- Faculty of Physics and Earth System Science, Peter Debye Institute of Soft Matter Physics, Biological Physics Division, Leipzig University, Leipzig, Germany
The endothelial cells of the blood circulation are exposed to hemodynamic forces, such as cyclic strain, hydrostatic forces, and shear stress caused by the blood fluid’s frictional force. Endothelial cells perceive mechanical forces via mechanosensors and thus elicit physiological reactions such as alterations in vessel width. The mechanosensors considered comprise ion channels, structures linked to the plasma membrane, cytoskeletal spectrin scaffold, mechanoreceptors, and junctional proteins. This review focuses on endothelial mechanosensors and how they alter the vascular functions of endothelial cells. The current state of knowledge on the dysregulation of endothelial mechanosensitivity in disease is briefly presented. The interplay in mechanical perception between endothelial cells and vascular smooth muscle cells is briefly outlined. Finally, future research avenues are highlighted, which are necessary to overcome existing limitations.
1 Introduction
Endothelial cells are subject of mechanical cues from their microenvironment. They form a continuous monolayer at the inner side of vessels, and thus, they are located at the interface of the blood flow and the vessel wall. This specific location of endothelial cells enables them to sense mechanical cues, such as forces that can alter endothelial cell functions. Moreover, due to the blood flow–vessel wall interface location, endothelial cells can serve as mechanical transducers for neighboring cells, such as vascular smooth muscle cells (VSMCs). In turn, from a mechanical viewpoint, VSMCs may act as regulators of the endothelial cell shape and function. Apart from VSMCs, the morphology and functions of endothelial cells are mainly controlled by the blood pressure and extracellular matrix (ECM). The intricate hemodynamic milieu exposes mechanical signals toward endothelial cells in a direct manner, such as tensile forces. These tensile forces strain endothelial cells (Raupach et al., 2007) and strengthen their cell–cell junctions. These mechanical cues are converted into biochemical messages and determine numerous facets of cell fate and determinacy, ranging from endothelial cell proliferation, differentiation, motility, adhesiveness, cell death, and survival. The hemodynamic shear stress has been revealed as a major and relevant determinant of endothelial functionality and shape. Arterial shear stress above 15 dyne/cm2 causes endothelial dormancy and an atheroprotective gene expression pattern. In contrast, a low shear stress of less than 4 dynes/cm2 is predominant at atherosclerosis-prone locations and induces an atherogenic phenotype. In large arteries of healthy individuals, average wall shear rates of 80–400 s-1 and maximum wall shear rates of 900–1,600 s-1 have been determined. Major veins have a larger diameter and less fluid velocity, which leads to lower shear rates (Stroev et al., 2007; Tinken et al., 2009). Likewise, the average shear stress is usually higher in the arterial system, which is between 4 and 70 dynes/cm2, than in the venous system, which ranges from 1 to 6 dynes/cm2 (Malek et al., 1999). Pathological vessels suffering from stenosis exhibit abrupt alterations in vessel diameter, which can modify the normal flow profile and generate shear stresses of over 1,000 dynes/cm2 (Strony et al., 1993). Even though smaller vessels demonstrate reduced fluid velocities, the reduction in the diameter of the vessel is accompanied by an escalation in shear stress and shear rate. In vivo determinations of maximum and average shear rates in 6–12-µm precapillary arteries yielded values of 733–6,562 s-1 and 587–3,515 s-1, respectively (Koutsiaris et al., 2013; Sakariassen et al., 2015).
In adults, maintaining normal blood pressure is also critical for the sustenance of vascular structure and functionality. The pulsatile characteristic of blood pressure produces radial and axial forces, mainly in the shape of cyclic dilation and shear flow, which have a lasting impact on the make-up of the vessel walls so that high blood pressure causes thickening and stiffening of the arterial walls (Laurent and Boutouyrie, 2020; Humphrey, 2021). The friction generated between the blood and the vessel wall produces fluid shear stresses running parallel to the vessel surface, acting primarily on the endothelial cells at the vessel wall boundary (Chiu and Chien, 2011; Roux et al., 2020); circumferential stresses act vertically to the vessel wall on all cells in the vessel wall, such as endothelial cells and VSMCs (Davis et al., 2023; Katoh, 2023).
Endothelial cells sense mechanical forces through specific molecules or structures referred to as mechanosensors. Mechanosensors are first responses to alterations in the mechanical surroundings, and many of them are located at the cell’s plasma membrane. Mechanosensors can transduce extracellular mechanical cues into intracellular chemical signals and initiate signaling pathways, following the engagement of adapter molecules. The process involved is known as mechanotransduction, a mechanism consisting of a series of signaling processes triggered by mechanical impulses. Apart from the activation of mechanosensors, physical forces impact the fluidity of the plasma membrane and cause alterations in protein assemblies on the plasma membrane, which is associated with changes in intercellular junctions, cell–ECM connectivity, cytoskeletal remodeling, and, subsequently, transcriptional reactions in sculpting certain cell phenotypes. The endothelium can perceive these mechanical signals and assimilate inputs from various kinds of physical stimuli, which is of vital importance for the control of vascular functions. The functions of endothelial cells lie in the production of messenger molecules that regulate vascular tone, vascular flow, activity of immune cells, and adhesion, which are all involved in the maintenance of blood pressure and perfusion (Félétou and Vanhoutte, 2006; Moncada and Higgs, 2006). These various endothelial functions are depicted in Figure 1 and also comprise regulation of overall vessel integrity, angiogenesis, hemostasis, vascular growth, vessel restructuring, and growth of tissues, as well as metabolism.
Endothelial function homeostasis is prevalent under physiological conditions, whereby the endothelium keeps an equilibrium between vasodilation and vasoconstriction, impairment and fostering of the migration and proliferation of VSMCs, and fibrinolysis and thrombogenesis, as well as hindering and encouraging the adhesion and platelet agglomeration (Risman et al., 2023). Endothelium-originated molecules with vasodilatory and antiproliferative actions comprise endothelium-derived hyperpolarizing factor (EDHF) (Chen et al., 1988), nitric oxide (NO) (Garty and Palmer, 1997), and prostacyclin (PGI2) (Moncada et al., 1976), whereas endothelin-1 (ET-1) (Yanagisawa et al., 1988), angiotensin II, and reactive oxygen species (ROS) belong to the factors that enhance vasoconstrictive processes (Endemann and Schiffrin, 2004; Just et al., 2008). Endothelial cells also secrete antithrombotic molecules such as NO and PGI2, both of which suppress platelet aggregation, and prothrombotic molecules, such as the von Willebrand factor that fosters the aggregation of platelets and plasminogen activator inhibitor-1 (PAI-1) that blocks fibrinolysis (Risman et al., 2023). In contrast, the dysregulation of homeostasis is tethered to pathological conditions, comprising atherosclerosis, hypertension, cancer metastasis, and diabetes (hampered endothelium-based vasodilation).
In mechanobiological research, the endothelium has been found to be predestined for mechanical analyses because of its appearance as a mostly closed monolayer, the internal apical–basal polarity of endothelial cells, the barrier function of the endothelium, the facilitation of transmigration of immune cells and cancer cells, angiogenesis, and the ease of stimulation. Several mechanosensory elements have been identified, and it has been proposed that the perception of mechanical signals enables the endothelial cells to mechanically analyze their environment and react to changes. There is still much to be done in the field of physical characterization of individual endothelial cells and the perception of physical changes in the environment by endothelial cells regarding their function. The key goals of this review article are, first, to introduce the mechanical environment of endothelial cells lining blood vessels. Second, the most important mechanosensory proteins on endothelial cells are presented. Third, the prominent membrane structures serving as mechanosensors are discussed. Fourth, the coupling between mechanosensation and endothelial cell functions is highlighted. Fifth, the deregulation of the mechanosensory system in pathological conditions is discussed. Sixth, future research directions in the fields of physiology and pathology are predicted.
2 Physical environment to which the endothelial cells in the blood vessels are exposed
It is important to describe which biomechanical forces are relevant for endothelial function and possibly for dysfunction, e.g., endothelial cells lining the lumen of different types of (mostly distensible) vessels. The physical environment of the endothelium consists of a series of interconnected stresses (for definitions see Box 1) that fit into two general classes. The blood vessels are, therefore, not exposed to hydrostatic pressure as such but to the distension that accompanies a rise in (blood) pressure, which stresses the contacts between the neighboring endothelial cells. Hydrostatic pressure is the amount of pressure that a fluid column, e.g., blood, creates when it is trapped in blood vessels or heart chambers. The circulatory system depends on hydrostatic pressure to govern the blood flow and ensure optimized perfusion of organs and tissues. The hydrostatic pressure inside the blood vessels fluctuates according to parameters such as the position of the vascular tree and the level of the pathological disorder. Gravity increases the hydrostatic pressure in the lower body limbs. In the case of pathological states such as high blood pressure, the hydrostatic pressure can rise and result in endothelial malfunction and damage to the blood vessels (Weenink and Wingelaar, 2021). The intensity of this force is several orders of magnitude higher than the shear stress to which only the endothelial cells (but not other cells inside the vessel wall) are subjected. This is the most physiologically pertinent biomechanical force for endothelial cells, which possess an augmentation mechanism at their cell–cell junctions to perceive it more effectively. Most notably, it controls the synthesis of endothelial NO (Vozzi et al., 2014). NO is a highly relevant mediator that mainly governs the phenotype of the endothelial cells themselves, both in the microcirculation in which unidirectional shear stress is mostly dictated by narrowing of the lumen caused by vasoconstriction and at bifurcations or bends of large conduit arteries, in which it declines and undergoes oscillatory behavior, leading to atherogenesis.
Vascular endothelial cells are affected in vivo by two different hemodynamic forces: cyclic strain due to the distension (dilatation) of the vessel wall caused by transmural pressure and shear stress, and the frictional force created through blood flow (Ballermann et al., 1998). Shear stress operates at the apical cell surface to distort cells in the blood flow direction; wall stretch tends to distort cells in all orientations (Ballermann et al., 1998). The response to shear stress is at least partially distinct from the reaction to cyclic elongation, implying that elongation of the cytoskeleton by itself is not sufficient to account for it. Acute shear stress in vitro causes fast cytoskeletal reorganization and activates endothelial cell signaling pathways, leading to acute liberation of NO and prostacyclin, activation of transcription factors, such as nuclear factor (NF)κB, c-fos, Nrf2, c-Jun, and SP-1 (Chien et al., 1998; Wilson et al., 2000; Dai et al., 2007; Hahn et al., 2009). Thereby, the transcription of genes, comprising ICAM-1, VCAM-1, MCP-1, tissue factor, platelet-derived growth factor-B (PDGF-B), transforming growth factor (TGF)-β1, cyclooxygenase-II, and endothelial nitric oxide synthase (eNOS), is activated (Wilcox et al., 1988; Nakashima et al., 1998; Viñals and Pouysségur, 2001; Chien, 2003; Tang et al., 2014; Tran et al., 2022). This type of reaction thus has analogies with the reactions of endothelial cells reacting to inflammatory cytokines (Wautier and Wautier, 2021). In sharp distinction, endothelial cells adjust to chronic shear stress through reshaping and thinning their architecture to mitigate shear stress (Zhong et al., 2020). These bordered cells adhere strongly to their substrate and exhibit indications of cell differentiation (Gifre-Renom et al., 2022). The elevated adhesion, following chronic shear stress, has been artificially generated to produce vascular grafts with confluent endothelial cell monolayers that are better preserved post-implantation in vivo, thereby surmounting a principal hurdle to endothelization of vascular grafts (Dardik et al., 1999). In the following, the cyclical strain and the shear stress are defined by physical equations.
2.1 Cyclical strain
Cyclic strain, especially in arteries and heart valves, is the periodic distortion of blood vessels due to pulsatile blood flow. The vessel wall is cyclically dilated and relaxed because of this mechanical stress, which triggers a reaction in the endothelial cells (Katoh, 2023). The blood flow triggered by myocardial spasm and relaxation generates cyclic tension on the walls of the arteries. The magnitude and length of cyclic stretch are determined by blood pressure, diameter of the vessel, and compliance (Brozovich et al., 2016). Cyclic stress has been found to impact the proliferation of endothelial cells, migration, and the liberation of vasoactive molecules. It has also been implicated in vascular smooth muscle cell attachment, inflammation, restructuring of the ECM, and expression of genes (transcriptional profile). For example, stretch-activated ion channels, focal adhesions and focal adhesion complexes, and integrins are representative mechanosensitive proteins that transmit the reaction of arterial endothelial cells toward cyclic stretching (Baeyens et al., 2014; Zhang et al., 2020).
In vivo, endothelial cells are subjected to two principal hemodynamic forces: transmural pressure gradients and wall shear stress. In blood vessels in vivo, these forces fluctuate in a pulsatile manner, apart from those points where the pressure curve of the heart has been attenuated due to high upstream resistances. Transmural pressure gradients cause vessel wall distention and generate the emergence of wall tension (T), as provided in Equation 1 using Laplace’s law:
where
Box 1 Definitions of mechanical terms
Axial stress = This refers to a normal stress that is positioned parallel to the symmetrical axis of the cylinder.
Circumferential stress (or hoop stress) = This is a normal stress in the tangential (azimuthal) orientation.
Longitudial stress = The axial force places the vessel either in tension or in compression.
Radial stress = It represents a stress that acts toward or away from the central axis of a construction element.
Shear stress = It refers to the tangential stress resulting from the friction of a fluid flowing alongside a solid interface.
Tensile stress = It is produced by the blood pressure, has a circumferential spread, and influences all elements of the vessel wall. It is directly proportional to the transmural pressure (P) and radius (r) and reciprocally related to the vessel wall thickness (w).
Transmural pressure = This is defined as the pressure difference between the intravascular pressure and the pressure applied to the external surface of the vessel wall.
Wall shear stress = It is the resistance that the flowing blood places on the vessel wall, i.e., the force applied by the blood movement against the arterial wall. This stress is assumed to have an important effect on the adaptive processes of the vascular wall by triggering the release of substances such as nitric oxide (NO), prostacyclin, and endothelin from the endothelial cells.
Vasoconstriction = It refers to the narrowing of blood vessels due to the tightening (constriction) of blood vessels, typically occurring when the muscles of the blood vessel walls contract, thereby reducing the vessel lumen. It is the opposite phenomenon of vasodilation.
Vasodilation = It is a process that occurs when the lumen of the blood vessels is enlarged by the muscles of the blood vessel walls expanding.
2.2 Shear stress
Endothelial cells are also exposed to the frictional force created by the shear force of blood rushing along their apical sites. This force is based on the mean fluid flow velocity, its viscosity, and the physical geometry of the blood vessels. For Newtonian fluids, which are defined as fluids for which the flow velocity has no influence on the viscosity, flowing in stiff vessels with constant internal geometry, uniform gradients of velocity arise so that the fluid velocity is lowest at the fixed vessel wall and increases with rising distance therefrom. A liquid flow with this typical uniform velocity gradient is referred to as laminar flow as the liquid can be considered a sequence of molecular layers (laminae) that slide along one another with accelerating velocity as one comes closer to the middle of the vessel. In a uniform, stiff cylinder, the shear stress (
whereby Q stands for the fluid flow rate and
2.3 In vivo shear stress
Biological systems deviate from the conditions defined in the previous section because the blood fluid is not a Newtonian fluid, i.e., the viscosity of the blood drops with rising speed, and the vessels are non-uniform, differently expandable containers (Katoh, 2023). At extremely low blood speeds, the aggregation of cell elements leads to a significant increase in blood viscosity, while the viscosity at very high speeds is just four times higher than that of water (Letcher et al., 1981). Even though the blood flow in vessels with a diameter of less than 0.5 mm is generally laminar, it deviates somewhat from this characteristic in very small vessels like the glomerular capillaries. Moreover, the shear stress is affected by transmural pressure gradients as vessel distention enlarges the vessel diameter and thus leads to a tendency to reduce the shear stress. The impact of vessel distention on shear stress can be substantial since shear stress is in inverse relation to the third power of the radius of the vessel. Differences in vessel wall folds and cell structure also lead to fluctuations in shear stress at various points within a single vessel (Satcher et al., 1992) and even on the single cell (Satcher et al., 1992). Wall shear stress is estimated at a variety of sites in the circulation (Giddens et al., 1993; Jones et al., 1997); some have considered circulating cellular components and the structure and distensibility of the vessel. Therefore, the analysis of the wall shear stress has been standardized later (Gijsen et al., 2019). The mean shear stress is usually at its lowest in the large veins, typically below 1 dynes/cm2. It is generally greatest in small arterioles, in which it can range from 60 to 80 dynes/cm2. It is remarkable that the mean shear stress in small venules is also high due to the large flow rates and the small diameter of these vessels (20–40 dynes/cm2) (Lipowsky et al., 1978). Human cervical artery bifurcation observations indicate that the curvature and shape of the vessel can influence wall shear stress in a dramatic fashion, with values varying from less than 1 dyne/cm2 to over 600 dyne/cm2 at various locations in the same vessel (Zarins et al., 1983). Shear stress in glomerular capillaries has been estimated with a computer modeling approach and varies from approximately 1 to approximately 95 dynes/cm2, with mean stress levels of 5–20 dynes/cm2 in the majority of circuits (Remuzzi et al., 1992).
2.4 Shear stress in different types of vessels
The shear stress is exerted on the endothelial cells through the blood circulation. It has a major impact on both vascular physiology and the functioning of endothelial cells. The impact of shear stress in arteries and its involvement in regulating endothelial cell response is reviewed in this section (Chien, 2007). The aorta and other large arteries experience laminar shear stress, which is characterized by the unidirectional blood flow of restricted spatial and temporal variations (Tarbell and Pahakis, 2006). Arterial shear stress, which fluctuates across the vascular system, is fundamental to the sustenance of endothelial functioning and integrity. Laminar shear stress is a condition with unidirectional flow and exhibits moderate temporal and spatial variations and occurs in large arteries like the aorta. The permeability of the endothelium is influenced, the expression of endothelial adhesion molecules is adjusted, and the formation of vasodilators, such as NO, is activated, whereas thrombosis and inflammation of the endothelium are suppressed (Cunningham and Gotlieb, 2005; Chiu and Chien, 2011). Mechanosensitive gene expression linked to vascular reorganization and evolution of atherosclerosis is impacted through the pulsatile and bidirectional flow in mid-sized arteries (Khan et al., 2021). Smaller arterioles have a branching and twisting shape, which leads to perturbed or fluctuating flow characteristics. Varying shear stress profiles in arteries impact endothelial cell physiology and gene expression in different ways (Cunningham and Gotlieb, 2005; Tarbell and Pahakis, 2006; Chiu and Chien, 2011). Capillaries are essential for exchanging nutrients and for perfusing the tissue. They are exposed to a lower shear stress compared to larger arteries. The shear stress in capillaries is generally at a minimum and exhibits considerable spatial and temporal fluctuations due to the fluctuating spread of the blood flow (Pries et al., 2000). The form and orientation of endothelial cells and the development of fenestrations in capillaries are altered when subjected to shear stress. Moreover, shear stress affects the formation of carriers that support the exchange of nutrients and angiogenesis. The sensitive interplay of shear stress and endothelial reactions within the capillaries is fundamental for homeostasis of the tissue and maintaining optimal microvascular functionality (Chien, 2007). A typical vessel’s shear stresses are the following: aorta ranges from 1 to 22 dyn/cm2 (Cheng C. P. et al., 2003), arteries are in the range of 10–70 dyn/cm2 (Cheng C. et al., 2003), veins are between 1 and 6 dyn/cm2 (Malek et al., 1999), and capillaries exhibit 3–95 dyn/cm2 (Koutsiaris et al., 2013).
Proliferation of endothelial and vascular smooth muscle cells takes place in reaction to a rise in axial stress due to arterial stretching, while shear stress and circumferential stretching stay undisturbed. Enhanced matrix metalloproteinase MMP activity (a family of zinc-dependent extracellular matrix proteins) and the buildup of ECM accompany this process, which leads to growth in length to compensate the effect and re-establish normal axial tension (Liu and Khalil, 2017; Wang and Khalil, 2018; Shimoda, 2019). In laminar flow conditions, the shear stress is dictated by parameters such as blood viscosity, flow speed, and the diameter of the vessel. The shear stress rises with declining vessel diameter if viscosity and speed are kept at a constant level. When blood vessels are exposed to elevated shear stress, they tend to dilate to accommodate and revert to their normal state. In theory, vessels are capable of unlimited expansion and remodeling within the boundaries of the body if this leads to a normalization of circumferential stretching and shear stress. Nevertheless, the mechanical adjustment capability of elastin and collagen within the vascular wall is restricted (Hoefer et al., 2013).
The wall tension is directly correlated with the blood pressure, particularly the pulse pressure. Blood pressure applies three forms of stress to the arterial wall: the longitudinal stress, the radial (or normal) stress (vertically to the vessel axis), and the tangential (or hoop) stress (for definitions see Box 1). The shear stress operates parallel to the surfaces of the intima, media, and adventitia sheets and has the effect that one sheet glides over the other sheet. The strain is the degree of deformation per initial material length and is linked to the stress. Shear stress is able to injure the vessel wall by causing harm to the intima, which is the inner vessel layer, as blood flows over the surface, and it is now considered that this is the gateway for the incorporation of plasma lipids into the wall, causing atherosclerosis (Wang S. et al., 2016). The effect of shear stress does not halt at the intima. Shear stress may alter the inner sheets of a multilayer artery so that one layer is displaced in relation to the other as a function of blood pressure and the varying characteristics of the individual layers (Mishani et al., 2021). Alterations in mechanical forces, like tensile or shear stresses, lead to adaptations in the vessel wall architecture to compensate for the altered circumstances and eventually bring the tensile and shear stresses back to their former values (Gimbrone et al., 1999; Cui et al., 2020). Temporary alterations in vessel diameter are caused by sudden alterations in mechanical stress. These alterations are predominantly governed by the liberation of vasoactive stimulants or alterations in myogenic tone. Conversely, chronic alterations lead to considerable alterations in the form and configuration of the vessel wall (Renna et al., 2013; Martinez-Quinones et al., 2018). This process is termed vascular restructuring, which is a term that refers to alterations in vessels exposed to mechanical forces. For instance, experimental hypertension results in an augmentation of wall thickness in resistance arterioles and arteries because of VSMC hyperplasia and in conduit arteries because of hypertrophy. In the same way, decreased mechanical stress results in vascular atrophy (Lehoux and Tedgui, 1998).
2.5 Vascular tone
Vascular tone refers to the extent to which the blood vessel walls, especially the arteries and arterioles, constrict or relax. Vasoconstriction means the narrowing (constriction) of blood vessels by vascular smooth muscle cells within the vessel walls. If the blood vessels narrow, the blood flow is reduced or obstructed. The relation to NOS (nitric oxide synthase) consists in its function as an essential controller of vascular tone. NO produced by endothelial cells fulfills a key function in governing the acute dilation of arteries that takes place when the blood flow rises inside these vessels (Rubanyi et al., 1986). Shear stress demonstrably enhances nitric oxide production by activating endothelial NO synthase and increasing its gene expression (Boo and Jo, 2003). Shear stress is also proven to activate NO synthesis in cultured endothelial cells (Ohno et al., 1993). Tetrahydrobiopterin, an essential cofactor of eNOS, and intracellular Ca2+ concentration rise in reaction to shear stress, and the activation of protein kinases switches on eNOS (Corson et al., 1996; Fleming et al., 1998). NF-κB, a shear stress response component in the promoter of the eNOS gene, and 3′-polyadenylation help in stabilizing eNOS mRNA to improve transcription in reaction to shear stress (Uematsu et al., 1995; Weber et al., 2005). Endothelial cells subjected to shear stress are also more likely to generate the potential vasodilators prostacyclin, adrenomedullin, and C-type natriuretic peptide (Chun et al., 1997). The generation of endothelin and the enzyme expression, which turns angiotensin into the powerful vasoconstrictor angiotensin II, are both reduced in reaction to shear stress (Rieder et al., 1997).
2.6 Endothelial barrier function
The endothelial barrier ensures the retention of vascular integrity and the transfer of substances between the bloodstream and the adjacent tissue. The function of the endothelium as a barrier can be perturbed through mechanical stresses, including tension, hydrostatic pressure, and shear stress (Cahill and Redmond, 2016). The intactness of the endothelial barrier is mostly sustained by two distinct kinds of junctional complexes, adherens junctions which offer cell–cell adhesion and provide mechanical stability, and tight junctions which serve as a physical border restricting paracellular permeability (Adil et al., 2021). Mechanical stress can influence the endothelial barrier capacity via the regulation of the production and breakdown of junctional complexes. For instance, shear stress encourages the maturation and growth of the tight junctions, which leads to increased barrier strength. In turn, cyclic stress causes harm to the proteins that comprise the tight junctions, thereby enhancing permeability (Gulino-Debrac, 2013). Mechanical stress impacts the permeability of endothelia; shear stress, in particular, reduces permeability through encouraging the creation of tight junctions and decreasing the expression of adhesion molecules (Sukriti et al., 2014; Claesson-Welsh et al., 2021). Inversely, excessive shear stress or prolonged cyclic stress can enhance permeability and interfere with the endothelial barrier. Alterations in the junctional proteins, the arrangement of the cytoskeleton, and the endothelial glycocalyx contribute to these irregularities (Sukriti et al., 2014; Schött et al., 2016; Claesson-Welsh et al., 2021). Studying the processes that control endothelial barrier function during mechanical stress is essential to gain an insight into vascular diseases characterized mainly due to enhanced permeability. This has previously been initiated by exerting shear stress on two kinds of endothelial cells, such as human aortic endothelial cells (HAECs) and human umbilical vein endothelial cells (HUVECs). Shear stress application increases the typical characteristic manifestation of a mature endothelium, which shows a linear arrangement of VE-cadherin at the cell–cell interface and a redistribution of actin filaments alongside the periphery of the endothelial cells (Silvani et al., 2021). An escalating sequence of ascending force levels, varying from 186 pN to 3.5 nN, has then been utilized in a single measurement to assess the force-dependent apparent stiffness of the membrane cortex in the kPa regime, indicating that the membrane cortex has become stiffer and can exert increased forces. Moreover, it has been found that the beads adhered to cells grown in dynamic conditions were more difficult to dislodge using acoustic force spectroscopy than those grown in static culture, indicating a more rigid membrane cortex located at the periphery of the cell. Based on the successful acoustic force spectroscopic measurements, it can be concluded that this biophysical method can be used in the future to determine changes in cell mechanics based on force measurements on adherent cells under conditions that mimic their native microenvironment and, thus, also to uncover the shear stress relationship of the mechanical characteristics of adjacent endothelial cells (Silvani et al., 2021).
2.7 Endothelial nitric oxide synthase (eNOS) and nitric oxide (NO) generation
Activity of eNOS and NO generation in endothelial cells is governed through mechanical stress, specifically shear stress. NO, which is a powerful vasodilator and signal molecule with numerous physiological roles (Figure 2), is generated upon shear stress activation of eNOS (Li et al., 2004; Sriram et al., 2016). The phosphorylation of eNOS at certain locations, which is triggered via shear stress, leads to the generation of NO because of its activation. NO is a key actor in maintaining vascular homeostasis. NO is produced in endothelial cells through eNOS upon converting L-arginine to L-citrulline (Vallance and Chan, 2001). It is predominantly secreted from endothelial cells in reaction to shear stress triggered by circulating blood or receptor-regulated compounds like acetylcholine, bradykinin, or serotonin (Boulanger and Lüscher, 1990). NO efficiently dissipates to the VSMCs and stimulates soluble guanylate cyclase (sGC), leading to elevated levels of cyclic guanosine-3,5-monophosphate (cGMP) and VSMC relaxation (Joannides et al., 1995; Vallance, 2001). In addition, NO also hinders the adhesion and migration of leukocytes, the proliferation of VSMCs, and the adhesion and agglomeration of platelets, and counteracts apoptosis and inflammation, which has an altogether anti-atherogenic impact (Wheatcroft et al., 2003). The natural half-life of NO is extremely short (under 4 s). It is quickly broken down into nitrite and subsequently nitrate, prior to being removed from the body via urine (Moncada and Higgs, 2006). At the same time, NO can also be an endocrine vasoregulator that regulates the flow of blood in the microcirculation (Datta et al., 2004). The key point is that decreased eNOS expression and/or NO bioavailability is linked to dysfunction of the endothelium (Oemar et al., 1998; Sena et al., 2008). When NO penetrates the smooth muscle cells of the vascular system, it causes them to relax and dilate. This helps control the vascular tone, the flow of blood, and the retention of endothelial functionality (Boo et al., 2002; Sriram et al., 2016). Moreover, NO exhibits multiple favorable actions on endothelial functioning, which includes anti-inflammatory and anti-thrombotic effects. It reduces the formation of adhesion molecules, inhibits platelet agglomeration, and inhibits the adhesion of leukocytes to endothelial cells (Kubes et al., 1991; Riddell and Owen, 1997; Liao, 2013; Gao et al., 2018). NO has additional impacts on the motility, angiogenesis, and proliferation of endothelial cells (Pilard et al., 2022). NO represents a signal transduction molecule that exhibits various functions in physiological events including vasodilation (relaxation of blood vessels), neurotransmission, and immune reactions (Ziegler et al., 1998; Balligand et al., 2009; Boycott et al., 2020; Uchida et al., 2021).
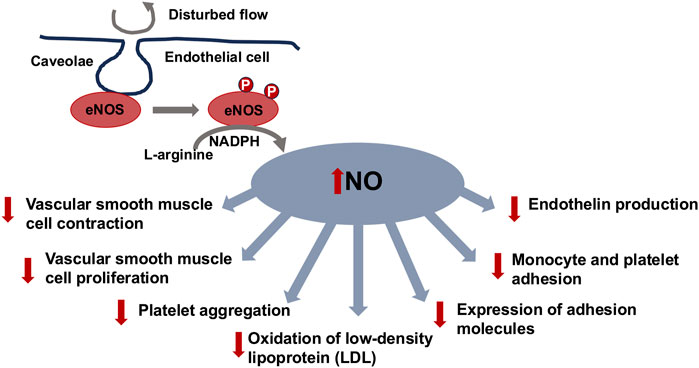
Figure 2. Endothelial nitric oxide synthetase (eNOS)-generated nitric oxide (NO) provides atheroprotective characteristics.
eNOS is induced through different agonists and shear stress by a multitude of cellular mechanisms, which includes elevated intracellular Ca2+, engagement with substrates and cofactors, protein phosphorylation, engagement with adapter and regulatory proteins, and inter-subcellular domain shuttling (Fleming, 2010). Certain serine and threonine residues of eNOS have been phosphorylated through PKA and Akt. Activation of eNOS relies on its degree of phosphorylation. eNOS usually exists as a monomer and is in an inactive form. The dimerization of eNOS is evoked through phosphorylation and the attachment of calcium–calmodulin, which leads to the generation of an active enzyme (Fleming and Busse, 1999). Mechanical forces can induce the stimulation of tyrosine kinases, which are enzymes that attach phosphate groups directly to tyrosine residues. The activity of NOS or other associated molecules can be influenced through subsequent signaling routes that are potentially induced. Moreover, NO can influence cellular events that affect tyrosine phosphorylation since NO is able to react with specific molecules to produce nitrosylated derivatives that can interfere with protein functioning (Gantner et al., 2020; Pourbagher-Shahri et al., 2021; Lundberg and Weitzberg, 2022). Importantly, the relation between NOS, mechanoresponsiveness, and tyrosine phosphorylation can be impacted from the environmental milieu and may potentially engage intricate signaling cascades that are still under exploration.
3 Mechanosensors of endothelial cells
Research into the pathways underpinning endothelial cell mechanotransduction has advanced remarkably. Several molecules located in the apical, junctional, and basal regions of endothelial cells have been found to be crucial for endothelial cell mechanotransduction. Therefore, an overview of the different mechanosensory elements is given and discussed below.
The groups of Chalfie and coworkers and Patapoutian and coworkers (Árnadóttir and Chalfie, 2010; Syeda et al., 2016) identified the following criteria for a well-functioning mechanosensitive ion channel, which had to meet stringent criteria to ensure that the channel is a direct and not an indirect mechanosensor.
I. The channel is designed to incorporate a pore-forming subunit that enables fast ion conduction.
II. When the purified channel is reformed in an artificial, cell-free lipid bilayer, it is expected to open in response to tension exerted on the bilayer.
III. Site-directed mutagenesis of key channel domains that influence pore selectivity or conductivity is expected to modify mechanosensitivity.
IV. Forced expression of the channel in a non-mechanosensitive cell is expected to provide mechanosensitive.
V. Both the gene and the protein of the channel need to be expressed in the presumably mechanosensitive cell.
VI. Genetic deletion of the channel is intended to eliminate mechanosensitivity in a manner that excludes the possibility that the channel plays solely a developmental function or is a downstream signaling partner of another mechanosensor. Genetic deletion, nevertheless, can perturb normal signaling complexes, resulting in off-target events, so expression of a dominant-negative (dead) channel construct might be an improved option.
Finally, whether these criteria are sufficient to distinguish true mechanosensitive channels from those that are indirect mechanosensors is a matter of debate.
The translation of shear forces into biological inputs is facilitated through mechanosensors that can be either specialized intercellular compartments or protein assemblies. These particularly comprise intercellular junctional complexes, integrins, the cytoskeletal components, specific ion channels, G-proteins and G-protein-coupled receptors (GPCRs), the cellular glycocalyx, caveolae, primary cilia (if present on mammalian endothelial cells), and plexin D1 (PLXND1) (Figure 3) (Chatterjee and Fisher, 2014; Mehta et al., 2020). In the case of stimulation, the transmission of the mechanical force commences at the neighboring cell circumference and then spreads across the entire cell (Vogel and Sheetz, 2006). Several signal paths arranged after the mechanosensors are triggered at almost the same time.
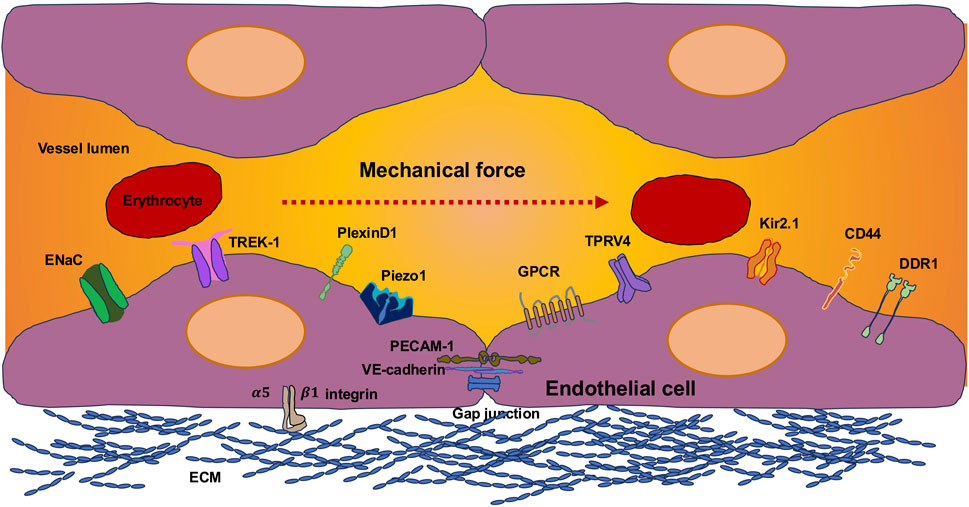
Figure 3. Types of endothelial mechanoreceptors at their luminal site are permanently subject to vascular mechanical forces. The sensing of these forces can be performed through specific mechanosensors. Among them are ion channels, such as Piezo1, transient receptor potential vanilloid 4 channel (TRPV4), K+ channel inwardly rectifying 2.1 (Kir 2.1), epithelial sodium channel (ENaC), TREK-1, DDR1, CD44, and mechanoreceptors, comprising the G-protein-coupled receptor (GPCR).
Messenger substances like cytosolic calcium (Ca2+) and NO are subsequently produced, and the mechanical transduction of nuclear transcription factors becomes enabled. Such shear stress-responsive transcription factors comprise Krüppel-like factor 2 (KLF2) and KLF4, nuclear factor erythroid 2-like (NRF2), and Yes-associated protein (YAP)/transcriptional coactivator with a PDZ-binding domain (TAZ) (Tsaryk et al., 2022). KLF2, which is expressed in vascular endothelial cells of mouse embryos even at E9.5, is triggered through atheroprotective streaming (Dekker et al., 2002) and controls nuclear gene transcription (Dekker et al., 2005). Nevertheless, endothelial cells in arteries with perturbed flow exhibited, amongst other characteristics, an accumulation of TEAD1 and ETV3 tethering sites for transcription factors (Andueza et al., 2020). Shear stress-responsive elements have been found in endothelial cells that are susceptible to atheroporotic oscillatory shear stress. Their activation of the YAP/TAZ signaling pathway was implicated in facilitating the endothelial cell reaction to this mode of shear stress (Bondareva et al., 2019).
In the study of histone changes in reaction to pulsatile shear stress, KLF4 was recognized as a cognate downstream transcription factor (He et al., 2019). In addition, the early growth response (EGR1) and SMAD1/5 are involved in the control of shear stress-induced alterations in gene expression (Khachigian et al., 1997; Zhou J. et al., 2012), whereas the transcription factor Snail reacts to low shear stress and facilitates the switch from an endothelial to a mesenchymal state (Mahmoud et al., 2017). Twist transcription factor is also expressed at low shear stress (Mahmoud et al., 2016). Consequently, the comprehension of transcriptional organization of the shear stress reaction is by no means exhaustive and could imply the involvement of different groups of transcription factors based on the flow scheme and/or endothelial cell type studied (Nakajima and Mochizuki, 2017).
The diverse kinds of mechanosensors can be broadly grouped into three major types, such as apical mechanosensors, cell–cell junctions, and cell-matrix interactions. Apical mechanosensors comprise mechanosensitive ion channels, including PIEZO (Barakat et al., 1999; Yamamoto et al., 2000; Ranade et al., 2014; Wang et al., 2020; Cudmore and Santana, 2022), primary cilia (Iomini et al., 2004; Hierck et al., 2008), the glycocalyx—like glypican-1, heparan sulfate, hyaluronan (HA), and sialic acid—(Weinbaum et al., 2003; Foote et al., 2022), GTP-binding proteins (Gudi et al., 2003; Iomini et al., 2004), and the caveolae (Boyd et al., 2003; Shihata et al., 2016). There are convergent roles of Piezo1 action as, on one hand, Piezo1-driven Akt activity triggers vasodilation and vasorelaxation due to increased flow conditions and, on the other hand, conveys in vasoconstriction (Porto Ribeiro et al., 2022). The cooperation between these two mechanisms is presumably key to controlling the blood pressure. In cell–cell contacts, platelet endothelial adhesion molecule-1 (PECAM-1), vascular endothelial cadherin (VE-cadherin), and vascular endothelial growth factor receptors (VEGFRs), such as VEGFR2, have been demonstrated to constitute an intricate mechanosensory system (Osawa et al., 2002; Tzima et al., 2005; Chiu et al., 2008). PECAM-1 can perceive unidirectional and perturbed flow and consequently transmit protecting and inflammatory messages. Therefore, PECAM-1 knockouts exhibit an intriguing phenotype consisting of amplified lesion formation at locations of the unidirectional flow and diminished lesion development at locations of the perturbed flow (Goel et al., 2008; Harry et al., 2008). In contrast, syndecan-4, which belongs to transmembrane heparan sulfate proteoglycans, is necessary for aligning endothelial cells during shear stress but not required for other mechanoreactions, pointing to a function in flow direction recognition (Baeyens et al., 2014). Depletion of syndecan-4 leads to atherosclerotic plaque formation.
There are important and comprehensive review articles on cell–cell junctions acting as mechanosensors (Dorland and Huveneers, 2017; Angulo-Urarte et al., 2020), but nonetheless, some main cell–cell adhesion receptors are discussed in this review. The major class of mechanosensory receptors comprises ion channels, and thus, they are presented and discussed in the following.
3.1 Ion channels
Several classes of ion channels operate under the control of ligands or are voltage-activated, whereas others are mechanically activated. Mechanosensitive ion channels can be directly activated through physiological mechanical forces. Because of the vital importance of endothelial cells, the question of how the endothelium perceives and reacts to blood flow and the accompanying mechanical forces is something that has been of great concern to scientists for decades. The first proof of a mechanosensitive endothelial channel that transmits the mechanically triggered cation inflow occurred over 40 years ago (Guharay and Sachs, 1984). This groundbreaking revelation was soon afterward followed by the finding of shear stress-activated K+ currents within endothelial cells (Olesen et al., 1988).
Endothelial mechanosensory channel activity varies in direct reaction to shear forces, while the activity of other channels can be modified by forces in an indirect way. For an ion channel to be regarded as a genuine mechanosensory channel, several criteria must be satisfied. First, channel overexpression in a null cell needs to impart mechanosensitivity, and removal or blocking of the channel needs to abrogate the mechanoreaction. The second is that the signal and the protein expression of the channel need to be detectable in the mechanosensitive cell—here the endothelial cell. The third is that the alleged mechanosensitive channel needs to have a direct part in ion permeation as a force reaction. Fourth, the expression of channels is essential to the mechanosensing mechanism and cannot be a downstream objective of any other mechanosensing mechanism. Lastly, the targeted mutagenesis of the ion channel needs to change the mechanoresponse.
Mechanosensitive ion channels are an entrenched group of biological molecules that have an exceptional ability to perceive and handle external mechanical stimuli by triggering an electrical or chemical response that is ultimately incorporated into a coherent cellular reaction (Brohawn, 2015). Several ion channels, such as the ion channels TREK-1 (Honoré, 2007) and TRPC1 (Maroto et al., 2005), are found to be triggered directly by the lipid bilayer during force propagation with no additional associated cellular constituents, such as the cytoskeleton. This corresponds to a kind of gating model that is commonly seen in bacterial ion channels and is referred to as the bilayer mechanism. In sharp distinction, the overwhelming majority of mechanosensitive ion channels present in the membranes of animal cells, which comprise multiple TRP channels (Kanzaki et al., 1999; Liman, 2007; Nilius et al., 2007; Pedersen and Nilius, 2007; Zhu et al., 2011), are controlled through a process referred to as the tethered mechanism. The tethered mechanism exerts a force on them indirectly through the cytoskeleton via ECM molecules like fibronectin and auxiliary scaffold constituents (Rohács et al., 1999; Matsuda et al., 2003). As a multitude of physical stimuli act on the plasma membrane, they are transmitted via the ECM toward the cytoskeleton, which is referred to as the dual tether model, by activating overlapping integrin receptors situated at focal adhesions (Geiger et al., 2009). Consequently, cytoskeletal actin and other structural elements may represent pivotal pathways of cellular force transduction that distinctly affect the mechanosensitivity of ion channels.
The opening of mechanosensitive ion channels, such as Piezo1, triggered upon shear stress or flow-induced membrane elongation constitutes the initial phase of mechanosignaling (Christensen and Corey, 2007; Murthy et al., 2017; Martino et al., 2018). These ion channels exhibit opposing characteristics sometimes, varying from hyperpolarization by K+-selective TREK channels to depolarization through Ca2+- and Na+-permeable Piezo1 channels. The primary area of focus was on mechanosensitive cation-permeable ion channels, causing Ca2+ entry in the endothelial cell. Ample evidence indicates that the increase in intracellular Ca2+ occurs as a first step in reaction to shear stress. In the endothelium, augmented Ca2+ is followed by the activation of eNOS and Ca2+-activated intermediate conductance K+ channels (IKCa), all of which leads to vasodilation via eNOS-driven NO liberation and possibly hyperpolarization of the plasma membrane. Ion channels appear to be exceptionally skilled at sensing forces, and it is widely assumed that they perform a pivotal task in the perception of shear stress (Hyman et al., 2017).
Transient receptor potential (TRP) channels operate as non-voltage-driven cation channels that are controlled by a variety of stimuli and are associated with a wide range of cellular processes (Nilius and Owsianik, 2011). There are, at minimum, 10 different TRP channels (TRPC1, 5, 6; TRPV1, 2, 4; TRPM3, 7; TRPA1; and TRPP2) that are suggested to act mechanosensitive (Tobin et al., 2002; Sidi et al., 2003; Christensen and Corey, 2007; Inoue et al., 2009b; Malsch et al., 2014). TRP can moderate Ca2+ transmission, although it may be regulated by direct Ca2+ engagement with the channel or by the Ca2+–calmodulin complex-driven activation mechanism. The inward Ca2+ current from TRP channels in VSMCs results in the depolarization of the plasma membrane and a compelled inward current via voltage-gated Ca2+ channels, comprising L-type or T-type Ca2+ channels, CaV1.2/CaV3.1. The Ca2+–calmodulin compound triggers myosin light-chain kinase and starts the contractile response (Hill-Eubanks et al., 2014). TRPV1 can exercise an anti-inflammatory impact on endothelial cells through the activation of eNOS over the eNOS/NO signaling route (Wang Y. et al., 2017). In the last two decades, there have been major advances in research on TRP channels, including their involvement in diseases and their function as mechanosensors (Zhang et al., 2023). The subsequent mechanisms have been proposed: first, direct activation through extracellular forces including membrane elongation and shear-induced alterations of the lipid bilayer structure and consequent distortion of channel domains (Gao et al., 2003; Maroto et al., 2005; Spassova et al., 2006); second, the engagement of ion channel architecture with cellular or matrix constituents including ECM, intracellular cytoskeleton, or proteins (Becker et al., 2009; Matthews et al., 2010); and third, secondary activation through other well-defined primary mechanosensors and their consequent biochemical conversion into effector TRP channels (Köhler et al., 2006; Loot et al., 2008; y Schnitzler et al., 2008). In the following, after the discussion of Piezo1, specific mechanosensitive receptor types of the TRP family are also presented and discussed, including TRPV4, TRPC1, TRPC6, TRPM7, TRPP2, and ENaC.
3.1.1 Piezo1
Piezo1 and Piezo2 constitute mechanically excited cation channels that function as large homomultimeric assemblies capable of facilitating cation fluxes in multiple tissues (Coste et al., 2015; Volkers et al., 2015). Both isoforms function as mechanically driven gates and convey non-selective Na+, K+, and Ca2+ ion flows characterized by rapid activation kinetics. However, Piezo2 is mainly expressed in tactile epithelial cells (Merkel) (Woo et al., 2014) and mechanosensory nerve cells (Murthy et al., 2017). Piezo1 can convey mechanically derived flows in different cell types, comprising endothelial cells and VSMCs. (Ranade et al., 2014; Retailleau et al., 2015). Piezo1 protein represents a trimeric, propeller-shaped channel protein consisting of a central anchor, three long rods, and three blade-like structures (Zhao et al., 2019).
Ion channels respond extremely sensitively toward mechanical forces and change their conformation when mechanically excited (Martinac, 2004). The most well-characterized ion channel in endothelial shear stress reactions is Piezo1, which is necessary for shear stress-imposed endothelial orientation, Ca2+ influx, and reshaping of focal adhesions in reaction to the flow (Li et al., 2014). Both the stable/laminar and disturbed flow trigger a signaling route through Piezo1 and the GPCRs Gq/G11 and P2Y2 (P2RY2) (Albarrán-Juárez et al., 2018). When the flow is disturbed, this route activates the mechanosensory complex and integrins, thereby initiating NFκB activity and inflammation, which ultimately leads to atherosclerosis. In addition, Piezo1 has been shown in zebrafish embryos to react to mechanical forces in heart valve development, where it induces endothelial expression of the transcription factor KLF2 (Duchemin et al., 2019). Piezo1 also acts in the flow-induced mitochondrial signaling route, resulting in the activation of ERK and upregulation of KLF2 (Coon et al., 2022). Intriguingly, Piezo1 associates with PECAM1, the Ca2+ influx, and dynamical remodeling of the actin cytoskeleton under flow (Chuntharpursat-Bon et al., 2023).
VE-cadherin at the endothelial cell–cell junction, where it maintains Ca2+, is a bone-fide mechanosensitive ion channel. Piezo1 is a true mechanosensory since it fulfills the criteria as such. Piezo1 acts as a non-selective cation channel, which is somewhat better permeable for Ca2+ compared to Na+. Electrophysiological and imaging investigations on endothelial cells derived from several vascular beds revealed that activation of Piezo1 results in cationic currents and intracellular Ca2+ signaling (Porto Ribeiro et al., 2022). Activation of Piezo1 can be accomplished in experiments by mechanical (for example shear stress) or chemical activation, such as by Yoda1—which is a selective Piezo1 activator (Servin-Vences et al., 2017). The impact of the endothelial cell Yoda1 imitates shear stress-induced reactions and is mitigated by inhibitors of mechanically gated channels (Wang S. et al., 2016).
Piezo1 has been found to be an effective sensor for shear forces in endothelial cells and is implicated in the orientation of the endothelial cells in the direction of the flow (Li et al., 2014). Endothelial Ca2+ signaling fulfills a critical task in the development of the vasculature. Therefore, after the identification of Piezo1 in endothelial cells, previous endeavors were focused on comprehending the impact of Piezo1-mediated Ca2+ transients on vessel evolution. Piezo1 also activates multiple Ca2+-dependent endothelial metalloproteinases implicated in the process of angiogenesis (Ichioka et al., 1997; Zhou et al., 2000; Kang et al., 2019). Piezo1-based Ca2+ events regulate wayfinding and cerebrovascular organization within brain endothelial cells (Liu T. et al., 2020). Collectively, these investigations have revealed that Piezo1-faciliated Ca2+ signaling is necessary for vascular evolution, patterning development, and angiogenesis. The activation of Ca2+-dependent enzymes is essential for the three-dimensional arrangement and orientation of endothelial cells throughout developmental processes. Ca2+-activated protease calpain, which cleaves focal adhesion proteins necessary for cellular orientation, becomes upregulated with the activation of Piezo1 and is critical during vessel evolution (Lai et al., 2022). Piezo1 activation has been found to be important for stress fiber orientation and the alignment of endothelial cells (Ranade et al., 2014). Laminar flow-based activation of Piezo1 induces the flow-triggered liberation of ATP from endothelial cells, leading to Gq/G11-coupled purinergic P2Y2 receptor engagement (Wang et al., 2015; Wang S et al., 2016). P2Y2 receptor and Gq/G11 pathways cause the activation of AKT and eNOS and facilitate flow-based vasodilation. Laminar and perturbed currents equally activate the identical early mechanosignaling route that integrates Piezo1- and Gq/G11-based paths (Albarrán-Juárez et al., 2018). Consequently, the Piezo1 channel activator Yoda1 triggers NO-driven relaxation of intrapulmonary arteries of mice (Lhomme et al., 2019; Porto Ribeiro et al., 2022). Moreover, in cultured microvascular endothelial cells, Piezo1 channel activation induced, either by shear stress or by the chemical agonist Yoda1, a disintegrin and metalloproteinase domain-containing protein 10 (ADAM10), which is a Ca2+-regulated transmembrane sheddase that facilitates S2-Notch1 modification by cleavage. In accordance with this finding, a Piezo1-induced abundance enhancement of the Notch1 intracellular domain (NICD) has been identified, which relies on ADAM10 and the subsequent S3 cleavage enzyme, namely, γ-secretase. Conditional endothelial-specific silencing of Piezo1 of adult mice repressed the expression of several Notch1 target genes in the liver vasculature, implying a constitutive functional importance of Piezo1 in vivo. Together, the data imply that Piezo1 functions as a mechanism that imparts force responsiveness to ADAM10 and Notch1, with subsequent implications for the persistent activation of Notch1 target genes and possibly related processes. Ca2+ translocations trigger subsequent signaling cascades and stimulate Ca2+-based endothelial mechanisms that control vascular diameter guidance (Caolo et al., 2020).
