- 1Instituto de Medicina y Biología Experimental de Cuyo (IMBECU)-CONICET (Consejo Nacional de Investigaciones Científicas y Técnicas), Argentina
- 2Facultad de Ciencias Médicas, Universidad Nacional de Cuyo, Mendoza, Argentina
- 3Instituto de Histología y Embriología de Mendoza (IHEM) “Dr. Mario H. Burgos”, CONICET (Consejo Nacional de Investigaciones Científicas y Técnicas), Universidad Nacional de Cuyo, Mendoza, Argentina
It has long been thought that exocytosis was driven exclusively by well-studied fusion proteins. Some decades ago, the role of lipids became evident and escalated interest in the field. Our laboratory chose a particular cell to face this issue: the human sperm. What makes this cell special? Sperm, as terminal cells, are characterized by their scarcity of organelles and the complete absence of transcriptional and translational activities. They are specialized for a singular membrane fusion occurrence: the exocytosis of the acrosome. This unique trait makes them invaluable for the study of exocytosis in isolation. We will discuss the lipids’ role in human sperm acrosome exocytosis from various perspectives, with a primary emphasis on our contributions to the field. Sperm cells have a unique lipid composition, very rare and not observed in many cell types, comprising a high content of plasmalogens, long-chain, and very-long-chain polyunsaturated fatty acids that are particular constituents of some sphingolipids. This review endeavors to unravel the impact of membrane lipid composition on the proper functioning of the exocytic pathway in human sperm and how this lipid dynamic influences its fertilizing capability. Evidence from our and other laboratories allowed unveiling the role and importance of multiple lipids that drive exocytosis. This review highlights the role of cholesterol, diacylglycerol, and particular phospholipids like phosphatidic acid, phosphatidylinositol 4,5-bisphosphate, and sphingolipids in driving sperm acrosome exocytosis. Furthermore, we provide a comprehensive overview of the factors and enzymes that regulate lipid turnover during the exocytic course. A more thorough grasp of the role played by lipids transferred from sperm can provide insights into certain causes of male infertility. It may lead to enhancements in diagnosing infertility and techniques like assisted reproductive technology (ART).
1 Introduction
During exocytosis, vesicles within the cell move toward and fuse with the plasma membrane. In constitutive secretion, the vesicles provide proteins and lipids that will become components of the cell membrane. Otherwise, regulated exocytosis is accomplished by an intracellular calcium increase, and vesicles usually contain soluble bioactive molecules to be secreted to the extracellular environment. Neurons and endocrine and neuroendocrine cells are highly specialized in releasing active biological compounds. However, regulated exocytosis is a central issue for fertilization. The oocyte and spermatozoon require exocytosis for successful fertilization to proceed. Fertilization implies a meticulously coordinated series of events, leading to the formation of a zygote (Bhakta et al., 2019; Evans and Florman, 2002). Penetration of the oocyte coat, fusion with its plasma membrane, and ultimately fertilization necessitate the secretion of the special sperm vesicle, named acrosome, resulting in the exposure of sperm membranes required to complete the process (Belmonte et al., 2016; Buffone et al., 2014; Tomes, 2007).
The spermatozoon represents one of the most divergent cell types within the animal kingdom. In mammals, it is the smallest cell in the body and the only cell that performs its function in a different organism than where it was produced. After being formed in the seminiferous tubules of the testicle (spermatogenesis), sperm cells are conducted through the epididymal duct where they mature; once matured, the sperm cells are stored in the epididymal cauda until ejaculation, ultimately navigating through the female reproductive tract to reach the site of fertilization. The spermatozoon’s journey exposes it to numerous environmental, physical, and chemical barriers, along with selective pressures, which have driven its evolution toward deep morphological and functional specialization (Inaba, 2011). Therefore, the spermatozoon is a terminally differentiated cell characterized by its highly polarized structure. It contains a head and a tail or flagellum. The head exhibits distinct regions, namely, the acrosomal and postacrosomal regions, divided by the equatorial segment, with the nucleus and acrosome enclosed. The acrosome is a large granule that covers approximately half of the nucleus in the human sperm (Florman et al., 2008). The flagellum of mature sperm cells is in charge of cell movement, and it represents approximately 90% of the total length and contains the axoneme. This central structure runs throughout its entire length, starting at the neck region and ending at the terminal piece. It comprises a 9 + 2 arrangement of microtubules, sheath proteins, and mitochondria, ordered spirally only in the midpiece. In the following section, the principal piece, the mitochondrial sheath is absent and is replaced by a fibrous sheath. The terminal piece comprises a naked axoneme, and the microtubules end at different levels. The head and the tail are connected at the neck, enveloped by the plasma membrane with minimal cytoplasm inside. Sperm cells lack the capacity for protein or nucleic acid synthesis, and they have the sole purpose of locating, merging with, and transmitting genetic material to the oocyte for fertilization (Yanagimachi, 1994) (Figure 1).
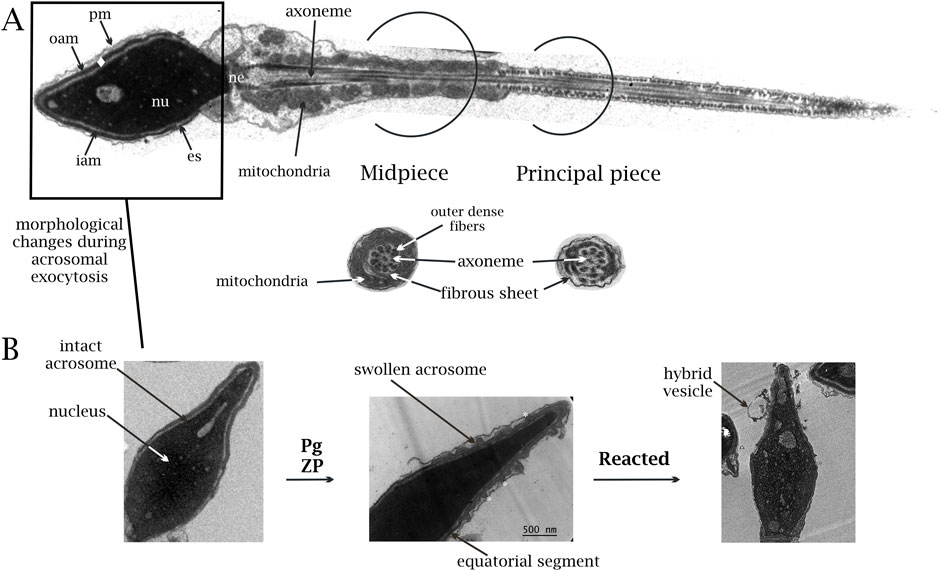
Figure 1. (A) Transmission electron micrographs of human sperm cells (scale 500 nm). Normal ultrastructural pattern of a human spermatozoon, showing the nucleus (nu), acrosome (♦), equatorial segment (es), neck (ne), plasma membrane (pm), and outer and inner acrosomal membranes (OAM and IAM, respectively). The cytoskeletal structures at the principal and middle pieces are shown (axoneme, mitochondria, outer dense fibers, and fibrous sheet). The tail’s terminal piece is not shown (given that the cut section did not reach it). (B) Ultrastructural changes of the human sperm head. Electron micrographs shown in I–III illustrate different stages of the acrosome exocytosis. I. Sperm cell with an intact acrosome. II. Changes occurring upon receiving different stimuli, such as zona pellucida (ZP) or progesterone (Pg). A swollen acrosome with a waving outer acrosomal membrane (*) shows where membrane apposition occurs. III. Last stage (reacted). A sperm cell that has completed the acrosome reaction. Notice that the equatorial segment remains unaltered during the acrosome exocytosis. At the end of the reaction, the continuity between the plasma membrane and the outer acrosomal membrane is established, and the inner acrosomal membrane becomes part of the limiting membrane of the cell. A noticeable hybrid vesicle is still attached to the head.
The acrosome is an atypical secretory vesicle that goes through regulated exocytosis when stimulated by physiological inducers like progesterone or zona pellucida glycoproteins. The acrosomal membrane is partitioned into distinct segments, each serving unique roles. The segment positioned over the nucleus is dubbed the inner acrosomal membrane (IAM), while the region beneath the plasma membrane is termed the outer acrosomal membrane (OAM). It contains soluble acid hydrolases and other enzymes immersed in a dense protein matrix that makes it electron-opaque (Buffone et al, 2008) (Figure 1).
Millions of sperm ascend the uterus upon ejaculation in the female genital tract. However, only a few pass through the oviduct to reach the ampulla, where fertilization proceeds. This journey involves capacitation, a process comprising physiological, biophysical, and molecular changes, enabling the sperm to fertilize oocytes. Capacitated sperm acquire hyperactivated motility and the ability to release the acrosome content in the presence of physiological stimuli (Cohen et al., 2004; Gadella et al., 2008; Puga Molina et al., 2017; Stival et al., 2016; Visconti, 2009). The exocytosis of the sperm acrosome, known as acrosome reaction (AR), is modulated by Ca2+ and is necessary for oocyte fertilization (Belmonte et al., 2016; Buffone et al., 2014; Kirkman-Brown et al., 2002; Lishko et al., 2011; Michaut et al., 2000; Suhaiman et al., 2021; Suhaiman et al., 2010; Tomes, 2007; Trevino et al., 2006). The AR initiates a deep membrane rearrangement within the sperm head, and the acrosome undergoes striking morphological changes. In quiescent sperm, the acrosome typically exhibits dense electron content, positioned closely and parallel to the plasma membrane. The interaction between the OAM and PM occurs only under sperm activation upon stimulation by calcium ionophore or progesterone. Therefore, approximately 30%–40% of sperm display morphologically altered acrosomes (Sosa et al., 2015; Sosa et al., 2016; Zanetti and Mayorga, 2009). The acrosomes undergo swelling and waving on the OAM surface, which makes contact with the PM at multiple points, forming thousands of fusion pores and deep invaginations. A subset of cells demonstrates vesiculated or lost acrosomes, which is indicative of a reacted state. The AR is strongly regulated and irreversible. After the acrosome releases its contents, the cell undergoes a complete modification of its membrane components, exposing the IAM to the extracellular environment becoming the limiting membrane of the sperm while preserving the equatorial segment (Figure 1).
The AR involves the exocytosis of a huge granule with a dense matrix; therefore, whereas secretion in neurons and neuroendocrine cells is a quick process, the acrosome reaction requires several minutes to proceed after challenging sperm with different stimuli. During AR, Ca2+ increases with fast kinetics but the swelling of the acrosome preceding exocytosis is a slow process (t1/2 = 13 min). When swelling finishes, the fusion pore opening is fast. Acrosome swelling is the slowest step, and it defines the kinetics of acrosome secretion (Sosa et al., 2015; Sosa et al., 2016).
2 Unique lipids: the remarkable composition of human sperm membranes
The lipid composition of the sperm plasma membrane has been determined for different mammal species [for a review, see Gautier and Aurich (2022); Shan et al. (2021); Yanagimachi (1994). There are some differences between them, but in general, it is known that 70% are phospholipids, 25% are neutral lipids, and 5% are glycolipids (on molar base) of the total sperm plasma membrane lipids. Glycerophospholipids (GPLs) are the most representative molecules in the phospholipid fraction, with phosphatidylcholine and phosphatidylethanolamine being predominant. Notably, a significant proportion of these phospholipids comprises plasmalogens, which contain one fatty acid esterified with glycerol and an alkyl or alkenyl moiety forming an ether bond constituting two types of ether phospholipids, namely, plasmanyl (alkyl moiety at sn-1) and plasmenyl (alkenyl moiety with vinyl ether linkage at sn-1) (Tapia et al., 2012). Particularly, sperm membrane lipids contain a high proportion of polyunsaturated fatty acids such as arachidonic (20:4), docosapentaenoic (22:5), and docosahexaenoic acid (22:6) (Henkel, 2011). Another characteristic that distinguishes sperm membranes from somatic cell membranes is the presence of very long polyunsaturated fatty acids (VLC-PUFAs) within sphingolipids (Furland et al., 2007a; Furland et al., 2007b; Oresti et al., 2011). Remarkably, these polyenoic acyl moieties were of considerable length, ranging from 28 to 34 carbon atoms with 4–6 double bonds. The amount of double bonds makes sperm especially vulnerable to lipid peroxidation by reactive oxygen species (ROS) (Aitken et al., 2007). Regarding neutral lipids, sperm membranes mainly contain diacylglycerol (DAG) and cholesterol. Common glycolipid synthesis involves a glycosidic headgroup binding to ceramide (Sandhoff and Kolter, 2003). Still, in sperm, there is one notable exception: seminolipid, also known as the sulfogalactosylglycerolipid (SGG). This unique glycolipid, featuring a sulfo-galactosyl glycerol structure, is exclusively found in sperm membranes. It plays a crucial role in various stages of sperm development and function (Di Nisio et al., 2023; Gadella et al., 1995; Tanphaichitr et al., 2018). The main lipids building sperm membranes are summarized in Table 1 (Henkel, 2011; Sanocka and Kurpisz, 2004; Zalata et al., 2010; Zalata et al., 1998a; Zalata et al., 1998b).
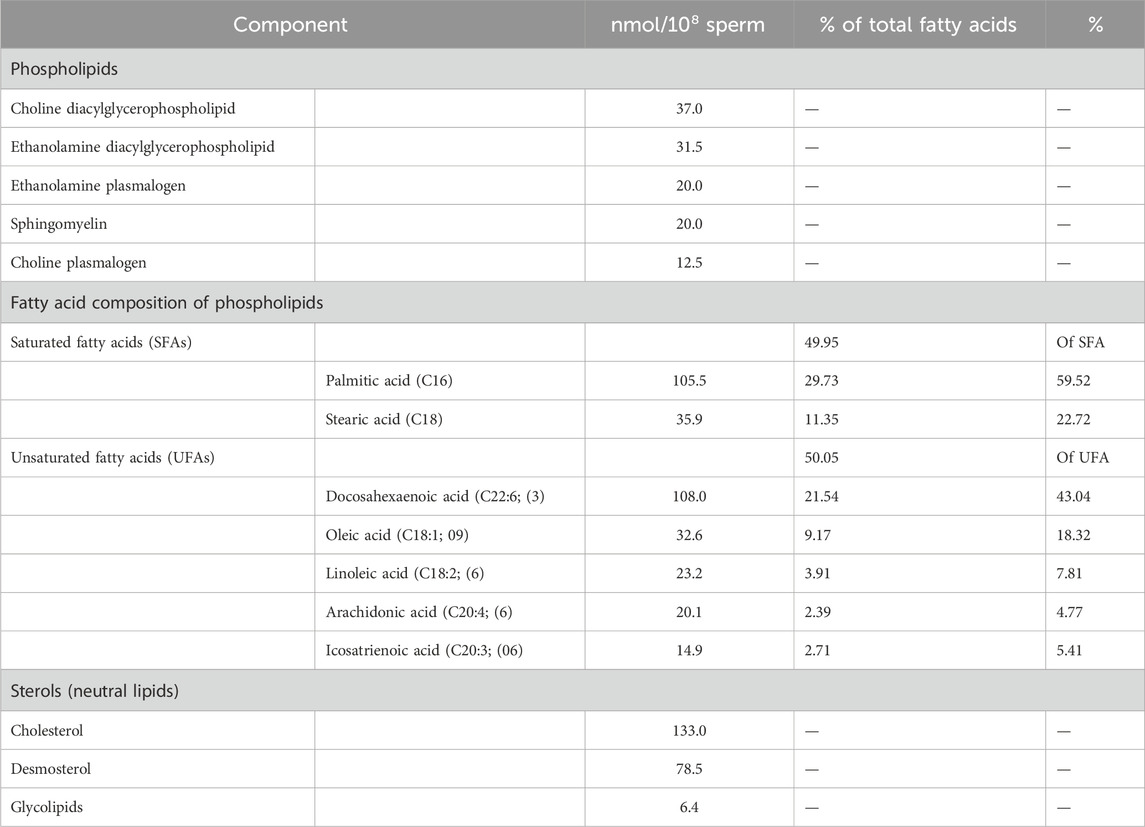
Table 1. Main lipids of human sperm membranes (Henkel, 2011; Sanocka and Kurpisz, 2004; Zalata et al., 2010; Zalata et al., 1998a).
