- 1Student Research and Technology Committee, Bushehr University of Medical Sciences, Bushehr, Iran
- 2The Persian Gulf Marine Biotechnology Research Center, The Persian Gulf Biomedical Sciences Research Institute, Bushehr University of Medical Sciences, Bushehr, Iran
- 3The Pharmacogenomics Laboratory, Department of Pharmacology, Faculty of Medicine, University of Malaya, Kuala Lumpur, Malaysia
- 4Food Control Laboratory, Food and Drug Deputy, Bushehr University of Medical Sciences, Bushehr, Iran
Marine invertebrate stem cells (MISCs) represent a distinct category of pluripotent and totipotent cells with remarkable abilities for self-renewal and differentiation into multiple germ layers, akin to their vertebrate counterparts. These unique cells persist throughout an organism’s adult life and have been observed in various adult marine invertebrate phyla. MISCs play crucial roles in numerous biological processes, including developmental biology phenomena specific to marine invertebrates, such as senescence, delayed senescence, whole-body regeneration, and asexual reproduction. Furthermore, they serve as valuable models for studying stem cell biology. Despite their significance, information about MISCs remains scarce and scattered in the scientific literature. In this review, we have carefully collected and summarized valuable information about MISC detection by perusing the articles that study and detect MISCs in various marine invertebrate organisms. The review begins by defining MISCs and highlighting their unique features compared to vertebrates. It then discusses the common markers for MISC detection and in vitro techniques employed in invertebrate and vertebrates investigation. This comprehensive review provides researchers and scientists with a cohesive and succinct overview of MISC characteristics, detection methods, and associated biological phenomena in marine invertebrate organisms. We aim to offer a valuable resource to researchers and scientists interested in marine invertebrate stem cells, fostering a better understanding of their broader implications in biology. With ongoing advancements in scientific techniques and the continued exploration of marine invertebrate species, we anticipate that further discoveries will expand our knowledge of MISCs and their broader implications in biology.
1 Introduction
The diversity and phylogenetic range of marine invertebrates are unparalleled on Earth, from the simplest organisms like sponges and cnidarians to more complex creatures such as mollusks, crustaceans, echinoderms, and protochordate (Ballarin et al., 2018). A wide variety of cell and tissue types in invertebrates show remarkable flexibility in their shapes, structures, regenerative properties, proliferation processes, and cell lineage (Rosenfield et al., 1994; Rinkevich, 1999; Afshar et al., 2023; Baghban et al., 2023). Moreover, marine organisms produce different molecules including enzymes, biopolymers, bioactive compounds, and secondary metabolites which have applications in different fields (Ballarin et al., 2018; Keshavarz et al., 2021; Baghban et al., 2022; Carroll et al., 2022; Miri et al., 2022; Rasekh et al., 2023; Zare et al., 2023; Dehghani et al., 2024; Shamsian et al., 2024). For more than 150 years, due to these significant properties of marine invertebrates, they have served as laboratory models, illustrating various biological phenomena (Bellé et al., 2007; Ballarin et al., 2018). Besides, they also enable the production of numerous innovative bioactive molecules based on a kaleidoscope of marine invertebrate stem cell (MISC) types, many of which have significant potential for human health applications (Ballarin et al., 2018). These bioactive molecules will be used in various biological experiments, including stem cell research, further developing our understanding of marine invertebrate models (Ballarin et al., 2018).
Recent studies, have shown that marine invertebrates are ideal for stem cell research. These organisms provide various types and lineages of stem cells, which serve as significant models for studying stem cell biology (Ballarin et al., 2018). Stem cells, found in both embryonic development and organogenesis (embryonic stem cells), as well as tissue regeneration (adult stem cells), play pivotal roles in self-renewal and differentiation (Zhan, 2008). In multicellular organisms, stem cells have the unique ability to remain in an undifferentiated conditions and when needed, generate differentiated target cells (Varghese et al., 2009; Taichman et al., 2010; Ballarin et al., 2018). Instead of strictly interpreting stem cells as undifferentiated cells, it might be helpful to consider a broader interpretation that encompasses dedifferentiated cells in addition to stem cells (Kang et al., 2006; Xu et al., 2015; Ferrario et al., 2020), as defined by Post and Clevers’s (2019). Which places more emphasis on their ability to replace lost cells rather than on their morphological or molecular characteristics (Post and Clevers, 2019).
It is also noteworthy that trans-differentiation (a topic of great interest today when it comes to understanding “reprogramming” a cell) is found in both anatomically simple and “morphologically complex” invertebrates (Arenas-Mena, 2010; Knapp and Tanaka, 2012). There are many activities in which invertebrates can replace vertebrates, and invertebrates can also offer new perspectives on the function, properties, and evolution of MISCs (Ballarin et al., 2018). Research on MISCs contributes to our understanding of various biological processes, including cellular growth and differentiation, aging, and senescence, as well as regeneration and budding that occur in marine invertebrates (Ballarin et al., 2018). Therefore, effective detection approaches for these cells become more significant. In this regard, this review summarizes the most common techniques used to detect MISCs based on previous studies on stem cell investigation, and the types of detected stem cell markers in marine invertebrates.
Figure 1 is a comprehensive phylogenetic tree to elucidate the evolutionary relationships among diverse marine and terrestrial invertebrates. This tree includes major invertebrate groups such as Arthropods, Crustaceans, Molluscs, Bivalves, Gastropods, Annelids, Polychaetes, Echinoderms, Cnidarians, Sponges, Hemichordates, and Ctenophores (Zhang et al., 2021). By incorporating a range of invertebrates, this phylogenetic tree aims to depict the complex web of evolutionary paths these organisms have followed. The construction of this tree is based on both morphological characteristics and molecular data, providing a robust framework for understanding the evolutionary history and relationships among these diverse groups. Such a phylogenetic tree not only highlights the shared ancestry and divergence of these invertebrates but also aids in the identification of unique evolutionary traits and adaptations that have arisen in response to different ecological niches. This visual representation is a valuable tool for comparative studies, helping unravel the evolutionary processes that have shaped the rich biodiversity of invertebrates in marine and terrestrial environments.
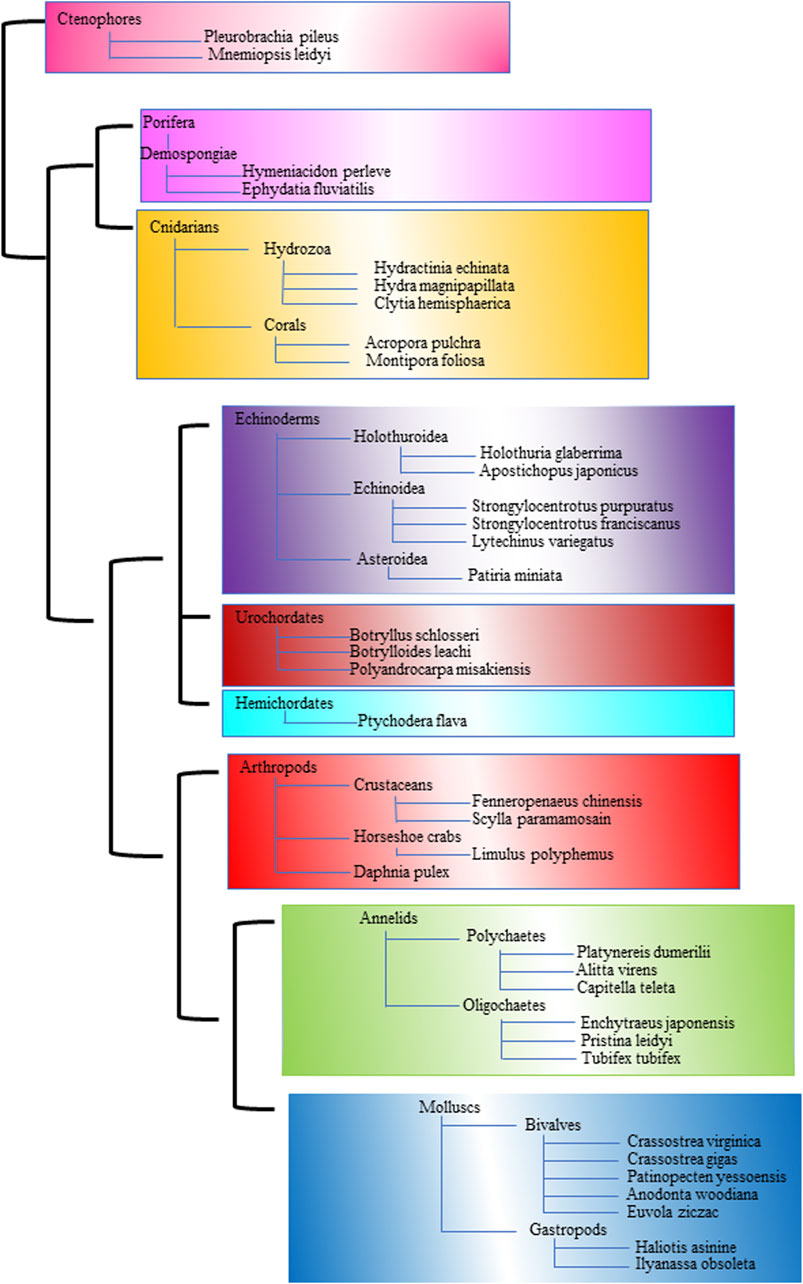
Figure 1. The phylogenetic tree illustrates the evolutionary relationships among various marine and terrestrial invertebrates. A distinct color represents each major group.
2 Marine invertebrates stem cells (MISCs)
Stem cells are believed to exist in various marine organisms, ranging from simple species like sponges to more complex echinoderms and crustaceans (Ballarin et al., 2018). While adult stem cells are prevalent across marine invertebrate phyla, extensive examination has primarily focused on porifera, cnidaria, and platyhelminths. Despite this, our understanding of Marine Invertebrate Stem Cells (MISCs) is limited, especially when compared to vertebrates, mammals, and terrestrial invertebrates (Rinkevich et al., 2009).
MISCs exhibit distinctive cytomorphological features, being smaller in size with a higher nucleo-cytoplasmic ratio, basophilic staining, and rounded shapes (Cima et al., 2001). Hemocytes in Botryllus, as well as neoblasts in many species of oligochaete worms and Enchytraeus japonensis, display stem cell characteristics (Randolph, 1892; Oka and Watanabe, 1957; Rinkevich et al., 2007; Rinkevich et al., 2008; Yoshida-Noro and Tochinai, 2010). Unlike vertebrates, MISCs have a widespread distribution throughout the body, lacking clear distinctions between germ and somatic stem cell lineages (Rinkevich and Matranga, 2009; Sköld et al., 2009). In some marine invertebrates like Platyhelminthes and hydra, stem cells can differentiate into both germ and stem lineages, allowing germline establishment in the adult phase (Hwang et al., 2007). Notably, germ-stem cells in marine invertebrates have been observed to trans-differentiate into somatic adult stem cells in specific regeneration scenarios (Gremigni and Puccinelli, 1977; Gremigni, 1981). Marine invertebrates exhibit pluripotency and totipotency, differentiating into cell lines from multiple germ layers, including the germline, a unique trait when compared to the unipotent or oligopotent nature of vertebrate stem cells (Rinkevich et al., 2009). MISCs can proliferate, differentiate, and migrate to various tissues throughout adulthood, playing pivotal roles in various biological processes. These processes include senescence, delayed senescence, longevity, whole-body regeneration, asexual reproduction methods like budding, fragmentation, gemmule-hatching, indeterminate growth, fission, and torpor phenomena. Additionally, MISCs contribute to unique stemness systems in marine invertebrates, without forming tumors (Shimomura et al., 1962). Ongoing research into dedifferentiation and the spontaneous reacquisition of stem cell phenotypes, has potential implications in reprogramming, as seen with the addition of Yamanaka’s factors in mammalian cultures. Therefore, MISCs, are central to the developmental biology phenomena of marine invertebrates (Okita et al., 2007).
3 MISC identification
Scientists use stem cell markers, which are genes and their protein products, to identify and isolate stem cells, including MISCs (Pazhanisamy, 2013). These markers can be specific proteins on the cell surface or intracellular proteins within the cell (Nguyen et al., 2010). Using antibodies, either monoclonal or polyclonal, to identify stem cell lineages is one of the strategies to assign the stemness phenotype based on an analysis of surface molecules and intracellular markers (Cai et al., 2005).
In recent years, there has been a surge in the development and utilization of various methods for detecting and characterizing stem cell markers. These techniques, encompassing flow cytometry, immunofluorescence/immunocytochemistry, protein array, real-time RT-PCR, and Western blot, have significantly advanced our understanding of stem cell biology. Their continued development promises to further enhance stem cell research and contribute significantly to the field of regenerative medicine.
3.1 Markers used to identify MISCs
Accurate identification of stem cells relies on specific molecular markers, which are recognized by their distinct expression patterns rather than their functions (Cai et al., 2005). Although no single marker is sufficient to identify a specific stem cell, several molecules have been identified, such as Vasa, Sox2, Oct3/4, Nanog, alkaline phosphatase, and telomerase activity (Sköld et al., 2009; Zito and Matranga, 2009). Additionally, common “stemness” genes like Piwi, Nanos, PCNA, and Aldh are utilized in both vertebrate and invertebrate stem cells (Rinkevich et al., 2009). Studies reveal molecular similarities between ascidian and mammalian stem cells, including Aldh, alkaline phosphatase, PL10, and PCNA. Genes related to the Germline/Multipotency program (GMP) like Piwi, Vasa, Nanos, and PL10 are highly conserved across various metazoan species (Juliano et al., 2010). The markers listed in Table 1 are frequently employed for identifying MISCs and will be further elaborated in more detail in the following sections.
3.1.1 Aldehyde dehydrogenase
Aldehyde dehydrogenase (ALDH) plays diverse roles in cells, including detoxification of aldehydes, retinoic acid production, biomolecule synthesis, promoting cell survival, and hormonal interactions (Marchitti et al., 2008; Zhou et al., 2019). There are 24 ALDH protein families with varied functions, that are crucial for drug resistance and stem cell protection (Moreb, 2008; Jackson et al., 2011; Vasiliou et al., 2013). ALDH activity is essential for adult stem cell self-renewal, and is closely linked to stemness and differs during hypoxia in species like shrimp Fenneropenaeus chinensis (Jiang et al., 2009). ALDH also contributes to ascidian species development and has been studied in somatic tissue of marine species like Limulus polyphemus and Crassostrea virginica for stress adaptation (Dragolovich and Pierce, 1994; Perrino and Pierce, 2000; Sköld et al., 2009).
It is important to note that while ALDH is vital for transforming progenitors cells into mature cells, its specificity for stem cells is limited (Vassalli, 2019). Certain ALDH isozymes may not maintain stemness, emphasizing the need to identify stemness-related ALDH isozymes and their roles in renewing and differentiating cells (Moreb, 2008). ALDH has been detected in the asexual buds of the ascidian Polyandrocarpa misakiensis. This was found using the ALDEFLUOR assay, aiding in ALDH detection through flow cytometry (Storms et al., 1999; Zhou et al., 2019).