A pivotal mechanism enabled through Ca2+ signals involves the synthesis of the powerful vasodilator NO. Flow-mediated vasodilation is due to Piezo1-driven Ca2+ inward current and Ca2+-driven eNOS activation, NO formation, and ultimately relaxation of VSMC. Endothelial-cell specific loss of NO-driven vasodilation in lead to hypertension in Piezo1-knockout mice (Wang S. et al., 2016). In addition, the downregulation of Piezo1 leads to a reduction in renin and thus to blood pressure control in the kidney (Yang et al., 2022). Conditional endothelial cell-specific ablation of Piezo1 in adult mice resulted in a reduction in physical fitness (Bartoli et al., 2022). Apoptosis of muscle microvascular endothelial cells and capillary dilution were apparent and enough to account for the impact on performance. Selective high regulation of thrombospondin-2 (TSP2), which triggers apoptosis of endothelial cells, occurred without affecting TSP1, which is a cognate key actor in the physiology of muscle. In muscle endothelial cells, TSP2 was barely expressed but strongly expressed in muscle pericytes, where NO suppressed the Tsp2 gene without affecting Tsp1. In endothelial cells, Piezo1 was necessary for the normal endothelial NOS expression. The findings point to a cooperative partnership between endothelial cells and muscle pericytes, where the endothelial Piezo1 perceives the blood flow to preserve the capillary density and thus preserve the physical performance (Bartoli et al., 2022).
Nevertheless, Piezo1-driven vasodilation in several vascular patches is facilitated through NO generation (Li et al., 2014; Evans et al., 2018; John et al., 2018; Lhomme et al., 2019). Endothelial Piezo1 has been demonstrated to affect the activity of GPCRs. Laminar flow triggers Piezo1 activation and facilitates the liberation of adenosine triphosphate (ATP), which then stimulates the activation of purinergic Gq/11 PCRs to finally enhance NO generation (Albarrán-Juárez et al., 2018). Specifically, Gq-PCR activity is a key controller of ion channels in endothelial cells (Harraz et al., 2018a; Harraz et al., 2018a), but it is uncertain whether Gq-PCR signal transduction impacts endothelial Piezo1 activity.
The cation inward current connected with activation of Piezo1 causes depolarization of cells, such as endothelial cells (Rode et al., 2017; Ye et al., 2022). Endothelial cells are linked with VSMCs in structural and electrical terms through myo-endothelial protrusions and gap junctions (Yamamoto et al., 1999; Wu et al., 2006). This electrical pairing eases endothelial cell communication with adjacent VSMCs and probably facilitates the propagation of Piezo1-facilitated electrical cues from endothelial cells to VSMCs. Evidence indicates that Piezo1-triggered endothelial cell depolarization spills over to VSMCs and triggers the activation voltage-gated Ca2+ channels that induce vasoconstriction of the mesenteric artery (Rode et al., 2017). This accounts for the reported flow-mediated vasoconstriction rather than vasodilation and the complete cessation of this reaction in endothelial cells from Piezo1-knockout mice. These results, nonetheless, are in contrast to other investigations, demonstrating that blood flow-triggered vasodilation relies on NO and is mitigated in endothelial cells of Piezo1-knockout mice (Li et al., 2014). These disparities need to be examined more closely and may be partly attributable to differing expression profiles in the various arterial branches or to variations between the mouse models. It is also an open question whether Piezo1 stimulates Ca2+-activated ion channels within endothelial cells. When this is the case, Piezo1 activity potentially hyperpolarizes or depolarizes the Vm of endothelial cells through the activation of Ca2+-activated K+ or Cl− channels, which is a theory that needs to be verified experimentally. In general, Piezo1 is an essential mechanosensory mechanism within endothelial cells. Two envisionable potential scenarios exist for the prospective regulatory actions of capillary Piezo1 channels in the cerebral blood flow (CBF) (Harraz et al., 2022). In the first case, mechanical forces cause Piezo1-driven Ca2+ signaling, which might influence CBF at a local level through the production of NO and consequent relaxation of mural cells, such as pericytes or VSMCs. In the second place, Piezo1, acting as a non-selective cation channel, might convey a depolarizing conductance within capillary endothelial cells. From a conceptual perspective, hyperemia-induced Piezo1 activation potentially depolarizes capillary endothelial cells, which is an action that is proposed to be a built-in restraint system that enables membrane potential restoration, thus aiding the repetitive function of the previously described hyperpolarization-based NVC mechanism (Longden et al., 2017). The two outlined mechanisms are not strictly mutually independent; they may occur in parallel and control CBF by spatially and temporally different mechanisms. In confirmation of this hypothesis, the Ca2+/Na+-permeable TRPV4 channel within the cerebral capillaries appears to fulfill two tasks: control of membrane potential (Harraz et al., 2018b) and facilitation of Ca2+ signal transduction (Dabertrand et al., 2021). It remains unclear as to whether and how Piezo1 channels control CBF and, therefore, needs experimental modification of Piezo1 with simultaneous surveillance of CBF, which is a key aspect under investigation.
Piezo1 channels open as a reaction to a variety of mechanical cues, such as physical membrane deformations that regulate the curvature of flexible domains known as blades. It has been revealed that flow-induced blade movements are functionally coupled to the pore and that at least two widely separated blade regions distinguish flow from two other stimuli, indicating that Piezo1 utilizes different mechanisms to perceive a wide spectrum of mechanical stimuli (Ozkan et al., 2022). An uncommon characteristic of Piezo1 is the highly curved blade, which allows the protein to locally distort the membrane to form a dome shape (Guo and MacKinnon, 2017). When the channel opens, the enclosing membrane is flattened, which expands the dome into an in-plane configuration (Guo and MacKinnon, 2017). This specific event produces free energy, which could act as a cause for the mechanical gating of Piezo1 (Guo and MacKinnon, 2017). Membrane dome hypothesis is a piece of evidence that piezoelectric channels obey the force-from-lipid (FFL) model, which proposes that mechanosensitive channels are directly manipulated through variations from nearby membrane curvature and tension (Martinac and Kung, 2022). A further model that can account for the sensitivity of piezo channels to wide-ranging mechanical cues is the force-from-filament (FFF) model, which enables full-cell mechanosensing across the cytoskeleton (Jiang et al., 2021; Chuang and Chen, 2022). In fact, piezo channels act as a physical connection to the actin cytoskeleton through the cadherin–β-catenin–vinculin complex, and the Cap domain of Piezo1 also interfaces in a direct manner with the extracellular domain of E-cadherin (Wang et al., 2022b) and possibly also VE-cadherin in a similar way (Chuntharpursat-Bon et al., 2019). In living cells, Piezo1 is able to exploit the FFF model in concert with the FFL model, allowing them to act as multipurpose and adaptable mechanotransducers (Jiang et al., 2021). Apart from the activation of the plasma membrane or the filaments of the cytoskeleton, it was found that the activation of Piezo by the ligand Yoda1 is independent of the presence of TRPV4 (Harraz et al., 2022), all of which leads to the hypothesis that Piezo also possibly fulfills a prominent and unique function in mechanosensation and mechanotransductions. The lack of specific and selective inhibitors, given that the molecular structure and ligand binding mechanism are not fully elucidated, is a major challenge in Piezo1 research. Progress has recently been achieved in the design of novel selective inhibitory substance of the Piezo1 channel (Thien et al., 2024). The discovery of inhibitors, although, is so far in its fledgling stages.
3.1.2 TRPV4
TRPV4 was first recognized in the endothelium in 2002 (Watanabe et al., 2002). TRPV4 has since been detected in endothelial cells of various vascular sites, including carotid arteries, mesenteric resistance arteries, pulmonary arteries, arterioles of the skeletal muscle, and cerebral capillaries (Köhler et al., 2006; Hartmannsgruber et al., 2007; Mendoza et al., 2010; Earley and Brayden, 2015). TRPV4 acts as a non-selective cation channel, which is better permeable for Ca2+ compared to Na+ ions (Liedtke et al., 2000; Strotmann et al., 2000). The endothelial TRPV4 channel participates in multiple vascular processes, which includes control of vascular tone, endothelial cell alignment, angiogenesis, and perfusion (Earley and Brayden, 2015; White et al., 2016). TRPV4 appears to be a prospective receptor for the molecular blood flow sensor that triggers flow-based vasodilation, which is a reaction to elevated blood fluid speed or enhanced blood fluid viscosity. In agreement, TRPV4 has been found to be activated during hypertonic circumstances and membrane elongation induced through cell swelling (Liedtke et al., 2000). The TRPV4 channel reacts sensitively to forces and is essential for recognizing shear stress in endothelial cells (Köhler et al., 2006; Hartmannsgruber et al., 2007; Mendoza et al., 2010). Whether TRPV4 is a genuine mechanosensor is, nonetheless, controversial. Cell-attached patch-clamp approaches failed to activate TRPV4 directly through pipette suction, indicating its indirect activation via force-driven signaling pathways (Earley and Brayden, 2015). Using the rat carotid artery, it has been demonstrated that activation of TRPV4 triggered by endothelial cell agonists or shear stress causes expansion of the rat gracilis arteries. The impairment of eNOS mitigates the TRPV4-driven response (Köhler et al., 2006). In addition, TRPV4 knockouts exhibited markedly decreased flow-driven vasodilation (Hartmannsgruber et al., 2007). In this context, TRPV4-driven relaxation has been identified engaging NO and EDHFs, as well as Ca2+ inward flow across endothelial TRPV4 channels in reaction to flux (Mendoza et al., 2010). Thus, direct activation of the channel by mechanical forces has not yet been clearly identified, which indicates that TRPV4 may not be a true mechanosensor (Liedtke et al., 2000; Loukin et al., 2010; Servin-Vences et al., 2017; Nikolaev et al., 2019). Beyond this, the fact that TRPV4 can be stimulated in a physiological manner through endogenous compounds like arachidonic acid (AA) and epoxyeicosatrienoic acids (EETs), which are liberated as a reaction to shear stress, would account for how TRPV4 activity rises with force (Watanabe et al., 2003; Köhler et al., 2006; Loot et al., 2008). In this sense, Piezo1 activation has been found to induce AA metabolite formation in tissue-engineered endothelial cells, which ultimately increases the activity of TRPV4 (Swain and Liddle, 2021). These results imply that Piezo1 functions as the mechanosensor and TRPV4 is functionally downstream to augment and maintain the mechanically generated Ca2+ inward current.
Endothelial TRPV4 activation causes vasodilation via NO liberation and/or by changing the Vm of endothelial cells. These two regulatory principles are not inherently mutually independent and potentially synergistic. Strongly localized Ca2+ effects during the activation of TRPV4 channels, referred to as TRPV4 sparklets, are linked to, first, the activation of eNOS, NO generation, and vasodilation, or second, the engagement of Ca2+-activated K+ channels and subsequent endothelial cell hyperpolarization (Köhler et al., 2006; Hartmannsgruber et al., 2007; Mendoza et al., 2010; Sonkusare et al., 2012; Longden et al., 2021). Genetic ablation of TRPV4 causes dampened endothelial Ca2+ reactions and impaired NO liberation (McFarland et al., 2020). The enhancement of TRPV4-driven Ca2+ signal transduction is achieved by sensitization of the inositol 1,4,5-trisphosphate receptor (IP3R) (Heathcote et al., 2019).
TRPV4 channels engage with a variety of proteins and molecules, including proteins and molecules that are manipulated directly through mechanical forces. Similar to various other TRP channels, TRPV4 undergoes activation, following GqPCR induction. Activation of endothelial GqPCR increases the activity of phospholipase C (PLC) and the hydrolytic breakdown of phosphatidylinositol 4,5-bisphosphate (PIP2) into IP3, which results in the liberation of Ca2+ from intracellular storage, and diacylglycerol (DAG), inducing protein kinase C (PKC) activation. GqPCR signal transduction in endothelial cells triggers the activation of TRPV4 channels in a PKC- or PIP2-dependent mode (Sonkusare et al., 2014; Harraz et al., 2018b), and IP3/IP3 R signal transduction enhances the activity of TRPV4 (Heathcote et al., 2019). Since some GPCRs exhibit mechanosensitivity (Xu et al., 2018) (see section below), the activation of these GPCRs through forces could increase endothelial cell TRPV4 activity, prompting the hypothesis that TRPV4 channels are integral mechanosensors. Notably, GqPCR signal transduction and subsequent regulation of ion channels like TRPV4 and Piezo1 may represent an important interface between various mechanosensing mechanisms. CAV1, a scaffold protein, directly engages with TRPV4 and colocalizes with gap junction proteins to promote electrical pairing. In fact, CAV1 is necessary for the endothelial Ca2+ inward flow and consequent vasodilation (Saliez et al., 2008; Rath et al., 2012). Overall, there is strong support that caveolae are critical microdomains participating in the activity of TRPV4 and vasodilation. In addition to mechanosensing and flow-induced vasodilation, there are several other TRP channels that are key for signal transmission in endothelial cells. For instance, the cerebral endothelial transient receptor potential ankyrin 1 channel (TRPA1) is part of the neurovascular connection, and the activation of TRPA1 through reactive oxygen species causes vasodilation (Sullivan et al., 2015; Thakore et al., 2021).
Shear stress also results in exocytosis-facilitated enrollment of TRPV4 channels and mechanical stress awareness of the endothelium (Baratchi et al., 2016). TRPV4 can colocalize with TRPC1 proteins in endothelial cells derived from rabbit mesenteric arteries. High external Ca2+-based endothelial cell-driven vasodilation exhibited TRPV4- and TRPC1-driven Ca2+ affluence and NO production stimulation. Activation of TRPV4-based triggered NO formation and consecutive vasodilation could be impeded with N(ω)-nitro-L-arginine methyl ester (L-NAME), which is an eNOS inhibitory substance, the TRPC1 antagonist T1E3, which is an inhibitory peptide or TRPV4 antagonist RN1734. Heteromeric TRPV4 and TRPC1 channels convey calcium receptor-triggered vasorelaxation via NO generation (Greenberg et al., 2017). In HUVECs, agonist-driven stimulation of the calcium-sensing receptor (CaSR) results in a TRPC1-driven elevation of intracellular Ca2+ ion levels and encourages the generation of NO. It has been postulated that engagement of TRPC1 with CaSR and TRPC1-mediated store-operated Ca2+ entry (SOCE) mechanisms have been hypothesized to promote an inward flow of Ca2+ ions (Qu et al., 2017). TRPC1 co-localizes with TRPV4 in mesenteric artery endothelial cells. This kind of heteromeric channel is triggered through CaSR and enhances NO formation and vasorelaxation (Greenberg et al., 2017; Greenberg et al., 2019).
3.1.3 TRPC6
TRPC6 appears to be a mechanosensitive TRP channel that can be specifically and directly activated via diacylglycerol (Hofmann et al., 1999; Kress et al., 2008). TRPC6 functions to modulate endothelial permeability in reaction to proinflammatory cytokines and inflammatory mediators (Leung et al., 2006; Singh et al., 2007). In pulmonary artery ECs, TRPC6 silencing reduced TRPC6 agonist-driven rise in intracellular Ca2+ ion levels, vascular perfusion, and edema production (Samapati et al., 2012). Cytochrome P450 (CYP)-based epoxyeicosatrienoic acids (EETs), which are among the other mechanically generated compounds, promote the displacement of TRPC6 into caveolin-1-rich cell plasma membrane areas, with increased caveola generation upon fluid shear stress (Fleming et al., 2007). The direct mechanical activation of TRPC6 has not yet been clearly identified. The synergistic activation of a dual mechanical and muscarinic receptor agonist carbachol-mediated activation mechanism has been postulated (Inoue et al., 2009a).
3.1.4 TRPM7
TRPM7 expression has been detected within HUVECs (Baldoli et al., 2013), wherein it is coupled to the trafficking of magnesium. TRPM7 is, to some extent, unique relative to other TRPs, in which it contains a regulatory kinase domain located near the C-terminus (Ryazanova et al., 2014). The mechanosensitive capability of TRPM7 has been confirmed in pressure-loaded patch-clamp assays (Xiao E. et al., 2015) and in fluid shear stress studies of mesenchymal stromal cells (Liu et al., 2015).
3.1.5 TRPP2
TRPP2, synonymously referred to as polycystin-2 or polycystic kidney disease 2 (PKD2), has been coupled to mechanosensitive functions of primary cilia. Decreased expression of TRPP2 decreases NO formation of mouse endothelial cells (AbouAlaiwi et al., 2009). Elimination of TRPP2 prevents endothelial cells from converting external shear stress into intracellular Ca2+ signals and nitric oxide production (Nauli et al., 2008). An interplay of TRPP2 and TRPC1 and a hypothesized involvement in stretch-driven blood–brain barrier endothelial cell damage are also proposed (Patel et al., 2010; Berrout et al., 2012). Moreover, it has been found that, solely, a heteromeric channel assemblage of TRPP2, TRPC1, and TRPV4 is capable of imparting flux-driven cation flows (Earley and Brayden, 2015).
3.1.6 Kir2.1
In an early effort to identify mechanosensors in endothelial cells, a K+ channel was revealed that responds to forces (Olesen et al., 1988). Expression of functional Kir2 channels has been identified in VSMCs (Sancho et al., 2022). Kir2 channels are not subject to conventional regulatory oversight, which is why they are frequently regarded as being merely background conductance (Sancho et al., 2022). Two membrane lipids, phosphatidylinositol 4,5-bisphosphate (PIP2) and cholesterol, provide stabilization of Kir2 channels in a favored open or closed configuration and, in conjunction with the cytoskeleton, CAV1 and syntrophin, endow them with hemodynamic sensitivity.
Kir2.1 channels, which are part of the inwardly rectifying potassium Kir channel family that let larger amounts of potassium ions into the cell than out of it, have been linked to neurovascular coupling (NVC). The cerebral circulation consists of a mesh of interlinked surface vessels with a substantial capacity to reroute the blood flow (Blinder et al., 2013). Arterioles enter the brain in an orthogonal fashion, ramify into smaller arterioles, and merge into capillaries, which vastly expand the zone of blood flow. Capillaries, which comprise the tiniest of all blood vessels, are small of approximately 5-μm diameter ducts composed of a monolayer of capillary endothelial cells. It has been observed that inwardly rectifying Kir2.1 channels in capillary endothelial cells are activated by K+ liberated by active neurons, causing a spreading hyperpolarization that propagates upstream and enlarges arterioles, referred to as dilation (Longden et al., 2017). This mechanism is a key driver of the NVC mechanism, which conveys an enhancement of the local blood flow toward active neurons, which is referred to as functional hyperemia (Longden et al., 2017; Harraz et al., 2018a; Dabertrand et al., 2021). Since the red blood cells are a little more than capillary diameter, the blood cells get “squeezed” through the capillary in a single stream, thereby exerting a unique mechanical force on the capillary endothelium. It is currently uncertain how the capillaries of the brain perceive the forces connected with blood motion and whether this mechanosensitivity plays a role in NVC. There is, therefore, a need to investigate this in further studies.
In cultured aortic endothelial cells, a Kir channel has been found to be activated by shear stress. It was hypothesized that this channel participates in flow-based hyperpolarization and the regulatory control of vascular tone (Olesen et al., 1988). This research sparked the pursuit of the function of Kir channels in mechanosensation and regulation of blood flow. The Kir2.1 channel found to be expressed in endothelial cells from various vascular types (Zaritsky et al., 2000; Ahn et al., 2017; Longden et al., 2017), is responsive to extracellular K+ levels, and its activation is triggered by hyperpolarization (Hibino et al., 2010). The flow activates Kir2.1 in a way that relies on the level of shear stress and oscillation frequency, which results in K+ efflux and hyperpolarization. In addition to regulation of Vm, Kir2.1 activity has been implicated in NO generation (Hoger et al., 2002; Lieu et al., 2004; Ahn et al., 2017). It has been hypothesized that Kir2.1 in endothelial cells is critical for flow-triggered phosphorylation and activation of eNOS and Akt and downstream NO production, probably in a Ca2+-independent fashion (Ahn et al., 2017). Shear stress enhances Kir2.1 activity, although the channel may not serve as the primary mechanosensor. Cell-attached electrophysiology was utilized to induce shear activation of Kir channels, although the cell-tethered membrane was not necessarily directly subjected to shear stress (Jacobs et al., 1995). It is probable that some upstream mechanosensing mechanisms are implicated in the reaction of Kir2.1 to shear stress. It is speculated that shear stress changes the lipids and fluidity of the plasma membrane, which then, in turn, impacts the activity of Kir2.1. Kir2.1 is, in fact, an essential regulatory target of phosphoinositides such as PIP2 and cholesterol (Romanenko et al., 2004; Harraz et al., 2018a). In arterial endothelial cells, among ion channel mechanosensors, Piezo1 and Kir2.1 channels convey shear stress-initiated NO liberation, although the Piezo1 channel functions in a depolarizing manner via the Ca2+/Na+ influx and Kir2.1 in a hyperpolarizing manner via the K+ efflux (Wang S. et al., 2016; Ahn et al., 2017). In other investigations, flow-driven activation of the TRP polycystin (PKD2) channel has been connected to the activation of eNOS and K+ channel-based hyperpolarization (MacKay et al., 2020). These findings and other related findings underscore the versatility of endothelial signal transduction. Endothelial cells use various mechanisms to provide resilient signaling and mechanotransduction paths in reaction to hemodynamic forces.
3.1.7 ENaC
ENaC is a type of Na+ channel that is nonvoltage-dependent, composed of three subunits, constitutive active channel, and an amiloride-sensitive channel. It belongs to the ENaC/degenerin family (Kellenberger and Schild, 2002). ENaC has been originally identified in epithelial cells, and it has been suggested to express exclusively on these cells. The epithelial sodium channel ENaC has been characterized mainly in the principal cells of the distal renal nephron, where it is mostly implicated in water and salt balance homeostasis (Garty and Palmer, 1997; Bhalla and Hallows, 2008). Evidently, ENaC is widely expressed in several different tissues, where it performs various tasks (Zhang et al., 2022). In specific, ENaC has been detected in the vascular endothelium, in which it regulates endothelial mechanical characteristics at the nanoscale (Fels et al., 2010; Tarjus et al., 2017). The endothelial ENaC channel seems to be activated through laminar flow in a direct fashion. ENaC, along with several other ion channels, is connected to cytoskeletal elements, and these connections serve for mechanotransduction purposes (Mazzochi et al., 2006; Ilatovskaya et al., 2011; Fels et al., 2012; Warnock et al., 2014). It is assumed that elevated ENaC activity within the endothelial cells and, thus, enhanced sodium inflow cause the cortical actin to become stable in its filamentous form (F-actin), thereby creating a stiffer cell cortex (Oda et al., 2001; Warnock et al., 2014). AFM-based nanoindentation approaches were employed to demonstrate a relationship between shear stress-induced ENaC membrane abundance, polymerization of actin, and the nanomechanical characteristics of the endothelial cortex. In other words, shear stress under laminar flow resulted in a stiffening of the cortex of the cells by 18.9% ± 5.5% (N = 3, n = 37, p ≤ 0.01) relative to static control conditions, which can be attributed to an elevated quantity of F-actin in laminar shear stress (Cosgun et al., 2022). It is important to note that the implementation of non-laminar shear stress enhanced cortical stiffness by a further 67.9% ± 8.9% (N = 3, n = 36, p ≤ 0.01), which was accompanied by additional non-laminar shear stress-driven actin polymerization (Cosgun et al., 2022). Consequently, these results emphasized the impact of non-laminar shear stress in a robust in vitro model. Moreover, an increase in the ENaC channel density and/or activity, referred to as ENaC gain-of-function (GOF), has been demonstrated to cause endothelial cells to stiffen and subsequently lead to vasoconstriction (Jernigan and Drummond, 2005). ENaC GOF, resulting from elevated channel expression, has been seen in hypertension (Mills et al., 2016; Ahmad et al., 2023). The mechanisms through which ENaC GOF impairs endothelial functioning and shear stress-driven vasodilation include decreased generation of NO. NO loss is the outcome of increased Na+ influx, which weakens the driving force for L-arginine, which is a NO precursor, incorporation through cationic amino acid transporters. As an alternative, NO depletion can result from the negative regulation of the phosphoinositide 3-kinase (PI3K)/Akt pathway, which is essential for the synthesis of NO (Stojanovic et al., 2006).
Functional blockade of ENaC can cause a switch from F- to G-actin, resulting in a softening of the cell cortex (Fels and Kusche-Vihrog, 2020). On the contrary, the fixation of the actin cytoskeleton through chemical substances cancels this switching effect. In addition, the decrease in the spacing between the cortex and actin filaments is crucial for the thickness and tension of the cortex (Lembo et al., 2023). Thus, ENaC functioning and actin dynamism are tightly coupled in endothelial cells. In addition, ENaC could be triggered due to the flow and elevated hydrostatic pressure, and raised intracellular sodium concentrations cause lower NO formation in endothelial cells (Guo et al., 2015). In agreement with these results, ENaC has been observed to insert into the plasma membrane of HUVECs (cultured as a 2D monolayer) due to acute shear stress application or alterations (Cosgun et al., 2022). Consequently, an enhanced Na+ flow into the endothelial cells and the polymerization of the cortical actin take place. ENaC shear force detection relies on the engagement of sugar residues with the endothelial glycocalyx. Extracellular N-glycosylated asparagine moieties of ENaC bind the channel to both the ECM and endothelial glycocalyx, and elimination of these N-glycans decreases shear force-triggered ENaC flows (Leung et al., 2006). Notably, the endothelial glycocalyx is also engaged in the capacity of endothelial ENaC to moderate flow-mediated vasodilation, and removal of ENaC in endothelial cells abrogates shear stress-based relaxation.
Together, these findings reinforce the concept of a close cooperation and mutual relationship between the endothelial glycocalyx, ion channel functionality, and the endothelial cytoskeleton as linked mechanosensors. Finally, ENaC has a dual function, such as being beneficial or detrimental for the vascular function (Zhang et al., 2022). In summary, normal endothelial ENaC activity promotes shear stress-mediated vasodilation, whereas increased ENaC activity is associated with dysfunction of the endothelium. The differential effect of ENaC on vascular functioning can be attributed to the heterogeneity of the vascular sites and whether ENaC activity is increased in physiological or pathological manners (Zhang et al., 2022).
3.1.8 TREK-1
Two-pore K+ channel (K2P channel) superfamily members have been hypothesized to act as endothelial mechanosensors. The K2P channels are widely found to be expressed in various vascular patches, where they control the vascular function (Gurney and Manoury, 2009). TRAAK, TREK-1, and TREK-2 belong to the K2P channel family, and the TREK-1 channel has been thoroughly characterized in endothelial cells (Lesage et al., 2000; Brohawn et al., 2014). TREK-1 exhibits a direct responsiveness to positive and negative pressure within lipid bilayers, and the mechanosensitivity of the channel is maintained in various electrophysiological settings (Patel et al., 1998; Brohawn et al., 2014). TREK-1 is directly controlled through membrane tension; distortion of the plasma membrane removes the lipid blockade of ion conductance and consequently increases the activity of the channel, which results in hyperpolarizing flows (Honoré et al., 2006; Brohawn et al., 2014; Brohawn, 2015; Schmidpeter et al., 2023). TREK-1 not only modifies the Vm value of endothelial cells but is also involved in endothelium-driven, NO-facilitated vasodilation (Garry et al., 2007). The TREK-1 channel is not only solely mechanosensitive but also sensitive to osmotic pressure and becomes activated through ischemia-associated swelling. The cerebrovascular TREK-1 channel, therefore, plays a preservative role in the event of ischemia as it hyperpolarizes the endothelial cells and dilates the vessels (Patel et al., 1998; Maingret et al., 1999; Rao et al., 1999). It is hypothesized that hyperpolarization conveyed by TREK-1 may act in synergy with some other hyperpolarizing channels or mechanosensors, such as Kir2.1, or antagonize depolarizing channels, such as Piezo1 or TRPV4. The TREK-1 channel is regulated through GPCRs, with several of them being mechanosensors (Maingret et al., 1999; Lesage et al., 2000; Chemin et al., 2005; Mathie, 2007; Brohawn et al., 2014; Brohawn, 2015). PIP2 increases the activity of TREK-1, and cyclic adenosine monophosphate/protein kinase A (cAMP/PKA) acts to repress the channel. Consequently, TREK-1 activity is inhibited through Gs-PKA (enhanced inhibitory PKA) and Gq-PKA (reduced stimulatory PIP2) but is not blocked through Gi-PKA (reduced PKA) (Chemin et al., 2005; Mathie, 2007). Overall, the function of endothelial TREK-1 in mechanosensing is probably influenced through the other mechanosensitive GPCRs in place.
3.1.9 Future perspectives of ion channel actin as mechanosensors
Many endothelial mechanosensitive ion channels have been examined, and the emerging lines of evidence that Piezo1 is a genuine mechanosensor are very strong. Piezo1 appears to be expressed in most vascular grafts investigated so far, but the functions of endothelial Piezo1 are still not sufficiently characterized, which has been due to the recent detection of Piezo1 and the general relatively low number of specific/selective blockers. There exist inhibitors against the Piezo-ligand Yoda1, such as salvianolic acid B (Pan et al., 2022), and the Yoda1 derivative Dooku1 (Evans et al., 2018; Hatem et al., 2023). Recently, there have also been inhibitors that target Piezo1, comprising the toxin GsMTx4 from tarantulas; specific fatty acids; amyloid beta (Aβ) peptides; metal-containing compounds, such as ruthenium red and gadolinium; the Yoda1 derivative Dooku1; and natural compounds, such as tubeimoside I, salvianolic acid B, jatrorrhizine, and escin (Tapia and Velasco, 1997; Peng et al., 2016; Evans et al., 2018; Thien et al., 2024). The results showed that Piezo1 misexpression can be linked to a variety of chronic illnesses, which range from hypertension to cancer and hemolytic anemia. As a result, the blockade of Piezo1 and the resulting calcium inward current may have favorable implications for several pathological processes as several in vitro and in vivo investigations have been revealed (Thien et al., 2024). The development of Piezo1 inhibitors is still in its infancy, although, and there are still plenty of options and many challenges to be exploited.
As mentioned above, several mechanosensors cooperate, and Piezo1 may be a crucial element in these mechanisms. It is assumed that progress will be made in the near future in gaining an improved insight into mechanosensors, in general, and Piezo1 as the prevailing channel mechanosensor in endothelial cells. Remarkably, the expression of these ion channel species differs according to the anatomical position of the endothelial cells in the vascular tree. Arterial/arteriolar endothelial cells exhibit IK and SK channels, which are crucial in converting increases in intracellular Ca2+ levels into hyperpolarizing/dilator cues, whereas in the capillary endothelium, this ion channel family is lacking (Jackson, 2022). Notably, endothelial cells that do not express voltage-gated channels can be electrically coupled to VSMCs and can, therefore, directly control the basal tone of the vessels (Ebong et al., 2014). Finally, the characteristics of the mechanosensors must be defined to be able to better work out the similarities and differences. In addition, these hallmarks could be used to focus on the essential mechanisms of these receptors in the regulation of vascular functions to be able to intervene in these mechanisms with inhibitors or activators.
3.2 G-protein-coupled receptors
Selected G-protein-coupled receptors are briefly presented as mechanosensory units. Canonical activation of a GPCR is induced when an extracellular ligand engages the receptor, thereby initiating second messenger cascades that rely on Ca2+ signal transduction, cyclic nucleotides, or β-arrestin. Nevertheless, some GPCRs transduce mechanochemical signaling, regardless of ligand tethering (Takada et al., 1997; Chachisvilis et al., 2006). The mechanisms behind the mechanosensitivity of GPCRs are not yet completely elucidated but probably comprise alterations in membrane fluidity that permit GPCRs to acquire an active configuration even in the complete lack of a receptor agonist (Gudi et al., 1998; Yamamoto and Ando, 2013; 2015). In addition, helix 8, which is a conserved helical motif situated adjacent to transmembrane segment 7, has been demonstrated to confer mechanosensitivity to GPCRs. Structural and functional investigations have revealed that mechanical pacing extends helix 8 and thus stimulates the activation of GPCR (Erdogmus et al., 2019). Multiple mechanosensory mechanisms intertwine. Activation of an endothelial cell mechanosensor is frequently accompanied by metabolic alterations. Shear stress triggers Gq-PCRs and subsequently enhances the production of DAG and IP3 and the degradation of PIP2. Alterations in the intracellular metabolite content have considerable effects on mechanosensitive ion channels. DAG increases the activity of TRPV4 in arterial endothelial cells. Depletion of PIP2 increases TRPV4 and TREK-1 activity and represses the Kir2.1 activity. The activation of Piezo1 can increase AA synthesis, and then, AA triggers TRPV4 and TREK-1 and subsequent reactions (Patel et al., 1998; Chemin et al., 2005; Sonkusare et al., 2014; Harraz et al., 2018b; Harraz et al., 2018b; Swain and Liddle, 2021). The overlaps among various metabolic routes are hence key components of mechanosensory mechanisms.
3.2.1 GPR68
G protein-coupled receptor 68 (GPR68) has been shown to be an endothelial Gq/11-coupled receptor that acts to promote flow-induced vasodilation by increasing NO levels. Deletion of GPR68 compromised flow-based dilation of mesenteric arteries and had no impact on the regulation of blood pressure (Xu et al., 2018). Disruption of the structural integrity of the helix 8 (H8) domain of GPR68 by amino acid replacement limits mechanosensitivity, suggesting that H8 is an essential structural motif that provides mechanosensitivity to GPCRs (Erdogmus et al., 2019). Inconsistent findings employing cultured cells, nevertheless, revealed that flow-driven GPR68 activation is sustained, regardless of H8 removal or the pharmacological blockade of G protein signal transduction (Ozkan et al., 2021). The latter is in accordance with the piece of evidence that shear stress triggers the activation of Gq/11, regardless of the activation of GPCRs in cultivated endothelial cells (Dela Paz et al., 2017).
3.2.2 Bradykinin 2 receptor
Another type of endothelial mechanosensors is the Gq/11-coupled bradykinin-2 (B2) receptors (Chachisvilis et al., 2006). Shear stress, hypo-osmotic excitation, or a modification of plasma membrane fluidity stimulates the B2 receptor, resulting in an elevation of intracellular Ca2+ ion levels. Ca2+ signal transduction facilitated through the endothelial B2 receptor causes vasodilation through enhancing NO generation or stimulating Ca2+-driven hyperpolarization (Liao and Homcy, 1993; Chachisvilis et al., 2006; Hu et al., 2022). In addition, the endothelial B2 receptor increases the generation of AA, which can elevate the Ca2+ flux through TRPV4 (Watanabe et al., 2003), indicating a possible link between the B2 receptor and other mechanosensors.
3.2.3 Endothelial sphingosine-1-phosphate receptor 1 and histamine H1 receptor
Shear stress has been found to induce activation of sphingosine-1-phosphate receptor 1 (S1PR1) and the histamine H1 receptor (H1R) in endothelial cells. Activation of S1PR1 triggers NO synthesis, which causes vasodilation. S1PR1 is likewise critical for vessel development and homeostasis of blood pressure (Jung et al., 2012; Cantalupo et al., 2017). Overall, the results indicate that S1PR1 acts as a pivotal element in an endothelial mechanosensory signal transduction route, behaving more as a mechanotransducer than as a sensor. H1R contains helix 8 and has thus been described as an endothelial mechanosensitive GPCR that can promote NO signal transduction and vasodilation (Erdogmus et al., 2019).
3.2.4 Perspectives for the mechanosensitive GPCRs
Endothelial GPCRs that act as putative mechano-GPCRs comprise GPR68, B2 receptor, S1PR1, and H1R. Mechanosensing evidence indicates that GPR68 may fulfill the most important function. The expression at the messenger RNA (mRNA) stage, nevertheless, is most prominent for S1PR1 and tends to be minor for other culprits (Vanlandewijck et al., 2018). Moreover, some putative mechanosensitive Gq/11-coupled receptors are lacking indications of a direct activation through mechanical forces when exposed to heterologous expression networks, confirming the concept that GPCRs are implicated in mechanosensation in an indirect manner (Marullo et al., 2020). The downstream actions of GPCR signaling intersect with and modify the activity of other mechanosensitive ion channels; the complement of the endothelial cell receptors may hence orchestrate and control the mechanosensitive ion channel activity (Harraz et al., 2018b). This indicates that the mechanosensors work together, are functionally linked, and thus may be able to cancel each other out.
3.3 Cell matrix receptors such as integrins
Another focus of the review article is placed on apical mechanotransduction and on cell–matrix mechanotransduction. Cell–matrix receptors like integrins act as the major mechanosensors on the basal face of the endothelial cell, establish a direct connection from the actin cytoskeleton to the ECM (Geiger et al., 2009; Eyckmans et al., 2011), and are implicated in the reaction toward substrate-related and flow-related stimuli (Tzima et al., 2005).
Integrins constitute heterodimeric adhesion receptors consisting of α- and β-subunits that are capable of tethering extracellular matrix compounds, among other ligands, that are essential for basement membrane attachment (Hynes et al., 2002). Integrins can create 24 different, specific integrin heterodimers. Various integrin heterodimers couple to various ligands, such as fibronectin receptors, collagen receptors, laminin receptors, and leukocyte-specific receptors (Hynes, 2002). Expression of integrins is specific to cells, and the various α/β-subunit pairs generated determine the types of extrinsic ligands that cells can engage, impacting their differentiation and performance. Integrins are connected to the actin cytoskeleton via focal adhesion proteins, such as talin, vinculin, kindlin, and paxillin, which also serve as signal transduction centers that promote intracellular signal transmission (Sun et al., 2019; Kadry and Calderwood, 2020). Integrin signaling is unique because it is bidirectional as it can be triggered by the engagement of external ligands (outside-in signaling) or by the engagement of cytosolic scaffold proteins (inside-out signaling) (Hynes, 2002; Ye et al., 2012; Humphries et al., 2019; Kadry and Calderwood, 2020). Most of the knowledge about integrins and their functioning stems from the examination of cell–cell interactions among leukocytes (Chigaev and Sklar, 2012; Martín-Cófreces et al., 2018) and cell/ECM adhesion at the basolateral cell surface (Schwartz, 2010; Geiger and Yamada, 2011).
The structure and conformation of integrins are crucial to each part of its operation, ranging from adhesion to signal transduction. The α-subunit comprises a head domain and two leg domains (Luo et al., 2007; Campbell and Humphries, 2011). Half of the α-subunits possess an I domain incorporated into the head domain, which enables them to bind divalent metal ions that can function as an activation switch (Luo et al., 2007; Campbell and Humphries, 2011). Similar to the α-subunit, the β-subunit contains leg domains in the upper and lower parts of the molecule, as well as a head domain with a cation-tethering I-like domain (Luo et al., 2007; Campbell and Humphries, 2011). This structure allows both α- and β-subunits to take on conformations with bent and stretched head groups (Luo et al., 2007; Campbell and Humphries, 2011). In inactive conditions, integrins exist in a bent conformation (Takagi and Springer, 2002; Luo et al., 2007; Ye et al., 2012). The conformational rearrangement that enables the head group to switch to an outstretched conformation coincides with activation (Takagi and Springer, 2002; Ye et al., 2012). Activation is a prerequisite for the engagement of integrins with ligands and for the transduction of intracellular cues. Following activation, integrins exhibit a higher affinity for ligand engagement (Luo et al., 2007; Ye et al., 2012). Although the elongated head group is a characteristic of activated integrins, they can also assume intermediate conformations, such as an elongated closed conformation in which the head group is turned outward but still exhibits a low affinity for the linking ligand (Ye et al., 2012; Su et al., 2016; Li and Springer, 2017).
The resting endothelium expresses a minimum of seven classes of integrins, where β1-integrin, being one of the most well-characterized in the endothelium, confers the specificity for fibronectin (α5β1), laminin (α3β1 and α6β1), and collagen (α1β1 and α2β1) (Stupack and Cheresh, 2002). Simultaneous endothelial-specific α5 and αv deletion results in heart vascular defects and in embryonic lethality up to E14.5 (Van Der Flier et al., 2010). Endothelial cell-specific deletion of β1-integrin throughout development led to embryonic lethality ranging from E9.5-E.10 .5, which has been found to be marked through defective vascular patterning and vascular abnormalities (Lei et al., 2008). Inducible genetic knockdown of β1-integrin in endothelial cells or postnatal pharmacological inhibition of β1-integrin compromised lumen generation and caused apical–basal polarity defects in endothelial cells throughout new vessel outgrowth (Zovein et al., 2010). Moreover, endothelial cell-specific genetic deletion of β1-integrin in mice provides strong evidence for a regulatory mechanism of β1-integrin expression in the stabilization of VE-cadherin at cell–cell junctions (Yamamoto et al., 2015). Overall, these findings imply that the expression of β1-integrin in endothelial cells is decisive for proper vascular development and the stability of blood vessels.
Even though the abovementioned examples only deal with monovalent molecular interactions, there are multivalent interactions in several receptor–ligand systems. P-selectins and E-selectins, for example, can form dimeric links with the dimeric P-selectin-GP ligand 1 (Ramachandran et al., 2001; Zhang et al., 2013). Fibrinogen and VWF are each able to attach multiple replicas of their corresponding receptors to the identical or distinct platelets (Rooney et al., 1998; Zhou Y.-F. et al., 2012). The assembly and disassembly of these intricate linkages involve multi-step kinetic mechanisms that include intermediary events with additional speed variables. In addition, the force loading is probably non-uniformly spread over multiple bonds, which complicates the force sensitivity to bond lifetime (Friddle et al., 2012). Nevertheless, it is probable that a multimeric ligand has a higher probability to reach the cell receptors for elevated mechanopresentation. The total lifetime of attachment is likely also extended by the fact that disconnected elements of the receptor–ligand cluster are maintained in close distance by other members and stay assigned so that they can rapidly reconnect, leading to enhanced mechanoreception. Although cross-linking of receptors by multimeric ligands promotes cell signaling in multiple settings, multivalence may eventually guarantee enhanced mechanosensing. Although the formation of clusters results in a higher local accumulation of signaling molecules to amplify the signaling response, the generation of multiple tethers requires a reduction in the force distributed across the individual linkages, which can attenuate the signaling effect of the individual receptors. When the mechanosensors switch to a threshold mechanism that demands a specific force amplitude at individual receptors, then excessive force scattering may disable the transmission of signals. Moreover, although several proteins lack a dimeric/multimeric architecture, they include binary or plural binding sites that can independently or cooperatively engage. For instance, fibronectin incorporates a synergistic site in its III9 domain that enhances the engagement of the RGD sequence of the III10 domain with integrin α5β1 (Friedland et al., 2009; Kong et al., 2009). Thy-1 is able to concomitantly engage the integrin α5β1 and syndecan-4 in a force-triggered cooperative fashion (Fiore et al., 2014). Consequently, mechanoreception is amplified in these two events.
β1-integrins can sense the direction of the vascular flow (Xanthis et al., 2019; Su et al., 2024). Thereby, the activation of β1-integrins seems to be critical and mandatory for endothelial cell-alignment at locations of the unidirectional flow (Vion et al., 2021). In addition, reduced tension across intercellular VE-cadherin junctions and, thus, impaired lateral forces between adjacent endothelial cells have been demonstrated to prevent endothelial cells from collectively polarizing, such as in processes of vascular wounding, following physical damage. At sites of bidirectional flux, β1-integrins fail to be activated, which is a pivotal mechanism for unraveling the mechanics of blood flow to foster vascular homeostasis. A unidirectional shear stress leads to orientation of the endothelial cells with a simultaneous rest phase, while bidirectional and other irregular shear stress patterns fail to encourage orientation (Sorescu et al., 2004; Wang et al., 2006; Wang et al., 2013; Wu et al., 2011; Feaver et al., 2013; Ajani et al., 2015). The shear stress-mediated activation of α5β1 integrins induces Ca2+-dependent signal transduction processes (Matthews et al., 2006; Loufrani et al., 2008; Thodeti et al., 2009; Buschmann et al., 2010; Yang et al., 2011, p. 190), which then guides the migration and invasion of endothelial cells (Urbich et al., 2002) and endothelial inflammatory events (Bhullar et al., 1998; Dekker et al., 2005; Luu et al., 2013; Chen et al., 2015; Sun X. et al., 2016; Yun et al., 2016; Budatha et al., 2018). A model for the integrin involvement in shear stress signal transduction assumes that the tension produced at the apical surface is transferred through the cytoskeleton to the integrins positioned at the basal surface, causing structural alterations that raise their binding capabilities for ECM ligands (Bhullar et al., 1998; Tzima et al., 2001; Orr et al., 2005; Orr et al., 2006; Puklin-Faucher and Sheetz, 2009). Experiments with a chimeric form of the α5 integrin, replacing the cytoplasmic region with that of the α2 integrin, have revealed that the flow fuels ECM-driven signal transduction through the basally situated α5 integrin to facilitate the inflammatory activation of endothelial cells (Yun et al., 2016; Budatha et al., 2018). Nevertheless, further investigations revealed that integrins expressed on the apical face of endothelial cells can also react to mechanical force (Conforti et al., 1992; Matthews et al., 2006), which has been refined thereafter (Xanthis et al., 2019). The following indications lead to the deduction that β1-integrins are force direction-sensitive elements. First, β1-integrin transforms from a deflected inactive shape to an elongated active mode as a reaction to a unidirectional shear force, as contrasted to a bidirectional shear force. Second, steered molecular dynamics (SMD) simulations demonstrated that a force exerted along parallel to the membrane can produce structural redistributions, resulting in elongation of the β1-integrin. Third, unidirectional shear force initiates Ca2+ signal transduction through a β1-integrin-driven pathway, while the response to bidirectional force is completely unrelated to β1-integrins. Fourth, deletion of β1-integrin inhibited the orientation of cultured endothelial cells subjected to unidirectional shear stress, whereas it failed to change the shape of cells undergoing bidirectional shear stress. Fifth, β1-integrins underwent selective activation at locations of unidirectional shear stress within the mouse aorta (Xanthis et al., 2019), and sixth, removal of β1-integrin from endothelial cells diminished the orientation of endothelial cells at locations of unidirectional shear stress across the aorta of mice but made no changes to their shape at locations of the disturbed flow (Xanthis et al., 2019).
3.3.1 Basal or apical position of integrins and their reaction to flow
A question arises whether the location of integrins, such as at the basal or apical site of endothelial cells, plays a role in the sensing and reaction to flow-induced shear stress. Another important question that arises is whether apical integrins can transduce physical signals without being tethered to an extracellular substrate. In contrast to basal integrins, which organize focal adhesions or cell–cell interactions, apical integrins are usually distributed over the entire plasma membrane, complicating their assignment to specific structures or molecular complexes. The treatment of human umbilical vein endothelial cells (HUVECs) with a type I collagen hydrogel on the apical surface causes the rearrangement of α2β1 integrins on the apical cell surface (Turner et al., 2020). Activation of α2β1 integrins has also been shown to be an essential step in fast tube assembly and angiogenesis (Turner et al., 2020). The administration of collagen coating on the apical surface of the cell has no influence on the size of the apical β1-integrin pools (Zuk and Matlin, 1996). This indicates that the apical integrins themselves do not influence polarity but that the interplay between the apical integrins and external ECM proteins favors a reorientation of the apical/basolateral polarity axis (Ojakian and Schwimmer, 1994; Zuk and Matlin, 1996).
Interrupting integrin–ligand adhesion with blocking antibodies against α5 or β1 reduces cell adhesion and enhances cell migration (Akiyama et al., 1989). In accordance with this model, integrins are present on the upper surface of migrating skin fibroblasts, as determined by FRAP, whereas in stationary cells, they are clustered into fibrillar streaks (Duband et al., 1988). In line with this finding, cells exhibit high migratory activity when the apical pools of β1-integrins on F98 cells adopt the closed conformation (Holz et al., 2000). Conversely, exposure of cells to ligands that both cluster and activate apical β1-subunits decreases focal adhesion and cellular stretch, thereby impeding migration (Mang et al., 2020). Consequently, these investigations imply that the interaction between apical integrins and specific ligands acts as a molecular on–off switch that regulates cell motility.
At first glance, it seems reasonable that the functionality of α5β1-heterodimers differs according to their position on the basal or apical site. For example, basally positioned integrins are activated upon perturbed flow (Figure 4) (Sun Z. et al., 2016), while apically situated integrins are exclusively activated upon unidirectional flow. The mechanisms by which endothelial cells incorporate these diverse types of proximal cues from apical and basal compartments of β1-integrins deserve closer examination. Flow dynamics dictate that blood flow near the vessel wall approaches zero speed (no slippage), and therefore, it is uncertain how shear stress acts to activate proteins at the apical surface. Nevertheless, endothelial cells harbor structures that protrude into the vessel lumen and could be relevant for mechanosensing; among them are the primary cilium and the glycocalyx, which is a coating of glycolipids, glycoproteins, and proteoglycans present on the apical face of endothelial cells. Despite the glycocalyx frequently being lacking in cultured endothelial cells, like HUVECs (Chappell et al., 2009), the glycocalyx has been detected on the arterial endothelium where it may impart shear forces toward the apical face of endothelial cells (Bartosch et al., 2017). It has been established that β1-integrins became activated at the apical face of the aortic endothelium of mice, and it seems to be intriguing to conduct future experiments on the regulatory impact of the glycosylation of β1-integrins (Xu Z. et al., 2019) and the glycocalyx of this kind of process.