Due to the absence of most cellular organelles and the DNA transcription machinery, as far as we know, sperm cells cannot synthesize lipids de novo. However, they possess an active and highly regulated system that keeps a dynamic refurbishing of sperm membranes adapting them for different functions throughout their lifespan. Membrane lipid remodeling occurs during epididymal sperm maturation (Dacheux and Dacheux, 2014; Flesch and Gadella, 2000; Sosnicki et al., 2023), capacitation (Asano et al., 2013b; Visconti, 2009), and the AR (Cheng et al., 2023; Lopez et al., 2012; Pelletan et al., 2015; Vaquer et al., 2020).
3 Cholesterol dynamics during acrosome exocytosis
Cholesterol is an abundant neutral lipid in sperm cells (Table 1) that plays a crucial role in modulating membrane properties during acrosome exocytosis. It is primarily synthesized by Sertoli cells in the testes and transferred by different mechanisms to spermatogenic cells (Shi et al., 2018). Cholesterol is distributed asymmetrically between the outer and inner leaflets of the plasma membrane. Typically, there is a slight enrichment of cholesterol in the outer leaflet compared to the inner leaflet (Ingolfsson et al., 2014). This asymmetry is crucial for maintaining the membrane’s structural integrity and functionality. Cholesterol can flip-flop between the inner and outer leaflets of the plasma membrane without the assistance of a transporter. However, this process is relatively slow compared to its lateral diffusion within the same leaflet. Transporter proteins, like ATP-binding cassette transporters, can facilitate and speed up this process, ensuring efficient cholesterol distribution across the membrane (Ogasawara and Ueda, 2022).
This sterol acts by influencing membrane fluidity, modulating the function of ion channels and key fusion proteins, and organizing lipid microdomains.
Due to deep lipid changes in the sperm plasma membrane accomplished during capacitation, the membranes become fusogenic and reactive to progesterone and zona pellucida glycoproteins (Harrison and Gadella, 2005; Spungin et al., 1992). Capacitation is a multifaceted and complex process within the female reproductive tract (Matamoros-Volante and Trevino, 2020; Orta et al., 2018; Stival et al., 2016; Visconti, 2009). Cholesterol loss is a hallmark of sperm capacitation, and a variety of functions have been assigned to this event that actively influences the function of the membrane during the AR. Cholesterol depletion starts shortly after sperm separation from seminal plasma. During the sperm transit in the female genital tract, cholesterol efflux occurs due to the presence of cholesterol acceptors such as high-density lipoproteins and albumin in the oviductal and follicular fluids (Bernecic et al., 2021; Bernecic et al., 2020; Ehrenwald et al., 1990; Leahy and Gadella, 2015). Sperm cells leap from an albumin concentration of 1 mg/mL (5 μM) in the semen (Elzanaty et al., 2007) to 34 mg/mL (500 μM) in the uterine fluid (Casslen and Nilsson, 1984). The human tubal fluid composition is usually mimicked in an in vitro-defined media to capacitate sperm artificially, and serum albumin is used as a cholesterol acceptor. During this process, the cholesterol content can decrease by up to 40% (Belmonte et al., 2005; Flesch et al., 2001; Flesch and Gadella, 2000). A direct consequence of cholesterol removal is that the plasma membrane fluidity of sperm cells increases and lateral redistribution of the sterol occurs toward the apical edge of the sperm head. These shifts in sperm lipid dynamics take place before the initiation of the AR (Companyo et al., 2007; Iborra et al., 2000; Leahy and Gadella, 2015).
Albumin has long been utilized as a cholesterol acceptor in sperm culture and in vitro fertilization (IVF) media (Balaban et al., 2014); however, Zhao et al. (2021) demonstrated that in addition to having this function albumin binds to the human sperm proton channel, hHv1, activating it by interacting with the voltage sensor domains (VSDs). Its opening decreases the H+ concentration inside human sperm, inducing alkalization required for the capacitation and consequently the AR (Zhao et al., 2021) (Figure 2).
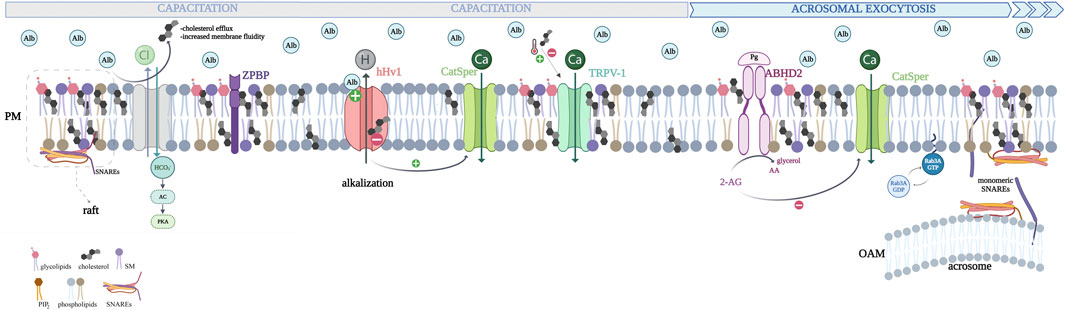
Figure 2. Schematic representation of the human sperm plasma membrane and changes induced by cholesterol efflux during capacitation and acrosome exocytosis. Major changes occur during capacitation, including increases in intracellular pH and stimulation of cAMP-dependent protein kinase (PKA). Cholesterol depletion is mediated by albumin in the female genital tract fluids (Alb, light blue circles). Albumin also binds to the human sperm proton channel, hHv1, activating it by interacting with its voltage sensor domains (VSDs). Its opening decreases the H+ concentration inside the human sperm, provoking alkalization required for the capacitation and consequently the AR. Cholesterol blocks the hHv1 channel function by stabilizing the voltage-sensing S4 at resting conformations. Thus, cholesterol efflux releases the channel from its inhibitory effect, favoring cytoplasmic alkalization. Cholesterol-depleted membranes become prone to facilitate sperm acrosome exocytosis via different complementary pathways. Cholesterol inhibits TRPV1 (transient receptor potential channel-vanilloid receptor 1) by interacting with an amino acid consensus sequence, which is known as the cholesterol recognition amino acid consensus (CRAC); on the other hand, it has been shown that mild temperatures activate this channel (thermotaxis). Cholesterol efflux allows the proper motility of sperm. Progesterone binds to and activates ABHD2 (progesterone receptor: α/β hydrolase domain-containing protein 2), a lipid hydrolase that cleaves the 2-AG (endocannabinoid 2- arachidonoylglycerol), which is a natural inhibitor of sperm CatSper (cation channel of the sperm). ABHD2 activation hydrolyzes 2-AG releasing glycerol and arachidonic acid (AA), opening CatSper and increasing [Ca2+]i. Recently, a computational-based approach suggested that cholesterol interacts directly with ABHD2 impairing progesterone-mediated sperm function through possible hydrolase inhibition. Cholesterol efflux allows the proper function of the receptor. Sphingolipids and cholesterol cluster in dynamic nanoscale domains embedded inside the plasma membrane phospholipid bilayer (lipid rafts). ZP-binding proteins are aggregated in the lipid-ordered fraction, as well as TRPV1 and ABHD2. Members of the SNARE family are concentrated in cholesterol-dependent domains: SNAP25 and syntaxin clusters. Certain molecules, such as isoprenylated proteins like the small GTPases, Rabs, tend to avoid lipid rafts and are generally excluded from these regions. The sterol content regulates its attachment to the membrane. When cholesterol levels decrease, active Rab3A can incorporate its geranylgeranyl groups into sperm membranes, recruiting effectors and initiating the tethering of the OAM to the plasma membrane. In summary, cholesterol efflux enhances fertilization competence by promoting the conditions necessary for the effective assembly of fusion machinery, leading to acrosome exocytosis.
As stated before, cholesterol also plays a role in regulating channels and protein activity. Next, we will examine this function in sperm. Dr. Travis’s group demonstrated that the cholesterol efflux and focal enrichment of the ganglioside GM1 induce murine sperm calcium influx through the CaV2.3 channel (Cohen et al., 2014), triggering calcium transients required for AR [reviewed in (Cohen et al., 2016)]. The cholesterol decrease also results in proteolytic activation by a furin-class protease of phospholipase B (PLB) that hydrolyzes both fatty acids from phospholipids. The products may alter membrane curvature, favoring membrane fusion during physiologically induced AR in mouse sperm (Asano et al., 2013a; Asano et al., 2013b).
Recently, Han et al. (2022) demonstrated that cholesterol hinders the hHv1 channel function by stabilizing the voltage-sensing S4 at resting conformations. Therefore, cholesterol efflux releases the channel from its inhibitory effect, inducing human sperm cytoplasmic alkalization (Han et al., 2022). Furthermore, these authors showed that arachidonic acid reverses cholesterol inhibition (Han et al., 2023).
Moreover, the transient receptor potential channel-vanilloid receptor 1 (TRPV1) regulates, partly, sperm-oriented motility to reach the oocyte (Bahat et al., 2003). De Toni et al. (2021) demonstrated that in human sperm, cholesterol inhibits TRPV1 by interacting with an amino acid consensus sequence, known as the cholesterol recognition amino acid consensus (CRAC), present in the channel S5 helix domain (Picazo-Juarez et al., 2011).
Recently, the same group described the mechanism by which membrane cholesterol regulates progesterone-mediated sperm activation. Membrane cholesterol levels are linearly and inversely correlated with the progesterone-induced effects in human sperm cells, such as increases in [Ca2+]i, chemotaxis, motility changes, and AR. Progesterone binds to and activates ABHD2 (progesterone receptor: α/β hydrolase domain-containing protein 2), a lipid hydrolase that cleaves the endocannabinoid 2-arachidonoylglycerol (2-AG), releasing glycerol and arachidonic acid (AA) and increasing [Ca2+]i (Miller et al., 2016). The 2-AG is a natural inhibitor of sperm CatSper (cation channel of sperm). CatSper mediates hyperactivated motility during capacitation (Lishko et al., 2011), and the calcium current it produces is required for the AR (Vaquer et al., 2023; Vaquer et al., 2020). A computational-based approach suggested that cholesterol interacts directly with ABHD2 impairing progesterone-mediated sperm function through possible hydrolase inhibition. Molecular dynamic analysis indicates that cholesterol binding affects the conformational freedom of key amino acids involved in the binding of the enzyme substrates (De Toni et al., 2023) (Figure 2). Cholesterol efflux allows the proper function of the progesterone receptor.
We will discuss another function of cholesterol: its role in forming microdomains, which may influence the localization of exocytosis-related proteins by determining the most suitable membrane regions for their activity. Sphingolipids and cholesterol clusters in dynamic nanoscale domains embedded within the phospholipid bilayer (lipid rafts) (Brown and Rose, 1992; de Almeida et al., 2003). Lipid rafts demonstrate less fluidity than the neighboring membrane (Calder & Yaqoob, 2007; Lingwood et al, 2009). Since 2001, biochemical evidence and high-resolution imaging support a direct role for cholesterol in exocytosis, given that members of the SNAREs (soluble N-ethylmaleimide-sensitive factor attachment protein receptors), like syntaxin 1 and synaptosome-associated protein 25 (SNAP25), are concentrated in cholesterol-dependent domains, known as SNAP25 and syntaxin clusters (Chamberlain et al., 2001; Lang, 2007; Lang et al., 2001; Weisgerber et al., 2024). The presence of SNAREs in lipid rafts concentrates these proteins at precise plasma membrane zones. R-SNAREs like synaptobrevin (VAMP) and Q-SNAREs like syntaxin, as well as the Ca2+ sensor protein synaptotagmin, are enriched in sperm-derived lipid rafts from different mammal species, including humans (Gadella et al., 2008; Gamboa and Ramalho-Santos, 2005; Lv et al., 2008; Nixon and Aitken, 2009; Nixon et al., 2011). The complementary SNARE proteins, syntaxin, and VAMP have been localized to the apical region of the mouse sperm head (Brahmaraju et al., 2004; Tsai et al., 2007; Zitranski et al., 2010) and sperm cells of other species. In murine and guinea sperm cells, Syntaxin 2 can be found in both raft and non-raft fractions (Asano et al., 2009; Selvaraj et al., 2006; Travis et al., 2001). Importantly, molecules involved in sperm-zona pellucida binding have been recovered from detergent-resistant membrane preparations (DRM) obtained from capacitated sperm (Zitranski et al., 2010). The sperm SNARE proteins, including VAMP from the outer acrosomal membrane and syntaxin from the apical plasma membrane of the sperm head, exhibited similar lateral redistribution characteristics during capacitation. The same redistribution pattern was also observed in the ZP-binding protein complex and raft marker proteins (Boerke et al., 2008). Interestingly, sperm SNAREs and ZP-binding proteins are aggregated in the same lipid-ordered fraction of the sperm head, and this is relevant for ZP-binding and ZP-induced AR (Zitranski et al., 2010) (Figure 2).
Due to the physical properties of cholesterol microdomains, proteins can distribute themselves among various membrane regions. Certain molecules, such as isoprenylated proteins like the small GTPases, Rabs, tend to avoid lipid rafts and are generally excluded from these regions (Pyenta et al., 2001). The effect of cholesterol efflux has been extensively studied during capacitation. Our group proposed that cholesterol might directly influence the AR. To investigate this, we induced acute cholesterol depletion in capacitated human sperm just before stimulating them with progesterone. This cholesterol removal resulted in the increased occurrence of the AR. Cholesterol seems to affect an early step of the exocytic cascade rather than the mixing of lipid bilayers. One specific target of cholesterol’s effect is Rab3A. The sterol content does not influence the activation–deactivation cycle of Rab3A but regulates its membrane attachment. Our results suggest that initially, sperm SNAREs concentrate in lipid rafts, while Rab3A remains in the cytosol. However, when cholesterol levels decrease, active Rab3A can incorporate its geranylgeranyl groups into sperm membranes, recruiting effectors and initiating the tethering of OAM to the plasma membrane. In summary, cholesterol efflux enhances fertilization competence, by promoting the conditions necessary for the effective assembly of fusion machinery, leading to acrosome exocytosis (Belmonte et al., 2005) (Figure 2). Thus, cholesterol is a critical regulator of sperm AR.
4 Bioactive synergy: the power of sphingolipids in exocytosis
Sphingolipids represent ∼10–20% of total membrane lipids (Bartke and Hannun, 2009). Sphingolipid’s de novo synthesis pathway starts exclusively with the activity of a serine palmitoyl transferase (SPT) from serine and palmitate which forms the first sphingolipid, ceramide. This last sphingolipid can further be synthesized via the cleavage of sphingomyelin (SM) as a result of the sphingomyelinase (SMase) activity (SM pathway). The metabolism ends with S1P lyase, which degrades sphingosine-1-phosphate (S1P) into non-sphingolipid molecules. De novo synthesis of sphingolipids occurs in the endoplasmic reticulum [for a review, see Gault et al. (2010); Hannun and Obeid (2008)]. The intermediary steps form a complex network connecting various sphingolipids. In this network, ceramide acts as a central core, given that it is the only one synthesized de novo and can originate all the sphingolipids of the pathway (Petrache et al., 2013; Silva et al., 2012; Sridevi et al., 2009). The sphingolipids ceramide, S1P, and ceramide 1-phosphate (C1P) are critical signaling molecules regulating numerous physiological functions such as exocytosis [reviewed in Gomez-Munoz et al. (2016)].
