- 1Laboratory of Skeletal Development and Regeneration, Key Laboratory of Clinical Laboratory Diagnostics (Ministry of Education), College of Laboratory Medicine, Chongqing Medical University, Chongqing, China
- 2Department of Clinical Application, Center for iPS Cell Research and Application (CiRA), Kyoto University, Kyoto, Japan
Fibro/adipogenic progenitors (FAPs) that reside in muscle tissue are crucial for muscular homeostasis and regeneration as they secrete signaling molecules and components of the extracellular matrix. During injury or disease, FAPs differentiate into different cell types and significantly modulate muscular function. Recent advances in lineage tracing and single-cell transcriptomics have proven that FAPs are heterogeneous both in resting and post-injury or disease states. Their heterogeneity may be owing to the varied tissue microenvironments and their diverse developmental origins. Therefore, understanding FAPs’ developmental origins can help predict their characteristics and behaviors under different conditions. FAPs are thought to be the major cell populations in the muscle connective tissue (MCT). During embryogenesis, the MCT directs muscular development throughout the body and serves as a prepattern for muscular morphogenesis. The developmental origins of FAPs as stromal cells in the MCT were studied previously. In adult tissues, FAPs are important precursors for heterotopic ossification, especially in the context of the rare genetic disorder fibrodysplasia ossificans progressiva. A new developmental origin for FAPs have been suggested that differs from conventional developmental perspectives. In this review, we summarize the developmental origins and functions of FAPs as stromal cells of the MCT and present novel insights obtained by using patient-derived induced pluripotent stem cells and mouse models of heterotopic ossification. This review broadens the current understanding of FAPs and suggests potential avenues for further investigation.
1 Introduction
In 2010, fibro/adipogenesis-associated muscle-resident cells were identified in adult mice based on PDGFRα expression (Joe et al., 2010; Uezumi et al., 2010). These cells were isolated using the expression of specific surface antigens, such as Sca1 and Tie2, while excluding muscle satellite (SM/C-2.6+), hematopoietic (CD45+), and endothelial (CD31+) cell lineages and were termed fibro/adipogenic progenitors (FAPs). In 2014, FAPs from human skeletal muscle were also identified (Uezumi et al., 2014). FAPs reportedly orchestrate regenerative signals via paracrine functions to modulate the proliferation, differentiation, and myogenic capacity of muscle stem cells in various body regions (Farup et al., 2015; Wosczyna et al., 2019; Kim et al., 2022). They are the primary source of cells for regenerative matrix deposition and contribute to fibrous deformation/fatty infiltration in muscle tissues through differentiation into fibroblasts and adipocytes, particularly in the context of muscle damage and neuromuscular diseases (Cordani et al., 2014; Lemos et al., 2015; Contreras et al., 2016; Gonzalez et al., 2017; Malecova et al., 2018; Moratal et al., 2018; Moratal et al., 2019; Farup et al., 2021; Engquist et al., 2024; Flores-Opazo et al., 2024).
FAPs’ diversity and heterogeneity have hindered the understanding of their characteristics and predicting their functions and behaviors. FAPs have been found to exhibit altered gene regulatory networks or express state-specific markers under different conditions, which in turn regulates their fate and plasticity. Therefore, Resting FAPs in undamaged mouse muscles (Scott et al., 2019; De Micheli et al., 2020a; Oprescu et al., 2020) and FAPs activated during muscle regeneration (Malecova et al., 2018; Scott et al., 2019; De Micheli et al., 2020a; De Micheli et al., 2020b; Oprescu et al., 2020; Rubenstein et al., 2020) are heterogeneous populations with different functions or at different stages. Their heterogeneity may be caused by varied muscle tissue microenvironments and their diverse developmental pathways. Tracing the developmental origins of FAPs is an important entry point for understanding their intrinsic properties. During embryonic muscle development, FAPs are identified as the main sources of developmental extracellular matrix (ECM). Genetic lineage tracing has shown that the transcription factor odd-skipped-related 1 (Osr1) marks a subpopulation of FAP-like cells that supports myogenesis by promoting myogenic progenitor proliferation and survival (Vallecillo-García et al., 2017). Adult FAPs are reported to share a common lineage with embryonic muscle connective tissue (MCT) cells, which are responsible for the development of the macroscopic and microscopic attributes of muscle (Nassari et al., 2017; Sefton and Kardon, 2019).
MCT cells, the primary cell type in the muscle tissue, are responsible for producing and depositing ECM components, such as fibrous collagen and proteoglycans (Light and Champion, 1984). They are associated with the healing process following muscle injury and exhibit phenotypic characteristics akin to those of fibroblasts (Williams and Goldspink, 1984; Gatchalian et al., 1989; Schmitt-Gräff et al., 1994; Gabbiani, 1998; Chapman et al., 2017). With contributions from many researchers, it has been established that adult MCT is primarily derived from muscle-resident PDGFRα+ FAPs. These cells exhibit the characteristics of tissue-resident mesenchymal stem/progenitor cells (MSCs/MPCs) with multilineage differentiation potential and a fibroblast-like phenotype (Nesti et al., 2008; Jackson et al., 2009; Judson et al., 2013; Scott et al., 2019). However, these discoveries have led to some confusion in terminology. Contreras et al. (2021) proposed that these muscle-resident multipotent progenitor cells, whether called FAPs or fibroblasts, are essentially the same cell populations in studies related to MCT. Although careful identification of these cell types and the use of context-appropriate terminology are necessary, this review integrates previous literature on the developmental origins of FAPs by adopting the perspective that MCT cells serve as a collective term encompassing fibroblasts, MSCs/MPCs, and FAPs.
Relevant studies have provided valuable prospects into the origins of FAPs. As the primary precursor cells give rise to heterotopic ossification (HO) (Wosczyna et al., 2012), recent investigations utilizing patient-derived induced pluripotent stem cells (iPSCs) and mouse models have introduced new perspectives on FAPs’ embryonic origins. We discuss the conventional developmental perspectives on the origins and functions of MCT cells/FAPs during myogenesis and introduce novel insights emerging from diseased iPSCs and mouse models to deepen our understanding of related research field.
2 Embryonic origins and roles of MCT cells and FAPs during muscle development
Muscle formation and development involve two parallel processes: myogenesis and morphogenesis. Myogenesis involves the formation of muscle progenitor cells that express Pax3 and Pax7, followed by differentiation into myoblasts. Committed myoblasts then proliferate, express myogenic regulatory factors (including Myf5, MyoD, and myogenin), undergo morphological changes, and fuse to form multinucleated muscle fibers (Rudnicki et al., 1992; Braun and Gautel, 2011; Abmayr and Pavlath, 2012; Sampath et al., 2018) (Figure 1A). Early development and initial differentiation into myoblasts are not controlled by MCT cells. Instead, the MCT stromal cells control multiple aspects of muscle morphogenesis (Sefton and Kardon, 2019). They direct myoblasts to migrate to the target region, as well as promote expansion, viability, and maturation of adjacent myoblasts into myofibers by secreting complex signaling molecules and ECM components (Bladt et al., 1995; Dietrich et al., 1999; Vasyutina et al., 2005; Hasson et al., 2010; Swartz et al., 2012; Iwata et al., 2013; Vallecillo-García et al., 2017; Stumm et al., 2018) (Figure 1B). MCT may act as a pre-model to determine the location of myofiber differentiation, which in turn determines the quantity, position, scale, and structure of a muscle. The following sections introduce the origins and functions of MCT cells/FAPs in major regions of the body.
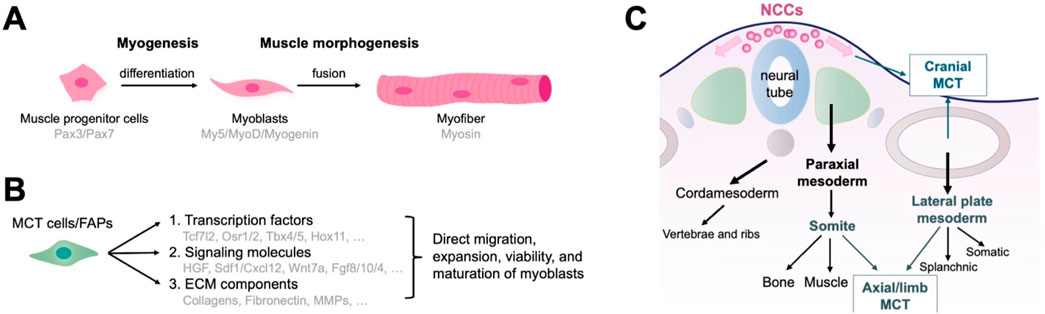
Figure 1. Function and embryonic origin of fibro/adipogenic progenitors (FAPs) as muscle connective tissue (MCT) cells during muscle development. (A) The development of muscle tissue. Myogenesis encompasses the formation of muscle progenitor cells, differentiation into myoblasts, and the fusion of myoblasts into multinucleated muscle fibers. (B) MCT cells control multiple aspects of muscle morphogenesis by directing myoblasts to migrate to the target region and promoting their expansion, viability, and maturation through the production of signaling molecules and ECM components. (C) Embryonic origin of MCTs representing the traditional developmental biology perspectives. Axial MCT originates from the somites and lateral plate mesoderm, limb MCT from the lateral plate mesoderm, and cranial MCT from the neural crest.