3.1.2 Alkaline phosphatase
Alkaline phosphatases (ALPs) are essential metalloenzymes found in various life forms and have been studied since 1954, serving as markers for germ cells in mice (Chiquoine, 1954; Cavelier et al., 2017). These enzymes are predominantly present in stem cells and germline cells, contributing to metabolite transport, secretion, stem cell maintenance, biomineralization, and tissue regeneration in specific animals (Buchet et al., 2013). In human embryonic stem cells, ALP activity indicates pluripotency and diverse differentiation potential. Notably, ALP activity is not limited to mammals but extends to diverse organisms, including oysters and invertebrates like ascidians, parasitic crustaceans, hydrozoans, planarian neoblasts, and crustaceans (Osborne and Miller, 1963; Millan and Manes, 1988; Extavour and Akam, 2003; Shukalyuk et al., 2005; O’Connor et al., 2008; Isaeva et al., 2011). This underscores ALP’s wide-ranging importance in stem cell functions. For instance, in oysters, robust ALP activity in reproductive areas and metabolic processes has been detected (Bevelander, 1952). Similarly, ALP activity in Polyascus polygenea’s stolons, primordial cells, and the polychaete Pomatoceros lamarckii highlights its role in stem cell-related functions (Isaeva et al., 2003; Szabo and Ferrier, 2014).
3.1.3 BMI1
BMI1, a transcription repressor in the Polycomb family of transcription factors, is essential for governing gene expression and preserving stem cell reservoirs, ensuring self-renewal, and maintaining these critical cell populations. Its primary role is to prevent premature senescence, ensuring stem cell longevity and functionality (Siddique and Saleem, 2012). BMI1’s presence in mice suggests that it plays a crucial role in sustaining somatic stem cells throughout the body and is associated with oncogenic potential (Schmitt, 2003). Moreover, BMI1 is not limited to mice; it also plays a role in self-renewal in various stem cell populations, including adult hematopoietic stem cells (HSCs), peripheral neural stem cells, and central nervous system neural stem cells (Haupt et al., 1993; Jacobs et al., 1999). In non-mammalian species like the sea cucumber Holothuria glaberrima, BMI1 serves as an analogue to mammalian intestinal stem cell markers, indicating its conserved role in regulating stem cells across different organisms (Molofsky et al., 2003; Park et al., 2003; Mashanov V. et al., 2017a). This underscores the wide-reaching importance of BMI1 in stem cell biology.
3.1.4 BrdU
BrdU (5-bromo-2′-deoxyuridine) labeling is widely used to identify cell divisions and serves as a valuable marker for DNA synthesis and cell division rates (Mashanov et al., 2017b). It is particularly effective for tracking actively dividing cells during the S-phase of the cell cycle, although caution is needed due to potential incorporation during DNA repair processes (Dolbeare and Selden, 1994; Taupin, 2007; Benton et al., 2014). In marine organisms like Hymeniacidon perleve, BrdU incorporation in archaeocytes (toti/multipotent stem cells), along with increased PCNA expression and telomerase activity, indicates DNA synthesis and cell proliferation (Sun et al., 2007).
Cnidarians like Hydractinia echinata and Clytia hemisphaerica utilize BrdU in pulse-chase assays, shedding light on mitotic activity and cellular self-renewal dynamics (Müller et al., 2004; Denker et al., 2008). In the hatchings and adults marine platyhelminth Macrostomum sp., BrdU incorporation reveals neoblast migration, renewal capabilities, and differentiation into various somatic and germ cells, enhancing understanding of stem cell dynamics (Ladurner et al., 2000; Schärer et al., 2004). BrdU labeling proves versatile in exploring cell proliferation, stem cell behavior, and tissue renewal in diverse marine organisms, advancing our understanding of cellular processes and promising further research in regenerative biology and developmental studies, with necessary acknowledgment of its limitations for accurate interpretation.
3.1.5 c-Myc
The c-Myc gene, a pivotal transcription factor, regulates essential cellular functions such as proliferation, growth, and apoptosis (Lehr, 2003). In both invertebrates and vertebrates, Myc proteins play a crucial role in managing cell growth and proliferation, highlighting their conserved regulatory function across species (Gallant, 2006; Takahashi et al., 2007; Morita et al., 2009; McConnell and Yang, 2010; Mahani et al., 2013). For instance, marine invertebrates like the sea cucumber H. glaberrima possess a c-Myc gene family, with elevated Myc levels correlating with tissue dedifferentiation and regeneration, emphasizing Myc’s importance in tissue growth and repair in marine organisms (Mashanov et al., 2015).
Studies on the sea star species Asterias vulgaris have identified c-myc proto-oncogene expression as a sensitive indicator for assessing mitotic activity in the testicular germinal epithelium, shedding light on growth and reproduction regulatory mechanisms in marine organisms (Marsh and Walker 1995).
Understanding proto-oncogenes like c-Myc in marine organisms provides crucial insights into the molecular mechanisms driving growth, development, and regeneration. Exploring Myc-related pathways reveals fundamental processes enabling marine organisms to adapt and respond to environmental changes, including tissue repair and regeneration. These insights not only expand our knowledge of fundamental biological processes but also have potential implications for regenerative medicine and stem cell research in humans. The universal functions of Myc across species emphasize the importance of studying marine invertebrates as model organisms, enriching our understanding of these processes.
3.1.6 EdU
EdU (5-ethynyl-2′-deoxyuridine) is a groundbreaking thymidine analog used for DNA labeling in actively dividing cells. Its unique feature, employing fluorescent azides for detection, eliminating the need for DNA denaturation and providing deep tissue penetration for in vivo studies, making it invaluable for tracking stem cells and cell proliferation in living organisms (Lin et al., 2010; Qu et al., 2011). EdU labeling has provided crucial insights into cell proliferation patterns in various organisms. For instance, in Aeolosoma viride (Chen et al., 2020) and Pristina leidyi (Bely and Sikes, 2010) distinct proliferation patterns highlighted by EdU labeling led to variations in blastema size during regeneration (Bely and Sikes, 2010). In Playtnereis dumerilli, EdU pulse-labeling revealed unique primordial germ cell (PGC) clusters anterior to the mesodermal posterior growth zone, demonstrating its specificity in detecting cell populations (Rebscher et al., 2012; Zattara and Bely, 2013; Ćorić et al., 2022). EdU has illuminated cell proliferation during adult regeneration in species like the Sea Anemone Nematostella vectensis and Ptychodera flava, showing its applicability in studying tissue regeneration and cell dynamics (Amiel et al., 2015; Luttrell et al., 2018).
In hydra, EdU labeling indicated the presence of slow-cycling adult stem cells in early multicellular organisms, emphasizing its role in studying stem cell biology and evolution (Govindasamy et al., 2014). Additionally, EdU assays conducted on hammer coral (Fimbriaphyllia ancora) elucidated somatic cell proliferation during gametogenesis which advanced our understanding of coral reproduction (Chiu et al., 2021). Furthermore, experiments with Pleurobrachia pileus demonstrated EdU’s ability for DNA-labeling and long-term retention. This helped link stem cell concentrations with gene expression areas, emphasizing its role in pinpointing stem cell niches and connecting somatic stem cells to gene regulation (Alié et al., 2011). EdU’s non-invasive in vivo detection method, coupled with its versatility, makes it a crucial tool for studying cell proliferation, stem cell biology, and regeneration across diverse organisms. As research progresses, it is expected that EdU will play a pivotal role in enhancing our understanding of stem cells within multicellular development and regeneration contexts.
3.1.7 Grimp
The Grimp gene, which has been identified in E. japonensis, serves as a crucial regulator of cell proliferation, particularly in multipotent mesodermal cells (neoblasts), highlighting its significant role in the early stages of regeneration. Its impact extends to various mesodermal cells beyond neoblasts, emphasizing its vital function in regulating mesodermal cell proliferation during the onset of the regenerative process. When the Grimp mRNA is suppressed, it disrupts cell proliferation in the mesoderm, and inhibits anterior structure differentiation, indicating the pivotal role of Grimp in mesodermal regeneration in E. japonensis (Takeo et al., 2009).
It is important to understand the function of the Grimp gene in order to advance regeneration and stem cell biology. Its promotion of neoblast proliferation provides insights into the mechanisms governing tissue repair and regrowth, considering the crucial role of neoblasts in regeneration across organisms (Yoshida-Noro and Tochinai, 2010). Exploring non-neoblast Grimp-expressing cells in E. japonensis mesoderm could offer insights into complex mesodermal cell proliferation and differentiation during regeneration. Furthermore, investigating Grimp-related regulatory networks may deepen our understanding of the molecular mechanisms driving tissue regeneration and repair. Grimp research stands as a promising potential in regenerative biology, with implications for enhancing tissue repair and regeneration in various organisms, including humans. Exploring its mechanisms could lead to innovative approaches in regenerative medicine and illuminate fundamental principles of stem cell biology (Takeo et al., 2009).
3.1.8 Klf
The Krüppel-like factor (Klf) gene family is known for its involvement in fundamental processes like stem cell renewal, pluripotency, and differentiation. This gene family consists of zinc-finger transcription factors, specifically KLF2 and KLF4. These genes exhibit tumor suppressor properties, inhibiting cell growth, DNA synthesis, and cell cycle progression, with KLF4 having dual capabilities in promoting cell survival and counteracting c-Myc-induced programmed cell death (Takahashi et al., 2007; McConnell and Yang, 2010).
While most research on Klf genes has focused on select bilaterian animals, there is growing interest in their potential evolutionary. Studies on the ctenophore Mnemiopsis leidyi have revealed Klf genes in stem cells that regulate proliferation in developmental stem cell niches, suggesting their contribution to the stem lineage leading to Metazoa, emphasizing their role in governing stem cell proliferation in metazoans (Presnell, 2017). Sea urchin genomes contain multiple Klf genes, and Klf1/2/4 have been identified as orthologs of Holothuria glaberrima, indicating their conservation across echinoderms (Materna et al., 2006; Mashanov et al., 2015).
In Schmidtea mediterranea planarians, the Klf4-like gene (klf4l) has been studied for its role in maintaining germ cells and yolk cells. Knocking down Klf4l impedes these cell types, highlighting its crucial role in their regulation. Moreover, Klf4 marks expression in PGCs and presumptive germline stem cells (GSCs) of planarians, emphasizing its significance in germ cell regulation. In the sea cucumber Apostichopus japonicus, Aj-klf13 plays a pivotal role in intestinal regeneration, showcasing diverse roles of KLFs in tissue regeneration and repair across organisms (Chen et al., 2020b; Issigonis et al., 2022).
Hydra polyps as a simple multicellular animal, demonstrate intricate KLF roles, particularly in interlineage communication and cell type-specific functioning within three stem cell populations. Overall, the multifaceted roles of the Klf gene family, along with their conservation across metazoans, underscore their importance in developmental processes, stem cell regulation, and tissue regeneration. Deeper exploration of KLF molecular mechanisms and evolution is expected to yield new insights into stem cell biology and regenerative medicine, offering innovative avenues for future therapeutic interventions and treatments (Hemmrich et al., 2012).
3.1.9 Lgr5
The trio of receptors, Lgr5, Lgr4, and Lgr6, which belong to the LGR family, play a pivotal role in adult stem/progenitor cells. These receptors, characterized by a large extracellular domain with leucine-rich repeat (LRR) units, are regulated by Wnt signaling and are crucial for cellular growth and division. Dysregulated Wnt activation, often due to mutations, leads to strong Lgr5 expression in various cancers (de Lau et al., 2014).
In sea cucumber H. glaberrima, homologs of mammalian Lgr5 (Lgr4/5/6) are expressed in peritoneal cells, suggesting their candidacy as gut mesothelium stem cells. Both Lgr5 and Bmi1 stem cell gene markers are found in epithelial and peritoneal cells of the digestive tube, highlighting their role in tissue maintenance and regeneration (de Lau et al., 2014). Investigating Lgr4/5/6 interactions with R-spondins in sea cucumbers can shed light on stem cell self-renewal and tissue repair pathways, underscoring evolutionary stem cell regulatory conservation (Mashanov et al., 2017a).
Advancements in understanding LGR roles, such as Lgr5, Lgr4, and Lgr6, hold therapeutic potential in regenerative medicine and cancer therapy. Exploring LGR signaling pathways and mechanisms enriches stem cell biology knowledge, with implications for human health. The identification of Lgr4/5/6 in sea cucumbers and their expression in peritoneal cells hints at their diverse roles in stem cell regulation and tissue balance, promising novel therapies in regenerative medicine driven by exciting discoveries (Mashanov et al., 2017b).
3.1.10 Nanog
Nanog, a vital transcription factor, plays a key role in maintaining pluripotent cells and embryonic stem cells’ (ESCs) self-renewal capacities. In marine organisms like Hydra and Nematostella, transcription factors including Nanog, Oct-4, and Klf4 regulate stemness and robust proliferation in vertebrate ESCs. Despite the challenges associated with influencing specific genes in marine invertebrates due to limited understanding of growth factor gene expression, the sea urchin S. purpuratus possesses conserved genes like SpOct and a nanog homolog that are associated with pluripotency. Exploration of DNA sequences has led to the identification of a nanog gene homolog in marine invertebrates, particularly within the sea urchin genome, highlighting its significance in understanding stem cell regulation and pluripotency (Char et al., 1993; Yuh et al., 1998; Chambers et al., 2003; Mitsui et al., 2003; Rodda et al., 2005; Odintsova et al., 2007; Watanabe et al., 2009; Ballarin et al., 2022).
3.1.11 Nanos
Nanos family proteins, which characterized by conserved CCHC zinc fingers, play crucial roles in the development of somatic and germ line cells across various Metazoa species. While they are primarily associated with germline functions, nanos genes exhibit diverse expression patterns, suggesting multiple roles in embryonic and larval development (Curtis et al., 1997; Extavour and Akam, 2003; Wang et al., 2007; Rinkevich et al., 2022). They contribute to the germline multipotency program (GMP) alongside genes like piwi and vasa, and are served as robust molecular markers for multipotent cells. In certain contexts, such as regenerative blastema, de novo nanos expression may indicate local cell dedifferentiation (Juliano et al., 2010; Nikanorova et al., 2020; Kostyuchenko and Kozin, 2021).
Nanos expression patterns vary in embryonic and larval development - among various marine organisms. For instance, in P. ornatus, nanos expression increases in day 3 embryos, aligning with gastrula-specific gene expressions (Helluy and Beltz, 1991). In E. sinensis embryos, genes like Nanos are upregulated during early developmental stages (Wang et al., 2020).
