- 1Physiology Research Center, Iran University of Medical Sciences, Tehran, Iran
- 2Student Research Committee, USERN Office, Lorestan University of Medical Sciences, Khorramabad, Iran
- 3Department of Chemistry, Iran University of Science and Technology, Tehran, Iran
- 4Master of Health Education, Research Expert, Clinical Research Development Unit, Emam Khomeini Hospital, Ilam University of Medical Sciences, Ilam, Iran
- 5Faculty of Medicine, Tehran Medical Sciences Branch, Islamic Azad University, Tehran, Iran
- 6Cellular and Molecular Research Center, Qom University of Medical Sciences, Qom, Iran
- 7Nervous System Stem Cells Research Center, Semnan University of Medical Sciences, Semnan, Iran
- 8Department of Medical Microbiology, Faculty of Medicine, Shahed University, Tehran, Iran
Mortality and morbidity from cardiovascular diseases are common worldwide. In order to improve survival and quality of life for this patient population, extensive efforts are being made to establish effective therapeutic modalities. New treatment options are needed, it seems. In addition to treating cardiovascular diseases, cell therapy is one of the most promising medical platforms. One of the most effective therapeutic approaches in this area is stem cell therapy. In stem cell biology, multipotent stem cells and pluripotent stem cells are divided into two types. There is evidence that stem cell therapy could be used as a therapeutic approach for cardiovascular diseases based on multiple lines of evidence. The effectiveness of stem cell therapies in humans has been studied in several clinical trials. In spite of the challenges associated with stem cell therapy, it appears that resolving them may lead to stem cells being used in cardiovascular disease patients. This may be an effective therapeutic approach. By mounting these stem cells on biological scaffolds, their effect can be enhanced.
Introduction
Despite great progress in medical research over the past few decades, cardiovascular diseases (CVDs) remain the primary cause of mortality and morbidity globally, imposing a substantial burden on global health. Approximately 30% of worldwide mortality is attributed to cardiovascular diseases (CVDs), rendering them one of the most urgent public health concerns of our era. Projections from the World Health Organization (WHO) and the American Heart Association indicate that by 2030, the number of deaths caused by cardiovascular diseases would exceed 23 million per year. The presented data highlights the increasing severity of the problem and emphasizes the pressing requirement for creative approaches to decrease the occurrence and consequences of cardiovascular disease (Di Cesare et al., 2024; Correction to: heart disease and stroke statistics—2023 update: a report from the American heart association, 2023; Xu et al., 2022).
In addition to becoming a health concern, the increasing prevalence of cardiovascular illnesses imposes a significant economic cost. The expenses related to the treatment of cardiovascular diseases (CVDs) are immense, amounting to billions of dollars each year on healthcare services, hospital stays, drugs, and long-term care. As populations age and the prevalence of risk factors such as obesity, diabetes, and hypertension increases, the economic burden is anticipated to deteriorate. Furthermore, the financial strain is exacerbated by the indirect expenses linked to decreased production and the extended care needed for patients who survive myocardial infarctions, cerebrovascular accidents, or suffer from chronic heart failure (Brown et al., 2020). Based on these considerations, the advancement of treatment alternatives that are more economical, effective, and less intrusive has become a crucial focus for healthcare systems globally (Csöbönyeiová et al., 2022; Ge et al., 2023). Heart failure, a medical disorder characterized by a significant impairment in the heart’s capacity to efficiently circulate blood, is a primary factor contributing to hospitalization and mortality in individuals with cardiovascular disease (CVD). Although pharmaceutical medicines have made significant progress and mechanical support devices like ventricular assist devices (VADs) have been developed, heart transplantation is still the only definitive therapy for people suffering from end-stage heart failure (Fadini et al., 2020). Yet, heart transplantation is riddled with obstacles, such as a critical scarcity of donor organs, the requirement for lifelong immunosuppression, and the possibility of transplant rejection. The aforementioned constraints emphasize the necessity for alternative therapeutic approaches that can effectively target the root causes of heart failure and provide enduring advantages without the consequent hazards of transplantation (Haba et al., 2021; Feng et al., 2024).
Recent years have seen the emergence of stem cell therapy as a promising method for treating cardiac illness, including heart failure. Stem cells inherently possess the distinctive capacity to undergo differentiation into many cell types, including cardiomyocytes, the cells accountable for the contractile activity of the heart. Two kinds of stem cells now under investigation for cardiac treatment, resident cardiac stem cells (CSCs) and induced pluripotent stem cells (iPSCs), have demonstrated significant promise. cardiac stem cells (CSCs), located in the heart, has the capacity to undergo differentiation into several types of cardiogenic cells, therefore presenting a promising opportunity for the regeneration of impaired cardiac tissue. Likewise, induced pluripotent stem cells (iPSCs), produced by reprogramming mature cells to exhibit pluripotency, may be manipulated to differentiate into cardiac cells, offering a flexible and individualized therapeutic alternative (Golpanian et al., 2016; Hu and Feng, 2017; Huang et al., 2024; Farokhi et al., 2024). Numerous clinical trials have investigated different sources of stem cells, such as skeletal myoblasts, bone marrow mononuclear cells (BMMNCs), and more recently, induced pluripotent stem cells (iPSCs), to assess their capacity to restore and regenerate cardiac tissue. Experimental studies have demonstrated that stem cells have the potential to boost cardiac function, diminish scar tissue, and facilitate the general recuperation of individuals afflicted with heart illness. However, the therapeutic use of stem cells in the treatment of cardiovascular disorders is still in its nascent phase, and various obstacles persist (Xu et al., 2002). One of the primary challenges is to determine the optimal stem cell type and delivery technique to obtain reliable and enduring outcomes. Furthermore, there are apprehensions regarding the possible hazards linked to stem cell treatment, such as the development of malignancies or undesired immunological reactions, which must be duly tackled by meticulous clinical experimentation (Gallina et al., 2015; Simon et al., 2008).
Furthermore, although stem cell treatment has significant potential, it is not devoid of its constraints. One example is the restricted availability of very desirable stem cell characteristics, especially in the context of adult-derived stem cells, which often decrease in quantity and effectiveness as they age. Recent studies have demonstrated that a mere 1%–2% of cardiac c-kit + cells exhibit the requisite multifunctional capability for efficient cardiac repair, rendering them scarce and difficult to separate. Moreover, research has shown that the levels of cardiac progenitor cells (CPCs) in humans decline considerably beyond the age of two, therefore restricting their potential for therapeutic applications in later stages of life (Fathi et al., 2020; Yu et al., 2022). The reduction associated with aging poses a substantial challenge in using these cells for the treatment of cardiac diseases in elderly people, who are the most likely to get benefits from such treatments (Guo et al., 2020; Trzyna and Banaś-Ząbczyk, 2021; Zhao Y. et al., 2021a). This study explores the growing significance of bio-scaffolds in combination with stem cells for the management of cardiovascular disorders, providing novel perspectives on how these technologies might be employed to improve therapeutic results. Biological scaffolds offer a supporting structure that can direct the development, specialization, and incorporation of stem cells into injured cardiac tissue, therefore enhancing the effectiveness of stem cell-based treatments. Through the emulation of the extracellular matrix of the heart, these scaffolds have the ability to establish a more advantageous milieu for tissue regeneration, therefore facilitating enhanced integration of the transplanted cells and ultimately enhancing the total structural and functional restoration of the heart. Continued progress in research in this field suggests that the integration of stem cells and bio-scaffolds has the potential to completely transform the management of heart failure and other cardiovascular disorders, offering fresh optimism for patients with few treatment alternatives (Figure 1).
Types of cells
During reimplantation, autologous cells are obtained from the same individual (Simeon et al., 2021). The least susceptible to pathogen rejection and transmission are autologous cells, however these are occasionally unavailable. For instance, hereditary illness lacks adequate autologous cells (Ma et al., 2017). Moreover, patients with severe burns, the older, or those who are severely ill may not have sufficient autologous cells to create the cell lines (Eschenhagen and Carrier, 2019). Concerns exist over the necessity of such procedures due to the fact that certain cell types must be removed from the patient and could result in persistent discomfort or donor site infection (Palà et al., 2020). Before usage, autologous cells must also be cultivated from specimens: Autologous solutions could not be particularly quick because this could take some time (Moghaddam et al., 2019). The use of bone marrow and adipocyte mesenchymal stem cells has lately become popular. These cells can develop into many tissues, such as bone, cartilage, fat, and nerve. Fat may be swiftly and readily used to separate many cells, opening up the possibility of producing plenty of cells in a hurry (Henning, 2021; Andalib et al., 2023).
Allogeneic cells are those that originate from a single donor. It has been shown that the use of dermal fibroblasts from human skin is a risk-free and, as a result, acceptable choice for the engineering of skin tissue. This is despite the fact that there are ethical restrictions placed on the utilization of human cells in the context of laboratory research (Xia et al., 2020).
These xenogeneic cells cannot be compared to those of any other species. Particularly animal cells have just seen extensive application in research efforts directed towards the development of cardiovascular implants (Moghaddam et al., 2019).
Syngeneic cells, also known as isogenic cells, are those that have been extracted from genetically identical creatures like twins, clones, or carefully researched animal models (Csöbönyeiová et al., 2022).
There is just one creature that has primary cells. There is only 1 cell present in each of the secondary cells (Eschenhagen and Carrier, 2019). The choice of stem cell source and differentiation for therapeutic purposes is determined by the specific medical condition being targeted. Multiple types of stem cells, such as embryonic, adult, and induced pluripotent stem cells, have been extensively studied for their potential in therapy. Every type has its own set of benefits and constraints (Thanaskody et al., 2022).
Best source for stem cells
Embryonic Stem Cells (ESCs): These stem cells are derived from embryos and have the ability to develop into different cell types. These cells possess the remarkable ability to transform into various cell types within the body, which greatly enhances their significance in the field of regenerative medicine. Nevertheless, their utilization raises ethical concerns Adult stem cells are a remarkable type of cells that exist in various parts of the body. They possess the incredible ability to divide and transform into different cell types, allowing them to replace dying cells and repair injured tissues (Vazin and Freed, 2010). They are widely accepted and have been utilized in a range of therapies, including bone marrow transplants. Induced Pluripotent Stem Cells (iPSCs) are adult cells that have undergone reprogramming to acquire a state similar to embryonic stem cells. They have the potential to be tailored to individual patients, which can help minimize the chances of immune rejection. However, additional research is needed to ensure the safety and effectiveness of their clinical use (Chang et al., 2019).
Maximizing differentiation
The differentiation of stem cells into specific cell types is essential for their therapeutic efficacy. As an illustration, in the treatment of neurodegenerative diseases, it is desirable to differentiate stem cells into neurons or glial cells (Mustafa et al., 2023). On the other hand, in the context of heart disease, the focus is on differentiating them into cardiac muscle cells. When selecting a stem cell source and differentiation method, it is crucial to consider their demonstrated effectiveness for the specific condition being targeted (Augustine et al., 2021).
Ensuring safety is of utmost importance when evaluating the stem cell type and the differentiation process, as it helps to minimize any potential side effects.
Consideration should be given to the ethical and legal regulations regarding the use of various stem cell types (Fadini et al., 2020; Rigato and Fadini, 2018; Mahjoor et al., 2021) (Figure 2). In the table below, the types of stem cells and their different characteristics are listed in full (Table 1).
Stem cells for tissue engineering
In selecting an appropriate biological cellular material, also known as scaffolding, one of the most critical aspects to focus on is choosing the cell type that is most ideal for tissue engineering. (SCs), also known as a source of cells capable of developing into cardiac muscle/cardiomyocytes (CMCs), smooth muscle cells (SMCs), and endothelial cells (ECs), have been shown to be able to repair heart tissue. They play an important part in the study of TE due to the qualities that have been listed (Wang et al., 2021).
Embryonic stem cell
Embryonic stem cells, often known as ESCs, are one of the cellular sources that are applied in techniques involving TE. Internal cell mass of the preimplantation blastocyst is the source of embryonic stem cells (ESCs) (Nasser et al., 2020). They are capable of differentiating into a variety of three-layer germ cells of varying kinds. The use of human embryonic stem cells (hESC) may be advantageous in the field of tissue engineering for the heart. HESC-derived cardiomyocytes (hESC-CMC) demonstrated extremely good characteristics in a research carried out by Landry et al. These phenotypes included myofibril alignment, density, morphology, contractile performance, and gene expression profiles. Nevertheless, only laboratory cultures were validated after days in vitro (Lundy et al., 2013). According to the findings given in a research by Henning (2021), several experiments have been carried out to validate the effective differentiation of embryonic stem cells (ESCs) into cardiomyocytes. The researchers Andalib et al. (2023) investigated how the native cardiac ECM might influence the differentiation of hESC. After the extraction of cardiac ECM from the digested pig hearts, the cardiac ECM was combined with collagen and used to generate hydrogels for use in cell culture. When compared with HESCs grown on less ECM hydrogels, HESCs cultured on biomass material consisting of 75% ECC of native goats and 25% hydrogels with added growth factors did not demonstrate adequate differentiation with cardiac T and troponin expression and contractile function. Native. ESCs have been shown to be a viable treatment option for cardiac TE, according to a number of studies (Xiao et al., 2021). In addition, some factors such as ethical concerns, immune stimulation and the risk of tumorigenesis make ESCs a very inconsistent choice of cell source for TE (Nugraha et al., 2019) (Table 2).