2.1 Roles of MCT/FAPs in development of axial muscles
The axial or trunk muscles adjacent to the dorsal notochord, vertebrae, and abdomen originate from somites of the paraxial mesoderm (Buckingham, 2006; Bryson-Richardson and Currie, 2008; Wotton et al., 2015). Lineage studies using Prx1Cre transgenic mice identified two distinct developmental origins of axial muscle MCT: 1. MCT surrounding the distal intercostal, pectoralis, transverse, internal/external oblique, and rectus abdominis (medial/lateral muscles) originate from the lateral plate mesoderm; 2. MCT surrounding the intercostal and longissimus (proximal muscles) are derived from somites (Durland et al., 2008) (Figure 1C). Additionally, ScxGFP-transgenic mice, marking syndetome and tenocyte cells, also labeled MCT cells in some lateral muscles (Deries et al., 2010; Ono et al., 2023). Morphogenesis of the medial/lateral and proximal muscles is closely linked to ECM alterations concerning MCT of the lateral plate mesoderm and somite. MCT-derived fibronectin may contribute to this stage (Deries et al., 2010; Deries et al., 2012). Fat1 is associated with planar cell polarity and plays a crucial role in the distribution and differentiation of myoblasts. Fat1 absence in the lateral plate mesoderm of Prx1-expressing cells causes myofibril dysfunction and low count of myogenic cells, implying that mesenchymal cells derived from the lateral plate mesoderm control axial muscle morphogenesis by secreting ECM and regulating critical signaling pathways (Helmbacher, 2018). Furthermore, the axial muscles, tendons, and bones are derived from different somite regions, and their spatial interrelationships enable coordinated development. MCT derived from somite and lateral plate mesoderm likely regulate these interactions.
2.2 MCT/FAPs and limb muscle formation
The first step in limb muscle morphogenesis is the migration of myoblasts from the somites to the limbs. Once in the limbs, myoblasts undergo a complex morphogenetic process that ultimately forms the limb muscle pattern.
During the initial migration, attraction and repulsion signals in the limb bud mesoderm, produced by MCT cells derived from embryonic lateral plate mesoderm, are essential at this stage (Helmbacher and Stricker, 2020). For instance, stromal cell-derived factor 1 (SDF1 or Cxcl12) produced in this area, binds to CXCR4 produced by migrating myoblasts, actively regulating their survival and entry into the limbs (Vasyutina et al., 2005). Meanwhile, the ligand EphrinA5 expressed in the MCT repels myoblasts that express the receptor Epha4, preventing their settlement in the limb regions (Swartz et al., 2001).
In the subsequent step, the prepattern established by limb MCT determines the differentiation location and the number of myoblasts. Several key transcription factors, which have been identified in mouse MCT, importantly regulate this process. Knockout of the T-box transcription factors Tbx5 and Tbx4, respectively, cause misplacement of the forelimb and hindlimb muscles (Agarwal et al., 2003; Hasson et al., 2007; Naiche and Papaioannou, 2007). Tcf7l2 mutations cause truncation of the muscle near the knee and affect myofiber development and profile (Mathew et al., 2011). Tbx3-mutant mice lack two specific anterior muscles: the lateral triceps and brachialis (Colasanto et al., 2016). Compound mutations in Hoxa11 and Hoxd11 cause abnormalities in the forearm and calf muscles (Swinehart et al., 2013).
In summary, the MCT cells of the lateral plate mesodermal origin is essential for limb muscle morphogenesis. Activities of the signaling and transcription factors determine the position and number of myoblasts in the limbs, thereby influencing muscular pattern formation.
2.3 MCT/FAPs in cranial muscle development
The head muscles and MCT develop from diverse origins. The cranial mesoderm is the primary source of head muscles. It is positioned bilaterally along the neural tube and spans somites across the forebrain during embryonic development (Michailovici et al., 2015; Ziermann et al., 2018). MCT cells within the head muscles typically derives from the cranial neural crest. Moreover, neck muscles derive from somites and the head mesoderm. The MCT cells of the neck originates in the neural crest and lateral plate mesoderm (Matsuoka et al., 2005; Durland et al., 2008; Lescroart et al., 2015; Sefton and Kardon, 2019) (Figure 1C).
Formation of head muscles is initiated by the simultaneous migration of myogenic and neural crest cells (NCCs), accompanied by myoblast specialization. Within the pharynx, the myogenic cells constitute the central structure of each arch. Neural crest-derived MCT cells surround and invade them, causing significant mixing of both cell types. Simultaneously, myogenic cells begin myogenesis and specialize into myoblasts (Trainor et al., 1994; Grenier et al., 2009). Although neural crest-derived MCT cells are not required for the initiation of craniomandibular myogenesis (Heude et al., 2018), they critically regulate the migration, stereotyping, and differentiation of craniofacial myogenic precursors (Tzahor et al., 2003; Rinon et al., 2007). Deletion of Pitx2 in NCCs in Wnt1Cre mice proves that it crucially regulates positioning of the extraocular muscles (Evans and Gage, 2005). Deletion of the transcription factors Dlx5 and Dlx6 in the murine cranial NCCs causes mandibular absence, indicating that they are critical for morphogenesis of jaw muscles (Heude et al., 2010). Lingual muscle development commences with the migration of myogenic and NCCs into the tongue bud (Han et al., 2012). Conditional knockout studies in mice indicated that cilium-dependent GLI in the neural crest is crucial for the viability and movement of myogenic cells toward the bud (Millington et al., 2017). Thus, signaling by the MCT cells originated from neural crest modulates myoblast expansion and maturation, eventually developing the cranial muscle patterns.
3 Novel insights into the origin of FAPs: perspectives from iPSCs and mouse models of HO
Studies based on traditional perspectives, which classify FAPs as MCT cells, have provided a comprehensive understanding of the embryonic origins of MCT/FAPs and their roles in muscle development (Table 1). However, it remains a challenge to confirm whether the FAPs present in different regions of adult muscle are completely originate from the MCTs described in the previous sections. In recent years, studies related to HO using patient-derived iPSCs and mouse models have provided new insights into the origin of FAPs.
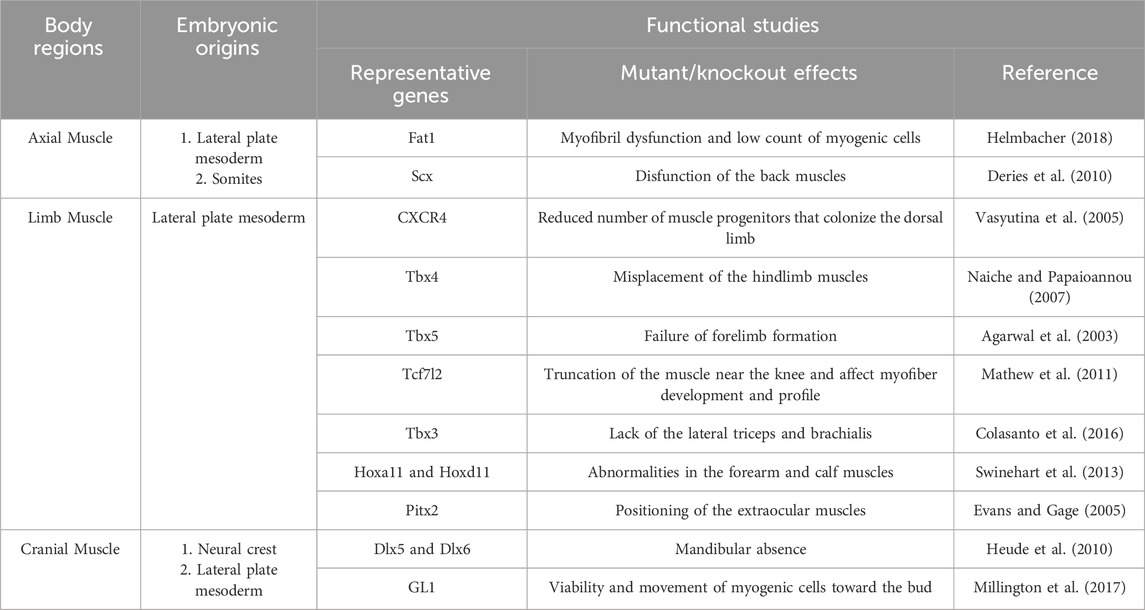
Table 1. Embryonic origins and function of fibro/adipogenic progenitors as muscle connective tissue stromal cells in major regions of the body.