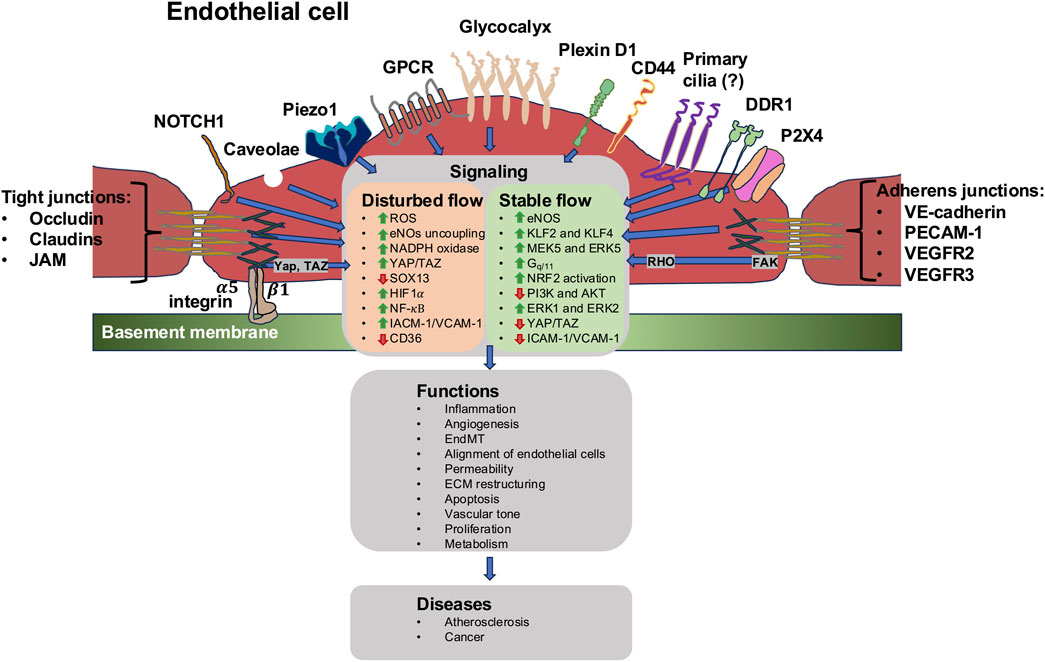
Figure 4. The apical surface of the endothelial cell possesses mechanosensory proteins, such as Plexin D1, NOTCH, Piezo1, P2X4, DDR1, CD44, and G protein-coupled receptors, such as GPR68 and mechanosensitive structures like caveolar, glycocalyx, and primary cilia. Cell–cell junctions, such as adherens junctions, compromise VE-cadherin, PECAM-1, vascular endothelial growth factor 2 (VEGFR2), and VEGFR3. At the basal site of the endothelium is the contact to the basement membrane via integrins, such as
3.3.2 Force sensors and anisotropic distribution of forces
Typically, integrins are located on basolateral and lateral cell surfaces, where they promote tethering to the ECM and intercellular adhesion via cytosolic engagement proteins that drive the arrangement of actin microfilaments. Thereby, force transmission plays a pivotal role. So far, it appears that the molecular complexes utilized to drive cell signal transduction via apical integrins are the same as those utilized by integrins in the general setting. Apical-localized integrins, nevertheless, diverge from ECM-localized integrins with respect to their ability to bind ECM proteins, a feature that may affect their physiological role. Apical integrins are not frequently organized into immobile focal adhesions and may, thus, be more quickly rearranged in reaction to different stimuli, as compared to ECM-bound integrins. Moreover, the apical integrin function is most likely affected by the local environment of the apical plasma membrane, which is clearly distinct from the basolateral plasma membrane. They also have entry to apical ligands, comprising ECM elements that are apically secreted, particularly fibronectin.
The accumulation of apical integrins, especially in activated integrins, seems to be a critical step in triggering cellular responses ranging from cell migration to enhanced permeability (Ojakian and Schwimmer, 1994; Zuk and Matlin, 1996; Walsh et al., 2015; Stewart et al., 2017; Badaoui et al., 2020; Mang et al., 2020; Turner et al., 2020). Apical integrin clusters can also promote endocytosis, which may be physiological in the event of integrin turnover in the course of cell migration. How apical integrin clustering is connected to integrin conformation and activation is poorly understood. A number of investigations have shown that integrin activation, typically by ligand binding (Ojakian and Schwimmer, 1994; Zuk and Matlin, 1996; Badaoui et al., 2020; Mang et al., 2020; Turner et al., 2020) but not strictly in clustering (Xu et al., 2016), was needed to trigger a reaction in the cells. β1-integrins tend to be linked to focal adhesion, which comprise nanoclusters with specific populations of both active and inactive integrins, indicating two independent reservoirs according to their activation state (Spiess et al., 2018). Considering the transition from freely mobile integrins to clustered, immobile integrins that occur when cells stabilize (Duband et al., 1988), it is probable that there is also a connection between clustering and the integrin inactivation state at the apical surface.
Similar to their basolaterally localized equivalents, apically localized integrins engage with the actin cytoskeleton (Tafazoli et al., 2000; Li et al., 2009; Yang and Rizzo, 2013; Uehara and Uehara, 2014; Walsh et al., 2015; Badaoui et al., 2020; Mang et al., 2020). The apical integrins may access unique actin pools that differ from actin engaged by integrins involved in focal adhesion. For example, a membrane-proximal pool of F-actin has been characterized, and if apical integrins mainly recruit to this pool of actin, this could account for the participation of apical integrins in processes like apical signal transduction, mechanosensing, and control of barrier function (Bisaria et al., 2020). Identification of specific actin patches preferentially controlled by apical integrins will contribute to the refinement of hypotheses associating them with the organization of other actin-binding proteins, like ZO-1, involved in tight junctions (Belardi et al., 2020).
To elucidate how apical integrins engage the actin cytoskeleton, it is critical to identify how scaffolding proteins can be attracted by stimulation of apical integrins. At present, there is little data on how apical integrin stimulation influences association with scaffold proteins, like kindlin, vinculin, or focal adhesion kinase, even though some indirect evidence indicates a rearrangement of talin in reaction to stimulation with apically presented nanostructured surfaces (Huang et al., 2020). In the actin cytoskeleton, the apical integrins are also associated with alterations in lipid organization of the plasma membrane, increasing ceramide accumulation and decreasing sphingosine (Grassmé et al., 2017). Similarly, data connecting caveolin, eNOS, and connexin hemichannels to apical integrin-facilitated mechanosensing (Batra et al., 2012; Yang and Rizzo, 2013; Liu J. et al., 2020) broaden the range of proteins that associate with integrin past actin and canonical integrin scaffolding proteins. A technique that uses antibodies to coat polystyrene beads mimicking the polyvalency of collagen fibrils causes the formation of tubes similar to the effect of collagen (Turner et al., 2020). This implies that, at a minimum, apical integrin stimulation on its own is capable of producing the same results.
Nevertheless, the apical β1-integrins can perceive force even without ligand engagement. Although apical β1-integrins are unattached to the ECM scaffold, it can be hypothesized that in cells subjected to flows, they function as “sea anchors,” facilitating the transmission of force toward the cell. Therefore, it was hypothesized that unidirectional shear stress triggers tension in β1-integrins that results in downstream signal transduction cascades, while bidirectional shear stress is inadequate as it reverses the flow direction prior to tension buildup (Xanthis et al., 2019). This concept is in agreement with similar findings, which have revealed that mechanical forces are capable of activating β1-integrins, regardless of whether the ligand is tethered or not (Ferraris et al., 2010; Petridou and Skourides, 2016). The direction-specific β1-integrin signal transduction event is anisotropic as it is increased in cells oriented in the force direction, whereas it is diminished in cells subjected to the flow in the reverse or tangential direction. The underlying principle of anisotropy is not determined, but it can be assumed that mechanisms are implicated that restrict rotational diffusion and keep the β1-integrin aligned. The mechanism is likely to entail a flow-driven alteration of actin dynamism or membranous fluid dynamics (Sun Z. et al., 2016), both of which are characterized to affect the alignment and activity of integrins. Because β1-integrin advances endothelial cell orientation, it can be conversely inferred that there is a forward loop present from the activation of β1-integrin to the orientation. Feed-forward schemes are essential for maintaining physiological robustness, and thus, the positive interplay between endothelial cell orientation and β1-mechanosensation is postulated to sustain long-term homeostasis at areas of unidirectional flow.
3.3.3 β1-integrin-dependent Ca2+ signaling engages with TRPV4 and Piezo1
There is a requirement to clarify whether β1-integrin-driven Ca2+ signals engage Piezo1 and TRPV4 as these Ca2+-permeable channels are recognized to perceive shear stress (Köhler et al., 2006; Thodeti et al., 2009; Mendoza et al., 2010). Therefore, HUVECs have been modified with specified siRNAs to inhibit Piezo1 or TRPV4 before applying unidirectional force via magnetic tweezers paired with 12G10 (anti-β1-integrin antibody that targets the β1-domain)-coated superparamagnetic beads (Xanthis et al., 2019). Piezo1 or TRPV4 gene silencing markedly decreased the buildup of Ca2+ in HUVECs subjected to the unidirectional flow, implying that the two channels are engaged in Ca2+ signal transduction. In agreement with this, β1-integrin-driven Ca2+ signal transduction is markedly diminished upon EGTA challenge, suggesting a need for extracellular Ca2+. In sharp distinction, the reaction to bidirectional flux upon application of EGTA was only marginally and not significantly decreased. Turning off Piezo1 or TRPV4, nonetheless, had no effect on β1-integrin signal transduction in reaction to unidirectional flow, indicating that these channels are not operating upstream of β1-integrin. It can be inferred that a unidirectional force that triggers Ca2+ signaling through a mechanism necessitates β1-integrin-driven activation of Piezo1 and TRPV4 linked to extracellular Ca2+, while in contrast, the bidirectional force is initiated by a β1-integrin-independent route. As the shear stress-induced integrin αvβ1 primarily tethers with TGF-β, fibronectin, neural cell-adhesion molecule L1, and osteopontin (Takada et al., 2007), it is likely that, thereby, the ligands contribute to the phenotypic switch of endothelial cells to mesenchymal cells. Consistent with these results, both laminar and perturbed flows can trigger activation of the identical early mechanosignaling route comprising Piezo1- and Gq/G11-based signal transduction (Albarrán-Juárez et al., 2018). According to the flow characteristics, however, the endothelial cells interpret these signaling events alternatively as atheroprotective cues, leading to the activation of eNOS or as inflammatory cues, causing the activation of NF-κB. This variable responsiveness of cells to the initial mechanotransduction event relies on α5-integrin activation, which is enabled only by the perturbed flow and not by the persistent laminar flow (Albarrán-Juárez et al., 2018).
3.3.4 Integrins engage via talin with the adherence junction protein VE-cadherin
A key feature of integrins is well known to be affinity control for extracellular ligands, a process referred to as integrin activation or “inside-out integrin signaling.” An essential terminal stage in the activation of integrins is the tethering of the N-terminal head domain of the cytoskeletal protein talin to the cytoplasmic domain of β-integrins (Calderwood et al., 1999; Tadokoro et al., 2003; Wegener et al., 2007; Ye et al., 2011). Although evidence has emerged on a lot of molecular and structural particulars of how talin engagement activates integrins (Wegener et al., 2007) and the biological relevance of talin-driven integrin activation in hematopoietic cells (Nieswandt et al., 2007; Petrich et al., 2007; Stefanini et al., 2014; Yago et al., 2015; Klann et al., 2017), the precondition for talin-driven integrin activation in committed blood vessels has not yet been addressed so far. This issue was addressed by using a mouse model with deleted talin. Embryonic lethality results from endothelial cell-specific deletion of Tln1 in mice because of deficiencies in angiogenesis, leading to widespread vascular hemorrhage and lethality up to E9.5 (Monkley et al., 2011). This finding substantiates a distinct role of talin in angiogenesis during embryonic development. Mice were studied where Tln1 was genetically removed selectively in the endothelium of mature adult mouse blood vessels utilizing an inducible conditional Cre/loxP recombination setup. These results highlight the significance of talin1 in endothelial cells for the stabilization and barrier role of the visceral microvasculature (Pulous et al., 2019). In addition, both in vivo and in vitro studies support a function for talin in VE-cadherin regulation, and talin-driven activation of β1-integrin is a pivotal hub in this signaling pathway necessary for adherence junction stability and endothelial integrity (Pulous et al., 2019). Consequently, VE-cadherin is not independently regulated of other cell surface receptors.
3.3.5 Discoidin domain receptor 1 (DDR1)
The tyrosine kinase discoidin domain receptor 1 (DDR1) is critical for normal embryonic development and organogenesis and is also associated with the advancement of several diseases, such as different types of cancer, atherosclerosis, and fibrotic illnesses (Borza and Pozzi, 2014). DDR1 exhibits mechanoresponsivity to the stiffness of the ECM scaffold (Coelho et al., 2017; Ngai et al., 2022; Wang et al., 2022c; Liu et al., 2023a). DDR1 consists of an N-terminal extracellular discoidin (DS) domain and a DS-like domain, a juxtamembrane (JM) extracellular region, a transmembrane helix, a large cytosolic JM region, and a tyrosine kinase domain at its C-terminal end (Leitinger, 2014). The binding of collagen to the DS domain of dimerized DDR1 leads to activation of the receptor by oligomerization and consequent autophosphorylation of cytoplasmic tyrosine residues (Chen and Lin, 2019). A disturbed flow enhances, while a laminar flow attenuates endothelial YAP activity with respect to dephosphorylation and nuclear translocation (Wang K.-C. et al., 2016; Wang L. et al., 2016). The activation of the YAP signal transduction path fosters endothelial inflammation and impaired vascular function, leading to atherogenesis (Wang L. et al., 2016; Wang K.-C et al., 2016). A key outstanding question in YAP signal transduction pertains to the way in which external mechanical stimuli are converted and transmitted to the YAP upstream regulator. Several mechanosensory molecules and complexes, including integrins and focal adhesion proteins, have been linked together (Elosegui-Artola et al., 2017; Nardone et al., 2017). DDR1 was shown to transduce ECM stiffness-triggered YAP activation in vascular smooth muscle cells (Ngai et al., 2022; Liu et al., 2023a). It is uncertain, nevertheless, as to whether endothelial DDR1 senses the flow to orchestrate YAP. DDR1 has been seen to act as a direct mechanosensor in endothelial cells, which governs the cellular responsiveness toward shear flow and consequently the site-specific distribution of atherosclerosis (Liu et al., 2023b). The function and mechanism of its DS-like domain in force-driven oligomerization of DDR1 have been elucidated, and it has been found that force-activated DDR1 assembles liquid-like biomolecular condensates with the 14-3-3ε protein (YWHAE) to block phosphorylation and cytoplasmic retention of YAP. Subsequently, DDR1 tyrosine kinase was identified as a direct mechanosensor and proved to be indispensable for transmitting the force exerted by shearing to endothelial reactions. The flow-driven activation of endothelial DDR1 seems to be atherogenic. The shear force probably results in conformational rearrangement of the DDR1 ectodomain, deploying its DS-like domain to uncover the hidden cysteine-287, whose uncovering promotes force-based receptor oligomerization and phase separation. Upon shear stress, DDR1 generates liquid-like biomolecular condensates and co-condenses together with YWHAE, resulting in YAP nuclear translocation. These results demonstrate a hitherto underappreciated role of DDR1 in directly sensing the flow, suggest a conceptual scheme for how endothelial DDR1 upstream governs YAP signaling, and offer a mechanism through which endothelial DDR1 activation fosters atherosclerosis. Among these mechanosensors, YAP/TAZ has been shown to promote an inflammatory and atherogenic phenotype in endothelial cells of the aortic arch (Wang K.-C. et al., 2016; Wang L. et al., 2016). Laminar flow enhances integrin α5β1–Gα13 coupling and represses YAP through decreased RhoA activity (Wang L. et al., 2016), whereas the disturbed flow enhances active nuclear YAP through the integrin α5β1 signaling path and c-Abl kinase (or ABL1) to boost downstream ICAM1 and VCAM1 expression and atherosclerosis (Li et al., 2019). The YAP/TAZ target CCN1 enhances atherosclerosis through tension-driven engagement of α6β1 integrin to activate downstream NFκB (Hsu et al., 2019). In particular, the transcription factor BACH1 has been characterized as a mechanosensor that moves into the cell nucleus when flow is disturbed, where it functions as a YAP-binding partner (Jia et al., 2022). In cases of the disturbed blood flow, the pro-inflammatory transcription program BACH1-YAP promotes the progression of atherosclerosis. In addition, it has been shown that BACH1 directly activates YAP expression by interacting with the YAP promoter. The mechanosensor JCAD has been pinpointed in genome-wide association analyses of patients with coronary heart disease (Erdmann et al., 2011), and JCAD deficiency attenuated atherosclerosis in Apoe-knockout mice (Xu S. et al., 2019; Douglas et al., 2020). In human endothelial cells, JCAD depletion has also been demonstrated to suppress YAP activity through stabilizing its interaction with the cytoskeletal modulator TRIOBP to prevent its entrance into the cell nucleus (Xu S. et al., 2019). This blocked the expression of its subsequent atherogenic target CCN1 and verified that JCAD encourages endothelial malfunction, inflammation, and atherosclerosis (Xu S. et al., 2019). Despite reduced VCAM1 and ICAM1 levels detected in the aortic arch, consistent with an atheroprotective phenotype, enhanced VCAM1 expression has been observed in the descending aorta of Jcad-knockout mice, indicating that Jcad could also have a site-specific effect in contributing to atherosclerosis (Douglas et al., 2020). Impairment of function experiments showed that DDR1 is important for shear stress-driven alignment of endothelial cells and affects the modulation of mechanoresponsive genes. In addition, direct mechanical force measurements employing magnetic tweezers to exert minimal tensile force on DDR1 expressed on endothelial cell surfaces led to an inflow of calcium. This is critical since small-scale forces, like 4 pN, do not affect the plasma membrane or some other mechanosensors (Monticelli et al., 2016). Using a FRET-based membrane-bound tension biosensor provided evidence that shear force-based DDR1 activation was, at minimum, partially tension-based in terms of DDR1 droplet formation and droplet number enhancement. This mechanism has earlier been proposed for other well-known mechanosensors like the mechanosensitive ion channel Piezo1 (Lewis and Grandl, 2015; Lai et al., 2022) and mechanosensitive GPCRs (Erdogmus et al., 2019).
3.4 Junctional proteins act as mechanosensors
There are three major types of cell–cell junctions: adherence junctions, tight junctions, and gap junctions. The adherence junctions have been identified to be highly active in mechanosensation processes, and thus, the focus is placed on them. A brief discussion on the role of tight junctions and gap junction is provided. The adherence junctional proteins PECAM-1, VE-cadherin, and VEGFR2, a tyrosine kinase receptor, have been shown to be abundantly present and required for shear stress signaling within endothelial cells (Yamada et al., 2005). Recently, another junctional protein plexin D1 has been revealed. They are all described briefly in the following.
3.4.1 PECAM-1
The adhesion molecule PECAM-1 is a member of the immunoglobulin superfamily. The extracellular domain of PECAM-1 that facilitates hemophilic engagement with adjacent endothelial cells comprises six Ig-like subunits. The intracellular and extracellular domains of PECAM-1 are linked through a short transmembrane domain. The most crucial characteristics of the intracellular domain include two immunoreceptor tyrosine-based inhibitory motif domains. These domains comprise two tyrosine residues, namely, 663 and 686, which are subject to fast phosphorylation upon the initial application of shear stress. Together with the Ca2+ inward current and the activation of the K+ channel, the phosphorylation of PECAM-1 is the first recognized reaction to shear stress. It is assumed that the phosphorylation of PECAM-1 occurs regardless of the calcium influx since Ca2+ agonists themselves cannot enhance PECAM-1 phosphorylation levels. There are abundant in vitro investigations on the involvement of PECAM-1 in shear stress signal transduction. Early supporting data for the involvement of PECAM-1 in endothelial cell force sensing revealed that phosphorylation of PECAM-1, enrollment of Shp2 and Gab1, and phosphorylation of ERK accelerate in reaction to hypoosmotic shock and fluid shear stress (Osawa et al., 2002). In addition, the phosphatase activity of Shp2 is necessary for PECAM-1-driven ERK activation. Fyn has been identified as the kinase acting as a mediator of stretch- and shear stress-induced PECAM-1 phosphorylation, utilizing an extracted endothelial cell model (Chiu et al., 2008). Subsequently, PECAM-1 has been found to be involved with VE-cadherin and VEGFR2 in facilitating multiple reactions to shear stress. These reactions comprise endothelial cell orientation, NF-kB activation, and Akt phosphorylation induced by shear stress (Fleming et al., 2005; Tzima et al., 2005). Crucially, PECAM-1 is necessary for both anti-inflammatory and inflammatory signal transduction in endothelial cells (Tzima et al., 2005). PECAM-1 is needed for polarity determination in endothelial cells, following the onset of shear stress through the spatiotemporal activation of Rac GTPase (Liu Y. et al., 2013). In this model, PECAM-1 is necessary for the activation of Rac subsequent to the phosphorylation of Src and Vav2, whereas VE-cadherin serves as a framework for the localized activation of Rac at the endothelial cell downstream edge. Knockout of PECAM-1 also results in dysregulation of eNOS localization and a consequent rise in NO generation in non-stimulated endothelial cells (McCormick et al., 2011). Moreover, in endothelial cells exhibiting PECAM-1 knockout, the activation of eNOS is reduced in reaction to shear stress (Fleming et al., 2005).
Direct force exposure to PECAM-1 through ferromagnetic beads has permitted the investigation of specific PECAM-1-based signaling cascades. Early work using magnetic beads covered with PECAM-1 antibodies has revealed that direct force exposure to PECAM-1 triggers phosphorylation of both PECAM-1 and ERK (Osawa et al., 2002). The action of PECAM-1 is necessary for these reactions, underlining the significance of confluence in investigations of endothelial cell shear stress signal transduction. It has been demonstrated that a localized force directly exerted on PECAM-1 can activate overall cellular stiffening responses in endothelial cells. Force exerted on PECAM-1 with magnetic beads affects only a small region of the endothelial cell; nevertheless, global growth of focal adhesions and activation of RhoA are monitored, following 5 min of force exposure (Collins et al., 2012). The monitored growth of focal adherence junctions and RhoA signal transduction peaks in a stiffening of the cells in reaction to the exertion of force. Importantly, cellular stiffening, associated cytoskeletal dynamics, and focal adhesion growth are ECM-specific phenomena. When endothelial cells are seeded on collagen, the force-triggered growth of focal adhesion and cytoskeletal dynamics usually witnessed in endothelial cells cultured on fibronectin are impeded (Collins et al., 2014). This indicates that PECAM-1 and integrins work together in transducing subsequent signal transduction for force-driven processes. As the pharmacological blockade of PI3 kinase prevents force-based focal adhesion generation and the activation of integrins (Collins et al., 2012), PECAM-1 possibly engages in biochemical interactions with integrins rather than direct cytoskeletal force transmission.
PECAM-1-null mice remain healthy and fertile (Duncan et al., 1999), which is unexpected considering the involvement of shear stress signal transduction in vascular plexus formation. Starting at 4 weeks of age, nonetheless, PECAM-1-knockout mice exhibit cardiac deficiencies, comprising an enlarged left ventricular diameter, diminished fractional shortening, and decreased cardiac ejection fraction (McCormick et al., 2011). Blood flow-induced dilation is inhibited in ex vivo arteries of PECAM-KO mice because eNOS is not activated (Bagi et al., 2005). Moreover, PECAM-1 KO mice undergoing regional carotid artery ligation exhibit defective flow-based vascular remodeling and intima-based thickening because of deficiencies in the NF-kB signal transduction route (Chen and Tzima, 2009). PECAM-1 KO mice are also characterized by less collateral rearrangement, following ligation of the femoral artery, which again is attributed to decreased NF-kB-dependent transcriptional activity (Chen Z. et al., 2010). The results of atherosclerosis experiments with PECAM-1-knockout mice are challenging to understand, perhaps due to variations in the atherogenic genetic background and the period of feeding. PECAM-1-knockout mice, by contrast, have been found to exhibit less atherosclerotic plaque buildup in the smaller curvature of the aortic arch (Goel et al., 2008; Harry et al., 2008; Stevens et al., 2008; Harrison et al., 2013). This region is permanently subjected to perturbed flow, indicating that silencing of PECAM-1 attenuates the inflammatory signaling of the endothelial cells caused by the perturbed flow (Figure 4).
3.4.2 VE-cadherin
When VE-cadherin-expressing cells are pooled with VE-cadherin-knockout cells and subjected to the flow, the VE-cadherin-expressing cells align toward the flow, while VE-cadherin-knockout cells fail to do so (Tzima et al., 2005). Endothelial cells align properly even in the complete lack of homophilic VE-cadherin adhesion. This indicates that VE-cadherin functions as an adapter in shear stress signal transduction. VE-cadherin knockout also prevents integrin activation, following the imposition of shear stress; however, it does not impede the activation of Src (Tzima et al., 2005). Src activation occurs immediately after phosphorylation of PECAM-1 and is among the earliest steps in shear stress signal transduction. This implies that VE-cadherin operates downstream of PECAM-1 in shear stress signal transduction, enabling VEGFR2 transactivation and subsequent signaling pathway initiation (Tzima et al., 2005; Liu et al., 2008). The transmembrane domain of VE-cadherin appears to be also necessary for the enrollment of VEGFR2 to the mechanosensory system, which further implicates VE-cadherin being an adapting molecule in this network (Coon et al., 2015). Emerging indications point to the potential for VE-cadherin to also be a mechanosensitive protein. Previous research indicates that exerting force on VE-cadherin utilizing antibody-coated magnetic beads fails to activate established force-sensitive signaling cascades; nevertheless, more recent findings indicate that this phenomenon could be epitope-specific (Tzima et al., 2005; Barry et al., 2015). Exposure of VE-cadherin to force utilizing the VE-cadherin FC antibody triggered reactions resembling those induced via force on PECAM-1, specifically cell stiffness and rearrangement of actin (Barry et al., 2015). Moreover, FRET experiments employing PECAM-1 and VE-cadherin tension sensors indicate that VE-cadherin bears a high-tension load in non-stimulated endothelial cells. This stress decreases as soon as a shear stress is imposed. Curiously, the tension on PECAM-1 is extremely low before shear stress is exerted. Following the introduction of shear, tension on PECAM-1 rises due to its engagement with vimentin (Conway et al., 2013). The primary function of VE-cadherin in shear stress signaling is probably to act as an adapting factor. The recent reports referred to earlier, however, indicate that VE-cadherin may also play additional functions in shear stress signal transduction in endothelial cells. Further investigations are required to ascertain whether the force-measuring characteristics of VE-cadherin are pertinent to physiological signal transmission.
3.4.3 VEGF receptor 2
VEGF receptors 2 and 3 (VEGFR2 and VEGFR3) are likewise implicated in the perception of shear stress. The ligand VEGF and one of its receptors VEGFR-2 are the master regulatory agents in angiogenesis (Miller and Sewell-Loftin, 2022); nevertheless, VEGFR-2 is recognized to be activated, regardless of whether its ligand is bound or not (Jin et al., 2003; Warren et al., 2014). Multiple studies have demonstrated that mechanical forces can modulate the expression and activation of VEGFR-2 (Chen et al., 1999; Jin et al., 2003; Chen T. T. et al., 2010; Tian et al., 2016; LaValley et al., 2017; Vion et al., 2021; DeCastro et al., 2022). For instance, endothelial cells subjected to shear stress comprised the creation of a VEGFR-2 and VE-cadherin-β-catenin complex, which served as a mechanotransducer and permitted the cells to activate subsequent signal transduction pathways like p38 and Akt (Shay-Salit et al., 2002). VEGFR2, which appears to be the more well-defined of the two VEGFRs, is quickly phosphorylated at the initiation of shear stress exposure (Shay-Salit et al., 2002; Tzima et al., 2005; Liu et al., 2008). Shear stress-derived activation of Akt is inhibited when tyrosines 801 and 1,175 of VEGFR2 become mutated, indicating that VEGFR2 is necessary for shear stress-based PI3-kinase activation and the consequent subsequent signal transduction process. Therefore, although there is a lack of proof that VEGFR2 is directly mechanosensitive, its activity is necessary for proper shear stress signal transduction. Two further members of the VEGF receptor family are VEGFR-1 and VEGFR-3, both of which also engage members of the VEGF family (Takahashi and Shibuya, 2005). Neuropilin-1 (NRP-1) and neuropilin-2 (NRP-2) function as co-receptors for members of the VEGF receptor family and are proven to be involved in vascular growth, in developmental angiogenesis, and tumor-driven angiogenesis (Gelfand et al., 2014; Melincovici et al., 2018; Luo et al., 2020). VEGFR-1, similar to VEGFR-2, modulates angiogenesis, through the repression of pro-angiogenic signal transduction caused by the kinetics of engagement with the VEGF ligand. The extracellular domain of VEGFR-1 exhibits a higher affinity for VEGF relative to VEGFR-2, and there is emerging support that this linkage is involved in the angiogenesis restraint; however, VEGFR-2 displays higher tyrosine kinase activity, which leads to its greater involvement in angiogenesis (Takahashi and Shibuya, 2005; Koch and Claesson-Welsh, 2012; Melincovici et al., 2018). Alternative splicing can result in a soluble VEGFR-1 (sVEGFR-1) that lacks a transmembrane or intracellular domain, and it has been demonstrated that this variant inhibits the proliferation of vascular endothelial cells (Kendall and Thomas, 1993). Conversely, VEGFR-3 is generally linked to the lymphatic system development or lymphangiogenesis, although it is implicated in controlling some other angiogenic characteristics like VEGFR-2 expression (Mäkinen et al., 2001; Veikkola et al., 2001; Heinolainen et al., 2017). In a similar fashion to VEGFR-2, VEGFR-3 can be mechanically activated under sheer stress and produces complexes with VEGFR-2, PECAM-1, and VE-cadherin at the plasma membrane (Coon et al., 2015). These supporting data also suggest that VEGFR3 is involved in the perception of distinct shear forces, which advances the understanding of how distinct shear profiles are perceived. Upon initiation of shear, VEGFR2 and VEGFR3 are attracted to the mechanosensory complex through the transmembrane domain of VE-cadherin, which is immediately succeeded by the phosphorylation of VEGFR2 and VEGFR3 (Coon et al., 2015). There is also indication that VEGFR2 and VEGFR3 respond in a redundant manner to shear stress signals. Silencing both proteins, and not VEGFR2 or VEGFR3 separately, inhibits shear-driven Akt activation (Coon et al., 2015). Intriguingly, VEGFR3 has been involved in assessing the sensitivity of various types of endothelial cells toward shear stress (Baeyens et al., 2015). For example, human dermal lymphatic endothelial cells (HDLECs) and HUVECs exhibit tip alignment at various shear intensities, when subject to a series of shear stresses. HDLECs orient themselves most efficaciously at 5 dynes/cm2, whereas HUVECs orient themselves most efficaciously at 10 dynes/cm2. Moreover, HDLECs express larger amounts of VEGFR3 compared to HUVECs. HDLECs replicate HUVECs’ phenotype after VEGFR3 silencing and align at higher shear forces relative to normal HDLECs. Collectively, these findings imply that the perception of shear stress could be more diverse in the phenotypic landscape of endothelial cells than initially envisioned. Endothelial mechanosensitivity might not just be an efficient transmission of force and biochemical communication of information through a specialized endothelial cell protein(s), whose mechanisms are the same in all endothelial cells. Mechanosensing mechanisms can differ depending on the endothelial specific niche conferred by the varying expression of mechanosensory components.
3.4.4 Tie receptors and angiopoietin 1
The endothelial TIE1 and TIE2 receptor tyrosine kinases constitute a dedicated subfamily identified through their unique extracellular domains. Tie family members of receptor tyrosine kinases have also been associated with the perception of shear stress (Chen-Konak et al., 2003; Porat et al., 2004). Tie1, which is a tyrosine kinase containing immunoglobulin-like and EGF-like domains 1, is expressed within endothelial cells, and its expression is clearly found in areas subjected to perturbed shear stress (Woo et al., 2011). Its expression is downregulated under laminar shear stress in vitro, whereas the perturbed flow enhances the activity of the Tie1 promoter. Deletion of Tie1 augmented eNOS activation and reduced inflammatory signaling in direct reaction to laminar flow in vitro. The same group detected a dose-driven decrease in atherosclerosis in Tie1-attenuated ApoE−/− mice, supporting the concept that Tie1 is a crucial controller of the endothelial reaction to perturbed shear stress (Woo et al., 2011). Although it is mechanoresponsive that it changes its expression and thereby alters downstream signal transduction, it is uncertain whether it acts as a direct sensing element for shear stress. The ANG receptors TIE1 and TIE2, which are synonymously referred to as TEK, constitute a small subfamily of growth factor receptor tyrosine kinases (Saharinen et al., 2015). TIE1 and TIE2 are expressed nearly entirely in the endothelium, but there is also evidence of expression in hematopoietic cells, like M2 monocytes (Skiba et al., 2022). Along with angiopoietin growth factors, such as ANGPT1, ANGPT2, and ANGPT4, which is also shortened as ANG, the TIE receptors constitute an endothelial-specific signal transduction pathway with key roles in the control of lymphatic and cardiovascular development and in maintaining vascular homeostasis. The growth factors angiopoietin-1 (ANG1), ANG2, and ANG4, which comprise the human ortholog of mouse ANG3, are the ligands for TIE2 (Suri et al., 1996; Maisonpierre et al., 1997; Lee et al., 2004), while TIE1 is an orphan receptor that can still be triggered by ANG proteins through its engagement with TIE2 (Marron et al., 2000). Angiopoietins are present in multimeric versions that trigger TIE receptors through distinct mechanisms. Upon an endothelial cell–cell interface, angiopoietins stimulate the generation of homomeric in trans-TIE receptor complexes spanning the cell junctions, while matrix-bound ANG1 induces TIE receptor activation in a cis configuration. Compared to vascular endothelial growth factor receptors, TIE receptors are only slightly catabolized after activation by ubiquitin, while TIE2 signaling is negatively controlled via the vascular endothelial protein tyrosine phosphatase VE-PTP. ANG1 activation of TIE2 promotes stabilization of vessels, while ANG2, a context-dependent mild TIE2 agonist/antagonist, enhances abnormal tumor angiogenesis, permeability of vessels, and inflammation. ANG2 has been shown to convey some of its vessel-destabilizing and angiogenic roles through integrin signal transduction. Circulating ANG2 concentrations are increased in cancer and in several human diseases accompanied by inflammation and vascular leakage, such as sepsis. ANG2 blockade has proven to be a promising new therapeutic option for these diseases. Moreover, preclinical findings demonstrate that genetic deletion of TIE1 in mice retards vascularization and growth of tumor isografts and prevents atherosclerosis, with minimal impact on normal vascular homeostasis of adult mice (Saharinen et al., 2015). The ANG-TIE pathway’s capacity to regulate vascular stability and angiogenesis renders it an attractive vascular objective for the therapy of several diseases (Saharinen et al., 2011; Saharinen et al., 2015; Saharinen et al., 2017).
3.4.5 Plexin D1
A novel membrane receptor, namely, plexin D1 (PLXND1), has been identified on endothelial cells that may operate as a mechanoreceptor for sensing forces (Mehta et al., 2020). More specifically, PLXND1 controls the functionality of the vessels and is responsible for the location-specific spread of atherosclerosis. Plexins are generally plasma membrane counterreceptors for semaphorins and are implicated in the axonal guidance, cancer propagation, and immune cell control (Valentini et al., 2021). In addition to its function as a counterreceptor for semaphorin 3E, PLXND1 is capable of perceiving physical forces that are translated into biochemical cues within the cell that subsequently determine the formation of atherosclerotic sites. In general, plexins are critical cell surface receptors belonging to the semaphorin family of signaling molecules and can govern the architecture of cells through modulation of the cytoskeleton and focal adhesion (Sakurai et al., 2010; Aghajanian et al., 2014). First, based on in vitro and in vivo models, it has been revealed that PLXND1 is necessary for the endothelial cell reaction to shear stress and that PLXND1-based mechanotransduction is not reliant on its ligand semaphorin 3E. Under laminar flow regimes, PLXND1 is necessary for the orientation of endothelial cells in the forward flow direction, which represents a characteristic reaction toward atheroprotective shear stress, and for the elevated expression of Klf2 and Klf4 that encode anti-inflammatory transcription factors. In disturbed flow regimes, PLXND1 is implicated in the elevated expression of pro-inflammatory genes, which include Ccl2 and VCAM-1. PLXND1 has been found to control the site-specific spread of atherosclerotic plaques. In atheroprone mice on a high-fat diet, a lack of PLXND1 in endothelial cells reduced aortic plaque load in the entire aorta and in atheroprone areas, such as the aortic arch, when compared to mice expressing wildtype levels of PLXND1 in ECs. PLXND1 lack resulted in a decreased concentration of CCL2 and VCAM1 in the inside bend of the aortic arch. Finally, PLXND1 was found to act as a direct force sensor that can trigger mechanical responses in endothelial cells, and the mechanism by which a single receptor can have a binary function was uncovered. In specific detail, PLXND1 builds a complex with neuropilin 1 and VEGFR2 in reaction to the fluid flow. This complex functions by acting upstream of the PECAM-1 junctional compound complex and integrins, which comprise two mechanosensory hubs participating in cell–cell and cell–extracellular matrix attachment, and the tri-complex can react to shear stress. PLXND1 fulfills its binary functions as a ligand receptor and force receptor, in which it alternates between two conformations: a ring-shaped state preserves its ligand-dependent features, while flexion of the ectodomain yields a more open configuration necessary for mechanotransduction.
Multiple mechanosensors have been found in the endothelial cell, and among the most well-described mechanosensors is the PECAM-1 complex used for mechanosensing. These studies, nonetheless, failed to provide structural insights into the way the receptors exert mechanosensitivity, their role in disorders, and how they interact with the other established mechanosensors.
3.4.6 Future perspective of junctional proteins as mechanosensors
The findings obtained for endothelial cells may be transferable to epithelial cells exposed to mechanical cues at interfaces. Moreover, the identification of mechanosensors on endothelial cells may help understand the interaction between the endothelium and immune cells or cancer cell transmigration. Apart from adherence junctions, tight junctions and gap junctions may act as mechanosensory proteins. Thus, elevated research effort is needed to fully reveal the function of these two other types of endothelial cell–cell junctions in mechanosensation. Endothelial mechanosensing is certainly essential for vascular functions. In this conjunction, it is important to recognize that the mechanosensory system of endothelial cells is by no means a uniform system. Instead, there exists a diverse nature of endothelial mechanosensing. The perception of hemodynamic forces involves several components: first, mechanosensors, some of which function in tandem and others that act as possible alternative or counter mechanisms; second, signaling proteins that act as regulators or amplifiers; and third, multiprotein assemblies that operate as integrated entities. The mechanotransduction mechanisms of the endothelium include fast reactions, such as acute alterations in vessel diameter, or lingering responses, involving alterations in the cytoskeleton of endothelial cells, or perhaps speculating on various physiological settings where Piezo1 is involved in vascular functioning and blood flow management. Mechanosensory elements can act together and can operate differently due to their location. Endothelial cells can even switch their phenotype upon mechanical stimulation. When examining mechanosensing and mechanotransduction, the physiology is complicated and sometimes enigmatic. The solution requires a comprehensive approach that recognizes that unrelated mechanosensors share downstream effectors and can, therefore, work together. Mechanosensors additionally transmit signals via molecules that reinforce or inhibit the activity of other mechanosensors. The concept also acknowledges the physical connection or colocalization of mechanosensors and mechanotransducers, whereby a common characteristic is the ability of various mechanosensors to trigger the exact same effector.
3.5 Membrane structures function as mechanosensors
Several protein microdomains are incorporated in the plasma membrane of endothelial cells. These microdomains endow the various areas with unique mechanical characteristics and thus lead to the heterogeneity of endothelial cells. Exposure of endothelial cells to shear forces generates a buildup of forces in plasma membrane areas with higher mechanical strength, such as focal adhesion. The latter results in the formation of regions with high strain or rigidity, which can function as mechanosensors. Other membrane structures that can be considered mechanosensors are the glycocalyx and caveolae.
3.5.1 Glycocalyx acts as a mechanosensor
Endothelial cells possess a thick pericellular coating that is abundant in carbohydrates termed the glycocalyx. The endothelial glycocalyx functions as a critical interface that regulates the interaction of cells with multiple components of the microenvironment (Purcell and Godula, 2019). The endothelial glycocalyx acts in the apical mechanosensory system. The endothelial glycocalyx consists of a gel-like coating that covers the luminal surfaces of all endothelial cells. In the endothelial glycocalyx, the carbohydrates are linked to glycoproteins and proteoglycans (PGs), which are either sequestered or attached to the plasma membrane (Sprovieri and Martino, 2018). Due to its composition of glycosaminoglycans, proteoglycans, and glycoproteins, as well as its ability to adsorb proteins of the blood plasma (Weinbaum et al., 2007), it was first assumed that the glycocalyx acts as a structural shield and has been referred to as a permeability barrier (Dull and Hahn, 2022).
Determining the structure of the glycocalyx continues to be a difficult endeavor as the physical characteristics appear to vary according to a range of parameters, which includes the species and vascular cradle, resulting in a broad spectrum of assumptions about the thickness and architecture of the glycocalyx (Fronius, 2022). Most of these reciprocal effects are conveyed through the chondroitin sulfate (CS), glycosaminoglycans (GAGs), HA, and heparan sulfate (HS). These GAGs are linked to the PGs of the glycocalyx via covalent linkages, like HS and CS, or via weak binding interactions with non-PG membrane glycoproteins, like HA. PGs, which form a subclass of glycoproteins, have different HS/CS transport properties with different amounts of covalently bound HS and CS, according to the specific PG and PG isoform. The proportion of HS to CS chains in the vascular endothelium is about 4:1, and the amount of sulfation within each chain can also differ based on the underlying cellular microenvironment. In contrary to the sulfated GAGs HS and CS, HA misses sulfation and builds linear polymer chains that meander through the other constituents of the glycocalyx and attach themselves only weakly to certain membranous adhesion glycoproteins (Gao and Lipowsky, 2010; Kolářová et al., 2014). The availability of monosaccharides and the extent of enzymatic glycan activity finally govern the make-up of the glycocalyx, which is constantly changing and contributes to the diversity in building a “homogeneous” glycocalyx structure (Becker et al., 2015). Nevertheless, it has been proven that proteins can utilize saccharide-rich structures of the glycocalyx for several purposes. These involve adhesion to tissue and detection through the immune system, the adjustment of microvascular tonicity, and permeability of the endothelium, as well as the inhibition of excessive inflammation and coagulation reactions. Moreover, these molecular remnants have a decisive function within glycoproteins as they enable proper membrane folding and arrangement (Suzuki and Fujihira, 2021). The endothelial glycocalyx plays numerous roles in the vascular network, extending from the regulation of vascular tonicity to immune response and coagulation of blood. These functions comprise, first, the regulation of the coarse permeability of the vascular wall; second, the modification of the reciprocal exchange between endothelial cells and flowing circulation cells; and third, the perception and transmission of shear forces to endothelial cells, which—in the majority of instances—favors vasodilation (Bai and Wang, 2012). The glycocalyx serves as the principal mechanosensor. Nevertheless, the sole purpose of triggering the countless cell events that arise as a reaction to mechanical forces on the membrane cannot be the mere stimulation of the glycocalyx. Therefore, the force needs to be transferred to other proteins that probably engage in a direct interaction with the glycocalyx and experience conformational shifts as a function of physical disturbances of the glycocalyx, thereby eliciting stimuli for certain cell signaling phenomena (Askari et al., 2023). The glycocalyx has been proposed to fulfill a critical mechanosensing function (Zeng et al., 2018). The glycocalyx acts as an interface between the flowing blood and endothelial cells and transforms mechanical signals into intracellular cues. The glycocalyx engages with mechanosensitive ion channels such as ENaC (Reitsma et al., 2007; Fels and Kusche-Vihrog, 2020) and participates in shear-induced signal transduction routes that implicate endothelial cell junctional proteins like PECAM-1 (Kršek et al., 2024). The glycocalyx is also an important participant in shear stress-driven proteoglycan spreading and the expression of caveolin-1 (CAV1) (Ebong et al., 2014).
The glycocalyx, a layer of sialic acid and glycosaminoglycans, containing hyaluronic acid (HA) and heparan, is attached to the apical membrane through the HA receptor (Aruffo et al., 1990) and transmits shear stress sensing through endothelial cells (Mochizuki et al., 2003; Pahakis et al., 2007). Earlier work has shown that the glycocalyx is disrupted under disturbed flow regimes, undergoes erosion in the internal aortic arch, and modulates CAV1 expression and eNOS signal transduction (Harding et al., 2018). Additionally, it has been proposed that enzymatic cleavage of heparan sulfate fosters the flow-facilitated angiopoietin-2 expression through the AMPK/FoxO1 signaling route, which does not rely on KLF2 (Richter et al., 2022). A signaling cascade was identified that connects the glycocalyx, apical CD44 expression, and the cytoskeletal network of intracellular spectrin filaments, which governs the orientation of endothelial cells in response to shear stress (Mylvaganam et al., 2022). This orientation is junction-independent as subconfluent endothelial cells orient under in vitro flow situations, a behavior that necessitates HA, glycocalyx, CD44, and the spectrin scaffold. The importance of the integrity of the spectrin reticulum for preserving the orientation of aortic endothelial cells in vivo was also confirmed in the same investigation. In addition, shear stress has been shown to impact plasma membrane tension, which consequently induced caveolae-localized Piezo1 activity and the inflow of Ca2+ ions that was needed for downstream mechanosignaling and alignment of endothelial cells (Mylvaganam et al., 2022). This result raises new questions about the glycocalyx and the HA–CD44–spectrin–mechanosensor complex. Moreover, shear-induced generation of NO is controlled by spectrin (Mylvaganam et al., 2022). In the vasculature, NO is produced by eNOS that interferes with CAV1 under basal conditions. CAV1 keeps eNOS inactive through its sequestering via caveolae. Elevations in cytoskeletal Ca2+ levels lead to the activation of calmodulin that complexes with Ca2+ and tethers with eNOS, which perturbs its inhibitory interference with CAV1 (Schlessinger, 2000). When eNOS is separated from caveolae, it can be phosphorylated and hence activated (Noordeen et al., 2006; Mihai et al., 2009; Carafoli et al., 2012; Su et al., 2021). An apical spectrin scaffold is crucial for transmitting the force exerted by shearing on endothelial mechanosensors. When CD44 is attached, spectrins regulate the cell surface density of hyaluronan and sense shear stress and convert it into alterations of the plasma membrane tension. Spectrins also stabilize apical caveolae, which are likely to contain PIEZO1 mechanosensitive channels. Consequently, the shear-facilitated PIEZO1 activation and the related calcium influx were lacking in cells deficient in spectrin. Subsequently, cell alignment and blood flow-based endothelial nitric oxide synthase stimulation were found to be equally reliant on spectrin. Thus, the apical spectrin mesh is not just required for determining the shear force but also transfers and disperses the resulting tensile forces to mechanosensors that fulfill protective and vasoactive reactions.
Additional junction-independent processes may contribute to endothelial mechanotransduction. In this context, the glycocalyx has been associated with shear-induced reactions, and HA appears to be specifically important in this regard. HA, a non-sulfated glycosaminoglycan, is attached to the cell surface by attaching to its receptors, mainly CD44 (Coelho et al., 2017). Enzymatic breakdown of HA in vitro and in vivo interferes with endothelial reactions to shear forces (Ngai et al., 2022; Wang et al., 2022c; Liu et al., 2023a). Mechanosensitive ion channels, including PIEZO1, are also involved in endothelial responsiveness toward shear stress (Leitinger, 2014). In this way, several apparently unrelated molecules work together to produce endothelial reactions to the blood flow. However, there is still no coherent mechanism that reveals the coordination of the elements.
3.5.2 Caveolae serve as mechanosensors
Caveolae are 50–100 nm small bottle-shaped indentations in the plasma membrane that are encrusted with cholesterol, caveolin, sphingolipids, and some other signaling molecules. Their creation and sustenance are based on a cholesterol-binding protein, CAV1, in non-muscle cells, and it is implicated in the control of multiple signaling pathways (Drab et al., 2001; Razani et al., 2001; Razani et al., 2002; Park et al., 2002). Caveolae are engaged in several vascular cell behaviors, and endothelial caveolae are probably implicated in mechanosensation and are, therefore, considered mechanosensory elements. Thereby, caveolae have been shown to alter their assembly state, the structural architecture of their membrane liquids, and/or activate mechanosensory receptors in their caveolae membrane. Caveolae respond to mechanical disturbances by breaking down quickly and in a reversible manner, liberating membrane regions and molecules as they flatten (Tomita et al., 2024). In reaction to tension, caveolae liberate free caveolins, the basic component of caveolae, and thus function as a cushion for the membrane tension (Yu et al., 2006; Sinha et al., 2011). Liberated caveolins interfere with other signaling molecules to trigger the initiation of mechanosensing cues.
