/embj.2021109996
Wroblewski, L. E., Choi, E., Petersen, C., Delgado, A. G., Piazuelo, M. B., Romero-Gallo, J., et al. (2019). Targeted mobilization of Lrig1 + gastric epithelial stem cell populations by a carcinogenic Helicobacter pylori type IV secretion system. Proc. Natl. Acad. Sci. U.S.A. 116, 19652–19658. doi:10.1073/pnas.1903798116
Wu, Y., Shen, L., Liang, X., Li, S., Ma, L., Zheng, L., et al. (2019). Helicobacter pylori-induced YAP1 nuclear translocation promotes gastric carcinogenesis by enhancing IL-1β expression. Cancer Med. 8, 3965–3980. doi:10.1002/cam4.2318
Xiao, S., and Zhou, L. (2020). Gastric stem cells: physiological and pathological perspectives. Front. Cell Dev. Biol. 8, 571536. doi:10.3389/fcell.2020.571536
Xie, C., Li, N., Wang, H., He, C., Hu, Y., Peng, C., et al. (2020). Inhibition of autophagy aggravates DNA damage response and gastric tumorigenesis via Rad51 ubiquitination in response to H. pylori infection. Gut Microbes 11, 1567–1589. doi:10.1080/19490976.2020.1774311
Xie, Y., and Liu, L. (2018). Analysis of correlation between HP infection and activation of PI3K/Akt pathway in mucosal tissues of gastric cancer and precancerous lesions. Oncol. Lett. 16, 5615–5620. doi:10.3892/ol.2018.9329
Xu, B., Kong, J., Lin, Y., Tang, Z., Liu, J., Chen, Z., et al. (2023). Anti- Helicobacter pylori activity and gastroprotective effects of human stomach-derived Lactobacillus paragasseri strain LPG-9. Food Funct. 14, 10882–10895. doi:10.1039/D3FO03562J
Xu, H., Ruan, L.-Y., Chen, C., Fan, J.-T., Chen, J.-F., Zhao, W.-L., et al. (2022). Therapeutic assessment of fractions of Gastrodiae Rhizoma on chronic atrophic gastritis by 1H NMR-Based metabolomics. J. Ethnopharmacol. 254, 112403. doi:10.1016/j.jep.2019.112403
Yamaguchi, N., Sakaguchi, T., Isomoto, H., Inamine, T., Ueda, H., Fukuda, D., et al. (2023). ATG16L1 and ATG12 gene polymorphisms are involved in the progression of atrophic gastritis. JCM 12, 5384. doi:10.3390/jcm12165384
Yamaguchi, N., Sakaguchi, T., Taira, M., Fukuda, D., Ohnita, K., Hirayama, T., et al. (2024). Autophagy-related gene ATG7 polymorphism could potentially serve as a biomarker of the progression of atrophic gastritis. JCM 13, 629. doi:10.3390/jcm13020629
Yamaguchi, T., Nakajima, N., Kuwayama, H., Ito, Y., Iwasaki, A., and Arakawa, Y. (2000). Gastric epithelial cell proliferation and apoptosis in Helicobacter Pylori-infected mice. Aliment. Pharmacol. Ther. 1, 68–73. doi:10.1046/j.1365-2036.2000.014s1068.x
Yan, H., Zou, T., Tuo, Q., Xu, S., Li, H., Belaidi, A. A., et al. (2021). Ferroptosis: mechanisms and links with diseases. Sig. Transduct.Target. Ther. 6, 49. doi:10.1038/s41392-020-00428-9
Yanaka, A., Fahey, J. W., Fukumoto, A., Nakayama, M., Inoue, S., Zhang, S., et al. (2009). Dietary sulforaphane-rich broccoli sprouts reduce colonization and attenuate gastritis in Helicobacter Pylori –infected mice and humans. Cancer Prev. Res. 2, 353–360. doi:10.1158/1940-6207.CAPR-08-0192
Yang, C., Scheibenbogen, C., Bauer, S., Kleinle, C., Wex, T., Bornschein, J., et al. (2013). A rrequent Toll-Like Receptor 1 gene polymorphism affects NK - and T-cell IFN -γ production and is associated with Helicobacter pylori -induced gastric disease. Helicobacter 18, 13–21. doi:10.1111/hel.12001
Yang, J.-C., and Chien, C.-T. (2009). A new approach for the prevention and treatment of Helicobacter pylori infection via upregulation of autophagy and downregulation of apoptosis. Autophagy 5, 413–414. doi:10.4161/auto.5.3.7826
Yao, Y., Li, G., Wu, J., Zhang, X., and Wang, J. (2015). Inflammatory response of macrophages cultured with Helicobacter pylori strains was regulated by miR-155. Int. J. Clin. Exp. Pathol. 8, 4545–4554.