3.1 FAPs are the principal precursor cells for HO
HO is abnormal bone formation in soft tissues, such as muscles, tendons, and ligaments. HO has two main subtypes: acquired and hereditary. Acquired HO is a common complication of major injuries to connective tissues, traumatic injuries to the central nervous system, and surgical interventions, and may cause pain and postoperative disability. Fibrodysplasia ossificans progressiva (FOP) is a rare but destructive form of hereditary HO that is caused by ACVR1 mutations, which activate the bone morphogenetic protein (BMP) signaling pathway and subsequent ossification. FOP usually begins during childhood and manifests as soft-tissue swelling and inflammatory episodes that may progress to form ectopic bones (Shore et al., 2006; Kaplan et al., 2012).
FAPs residing in the interstitium of skeletal muscles, labeled with Tie2Cre, PDGFRα, and Sca-1, are identified as the major precursor cells driving HO (Uezumi et al., 2010; Wosczyna et al., 2012). Despite evidence from various studies suggesting that other cell types, including Prrx1+ skeletal progenitor cells (Chakkalakal et al., 2016; Hesse, 2016), Scx+ tendon-derived progenitor and muscle-resident interstitial Mx1+ population (Dey et al., 2016) and Tie2-expressing endothelial-related cells (Medici et al., 2010), as potential contributors to HO lesions, lineage-tracing studies have demonstrated the decisive role of FAPs in HO. PDGFRα-expressing cells labeled with fusion of CreERT and glutamate–aspartate transporter (GLAST–CreERT) or α-smooth muscle actin (αSMA–CreERT2) significantly contribute at all stages of BMP-induced HO (Kan et al., 2013; Matthews et al., 2016). The FAPs are identified as the major cell population in muscles capable of ectopically depositing cartilage or bone after BMP2 injection or overexpression (Lees-Shepard et al., 2018; Wang et al., 2020; Eisner et al., 2020). In models of HO induced by burns or tendon excisions, highly proliferative PDGFRα+ MSC-like cells accumulate at injury sites, subsequently generating HO (Agarwal et al., 2016; Agarwal et al., 2017). Furthermore, mouse model expressing ACVR1 (R206H) in FAPs recapitulates the full HO spectrum observed in patients with FOP, including injury-induced and spontaneous HO production (Lees-Shepard et al., 2018). These studies collectively demonstrate that FAPs give rise to HO, whether acquired or owing to FOP. Originally defined by their fibrogenic and adipogenic capabilities, the term ‘FAPs’ did not consider their chondrogenic and osteogenic potentials revealed in recent HO studies. A reconsideration of this terminology might better reflect the evolving understanding of these progenitors and their diverse functions.
3.2 New perspectives from iPSC-based disease models derived from patients with FOP
Studies of iPSCs derived from patients with FOP have provided new insights into FAPs’ origins. Many studies on the pathogenic mechanisms of FOP have relied on animal experiments; however, specific mechanisms in humans remain largely unknown. Additionally, obtaining clinical samples for FOP research is difficult, with triggering factors such as surgery possibly complicating the patients’ conditions. Human iPSCs derived from patients with FOP provide unique opportunities to study the mechanisms underlying the human disease. We previously generated a series of iPSC lines using the dermal fibroblasts of patients with FOP (FOP-iPSCs) (Matsumoto et al., 2013). Rescued iPSC clones (resFOP-iPSCs), with the pathogenic ACVR1R206H mutation was corrected by BAC-based homologous recombination to correct the FOP mutation (617G > A) existing in exon 7, were generated using FOP-iPSCs as genetically matched controls. Using a stepwise induction method that mimics embryonic development (Fukuta et al., 2014), induced MSCs (iMSCs) were generated from resFOP/FOP-iPSCs using the NCC lineage. Using these methods, we previously constructed a human-derived in vitro disease model of FOP (Matsumoto et al., 2015). In FOP-iMSCs, BMP and transforming growth factor (TGF)-β signaling were stronger compared to resFOP-iMSCs. FOP-iMSCs exhibited enhanced chondrogenic capacity, which is crucial for promoting endochondral ossification. Furthermore, enhanced mineralization was confirmed in FOP-iMSCs cultured in a mineralization medium.
Research has shown that chondroprogenitor cells derived from FOP-iPSCs via sclerotome, the developmental origin of tendons and ligaments, did not display enhanced chondrogenesis (Nakajima et al., 2018). These results indicate target cells induced via a specific pathway that exhibit a disease-prone phenotype might to some content reflect the cellular origins of the disease. MSCs derived from NCCs could be a potential cellular origin for ectopic bone in FOP (Figure 2A). Consist with these observations, by tracing cell lineages in mice, we investigated the correlation between NCC-derived FAPs and HO by different stimuli in mouse limbs. NCCs were marked in mice using P0-Cre or Wnt1-Cre (P0, migrating NCCs; Wnt1, dorsal neural tube before migration to neural crest) with a floxed LacZ reporter gene. In HO tissues induced by BMP7 injection, approximately 80% of cells positive for P0-Cre and Wnt1-Cre were co-labeled with the osteoblast marker SP7 and co-localized with regions positive for COL2 and COL1, which label the cartilage and bone matrix. Thus, NCCs are the origin for ectopic formation of cartilage and bone. Moreover, expression FOP-ACVR1 specifically in the P0-lineage cells was sufficient to cause HO under CTX-induced muscle injury. BMP7-induced P0-Cre-positive and Wnt1-Cre-positive cells, as well as RFP-labeled P0-Cre-FOP-ACVR1-expressing cells, co-stained with approximately 90% of the PDGFRα- and Vimentin-expressing cells in HO tissues (Zhao et al., 2023). These findings affirm the pivotal contribution of FAPs originating from the neural crest to the pathogenesis of limb HO, regardless of etiology (Figure 2B). However, both the aforementioned results and other studies suggest that NCC-derived FAPs are not the exclusive source of HO. For instance, research has indicated that Prx1-Cre labeled mesoderm-derived adipose precursor cells are found in the muscle interstitium (Krueger et al., 2014). This may suggest the presence of HO-related mesenchymal precursors with distinct developmental origins. Additionally, another study using WNT1-Cre/R26-YFP mice did not find NCC-derived FAPs in the interstitial space of uninjured limb muscle (tibialis anterior) (Lemos et al., 2012). This discrepancy with the aforementioned study may be due to differences in the YFP and LacZ marking methods, or variations in the muscle microenvironment of the model mice under static conditions. Further validation from different perspectives, such as comparing the proportions of corresponding cells in injured limb muscle or HO tissue between the two model mice, would provide greater clarity on these findings.
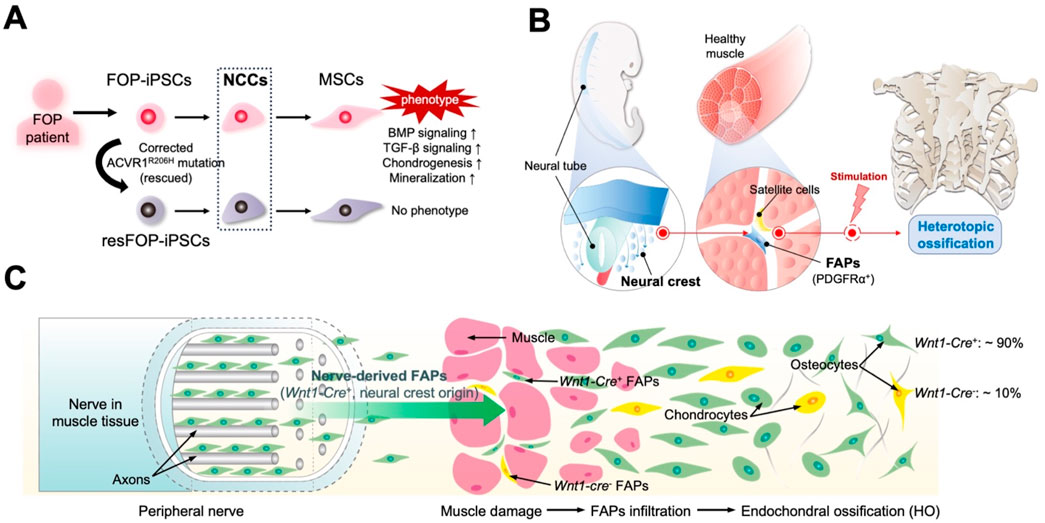
Figure 2. Novel perspectives developed by studying patient-derived induced pluripotent stem cells (iPSCs) and mouse models. (A) Mesenchymal stem cells (MSCs) derived from fibrodysplasia ossificans progressiva (FOP) patient-derived iPSCs (FOP-iPSCs) via neural crest cells (NCCs) are the fundamental origin cells of heterotopic ossification (HO) in FOP. Rescued iPSC clones (resFOP-iPSCs), with the pathogenic ACVR1R206H mutation was corrected, were generated using FOP-iPSCs as genetically matched controls. Only induced MSCs (iMSCs) generated from FOP-iPSCs through NCC lineage exhibit the phenotype of FOP. (B) Critical role of FAPs derived from the neural crest in HO. (C) FAPs originate from neural crest lineage (Wnt1-Cre+) located in the peripheral nerves contribute to HO.