Spectrin is required for endothelial caveolae stabilization that govern PIEZO1 regulation. Consistent with this, immunofluorescence in wildtype endothelial cells showed substantial colocalization of PIEZO1 and CAV-1, which is a key constituent of caveolae. Since caveolae are stably maintained at the plasma membrane via associations with cytoskeletal proteins (Parton and Del Pozo, 2013), the impact of spectrin on caveolae can be assessed as it may indirectly affect PIEZO1 activity. Although they were still available, significantly fewer caveolae were seen on the surface of SPTBN1-KO cells. Enhanced surface tension at quiescence in SPTBN1-KO cells could be responsible for the loss of caveolae and thus cell flattening. Shear forces lead to caveolae flattening. Collectively, these data indicate that spectrin transmits forces that are perceived by HA to elicit alterations in plasma membrane tension that cause caveolae to flatten, which then activates the tension-sensitive PIEZO1. Spectrin is a protein that is essential for the integrity of apical caveolae in endothelial cells. As an essential membrane reservoir, caveolae provide cell deformability (Sinha et al., 2011) and have been suggested to be part of the shear force sensing apparatus (Parton and Simons, 2007). It was uncertain whether shear forces lead to caveolae flattening or whether they primarily act as mechanosensitive molecular organizers. Spectrin modulates PIEZO1 sensitivity and activation in a dual manner: by affecting caveolae density and sustaining relatively low membrane tension. These findings offer a preliminary unified picture of how shear stress signaling is integrated and relayed to intracellular target effectors. Glycocalyx constituents, particularly HA, appear to be the primary sensors for fluid shear, and CD44 appears indispensable to relay the messages to the subjacent spectrin network. The latter is also associated with caveolae and determines their stability and dispersion. A shift in the spectrin mesh could slightly change the curvature or tension applied to caveolae, thereby activating PIEZO1. The subsequent calcium influx, possibly together with a mechanical deformation of the caveolae, leads to the stimulated liberation and activation of eNOS. In this scheme, spectrin plays a twofold function: on one hand, it modulates the stability of glycocalyx constituents (most directly exposed to the fluid flow) and senses and spreading them; on the other hand, it regulates plasma membrane tension through the stabilization of curved membrane microdomains like caveolae to affect the activation of mechanosensitive ion channels.
Laminar shear stress (15 dynes/cm2) elevated the expression of CAV1 at the plasma membrane, which is implicated in inflammation, adhesion, and phagocytosis. CAV1 changes ERK and Akt signaling profiles and promotes the enrichment and activation of eNOS inside the caveolae (Rizzo et al., 1998b; Rizzo et al., 1998a). Caveolae are able to engage with focal adhesion molecules, and this interplay is implicated in flow-induced reactions (Sonveaux et al., 2004; Radel and Rizzo, 2005). In addition, caveolae link mechanical stress to the activation of integrins and thus control the early stages of the mechanosensing reaction (Lolo et al., 2022). These effects underpin the participation of caveolae in endothelial cells in mechanosensitivity. Caveolae are key regulators of some other proteins and signaling molecules, including those implicated in endothelial cell Ca2+ signal transduction. Candidate mechanosensors like GPCRs and TRPV4 are two examples, both of which are implicated in endothelium-based vasodilation triggered through shear stress (Frank et al., 2003; Saliez et al., 2008; Rath et al., 2012). Caveolae are engaged in flow-driven ATP liberation of endothelial cells, which, in turn, activates purinergic signal transduction to augment Ca2+ signals (Yamamoto et al., 2011). Whether the endothelial Piezo1 channel is controlled through CAV1 and whether Piezo1 is primarily distributed in the caveolae of endothelial cells are still uncertain. In summary, caveolae are implicated in facilitating endothelial mechanosensation and various mechanotransduction events. Thus, CAV1 modulates endothelial NO synthesis, the microvascular permeability, and remodeling (Murata et al., 2007). In addition to caveolins, several accessory proteins have been recognized as fundamental components for the development and functioning of caveolae. Cavin-1, for example, which is synonymously referred to as polymerase transcript release factor (PTRF), and cavin-2, known synonymously as serum deprivation protein response (SDPR), are both necessary for the generation and operation of caveolae (Murata et al., 2007). CAV1 and Cavin-1 are major constituents of caveolae, both of which interfere with caveolae and affect their assembly and stabilization. CAV1 is linked with pulmonary arterial hypertension (PAH). The bone morphogenetic protein (BMP) receptor type 2 (BMPR2) is found in the caveolae linked with CAV1 and is frequently mutated in PAH. BMP/Smad signal transduction has been observed to be reduced in pulmonary microvascular endothelial cells isolated from CAV1-knockout mice. In addition, hypoxia increases the CAV1/Cavin-1 engagement while it weakens the CAV1/BMPR2 engagement and BMPR2 membrane targeting within pulmonary artery endothelial cells (PAECs). Cavin-1 and BMPR2 are both connected to the scaffold domain of CAV1. Cavin-1 reduces membrane localization of BMPR2 through blocking the interplay of BMPR2 with CAV1 and decreases Smad signal transduction within PAECs. Moreover, knockdown of cavin-1 renders it resilient to CAV1-driven pulmonary hypertension in vivo. The interaction between cavin-1 and CAV1 has been found to mitigate BMP/Smad signal transduction and represent a very encouraging therapeutic target for PAH (Tomita et al., 2024).
It is possible that caveolae in endothelial cells operate as mechanosensors of shear stress to trigger a set of events that enhance NO generation and vasodilation, but instead, they trigger a structural type of reaction, following stretch. Since flattening occurs as an intrinsic feature of caveolae, it has been hypothesized that this is a rapid cell survival strategy that provides a broader endothelial cell surface area (Parton and Del Pozo, 2013; Parton et al., 2020). This is consistent with other studies that emphasize caveolae as a membrane resource (Dulhunty and Franzini-Armstrong, 1975; Sinha et al., 2011). Flattening activity has been demonstrated to degrade caveolar scaffold proteins and liberate them into the plasma membrane (caveolin) and cytosol (cavin) (Sinha et al., 2011). Beyond conformational modifications, such degradation has no apparent effect. It remains to be seen whether this results in any compensatory responses that encourage the development of caveolae because of their fewer abundance. Based on the breakdown of the caveolae, it was hypothesized that the subunits of the protein can carry out independent functions, such as interactions with other proteins (McMahon et al., 2019). Underpinning evidence for this is a study proving that dissociated cavin-1 can tie to a transcription factor famously referred to as a type I collagen promoter-binding factor (BFCOL1), which, in turn, increases type I collagen, which is a key driver of depositing of the ECM (Hasegawa et al., 2000). CAV1 is able to engage with different types of intracellular signaling molecules accumulated in caveolae, including G-protein-coupled receptors, tyrosine kinases, eNOS, and several members of the MAPK signaling route (Li et al., 1995; García-Cardeña et al., 1996; Huang et al., 1997; Engelman et al., 1998; Dudãu et al., 2020; Zhao et al., 2022). There is an agreement that these molecules act as key effectors in shear stress-based induction of the endothelial cell’s activity. In line with this, exposing cells to chronic shear stress has revealed enhanced tyrosine phosphorylation and activation of surface proteins associated with shear-responsive signaling cascades, such as Akt, eNOS, and ERK (Sun et al., 2002; Boyd et al., 2003; Rizzo et al., 2003; Sun et al., 2003).
Although three isoforms have been recognized in the vascular smooth muscle, CAV1 is the sole compound necessary for caveolae generation, and its removal decreased contractile responsiveness (Gosens et al., 2011). The absence of this isoform results in an inhibition of endothelium-based relaxation, contractility, and myogenic tone (Brozovich et al., 2016). CAV1 and caveolae functionally amplify signaling pathways by incorporating important molecular elements like ion channels, adapter proteins, and receptors into membrane microdomains (Fridolfsson et al., 2014). In addition, CAV1 can control the activity of several ion channels and enzymes. For instance, swelling-activated Cl− flows are inhibited in cell strains deficient in CAV1, whereas the transient expression of CAV1 can restore the phenotype. Actin filaments are reported to bind to and support stabilization of caveolae since depolymerization of the cytoskeleton results in the migration of CAV1 across the cell (Fridolfsson et al., 2014). This connection establishes a highly efficacious mechanosensitive region, which is a localized zone where mechanical signals can be gated, perceived, and handled. This mechanism has been hypothesized for stretch-activated Ca2+ channels because they are located in caveolae, and their activity is closely governed through the actin cytoskeleton (Fridolfsson et al., 2014; Davis et al., 2023). CAV1 has been reported to engage directly with Kir2 channels, thereby reducing the current density without affecting the characteristics of individual channels or the expression of membrane proteins. In this respect, it has been revealed that CAV1 serves as a negative controller of Kir channel activity and that CAV1 and cholesterol function to stabilize the channel in a closed, quiescent condition through a common modulatory mechanism (Han et al., 2014), albeit CAV1 is not necessary to impart cholesterol sensitivity on the channel. Using crystallography, two presumptive CAV1-binding domains have been observed, whereby the first lies at the interface between the outer transmembrane helix and the N-terminus and the second is located in the outer transmembrane helix adjacent to the channels’ extracellular domain. In terms of cerebral circulation, it has been revealed that there is a tight connection (<40 nm) of Kir2 channel subunits and CAV1 located within caveolae formations in the membrane of cerebral vascular smooth muscle cells. Through this tandem arrangement, the mechanosensitivity of the Kir2 channel is potentially increased (Sancho et al., 2022). Importantly, simulated pressure utilizing a hypoosmotic stimulus challenge has been demonstrated to inhibit cerebral arterial smooth muscle cell Kir2 flows in whole-cell recordings, which was fully restored by pre-incubation with actin-disrupting agents, such as latrunculin A and/or cytochalasin D. Similarly, disconnection of caveolae-forming proteins hindered the inhibition of Kir2 smooth muscle flows during cell swelling. In addition, immunofluorescence assays revealed the expression of both scaffold proteins, syntrophin and CAV1, in vascular smooth muscle, and a proximity ligation assay emphasized the tight structural connection with Kir2.2 subunits, which was seen within 40 nm of one another, pointing to their participation in mechanosensing of the channel. These results offered convincing support that the mechanosensitivity of Kir2 channels entails distinct interactions with the cytoskeleton that are probably regulated by scaffold proteins.
Knockdown of CAV1 using siRNA (Wang et al., 2009) or caveolae abrogation by methyl-β-cyclodextrin (MβCD) abolished shear stress-driven ATP liberation and Ca2+ signal transduction (Albrakati, 2022). Laurdan imaging revealed that caveolar membrane domains react to laminar shear stress (15 dyn/cm2) through fast shifting their lipid-ordered configuration from the liquid-ordered state toward the liquid-disturbed state. Moreover, the addition of cholesterol to the endothelial cells inhibits the lipid-ordering reaction of the caveolae and significantly represses shear stress-driven ATP liberation (Parasassi et al., 1997). These results imply that the transition in the caveolar lipid order is engaged in mechanotransduction through shear stress, resulting in ATP liberation. The caveolae are likewise implicated in cellular reactions to stretching. A sustained 20% stretch induced activation of the small Rho family GTPases, such as RhoA and Rac1, in rat cardiomyocytes and then disengaged them from the caveolae. Caveolar disconnection with MβCD prevented stretch-induced activation of RhoA and Rac1 (Sinha et al., 2011). Subjecting mouse mesangial cells to cyclic 1 Hz loading for 10 min induced transactivation of the epidermal growth factor receptor (EGFR) and activation of protein kinase B (Akt). Caveolae disconnection with MβCD or filipin inhibited stretch-induced Akt activation, and both EGFR and Akt activation from stretch were abolished in mice with CAV1 knockout, implying that healthy caveolae are necessary for stretch signals to emerge (Luo et al., 2021).
3.5.3 Primary cilia function as mechanosensors
The contributing function of cilia as endothelial sensors of fluid shear stress is poorly understood, although there is considerable evidence that cilia serve as primary sensors of the flow (Nauli et al., 2008; AbouAlaiwi et al., 2009). The presence of primary cilia on mammalian is still to be confirmed and under debate. Primary cilia appear to be deficient in calcification of mammalian endothelial cells (Sánchez-Duffhues et al., 2015). Shear stress distorts the cilia and initiates downstream calcium signaling necessary for endothelial responsiveness toward the flow (Goetz et al., 2014). In addition, endothelial cells have cilia with a specialized architecture that enables a flexible responsiveness to the flow (Goetz et al., 2014). In the mammalian aorta, primary cilia exist in areas of low disturbed flow (Van der Heiden et al., 2008) and control atherosclerosis (Dinsmore and Reiter, 2016). Recent studies also point to the fact that the deciliation of endothelial cells in high-flow settings could even be a possible biomarker for endothelial injury (Gupta et al., 2022).
Primary cilia consist of 3–5-μm-long cellular protuberances that are organized by microtubule bundles. In the embryonic phase, there is indication that cilia are needed in the evolving vessels, where the flow conditions are low. In a developing zebrafish, disruption of the intraflagellar transport protein Ift81 leads to cranial hemorrhages, whereas silencing of Pkd2, which is specific for endothelial cells, leads to hemorrhages in the skull and back of the developing mice (Sen-Banerjee et al., 2005; Parmar et al., 2006; Hamik et al., 2007; Garcia-Gonzalez et al., 2010; Kallakuri et al., 2015). Primary cilia of endothelial cells are mainly located in parts of the vascular network where the blood flow is low or disturbed. Their existence has been detected in embryonic endocardial and venous endothelial cells of the chicken (Van der Heiden et al., 2006). Cilia are present in the embryonic aorta of mice and in the inner curvature within the aorta in adult mice; both regions of the vascular system are characterized by a low flow (Nauli et al., 2008; Van der Heiden et al., 2008). Moreover, it has been found that zebrafish arteries and veins display cilia (Goetz et al., 2014). Cilia have been identified in primary chicken endothelial cells (Hierck et al., 2008), embryonic mouse aortic endothelial cells (Nauli et al., 2008), and HUVECs (Iomini et al., 2004). Laminar shear forces lead to fast breakdown of cilia in endothelial cells, so the potential role of cilia in prolonged shear stress signal transduction is still uncertain (Iomini et al., 2004).
Several research projects dealing with the reaction of cilia to shear forces indicate that cilia are involved in early calcium signal transduction and NO synthesis. Primary cilia cooperate with polycystin 1 and 2 (PKD1 and PKD2), which are expressed by the Pkd1 and Pkd2 genes, to transduce shear-induced calcium and NO signaling. PKD1, which represents a GPCR, activates Gi/o proteins in a constitutive manner. Physical linkages with PKD2, which belongs to the Ca2+ channels, impair this constitutive activation (Delmas et al., 2002). Microfluorimetry has been employed to investigate early shear-dependent calcium inward flow and NO generation in vitro in aortic endothelial cells of Pkd1−/− mice. High levels of calcium influx and NO generation have been detected in wildtype cells 20 seconds after shear stress application (Nauli et al., 2008). Nevertheless, early calcium inward flow and NO synthesis have been obstructed in endothelial cells of Pkd1−/− mice. Moreover, Pkd2 is necessary for the shear-triggered calcium inward flow and NO generation. The silencing of Pkd2 within endothelial cells suppresses these initial processes (AbouAlaiwi et al., 2009). Most interestingly, endothelial cells derived from a patient with autosomal dominant polycystic kidney disease exhibit heterogeneous expression of PKD2. In these patients, PKD2-null endothelial cells reveal abnormal eNOS positioning upon shear stress exposure (AbouAlaiwi et al., 2009). In vivo investigations on primary cilia add to the earlier in vitro investigations on cilia. A morpholino against Pkd2 inhibits the calcium inward flow into the arterial endothelium of zebrafish embryos (Goetz et al., 2014). There are also Tnnt2 and gata1 morpholinos utilized, which inhibit the heartbeat and decrease the hematocrit, respectively. Tnnt2 morphants show no deflection of the cilia, whereas the cilia in gata1 morphants are only mildly deflected. It is significant that the calcium entry into the endothelial cells relates to the level of deflection of the cilia as tnnt2 morphants exhibit a marked decrease in calcium entry into the endothelial cells, whereas gata1 morphants have a moderate decrease (Nauli et al., 2008; Goetz et al., 2014). Notwithstanding the reported evidence of cilia participation in Ca2+ and NO signal transduction, the role of primary cilia functioning as shear sensors is not yet clearly elucidated. Cilia are located in areas of the vasculature with low blood flow (Tzima et al., 2001; Li et al., 2005; Liu et al., 2008; Van der Heiden et al., 2008) and tend to be missing in high-flow zones. This raises the fascinating prospect that cilia generation is itself a flow-dependent event as cilia generate when blood flow is low or perturbed. Although this does not rule out cilia acting as mechanosensors, their function as reinforcers of shear signatures (Lan et al., 1994; Hierck et al., 2008; Cuhlmann et al., 2011) is surely coherent with their existence in regions of low blood flow. Cilia can function as a sensor of low flow in the endothelium, but the disentanglement of cilia roles in development and the overall shear signaling of endothelial cells continue to be a major effort.
3.5.4 Cytoskeleton is engaged in endothelial mechanoresponse
It has been postulated that the cytoskeleton serves as an integrative mechanism to transmit forces from mechanosensory receptors to mechanosensitive molecular entities in cells (Davies, 1995). Most studies have concentrated on the actin cytoskeleton due to its fundamental involvement in controlling the activity of integrins and junctional protein complexes, which are frequently implicated in mechanotransduction (Hahn and Schwartz, 2009). Actin filaments, in contrast, cannot sustain large forces and are usually highly dynamic (Palmer et al., 1999). This indicates that actin-rich structures on their own are not well-adapted to serve as a framework for mechanosensitive cells. In addition, the hypothesis was put forward that the spectrin cytoskeleton, which includes only short, relatively stable actin filaments, is a basic integrator of mechanical signal transduction on the apical surface of the endothelium. Spectrins, found abundantly and highly conserved in the tissues of multicellular organisms, are involved in stabilizing cell membranes and regulating the activity of ion channels (Bennett and Healy, 2009). Albeit the underlying mechanism is still unclear, spectrins appear to be especially critical for vascular function. Spectrins are highly flexible and bind with short actin filaments, making them very stable. The ensuing spectrin–actin lattice is particularly flexible and durable (Lambert and Bennett, 1993). The flexibility imparts enhanced conformance and deformability to the membrane and is optimal for cells subjected to the dynamic vascular environment. In fact, the spectrin cytoskeleton is needed to preserve the integrity of the erythrocytes when they are put under hemodynamic stresses (Sheetz and Singer, 1977; Sheetz et al., 1980) and have to pass through narrow constrictions (Lux et al., 1976; Agre et al., 1982; Chasis et al., 1988). Endothelial cells are likewise exposed to hemodynamic stress, notably in the aorta, and in constricted capillaries, where the endothelium is distorted by streaming erythrocytes. Ultimately, it has been found that shear stress causes endothelial spectrin to reshape, a characteristic that is likely to be pivotal in vascular homeostasis (Mylvaganam et al., 2022).
4 Mechanical interplay between endothelial cells and vascular smooth muscle cells
Not only mechanosensory cells can interact but also different cell types such as endothelial cells and VSMCs can cooperate in mechanosensation, mechanotransduction, and vascular function. Resistance arteries make up small blood vessels that are less than 400 μm in diameter and, therefore, account for peripheral vascular resistance. The vessel wall of these blood vessels responds structurally and intrinsically (locally) to the constantly arising dynamic forces in such a way as to finally control the vascular tone and adjust the blood flow to the metabolic needs of the tissue. This capability is accomplished through the plasma membranes of two of the major parts of the vascular wall, such as smooth muscle and the endothelium, which are permanently subjected to mechanical irritations caused mainly by the pulsatile blood flow, including stretch (tensile stress) and/or shear stress (Lehoux and Tedgui, 1998). In this regard, certain mechanosensitive molecules located in the plasma membrane, such as ion channels, caveolae, and/or surface receptors, can change their conformational status and electrical/chemical characteristics in reaction to mechanical perturbations, transforming them into a physiological reaction (Ingber, 2006; Martinac, 2014). The endothelium is not a closed system in mechanical terms; it can interact with other nearby cells, such as VSMCs (Figure 5). Once the mechanical forces have been perceived from the endothelial cells, they are codified and passed on to the VSMCs, which react by either relaxing or contracting. A tight functional engagement between the endothelial cells and the neighboring VSMCs is necessary for the control of vascular tonicity and the capacity of the cells to respond to different biochemical and mechanical impulses received from the flowing blood. In recent years, it has been found that the mechanical properties of endothelial cells rely on flow-induced forces, on one hand, and govern the contractile nature of VSMCs, on the other. At the heart of this well-known process is the capacity of endothelial cells to liberate NO in accordance with shear stress, causing it to diffuse toward neighboring VSMCs, where it induces vasodilation through cGMP-dependent routes (Bolotina et al., 1994). A decrease in NO is closely related to elevated amounts of reactive oxygen species (ROS) produced by NAD(P)H oxidase, xanthine oxidase, or uncoupled endothelial nitric oxide synthase (eNOS) in the vessel wall, resulting not solely in a depletion of NO but also in an interruption of several signaling cascades that convey NO generation (Lubos et al., 2008). Therefore, the close communication between endothelial cells and VSMCs regulates vascular performance and vascular tonicity. An important physiological feature of the endothelium is mainly the capacity of endothelial cells to modify their mechanical characteristics, that is, to switch between “stiff” and “soft” states. Endothelial cells that have lost this capacity and are stuck in chronic stiffening can be considered dysfunctional (Marti et al., 2012). Moreover, the mechanoresponse involves the interaction of endothelial cells with VSMCs, stromal cells, and immune cells in the local vessel walls via microRNA-driven and extracellular vesicle-driven mechanisms (Tan et al., 2020; Li et al., 2022). Endothelial cells and VSMCs constitute the key adjacent wall cells that build barriers. They can exchange information through direct proximity, the transfer of signaling agents, and the accumulation of ECM. Their interaction is a key mechanism in the control of vascular tonicity. Endothelium-derived factors (PDGF-B, CXCL12, ET-1, and MIF) have been found to drive VSMC proliferation, thereby promoting vascular restructuring and pulmonary arterial hypertension (Dai et al., 2018). Consequently, the endothelial-specific alk5 overexpression in alk5−/− has been hypothesized to reestablish VSMC proliferation in the wall of the coronary effluent tract (Boezio et al., 2020). Finally, the emphasis is the role of endothelial cells in the process of mechanosensing and mechanotransduction, and therefore, in the following, the three main types of endothelial mechanosensors are presented and discussed.
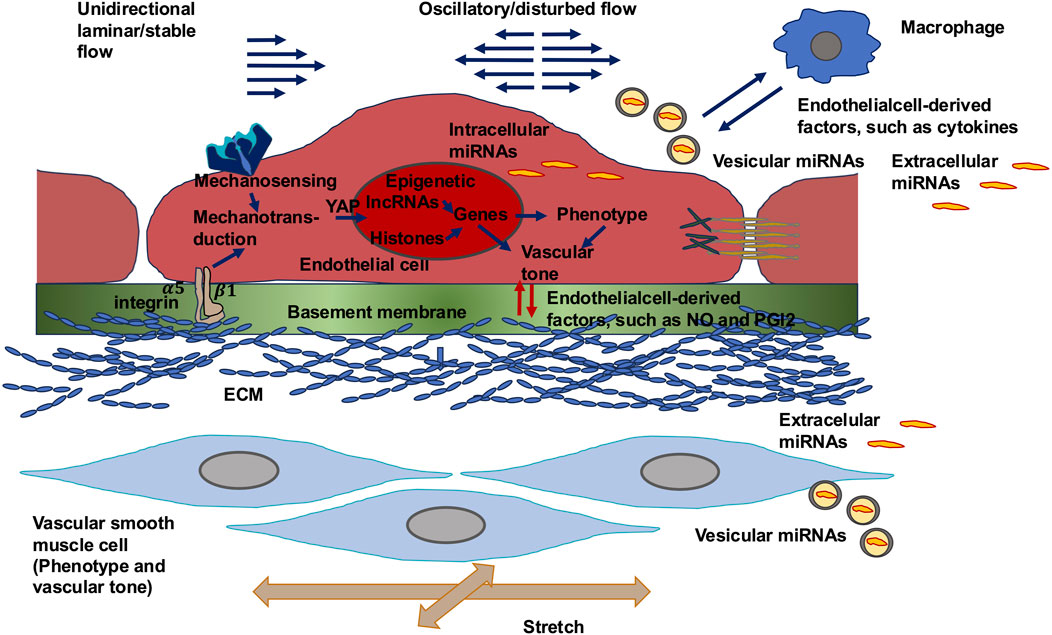
Figure 5. Vascular interference is regulated by shear flow. Shear stress can alter the endothelial cell function via endothelial mechanosensing and mechanotransduction events to govern the epigenome, transcriptome, phenotype, and interplay between endothelial cells and VSMCs and other adjacent cells, such as macrophages. The regulation of this interferences results in homeostasis under normal conditions, and in pathological conditions, it leads to diseases. lncRNAs, long non-coding RNAs.
4.1 Epigenomic mechanoregulation of vascular smooth muscle cells
Epigenetic signatures characterize the non-genetic alterations to the genome through chemical changes to DNA and its associated proteins like DNA methylation and histone modifications (Rivera and Ren, 2013). These heritable alterations in expression are regulated through various chemical changes of bases in DNA, involving DNA methylation and histone modification, and non-coding RNA (ncRNA) regulatory mechanisms. In contrast to the majority of cell types, nevertheless, VSMCs are non-terminally differentiated and, therefore, exhibit phenotypic plasticity (Babaev et al., 1990). This plasticity varies widely from contractile-resting to migratory-proliferative-synthetic to osteogenic or macrophage-like cells (Jaminon et al., 2019). Endothelial cells may possibly alter the phenotype of VSMCs by changing their epigenetic signatures upon mechanical sensing and signaling, More generally, several genes that determine the VSMC phenotype have been identified to be governed through DNA methylation, involving SRF, platelet-derived growth factor B (PDGF-B) of the GATA-6-PDGF-B pathway in endothelial cells, and TAGLN (De Oca et al., 2010). Thereby, TET2 can serve as a key epigenetic modulator of the phenotype of VSMCs (Liu R. et al., 2013). In addition, knockdown of TET2 attenuates the expression of crucial VSMC genes like MYOCD and SRF with concomitant transcriptional upregulation of KLF4, which enhances pluripotency network reactivation and consequently phenotype transition. Conversely, overexpression of TET2 leads to a contractile VSMC phenotype, reestablishes the epigenetic 5-hmC map, and substantially decreases intimal hyperplasia in vivo (Liu R. et al., 2013). Post-transcriptional histone modifications comprise, among others, acetylation, methylation, and ubiquitinylation, which are primarily occurring at arginine (R) and lysine (K) residues (Lawrence et al., 2016). Usually, these changes can take place in combined form, like, for example, dimethylation (me2) or trimethylation (me3) at histone H3K4 in combination with H3K9ac or H3K14ac, all of which activate gene expression (Kimura, 2013). Acetylation by histone acetyltransferases (HATs) generally attenuates the DNA–histone interactivity, thereby rendering genes more amenable to transcription. In contrast, deleting an acetyl group by histone deacetylases (HDACs) reinforces the coupling between DNA and histone, leading to suppression of gene expression (Gomez et al., 2015; Jiang et al., 2018). Several alterations in the levels of histone methylation and acetylation have been determined to have a critical impact on the progression of diseases, such as atherosclerosis, and the transition of VSMC toward the synthetic phenotype, which involves a marked reduction in H3K9 and H3K27 methylation (Greißel et al., 2015) with a simultaneous rise in H3K9 and H3K27 acetylation in progressing atherosclerotic plaques (Greißel et al., 2016). The involvement of H3K27 methylation in the VSMC transition phenotype has been confirmed by experiments, in which a decrease of H3K27me3 in tunica media cells has a key function in the differentiation and proliferation of VSMCs in endothelial dysfunction like atherosclerosis (Wierda et al., 2015). Reduced levels of H3K9me2 have been detected in VSMCs in atherosclerotic lesions and arteries experiencing lesion-related restructuring and are linked to increased transcription of inflammation-responsive genes (Harman et al., 2019). Elevated expression of the histone demethylase KDM3a in diabetic rats has been demonstrated to promote neointimal hyperplasia by diminishing H3K9 dimethylation within the ROCK2 and AGTR1 sites, suggesting that the shift from the contractile to the synthetic VSMC phenotype is amplified through activation of the Rho/ROCK and AngII/AGTR1 signal transduction routes (Chen et al., 2017). Finally, several epigenetic alterations can either enhance or impede arterial restructuring in diseases like atherosclerosis by influencing directly and indirectly the VSMC phenotype. Several target genes and molecules that regulate all forms of epigenetic modifications have been evaluated in animal models and clinical studies for their efficacy in the general therapy of atherosclerosis (Jiang et al., 2018). For instance, the DNMT inhibitor 5-aza-2′-deoxycytidine (5-aza-dC) has inhibited the depressed expression of methylated genes (Mossman et al., 2010), reduced overall 5-mC levels, and re-established the expression of myocardin expression in VSMCs triggered through PDGF, which thereby impedes excessive dedifferentiation of VSMCs (Zhuang et al., 2017).
4.2 MIRs facilitate exchange between endothelial cells and vascular smooth muscle cells
MIRs can be secreted by endothelial exosomes, which are small extracellular vesicles of a diameter of 40–150 nm that emanate from endosomal multivesicular bodies (Stoorvogel et al., 2002; Tkach and Théry, 2016). Numerous studies have revealed that exosomes are key paracrine facilitators of intercellular signaling (Loyer et al., 2014; Wu et al., 2019) and modulate the functionality of recipient cells by transmitting various functional molecules, including proteins, lipids, and nucleic acids, such as miRNA, mRNA, and lncRNA (Hergenreider et al., 2012; Deng et al., 2015; Lin et al., 2019). Endothelial cells can sequester exosomal miR-143/145 clusters that are conferred to VSMCs and regulate atherosclerotic lesion development by acting on KLF2 (Hergenreider et al., 2012). Several studies have described the transfer of microRNAs, like miR-143/145, between endothelial cells and their connected VSMCs (Hergenreider et al., 2012; Climent et al., 2015; Deng et al., 2015). It has been observed that miR-143–3p regulates exosome-based intercellular communication between pulmonary arterial endothelial cells and VSMCs in the development of pulmonary arterial hypertension (Deng et al., 2015). Nevertheless, it is uncertain as to whether and in what way exosomes also facilitate endothelial cell–VSMC intercellular communication in diseases (Lin et al., 2022). Finally, a critical role of endothelial cell-derived exosomal miR-670–3p in the regulation of arteriosclerosis has been established. Therefore, it can be hypothesized that miR-670–3p secreted by endothelial cells could be a candidate target for the treatment and prediction of arteriosclerosis and other diseases. Both endothelial dysfunction and VSMC plasticity are key factors in the pathogenesis of hypertension and stiffness of arteries. MicroRNAs can facilitate cellular signaling between vascular endothelial cells and VSMCs. The role of endothelium-derived extracellular miR-92a in promoting arterial stiffness by modulating intercellular communication of endothelial cells and VSMCs has been investigated (Wang C. et al., 2022). The serum level of miR-92a has been seen to be elevated in hypertensive patients compared to control subjects. Circulating miR-92a levels correlated positively with pulse wave velocity (PWV), systolic blood pressure (SBP), diastolic blood pressure (DBP), and serum endothelin-1 (ET-1) levels, although inversely with levels of serum NO. In vitro, elevated levels of miR-92a in endothelial cells induced by angiotensin II (Ang II) caused a phenotype shift from contractile to synthetic in cocultured VSMCs. In mice infused with Ang II, a lock nucleic acid-modified antisense miR-92a (LNA-miR-92a) ameliorated Ang II-induced PWV, SBP, and DBP and compromised vasodilation. Application of LNA-miR-92a also completely reverted the elevated levels of proliferative genes and the reduced amounts of contractile genes triggered in the mouse aorta with Ang II. The circulating serum concentration of miR-92a and vascular stiffness were positively related in these mice. These results lead to the hypothesis that endothelial cell miR-92a can be delivered to VSMCs through extracellular vesicles to control phenotypic transitions of VSMCs, which consequently results in elevated arterial stiffness. Moreover, extracellular vesicles secreted by endothelial cells contributed to the contractile phenotype of VSMCs through the miR-206/ARF6&NCX1/exosome route (Lin et al., 2016). MiR-582 secreted by endothelial cells acts on VSMCs (Fontaine et al., 2021). The proliferation of VSMCs is also affected with miR-582 since this miRNA reduces the expression of CASP3 and PTPRJ (Woo et al., 2003; Smart et al., 2012). The regulation of ITGA3 and PDCD6 by miR-582, which is implicated in cell migration, could finally be affirmed (Su et al., 2012; Kurozumi et al., 2016).
4.3 Myogenic tone (pressure-driven constriction) control of vessels via vascular smooth muscle cells
The VSMC layer of a resistance artery or of arterioles penetrating inferiorly has an intrinsic capacity, which is regardless of the endothelium and nerves, to contract and decrease luminal diameter in reaction to an abrasive rise in transmural pressure, such as radial stretch (Bayliss, 1902). Within the brain, this process, termed ‘myogenic tone,’ enhances opposition to the blood flow (Davis and Hill, 1999; Kirby et al., 2007) and is critical for setting marrow basal vascular tone, sustaining steady perfusion across a wide array of intraluminal pressures and finely adjusting local CBF simultaneously safeguarding the downstream capillary meshwork against injury (Figure 6) (Hill and Davis, 2007; Cole and Welsh, 2011). Curiously, the reaction to myogenic tone gets stronger as the vessel size reduces (Russell et al., 1970; Davis, 1993), which may be attributed to an elevation in vessel wall distensibility with the ramification of the vascular tree (Thorin-Trescases et al., 1997). In addition, the myogenic tone of resistance arteries can be affected via hemodynamic forces, such as the flow, metabolic agents, and vasoactive modulators secreted by other cell types, such as the endothelium (Bevan and Joyce, 1990; Garcia-Roldan and Bevan, 1990; Ngai and Winn, 1995). The mechanisms behind this phenomenon have been thoroughly investigated (Davis and Hill, 1999; Welsh et al., 2000; Hill et al., 2001; Osol et al., 2002; Brayden et al., 2008; Sharif-Naeini et al., 2010). An elevation in intraluminal pressure acts to modify the activity of stretch-sensitive ion channels that are expressed in VSMCs, leading to membrane depolarization and the engagement of voltage-gated calcium channels (VGCCs), resulting in elevated intracellular levels of Ca2+ ions (Knot and Nelson, 1998; Thorneloe and Nelson, 2005). Therefore, mechanosensitive ion channels in VSMCs are frequently studied because they function as pressure sensors through depolarizing the arterial membrane potential (VM) and triggering the myogenic reaction (Davis and Hill, 1999; Welsh et al., 2002). Cerebral myogenic reaction is essential for accurate VSMC functionality, and consequently, disruption of myogenic tone has been linked to multiple vascular diseases, like stroke, hypertension, and dementia (Izzard et al., 2003; Toth et al., 2013; Toth et al., 2017).
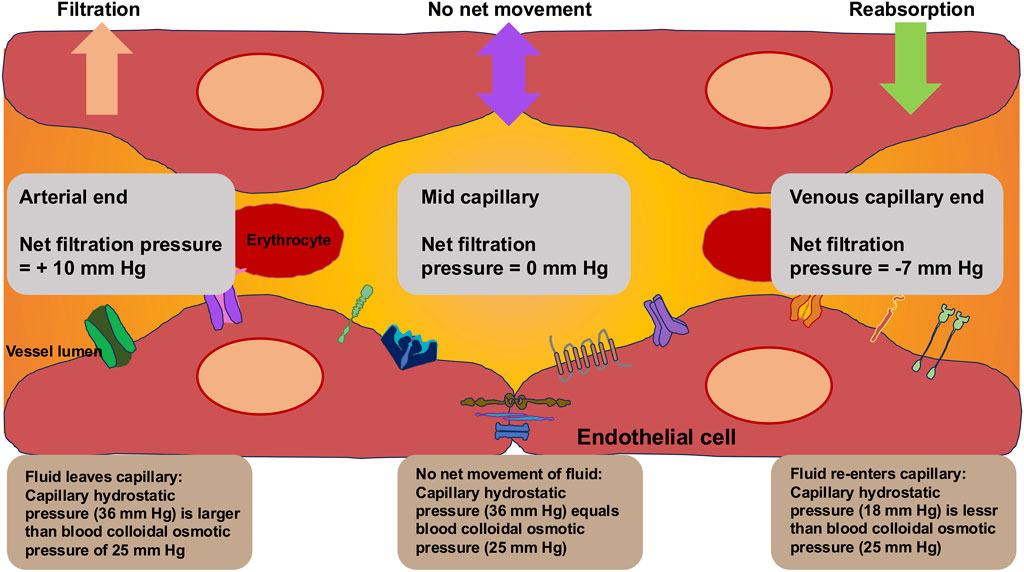
Figure 6. The net filtration takes place close to the arterial end of the capillary, when capillary hydrostatic pressure (CHP) is larger than the blood colloidal osmotic pressure (BCOP). There is no motion of fluid near the midpoint (CHP = BCOP). Net reabsorption takes place close to the venous end of the capillary (BCOP is larger than CHP).
5 Endothelial mechanosensing in pathological settings, such as cancer
The process of mechanosensing involves a force and a sensor. When one or both are changed, mechanosensing and/or mechanotransduction is compromised, causing endothelial malfunction. Disturbances also arise in ambiguous circumstances, for example, in collateral vessels and in cases of ischemia-reperfusion. At the same time, mechanosensors are susceptible to post-translational alterations and mutations that impair their performance. In the following, pathologies associated with altered mechanosensing are briefly presented with emphasis on cancer.
5.1 Flow pattern regulates the signal transduction processes in endothelial cells
Hemodynamic disturbances may cause pathologic results rather than physiologic reactions. Atherosclerosis is a prime illustration of changing forces acting on endothelial cells. Atheroprotective or atherogenic endothelial signals mark vascular areas in relation to blood flow profiles (Albarrán-Juárez et al., 2018). Blood vessels with a high laminar flow show an orientation of endothelial cells in the direction of the flow, an anti-inflammatory phenotype, reduced oxidative stress, turnover of cells, and permeability (Hahn and Schwartz, 2009). In contrast, vascular areas with a low or oscillating flow, like arterial bifurcations, are marked through an inflammatory phenotype, increased endothelial cell proliferation, apoptosis, enhanced permeability, endothelial cell misalignment, enhanced proinflammatory gene expression, and increased extracellular matrix production (Mohan et al., 1997; Brooks et al., 2004; Cheng et al., 2006; Hahn and Schwartz, 2009). This shows that various hemodynamic patterns can alter endothelial signal transduction and thus protect from or exacerbate vascular disease. Shear stress and flow patterns determine inflammatory signal cascades in endothelial cells. Impaired flow activates an inflammatory phenotype centered on elevated regulation of reactive oxygen species generation, oxidative stress, expression of cytokines, alterations in the ECM, and persistent alterations in inflammatory gene expression from hours to days, which can even be seen in atherosclerosis-resistant animal models (Thoumine et al., 1995; Orr et al., 2005; Jongstra-Bilen et al., 2006; Hahn and Schwartz, 2009). These alterations based on perturbed flow led to a permanently activated inflammatory phenotype, sustained inflammation, and reorganization of the blood vessels. In opposition, laminar flow stimulates only transient endothelial inflammation symptoms that decay fast (Hsieh et al., 1998).
5.2 Hypertension governs signal transduction processes and mechanosensors of endothelial cells
Chronic hypertension is marked with substantial perturbations of hemodynamic forces (Mayet and Hughes, 2003), to which endothelial cells are exposed and which probably affect mechanosensation. Hypertension leads to oxidative stress elevation, the formation of reactive oxygen species, and inflammation. Blood flow disorders are further risks for the development of atherosclerotic plaques (Figure 7) (Cheng C. et al., 2003; Jongstra-Bilen et al., 2006; Hahn and Schwartz, 2009). It is well known that hypertension is linked to impaired expression and/or dysfunction of mechanosensors and mechanotransducers. Reactive oxygen species produced in hypertension react with NO and remove it, thus preventing vasodilation (Harrison et al., 2006). Animal experiments have also shown that the activation of mechanosensitive channels is compromised because of disease. Whether the changed activity of a mechanosensor is a reason or a consequence of the altered forces is still uncertain. Hypertension influences the vascular channels, such as TRPV4, Kir2.1, and Piezo1 (Koide et al., 2021). Mutations in genes that encode mechanosensors, such as PIEZO1, are widespread in humans and may be cardiovascular disease risk mechanisms (Ma et al., 2018). Overall, impaired hemodynamics and dysregulated mechanosensors are closely associated with vascular impairment and disease.
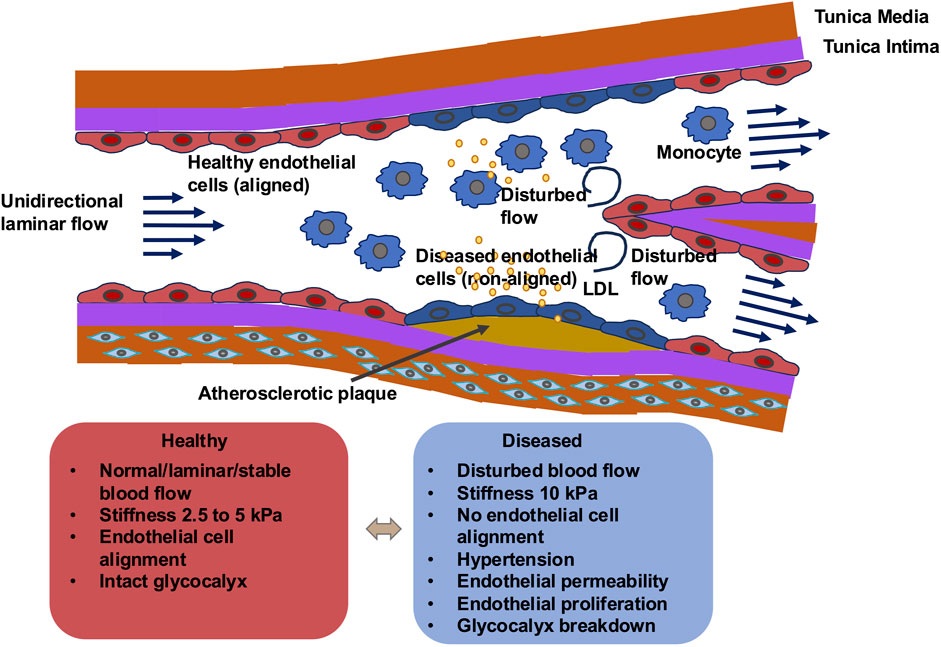
Figure 7. Development of diseases such as atherosclerosis: the behavior of endothelial cells is guided by dynamics of the vascular microenvironment. Most commonly, vessel walls are subject to uniform/laminar/stable flow that fosters the healthy state of endothelial cells. Vessel bifurcations and curvatures can lead to perturbed flow areas, which cause altered endothelial cells with development toward a diseased behavior. For instance, endothelial cells possess hyperpermeability, and they get proinflammatory. Consequently, endothelial cells enable the transmigration of low-density lipoprotein (LDL) and immune cells into the blood vessel wall, whereby atherosclerotic plaques can be built-up.
5.3 Mechanoswitching of endothelial cell phenotypes
Endothelial cells sense changes in their environment, must respond to the neighboring mechanical stimuli, and accommodate to the ongoing alterations in their microenvironment. The cellular adaptation of endothelial cells involves a broad spectrum of responses, including (de)activation, (de)differentiation, and cell proliferation/apoptosis. It is, therefore, helpful for the adaptation of endothelial cells to changes in their environment that they themselves can exhibit plastic behavior. Endothelial cells are capable of significant phenotypic plasticity (Dejana et al., 2017), exemplified by their capacity to change their endothelial phenotype to a mesenchymal phenotype. This plasticity, termed endothelial-to-mesenchymal transition (EndMT), was originally reported in cardiac embryonic development; however, it has also been emphasized in different postnatal pathologies like atherosclerosis, cardiac fibrosis, calcification of the vessels, and pulmonary hypertension (Li et al., 2018a). EndMT seems to be important in these inflammation-related diseases and could be an important connecting element between endothelial dysfunction and inflammation (Cho et al., 2018). In the case of cancer, the EndMT mechanism has been identified in melanoma and pancreatic cancer mouse models (Zeisberg et al., 2007) and is significantly implicated in cancer propagation. Due to their plasticity and their capacity to transdifferentiate into mesenchymal cells, these cells have been characterized through a process referred to as EndMT. This complex process is driven by several different factors that convert endothelial cells into a phenotype marked with mesenchymal protein expression and a mobile, contractile shape. EndMT has originally been characterized in normal heart development, but it is now also found in various pathologies and, in particular, in cancer (Clere et al., 2020). This crossover is linked to a marked reduction in endothelial markers, like VE-cadherin, PECAM-1, Tie-1, Tie-2, and vWF, whereas the expressions of mesenchymal biomarkers, like α-SMA, CD44, COL I/III, FSP-1 (S100A4), SM22a, N-cadherin, and vimentin, rise (Piera-Velazquez and Jimenez, 2019). EndMT markers develop from an early stage with regional reduction of endothelial biomarkers and elevation of some early mesenchymal biomarkers (α-SMA, SM22a, and FSP-1) to a subsequent stage marked by a reduction of endothelial biomarkers and elevation of mesenchymal biomarkers such as matrix proteins, such as fibronectin and COL I, and matrix metalloproteases (MMPs) (Dejana et al., 2017). Originally, EndMT has been viewed as a complete differentiation event; however, in the pathophysiological setting, especially in cancer, it can also be partial. Intermediate levels of differentiation of cancer-derived endothelial cells have been observed (Xiao L. et al., 2015), and these cancer endothelial cells exhibit heterogeneity in their capacity for EndMT. It is in agreement with the phenotypic heterogeneity of endothelial cells within various cancer types (Maishi et al., 2019; Goveia et al., 2020), coupled with the variety of cues emanating from the tumor microenvironment, as EndMT subtuning seems to be regulated by various drivers like TGF-β and basic fibroblast growth factor (bFGF) (Xiao and Dudley, 2017). In addition, partial EndMT has been considered a first step of endothelial sprouting in the course of angiogenesis (Welch-Reardon et al., 2015; Wang S.-H. et al., 2017). The reversibility of EndMT in cancer has hardly been characterized; however, a transition from mesenchyme to the endothelium has recently been implicated in Kaposi’s sarcoma (Li et al., 2018b). Limited in vitro research indicates that reversibility of EndMT may arise on exposure to EndMT inducers within a brief period (Rieder et al., 2011), while extended exposure compels mesenchymal differentiated cells to progress to a point where there is no reversion to endothelial characteristics (Xiao L. et al., 2015). Apart from their signal mechanotransduction function of endothelial cells, it is possible that they may undergo a mechanoswitch upon mechanical stimulation, like the epithelial-to-mesenchymal transition of epithelial cells. As expected, EndMT can be induced by mechanical cues via the Alk5-Shc mechanotransduction pathway (Egorova et al., 2011b; Egorova et al., 2011a). Vascular pathologies like atherosclerosis, which are typified by aberrant mechanical forces, are often paralleled by EndMT. Nevertheless, it is not well enough established how the forces impact the mechanotransduction pathways that govern cellular plasticity, inflammation, and, finally, pathology of the vessels. A mechanoreceptor unique to EndMT has been identified, and a molecular Alk5-Shc pathway, resulting in EndMT and atherosclerosis, has been elucidated. Depleting Alk5 abolishes shear stress-induced EndMT reactions, and targeting endothelial Shc genetically decreases EndMT and atherosclerosis in zones of impaired flow. Tensile force and readjustment studies highlight a mechanosensory role for Alk5 in EndMT signaling that appears to be unique and separate from that of other mechanosensors. Despite the multifunctional cytokine TGFβ performing a pivotal function in EndMT (Moustakas and Heldin, 2016; Ma et al., 2020), emerging evidence has indicated that the imposition of atheroporotic (or oscillatory/perturbed) shear stress on endothelial cells induces the expression of EndMT transcription factors and mesenchymal hallmarks in vitro and in vivo (Saito, 2013; Kovacic et al., 2019). In addition to its embryonic developmental involvement, EndMT has also been linked to inflammatory cardiovascular pathologies (Kovacic et al., 2019), including atherosclerosis (Islam et al., 2021). TGFβ-Alk5 signaling is critical for the reaction to shear stress (Egorova et al., 2011b), and emerging evidence has revealed that endothelial-specific deletion of both TGFβR1 (Alk5) and TGFβR2 retards the outgrowth of atherosclerotic lesions and causes complete remission of well-established plaques, demonstrating a clear cause-and-effect connection of EndMT to atherosclerosis (Frutkin et al., 2009). Finally, Alk5 has been implicated as the receptor in charge of mechano-EndMT, and Shc has been established as a candidate driver of EndMT and atherosclerosis in zones of disrupted shear stress (Liu et al., 2008; Mehta et al., 2021). There is even the possibility for endothelial cells to undergo differentiation into adipocytes via EndMT and into mural cells like pericytes and smooth muscle cells (Huang et al., 2015). Endothelial progenitor cells have also been previously shown to undergo smooth muscle-like progenitor cell differentiation through TGF-β1-driven EndMT (Moonen et al., 2015). In addition, tumor EndMT causes the creation of a tumor microenvironment that is accompanied by cancer-associated fibroblasts (CAFs) and abnormal tumor vessels (Huang et al., 2016; Choi et al., 2018; Choi et al., 2020).
6 Conclusion and future directions
There is a need for advanced cell culture systems, such as organoids, to study the effects of shear stress on vessel formation in a sophisticated 3D environment. 3D models are needed instead of 2D monolayer cultures that mimic the structural and mechanical properties of the ECM of tissues as closely as possible. In many studies, magnetic tweezers or several magnetic tweezers were used to mimic unidirectional and bidirectional flows. The question now arises whether there are not much better biophysical systems to simulate the fluid flow through vessels. Alternative ways are needed (interaction of magnetized superparamagnetic beads as they induce a magnetic field, hysteresis). Alternative biophysical methods seem to be needed since the interaction of cells with magnetized beads, as they induce a magnetic field, is given, and an unwanted interaction could falsify the results in this case. Another limitation that needs to be overcome is the type of endothelial cells used as there are very large organ-specific differences that need to be considered when comparing results from different groups and identifying general mechanisms. In addition, the interaction of endothelial cells with immune cells also plays a role regarding their mechanosensing and mechanotransduction properties. The possibility of transition of endothelial cells to mesenchymal cells appears to be of crucial importance in angiogenesis and is likely to play a role in tumor angiogenesis, which requires more research activity in this field. All these new research activities will contribute to a more comprehensive understanding of the function of endothelial cells in mechanosensation and mechanotransduction, which may also have a positive impact on the treatment of diseases, such as cancer, which results in an altered vascular system. Finally, the possibility of personalized medicine for diseases that affect the endothelial cells appears to be given here via special “reprogramming” of the endothelial cells from the patient’s own pool.