Mesenchymal stem cells
Bone marrow, adipose tissue, tonsil tissue, and mesenchymal stem cells (MSCs) are some of the tissues from which mesenchymal stem cells can be isolated (Liu et al., 2022; Mahjoor et al., 2023a; Mahjoor et al., 2023b; Fakouri et al., 2024). Under normal culture conditions, MSCs have plastic adherent properties and show characteristics similar to fibroblasts in terms of their morphology. As well as CD73, CD90, and CD105, there are specific cell surface markers expressed on them. In addition to self-renewing cells, MSCs also can differentiate into a wide range of mesodermal lineages, including adipocytes, muscles, chondrocytes, and osteoblasts (Mazini et al., 2020; Mirshekar et al., 2023). Furthermore, there is growing evidence that MSCs possess both immune-modulatory functions and pro-antigenic activity, which are beneficial for tissue regeneration, in addition to their differentiation potential (Jiang and Xu, 2020; Mahmoudvand et al., 2023). Through the secretion of various immune-modulatory cytokines, MSCs impair dendritic cell and T-cell function in addition to generating an immunosuppressive environment at the local level (Fu et al., 2019; Mahjoor et al., 2023b). As a matter of fact, MSCs have been shown to promote angiogenesis by secreting factors that promote angiogenesis (Andrzejewska et al., 2019). The use of MSCs in treating a wide range of human diseases has been investigated through clinical trials worldwide. These include cardiovascular diseases, bone and cartilage diseases, neurological disorders, and inflammatory disorders (Yu et al., 2017). In the field of cell therapy, there are a number of MSC-based products available on the market, although their therapeutic efficacy is still under debate (Brown et al., 2020).
Induced pluripotent stem cells
SCs that have been induced to become pluripotent are another kind employed in TE (iPSCs). Induced pluripotent stem cells (iPSCs) are somatic cells that have been reprogrammed to act and display features similar to embryonic stem cells (ESCs). Differentiation of iPSCs into the three germ layers is possible (Takahashi et al., 2007). In order to address the four critical elements of OCT4, SOX2, c-Myc, and KFL-4, the group led by Takahashi was the first to employ viral vectors to reprogram somatic adult cells like fibroblasts. The Cell Kind Known as Fibroblasts (Zaehres and Schöler, 2007). In spite of the fact that induced pluripotent stem cells (also known as iPSCs) have a great lot of promise, the therapeutic use of these cells is contingent on the discovery of methods that will reduce the likelihood that these cells would give rise to tumors (Ishida et al., 2020). It has been demonstrated that the genes Oct4, Sox2, Klf4, c-Myc, and Nanog play an important part in preserving the stemness of the cells that are produced by the process of induced pluripotent stem cells (iPSCs). As a consequence of this, there is a significant correlation between the existence of these genes and the development of teratomas from iPSCs (Chen et al., 2021). Cells derived from this P19 teratocarcinoma cell line have properties that are comparable to those observed in iPSCs. Moreover, these cells are capable of developing into a wide variety of cell types (Ebrahimi et al., 2020). We individually knocked down Oct4, Sox2, KLF4, c-Myc, and Nanog expression in P19 cells and analyzed their impact in vivo on gene expression (Karagiannis et al., 2019). Teratomas containing mesodermal tissue were the most common kind seen in immunosuppressed animals whose Oct4, Sox2, and KLF4 genes were activated (Kim et al., 2021). With this technique, fibroblasts may be reprogrammed into embryonic-like cells and then differentiated into a similar cell type. Heart muscle cells grown from human induced pluripotent stem cells (hiPSCs) (hiPSC-CMCs) have been found to share key properties with their hESC-derived counterparts after prolonged in vitro incubation (Lundy et al., 2013). Restoring the decellularized heart of a cadaver mouse with multifunctional ancestors obtained from hiPSC was proposed by Lu et al. (2013). They also demonstrate the expansion, differentiation, and myogenesis of cardiac ECM from hiPSC-derived cells (Yan et al., 2021). They used an electrocardiogram that induced arrhythmia to investigate the repopulated heart’s capability for normal rhythmic activity. Significant responses have been detected when studying the impact of drugs on the retransmitted heart. In the context of diagnostics and pharmaceutical research, this framework has been investigated as an alternate to individualized medicine (Gorshkov et al., 2019). Because of the wide variety of CHD cases, it is important to create individual patient patterns to better understand how each patient responds to current pharmacological therapy (Henning, 2021). It may even be impossible to generate genuine cardiac patterns that exhibit each patient’s unique clinical manifestations of the condition (Smith et al., 2015). Nevertheless, iPSC has showed cancer, much as ESC (Wang et al., 2022). Using the three key factors Gata4, Mef2c, and Tbx5, Ieda and colleagues showed that fibroblasts may be transformed into functioning cardiomyocytes in a short amount of time, and that this direct reprogramming can lower the chance of cancer (Brown et al., 2020). The use of viral vectors in reprogramming techniques, however, is very difficult and has a number of dangers (Bian et al., 2022). In order to identify safer and more successful alternatives to this rescheduling procedure, researchers have utilized and investigated a variety of iPSC production methods (Simeon et al., 2021). iPSCs do not cause an immunological response, and the harvest of cells from patients who are in critical condition is not expected to result in iPSCs, therefore they may be the safest cellular source for TE. In addition, there is less ethical debate around the use of iPSCs than there is about the use of fetal ESCs or SCs (Cruz-Samperio et al., 2021) (Table 3).
Prenatal, perinatal and postnatal stem cells
In addition to (CV-MSC) SCs and amniotic fluid-derived SCs (AFSCs), stem cell-producing cells from prenatal, perinatal, and postnatal stages are employed in TE. Blood from the umbilical cord is known to contain multifunctional microorganisms thanks to its endothelial lining (Eschenhagen and Carrier, 2019). Endothelial progenitor cells (EPCs) are one type of UCB progenitor that stands out in terms of their proliferation potential (Zhao et al., 2020). For prenatally diagnosed kids with congenital heart disease, this type of SC is very useful since it may be utilized as a child’s individual SC for the heart. This strategy is useful for preventing immunogenicity or for eliminating autologous SCs from a newborn or young kid by other means. It has also been demonstrated that, unlike ESCs and iPSCs, AFSCs do not result in the formation of teratomas (Palà et al., 2020). All groups of these cells have been studied with remarkable results in engineered valves and vascular grafts (Banerjee et al., 2018). This type of SC is not applicable for adults who are later diagnosed with CHD (Pomatto et al., 2021) (Table 4).
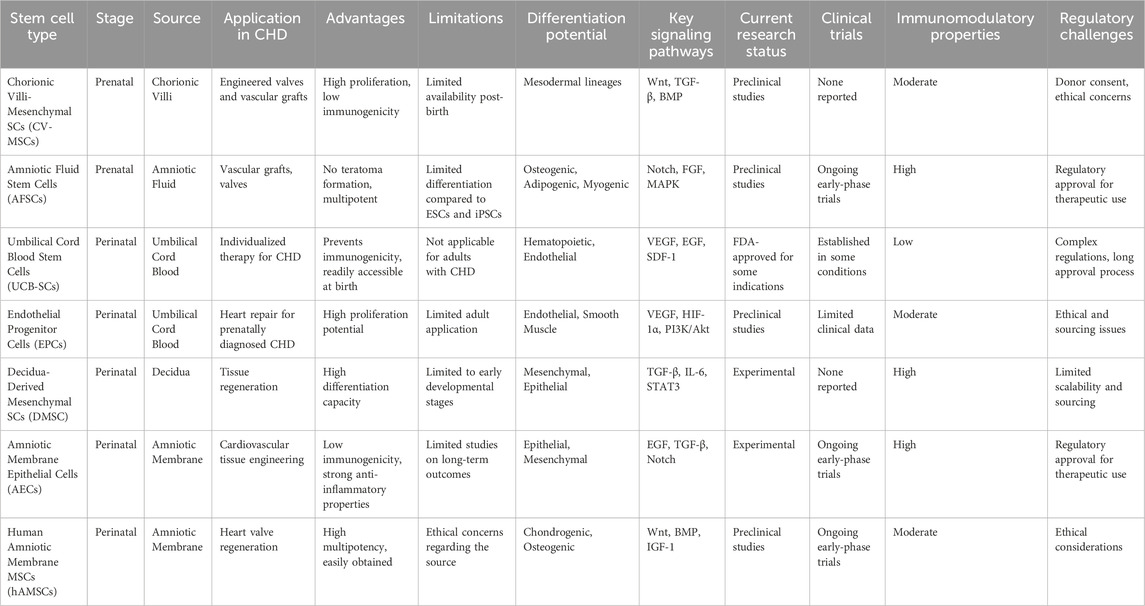
Table 4. Comparative analysis of various stem cell types and their applications in congenital heart disease (CHD). The table highlights the source, stage, and differentiation potential of each stem cell type, along with their advantages, limitations, key signaling pathways, current research status, clinical trial involvement, immunomodulatory properties, and regulatory challenges associated with their use in CHD therapies.
Here is a further expanded table with additional columns based on the most recent data from PubMed.
Adult stem cells
Bone marrow derived stem cells
EPCs are not only found in cord blood, but also in the peripheral blood of adults (PB-EPCs) and in bone marrow (BM-EPCs) (Trzyna and Banaś-Ząbczyk, 2021). Yet, in contrast to peripheral blood, bone marrow is a far more abundant source of blood nerve cells. CD34+ mononuclear hematopoietic cells were isolated from peripheral blood and shown to have endothelial characteristics in vitro by Asahara. Endothelial progenitor cells generated from bone marrow have shown great promise in the treatment of patients with ischemic and vascular TE (De Bartolo et al., 2013; Schmitt et al., 2004). Complete endothelial re-engineering of canine cerebral arteries utilising peripheral blood endothelial progenitor cells has been documented in animal research. Tissue-engineered pluripotent stem cells (TE PSCs) are another important component of the tissue-engine (Hao et al., 2014). Complete endothelial re-engineering of canine cerebral arteries utilising peripheral blood endothelial progenitor cells has been documented in animal research. Tissue-engineered pluripotent stem cells (TE PSCs) are another important component of the tissue-engine (Ye and Zhang, 2017). Using bone marrow-derived stem cells, PB-EPCs have been used in a manner analogous to that of prenatal EPCs in vascular transplantation during congenital heart surgery (Oliveira et al., 2017). Mirensky and his colleagues utilized non-woven PGA laminate as a scaffold to generate vascular grafts in conjunction with human bone marrow mononuclear cells (BM-MNCs) (BM-MNCs) (Fukunishi et al., 2018). No aneurysms or thrombotic events were reported, which is a very encouraging finding given the lack of anticoagulants. Six weeks after transplant implantation, the team concludes that the transplant has been completely accommodated by the host cells, and they propose using this technique as a vascular treatment for CHD (Yoshida and Yamanaka, 2017). No aneurysms or thrombotic events were reported, which is a very encouraging finding given the lack of anticoagulants. Six weeks after transplant implantation, the team concludes that the transplant has been completely accommodated by the host cells, and they propose using this technique as a vascular treatment for CHD (Tsilimigras et al., 2017). Similar results have been reported by more research with the same conclusion (Chang et al., 2018). In addition, a research including 25 young patients under the age of 30 who had whole-heart cardiopulmonary transplantation with engineered vascular BM-MNCs yielded encouraging outcomes. Long-term patient follow-up did not reveal zero death related to transplantation, thromboembolic problems, hemorrhoids, or infection; nevertheless, six of the patients developed effectively managed graft stenosis (Mittal et al., 2018). Positive outcomes with BM-MNC are promising, while BM-MSC provides even greater advantages. Advantages include, for instance, the ability to develop into many cell types, including progenitor cells (Zhang et al., 2018). They can be collected, isolated, stored, and replicated with little effort. displaying a behavior pattern typical of valve cells. Antithrombogenic characteristics; and their immunity can be managed. Decellularized pig scaffolds were treated by in vivo injection of BM-MNCs and BM-MSC before transplantation in an animal model, and the short- and long-term properties of these scaffolds were compared and contrasted (Ahmed et al., 2021). There was no discernible improvement in the short term. The transverse and distal gradients were significantly lower, the inflammatory response was greater, the structural degradation was greater, there was calcification and a thick fibrous wound around the suture line in the BM-MNCs group after 4 months of follow-up. When comparing BM-MNCs and BM-MSCs, these differences were striking (Ahmed and Al-Massri, 2021) (Table 5).