3.3 FAPs derived from neural crest in the peripheral nerves contribute to HO
The neural crest is a stem cell/progenitor cell population that contributes to various conditions, including formation of the craniofacial cartilages and bones, smooth muscles, peripheral and intestinal neurons, and neuroglia (Le Douarin and Kalcheim, 1999). Cells derived from the neural crest are localized in various adult tissues and possess differentiate potential, associated with the new tissue formation following stimuli, injuries, or stresses (Neirinckx et al., 2013; Parfejevs et al., 2018). Studies based on muscle development have shown that neural crest-derived MCT cells/FAPs are primarily found in the craniofacial muscles, rather than in the axial or limb muscles. However, research discussed earlier has indicated that in the limb muscles, FAPs originating from neural crests play a significant role in HO. Consistently, studies of mouse models have revealed osteogenic precursor cells, which have originated from neural crests, within the peripheral nerves (Lazard et al., 2015; Olmsted-Davis et al., 2017; Carr et al., 2019).
The connective tissue sheath that encases each peripheral nerve bundle, the perineurium, contains MSCs that are tightly intertwined with axons and Schwann cells. These cells originate from the neural crest, and this unique embryonic origin may enhance their regenerative capacity (Davis et al., 2018; Joseph et al., 2004; Richard et al., 2014). These cells exhibit features of FAPs and are associated with tissue regeneration and ectopic bone formation. Storer et al. demonstrate that Pdgfra-expressing mesenchymal cells in uninjured digits establish the regenerative blastema and are essential for regeneration (Storer et al., 2020). Interestingly, single-cell RNA sequencing and lineage tracing of injured sciatic nerves in adult mice transfected with an EGFP reporter knocked into the Pdgfra gene revealed that a substantial population of Pdgfra-EGFP-positive cells exhibit a mesenchymal precursor and embryonic mesenchyme transcriptional signature. These cells proliferate after injury, express genes related to chondrogenesis and osteogenesis, such as Sox9 and Alpl, and can differentiate into osteo/chondro/adipogenic lineages. Crossing Wnt1CreR26-LSL-tdTomato (TdT) mice with the above mice showed that most Pdgfra-EGFP-positive endoneurial FAPs were Wnt1Cre-TdT-positive, indicating a neural crest origin in the injured nerves. These FAPs contribute to the formation of the regenerative blastema and, ultimately, to the regenerated bone following digit-tip amputation. Similar results were obtained in mice with another neural crest lineage tracing line, DhhCre mice (Carr et al., 2019). These findings support the hypothesis that the peripheral nerves facilitate tissue repair and regeneration by providing FAPs derived from the neural crest.
In the HO mouse model induced by muscle injection of Ad5BMP-2, cells expressing the osteoblast-specific transcription factor osterix appeared in the neurons at the injection site (Lazard et al., 2015). Subsequently, these cells migrated away from the endoneurial compartment and entered the site of new bone formation. These cells expressed both PDGFRα and the NCC marker low-affinity nerve growth factor receptor p75, suggesting that this HO precursor cell may be FAPs of NCC origin. Lineage tracing in mice that contained a tamoxifen-regulated Wnt1-Cre recombinase crossed with a TdT reporter (Wnt1CreErt:Ai9Tm) confirmed that following BMP-2 intramuscular injection, TdT-positive cells within the endoneurium co-expressed SP7 (osterix), pre-chondrocytes (Sox9), and transient brown fat (tBAT, UCP1), which is closely associated with subsequent HO (Olmsted-Davis et al., 2017). Additionally, human nerves near the HO site contain many phosphoSMAD1/5/8-positive cells. Constitutively activated ACVR1 in the cranial NCCs of mice induces ectopic cartilage formation in the craniofacial region by enhancing BMP signaling (Mishina and Snider, 2014). This finding may explain why patients with FOP develop HO in the craniofacial regions specifically through endochondral ossification. These studies indicate that, in both mouse and human acquired HO and FOP, the precursor cells for HO originate from NCCs in peripheral nerves (Figure 2C).
NCCs may persist in the endoneurium because of their immune-privileged position behind the blood–nerve barrier. Meanwhile, PDGFRα, a factor crucial for glia–endothelium interactions and the maintenance of the blood–brain barrier, is expressed in FAPs of neural crest origin and may play a key role in their transition from a neural to mesodermal fate (Lazard et al., 2015). Wnt1 is a major inducer of the neural crest (García-Castro et al., 2002; Ikeya et al., 1997). When not inhibited, Wnt1 signaling causes the formation of sensory neurons from neural stem cells (Lee et al., 2004). However, when inhibited by BMP2, Wnt1 signaling in NCCs leads to the formation of other cell types including osteogenic precursors (Kléber et al., 2005). Therefore, in addition to the FAPs present in the muscle interstitium, FAPs in adult muscle tissues may originate from NCCs deposited in the endoneurium during neural crest migration and sensory nerve formation, which re-enact embryonic processes for tissue regeneration upon activation by specific signals. This may explain the NCC-derived FAPs in the peripheral nerves that contribute to HO and their mechanisms of action.
3.4 Application of iPSCs-derived FAPs as a tool to investigate HO
iPSCs derived from patients with HO offers potential for understanding of the disease. Utilizing MSCs derived from FOP-iPSCs, a mechanism has been discovered in which FOP-ACVR1 abnormally transduces BMP signaling in response to Activin-A. This mechanism is distinct from the previously identified FOP-ACVR1-mediated ligand-independent constitutive activity and BMP ligand-dependent hyperactivity in BMP signaling. Activin-A enhanced the chondrogenesis of FOP-iMSCs via aberrant activation of BMP signaling in vitro, and induced endochondral ossification of FOP-iMSCs in vivo (Hatsell et al., 2015; Hino et al., 2015). Further research revealed that mTOR signaling as a critical pathway for the aberrant chondrogenesis induced by Activin-A in FOP-MSCs (Hino et al., 2017). Furthermore, drug screening based on the FOP-iMSCs has identified specific small-molecule compounds, such as rapamycin (Hino et al., 2017) and AZD0530 (Hino et al., 2018), as potential candidates which have entered clinical trials for treating FOP, supporting the reliability of the iPSC-based disease models.
However, FAPs and MSCs originated from iPSCs may not be entirely congruent cell populations. FACS sorting was used to isolate different subsets of MSC-like cells derived from FOP-iPSCs via somite (Nakajima et al., 2018). The results showed that PDGFRα+/CD31− cells exhibited enhanced chondrogenesis compared to PDGFRα−/CD31- cells, suggesting that specific differentiation protocols and isolation based on surface markers such as PDGFRα can be employed to obtain FAPs from iPSCs.
Building on the insights into the distinct origins of FAPs, iPSC-derived FAPs can serve as a valuable tool to investigate the role of embryonic origin on the behavior of FAPs in heterotopic ossification. By comparing the characteristics and functional capacities of FAPs derived from different embryonic origins, researchers can elucidate how these populations contribute to the pathogenesis of ectopic bone formation and potentially identify novel therapeutic targets. Furthermore, recent studies have shown that iMSC-mediated delivery of therapeutic agents, such as the ACVR2B-Fc fusion protein, can effectively alleviate HO symptoms in FOP model mice (Gao et al., 2024). Based on the different embryonic origins of FAPs, these cells may also provide a promising avenue for developing targeted therapies aimed at mitigating HO.
4 Conclusion
FAPs are essential for muscle homeostasis and regeneration in adults. They are vital for the regulation of muscle development. The previous literatures, by adopting the perspective that FAPs are MCT cells, mapped their origin and fate during embryonic development and elucidated their roles in regulating muscle morphogenesis. The axial MCT is thought to originate from the somites and lateral plate mesoderm; the limb MCT originates from the lateral plate mesoderm; and the cranial MCT originates from the neural crest and lateral plate mesoderm. Recent studies using iPSCs derived from patients with FOP and HO mouse models revealed the contribution of NCC-derived FAPs to HO. This novel finding, departing from traditional developmental perspectives, suggests that the heterogeneity of FAPs may be attributed to specific environments and signals that activate FAPs of different embryonic origins, leading to diverse responses. These perspectives aid in understanding the intricate roles of FAPs in muscle homeostasis, regeneration, and pathological conditions.
Author contributions
CZ: Conceptualization, Funding acquisition, Validation, Writing–original draft. MI: Conceptualization, Supervision, Validation, Writing–review and editing.
Funding
The author(s) declare that financial support was received for the research, authorship, and/or publication of this article. This work was supported by the Young Scientists Fund of the National Natural Science Foundation of China (Grant No. 82202655) to CZ.