The literature analysis outlined in this review article has led to the development and deployment of a human cell-based experimental model that subjects endothelial cells to an ambient environment that includes a properly managed dynamic fluid flow with support that provides mechanical stiffness values corresponding to physiological and pathological states. Based on this experimental approach, the goal is to elucidate how the natural mechanical environment with a mixture of fluid and solid tissue affects the organization of the endothelial glycocalyx, which acts as the foremost sensor of the mechanical surrounding, transferring mechanical force to the endothelial cells and converting the force into intracellular activity. It can be postulated that the endothelial glycocalyx is at its most uninterrupted and at minimum porosity under physiological flow conditions in conjunction with relatively soft subendothelial tissue, whereas it is at its most fragmented and at its thinnest under perturbed (pathological) flow regimes in conjunction with fairly stiff subendothelial tissue. Moreover, it is necessary to investigate the mechanisms through which endothelial glycocalyx translates extracellular forces into the biological response of endothelial cells. The cytoskeleton, such as the spectrin scaffold containing short and stable actin filaments, underneath these mechanosensory structures, comprising caveolae, glycocalyx, and membrane receptors, such as integrins, DDR1, and PIEZO, seem to be key elements that are interrelated. Spectrin is needed for stabilization endothelial caveolae, which controls the regulation of PIEZO1. Glycocalyx constituents, especially HA, can be seen as the primary mechanosensors for fluid shear, and CD44 may be indispensable to transduce cues to the nearby spectrin scaffold. When bound to CD44, spectrins control the density of hyaluronan on the cell surface, sensing shear forces and translating them into changes in plasma membrane tension. In turn, an alteration in the spectrin scaffold could slightly alter the curvature or tension exerted on caveolae, which subsequently activates PIEZO1. Consequently, the calcium influx, probably the mechanical deformation of the caveolae, leads to the release of eNOS from the caveolae and to the activation of eNOS. This dual function of spectrin seems to be essential for the mechanotransduction process in endothelial cells.
It is important, in the future, to investigate the participation of YAP/TAZ as a possible mechanism, whereby the glycocalyx exerts an influence on endothelial cells. In addition, it should also be analyzed whether YAP/TAZ acts upstream of the endothelial glycocalyx and regulates the glycocalyx instead. The research findings were expected to yield evidence on how to regulate mechanotransduction in the cells to control and alter cell performance and how cell-dependent vascular functionality can be fixed or restored. DDR1 has been identified as a mechanosensory receptor. Upon shear stress, DDR1 produces liquid-like biomolecular condensates and co-condenses together with YWHAE, which causes the nuclear translocation of YAP that activates transcription of shear stress-induced genes, such as ICAM1 and VCAM1. Developing in vitro models is required to examine the impact of mechanical stress on endothelial cells in a defined microenvironment. Complex models that closely mirror the complex microenvironment in vivo can be created using dynamic mechanical loading of endothelial cells.
A better insight into cellular reactions can be gained, and high-throughput testing for potential therapeutic treatments can be carried out (He et al., 2015; Zhang et al., 2017). The emergence of high-throughput screening instruments able to systematically investigate the effects of various mechanical stressors and their associations on endothelial cells would be of great benefit to mechanobiology. This can make it easier to identify key mechanosensors, signaling routes, and pharmaceutical targets (Nazari-Jahantigh et al., 2012; Michalik et al., 2014). The incorporation of multi-omics techniques, comprising proteomics, transcriptomics, epigenomics, and genomics, may enable a comprehensive insight into the molecular alterations that arise in endothelial cells in reaction to mechanical stress. With the help of a multidisciplinary analysis, it is potentially feasible to detect new mechanotransduction processes, possible biomarkers, and therapeutic objectives for cardiovascular illnesses (Wang et al., 2019). Progress in omics techniques and experimental models has contributed to the identification of several new potential therapeutic candidates for atherosclerosis (Tamargo et al., 2023). Omics-based analyses have emerged as a standard procedure to assess alterations in endothelial cells reacting to different flow and disease states. In contrast to conventional reductionist strategies, where only a single or a handful of candidate genes or proteins are investigated at a time, amazing breakthroughs in omics technologies and computational bioinformatics have opened the opportunity to identify alterations in genes, proteins, and metabolites at genome-wide, epigenome-wide, proteome-wide, and metabolome-wide scales, often at single-cell-level resolution and utilizing a limited quantity of the sample. The utilization of these methods with in vitro and in vivo models has generated a wealth of datasets with flow-based transcriptomic, epigenomic, proteomic, and metabolomic patterns in endothelial cells and blood vessels under both healthy and diseased states (Wu et al., 2007; Chen X. et al., 2010; Ni et al., 2010; Dunn et al., 2014; Goveia et al., 2014; Andueza et al., 2020; Ajami et al., 2017). Initial transcriptomic studies utilized bulk RNA and miRNA probes from pooled endothelial cell cultures and animal tissues to perform microarray and RNA sequencing studies. These investigations revealed many unsuspected flow-responsive genes, miRNAs, and lncRNAs, leading to far-reaching new theories concerning their various functions in endothelial cells and atherosclerosis (Tarbell et al., 2014; Simmons et al., 2016a; Demos et al., 2021). Proteomics investigations with modern mass spectrometry have revealed a large number of flow-sensitive proteins that are expressed differently in endothelial cells as a reaction to the flow or are post-translationally altered (Burghoff and Schrader, 2011; Firasat et al., 2014; Simmons et al., 2016b). Endothelial cell secretome studies indicate that the impaired flow changes the abundance of hundreds of proteins, including ANGPT2 and endothelin 1 (Burghoff and Schrader, 2011). A proteome-wide S-sulfhydration of reactive cysteines (S-sulfhydrome) in endothelial cells in reaction to pro-atherogenic stimuli in vitro and in vivo revealed hundreds of flow-sensitive S-sulfhydrated proteins (Bibli et al., 2021), such as integrins that are involved in the flow-based vascular relaxation reaction.
Future perspectives of the coupling between mechanosensation and function are still needed for a comprehensive and detailed view of the function of endothelial cells in mechanobiology. Mechanotransduction is frequently assessed after the cell has achieved equilibrium and is depicted as a unidirectional pathway from cytoskeletal activation to the transfer of nuclear events, leading to a displacement of state parameters, such as lineage determination. The mechanotransduction can, nevertheless, also activate feedback circuits that change the cell’s reaction to downstream mechanical impulses. These feedback circuits are essential to keep the cytoskeleton in a reactive equilibrium or to maintain an adapted status. A disturbance of these components impairs the mechanosensory system of the endothelial cells and may form the basis of the pathophysiology. Finally, it can be assumed that future endeavors to investigate the colocalization of mechanosensors will largely profit from new technological progress like super-resolution microscopy.
Author contributions
CM: conceptualization, data curation, formal analysis, funding acquisition, investigation, methodology, project administration, resources, software, supervision, validation, visualization, writing–original draft, and writing–review and editing.
Funding
The author(s) declare that no financial support was received for the research, authorship, and/or publication of this article.
Acknowledgments
The author thanks Thomas Michael L. Mierke for proofreading their manuscript.
Conflict of interest
The author declares that the research was conducted in the absence of any commercial or financial relationships that could be construed as a potential conflict of interest.
The author(s) declared that they were an editorial board member of Frontiers, at the time of submission. This had no impact on the peer review process and the final decision.
Publisher’s note
All claims expressed in this article are solely those of the authors and do not necessarily represent those of their affiliated organizations, or those of the publisher, the editors, and the reviewers. Any product that may be evaluated in this article, or claim that may be made by its manufacturer, is not guaranteed or endorsed by the publisher.
References
AbouAlaiwi, W. A., Takahashi, M., Mell, B. R., Jones, T. J., Ratnam, S., Kolb, R. J., et al. (2009). Ciliary polycystin-2 is a mechanosensitive calcium channel involved in nitric oxide signaling cascades. Circulation Res. 104, 860–869. doi:10.1161/CIRCRESAHA.108.192765
Adil, M. S., Narayanan, S. P., and Somanath, P. R. (2021). Cell-cell junctions: structure and regulation in physiology and pathology. Tissue Barriers 9, 1848212. doi:10.1080/21688370.2020.1848212
Aghajanian, H., Choi, C., Ho, V. C., Gupta, M., Singh, M. K., and Epstein, J. A. (2014). Semaphorin 3d and Semaphorin 3e Direct Endothelial Motility through Distinct Molecular Signaling Pathways. J. Biol. Chem. 289, 17971–17979. doi:10.1074/jbc.M113.544833
Agre, P., Orringer, E. P., and Bennett, V. (1982). Deficient red-cell spectrin in severe, recessively inherited spherocytosis. N. Engl. J. Med. 306, 1155–1161. doi:10.1056/NEJM198205133061906
Ahmad, T., Ertuglu, L. A., Masenga, S. K., Kleyman, T. R., and Kirabo, A. (2023). The epithelial sodium channel in inflammation and blood pressure modulation. Front. Cardiovasc. Med. 10, 1130148. doi:10.3389/fcvm.2023.1130148
Ahn, S. J., Fancher, I. S., Bian, J.-T., Zhang, C. X., Schwab, S., Gaffin, R., et al. (2017). Inwardly rectifying K+ channels are major contributors to flow-induced vasodilatation in resistance arteries. J. Physiol. 595, 2339–2364. doi:10.1113/JP273255
Ajami, N. E., Gupta, S., Maurya, M. R., Nguyen, P., Li, J. Y.-S., Shyy, J. Y.-J., et al. (2017). Systems biology analysis of longitudinal functional response of endothelial cells to shear stress. Proc. Natl. Acad. Sci. U.S.A. 114, 10990–10995. doi:10.1073/pnas.1707517114
Ajani, J. A., Song, S., Hochster, H. S., and Steinberg, I. B. (2015). Cancer stem cells: the promise and the potential. Seminars Oncol. 42, S3–S17. doi:10.1053/j.seminoncol.2015.01.001
Akiyama, S. K., Yamada, S. S., Chen, W. T., and Yamada, K. M. (1989). Analysis of fibronectin receptor function with monoclonal antibodies: roles in cell adhesion, migration, matrix assembly, and cytoskeletal organization. J. Cell Biol. 109, 863–875. doi:10.1083/jcb.109.2.863
Albarrán-Juárez, J., Iring, A., Wang, S., Joseph, S., Grimm, M., Strilic, B., et al. (2018). Piezo1 and Gq/G11 promote endothelial inflammation depending on flow pattern and integrin activation. J. Exp. Med. 215, 2655–2672. doi:10.1084/jem.20180483
Albrakati, A. (2022). Caveolar disruption with methyl-β-cyclodextrin causes endothelium-dependent contractions in Wistar rat carotid arteries. Environ. Sci. Pollut. Res. 29, 63071–63080. doi:10.1007/s11356-022-20226-w
Andueza, A., Kumar, S., Kim, J., Kang, D.-W., Mumme, H. L., Perez, J. I., et al. (2020). Endothelial reprogramming by disturbed flow revealed by single-cell RNA and chromatin accessibility study. Cell Rep. 33, 108491. doi:10.1016/j.celrep.2020.108491
Angulo-Urarte, A., van der Wal, T., and Huveneers, S. (2020). Cell-cell junctions as sensors and transducers of mechanical forces. Biochimica Biophysica Acta (BBA) - Biomembr. 1862, 183316. doi:10.1016/j.bbamem.2020.183316
Árnadóttir, J., and Chalfie, M. (2010). Eukaryotic mechanosensitive channels. Annu. Rev. Biophys. 39, 111–137. doi:10.1146/annurev.biophys.37.032807.125836
Aruffo, A., Stamenkovic, I., Melnick, M., Underhill, C. B., and Seed, B. (1990). CD44 is the principal cell surface receptor for hyaluronate. Cell 61, 1303–1313. doi:10.1016/0092-8674(90)90694-A
Askari, H., Sadeghinejad, M., and Fancher, I. S. (2023). “Mechanotransduction and the endothelial glycocalyx: interactions with membrane and cytoskeletal proteins to transduce force,” in Current topics in membranes (Elsevier), 43–60. doi:10.1016/bs.ctm.2023.02.003
Babaev, V. R., Bobryshev, Y. V., Stenina, O. V., Tararak, E. M., and Gabbiani, G. (1990). Heterogeneity of smooth muscle cells in atheromatous plaque of human aorta. Am. J. Pathol. 136, 1031–1042.
Badaoui, M., Zoso, A., Idris, T., Bacchetta, M., Simonin, J., Lemeille, S., et al. (2020). Vav3 mediates Pseudomonas aeruginosa adhesion to the cystic fibrosis airway epithelium. Cell Rep. 32, 107842. doi:10.1016/j.celrep.2020.107842
Baeyens, N., Mulligan-Kehoe, M. J., Corti, F., Simon, D. D., Ross, T. D., Rhodes, J. M., et al. (2014). Syndecan 4 is required for endothelial alignment in flow and atheroprotective signaling. Proc. Natl. Acad. Sci. U.S.A. 111, 17308–17313. doi:10.1073/pnas.1413725111
Baeyens, N., Nicoli, S., Coon, B. G., Ross, T. D., Van den Dries, K., Han, J., et al. (2015). Vascular remodeling is governed by a VEGFR3-dependent fluid shear stress set point. Elife 4, e04645. doi:10.7554/eLife.04645
Bagi, Z., Frangos, J. A., Yeh, J.-C., White, C. R., Kaley, G., and Koller, A. (2005). PECAM-1 mediates NO-dependent dilation of arterioles to high temporal gradients of shear stress. Arterioscler. Thromb. Vasc. Biol. 25, 1590–1595. doi:10.1161/01.ATV.0000170136.71970.5f
Bai, K., and Wang, W. (2012). Spatio-temporal development of the endothelial glycocalyx layer and its mechanical property in vitro. J. R. Soc. Interface. 9, 2290–2298. doi:10.1098/rsif.2011.0901
Baldoli, E., Castiglioni, S., and Maier, J. A. M. (2013). Regulation and function of TRPM7 in human endothelial cells: TRPM7 as a potential novel regulator of endothelial function. PLoS ONE 8, e59891. doi:10.1371/journal.pone.0059891
Ballermann, B. J., Dardik, A., Eng, E., and Liu, A. (1998). Shear stress and the endothelium. Kidney Int. 54, S100–S108. doi:10.1046/j.1523-1755.1998.06720.x
Balligand, J.-L., Feron, O., and Dessy, C. (2009). eNOS activation by physical forces: from short-term regulation of contraction to chronic remodeling of cardiovascular tissues. Physiol. Rev. 89, 481–534. doi:10.1152/physrev.00042.2007
Barakat, A. I., Leaver, E. V., Pappone, P. A., and Davies, P. F. (1999). A flow-activated chloride-selective membrane current in vascular endothelial cells. Circulation Res. 85, 820–828. doi:10.1161/01.RES.85.9.820
Baratchi, S., Almazi, J. G., Darby, W., Tovar-Lopez, F. J., Mitchell, A., and McIntyre, P. (2016). Shear stress mediates exocytosis of functional TRPV4 channels in endothelial cells. Cell. Mol. Life Sci. 73, 649–666. doi:10.1007/s00018-015-2018-8
Barry, A. K., Wang, N., and Leckband, D. E. (2015). Local VE-cadherin mechanotransduction triggers long-ranged remodeling of endothelial monolayers. J. Cell Sci. 128, 1341–1351. doi:10.1242/jcs.159954
Bartoli, F., Debant, M., Chuntharpursat-Bon, E., Evans, E. L., Musialowski, K. E., Parsonage, G., et al. (2022). Endothelial Piezo1 sustains muscle capillary density and contributes to physical activity. J. Clin. Investigation 132, e141775. doi:10.1172/JCI141775
Bartosch, A. M. W., Mathews, R., and Tarbell, J. M. (2017). Endothelial glycocalyx-mediated nitric oxide production in response to selective AFM pulling. Biophysical J. 113, 101–108. doi:10.1016/j.bpj.2017.05.033
Batra, N., Burra, S., Siller-Jackson, A. J., Gu, S., Xia, X., Weber, G. F., et al. (2012). Mechanical stress-activated integrin α5β1 induces opening of connexin 43 hemichannels. Proc. Natl. Acad. Sci. U.S.A. 109, 3359–3364. doi:10.1073/pnas.1115967109
Bayliss, W. M. (1902). On the local reactions of the arterial wall to changes of internal pressure. J. Physiology 28, 220–231. doi:10.1113/jphysiol.1902.sp000911
Becker, B. F., Jacob, M., Leipert, S., Salmon, A. H. J., and Chappell, D. (2015). Degradation of the endothelial glycocalyx in clinical settings: searching for the sheddases. Brit J. Clin. Pharma 80, 389–402. doi:10.1111/bcp.12629
Becker, D., Bereiter-Hahn, J., and Jendrach, M. (2009). Functional interaction of the cation channel transient receptor potential vanilloid 4 (TRPV4) and actin in volume regulation. Eur. J. Cell Biol. 88, 141–152. doi:10.1016/j.ejcb.2008.10.002
Belardi, B., Hamkins-Indik, T., Harris, A. R., Kim, J., Xu, K., and Fletcher, D. A. (2020). A weak link with actin organizes tight junctions to control epithelial permeability. Dev. Cell 54, 792–804. doi:10.1016/j.devcel.2020.07.022
Bennett, V., and Healy, J. (2009). Membrane domains based on ankyrin and spectrin associated with cell-cell interactions. Cold Spring Harb. Perspect. Biol. 1, a003012. doi:10.1101/cshperspect.a003012
Berrout, J., Jin, M., and O’Neil, R. G. (2012). Critical role of TRPP2 and TRPC1 channels in stretch-induced injury of blood–brain barrier endothelial cells. Brain Res. 1436, 1–12. doi:10.1016/j.brainres.2011.11.044
Bevan, J. A., and Joyce, E. H. (1990). Flow-induced resistance artery tone: balance between constrictor and dilator mechanisms. Am. J. Physiology-Heart Circulatory Physiology 258, H663–H668. doi:10.1152/ajpheart.1990.258.3.H663
Bhalla, V., and Hallows, K. R. (2008). Mechanisms of ENaC regulation and clinical implications. J. Am. Soc. Nephrol. 19, 1845–1854. doi:10.1681/ASN.2008020225
Bhullar, I. S., Li, Y.-S., Miao, H., Zandi, E., Kim, M., Shyy, J. Y.-J., et al. (1998). Fluid shear stress activation of IkappaB kinase is integrin-dependent. J. Biol. Chem. 273, 30544–30549. doi:10.1074/jbc.273.46.30544
Bibli, S.-I., Hu, J., Looso, M., Weigert, A., Ratiu, C., Wittig, J., et al. (2021). Mapping the endothelial cell S-sulfhydrome highlights the crucial role of integrin sulfhydration in vascular function. Circulation 143, 935–948. doi:10.1161/CIRCULATIONAHA.120.051877
Bisaria, A., Hayer, A., Garbett, D., Cohen, D., and Meyer, T. (2020). Membrane-proximal F-actin restricts local membrane protrusions and directs cell migration. Science 368, 1205–1210. doi:10.1126/science.aay7794
Blinder, P., Tsai, P. S., Kaufhold, J. P., Knutsen, P. M., Suhl, H., and Kleinfeld, D. (2013). The cortical angiome: an interconnected vascular network with noncolumnar patterns of blood flow. Nat. Neurosci. 16, 889–897. doi:10.1038/nn.3426
Boezio, G. L., Bensimon-Brito, A., Piesker, J., Guenther, S., Helker, C. S., and Stainier, D. Y. (2020). Endothelial TGF-β signaling instructs smooth muscle cell development in the cardiac outflow tract. eLife 9, e57603. doi:10.7554/eLife.57603
Bolotina, V. M., Najibi, S., Palacino, J. J., Pagano, P. J., and Cohen, R. A. (1994). Nitric oxide directly activates calcium-dependent potassium channels in vascular smooth muscle. Nature 368, 850–853. doi:10.1038/368850a0
Bondareva, O., Tsaryk, R., Bojovic, V., Odenthal-Schnittler, M., Siekmann, A. F., and Schnittler, H.-J. (2019). Identification of atheroprone shear stress responsive regulatory elements in endothelial cells. Cardiovasc. Res. 115, 1487–1499. doi:10.1093/cvr/cvz027
Boo, Y. C., Hwang, J., Sykes, M., Michell, B. J., Kemp, B. E., Lum, H., et al. (2002). Shear stress stimulates phosphorylation of eNOS at Ser 635 by a protein kinase A-dependent mechanism. Am. J. Physiology-Heart Circulatory Physiology 283, H1819–H1828. doi:10.1152/ajpheart.00214.2002
Boo, Y. C., and Jo, H. (2003). Flow-dependent regulation of endothelial nitric oxide synthase: role of protein kinases. Am. J. Physiology-Cell Physiology 285, C499–C508. doi:10.1152/ajpcell.00122.2003
Borza, C. M., and Pozzi, A. (2014). Discoidin domain receptors in disease. Matrix Biol. 34, 185–192. doi:10.1016/j.matbio.2013.12.002
Boulanger, C., and Lüscher, T. F. (1990). Release of endothelin from the porcine aorta. Inhibition by endothelium-derived nitric oxide. J. Clin. Invest. 85, 587–590. doi:10.1172/JCI114477
Boycott, H. E., Nguyen, M.-N., Vrellaku, B., Gehmlich, K., and Robinson, P. (2020). Nitric oxide and mechano-electrical transduction in cardiomyocytes. Front. Physiol. 11, 606740. doi:10.3389/fphys.2020.606740
Boyd, N. L., Park, H., Yi, H., Boo, Y. C., Sorescu, G. P., Sykes, M., et al. (2003). Chronic shear induces caveolae formation and alters ERK and Akt responses in endothelial cells. Am. J. Physiology-Heart Circulatory Physiology 285, H1113–H1122. doi:10.1152/ajpheart.00302.2003
Brayden, J. E., Earley, S., Nelson, M. T., and Reading, S. (2008). Transient receptor potential (trp) channels, vascular tone and autoregulation of cerebral blood flow. Clin. Exp. Pharma Physio 35, 1116–1120. doi:10.1111/j.1440-1681.2007.04855.x
Brohawn, S. G. (2015). How ion channels sense mechanical force: insights from mechanosensitive K2P channels TRAAK, TREK1, and TREK2. Ann. N.Y. Acad. Sci. 1352, 20–32. doi:10.1111/nyas.12874
Brohawn, S. G., Su, Z., and MacKinnon, R. (2014). Mechanosensitivity is mediated directly by the lipid membrane in TRAAK and TREK1 K+ channels. Proc. Natl. Acad. Sci. U. S. A. 111, 3614–3619. doi:10.1073/pnas.1320768111
Brooks, A. R., Lelkes, P. I., and Rubanyi, G. M. (2004). Gene expression profiling of vascular endothelial cells exposed to fluid mechanical forces: relevance for focal susceptibility to atherosclerosis. Endothelium 11, 45–57. doi:10.1080/10623320490432470
Brozovich, F. V., Nicholson, C. J., Degen, C. V., Gao, Y. Z., Aggarwal, M., and Morgan, K. G. (2016). Mechanisms of vascular smooth muscle contraction and the basis for pharmacologic treatment of smooth muscle disorders. Pharmacol. Rev. 68, 476–532. doi:10.1124/pr.115.010652
Budatha, M., Zhang, J., Zhuang, Z. W., Yun, S., Dahlman, J. E., Anderson, D. G., et al. (2018). Inhibiting integrin α5 cytoplasmic domain signaling reduces atherosclerosis and promotes arteriogenesis. JAHA 7, e007501. doi:10.1161/JAHA.117.007501
Burghoff, S., and Schrader, J. (2011). Secretome of human endothelial cells under shear stress. J. Proteome Res. 10, 1160–1169. doi:10.1021/pr100937a
Buschmann, I., Pries, A., Styp-Rekowska, B., Hillmeister, P., Loufrani, L., Henrion, D., et al. (2010). Pulsatile shear and Gja5 modulate arterial identity and remodeling events during flow-driven arteriogenesis. Development 137, 2187–2196. doi:10.1242/dev.045351
Cahill, P. A., and Redmond, E. M. (2016). Vascular endothelium – gatekeeper of vessel health. Atherosclerosis 248, 97–109. doi:10.1016/j.atherosclerosis.2016.03.007
Calderwood, D. A., Zent, R., Grant, R., Rees, D. J. G., Hynes, R. O., and Ginsberg, M. H. (1999). The Talin head domain binds to integrin beta subunit cytoplasmic tails and regulates integrin activation. J. Biol. Chem. 274, 28071–28074. doi:10.1074/jbc.274.40.28071
Campbell, I. D., and Humphries, M. J. (2011). Integrin structure, activation, and interactions. Cold Spring Harb. Perspect. Biol. 3, a004994. doi:10.1101/cshperspect.a004994
Cantalupo, A., Gargiulo, A., Dautaj, E., Liu, C., Zhang, Y., Hla, T., et al. (2017). S1PR1 (Sphingosine-1-Phosphate receptor 1) signaling regulates blood flow and pressure. Hypertension 70, 426–434. doi:10.1161/HYPERTENSIONAHA.117.09088
Caolo, V., Debant, M., Endesh, N., Futers, T. S., Lichtenstein, L., Bartoli, F., et al. (2020). Shear stress activates ADAM10 sheddase to regulate Notch1 via the Piezo1 force sensor in endothelial cells. eLife 9, e50684. doi:10.7554/eLife.50684
Carafoli, F., Mayer, M. C., Shiraishi, K., Pecheva, M. A., Chan, L. Y., Nan, R., et al. (2012). Structure of the discoidin domain receptor 1 extracellular region bound to an inhibitory fab fragment reveals features important for signaling. Structure 20, 688–697. doi:10.1016/j.str.2012.02.011
Chachisvilis, M., Zhang, Y.-L., and Frangos, J. A. (2006). G protein-coupled receptors sense fluid shear stress in endothelial cells. Proc. Natl. Acad. Sci. U.S.A. 103, 15463–15468. doi:10.1073/pnas.0607224103
Chappell, D., Jacob, M., Paul, O., Rehm, M., Welsch, U., Stoeckelhuber, M., et al. (2009). The glycocalyx of the human umbilical vein endothelial cell: an impressive structure ex vivo but not in culture. Circulation Res. 104, 1313–1317. doi:10.1161/CIRCRESAHA.108.187831
Chasis, J. A., Agre, P., and Mohandas, N. (1988). Decreased membrane mechanical stability and in vivo loss of surface area reflect spectrin deficiencies in hereditary spherocytosis. J. Clin. Invest. 82, 617–623. doi:10.1172/JCI113640
Chatterjee, S., and Fisher, A. B. (2014). Mechanotransduction in the endothelium: role of membrane proteins and reactive oxygen species in sensing, transduction, and transmission of the signal with altered blood flow. Antioxidants and Redox Signal. 20, 899–913. doi:10.1089/ars.2013.5624
Chemin, J., Patel, A. J., Duprat, F., Lauritzen, I., Lazdunski, M., and Honoré, E. (2005). A phospholipid sensor controls mechanogating of the K+ channel TREK-1. EMBO J. 24, 44–53. doi:10.1038/sj.emboj.7600494
Chen, E. A., and Lin, Y.-S. (2019). Using synthetic peptides and recombinant collagen to understand DDR–collagen interactions. Biochimica Biophysica Acta (BBA) - Mol. Cell Res. 1866, 118458. doi:10.1016/j.bbamcr.2019.03.005
Chen, G., Suzuki, H., and Weston, A. H. (1988). Acetylcholine releases endothelium-derived hyperpolarizing factor and EDRF from rat blood vessels. Br. J Pharmacol. 95, 1165–1174. doi:10.1111/j.1476-5381.1988.tb11752.x
Chen, J., Green, J., Yurdagul, A., Albert, P., McInnis, M. C., and Orr, A. W. (2015). αvβ3 integrins mediate flow-induced NF-κB activation, proinflammatory gene expression, and early atherogenic inflammation. Am. J. Pathology 185, 2575–2589. doi:10.1016/j.ajpath.2015.05.013
Chen, J., Zhang, J., Yang, J., Xu, L., Hu, Q., Xu, C., et al. (2017). Histone demethylase KDM3a, a novel regulator of vascular smooth muscle cells, controls vascular neointimal hyperplasia in diabetic rats. Atherosclerosis 257, 152–163. doi:10.1016/j.atherosclerosis.2016.12.007
Chen, K.-D., Li, Y.-S., Kim, M., Li, S., Yuan, S., Chien, S., et al. (1999). Mechanotransduction in response to shear stress. Roles of receptor tyrosine kinases, integrins, and Shc. J. Biol. Chem. 274, 18393–18400. doi:10.1074/jbc.274.26.18393
Chen, T. T., Luque, A., Lee, S., Anderson, S. M., Segura, T., and Iruela-Arispe, M. L. (2010a). Anchorage of VEGF to the extracellular matrix conveys differential signaling responses to endothelial cells. J. Cell Biol. 188, 595–609. doi:10.1083/jcb.200906044
Chen, X., Liu, L., Palacios, G., Gao, J., Zhang, N., Li, G., et al. (2010b). Plasma metabolomics reveals biomarkers of the atherosclerosis. J. Sep. Sci. 33, 2776–2783. doi:10.1002/jssc.201000395
Chen, Z., Rubin, J., and Tzima, E. (2010c). Role of PECAM-1 in arteriogenesis and specification of preexisting collaterals. Circ. Res. 107, 1355–1363. doi:10.1161/CIRCRESAHA.110.229955
Chen, Z., and Tzima, E. (2009). PECAM-1 is necessary for flow-induced vascular remodeling. Arterioscler. Thromb. Vasc. Biol. 29, 1067–1073. doi:10.1161/ATVBAHA.109.186692
Cheng, C., Herfkens, R. J., and Taylor, C. A. (2003a). Abdominal aortic hemodynamic conditions in healthy subjects aged 50–70 at rest and during lower limb exercise: in vivo quantification using MRI. Atherosclerosis 168, 323–331. doi:10.1016/S0021-9150(03)00099-6
Cheng, C., Tempel, D., van Haperen, R., van der Baan, A., Grosveld, F., Daemen, M. J. A. P., et al. (2006). Atherosclerotic lesion size and vulnerability are determined by patterns of fluid shear stress. Circulation 113, 2744–2753. doi:10.1161/CIRCULATIONAHA.105.590018
Cheng, C. P., Herfkens, R. J., and Taylor, C. A. (2003b). Comparison of abdominal aortic hemodynamics between men and women at rest and during lower limb exercise. J. Vasc. Surg. 37, 118–123. doi:10.1067/mva.2002.107
Chen-Konak, L., Guetta-Shubin, Y., Yahav, H., Shay-Salit, A., Zilberman, M., Binah, O., et al. (2003). Transcriptional and post-translation regulation of the Tie1 receptor by fluid shear stress changes in vascular endothelial cells. FASEB J. 17, 2121–2123. doi:10.1096/fj.02-1151fje
Chien, S. (2003). Molecular and mechanical bases of focal lipid accumulation in arterial wall. Prog. Biophysics Mol. Biol. 83, 131–151. doi:10.1016/S0079-6107(03)00053-1
Chien, S. (2007). Mechanotransduction and endothelial cell homeostasis: the wisdom of the cell. Am. J. Physiology-Heart Circulatory Physiology 292, H1209–H1224. doi:10.1152/ajpheart.01047.2006
Chien, S., Li, S., and Shyy, J. Y.-J. (1998). Effects of mechanical forces on signal transduction and gene expression in endothelial cells. Hypertension 31, 162–169. doi:10.1161/01.HYP.31.1.162
Chigaev, A., and Sklar, L. A. (2012). Aspects of VLA-4 and LFA-1 regulation that may contribute to rolling and firm adhesion. Front. Immun. 3, 242. doi:10.3389/fimmu.2012.00242
Chiu, J.-J., and Chien, S. (2011). Effects of disturbed flow on vascular endothelium: pathophysiological basis and clinical perspectives. Physiol. Rev. 91, 327–387. doi:10.1152/physrev.00047.2009
Chiu, Y.-J., McBeath, E., and Fujiwara, K. (2008). Mechanotransduction in an extracted cell model: fyn drives stretch- and flow-elicited PECAM-1 phosphorylation. J. Cell Biol. 182, 753–763. doi:10.1083/jcb.200801062
Cho, J. G., Lee, A., Chang, W., Lee, M.-S., and Kim, J. (2018). Endothelial to mesenchymal transition represents a key link in the interaction between inflammation and endothelial dysfunction. Front. Immunol. 9, 294. doi:10.3389/fimmu.2018.00294
Choi, K. J., Nam, J.-K., Kim, J.-H., Choi, S.-H., and Lee, Y.-J. (2020). Endothelial-to-mesenchymal transition in anticancer therapy and normal tissue damage. Exp. Mol. Med. 52, 781–792. doi:10.1038/s12276-020-0439-4
Choi, S.-H., Kim, A.-R., Nam, J.-K., Kim, J.-M., Kim, J.-Y., Seo, H. R., et al. (2018). Tumour-vasculature development via endothelial-to-mesenchymal transition after radiotherapy controls CD44v6+ cancer cell and macrophage polarization. Nat. Commun. 9, 5108. doi:10.1038/s41467-018-07470-w
Christensen, A. P., and Corey, D. P. (2007). TRP channels in mechanosensation: direct or indirect activation? Nat. Rev. Neurosci. 8, 510–521. doi:10.1038/nrn2149
Chuang, Y.-C., and Chen, C.-C. (2022). Force from filaments: the role of the cytoskeleton and extracellular matrix in the gating of mechanosensitive channels. Front. Cell Dev. Biol. 10, 886048. doi:10.3389/fcell.2022.886048
Chun, T.-H., Itoh, H., Ogawa, Y., Tamura, N., Takaya, K., Igaki, T., et al. (1997). Shear stress augments expression of C-type natriuretic peptide and adrenomedullin. Hypertension 29, 1296–1302. doi:10.1161/01.HYP.29.6.1296
Chuntharpursat-Bon, E., Povstyan, O., Ludlow, M., Gaunt, H., Baxter, P., and Beech, D. (2019). Cell adhesion molecule interaction with Piezo1 channels is a mechanism for sub cellular regulation of mechanical sensitivity. bioRxiv. doi:10.1101/602532
Chuntharpursat-Bon, E., Povstyan, O. V., Ludlow, M. J., Carrier, D. J., Debant, M., Shi, J., et al. (2023). PIEZO1 and PECAM1 interact at cell-cell junctions and partner in endothelial force sensing. Commun. Biol. 6, 358. doi:10.1038/s42003-023-04706-4
Claesson-Welsh, L., Dejana, E., and McDonald, D. M. (2021). Permeability of the endothelial barrier: identifying and reconciling controversies. Trends Mol. Med. 27, 314–331. doi:10.1016/j.molmed.2020.11.006
Clere, N., Renault, S., and Corre, I. (2020). Endothelial-to-Mesenchymal transition in cancer. Front. Cell Dev. Biol. 8, 747. doi:10.3389/fcell.2020.00747
Climent, M., Quintavalle, M., Miragoli, M., Chen, J., Condorelli, G., and Elia, L. (2015). TGFβ triggers miR-143/145 transfer from smooth muscle cells to endothelial cells, thereby modulating vessel stabilization. Circulation Res. 116, 1753–1764. doi:10.1161/CIRCRESAHA.116.305178
Coelho, N. M., Arora, P. D., Van Putten, S., Boo, S., Petrovic, P., Lin, A. X., et al. (2017). Discoidin domain receptor 1 mediates myosin-dependent collagen contraction. Cell Rep. 18, 1774–1790. doi:10.1016/j.celrep.2017.01.061
Cole, W. C., and Welsh, D. G. (2011). Role of myosin light chain kinase and myosin light chain phosphatase in the resistance arterial myogenic response to intravascular pressure. Archives Biochem. Biophysics 510, 160–173. doi:10.1016/j.abb.2011.02.024
Collins, C., Guilluy, C., Welch, C., O’Brien, E. T., Hahn, K., Superfine, R., et al. (2012). Localized tensional forces on PECAM-1 elicit a global mechanotransduction response via the integrin-RhoA pathway. Curr. Biol. 22, 2087–2094. doi:10.1016/j.cub.2012.08.051
Collins, C., Osborne, L. D., Guilluy, C., Chen, Z., O’Brien, E. T., Reader, J. S., et al. (2014). Haemodynamic and extracellular matrix cues regulate the mechanical phenotype and stiffness of aortic endothelial cells. Nat. Commun. 5, 3984. doi:10.1038/ncomms4984
Conforti, G., Dominguez-Jimenez, C., Zanetti, A., Gimbrone, M. A., Cremona, O., Marchisio, P. C., et al. (1992). Human endothelial cells express integrin receptors on the luminal aspect of their membrane. Blood 80, 437–446. doi:10.1182/blood.v80.2.437.437
Conway, D. E., Breckenridge, M. T., Hinde, E., Gratton, E., Chen, C. S., and Schwartz, M. A. (2013). Fluid shear stress on endothelial cells modulates mechanical tension across VE-cadherin and PECAM-1. Curr. Biol. 23, 1024–1030. doi:10.1016/j.cub.2013.04.049
Coon, B. G., Baeyens, N., Han, J., Budatha, M., Ross, T. D., Fang, J. S., et al. (2015). Intramembrane binding of VE-cadherin to VEGFR2 and VEGFR3 assembles the endothelial mechanosensory complex. J. Cell Biol. 208, 975–986. doi:10.1083/jcb.201408103
Coon, B. G., Timalsina, S., Astone, M., Zhuang, Z. W., Fang, J., Han, J., et al. (2022). A mitochondrial contribution to anti-inflammatory shear stress signaling in vascular endothelial cells. J. Cell Biol. 221, e202109144. doi:10.1083/jcb.202109144
Corson, M. A., James, N. L., Latta, S. E., Nerem, R. M., Berk, B. C., and Harrison, D. G. (1996). Phosphorylation of endothelial nitric oxide synthase in response to fluid shear stress. Circulation Res. 79, 984–991. doi:10.1161/01.RES.79.5.984
Cosgun, Z. C., Sternak, M., Fels, B., Bar, A., Kwiatkowski, G., Pacia, M. Z., et al. (2022). Rapid shear stress-dependent ENaC membrane insertion is mediated by the endothelial glycocalyx and the mineralocorticoid receptor. Cell. Mol. Life Sci. 79, 235. doi:10.1007/s00018-022-04260-y
Coste, B., Murthy, S. E., Mathur, J., Schmidt, M., Mechioukhi, Y., Delmas, P., et al. (2015). Piezo1 ion channel pore properties are dictated by C-terminal region. Nat. Commun. 6, 7223. doi:10.1038/ncomms8223
Cudmore, R. H., and Santana, L. F. (2022). Piezo1 tunes blood flow in the central nervous system. Circ. Res. 130, 1547–1549. doi:10.1161/CIRCRESAHA.122.321144
Cuhlmann, S., Van der Heiden, K., Saliba, D., Tremoleda, J. L., Khalil, M., Zakkar, M., et al. (2011). Disturbed blood flow induces RelA expression via c-Jun N-terminal kinase 1: a novel mode of NF-κB regulation that promotes arterial inflammation. Circ. Res. 108, 950–959. doi:10.1161/CIRCRESAHA.110.233841
Cui, X., Tong, J., Yau, J., Bajpai, A., Yang, J., Peng, Y., et al. (2020). Mechanical forces regulate asymmetric vascular cell alignment. Biophysical J. 119, 1771–1780. doi:10.1016/j.bpj.2020.09.020
Cunningham, K. S., and Gotlieb, A. I. (2005). The role of shear stress in the pathogenesis of atherosclerosis. Lab. Investig. 85, 9–23. doi:10.1038/labinvest.3700215
Dabertrand, F., Harraz, O. F., Koide, M., Longden, T. A., Rosehart, A. C., Hill-Eubanks, D. C., et al. (2021). PIP 2 corrects cerebral blood flow deficits in small vessel disease by rescuing capillary Kir2.1 activity. Proc. Natl. Acad. Sci. U.S.A. 118, e2025998118. doi:10.1073/pnas.2025998118
Dai, G., Vaughn, S., Zhang, Y., Wang, E. T., Garcia-Cardena, G., and Gimbrone, M. A. (2007). Biomechanical forces in atherosclerosis-resistant vascular regions regulate endothelial redox balance via phosphoinositol 3-kinase/akt-dependent activation of Nrf2. Circulation Res. 101, 723–733. doi:10.1161/CIRCRESAHA.107.152942
Dai, Z., Zhu, M. M., Peng, Y., Jin, H., Machireddy, N., Qian, Z., et al. (2018). Endothelial and smooth muscle cell interaction via FoxM1 signaling mediates vascular remodeling and pulmonary hypertension. Am. J. Respir. Crit. Care Med. 198, 788–802. doi:10.1164/rccm.201709-1835OC
Dardik, A., Liu, A., and Ballermann, B. J. (1999). Chronic in vitro shear stress stimulates endothelial cell retention on prosthetic vascular grafts and reduces subsequent in vivo neointimal thickness. J. Vasc. Surg. 29, 157–167. doi:10.1016/S0741-5214(99)70357-5
Datta, B., Tufnell-Barrett, T., Bleasdale, R. A., Jones, C. J. H., Beeton, I., Paul, V., et al. (2004). Red blood cell nitric oxide as an endocrine vasoregulator: a potential role in congestive heart failure. Circulation 109, 1339–1342. doi:10.1161/01.CIR.0000124450.07016.1D
Davies, P. F. (1995). Flow-mediated endothelial mechanotransduction. Physiol. Rev. 75, 519–560. doi:10.1152/physrev.1995.75.3.519
Davis, M. J. (1993). Myogenic response gradient in an arteriolar network. Am. J. Physiology-Heart Circulatory Physiology 264, H2168–H2179. doi:10.1152/ajpheart.1993.264.6.H2168
Davis, M. J., Earley, S., Li, Y.-S., and Chien, S. (2023). Vascular mechanotransduction. Physiol. Rev. 103, 1247–1421. doi:10.1152/physrev.00053.2021
Davis, M. J., and Hill, M. A. (1999). Signaling mechanisms underlying the vascular myogenic response. Physiol. Rev. 79, 387–423. doi:10.1152/physrev.1999.79.2.387
DeCastro, A. J. L., Pranda, M. A., Gray, K. M., Merlo-Coyne, J., Girma, N., Hurwitz, M., et al. (2022). Morphological phenotyping of organotropic brain- and bone-seeking triple negative metastatic breast tumor cells. Front. Cell Dev. Biol. 10, 790410. doi:10.3389/fcell.2022.790410
Dejana, E., Hirschi, K. K., and Simons, M. (2017). The molecular basis of endothelial cell plasticity. Nat. Commun. 8, 14361. doi:10.1038/ncomms14361
Dekker, R. J., Van Soest, S., Fontijn, R. D., Salamanca, S., De Groot, P. G., VanBavel, E., et al. (2002). Prolonged fluid shear stress induces a distinct set of endothelial cell genes, most specifically lung Krüppel-like factor (KLF2). Blood 100, 1689–1698. doi:10.1182/blood-2002-01-0046
Dekker, R. J., Van Thienen, J. V., Rohlena, J., De Jager, S. C., Elderkamp, Y. W., Seppen, J., et al. (2005). Endothelial KLF2 links local arterial shear stress levels to the expression of vascular tone-regulating genes. Am. J. Pathology 167, 609–618. doi:10.1016/S0002-9440(10)63002-7
Dela Paz, N. G., Melchior, B., and Frangos, J. A. (2017). Shear stress induces Gαq/11 activation independently of G protein-coupled receptor activation in endothelial cells. Am. J. Physiol. Cell Physiol. 312, C428–C437. doi:10.1152/ajpcell.00148.2016
Delmas, P., Nomura, H., Li, X., Lakkis, M., Luo, Y., Segal, Y., et al. (2002). Constitutive activation of G-proteins by polycystin-1 is antagonized by polycystin-2. J. Biol. Chem. 277, 11276–11283. doi:10.1074/jbc.M110483200
Demos, C., Tamargo, I., and Jo, H. (2021). “Biomechanical regulation of endothelial function in atherosclerosis,” in Biomechanics of coronary atherosclerotic plaque (Elsevier), 3–47. doi:10.1016/B978-0-12-817195-0.00001-9
Deng, L., Blanco, F. J., Stevens, H., Lu, R., Caudrillier, A., McBride, M., et al. (2015). MicroRNA-143 activation regulates smooth muscle and endothelial cell crosstalk in pulmonary arterial hypertension. Circulation Res. 117, 870–883. doi:10.1161/CIRCRESAHA.115.306806
De Oca, A. M., Madueño, J. A., Martinez-Moreno, J. M., Guerrero, F., Muñoz-Castañeda, J., Rodriguez-Ortiz, M. E., et al. (2010). High-phosphate-induced calcification is related to SM22α promoter methylation in vascular smooth muscle cells. J. Bone Mineral Res. 25, 1996–2005. doi:10.1002/jbmr.93
Dorland, Y. L., and Huveneers, S. (2017). Cell–cell junctional mechanotransduction in endothelial remodeling. Cell. Mol. Life Sci. 74, 279–292. doi:10.1007/s00018-016-2325-8
Douglas, G., Mehta, V., Al Haj Zen, A., Akoumianakis, I., Goel, A., Rashbrook, V. S., et al. (2020). A key role for the novel coronary artery disease gene JCAD in atherosclerosis via shear stress mechanotransduction. Cardiovasc. Res. 116, 1863–1874. doi:10.1093/cvr/cvz263
Drab, M., Verkade, P., Elger, M., Kasper, M., Lohn, M., Lauterbach, B., et al. (2001). Loss of caveolae, vascular dysfunction, and pulmonary defects in caveolin-1 gene-disrupted mice. Science 293, 2449–2452. doi:10.1126/science.1062688
Duband, J. L., Nuckolls, G. H., Ishihara, A., Hasegawa, T., Yamada, K. M., Thiery, J. P., et al. (1988). Fibronectin receptor exhibits high lateral mobility in embryonic locomoting cells but is immobile in focal contacts and fibrillar streaks in stationary cells. J. Cell Biol. 107, 1385–1396. doi:10.1083/jcb.107.4.1385
Duchemin, A.-L., Vignes, H., and Vermot, J. (2019). Mechanically activated piezo channels modulate outflow tract valve development through the Yap1 and Klf2-Notch signaling axis. eLife 8, e44706. doi:10.7554/eLife.44706
Dudãu, M., Codrici, E., Tanase, C., Gherghiceanu, M., Enciu, A.-M., and Hinescu, M. E. (2020). Caveolae as potential hijackable gates in cell communication. Front. Cell Dev. Biol. 8, 581732. doi:10.3389/fcell.2020.581732
Dulhunty, A. F., and Franzini-Armstrong, C. (1975). The relative contributions of the folds and caveolae to the surface membrane of frog skeletal muscle fibres at different sarcomere lengths. J. Physiology 250, 513–539. doi:10.1113/jphysiol.1975.sp011068
Dull, R. O., and Hahn, R. G. (2022). The glycocalyx as a permeability barrier: basic science and clinical evidence. Crit. Care 26, 273. doi:10.1186/s13054-022-04154-2
Duncan, G. S., Andrew, D. P., Takimoto, H., Kaufman, S. A., Yoshida, H., Spellberg, J., et al. (1999). Genetic evidence for functional redundancy of Platelet/Endothelial cell adhesion molecule-1 (PECAM-1): CD31-deficient mice reveal PECAM-1-dependent and PECAM-1-independent functions. J. Immunol. 162, 3022–3030. doi:10.4049/jimmunol.162.5.3022
Dunn, J., Qiu, H., Kim, S., Jjingo, D., Hoffman, R., Kim, C. W., et al. (2014). Flow-dependent epigenetic DNA methylation regulates endothelial gene expression and atherosclerosis. J. Clin. Invest. 124, 3187–3199. doi:10.1172/JCI74792