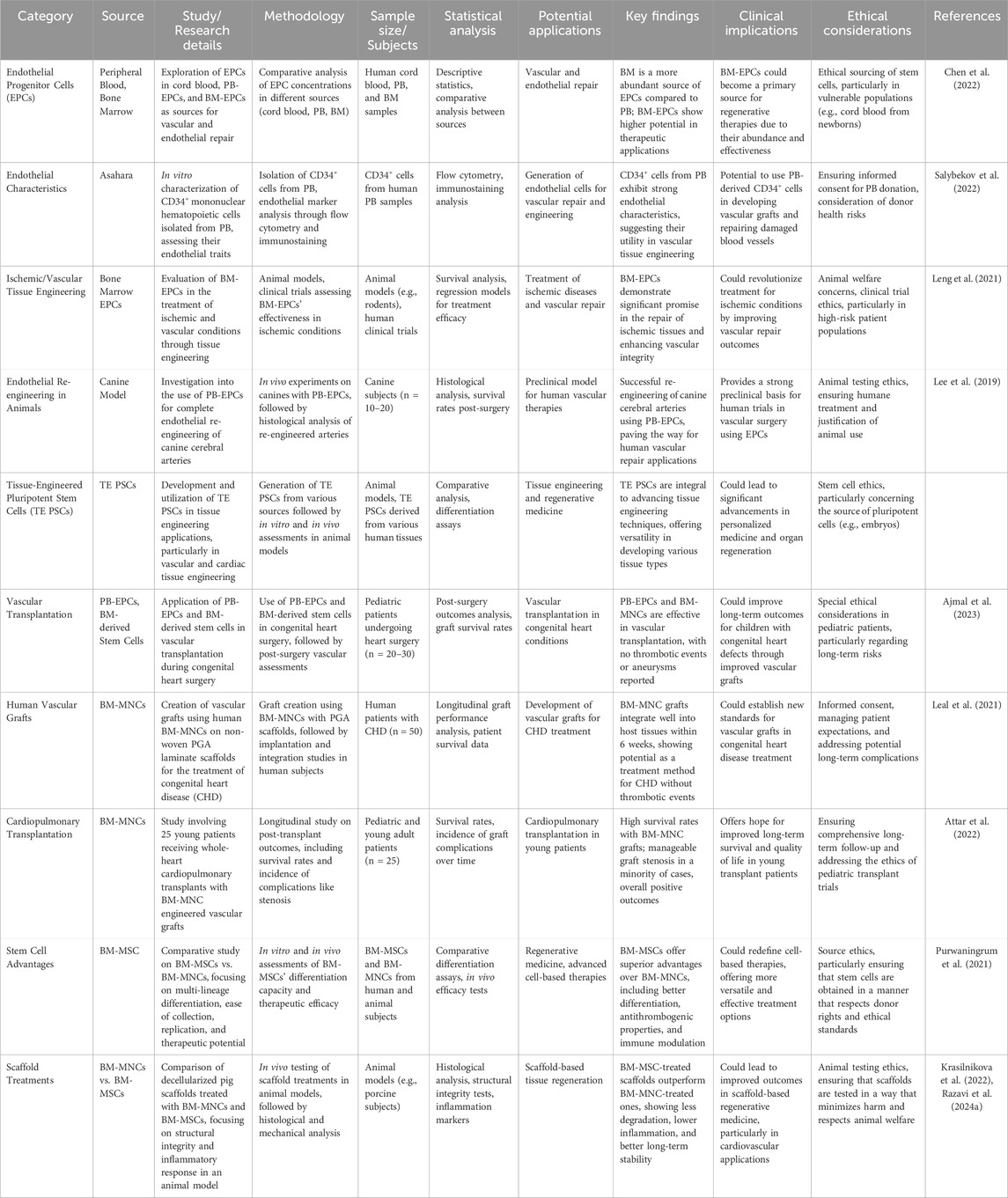
Table 5. Overview of studies on endothelial progenitor cells (EPCs) and tissue-engineered pluripotent stem cells (TE PSCs) in vascular and cardiac applications. The table highlights key research details, methodologies, findings, clinical implications, and ethical considerations, emphasizing their roles in regenerative medicine and congenital heart disease (CHD) treatment.
Cardiac progenitor cells
Stem cell receptor kinase + is a blood marker for cardiac progenitor cells (CPCs), also recognized as cardiac stem cells (CSCs), which are a specific type of cell present in the adult heart (Eschenhagen and Carrier, 2019; Yoshida and Yamanaka, 2017; Shouman et al., 2021) Beltrami et al., who first demonstrated CPC’s repeatable and multipotent features, deserve much of the credit for its short existence (15 years). Differentiation into cardiomyocytes, smooth muscle cells, and endothelial cells; all three kinds of cardiogenic cells (Castaldo and Chimenti, 2018) In more recent years, research led by Vincenza et al. has demonstrated that only a tiny percentage of adult stem cells exhibit congenital tissue-specific features, facilitating c-kit + cardiac cell divisions (Yeung et al., 2019; Abou-Saleh et al., 2018) Acute myocardial infarction (MI) was created in adult Wistar rats, and the infarcted boundary was injected with GFP + c-kit + for one group, CDF-c-kit-GFP-expressing GFP CSC (CSCGFP) was injected into PBS for a second group, and a placebo group received PBS injections. Research here revealed that CSCGFP did more than only minimize apoptosis and cardiomyocyte hypertrophy; it also reduced scar and enhanced ventricular function (Mahmoudvand et al., 2023; Khan et al., 2022). According to the results, only approximately 10% of CD45-c-kit + c cells are cardiovascular, and only about 10% are clonogenic and multiple. This suggests that only a small percentage of cardiac C+ cells, about 1%–2%, actually possess the highly desirable CSC phenotype that allows them to perform several functions (Bianconi et al., 2018). It has also been demonstrated that CP-C + CD45− (delete CD31, CD34) cells display low levels of Sca-1, Abcg2, CD105, CD166, PDGFR-, Flk-1, ROR2, CD13, and CD90 (Rigato and Fadini, 2018). While there are far more CPCs in newborns, that number drops considerably after the age of 2 (Müller et al., 2018). Consequently, as CPCs may be harvested during palliative surgery or during endomycopath biopsy, these cells, in conjunction with suitable scaffolds, can be a response to the therapy of a variety of CHDs (Csöbönyeiová et al., 2022; Yu et al., 2017; Molaei et al., 2022) (Table 6).
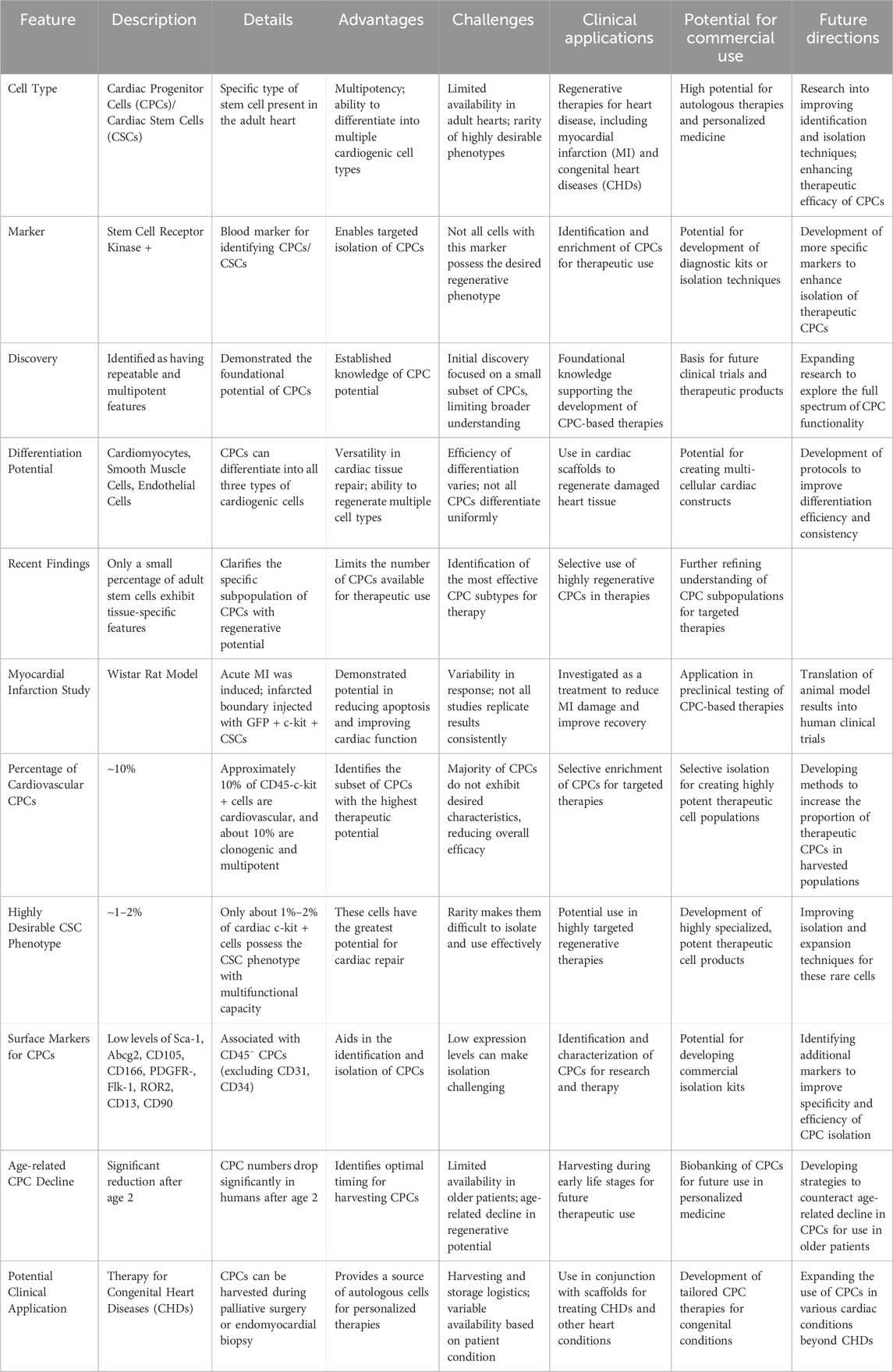
Table 6. Summary of key features, advantages, challenges, and clinical applications of cardiac progenitor cells (CPCs) and cardiac stem cells (CSCs). The table highlights their differentiation potential, recent findings, and the potential for commercial use, along with future directions for improving their therapeutic efficacy and isolation techniques.
Bio-scaffold in cardiac disorders
Bio-scaffolds have emerged as a promising avenue in the treatment and management of cardiac disorders, offering innovative approaches to heart tissue regeneration and repair. These scaffolds, often designed to mimic the extracellular matrix (ECM) of the heart, play a crucial role in promoting cell adhesion, proliferation, and differentiation. The complexity and multifunctionality of cardiac tissue require that these scaffolds not only support cellular functions but also replicate the mechanical and electrical properties of the myocardium. Recent studies highlight the advancements and potential of various bio-scaffold technologies in addressing cardiac diseases, especially following myocardial infarction (MI), where the loss of viable myocardial tissue poses a significant challenge (Zielińska et al., 2023). One of the pivotal advancements in this field is the development of electrospun ECM-based scaffolds. These scaffolds are created from decellularized porcine cardiac ECM using electrospinning technology, which preserves the composition, microstructure, and mechanical properties of the original cardiac ECM. This approach has demonstrated considerable success in supporting cell growth and function, both in vitro and in vivo, showcasing its potential for broader biomedical applications in cardiac tissue engineering. By retaining key cardiac mechanical properties, these scaffolds offer a reproducible, scalable, and controllable platform for heart regeneration efforts (Gokce et al., 2022). In another study, acellular cardiac ECM scaffolds have been shown to possess desirable mechanical properties such as elasticity, strength, and durability, which are essential for cardiac tissue repair. These scaffolds support the proliferation and viability of various cell types, including cardiomyocytes and mesenchymal stem cells (MSCs). Notably, cardiomyocyte-seeded scaffolds exhibited synchronous beating, an indication of functional tissue integration, thus reinforcing the potential use of acellular ECM in cardiac tissue engineering. The ability of these scaffolds to support functional cardiac markers like alpha-actinin and connexin43 further underscores their therapeutic potential (Zhao X. et al., 2021b; Otaghvar et al., 2022).
Moreover, the integration of conductive materials into bio-scaffolds has been explored to enhance the electrical properties of the engineered cardiac tissues. For instance, silk-polypyrrole composite scaffolds have been developed to mimic the native ECM’s nanotopography while also improving electrical conductivity. These scaffolds have been shown to promote cellular organization and enhance the expression of critical cardiac proteins, such as connexin43, which is vital for cell-cell electrical coupling. This combination of biomimetic topography and electroconductivity significantly improves the contractile and electrophysiological function of the cultured cardiomyocytes, making it a promising strategy for cardiac tissue engineering (Wang et al., 2023; Taheripak et al., 2024). A novel approach involves the use of shape-memory scaffolds that can be delivered minimally invasively. These scaffolds, designed from biodegradable polymers, are capable of recovering their original shape after injection, which is crucial for their application in minimally invasive surgeries. The successful delivery and integration of these scaffolds into the heart tissue have shown promising results in improving cardiac function post-MI, as demonstrated in animal models. Such innovations highlight the potential for minimally invasive cardiac repair techniques, which could significantly reduce the recovery time and complications associated with traditional surgical methods.
Additionally, the development of dual bioactive embedded nanofibrous scaffolds has shown promise in regenerating damaged myocardial tissue. These scaffolds, fabricated using coaxial electrospinning, are designed to mimic the topographical and chemical cues of the natural cardiac ECM. The sustained delivery of bioactive signals from these scaffolds has been shown to improve cell adhesion, proliferation, and differentiation, leading to enhanced myocardial repair and regeneration. The success of these scaffolds in inducing angiogenesis and maintaining cell viability post-transplantation further solidifies their role in cardiac therapeutics.
Recent advancements in bio-scaffolds for cardiac disorders have introduced groundbreaking technologies that improve both the mechanical and biochemical properties of these scaffolds, enhancing their application in cardiac tissue regeneration. For instance, bioresorbable scaffolds (BRS) have emerged as a new paradigm in cardiovascular interventions. These scaffolds provide temporary support to maintain vessel patency and then gradually dissolve, avoiding long-term complications like stent thrombosis. New-generation BRS devices offer thinner struts and enhanced mechanical properties, improving safety and efficacy in treating coronary artery disease. Such advancements allow for positive remodeling of the vasculature, restoring natural vessel function post-implantation (Tenekecioglu et al., 2016). Electrospun nanofibrous scaffolds have also seen significant innovations. These scaffolds, mimicking the structure of native ECM, are designed to enhance mechanical strength and biological properties suitable for cardiac tissue regeneration. Recent studies emphasize the incorporation of bioactive molecules into nanofibers, allowing the scaffolds to support functional cardiac tissue regeneration while ensuring long-term cell viability and functionality. Electrospun scaffolds are now being tailored for improved cardiomyocyte alignment and electrical conductivity, which are critical for restoring cardiac tissue function (Zhao et al., 2015).
3D bioprinting and bioprinted scaffolds have gained traction in recent years, offering more sophisticated options for fabricating personalized cardiac scaffolds. This technology enables the precise layering of cells and biomaterials to create complex structures that closely resemble cardiac tissues. The use of biomaterials such as hydrogels and bioinks in conjunction with stem cells can promote the formation of cardiac valves and vascular networks. These advancements have shown promise in improving cell viability, reducing inflammation, and fostering the regeneration of cardiac tissues (Kozaniti et al., 2021).