Yepuri, G., Sukhovershin, R., Nazari-Shafti, T. Z., Petrascheck, M., Ghebre, Y. T., and Cooke, J. P. (2016). Proton pump inhibitors accelerate endothelial senescence. Circ. Res. 118, e36–e42. doi:10.1161/CIRCRESAHA.116.308807
Yu, B., De Vos, D., Guo, X., Peng, S., Xie, W., Peppelenbosch, M. P., et al. (2024). IL-6 facilitates cross-talk between epithelial cells and tumor-associated macrophages in Helicobacter pylori-linked gastric carcinogenesis. Neoplasia 50, 100981. doi:10.1016/j.neo.2024.100981
Yu, C., Su, Z., Li, Y., Li, Y., Liu, K., Chu, F., et al. (2020). Dysbiosis of gut microbiota is associated with gastric carcinogenesis in Rats. Biomed. Pharmacother. 126, 110036. doi:10.1016/j.biopha.2020.110036
Yu, F., Yu, C., Li, F., Zuo, Y., Wang, Y., Yao, L., et al. (2021). Wnt/β-catenin signaling in cancers and targeted therapies. Sig. Transduct. Target .Ther. 6, 307. doi:10.1038/s41392-021-00701-5
Yu, G., Torres, J., Hu, N., Medrano-Guzman, R., Herrera-Goepfert, R., Humphrys, M. S., et al. (2017). Molecular characterization of the human stomach microbiota in gastric cancer patients. Front. Cell. Infect. Microbiol. 7, 302. doi:10.3389/fcimb.2017.00302
Yu, H., Lin, L., Zhang, Z., Zhang, H., and Hu, H. (2020). Targeting NF-κB pathway for the therapy of diseases: mechanism and clinical study. Sig. Transduct. Target Ther. 5, 209. doi:10.1038/s41392-020-00312-6
Yu, J., Chen, Z., Zhou, Q., Li, P., Wu, S., Zhou, T., et al. (2024). Exopolysaccharide from Lacticaseibacillus paracasei alleviates gastritis in Helicobacter pylori-infected mice by regulating gastric microbiota. Front. Nutr. 11, 1426358. doi:10.3389/fnut.2024.1426358
Yu, L., Li, R., Liu, W., Zhou, Y., Li, Y., Qin, Y., et al. (2020). Protective effects of wheat peptides against ethanol-induced gastric mucosal lesions in rats: vasodilation and anti-inflammation. Nutrients 12, 2355. doi:10.3390/nu12082355
Yu, P., Zhang, X., Liu, N., Tang, L., Peng, C., and Chen, X. (2021). Pyroptosis: mechanisms and diseases. Sig. Transduct. Target. Ther. 6, 128. doi:10.1038/s41392-021-00507-5
Yuan, L.-Z., Shi, X., Tang, D., Zheng, S.-P., Xiao, Z.-M., and Wang, F. (2021). Construction and preservation of a stable and highly expressed recombinant Helicobacter Pylori vacuolating cytotoxin A with apoptotic activity. BMC Microbiol. 21, 229. doi:10.1186/s12866-021-02262-7
Zamperone, A., Cohen, D., Stein, M., Viard, C., and Müsch, A. (2019). Inhibition of polarity-regulating kinase PAR1b contributes to Helicobacter pylori inflicted DNA Double Strand Breaks in gastric cells. Cell Cycle 18, 299–311. doi:10.1080/15384101.2018.1560121
Zeng, Z., Nian, Q., Chen, N., Zhao, M., Zheng, Q., Zhang, G., et al. (2022). Ginsenoside Rg3 inhibits angiogenesis in gastric precancerous lesions through downregulation of Glut1 and Glut4. Biomed. Pharmacother. 145, 112086. doi:10.1016/j.biopha.2021.112086
Zhang, J., Sayakoummane, S., Kim, S. A., Lee, J. S., Choung, E. S., Kim, E. S., et al. (2022). Hymenocallis littoralis ameliorates inflammatory responses in LPS-stimulated RAW264.7 cells and HCl/EtOH-induced gastric mucosal injury via targeting the MAPK pathway. J. Ethnopharmacol. 295, 115400. doi:10.1016/j.jep.2022.115400