The transcripts of CapI-vasa and CapI-nanos are present in developing gametes of mature adults, and their expression is observed across embryonic, larval, and juvenile stages. These transcripts are found in various somatic tissues, with broad expression during early cleavage stages. As gastrulation progresses, their expression becomes prominent in areas such as the presumptive brain, mesodermal bands, and developing foregut. Researchers have used CapI-nanos and CapI-vasa as markers to identify potential PGCs in larvae, initially forming small bilateral clusters in segment four and later consolidating into a single cluster in late larval stages (Dill and Seaver, 2008)
Nanos-related genes are crucial in lophotrochozoans like Helobdella robusta and P. dumerilii, expressed in germ cells and PGCs (Kang et al., 2002; Zelada González, 2005; Rebscher et al., 2007). In these species, Nanos expression extends to both germ cells and somatic tissues (Kang et al., 2002; Zelada González, 2005). Knock down experiments in Ilyanassa obsoleta demonstrate Nanos’s importance in mesodermal and endodermal tissue development (Rabinowitz et al., 2008).
Patinopecten yessoensis possesses two Nanos family genes, PyNanos1 and PyNanos2/3, expressed in distinct gonadal cells. In the Tritia obsoleta, Nanos expression is limited to the 4d mesoderm lineage, and it is vital for mesodermal and endodermal tissue preservation. Haliotis asinine displays varied HasNanos expression, which is crucial in embryonic development and is localized in the dorsal quadrant (Rabinowitz et al., 2008; Liu et al., 2022).
During trochophore development in Haliotis asinine, Nanos is found in putative mesodermal bands and PGCs. In leeches, polychaetes (Platynereis dumerilii and Capitella sp. I), Nanos is integral to germ cell and PGC formation (Kang et al., 2002; Zelada González, 2005; Dill and Seaver, 2008; Kranz et al., 2010; Ponz-Segrelles et al., 2018). Nanos is expressed diversely in somatic tissues, including ectodermal stem cells in leeches, and brain, foregut, mesodermal bands, and growth zones in P. dumerilii and Capitella sp. I. Furthermore, Pdu-nanos is involved in the regeneration process of P. dumerilii worms (Kang et al., 2002; Zelada González, 2005; Planques et al., 2019).
3.1.12 Oct-4
The Oct4 gene, a member of the POU protein family, is essential for establishing and maintaining pluripotent stem cells, governing key target genes to preserve pluripotency and inhibit differentiation. Oct4 downregulation during cell differentiation is harnessed for reprogramming somatic cells into pluripotent stem cells (Pesce et al., 1998; Niwa et al., 2000; Cavaleri and Schöler, 2003; Boyer et al., 2005; Anderson et al., 2007; Takahashi et al., 2007; Park et al., 2013).
Oct4-like POU domain gene expression is observed in pluripotent interstitial cells (I cells) in hydrozoan cnidarians Hydractinia stolons and in sea urchins. In the sea cucumber H. glaberrima, Oct1/2/11 shows homology with mammalian Oct4 (Eddy, 1976; Frank et al., 2009; Range and Lepage, 2011; Mashanov, et al., 2015).
Introducing the Oct4-like transcription factor Polynem (Pln) into Hydractinia’s epithelial cells transforms them into stem cells, leading to neoplasm development. Oct4 induces MALAT1 transcription, promoting cell proliferation and motility, and regulates Dnmt1 activity. Strong Oct4 expression is found in mature ovaries of mud crabs, particularly in the eyestalk of female crabs. Oct4/Sox9 interaction enhances SpVih expression, while interference with Oct4 and Sox9 reduces SpVih expression and increases SpVtg expression in ovaries and hepatopancreas. GST pull-down experiments support the interactions between Oct4 and Sox9, emphasizing their role in SpVih regulation (Plickert et al., 2012; Jen et al., 2017; Wu et al., 2018; Liao et al., 2020).
3.1.13 PCNA
The Proliferating Cell Nuclear Antigen (PCNA) serves as a crucial biomarker for active cell division and plays essential roles in DNA replication, damage repair, and cell cycle progression. Studies in Cnidarian species like Montipora foliosa and Acropora pulchra have utilized PCNA protein expression to investigate mitotic activity, indicating its evolutionary conservation (D’Angelo et al., 2012). In sponges, specifically in archaeocytes of Hymeniacidon, remarkably high PCNA levels were observed (Sun et al., 2007).
Oysters such as Crassostrea gigas exhibited elevated CgPCNA mRNA transcripts in various tissues, including gonads, gills, and haemolymph (Yu et al., 2022). Invertebrate stem cells, identified through markers like germinal granules and alkaline phosphatase, demonstrated specific PCNA activity (Isaeva et al., 2008).
Additionally, the introduction of recombinant protein CgAstakine in oysters increased CgPCNA protein abundance in agranulocytes and gills, implicating its role in hematopoiesis (Yu et al., 2022). Furthermore, spatial distribution studies using BrdU-PCNA immuno-detection, neuron-specific staining, and electron microscopy provided insights into mesenchymal stem cells and neurons in the striated adductor muscle of scallops (Sun et al., 2021).
3.1.14 Piwi
The Piwi-like genes, part of the Argonaute protein subfamily, are crucial in stem cell epigenetics and RNA interference across various biological systems. They participate in mRNA degradation and gene silencing, impacting germline development and stem cell regulation (Hutvágner and Zamore, 2002; Hatfield et al., 2005; Thomson and Lin, 2009; Giani et al., 2011). Studies on sea urchin have highlighted their role in spine and tube foot regeneration, indicating multipotent progenitor cells’ involvement (Juliano et al., 2006; Karthik et al., 2014; Bodnar and Coffman, 2016).
Piwi gene expression is predominant during gametogenesis and embryonic development, exhibiting varying patterns across species (Deng and Lin, 2002; Carmell et al., 2007; Houwing et al., 2008; Wang and Reinke, 2008; Solana et al., 2012; Juliano et al., 2014). Across diverse organisms, Piwi influences regeneration, with examples in Botrylloides species and cnidarians like jellyfish (Seipel et al., 2003; Juliano et al., 2006; Frank et al., 2009; Alié et al., 2011; Brown and Swalla, 2012; Vickers, 2017).
Capitella teleta’s Piwi genes have dual roles in somatic and germline stem cells (Giani et al., 2011). Piwi’s presence in stony coral, sea anemone, jellyfish, and hydroids influences germ cell formation (Rabinowitz and Rinkevich, 2011).
Specific Piwi-like genes in species like Lygdamis sanguineus play tissue-specific roles, particularly in early regeneration. While they are typically involved in silencing, there are unique cases such as flatworms and acoels expressing Piwi-like genes in somatic stem cells. Annelid species exhibit Piwi-like gene expression in posterior growth zone cells and gonadal stem cells (Extavour et al., 2005; Rebscher et al., 2008; Alié et al., 2011; Leclère et al., 2012; Shikina et al., 2012; Weigert et al., 2013; Hartung et al., 2014; Kozin and Kostyuchenko, 2015; Shikina et al., 2015; Xu and Sun, 2020).
3.1.15 PL10
The Vasa and PL10 homologs, both are members of the DEAD-box family, have a close relationship (Yoshizaki et al., 2000; Mochizuki et al., 2001). These genes are present in various organisms such as sponges, Hydra, and planaria, which are belonging to invertebrates and non-mammal vertebrates (Mochizuki et al., 2001). In these groups, Ded1/DDX3 genes are often referred to as PL10. Notably, PL10 plays a significant role in spermatogenesis and differentiation in invertebrates like Hydra and planarians. While PL10 is typically the only member of the subfamily in non-mammal animals and some noneutherian mammals, it does show a distinct localization pattern in sponges and cnidarians but has been less studied in vertebrates.
Research on zebrafish germ cells has revealed a high transcription of the PL10-homologous gene (pl10a) using RNA in situ hybridization. This suggests that PL10 has an important function in maintaining the undifferentiated state of PGCs and multipotent stem cells among invertebrates like the nereid polychaete Alitta virens (Kozin and Kostyuchenko, 2015). Furthermore, PL10 expression has been identified in the germlines of both male and female Pleurobrachia pileus (Alié et al., 2011).
PL10, a DEAD-box RNA helicase closely related to Vasa, and Bruno, an RNA-binding protein with RRM domains, play crucial roles in cellular processes. In mammals, PL10 (also known as DDX3) is indispensable for spermatogenesis, ensuring normal cellular differentiation during this process (Abdelhaleem, 2005). Expression of PL10 and Bruno genes is observed in planarian neoblasts (Shibata et al., 1999; Guo et al., 2006), while Piwi, Vasa, and PL10 genes are co-expressed in the posterior growth zone of Platynereis larvae (Rebscher et al., 2007), playing a role in somatic and germinal stem cell activity. PL10 is expressed in progenitor cell clusters, gonads, and the posterior growth zone of C. teleta, indicating a potential dual role in somatic and germline cells (Seaver and de Jong, 2021).
3.1.16 Sox2
Sox2, a stem cell-specific transcription factor, possesses a high mobility group (HMG) DNA binding domain that interacts with the 5-CATTGTT-3 consensus motif (Chambers et al., 2007) Functioning within the core regulatory network, Sox2 maintains pluripotency and suppresses differentiation-inducing genes in stem and precursor cells (Boyer et al., 2005; Park et al., 2013; Liu and Nonomura, 2016; Cavelier et al., 2017). Reprogramming of differentiated cells into pluripotent stem cells is achieved using Sox2 (Takahashi et al., 2007). Notably, an anti-Sox2 antibody aids in identifying oyster stem cells, covering chromatin in oyster gonia. In C. gigas, Sox2 is present in gonad ducts during the first sexual cycle, indicating its role in proliferation (Franco et al., 2008; Jemaà et al., 2014; Patra et al., 2015; Nuurai et al., 2016; Cavelier et al., 2017). Sox2 is distributed in a pattern similar to fish gonial proliferation, further supported by phylogenetic analysis identifying SoxB1 as a sea urchin homolog (Wei et al., 2011; Mashanov et al., 2015).
The orthologs of C-Myc and Sox2 are found in adult stem cell lines from cnidarians like Hydra, C. hemisphaerica, and planula larvae, indicating their involvement in proliferation (Pennati et al., 2019). Botryllus schlosseri, a model for pluripotency, also contains orthologs of Yamanaka factors, including Sox2 and c-Myc, hinting at their importance in stemness (Vanni et al., 2022).
In sea stars, Sox2 plays a role in nervous system regeneration, with Sox2-positive cells generating neural precursors (Zheng et al., 2022).
In the scallop Chlamys farreri’s the expression of Sox2 (Cf-Sox2) is observed during embryogenesis, resembling the pattern seen in mammals, implying its role in early development (Liang et al., 2015). Furthermore, the expression pattern of Cf-sox2 was investigated in scallop C. farreri using the whole mount in situ hybridization technique. Positive hybridization signals were observed in the central region of unfertilized oocytes and fertilized eggs, and subsequently, strong signals were dispersed throughout the embryos from the 2-cell stage to gastrula. The observed expression pattern of Cf-sox2 during embryogenesis resembled that of mammalian sox2, suggesting that Cf-Sox2 likely regulates the early development of C. farreri (Liang et al., 2015).
In scallops, Cf-Sox2 is linked to spermatogenesis and testis development, influencing apoptosis and proliferation (Liang et al., 2019).
The genes LsSox2 and LsSox9, homologs to the genes found in Lutraria sieboldii, exhibit differential expression in the ovary and testis, suggesting roles in embryonic and gonadal development (Lu et al., 2022). In the freshwater bivalve Anodonta woodiana, upregulated AwSox2 relates to apoptosis of spermatogonial stem cells and enhanced immune defense (Xia et al., 2020).
Echinococcus multilocularis exhibits dynamic Sox2 expression in germinative cells, which decreases upon germinative cell depletion (Cheng et al., 2017). In Mulinia lateralis, SOX2, FOXZ, HSFY, FOXL2, and HES1 have been identified offering insights into gonadal development and sex differentiation (Li et al., 2022). Furthermore, the regulatory effect of Oct4 and Sox9 on vitellogenesis-inhibiting hormone (SpVih) in mud crab gonadal development has been established (Liao et al., 2020). These findings collectively emphasize the significance of Sox2 in diverse developmental and regulatory processes across various organisms.
3.1.17 Telomerase activity
Stem cells possess remarkable self-renewal abilities, which contribute to their immortality or prolonged existence. The maintenance of chromosomal telomeres and the presence of telomerase are vital for cellular immortality (Blackburn, 1991). Higher telomerase activity in stem cells and immortal cells indicates enhanced self-renewal potential (Morrison et al., 1996). This concept is exemplified in the colonial ascidian B. schlosseri, where telomerase and telomere length influence self-renewal (Laird and Weissman, 2004), and in shrimp tissues where telomerase activity predicts proliferation (Lang et al., 2004). Telomerase activity can be quantified using the telomeric repeat amplification protocol (TRAP) (Kim et al., 1994), which is extensively used to study various cell types (Belair et al., 1997). For instance, in B. schlosseri, telomerase activity was measured using a real-time quantitative protocol (Laird et al., 2005).
Notably, telomerase activity in shrimp lymphoid tissue increases during cultivation, peaking at 20 days (Lang et al., 2004). Immortality in stem cells comes from long telomeres and sustained telomerase activity, which is a widespread phenomenon across the animal kingdom (Traut et al., 2007). The colonial ascidian Botrylloides exhibits heightened telomerase activity linked to its regenerative capacity (Durham et al., 2016).
Telomerase expression was discovered in the sand scallop Euvola ziczac across developmental stages and adult tissues (Owen et al., 2007). Telomerase is present in various organisms, including marine species, and it safeguards telomere stability and cellular division (Greider, 1990; Campisi et al., 2001; Forsyth et al., 2002). High levels of BrdU incorporation, PCNA expression, and telomerase activity in H. perleve’s archaeocytes demonstrate their impressive proliferation potential. In primary cultures lasting 4 days, the purified archaeocytes exhibit a substantial 2.5-fold increase in total cell number, highlighting the potential for developing sponge cell cultures to produce valuable sponge-derived drugs (Sun et al., 2007).
In the case of Arctica islandica, telomerase activity and telomere lengths remain constant across ages and tissues, contributing to its exceptional lifespan (Gruber et al., 2014). Telomerase activity is detected in corals and their symbiotic algae, contributing to growth and reproductive adaptation (Zielke and Bodnar, 2010).
Diplosoma listerianum demonstrates extensive cell proliferation, with higher telomerase activity in bud tissue than adult tissue (Sköld et al., 2011). Telomerase functions in yeast Saccharomyces cerevisiae during S-phase, coinciding with chromosome end replication (Luciano et al., 2012).
Activation of telomerase in Penaeus monodon lymphoid cells could overcome cellular aging barriers for in vitro transformation (Jayesh et al., 2016). Lifespan differences in sea urchins, Strongylocentrotus franciscanus and Lytechinus variegatus, do not correlate with age-related telomere shortening (Francis et al., 2006). Daphnia species, D. pulex and D. pulicaria, exhibit no age-related decline in telomere length or telomerase activity, suggesting other factors influence their distinct lifespans (Schumpert et al., 2015).