Further developments in tissue-engineered cardiac scaffolds focus on biomimetic design to replicate the mechanical behaviors of the myocardium. Scaffolds that incorporate nonlinear elasticity, anisotropy, and viscoelasticity mimic the natural biomechanics of heart tissues more effectively. This advancement provides a closer match to the physiological environment, enhancing the potential for better integration and functionality of the transplanted tissue. Improvements in mechanical behavior have been critical for developing scaffolds that promote cardiac regeneration (Baghersad et al., 2023). Another promising approach involves the use of conductive bio-scaffolds that support cardiac cell growth while enhancing electrical signaling between cardiomyocytes. Carbon nanotube (CNT) scaffolds have shown significant potential in improving the electrical and mechanical properties of engineered cardiac tissue. By incorporating CNTs, these scaffolds foster better organization, retention, and physiological function of cardiomyocytes, promoting synchronous beating and improved contractile activity. This innovation could lead to more effective cardiac tissue regeneration therapies (Dozois et al., 2017).
In summary, bio-scaffolds represent a cutting-edge approach to treating cardiac disorders, with various studies demonstrating their effectiveness in supporting cardiac cell functions, promoting tissue regeneration, and integrating seamlessly with the host tissue. These scaffolds not only mimic the physical and biochemical properties of the native ECM but also introduce innovative features such as electrical conductivity and shape-memory capabilities, which are critical for the successful restoration of heart function. As research continues to advance, bio-scaffolds are poised to play a transformative role in cardiac regenerative medicine, offering hope for more effective and less invasive treatments for heart disease (Table 7).
Nanomaterials currently being used for cardiac cell therapy
Nanomaterials are revolutionizing cardiac cell therapy by offering novel ways to enhance the repair and regeneration of heart tissue, which is crucial given the high mortality and morbidity associated with cardiovascular diseases. Gold nanoparticles (GNPs) are among the most extensively studied nanomaterials in this field due to their remarkable electrical conductivity and ease of functionalization. The work of Esmaeili et al. (2022) underscores the potential of GNPs in cardiac tissue engineering, particularly in their ability to facilitate the synchronized contraction of cardiac cells by enhancing electrical signaling pathways. This property is essential for the restoration of normal heart rhythm and function in damaged myocardium. Moreover, GNPs have been shown to support the proliferation and differentiation of cardiac cells, which is critical for the regeneration of heart tissue following injury such as myocardial infarction (Figure 3).
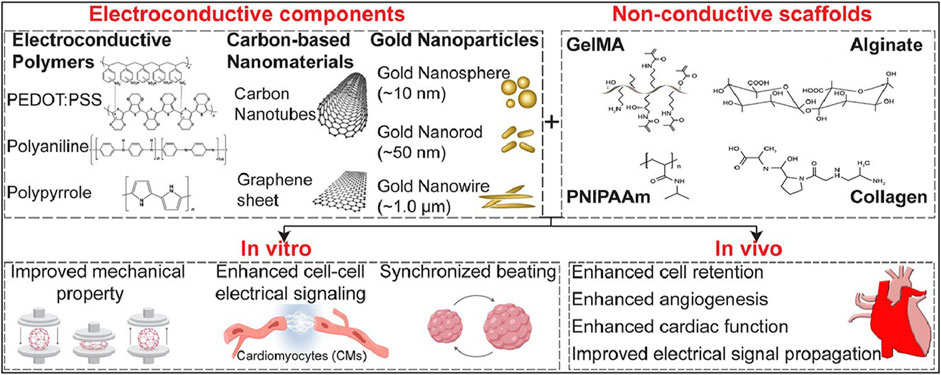
Figure 3. This figure covers the recent development in the use of engineered electroconductive tissues for in vivo cardiac regeneration applications. We will discuss the prospects and challenges of each approach and provide our viewpoints on possible paths for enhanced cTE using different types of nanomaterials including gold nanoparticles (GNPs), silicon-derived nanomaterials, carbon-based nanomaterials (CBNs), as well as electroconductive polymers (ECPs) (Esmaeili et al., 2022).
Despite these benefits, the application of GNPs in clinical settings is not without challenges. Their potential cytotoxicity, especially at higher concentrations or with prolonged exposure, raises significant safety concerns. Additionally, the high cost associated with gold materials presents a barrier to the widespread adoption of GNP-based therapies. However, advances in surface modification techniques, such as PEGylation, have been developed to improve the biocompatibility of GNPs, reduce their immunogenicity, and enhance their stability in biological environments, thereby mitigating some of these challenges. Carbon-based nanomaterials, including carbon nanotubes (CNTs) and graphene oxide (GO), represent another promising avenue in the development of cardiac therapies. CNTs, with their exceptional mechanical strength and electrical conductivity, are particularly well-suited for use in cardiac scaffolding, where they can mimic the structural and functional properties of native myocardium. Research by Jalilinejad et al. (2023) highlights how CNTs can enhance the adhesion, proliferation, and alignment of cardiac cells, which are vital processes in the formation of functional cardiac tissue (Figure 4).
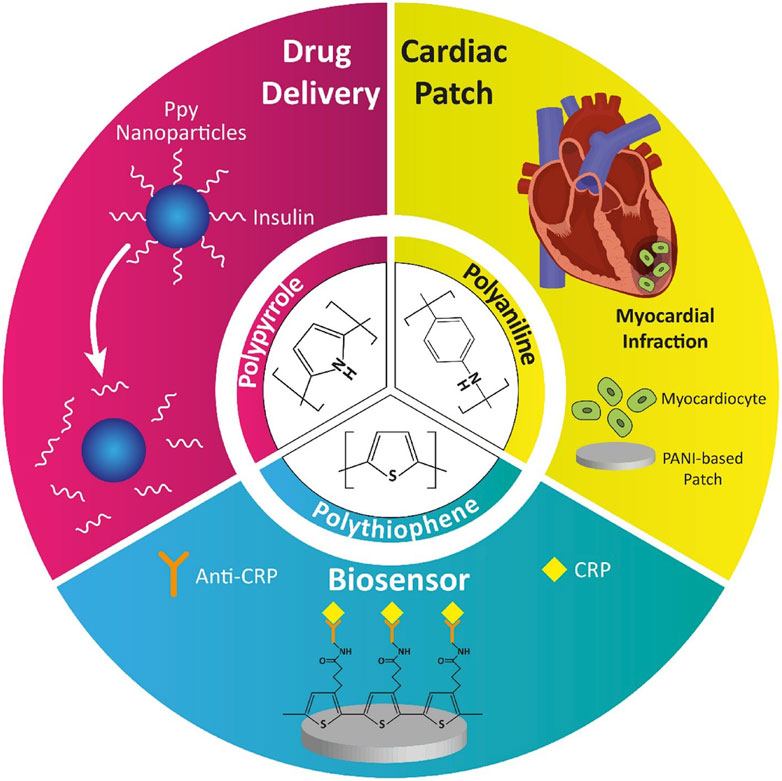
Figure 4. Application of conductive polymers in nanomedicine (Jalilinejad et al., 2023).
This makes CNTs highly effective in supporting the regeneration of heart tissue following damage. However, their application is complicated by issues related to bio-persistence and potential pulmonary toxicity, particularly when inhaled. The inherent difficulty in removing CNTs from biological systems poses a long-term safety risk, which is compounded by their tendency to accumulate in tissues (Razavi et al., 2024c). To address these issues, CNTs are often functionalized with biocompatible molecules such as proteins or peptides, which can reduce their toxicity and improve their integration with biological tissues. On the other hand, GO, with its high surface area and flexible structure, has been shown to enhance the differentiation of stem cells into cardiomyocytes, as reported by Qu et al. (2018). GO’s ability to create a conducive environment for cell growth and differentiation is particularly beneficial in cardiac repair applications. However, its propensity to aggregate and its potential cytotoxicity require careful management, often necessitating surface modifications to improve its dispersion and reduce harmful interactions with cells.
Magnetic nanoparticles (MNPs), particularly those composed of iron oxide, are another class of nanomaterials gaining traction in cardiac cell therapy. These nanoparticles are valued for their magnetic properties, which allow for precise manipulation and targeted delivery of therapeutic agents to specific areas of the heart. Cassani et al. (2020) have demonstrated that MNPs can be used not only for targeted drug delivery but also for imaging, thereby providing a dual function in both the treatment and diagnosis of cardiac conditions such as myocardial infarction (Figure 5).
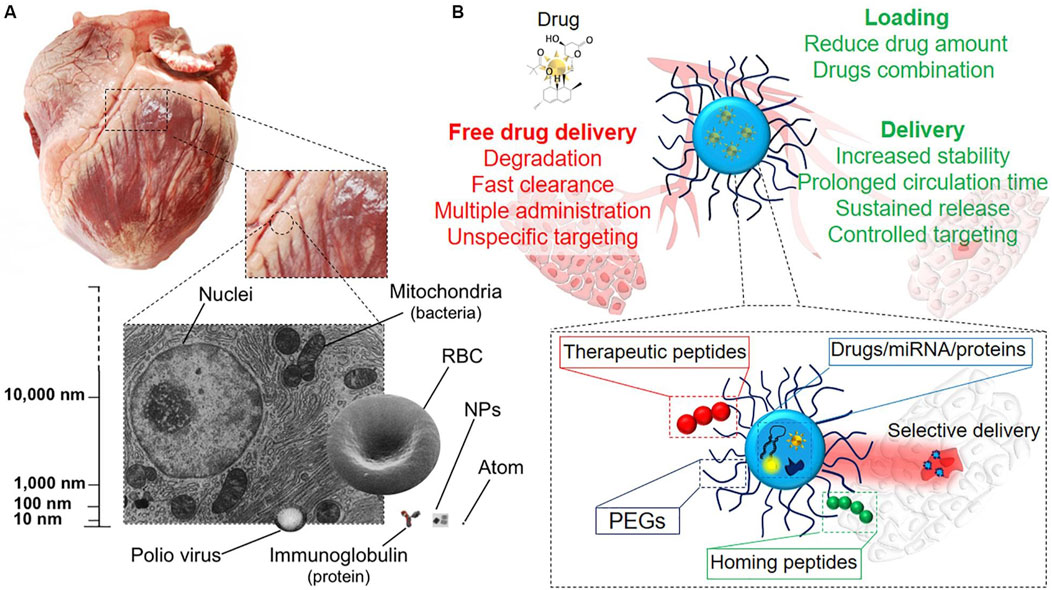
Figure 5. (A) Nanoscale representation, scaling from pig heart to single cells and intracellular organelles, which contain NPs. (B) Diagram of medication delivery methods. NPs offer several benefits over systemic drug administration, including tailored release and dose reduction, due to their many functional blocks (Cassani et al., 2020).
The magnetic properties of MNPs enable them to be directed to specific sites within the body using external magnetic fields, thereby increasing the concentration of therapeutic agents at the site of injury and reducing systemic side effects (Farokhi et al., 2024; Sarmadi et al., 2024). However, the potential for oxidative stress caused by the release of iron ions from these nanoparticles is a significant concern. This oxidative stress can lead to further damage to cardiac cells, negating the therapeutic benefits. To mitigate these risks, MNPs are often coated with biocompatible materials such as polymers or silica, which can help control the release of iron ions and reduce the potential for oxidative damage. Furthermore, the use of exosomes, which are cell-derived vesicles, in cardiac therapy has been explored as a natural and biocompatible alternative for delivering therapeutic agents. Han et al. (2015) found that exosomes can play a crucial role in facilitating intercellular communication and enhancing the reparative processes in damaged cardiac tissues.
Due to their natural origin, exosomes have low immunogenicity and can be engineered to carry specific therapeutic molecules, making them a highly targeted and efficient delivery system. However, challenges related to the production scalability and rapid clearance of exosomes from the body limit their current clinical applications (Fakouri et al., 2024). Polymeric nanomaterials, such as poly(lactic-co-glycolic acid) (PLGA), represent a versatile platform for cardiac cell therapy, particularly in the context of controlled drug delivery and tissue engineering. These biodegradable polymers are highly valued for their ability to degrade into non-toxic byproducts within the body, which makes them suitable for temporary scaffolding applications in cardiac tissue regeneration. Su and Kang (2020) emphasize that the tunable degradation rates of PLGA allow for the sustained release of therapeutic agents, ensuring that the treatment remains effective over the critical period of tissue repair. This is particularly important in cardiac therapy, where the timing and dosage of drug release can significantly impact the outcome. PLGA scaffolds can be engineered to degrade over weeks or months, depending on the specific needs of the treatment, providing a controlled environment for cell growth and differentiation. However, achieving precise control over the degradation rate is challenging and critical to ensure that the scaffold provides adequate support throughout the healing process but does not persist long enough to interfere with the newly formed tissue. Additionally, while PLGA is FDA-approved and widely regarded as safe, there is still a risk of immune responses, particularly when the polymer is used in combination with other materials or in patients with pre-existing conditions. Surface modifications, such as the addition of growth factors or adhesion molecules, can enhance the integration of PLGA scaffolds with cardiac tissues, promoting cell attachment and proliferation. Moreover, the combination of PLGA with other nanomaterials, such as magnetic nanoparticles or gold nanoparticles, is being explored to harness the benefits of multiple materials, thereby advancing the effectiveness of cardiac cell therapy. These studies collectively underscore the vast potential and the inherent complexities of using nanomaterials in cardiac cell therapy. While each type of nanomaterial offers distinct advantages—such as the electrical conductivity of gold nanoparticles, the mechanical strength of carbon nanotubes, the targeted delivery capabilities of magnetic nanoparticles, and the biodegradability of polymeric materials—each also presents unique challenges. These include issues related to cytotoxicity, long-term biocompatibility, immune response, and the need for precise control over material properties and interactions with biological systems. Ongoing research is focused on overcoming these challenges through the development of advanced surface modification techniques, the combination of multiple nanomaterials to exploit their synergistic effects, and the refinement of delivery systems to ensure that therapeutic agents reach their target sites in the most effective manner. As the field continues to evolve, the integration of nanomaterials with cutting-edge biological and engineering approaches holds the promise of significantly improving the outcomes of cardiac regeneration therapies, offering new hope for patients suffering from heart disease. The future of cardiac cell therapy lies in the continued exploration and optimization of these nanomaterials, with the ultimate goal of achieving safe, effective, and scalable treatments that can be widely implemented in clinical practice (Table 8).