Zhang, L., Hu, W., Cho, C. H., Chan, F. K., Yu, J., Fitzgerald, J. R., et al. (2018). Reduced lysosomal clearance of autophagosomes promotes survival and colonization of Helicobacter Pylori. J. Pathol. 244, 432–444. doi:10.1002/path.5033
Zhang, L. Y., Zhang, J., Li, D., Liu, Y., Zhang, D. L., Liu, C. F., et al. (2021). Bile reflux is an independent risk factor for precancerous gastric lesions and gastric cancer: an observational cross-sectional study. J.Digest. Dis. 22, 282–290. doi:10.1111/1751-2980.12986
Zhang, X., He, Y., Zhang, X., Fu, B., Song, Z., Wang, L., et al. (2024). Sustained exposure to Helicobacter pylori induces immune tolerance by desensitizing TLR6. Gastric Cancer 27, 324–342. doi:10.1007/s10120-023-01461-7
Zhang, X., Li, C., Chen, D., He, X., Zhao, Y., Bao, L., et al. (2022). H. pylori CagA activates the NLRP3 inflammasome to promote gastric cancer cell migration and invasion. Inflamm. Res. 71, 141–155. doi:10.1007/s00011-021-01522-6
Zhang, Z., Liu, J., Wang, Y., Zhang, L., Zhou, T., Huang, Y., et al. (2024). Toll-like receptor 4 signaling mediates gastritis and gastric cancer. CMM 24. doi:10.2174/0115665240276139231206071742
Zhao, H., Yan, P., Zhang, N., Feng, L., Chu, X., Cui, G., et al. (2021). The recurrence rate of Helicobacter pylori in recent 10 years: a systematic review and meta-analysis. Helicobacter 26, e12852. doi:10.1111/hel.12852
Zhao, Q., Jia, Q., and Chi, T. (2022). Deep learning as a novel method for endoscopic diagnosis of chronic atrophic gastritis: a prospective nested case–Control study. BMC Gastroenterol. 22, 352. doi:10.1186/s12876-022-02427-2
Zhao, T., and Yu, Z. (2024). Modified Gexia-Zhuyu Tang inhibits gastric cancer progression by restoring gut microbiota and regulating pyroptosis. Cancer Cell Int. 24, 21. doi:10.1186/s12935-024-03215-6
Zhao, X., Wu, M., Zhang, D., Sun, Y., Yang, Y., Xie, H., et al. (2018). The relationship of interpersonal sensitivity and depression among patients with chronic atrophic gastritis: the mediating role of coping styles. J. Clin. Nurs. 27, e984–e991. doi:10.1111/jocn.14114
Zhao, Y., Zhao, J., Ma, H., Han, Y., Xu, W., Wang, J., et al. (2023). High hepcidin levels promote abnormal iron metabolism and ferroptosis in chronic atrophic gastritis. Biomedicines 11, 2338. doi:10.3390/biomedicines11092338
Zheng, J., Jiang, X., Jiang, K., Yan, Y., Pan, J., Liu, F., et al. (2023). MiR-196a-5p correlates with chronic atrophic gastritis progression to gastric cancer and induces malignant biological behaviors of gastric cancer cells by targeting ACER2. Mol. Biotechnol. 65, 1306–1317. doi:10.1007/s12033-022-00589-8
Zhou, B., Lin, W., Long, Y., Yang, Y., Zhang, H., Wu, K., et al. (2022). Notch signaling pathway: architecture, disease, and therapeutics. Sig. Transduct. Target.Ther. 7, 95. doi:10.1038/s41392-022-00934-y
Zhou, H., Ni, Z., Li, T., Su, L., Zhang, L., Liu, N., et al. (2018). Activation of FXR promotes intestinal metaplasia of gastric cells via SHP-dependent upregulation of the expression of CDX2. Oncol. Lett. doi, 7617–7624. doi:10.3892/ol.2018.8342