A defining trait of the plasma membrane (PM) in mammalian cells is the asymmetric distribution of lipids across the bilayer (Doktorova et al., 2020). Sphingomyelin (SM) is the main sphingolipid in the outer leaflet and is present in membrane nanodomains alongside cholesterol. SM has two aliphatic chains and a zwitterionic headgroup. Hence, it only occasionally flip-flops across bilayers. However, Abe et al. showed that SM might move from the outer leaflet to the inner leaflet of the PM by a mechanism involving a peripheral myelin protein 2 (PMP2), which is a phosphatidylinositol 4,5-bisphosphate (PIP2)-binding protein. They showed that PMP2 provokes the tubulation of membranes in a PIP2-dependent manner, coupled with changes in sphingomyelin transbilayer localization (Abe et al., 2021). In secretory cells, SM is considered mostly a structural lipid that can release metabolites considering highly bioactive molecules (Gafurova et al., 2024; Tsentsevitsky et al., 2023).
Focusing on the sperm of different species, during the steady state, Xenopus, bull, ram, rat, and boar sperm exhibit notably high levels of SM (approximately 12–20 mol%) (Stith, 2015). However, in rodents and boar sperm, SMase activity increases just before fertilization hydrolyzing SM, mainly containing VLC-PUFA, to produce ceramides during the AR, with a consistent SM decrease (Ahumada-Gutierrez et al., 2019; Nikolopoulou et al., 1986a; Nikolopoulou et al., 1986b; Penalva et al., 2018; Petcoff et al., 2008; Zanetti et al., 2010a; Zanetti et al., 2010b). Cross (2000) reported that exogenous SMase accelerates capacitation by promoting sterol loss and ceramide production in human sperm. As a result, sperm become responsive to progesterone stimuli, releasing the acrosome content. The sperm SM likely serves a primarily structural role and acts as a source of ceramide that exerts its specific biological activity (Figure 3). Murase et al. (2004) reported that ceramide increased the AR induced by calcium ionophore in boar sperm cells. Given that the effect of ceramides on secretory cell exocytosis is controversial (Abousalham et al., 1997; Ji et al., 2011; Mansfield et al., 2004; Tang et al., 2007; Won et al., 2018) and the molecular mechanism has not been fully explained, our group focused on the role of ceramide during the AR. Ceramide contains two aliphatic chains and a neutral headgroup, enabling it to flip-flop within membranes. However, for ceramide to move between different membranes, it requires the presence of vesicles or specific transfer proteins. The ceramide transfer protein (CERT) plays a crucial role in transporting ceramide from its synthesis site to the required location (Hannun and Obeid, 2008). Vaquer et al. (2020) provided a comprehensive exploration of the molecular mechanisms initiated by ceramide during acrosome exocytosis. The study identifies and localizes key sphingolipid metabolism enzymes—such as nSMase, ceramide synthase, and neutral ceramidase—in human sperm, highlighting both basal and regulated ceramidase activity during the AR (Vaquer et al., 2020). The neutral ceramidase degrades ceramide into sphingosine and free fatty acids. It is localized to the plasma membrane and the endoplasmic reticulum membrane in somatic cells, where it functions optimally at a neutral pH. Endogenous and exogenous ceramide leads to a rapid and early rise in intracellular calcium levels involving at least three channels: CatSper channels, ryanodine receptors (RyR), and store-operated calcium channels (SOCCs). This initial calcium wave activates a PLC, which hydrolyzes PIP2 into inositol 3-phosphate (IP3) and DAG. IP3 then promotes the release of intracellular calcium stores by activating IP3-sensitive calcium channels (IP3R), leading to prolonged SOCC opening and sustained calcium augment, which drives the acrosome exocytosis.
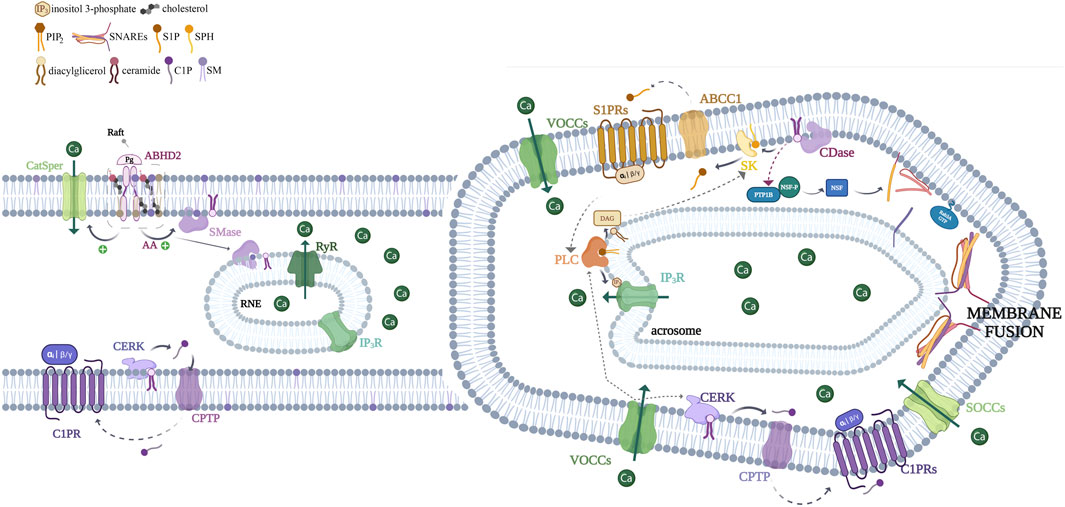
Figure 3. Schematic representation depicting the role of sphingolipids in acrosome exocytosis. We present here a working model hypothesized for the sphingolipid pathway involved in the acrosome exocytosis. Progesterone interacts with its receptor (α/β hydrolase domain-containing protein 2, ABHD2) to trigger lipid hydrolysis, leading to the generation of arachidonic acid (AA), an activator of neutral sphingomyelinase (nSMase). In turn, this enzyme hydrolyzes sphingomyelin to produce ceramide. However, it remains possible that nSMase triggers the pathway, and due to that, it might also be activated by other stimuli (AA from other sources, reactive oxygen species, and anionic phospholipids) (Airola and Hannun, 2013). As already known, the activation of ABHD2 leads to the stimulation of the sperm cation channel (CatSper). This occurs because ABHD2’s lipid hydrolase activity, associated with the progesterone receptor, cleaves the endocannabinoid 2-arachidonoylglycerol (2-AG), thereby releasing CatSper from its inhibition. This allows the calcium current through CatSper, which is required for hyperactivated motility during capacitation and later for the AR. Endogenous ceramide exerts various effects, for example, it activates CatSper and ryanodine sensitive-calcium channels (RyR) located in the redundant nuclear envelope (RNE), contributing to calcium mobilization toward the sperm head. On the other hand, the first calcium wave activates ceramide kinase (CERK), leading to the C1P synthesis. Given that C1P-induced AR requires CatSper activity, we suppose that the sphingolipid can set off CatSper via an unknown, alternative mechanism or by using the same machinery utilized by Pg. The last possibility makes sense due to the CERK activity requirement for Pg-triggered exocytosis. The CERK phosphorylates ceramide to C1P, which can be transported through the plasma membrane to the extracellular environment by a C1P transfer protein (CPTP). Extracellular C1P interacts with a Gi-coupled receptor (C1PR) activating VOC channels and a heterotrimeric Gi-protein. In the same manner, S1P is generated by the phosphorylation of sphingosine by the action of sphingosine kinase 1 (SK1). The S1P is probably transported to the extracellular space by the ATP-binding cassette family member of transporters named ABCC1, where it interacts with a Gi-coupled receptor (S1PR). Both GPCR pathways lead to calcium increase and the heterotrimeric Gi-protein switch on a PLC, which hydrolyzes phosphatidylinositol 4,5-bisphosphate (PIP2) producing DAG and IP3. The last one binds to IP3-sensitive calcium channels, which is present on the outer acrosomal and redundant nuclear envelope (RNE) membranes, inducing calcium efflux from the reservoirs. The voiding of the stores triggers the opening of SOCCs at the plasma membrane, allowing a sustained calcium increase. On the other hand, ceramide activates a signal transduction cascade involving the activity of protein tyrosine phosphatase 1B (PTP1B), which dephosphorylates NSF (N-ethylmaleimide-sensitive factor) and elicits the SNARE (SNAP receptor) complex disassembly during human sperm exocytosis. Both a local increase in calcium coming from IP3-sensitive channels and SNAREs converge to accomplish the final steps of membrane fusion. The SK/S1P pathway seems to follow a pathway independent of an acute ceramide increase.
Although ceramide synthase (CerS, Lass) is found in the acrosomal region of human sperm (Vaquer et al., 2020), we do not believe that ceramides can be synthesized “de novo” in a terminal cell lacking the endoplasmic reticulum, where this process occurs in somatic cells. Instead, evidence suggests that the increase in ceramides during the AR is due to the activity of nSMases present in the inner leaflet of the plasma membrane (Vaquer et al., 2020).
Ceramide increases in human sperm activate protein tyrosine phosphatase 1B (PTP1B), resulting in the dephosphorylation of the N-ethylmaleimide-sensitive factor (NSF) and subsequent disassembly of the SNARE complex. The study also reveals that both progesterone and ceramide activate PTP1B and necessitate VAMP2 for the AR, so both pathways converge in the final steps of membrane fusion. This study provides compelling evidence for the critical pathways regulated by ceramide during sperm acrosome secretion, clarifying the debated role of this lipid in exocytosis signaling pathways (Vaquer et al., 2020).
Due to the sperm sphingolipid metabolism appearing very active and the ability of ceramide to be converted into all sphingolipids in the pathway, the acute ceramide increase might be exerted by itself or by its generated products. Among the molecules with biological activities related to exocytosis, S1P and C1P are the most attractive candidates. They are bioactive lipids, meaning that, slight concentration changes can have significant functional consequences. S1P and C1P, far from structural lipids, are present in minimal concentrations, undergo rapid turnover, and achieve signaling function. S1P has a zwitterionic headgroup, including a charged phosphate, and is likely to move freely among membranes but is unlikely to flip-flop spontaneously. S1P acts as an intracellular second messenger or extracellularly by binding to its receptors. S1P interacts with five G-protein coupled receptors (S1PR1-5) that belong to the lysophospholipid receptor family (Spiegel and Milstien, 2003).
Our laboratory has made significant strides in understanding the S1P signaling mechanisms underlying human sperm acrosome exocytosis. S1P induces the AR in human sperm by activating a Gi-coupled receptor (Suhaiman et al., 2010), leading to [Ca2+]i increase, driven by extracellular ion influx through VOCCs and SOCCs, as well as release from intracellular stores via IP3Rs. The pathway’s efficacy relies on the activity of phospholipase C (PLC), protein kinase C (PKC), and the small GTPase Rab3A, which inserts into sperm membranes recruiting effectors to initiate membrane fusion. Furthermore, we demonstrated the presence and activity of sphingosine kinase 1 (SK1) in human sperm cells. DAG and its analog, the phorbol ester PMA, induce SK1 translocation/activation from the cytosol to the membrane and S1P synthesis. Importantly, exocytosis induced by PMA is dependent on SK1 activity. Our findings reveal that PMA stimulates S1P synthesis, which can be transported extracellularly, indicating the existence of an S1P transporter. The extracellular S1P then binds to specific Gi-coupled receptors, triggering the signaling cascade which results in AR. Thus, S1P can induce the AR through an autocrine/paracrine effect (Belmonte and Suhaiman, 2012; Suhaiman et al., 2010). Our work highlights the crucial role of the SK1/S1P/S1PR signaling pathway in regulating acrosome secretion, as depicted in Figure 3.
Since ceramide stimulates the AR (Vaquer et al., 2020) and is a near-direct precursor of S1P, which is synthesized by human sperm cells inducing acrosome secretion (Suhaiman et al., 2010), we hypothesized that an acute rise in ceramide could exert its effect on exocytosis through the synthesis of S1P. However, an increase in ceramide does not provoke S1P synthesis in sperm cells and induces exocytosis in an S1P-independent manner (Vaquer et al., 2023). Instead, the rise in ceramide leads to the production of C1P (Vaquer et al., 2023). C1P carries ionic charges at neutral pH and has two hydrophobic chains. It likely remains in its synthesis compartment and is unlikely to flip spontaneously across bilayers. The C1P synthesis in human sperm implies the presence of CERK. Our group demonstrated the existence of an active CERK in human sperm, further showing that its activity is regulated by calcium. C1P induces exocytosis in capacitated human sperm cells, causing a rise in [Ca2+]i that involves calcium influx from the extracellular medium and calcium efflux from internal reservoirs. Ceramide induces the AR, primarily due to C1P production. Notably, progesterone required the activity of CERK to evoke a [Ca2+]i increase and acrosome exocytosis. Our research is pioneering in describing the molecular mechanism triggered by the bioactive sphingolipid C1P in the physiological progesterone pathway that triggers membrane fusion during sperm AR. In conclusion, the physiological activity of progesterone in human sperm depends on ceramide (Vaquer et al., 2020) and C1P (Vaquer et al., 2023) but is S1P-independent (Suhaiman et al., 2010) (Figure 3).
In light of the findings discussed, we propose a mechanism in which C1P interacts with a C1P receptor in the human sperm plasma membrane, inducing signaling. C1P is produced intracellularly as CERK catalyzes ceramide phosphorylation in a calcium-dependent manner within the sperm. The bioactive sphingolipid can be transported to the extracellular medium by a C1P transfer protein (CPTP) (Presa et al., 2020). It may then bind to a putative Gi protein-coupled receptor. Despite the partial characterization of a potential C1P receptor, the receptor has not yet been cloned or isolated (Arana et al., 2013; Ouro et al., 2013). We suggest that the progesterone present in the female tubal fluid induces a calcium influx through CatSper by activating α/β hydrolase domain-containing protein 2 (ABHD2) (Miller et al., 2016). This increase in calcium stimulates the CERK in human sperm cells to produce C1P, triggering the mechanism described (Figure 3).
We hypothesize that the physiological stimulus eliciting S1P signaling is the oocyte zona pellucida and that it should be produced from ceramide. An acute rise in ceramide induces C1P synthesis, whereas progesterone requires both ceramide and C1P—but may bypass the S1P pathway—to trigger acrosome exocytosis.
In conclusion, sphingolipids play a pivotal role in membrane remodeling during membrane fusion, and maintaining a precise balance of these lipids in human sperm is essential for successful fertilization.
5 Diacylglycerol (DAG)
Phosphoinositide-specific PLC catalyzes the cleavage of PIP2 into DAG and IP3. Non-specific PLC generates DAG by hydrolyzing phosphatidylethanolamine (PE) or phosphatidylcholine (PC) (Sim et al., 2020). DAG induces the fusion of liposomes and is required to merge organelle membranes (Fratti et al., 2004; Jun et al., 2004). It induces vesicle fusion through various mechanisms, such as those mediated by TRP channel activation (Albert, 2011). DAG and PMA significantly enhance neurotransmitter release and promote exocytosis in PC12 cells (Xue et al., 2009). They bind to and activate C1-domain-containing proteins, such as PKCs and Munc13, the latter being a prime factor for SNARE-dependent exocytosis (Houy et al., 2022; Rhee et al., 2002; Trexler and Taraska, 2017). Recently, the reconstitution of complete fusion protein machinery demonstrated a new function for DAG in vesicle priming and the cargo release rate (Sundaram et al., 2023).