Acknowledgments
We would like to thank Editage (www.editage.cn) for English language editing.
Conflict of interest
The authors declare that the research was conducted in the absence of any commercial or financial relationships that could be construed as a potential conflict of interest.
Publisher’s note
All claims expressed in this article are solely those of the authors and do not necessarily represent those of their affiliated organizations, or those of the publisher, the editors and the reviewers. Any product that may be evaluated in this article, or claim that may be made by its manufacturer, is not guaranteed or endorsed by the publisher.
Abbreviations
BMP, Bone morphogenetic protein; ECM, Extracellular matrix; FAPs, Fibro/adipogenic progenitors; FOP, fibrodysplasia ossificans progressiva; HO, Heterotopic ossification; iPSCs, Induced pluripotent stem cells; MCT, Muscle connective tissue; MPC, Mesenchymal progenitor cells; MSC, Mesenchymal stem cells; NCCs, Neural crest cells; TGF, transforming growth factor.
References
Abmayr, S. M., and Pavlath, G. K. (2012). Myoblast fusion: lessons from flies and mice. Development 139, 641–656. doi:10.1242/dev.068353
Agarwal, P., Wylie, J. N., Galceran, J., Arkhitko, O., Li, C., Deng, C., et al. (2003). Tbx5 is essential for forelimb bud initiation following patterning of the limb field in the mouse embryo. Development 130, 623–633. doi:10.1242/dev.00191
Agarwal, S., Loder, S., Brownley, C., Cholok, D., Mangiavini, L., Li, J., et al. (2016). Inhibition of Hif1α prevents both trauma-induced and genetic heterotopic ossification. Proc. Natl. Acad. Sci. U. S. A. 113, E338–E347. doi:10.1073/pnas.1515397113
Agarwal, S., Loder, S., Cholok, D., Li, J., Breuler, C., Drake, J., et al. (2017). Surgical excision of heterotopic ossification leads to Re-emergence of mesenchymal stem cell populations responsible for recurrence. Stem Cells Transl. Med. 6, 799–806. doi:10.5966/sctm.2015-0365
Bladt, F., Riethmacher, D., Isenmann, S., Aguzzi, A., and Birchmeier, C. (1995). Essential role for the c-met receptor in the migration of myogenic precursor cells into the limb bud. Nature 376, 768–771. doi:10.1038/376768a0
Braun, T., and Gautel, M. (2011). Transcriptional mechanisms regulating skeletal muscle differentiation, growth and homeostasis. Nat. Rev. Mol. Cell Biol. 12, 349–361. doi:10.1038/nrm3118
Bryson-Richardson, R. J., and Currie, P. D. (2008). The genetics of vertebrate myogenesis. Nat. Rev. Genet. 9, 632–646. doi:10.1038/nrg2369
Buckingham, M. (2006). Myogenic progenitor cells and skeletal myogenesis in vertebrates. Curr. Opin. Genet. Dev. 16, 525–532. doi:10.1016/j.gde.2006.08.008
Carr, M. J., Toma, J. S., Johnston, A. P. W., Steadman, P. E., Yuzwa, S. A., Mahmud, N., et al. (2019). Mesenchymal precursor cells in adult nerves contribute to mammalian tissue repair and regeneration. Cell Stem Cell 24, 240–256. doi:10.1016/j.stem.2018.10.024
Chakkalakal, S. A., Uchibe, K., Convente, M. R., Zhang, D., Economides, A. N., Kaplan, F. S., et al. (2016). Palovarotene inhibits heterotopic ossification and maintains limb mobility and growth in mice with the human ACVR1(r206H) fibrodysplasia ossificans progressiva (FOP) mutation. J. Bone Min. Res. 31, 1666–1675. doi:10.1002/jbmr.2820
Chapman, M. A., Mukund, K., Subramaniam, S., Brenner, D., and Lieber, R. L. (2017). Three distinct cell populations express extracellular matrix proteins and increase in number during skeletal muscle fibrosis. Am. J. Physiol. Cell Physiol. 312, C131–c143. doi:10.1152/ajpcell.00226.2016
Colasanto, M. P., Eyal, S., Mohassel, P., Bamshad, M., Bonnemann, C. G., Zelzer, E., et al. (2016). Development of a subset of forelimb muscles and their attachment sites requires the ulnar-mammary syndrome gene Tbx3. Dis. Model Mech. 9, 1257–1269. doi:10.1242/dmm.025874
Contreras, O., Rebolledo, D. L., Oyarzún, J. E., Olguín, H. C., and Brandan, E. (2016). Connective tissue cells expressing fibro/adipogenic progenitor markers increase under chronic damage: relevance in fibroblast-myofibroblast differentiation and skeletal muscle fibrosis. Cell Tissue Res. 364, 647–660. doi:10.1007/s00441-015-2343-0
Contreras, O., Rossi, F. M. V., and Theret, M. (2021). Origins, potency, and heterogeneity of skeletal muscle fibro-adipogenic progenitors-time for new definitions. Skelet. Muscle 11, 16. doi:10.1186/s13395-021-00265-6
Cordani, N., Pisa, V., Pozzi, L., Sciorati, C., and Clementi, E. (2014). Nitric oxide controls fat deposition in dystrophic skeletal muscle by regulating fibro-adipogenic precursor differentiation. Stem Cells 32, 874–885. doi:10.1002/stem.1587
Davis, E. L., Davis, A. R., Gugala, Z., and Olmsted-Davis, E. A. (2018). Is heterotopic ossification getting nervous? the role of the peripheral nervous system in heterotopic ossification. Bone 109, 22–27. doi:10.1016/j.bone.2017.07.016
De Micheli, A. J., Laurilliard, E. J., Heinke, C. L., Ravichandran, H., Fraczek, P., Soueid-Baumgarten, S., et al. (2020b). Single-cell analysis of the muscle stem cell hierarchy identifies heterotypic communication signals involved in skeletal muscle regeneration. Cell Rep. 30, 3583–3595. doi:10.1016/j.celrep.2020.02.067
De Micheli, A. J., Spector, J. A., Elemento, O., and Cosgrove, B. D. (2020a). A reference single-cell transcriptomic atlas of human skeletal muscle tissue reveals bifurcated muscle stem cell populations. Skelet. Muscle 10, 19. doi:10.1186/s13395-020-00236-3
Deries, M., Gonçalves, A. B., Vaz, R., Martins, G. G., Rodrigues, G., and Thorsteinsdóttir, S. (2012). Extracellular matrix remodeling accompanies axial muscle development and morphogenesis in the mouse. Dev. Dyn. 241, 350–364. doi:10.1002/dvdy.23703
Deries, M., Schweitzer, R., and Duxson, M. J. (2010). Developmental fate of the mammalian myotome. Dev. Dyn. 239, 2898–2910. doi:10.1002/dvdy.22425
Dey, D., Bagarova, J., Hatsell, S. J., Armstrong, K. A., Huang, L., Ermann, J., et al. (2016). Two tissue-resident progenitor lineages drive distinct phenotypes of heterotopic ossification. Sci. Transl. Med. 8, 366ra163. doi:10.1126/scitranslmed.aaf1090
Dietrich, S., Abou-Rebyeh, F., Brohmann, H., Bladt, F., Sonnenberg-Riethmacher, E., Yamaai, T., et al. (1999). The role of SF/HGF and c-Met in the development of skeletal muscle. Development 126, 1621–1629. doi:10.1242/dev.126.8.1621
Durland, J. L., Sferlazzo, M., Logan, M., and Burke, A. C. (2008). Visualizing the lateral somitic frontier in the Prx1Cre transgenic mouse. J. Anat. 212, 590–602. doi:10.1111/j.1469-7580.2008.00879.x
Eisner, C., Cummings, M., Johnston, G., Tung, L. W., Groppa, E., Chang, C., et al. (2020). Murine tissue-resident PDGFRα+ fibro-adipogenic progenitors spontaneously acquire osteogenic phenotype in an altered inflammatory environment. J. Bone Mineral Res. 35, 1525–1534. doi:10.1002/jbmr.4020
Engquist, E. N., Greco, A., Joosten, L. A. B., van Engelen, B. G. M., Zammit, P. S., and Banerji, C. R. S. (2024). FSHD muscle shows perturbation in fibroadipogenic progenitor cells, mitochondrial function and alternative splicing independently of inflammation. Hum. Mol. Genet. 33, 182–197. doi:10.1093/hmg/ddad175
Evans, A. L., and Gage, P. J. (2005). Expression of the homeobox gene Pitx2 in neural crest is required for optic stalk and ocular anterior segment development. Hum. Mol. Genet. 14, 3347–3359. doi:10.1093/hmg/ddi365
Farup, J., Just, J., de Paoli, F., Lin, L., Jensen, J. B., Billeskov, T., et al. (2021). Human skeletal muscle CD90(+) fibro-adipogenic progenitors are associated with muscle degeneration in type 2 diabetic patients. Cell Metab. 33, 2201–2214.e11. doi:10.1016/j.cmet.2021.10.001