Zhou, J., Qiu, J., Song, Y., Liang, T., Liu, S., Ren, C., et al. (2023). Pyroptosis and degenerative diseases of the elderly. Cell Death Dis. 14, 94. doi:10.1038/s41419-023-05634-1
Zhou, Q., Meng, Y., Li, D., Yao, L., Le, J., Liu, Y., et al. (2024). Ferroptosis in cancer: from molecular mechanisms to therapeutic strategies. Sig. Transduct. Target. Ther. 9, 55. doi:10.1038/s41392-024-01769-5
Zhou, Z., Hu, C., Cui, B., You, L., An, R., Liang, K., et al. (2024). Ginsenoside Rg1 suppresses proptosis via the NF-κB/NLRP3/GSDMD pathway to alleviate chronic atrophic gastritis in vitro and in vivo. J. Agric. Food Chem. 72, 13668–13683. doi:10.1021/acs.jafc.4c01271
Zhu, P., Xue, J., Zhang, Z., Jia, Y., Tong, Y., Han, D., et al. (2017). Helicobacter Pylori VacA induces autophagic cell death in gastric epithelial cells via the endoplasmic reticulum stress pathway. Cell Death Dis. 8, 3207. doi:10.1038/s41419-017-0011-x
Zhu, W., Liu, D., Lu, Y., Sun, J., Zhu, J., Xing, Y., et al. (2023). PHKG2 regulates RSL3-induced ferroptosis in Helicobacter Pylori related gastric Cancer. Arch. Biochem. Biophys. 740, 109560. doi:10.1016/j.abb.2023.109560
Zhu, Y., Ma, R., Cheng, W., Qin, M., Guo, W., Qi, Y., et al. (2024). Sijunzi Decoction ameliorates gastric precancerous lesions via regulating oxidative phosphorylation based on proteomics and metabolomics. J. Ethnopharmacol. 318, 116925. doi:10.1016/j.jep.2023.116925
Zou, T. H., Gao, Q. Y., Liu, S. D., Li, Y. Q., Meng, X. J., Zhang, G. X., et al. (2024). Effectiveness and safety of Moluodan in the treatment of precancerous lesions of gastric cancer: a randomized clinical trial. J. Dig. Dis. 25, 27–35. doi:10.1111/1751-2980.13251
Keywords: chronic atrophic gastritis, pathogenetic mechanisms, gastric precancerous lesions, biomarkers, therapeutic targets
Citation: Kuang W, Xu J, Xu F, Huang W, Majid M, Shi H, Yuan X, Ruan Y and Hu X (2024) Current study of pathogenetic mechanisms and therapeutics of chronic atrophic gastritis: a comprehensive review. Front. Cell Dev. Biol. 12:1513426. doi: 10.3389/fcell.2024.1513426
Received: 18 October 2024; Accepted: 25 November 2024;
Published: 10 December 2024.
Edited by:
Tadahiro Nagaoka, Fujita Health University, JapanReviewed by:
Sara Massironi, Vita-Salute San Raffaele University, ItalyKayoko Ozeki, Aichi Gakuin University, Japan
Copyright © 2024 Kuang, Xu, Xu, Huang, Majid, Shi, Yuan, Ruan and Hu. This is an open-access article distributed under the terms of the Creative Commons Attribution License (CC BY). The use, distribution or reproduction in other forums is permitted, provided the original author(s) and the copyright owner(s) are credited and that the original publication in this journal is cited, in accordance with accepted academic practice. No use, distribution or reproduction is permitted which does not comply with these terms.
*Correspondence: Xia Yuan, eXVhbnhpYV96bG5rQDE2My5jb20=; Yongdui Ruan, UnVhbnlkMjAyNEAxNjMuY29t; Xianjing Hu, aHV4ajIwMDNAMTYzLmNvbQ==
†These authors have contributed equally to this work