DAG and phorbol esters induce the AR in sperm from different species. The endogenous sperm synthesis of DAG is regulated by physiological agonists (Murase and Roldan, 1996; O’Toole et al., 1996a; O’Toole et al., 1996b; O'Toole et al., 1996c; Vazquez and Roldan, 1997). DAG activates TRP channels in the sperm plasma membrane, inducing the Ca2+ influx from the extracellular medium (Jungnickel et al., 2001; Jungnickel et al., 2007). This lipid can also impact other factors involved in exocytosis. Given the diverse actions of DAG, our group investigated whether this lipid plays further roles after the opening of TRP calcium channels localized in the sperm plasma membrane. To achieve this, we performed selective plasma membrane permeabilization with streptolysin O (SLO), abrogating ion gradients to dissect DAG additional intracellular actions (Lopez et al., 2012). We developed this protocol specifically for human sperm (Bello et al., 2012; Bustos et al., 2012; Diaz et al., 1996; Lopez et al., 2007; Michaut et al., 2001; Roggero et al., 2005). Our research demonstrated that DAG/PMA induces exocytosis without the extracellular calcium requirement, instead necessitating calcium release from internal stores via IP3-dependent channels and the presence of functional SNAP-25. DAG activates a signaling cascade by stimulating PKC, which activates PLD1 to synthesize phosphatidic acid (PA). This PA drives the production of PIP2 and phosphatidylinositol-(3,4,5)-trisphosphate (PIP3) (Figure 4). The exocytosis triggered by DAG and calcium converges within the same signaling pathway. Therefore, DAG sustains elevated IP3 levels through a positive feedback loop that results in the continuous generation of PIP2. Additionally, DAG stimulates a GTPase exchange factor (GEF) for Rab3A, activating it and enabling the tethering of the plasma membrane to the OAM, facilitating protein interactions that drive the SNARE complex assembly and membrane fusion.
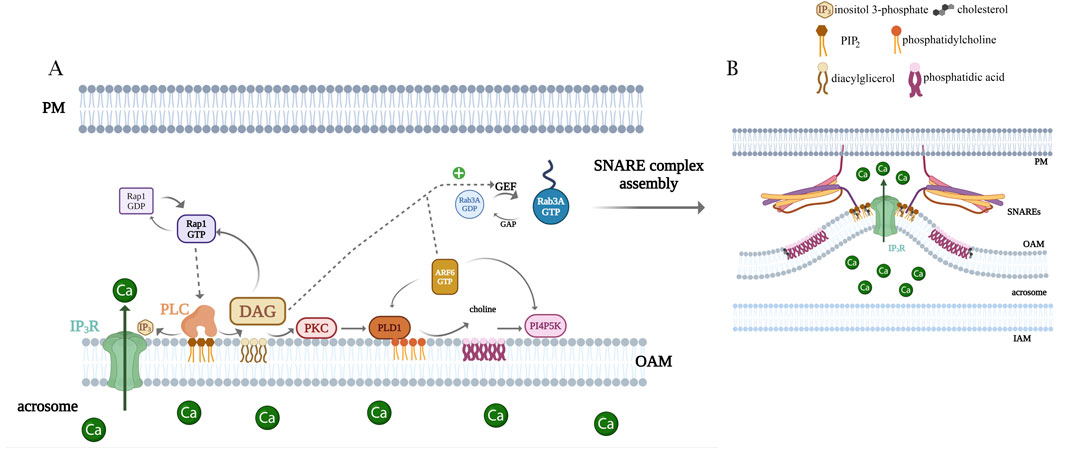
Figure 4. Model highlighting the importance of lipids as signaling molecules for the AR. (A) Rap1 GDP/GTP exchange is promoted by DAG and/or Epac1 and triggers PLC epsilon activation, generating the loop shown in the cartoon. DAG also activates PLD1 via PKC. Active PLD1 hydrolyzes phosphatidylcholine, generating choline and PA. The latter could be activating phosphatidylinositol 4-phosphate 5-kinase (PI4P5K), which catalyzes PIP2 and closes a positive feedback loop. PIP2 hydrolysis generates IP3, which elicits the efflux of calcium from the acrosome. Simultaneously, DAG connects with the proteinaceous membrane fusion machinery by activating Rab3A, which leads to the assembly of trans SNARE complexes. Exocytic stimuli also promote the GDP/GTP exchange in ARF6 that stimulates PLD1, PI4P5K, and PLC activities, driving PIP2 turnover and contributing to the lipid cascade. (B) There is a remarkable bending of the outer acrosomal membrane associated with acrosomal swelling. We postulate a significant role for cone (PA)- and inverted cone (PIP2)-shaped lipids during the AR. Lipid modifications in the cytosolic hemileaflet of the OAM during the exocytotic stimulus may induce changes in the membrane curvature that may foster the growing of deep invaginations of the OAM and the recruitment of SNARE proteins.
6 Tiny but mighty: the diverse functions of small phospholipids
6.1 Phosphatidylinositol-4,5-bisphosphate (PIP2)
In eukaryotic cells, PIP2 is produced in the inner leaflet of the plasma membrane by the highly regulated activity of kinases and phosphatases that modify phosphoinositides (Olivenca et al., 2018). It constitutes less than 2 mol% of the total phospholipid content and is also present in vesicle membranes. Unlike structural lipids, PIP2 exists in minimal quantities and undergoes rapid turnover, which makes it particularly suited for signaling roles (Delage et al., 2013).
PIP2 plays multiple roles during exocytosis by clustering into platforms that delineate membrane domains, attracting proteins from the cytosol and membrane and concentrating them in a signaling spot. PIP2 signaling is transient and localized. It functions as the first and second messenger in signal transduction and molecular recognition processes, and it shapes membranes poised to fuse. Membrane fusion necessitates the transformation of flat lipid bilayers into highly curved structures, making membrane bending a crucial force. In general, PIP2 assembles into membrane microdomains affecting the membrane curvature and tension (Bradley et al., 2020; Slochower et al., 2013; Wang et al., 2014). The effect is due to its inverted cone shape, where the hydrophobic portion occupies the less surface area than the head [for a review consult (Bura et al., 2023; Martin, 2012; Martin, 2015)]. PIP2 clusters induce powerful electrostatic interactions with positively charged molecules (McLaughlin and Murray, 2005; Williams et al., 2009) and recruit proteins containing specific PH domains involved in exocytosis (Martin, 2012; Martin, 2015). Calcium, through its positive charge, bridges reversibly syntaxin 1/PIP2 complexes into mesoscale domains (Milovanovic et al., 2016); therefore, physiological [Ca2+] drives transient lipid plasma membrane reorganization for secretion. The synaptic vesicle calcium sensor, synaptotagmin, binds to PIP2 regulating exocytosis (Park et al., 2015), e.g., phosphoinositide enhances 40-fold the affinity of synaptotagmin-1 for calcium (Honigmann et al., 2013; van den Bogaart et al., 2012). Ji et al. (2017) found that local PIP2 metabolism is essential for vesicle tethering and docking. PIP2 regulates different types of channels including voltage-gated K+ channels, inward-rectifier K+ channels, sensory transduction channels, and voltage-gated Ca2+ channels (Hille et al., 2015; Suh and Hille, 2005; Suh et al., 2012; Suh et al., 2010).
Physiological inducers of the AR elicit a transient and quick calcium entry from the extracellular space into the cytosol, which is crucial for phospholipase activation. Then, PI-PLCs bind PIP2 through their specific PH domain, hydrolyzing the substrate during an early step in the AR [for a review, see Roldan and Shi (2007)]. Our laboratory unveiled a new cascade occurring after the first calcium wave during the acrosome exocytosis (Figure 4), where PIP2 hydrolysis occurs again at a later step, but the phosphoinositide is re-synthesized entering a feedback cycle (Lopez et al., 2012).
Our research has provided compelling evidence showing that ADP-ribosylation ractor 6 (ARF6) is present in human sperm cells, undergoing GDP to GTP exchange in response to exocytic signals and subsequently governing lipid exchange (Pelletan et al., 2015). ARF6 alternates between a GTP, membrane-bound “on” state, and a GDP-bound, cytosolic “off” state. During the activation phase, a guanine nucleotide exchange factor (GEF) releases GDP bound in the nucleotide pocket, and ARF binds GTP. This is the rate-limiting step in ARF activation. During the inactivation phase, hydrolysis converts GTP to GDP, catalyzed by ARF’s intrinsic GTPase activity assisted by GTPase-activating proteins (GAPs). ARF6 regulates actin cytoskeleton reorganization and different membrane trafficking pathways. Suzuki et al. (2009) demonstrated the presence of EFA6, an ARF6-GEF, in spermatogenic cells of adult mouse testes. Proteomic analysis of the human sperm revealed that ARF6 is present in the male gamete and different GAPs and GEFs for these GTPases (Wang et al., 2013). Given that we demonstrated that exocytic stimuli in human sperm induce changes in their lipid profile (Belmonte et al., 2016; Lopez et al., 2012; Pelletan et al., 2015), and, in particular, that PIP2 and PIP3 levels increased, we hypothesize that both lipids contribute to ARF6-GEFs binding to membranes via their pleckstrin homology (PH) domain. Although we previously reported that calcium and DAG activate a GEF for ARF6 (Pelletan et al., 2015), we have not yet detected the presence of GEFs for ARF6 in human sperm cells.
Upon GTP binding, ARF6 activates PLC, which hydrolyzes PIP2-producing DAG and IP3-reliant intra-acrosomal calcium release. Additionally, ARF6, once activated, stimulates PLD1 and PIP kinase, resulting in increased levels of PA and PIP2, respectively. The small GTPase promotes phospholipid remodeling for the continuous synthesis and hydrolysis of PIP2. ARF6-GTP is the master modulator of the feedback loop and consequently the lipid turnover essential for acrosome exocytosis. Concomitantly, ARF6 activates Rab3A, driving the fusion protein machinery assembly, becoming the OAM and PM physically attached (Pelletan et al., 2015). Our study unveils the molecular connection involving ARF6, PLC, PLD, PIP kinase, and Rab3A, shedding light on the intricate interplay between lipids and proteins during AR (summarized in Figure 4).
6.2 Phosphatidic acid (PA)
Three alternative routes are known to synthesize the signaling lipid PA. First, the DAG kinase (DGK) catalyzes the phosphorylation of DAG to produce PA. Second, the acylation of lysophosphatidic acid (LPA) is driven by specific LPA-acyltransferases (LPAAT) producing PA (Jenkins and Frohman, 2005). Third, PLD catalyzes the hydrolysis of the distal phosphodiester link of PC generating PA and choline. PLDs are mainly responsible for PA synthesis during exocytosis. Mammals have six PLD isoforms, with PLD1 and PLD2 functioning as lipases involved in exocytosis. PLD2 is constitutively active and PLD1 activity is highly regulated by different stimuli (Bowling et al., 2021; Frohman, 2015; Humeau et al., 2001; Zeniou-Meyer et al., 2007).
PA contains a tiny headgroup, negatively charged with two fatty acids. Due to this chemical structure, it adopts a conical shape. A localized build-up of PA in a single membrane leaflet can alter membrane topology by inducing a negative curvature in in vitro experiments (Kooijman and Burger, 2009; Kooijman et al., 2005a; Kooijman et al., 2005b). One theory posits that PA plays a crucial role in the secretion process by stabilizing the intensely curved structure of the fusion pore during membrane fusion (Chernomordik and Kozlov, 2005; Chernomordik and Kozlov, 2008). Nevertheless, the exact timing and location of PA formation during exocytosis remain unknown. PA is a main signaling molecule and forms microdomains via intermolecular hydrogen bonds, recruiting proteins to their proper location to achieve their role (Tanguy et al., 2020; Tanguy et al., 2021; Tanguy et al., 2022). There are a lot of data involving PLD1/PA in the fusion of secretory vesicles during regulated exocytosis in neurons and neuroendocrine cells; however, the molecular mechanism is not clear enough [for a review, see Ammar et al. (2013); Chasserot-Golaz et al. (2010); Tanguy et al. (2021); Tanguy et al. (2022); Vitale, (2010)]. Most results point out its effect on the membrane curvature, and some results indicate that PA modulates the membrane penetration of dynamin (Barber et al., 2018; Raben and Barber, 2017). Lam et al. (2008) showed that syntaxin-1A interacted with PA and defined a polybasic region within this protein considered as the lipid-binding domain.
During bovine sperm capacitation, a PLD activity is necessary for actin polymerization (Cohen et al., 2004; Cohen et al., 2016). Bates et al. (2014) described that in Xenopus laevis sperm, PLD is activated to increase PA 2.7-fold by 1 min after insemination. The inhibition of PA synthesis abolished PLCγ and tyrosine kinase Src activation. Our laboratory showed that PLD1/PA is part of a signaling cascade that sustains PIP2/IP3 synthesis. PLD is present in human sperm cells and that DAG activates the enzyme, leading to PA increase through PKC activation. PA is indispensable for membrane fusion during the AR (Lopez et al., 2012). The activity of PLD1 is highly regulated by active ARF6 in acrosome exocytosis (Belmonte et al., 2016; Pelletan et al., 2015). Another protein that interacts directly with PA is Epac1 (Consonni et al., 2012). Epac1 is a cAMP-modulated guanine nucleotide exchange factor for the small G-protein Rap. Epac and Rap are present in the human sperm and connect the cAMP signal (Branham et al., 2009; Branham et al., 2006; Lucchesi et al., 2016) to lipid synthesis shown in the loop described (Figure 4).
Considering that the membranes cannot stretch or deform easily, how do the invaginations form? Where do the lipids that create these invaginations originate? We propose that PIP2 (inverted-cone) and PA (cone) are synthesized mainly in the OAM, clustering in microdomains, which induce membrane bending that originates invaginations (Figure 1). This synthesis causes the OAM to wave during acrosome swelling. This hypothesis is illustrated in Figure 4.
7 Discussion
The WHO defines infertility as a disease characterized by the inability to achieve pregnancy after 12 months of unprotected sexual intercourse (https://www.who.int/news-room/fact-sheets/detail/infertility). It is estimated that there are 186 million people worldwide with infertility, and with current trends, it is projected that there will be two million more cases per year (Szkodziak et al., 2016). Male reproductive failure accounts for half of couples’ infertility (Agarwal et al., 2015). The causes of male infertility include numerous factors: genetic, endocrine, male reproductive system pathologies, systemic diseases, and lifestyle factors (Durairajanayagam, 2018). Idiopathic infertility is diagnosed in men with a normal spermogram after excluding all contributing factors, and it accounts for up to 15% of male reproductive dysfunction (Tvrda et al., 2021).
This review underscores lipid balance and dynamic changes in sperm membranes must be tightly regulated to guarantee physiological processes. Increasing attention is focused on how paternal factors influence fertilization and embryo development (Vallet-Buisan et al., 2023). The lipid composition of the membrane holds sperm structure and function and could be related to altered semen analysis and fertilizing ability. Research on how sperm lipid composition affects male fertility remains insufficient. The results clustered here closely link with data demonstrating that infertile patients with abnormal sperm parameters presented augmented levels of membrane cholesterol (Chen et al., 2021; Di Nisio et al., 2023; Force et al., 2001; Garolla et al., 2018; Hou et al., 2023; Samavat et al., 2014; Sedes et al., 2018). As previously stated, sperm membrane SMs contain high levels of VLC-PUFA. Recently, a study on human sperm found a correlation between the health of the human male gamete and VLC-PUFA concentration, suggesting that sperm quantity and quality possibly depend on these fatty acid levels (Craig et al., 2019).
Some studies have allowed the identification of sperm lipid markers related to mammalian fertility (Shan et al., 2020; Shan et al., 2021). In a preliminary investigation, a lipid cluster has been recognized to differ significantly between fertile and infertile men and associated with the semen parameters. Indeed, SGG, cholesterol sulfate, and PUFAs represented the most important lipid markers to predict semen quality (Di Nisio et al., 2023). Validating these results and tuning up a routine analysis of lipid markers for the laboratory of assisted reproductive technology (ART) might be helpful in predicting the ability of sperm samples to render successful FIV (in vitro fertilization) and ICSI (intra cytoplasmic sperm injection) procedures. A deeper understanding of how paternal factors transported from sperm cells to embryos contribute could illuminate ways to enhance ART from an andrological standpoint.