Earley, S., and Brayden, J. E. (2015). Transient receptor potential channels in the vasculature. Physiol. Rev. 95, 645–690. doi:10.1152/physrev.00026.2014
Ebong, E. E., Lopez-Quintero, S. V., Rizzo, V., Spray, D. C., and Tarbell, J. M. (2014). Shear-induced endothelial NOS activation and remodeling via heparan sulfate, glypican-1, and syndecan-1. Integr. Biol. (Camb) 6, 338–347. doi:10.1039/c3ib40199e
Egorova, A. D., Khedoe, P. P. S. J., Goumans, M.-J. T. H., Yoder, B. K., Nauli, S. M., ten Dijke, P., et al. (2011a). Lack of primary cilia primes shear-induced endothelial-to-mesenchymal transition. Circ. Res. 108, 1093–1101. doi:10.1161/CIRCRESAHA.110.231860
Egorova, A. D., Van Der Heiden, K., Van De Pas, S., Vennemann, P., Poelma, C., DeRuiter, M. C., et al. (2011b). Tgfβ/Alk5 signaling is required for shear stress induced klf2 expression in embryonic endothelial cells. Dev. Dyn. 240, 1670–1680. doi:10.1002/dvdy.22660
Elosegui-Artola, A., Andreu, I., Beedle, A. E. M., Lezamiz, A., Uroz, M., Kosmalska, A. J., et al. (2017). Force triggers YAP nuclear entry by regulating transport across nuclear pores. Cell 171, 1397–1410. doi:10.1016/j.cell.2017.10.008
Endemann, D. H., and Schiffrin, E. L. (2004). Endothelial dysfunction. J. Am. Soc. Nephrol. 15, 1983–1992. doi:10.1097/01.ASN.0000132474.50966.DA
Engelman, J. A., Chu, C., Lin, A., Jo, H., Ikezu, T., Okamoto, T., et al. (1998). Caveolin-mediated regulation of signaling along the p42/44 MAP kinase cascade in vivo: a role for the caveolin-scaffolding domain. FEBS Lett. 428, 205–211. doi:10.1016/S0014-5793(98)00470-0
Erdmann, J., Willenborg, C., Nahrstaedt, J., Preuss, M., Konig, I. R., Baumert, J., et al. (2011). Genome-wide association study identifies a new locus for coronary artery disease on chromosome 10p11.23. Eur. Heart J. 32, 158–168. doi:10.1093/eurheartj/ehq405
Erdogmus, S., Storch, U., Danner, L., Becker, J., Winter, M., Ziegler, N., et al. (2019). Helix 8 is the essential structural motif of mechanosensitive GPCRs. Nat. Commun. 10, 5784. doi:10.1038/s41467-019-13722-0
Evans, E. L., Cuthbertson, K., Endesh, N., Rode, B., Blythe, N. M., Hyman, A. J., et al. (2018). Yoda1 analogue (Dooku1) which antagonizes Yoda1-evoked activation of Piezo1 and aortic relaxation. Br. J. Pharmacol. 175, 1744–1759. doi:10.1111/bph.14188
Eyckmans, J., Boudou, T., Yu, X., and Chen, C. S. (2011). A hitchhiker’s guide to mechanobiology. Dev. Cell 21, 35–47. doi:10.1016/j.devcel.2011.06.015
Feaver, R. E., Gelfand, B. D., and Blackman, B. R. (2013). Human haemodynamic frequency harmonics regulate the inflammatory phenotype of vascular endothelial cells. Nat. Commun. 4, 1525. doi:10.1038/ncomms2530
Félétou, M., and Vanhoutte, P. M. (2006). Endothelial dysfunction: a multifaceted disorder (the wiggers award lecture). Am. J. Physiology-Heart Circulatory Physiology 291, H985–H1002. doi:10.1152/ajpheart.00292.2006
Fels, B., and Kusche-Vihrog, K. (2020). It takes more than two to tango: mechanosignaling of the endothelial surface. Pflugers Arch. - Eur. J. Physiol. 472, 419–433. doi:10.1007/s00424-020-02369-2
Fels, J., Jeggle, P., Kusche-Vihrog, K., and Oberleithner, H. (2012). Cortical actin nanodynamics determines nitric oxide release in vascular endothelium. PLoS ONE 7, e41520. doi:10.1371/journal.pone.0041520
Fels, J., Oberleithner, H., and Kusche-Vihrog, K. (2010). Ménage à trois: Aldosterone, sodium and nitric oxide in vascular endothelium. Biochimica Biophysica Acta (BBA) - Mol. Basis Dis. 1802, 1193–1202. doi:10.1016/j.bbadis.2010.03.006
Ferraris, D. M., Gherardi, E., Di, Y., Heinz, D. W., and Niemann, H. H. (2010). Ligand-mediated dimerization of the met receptor tyrosine kinase by the bacterial invasion protein InlB. J. Mol. Biol. 395, 522–532. doi:10.1016/j.jmb.2009.10.074
Fiore, V. F., Ju, L., Chen, Y., Zhu, C., and Barker, T. H. (2014). Dynamic catch of a Thy-1-α5β1+syndecan-4 trimolecular complex. Nat. Commun. 5, 4886. doi:10.1038/ncomms5886
Firasat, S., Hecker, M., Binder, L., and Asif, A. R. (2014). Advances in endothelial shear stress proteomics. Expert Rev. Proteomics 11, 611–619. doi:10.1586/14789450.2014.933673
Fleming, I. (2010). Molecular mechanisms underlying the activation of eNOS. Pflugers Arch. - Eur. J. Physiol. 459, 793–806. doi:10.1007/s00424-009-0767-7
Fleming, I., Bauersachs, J., Fisslthaler, B., and Busse, R. (1998). Ca 2+ -independent activation of the endothelial nitric oxide synthase in response to tyrosine phosphatase inhibitors and fluid shear stress. Circulation Res. 82, 686–695. doi:10.1161/01.RES.82.6.686
Fleming, I., and Busse, R. (1999). Signal transduction of eNOS activation. Cardiovasc. Res. 43, 532–541. doi:10.1016/S0008-6363(99)00094-2
Fleming, I., Fisslthaler, B., Dixit, M., and Busse, R. (2005). Role of PECAM-1 in the shear-stress-induced activation of Akt and the endothelial nitric oxide synthase (eNOS) in endothelial cells. J. Cell Sci. 118, 4103–4111. doi:10.1242/jcs.02541
Fleming, I., Rueben, A., Popp, R., Fisslthaler, B., Schrodt, S., Sander, A., et al. (2007). Epoxyeicosatrienoic acids regulate trp channel–dependent Ca 2+ signaling and hyperpolarization in endothelial cells. ATVB 27, 2612–2618. doi:10.1161/ATVBAHA.107.152074
Fontaine, M., Herkenne, S., Ek, O., Paquot, A., Boeckx, A., Paques, C., et al. (2021). Extracellular vesicles mediate communication between endothelial and vascular smooth muscle cells. IJMS 23, 331. doi:10.3390/ijms23010331
Foote, C. A., Soares, R. N., Ramirez-Perez, F. I., Ghiarone, T., Aroor, A., Manrique-Acevedo, C., et al. (2022). “Endothelial glycocalyx,” in Comprehensive physiology. Editor Y. S. Prakash (Wiley), 3781–3811. doi:10.1002/cphy.c210029
Frank, P. G., Woodman, S. E., Park, D. S., and Lisanti, M. P. (2003). Caveolin, caveolae, and endothelial cell function. Arterioscler. Thromb. Vasc. Biol. 23, 1161–1168. doi:10.1161/01.ATV.0000070546.16946.3A
Friddle, R. W., Noy, A., and De Yoreo, J. J. (2012). Interpreting the widespread nonlinear force spectra of intermolecular bonds. Proc. Natl. Acad. Sci. U.S.A. 109, 13573–13578. doi:10.1073/pnas.1202946109
Fridolfsson, H. N., Roth, D. M., Insel, P. A., and Patel, H. H. (2014). Regulation of intracellular signaling and function by caveolin. FASEB J. 28, 3823–3831. doi:10.1096/fj.14-252320
Friedland, J. C., Lee, M. H., and Boettiger, D. (2009). Mechanically activated integrin switch controls α 5 β 1 function. Science 323, 642–644. doi:10.1126/science.1168441
Fronius, M. (2022). Epithelial Na+ channel and the glycocalyx: a sweet and salty relationship for arterial shear stress sensing. Curr. Opin. Nephrol. and Hypertens. 31, 142–150. doi:10.1097/MNH.0000000000000779
Frutkin, A. D., Otsuka, G., Stempien-Otero, A., Sesti, C., Du, L., Jaffe, M., et al. (2009). TGF-[beta]1 limits plaque growth, stabilizes plaque structure, and prevents aortic dilation in apolipoprotein E-null mice. ATVB 29, 1251–1257. doi:10.1161/ATVBAHA.109.186593
Gantner, B. N., LaFond, K. M., and Bonini, M. G. (2020). Nitric oxide in cellular adaptation and disease. Redox Biol. 34, 101550. doi:10.1016/j.redox.2020.101550
Gao, F., Lucke-Wold, B. P., Li, X., Logsdon, A. F., Xu, L.-C., Xu, S., et al. (2018). Reduction of endothelial nitric oxide increases the adhesiveness of constitutive endothelial membrane ICAM-1 through src-mediated phosphorylation. Front. Physiol. 8, 1124. doi:10.3389/fphys.2017.01124
Gao, L., and Lipowsky, H. H. (2010). Composition of the endothelial glycocalyx and its relation to its thickness and diffusion of small solutes. Microvasc. Res. 80, 394–401. doi:10.1016/j.mvr.2010.06.005
Gao, X., Wu, L., and O’Neil, R. G. (2003). Temperature-modulated diversity of TRPV4 channel gating: activation by physical stresses and phorbol ester derivatives through protein kinase C-dependent and -independent pathways. J. Biol. Chem. 278, 27129–27137. doi:10.1074/jbc.M302517200
García-Cardeña, G., Fan, R., Stern, D. F., Liu, J., and Sessa, W. C. (1996). Endothelial nitric oxide synthase is regulated by tyrosine phosphorylation and interacts with caveolin-1. J. Biol. Chem. 271, 27237–27240. doi:10.1074/jbc.271.44.27237
Garcia-Gonzalez, M. A., Outeda, P., Zhou, Q., Zhou, F., Menezes, L. F., Qian, F., et al. (2010). Pkd1 and Pkd2 are required for normal placental development. PLoS One 5, e12821. doi:10.1371/journal.pone.0012821
Garcia-Roldan, J. L., and Bevan, J. A. (1990). Flow-induced constriction and dilation of cerebral resistance arteries. Circulation Res. 66, 1445–1448. doi:10.1161/01.RES.66.5.1445
Garry, A., Fromy, B., Blondeau, N., Henrion, D., Brau, F., Gounon, P., et al. (2007). Altered acetylcholine, bradykinin and cutaneous pressure-induced vasodilation in mice lacking the TREK1 potassium channel: the endothelial link. EMBO Rep. 8, 354–359. doi:10.1038/sj.embor.7400916
Garty, H., and Palmer, L. G. (1997). Epithelial sodium channels: function, structure, and regulation. Physiol. Rev. 77, 359–396. doi:10.1152/physrev.1997.77.2.359
Geiger, B., Spatz, J. P., and Bershadsky, A. D. (2009). Environmental sensing through focal adhesions. Nat. Rev. Mol. Cell Biol. 10, 21–33. doi:10.1038/nrm2593
Geiger, B., and Yamada, K. M. (2011). Molecular architecture and function of matrix adhesions. Cold Spring Harb. Perspect. Biol. 3, a005033. doi:10.1101/cshperspect.a005033
Gelfand, M. V., Hagan, N., Tata, A., Oh, W.-J., Lacoste, B., Kang, K.-T., et al. (2014). Neuropilin-1 functions as a VEGFR2 co-receptor to guide developmental angiogenesis independent of ligand binding. eLife 3, e03720. doi:10.7554/eLife.03720
Giddens, D. P., Zarins, C. K., and Glagov, S. (1993). The role of fluid mechanics in the localization and detection of atherosclerosis. J. Biomechanical Eng. 115, 588–594. doi:10.1115/1.2895545
Gifre-Renom, L., Daems, M., Luttun, A., and Jones, E. A. V. (2022). Organ-specific endothelial cell differentiation and impact of microenvironmental cues on endothelial heterogeneity. IJMS 23, 1477. doi:10.3390/ijms23031477
Gijsen, F., Katagiri, Y., Barlis, P., Bourantas, C., Collet, C., Coskun, U., et al. (2019). Expert recommendations on the assessment of wall shear stress in human coronary arteries: existing methodologies, technical considerations, and clinical applications. Eur. Heart J. 40, 3421–3433. doi:10.1093/eurheartj/ehz551
Gimbrone, M. A., Anderson, K. R., Topper, J. N., Langille, B. L., Clowes, A. W., Bercel, S., et al. (1999). Special communicationthe critical role of mechanical forces in blood vessel development, physiology and pathology. J. Vasc. Surg. 29, 1104–1151. doi:10.1016/S0741-5214(99)70252-1
Goel, R., Schrank, B. R., Arora, S., Boylan, B., Fleming, B., Miura, H., et al. (2008). Site-specific effects of PECAM-1 on atherosclerosis in LDL receptor–deficient mice. ATVB 28, 1996–2002. doi:10.1161/ATVBAHA.108.172270
Goetz, J. G., Steed, E., Ferreira, R. R., Roth, S., Ramspacher, C., Boselli, F., et al. (2014). Endothelial cilia mediate low flow sensing during zebrafish vascular development. Cell Rep. 6, 799–808. doi:10.1016/j.celrep.2014.01.032
Gomez, D., Swiatlowska, P., and Owens, G. K. (2015). Epigenetic control of smooth muscle cell identity and lineage memory. ATVB 35, 2508–2516. doi:10.1161/ATVBAHA.115.305044
Gosens, R., Stelmack, G. L., Bos, S. T., Dueck, G., Mutawe, M. M., Schaafsma, D., et al. (2011). Caveolin-1 is required for contractile phenotype expression by airway smooth muscle cells. J. Cell. Mol. Med. 15, 2430–2442. doi:10.1111/j.1582-4934.2010.01246.x
Goveia, J., Rohlenova, K., Taverna, F., Treps, L., Conradi, L.-C., Pircher, A., et al. (2020). An integrated gene expression landscape profiling approach to identify lung tumor endothelial cell heterogeneity and angiogenic candidates. Cancer Cell 37, 421–436.e13. doi:10.1016/j.ccell.2020.03.002
Goveia, J., Stapor, P., and Carmeliet, P. (2014). Principles of targeting endothelial cell metabolism to treat angiogenesis and endothelial cell dysfunction in disease. EMBO Mol. Med. 6, 1105–1120. doi:10.15252/emmm.201404156
Grassmé, H., Henry, B., Ziobro, R., Becker, K. A., Riethmüller, J., Gardner, A., et al. (2017). β1-Integrin accumulates in cystic fibrosis luminal airway epithelial membranes and decreases sphingosine, promoting bacterial infections. Cell Host and Microbe 21, 707–718. doi:10.1016/j.chom.2017.05.001
Greenberg, H. Z. E., Carlton-Carew, S. R. E., Khan, D. M., Zargaran, A. K., Jahan, K. S., Vanessa Ho, W.-S., et al. (2017). Heteromeric TRPV4/TRPC1 channels mediate calcium-sensing receptor-induced nitric oxide production and vasorelaxation in rabbit mesenteric arteries. Vasc. Pharmacol. 96–98, 53–62. doi:10.1016/j.vph.2017.08.005
Greenberg, H. Z. E., Carlton-Carew, S. R. E., Zargaran, A. K., Jahan, K. S., Birnbaumer, L., and Albert, A. P. (2019). Heteromeric TRPV4/TRPC1 channels mediate calcium-sensing receptor-induced relaxations and nitric oxide production in mesenteric arteries: comparative study using wild-type and TRPC1 −/- mice. Channels 13, 410–423. doi:10.1080/19336950.2019.1673131
Greißel, A., Culmes, M., Burgkart, R., Zimmermann, A., Eckstein, H.-H., Zernecke, A., et al. (2016). Histone acetylation and methylation significantly change with severity of atherosclerosis in human carotid plaques. Cardiovasc. Pathol. 25, 79–86. doi:10.1016/j.carpath.2015.11.001
Greißel, A., Culmes, M., Napieralski, R., Wagner, E., Gebhard, H., Schmitt, M., et al. (2015). Alternation of histone and DNA methylation in human atherosclerotic carotid plaques. Thromb. Haemost. 114, 390–402. doi:10.1160/TH14-10-0852
Gudi, S., Huvar, I., White, C. R., McKnight, N. L., Dusserre, N., Boss, G. R., et al. (2003). Rapid activation of ras by fluid flow is mediated by gα q and gβγ subunits of heterotrimeric G proteins in human endothelial cells. ATVB 23, 994–1000. doi:10.1161/01.ATV.0000073314.51987.84
Gudi, S., Nolan, J. P., and Frangos, J. A. (1998). Modulation of GTPase activity of G proteins by fluid shear stress and phospholipid composition. Proc. Natl. Acad. Sci. U. S. A. 95, 2515–2519. doi:10.1073/pnas.95.5.2515
Guharay, F., and Sachs, F. (1984). Stretch-activated single ion channel currents in tissue-cultured embryonic chick skeletal muscle. J. Physiology 352, 685–701. doi:10.1113/jphysiol.1984.sp015317
Gulino-Debrac, D. (2013). Mechanotransduction at the basis of endothelial barrier function. Tissue Barriers 1, e24180. doi:10.4161/tisb.24180
Guo, D., Liang, S., Wang, S., Tang, C., Yao, B., Wan, W., et al. (2015). Role of epithelial sodium channels (ENaCs) in endothelial function. J. Cell Sci., jcs168831. doi:10.1242/jcs.168831
Guo, Y. R., and MacKinnon, R. (2017). Structure-based membrane dome mechanism for Piezo mechanosensitivity. eLife 6, e33660. doi:10.7554/eLife.33660
Gupta, A., Thirugnanam, K., Thamilarasan, M., Mohieldin, A. M., Zedan, H. T., Prabhudesai, S., et al. (2022). Cilia proteins are biomarkers of altered flow in the vasculature. JCI Insight 7 (6), e151813. doi:10.1172/jci.insight.151813
Gurney, A., and Manoury, B. (2009). Two-pore potassium channels in the cardiovascular system. Eur. Biophys. J. 38, 305–318. doi:10.1007/s00249-008-0326-8
Hahn, C., Orr, A. W., Sanders, J. M., Jhaveri, K. A., and Schwartz, M. A. (2009). The subendothelial extracellular matrix modulates JNK activation by flow. Circulation Res. 104, 995–1003. doi:10.1161/CIRCRESAHA.108.186486
Hahn, C., and Schwartz, M. A. (2009). Mechanotransduction in vascular physiology and atherogenesis. Nat. Rev. Mol. Cell Biol. 10, 53–62. doi:10.1038/nrm2596
Hamik, A., Lin, Z., Kumar, A., Balcells, M., Sinha, S., Katz, J., et al. (2007). Kruppel-like factor 4 regulates endothelial inflammation. J. Biol. Chem. 282, 13769–13779. doi:10.1074/jbc.M700078200
Han, H., Rosenhouse-Dantsker, A., Gnanasambandam, R., Epshtein, Y., Chen, Z., Sachs, F., et al. (2014). Silencing of Kir2 channels by caveolin-1: cross-talk with cholesterol. J. Physiology 592, 4025–4038. doi:10.1113/jphysiol.2014.273177
Harding, I. C., Mitra, R., Mensah, S. A., Herman, I. M., and Ebong, E. E. (2018). Pro-atherosclerotic disturbed flow disrupts caveolin-1 expression, localization, and function via glycocalyx degradation. J. Transl. Med. 16, 364. doi:10.1186/s12967-018-1721-2
Harman, J. L., Dobnikar, L., Chappell, J., Stokell, B. G., Dalby, A., Foote, K., et al. (2019). Epigenetic regulation of vascular smooth muscle cells by histone H3 lysine 9 dimethylation attenuates target gene-induction by inflammatory signaling. ATVB 39, 2289–2302. doi:10.1161/ATVBAHA.119.312765
Harraz, O. F., Klug, N. R., Senatore, A. J., Hill-Eubanks, D. C., and Nelson, M. T. (2022). Piezo1 is a mechanosensor channel in central nervous system capillaries. Circulation Res. 130, 1531–1546. doi:10.1161/CIRCRESAHA.122.320827
Harraz, O. F., Longden, T. A., Dabertrand, F., Hill-Eubanks, D., and Nelson, M. T. (2018a). Endothelial GqPCR activity controls capillary electrical signaling and brain blood flow through PIP 2 depletion. Proc. Natl. Acad. Sci. U.S.A. 115, E3569–E3577. doi:10.1073/pnas.1800201115
Harraz, O. F., Longden, T. A., Hill-Eubanks, D., and Nelson, M. T. (2018b). PIP2 depletion promotes TRPV4 channel activity in mouse brain capillary endothelial cells. eLife 7, e38689. doi:10.7554/eLife.38689
Harrison, D. G., Widder, J., Grumbach, I., Chen, W., Weber, M., and Searles, C. (2006). Endothelial mechanotransduction, nitric oxide and vascular inflammation. J. Intern Med. 259, 351–363. doi:10.1111/j.1365-2796.2006.01621.x
Harrison, M., Smith, E., Ross, E., Krams, R., Segers, D., Buckley, C. D., et al. (2013). The role of platelet-endothelial cell adhesion molecule-1 in atheroma formation varies depending on the site-specific hemodynamic environment. Arterioscler. Thromb. Vasc. Biol. 33, 694–701. doi:10.1161/ATVBAHA.112.300379
Harry, B. L., Sanders, J. M., Feaver, R. E., Lansey, M., Deem, T. L., Zarbock, A., et al. (2008). Endothelial cell PECAM-1 promotes atherosclerotic lesions in areas of disturbed flow in ApoE-deficient mice. ATVB 28, 2003–2008. doi:10.1161/ATVBAHA.108.164707
Hartmannsgruber, V., Heyken, W.-T., Kacik, M., Kaistha, A., Grgic, I., Harteneck, C., et al. (2007). Arterial response to shear stress critically depends on endothelial TRPV4 expression. PLoS ONE 2, e827. doi:10.1371/journal.pone.0000827
Hasegawa, T., Takeuchi, A., Miyaishi, O., Xiao, H., Mao, J., and Isobe, K. (2000). PTRF (polymerase I and transcript-release factor) is tissue-specific and interacts with the BFCOL1 (binding factor of a type-I collagen promoter) zinc-finger transcription factor which binds to the two mouse type-I collagen gene promoters. Biochem. J. 347, 55–59. doi:10.1042/0264-6021:3470055
Hatem, A., Poussereau, G., Gachenot, M., Pérès, L., Bouyer, G., and Egée, S. (2023). Dual action of Dooku1 on PIEZO1 channel in human red blood cells. Front. Physiol. 14, 1222983. doi:10.3389/fphys.2023.1222983
He, M., Huang, T.-S., Li, S., Hong, H.-C., Chen, Z., Martin, M., et al. (2019). Atheroprotective flow upregulates ITPR3 (inositol 1,4,5-trisphosphate receptor 3) in vascular endothelium via KLF4 (Krüppel-Like factor 4)-mediated histone modifications. ATVB 39, 902–914. doi:10.1161/ATVBAHA.118.312301
He, S., Yang, L., Li, D., and Li, M. (2015). Kruppel-like factor 2-mediated suppression of MicroRNA-155 reduces the proinflammatory activation of macrophages. PLoS One 10, e0139060. doi:10.1371/journal.pone.0139060
Heathcote, H. R., Lee, M. D., Zhang, X., Saunter, C. D., Wilson, C., and McCarron, J. G. (2019). Endothelial TRPV4 channels modulate vascular tone by Ca2+ -induced Ca2+ release at inositol 1,4,5-trisphosphate receptors. Br. J. Pharmacol. 176, 3297–3317. doi:10.1111/bph.14762
Heinolainen, K., Karaman, S., D’Amico, G., Tammela, T., Sormunen, R., Eklund, L., et al. (2017). VEGFR3 modulates vascular permeability by controlling VEGF/VEGFR2 signaling. Circulation Res. 120, 1414–1425. doi:10.1161/CIRCRESAHA.116.310477
Hergenreider, E., Heydt, S., Tréguer, K., Boettger, T., Horrevoets, A. J. G., Zeiher, A. M., et al. (2012). Atheroprotective communication between endothelial cells and smooth muscle cells through miRNAs. Nat. Cell Biol. 14, 249–256. doi:10.1038/ncb2441
Hibino, H., Inanobe, A., Furutani, K., Murakami, S., Findlay, I., and Kurachi, Y. (2010). Inwardly rectifying potassium channels: their structure, function, and physiological roles. Physiol. Rev. 90, 291–366. doi:10.1152/physrev.00021.2009
Hierck, B. P., Van Der Heiden, K., Alkemade, F. E., Van De Pas, S., Van Thienen, J. V., Groenendijk, B. C. W., et al. (2008). Primary cilia sensitize endothelial cells for fluid shear stress. Dev. Dyn. 237, 725–735. doi:10.1002/dvdy.21472
Hill, M. A., and Davis, M. J. (2007). Coupling a change in intraluminal pressure to vascular smooth muscle depolarization: still stretching for an explanation. Am. J. Physiology-Heart Circulatory Physiology 292, H2570–H2572. doi:10.1152/ajpheart.00331.2007
Hill, M. A., Zou, H., Potocnik, S. J., Meininger, G. A., and Davis, M. J. (2001). Invited Review: arteriolar smooth muscle mechanotransduction: Ca 2+ signaling pathways underlying myogenic reactivity. J. Appl. Physiology 91, 973–983. doi:10.1152/jappl.2001.91.2.973
Hill-Eubanks, D. C., Gonzales, A. L., Sonkusare, S. K., and Nelson, M. T. (2014). Vascular TRP channels: performing under pressure and going with the flow. Physiology 29, 343–360. doi:10.1152/physiol.00009.2014
Hoefer, I. E., Den Adel, B., and Daemen, M. J. A. P. (2013). Biomechanical factors as triggers of vascular growth. Cardiovasc. Res. 99, 276–283. doi:10.1093/cvr/cvt089
Hofmann, T., Obukhov, A. G., Schaefer, M., Harteneck, C., Gudermann, T., and Schultz, G. (1999). Direct activation of human TRPC6 and TRPC3 channels by diacylglycerol. Nature 397, 259–263. doi:10.1038/16711
Hoger, J. H., Ilyin, V. I., Forsyth, S., and Hoger, A. (2002). Shear stress regulates the endothelial Kir2.1 ion channel. Proc. Natl. Acad. Sci. U. S. A. 99, 7780–7785. doi:10.1073/pnas.102184999
Holz, M., Heil, S. R., and Sacco, A. (2000). Temperature-dependent self-diffusion coefficients of water and six selected molecular liquids for calibration in accurate 1H NMR PFG measurements. Phys. Chem. Chem. Phys. 2, 4740–4742. doi:10.1039/b005319h
Honoré, E. (2007). The neuronal background K2P channels: focus on TREK1. Nat. Rev. Neurosci. 8, 251–261. doi:10.1038/nrn2117
Honoré, E., Patel, A. J., Chemin, J., Suchyna, T., and Sachs, F. (2006). Desensitization of mechano-gated K2P channels. Proc. Natl. Acad. Sci. U. S. A. 103, 6859–6864. doi:10.1073/pnas.0600463103
Hsieh, H. J., Cheng, C. C., Wu, S. T., Chiu, J. J., Wung, B. S., and Wang, D. L. (1998). Increase of reactive oxygen species (ROS) in endothelial cells by shear flow and involvement of ROS in shear-induced c-fos expression. J. Cell Physiol. 175, 156–162. doi:10.1002/(SICI)1097-4652(199805)175:2<156::AID-JCP5>3.0.CO;2-N
Hsu, P.-L., Chen, J.-S., Wang, C.-Y., Wu, H.-L., and Mo, F.-E. (2019). Shear-induced CCN1 promotes atheroprone endothelial phenotypes and atherosclerosis. Circulation 139, 2877–2891. doi:10.1161/CIRCULATIONAHA.118.033895
Hu, Y., Chen, M., Wang, M., and Li, X. (2022). Flow-mediated vasodilation through mechanosensitive G protein-coupled receptors in endothelial cells. Trends Cardiovasc. Med. 32, 61–70. doi:10.1016/j.tcm.2020.12.010
Huang, C., Hepler, J. R., Chen, L. T., Gilman, A. G., Anderson, R. G. W., and Mumby, S. M. (1997). Organization of G Proteins and adenylyl cyclase at the plasma membrane. MBoC 8, 2365–2378. doi:10.1091/mbc.8.12.2365
Huang, L., Nakayama, H., Klagsbrun, M., Mulliken, J. B., and Bischoff, J. (2015). Glucose transporter 1-positive endothelial cells in infantile hemangioma exhibit features of facultative stem cells. Stem Cells 33, 133–145. doi:10.1002/stem.1841
Huang, M., Liu, T., Ma, P., Mitteer, R. A., Zhang, Z., Kim, H. J., et al. (2016). c-Met-mediated endothelial plasticity drives aberrant vascularization and chemoresistance in glioblastoma. J. Clin. Invest. 126, 1801–1814. doi:10.1172/JCI84876
Huang, X., Shi, X., Hansen, M. E., Setiady, I., Nemeth, C. L., Celli, A., et al. (2020). Nanotopography enhances dynamic remodeling of tight junction proteins through cytosolic liquid complexes. ACS Nano 14, 13192–13202. doi:10.1021/acsnano.0c04866
Humphrey, J. D. (2021). Mechanisms of vascular remodeling in hypertension. Am. J. Hypertens. 34, 432–441. doi:10.1093/ajh/hpaa195
Humphries, J. D., Chastney, M. R., Askari, J. A., and Humphries, M. J. (2019). Signal transduction via integrin adhesion complexes. Curr. Opin. Cell Biol. 56, 14–21. doi:10.1016/j.ceb.2018.08.004
Hyman, A. J., Tumova, S., and Beech, D. J. (2017). “Piezo1 channels in vascular development and the sensing of shear stress,” in Current topics in membranes (Elsevier), 37–57. doi:10.1016/bs.ctm.2016.11.001
Hynes, R. O., Lively, J. C., Mccarty, J. H., Taverna, D., Francis, S. E., Hodivala-Dilke, K., et al. (2002). The diverse roles of integrins and their ligands in angiogenesis. Cold Spring Harb. Symposia Quantitative Biol. 67, 143–153. doi:10.1101/sqb.2002.67.143
Ichioka, S., Shibata, M., Kosaki, K., Sato, Y., Harii, K., and Kamiya, A. (1997). Effects of shear stress on wound-healing angiogenesis in the rabbit ear chamber. J. Surg. Res. 72, 29–35. doi:10.1006/jsre.1997.5170
Ilatovskaya, D. V., Pavlov, T. S., Levchenko, V., Negulyaev, Y. A., and Staruschenko, A. (2011). Cortical actin binding protein cortactin mediates ENaC activity via Arp2/3 complex. FASEB J. 25, 2688–2699. doi:10.1096/fj.10-167262
Ingber, D. E. (2006). Cellular mechanotransduction: putting all the pieces together again. FASEB J. 20, 811–827. doi:10.1096/fj.05-5424rev
Inoue, R., Jensen, L. J., Jian, Z., Shi, J., Hai, L., Lurie, A. I., et al. (2009a). Synergistic activation of vascular TRPC6 channel by receptor and mechanical stimulation via phospholipase C/diacylglycerol and phospholipase A 2/ω-Hydroxylase/20-HETE pathways. Circulation Res. 104, 1399–1409. doi:10.1161/CIRCRESAHA.108.193227
Inoue, R., Jian, Z., and Kawarabayashi, Y. (2009b). Mechanosensitive TRP channels in cardiovascular pathophysiology. Pharmacol. and Ther. 123, 371–385. doi:10.1016/j.pharmthera.2009.05.009
Iomini, C., Tejada, K., Mo, W., Vaananen, H., and Piperno, G. (2004). Primary cilia of human endothelial cells disassemble under laminar shear stress. J. Cell Biol. 164, 811–817. doi:10.1083/jcb.200312133
Islam, S., Boström, K. I., Di Carlo, D., Simmons, C. A., Tintut, Y., Yao, Y., et al. (2021). The mechanobiology of endothelial-to-mesenchymal transition in cardiovascular disease. Front. Physiol. 12, 734215. doi:10.3389/fphys.2021.734215
Izzard, A. S., Graham, D., Burnham, M. P., Heerkens, E. H., Dominiczak, A. F., and Heagerty, A. M. (2003). Myogenic and structural properties of cerebral arteries from the stroke-prone spontaneously hypertensive rat. Am. J. Physiology-Heart Circulatory Physiology 285, H1489–H1494. doi:10.1152/ajpheart.00352.2003
Jackson, W. F. (2022). Endothelial ion channels and cell-cell communication in the microcirculation. Front. Physiol. 13, 805149. doi:10.3389/fphys.2022.805149
Jacobs, E. R., Cheliakine, C., Gebremedhin, D., Birks, E. K., Davies, P. F., and Harder, D. R. (1995). Shear activated channels in cell-attached patches of cultured bovine aortic endothelial cells. Pflugers Arch. 431, 129–131. doi:10.1007/BF00374386
Jaminon, A., Reesink, K., Kroon, A., and Schurgers, L. (2019). The role of vascular smooth muscle cells in arterial remodeling: focus on calcification-related processes. IJMS 20, 5694. doi:10.3390/ijms20225694
Jernigan, N. L., and Drummond, H. A. (2005). Vascular ENaC proteins are required for renal myogenic constriction. Am. J. Physiology-Renal Physiology 289, F891–F901. doi:10.1152/ajprenal.00019.2005
Jia, M., Li, Q., Guo, J., Shi, W., Zhu, L., Huang, Y., et al. (2022). Deletion of BACH1 attenuates atherosclerosis by reducing endothelial inflammation. Circulation Res. 130, 1038–1055. doi:10.1161/CIRCRESAHA.121.319540
Jiang, W., Agrawal, D., and Boosani, C. (2018). Cell-specific histone modifications in atherosclerosis (Review). Mol. Med. Rep. 18, 1215–1224. doi:10.3892/mmr.2018.9142
Jiang, Y., Yang, X., Jiang, J., and Xiao, B. (2021). Structural designs and mechanogating mechanisms of the mechanosensitive piezo channels. Trends Biochem. Sci. 46, 472–488. doi:10.1016/j.tibs.2021.01.008
Jin, Z.-G., Ueba, H., Tanimoto, T., Lungu, A. O., Frame, M. D., and Berk, B. C. (2003). Ligand-independent activation of vascular endothelial growth factor receptor 2 by fluid shear stress regulates activation of endothelial nitric oxide synthase. Circulation Res. 93, 354–363. doi:10.1161/01.RES.0000089257.94002.96
Joannides, R., Haefeli, W. E., Linder, L., Richard, V., Bakkali, E. H., Thuillez, C., et al. (1995). Nitric oxide is responsible for flow-dependent dilatation of human peripheral conduit arteries in vivo. Circulation 91, 1314–1319. doi:10.1161/01.CIR.91.5.1314
John, L., Ko, N. L., Gokin, A., Gokina, N., Mandalà, M., and Osol, G. (2018). The Piezo1 cation channel mediates uterine artery shear stress mechanotransduction and vasodilation during rat pregnancy. Am. J. Physiol. Heart Circ. Physiol. 315, H1019–H1026. doi:10.1152/ajpheart.00103.2018
Jones, S. A., Giddens, D. P., Loth, F., Zarins, C. K., Kajiya, F., Morita, I., et al. (1997). In-Vivo measurements of blood flow velocity profiles in canine ilio-femoral anastomotic bypass grafts. J. Biomechanical Eng. 119, 30–38. doi:10.1115/1.2796061
Jongstra-Bilen, J., Haidari, M., Zhu, S.-N., Chen, M., Guha, D., and Cybulsky, M. I. (2006). Low-grade chronic inflammation in regions of the normal mouse arterial intima predisposed to atherosclerosis. J. Exp. Med. 203, 2073–2083. doi:10.1084/jem.20060245
Jung, B., Obinata, H., Galvani, S., Mendelson, K., Ding, B., Skoura, A., et al. (2012). Flow-regulated endothelial S1P receptor-1 signaling sustains vascular development. Dev. Cell 23, 600–610. doi:10.1016/j.devcel.2012.07.015
Just, A., Whitten, C. L., and Arendshorst, W. J. (2008). Reactive oxygen species participate in acute renal vasoconstrictor responses induced by ET A and ET B receptors. Am. J. Physiology-Renal Physiology 294, F719–F728. doi:10.1152/ajprenal.00506.2007
Kadry, Y. A., and Calderwood, D. A. (2020). Chapter 22: structural and signaling functions of integrins. Biochimica Biophysica Acta (BBA) - Biomembr. 1862, 183206. doi:10.1016/j.bbamem.2020.183206
Kallakuri, S., Yu, J. A., Li, J., Li, Y., Weinstein, B. M., Nicoli, S., et al. (2015). Endothelial cilia are essential for developmental vascular integrity in zebrafish. J. Am. Soc. Nephrol. 26, 864–875. doi:10.1681/ASN.2013121314
Kang, H., Hong, Z., Zhong, M., Klomp, J., Bayless, K. J., Mehta, D., et al. (2019). Piezo1 mediates angiogenesis through activation of MT1-MMP signaling. Am. J. Physiology-Cell Physiology 316, C92–C103. doi:10.1152/ajpcell.00346.2018
Kanzaki, M., Zhang, Y.-Q., Mashima, H., Li, L., Shibata, H., and Kojima, I. (1999). Translocation of a calcium-permeable cation channel induced by insulin-like growth factor-I. Nat. Cell Biol. 1, 165–170. doi:10.1038/11086
Katoh, K. (2023). Effects of mechanical stress on endothelial cells in situ and in vitro. IJMS 24, 16518. doi:10.3390/ijms242216518
Kellenberger, S., and Schild, L. (2002). Epithelial sodium channel/degenerin family of ion channels: a variety of functions for a shared structure. Physiol. Rev. 82, 735–767. doi:10.1152/physrev.00007.2002
Kendall, R. L., and Thomas, K. A. (1993). Inhibition of vascular endothelial cell growth factor activity by an endogenously encoded soluble receptor. Proc. Natl. Acad. Sci. U.S.A. 90, 10705–10709. doi:10.1073/pnas.90.22.10705
Khachigian, L. M., Anderson, K. R., Halnon, N. J., Gimbrone, M. A., Resnick, N., and Collins, T. (1997). Egr-1 is activated in endothelial cells exposed to fluid shear stress and interacts with a novel shear-stress-response element in the PDGF A-chain promoter. ATVB 17, 2280–2286. doi:10.1161/01.ATV.17.10.2280
Khan, A. W., Paneni, F., and Jandeleit-Dahm, K. A. M. (2021). Cell-specific epigenetic changes in atherosclerosis. Clin. Sci. 135, 1165–1187. doi:10.1042/CS20201066
Kimura, H. (2013). Histone modifications for human epigenome analysis. J. Hum. Genet. 58, 439–445. doi:10.1038/jhg.2013.66
Kirby, B. S., Carlson, R. E., Markwald, R. R., Voyles, W. F., and Dinenno, F. A. (2007). Mechanical influences on skeletal muscle vascular tone in humans: insight into contraction-induced rapid vasodilatation. J. Physiology 583, 861–874. doi:10.1113/jphysiol.2007.131250
Klann, J. E., Remedios, K. A., Kim, S. H., Metz, P. J., Lopez, J., Mack, L. A., et al. (2017). Talin plays a critical role in the maintenance of the regulatory T cell pool. J. Immunol. 198, 4639–4651. doi:10.4049/jimmunol.1601165
Knot, H. J., and Nelson, M. T. (1998). Regulation of arterial diameter and wall [Ca 2+ ] in cerebral arteries of rat by membrane potential and intravascular pressure. J. Physiology 508, 199–209. doi:10.1111/j.1469-7793.1998.199br.x
Koch, S., and Claesson-Welsh, L. (2012). Signal transduction by vascular endothelial growth factor receptors. Cold Spring Harb. Perspect. Med. 2, a006502. doi:10.1101/cshperspect.a006502
Köhler, R., Heyken, W.-T., Heinau, P., Schubert, R., Si, H., Kacik, M., et al. (2006). Evidence for a functional role of endothelial transient receptor potential V4 in shear stress–induced vasodilatation. ATVB 26, 1495–1502. doi:10.1161/01.ATV.0000225698.36212.6a
Koide, M., Harraz, O. F., Dabertrand, F., Longden, T. A., Ferris, H. R., Wellman, G. C., et al. (2021). Differential restoration of functional hyperemia by antihypertensive drug classes in hypertension-related cerebral small vessel disease. J. Clin. Invest. 131, e149029. doi:10.1172/JCI149029
Kolářová, H., Ambrůzová, B., Švihálková Šindlerová, L., Klinke, A., and Kubala, L. (2014). Modulation of endothelial glycocalyx structure under inflammatory conditions. Mediat. Inflamm. 2014, 694312–694317. doi:10.1155/2014/694312
Kong, F., García, A. J., Mould, A. P., Humphries, M. J., and Zhu, C. (2009). Demonstration of catch bonds between an integrin and its ligand. J. Cell Biol. 185, 1275–1284. doi:10.1083/jcb.200810002
Koutsiaris, A. G., Tachmitzi, S. V., and Batis, N. (2013). Wall shear stress quantification in the human conjunctival pre-capillary arterioles in vivo. Microvasc. Res. 85, 34–39. doi:10.1016/j.mvr.2012.11.003
Kovacic, J. C., Dimmeler, S., Harvey, R. P., Finkel, T., Aikawa, E., Krenning, G., et al. (2019). Endothelial to mesenchymal transition in cardiovascular disease: JACC state-of-the-art review. J. Am. Coll. Cardiol. 73, 190–209. doi:10.1016/j.jacc.2018.09.089
Kress, M., Karasek, J., Ferrer-Montiel, A. V., Scherbakov, N., and Haberberger, R. V. (2008). TRPC channels and diacylglycerol dependent calcium signaling in rat sensory neurons. Histochem Cell Biol. 130, 655–667. doi:10.1007/s00418-008-0477-9
Kršek, A., Batičić, L., Ćurko-Cofek, B., Batinac, T., Laškarin, G., Miletić-Gršković, S., et al. (2024). Insights into the molecular mechanism of endothelial glycocalyx dysfunction during heart surgery. CIMB 46, 3794–3809. doi:10.3390/cimb46050236
Kubes, P., Suzuki, M., and Granger, D. N. (1991). Nitric oxide: an endogenous modulator of leukocyte adhesion. Proc. Natl. Acad. Sci. U.S.A. 88, 4651–4655. doi:10.1073/pnas.88.11.4651
Kurozumi, A., Goto, Y., Matsushita, R., Fukumoto, I., Kato, M., Nishikawa, R., et al. (2016). Tumor-suppressive micro RNA -223 inhibits cancer cell migration and invasion by targeting ITGA 3/ITGB 1 signaling in prostate cancer. Cancer Sci. 107, 84–94. doi:10.1111/cas.12842
Lai, A., Cox, C. D., Chandra Sekar, N., Thurgood, P., Jaworowski, A., Peter, K., et al. (2022). Mechanosensing by Piezo1 and its implications for physiology and various pathologies. Biol. Rev. 97, 604–614. doi:10.1111/brv.12814
Lambert, S., and Bennett, V. (1993). From anemia to cerebellar dysfunction: a review of the ankyrin gene family. Eur. J. Biochem. 211, 1–6. doi:10.1111/j.1432-1033.1993.tb19863.x
Lan, Q., Mercurius, K. O., and Davies, P. F. (1994). Stimulation of transcription factors NF kappa B and AP1 in endothelial cells subjected to shear stress. Biochem. Biophys. Res. Commun. 201, 950–956. doi:10.1006/bbrc.1994.1794
Laurent, S., and Boutouyrie, P. (2020). Arterial stiffness and hypertension in the elderly. Front. Cardiovasc. Med. 7, 544302. doi:10.3389/fcvm.2020.544302
LaValley, D. J., Zanotelli, M. R., Bordeleau, F., Wang, W., Schwager, S. C., and Reinhart-King, C. A. (2017). Matrix stiffness enhances VEGFR-2 internalization, signaling, and proliferation in endothelial cells. Converg. Sci. Phys. Oncol. 3, 044001. doi:10.1088/2057-1739/aa9263
Lawrence, M., Daujat, S., and Schneider, R. (2016). Lateral thinking: how histone modifications regulate gene expression. Trends Genet. 32, 42–56. doi:10.1016/j.tig.2015.10.007
Lee, H. J., Cho, C.-H., Hwang, S.-J., Choi, H.-H., Kim, K.-T., Ahn, S. Y., et al. (2004). Biological characterization of angiopoietin-3 and angiopoietin-4. FASEB J. 18, 1200–1208. doi:10.1096/fj.03-1466com
Lehoux, S., and Tedgui, A. (1998). Signal transduction of mechanical stresses in the vascular wall. Hypertension 32, 338–345. doi:10.1161/01.HYP.32.2.338
Lei, L., Liu, D., Huang, Y., Jovin, I., Shai, S.-Y., Kyriakides, T., et al. (2008). Endothelial expression of beta1 integrin is required for embryonic vascular patterning and postnatal vascular remodeling. Mol. Cell. Biol. 28, 794–802. doi:10.1128/MCB.00443-07
Leitinger, B. (2014). “Discoidin domain receptor functions in physiological and pathological conditions,” in International review of cell and molecular biology (Elsevier), 39–87. doi:10.1016/B978-0-12-800180-6.00002-5
Lembo, S., Strauss, L., Cheng, D., Vermeil, J., Siggel, M., Toro-Nahuelpan, M., et al. (2023). The distance between the plasma membrane and the actomyosin cortex acts as a nanogate to control cell surface mechanics. Biophysics. doi:10.1101/2023.01.31.526409
Lesage, F., Terrenoire, C., Romey, G., and Lazdunski, M. (2000). Human TREK2, a 2P domain mechano-sensitive K+ channel with multiple regulations by polyunsaturated fatty acids, lysophospholipids, and Gs, Gi, and Gq protein-coupled receptors. J. Biol. Chem. 275, 28398–28405. doi:10.1074/jbc.M002822200
Letcher, R. L., Chien, S., Pickering, T. G., Sealey, J. E., and Laragh, J. H. (1981). Direct relationship between blood pressure and blood viscosity in normal and hypertensive subjects. Role of fibrinogen and concentration. Am. J. Med. 70, 1195–1202. doi:10.1016/0002-9343(81)90827-5
Leung, P.-C., Cheng, K.-T., Liu, C., Cheung, W.-T., Kwan, H.-Y., Lau, K.-L., et al. (2006). Mechanism of non-capacitative Ca2+ influx in response to bradykinin in vascular endothelial cells. J. Vasc. Res. 43, 367–376. doi:10.1159/000094096
Lewis, A. H., and Grandl, J. (2015). Mechanical sensitivity of Piezo1 ion channels can be tuned by cellular membrane tension. eLife 4, e12088. doi:10.7554/eLife.12088
Lhomme, A., Gilbert, G., Pele, T., Deweirdt, J., Henrion, D., Baudrimont, I., et al. (2019). Stretch-activated Piezo1 channel in endothelial cells relaxes mouse intrapulmonary arteries. Am. J. Respir. Cell Mol. Biol. 60, 650–658. doi:10.1165/rcmb.2018-0197OC
Li, B., He, J., Lv, H., Liu, Y., Lv, X., Zhang, C., et al. (2019). c-Abl regulates YAPY357 phosphorylation to activate endothelial atherogenic responses to disturbed flow. J. Clin. Investigation 129, 1167–1179. doi:10.1172/JCI122440
Li, H., Zhou, W.-Y., Xia, Y.-Y., and Zhang, J.-X. (2022). Endothelial mechanosensors for atheroprone and atheroprotective shear stress signals. JIR 15, 1771–1783. doi:10.2147/JIR.S355158
Li, J., Hou, B., Tumova, S., Muraki, K., Bruns, A., Ludlow, M. J., et al. (2014). Piezo1 integration of vascular architecture with physiological force. Nature 515, 279–282. doi:10.1038/nature13701
Li, J., and Springer, T. A. (2017). Integrin extension enables ultrasensitive regulation by cytoskeletal force. Proc. Natl. Acad. Sci. U.S.A. 114, 4685–4690. doi:10.1073/pnas.1704171114
Li, R., Maminishkis, A., Zahn, G., Vossmeyer, D., and Miller, S. S. (2009). Integrin alpha5beta1 mediates attachment, migration, and proliferation in human retinal pigment epithelium: relevance for proliferative retinal disease. Invest. Ophthalmol. Vis. Sci. 50, 5988–5996. doi:10.1167/iovs.09-3591
Li, S., Okamoto, T., Chun, M., Sargiacomo, M., Casanova, J. E., Hansen, S. H., et al. (1995). Evidence for a regulated interaction between heterotrimeric G proteins and caveolin. J. Biol. Chem. 270, 15693–15701. doi:10.1074/jbc.270.26.15693
Li, Y., Lui, K. O., and Zhou, B. (2018a). Reassessing endothelial-to-mesenchymal transition in cardiovascular diseases. Nat. Rev. Cardiol. 15, 445–456. doi:10.1038/s41569-018-0023-y
Li, Y., Zheng, J., Bird, I. M., and Magness, R. R. (2004). Mechanisms of shear stress-induced endothelial nitric-oxide synthase phosphorylation and expression in ovine fetoplacental artery endothelial cells. Biol. Reproduction 70, 785–796. doi:10.1095/biolreprod.103.022293
Li, Y., Zhong, C., Liu, D., Yu, W., Chen, W., Wang, Y., et al. (2018b). Evidence for Kaposi sarcoma originating from mesenchymal stem cell through KSHV-induced mesenchymal-to-endothelial transition. Cancer Res. 78, 230–245. doi:10.1158/0008-5472.CAN-17-1961
Li, Y.-S. J., Haga, J. H., and Chien, S. (2005). Molecular basis of the effects of shear stress on vascular endothelial cells. J. Biomech. 38, 1949–1971. doi:10.1016/j.jbiomech.2004.09.030
Liao, J. K. (2013). Linking endothelial dysfunction with endothelial cell activation. J. Clin. Invest. 123, 540–541. doi:10.1172/JCI66843
Liao, J. K., and Homcy, C. J. (1993). The G proteins of the G alpha i and G alpha q family couple the bradykinin receptor to the release of endothelium-derived relaxing factor. J. Clin. Invest. 92, 2168–2172. doi:10.1172/JCI116818
Liedtke, W., Choe, Y., Martí-Renom, M. A., Bell, A. M., Denis, C. S., Sali, A., et al. (2000). Vanilloid receptor–related osmotically activated channel (VR-OAC), a candidate vertebrate osmoreceptor. Cell 103, 525–535. doi:10.1016/S0092-8674(00)00143-4
Lieu, D. K., Pappone, P. A., and Barakat, A. I. (2004). Differential membrane potential and ion current responses to different types of shear stress in vascular endothelial cells. Am. J. Physiol. Cell Physiol. 286, C1367–C1375. doi:10.1152/ajpcell.00243.2003
Liman, E. R. (2007). “The Ca2+-activated TRP channels: TRPM4 and TRPM5,” in TRP ion channel function in sensory transduction and cellular signaling cascades. Editors W. B. Liedtke,, and S. Heller (Boca Raton (FL): CRC Press/Taylor and Francis). Available at: http://www.ncbi.nlm.nih.gov/books/NBK5257/(Accessed May 27, 2024).