Farup, J., Madaro, L., Puri, P. L., and Mikkelsen, U. R. (2015). Interactions between muscle stem cells, mesenchymal-derived cells and immune cells in muscle homeostasis, regeneration and disease. Cell Death Dis. 6, e1830. doi:10.1038/cddis.2015.198
Flores-Opazo, M., Kopinke, D., Helmbacher, F., Fernández-Verdejo, R., Tuñón-Suárez, M., Lynch, G. S., et al. (2024). Fibro-adipogenic progenitors in physiological adipogenesis and intermuscular adipose tissue remodeling. Mol. Asp. Med. 97, 101277. doi:10.1016/j.mam.2024.101277
Fukuta, M., Nakai, Y., Kirino, K., Nakagawa, M., Sekiguchi, K., Nagata, S., et al. (2014). Derivation of mesenchymal stromal cells from pluripotent stem cells through a neural crest lineage using small molecule compounds with defined media. PLoS One 9, e112291. doi:10.1371/journal.pone.0112291
Gabbiani, G. (1998). Evolution and clinical implications of the myofibroblast concept. Cardiovasc Res. 38, 545–548. doi:10.1016/s0008-6363(98)00065-0
Gao, P., Inada, Y., Hotta, A., Sakurai, H., and Ikeya, M. (2024). iMSC-mediated delivery of ACVR2B-Fc fusion protein reduces heterotopic ossification in a mouse model of fibrodysplasia ossificans progressiva. Stem Cell Res. Ther. 15, 83. doi:10.1186/s13287-024-03691-7
García-Castro, M. I., Marcelle, C., and Bronner-Fraser, M. (2002). Ectodermal Wnt function as a neural crest inducer. Science 297, 848–851. doi:10.1126/science.1070824
Gatchalian, C. L., Schachner, M., and Sanes, J. R. (1989). Fibroblasts that proliferate near denervated synaptic sites in skeletal muscle synthesize the adhesive molecules tenascin(J1), N-CAM, fibronectin, and a heparan sulfate proteoglycan. J. Cell Biol. 108, 1873–1890. doi:10.1083/jcb.108.5.1873
Gonzalez, D., Contreras, O., Rebolledo, D. L., Espinoza, J. P., van Zundert, B., and Brandan, E. (2017). ALS skeletal muscle shows enhanced TGF-β signaling, fibrosis and induction of fibro/adipogenic progenitor markers. PLoS One 12, e0177649. doi:10.1371/journal.pone.0177649
Grenier, J., Teillet, M. A., Grifone, R., Kelly, R. G., and Duprez, D. (2009). Relationship between neural crest cells and cranial mesoderm during head muscle development. PLoS One 4, e4381. doi:10.1371/journal.pone.0004381
Han, D., Zhao, H., Parada, C., Hacia, J. G., Bringas, P., and Chai, Y. (2012). A TGFβ-Smad4-Fgf6 signaling cascade controls myogenic differentiation and myoblast fusion during tongue development. Development 139, 1640–1650. doi:10.1242/dev.076653
Hasson, P., DeLaurier, A., Bennett, M., Grigorieva, E., Naiche, L. A., Papaioannou, V. E., et al. (2010). Tbx4 and tbx5 acting in connective tissue are required for limb muscle and tendon patterning. Dev. Cell 18, 148–156. doi:10.1016/j.devcel.2009.11.013
Hasson, P., Del Buono, J., and Logan, M. P. (2007). Tbx5 is dispensable for forelimb outgrowth. Development 134, 85–92. doi:10.1242/dev.02622
Hatsell, S. J., Idone, V., Wolken, D. M., Huang, L., Kim, H. J., Wang, L., et al. (2015). ACVR1R206H receptor mutation causes fibrodysplasia ossificans progressiva by imparting responsiveness to activin A. Sci. Transl. Med. 7, 303ra137. doi:10.1126/scitranslmed.aac4358
Helmbacher, F. (2018). Tissue-specific activities of the Fat1 cadherin cooperate to control neuromuscular morphogenesis. PLoS Biol. 16, e2004734. doi:10.1371/journal.pbio.2004734
Helmbacher, F., and Stricker, S. (2020). Tissue cross talks governing limb muscle development and regeneration. Semin. Cell Dev. Biol. 104, 14–30. doi:10.1016/j.semcdb.2020.05.005
Hesse, E. (2016). Muscle and bone: combating the evil side of the connection. J. Bone Mineral Res. 31, 1647–1651. doi:10.1002/jbmr.2912
Heude, E., Bouhali, K., Kurihara, Y., Kurihara, H., Couly, G., Janvier, P., et al. (2010). Jaw muscularization requires Dlx expression by cranial neural crest cells. Proc. Natl. Acad. Sci. U. S. A. 107, 11441–11446. doi:10.1073/pnas.1001582107
Heude, E., Tesarova, M., Sefton, E. M., Jullian, E., Adachi, N., Grimaldi, A., et al. (2018). Unique morphogenetic signatures define mammalian neck muscles and associated connective tissues. Elife 7, e40179. doi:10.7554/eLife.40179
Hino, K., Horigome, K., Nishio, M., Komura, S., Nagata, S., Zhao, C., et al. (2017). Activin-A enhances mTOR signaling to promote aberrant chondrogenesis in fibrodysplasia ossificans progressiva. J. Clin. Invest. 127, 3339–3352. doi:10.1172/JCI93521
Hino, K., Ikeya, M., Horigome, K., Matsumoto, Y., Ebise, H., Nishio, M., et al. (2015). Neofunction of ACVR1 in fibrodysplasia ossificans progressiva. Proc. Natl. Acad. Sci. U. S. A. 112, 15438–15443. doi:10.1073/pnas.1510540112
Hino, K., Zhao, C., Horigome, K., Nishio, M., Okanishi, Y., Nagata, S., et al. (2018). An mTOR signaling modulator suppressed heterotopic ossification of fibrodysplasia ossificans progressiva. Stem Cell Rep. 11, 1106–1119. doi:10.1016/j.stemcr.2018.10.007
Ikeya, M., Lee, S. M., Johnson, J. E., McMahon, A. P., and Takada, S. (1997). Wnt signalling required for expansion of neural crest and CNS progenitors. Nature 389, 966–970. doi:10.1038/40146
Iwata, J., Suzuki, A., Pelikan, R. C., Ho, T. V., and Chai, Y. (2013). Noncanonical transforming growth factor β (TGFβ) signaling in cranial neural crest cells causes tongue muscle developmental defects. J. Biol. Chem. 288, 29760–29770. doi:10.1074/jbc.M113.493551
Jackson, W. M., Aragon, A. B., Djouad, F., Song, Y., Koehler, S. M., Nesti, L. J., et al. (2009). Mesenchymal progenitor cells derived from traumatized human muscle. J. Tissue Eng. Regen. Med. 3, 129–138. doi:10.1002/term.149
Joe, A. W., Yi, L., Natarajan, A., Le Grand, F., So, L., Wang, J., et al. (2010). Muscle injury activates resident fibro/adipogenic progenitors that facilitate myogenesis. Nat. Cell Biol. 12, 153–163. doi:10.1038/ncb2015
Joseph, N. M., Mukouyama, Y. S., Mosher, J. T., Jaegle, M., Crone, S. A., Dormand, E. L., et al. (2004). Neural crest stem cells undergo multilineage differentiation in developing peripheral nerves to generate endoneurial fibroblasts in addition to Schwann cells. Development 131, 5599–5612. doi:10.1242/dev.01429
Judson, R. N., Zhang, R. H., and Rossi, F. M. (2013). Tissue-resident mesenchymal stem/progenitor cells in skeletal muscle: collaborators or saboteurs? Febs J. 280, 4100–4108. doi:10.1111/febs.12370
Kan, L., Peng, C. Y., McGuire, T. L., and Kessler, J. A. (2013). Glast-expressing progenitor cells contribute to heterotopic ossification. Bone 53, 194–203. doi:10.1016/j.bone.2012.12.008
Kaplan, F. S., Chakkalakal, S. A., and Shore, E. M. (2012). Fibrodysplasia ossificans progressiva: mechanisms and models of skeletal metamorphosis. Dis. Model Mech. 5, 756–762. doi:10.1242/dmm.010280
Kim, E., Wu, F., Lim, D., Zeuthen, C., Zhang, Y., Allen, J., et al. (2022). Fibroadipogenic progenitors regulate the basal proliferation of satellite cells and homeostasis of pharyngeal muscles via HGF secretion. Front. Cell Dev. Biol. 10, 875209. doi:10.3389/fcell.2022.875209
Kléber, M., Lee, H. Y., Wurdak, H., Buchstaller, J., Riccomagno, M. M., Ittner, L. M., et al. (2005). Neural crest stem cell maintenance by combinatorial Wnt and BMP signaling. J. Cell Biol. 169, 309–320. doi:10.1083/jcb.200411095
Krueger, K. C., Costa, M. J., Du, H., and Feldman, B. J. (2014). Characterization of Cre recombinase activity for in vivo targeting of adipocyte precursor cells. Stem Cell Rep. 3, 1147–1158. doi:10.1016/j.stemcr.2014.10.009
Lazard, Z. W., Olmsted-Davis, E. A., Salisbury, E. A., Gugala, Z., Sonnet, C., Davis, E. L., et al. (2015). Osteoblasts have a neural origin in heterotopic ossification. Clin. Orthop. Relat. Res. 473, 2790–2806. doi:10.1007/s11999-015-4323-9
Le Douarin, N., and Kalcheim, C. (1999). “General Introduction,” in The neural crest. Developmental and cell biology series. Cambridge University Press; 1999:xxi-xxiv.