Future research is required to determine whether there is a correlation between the sperm lipid profile and different reproductive outcomes, such as sperm health, fertilization rates in FIV and ICSI procedures, pregnancy, and live birth. A reliable parameter is urgently required because the current standard semen analysis has low accuracy in predicting the sperm-fertilizing capability and male fertility. The next challenge would be to analyze whether sperm membrane lipid homeostasis can be restored by supplementing the diet or culture media with the correct factors. If successful, such interventions could offer a revolutionary approach to the treatment of male infertility, providing hope to millions of couples worldwide.
Author contributions
LS: writing–original draft and writing–review and editing. SB: conceptualization, funding acquisition, project administration, visualization, writing–original draft, and writing–review and editing.
Funding
The author(s) declare that financial support was received for the research, authorship, and/or publication of this article. This research was funded by grants from CONICET (PIP number 11220210100232, Argentina) and SIIP-Universidad Nacional de Cuyo (grant number 06/J003-T1, Argentina) to SB.
Acknowledgments
The authors would like to thank E. Bocanegra for technical assistance. They would also like to thank P. López, MS from the STAN: ST3371 of TEM samples preparation, IHEM-CONICET-UNCuyo. They are thankful to Dr. L. Mayorga for useful discussions and critical revision of this manuscript.
Conflict of interest
The authors declare that the research was conducted in the absence of any commercial or financial relationships that could be construed as a potential conflict of interest.
Publisher’s note
All claims expressed in this article are solely those of the authors and do not necessarily represent those of their affiliated organizations, or those of the publisher, the editors, and the reviewers. Any product that may be evaluated in this article, or claim that may be made by its manufacturer, is not guaranteed or endorsed by the publisher.
References
Abe, M., Makino, A., Murate, M., Hullin-Matsuda, F., Yanagawa, M., Sako, Y., et al. (2021). PMP2/FABP8 induces PI(4,5)P(2)-dependent transbilayer reorganization of sphingomyelin in the plasma membrane. Cell Rep. 37 (6), 109935. doi:10.1016/j.celrep.2021.109935
Abousalham, A., Liossis, C., O'Brien, L., and Brindley, D. N. (1997). Cell-permeable ceramides prevent the activation of phospholipase D by ADP-ribosylation factor and RhoA. J. Biol. Chem. 272 (2), 1069–1075. doi:10.1074/jbc.272.2.1069
Agarwal, A., Mulgund, A., Hamada, A., and Chyatte, M. R. (2015). A unique view on male infertility around the globe. Reprod. Biol. Endocrinol. 13, 37. doi:10.1186/s12958-015-0032-1
Ahumada-Gutierrez, H., Penalva, D. A., Enriz, R. D., Antollini, S. S., and Cascales, J. J. L. (2019). Mechanical properties of bilayers containing sperm sphingomyelins and ceramides with very long-chain polyunsaturated fatty acids. Chem. Phys. Lipids 218, 178–186. doi:10.1016/j.chemphyslip.2018.12.008
Airola, M. V., and Hannun, Y. A. (2013). Sphingolipid metabolism and neutral sphingomyelinases. Handb. Exp. Pharmacol. 215, 57–76. doi:10.1007/978-3-7091-1368-4_3
Aitken, R. J., Wingate, J. K., De Iuliis, G. N., and McLaughlin, E. A. (2007). Analysis of lipid peroxidation in human spermatozoa using BODIPY C11. Mol. Hum. Reprod. 13 (4), 203–211. doi:10.1093/molehr/gal119
Albert, A. P. (2011). Gating mechanisms of canonical transient receptor potential channel proteins: role of phosphoinositols and diacylglycerol. Adv. Exp. Med. Biol. 704, 391–411. doi:10.1007/978-94-007-0265-3_22
Ammar, M. R., Kassas, N., Chasserot-Golaz, S., Bader, M. F., and Vitale, N. (2013). Lipids in regulated exocytosis: what are they doing? Front. Endocrinol. (Lausanne) 4, 125. doi:10.3389/fendo.2013.00125
Arana, L., Ordonez, M., Ouro, A., Rivera, I. G., Gangoiti, P., Trueba, M., et al. (2013). Ceramide 1-phosphate induces macrophage chemoattractant protein-1 release: involvement in ceramide 1-phosphate-stimulated cell migration. Am. J. Physiol. Endocrinol. Metab. 304 (11), E1213–E1226. doi:10.1152/ajpendo.00480.2012
Asano, A., Nelson-Harrington, J. L., and Travis, A. J. (2013a). Membrane rafts regulate phospholipase B activation in murine sperm. Commun. Integr. Biol. 6 (6), e27362. doi:10.4161/cib.27362
Asano, A., Nelson-Harrington, J. L., and Travis, A. J. (2013b). Phospholipase B is activated in response to sterol removal and stimulates acrosome exocytosis in murine sperm. J. Biol. Chem. 288 (39), 28104–28115. doi:10.1074/jbc.M113.450981
Asano, A., Selvaraj, V., Buttke, D. E., Nelson, J. L., Green, K. M., Evans, J. E., et al. (2009). Biochemical characterization of membrane fractions in murine sperm: identification of three distinct sub-types of membrane rafts. J. Cell Physiol. 218 (3), 537–548. doi:10.1002/jcp.21623
Bahat, A., Tur-Kaspa, I., Gakamsky, A., Giojalas, L. C., Breitbart, H., and Eisenbach, M. (2003). Thermotaxis of mammalian sperm cells: a potential navigation mechanism in the female genital tract. Nat. Med. 9 (2), 149–150. doi:10.1038/nm0203-149
Balaban, B., Sakkas, D., and Gardner, D. K. (2014). Laboratory procedures for human in vitro fertilization. Semin. Reprod. Med. 32 (4), 272–282. doi:10.1055/s-0034-1375179
Barber, C. N., Huganir, R. L., and Raben, D. M. (2018). Phosphatidic acid-producing enzymes regulating the synaptic vesicle cycle: role for PLD? Adv. Biol. Regul. 67, 141–147. doi:10.1016/j.jbior.2017.09.009
Bartke, N., and Hannun, Y. A. (2009). Bioactive sphingolipids: metabolism and function. J. Lipid Res. 50, S91–S96. doi:10.1194/jlr.R800080-JLR200
Bates, R. C., Fees, C. P., Holland, W. L., Winger, C. C., Batbayar, K., Ancar, R., et al. (2014). Activation of Src and release of intracellular calcium by phosphatidic acid during Xenopus laevis fertilization. Dev. Biol. 386 (1), 165–180. doi:10.1016/j.ydbio.2013.11.006
Bello, O. D., Zanetti, M. N., Mayorga, L. S., and Michaut, M. A. (2012). RIM, Munc13, and Rab3A interplay in acrosomal exocytosis. Exp. Cell Res. 318 (5), 478–488. doi:10.1016/j.yexcr.2012.01.002
Belmonte, S. A., Lopez, C. I., Roggero, C. M., De Blas, G. A., Tomes, C. N., and Mayorga, L. S. (2005). Cholesterol content regulates acrosomal exocytosis by enhancing Rab3A plasma membrane association. Dev. Biol. 285 (2), 393–408. doi:10.1016/j.ydbio.2005.07.001
Belmonte, S. A., Mayorga, L. S., and Tomes, C. N. (2016). The molecules of sperm exocytosis. Adv. Anat. Embryol. Cell Biol. 220, 71–92. doi:10.1007/978-3-319-30567-7_4
Belmonte, S. A., and Suhaiman, L. (2012). Optimized protocols to analyze sphingosine-1-phosphate signal transduction pathways during acrosomal exocytosis in human sperm. Methods Mol. Biol. 874, 99–128. doi:10.1007/978-1-61779-800-9_9
Bernecic, N. C., de Graaf, S. P., Leahy, T., and Gadella, B. M. (2021). HDL mediates reverse cholesterol transport from ram spermatozoa and induces hyperactivated motility. Biol. Reprod. 104 (6), 1271–1281. doi:10.1093/biolre/ioab035
Bernecic, N. C., Gadella, B. M., de Graaf, S. P., and Leahy, T. (2020). Synergism between albumin, bicarbonate and cAMP upregulation for cholesterol efflux from ram sperm. Reproduction 160 (2), 269–280. doi:10.1530/REP-19-0430
Bhakta, H. H., Refai, F. H., and Avella, M. A. (2019). The molecular mechanisms mediating mammalian fertilization. Development 146 (15), dev176966. doi:10.1242/dev.176966
Boerke, A., Tsai, P. S., Garcia-Gil, N., Brewis, I. A., and Gadella, B. M. (2008). Capacitation-dependent reorganization of microdomains in the apical sperm head plasma membrane: functional relationship with zona binding and the zona-induced acrosome reaction. Theriogenology 70 (8), 1188–1196. doi:10.1016/j.theriogenology.2008.06.021
Bowling, F. Z., Frohman, M. A., and Airola, M. V. (2021). Structure and regulation of human phospholipase D. Adv. Biol. Regul. 79, 100783. doi:10.1016/j.jbior.2020.100783
Bradley, R. P., Slochower, D. R., Janmey, P. A., and Radhakrishnan, R. (2020). Divalent cations bind to phosphoinositides to induce ion and isomer specific propensities for nano-cluster initiation in bilayer membranes. R. Soc. Open Sci. 7 (5), 192208. doi:10.1098/rsos.192208
Brahmaraju, M., Shoeb, M., Laloraya, M., and Kumar, P. G. (2004). Spatio-temporal organization of Vam6P and SNAP on mouse spermatozoa and their involvement in sperm-zona pellucida interactions. Biochem. Biophys. Res. Commun. 318 (1), 148–155. doi:10.1016/j.bbrc.2004.04.006
Branham, M. T., Bustos, M. A., De Blas, G. A., Rehmann, H., Zarelli, V. E., Trevino, C. L., et al. (2009). Epac activates the small G proteins Rap1 and Rab3A to achieve exocytosis. J. Biol. Chem. 284 (37), 24825–24839. doi:10.1074/jbc.M109.015362
Branham, M. T., Mayorga, L. S., and Tomes, C. N. (2006). Calcium-induced acrosomal exocytosis requires cAMP acting through a protein kinase A-independent, Epac-mediated pathway. J. Biol. Chem. 281 (13), 8656–8666. doi:10.1074/jbc.M508854200
Brown, D. A., and Rose, J. K. (1992). Sorting of GPI-anchored proteins to glycolipid-enriched membrane subdomains during transport to the apical cell surface. Cell 68 (3), 533–544. doi:10.1016/0092-8674(92)90189-j
Buffone, M. G., Foster, J. A., and Gerton, G. L. (2008). The role of the acrosomal matrix in fertilization. Int. J. Dev. Biol. 52 (5-6), 511–522.
Buffone, M. G., Hirohashi, N., and Gerton, G. L. (2014). Unresolved questions concerning mammalian sperm acrosomal exocytosis. Biol. Reprod. 90 (5), 112. doi:10.1095/biolreprod.114.117911
Bura, A., Cabrijan, S., Duric, I., Bruketa, T., and Jurak Begonja, A. (2023). A plethora of functions condensed into tiny phospholipids: the story of PI4P and PI(4,5)P(2). Cells 12 (10), 1411. doi:10.3390/cells12101411
Bustos, M. A., Lucchesi, O., Ruete, M. C., Mayorga, L. S., and Tomes, C. N. (2012). Rab27 and Rab3 sequentially regulate human sperm dense-core granule exocytosis. Proc. Natl. Acad. Sci. U. S. A. 109 (30), E2057–E2066. doi:10.1073/pnas.1121173109
Calder, P. C., and Yaqoob, P. (2007). Lipid rafts--composition, characterization, and controversies. J. Nutr. 137 (3), 545–547.