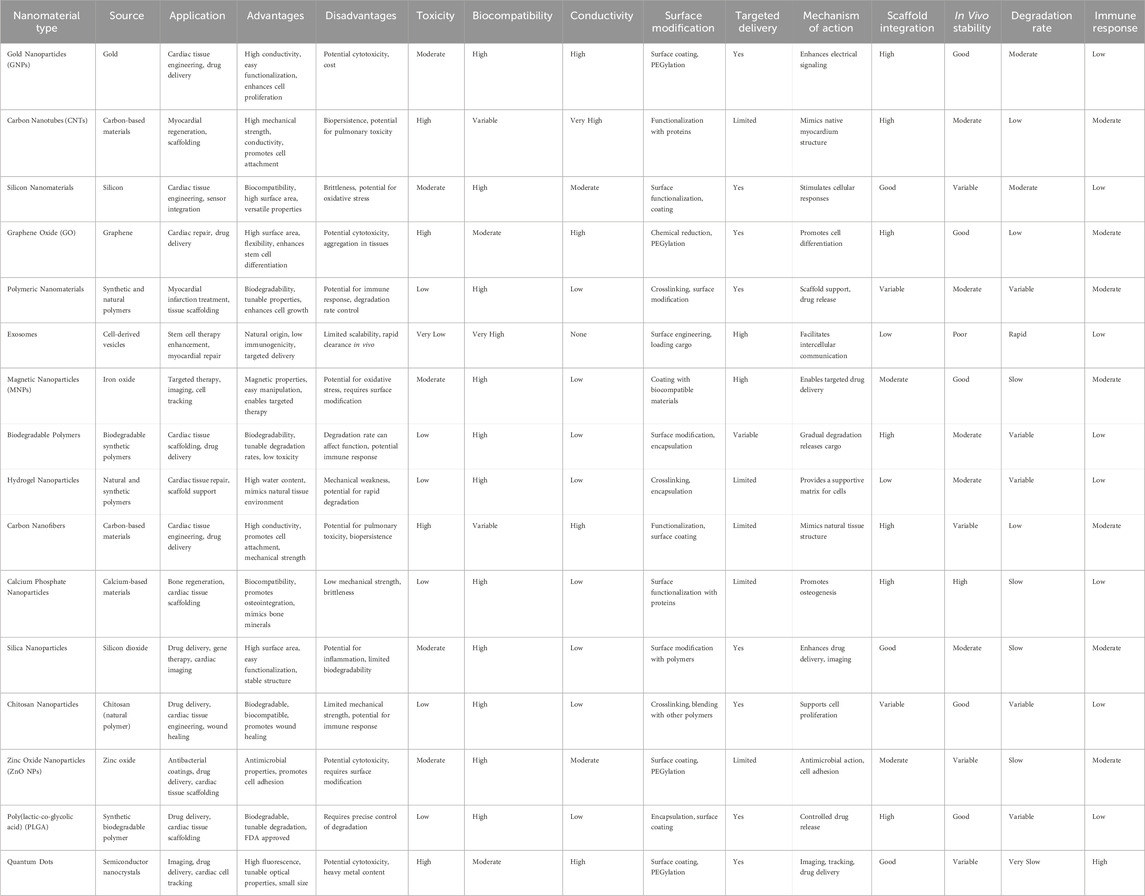
Table 8. This table includes variety of additional nanomaterials along with more detailed columns to cover a broader spectrum of their characteristics and applications. This comprehensive overview provides insights into the use of nanomaterials in cardiac cell therapy, along with their advantages, challenges, and therapeutic roles.
Cell type used in cardiac scaffolding
Whether it's 3D printed scaffolding for the heart or 3D printed cells of some other kind, the field of tissue engineering is expanding rapidly. Each type of cell has its own category, such as human cells and non-human cells. They can function alone or in tandem with one another. Recently, it has been shown that myoblast cells, which originate from rats, are functionally expressed in a variety of cardiac tissues (Khan et al., 2022). Until far, ESCs, produced from bone marrow or adipose tissue, as well as skeletal myoblasts and localized cardiac stem cells, have been the most often employed human cells for cardiac tissue engineering. Precursor cells from a wide range of origins are being employed in regenerative medicine for heart repair thanks to recent developments in stem cell biology (Khan et al., 2018). There is a growing body of evidence suggesting that regenerative medicine and engineering based on stem cells can be an effective therapy for CVDs. Prior to placentation and renewal, ESCs are in their prime for cardiac tissue engineering (Scott et al., 2022). They were originally cultivated in vitro in 1998 and were obtained by isolating them from human blastocysts. This need for cells was addressed by the generation of stem cells by creating iPSCs from somatic cells of patients. Similar to ESCs, these cells have the potential to generate an infinite number of distinct cell types, one of which is functioning CMs. The addition of the transcription factors kfl-4, c-Myc, Oct4, and Sox two reprograms somatic cells into iPSCs. To a greater extent, it finds application in heart tissue engineering. These iPSCs can be employed for cardiac tissue engineering after being prompted to develop into cardiac progenitor cells by the addition of the transcription factors Flk1, Isl1, or Nkx2-5. Additionally, the Wnt/Catenin signalling pathway permits the differentiation of ESCs and iPSCs into CMs and vascular cells. Stopping glycogen synthase kinase three prior to ESC or iPSC development activates the Wnt/Catenin signalling pathway. Due to the fact that iPSCs will be produced from the patient’s own somatic cells, they will not trigger an immune response (Brown et al., 2020; Fathi et al., 2020; Wang et al., 2019). As autologous CMs are a necessary component in the development of a synthetic cardiac tissue construct, iPSCs are a promising source for their generation. For the purpose of tissue engineering, embryonic stem cells (ESCs) and induced pluripotent stem cells (iPSCs) can be differentiated into CM using a variety of procedures. Yet, insufficient maturity of stem cells presents a significant barrier to the eventual objective of cardiac remodelling therapy, making the promotion of CM maturation crucial (Yeung et al., 2019; Musunuru et al., 2018). Human embryonic stem cell-derived cardiac myocytes (hESC-CMs) were shown to significantly resuscitate, albeit with impaired maturation, in an inhuman model of ischemia-reperfusion ischemia by Chong and colleagues. Arrhythmia in the ventricles seldom results in death. Most electrophysiologically functioning mature CMs display cardiac markers with distinct potential for MI therapy, and recent advances in reprogramming mouse somatic cells into pluripotent stem cells have made this possible (Augustine et al., 2021). When it comes to human cells, some typical possibilities for 3D printing of bioscaffold are hCMPCs and hiPSC-CM. When the biomass was printed using the procedures described above, these cells displayed the genetic profile and expression of the native cardiac protein (Williams et al., 2022) (Table 9).
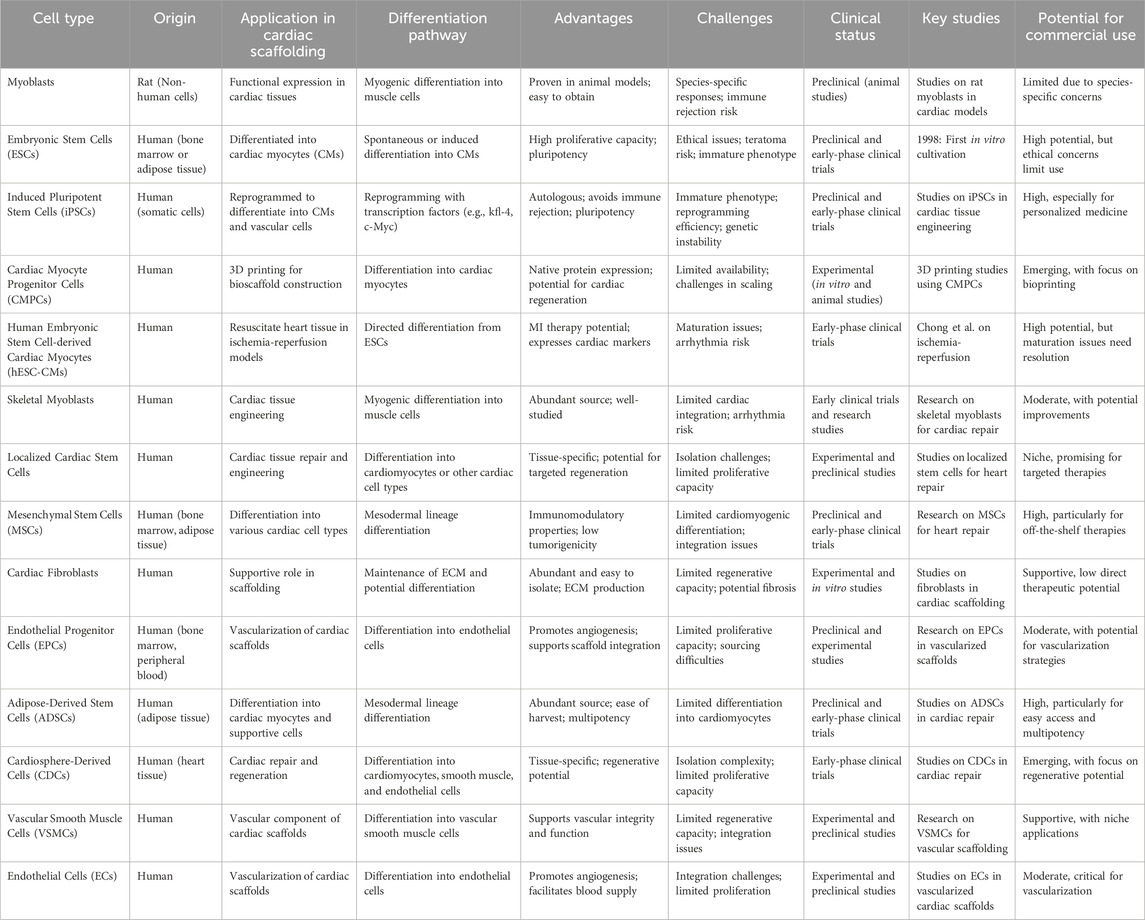
Table 9. This table includes a more comprehensive range of cell types used in cardiac scaffolding, with additional columns for differentiation pathways, advantages, challenges, clinical status, key studies, and potential for commercial use.
Conclusion
The use of stem cell treatment, including the use of exosomes and other minuscule vesicles that are released by stem cells, as well as tissue engineering, are all potential ways for rebuilding injured cardiac tissue. In spite of the extremely high expectations, the majority of clinical studies have resulted in unsatisfactory findings. There is compelling evidence that stem cell-derived paracrine factors contribute to myocardial cardioprotection and regeneration. This is the case despite the fact that stem cells injected into heart muscle have a low engraftment and survival rate. Numerous distinct subgroups of stem cells have been investigated in both animal and human research. All other types of stem cells, with the exception of embryonic and induced pluripotent stem cells (ESCs and iPSCS), have been examined and evaluated in human subjects. Stem cells generated from bone marrow are often used in clinical research. The easy availability of these stem cells and the low risk associated with their use in therapeutic settings are two factors that favor their utilization. Nevertheless, the prospective applications of hESCs may be limited because of ethical considerations, the possibility of teratoma formation, and immune system rejection. The transplantation of human embryonic stem cells (hESCs) is met with immunological rejection and presents ethical issues; nevertheless, these obstacles may be overcome with a clearer understanding of the molecular and genetic pathways involved in ESC differentiation and heart development. In addition, the usage of iPSCs may assist circumvent some of the challenges that are presented by the utilization of hESCs.
Author contributions
ZR: Conceptualization, Data curation, Investigation, Software, Writing–original draft, Writing–review and editing. SF: Conceptualization, Investigation, Writing–original draft, Writing–review and editing. GM: Conceptualization, Writing–original draft. AK-R: Conceptualization, Writing–original draft. BF-F: Writing–review and editing. ST-G: Writing–review and editing. HP-T: Writing–review and editing. MS-F: Methodology, Project administration, Supervision, Validation, Writing–review and editing. HA: Methodology, Project administration, Supervision, Validation, Writing–review and editing.
Funding
The author(s) declare that no financial support was received for the research, authorship, and/or publication of this article.
Conflict of interest
The authors declare that the research was conducted in the absence of any commercial or financial relationships that could be construed as a potential conflict of interest.
Publisher’s note
All claims expressed in this article are solely those of the authors and do not necessarily represent those of their affiliated organizations, or those of the publisher, the editors and the reviewers. Any product that may be evaluated in this article, or claim that may be made by its manufacturer, is not guaranteed or endorsed by the publisher.