Lin, X., He, Y., Hou, X., Zhang, Z., Wang, R., and Wu, Q. (2016). Endothelial cells can regulate smooth muscle cells in contractile phenotype through the miR-206/arf6&ncx1/exosome Axis. PLoS ONE 11, e0152959. doi:10.1371/journal.pone.0152959
Lin, X., Li, S., Wang, Y.-J., Wang, Y., Zhong, J.-Y., He, J.-Y., et al. (2019). Exosomal Notch3 from high glucose-stimulated endothelial cells regulates vascular smooth muscle cells calcification/aging. Life Sci. 232, 116582. doi:10.1016/j.lfs.2019.116582
Lin, X., Shan, S.-K., Xu, F., Zhong, J.-Y., Wu, F., Duan, J.-Y., et al. (2022). The crosstalk between endothelial cells and vascular smooth muscle cells aggravates high phosphorus-induced arterial calcification. Cell Death Dis. 13, 650. doi:10.1038/s41419-022-05064-5
Lipowsky, H. H., Kovalcheck, S., and Zweifach, B. W. (1978). The distribution of blood rheological parameters in the microvasculature of cat mesentery. Circulation Res. 43, 738–749. doi:10.1161/01.RES.43.5.738
Liu, J., and Khalil, R. A. (2017). “Matrix metalloproteinase inhibitors as investigational and therapeutic tools in unrestrained tissue remodeling and pathological disorders,” in Progress in molecular biology and translational science (Elsevier), 355–420. doi:10.1016/bs.pmbts.2017.04.003
Liu, J., Riquelme, M. A., Li, Z., Li, Y., Tong, Y., Quan, Y., et al. (2020a). Mechanosensitive collaboration between integrins and connexins allows nutrient and antioxidant transport into the lens. J. Cell Biol. 219, e202002154. doi:10.1083/jcb.202002154
Liu, J., Wang, J., Liu, Y., Xie, S.-A., Zhang, J., Zhao, C., et al. (2023a). Liquid-liquid phase separation of DDR1 counteracts the hippo pathway to orchestrate arterial stiffening. Circulation Res. 132, 87–105. doi:10.1161/CIRCRESAHA.122.322113
Liu, J., Zhao, C., Xiao, X., Li, A., Liu, Y., Zhao, J., et al. (2023b). Endothelial discoidin domain receptor 1 senses flow to modulate YAP activation. Nat. Commun. 14, 6457. doi:10.1038/s41467-023-42341-z
Liu, R., Jin, Y., Tang, W. H., Qin, L., Zhang, X., Tellides, G., et al. (2013a). Ten-eleven translocation-2 (TET2) is a master regulator of smooth muscle cell plasticity. Circulation 128, 2047–2057. doi:10.1161/CIRCULATIONAHA.113.002887
Liu, T., Du, X., Zhang, B., Zi, H., Yan, Y., Yin, J., et al. (2020b). Piezo1-Mediated Ca2+ activities regulate brain vascular pathfinding during development. Neuron 108, 180–192. doi:10.1016/j.neuron.2020.07.025
Liu, Y., Collins, C., Kiosses, W. B., Murray, A. M., Joshi, M., Shepherd, T. R., et al. (2013b). A novel pathway spatiotemporally activates Rac1 and redox signaling in response to fluid shear stress. J. Cell Biol. 201, 863–873. doi:10.1083/jcb.201207115
Liu, Y., Sweet, D. T., Irani-Tehrani, M., Maeda, N., and Tzima, E. (2008). Shc coordinates signals from intercellular junctions and integrins to regulate flow-induced inflammation. J. Cell Biol. 182, 185–196. doi:10.1083/jcb.200709176
Liu, Y.-S., Liu, Y.-A., Huang, C.-J., Yen, M.-H., Tseng, C.-T., Chien, S., et al. (2015). Mechanosensitive TRPM7 mediates shear stress and modulates osteogenic differentiation of mesenchymal stromal cells through Osterix pathway. Sci. Rep. 5, 16522. doi:10.1038/srep16522
Lolo, F.-N., Pavón, D. M., Grande-García, A., Elosegui-Artola, A., Segatori, V. I., Sánchez, S., et al. (2022). Caveolae couple mechanical stress to integrin recycling and activation. Elife 11, e82348. doi:10.7554/eLife.82348
Longden, T. A., Dabertrand, F., Koide, M., Gonzales, A. L., Tykocki, N. R., Brayden, J. E., et al. (2017). Capillary K+-sensing initiates retrograde hyperpolarization to increase local cerebral blood flow. Nat. Neurosci. 20, 717–726. doi:10.1038/nn.4533
Longden, T. A., Mughal, A., Hennig, G. W., Harraz, O. F., Shui, B., Lee, F. K., et al. (2021). Local IP3 receptor-mediated Ca2+ signals compound to direct blood flow in brain capillaries. Sci. Adv. 7, eabh0101. doi:10.1126/sciadv.abh0101
Loot, A. E., Popp, R., Fisslthaler, B., Vriens, J., Nilius, B., and Fleming, I. (2008). Role of cytochrome P450-dependent transient receptor potential V4 activation in flow-induced vasodilatation. Cardiovasc. Res. 80, 445–452. doi:10.1093/cvr/cvn207
Loufrani, L., Retailleau, K., Bocquet, A., Dumont, O., Danker, K., Louis, H., et al. (2008). Key role of α 1 β 1 -integrin in the activation of PI3-kinase-Akt by flow (shear stress) in resistance arteries. Am. J. Physiology-Heart Circulatory Physiology 294, H1906–H1913. doi:10.1152/ajpheart.00966.2006
Loukin, S., Zhou, X., Su, Z., Saimi, Y., and Kung, C. (2010). Wild-type and brachyolmia-causing mutant TRPV4 channels respond directly to stretch force. J. Biol. Chem. 285, 27176–27181. doi:10.1074/jbc.M110.143370
Loyer, X., Vion, A.-C., Tedgui, A., and Boulanger, C. M. (2014). Microvesicles as cell–cell messengers in cardiovascular diseases. Circulation Res. 114, 345–353. doi:10.1161/CIRCRESAHA.113.300858
Lubos, E., Handy, D. E., and Loscalzo, J. (2008). Role of oxidative stress and nitric oxide in atherothrombosis. Front. Biosci. 13, 5323–5344. doi:10.2741/3084
Lundberg, J. O., and Weitzberg, E. (2022). Nitric oxide signaling in health and disease. Cell 185, 2853–2878. doi:10.1016/j.cell.2022.06.010
Luo, B.-H., Carman, C. V., and Springer, T. A. (2007). Structural basis of integrin regulation and signaling. Annu. Rev. Immunol. 25, 619–647. doi:10.1146/annurev.immunol.25.022106.141618
Luo, S., Yang, M., Zhao, H., Han, Y., Jiang, N., Yang, J., et al. (2021). Caveolin-1 regulates cellular metabolism: a potential therapeutic target in kidney disease. Front. Pharmacol. 12, 768100. doi:10.3389/fphar.2021.768100
Luo, X., He, J., Xu, J., Hu, S., Mo, B., Shu, Q., et al. (2020). Vascular NRP2 triggers PNET angiogenesis by activating the SSH1-cofilin axis. Cell Biosci. 10, 113. doi:10.1186/s13578-020-00472-6
Luu, T., Glen, K., Egginton, S., Rainger, E., and Nash, G. (2013). Integrin-substrate interactions underlying shear-induced inhibition of the inflammatory response of endothelial cells. Thromb. Haemost. 109, 298–308. doi:10.1160/TH12-06-0400
Lux, S. E., John, K. M., and Karnovsky, M. J. (1976). Irreversible deformation of the spectrin-actin lattice in irreversibly sickled cells. J. Clin. Invest. 58, 955–963. doi:10.1172/JCI108549
Ma, J., Sanchez-Duffhues, G., Goumans, M.-J., and Ten Dijke, P. (2020). TGF-β-Induced endothelial to mesenchymal transition in disease and tissue engineering. Front. Cell Dev. Biol. 8, 260. doi:10.3389/fcell.2020.00260
Ma, S., Cahalan, S., LaMonte, G., Grubaugh, N. D., Zeng, W., Murthy, S. E., et al. (2018). Common PIEZO1 allele in african populations causes RBC dehydration and attenuates plasmodium infection. Cell 173, 443–455. doi:10.1016/j.cell.2018.02.047
MacKay, C. E., Leo, M. D., Fernández-Peña, C., Hasan, R., Yin, W., Mata-Daboin, A., et al. (2020). Intravascular flow stimulates PKD2 (polycystin-2) channels in endothelial cells to reduce blood pressure. Elife 9, e56655. doi:10.7554/eLife.56655
Mahmoud, M. M., Kim, H. R., Xing, R., Hsiao, S., Mammoto, A., Chen, J., et al. (2016). TWIST1 integrates endothelial responses to flow in vascular dysfunction and atherosclerosis. Circulation Res. 119, 450–462. doi:10.1161/CIRCRESAHA.116.308870
Mahmoud, M. M., Serbanovic-Canic, J., Feng, S., Souilhol, C., Xing, R., Hsiao, S., et al. (2017). Shear stress induces endothelial-to-mesenchymal transition via the transcription factor Snail. Sci. Rep. 7, 3375. doi:10.1038/s41598-017-03532-z
Maingret, F., Patel, A. J., Lesage, F., Lazdunski, M., and Honoré, E. (1999). Mechano- or acid stimulation, two interactive modes of activation of the TREK-1 potassium channel. J. Biol. Chem. 274, 26691–26696. doi:10.1074/jbc.274.38.26691
Maishi, N., Annan, D. A., Kikuchi, H., Hida, Y., and Hida, K. (2019). Tumor endothelial heterogeneity in cancer progression. Cancers 11, 1511. doi:10.3390/cancers11101511
Maisonpierre, P. C., Suri, C., Jones, P. F., Bartunkova, S., Wiegand, S. J., Radziejewski, C., et al. (1997). Angiopoietin-2, a natural antagonist for Tie2 that disrupts in vivo angiogenesis. Science 277, 55–60. doi:10.1126/science.277.5322.55
Mäkinen, T., Jussila, L., Veikkola, T., Karpanen, T., Kettunen, M. I., Pulkkanen, K. J., et al. (2001). Inhibition of lymphangiogenesis with resulting lymphedema in transgenic mice expressing soluble VEGF receptor-3. Nat. Med. 7, 199–205. doi:10.1038/84651
Malek, A. M., Alper, S. L., and Izumo, S. (1999). Hemodynamic shear stress and its role in atherosclerosis. JAMA 282, 2035–2042. doi:10.1001/jama.282.21.2035
Malsch, P., Andratsch, M., Vogl, C., Link, A. S., Alzheimer, C., Brierley, S. M., et al. (2014). Deletion of interleukin-6 signal transducer gp130 in small sensory neurons attenuates mechanonociception and down-regulates TRPA1 expression. J. Neurosci. 34, 9845–9856. doi:10.1523/JNEUROSCI.5161-13.2014
Mang, D., Roy, S. R., Hoh, H. H., Wu, X., Zhang, J., Jin, C., et al. (2020). Self-assembly of integrin ligands on the apical membrane inhibits the migration of glioma cells. Langmuir 36, 3750–3757. doi:10.1021/acs.langmuir.0c00291
Maroto, R., Raso, A., Wood, T. G., Kurosky, A., Martinac, B., and Hamill, O. P. (2005). TRPC1 forms the stretch-activated cation channel in vertebrate cells. Nat. Cell Biol. 7, 179–185. doi:10.1038/ncb1218
Marron, M. B., Hughes, D. P., Edge, M. D., Forder, C. L., and Brindle, N. P. J. (2000). Evidence for heterotypic interaction between the receptor tyrosine kinases TIE-1 and TIE-2. J. Biol. Chem. 275, 39741–39746. doi:10.1074/jbc.M007189200
Marti, C. N., Gheorghiade, M., Kalogeropoulos, A. P., Georgiopoulou, V. V., Quyyumi, A. A., and Butler, J. (2012). Endothelial dysfunction, arterial stiffness, and heart failure. J. Am. Coll. Cardiol. 60, 1455–1469. doi:10.1016/j.jacc.2011.11.082
Martinac, B. (2004). Mechanosensitive ion channels: molecules of mechanotransduction. J. Cell Sci. 117, 2449–2460. doi:10.1242/jcs.01232
Martinac, B. (2014). The ion channels to cytoskeleton connection as potential mechanism of mechanosensitivity. Biochimica Biophysica Acta (BBA) - Biomembr. 1838, 682–691. doi:10.1016/j.bbamem.2013.07.015
Martinac, B., and Kung, C. (2022). The force-from-lipid principle and its origin, a ‘ what is true for E. coli is true for the elephant’ refrain. J. Neurogenetics 36, 44–54. doi:10.1080/01677063.2022.2097674
Martín-Cófreces, N. B., Vicente-Manzanares, M., and Sánchez-Madrid, F. (2018). Adhesive interactions delineate the topography of the immune synapse. Front. Cell Dev. Biol. 6, 149. doi:10.3389/fcell.2018.00149
Martinez-Quinones, P., McCarthy, C. G., Watts, S. W., Klee, N. S., Komic, A., Calmasini, F. B., et al. (2018). Hypertension induced morphological and physiological changes in cells of the arterial wall. Am. J. Hypertens. 31, 1067–1078. doi:10.1093/ajh/hpy083
Martino, F., Perestrelo, A. R., Vinarský, V., Pagliari, S., and Forte, G. (2018). Cellular mechanotransduction: from tension to function. Front. Physiol. 9, 824. doi:10.3389/fphys.2018.00824
Marullo, S., Doly, S., Saha, K., Enslen, H., Scott, M. G. H., and Coureuil, M. (2020). Mechanical GPCR activation by traction forces exerted on receptor N -glycans. ACS Pharmacol. Transl. Sci. 3, 171–178. doi:10.1021/acsptsci.9b00106
Mathie, A. (2007). Neuronal two-pore-domain potassium channels and their regulation by G protein-coupled receptors. J. Physiol. 578, 377–385. doi:10.1113/jphysiol.2006.121582
Matsuda, H., Oishi, K., and Omori, K. (2003). Voltage-dependent gating and block by internal spermine of the murine inwardly rectifying K+ channel, Kir2.1. J. Physiology 548, 361–371. doi:10.1113/jphysiol.2003.038844
Matthews, B. D., Overby, D. R., Mannix, R., and Ingber, D. E. (2006). Cellular adaptation to mechanical stress: role of integrins, Rho, cytoskeletal tension and mechanosensitive ion channels. J. Cell Sci. 119, 508–518. doi:10.1242/jcs.02760
Matthews, B. D., Thodeti, C. K., Tytell, J. D., Mammoto, A., Overby, D. R., and Ingber, D. E. (2010). Ultra-rapid activation of TRPV4 ion channels by mechanical forces applied to cell surface beta1 integrins. Integr. Biol. 2, 435–442. doi:10.1039/c0ib00034e
Mayet, J., and Hughes, A. (2003). Cardiac and vascular pathophysiology in hypertension. Heart 89, 1104–1109. doi:10.1136/heart.89.9.1104
Mazzochi, C., Bubien, J. K., Smith, P. R., and Benos, D. J. (2006). The carboxyl terminus of the alpha-subunit of the amiloride-sensitive epithelial sodium channel binds to F-actin. J. Biol. Chem. 281, 6528–6538. doi:10.1074/jbc.M509386200
McCormick, M. E., Goel, R., Fulton, D., Oess, S., Newman, D., and Tzima, E. (2011). Platelet-endothelial cell adhesion molecule-1 regulates endothelial NO synthase activity and localization through signal transducers and activators of transcription 3-dependent NOSTRIN expression. Arterioscler. Thromb. Vasc. Biol. 31, 643–649. doi:10.1161/ATVBAHA.110.216200
McFarland, S. J., Weber, D. S., Choi, C.-S., Lin, M. T., and Taylor, M. S. (2020). Ablation of endothelial TRPV4 channels alters the dynamic Ca2+ signaling profile in mouse carotid arteries. Int. J. Mol. Sci. 21, 2179. doi:10.3390/ijms21062179
McMahon, K.-A., Wu, Y., Gambin, Y., Sierecki, E., Tillu, V. A., Hall, T., et al. (2019). Identification of intracellular cavin target proteins reveals cavin-PP1alpha interactions regulate apoptosis. Nat. Commun. 10, 3279. doi:10.1038/s41467-019-11111-1
Mehta, V., Pang, K.-L., Givens, C. S., Chen, Z., Huang, J., Sweet, D. T., et al. (2021). Mechanical forces regulate endothelial-to-mesenchymal transition and atherosclerosis via an Alk5-Shc mechanotransduction pathway. Sci. Adv. 7, eabg5060. doi:10.1126/sciadv.abg5060
Mehta, V., Pang, K.-L., Rozbesky, D., Nather, K., Keen, A., Lachowski, D., et al. (2020). The guidance receptor plexin D1 is a mechanosensor in endothelial cells. Nature 578, 290–295. doi:10.1038/s41586-020-1979-4
Melincovici, C. S., Boşca, A. B., Şuşman, S., Mărginean, M., Mihu, C., Istrate, M., et al. (2018). Vascular endothelial growth factor (VEGF) - key factor in normal and pathological angiogenesis. Rom. J. Morphol. Embryol. 59, 455–467.
Mendoza, S. A., Fang, J., Gutterman, D. D., Wilcox, D. A., Bubolz, A. H., Li, R., et al. (2010). TRPV4-mediated endothelial Ca 2+ influx and vasodilation in response to shear stress. Am. J. Physiology-Heart Circulatory Physiology 298, H466–H476. doi:10.1152/ajpheart.00854.2009
Michalik, K. M., You, X., Manavski, Y., Doddaballapur, A., Zörnig, M., Braun, T., et al. (2014). Long noncoding RNA MALAT1 regulates endothelial cell function and vessel growth. Circ. Res. 114, 1389–1397. doi:10.1161/CIRCRESAHA.114.303265
Mihai, C., Chotani, M., Elton, T. S., and Agarwal, G. (2009). Mapping of DDR1 distribution and oligomerization on the cell surface by FRET microscopy. J. Mol. Biol. 385, 432–445. doi:10.1016/j.jmb.2008.10.067
Miller, B., and Sewell-Loftin, M. K. (2022). Mechanoregulation of vascular endothelial growth factor receptor 2 in angiogenesis. Front. Cardiovasc. Med. 8, 804934. doi:10.3389/fcvm.2021.804934
Mills, K. T., Bundy, J. D., Kelly, T. N., Reed, J. E., Kearney, P. M., Reynolds, K., et al. (2016). Global disparities of hypertension prevalence and control: a systematic analysis of population-based studies from 90 countries. Circulation 134, 441–450. doi:10.1161/CIRCULATIONAHA.115.018912
Mishani, S., Belhoul-Fakir, H., Lagat, C., Jansen, S., Evans, B., and Lawrence-Brown, M. (2021). Stress distribution in the walls of major arteries: implications for atherogenesis. Quant. Imaging Med. Surg. 11, 3494–3505. doi:10.21037/qims-20-614
Mochizuki, S., Vink, H., Hiramatsu, O., Kajita, T., Shigeto, F., Spaan, J. A. E., et al. (2003). Role of hyaluronic acid glycosaminoglycans in shear-induced endothelium-derived nitric oxide release. Am. J. Physiology-Heart Circulatory Physiology 285, H722–H726. doi:10.1152/ajpheart.00691.2002
Mohan, S., Mohan, N., and Sprague, E. A. (1997). Differential activation of NF-kappa B in human aortic endothelial cells conditioned to specific flow environments. Am. J. Physiol. 273, C572–C578. doi:10.1152/ajpcell.1997.273.2.C572
Moncada, S., Gryglewski, R., Bunting, S., and Vane, J. R. (1976). An enzyme isolated from arteries transforms prostaglandin endoperoxides to an unstable substance that inhibits platelet aggregation. Nature 263, 663–665. doi:10.1038/263663a0
Moncada, S., and Higgs, E. A. (2006). “Nitric oxide and the vascular endothelium,” in The vascular endothelium I. Editors S. Moncada,, and A. Higgs (Berlin, Heidelberg: Springer Berlin Heidelberg), 213–254. doi:10.1007/3-540-32967-6_7
Monkley, S. J., Kostourou, V., Spence, L., Petrich, B., Coleman, S., Ginsberg, M. H., et al. (2011). Endothelial cell talin1 is essential for embryonic angiogenesis. Dev. Biol. 349, 494–502. doi:10.1016/j.ydbio.2010.11.010
Monticelli, M., Conca, D. V., Albisetti, E., Torti, A., Sharma, P. P., Kidiyoor, G., et al. (2016). Magnetic domain wall tweezers: a new tool for mechanobiology studies on individual target cells. Lab. Chip 16, 2882–2890. doi:10.1039/C6LC00368K
Moonen, J.-R. A. J., Lee, E. S., Schmidt, M., Maleszewska, M., Koerts, J. A., Brouwer, L. A., et al. (2015). Endothelial-to-mesenchymal transition contributes to fibro-proliferative vascular disease and is modulated by fluid shear stress. Cardiovasc Res. 108, 377–386. doi:10.1093/cvr/cvv175
Mossman, D., Kim, K.-T., and Scott, R. J. (2010). Demethylation by 5-aza-2’-deoxycytidine in colorectal cancer cells targets genomic DNA whilst promoter CpG island methylation persists. BMC Cancer 10, 366. doi:10.1186/1471-2407-10-366
Moustakas, A., and Heldin, C.-H. (2016). Mechanisms of tgfβ-induced epithelial-mesenchymal transition. JCM 5, 63. doi:10.3390/jcm5070063
Murata, T., Lin, M. I., Huang, Y., Yu, J., Bauer, P. M., Giordano, F. J., et al. (2007). Reexpression of caveolin-1 in endothelium rescues the vascular, cardiac, and pulmonary defects in global caveolin-1 knockout mice. J. Exp. Med. 204, 2373–2382. doi:10.1084/jem.20062340
Murthy, S. E., Dubin, A. E., and Patapoutian, A. (2017). Piezos thrive under pressure: mechanically activated ion channels in health and disease. Nat. Rev. Mol. Cell Biol. 18, 771–783. doi:10.1038/nrm.2017.92
Mylvaganam, S., Plumb, J., Yusuf, B., Li, R., Lu, C.-Y., Robinson, L. A., et al. (2022). The spectrin cytoskeleton integrates endothelial mechanoresponses. Nat. Cell Biol. 24, 1226–1238. doi:10.1038/s41556-022-00953-5
Nakajima, H., and Mochizuki, N. (2017). Flow pattern-dependent endothelial cell responses through transcriptional regulation. Cell Cycle 16, 1893–1901. doi:10.1080/15384101.2017.1364324
Nakashima, Y., Raines, E. W., Plump, A. S., Breslow, J. L., and Ross, R. (1998). Upregulation of VCAM-1 and ICAM-1 at atherosclerosis-prone sites on the endothelium in the ApoE-deficient mouse. ATVB 18, 842–851. doi:10.1161/01.ATV.18.5.842
Nardone, G., Oliver-De La Cruz, J., Vrbsky, J., Martini, C., Pribyl, J., Skládal, P., et al. (2017). YAP regulates cell mechanics by controlling focal adhesion assembly. Nat. Commun. 8, 15321. doi:10.1038/ncomms15321
Nauli, S. M., Kawanabe, Y., Kaminski, J. J., Pearce, W. J., Ingber, D. E., and Zhou, J. (2008). Endothelial cilia are fluid shear sensors that regulate calcium signaling and nitric oxide production through polycystin-1. Circulation 117, 1161–1171. doi:10.1161/CIRCULATIONAHA.107.710111
Nazari-Jahantigh, M., Wei, Y., Noels, H., Akhtar, S., Zhou, Z., Koenen, R. R., et al. (2012). MicroRNA-155 promotes atherosclerosis by repressing Bcl6 in macrophages. J. Clin. Invest. 122, 4190–4202. doi:10.1172/JCI61716
Ngai, A. C., and Winn, H. R. (1995). Modulation of cerebral arteriolar diameter by intraluminal flow and pressure. Circulation Res. 77, 832–840. doi:10.1161/01.RES.77.4.832
Ngai, D., Mohabeer, A. L., Mao, A., Lino, M., and Bendeck, M. P. (2022). Stiffness-responsive feedback autoregulation of DDR1 expression is mediated by a DDR1-YAP/TAZ axis. Matrix Biol. 110, 129–140. doi:10.1016/j.matbio.2022.05.004
Ni, C.-W., Qiu, H., Rezvan, A., Kwon, K., Nam, D., Son, D. J., et al. (2010). Discovery of novel mechanosensitive genes in vivo using mouse carotid artery endothelium exposed to disturbed flow. Blood 116, e66–e73. doi:10.1182/blood-2010-04-278192
Nieswandt, B., Moser, M., Pleines, I., Varga-Szabo, D., Monkley, S., Critchley, D., et al. (2007). Loss of talin1 in platelets abrogates integrin activation, platelet aggregation, and thrombus formation in vitro and in vivo. J. Exp. Med. 204, 3113–3118. doi:10.1084/jem.20071827
Nikolaev, Y. A., Cox, C. D., Ridone, P., Rohde, P. R., Cordero-Morales, J. F., Vásquez, V., et al. (2019). Mammalian TRP ion channels are insensitive to membrane stretch. J. Cell Sci. 132, jcs238360. doi:10.1242/jcs.238360
Nilius, B., and Owsianik, G. (2011). The transient receptor potential family of ion channels. Genome Biol. 12, 218. doi:10.1186/gb-2011-12-3-218
Nilius, B., Owsianik, G., Voets, T., and Peters, J. A. (2007). Transient receptor potential cation channels in disease. Physiol. Rev. 87, 165–217. doi:10.1152/physrev.00021.2006
Noordeen, N. A., Carafoli, F., Hohenester, E., Horton, M. A., and Leitinger, B. (2006). A transmembrane leucine zipper is required for activation of the dimeric receptor tyrosine kinase DDR1. J. Biol. Chem. 281, 22744–22751. doi:10.1074/jbc.M603233200
Oda, T., Makino, K., Yamashita, I., Namba, K., and Maéda, Y. (2001). Distinct structural changes detected by X-ray fiber diffraction in stabilization of F-actin by lowering pH and increasing ionic strength. Biophysical J. 80, 841–851. doi:10.1016/S0006-3495(01)76063-8
Oemar, B. S., Tschudi, M. R., Godoy, N., Brovkovich, V., Malinski, T., and Lüscher, T. F. (1998). Reduced endothelial nitric oxide synthase expression and production in human atherosclerosis. Circulation 97, 2494–2498. doi:10.1161/01.CIR.97.25.2494
Ohno, M., Gibbons, G. H., Dzau, V. J., and Cooke, J. P. (1993). Shear stress elevates endothelial cGMP. Role of a potassium channel and G protein coupling. Circulation 88, 193–197. doi:10.1161/01.CIR.88.1.193
Ojakian, G. K., and Schwimmer, R. (1994). Regulation of epithelial cell surface polarity reversal by β1 integrins. J. Cell Sci. 107 (Pt 3), 561–576. doi:10.1242/jcs.107.3.561
Olesen, S.-P., Claphamt, D., and Davies, P. (1988). Haemodynamic shear stress activates a K+ current in vascular endothelial cells. Nature 331, 168–170. doi:10.1038/331168a0
Orr, A. W., Ginsberg, M. H., Shattil, S. J., Deckmyn, H., and Schwartz, M. A. (2006). Matrix-specific suppression of integrin activation in shear stress signaling. MBoC 17, 4686–4697. doi:10.1091/mbc.e06-04-0289
Orr, A. W., Sanders, J. M., Bevard, M., Coleman, E., Sarembock, I. J., and Schwartz, M. A. (2005). The subendothelial extracellular matrix modulates NF-kappaB activation by flow: a potential role in atherosclerosis. J. Cell Biol. 169, 191–202. doi:10.1083/jcb.200410073
Osawa, M., Masuda, M., Kusano, K., and Fujiwara, K. (2002). Evidence for a role of platelet endothelial cell adhesion molecule-1 in endothelial cell mechanosignal transduction. J. Cell Biol. 158, 773–785. doi:10.1083/jcb.200205049
Osol, G., Brekke, J. F., McElroy-Yaggy, K., and Gokina, N. I. (2002). Myogenic tone, reactivity, and forced dilatation: a three-phase model of in vitro arterial myogenic behavior. Am. J. Physiology-Heart Circulatory Physiology 283, H2260–H2267. doi:10.1152/ajpheart.00634.2002
Ozkan, A. D., Gettas, T., Sogata, A., Phaychanpheng, W., Zhou, M., and Lacroix, J. J. (2021). Mechanical and chemical activation of GPR68 probed with a genetically encoded fluorescent reporter. J. Cell Sci. 134, jcs255455. doi:10.1242/jcs.255455
Ozkan, A. D., Wijerathne, T. D., Gettas, T., and Lacroix, J. J. (2022). PIEZO1 discriminates mechanical stimuli. doi:10.1101/2022.06.23.497409
Pahakis, M. Y., Kosky, J. R., Dull, R. O., and Tarbell, J. M. (2007). The role of endothelial glycocalyx components in mechanotransduction of fluid shear stress. Biochem. Biophysical Res. Commun. 355, 228–233. doi:10.1016/j.bbrc.2007.01.137
Palmer, A., Mason, T. G., Xu, J., Kuo, S. C., and Wirtz, D. (1999). Diffusing wave spectroscopy microrheology of actin filament networks. Biophysical J. 76, 1063–1071. doi:10.1016/S0006-3495(99)77271-1
Pan, X., Wan, R., Wang, Y., Liu, S., He, Y., Deng, B., et al. (2022). Inhibition of chemically and mechanically activated Piezo1 channels as a mechanism for ameliorating atherosclerosis with salvianolic acid B. Br. J Pharmacol. 179, 3778–3814. doi:10.1111/bph.15826
Parasassi, T., Gratton, E., Yu, W. M., Wilson, P., and Levi, M. (1997). Two-photon fluorescence microscopy of laurdan generalized polarization domains in model and natural membranes. Biophysical J. 72, 2413–2429. doi:10.1016/S0006-3495(97)78887-8
Park, D. S., Woodman, S. E., Schubert, W., Cohen, A. W., Frank, P. G., Chandra, M., et al. (2002). Caveolin-1/3 double-knockout mice are viable, but lack both muscle and non-muscle caveolae, and develop a severe cardiomyopathic phenotype. Am. J. Pathology 160, 2207–2217. doi:10.1016/S0002-9440(10)61168-6
Parmar, K. M., Larman, H. B., Dai, G., Zhang, Y., Wang, E. T., Moorthy, S. N., et al. (2006). Integration of flow-dependent endothelial phenotypes by Kruppel-like factor 2. J. Clin. Invest. 116, 49–58. doi:10.1172/JCI24787
Parton, R. G., and Del Pozo, M. A. (2013). Caveolae as plasma membrane sensors, protectors and organizers. Nat. Rev. Mol. Cell Biol. 14, 98–112. doi:10.1038/nrm3512
Parton, R. G., Del Pozo, M. A., Vassilopoulos, S., Nabi, I. R., Le Lay, S., Lundmark, R., et al. (2020). Caveolae: the FAQs. Traffic 21, 181–185. doi:10.1111/tra.12689
Parton, R. G., and Simons, K. (2007). The multiple faces of caveolae. Nat. Rev. Mol. Cell Biol. 8, 185–194. doi:10.1038/nrm2122
Patel, A., Sharif-Naeini, R., Folgering, J. R. H., Bichet, D., Duprat, F., and Honoré, E. (2010). Canonical TRP channels and mechanotransduction: from physiology to disease states. Pflugers Arch. - Eur. J. Physiol. 460, 571–581. doi:10.1007/s00424-010-0847-8
Patel, A. J., Honoré, E., Maingret, F., Lesage, F., Fink, M., Duprat, F., et al. (1998). A mammalian two pore domain mechano-gated S-like K+ channel. EMBO J. 17, 4283–4290. doi:10.1093/emboj/17.15.4283
Pedersen, S. F., and Nilius, B. (2007). “Transient receptor potential channels in mechanosensing and cell volume regulation,” in Methods in enzymology (Elsevier), 183–207. doi:10.1016/S0076-6879(07)28010-3
Peng, Y.-L., Meng, Q.-L., Qiao, J., Xie, K., Chen, C., Liu, T.-L., et al. (2016). The roles of noncoding RNA Rli60 in regulating the virulence of Listeria monocytogenes. J. Microbiol. Immunol. Infect. 49, 502–508. doi:10.1016/j.jmii.2014.08.017
Petrich, B. G., Marchese, P., Ruggeri, Z. M., Spiess, S., Weichert, R. A. M., Ye, F., et al. (2007). Talin is required for integrin-mediated platelet function in hemostasis and thrombosis. J. Exp. Med. 204, 3103–3111. doi:10.1084/jem.20071800
Petridou, N. I., and Skourides, P. A. (2016). A ligand-independent integrin β1 mechanosensory complex guides spindle orientation. Nat. Commun. 7, 10899. doi:10.1038/ncomms10899
Piera-Velazquez, S., and Jimenez, S. A. (2019). Endothelial to mesenchymal transition: role in physiology and in the pathogenesis of human diseases. Physiol. Rev. 99, 1281–1324. doi:10.1152/physrev.00021.2018
Pilard, M., Ollivier, E. L., Gourdou-Latyszenok, V., Couturaud, F., and Lemarié, C. A. (2022). Endothelial cell phenotype, a major determinant of venous thrombo-inflammation. Front. Cardiovasc. Med. 9, 864735. doi:10.3389/fcvm.2022.864735
Porat, R. M., Grunewald, M., Globerman, A., Itin, A., Barshtein, G., Alhonen, L., et al. (2004). Specific induction of tie1 promoter by disturbed flow in atherosclerosis-prone vascular niches and flow-obstructing pathologies. Circ. Res. 94, 394–401. doi:10.1161/01.RES.0000111803.92923.D6
Porto Ribeiro, T., Barbeau, S., Baudrimont, I., Vacher, P., Freund-Michel, V., Cardouat, G., et al. (2022). Piezo1 channel activation reverses pulmonary artery vasoconstriction in an early rat model of pulmonary hypertension: the role of Ca2+ influx and akt-eNOS pathway. Cells 11, 2349. doi:10.3390/cells11152349
Pourbagher-Shahri, A. M., Farkhondeh, T., Talebi, M., Kopustinskiene, D. M., Samarghandian, S., and Bernatoniene, J. (2021). An overview of NO signaling pathways in aging. Molecules 26, 4533. doi:10.3390/molecules26154533
Pries, A. R., Secomb, T. W., and Gaehtgens, P. (2000). The endothelial surface layer. Pflugers Arch. - Eur. J. Physiol. 440, 653–666. doi:10.1007/s004240000307
Puklin-Faucher, E., and Sheetz, M. P. (2009). The mechanical integrin cycle. J. Cell Sci. 122, 179–186. doi:10.1242/jcs.042127
Pulous, F. E., Grimsley-Myers, C. M., Kansal, S., Kowalczyk, A. P., and Petrich, B. G. (2019). Talin-dependent integrin activation regulates VE-cadherin localization and endothelial cell barrier function. Circulation Res. 124, 891–903. doi:10.1161/CIRCRESAHA.118.314560
Purcell, S. C., and Godula, K. (2019). Synthetic glycoscapes: addressing the structural and functional complexity of the glycocalyx. Interface Focus 9, 20180080. doi:10.1098/rsfs.2018.0080
Qu, Y.-Y., Wang, L.-M., Zhong, H., Liu, Y.-M., Tang, N., Zhu, L.-P., et al. (2017). TRPC1 stimulates calcium-sensing receptor-induced store-operated Ca2+ entry and nitric oxide production in endothelial cells. Mol. Med. Rep. 16, 4613–4619. doi:10.3892/mmr.2017.7164
Radel, C., and Rizzo, V. (2005). Integrin mechanotransduction stimulates caveolin-1 phosphorylation and recruitment of Csk to mediate actin reorganization. Am. J. Physiol. Heart Circ. Physiol. 288, H936–H945. doi:10.1152/ajpheart.00519.2004
Ramachandran, V., Yago, T., Epperson, T. K., Kobzdej, M. M. A., Nollert, M. U., Cummings, R. D., et al. (2001). Dimerization of a selectin and its ligand stabilizes cell rolling and enhances tether strength in shear flow. Proc. Natl. Acad. Sci. U.S.A. 98, 10166–10171. doi:10.1073/pnas.171248098
Ranade, S. S., Qiu, Z., Woo, S.-H., Hur, S. S., Murthy, S. E., Cahalan, S. M., et al. (2014). Piezo1, a mechanically activated ion channel, is required for vascular development in mice. Proc. Natl. Acad. Sci. 111, 10347–10352. doi:10.1073/pnas.1409233111
Rao, A. M., Hatcher, J. F., Kindy, M. S., and Dempsey, R. J. (1999). Arachidonic acid and leukotriene C4: role in transient cerebral ischemia of gerbils. Neurochem. Res. 24, 1225–1232. doi:10.1023/a:1020916905312
Rath, G., Saliez, J., Behets, G., Romero-Perez, M., Leon-Gomez, E., Bouzin, C., et al. (2012). Vascular hypoxic preconditioning relies on TRPV4-dependent calcium influx and proper intercellular gap junctions communication. Arterioscler. Thromb. Vasc. Biol. 32, 2241–2249. doi:10.1161/ATVBAHA.112.252783
Raupach, C., Zitterbart, D. P., Mierke, C. T., Metzner, C., Müller, F. A., and Fabry, B. (2007). Stress fluctuations and motion of cytoskeletal-bound markers. Phys. Rev. E 76, 011918. doi:10.1103/PhysRevE.76.011918
Razani, B., Combs, T. P., Wang, X. B., Frank, P. G., Park, D. S., Russell, R. G., et al. (2002). Caveolin-1-deficient mice are lean, resistant to diet-induced obesity, and show hypertriglyceridemia with adipocyte abnormalities. J. Biol. Chem. 277, 8635–8647. doi:10.1074/jbc.M110970200
Razani, B., Schlegel, A., Liu, J., and Lisanti, M. P. (2001). Caveolin-1, a putative tumour suppressor gene. Biochem. Soc. Trans. 29, 494–499. doi:10.1042/bst0290494
Reitsma, S., Slaaf, D. W., Vink, H., Van Zandvoort, M. A. M. J., and Oude Egbrink, M. G. A. (2007). The endothelial glycocalyx: composition, functions, and visualization. Pflugers Arch. - Eur. J. Physiol. 454, 345–359. doi:10.1007/s00424-007-0212-8
Remuzzi, A., Brenner, B. M., Pata, V., Tebaldi, G., Mariano, R., Belloro, A., et al. (1992). Three-dimensional reconstructed glomerular capillary network: blood flow distribution and local filtration. Am. J. Physiology-Renal Physiology 263, F562–F572. doi:10.1152/ajprenal.1992.263.3.F562
Renna, N. F., De Las Heras, N., and Miatello, R. M. (2013). Pathophysiology of vascular remodeling in hypertension. Int. J. Hypertens. 2013, 808353–808357. doi:10.1155/2013/808353
Retailleau, K., Duprat, F., Arhatte, M., Ranade, S. S., Peyronnet, R., Martins, J. R., et al. (2015). Piezo1 in smooth muscle cells is involved in hypertension-dependent arterial remodeling. Cell Rep. 13, 1161–1171. doi:10.1016/j.celrep.2015.09.072
Richter, R. P., Ashtekar, A. R., Zheng, L., Pretorius, D., Kaushlendra, T., Sanderson, R. D., et al. (2022). Glycocalyx heparan sulfate cleavage promotes endothelial cell angiopoietin-2 expression by impairing shear stress–related AMPK/FoxO1 signaling. JCI Insight 7, e155010. doi:10.1172/jci.insight.155010
Riddell, D. R., and Owen, J. S. (1997). “Nitric oxide and platelet aggregation,” in Vitamins and hormones (Elsevier), 25–48. doi:10.1016/S0083-6729(08)60639-1
Rieder, F., Kessler, S. P., West, G. A., Bhilocha, S., De La Motte, C., Sadler, T. M., et al. (2011). Inflammation-induced endothelial-to-mesenchymal transition: a novel mechanism of intestinal fibrosis. Am. J. Pathology 179, 2660–2673. doi:10.1016/j.ajpath.2011.07.042
Rieder, M. J., Carmona, R., Krieger, J. E., Pritchard, K. A., and Greene, A. S. (1997). Suppression of angiotensin-converting enzyme expression and activity by shear stress. Circulation Res. 80, 312–319. doi:10.1161/01.RES.80.3.312
Risman, R. A., Kirby, N. C., Bannish, B. E., Hudson, N. E., and Tutwiler, V. (2023). Fibrinolysis: an illustrated review. Res. Pract. Thrombosis Haemostasis 7, 100081. doi:10.1016/j.rpth.2023.100081
Rivera, C. M., and Ren, B. (2013). Mapping human epigenomes. Cell 155, 39–55. doi:10.1016/j.cell.2013.09.011
Rizzo, V., McIntosh, D. P., Oh, P., and Schnitzer, J. E. (1998a). In situ flow activates endothelial nitric oxide synthase in luminal caveolae of endothelium with rapid caveolin dissociation and calmodulin association. J. Biol. Chem. 273, 34724–34729. doi:10.1074/jbc.273.52.34724
Rizzo, V., Morton, C., DePaola, N., Schnitzer, J. E., and Davies, P. F. (2003). Recruitment of endothelial caveolae into mechanotransduction pathways by flow conditioning in vitro. Am. J. Physiology-Heart Circulatory Physiology 285, H1720–H1729. doi:10.1152/ajpheart.00344.2002
Rizzo, V., Sung, A., Oh, P., and Schnitzer, J. E. (1998b). Rapid mechanotransduction in situ at the luminal cell surface of vascular endothelium and its caveolae. J. Biol. Chem. 273, 26323–26329. doi:10.1074/jbc.273.41.26323
Rode, B., Shi, J., Endesh, N., Drinkhill, M. J., Webster, P. J., Lotteau, S. J., et al. (2017). Piezo1 channels sense whole body physical activity to reset cardiovascular homeostasis and enhance performance. Nat. Commun. 8, 350. doi:10.1038/s41467-017-00429-3
Rohács, T., Chen, J., Prestwich, G. D., and Logothetis, D. E. (1999). Distinct specificities of inwardly rectifying K+Channels for phosphoinositides. J. Biol. Chem. 274, 36065–36072. doi:10.1074/jbc.274.51.36065
Romanenko, V. G., Fang, Y., Byfield, F., Travis, A. J., Vandenberg, C. A., Rothblat, G. H., et al. (2004). Cholesterol sensitivity and lipid raft targeting of Kir2.1 channels. Biophys. J. 87, 3850–3861. doi:10.1529/biophysj.104.043273
Rooney, M. M., Farrell, D. H., van Hemel, B. M., de Groot, P. G., and Lord, S. T. (1998). The contribution of the three hypothesized integrin-binding sites in fibrinogen to platelet-mediated clot retraction. Blood 92, 2374–2381. doi:10.1182/blood.v92.7.2374.2374_2374_2381
Roux, E., Bougaran, P., Dufourcq, P., and Couffinhal, T. (2020). Fluid shear stress sensing by the endothelial layer. Front. Physiol. 11, 861. doi:10.3389/fphys.2020.00861
Rubanyi, G. M., Romero, J. C., and Vanhoutte, P. M. (1986). Flow-induced release of endothelium-derived relaxing factor. Am. J. Physiology-Heart Circulatory Physiology 250, H1145–H1149. doi:10.1152/ajpheart.1986.250.6.H1145
Russell, R. W. R., Simcock, J. P., Wilkinson, I. M. S., and Frears, C. C. (1970). The effect of blood pressure changes on the leptomeningeal circulation of the rabbit. Brain 93, 491–504. doi:10.1093/brain/93.3.491
Ryazanova, L. V., Hu, Z., Suzuki, S., Chubanov, V., Fleig, A., and Ryazanov, A. G. (2014). Elucidating the role of the TRPM7 alpha-kinase: TRPM7 kinase inactivation leads to magnesium deprivation resistance phenotype in mice. Sci. Rep. 4, 7599. doi:10.1038/srep07599
Saharinen, P., Eklund, L., and Alitalo, K. (2017). Therapeutic targeting of the angiopoietin–TIE pathway. Nat. Rev. Drug Discov. 16, 635–661. doi:10.1038/nrd.2016.278
Saharinen, P., Eklund, L., Pulkki, K., Bono, P., and Alitalo, K. (2011). VEGF and angiopoietin signaling in tumor angiogenesis and metastasis. Trends Mol. Med. 17, 347–362. doi:10.1016/j.molmed.2011.01.015
Saharinen, P., Jeltsch, M., Santoyo, M. M., Leppänen, V.-M., and Alitalo, K. (2015). “The TIE receptor family,” in Receptor tyrosine kinases: family and subfamilies. Editors D. L. Wheeler,, and Y. Yarden (Cham: Springer International Publishing), 743–775. doi:10.1007/978-3-319-11888-8_16
Saito, A. (2013). EMT and EndMT: regulated in similar ways? J. Biochem. 153, 493–495. doi:10.1093/jb/mvt032
Sakariassen, K. S., Orning, L., and Turitto, V. T. (2015). The impact of blood shear rate on arterial thrombus formation. Future Sci. OA 1, FSO30. doi:10.4155/fso.15.28
Sakurai, A., Gavard, J., Annas-Linhares, Y., Basile, J. R., Amornphimoltham, P., Palmby, T. R., et al. (2010). Semaphorin 3E initiates antiangiogenic signaling through plexin D1 by regulating Arf6 and R-ras. Mol. Cell. Biol. 30, 3086–3098. doi:10.1128/MCB.01652-09
Saliez, J., Bouzin, C., Rath, G., Ghisdal, P., Desjardins, F., Rezzani, R., et al. (2008). Role of caveolar compartmentation in endothelium-derived hyperpolarizing factor-mediated relaxation: Ca2+ signals and gap junction function are regulated by caveolin in endothelial cells. Circulation 117, 1065–1074. doi:10.1161/CIRCULATIONAHA.107.731679
Samapati, R., Yang, Y., Yin, J., Stoerger, C., Arenz, C., Dietrich, A., et al. (2012). Lung endothelial Ca 2+ and permeability response to platelet-activating factor is mediated by acid sphingomyelinase and transient receptor potential classical 6. Am. J. Respir. Crit. Care Med. 185, 160–170. doi:10.1164/rccm.201104-0717OC
Sánchez-Duffhues, G., De Vinuesa, A. G., Lindeman, J. H., Mulder-Stapel, A., DeRuiter, M. C., Van Munsteren, C., et al. (2015). SLUG is expressed in endothelial cells lacking primary cilia to promote cellular calcification. ATVB 35, 616–627. doi:10.1161/ATVBAHA.115.305268
Sancho, M., Fletcher, J., and Welsh, D. G. (2022). Inward rectifier potassium channels: membrane lipid-dependent mechanosensitive gates in brain vascular cells. Front. Cardiovasc. Med. 9, 869481. doi:10.3389/fcvm.2022.869481
Satcher, R. L., Bussolari, S. R., Gimbrone, M. A., and Dewey, C. F. (1992). The distribution of fluid forces on model arterial endothelium using computational fluid dynamics. J. Biomechanical Eng. 114, 309–316. doi:10.1115/1.2891388
Schlessinger, J. (2000). Cell signaling by receptor tyrosine kinases. Cell 103, 211–225. doi:10.1016/S0092-8674(00)00114-8
Schmidpeter, P. A. M., Petroff, J. T., Khajoueinejad, L., Wague, A., Frankfater, C., Cheng, W. W. L., et al. (2023). Membrane phospholipids control gating of the mechanosensitive potassium leak channel TREK1. Nat. Commun. 14, 1077. doi:10.1038/s41467-023-36765-w
Schött, U., Solomon, C., Fries, D., and Bentzer, P. (2016). The endothelial glycocalyx and its disruption, protection and regeneration: a narrative review. Scand. J. Trauma Resusc. Emerg. Med. 24, 48. doi:10.1186/s13049-016-0239-y
Schwartz, M. A. (2010). Integrins and extracellular matrix in mechanotransduction. Cold Spring Harb. Perspect. Biol. 2, a005066. doi:10.1101/cshperspect.a005066
Sena, C. M., Nunes, E., Louro, T., Proença, T., Fernandes, R., Boarder, M. R., et al. (2008). Effects of alpha-lipoic acid on endothelial function in aged diabetic and high-fat fed rats. Br. J Pharmacol. 153, 894–906. doi:10.1038/sj.bjp.0707474
Sen-Banerjee, S., Mir, S., Lin, Z., Hamik, A., Atkins, G. B., Das, H., et al. (2005). Kruppel-like factor 2 as a novel mediator of statin effects in endothelial cells. Circulation 112, 720–726. doi:10.1161/CIRCULATIONAHA.104.525774
Servin-Vences, M. R., Moroni, M., Lewin, G. R., and Poole, K. (2017). Direct measurement of TRPV4 and PIEZO1 activity reveals multiple mechanotransduction pathways in chondrocytes. Elife 6, e21074. doi:10.7554/eLife.21074
Sharif-Naeini, R., Folgering, J. H. A., Bichet, D., Duprat, F., Delmas, P., Patel, A., et al. (2010). Sensing pressure in the cardiovascular system: Gq-coupled mechanoreceptors and TRP channels. J. Mol. Cell. Cardiol. 48, 83–89. doi:10.1016/j.yjmcc.2009.03.020
Shay-Salit, A., Shushy, M., Wolfovitz, E., Yahav, H., Breviario, F., Dejana, E., et al. (2002). VEGF receptor 2 and the adherens junction as a mechanical transducer in vascular endothelial cells. Proc. Natl. Acad. Sci. U. S. A. 99, 9462–9467. doi:10.1073/pnas.142224299
Sheetz, M. P., Schindler, M., and Koppel, D. E. (1980). Lateral mobility of integral membrane proteins is increased in spherocytic erythrocytes. Nature 285, 510–511. doi:10.1038/285510a0
Sheetz, M. P., and Singer, S. J. (1977). On the mechanism of ATP-induced shape changes in human erythrocyte membranes. I. The role of the spectrin complex. J. Cell Biol. 73, 638–646. doi:10.1083/jcb.73.3.638
Shihata, W. A., Michell, D. L., Andrews, K. L., and Chin-Dusting, J. P. F. (2016). Caveolae: a role in endothelial inflammation and mechanotransduction? Front. Physiol. 7, 628. doi:10.3389/fphys.2016.00628
Shimoda, M. (2019). “Extracellular vesicle-associated MMPs: a modulator of the tissue microenvironment,” in Advances in clinical chemistry (Elsevier), 35–66. doi:10.1016/bs.acc.2018.10.006
Sidi, S., Friedrich, R. W., and Nicolson, T. (2003). NompC TRP channel required for vertebrate sensory hair cell mechanotransduction. Science 301, 96–99. doi:10.1126/science.1084370
Silvani, G., Romanov, V., Cox, C. D., and Martinac, B. (2021). Biomechanical characterization of endothelial cells exposed to shear stress using acoustic force spectroscopy. Front. Bioeng. Biotechnol. 9, 612151. doi:10.3389/fbioe.2021.612151
Simmons, R. D., Kumar, S., and Jo, H. (2016a). The role of endothelial mechanosensitive genes in atherosclerosis and omics approaches. Arch. Biochem. Biophys. 591, 111–131. doi:10.1016/j.abb.2015.11.005
Simmons, R. D., Kumar, S., Thabet, S. R., Sur, S., and Jo, H. (2016b). Omics-based approaches to understand mechanosensitive endothelial biology and atherosclerosis. Wiley Interdiscip. Rev. Syst. Biol. Med. 8, 378–401. doi:10.1002/wsbm.1344
Singh, I., Knezevic, N., Ahmmed, G. U., Kini, V., Malik, A. B., and Mehta, D. (2007). Galphaq-TRPC6-mediated Ca2+ entry induces RhoA activation and resultant endothelial cell shape change in response to thrombin. J. Biol. Chem. 282, 7833–7843. doi:10.1074/jbc.M608288200
Sinha, B., Köster, D., Ruez, R., Gonnord, P., Bastiani, M., Abankwa, D., et al. (2011). Cells respond to mechanical stress by rapid disassembly of caveolae. Cell 144, 402–413. doi:10.1016/j.cell.2010.12.031
Skiba, W., Suszczyk, D., Pawłowska, A., Włodarczyk, K., Pańczyszyn, A., and Wertel, I. (2022). Clinical significance of tie-2-expressing monocytes/macrophages and angiopoietins in the progression of ovarian cancer—state-of-the-art. Cells 11, 3851. doi:10.3390/cells11233851
Smart, C. E., Askarian Amiri, M. E., Wronski, A., Dinger, M. E., Crawford, J., Ovchinnikov, D. A., et al. (2012). Expression and function of the protein tyrosine phosphatase receptor J (PTPRJ) in normal mammary epithelial cells and breast tumors. PLoS ONE 7, e40742. doi:10.1371/journal.pone.0040742
Sonkusare, S. K., Bonev, A. D., Ledoux, J., Liedtke, W., Kotlikoff, M. I., Heppner, T. J., et al. (2012). Elementary Ca2+ signals through endothelial TRPV4 channels regulate vascular function. Science 336, 597–601. doi:10.1126/science.1216283
Sonkusare, S. K., Dalsgaard, T., Bonev, A. D., Hill-Eubanks, D. C., Kotlikoff, M. I., Scott, J. D., et al. (2014). AKAP150-dependent cooperative TRPV4 channel gating is central to endothelium-dependent vasodilation and is disrupted in hypertension. Sci. Signal 7, ra66. doi:10.1126/scisignal.2005052
Sonveaux, P., Martinive, P., DeWever, J., Batova, Z., Daneau, G., Pelat, M., et al. (2004). Caveolin-1 expression is critical for vascular endothelial growth factor-induced ischemic hindlimb collateralization and nitric oxide-mediated angiogenesis. Circ. Res. 95, 154–161. doi:10.1161/01.RES.0000136344.27825.72
Sorescu, G. P., Song, H., Tressel, S. L., Hwang, J., Dikalov, S., Smith, D. A., et al. (2004). Bone morphogenic protein 4 produced in endothelial cells by oscillatory shear stress induces monocyte adhesion by stimulating reactive oxygen species production from a nox1-based NADPH oxidase. Circulation Res. 95, 773–779. doi:10.1161/01.RES.0000145728.22878.45
Spassova, M. A., Hewavitharana, T., Xu, W., Soboloff, J., and Gill, D. L. (2006). A common mechanism underlies stretch activation and receptor activation of TRPC6 channels. Proc. Natl. Acad. Sci. U.S.A. 103, 16586–16591. doi:10.1073/pnas.0606894103
Spiess, M., Hernandez-Varas, P., Oddone, A., Olofsson, H., Blom, H., Waithe, D., et al. (2018). Active and inactive β1 integrins segregate into distinct nanoclusters in focal adhesions. J. Cell Biol. 217, 1929–1940. doi:10.1083/jcb.201707075
Sprovieri, P., and Martino, G. (2018). The role of the carbohydrates in plasmatic membrane. Physiol. Res. 67, 1–11. doi:10.33549/physiolres.933593
Sriram, K., Laughlin, J. G., Rangamani, P., and Tartakovsky, D. M. (2016). Shear-induced nitric oxide production by endothelial cells. Biophysical J. 111, 208–221. doi:10.1016/j.bpj.2016.05.034
Stefanini, L., Ye, F., Snider, A. K., Sarabakhsh, K., Piatt, R., Paul, D. S., et al. (2014). A talin mutant that impairs talin-integrin binding in platelets decelerates αIIbβ3 activation without pathological bleeding. Blood 123, 2722–2731. doi:10.1182/blood-2013-12-543363
Stevens, H. Y., Melchior, B., Bell, K. S., Yun, S., Yeh, J.-C., and Frangos, J. A. (2008). PECAM-1 is a critical mediator of atherosclerosis. Dis. Model Mech. 1, 175–181. doi:10.1242/dmm.000547
Stewart, T., Koval, W. T., Molina, S. A., Bock, S. M., Lillard, J. W., Ross, R. F., et al. (2017). Calibrated flux measurements reveal a nanostructure-stimulated transcytotic pathway. Exp. Cell Res. 355, 153–161. doi:10.1016/j.yexcr.2017.03.065
Stojanovic, A., Marjanovic, J. A., Brovkovych, V. M., Peng, X., Hay, N., Skidgel, R. A., et al. (2006). A phosphoinositide 3-kinase-AKT-nitric oxide-cGMP signaling pathway in stimulating platelet secretion and aggregation. J. Biol. Chem. 281, 16333–16339. doi:10.1074/jbc.M512378200
Stoorvogel, W., Kleijmeer, M. J., Geuze, H. J., and Raposo, G. (2002). The biogenesis and functions of exosomes. Traffic 3, 321–330. doi:10.1034/j.1600-0854.2002.30502.x
Stroev, P. V., Hoskins, P. R., and Easson, W. J. (2007). Distribution of wall shear rate throughout the arterial tree: a case study. Atherosclerosis 191, 276–280. doi:10.1016/j.atherosclerosis.2006.05.029
Strony, J., Beaudoin, A., Brands, D., and Adelman, B. (1993). Analysis of shear stress and hemodynamic factors in a model of coronary artery stenosis and thrombosis. Am. J. Physiology-Heart Circulatory Physiology 265, H1787–H1796. doi:10.1152/ajpheart.1993.265.5.H1787
Strotmann, R., Harteneck, C., Nunnenmacher, K., Schultz, G., and Plant, T. D. (2000). OTRPC4, a nonselective cation channel that confers sensitivity to extracellular osmolarity. Nat. Cell Biol. 2, 695–702. doi:10.1038/35036318
Stupack, D. G., and Cheresh, D. A. (2002). ECM remodeling regulates angiogenesis: endothelial integrins look for new ligands. Sci. STKE 2002, pe7. doi:10.1126/stke.2002.119.pe7
Su, C., Mo, J., Dong, S., Liao, Z., Zhang, B., and Zhu, P. (2024). Integrinβ-1 in disorders and cancers: molecular mechanisms and therapeutic targets. Cell Commun. Signal 22, 71. doi:10.1186/s12964-023-01338-3
Su, D., Xu, H., Feng, J., Gao, Y., Gu, L., Ying, L., et al. (2012). PDCD6 is an independent predictor of progression free survival in epithelial ovarian cancer. J. Transl. Med. 10, 31. doi:10.1186/1479-5876-10-31
Su, Q., Mehta, S., and Zhang, J. (2021). Liquid-liquid phase separation: orchestrating cell signaling through time and space. Mol. Cell 81, 4137–4146. doi:10.1016/j.molcel.2021.09.010
Su, Y., Xia, W., Li, J., Walz, T., Humphries, M. J., Vestweber, D., et al. (2016). Relating conformation to function in integrin α 5 β 1. Proc. Natl. Acad. Sci. U.S.A. 113, E3872–E3881. doi:10.1073/pnas.1605074113
Sukriti, S., Tauseef, M., Yazbeck, P., and Mehta, D. (2014). Mechanisms regulating endothelial permeability. Pulm. Circ. 4, 535–551. doi:10.1086/677356
Sullivan, M. N., Gonzales, A. L., Pires, P. W., Bruhl, A., Leo, M. D., Li, W., et al. (2015). Localized TRPA1 channel Ca2+ signals stimulated by reactive oxygen species promote cerebral artery dilation. Sci. Signal 8, ra2. doi:10.1126/scisignal.2005659
Sun, R. J., Muller, S., Zhuang, F. Y., Stoltz, J. F., and Wang, X. (2003). Caveolin-1 redistribution in human endothelial cells induced by laminar flow and cytokine. Biorheology 40, 31–39.