Casslen, B., and Nilsson, B. (1984). Human uterine fluid, examined in undiluted samples for osmolarity and the concentrations of inorganic ions, albumin, glucose, and urea. Am. J. Obstet. Gynecol. 150 (7), 877–881. doi:10.1016/0002-9378(84)90466-6
Chamberlain, L. H., Burgoyne, R. D., and Gould, G. W. (2001). SNARE proteins are highly enriched in lipid rafts in PC12 cells: implications for the spatial control of exocytosis. Proc. Natl. Acad. Sci. U. S. A. 98 (10), 5619–5624. doi:10.1073/pnas.091502398
Chasserot-Golaz, S., Coorssen, J. R., Meunier, F. A., and Vitale, N. (2010). Lipid dynamics in exocytosis. Cell Mol. Neurobiol. 30 (8), 1335–1342. doi:10.1007/s10571-010-9577-x
Chen, S., Wang, M., Li, L., Wang, J., Ma, X., Zhang, H., et al. (2021). High-coverage targeted lipidomics revealed dramatic lipid compositional changes in asthenozoospermic spermatozoa and inverse correlation of ganglioside GM3 with sperm motility. Reprod. Biol. Endocrinol. 19 (1), 105. doi:10.1186/s12958-021-00792-3
Cheng, X., Xie, H., Xiong, Y., Sun, P., Xue, Y., and Li, K. (2023). Lipidomics profiles of human spermatozoa: insights into capacitation and acrosome reaction using UPLC-MS-based approach. Front. Endocrinol. (Lausanne) 14, 1273878. doi:10.3389/fendo.2023.1273878
Chernomordik, L. V., and Kozlov, M. M. (2005). Membrane hemifusion: crossing a chasm in two leaps. Cell 123 (3), 375–382. doi:10.1016/j.cell.2005.10.015
Chernomordik, L. V., and Kozlov, M. M. (2008). Mechanics of membrane fusion. Nat. Struct. Mol. Biol. 15 (7), 675–683. doi:10.1038/nsmb.1455
Cohen, G., Rubinstein, S., Gur, Y., and Breitbart, H. (2004). Crosstalk between protein kinase A and C regulates phospholipase D and F-actin formation during sperm capacitation. Dev. Biol. 267 (1), 230–241. doi:10.1016/j.ydbio.2003.10.034
Cohen, R., Buttke, D. E., Asano, A., Mukai, C., Nelson, J. L., Ren, D., et al. (2014). Lipid modulation of calcium flux through CaV2.3 regulates acrosome exocytosis and fertilization. Dev. Cell 28 (3), 310–321. doi:10.1016/j.devcel.2014.01.005
Cohen, R., Mukai, C., and Travis, A. J. (2016). Lipid regulation of acrosome exocytosis. Adv. Anat. Embryol. Cell Biol. 220, 107–127. doi:10.1007/978-3-319-30567-7_6
Companyo, M., Iborra, A., Villaverde, J., Martinez, P., and Morros, A. (2007). Membrane fluidity changes in goat sperm induced by cholesterol depletion using beta-cyclodextrin. Biochim. Biophys. Acta 1768 (9), 2246–2255. doi:10.1016/j.bbamem.2007.06.011
Consonni, S. V., Gloerich, M., Spanjaard, E., and Bos, J. L. (2012). cAMP regulates DEP domain-mediated binding of the guanine nucleotide exchange factor Epac1 to phosphatidic acid at the plasma membrane. Proc. Natl. Acad. Sci. U. S. A. 109 (10), 3814–3819. doi:10.1073/pnas.1117599109
Craig, L. B., Brush, R. S., Sullivan, M. T., Zavy, M. T., Agbaga, M. P., and Anderson, R. E. (2019). Decreased very long chain polyunsaturated fatty acids in sperm correlates with sperm quantity and quality. J. Assist. Reprod. Genet. 36 (7), 1379–1385. doi:10.1007/s10815-019-01464-3
Cross, N. L. (2000). Sphingomyelin modulates capacitation of human sperm in vitro. Biol. Reprod. 63 (4), 1129–1134. doi:10.1095/biolreprod63.4.1129
Dacheux, J. L., and Dacheux, F. (2014). New insights into epididymal function in relation to sperm maturation. Reproduction 147 (2), R27–R42. doi:10.1530/REP-13-0420
de Almeida, R. F., Fedorov, A., and Prieto, M. (2003). Sphingomyelin/phosphatidylcholine/cholesterol phase diagram: boundaries and composition of lipid rafts. Biophys. J. 85 (4), 2406–2416. doi:10.1016/S0006-3495(03)74664-5
Delage, E., Puyaubert, J., Zachowski, A., and Ruelland, E. (2013). Signal transduction pathways involving phosphatidylinositol 4-phosphate and phosphatidylinositol 4,5-bisphosphate: convergences and divergences among eukaryotic kingdoms. Prog. Lipid Res. 52 (1), 1–14. doi:10.1016/j.plipres.2012.08.003
De Toni, L., Cosci, I., Sabovic, I., Di Nisio, A., Guidolin, D., Pedrucci, F., et al. (2023). Membrane cholesterol inhibits progesterone-mediated sperm function through the possible involvement of ABHD2. Int. J. Mol. Sci. 24 (11), 9254. doi:10.3390/ijms24119254
De Toni, L., Sabovic, I., De Filippis, V., Acquasaliente, L., Peterle, D., Guidolin, D., et al. (2021). Sperm cholesterol content modifies sperm function and TRPV1-mediated sperm migration. Int. J. Mol. Sci. 22 (6), 3126. doi:10.3390/ijms22063126
Diaz, A., Dominguez, L., Fornes, M. W., Burgos, M. H., and Mayorga, L. S. (1996). Acrosome content release in streptolysin O permeabilized mouse spermatozoa. Andrologia 28 (1), 21–26. doi:10.1111/j.1439-0272.1996.tb02753.x
Di Nisio, A., De Toni, L., Sabovic, I., Vignoli, A., Tenori, L., Dall'Acqua, S., et al. (2023). Lipidomic profile of human sperm membrane identifies a clustering of lipids associated with semen quality and function. Int. J. Mol. Sci. 25 (1), 297. doi:10.3390/ijms25010297
Doktorova, M., Symons, J. L., and Levental, I. (2020). Structural and functional consequences of reversible lipid asymmetry in living membranes. Nat. Chem. Biol. 16 (12), 1321–1330. doi:10.1038/s41589-020-00688-0
Durairajanayagam, D. (2018). Lifestyle causes of male infertility. Arab. J. Urol. 16 (1), 10–20. doi:10.1016/j.aju.2017.12.004
Ehrenwald, E., Foote, R. H., and Parks, J. E. (1990). Bovine oviductal fluid components and their potential role in sperm cholesterol efflux. Mol. Reprod. Dev. 25 (2), 195–204. doi:10.1002/mrd.1080250213
Elzanaty, S., Erenpreiss, J., and Becker, C. (2007). Seminal plasma albumin: origin and relation to the male reproductive parameters. Andrologia 39 (2), 60–65. doi:10.1111/j.1439-0272.2007.00764.x
Evans, J. P., and Florman, H. M. (2002). The state of the union: the cell biology of fertilization. Nat. Cell Biol. 4 (Suppl. l), s57–s63. doi:10.1038/ncb-nm-fertilityS57
Flesch, F. M., Brouwers, J. F., Nievelstein, P. F., Verkleij, A. J., van Golde, L. M., Colenbrander, B., et al. (2001). Bicarbonate stimulated phospholipid scrambling induces cholesterol redistribution and enables cholesterol depletion in the sperm plasma membrane. J. Cell Sci. 114 (Pt 19), 3543–3555. doi:10.1242/jcs.114.19.3543
Flesch, F. M., and Gadella, B. M. (2000). Dynamics of the mammalian sperm plasma membrane in the process of fertilization. Biochim. Biophys. Acta 1469 (3), 197–235. doi:10.1016/s0304-4157(00)00018-6
Florman, H. M., Jungnickel, M. K., and Sutton, K. A. (2008). Regulating the acrosome reaction. Int. J. Dev. Biol. 52 (5-6), 503–510. doi:10.1387/ijdb.082696hf
Force, A., Grizard, G., Giraud, M. N., Motta, C., Sion, B., and Boucher, D. (2001). Membrane fluidity and lipid content of human spermatozoa selected by swim-up method. Int. J. Androl. 24 (6), 327–334. doi:10.1046/j.1365-2605.2001.00309.x
Fratti, R. A., Jun, Y., Merz, A. J., Margolis, N., and Wickner, W. (2004). Interdependent assembly of specific regulatory lipids and membrane fusion proteins into the vertex ring domain of docked vacuoles. J. Cell Biol. 167 (6), 1087–1098. doi:10.1083/jcb.200409068
Frohman, M. A. (2015). The phospholipase D superfamily as therapeutic targets. Trends Pharmacol. Sci. 36 (3), 137–144. doi:10.1016/j.tips.2015.01.001
Furland, N. E., Oresti, G. M., Antollini, S. S., Venturino, A., Maldonado, E. N., and Aveldano, M. I. (2007a). Very long-chain polyunsaturated fatty acids are the major acyl groups of sphingomyelins and ceramides in the head of mammalian spermatozoa. J. Biol. Chem. 282 (25), 18151–18161. doi:10.1074/jbc.M700709200
Furland, N. E., Zanetti, S. R., Oresti, G. M., Maldonado, E. N., and Aveldano, M. I. (2007b). Ceramides and sphingomyelins with high proportions of very long-chain polyunsaturated fatty acids in mammalian germ cells. J. Biol. Chem. 282 (25), 18141–18150. doi:10.1074/jbc.M700708200
Gadella, B. M., Lopes-Cardozo, M., van Golde, L. M., Colenbrander, B., and Gadella, T. W. (1995). Glycolipid migration from the apical to the equatorial subdomains of the sperm head plasma membrane precedes the acrosome reaction. Evidence for a primary capacitation event in boar spermatozoa. J. Cell Sci. 108 (Pt 3), 935–946. doi:10.1242/jcs.108.3.935
Gadella, B. M., Tsai, P. S., Boerke, A., and Brewis, I. A. (2008). Sperm head membrane reorganisation during capacitation. Int. J. Dev. Biol. 52 (5-6), 473–480. doi:10.1387/ijdb.082583bg
Gafurova, C. R., Tsentsevitsky, A. N., Fedorov, N. S., Khaziev, A. N., Malomouzh, A. I., and Petrov, A. M. (2024). β2-Adrenergic regulation of the neuromuscular transmission and its lipid-dependent switch. Mol. Neurobiol. 61, 6805–6821. doi:10.1007/s12035-024-03991-2
Gamboa, S., and Ramalho-Santos, J. (2005). SNARE proteins and caveolin-1 in stallion spermatozoa: possible implications for fertility. Theriogenology 64 (2), 275–291. doi:10.1016/j.theriogenology.2004.11.021
Garolla, A., Sabovic, I., Tescari, S., De Toni, L., Menegazzo, M., Cosci, I., et al. (2018). Impaired sperm function in infertile men relies on the membrane sterol pattern. Andrology 6 (2), 325–334. doi:10.1111/andr.12468
Gault, C. R., Obeid, L. M., and Hannun, Y. A. (2010). An overview of sphingolipid metabolism: from synthesis to breakdown. Adv. Exp. Med. Biol. 688, 1–23. doi:10.1007/978-1-4419-6741-1_1
Gautier, C., and Aurich, C. (2022). “Fine feathers make fine birds” - the mammalian sperm plasma membrane lipid composition and effects on assisted reproduction. Anim. Reprod. Sci. 246, 106884. doi:10.1016/j.anireprosci.2021.106884
Gomez-Munoz, A., Presa, N., Gomez-Larrauri, A., Rivera, I. G., Trueba, M., and Ordonez, M. (2016). Control of inflammatory responses by ceramide, sphingosine 1-phosphate and ceramide 1-phosphate. Prog. Lipid Res. 61, 51–62. doi:10.1016/j.plipres.2015.09.002
Han, S., Applewhite, S., DeCata, J., Jones, S., Cummings, J., and Wang, S. (2023). Arachidonic acid reverses cholesterol and zinc inhibition of human voltage-gated proton channels. J. Biol. Chem. 299 (7), 104918. doi:10.1016/j.jbc.2023.104918
Han, S., Chu, X. P., Goodson, R., Gamel, P., Peng, S., Vance, J., et al. (2022). Cholesterol inhibits human voltage-gated proton channel hHv1. Proc. Natl. Acad. Sci. U. S. A. 119 (36), e2205420119. doi:10.1073/pnas.2205420119
Hannun, Y. A., and Obeid, L. M. (2008). Principles of bioactive lipid signalling: lessons from sphingolipids. Nat. Rev. Mol. Cell Biol. 9 (2), 139–150. doi:10.1038/nrm2329
Harrison, R. A., and Gadella, B. M. (2005). Bicarbonate-induced membrane processing in sperm capacitation. Theriogenology 63 (2), 342–351. doi:10.1016/j.theriogenology.2004.09.016
Henkel, R. R. (2011). Leukocytes and oxidative stress: dilemma for sperm function and male fertility. Asian J. Androl. 13 (1), 43–52. doi:10.1038/aja.2010.76
Hille, B., Dickson, E. J., Kruse, M., Vivas, O., and Suh, B. C. (2015). Phosphoinositides regulate ion channels. Biochim. Biophys. Acta 1851 (6), 844–856. doi:10.1016/j.bbalip.2014.09.010
Honigmann, A., van den, B. G., Iraheta, E., Risselada, H. J., Milovanovic, D., Mueller, V., et al. (2013). Phosphatidylinositol 4,5-bisphosphate clusters act as molecular beacons for vesicle recruitment. Nat. Struct. Mol. Biol. 20 (6), 679–686. doi:10.1038/nsmb.2570
Hou, Y., He, Y., Wang, J., Zhou, Y., Ma, B., Li, X., et al. (2023). Comprehensive metabolomics profiling of seminal plasma in asthenozoospermia caused by different etiologies. Clin. Chim. Acta 548, 117530. doi:10.1016/j.cca.2023.117530
Houy, S., Martins, J. S., Lipstein, N., and Sorensen, J. B. (2022). Phorbolester-activated Munc13-1 and ubMunc13-2 exert opposing effects on dense-core vesicle secretion. Elife 11, e79433. doi:10.7554/eLife.79433
Humeau, Y., Vitale, N., Chasserot-Golaz, S., Dupont, J. L., Du, G., Frohman, M. A., et al. (2001). A role for phospholipase D1 in neurotransmitter release. Proc. Natl. Acad. Sci. U. S. A. 98 (26), 15300–15305. doi:10.1073/pnas.261358698
Iborra, A., Companyo, M., Martinez, P., and Morros, A. (2000). Cholesterol efflux promotes acrosome reaction in goat spermatozoa. Biol. Reprod. 62 (2), 378–383. doi:10.1095/biolreprod62.2.378
Inaba, K. (2011). Sperm flagella: comparative and phylogenetic perspectives of protein components. Mol. Hum. Reprod. 17 (8), 524–538. doi:10.1093/molehr/gar034
Ingolfsson, H. I., Melo, M. N., van Eerden, F. J., Arnarez, C., Lopez, C. A., Wassenaar, T. A., et al. (2014). Lipid organization of the plasma membrane. J. Am. Chem. Soc. 136 (41), 14554–14559. doi:10.1021/ja507832e
Jenkins, G. M., and Frohman, M. A. (2005). Phospholipase D: a lipid centric review. Cell Mol. Life Sci. 62 (19-20), 2305–2316. doi:10.1007/s00018-005-5195-z
Ji, C., Fan, F., and Lou, X. (2017). Vesicle docking is a key target of local PI(4,5)P(2) metabolism in the secretory pathway of INS-1 cells. Cell Rep. 20 (6), 1409–1421. doi:10.1016/j.celrep.2017.07.041
Ji, J. E., Kim, S. K., Ahn, K. H., Choi, J. M., Jung, S. Y., Jung, K. M., et al. (2011). Ceramide induces serotonin release from RBL-2H3 mast cells through calcium mediated activation of phospholipase A2. Prostagl. Other Lipid Mediat 94 (3-4), 88–95. doi:10.1016/j.prostaglandins.2011.01.001
Jun, Y., Fratti, R. A., and Wickner, W. (2004). Diacylglycerol and its formation by phospholipase C regulate Rab- and SNARE-dependent yeast vacuole fusion. J. Biol. Chem. 279 (51), 53186–53195. doi:10.1074/jbc.M411363200
Jungnickel, M. K., Marrero, H., Birnbaumer, L., Lemos, J. R., and Florman, H. M. (2001). Trp2 regulates entry of Ca2+ into mouse sperm triggered by egg ZP3. Nat. Cell Biol. 3 (5), 499–502. doi:10.1038/35074570
Jungnickel, M. K., Sutton, K. A., Wang, Y., and Florman, H. M. (2007). Phosphoinositide-dependent pathways in mouse sperm are regulated by egg ZP3 and drive the acrosome reaction. Dev. Biol. 304 (1), 116–126. doi:10.1016/j.ydbio.2006.12.023
Kirkman-Brown, J. C., Punt, E. L., Barratt, C. L., and Publicover, S. J. (2002). Zona pellucida and progesterone-induced Ca2+ signaling and acrosome reaction in human spermatozoa. J. Androl. 23 (3), 306–315. doi:10.1002/j.1939-4640.2002.tb02232.x
Kooijman, E. E., and Burger, K. N. (2009). Biophysics and function of phosphatidic acid: a molecular perspective. Biochim. Biophys. Acta 1791 (9), 881–888. doi:10.1016/j.bbalip.2009.04.001
Kooijman, E. E., Carter, K. M., van Laar, E. G., Chupin, V., Burger, K. N., and de, K. B. (2005a). What makes the bioactive lipids phosphatidic acid and lysophosphatidic acid so special? Biochemistry 44 (51), 17007–17015. doi:10.1021/bi0518794
Kooijman, E. E., Chupin, V., Fuller, N. L., Kozlov, M. M., de, K. B., Burger, K. N., et al. (2005b). Spontaneous curvature of phosphatidic acid and lysophosphatidic acid. Biochemistry 44 (6), 2097–2102. doi:10.1021/bi0478502
Lam, A. D., Tryoen-Toth, P., Tsai, B., Vitale, N., and Stuenkel, E. L. (2008). SNARE-Catalyzed fusion events are regulated by Syntaxin1A-lipid interactions. Mol. Biol. Cell 19 (2), 485–497. doi:10.1091/mbc.e07-02-0148
Lang, T. (2007). SNARE proteins and ‘membrane rafts. J. Physiol. 585 (Pt 3), 693–698. doi:10.1113/jphysiol.2007.134346
Lingwood, D., Kaiser, H. J., Levental, I., and Simons, K. (2009). Lipid rafts as functional heterogeneity in cell membranes. Biochem Soc Trans. 37 (Pt 5), 955–960.