3.1.18 Vasa
The vasa gene family products are widely used as molecular markers for PGCs, encoding a conserved RNA-dependent helicase expressed in the germ line (Extavour and Akam, 2003). Vasa presence has been confirmed in various bivalve species through confocal microscopy (Milani et al., 2015; Milani et al., 2017).
Additionally, Vasa expression in Hydra, Hydractinia, polychaeta, and planarians supports the origin of germ cells from primitive totipotent stem cells (Shibata et al., 1999; Mochizuki et al., 2001; Rebscher et al., 2007; Rinkevich et al., 2009). In Hydractinia, vasa protein is found in multipotent or totipotent stem cells generating somatic and germ cells (Frank et al., 2009). Vasa’s role in germline cell formation and maintenance is established in Drosophila, Caenorhabditis, Xenopus, and zebrafish (Mochizuki et al., 2001; Fabioux et al., 2004a), making it a valuable marker for investigating totipotent stem cells and germline development.
The presence of Vasa-related genes in Rhizocephalans, such as Polyascus polygenea, Clistosaccus paguri, and Athelgis takanoshimensis, was studied (Shukalyuk et al., 2007). Co-expression of Vasa with PL10, Oct4, and Bl-piwi was observed in germ line derivatives and somatic tissues of B. schlosseri (Extavour et al., 2005). Nanomia bijuga showed co-expression of Vasa-1, PL10, piwi, nanos-1, and nanos-2 in interstitial stem cells of young zooid buds (Siebert et al., 2015).
In B. schlosseri, Vasa, PL10, and Oct4 co-expression identified PGC-like cells during embryonic and colony development (Rosner et al., 2009). In some cases, Vasa expression extends beyond germ cells. Vasa is found in somatic tissues of P. dumerilii, and in segmental tissues of Tubifex tubifex (Zelada González, 2005; Oyama and Shimizu, 2007; Rebscher et al., 2007). Acropora tenuis displays Vasa’s role in germline regulation throughout the oogenic cycle (Tan et al., 2020).
The study of A. virens investigates the Vasa, PL10, and Piwi genes, and their role in maintaining undifferentiated states in PGCs and multipotent stem cells (Kozin and Kostyuchenko, 2015). The Vasa orthologue in C. gigas is found only in germline cells (Fabioux et al., 2004b), while Mb vasa is identified in the testis of Maja brachydactyla, confirming its expression in the gonadal (Simeó et al., 2011). These findings collectively enhance our understanding of germ cell development and totipotent stem cells across various invertebrate species.
3.2 Applied techniques for MISC identification
Our -efforts to unlock the secrets of stem cells is guided by a wide assortment of techniques, each contributing a unique perspective to the puzzle. These techniques help us to unravel the multifaceted roles of stem cells, encompassing their involvement in developmental pathways, the stability of tissues, and the intricate mechanisms underlying diseases. By artfully combining these methods, researchers can comprehensively study stem cell populations, and gain a deeper comprehension of their functions. These versatile methodologies extend their utility not only for studying invertebrate but also for exploring mammalian systems studies. Some of the common techniques include flow cytometry, immunofluorescence/immunocytochemistry, in situ hybridization, Real-time RT-PCR, and western blotting. In the following, we embark on a detailed journey through these techniques, to uncover the valuable insights they provide (Tables 2, 3).
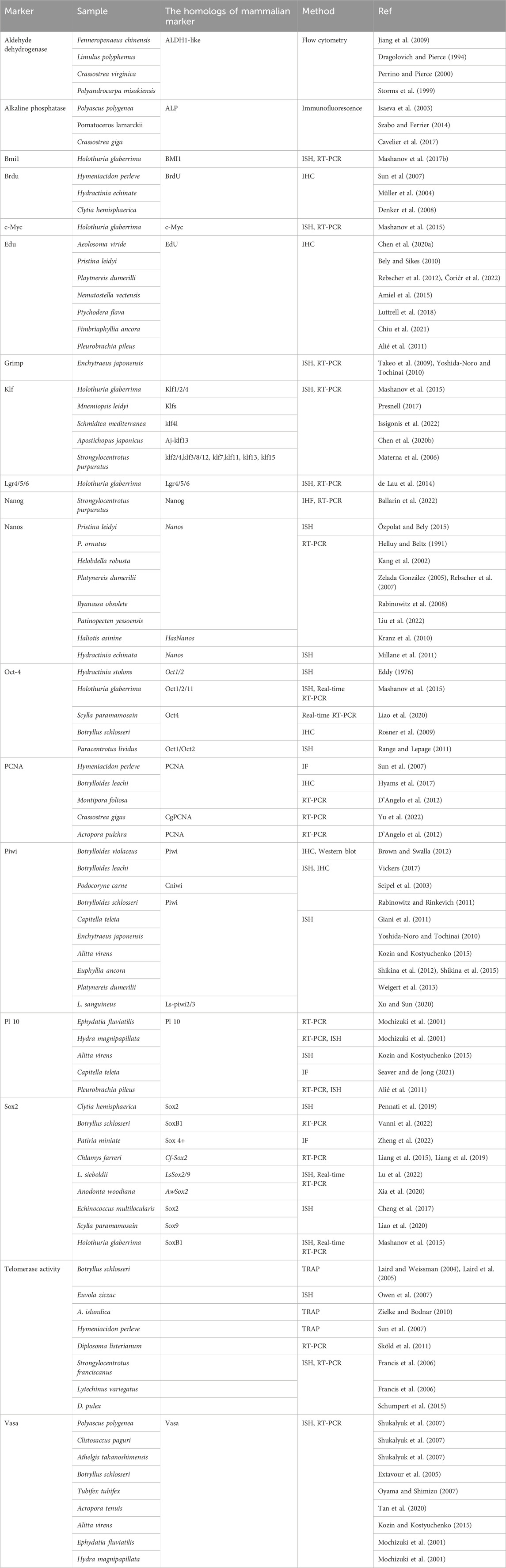
Table 2. Diversity of marine stem cell markers: comparative study across different marine organisms using various methods.
3.2.1 Flow cytometry
Flow cytometry, a potent analytical tool, enables for the simultaneous assessment of multiple cell markers within single cells. This method employs fluorescently labeled antibodies that bind to cell surface or intracellular markers. Subsequently, the labeled cells flow through a cytometer, which measures emitted fluorescence to quantify marker expression. Researchers utilize flow cytometry to delineate diverse cell populations based on marker profiles, making it useful for investigating heterogeneous groups, and identifying rare subsets (Schachtele et al., 2020). Additionally, this technique can be used for cell proliferation and cycle analysis (Wlodkowic et al., 2011; Wlodkowic et al., 2013). Flow cytometry dissects cell functions via surface and intracellular staining, hinges on cell surface antigens for cell type identification. Moreover, flow cytometry provides deeper insights by concurrently staining both cell surface and intracellular contents within single cells (Adan et al., 2017).
Flow cytometry is a valuable tool for both researchers and clinicians, facilitates identifying, characterizing, and isolating stem and progenitor cells for research and potential clinical applications (Adams et al., 2009). Multicolor flow cytometry enables the simultaneous examination of diverse stem cell markers (Schachtele, Clouser and Aho). This method has been effectively revealed stem cells in various organisms, including post-blastema tissue analysis in regenerating planarians, shedding light on neoblasts, or planarian stem cells (Ermakov et al., 2012).
In the case of Eupentacta fraudatrix, flow cytometry unveiled quantitative variations in coelomic fluid cells post-evisceration over 24 h. Notable dynamics emerged between minimally differentiated juvenile cells and well-differentiated cells during regeneration. The juvenile coelomocyte population increased, while differentiated cell quantity decreased, establishing a reciprocal pattern (Zavalnaya et al., 2020).
3.2.2 Immunofluorescence: immunocytochemistry/immunohistochemistry
Immunofluorescence, including immunocytochemistry and immunohistochemistry, employs antibodies to visualize specific markers within cells. Cells fixed on slides are first incubated with primary antibodies binding to target markers. Subsequently, fluorescently labeled secondary antibodies recognize the primary antibodies, producing a fluorescent signal at marker locations. This technique provides spatial insights into marker expression and subcellular localization (Odell and Cook, 2013). To maintain signal quality, samples must be shielded from light when not in use, ensuring reliable results (Lichtman and Conchello, 2005). Signal strength depends on antibody quality, sample handling, and secondary antibody detection (Odell and Cook, 2013).
An innovative approach uses immunocytochemistry to identify my-Vasa-positive germ cells in Yesso scallops, shedding light on reproductive cycle dynamics and shellfish hatcheries (Mokrina et al., 2021).
Another study utilizes immunocytochemistry with vasa antibodies, reverse transcription, and polymerase chain reaction to detect germline cell markers, providing a methodological basis for investigating oogonia in sea urchins (Yakovlev et al., 2010). Microfilament elongation towards the vegetal hemisphere is observed using time-lapse video and fluorescent labeling, emphasizing their role in germ plasm formation (Prodon et al., 2009). Immunocytochemistry with anti-Vasa antibodies reveals germ plasm and nuage material aggregation in chaetognaths (Carré et al., 2002).
Fluorescent and non-fluorescent recombinant proteins are effectively employed to track events in sea urchin post-fertilization. Microinjection of proteins and dyes into unfertilized eggs, observed via fluorescence, confocal, or two-photon microscopy, provides insights into the first cell cycle (Kisielewska and Whitaker, 2014).
3.2.3 In situ hybridization
In situ hybridization (ISH) seamlessly merges molecular biology and histochemical techniques to reveal gene expression within tissues and cytological samples. By pinpointing gene sequences and visualizing their products within their native context, ISH preserves cellular integrity in complex tissues, yielding anatomical insights (Jin and Lloyd, 1997). ISH uniquely identifies cells based on mRNA content, ensuring accuracy compared to potential false positives in immunostaining (Jin and Lloyd, 1997). A significant advantage is ISH’s ability to precisely localize mRNA at a cellular level, enhancing our understanding of intricate tissues and complementing other molecular methods. It is worth noting that false negatives can arise from RNA loss or probe issues, especially in retrospective paraffin-embedded studies (Jin and Lloyd, 1997).
Vasa orthologs serve as dependable markers for germline identification across species. In adult and juvenile pearl oysters (Pinctada fucata), ISH of the vasa ortholog (povlg1) unveils immature germ cells undetectable with traditional staining, benefiting pearl quality enhancement (Sano et al., 2015). Similarly, in L. sanguineus, ISH exposes distinct ovarian expression of Ls-piwi1 compared to absent Ls-piwi2 and Ls-piwi3 (Xu and Sun, 2020).
For another annelid, adult Myzostoma cirriferum, ISH highlights exclusive Piwi-like gene (Mc-Piwi1, Mc-Piwi2) presence in gonadal stem cells, excluding the posterior growth zone or somatic stem cells (Weigert et al., 2013). In C. hemisphaerica, a hydrozoan cnidarian, ISH reveals germ cell marker expressions (Vasa, Nanos1, Piwi, PL10) in multipotent interstitial cells during larval and adult medusa stages (Leclère et al., 2012). Focusing on F. chinensis, ISH confirms Fc-vasa transcript localization in spermatogonia and oocytes, illuminating Fc-Vasa’s role in germ-line development and potential as a marker (Zhou et al., 2010).
3.2.4 Real-time RT-PCR
The quantitative polymerase chain reaction (Q-PCR) is a valuable technique for assessing gene expression levels by measuring PCR product quantities in real-time. This method, also known as real-time PCR or quantitative reverse transcriptase PCR (RT-PCR), helps determine gene expression under specific conditions (Maddocks and Jenkins, 2017). In the study of prostate cancer cell lines and embryonic stem cells, reprogramming factor expression was evaluated using semi-quantitative RT-PCR (244). Fc-vasa exhibited gonadal-specific expression in Chinese shrimp, F. chinensis, with levels changing during gonadal development. Additionally, Fc-PL10a displayed widespread expression in examined tissues (Bae et al., 2010; Zhou et al., 2010).
Vasa is used as a molecular marker for PGCs visualization in various species. In tongue sole (Cynoglossus semilaevis), the vasa gene characterization identified to have three transcripts: vas-l, vas-m, and vas-s. Quantitative real-time PCR analysis has highlighted prevalent gonadal expression, particularly for vas-s during embryonic development (Wang et al., 2014). In Mytilus coruscus, gonad-specific genes and miRNAs were identified through transcriptome sequencing. The qRT-PCR analysis revealed differential expression, while miRNA targeting was confirmed through luciferase assays (Wang et al., 2022).
In the spider crab (Maja brachydactyla), the Mb vasa expression was examined using quantitative PCR during early post-embryonic development, showing fluctuations with developmental stages (Simeó et al., 2011). Furthermore, RNA interference targeting oyster vasa-like gene (Oyvlg) in C. gigas led to reduced germ cell proliferation and meiosis arrest (Fabioux et al., 2009).
In Botrylloides leachi, Mitomycin C treatments and siRNA knockdown of Bl-Piwi revealed its importance in regeneration and colony adaptation (Rinkevich et al., 2010). Analysis of BS-Vasa in Botryllus schlosseri demonstrated its presence in colony cells beyond germ cell lineages (Rosner et al., 2009).
3.2.5 Western blot
Western blotting is a sensitive technique employed to detect specific proteins or antibodies within complex mixtures that interact with discrete antigens (Laemmli, 1970). This method’s heightened sensitivity and specificity make it ideal for identifying low-concentration proteins. It allows the separation of intricate mixtures into individual components based on molecular weight and post-translational modifications, facilitating the identification of low-reactivity antigens (Kurien and Scofield, 2009).
However, it is important to acknowledge Western blotting’s limitations. Relying solely on a molecular weight ladder for comparison might lead to inaccurate conclusions. This is particularly pertinent for post-translational modification proteins, as the predicted molecular weight from the genome sequence might not precisely correspond to the actual gene product. Furthermore, the use of sodium dodecyl sulfate polyacrylamide gel electrophoresis (SDS-PAGE) could irreversibly denature all proteins in the lysate, potentially affecting the target protein’s detection (Algenäs et al., 2014).
In a study on Baltic Sea blue mussels, the impact of exposure to various doses of benzo [a]pyrene (BaP) for 3 days was investigated through the analysis of protein biomarkers. Notably, mussels exposed to the lowest dose, corresponding to the minimum reported dose for DNA adduct formation in their gills, exhibited significant changes in protein expression. Western blot analysis quantified the upregulated expression of proliferating cell nuclear antigen (PCNA). Conversely, immunocytochemistry analysis of the 5-bromo-deoxyuridine (BrdU) staining pattern revealed no substantial changes. These findings suggest that the PCNA response likely indicated non-proliferative activity, potentially linked to DNA damage (Prevodnik et al., 2007). Additionally, in order to gain insights into bivalve germ line development, VASA ortholog-specific antibodies were used to investigate different species, including Ruditapes philippinarum, Scapharca inaequivalvis, C. gigas, and Mya arenaria. Western blot analysis confirmed the immunoreactivity of the anti-VASA antibodies, displaying distinct bands in each species, with varying molecular weights. It`s worth noting that M. arenaria, like Ruditapes philippinarum, is reported as gonochoric (Milani et al., 2016).