References
Abou-Saleh, H., Zouein, F. A., El-Yazbi, A., Sanoudou, D., Raynaud, C., Rao, C., et al. (2018). The march of pluripotent stem cells in cardiovascular regenerative medicine. Stem Cell Res. Ther. 9 (1), 201. doi:10.1186/s13287-018-0947-5
Ahmed, E., Saleh, T., and Xu, M. (2021). Recellularization of native tissue derived acellular scaffolds with mesenchymal stem cells. Cells 10 (7), 1787. doi:10.3390/cells10071787
Ahmed, L. A., and Al-Massri, K. F. (2021). Directions for enhancement of the therapeutic efficacy of mesenchymal stem cells in different neurodegenerative and cardiovascular diseases: current status and future perspectives. Curr. Stem Cell Res. Ther. 16 (7), 858–876. doi:10.2174/1574888X16666210303151237
Ajmal, L., Ajmal, S., Ajmal, M., and Nawaz, G. (2023). Organ regeneration through stem cells and tissue engineering. Cureus 15 (1), e34336. doi:10.7759/cureus.34336
Alnasser, S. M. (2023). Advances and challenges in cancer stem cells for onco-therapeutics. Stem Cells Int. 2023, 8722803. doi:10.1155/2023/8722803
Andalib, E., Kashfi, M., Mahmoudvand, G., Rezaei, E., Mahjoor, M., Torki, A., et al. (2023). Application of hypoxia-mesenchymal stem cells in treatment of anaerobic bacterial wound infection: wound healing and infection recovery. Front. Microbiol. 14, 1251956. doi:10.3389/fmicb.2023.1251956
Andrzejewska, A., Lukomska, B., and Janowski, M. (2019). Concise review: mesenchymal stem cells: from roots to boost. Stem Cells 37 (7), 855–864. doi:10.1002/stem.3016
Atia, G. A., Rashed, F., Taher, E. S., Cho, S. G., Dayem, A. A., Soliman, M. M., et al. (2024). Challenges of therapeutic applications and regenerative capacities of urine based stem cells in oral, and maxillofacial reconstruction. Biomed. and Pharmacother. 177, 117005. doi:10.1016/j.biopha.2024.117005
Attar, A., Hosseinpour, A., Hosseinpour, H., and Kazemi, A. (2022). Major cardiovascular events after bone marrow mononuclear cell transplantation following acute myocardial infarction: an updated post-BAMI meta-analysis of randomized controlled trials. BMC Cardiovasc Disord. 22 (1), 259. doi:10.1186/s12872-022-02701-x
Augustine, R., Dan, P., Hasan, A., Khalaf, I. M., Prasad, P., Ghosal, K., et al. (2021). Stem cell-based approaches in cardiac tissue engineering: controlling the microenvironment for autologous cells. Biomed. and Pharmacother. 138, 111425. doi:10.1016/j.biopha.2021.111425
Baghersad, S., Sathish Kumar, A., Kipper, M. J., Popat, K., and Wang, Z. (2023). Recent advances in tissue-engineered cardiac scaffolds-the progress and gap in mimicking native myocardium mechanical behaviors. J. Funct. Biomater. 14 (5), 269. doi:10.3390/jfb14050269
Banerjee, M. N., Bolli, R., and Hare, J. M. (2018). Clinical studies of cell therapy in cardiovascular medicine: recent developments and future directions. Circ. Res. 123 (2), 266–287. doi:10.1161/CIRCRESAHA.118.311217
Bello, A. B., Park, H., and Lee, S.-H. (2018). Current approaches in biomaterial-based hematopoietic stem cell niches. Acta Biomater. 72, 1–15. doi:10.1016/j.actbio.2018.03.028
Bian, D., Wu, Y., Song, G., Azizi, R., and Zamani, A. (2022). The application of mesenchymal stromal cells (MSCs) and their derivative exosome in skin wound healing: a comprehensive review. Stem Cell Res. and Ther. 13 (1), 24–17. doi:10.1186/s13287-021-02697-9
Bianconi, V., Sahebkar, A., Kovanen, P., Bagaglia, F., Ricciuti, B., Calabrò, P., et al. (2018). Endothelial and cardiac progenitor cells for cardiovascular repair: a controversial paradigm in cell therapy. Pharmacol. Ther. 181, 156–168. doi:10.1016/j.pharmthera.2017.08.004
Biniazan, F., Stoian, A., and Haykal, S. (2024). Adipose-derived stem cells: angiogenetic potential and utility in tissue engineering. Int. J. Mol. Sci. 25 (4), 2356. doi:10.3390/ijms25042356
Brown, M. A., Rajamarthandan, S., Francis, B., O'Leary-Kelly, M. K., and Sinha, P. (2020). Update on stem cell technologies in congenital heart disease. J. Card. Surg. 35 (1), 174–179. doi:10.1111/jocs.14312
Bruno, A., Milillo, C., Anaclerio, F., Buccolini, C., Dell'Elice, A., Angilletta, I., et al. (2024). Perinatal tissue-derived stem cells: an emerging therapeutic strategy for challenging neurodegenerative diseases. Int. J. Mol. Sci. 25 (2), 976. doi:10.3390/ijms25020976
Cassani, M., Fernandes, S., Vrbsky, J., Ergir, E., Cavalieri, F., and Forte, G. (2020). Combining nanomaterials and developmental pathways to design new treatments for cardiac regeneration: the pulsing heart of advanced therapies. Front. Bioeng. Biotechnol. 8, 323. doi:10.3389/fbioe.2020.00323
Castaldo, C., and Chimenti, I. (2018). Cardiac progenitor cells: the matrix has you. Stem Cells Transl. Med. 7 (7), 506–510. doi:10.1002/sctm.18-0023
Cecerska-Heryć, E., Pękała, M., Serwin, N., Gliźniewicz, M., Grygorcewicz, B., Michalczyk, A., et al. (2023). The use of stem cells as a potential treatment method for selected neurodegenerative diseases: review. Cell Mol. Neurobiol. 43 (6), 2643–2673. doi:10.1007/s10571-023-01344-6
Cha, C., Shin, S. R., Annabi, N., Dokmeci, M. R., and Khademhosseini, A. (2013). Carbon-based nanomaterials: multifunctional materials for biomedical engineering. ACS nano 7 (4), 2891–2897. doi:10.1021/nn401196a
Chang, E. A., Jin, S. W., Nam, M. H., and Kim, S. D. (2019). Human induced pluripotent stem cells: clinical significance and applications in neurologic diseases. J. Korean Neurosurg. Soc. 62 (5), 493–501. doi:10.3340/jkns.2018.0222
Chang, Y. H., Wu, K. C., Harn, H. J., Lin, S. Z., and Ding, D. C. (2018). Exosomes and stem cells in degenerative disease diagnosis and therapy. Cell Transpl. 27 (3), 349–363. doi:10.1177/0963689717723636
Chen, A. C. H., Peng, Q., Fong, S. W., Lee, K. C., Yeung, W. S. B., and Lee, Y. L. (2021). DNA damage response and cell cycle regulation in pluripotent stem cells. Genes (Basel) 12 (10), 1548. doi:10.3390/genes12101548
Chen, K., Li, Y., Xu, L., Qian, Y., Liu, N., Zhou, C., et al. (2022). Comprehensive insight into endothelial progenitor cell-derived extracellular vesicles as a promising candidate for disease treatment. Stem Cell Res. and Ther. 13 (1), 238. doi:10.1186/s13287-022-02921-0
Heart disease and stroke statistics—2023 update: a report from the American heart association. (2023). Circulation, 147. doi:10.1161/CIR.0000000000001123
Cruz-Samperio, R., Jordan, M., and Perriman, A. (2021). Cell augmentation strategies for cardiac stem cell therapies. Stem Cells Transl. Med. 10 (6), 855–866. doi:10.1002/sctm.20-0489
Csöbönyeiová, M., Beerová, N., Klein, M., Debreová-Čeháková, M., and Danišovič, Ľ. (2022). Cell-based and selected cell-free therapies for myocardial infarction: how do they compare to the current treatment options? Int. J. Mol. Sci. 23 (18), 10314. doi:10.3390/ijms231810314
De Bartolo, L., and Bader, A., (2013). Biomaterials for stem cell therapy: state of art and vision for the future.
Di Cesare, M., Perel, P., Taylor, S., Kabudula, C., Bixby, H., Gaziano, T. A., et al. (2024). The heart of the World. Glob. Heart 19 (1), 11. doi:10.5334/gh.1288
Dozois, M. D., Bahlmann, L. C., Zilberman, Y., and Tang, X. S. (2017). Carbon nanomaterial-enhanced scaffolds for the creation of cardiac tissue constructs: a new frontier in cardiac tissue engineering. Carbon 120, 338–349. doi:10.1016/j.carbon.2017.05.050
Ebrahimi, M., Forouzesh, M., Raoufi, S., Ramazii, M., Ghaedrahmati, F., and Farzaneh, M. (2020). Differentiation of human induced pluripotent stem cells into erythroid cells. Stem Cell Res. Ther. 11 (1), 483. doi:10.1186/s13287-020-01998-9
Eschenhagen, T., and Carrier, L. (2019). Cardiomyopathy phenotypes in human-induced pluripotent stem cell-derived cardiomyocytes-a systematic review. Pflugers Arch. 471 (5), 755–768. doi:10.1007/s00424-018-2214-0
Esmaeili, H., Patino-Guerrero, A., Hasany, M., Ansari, M. O., Memic, A., Dolatshahi-Pirouz, A., et al. (2022). Electroconductive biomaterials for cardiac tissue engineering. Acta Biomater. 139, 118–140. doi:10.1016/j.actbio.2021.08.031
Fadini, G. P., Mehta, A., Dhindsa, D. S., Bonora, B. M., Sreejit, G., Nagareddy, P., et al. (2020). Circulating stem cells and cardiovascular outcomes: from basic science to the clinic. Eur. Heart J. 41 (44), 4271–4282. doi:10.1093/eurheartj/ehz923
Fakouri, A., Razavi, Z. S., Mohammed, A. T., Hussein, A. H. A., Afkhami, H., and Hooshiar, M. H. (2024). Applications of mesenchymal stem cell-exosome components in wound infection healing: new insights. Burns and Trauma 12, tkae021. doi:10.1093/burnst/tkae021
Farokhi, S., Razavi, Z. S., Mavaei, M., Shadab, A., Afkhami, H., and Sardarabadi, H. (2024). New perspectives on arteriosclerosis treatment using nanoparticles and mesenchymal stem cells. Discov. Appl. Sci. 6 (8), 411–440. doi:10.1007/s42452-024-06113-8
Fathi, E., Valipour, B., Vietor, I., and Farahzadi, R. (2020). An overview of the myocardial regeneration potential of cardiac c-Kit(+) progenitor cells via PI3K and MAPK signaling pathways. Future Cardiol. 16 (3), 199–209. doi:10.2217/fca-2018-0049
Feng, F., Wu, J., Chi, Q., Wang, S., Liu, W., Yang, L., et al. (2024). Lactylome analysis unveils lactylation-dependent mechanisms of stemness remodeling in the liver cancer stem cells. Adv. Sci., 2405975. doi:10.1002/advs.202405975
Fu, X., Liu, G., Halim, A., Ju, Y., Luo, Q., and Song, A. G. (2019). Mesenchymal stem cell migration and tissue repair. Cells 8 (8), 784. doi:10.3390/cells8080784
Fujii, Y., Hatori, A., Chikazu, D., and Ogasawara, T. (2023). Application of dental pulp stem cells for bone and neural tissue regeneration in oral and maxillofacial region. Stem Cells Int. 2023, 2026572. doi:10.1155/2023/2026572
Fukunishi, T., Best, C. A., Ong, C. S., Groehl, T., Reinhardt, J., Yi, T., et al. (2018). Role of bone marrow mononuclear cell seeding for nanofiber vascular grafts. Tissue Eng. Part A 24 (1-2), 135–144. doi:10.1089/ten.TEA.2017.0044
Gaetani, R., Feyen, D. A. M., Verhage, V., Slaats, R., Messina, E., Christman, K. L., et al. (2015). Epicardial application of cardiac progenitor cells in a 3D-printed gelatin/hyaluronic acid patch preserves cardiac function after myocardial infarction. Biomaterials 61, 339–348. doi:10.1016/j.biomaterials.2015.05.005
Gallina, C., Capelôa, T., Saviozzi, S., Accomasso, L., Catalano, F., Tullio, F., et al. (2015). Human mesenchymal stem cells labelled with dye-loaded amorphous silica nanoparticles: long-term biosafety, stemness preservation and traceability in the beating heart. J. Nanobiotechnology 13, 77. doi:10.1186/s12951-015-0141-1
Ge, H., Zhou, T., Zhang, C., Cun, Y., Chen, W., Yang, Y., et al. (2023). Targeting ASIC1a promotes neural progenitor cell migration and neurogenesis in ischemic stroke. Research 6, 0105. doi:10.34133/research.0105
Gokce, C., Gurcan, C., Delogu, L. G., and Yilmazer, A. (2022). 2D materials for cardiac tissue repair and regeneration. Front. Cardiovasc Med. 9, 802551. doi:10.3389/fcvm.2022.802551
Golpanian, S., Wolf, A., Hatzistergos, K. E., and Hare, J. M. (2016). Rebuilding the damaged heart: mesenchymal stem cells, cell-based therapy, and engineered heart tissue. Physiol. Rev. 96 (3), 1127–1168. doi:10.1152/physrev.00019.2015
Gorshkov, K., Chen, C. Z., Marshall, R. E., Mihatov, N., Choi, Y., Nguyen, D. T., et al. (2019). Advancing precision medicine with personalized drug screening. Drug Discov. Today 24 (1), 272–278. doi:10.1016/j.drudis.2018.08.010
Guo, Y., Yu, Y., Hu, S., Chen, Y., and Shen, Z. (2020). The therapeutic potential of mesenchymal stem cells for cardiovascular diseases. Cell Death Dis. 11 (5), 349. doi:10.1038/s41419-020-2542-9
Guyette, J. P., Charest, J. M., Mills, R. W., Jank, B. J., Moser, P. T., Gilpin, S. E., et al. (2016). Bioengineering human myocardium on native extracellular matrix. Circulation Res. 118 (1), 56–72. doi:10.1161/CIRCRESAHA.115.306874
Haba, M. Ș. C., Șerban, D. N., Șerban, L., Tudorancea, I. M., Haba, R. M., Mitu, O., et al. (2021). Nanomaterial-based drug targeted therapy for cardiovascular diseases: ischemic heart failure and atherosclerosis. Crystals 11 (10), 1172. doi:10.3390/cryst11101172
Han, J., Park, J., and Kim, B. S. (2015). Integration of mesenchymal stem cells with nanobiomaterials for the repair of myocardial infarction. Adv. Drug Deliv. Rev. 95, 15–28. doi:10.1016/j.addr.2015.09.002
Hao, C.-N., Huang, J. J., Shi, Y. Q., Cheng, X. W., Li, H. Y., Zhou, L., et al. (2014). Pulsed electromagnetic field improves cardiac function in response to myocardial infarction. Am. J. Transl. Res. 6 (3), 281–290.