Lang, T., Bruns, D., Wenzel, D., Riedel, D., Holroyd, P., Thiele, C., et al. (2001). SNAREs are concentrated in cholesterol-dependent clusters that define docking and fusion sites for exocytosis. EMBO J. 20 (9), 2202–2213. doi:10.1093/emboj/20.9.2202
Leahy, T., and Gadella, B. M. (2015). New insights into the regulation of cholesterol efflux from the sperm membrane. Asian J. Androl. 17 (4), 561–567. doi:10.4103/1008-682X.153309
Lishko, P. V., Botchkina, I. L., and Kirichok, Y. (2011). Progesterone activates the principal Ca2+ channel of human sperm. Nature 471 (7338), 387–391. doi:10.1038/nature09767
Lopez, C. I., Belmonte, S. A., De Blas, G. A., and Mayorga, L. S. (2007). Membrane-permeant Rab3A triggers acrosomal exocytosis in living human sperm. FASEB J. 21 (14), 4121–4130. doi:10.1096/fj.06-7716com
Lopez, C. I., Pelletan, L. E., Suhaiman, L., De Blas, G. A., Vitale, N., Mayorga, L. S., et al. (2012). Diacylglycerol stimulates acrosomal exocytosis by feeding into a PKC- and PLD1-dependent positive loop that continuously supplies phosphatidylinositol 4,5-bisphosphate. Biochim. Biophys. Acta 1821 (9), 1186–1199. doi:10.1016/j.bbalip.2012.05.001
Lucchesi, O., Ruete, M. C., Bustos, M. A., Quevedo, M. F., and Tomes, C. N. (2016). The signaling module cAMP/Epac/Rap1/PLCε/IP3 mobilizes acrosomal calcium during sperm exocytosis. Biochim. Biophys. Acta 1863 (4), 544–561. doi:10.1016/j.bbamcr.2015.12.007
Lv, J. H., He, L., and Sui, S. F. (2008). Lipid rafts association of synaptotagmin I on synaptic vesicles. Biochem. (Mosc) 73 (3), 283–288. doi:10.1134/s0006297908030073
Mansfield, P. J., Carey, S. S., Hinkovska-Galcheva, V., Shayman, J. A., and Boxer, L. A. (2004). Ceramide inhibition of phospholipase D and its relationship to RhoA and ARF1 translocation in GTP gamma S-stimulated polymorphonuclear leukocytes. Blood 103 (6), 2363–2368. doi:10.1182/blood-2002-11-3341
Martin, T. F. (2012). Role of PI(4,5)P(2) in vesicle exocytosis and membrane fusion. Subcell. Biochem. 59, 111–130. doi:10.1007/978-94-007-3015-1_4
Martin, T. F. (2015). PI(4,5)P₂-binding effector proteins for vesicle exocytosis. Biochim. Biophys. Acta 1851 (6), 785–793. doi:10.1016/j.bbalip.2014.09.017
Matamoros-Volante, A., and Trevino, C. L. (2020). Capacitation-associated alkalization in human sperm is differentially controlled at the subcellular level. J. Cell Sci. 133 (2), jcs238816. doi:10.1242/jcs.238816
McLaughlin, S., and Murray, D. (2005). Plasma membrane phosphoinositide organization by protein electrostatics. Nature 438 (7068), 605–611. doi:10.1038/nature04398
Michaut, M., De, B. G., Tomes, C. N., Yunes, R., Fukuda, M., and Mayorga, L. S. (2001). Synaptotagmin VI participates in the acrosome reaction of human spermatozoa. Dev. Biol. 235 (2), 521–529. doi:10.1006/dbio.2001.0316
Michaut, M., Tomes, C. N., De, B. G., Yunes, R., and Mayorga, L. S. (2000). Calcium-triggered acrosomal exocytosis in human spermatozoa requires the coordinated activation of Rab3A and N-ethylmaleimide-sensitive factor. Proc. Natl. Acad. Sci. U. S. A. 97 (18), 9996–10001. doi:10.1073/pnas.180206197
Miller, M. R., Mannowetz, N., Iavarone, A. T., Safavi, R., Gracheva, E. O., Smith, J. F., et al. (2016). Unconventional endocannabinoid signaling governs sperm activation via the sex hormone progesterone. Science 352 (6285), 555–559. doi:10.1126/science.aad6887
Milovanovic, D., Platen, M., Junius, M., Diederichsen, U., Schaap, I. A., Honigmann, A., et al. (2016). Calcium promotes the formation of syntaxin 1 mesoscale domains through phosphatidylinositol 4,5-bisphosphate. J. Biol. Chem. 291 (15), 7868–7876. doi:10.1074/jbc.M116.716225
Murase, T., Imaeda, N., Kondoh, N., and Tsubota, T. (2004). Ceramide enhances acrosomal exocytosis triggered by calcium and the calcium ionophore A23187 in boar spermatozoa. J. Reprod. Dev. 50 (6), 667–674. doi:10.1262/jrd.50.667
Murase, T., and Roldan, E. R. (1996). Progesterone and the zona pellucida activate different transducing pathways in the sequence of events leading to diacylglycerol generation during mouse sperm acrosomal exocytosis. Biochem. J. 320 (Pt 3), 1017–1023. doi:10.1042/bj3201017
Nikolopoulou, M., Soucek, D. A., and Vary, J. C. (1986a). Lipid composition of the membrane released after an in vitro acrosome reaction of epididymal boar sperm. Lipids 21 (9), 566–570. doi:10.1007/BF02534053
Nikolopoulou, M., Soucek, D. A., and Vary, J. C. (1986b). Modulation of the lipid composition of boar sperm plasma membranes during an acrosome reaction in vitro. Arch. Biochem. Biophys. 250 (1), 30–37. doi:10.1016/0003-9861(86)90698-3
Nixon, B., and Aitken, R. J. (2009). The biological significance of detergent-resistant membranes in spermatozoa. J. Reprod. Immunol. 83 (1-2), 8–13. doi:10.1016/j.jri.2009.06.258
Nixon, B., Mitchell, L. A., Anderson, A. L., McLaughlin, E. A., O’Bryan M, K., and Aitken, R. J. (2011). Proteomic and functional analysis of human sperm detergent resistant membranes. J. Cell Physiol. 226 (10), 2651–2665. doi:10.1002/jcp.22615
Ogasawara, F., and Ueda, K. (2022). ABCA1 and cholesterol transfer protein Aster-A promote an asymmetric cholesterol distribution in the plasma membrane. J. Biol. Chem. 298 (12), 102702. doi:10.1016/j.jbc.2022.102702
Olivenca, D. V., Uliyakina, I., Fonseca, L. L., Amaral, M. D., Voit, E. O., and Pinto, F. R. (2018). A mathematical model of the phosphoinositide pathway. Sci. Rep. 8 (1), 3904. doi:10.1038/s41598-018-22226-8
Oresti, G. M., Luquez, J. M., Furland, N. E., and Aveldano, M. I. (2011). Uneven distribution of ceramides, sphingomyelins and glycerophospholipids between heads and tails of rat spermatozoa. Lipids 46 (12), 1081–1090. doi:10.1007/s11745-011-3601-x
Orta, G., de la Vega-Beltran, J. L., Martin-Hidalgo, D., Santi, C. M., Visconti, P. E., and Darszon, A. (2018). CatSper channels are regulated by protein kinase A. J. Biol. Chem. 293 (43), 16830–16841. doi:10.1074/jbc.RA117.001566
O’Toole, C. M., Roldan, E. R., and Fraser, L. R. (1996a). Protein kinase C activation during progesterone-stimulated acrosomal exocytosis in human spermatozoa. Mol. Hum. Reprod. 2 (12), 921–927. doi:10.1093/molehr/2.12.921
O’Toole, C. M., Roldan, E. R., and Fraser, L. R. (1996b). Role for Ca2+ channels in the signal transduction pathway leading to acrosomal exocytosis in human spermatozoa. Mol. Reprod. Dev. 45 (2), 204–211. doi:10.1002/(SICI)1098-2795(199610)45:2<204::AID-MRD13>3.0.CO;2-0
O’Toole, C. M., Roldan, E. R., Hampton, P., and Fraser, L. R. (1996c). A role for diacylglycerol in human sperm acrosomal exocytosis. Mol. Hum. Reprod. 2 (5), 317–326. doi:10.1093/molehr/2.5.317
Ouro, A., Arana, L., Gangoiti, P., Rivera, I. G., Ordonez, M., Trueba, M., et al. (2013). Ceramide 1-phosphate stimulates glucose uptake in macrophages. Cell Signal 25 (4), 786–795. doi:10.1016/j.cellsig.2013.01.009
Park, Y., Seo, J. B., Fraind, A., Perez-Lara, A., Yavuz, H., Han, K., et al. (2015). Synaptotagmin-1 binds to PIP(2)-containing membrane but not to SNAREs at physiological ionic strength. Nat. Struct. Mol. Biol. 22 (10), 815–823. doi:10.1038/nsmb.3097
Pelletan, L. E., Suhaiman, L., Vaquer, C. C., Bustos, M. A., De Blas, G. A., Vitale, N., et al. (2015). ADP ribosylation factor 6 (ARF6) promotes acrosomal exocytosis by modulating lipid turnover and Rab3A activation. J. Biol. Chem. 290, 9823–9841. doi:10.1074/jbc.M114.629006
Penalva, D. A., Antollini, S. S., Ambroggio, E. E., Aveldano, M. I., and Fanani, M. L. (2018). Membrane restructuring events during the enzymatic generation of ceramides with very long-chain polyunsaturated fatty acids. Langmuir 34 (14), 4398–4407. doi:10.1021/acs.langmuir.7b04374
Petcoff, D. W., Holland, W. L., and Stith, B. J. (2008). Lipid levels in sperm, eggs, and during fertilization in Xenopus laevis. J. Lipid Res. 49 (11), 2365–2378. doi:10.1194/jlr.M800159-JLR200
Petrache, I., Kamocki, K., Poirier, C., Pewzner-Jung, Y., Laviad, E. L., Schweitzer, K. S., et al. (2013). Ceramide synthases expression and role of ceramide synthase-2 in the lung: insight from human lung cells and mouse models. PLoS One 8 (5), e62968. doi:10.1371/journal.pone.0062968
Picazo-Juarez, G., Romero-Suarez, S., Nieto-Posadas, A., Llorente, I., Jara-Oseguera, A., Briggs, M., et al. (2011). Identification of a binding motif in the S5 helix that confers cholesterol sensitivity to the TRPV1 ion channel. J. Biol. Chem. 286 (28), 24966–24976. doi:10.1074/jbc.M111.237537
Presa, N., Gomez-Larrauri, A., Dominguez-Herrera, A., Trueba, M., and Gomez-Munoz, A. (2020). Novel signaling aspects of ceramide 1-phosphate. Biochim. Biophys. Acta Mol. Cell Biol. Lipids 1865 (4), 158630. doi:10.1016/j.bbalip.2020.158630
Puga Molina, L. C., Pinto, N. A., Torres Rodriguez, P., Romarowski, A., Vicens Sanchez, A., Visconti, P. E., et al. (2017). Essential role of CFTR in PKA-dependent phosphorylation, alkalinization, and hyperpolarization during human sperm capacitation. J. Cell Physiol. 232 (6), 1404–1414. doi:10.1002/jcp.25634
Pyenta, P. S., Holowka, D., and Baird, B. (2001). Cross-correlation analysis of inner-leaflet-anchored green fluorescent protein co-redistributed with IgE receptors and outer leaflet lipid raft components. Biophys. J. 80 (5), 2120–2132. doi:10.1016/S0006-3495(01)76185-1
Raben, D. M., and Barber, C. N. (2017). Phosphatidic acid and neurotransmission. Adv. Biol. Regul. 63, 15–21. doi:10.1016/j.jbior.2016.09.004
Rhee, J. S., Betz, A., Pyott, S., Reim, K., Varoqueaux, F., Augustin, I., et al. (2002). Beta phorbol ester- and diacylglycerol-induced augmentation of transmitter release is mediated by Munc13s and not by PKCs. Cell 108 (1), 121–133. doi:10.1016/s0092-8674(01)00635-3
Roggero, C. M., Tomes, C. N., De Blas, G. A., Castillo, J., Michaut, M. A., Fukuda, M., et al. (2005). Protein kinase C-mediated phosphorylation of the two polybasic regions of synaptotagmin VI regulates their function in acrosomal exocytosis. Dev. Biol. 285 (2), 422–435. doi:10.1016/j.ydbio.2005.07.007
Roldan, E. R., and Shi, Q. X. (2007). Sperm phospholipases and acrosomal exocytosis. Front. Biosci. 12, 89–104. doi:10.2741/2050
Samavat, J., Natali, I., Degl’Innocenti, S., Filimberti, E., Cantini, G., Di Franco, A., et al. (2014). Acrosome reaction is impaired in spermatozoa of obese men: a preliminary study. Fertil. Steril. 102 (5), 1274–1281 e2. doi:10.1016/j.fertnstert.2014.07.1248
Sandhoff, K., and Kolter, T. (2003). Biosynthesis and degradation of mammalian glycosphingolipids. Philos. Trans. R. Soc. Lond B Biol. Sci. 358 (1433), 847–861. doi:10.1098/rstb.2003.1265
Sanocka, D., and Kurpisz, M. (2004). Reactive oxygen species and sperm cells. Reprod. Biol. Endocrinol. 2, 12. doi:10.1186/1477-7827-2-12
Sedes, L., Thirouard, L., Maqdasy, S., Garcia, M., Caira, F., Lobaccaro, J. A., et al. (2018). Cholesterol: a gatekeeper of male fertility? Front. Endocrinol. (Lausanne) 9, 369. doi:10.3389/fendo.2018.00369
Selvaraj, V., Asano, A., Buttke, D. E., McElwee, J. L., Nelson, J. L., Wolff, C. A., et al. (2006). Segregation of micron-scale membrane sub-domains in live murine sperm. J. Cell Physiol. 206 (3), 636–646. doi:10.1002/jcp.20504
Shan, S., Xu, F., Bleyer, M., Becker, S., Melbaum, T., Wemheuer, W., et al. (2020). Association of α/β-Hydrolase D16B with bovine conception rate and sperm plasma membrane lipid composition. Int. J. Mol. Sci. 21 (2), 627. doi:10.3390/ijms21020627
Shan, S., Xu, F., Hirschfeld, M., and Brenig, B. (2021). Sperm lipid markers of male fertility in mammals. Int. J. Mol. Sci. 22 (16), 8767. doi:10.3390/ijms22168767
Shi, J. F., Li, Y. K., Ren, K., Xie, Y. J., Yin, W. D., and Mo, Z. C. (2018). Characterization of cholesterol metabolism in Sertoli cells and spermatogenesis (Review). Mol. Med. Rep. 17 (1), 705–713. doi:10.3892/mmr.2017.8000
Silva, L. C., Ben David, O., Pewzner-Jung, Y., Laviad, E. L., Stiban, J., Bandyopadhyay, S., et al. (2012). Ablation of ceramide synthase 2 strongly affects biophysical properties of membranes. J. Lipid Res. 53 (3), 430–436. doi:10.1194/jlr.M022715
Sim, J. A., Kim, J., and Yang, D. (2020). Beyond lipid signaling: pleiotropic effects of diacylglycerol kinases in cellular signaling. Int. J. Mol. Sci. 21 (18), 6861. doi:10.3390/ijms21186861
Slochower, D. R., Huwe, P. J., Radhakrishnan, R., and Janmey, P. A. (2013). Quantum and all-atom molecular dynamics simulations of protonation and divalent ion binding to phosphatidylinositol 4,5-bisphosphate (PIP2). J. Phys. Chem. B 117 (28), 8322–8329. doi:10.1021/jp401414y
Sosa, C. M., Pavarotti, M. A., Zanetti, M. N., Zoppino, F. C., De Blas, G. A., and Mayorga, L. S. (2015). Kinetics of human sperm acrosomal exocytosis. Mol. Hum. Reprod. 21 (3), 244–254. doi:10.1093/molehr/gau110
Sosa, C. M., Zanetti, M. N., Pocognoni, C. A., and Mayorga, L. S. (2016). Acrosomal swelling is triggered by cAMP downstream of the opening of store-operated calcium channels during acrosomal exocytosis in human sperm. Biol. Reprod. 94 (3), 57. doi:10.1095/biolreprod.115.133231
Sosnicki, D. M., Cohen, R., Asano, A., Nelson, J. L., Mukai, C., Comizzoli, P., et al. (2023). Segmental differentiation of the murine epididymis: identification of segment-specific, GM1-enriched vesicles and regulation by luminal fluid factors†. Biol. Reprod. 109 (6), 864–877. doi:10.1093/biolre/ioad120