4 Current limitations/challenges
Despite significant advancements in the study of marine invertebrate stem cells, several limitations and challenges persist.
1) Genetic Tools: The lack of comprehensive genetic tools for many marine invertebrates limits the ability to perform detailed functional analyses. While techniques such as RNAi and CRISPR-Cas9 are promising, their application is not yet widespread across all species.
2) Marker Identification and Validation: Research on marine invertebrate stem cells requires the identification of more markers to distinguish between differentiation levels of cells in different species. This will allow for the identification and isolation of pluripotent/totipotent stem cells. Validating these markers to ensure they indicate stem cell activity, rather than simply being orthologous to vertebrate markers, is crucial. This issue is directly associated with the problem of de-differentiation. In several cases, such as ascidian palleal budding or starfish regeneration, it cannot be excluded that cells from injured tissues or budding areas de-differentiate, adopting a stem cell phenotype capable of forming a bud primordium or a blastema. Unlike in mammals, this spontaneous de-differentiation under certain circumstances in invertebrates requires additional investigation for a deeper understanding.
3) Species-Specific Protocols: Each invertebrate species may require specific protocols for successful gene perturbation, making it difficult to generalize findings across different taxa.
4) Functional Studies on Adult Organisms: Functional experiments on adult marine invertebrates are often more challenging than on their larval or juvenile stages due to the complexity and resilience of adult tissues.
5) Technical limitations: Further efforts are needed to foster cooperation among organizations working on aquatic invertebrate stem cells. Enhanced collaboration could increase the use of invertebrate adult stem cells in biological research and help overcome technical problems that have hindered the achievement of stable stem cell cultures from aquatic invertebrates.
To advance our understanding of invertebrate stem cells, it is crucial to leverage existing functional studies while acknowledging the current limitations. By focusing on the available gene perturbation assays and their findings, we can highlight the unique aspects of invertebrate stem cells and pave the way for future research that addresses the current challenges. Additionally, developing and refining genetic tools for a broader range of invertebrate species will enhance our ability to perform comprehensive functional analyses and uncover the intricate biology of these fascinating organisms.
5 Conclusion
This study underscores the critical role of marker expression analysis in advancing our understanding of marine invertebrate stem cells. The deployment of specific markers and sophisticated molecular techniques, such as flow cytometry, immunocytochemistry, protein arrays, and immunoblotting, offers profound insights into the characterization and identification of these stem cells (Figure 2). Through detailed investigation of marker expression patterns, researchers can explore the potential of marine invertebrate stem cells for applications in regenerative medicine, drug discovery, and the broader study of these unique organisms. The integration of these techniques with advanced methods like electron microscopy provides a comprehensive view of the distinctive properties of marine invertebrate stem cells. Looking ahead, ongoing advancements in marker detection, coupled with the integration of omics approaches and computational tools, promise to further refine our understanding of stem cell differentiation, cell heterogeneity, and therapeutic applications. These developments will enhance our ability to analyze individual cell profiles and marker expression across cell populations.
Overall, this article highlights the significance of marker expression analysis and the application of advanced techniques in marine invertebrate stem cell research. By offering valuable insights while circumventing ethical issues associated with human cell sources, these studies pave the way for future breakthroughs in regenerative medicine and marine biotechnology. Continued research in this field will undoubtedly lead to the discovery of novel markers, a deeper understanding of cellular mechanisms, and significant advancements in the application of marine invertebrate stem cells.
Author contributions
FM: Conceptualization, Data curation, Formal Analysis, Investigation, Writing–original draft. AK: Data curation, Writing–original draft. BR: Writing–original draft. TZ: Data curation, Writing–original draft. IN: Writing–original draft, Writing–review and editing. NB: Conceptualization, Data curation, Investigation, Methodology, Writing–original draft, Writing–review and editing.
Funding
The author(s) declare that no financial support was received for the research, authorship, and/or publication of this article.
Conflict of interest
The authors declare that the research was conducted in the absence of any commercial or financial relationships that could be construed as a potential conflict of interest.
Publisher’s note
All claims expressed in this article are solely those of the authors and do not necessarily represent those of their affiliated organizations, or those of the publisher, the editors and the reviewers. Any product that may be evaluated in this article, or claim that may be made by its manufacturer, is not guaranteed or endorsed by the publisher.
References
Abdelhaleem, M. (2005). RNA helicases: regulators of differentiation. Clin. Biochem. 38 (6), 499–503. doi:10.1016/j.clinbiochem.2005.01.010
Adams, V., Challen, G. A., Zuba-Surma, E., Ulrich, H., Vereb, G., and Tárnok, A. (2009). Where new approaches can stem from: focus on stem cell identification. Wiley Online Library.
Adan, A., Alizada, G., Kiraz, Y., Baran, Y., and Nalbant, A. (2017). Flow cytometry: basic principles and applications. Crit. Rev. Biotechnol. 37 (2), 163–176. doi:10.3109/07388551.2015.1128876
Afshar, A., Khoradmehr, A., Nowzari, F., Baghban, N., Zare, M., Najafi, M., et al. (2023). Tissue extract from brittle star undergoing arm regeneration promotes wound healing in rat. Mar. Drugs 21 (7), 381. doi:10.3390/md21070381
Algenäs, C., Agaton, C., Fagerberg, L., Asplund, A., Björling, L., Björling, E., et al. (2014). Antibody performance in western blot applications is context-dependent. Biotechnol. J. 9 (3), 435–445. doi:10.1002/biot.201300341
Alié, A., Leclère, L., Jager, M., Dayraud, C., Chang, P., Le Guyader, H., et al. (2011). Somatic stem cells express Piwi and Vasa genes in an adult ctenophore: ancient association of “germline genes” with stemness. Dev. Biol. 350 (1), 183–197. doi:10.1016/j.ydbio.2010.10.019
Amiel, A. R., Johnston, H. T., Nedoncelle, K., Warner, J. F., Ferreira, S., and Röttinger, E. (2015). Characterization of morphological and cellular events underlying oral regeneration in the sea anemone, Nematostella vectensis. Int. J. Mol. Sci. 16 (12), 28449–28471. doi:10.3390/ijms161226100
Anderson, R., Fulton, N., Cowan, G., Coutts, S., and Saunders, P. (2007). Conserved and divergent patterns of expression of DAZL, VASA and OCT4 in the germ cells of the human fetal ovary and testis. BMC Dev. Biol. 7, 136–145. doi:10.1186/1471-213X-7-136
Arenas-Mena, C. (2010). Indirect development, transdifferentiation and the macroregulatory evolution of metazoans. Philosophical Trans. R. Soc. B Biol. Sci. 365 (1540), 653–669. doi:10.1098/rstb.2009.0253
Bae, K.-M., Su, Z., Frye, C., McClellan, S., Allan, R. W., Andrejewski, J. T., et al. (2010). Expression of pluripotent stem cell reprogramming factors by prostate tumor initiating cells. J. urology 183 (5), 2045–2053. doi:10.1016/j.juro.2009.12.092
Baghban, N., Khoradmehr, A., Afshar, A., Jafari, N., Zendehboudi, T., Rasekh, P., et al. (2023). MRI tracking of marine proliferating cells in vivo using anti-oct4 antibody-conjugated iron nanoparticles for precision in regenerative medicine. Biosensors 13 (2), 268. doi:10.3390/bios13020268
Baghban, N., Khoradmehr, A., Nabipour, I., Tamadon, A., and Ullah, M. (2022). The potential of marine-based gold nanomaterials in cancer therapy: a mini-review. Gold Bull. 55 (1), 53–63. doi:10.1007/s13404-021-00304-6
Ballarin, L., Rinkevich, B., Bartscherer, K., Burzynski, A., Cambier, S., Cammarata, M., et al. (2018). Maristem—stem cells of marine/aquatic invertebrates: from basic research to innovative applications. Sustainability 10 (2), 526. doi:10.3390/su10020526
Ballarin, L., Rinkevich, B., and Hobmayer, B. (2022). Advances in aquatic invertebrate stem cell research: from basic research to innovative applications. MDPI-Multidisciplinary Digital Publishing Institute.
Belair, C. D., Yeager, T. R., Lopez, P. M., and Reznikoff, C. A. (1997). Telomerase activity: a biomarker of cell proliferation, not malignant transformation. Proc. Natl. Acad. Sci. 94 (25), 13677–13682. doi:10.1073/pnas.94.25.13677
Bellé, R., Le Bouffant, R., Morales, J., Cosson, B., Cormier, P., and Mulner-Lorillon, O. (2007). Sea urchin embryo, DNA-damaged cell cycle checkpoint and the mechanisms initiating cancer development. J. de Soc. de Biol. 201 (3), 317–327. doi:10.1051/jbio:2007030
Bely, A. E., and Sikes, J. M. (2010). Latent regeneration abilities persist following recent evolutionary loss in asexual annelids. Proc. Natl. Acad. Sci. 107 (4), 1464–1469. doi:10.1073/pnas.0907931107
Benton, J. L., Kery, R., Li, J., Noonin, C., Söderhäll, I., and Beltz, B. S. (2014). Cells from the immune system generate adult-born neurons in crayfish. Dev. Cell 30 (3), 322–333. doi:10.1016/j.devcel.2014.06.016
Bevelander, G. (1952). Calcification in molluscs. III. Intake and deposition of Ca45 and P32 in relation to shell formation. Biol. Bull. 102 (1), 9–15. doi:10.2307/1538617
Blackburn, E. H. (1991). Structure and function of telomeres. Nature 350 (6319), 569–573. doi:10.1038/350569a0
Bodnar, A. G., and Coffman, J. A. (2016). Maintenance of somatic tissue regeneration with age in short-and long-lived species of sea urchins. Aging cell 15 (4), 778–787. doi:10.1111/acel.12487
Boyer, L. A., Lee, T. I., Cole, M. F., Johnstone, S. E., Levine, S. S., Zucker, J. P., et al. (2005). Core transcriptional regulatory circuitry in human embryonic stem cells. Cell 122 (6), 947–956. doi:10.1016/j.cell.2005.08.020
Brown, F. D., and Swalla, B. J. (2012). Evolution and development of budding by stem cells: ascidian coloniality as a case study. Dev. Biol. 369 (2), 151–162. doi:10.1016/j.ydbio.2012.05.038
Buchet, R., Millán, J. L., and Magne, D. (2013). “Multisystemic functions of alkaline phosphatases,” in Phosphatase modulators (Springer), 27–51.
Cai, J., Olson, J. M., Rao, M. S., Stanley, M., Taylor, E., and Ni, H.-T. (2005). Development of antibodies to human embryonic stem cell antigens. BMC Dev. Biol. 5 (1), 26–27. doi:10.1186/1471-213X-5-26
Campisi, J., Kim, S.-h., Lim, C.-S., and Rubio, M. (2001). Cellular senescence, cancer and aging: the telomere connection. Exp. Gerontol. 36 (10), 1619–1637. doi:10.1016/s0531-5565(01)00160-7
Carmell, M. A., Girard, A., Van De Kant, H. J., Bourc'his, D., Bestor, T. H., de Rooij, D. G., et al. (2007). MIWI2 is essential for spermatogenesis and repression of transposons in the mouse male germline. Dev. cell 12 (4), 503–514. doi:10.1016/j.devcel.2007.03.001
Carré, D. l., Djediat, C., and Sardet, C. (2002). Formation of a large Vasa-positive germ granule and its inheritance by germ cells in the enigmatic Chaetognaths. Development 129 (3), 661–670. doi:10.1242/dev.129.3.661
Carroll, A. R., Copp, B. R., Davis, R. A., Keyzers, R. A., and Prinsep, M. R. (2022). Marine natural products. Nat. Product. Rep. 40, 275–325. doi:10.1039/D2NP00083K
Cavaleri, F., and Schöler, H. R. (2003). Nanog: a new recruit to the embryonic stem cell orchestra. Cell 113 (5), 551–552. doi:10.1016/s0092-8674(03)00394-5
Cavelier, P., Cau, J., Morin, N., and Delsert, C. (2017). Early gametogenesis in the Pacific oyster: new insights using stem cell and mitotic markers. J. Exp. Biol. 220 (21), 3988–3996. doi:10.1242/jeb.167734
Chambers, I., Colby, D., Robertson, M., Nichols, J., Lee, S., Tweedie, S., et al. (2003). Functional expression cloning of Nanog, a pluripotency sustaining factor in embryonic stem cells. Cell 113 (5), 643–655. doi:10.1016/s0092-8674(03)00392-1
Chambers, I., Silva, J., Colby, D., Nichols, J., Nijmeijer, B., Robertson, M., et al. (2007). Nanog safeguards pluripotency and mediates germline development. Nature 450 (7173), 1230–1234. doi:10.1038/nature06403
Char, B. R., Bell, J. R., Dovala, J., Coffman, J. A., Harrington, M. G., Becerra, J. C., et al. (1993). SpOct, a gene encoding the major octamer-binding protein in sea urchin embryos: expression profile, evolutionary relationships, and DNA binding of expressed protein. Dev. Biol. 158 (2), 350–363. doi:10.1006/dbio.1993.1194
Chen, C. P., Fok, S. K.-W., Hsieh, Y. W., Chen, C. Y., Hsu, F. M., Chang, Y. H., et al. (2020a). General characterization of regeneration in Aeolosoma viride (Annelida, Aeolosomatidae). Invertebr. Biol. 139 (1), e12277. doi:10.1111/ivb.12277
Chen, L., Yao, F., Qin, Y., Shao, Y., Fang, L., Yu, X., et al. (2020b). The potential role of Krüppel-like factor 13 (Aj-klf13) in the intestine regeneration of sea cucumber Apostichopus japonicus. Gene 735, 144407. doi:10.1016/j.gene.2020.144407
Cheng, Z., Liu, F., Dai, M., Wu, J., Li, X., Guo, X., et al. (2017). Identification of EmSOX2, a member of the Sox family of transcription factors, as a potential regulator of Echinococcus multilocularis germinative cells. Int. J. Parasitol. 47 (10), 625–632. doi:10.1016/j.ijpara.2017.03.005
Chiquoine, A. D. (1954). The identification, origin, and migration of the primordial germ cells in the mouse embryo. Anatomical Rec. 118 (2), 135–146. doi:10.1002/ar.1091180202
Chiu, Y.-L., Chang, C.-F., and Shikina, S. (2021). Development of an in vitro tissue culture system for hammer coral (Fimbriaphyllia ancora) ovaries. Sci. Rep. 11 (1), 24338–24410. doi:10.1038/s41598-021-03810-x
Cima, F., Perin, A., Burighel, P., and Ballarin, L. (2001). Morpho-functional characterization of haemocytes of the compound ascidian Botrylloides leachi (Tunicata, Ascidiacea). Acta Zool. 82 (4), 261–274. doi:10.1046/j.1463-6395.2001.00087.x
Ćorić, A., Stockinger, A. W., Schaffer, P., Rokvić, D., Tessmar-Raible, K., and Raible, F. (2022). A fast and versatile method for simultaneous HCR, immunohistochemistry and EdU labeling (SHInE). bioRxiv.