Hatami, S., Ghorabi, S. T., Mansouri, K., Razavi, Z., and Rouzbahani, A. K. (2023a). The role of human platelet-rich plasma in burn injury patients: a single center study. Canon J. Med. 4 (2), 41–45. doi:10.30477/cjm.2023.368686.1052
Hatami, S., Ghorabi, S. T., Ahmadi, P., Razavi, Z., and Rouzbahani, A. K. (2023b). Evaluation the effect of lipofilling in burn scar: a cross-sectional study. Canon J. Med. 4 (3), 78–82. doi:10.30477/cjm.2023.368788.1053
Henning, R. J. (2021). Cardiovascular exosomes and MicroRNAs in cardiovascular physiology and pathophysiology. J. Cardiovasc Transl. Res. 14 (2), 195–212. doi:10.1007/s12265-020-10040-5
Hu, R., and Feng, H. (2017). Lenticulostriate artery and lenticulostriate-artery neural complex: new concept for intracerebral hemorrhage. Curr. Pharm. Des. 23 (15), 2206–2211. doi:10.2174/1381612823666170220163750
Huang, Y., Wang, C., Zhou, T., Xie, F., Liu, Z., Xu, H., et al. (2024). Lumican promotes calcific aortic valve disease through H3 histone lactylation. Eur. Heart J., ehae407. doi:10.1093/eurheartj/ehae407
Ishida, T., Nakao, S., Ueyama, T., Harada, Y., and Kawamura, T. (2020). Metabolic remodeling during somatic cell reprogramming to induced pluripotent stem cells: involvement of hypoxia-inducible factor 1. Inflamm. Regen. 40, 8. doi:10.1186/s41232-020-00117-8
Jalilinejad, N., Rabiee, M., Baheiraei, N., Ghahremanzadeh, R., Salarian, R., Rabiee, N., et al. (2023). Electrically conductive carbon-based (bio)-nanomaterials for cardiac tissue engineering. Bioeng. and Transl. Med. 8 (1), e10347. doi:10.1002/btm2.10347
Jiang, W., and Xu, J. (2020). Immune modulation by mesenchymal stem cells. Cell Prolif. 53 (1), e12712. doi:10.1111/cpr.12712
Karagiannis, P., Takahashi, K., Saito, M., Yoshida, Y., Okita, K., Watanabe, A., et al. (2019). Induced pluripotent stem cells and their use in human models of disease and development. Physiol. Rev. 99 (1), 79–114. doi:10.1152/physrev.00039.2017
Khan, I., Saeed, K., and Khan, I. (2019). Nanoparticles: properties, applications and toxicities. Arabian J. Chem. 12 (7), 908–931. doi:10.1016/j.arabjc.2017.05.011
Khan, K., Gasbarrino, K., Mahmoud, I., Dufresne, L., Daskalopoulou, S. S., Schwertani, A., et al. (2022). Bioactive scaffolds in stem cell-based therapies for myocardial infarction: a systematic review and meta-analysis of preclinical trials. Stem Cell Rev. Rep. 18 (6), 2104–2136. doi:10.1007/s12015-021-10186-y
Khan, K., Gasbarrino, K., Mahmoud, I., Makhoul, G., Yu, B., Dufresne, L., et al. (2018). Bioactive scaffolds in stem-cell-based therapies for cardiac repair: protocol for a meta-analysis of randomized controlled preclinical trials in animal myocardial infarction models. Syst. Rev. 7 (1), 225. doi:10.1186/s13643-018-0845-z
Kim, K. P., Han, D. W., Kim, J., and Schöler, H. R. (2021). Biological importance of OCT transcription factors in reprogramming and development. Exp. Mol. Med. 53 (6), 1018–1028. doi:10.1038/s12276-021-00637-4
Kozaniti, F. K., Metsiou, D. N., Manara, A. E., Athanassiou, G., and Deligianni, D. D. (2021). Recent advancements in 3D printing and bioprinting methods for cardiovascular tissue engineering. Bioeng. (Basel) 8 (10), 133. doi:10.3390/bioengineering8100133
Krasilnikova, O. A., Baranovskii, D. S., Yakimova, A. O., Arguchinskaya, N., Kisel, A., Sosin, D., et al. (2022). Intraoperative creation of tissue-engineered grafts with minimally manipulated cells: new concept of bone tissue engineering in situ. Bioeng. (Basel) 9 (11), 704. doi:10.3390/bioengineering9110704
Leal, B. B. J., Wakabayashi, N., Oyama, K., Kamiya, H., Braghirolli, D. I., and Pranke, P. (2021). Vascular tissue engineering: polymers and methodologies for small caliber vascular grafts. Front. Cardiovasc. Med. 7. doi:10.3389/fcvm.2020.592361
Lee, S. H., Ra, J. C., Oh, H. J., Kim, M. J., Setyawan, E. M. N., Choi, Y. B., et al. (2019). Clinical assessment of intravenous endothelial progenitor cell transplantation in dogs. Cell Transpl. 28 (7), 943–954. doi:10.1177/0963689718821686
Leng, M., Peng, Y., Pan, M., and Wang, H. (2021). Experimental study on the effect of allogeneic endothelial progenitor cells on wound healing in diabetic mice. J. Diabetes Res. 2021, 1–15. doi:10.1155/2021/9962877
Liu, J., Gao, J., Liang, Z., Gao, C., Niu, Q., Wu, F., et al. (2022). Mesenchymal stem cells and their microenvironment. Stem Cell Res. Ther. 13 (1), 429. doi:10.1186/s13287-022-02985-y
Lu, T. Y., Lin, B., Kim, J., Sullivan, M., Tobita, K., Salama, G., et al. (2013). Repopulation of decellularized mouse heart with human induced pluripotent stem cell-derived cardiovascular progenitor cells. Nat. Commun. 4, 2307. doi:10.1038/ncomms3307
Lundy, S. D., Zhu, W. Z., Regnier, M., and Laflamme, M. A. (2013). Structural and functional maturation of cardiomyocytes derived from human pluripotent stem cells. Stem Cells Dev. 22 (14), 1991–2002. doi:10.1089/scd.2012.0490
Ma, T., Sun, J., Zhao, Z., Lei, W., Chen, Y., Wang, X., et al. (2017). A brief review: adipose-derived stem cells and their therapeutic potential in cardiovascular diseases. Stem Cell Res. Ther. 8 (1), 124. doi:10.1186/s13287-017-0585-3
Madrid, M., Lakshmipathy, U., Zhang, X., Bharti, K., Wall, D. M., Sato, Y., et al. (2024). Considerations for the development of iPSC-derived cell therapies: a review of key challenges by the JSRM-ISCT iPSC Committee. Cytotherapy. doi:10.1016/j.jcyt.2024.05.022
Mahjoor, M., Afkhami, H., Mollaei, M., Nasr, A., Shahriary, S., and Khorrami, S. (2021). MicroRNA-30c delivered by bone marrow-mesenchymal stem cells induced apoptosis and diminished cell invasion in U-251 glioblastoma cell line. Life Sci. 279, 119643. doi:10.1016/j.lfs.2021.119643
Mahjoor, M., Afkhami, H., Najafi, M., Nasr, A., and Khorrami, S. (2023b). The role of microRNA-30c in targeting interleukin 6, as an inflammatory cytokine, in the mesenchymal stem cell: a therapeutic approach in colorectal cancer. J. Cancer Res. Clin. Oncol. 149 (7), 3149–3160. doi:10.1007/s00432-022-04123-w
Mahjoor, M., Fakouri, A., Farokhi, S., Nazari, H., Afkhami, H., and Heidari, F. (2023a). Regenerative potential of mesenchymal stromal cells in wound healing: unveiling the influence of normoxic and hypoxic environments. Front. Cell Dev. Biol. 11, 1245872. doi:10.3389/fcell.2023.1245872
Mahmoudvand, G., Karimi Rouzbahani, A., Razavi, Z. S., Mahjoor, M., and Afkhami, H. (2023). Mesenchymal stem cell therapy for non-healing diabetic foot ulcer infection: new insight. Front. Bioeng. Biotechnol. 11, 1158484. doi:10.3389/fbioe.2023.1158484
Mazini, L., Rochette, L., Admou, B., Amal, S., and Malka, G. (2020). Hopes and limits of adipose-derived stem cells (ADSCs) and mesenchymal stem cells (MSCs) in wound healing. Int. J. Mol. Sci. 21 (4), 1306. doi:10.3390/ijms21041306
Mirshekar, M., Afkhami, H., Razavi, S., Masjedian Jazi, F., Darban-Sarokhalil, D., Ohadi, E., et al. (2023). Potential antibacterial activity and healing effect of topical administration of bone marrow and adipose mesenchymal stem cells encapsulated in collagen-fibrin hydrogel scaffold on full-thickness burn wound infection caused by Pseudomonas aeruginosa. Burns 49 (8), 1944–1957. doi:10.1016/j.burns.2023.01.005
Mittal, R., Jhaveri, V. M., McMurry, H. S., Kay, S. I. S., Sutherland, K. J., Nicole, L., et al. (2018). Recent treatment modalities for cardiovascular diseases with a focus on stem cells, aptamers, exosomes and nanomedicine. Artif. Cells Nanomed Biotechnol. 46 (Suppl. 1), 831–840. doi:10.1080/21691401.2018.1436555
Moghaddam, A. S., Afshari, J. T., Esmaeili, S. A., Saburi, E., Joneidi, Z., and Momtazi-Borojeni, A. A. (2019). Cardioprotective microRNAs: lessons from stem cell-derived exosomal microRNAs to treat cardiovascular disease. Atherosclerosis 285, 1–9. doi:10.1016/j.atherosclerosis.2019.03.016
Molaei, A., Milani, F. M., Motamedi, M. J., Sepehr, M., Alizadeh, O. H., Saboury, M., et al. (2022). Pharmacological and medical effect of modified skin grafting method in patients with chronic and severe neck burns. 369–375.
Müller, P., Lemcke, H., and David, R. (2018). Stem cell therapy in heart diseases - cell types, mechanisms and improvement strategies. Cell Physiol. Biochem. 48 (6), 2607–2655. doi:10.1159/000492704
Mustafa, G., Hassan, D., Zeeshan, M., Ruiz-Pulido, G., Ebrahimi, N., Mobashar, A., et al. (2023). Advances in nanotechnology versus stem cell therapy for the theranostics of Huntington's disease. J. Drug Deliv. Sci. Technol. 87, 104774. doi:10.1016/j.jddst.2023.104774
Musunuru, K., Sheikh, F., Gupta, R. M., Houser, S. R., Maher, K. O., Milan, D. J., et al. (2018). Induced pluripotent stem cells for cardiovascular disease modeling and precision medicine: a scientific statement from the American heart association. Circ. Genom Precis. Med. 11 (1), e000043. doi:10.1161/HCG.0000000000000043
Nasser, M. I., Qi, X., Zhu, S., He, Y., Zhao, M., Guo, H., et al. (2020). Current situation and future of stem cells in cardiovascular medicine. Biomed. Pharmacother. 132, 110813. doi:10.1016/j.biopha.2020.110813
Nugraha, B., Buono, M. F., von Boehmer, L., Hoerstrup, S. P., and Emmert, M. Y. (2019). Human cardiac organoids for disease modeling. Clin. Pharmacol. Ther. 105 (1), 79–85. doi:10.1002/cpt.1286
Oliveira, M. S., Saldanha-Araujo, F., Goes, A. M. d., Costa, F. F., and de Carvalho, J. L. (2017). Stem cells in cardiovascular diseases: turning bad days into good ones. Drug Discov. Today 22 (11), 1730–1739. doi:10.1016/j.drudis.2017.07.012
Otaghvar, H., Rouzbahani, A., Mahmoudvand, G., Moghaddam, S., Afkham, S., and Razavi, Z. (2022). A brief report on the effect of COVID 19 pandemic on patients undergoing skin graft surgery in a burns hospital from March 2019 to March 2020. J. Case Rep. Med. Hist. 2 (8).