Spiegel, S., and Milstien, S. (2003). Exogenous and intracellularly generated sphingosine 1-phosphate can regulate cellular processes by divergent pathways. Biochem. Soc. Trans. 31 (Pt 6), 1216–1219. doi:10.1042/bst0311216
Spungin, B., Levinshal, T., Rubinstein, S., and Breitbart, H. (1992). A cell free system reveals that capacitation is a prerequisite for membrane fusion during the acrosome reaction. FEBS Lett. 311 (2), 155–160. doi:10.1016/0014-5793(92)81388-3
Sridevi, P., Alexander, H., Laviad, E. L., Pewzner-Jung, Y., Hannink, M., Futerman, A. H., et al. (2009). Ceramide synthase 1 is regulated by proteasomal mediated turnover. Biochim. Biophys. Acta 1793 (7), 1218–1227. doi:10.1016/j.bbamcr.2009.04.006
Stith, B. J. (2015). Phospholipase C and D regulation of Src, calcium release and membrane fusion during Xenopus laevis development. Dev. Biol. 401 (2), 188–205. doi:10.1016/j.ydbio.2015.02.020
Stival, C., Puga Molina Ldel, C., Paudel, B., Buffone, M. G., Visconti, P. E., and Krapf, D. (2016). Sperm capacitation and acrosome reaction in mammalian sperm. Adv. Anat. Embryol. Cell Biol. 220, 93–106. doi:10.1007/978-3-319-30567-7_5
Suh, B. C., and Hille, B. (2005). Regulation of ion channels by phosphatidylinositol 4,5-bisphosphate. Curr. Opin. Neurobiol. 15 (3), 370–378. doi:10.1016/j.conb.2005.05.005
Suh, B. C., Kim, D. I., Falkenburger, B. H., and Hille, B. (2012). Membrane-localized β-subunits alter the PIP2 regulation of high-voltage activated Ca2+ channels. Proc. Natl. Acad. Sci. U. S. A. 109 (8), 3161–3166. doi:10.1073/pnas.1121434109
Suh, B. C., Leal, K., and Hille, B. (2010). Modulation of high-voltage activated Ca(2+) channels by membrane phosphatidylinositol 4,5-bisphosphate. Neuron 67 (2), 224–238. doi:10.1016/j.neuron.2010.07.001
Suhaiman, L., Altamirano, K. N., Morales, A., and Belmonte, S. A. (2021). Different approaches to record human sperm exocytosis. Methods Mol. Biol. 2233, 139–168. doi:10.1007/978-1-0716-1044-2_10
Suhaiman, L., De Blas, G. A., Obeid, L. M., Darszon, A., Mayorga, L. S., and Belmonte, S. A. (2010). Sphingosine 1-phosphate and sphingosine kinase are involved in a novel signaling pathway leading to acrosomal exocytosis. J. Biol. Chem. 285 (21), 16302–16314. doi:10.1074/jbc.M109.072439
Sundaram, R. V. K., Chatterjee, A., Bera, M., Grushin, K., Panda, A., Li, F., et al. (2023). Novel roles for diacylglycerol in synaptic vesicle priming and release revealed by complete reconstitution of core protein machinery. bioRxiv, 2023.06.05.543781. doi:10.1101/2023.06.05.543781
Suzuki, R., Saino-Saito, S., Sakagami, H., Toshimori, K., Abe, H., and Kondo, H. (2009). Localization of EFA6A, a guanine nucleotide exchange factor for ARF6, in spermatogenic cells of testes of adult mice. J. Mol. Histol. 40 (1), 77–80. doi:10.1007/s10735-008-9207-9
Szkodziak, P., Wozniak, S., Czuczwar, P., Wozniakowska, E., Milart, P., Mroczkowski, A., et al. (2016). Infertility in the light of new scientific reports - focus on male factor. Ann. Agric. Environ. Med. 23 (2), 227–230. doi:10.5604/12321966.1203881
Tang, N., Ong, W. Y., Zhang, E. M., Chen, P., and Yeo, J. F. (2007). Differential effects of ceramide species on exocytosis in rat PC12 cells. Exp. Brain Res. 183 (2), 241–247. doi:10.1007/s00221-007-1036-7
Tanguy, E., Coste de Bagneaux, P., Kassas, N., Ammar, M. R., Wang, Q., Haeberle, A. M., et al. (2020). Mono- and poly-unsaturated phosphatidic acid regulate distinct steps of regulated exocytosis in neuroendocrine cells. Cell Rep. 32 (7), 108026. doi:10.1016/j.celrep.2020.108026
Tanguy, E., Wolf, A., Montero-Hadjadje, M., Gasman, S., Bader, M. F., and Vitale, N. (2021). Phosphatidic acid: mono- and poly-unsaturated forms regulate distinct stages of neuroendocrine exocytosis. Adv. Biol. Regul. 79, 100772. doi:10.1016/j.jbior.2020.100772
Tanguy, E., Wolf, A., Wang, Q., Chasserot-Golaz, S., Ory, S., Gasman, S., et al. (2022). Phospholipase D1-generated phosphatidic acid modulates secretory granule trafficking from biogenesis to compensatory endocytosis in neuroendocrine cells. Adv. Biol. Regul. 83, 100844. doi:10.1016/j.jbior.2021.100844
Tanphaichitr, N., Kongmanas, K., Faull, K. F., Whitelegge, J., Compostella, F., Goto-Inoue, N., et al. (2018). Properties, metabolism and roles of sulfogalactosylglycerolipid in male reproduction. Prog. Lipid Res. 72, 18–41. doi:10.1016/j.plipres.2018.08.002
Tapia, J. A., Macias-Garcia, B., Miro-Moran, A., Ortega-Ferrusola, C., Salido, G. M., Pena, F. J., et al. (2012). The membrane of the mammalian spermatozoa: much more than an inert envelope. Reprod. Domest. Anim. 47 (Suppl. 3), 65–75. doi:10.1111/j.1439-0531.2012.02046.x
Tomes, C. N. (2007). Molecular mechanisms of membrane fusion during acrosomal exocytosis. Soc. Reprod. Fertil. Suppl. 65, 275–291.
Travis, A. J., Merdiushev, T., Vargas, L. A., Jones, B. H., Purdon, M. A., Nipper, R. W., et al. (2001). Expression and localization of caveolin-1, and the presence of membrane rafts, in mouse and Guinea pig spermatozoa. Dev. Biol. 240 (2), 599–610. doi:10.1006/dbio.2001.0475
Trevino, C. L., Jl, D. l. V.-B., Nishigaki, T., Felix, R., and Darszon, A. (2006). Maitotoxin potently promotes Ca2+ influx in mouse spermatogenic cells and sperm, and induces the acrosome reaction. J. Cell Physiol. 206 (2), 449–456. doi:10.1002/jcp.20487
Trexler, A. J., and Taraska, J. W. (2017). Regulation of insulin exocytosis by calcium-dependent protein kinase C in beta cells. Cell Calcium 67, 1–10. doi:10.1016/j.ceca.2017.07.008
Tsai, P. S., De Vries, K. J., De Boer-Brouwer, M., Garcia-Gil, N., Van Gestel, R. A., Colenbrander, B., et al. (2007). Syntaxin and VAMP association with lipid rafts depends on cholesterol depletion in capacitating sperm cells. Mol. Membr. Biol. 24 (4), 313–324. doi:10.1080/09687860701228692
Tsentsevitsky, A. N., Gafurova, C. R., Mukhutdinova, K. A., Giniatullin, A. R., Fedorov, N. S., Malomouzh, A. I., et al. (2023). Sphingomyelinase modulates synaptic vesicle mobilization at the mice neuromuscular junctions. Life Sci. 318, 121507. doi:10.1016/j.lfs.2023.121507
Tvrda, E., Benko, F., Slanina, T., and du Plessis, S. S. (2021). The role of selected natural biomolecules in sperm production and functionality. Molecules 26 (17), 5196. doi:10.3390/molecules26175196
Vallet-Buisan, M., Mecca, R., Jones, C., Coward, K., and Yeste, M. (2023). Contribution of semen to early embryo development: fertilization and beyond. Hum. Reprod. Update 29 (4), 395–433. doi:10.1093/humupd/dmad006
van den Bogaart, G., Meyenberg, K., Diederichsen, U., and Jahn, R. (2012). Phosphatidylinositol 4,5-bisphosphate increases Ca2+ affinity of synaptotagmin-1 by 40-fold. J. Biol. Chem. 287, 16447–16453. doi:10.1074/jbc.M112.343418
Vaquer, C. C., Suhaiman, L., Pavarotti, M. A., Arias, R. J., Pacheco Guinazu, A. B., De Blas, G. A., et al. (2023). The pair ceramide 1-phosphate/ceramide kinase regulates intracellular calcium and progesterone-induced human sperm acrosomal exocytosis. Front. Cell Dev. Biol. 11, 1148831. doi:10.3389/fcell.2023.1148831
Vaquer, C. C., Suhaiman, L., Pavarotti, M. A., De Blas, G. A., and Belmonte, S. A. (2020). Ceramide induces a multicomponent intracellular calcium increase triggering the acrosome secretion in human sperm. Biochim. Biophys. Acta Mol. Cell Res. 1867 (7), 118704. doi:10.1016/j.bbamcr.2020.118704
Vazquez, J. M., and Roldan, E. R. (1997). Diacylglycerol species as messengers and substrates for phosphatidylcholine re-synthesis during Ca2+-dependent exocytosis in boar spermatozoa. Mol. Reprod. Dev. 48 (1), 95–105. doi:10.1002/(SICI)1098-2795(199709)48:1<95::AID-MRD12>3.0.CO;2-V
Visconti, P. E. (2009). Understanding the molecular basis of sperm capacitation through kinase design. Proc. Natl. Acad. Sci. U. S. A. 106 (3), 667–668. doi:10.1073/pnas.0811895106
Vitale, N. (2010). Synthesis of fusogenic lipids through activation of phospholipase D1 by GTPases and the kinase RSK2 is required for calcium-regulated exocytosis in neuroendocrine cells. Biochem. Soc. Trans. 38, 167–171. doi:10.1042/BST0380167
Wang, G., Guo, Y., Zhou, T., Shi, X., Yu, J., Yang, Y., et al. (2013). In-depth proteomic analysis of the human sperm reveals complex protein compositions. J. Proteomics 79, 114–122. doi:10.1016/j.jprot.2012.12.008
Wang, Y. H., Slochower, D. R., and Janmey, P. A. (2014). Counterion-mediated cluster formation by polyphosphoinositides. Chem. Phys. Lipids 182, 38–51. doi:10.1016/j.chemphyslip.2014.01.001
Weisgerber, A. W., Otruba, Z., and Knowles, M. K. (2024). Syntaxin clusters and cholesterol affect the mobility of Syntaxin1a. Biophys. J. doi:10.1016/j.bpj.2024.01.012
Williams, D., Vicogne, J., Zaitseva, I., McLaughlin, S., and Pessin, J. E. (2009). Evidence that electrostatic interactions between vesicle-associated membrane protein 2 and acidic phospholipids may modulate the fusion of transport vesicles with the plasma membrane. Mol. Biol. Cell 20 (23), 4910–4919. doi:10.1091/mbc.e09-04-0284
Won, J. H., Kim, S. K., Shin, I. C., Ha, H. C., Jang, J. M., Back, M. J., et al. (2018). Dopamine transporter trafficking is regulated by neutral sphingomyelinase 2/ceramide kinase. Cell Signal 44, 171–187. doi:10.1016/j.cellsig.2018.01.006
Xue, R., Zhao, Y., and Chen, P. (2009). Involvement of PKC alpha in PMA-induced facilitation of exocytosis and vesicle fusion in PC12 cells. Biochem. Biophys. Res. Commun. 380 (2), 371–376. doi:10.1016/j.bbrc.2009.01.105
Yanagimachi, R. (1994). “Mammalian fertilization,” in The physiology of reproduction. Editors E. Knobil, and J. D. Neill (New York: Raven Press), 189–317.
Zalata, A., Hassan, A., Christophe, A., Comhaire, F., and Mostafa, T. (2010). Cholesterol and desmosterol in two sperm populations separated on Sil-Select gradient. Int. J. Androl. 33 (3), 528–535. doi:10.1111/j.1365-2605.2009.00961.x
Zalata, A. A., Christophe, A. B., Depuydt, C. E., Schoonjans, F., and Comhaire, F. H. (1998a). The fatty acid composition of phospholipids of spermatozoa from infertile patients. Mol. Hum. Reprod. 4 (2), 111–118. doi:10.1093/molehr/4.2.111
Zalata, A. A., Christophe, A. B., Depuydt, C. E., Schoonjans, F., and Comhaire, F. H. (1998b). White blood cells cause oxidative damage to the fatty acid composition of phospholipids of human spermatozoa. Int. J. Androl. 21 (3), 154–162. doi:10.1046/j.1365-2605.1998.00112.x
Zanetti, N., and Mayorga, L. S. (2009). Acrosomal swelling and membrane docking are required for hybrid vesicle formation during the human sperm acrosome reaction. Biol. Reprod. 81 (2), 396–405. doi:10.1095/biolreprod.109.076166
Zanetti, S. R., de Los Angeles, M. M., Rensetti, D. E., Fornes, M. W., and Aveldano, M. I. (2010a). Ceramides with 2-hydroxylated, very long-chain polyenoic fatty acids in rodents: from testis to fertilization-competent spermatozoa. Biochimie 92 (12), 1778–1786. doi:10.1016/j.biochi.2010.08.012
Zanetti, S. R., Monclus, M. L., Rensetti, D. E., Fornes, M. W., and Aveldano, M. I. (2010b). Differential involvement of rat sperm choline glycerophospholipids and sphingomyelin in capacitation and the acrosomal reaction. Biochimie 92 (12), 1886–1894. doi:10.1016/j.biochi.2010.08.015
Zeniou-Meyer, M., Zabari, N., Ashery, U., Chasserot-Golaz, S., Haeberle, A. M., Demais, V., et al. (2007). Phospholipase D1 production of phosphatidic acid at the plasma membrane promotes exocytosis of large dense-core granules at a late stage. J. Biol. Chem. 282 (30), 21746–21757. doi:10.1074/jbc.M702968200
Zhao, R., Dai, H., Arias, R. J., De Blas, G. A., Orta, G., Pavarotti, M. A., et al. (2021). Direct activation of the proton channel by albumin leads to human sperm capacitation and sustained release of inflammatory mediators by neutrophils. Nat. Commun. 12 (1), 3855. doi:10.1038/s41467-021-24145-1
Zitranski, N., Borth, H., Ackermann, F., Meyer, D., Vieweg, L., Breit, A., et al. (2010). The “acrosomal synapse”: subcellular organization by lipid rafts and scaffolding proteins exhibits high similarities in neurons and mammalian spermatozoa. Commun. Integr. Biol. 3 (6), 513–521. doi:10.4161/cib.3.6.13137
Keywords: acrosome exocytosis, human sperm, lipids, cholesterol, sphingolipids, phospholipids, membrane fusion
Citation: Suhaiman L and Belmonte SA (2024) Lipid remodeling in acrosome exocytosis: unraveling key players in the human sperm. Front. Cell Dev. Biol. 12:1457638. doi: 10.3389/fcell.2024.1457638
Received: 01 July 2024; Accepted: 03 September 2024;
Published: 23 September 2024.
Edited by:
Parijat Sarkar, Stanford University, United StatesReviewed by:
Alberto Darszon, National Autonomous University of Mexico, MexicoElisabeth Pinart, University of Girona, Spain
Copyright © 2024 Suhaiman and Belmonte. This is an open-access article distributed under the terms of the Creative Commons Attribution License (CC BY). The use, distribution or reproduction in other forums is permitted, provided the original author(s) and the copyright owner(s) are credited and that the original publication in this journal is cited, in accordance with accepted academic practice. No use, distribution or reproduction is permitted which does not comply with these terms.
*Correspondence: Silvia A. Belmonte, c2lsdmlhLmJlbG1vbnRlQGZjbS51bmN1LmVkdS5hcg==
†ORCID: Laila Suhaiman, orcid.org/0000-0001-8376-2673