Curtis, D., Treiber, D. K., Tao, F., Zamore, P. D., Williamson, J. R., and Lehmann, R. (1997). A CCHC metal-binding domain in Nanos is essential for translational regulation. EMBO J. 16 (4), 834–843. doi:10.1093/emboj/16.4.834
D’Angelo, C., Smith, E. G., Oswald, F., Burt, J., Tchernov, D., and Wiedenmann, J. (2012). Locally accelerated growth is part of the innate immune response and repair mechanisms in reef-building corals as detected by green fluorescent protein (GFP)-like pigments. Coral reefs 31 (4), 1045–1056. doi:10.1007/s00338-012-0926-8
Dehghani, H., Rashedinia, M., Mohebbi, G., Vazirizadeh, A., and Baghban, N. (2024). Antioxidant and anticholinesterase properties of Echinometra mathaei and Ophiocoma erinaceus venoms from the Persian Gulf. Front. Chem. 11, 1332921. doi:10.3389/fchem.2023.1332921
de Lau, W., Peng, W. C., Gros, P., and Clevers, H. (2014). The R-spondin/Lgr5/Rnf43 module: regulator of Wnt signal strength. Genes and Dev. 28 (4), 305–316. doi:10.1101/gad.235473.113
Deng, W., and Lin, H. (2002). Miwi, a murine homolog of piwi, encodes a cytoplasmic protein essential for spermatogenesis. Dev. cell 2 (6), 819–830. doi:10.1016/s1534-5807(02)00165-x
Denker, E., Manuel, M., Leclère, L., Le Guyader, H., and Rabet, N. (2008). Ordered progression of nematogenesis from stem cells through differentiation stages in the tentacle bulb of Clytia hemisphaerica (Hydrozoa, Cnidaria). Dev. Biol. 315 (1), 99–113. doi:10.1016/j.ydbio.2007.12.023
Dill, K. K., and Seaver, E. C. (2008). Vasa and nanos are coexpressed in somatic and germ line tissue from early embryonic cleavage stages through adulthood in the polychaete Capitella sp. I. Dev. genes Evol. 218, 453–463. doi:10.1007/s00427-008-0236-x
Dolbeare, F., and Selden, J. R. (1994). Immunochemical quantitation of bromodeoxyuridine: application to cell–cycle kinetics. Methods cell Biol. 41, 297–316. doi:10.1016/S0091-679X(08)61724-0
Dragolovich, J., and Pierce, S. K. (1994). Characterization of partially purified betaine aldehyde dehydrogenase from horseshoe crab (Limulus polyphemus) cardiac mitochondria. J. Exp. Zoology 270 (5), 417–425. doi:10.1002/jez.1402700502
Durham, M., Beck, C., and Keeling, E. (2016). Quantification of telomerase activity in colonial ascidians. FASEB J. 30, 1051–1053. doi:10.1096/fasebj.30.1_supplement.1051.3
Eddy, E. (1976). Germ plasm and the differentiation of the germ cell line. Int. Rev. Cytol. 43, 229–280. doi:10.1016/s0074-7696(08)60070-4
Ermakov, A. M., Ermakova, O. N., Kudravtsev, A. A., and Kreshchenko, N. D. (2012). Study of planarian stem cell proliferation by means of flow cytometry. Mol. Biol. Rep. 39 (3), 3073–3080. doi:10.1007/s11033-011-1070-1
Extavour, C. G., and Akam, M. (2003). Mechanisms of germ cell specification across the metazoans: epigenesis and preformation. Development 130, 5869–5884. doi:10.1242/dev.00804
Extavour, C. G., Pang, K., Matus, D. Q., and Martindale, M. Q. (2005). Vasa and nanos expression patterns in a sea anemone and the evolution of bilaterian germ cell specification mechanisms. Evol. and Dev. 7 (3), 201–215. doi:10.1111/j.1525-142X.2005.05023.x
Fabioux, C., Corporeau, C., Quillien, V., Favrel, P., and Huvet, A. (2009). In vivo RNA interference in oyster–vasa silencing inhibits germ cell development. FEBS J. 276 (9), 2566–2573. doi:10.1111/j.1742-4658.2009.06982.x
Fabioux, C., Huvet, A., Lelong, C., Robert, R., Pouvreau, S., Daniel, J.-Y., et al. (2004a). Oyster vasa-like gene as a marker of the germline cell development in Crassostrea gigas. Biochem. biophysical Res. Commun. 320 (2), 592–598. doi:10.1016/j.bbrc.2004.06.009
Fabioux, C., Pouvreau, S., Roux, F. L., and Huvet, A. (2004b). The oyster vasa-like gene: a specific marker of the germline in Crassostrea gigas. Biochem. Biophysical Res. Commun. 315 (4), 897–904. doi:10.1016/j.bbrc.2004.01.145
Ferrario, C., Sugni, M., Somorjai, I. M., and Ballarin, L. (2020). Beyond adult stem cells: dedifferentiation as a unifying mechanism underlying regeneration in invertebrate deuterostomes. Front. Cell Dev. Biol. 8, 587320. doi:10.3389/fcell.2020.587320
Forsyth, N. R., Wright, W. E., and Shay, J. W. (2002). Telomerase and differentiation in multicellular organisms: turn it off, turn it on, and turn it off again. Differentiation 69 (4-5), 188–197. doi:10.1046/j.1432-0436.2002.690412.x
Francis, N., Gregg, T., Owen, R., Ebert, T., and Bodnar, A. (2006). Lack of age-associated telomere shortening in long-and short-lived species of sea urchins. FEBS Lett. 580 (19), 4713–4717. doi:10.1016/j.febslet.2006.07.049
Franco, A., Berthelin, C. H., Goux, D., Sourdaine, P., and Mathieu, M. (2008). Fine structure of the early stages of spermatogenesis in the Pacific oyster, Crassostrea gigas (Mollusca, Bivalvia). Tissue Cell 40 (4), 251–260. doi:10.1016/j.tice.2007.12.006
Frank, U., Plickert, G., and Müller, W. A. (2009). “Cnidarian interstitial cells: the dawn of stem cell research,” in Stem cells in marine organisms (Springer), 33–59.
Gallant, P. (2006). “Myc/Max/Mad in invertebrates: the evolution of the Max network,” in The myc/max/mad transcription factor network (Springer), 235–253.
Giani, V. C., Yamaguchi, E., Boyle, M. J., and Seaver, E. C. (2011). Somatic and germline expression of piwi during development and regeneration in the marine polychaete annelid Capitella teleta. EvoDevo 2, 10–18. doi:10.1186/2041-9139-2-10
Glinka, A., Dolde, C., Kirsch, N., Huang, Y. L., Kazanskaya, O., Ingelfinger, D., et al. (2011). LGR4 and LGR5 are R-spondin receptors mediating Wnt/β-catenin and Wnt/PCP signalling. EMBO Rep. 12 (10), 1055–1061. doi:10.1038/embor.2011.175
Govindasamy, N., Murthy, S., and Ghanekar, Y. (2014). Slow-cycling stem cells in hydra contribute to head regeneration. Biol. open 3 (12), 1236–1244. doi:10.1242/bio.201410512
Greider, C. W. (1990). Telomeres, telomerase and senescence. Bioessays 12 (8), 363–369. doi:10.1002/bies.950120803
Gremigni, V. (1981). “The problem of cell totipotency, dedifferentiation and transdifferentiation in Turbellaria,” in The biology of the Turbellaria (Springer), 171–179.
Gremigni, V., and Puccinelli, I. (1977). A contribution to the problem of the origin of the blastema cells in planarians: a karyological and ultrastructural investigation. J. Exp. Zoology 199 (1), 57–71. doi:10.1002/jez.1401990108
Gruber, H., Schaible, R., Ridgway, I. D., Chow, T. T., Held, C., and Philipp, E. E. (2014). Telomere-independent ageing in the longest-lived non-colonial animal, Arctica islandica. Exp. Gerontol. 51, 38–45. doi:10.1016/j.exger.2013.12.014
Guo, T., Peters, A. H., and Newmark, P. A. (2006). A Bruno-like gene is required for stem cell maintenance in planarians. Dev. cell 11 (2), 159–169. doi:10.1016/j.devcel.2006.06.004
Hartung, O., Forbes, M. M., and Marlow, F. L. (2014). Zebrafish vasa is required for germ-cell differentiation and maintenance. Mol. reproduction Dev. 81 (10), 946–961. doi:10.1002/mrd.22414
Hatfield, S., Shcherbata, H., Fischer, K., Nakahara, K., Carthew, R., and Ruohola-Baker, H. (2005). Stem cell division is regulated by the microRNA pathway. Nature 435 (7044), 974–978. doi:10.1038/nature03816
Haupt, Y., Bath, M., Harris, A., and Adams, J. (1993). bmi-1 transgene induces lymphomas and collaborates with myc in tumorigenesis. Oncogene 8 (11), 3161–3164.
Helluy, S., and Beltz, B. (1991). Embryonic development of the American lobster (Homarus americanus): quantitative staging and characterization of an embryonic molt cycle. Biol. Bull. 180 (3), 355–371. doi:10.2307/1542337
Hemmrich, G., Khalturin, K., Boehm, A.-M., Puchert, M., Anton-Erxleben, F., Wittlieb, J., et al. (2012). Molecular signatures of the three stem cell lineages in hydra and the emergence of stem cell function at the base of multicellularity. Mol. Biol. Evol. 29 (11), 3267–3280. doi:10.1093/molbev/mss134
Houwing, S., Berezikov, E., and Ketting, R. F. (2008). Zili is required for germ cell differentiation and meiosis in zebrafish. EMBO J. 27 (20), 2702–2711. doi:10.1038/emboj.2008.204
Hutvágner, G., and Zamore, P. D. (2002). A microRNA in a multiple-turnover RNAi enzyme complex. Science 297 (5589), 2056–2060. doi:10.1126/science.1073827
Hwang, J. S., Ohyanagi, H., Hayakawa, S., Osato, N., Nishimiya-Fujisawa, C., Ikeo, K., et al. (2007). The evolutionary emergence of cell type-specific genes inferred from the gene expression analysis of Hydra. Proc. Natl. Acad. Sci. 104 (37), 14735–14740. doi:10.1073/pnas.0703331104
Hyams, Y., Paz, G., Rabinowitz, C., and Rinkevich, B. (2017). Insights into the unique torpor of Botrylloides leachi, a colonial urochordate. Dev. Biol. 428 (1), 101–117. doi:10.1016/j.ydbio.2017.05.020
Isaeva, V., Akhmadieva, A., Aleksandrova, Y. N., Shukalyuk, A., and Chernyshev, A. (2011). Germinal granules in interstitial cells of the colonial hydroids Obelia longissima pallas, 1766 and Ectopleura crocea Agassiz, 1862. Russ. J. Mar. Biol. 37 (4), 303–310. doi:10.1134/s1063074011040055
Isaeva, V., Shukaliuk, A., and Kizilova, E. (2003). Recognition of stem cells in the colonial internae of the Rhizocephala Peltogasterella gracilis and Sacculina polygenea at the parasitic life cycle stage. Tsitologiia 45 (8), 758–763.