Sun, S., Muller, J., Stoltz, X., and Wang, R. (2002). Shear stress induces caveolin-1 translocation in cultured endothelial cells. Eur. Biophysics J. 30, 605–611. doi:10.1007/s00249-001-0195-x
Sun, X., Fu, Y., Gu, M., Zhang, L., Li, D., Li, H., et al. (2016a). Activation of integrin α5 mediated by flow requires its translocation to membrane lipid rafts in vascular endothelial cells. Proc. Natl. Acad. Sci. U.S.A. 113, 769–774. doi:10.1073/pnas.1524523113
Sun, Z., Costell, M., and Fässler, R. (2019). Integrin activation by talin, kindlin and mechanical forces. Nat. Cell Biol. 21, 25–31. doi:10.1038/s41556-018-0234-9
Sun, Z., Guo, S. S., and Fässler, R. (2016b). Integrin-mediated mechanotransduction. J. Cell Biol. 215, 445–456. doi:10.1083/jcb.201609037
Suri, C., Jones, P. F., Patan, S., Bartunkova, S., Maisonpierre, P. C., Davis, S., et al. (1996). Requisite role of angiopoietin-1, a ligand for the TIE2 receptor, during embryonic angiogenesis. Cell 87, 1171–1180. doi:10.1016/S0092-8674(00)81813-9
Suzuki, T., and Fujihira, H. (2021). “Folding and quality control of glycoproteins,” in Comprehensive glycoscience (Elsevier), 1–28. doi:10.1016/B978-0-12-409547-2.14947-9
Swain, S. M., and Liddle, R. A. (2021). Piezo1 acts upstream of TRPV4 to induce pathological changes in endothelial cells due to shear stress. J. Biol. Chem. 296, 100171. doi:10.1074/jbc.RA120.015059
Syeda, R., Florendo, M. N., Cox, C. D., Kefauver, J. M., Santos, J. S., Martinac, B., et al. (2016). Piezo1 channels are inherently mechanosensitive. Cell Rep. 17, 1739–1746. doi:10.1016/j.celrep.2016.10.033
Tadokoro, S., Shattil, S. J., Eto, K., Tai, V., Liddington, R. C., De Pereda, J. M., et al. (2003). Talin binding to integrin beta tails: a final common step in integrin activation. Science 302, 103–106. doi:10.1126/science.1086652
Tafazoli, F., Holmström, A., Forsberg, Å., and Magnusson, K.-E. (2000). Apically exposed, tight junction-associated β1-integrins allow binding and YopE-mediated perturbation of epithelial barriers by wild-type Yersinia bacteria. Infect. Immun. 68, 5335–5343. doi:10.1128/IAI.68.9.5335-5343.2000
Takada, Y., Kato, C., Kondo, S., Korenaga, R., and Ando, J. (1997). Cloning of cDNAs encoding G protein-coupled receptor expressed in human endothelial cells exposed to fluid shear stress. Biochem. Biophys. Res. Commun. 240, 737–741. doi:10.1006/bbrc.1997.7734
Takada, Y., Ye, X., and Simon, S. (2007). The integrins. Genome Biol. 8, 215. doi:10.1186/gb-2007-8-5-215
Takagi, J., and Springer, T. A. (2002). Integrin activation and structural rearrangement. Immunol. Rev. 186, 141–163. doi:10.1034/j.1600-065X.2002.18613.x
Takahashi, H., and Shibuya, M. (2005). The vascular endothelial growth factor (VEGF)/VEGF receptor system and its role under physiological and pathological conditions. Clin. Sci. 109, 227–241. doi:10.1042/CS20040370
Tamargo, I. A., Baek, K. I., Kim, Y., Park, C., and Jo, H. (2023). Flow-induced reprogramming of endothelial cells in atherosclerosis. Nat. Rev. Cardiol. 20, 738–753. doi:10.1038/s41569-023-00883-1
Tan, S., Xia, L., Yi, P., Han, Y., Tang, L., Pan, Q., et al. (2020). Exosomal miRNAs in tumor microenvironment. J. Exp. Clin. Cancer Res. 39, 67. doi:10.1186/s13046-020-01570-6
Tang, S. Y., Monslow, J., Todd, L., Lawson, J., Puré, E., and FitzGerald, G. A. (2014). Cyclooxygenase-2 in endothelial and vascular smooth muscle cells restrains atherogenesis in hyperlipidemic mice. Circulation 129, 1761–1769. doi:10.1161/CIRCULATIONAHA.113.007913
Tapia, R., and Velasco, I. (1997). Ruthenium red as a tool to study calcium channels, neuronal death and the function of neural pathways. Neurochem. Int. 30, 137–147. doi:10.1016/S0197-0186(96)00056-3
Tarbell, J. M., and Pahakis, M. Y. (2006). Mechanotransduction and the glycocalyx. J. Intern. Med. 259, 339–350. doi:10.1111/j.1365-2796.2006.01620.x
Tarbell, J. M., Shi, Z.-D., Dunn, J., and Jo, H. (2014). Fluid mechanics, arterial disease, and gene expression. Annu. Rev. Fluid Mech. 46, 591–614. doi:10.1146/annurev-fluid-010313-141309
Tarjus, A., Maase, M., Jeggle, P., Martinez-Martinez, E., Fassot, C., Loufrani, L., et al. (2017). The endothelial αENaC contributes to vascular endothelial function in vivo. PLoS ONE 12, e0185319. doi:10.1371/journal.pone.0185319
Thakore, P., Alvarado, M. G., Ali, S., Mughal, A., Pires, P. W., Yamasaki, E., et al. (2021). Brain endothelial cell TRPA1 channels initiate neurovascular coupling. Elife 10, e63040. doi:10.7554/eLife.63040
Thien, N. D., Hai-Nam, N., Anh, D. T., and Baecker, D. (2024). Piezo1 and its inhibitors: overview and perspectives. Eur. J. Med. Chem. 273, 116502. doi:10.1016/j.ejmech.2024.116502
Thodeti, C. K., Matthews, B., Ravi, A., Mammoto, A., Ghosh, K., Bracha, A. L., et al. (2009). TRPV4 channels mediate cyclic strain–induced endothelial cell reorientation through integrin-to-integrin signaling. Circulation Res. 104, 1123–1130. doi:10.1161/CIRCRESAHA.108.192930
Thorin-Trescases, N., Bartolotta, T., Hyman, N., Penar, P. L., Walters, C. L., Bevan, R. D., et al. (1997). Diameter dependence of myogenic tone of human pial arteries: possible relation to distensibility. Stroke 28, 2486–2492. doi:10.1161/01.STR.28.12.2486
Thorneloe, K. S., and Nelson, M. T. (2005). Ion channels in smooth muscle: regulators of intracellular calcium and contractility. Can. J. Physiol. Pharmacol. 83, 215–242. doi:10.1139/y05-016
Thoumine, O., Nerem, R. M., and Girard, P. R. (1995). Oscillatory shear stress and hydrostatic pressure modulate cell-matrix attachment proteins in cultured endothelial cells. Vitro Cell Dev. Biol. Anim. 31, 45–54. doi:10.1007/BF02631337
Tian, Y., Gawlak, G., O’Donnell, J. J., Birukova, A. A., and Birukov, K. G. (2016). Activation of vascular endothelial growth factor (VEGF) receptor 2 mediates endothelial permeability caused by cyclic stretch. J. Biol. Chem. 291, 10032–10045. doi:10.1074/jbc.M115.690487
Tinken, T. M., Thijssen, D. H. J., Hopkins, N., Black, M. A., Dawson, E. A., Minson, C. T., et al. (2009). Impact of shear rate modulation on vascular function in humans. Hypertension 54, 278–285. doi:10.1161/HYPERTENSIONAHA.109.134361
Tkach, M., and Théry, C. (2016). Communication by extracellular vesicles: where we are and where we need to go. Cell 164, 1226–1232. doi:10.1016/j.cell.2016.01.043
Tobin, D. M., Madsen, D. M., Kahn-Kirby, A., Peckol, E. L., Moulder, G., Barstead, R., et al. (2002). Combinatorial expression of TRPV channel proteins defines their sensory functions and subcellular localization in C. elegans neurons. Neuron 35, 307–318. doi:10.1016/S0896-6273(02)00757-2
Tomita, S., Nakanishi, N., Ogata, T., Higuchi, Y., Sakamoto, A., Tsuji, Y., et al. (2024). The Cavin-1/Caveolin-1 interaction attenuates BMP/Smad signaling in pulmonary hypertension by interfering with BMPR2/Caveolin-1 binding. Commun. Biol. 7, 40. doi:10.1038/s42003-023-05693-2
Toth, P., Tarantini, S., Csiszar, A., and Ungvari, Z. (2017). Functional vascular contributions to cognitive impairment and dementia: mechanisms and consequences of cerebral autoregulatory dysfunction, endothelial impairment, and neurovascular uncoupling in aging. Am. J. Physiology-Heart Circulatory Physiology 312, H1–H20. doi:10.1152/ajpheart.00581.2016
Toth, P., Tucsek, Z., Sosnowska, D., Gautam, T., Mitschelen, M., Tarantini, S., et al. (2013). Age-related autoregulatory dysfunction and cerebromicrovascular injury in mice with angiotensin II-induced hypertension. J. Cereb. Blood Flow. Metab. 33, 1732–1742. doi:10.1038/jcbfm.2013.143
Tran, N., Garcia, T., Aniqa, M., Ali, S., Ally, A., and Nauli, S. M. (2022). Endothelial nitric oxide synthase (eNOS) and the cardiovascular system: in physiology and in disease states. Am. J. Biomed. Sci. Res. 15, 153–177.
Tsaryk, R., Yucel, N., Leonard, E. V., Diaz, N., Bondareva, O., Odenthal-Schnittler, M., et al. (2022). Shear stress switches the association of endothelial enhancers from ETV/ETS to KLF transcription factor binding sites. Sci. Rep. 12, 4795. doi:10.1038/s41598-022-08645-8
Turner, K. R., Adams, C., Staelens, S., Deckmyn, H., and San Antonio, J. (2020). Crucial role for endothelial cell α2β1 integrin receptor clustering in collagen-induced angiogenesis. Anatomical Rec. 303, 1604–1618. doi:10.1002/ar.24277
Tzima, E., del Pozo, M. A., Shattil, S. J., Chien, S., and Schwartz, M. A. (2001). Activation of integrins in endothelial cells by fluid shear stress mediates Rho-dependent cytoskeletal alignment. EMBO J. 20, 4639–4647. doi:10.1093/emboj/20.17.4639
Tzima, E., Irani-Tehrani, M., Kiosses, W. B., Dejana, E., Schultz, D. A., Engelhardt, B., et al. (2005). A mechanosensory complex that mediates the endothelial cell response to fluid shear stress. Nature 437, 426–431. doi:10.1038/nature03952
Uchida, T., Shimizu, S., Yamagishi, R., Tokuoka, S. M., Kita, Y., Honjo, M., et al. (2021). Mechanical stretch induces Ca2+ influx and extracellular release of PGE2 through Piezo1 activation in trabecular meshwork cells. Sci. Rep. 11, 4044. doi:10.1038/s41598-021-83713-z
Uehara, K., and Uehara, A. (2014). Integrin αvβ5 in endothelial cells of rat splenic sinus: an immunohistochemical and ultrastructural study. Cell Tissue Res. 356, 183–193. doi:10.1007/s00441-014-1796-x
Uematsu, M., Ohara, Y., Navas, J. P., Nishida, K., Murphy, T. J., Alexander, R. W., et al. (1995). Regulation of endothelial cell nitric oxide synthase mRNA expression by shear stress. Am. J. Physiology-Cell Physiology 269, C1371–C1378. doi:10.1152/ajpcell.1995.269.6.C1371
Urbich, C., Dernbach, E., Reissner, A., Vasa, M., Zeiher, A. M., and Dimmeler, S. (2002). Shear stress–induced endothelial cell migration involves integrin signaling via the fibronectin receptor subunits α 5 and β 1. ATVB 22, 69–75. doi:10.1161/hq0102.101518
Valentini, E., Di Martile, M., Del Bufalo, D., and D’Aguanno, S. (2021). SEMAPHORINS and their receptors: focus on the crosstalk between melanoma and hypoxia. J. Exp. Clin. Cancer Res. 40, 131. doi:10.1186/s13046-021-01929-3
Vallance, P. (2001). Importance of asymmetrical dimethylarginine in cardiovascular risk. Lancet 358, 2096–2097. doi:10.1016/S0140-6736(01)07229-4
Vallance, P., and Chan, N. (2001). Endothelial function and nitric oxide: clinical relevance. Heart 85, 342–350. doi:10.1136/heart.85.3.342
Van Der Flier, A., Badu-Nkansah, K., Whittaker, C. A., Crowley, D., Bronson, R. T., Lacy-Hulbert, A., et al. (2010). Endothelial alpha5 and alphav integrins cooperate in remodeling of the vasculature during development. Development 137, 2439–2449. doi:10.1242/dev.049551
Van der Heiden, K., Groenendijk, B. C. W., Hierck, B. P., Hogers, B., Koerten, H. K., Mommaas, A. M., et al. (2006). Monocilia on chicken embryonic endocardium in low shear stress areas. Dev. Dyn. 235, 19–28. doi:10.1002/dvdy.20557
Van der Heiden, K., Hierck, B. P., Krams, R., de Crom, R., Cheng, C., Baiker, M., et al. (2008). Endothelial primary cilia in areas of disturbed flow are at the base of atherosclerosis. Atherosclerosis 196, 542–550. doi:10.1016/j.atherosclerosis.2007.05.030
Vanlandewijck, M., He, L., Mäe, M. A., Andrae, J., Ando, K., Del Gaudio, F., et al. (2018). A molecular atlas of cell types and zonation in the brain vasculature. Nature 554, 475–480. doi:10.1038/nature25739
Veikkola, T., Jussila, L., Makinen, T., Karpanen, T., Jeltsch, M., Petrova, T. V., et al. (2001). Signalling via vascular endothelial growth factor receptor-3 is sufficient for lymphangiogenesis in transgenic mice. EMBO J. 20, 1223–1231. doi:10.1093/emboj/20.6.1223
Viñals, F., and Pouysségur, J. (2001). Transforming growth factor beta1 (TGF-beta1) promotes endothelial cell survival during in vitro angiogenesis via an autocrine mechanism implicating TGF-alpha signaling. Mol. Cell. Biol. 21, 7218–7230. doi:10.1128/MCB.21.21.7218-7230.2001
Vion, A.-C., Perovic, T., Petit, C., Hollfinger, I., Bartels-Klein, E., Frampton, E., et al. (2021). Endothelial cell orientation and polarity are controlled by shear stress and VEGF through distinct signaling pathways. Front. Physiol. 11, 623769. doi:10.3389/fphys.2020.623769
Vogel, V., and Sheetz, M. (2006). Local force and geometry sensing regulate cell functions. Nat. Rev. Mol. Cell Biol. 7, 265–275. doi:10.1038/nrm1890
Volkers, L., Mechioukhi, Y., and Coste, B. (2015). Piezo channels: from structure to function. Pflugers Arch. - Eur. J. Physiol. 467, 95–99. doi:10.1007/s00424-014-1578-z
Vozzi, F., Bianchi, F., Ahluwalia, A., and Domenici, C. (2014). Hydrostatic pressure and shear stress affect endothelin-1 and nitric oxide release by endothelial cells in bioreactors. Biotechnol. J. 9, 146–154. doi:10.1002/biot.201300016
Walsh, L., Ryu, J., Bock, S., Koval, M., Mauro, T., Ross, R., et al. (2015). Nanotopography facilitates in vivo transdermal delivery of high molecular weight therapeutics through an integrin-dependent mechanism. Nano Lett. 15, 2434–2441. doi:10.1021/nl504829f
Wang, C., Baker, B. M., Chen, C. S., and Schwartz, M. A. (2013). Endothelial cell sensing of flow direction. ATVB 33, 2130–2136. doi:10.1161/ATVBAHA.113.301826
Wang, C., Qu, Y., Suo, R., and Zhu, Y. (2019). Long non-coding RNA MALAT1 regulates angiogenesis following oxygen-glucose deprivation/reoxygenation. J. Cell. Mol. Medi 23, 2970–2983. doi:10.1111/jcmm.14204
Wang, C., Wu, H., Xing, Y., Ye, Y., He, F., Yin, Q., et al. (2022a). Endothelial-derived extracellular microRNA-92a promotes arterial stiffness by regulating phenotype changes of vascular smooth muscle cells. Sci. Rep. 12, 344. doi:10.1038/s41598-021-04341-1
Wang, J., Jiang, J., Yang, X., Wang, L., and Xiao, B. (2020). Tethering Piezo channels to the actin cytoskeleton for mechanogating via the E-cadherin-β-catenin mechanotransduction complex. Neuroscience. doi:10.1101/2020.05.12.092148
Wang, J., Jiang, J., Yang, X., Zhou, G., Wang, L., and Xiao, B. (2022b). Tethering Piezo channels to the actin cytoskeleton for mechanogating via the cadherin-β-catenin mechanotransduction complex. Cell Rep. 38, 110342. doi:10.1016/j.celrep.2022.110342
Wang, J., Xie, S., Li, N., Zhang, T., Yao, W., Zhao, H., et al. (2022c). Matrix stiffness exacerbates the proinflammatory responses of vascular smooth muscle cell through the DDR1-DNMT1 mechanotransduction axis. Bioact. Mater. 17, 406–424. doi:10.1016/j.bioactmat.2022.01.012
Wang, K.-C., Yeh, Y.-T., Nguyen, P., Limqueco, E., Lopez, J., Thorossian, S., et al. (2016a). Flow-dependent YAP/TAZ activities regulate endothelial phenotypes and atherosclerosis. Proc. Natl. Acad. Sci. U.S.A. 113, 11525–11530. doi:10.1073/pnas.1613121113
Wang, L., Luo, J.-Y., Li, B., Tian, X. Y., Chen, L.-J., Huang, Y., et al. (2016b). Integrin-YAP/TAZ-JNK cascade mediates atheroprotective effect of unidirectional shear flow. Nature 540, 579–582. doi:10.1038/nature20602
Wang, N., Miao, H., Li, Y.-S., Zhang, P., Haga, J. H., Hu, Y., et al. (2006). Shear stress regulation of Krüppel-like factor 2 expression is flow pattern-specific. Biochem. Biophysical Res. Commun. 341, 1244–1251. doi:10.1016/j.bbrc.2006.01.089
Wang, S., Chennupati, R., Kaur, H., Iring, A., Wettschureck, N., and Offermanns, S. (2016c). Endothelial cation channel PIEZO1 controls blood pressure by mediating flow-induced ATP release. J. Clin. Investigation 126, 4527–4536. doi:10.1172/JCI87343
Wang, S., Iring, A., Strilic, B., Albarrán Juárez, J., Kaur, H., Troidl, K., et al. (2015). P2Y₂ and Gq/G11 control blood pressure by mediating endothelial mechanotransduction. J. Clin. Invest. 125, 3077–3086. doi:10.1172/JCI81067
Wang, S., Jia, L., Zhou, H., Shi, W., and Zhang, J. (2009). Knockdown of caveolin-1 by siRNA inhibits the transformation of mouse hepatoma H22 cells in vitro and in vivo. Oligonucleotides 19, 81–88. doi:10.1089/oli.2008.0166
Wang, S.-H., Chang, J. S., Hsiao, J.-R., Yen, Y.-C., Jiang, S. S., Liu, S.-H., et al. (2017a). Tumour cell-derived WNT5B modulates in vitro lymphangiogenesis via induction of partial endothelial-mesenchymal transition of lymphatic endothelial cells. Oncogene 36, 1503–1515. doi:10.1038/onc.2016.317
Wang, X., and Khalil, R. A. (2018). “Matrix metalloproteinases, vascular remodeling, and vascular disease,” in Advances in pharmacology (Elsevier), 241–330. doi:10.1016/bs.apha.2017.08.002
Wang, Y., Cui, L., Xu, H., Liu, S., Zhu, F., Yan, F., et al. (2017b). TRPV1 agonism inhibits endothelial cell inflammation via activation of eNOS/NO pathway. Atherosclerosis 260, 13–19. doi:10.1016/j.atherosclerosis.2017.03.016
Warnock, D. G., Kusche-Vihrog, K., Tarjus, A., Sheng, S., Oberleithner, H., Kleyman, T. R., et al. (2014). Blood pressure and amiloride-sensitive sodium channels in vascular and renal cells. Nat. Rev. Nephrol. 10, 146–157. doi:10.1038/nrneph.2013.275
Warren, C. M., Ziyad, S., Briot, A., Der, A., and Iruela-Arispe, M. L. (2014). A ligand-independent VEGFR2 signaling pathway limits angiogenic responses in diabetes. Sci. Signal. 7, ra1. doi:10.1126/scisignal.2004235
Watanabe, H., Vriens, J., Prenen, J., Droogmans, G., Voets, T., and Nilius, B. (2003). Anandamide and arachidonic acid use epoxyeicosatrienoic acids to activate TRPV4 channels. Nature 424, 434–438. doi:10.1038/nature01807
Watanabe, H., Vriens, J., Suh, S. H., Benham, C. D., Droogmans, G., and Nilius, B. (2002). Heat-evoked activation of TRPV4 channels in a HEK293 cell expression system and in native mouse aorta endothelial cells. J. Biol. Chem. 277, 47044–47051. doi:10.1074/jbc.M208277200
Wautier, J.-L., and Wautier, M.-P. (2021). Endothelial cell participation in inflammatory reaction. IJMS 22, 6341. doi:10.3390/ijms22126341
Weber, M., Hagedorn, C. H., Harrison, D. G., and Searles, C. D. (2005). Laminar shear stress and 3′ polyadenylation of eNOS mRNA. Circulation Res. 96, 1161–1168. doi:10.1161/01.RES.0000170651.72198.fa
Weenink, R. P., and Wingelaar, T. T. (2021). The circulatory effects of increased hydrostatic pressure due to immersion and submersion. Front. Physiol. 12, 699493. doi:10.3389/fphys.2021.699493
Wegener, K. L., Partridge, A. W., Han, J., Pickford, A. R., Liddington, R. C., Ginsberg, M. H., et al. (2007). Structural basis of integrin activation by talin. Cell 128, 171–182. doi:10.1016/j.cell.2006.10.048
Weinbaum, S., Tarbell, J. M., and Damiano, E. R. (2007). The structure and function of the endothelial glycocalyx layer. Annu. Rev. Biomed. Eng. 9, 121–167. doi:10.1146/annurev.bioeng.9.060906.151959
Weinbaum, S., Zhang, X., Han, Y., Vink, H., and Cowin, S. C. (2003). Mechanotransduction and flow across the endothelial glycocalyx. Proc. Natl. Acad. Sci. U.S.A. 100, 7988–7995. doi:10.1073/pnas.1332808100
Welch-Reardon, K. M., Wu, N., and Hughes, C. C. W. (2015). A role for partial endothelial–mesenchymal transitions in angiogenesis? ATVB 35, 303–308. doi:10.1161/ATVBAHA.114.303220
Welsh, D. G., Morielli, A. D., Nelson, M. T., and Brayden, J. E. (2002). Transient receptor potential channels regulate myogenic tone of resistance arteries. Circulation Res. 90, 248–250. doi:10.1161/hh0302.105662
Welsh, D. G., Nelson, M. T., Eckman, D. M., and Brayden, J. E. (2000). Swelling-activated cation channels mediate depolarization of rat cerebrovascular smooth muscle by hyposmolarity and intravascular pressure. J. Physiology 527, 139–148. doi:10.1111/j.1469-7793.2000.t01-1-00139.x
Wheatcroft, S. B., Williams, I. L., Shah, A. M., and Kearney, M. T. (2003). Pathophysiological implications of insulin resistance on vascular endothelial function. Diabet. Med. 20, 255–268. doi:10.1046/j.1464-5491.2003.00869.x
White, J. P. M., Cibelli, M., Urban, L., Nilius, B., McGeown, J. G., and Nagy, I. (2016). TRPV4: molecular conductor of a diverse orchestra. Physiol. Rev. 96, 911–973. doi:10.1152/physrev.00016.2015
Wierda, R. J., Rietveld, I. M., Van Eggermond, M. C. J. A., Belien, J. A. M., Van Zwet, E. W., Lindeman, J. H. N., et al. (2015). Global histone H3 lysine 27 triple methylation levels are reduced in vessels with advanced atherosclerotic plaques. Life Sci. 129, 3–9. doi:10.1016/j.lfs.2014.10.010
Wilcox, J. N., Smith, K. M., Williams, L. T., Schwartz, S. M., and Gordon, D. (1988). Platelet-derived growth factor mRNA detection in human atherosclerotic plaques by in situ hybridization. J. Clin. Invest. 82, 1134–1143. doi:10.1172/JCI113671
Wilson, S. H., Caplice, N. M., Simari, R. D., Holmes, D. R., Carlson, P. J., and Lerman, A. (2000). Activated nuclear factor-kappaB is present in the coronary vasculature in experimental hypercholesterolemia. Atherosclerosis 148, 23–30. doi:10.1016/S0021-9150(99)00211-7
Woo, K. V., Qu, X., Babaev, V. R., Linton, M. F., Guzman, R. J., Fazio, S., et al. (2011). Tie1 attenuation reduces murine atherosclerosis in a dose-dependent and shear stress-specific manner. J. Clin. Invest. 121, 1624–1635. doi:10.1172/JCI42040
Woo, M., Hakem, R., Furlonger, C., Hakem, A., Duncan, G. S., Sasaki, T., et al. (2003). Caspase-3 regulates cell cycle in B cells: a consequence of substrate specificity. Nat. Immunol. 4, 1016–1022. doi:10.1038/ni976
Woo, S.-H., Ranade, S., Weyer, A. D., Dubin, A. E., Baba, Y., Qiu, Z., et al. (2014). Piezo2 is required for Merkel-cell mechanotransduction. Nature 509, 622–626. doi:10.1038/nature13251
Wu, D. M., Minami, M., Kawamura, H., and Puro, D. G. (2006). Electrotonic transmission within pericyte-containing retinal microvessels. Microcirculation 13, 353–363. doi:10.1080/10739680600745778
Wu, F., Li, F., Lin, X., Xu, F., Cui, R.-R., Zhong, J.-Y., et al. (2019). Exosomes increased angiogenesis in papillary thyroid cancer microenvironment. Endocrine-Related Cancer 26, 525–538. doi:10.1530/ERC-19-0008
Wu, J., Liu, W., Sousa, E., Qiu, Y., Pittman, D. D., Maganti, V., et al. (2007). Proteomic identification of endothelial proteins isolated in situ from atherosclerotic aorta via systemic perfusion. J. Proteome Res. 6, 4728–4736. doi:10.1021/pr070537c
Wu, W., Xiao, H., Laguna-Fernandez, A., Villarreal, G., Wang, K.-C., Geary, G. G., et al. (2011). Flow-dependent regulation of krüppel-like factor 2 is mediated by MicroRNA-92a. Circulation 124, 633–641. doi:10.1161/CIRCULATIONAHA.110.005108
Xanthis, I., Souilhol, C., Serbanovic-Canic, J., Roddie, H., Kalli, A. C., Fragiadaki, M., et al. (2019). β1 integrin is a sensor of blood flow direction. J. Cell Sci. 132, jcs229542. doi:10.1242/jcs.229542
Xiao, E., Yang, H. Q., Gan, Y.-H., Duan, D.-H., He, L.-H., Guo, Y., et al. (2015a). Brief reports: TRPM7 senses mechanical stimulation inducing osteogenesis in human bone marrow mesenchymal stem cells. Stem Cells 33, 615–621. doi:10.1002/stem.1858
Xiao, L., and Dudley, A. C. (2017). Fine-tuning vascular fate during endothelial–mesenchymal transition. J. Pathology 241, 25–35. doi:10.1002/path.4814
Xiao, L., Kim, D. J., Davis, C. L., McCann, J. V., Dunleavey, J. M., Vanderlinden, A. K., et al. (2015b). Tumor endothelial cells with distinct patterns of tgfβ-driven endothelial-to-mesenchymal transition. Cancer Res. 75, 1244–1254. doi:10.1158/0008-5472.CAN-14-1616
Xu, J., Mathur, J., Vessières, E., Hammack, S., Nonomura, K., Favre, J., et al. (2018). GPR68 senses flow and is essential for vascular physiology. Cell 173, 762–775. doi:10.1016/j.cell.2018.03.076
Xu, S., Xu, Y., Liu, P., Zhang, S., Liu, H., Slavin, S., et al. (2019a). The novel coronary artery disease risk gene JCAD/KIAA1462 promotes endothelial dysfunction and atherosclerosis. Eur. Heart J. 40, 2398–2408. doi:10.1093/eurheartj/ehz303
Xu, Y., Xu, J., Shan, W., Liu, M., Cui, Y., Li, L., et al. (2016). The transport mechanism of integrin αvβ3 receptor targeting nanoparticles in Caco-2 cells. Int. J. Pharm. 500, 42–53. doi:10.1016/j.ijpharm.2016.01.028
Xu, Z., Isaji, T., Fukuda, T., Wang, Y., and Gu, J. (2019b). O-GlcNAcylation regulates integrin-mediated cell adhesion and migration via formation of focal adhesion complexes. J. Biol. Chem. 294, 3117–3124. doi:10.1074/jbc.RA118.005923
Yago, T., Petrich, B. G., Zhang, N., Liu, Z., Shao, B., Ginsberg, M. H., et al. (2015). Blocking neutrophil integrin activation prevents ischemia-reperfusion injury. J. Exp. Med. 212, 1267–1281. doi:10.1084/jem.20142358
Yamada, S., Pokutta, S., Drees, F., Weis, W. I., and Nelson, W. J. (2005). Deconstructing the cadherin-catenin-actin complex. Cell 123, 889–901. doi:10.1016/j.cell.2005.09.020
Yamamoto, H., Ehling, M., Kato, K., Kanai, K., Van Lessen, M., Frye, M., et al. (2015). Integrin β1 controls VE-cadherin localization and blood vessel stability. Nat. Commun. 6, 6429. doi:10.1038/ncomms7429
Yamamoto, K., and Ando, J. (2013). Endothelial cell and model membranes respond to shear stress by rapidly decreasing the order of their lipid phases. J. Cell Sci. 126, 1227–1234. doi:10.1242/jcs.119628
Yamamoto, K., and Ando, J. (2015). Vascular endothelial cell membranes differentiate between stretch and shear stress through transitions in their lipid phases. Am. J. Physiol. Heart Circ. Physiol. 309, H1178–H1185. doi:10.1152/ajpheart.00241.2015
Yamamoto, K., Furuya, K., Nakamura, M., Kobatake, E., Sokabe, M., and Ando, J. (2011). Visualization of flow-induced ATP release and triggering of Ca2+ waves at caveolae in vascular endothelial cells. J. Cell Sci. 124, 3477–3483. doi:10.1242/jcs.087221
Yamamoto, K., Korenaga, R., Kamiya, A., and Ando, J. (2000). Fluid shear stress activates Ca 2+ influx into human endothelial cells via P2X4 purinoceptors. Circulation Res. 87, 385–391. doi:10.1161/01.RES.87.5.385
Yamamoto, Y., Imaeda, K., and Suzuki, H. (1999). Endothelium-dependent hyperpolarization and intercellular electrical coupling in Guinea-pig mesenteric arterioles. J. Physiol. 514 (Pt 2), 505–513. doi:10.1111/j.1469-7793.1999.505ae.x
Yanagisawa, M., Kurihara, H., Kimura, S., Tomobe, Y., Kobayashi, M., Mitsui, Y., et al. (1988). A novel potent vasoconstrictor peptide produced by vascular endothelial cells. Nature 332, 411–415. doi:10.1038/332411a0
Yang, B., Radel, C., Hughes, D., Kelemen, S., and Rizzo, V. (2011). p190 RhoGTPase-activating protein links the β1 integrin/caveolin-1 mechanosignaling complex to RhoA and actin remodeling. ATVB 31, 376–383. doi:10.1161/ATVBAHA.110.217794
Yang, B., and Rizzo, V. (2013). Shear stress activates eNOS at the endothelial apical surface through β1 containing integrins and caveolae. Cel. Mol. Bioeng. 6, 346–354. doi:10.1007/s12195-013-0276-9
Yang, X., Zeng, H., Wang, L., Luo, S., and Zhou, Y. (2022). Activation of Piezo1 downregulates renin in juxtaglomerular cells and contributes to blood pressure homeostasis. Cell Biosci. 12, 197. doi:10.1186/s13578-022-00931-2
Ye, F., Kim, C., and Ginsberg, M. H. (2011). Molecular mechanism of inside-out integrin regulation. J. Thrombosis Haemostasis 9, 20–25. doi:10.1111/j.1538-7836.2011.04355.x
Ye, F., Kim, C., and Ginsberg, M. H. (2012). Reconstruction of integrin activation. Blood 119, 26–33. doi:10.1182/blood-2011-04-292128
Ye, Y., Barghouth, M., Dou, H., Luan, C., Wang, Y., Karagiannopoulos, A., et al. (2022). A critical role of the mechanosensor PIEZO1 in glucose-induced insulin secretion in pancreatic β-cells. Nat. Commun. 13, 4237. doi:10.1038/s41467-022-31103-y
y Schnitzler, M. M., Storch, U., Meibers, S., Nurwakagari, P., Breit, A., Essin, K., et al. (2008). Gq-coupled receptors as mechanosensors mediating myogenic vasoconstriction. EMBO J. 27, 3092–3103. doi:10.1038/emboj.2008.233
Yu, J., Bergaya, S., Murata, T., Alp, I. F., Bauer, M. P., Lin, M. I., et al. (2006). Direct evidence for the role of caveolin-1 and caveolae in mechanotransduction and remodeling of blood vessels. J. Clin. Invest. 116, 1284–1291. doi:10.1172/JCI27100
Yun, S., Budatha, M., Dahlman, J. E., Coon, B. G., Cameron, R. T., Langer, R., et al. (2016). Interaction between integrin α5 and PDE4D regulates endothelial inflammatory signalling. Nat. Cell Biol. 18, 1043–1053. doi:10.1038/ncb3405
Zarins, C. K., Giddens, D. P., Bharadvaj, B. K., Sottiurai, V. S., Mabon, R. F., and Glagov, S. (1983). Carotid bifurcation atherosclerosis. Quantitative correlation of plaque localization with flow velocity profiles and wall shear stress. Circulation Res. 53, 502–514. doi:10.1161/01.RES.53.4.502
Zaritsky, J. J., Eckman, D. M., Wellman, G. C., Nelson, M. T., and Schwarz, T. L. (2000). Targeted disruption of Kir2.1 and Kir2.2 genes reveals the essential role of the inwardly rectifying K(+) current in K(+)-mediated vasodilation. Circ. Res. 87, 160–166. doi:10.1161/01.res.87.2.160
Zeisberg, E. M., Potenta, S., Xie, L., Zeisberg, M., and Kalluri, R. (2007). Discovery of endothelial to mesenchymal transition as a source for carcinoma-associated fibroblasts. Cancer Res. 67, 10123–10128. doi:10.1158/0008-5472.CAN-07-3127
Zeng, Y., Zhang, X. F., Fu, B. M., and Tarbell, J. M. (2018). “The role of endothelial surface glycocalyx in mechanosensing and transduction,” in Molecular, cellular, and tissue engineering of the vascular system. Editors B. M. Fu,, and N. T. Wright (Cham: Springer International Publishing), 1–27. doi:10.1007/978-3-319-96445-4_1
Zhang, H., Zhao, Z., Pang, X., Yang, J., Yu, H., Zhang, Y., et al. (2017). Genistein protects against ox-LDL-induced inflammation through MicroRNA-155/SOCS1-mediated repression of NF-ĸB signaling pathway in HUVECs. Inflammation 40, 1450–1459. doi:10.1007/s10753-017-0588-3
Zhang, J., Yuan, H., Chen, S., and Zhang, Z. (2022). Detrimental or beneficial: role of endothelial ENaC in vascular function. J. Cell. Physiology 237, 29–48. doi:10.1002/jcp.30505
Zhang, M., Ma, Y., Ye, X., Zhang, N., Pan, L., and Wang, B. (2023). TRP (transient receptor potential) ion channel family: structures, biological functions and therapeutic interventions for diseases. Sig Transduct. Target Ther. 8, 261. doi:10.1038/s41392-023-01464-x
Zhang, W., Huang, G., and Xu, F. (2020). Engineering biomaterials and approaches for mechanical stretching of cells in three dimensions. Front. Bioeng. Biotechnol. 8, 589590. doi:10.3389/fbioe.2020.589590
Zhang, Y., Jiang, N., Zarnitsyna, V. I., Klopocki, A. G., McEver, R. P., and Zhu, C. (2013). P-selectin glycoprotein ligand-1 forms dimeric interactions with E-selectin but monomeric interactions with L-selectin on cell surfaces. PLoS ONE 8, e57202. doi:10.1371/journal.pone.0057202
Zhao, Q., Zhou, H., Li, X., and Xiao, B. (2019). The mechanosensitive Piezo1 channel: a three-bladed propeller-like structure and a lever-like mechanogating mechanism. FEBS J. 286, 2461–2470. doi:10.1111/febs.14711
Zhao, X., Yang, X., An, Z., Liu, L., Yong, J., Xing, H., et al. (2022). Pathophysiology and molecular mechanism of caveolin involved in myocardial protection strategies in ischemic conditioning. Biomed. and Pharmacother. 153, 113282. doi:10.1016/j.biopha.2022.113282
Zhong, T., Li, Y., He, X., Liu, Y., Dong, Y., Ma, H., et al. (2020). Adaptation of endothelial cells to shear stress under atheroprone conditions by modulating internalization of vascular endothelial cadherin and vinculin. Ann. Transl. Med. 8, 1423. doi:10.21037/atm-20-3426
Zhou, J., Lee, P.-L., Tsai, C.-S., Lee, C.-I., Yang, T.-L., Chuang, H.-S., et al. (2012a). Force-specific activation of Smad1/5 regulates vascular endothelial cell cycle progression in response to disturbed flow. Proc. Natl. Acad. Sci. U.S.A. 109, 7770–7775. doi:10.1073/pnas.1205476109
Zhou, Y.-F., Eng, E. T., Zhu, J., Lu, C., Walz, T., and Springer, T. A. (2012b). Sequence and structure relationships within von Willebrand factor. Blood 120, 449–458. doi:10.1182/blood-2012-01-405134
Zhou, Z., Apte, S. S., Soininen, R., Cao, R., Baaklini, G. Y., Rauser, R. W., et al. (2000). Impaired endochondral ossification and angiogenesis in mice deficient in membrane-type matrix metalloproteinase I. Proc. Natl. Acad. Sci. U.S.A. 97, 4052–4057. doi:10.1073/pnas.060037197
Zhu, J., Yu, Y., Ulbrich, M. H., Li, M., Isacoff, E. Y., Honig, B., et al. (2011). Structural model of the TRPP2/PKD1 C-terminal coiled-coil complex produced by a combined computational and experimental approach. Proc. Natl. Acad. Sci. U.S.A. 108, 10133–10138. doi:10.1073/pnas.1017669108
Zhuang, J., Luan, P., Li, H., Wang, K., Zhang, P., Xu, Y., et al. (2017). The yin–yang dynamics of DNA methylation is the key regulator for smooth muscle cell phenotype switch and vascular remodeling. ATVB 37, 84–97. doi:10.1161/ATVBAHA.116.307923
Ziegler, T., Silacci, P., Harrison, V. J., and Hayoz, D. (1998). Nitric oxide synthase expression in endothelial cells exposed to mechanical forces. Hypertension 32, 351–355. doi:10.1161/01.HYP.32.2.351
Zovein, A. C., Luque, A., Turlo, K. A., Hofmann, J. J., Yee, K. M., Becker, M. S., et al. (2010). Beta1 integrin establishes endothelial cell polarity and arteriolar lumen formation via a Par3-dependent mechanism. Dev. Cell 18, 39–51. doi:10.1016/j.devcel.2009.12.006
Keywords: mechanoreceptors, ion channels, integrins, endothelial cell anisotropy, fluid shear stress, forces, vascular function, stiffness
Citation: Mierke CT (2024) Mechanosensory entities and functionality of endothelial cells. Front. Cell Dev. Biol. 12:1446452. doi: 10.3389/fcell.2024.1446452
Received: 09 June 2024; Accepted: 04 October 2024;
Published: 23 October 2024.
Edited by:
Akiko Mammoto, Medical College of Wisconsin, United StatesReviewed by:
Zhe Sun, University of Missouri, United StatesSivakami M. Mylvaganam, University of Toronto, Canada
Copyright © 2024 Mierke. This is an open-access article distributed under the terms of the Creative Commons Attribution License (CC BY). The use, distribution or reproduction in other forums is permitted, provided the original author(s) and the copyright owner(s) are credited and that the original publication in this journal is cited, in accordance with accepted academic practice. No use, distribution or reproduction is permitted which does not comply with these terms.
*Correspondence: Claudia Tanja Mierke, Y2xhdWRpYS5taWVya2VAdW5pLWxlaXB6aWcuZGU=
†ORCID: Claudia Tanja Mierke, orcid.org/0000-0002-6622-335X