Lee, H. Y., Kléber, M., Hari, L., Brault, V., Suter, U., Taketo, M. M., et al. (2004). Instructive role of Wnt/beta-catenin in sensory fate specification in neural crest stem cells. Science 303, 1020–1023. doi:10.1126/science.1091611
Lees-Shepard, J. B., Yamamoto, M., Biswas, A. A., Stoessel, S. J., Nicholas, S. E., Cogswell, C. A., et al. (2018). Activin-dependent signaling in fibro/adipogenic progenitors causes fibrodysplasia ossificans progressiva. Nat. Commun. 9, 471. doi:10.1038/s41467-018-02872-2
Lemos, D. R., Babaeijandaghi, F., Low, M., Chang, C. K., Lee, S. T., Fiore, D., et al. (2015). Nilotinib reduces muscle fibrosis in chronic muscle injury by promoting TNF-mediated apoptosis of fibro/adipogenic progenitors. Nat. Med. 21, 786–794. doi:10.1038/nm.3869
Lemos, D. R., Paylor, B., Chang, C., Sampaio, A., Underhill, T. M., and Rossi, F. M. (2012). Functionally convergent white adipogenic progenitors of different lineages participate in a diffused system supporting tissue regeneration. Stem Cells 30, 1152–1162. doi:10.1002/stem.1082
Lescroart, F., Hamou, W., Francou, A., Théveniau-Ruissy, M., Kelly, R. G., and Buckingham, M. (2015). Clonal analysis reveals a common origin between nonsomite-derived neck muscles and heart myocardium. Proc. Natl. Acad. Sci. U. S. A. 112, 1446–1451. doi:10.1073/pnas.1424538112
Light, N., and Champion, A. E. (1984). Characterization of muscle epimysium, perimysium and endomysium collagens. Biochem. J. 219, 1017–1026. doi:10.1042/bj2191017
Malecova, B., Gatto, S., Etxaniz, U., Passafaro, M., Cortez, A., Nicoletti, C., et al. (2018). Dynamics of cellular states of fibro-adipogenic progenitors during myogenesis and muscular dystrophy. Nat. Commun. 9, 3670. doi:10.1038/s41467-018-06068-6
Mathew, S. J., Hansen, J. M., Merrell, A. J., Murphy, M. M., Lawson, J. A., Hutcheson, D. A., et al. (2011). Connective tissue fibroblasts and Tcf4 regulate myogenesis. Development 138, 371–384. doi:10.1242/dev.057463
Matsumoto, Y., Hayashi, Y., Schlieve, C. R., Ikeya, M., Kim, H., Nguyen, T. D., et al. (2013). Induced pluripotent stem cells from patients with human fibrodysplasia ossificans progressiva show increased mineralization and cartilage formation. Orphanet J. Rare Dis. 8, 190. doi:10.1186/1750-1172-8-190
Matsumoto, Y., Ikeya, M., Hino, K., Horigome, K., Fukuta, M., Watanabe, M., et al. (2015). New protocol to optimize iPS cells for genome analysis of fibrodysplasia ossificans progressiva. Stem Cells 33, 1730–1742. doi:10.1002/stem.1981
Matsuoka, T., Ahlberg, P. E., Kessaris, N., Iannarelli, P., Dennehy, U., Richardson, W. D., et al. (2005). Neural crest origins of the neck and shoulder. Nature 436, 347–355. doi:10.1038/nature03837
Matthews, B. G., Torreggiani, E., Roeder, E., Matic, I., Grcevic, D., and Kalajzic, I. (2016). Osteogenic potential of alpha smooth muscle actin expressing muscle resident progenitor cells. Bone 84, 69–77. doi:10.1016/j.bone.2015.12.010
Medici, D., Shore, E. M., Lounev, V. Y., Kaplan, F. S., Kalluri, R., and Olsen, B. R. (2010). Conversion of vascular endothelial cells into multipotent stem-like cells. Nat. Med. 16, 1400–1406. doi:10.1038/nm.2252
Michailovici, I., Eigler, T., and Tzahor, E. (2015). Chapter one - craniofacial muscle development. Curr. Top. Dev. Biol. 115, 3–30. doi:10.1016/bs.ctdb.2015.07.022
Millington, G., Elliott, K. H., Chang, Y. T., Chang, C. F., Dlugosz, A., and Brugmann, S. A. (2017). Cilia-dependent GLI processing in neural crest cells is required for tongue development. Dev. Biol. 424, 124–137. doi:10.1016/j.ydbio.2017.02.021
Mishina, Y., and Snider, T. N. (2014). Neural crest cell signaling pathways critical to cranial bone development and pathology. Exp. Cell Res. 325, 138–147. doi:10.1016/j.yexcr.2014.01.019
Moratal, C., Arrighi, N., Dechesne, C. A., and Dani, C. (2019). Control of muscle fibro-adipogenic progenitors by myogenic lineage is altered in aging and duchenne muscular dystrophy. Cell Physiol. Biochem. 53, 1029–1045. doi:10.33594/000000196
Moratal, C., Raffort, J., Arrighi, N., Rekima, S., Schaub, S., Dechesne, C. A., et al. (2018). IL-1β- and IL-4-polarized macrophages have opposite effects on adipogenesis of intramuscular fibro-adipogenic progenitors in humans. Sci. Rep. 8, 17005. doi:10.1038/s41598-018-35429-w
Naiche, L. A., and Papaioannou, V. E. (2007). Tbx4 is not required for hindlimb identity or post-bud hindlimb outgrowth. Development 134, 93–103. doi:10.1242/dev.02712
Nakajima, T., Shibata, M., Nishio, M., Nagata, S., Alev, C., Sakurai, H., et al. (2018). Modeling human somite development and fibrodysplasia ossificans progressiva with induced pluripotent stem cells. Development 23, dev165431. doi:10.1242/dev.165431
Nassari, S., Duprez, D., and Fournier-Thibault, C. (2017). Non-myogenic contribution to muscle development and homeostasis: the role of connective tissues. Front. Cell Dev. Biol. 5, 22. doi:10.3389/fcell.2017.00022
Neirinckx, V., Coste, C., Rogister, B., and Wislet-Gendebien, S. (2013). Concise review: adult mesenchymal stem cells, adult neural crest stem cells, and therapy of neurological pathologies: a state of play. Stem Cells Transl. Med. 2, 284–296. doi:10.5966/sctm.2012-0147
Nesti, L. J., Jackson, W. M., Shanti, R. M., Koehler, S. M., Aragon, A. B., Bailey, J. R., et al. (2008). Differentiation potential of multipotent progenitor cells derived from war-traumatized muscle tissue. J. Bone Jt. Surg. Am. 90, 2390–2398. doi:10.2106/JBJS.H.00049
Olmsted-Davis, E. A., Salisbury, E. A., Hoang, D., Davis, E. L., Lazard, Z., Sonnet, C., et al. (2017). Progenitors in peripheral nerves launch heterotopic ossification. Stem Cells Transl. Med. 6, 1109–1119. doi:10.1002/sctm.16-0347
Ono, Y., Schlesinger, S., Fukunaga, K., Yambe, S., Sato, T., Sasaki, T., et al. (2023). Scleraxis-lineage cells are required for correct muscle patterning. Development 15, 150. doi:10.1242/dev.201101
Oprescu, S. N., Yue, F., Qiu, J., Brito, L. F., and Kuang, S. (2020). Temporal dynamics and heterogeneity of cell populations during skeletal muscle regeneration. iScience 23, 100993. doi:10.1016/j.isci.2020.100993
Parfejevs, V., Antunes, A. T., and Sommer, L. (2018). Injury and stress responses of adult neural crest-derived cells. Dev. Biol. 444 (Suppl. 1), S356–S365. doi:10.1016/j.ydbio.2018.05.011
Richard, L., Védrenne, N., Vallat, J. M., and Funalot, B. (2014). Characterization of endoneurial fibroblast-like cells from human and rat peripheral nerves. J. Histochem Cytochem 62, 424–435. doi:10.1369/0022155414530994
Rinon, A., Lazar, S., Marshall, H., Büchmann-Møller, S., Neufeld, A., Elhanany-Tamir, H., et al. (2007). Cranial neural crest cells regulate head muscle patterning and differentiation during vertebrate embryogenesis. Development 134, 3065–3075. doi:10.1242/dev.002501
Rubenstein, A. B., Smith, G. R., Raue, U., Begue, G., Minchev, K., Ruf-Zamojski, F., et al. (2020). Single-cell transcriptional profiles in human skeletal muscle. Sci. Rep. 10, 229. doi:10.1038/s41598-019-57110-6