Isaeva, V., Shukalyuk, A., and Akhmadieva, A. (2008). Stem cells in reproductive strategy of asexually reproducing invertebrates. Russ. J. Mar. Biol. 34, 1–8. doi:10.1134/s106307400801001x
Issigonis, M., Redkar, A. B., Rozario, T., Khan, U. W., Mejia-Sanchez, R., Lapan, S. W., et al. (2022). A Krüppel-like factor is required for development and regeneration of germline and yolk cells from somatic stem cells in planarians. PLoS Biol. 20 (7), e3001472. doi:10.1371/journal.pbio.3001472
Jackson, B., Brocker, C., Thompson, D. C., Black, W., Vasiliou, K., Nebert, D. W., et al. (2011). Update on the aldehyde dehydrogenase gene (ALDH) superfamily. Hum. genomics 5 (4), 283–303. doi:10.1186/1479-7364-5-4-283
Jacobs, J. J., Kieboom, K., Marino, S., DePinho, R. A., and Van Lohuizen, M. (1999). The oncogene and Polycomb-group gene bmi-1 regulates cell proliferation and senescence through the ink4a locus. Nature 397 (6715), 164–168. doi:10.1038/16476
Jayesh, P., Vrinda, S., Priyaja, P., Philip, R., and Singh, I. B. (2016). Impaired telomerase activity hinders proliferation and in vitro transformation of Penaeus monodon lymphoid cells. Cytotechnology 68, 1301–1314. doi:10.1007/s10616-015-9890-9
Jemaà, M., Morin, N., Cavelier, P., Cau, J., Strub, J. M., and Delsert, C. (2014). Adult somatic progenitor cells and hematopoiesis in oysters. J. Exp. Biol. 217 (17), 3067–3077. doi:10.1242/jeb.106575
Jen, J., Tang, Y.-A., Lu, Y.-H., Lin, C.-C., Lai, W.-W., and Wang, Y.-C. (2017). Oct4 transcriptionally regulates the expression of long non-coding RNAs NEAT1 and MALAT1 to promote lung cancer progression. Mol. cancer 16, 104–112. doi:10.1186/s12943-017-0674-z
Jiang, H., Li, F., Xie, Y., Huang, B., Zhang, J., Zhang, J., et al. (2009). Comparative proteomic profiles of the hepatopancreas in Fenneropenaeus chinensis response to hypoxic stress. Proteomics 9 (12), 3353–3367. doi:10.1002/pmic.200800518
Jin, L., and Lloyd, R. V. (1997). In situ hybridization: methods and applications. J. Clin. Lab. Anal. 11 (1), 2–9. doi:10.1002/(SICI)1098-2825(1997)11:1<2::AID-JCLA2>3.0.CO;2-F
Juliano, C. E., Reich, A., Liu, N., Götzfried, J., Zhong, M., Uman, S., et al. (2014). PIWI proteins and PIWI-interacting RNAs function in Hydra somatic stem cells. Proc. Natl. Acad. Sci. 111 (1), 337–342. doi:10.1073/pnas.1320965111
Juliano, C. E., Swartz, S. Z., and Wessel, G. M. (2010). A conserved germline multipotency program. Development 137 (24), 4113–4126. doi:10.1242/dev.047969
Juliano, C. E., Voronina, E., Stack, C., Aldrich, M., Cameron, A. R., and Wessel, G. M. (2006). Germ line determinants are not localized early in sea urchin development, but do accumulate in the small micromere lineage. Dev. Biol. 300 (1), 406–415. doi:10.1016/j.ydbio.2006.07.035
Kang, D., Pilon, M., and Weisblat, D. A. (2002). Maternal and zygotic expression of a nanos-class gene in the leech Helobdella robusta: primordial germ cells arise from segmental mesoderm. Dev. Biol. 245 (1), 28–41. doi:10.1006/dbio.2002.0615
Kang, S. K., Park, J. B., and Cha, S. H. (2006). Multipotent, dedifferentiated cancer stem-like cells from brain gliomas. Stem cells Dev. 15 (3), 423–435. doi:10.1089/scd.2006.15.423
Karthik, L., Kumar, G., Keswani, T., Bhattacharyya, A., Chandar, S., and Bhaskara Rao, K. V. (2014). Protease inhibitors from marine actinobacteria as a potential source for antimalarial compound. PLoS One 9, e90972. doi:10.1371/journal.pone.0090972
Keshavarz, M., Shamsizadeh, F., Tavakoli, A., Baghban, N., Khoradmehr, A., Kameli, A., et al. (2021). Chemical compositions and experimental and computational modeling activity of sea cucumber Holothuria parva ethanolic extract against herpes simplex virus type 1. Biomed. and Pharmacother. 141, 111936. doi:10.1016/j.biopha.2021.111936
Kim, N. W., Piatyszek, M. A., Prowse, K. R., Harley, C. B., West, M. D., Ho, P. d. L., et al. (1994). Specific association of human telomerase activity with immortal cells and cancer. Science 266 (5193), 2011–2015. doi:10.1126/science.7605428
Kisielewska, J., and Whitaker, M. (2014). Regulation of DNA synthesis at the first cell cycle in the sea urchin in vivo. Methods Mol. Biol. 1128, 223–236. doi:10.1007/978-1-62703-974-1_15
Knapp, D., and Tanaka, E. M. (2012). Regeneration and reprogramming. Curr. Opin. Genet. and Dev. 22 (5), 485–493. doi:10.1016/j.gde.2012.09.006
Kostyuchenko, R. P., and Kozin, V. V. (2021). Comparative aspects of annelid regeneration: towards understanding the mechanisms of regeneration. Genes 12 (8), 1148. doi:10.3390/genes12081148
Kozin, V. V., and Kostyuchenko, R. P. (2015). Vasa, PL10, and Piwi gene expression during caudal regeneration of the polychaete annelid Alitta virens. Dev. genes Evol. 225, 129–138. doi:10.1007/s00427-015-0496-1
Kranz, A. M., Tollenaere, A., Norris, B. J., Degnan, B. M., and Degnan, S. M. (2010). Identifying the germline in an equally cleaving mollusc: vasa and Nanos expression during embryonic and larval development of the vetigastropod Haliotis asinina. J. Exp. Zoology Part B Mol. Dev. Evol. 314 (4), 267–279. doi:10.1002/jez.b.21336
Kurien, B. T., and Scofield, R. H. (2009). Sending secret messages on nitrocellulose membrane and the use of a molecular pen for orientation in ECL membrane assays. Methods Mol. Biol. 536, 573–581. doi:10.1007/978-1-59745-542-8_57
Ladurner, P., Rieger, R., and Baguñà, J. (2000). Spatial distribution and differentiation potential of stem cells in hatchlings and adults in the marine platyhelminth Macrostomum sp.: a bromodeoxyuridine analysis. Dev. Biol. 226 (2), 231–241. doi:10.1006/dbio.2000.9867
Laemmli, U. K. (1970). Cleavage of structural proteins during the assembly of the head of bacteriophage T4. nature 227 (5259), 680–685. doi:10.1038/227680a0
Laird, D. J., De Tomaso, A. W., and Weissman, I. L. (2005). Stem cells are units of natural selection in a colonial ascidian. Cell 123 (7), 1351–1360. doi:10.1016/j.cell.2005.10.026
Laird, D. J., and Weissman, I. L. (2004). Telomerase maintained in self-renewing tissues during serial regeneration of the urochordate Botryllus schlosseri. Dev. Biol. 273 (2), 185–194. doi:10.1016/j.ydbio.2004.05.029
Lang, G., Wang, Y., Nomura, N., and Matsumura, M. (2004). Detection of telomerase activity in tissues and primary cultured lymphoid cells of Penaeus japonicus. Mar. Biotechnol. 6 (4), 347–354. doi:10.1007/s10126-003-0038-0
Leclère, L., Jager, M., Barreau, C., Chang, P., Le Guyader, H., Manuel, M., et al. (2012). Maternally localized germ plasm mRNAs and germ cell/stem cell formation in the cnidarian Clytia. Dev. Biol. 364 (2), 236–248. doi:10.1016/j.ydbio.2012.01.018
Lehr, N. (2003). The transcriptional function of the c-Myc oncoprotein and its regulation by the ubiquitin/proteasome pathway.
Li, Y., Liu, L., Zhang, L., Wei, H., Wu, S., Liu, T., et al. (2022). Dynamic transcriptome analysis reveals the gene network of gonadal development from the early history life stages in dwarf surfclam Mulinia lateralis. Biol. Sex Differ. 13 (1), 69. doi:10.1186/s13293-022-00479-3
Liang, S., Liu, D., Li, X., Wei, M., Yu, X., Li, Q., et al. (2019). SOX2 participates in spermatogenesis of Zhikong scallop Chlamys farreri. Sci. Rep. 9 (1), 76. doi:10.1038/s41598-018-35983-3
Liang, S., Ma, X., Han, T., Yang, D., and Zhang, Z. (2015). Expression pattern of Chlamys farreri sox2 in eggs, embryos and larvae of various stages. J. Ocean Univ. China 14, 731–738. doi:10.1007/s11802-015-2558-4
Liao, J., Zhang, Z., Jia, X., Zou, Z., Liang, K., and Wang, Y. (2020). Transcriptional regulation of vih by Oct4 and Sox9 in Scylla paramamosain. Front. Endocrinol. 11, 650. doi:10.3389/fendo.2020.00650
Lichtman, J. W., and Conchello, J.-A. (2005). Fluorescence microscopy. Nat. methods 2 (12), 910–919. doi:10.1038/nmeth817
Lin, G., Wang, G., Banie, L., Ning, H., Shindel, A. W., Fandel, T. M., et al. (2010). Treatment of stress urinary incontinence with adipose tissue-derived stem cells. Cytotherapy 12 (1), 88–95. doi:10.3109/14653240903350265
Liu, H., and Nonomura, K.-I. (2016). A wide reprogramming of histone H3 modifications during male meiosis I in rice is dependent on the Argonaute protein MEL1. J. cell Sci. 129 (19), 3553–3561. doi:10.1242/jcs.184937
Liu, L., Liu, T., Wu, S., Li, Y., Wei, H., Zhang, L., et al. (2022). Discovery of Nanos1 and Nanos2/3 as germ cell markers during scallop gonadal development. Mar. Biotechnol. 24 (2), 408–416. doi:10.1007/s10126-022-10124-0
Lu, M., Xing, Z., Zhou, Y., Xu, Y., Peng, H., Zou, J., et al. (2022). Cloning and expression of Sox2 and Sox9 in embryonic and gonadal development of lutraria sieboldii. Fishes 7 (6), 392. doi:10.3390/fishes7060392
Luciano, P., Coulon, S., Faure, V., Corda, Y., Bos, J., Brill, S. J., et al. (2012). RPA facilitates telomerase activity at chromosome ends in budding and fission yeasts. EMBO J. 31 (8), 2034–2046. doi:10.1038/emboj.2012.40
Luttrell, S. M., Su, Y.-H., and Swalla, B. J. (2018). Getting a head with Ptychodera flava larval regeneration. Biol. Bull. 234 (3), 152–164. doi:10.1086/698510
Maddocks, S., and Jenkins, R. (2017). “Chapter 4–Quantitative PCR: things to consider,” in Understanding PCR (Boston: Academic), 45–52.
Mahani, A., Henriksson, J., and Wright, A. P. (2013). Origins of Myc proteins–using intrinsic protein disorder to trace distant relatives. PloS one 8 (9), e75057. doi:10.1371/journal.pone.0075057
Marchitti, S. A., Brocker, C., Stagos, D., and Vasiliou, V. (2008). Non-P450 aldehyde oxidizing enzymes: the aldehyde dehydrogenase superfamily. Expert Opin. drug metabolism and Toxicol. 4 (6), 697–720. doi:10.1517/17425255.4.6.697
Marsh, A. G., and Walker, C. W. (1995). Effect of estradiol and progesterone on c-myc expression in the sea star testis and the seasonal regulation of spermatogenesis. Mol. Reprod. Dev. 40 (1), 62–68. doi:10.1002/mrd.1080400109
Mashanov, V., Zueva, O., Mashanova, D., and García-Arrarás, J. E. (2017a). Expression of stem cell factors in the adult sea cucumber digestive tube. Cell tissue Res. 370 (3), 427–440. doi:10.1007/s00441-017-2692-y
Mashanov, V. S., Zueva, O. R., and García-Arrarás, J. E. (2015). Expression of pluripotency factors in echinoderm regeneration. Cell tissue Res. 359 (2), 521–536. doi:10.1007/s00441-014-2040-4
Mashanov, V. S., Zueva, O. R., and García-Arrarás, J. E. (2017b). Inhibition of cell proliferation does not slow down echinoderm neural regeneration. Front. zoology 14 (1), 12–19. doi:10.1186/s12983-017-0196-y
Materna, S. C., Howard-Ashby, M., Gray, R. F., and Davidson, E. H. (2006). The C2H2 zinc finger genes of Strongylocentrotus purpuratus and their expression in embryonic development. Dev. Biol. 300 (1), 108–120. doi:10.1016/j.ydbio.2006.08.032
McConnell, B. B., and Yang, V. W. (2010). Mammalian Krüppel-like factors in health and diseases. Physiol. Rev. 90 (4), 1337–1381. doi:10.1152/physrev.00058.2009
Milani, L., Ghiselli, F., Pecci, A., Maurizii, M. G., and Passamonti, M. (2015). The expression of a novel mitochondrially-encoded gene in gonadic precursors may drive paternal inheritance of mitochondria. PloS one 10 (9), e0137468. doi:10.1371/journal.pone.0137468
Milani, L., Pecci, A., Ghiselli, F., Passamonti, M., Bettini, S., Franceschini, V., et al. (2017). VASA expression suggests shared germ line dynamics in bivalve molluscs. Histochem. cell Biol. 148 (2), 157–171. doi:10.1007/s00418-017-1560-x
Milani, L., Pecci, A., Ghiselli, F., Passamonti, M., and Maurizii, M. G. (2016). Germ line dynamics in bivalve molluscs. Invertebr. Surviv. J. 13, 54–55.
Millan, J. L., and Manes, T. (1988). Seminoma-derived Nagao isozyme is encoded by a germ-cell alkaline phosphatase gene. Proc. Natl. Acad. Sci. 85 (9), 3024–3028. doi:10.1073/pnas.85.9.3024
Millane, R. C., Kanska, J., Duffy, D. J., Seoighe, C., Cunningham, S., Plickert, G., et al. (2011). Induced stem cell neoplasia in a cnidarian by ectopic expression of a POU domain transcription factor. Development 138 (12), 2429–2439. doi:10.1242/dev.064931
Miri, M. R., Zare, A., Saberzadeh, J., Baghban, N., Nabipour, I., and Tamadon, A. (2022). Anti-lung cancer marine compounds: a review. Ther. Innovation and Regul. Sci. 56 (2), 191–205. doi:10.1007/s43441-022-00375-3
Mitsui, K., Tokuzawa, Y., Itoh, H., Segawa, K., Murakami, M., Takahashi, K., et al. (2003). The homeoprotein Nanog is required for maintenance of pluripotency in mouse epiblast and ES cells. Cell 113 (5), 631–642. doi:10.1016/s0092-8674(03)00393-3
Mochizuki, K., Nishimiya-Fujisawa, C., and Fujisawa, T. (2001). Universal occurrence of the vasa-related genes among metazoans and their germline expression in Hydra. Dev. genes Evol. 211 (6), 299–308. doi:10.1007/s004270100156
Mokrina, M., Nagasawa, K., Kanamori, M., Natsuike, M., and Osada, M. (2021). Seasonal composition of immature germ cells in the Yesso scallop identified by vasa-like gene (my-vlg) and protein expression, with evidence of irregular germ cell differentiation accompanied with a high mortality event. Aquac. Rep. 19, 100613. doi:10.1016/j.aqrep.2021.100613
Molofsky, A. V., Pardal, R., Iwashita, T., Park, I.-K., Clarke, M. F., and Morrison, S. J. (2003). Bmi-1 dependence distinguishes neural stem cell self-renewal from progenitor proliferation. Nature 425 (6961), 962–967. doi:10.1038/nature02060
Moreb, J. S. (2008). Aldehyde dehydrogenase as a marker for stem cells. Curr. stem cell Res. and Ther. 3 (4), 237–246. doi:10.2174/157488808786734006
Morita, M., Futami, K., Zhang, H., Kubokawa, K., Ojima, Y., and Okamoto, N. (2009). Evolutionary analysis of amphioxus myc gene. J. Tokyo Univ. Mar. Sci. Technol., 11–16.
Morrison, S. J., Prowse, K. R., Ho, P., and Weissman, I. L. (1996). Telomerase activity in hematopoietic cells is associated with self-renewal potential. Immunity 5 (3), 207–216. doi:10.1016/s1074-7613(00)80316-7
Müller, W. A., Teo, R., and Frank, U. (2004). Totipotent migratory stem cells in a hydroid. Dev. Biol. 275 (1), 215–224. doi:10.1016/j.ydbio.2004.08.006
Nguyen, P. K., Nag, D., and Wu, J. C. (2010). Methods to assess stem cell lineage, fate and function. Adv. drug Deliv. Rev. 62 (12), 1175–1186. doi:10.1016/j.addr.2010.08.008
Nikanorova, D., Kupriashova, E., and Kostyuchenko, R. (2020). Regeneration in annelids: cell sources, tissue remodeling, and differential gene expression. Russ. J. Dev. Biol. 51, 148–161. doi:10.1134/s1062360420030042
Niwa, H., Miyazaki, J.-i., and Smith, A. G. (2000). Quantitative expression of Oct-3/4 defines differentiation, dedifferentiation or self-renewal of ES cells. Nat. Genet. 24 (4), 372–376. doi:10.1038/74199
Nuurai, P., Panasophonkul, S., Tinikul, Y., Sobhon, P., and Wanichanon, R. (2016). Spermatogenesis in the rock oyster, Saccostrea forskali (Gmelin, 1791). Tissue Cell 48 (1), 43–48. doi:10.1016/j.tice.2015.11.001
O’Connor, M. D., Kardel, M. D., Iosfina, I., Youssef, D., Lu, M., Li, M. M., et al. (2008). Alkaline phosphatase-positive colony formation is a sensitive, specific, and quantitative indicator of undifferentiated human embryonic stem cells. Stem cells 26 (5), 1109–1116. doi:10.1634/stemcells.2007-0801
Odell, I. D., and Cook, D. (2013). Immunofluorescence techniques. J. investigative dermatology 133 (1), e4. doi:10.1038/jid.2012.455
Odintsova, N. A., Yakovlev, K. V., Diachuk, V. A., and Serov, O. L. (2007). Evolutionarily conserved pluripotency genes of marine invertebrates. Tsitologiia 46 (9), 780–781.