Ott, H. C., Matthiesen, T. S., Goh, S. K., Black, L. D., Kren, S. M., Netoff, T. I., et al. (2008). Perfusion-decellularized matrix: using nature's platform to engineer a bioartificial heart. Nat. Med. 14 (2), 213–221. doi:10.1038/nm1684
Palà, E., Bustamante, A., Jolkkonen, J., Hommel, M., Rosell, A., and Montaner, J. (2020). Blood-based biomarkers and stem cell therapy in human stroke: a systematic review. Mol. Biol. Rep. 47 (8), 6247–6258. doi:10.1007/s11033-020-05627-9
Pittenger, M. F., Discher, D. E., Péault, B. M., Phinney, D. G., Hare, J. M., and Caplan, A. I. (2019). Mesenchymal stem cell perspective: cell biology to clinical progress. npj Regen. Med. 4 (1), 22. doi:10.1038/s41536-019-0083-6
Pomatto, M., Gai, C., Negro, F., Cedrino, M., Grange, C., Ceccotti, E., et al. (2021). Differential therapeutic effect of extracellular vesicles derived by bone marrow and adipose mesenchymal stem cells on wound healing of diabetic ulcers and correlation to their cargoes. Int. J. Mol. Sci. 22 (8), 3851. doi:10.3390/ijms22083851
Purwaningrum, M., Jamilah, N. S., Purbantoro, S. D., Sawangmake, C., and Nantavisai, S. (2021). Comparative characteristic study from bone marrow-derived mesenchymal stem cells. J. Vet. Sci. 22 (6), e74. doi:10.4142/jvs.2021.22.e74
Qu, Y., He, F., Yu, C., Liang, X., Liang, D., Ma, L., et al. (2018). Advances on graphene-based nanomaterials for biomedical applications. Mater Sci. Eng. C Mater Biol. Appl. 90, 764–780. doi:10.1016/j.msec.2018.05.018
Razavi, Z., Soltani, M., Pazoki-Toroudi, H., and Chen, P. (2024b). CRISPR-microfluidics nexus: advancing biomedical applications for understanding and detection. Sensors Actuators A Phys. 376, 115625. doi:10.1016/j.sna.2024.115625
Razavi, Z., Soltani, M., Pazoki-Toroudi, H., and Dabagh, M. (2024c). Microfluidic systems for modeling digestive cancer: a review of recent progress. Biomed. Phys. and Eng. Express 10, 052002. doi:10.1088/2057-1976/ad6f15
Razavi, Z.-S., Soltani, M., Mahmoudvand, G., Farokhi, S., Karimi-Rouzbahani, A., Farasati-Far, B., et al. (2024a). Advancements in tissue engineering for cardiovascular health: a biomedical engineering perspective. Front. Bioeng. Biotechnol. 12, 1385124. doi:10.3389/fbioe.2024.1385124
Rigato, M., and Fadini, G. P. (2018). Circulating stem/progenitor cells as prognostic biomarkers in macro- and microvascular disease: a narrative review of prospective observational studies. Curr. Med. Chem. 25 (35), 4507–4517. doi:10.2174/0929867324666170920154020
Rosner, M., Horer, S., Feichtinger, M., and Hengstschläger, M. (2023). Multipotent fetal stem cells in reproductive biology research. Stem Cell Res. Ther. 14 (1), 157. doi:10.1186/s13287-023-03379-4
Salybekov, A. A., Kobayashi, S., and Asahara, T. (2022). Characterization of endothelial progenitor cell: past, present, and future. Int. J. Mol. Sci. 23 (14), 7697. doi:10.3390/ijms23147697
Sarmadi, A., Razavi, Z. S., van Wijnen, A. J., and Soltani, M. (2024). Comparative analysis of vision transformers and convolutional neural networks in osteoporosis detection from X-ray images. Sci. Rep. 14 (1), 18007. doi:10.1038/s41598-024-69119-7
Schmitt, T. M., de Pooter, R. F., Gronski, M. A., Cho, S. K., Ohashi, P. S., and Zúñiga-Pflücker, J. C. (2004). Induction of T cell development and establishment of T cell competence from embryonic stem cells differentiated in vitro. Nat. Immunol. 5 (4), 410–417. doi:10.1038/ni1055
Scott, L., Elídóttir, K., Jeevaratnam, K., Jurewicz, I., and Lewis, R. (2022). Electrical stimulation through conductive scaffolds for cardiomyocyte tissue engineering: systematic review and narrative synthesis. Ann. N. Y. Acad. Sci. 1515 (1), 105–119. doi:10.1111/nyas.14812
Shouman, S., Zaher, A., Abdelhameed, A., Elshaboury, S., Sakr, S., Fouda, B. E., et al. (2021). Cardiac progenitor cells. Adv. Exp. Med. Biol. 1312, 51–73. doi:10.1007/5584_2020_594
Simeon, M., Dangwal, S., Sachinidis, A., and Doss, M. X. (2021). Application of the pluripotent stem cells and genomics in cardiovascular research-what we have learnt and not learnt until now. Cells 10 (11), 3112. doi:10.3390/cells10113112
Simon, M. C., Liu, L., Barnhart, B. C., and Young, R. M. (2008). Hypoxia-induced signaling in the cardiovascular system. Annu. Rev. Physiol. 70 (1), 51–71. doi:10.1146/annurev.physiol.70.113006.100526
Smith, T., Rajakaruna, C., Caputo, M., and Emanueli, C. (2015). MicroRNAs in congenital heart disease. Ann. Transl. Med. 3 (21), 333. doi:10.3978/j.issn.2305-5839.2015.12.25
Su, S., and Kang, P. M. (2020). Systemic review of biodegradable nanomaterials in nanomedicine. Nanomaterials 10 (4), 656. doi:10.3390/nano10040656
Taheripak, G., Sabeti, N., Najar, N., Razavi, Z., Saharkhiz, S., and Alipourfard, I. (2024). SIRT1 activation attenuates palmitate induced apoptosis in C2C12 muscle cells. Mol. Biol. Rep. 51 (1), 354. doi:10.1007/s11033-024-09250-w
Takahashi, K., Tanabe, K., Ohnuki, M., Narita, M., Ichisaka, T., Tomoda, K., et al. (2007). Induction of pluripotent stem cells from adult human fibroblasts by defined factors. Cell 131 (5), 861–872. doi:10.1016/j.cell.2007.11.019
Tenekecioglu, E., Farooq, V., Bourantas, C. V., Silva, R. C., Onuma, Y., Yılmaz, M., et al. (2016). Bioresorbable scaffolds: a new paradigm in percutaneous coronary intervention. BMC Cardiovasc. Disord. 16 (1), 38. doi:10.1186/s12872-016-0207-5
Thanaskody, K., Jusop, A. S., Tye, G. J., Wan Kamarul Zaman, W. S., Dass, S. A., and Nordin, F. (2022). MSCs vs. iPSCs: potential in therapeutic applications. Front. Cell Dev. Biol. 10, 1005926. doi:10.3389/fcell.2022.1005926
Trzyna, A., and Banaś-Ząbczyk, A. (2021). Adipose-derived stem cells secretome and its potential application in stem cell-free therapy. Biomolecules 11 (6), 878. doi:10.3390/biom11060878
Tsilimigras, D. I., Oikonomou, E. K., Moris, D., Schizas, D., Economopoulos, K. P., and Mylonas, K. S. (2017). Stem cell therapy for congenital heart disease: a systematic review. Circulation 136 (24), 2373–2385. doi:10.1161/CIRCULATIONAHA.117.029607
Vazin, T., and Freed, W. J. (2010). Human embryonic stem cells: derivation, culture, and differentiation: a review. Restor. Neurol. Neurosci. 28 (4), 589–603. doi:10.3233/RNN-2010-0543
Wang, F., Cai, X., Shen, Y., and Meng, L. (2023). Cell–scaffold interactions in tissue engineering for oral and craniofacial reconstruction. Bioact. Mater. 23, 16–44. doi:10.1016/j.bioactmat.2022.10.029
Wang, H. S., Yi, M. Y., Wu, X., Liu, Q., Deng, Y. H., Wu, T., et al. (2022). Effects of mesenchymal stem cells in renovascular disease of preclinical and clinical studies: a systematic review and meta-analysis. Sci. Rep. 12 (1), 18080. doi:10.1038/s41598-022-23059-2
Wang, X., Han, Z., Yu, Y., Xu, Z., Cai, B., and Yuan, Y. (2019). Potential applications of induced pluripotent stem cells for cardiovascular diseases. Curr. Drug Targets 20 (7), 763–774. doi:10.2174/1389450120666181211164147
Wang, Z., Wang, L., Li, T., Liu, S., Guo, B., Huang, W., et al. (2021). 3D bioprinting in cardiac tissue engineering. Theranostics 11 (16), 7948–7969. doi:10.7150/thno.61621
Williams, M. A. C., Mair, D. B., Lee, W., Lee, E., and Kim, D. H. (2022). Engineering three-dimensional vascularized cardiac tissues. Tissue Eng. Part B Rev. 28 (2), 336–350. doi:10.1089/ten.TEB.2020.0343
Xia, L., Zeng, L., Pan, J., and Ding, Y. (2020). Effects of stem cells on non-ischemic cardiomyopathy: a systematic review and meta-analysis of randomized controlled trials. Cytotherapy 22 (12), 699–711. doi:10.1016/j.jcyt.2020.06.006
Xiao, X., Wang, M., Qiu, X., Ling, W., Chu, X., Huang, Y., et al. (2021). Construction of extracellular matrix-based 3D hydrogel and its effects on cardiomyocytes. Exp. Cell Res. 408 (1), 112843. doi:10.1016/j.yexcr.2021.112843
Xu, C., Police, S., Rao, N., and Carpenter, M. K. (2002). Characterization and enrichment of cardiomyocytes derived from human embryonic stem cells. Circulation Res. 91 (6), 501–508. doi:10.1161/01.res.0000035254.80718.91
Xu, Y., Zhang, F., Zhai, W., Cheng, S., Li, J., and Wang, Y. (2022). Unraveling of advances in 3D-printed polymer-based bone scaffolds. Polymers 14 (3), 566. doi:10.3390/polym14030566
Yan, C., Li, Y. Z., Luo, X. M., Quan, X. J., and Feng, Y. M. (2021). Roles of hematopoietic stem and progenitor cells in ischemic cardiovascular disease. Curr. Stem Cell Res. Ther. 16 (5), 589–598. doi:10.2174/1574888X15666200130091858
Ye, X., and Zhang, C. (2017). Effects of hyperlipidemia and cardiovascular diseases on proliferation, differentiation and homing of mesenchymal stem cells. Curr. Stem Cell Res. Ther. 12 (5), 377–387. doi:10.2174/1574888X12666170316105805
Yeung, E., Fukunishi, T., Bai, Y., Bedja, D., Pitaktong, I., Mattson, G., et al. (2019). Cardiac regeneration using human-induced pluripotent stem cell-derived biomaterial-free 3D-bioprinted cardiac patch in vivo. J. Tissue Eng. Regen. Med. 13 (11), 2031–2039. doi:10.1002/term.2954
Yoshida, Y., and Yamanaka, S. (2017). Induced pluripotent stem cells 10 Years later: for cardiac applications. Circ. Res. 120 (12), 1958–1968. doi:10.1161/CIRCRESAHA.117.311080
Yu, H., Lu, K., Zhu, J., and Wang, J. (2017). Stem cell therapy for ischemic heart diseases. Br. Med. Bull. 121 (1), 135–154. doi:10.1093/bmb/ldw059
Yu, Y., Wang, L., Ni, S., Li, D., Liu, J., Chu, H. Y., et al. (2022). Targeting loop3 of sclerostin preserves its cardiovascular protective action and promotes bone formation. Nat. Commun. 13 (1), 4241. doi:10.1038/s41467-022-31997-8
Zaehres, H., and Schöler, H. R. (2007). Induction of pluripotency: from mouse to human. Cell 131 (5), 834–835. doi:10.1016/j.cell.2007.11.020
Zakrzewski, W., Dobrzyński, M., Szymonowicz, M., and Rybak, Z. (2019). Stem cells: past, present, and future. Stem Cell Res. and Ther. 10 (1), 68. doi:10.1186/s13287-019-1165-5
Zhang, L., Issa Bhaloo, S., Chen, T., Zhou, B., and Xu, Q. (2018). Role of resident stem cells in vessel formation and arteriosclerosis. Circ. Res. 122 (11), 1608–1624. doi:10.1161/CIRCRESAHA.118.313058
Zhao, G., Zhang, X., Lu, T. J., and Xu, F. (2015). Tissue engineering: recent Advances in electrospun nanofibrous Scaffolds for cardiac tissue engineering (Adv. Funct. Mater. 36/2015). Adv. Funct. Mater. 25 (36), 5875. doi:10.1002/adfm.201570241
Zhao, L., Guo, Z., Chen, K., Yang, W., Wan, X., Zeng, P., et al. (2020). Combined transplantation of mesenchymal stem cells and endothelial colony-forming cells accelerates refractory diabetic foot ulcer healing. Stem Cells Int. 2020, 8863649. doi:10.1155/2020/8863649
Zhao, X., Li, Q., Guo, Z., and Li, Z. (2021b). Constructing a cell microenvironment with biomaterial scaffolds for stem cell therapy. Stem Cell Res. and Ther. 12 (1), 583. doi:10.1186/s13287-021-02650-w
Zhao, Y., Hu, J., Sun, X., Yang, K., Yang, L., Kong, L., et al. (2021a). Loss of m6A demethylase ALKBH5 promotes post-ischemic angiogenesis via post transcriptional stabilization of WNT5A. Clin. Transl. Med. 11 (5), e402. doi:10.1002/ctm2.402
Keywords: cardiovascular heart disease, heart failure, stem cell, cell therapy, bio scaffold
Citation: Razavi ZS, Farokhi S, Mahmoudvand G, Karimi-Rouzbahani A, Farasati-Far B, Tahmasebi-Ghorabi S, Pazoki-Toroudi H, Saadat-Fakhr M and Afkhami H (2024) Stem cells and bio scaffolds for the treatment of cardiovascular diseases: new insights. Front. Cell Dev. Biol. 12:1472103. doi: 10.3389/fcell.2024.1472103
Received: 28 July 2024; Accepted: 01 October 2024;
Published: 12 December 2024.
Edited by:
Munemasa Mori, Columbia University, United StatesReviewed by:
Xiaolei Li, University of Pennsylvania, United StatesDivya Sridharan, The Ohio State University, United States
Copyright © 2024 Razavi, Farokhi, Mahmoudvand, Karimi-Rouzbahani, Farasati-Far, Tahmasebi-Ghorabi, Pazoki-Toroudi, Saadat-Fakhr and Afkhami. This is an open-access article distributed under the terms of the Creative Commons Attribution License (CC BY). The use, distribution or reproduction in other forums is permitted, provided the original author(s) and the copyright owner(s) are credited and that the original publication in this journal is cited, in accordance with accepted academic practice. No use, distribution or reproduction is permitted which does not comply with these terms.
*Correspondence: Hamed Afkhami, aGFtZWRhZmtoYW1pNzBAZ21haWwuY29t