Rudnicki, M. A., Braun, T., Hinuma, S., and Jaenisch, R. (1992). Inactivation of MyoD in mice leads to up-regulation of the myogenic HLH gene Myf-5 and results in apparently normal muscle development. Cell 71, 383–390. doi:10.1016/0092-8674(92)90508-a
Sampath, S. C., Sampath, S. C., and Millay, D. P. (2018). Myoblast fusion confusion: the resolution begins. Skelet. Muscle 8, 3. doi:10.1186/s13395-017-0149-3
Schmitt-Gräff, A., Desmoulière, A., and Gabbiani, G. (1994). Heterogeneity of myofibroblast phenotypic features: an example of fibroblastic cell plasticity. Virchows Arch. 425, 3–24. doi:10.1007/BF00193944
Scott, R., Arostegui, M., Schweitzer, R., Rossi, F. M. V., and Underhill, T. M. (2019). Hic1 defines quiescent mesenchymal progenitor subpopulations with distinct functions and fates in skeletal muscle regeneration. Cell Stem Cell 25, 797–813.e799. doi:10.1016/j.stem.2019.11.004
Sefton, E. M., and Kardon, G. (2019). Connecting muscle development, birth defects, and evolution: an essential role for muscle connective tissue. Curr. Top. Dev. Biol. 132, 137–176. doi:10.1016/bs.ctdb.2018.12.004
Shore, E. M., Xu, M., Feldman, G. J., Fenstermacher, D. A., Cho, T. J., Choi, I. H., et al. (2006). A recurrent mutation in the BMP type I receptor ACVR1 causes inherited and sporadic fibrodysplasia ossificans progressiva. Nat. Genet. 38, 525–527. doi:10.1038/ng1783
Storer, M. A., Mahmud, N., Karamboulas, K., Borrett, M. J., Yuzwa, S. A., Gont, A., et al. (2020). Acquisition of a unique mesenchymal precursor-like blastema state underlies successful adult mammalian digit tip regeneration. Dev. Cell 52, 509–524. doi:10.1016/j.devcel.2019.12.004
Stumm, J., Vallecillo-García, P., Vom Hofe-Schneider, S., Ollitrault, D., Schrewe, H., Economides, A. N., et al. (2018). Odd skipped-related 1 (Osr1) identifies muscle-interstitial fibro-adipogenic progenitors (FAPs) activated by acute injury. Stem Cell Res. 32, 8–16. doi:10.1016/j.scr.2018.08.010
Swartz, M. E., Eberhart, J., Pasquale, E. B., and Krull, C. E. (2001). EphA4/ephrin-A5 interactions in muscle precursor cell migration in the avian forelimb. Development 128, 4669–4680. doi:10.1242/dev.128.23.4669
Swartz, M. E., Nguyen, V., McCarthy, N. Q., and Eberhart, J. K. (2012). Hh signaling regulates patterning and morphogenesis of the pharyngeal arch-derived skeleton. Dev. Biol. 369, 65–75. doi:10.1016/j.ydbio.2012.05.032
Swinehart, I. T., Schlientz, A. J., Quintanilla, C. A., Mortlock, D. P., and Wellik, D. M. (2013). Hox11 genes are required for regional patterning and integration of muscle, tendon and bone. Development 140, 4574–4582. doi:10.1242/dev.096693
Trainor, P. A., Tan, S. S., and Tam, P. P. (1994). Cranial paraxial mesoderm: regionalisation of cell fate and impact on craniofacial development in mouse embryos. Development 120, 2397–2408. doi:10.1242/dev.120.9.2397
Tzahor, E., Kempf, H., Mootoosamy, R. C., Poon, A. C., Abzhanov, A., Tabin, C. J., et al. (2003). Antagonists of Wnt and BMP signaling promote the formation of vertebrate head muscle. Genes Dev. 17, 3087–3099. doi:10.1101/gad.1154103
Uezumi, A., Fukada, S., Yamamoto, N., Ikemoto-Uezumi, M., Nakatani, M., Morita, M., et al. (2014). Identification and characterization of PDGFRα+ mesenchymal progenitors in human skeletal muscle. Cell Death Dis. 5, e1186. doi:10.1038/cddis.2014.161
Uezumi, A., Fukada, S., Yamamoto, N., Takeda, S., and Tsuchida, K. (2010). Mesenchymal progenitors distinct from satellite cells contribute to ectopic fat cell formation in skeletal muscle. Nat. Cell Biol. 12, 143–152. doi:10.1038/ncb2014
Vallecillo-García, P., Orgeur, M., Vom Hofe-Schneider, S., Stumm, J., Kappert, V., Ibrahim, D. M., et al. (2017). Odd skipped-related 1 identifies a population of embryonic fibro-adipogenic progenitors regulating myogenesis during limb development. Nat. Commun. 8, 1218. doi:10.1038/s41467-017-01120-3
Vasyutina, E., Stebler, J., Brand-Saberi, B., Schulz, S., Raz, E., and Birchmeier, C. (2005). CXCR4 and Gab1 cooperate to control the development of migrating muscle progenitor cells. Genes Dev. 19, 2187–2198. doi:10.1101/gad.346205
Wang, Y., Xu, J., Meyers, C. A., Gao, Y., Tian, Y., Broderick, K., et al. (2020). PDGFRα marks distinct perivascular populations with different osteogenic potential within adipose tissue. Stem Cells 38, 276–290. doi:10.1002/stem.3108
Williams, P. E., and Goldspink, G. (1984). Connective tissue changes in immobilised muscle. J. Anat. 138 (Pt 2), 343–350.
Wosczyna, M. N., Biswas, A. A., Cogswell, C. A., and Goldhamer, D. J. (2012). Multipotent progenitors resident in the skeletal muscle interstitium exhibit robust BMP-dependent osteogenic activity and mediate heterotopic ossification. J. Bone Min. Res. 27, 1004–1017. doi:10.1002/jbmr.1562
Wosczyna, M. N., Konishi, C. T., Perez Carbajal, E. E., Wang, T. T., Walsh, R. A., Gan, Q., et al. (2019). Mesenchymal stromal cells are required for regeneration and homeostatic maintenance of skeletal muscle. Cell Rep. 27, 2029–2035. doi:10.1016/j.celrep.2019.04.074
Wotton, K. R., Schubert, F. R., and Dietrich, S. (2015). Hypaxial muscle: controversial classification and controversial data? Results Probl. Cell Differ. 56, 25–48. doi:10.1007/978-3-662-44608-9_2
Zhao, C., Inada, Y., Sekiguchi, K., Hino, K., Nishio, M., Yamada, Y., et al. (2023). Myelin protein zero (P0)- and Wnt1-Cre marked muscle resident neural crest-derived mesenchymal progenitor cells give rise to heterotopic ossification in mouse models. Genes and Dis. 10, 731–734. doi:10.1016/j.gendis.2022.09.002
Keywords: fibro/adipogenic progenitors, induced pluripotent stem cells, muscle connective tissue, developmental origin, neural crest cells
Citation: Zhao C and Ikeya M (2024) Novel insights from human induced pluripotent stem cells on origins and roles of fibro/adipogenic progenitors as heterotopic ossification precursors. Front. Cell Dev. Biol. 12:1457344. doi: 10.3389/fcell.2024.1457344
Received: 30 June 2024; Accepted: 21 August 2024;
Published: 02 September 2024.
Edited by:
Atsushi Asakura, University of Minnesota Twin Cities, United StatesReviewed by:
Osvaldo Contreras, Victor Chang Cardiac Research Institute, AustraliaDavid Sassoon, Sorbonne Universités, France
Akiyoshi Uezumi, Kyushu University, Japan
Takahiko Sato, Fujita Health University, Japan
Sigmar Stricker, Free University of Berlin, Germany
Copyright © 2024 Zhao and Ikeya. This is an open-access article distributed under the terms of the Creative Commons Attribution License (CC BY). The use, distribution or reproduction in other forums is permitted, provided the original author(s) and the copyright owner(s) are credited and that the original publication in this journal is cited, in accordance with accepted academic practice. No use, distribution or reproduction is permitted which does not comply with these terms.
*Correspondence: Chengzhu Zhao, Y2hlbmd6aHUuemhhb0BjcW11LmVkdS5jbg==; Makoto Ikeya, bWlrZXlhQGNpcmEua3lvdG8tdS5hYy5qcA==