Oka, H., and Watanabe, H. (1957). Vascular budding, a new type of budding in Botryllus. Biol. Bull. 112 (2), 225–240. doi:10.2307/1539200
Okita, K., Ichisaka, T., and Yamanaka, S. (2007). Generation of germline-competent induced pluripotent stem cells. nature 448 (7151), 313–317. doi:10.1038/nature05934
Osborne, P. J., and Miller, A. (1963). Acid and alkaline phosphatase changes associated with feeding, starvation and regeneration in planarians. Biol. Bull. 124 (3), 285–292. doi:10.2307/1539478
Owen, R., Sarkis, S., and Bodnar, A. (2007). Developmental pattern of telomerase expression in the sand scallop, Euvola ziczac. Invertebr. Biol. 126 (1), 40–45. doi:10.1111/j.1744-7410.2007.00074.x
Oyama, A., and Shimizu, T. (2007). Transient occurrence of vasa-expressing cells in nongenital segments during embryonic development in the oligochaete annelid Tubifex tubifex. Dev. genes Evol. 217, 675–690. doi:10.1007/s00427-007-0180-1
Özpolat, B. D., and Bely, A. E. (2015). Gonad establishment during asexual reproduction in the annelid Pristina leidyi. Dev. Biol. 405 (1), 123–136. doi:10.1016/j.ydbio.2015.06.001
Park, I.-k., Qian, D., Kiel, M., Becker, M. W., Pihalja, M., Weissman, I. L., et al. (2003). Bmi-1 is required for maintenance of adult self-renewing haematopoietic stem cells. Nature 423 (6937), 302–305. doi:10.1038/nature01587
Park, K. S., Shin, S. W., Choi, J.-W., and Um, S. H. (2013). Specific protein markers for stem cell cross-talk with neighboring cells in the environment. Int. J. stem cells 6 (2), 75–86. doi:10.15283/ijsc.2013.6.2.75
Patra, S. K., Chakrapani, V., Panda, R. P., Mohapatra, C., Jayasankar, P., and Barman, H. K. (2015). First evidence of molecular characterization of rohu carp Sox2 gene being expressed in proliferating spermatogonial cells. Theriogenology 84 (2), 268–276. doi:10.1016/j.theriogenology.2015.03.017
Pazhanisamy, S. (2013). Adult stem cell and embryonic stem cell markers. Mater methods 3, 200. doi:10.13070/mm.en.3.200
Pennati, R., Miglietta, M., Matsumoto, Y., Mercurio, S., Bonasoro, F., Scari, G., et al. (2019). Reverse development and stem cells in the cnidarian Turritopsis dohrnii. ISJ-Invertebrate Surviv. J. 16, 63–64.
Perrino, L. A., and Pierce, S. K. (2000). Betaine aldehyde dehydrogenase kinetics partially account for oyster population differences in glycine betaine synthesis. J. Exp. Zoology 286 (3), 238–249. doi:10.1002/(sici)1097-010x(20000215)286:3<238::aid-jez3>3.0.co;2-e
Pesce, M., Wang, X., Wolgemuth, D. J., and Schöler, H. R. (1998). Differential expression of the Oct-4 transcription factor during mouse germ cell differentiation. Mech. Dev. 71 (1-2), 89–98. doi:10.1016/s0925-4773(98)00002-1
Planques, A., Malem, J., Parapar, J., Vervoort, M., and Gazave, E. (2019). Morphological, cellular and molecular characterization of posterior regeneration in the marine annelid Platynereis dumerilii. Dev. Biol. 445 (2), 189–210. doi:10.1016/j.ydbio.2018.11.004
Plickert, G., Frank, U., and Müller, W. A. (2012). Hydractinia, a pioneering model for stem cell biology and reprogramming somatic cells to pluripotency. Int. J. Dev. Biol. 56 (6-8), 519–534. doi:10.1387/ijdb.123502gp
Ponz-Segrelles, G., Bleidorn, C., and Aguado, M. T. (2018). Expression of vasa, piwi, and nanos during gametogenesis in Typosyllis antoni (Annelida, Syllidae). Evol. and Dev. 20 (5), 132–145. doi:10.1111/ede.12263
Post, Y., and Clevers, H. (2019). Defining adult stem cell function at its simplest: the ability to replace lost cells through mitosis. Cell stem cell 25 (2), 174–183. doi:10.1016/j.stem.2019.07.002
Presnell, J. S. (2017). The evolution of the krüppel-like factor gene family and their function during embryonic development in the ctenophore Mnemiopsis leidyi. United States: University of Miami.
Prevodnik, A., Lilja, K., and Bollner, T. (2007). Benzo [a] pyrene up-regulates the expression of the proliferating cell nuclear antigen (PCNA) and multixenobiotic resistance polyglycoprotein (P-gp) in Baltic Sea blue mussels (Mytilus edulis L.). Comp. Biochem. Physiology Part C Toxicol. and Pharmacol. 145 (2), 265–274. doi:10.1016/j.cbpc.2006.12.014
Prodon, F., Hanawa, K., and Nishida, H. (2009). Actin microfilaments guide the polarized transport of nuclear pore complexes and the cytoplasmic dispersal of Vasa mRNA during GVBD in the ascidian Halocynthia roretzi. Dev. Biol. 330 (2), 377–388. doi:10.1016/j.ydbio.2009.04.006
Qu, D., Wang, G., Wang, Z., Zhou, L., Chi, W., Cong, S., et al. (2011). 5-Ethynyl-2′-deoxycytidine as a new agent for DNA labeling: detection of proliferating cells. Anal. Biochem. 417 (1), 112–121. doi:10.1016/j.ab.2011.05.037
Rabinowitz, C., and Rinkevich, B. (2011). De novo emerged stemness signatures in epithelial monolayers developed from extirpated palleal buds. Vitro Cell. and Dev. Biology-Animal 47, 26–31. doi:10.1007/s11626-010-9357-4
Rabinowitz, J. S., Chan, X. Y., Kingsley, E. P., Duan, Y., and Lambert, J. D. (2008). Nanos is required in somatic blast cell lineages in the posterior of a mollusk embryo. Curr. Biol. 18 (5), 331–336. doi:10.1016/j.cub.2008.01.055
Randolph, H. (1892). The regeneration of the tail in lumbriculus. J. Morphol. 7, 317–344. doi:10.1002/jmor.1050070304
Range, R., and Lepage, T. (2011). Maternal Oct1/2 is required for Nodal and Vg1/Univin expression during dorsal–ventral axis specification in the sea urchin embryo. Dev. Biol. 357 (2), 440–449. doi:10.1016/j.ydbio.2011.07.005
Rasekh, P., Kameli, A., Khoradmehr, A., Baghban, N., Mohebbi, G., Barmak, A., et al. (2023). Proliferative effect of aqueous extract of sea cucumber (Holothuria parva) body wall on human umbilical cord mesenchymal stromal/stem cells. Mar. Drugs 21 (5), 267. doi:10.3390/md21050267
Rebscher, N., Lidke, A. K., and Ackermann, C. F. (2012). Hidden in the crowd: primordial germ cells and somatic stem cells in the mesodermal posterior growth zone of the polychaete Platynereis dumerillii are two distinct cell populations. EvoDevo 3 (1), 9–11. doi:10.1186/2041-9139-3-9
Rebscher, N., Volk, C., Teo, R., and Plickert, G. (2008). The germ plasm component Vasa allows tracing of the interstitial stem cells in the cnidarian Hydractinia echinata. Dev. Dyn. 237 (6), 1736–1745. doi:10.1002/dvdy.21562
Rebscher, N., Zelada-González, F., Banisch, T., Raible, F., and Arendt, D. (2007). Vasa unveils a common origin of germ cells and of somatic stem cells from the posterior growth zone in the polychaete Platynereis dumerilii. Dev. Biol. 306 (2), 599–611. doi:10.1016/j.ydbio.2007.03.521
Rinkevich, B. (1999). Cell cultures from marine invertebrates: obstacles, new approaches and recent improvements. J. Biotechnol. 70 (1-3), 133–153. doi:10.1016/s0079-6352(99)80107-6
Rinkevich, B., Ballarin, L., Martinez, P., Somorjai, I., Ben-Hamo, O., Borisenko, I., et al. (2022). A pan-metazoan concept for adult stem cells: the wobbling Penrose landscape. Biol. Rev. 97 (1), 299–325. doi:10.1111/brv.12801
Rinkevich, Y., Douek, J., Haber, O., Rinkevich, B., and Reshef, R. (2007). Urochordate whole body regeneration inaugurates a diverse innate immune signaling profile. Dev. Biol. 312 (1), 131–146. doi:10.1016/j.ydbio.2007.09.005
Rinkevich, Y., Matranga, V., and Rinkevich, B. (2009) “Stem cells in aquatic invertebrates: common premises and emerging unique themes,” in Stem cells in marine organisms. Springer, 61–103.
Rinkevich, Y., Rinkevich, B., and Reshef, R. (2008). Cell signaling and transcription factor genes expressed during whole body regeneration in a colonial chordate. BMC Dev. Biol. 8 (1), 100–111. doi:10.1186/1471-213X-8-100
Rinkevich, Y., Rosner, A., Rabinowitz, C., Lapidot, Z., Moiseeva, E., and Rinkevich, B. (2010). Piwi positive cells that line the vasculature epithelium, underlie whole body regeneration in a basal chordate. Dev. Biol. 345 (1), 94–104. doi:10.1016/j.ydbio.2010.05.500
Rodda, D. J., Chew, J.-L., Lim, L.-H., Loh, Y.-H., Wang, B., Ng, H.-H., et al. (2005). Transcriptional regulation of nanog by OCT4 and SOX2. J. Biol. Chem. 280 (26), 24731–24737. doi:10.1074/jbc.M502573200
Rosenfield, A., Kern, F., and Keller, B. (1994). “Invertebrate neoplasia: initiation and promotion mechanisms,” in NOAA technical memorandum NMFS-NE (Washington, United States: NMFS Scientific Publications Office (SPO) in Seattle), 107.
Rosner, A., Moiseeva, E., Rinkevich, Y., Lapidot, Z., and Rinkevich, B. (2009). Vasa and the germ line lineage in a colonial urochordate. Dev. Biol. 331 (2), 113–128. doi:10.1016/j.ydbio.2009.04.025
Sano, N., Kimata, S., Obata, M., and Komaru, A. (2015). Distribution and migration of immature germ cells in the pearl oyster Pinctada fucata with the expression pattern of the vasa ortholog by in situ hybridization. J. Shellfish Res. 34 (3), 803–809. doi:10.2983/035.034.0309
Schachtele, S., Clouser, C., and Aho, J. (2020). Markers and methods to verify mesenchymal stem cell identity, potency, and quality.
Schärer, L., Ladurner, P., and Rieger, R. M. (2004). Bigger testes do work more: experimental evidence that testis size reflects testicular cell proliferation activity in the marine invertebrate, the free-living flatworm Macrostomum sp. Behav. Ecol. Sociobiol. 56 (5), 420–425. doi:10.1007/s00265-004-0802-9
Schmitt, C. A. (2003). Senescence, apoptosis and therapy—cutting the lifelines of cancer. Nat. Rev. Cancer 3 (4), 286–295. doi:10.1038/nrc1044
Schumpert, C., Nelson, J., Kim, E., Dudycha, J. L., and Patel, R. C. (2015). Telomerase activity and telomere length in Daphnia. PloS one 10 (5), e0127196. doi:10.1371/journal.pone.0127196
Seaver, E. C., and de Jong, D. M. (2021). Regeneration in the segmented annelid Capitella teleta. Genes 12 (11), 1769. doi:10.3390/genes12111769
Seipel, K., Yanze, N., and Schmid, V. (2003). The germ line and somatic stem cell gene Cniwi in the jellyfish Podocoryne carnea. Int. J. Dev. Biol. 48 (1), 1–7. doi:10.1387/ijdb.15005568
Shamsian, S., Nabipour, I., Mohebbi, G., Baghban, N., Zare, M., Zandi, K., et al. (2024). In-vitro and in-silico anti-HSV-1 activity of a marine steroid from the jellyfish Cassiopea andromeda venom. Microb. Pathog. 186, 106486. doi:10.1016/j.micpath.2023.106486
Shibata, N., Umesono, Y., Orii, H., Sakurai, T., Watanabe, K., and Agata, K. (1999). Expression ofvasa (vas)-Related genes in germline cells and totipotent somatic stem cells of planarians. Dev. Biol. 206 (1), 73–87. doi:10.1006/dbio.1998.9130
Shikina, S., Chen, C.-J., Liou, J.-Y., Shao, Z.-F., Chung, Y.-J., Lee, Y.-H., et al. (2012). Germ cell development in the scleractinian coral Euphyllia ancora (Cnidaria, Anthozoa). PLoS One 7, e41569. doi:10.1371/journal.pone.0041569
Shikina, S., Chung, Y.-J., Wang, H.-M., Chiu, Y.-L., Shao, Z.-F., Lee, Y.-H., et al. (2015). Localization of early germ cells in a stony coral, Euphyllia ancora: potential implications for a germline stem cell system in coral gametogenesis. Coral Reefs 34, 639–653. doi:10.1007/s00338-015-1270-6
Shimomura, O., Johnson, F. H., and Saiga, Y. (1962). Extraction, purification and properties of aequorin, a bioluminescent protein from the luminous hydromedusan, Aequorea. J. Cell. Comp. physiology 59 (3), 223–239. doi:10.1002/jcp.1030590302
Shukalyuk, A., Isaeva, V., Kizilova, E., and Baiborodin, S. (2005). Stem cells in the reproductive strategy of colonial rhizocephalan crustaceans (Crustacea: cirripedia: Rhizocephala). Invertebr. Reproduction and Dev. 48 (1-3), 41–53. doi:10.1080/07924259.2005.9652169
Shukalyuk, A. I., Golovnina, K. A., Baiborodin, S. I., Gunbin, K. V., Blinov, A. G., and Isaeva, V. V. (2007). "vasa-related genes and their expression in stem cells of colonial parasitic rhizocephalan barnacle Polyascus polygenea (Arthropoda: Crustacea: cirripedia: Rhizocephala). Cell Biol. Int. 31 (2), 97–108. doi:10.1016/j.cellbi.2006.09.012
Siddique, H. R., and Saleem, M. (2012). Role of BMI1, a stem cell factor, in cancer recurrence and chemoresistance: preclinical and clinical evidences. Stem cells 30 (3), 372–378. doi:10.1002/stem.1035
Siebert, S., Goetz, F. E., Church, S. H., Bhattacharyya, P., Zapata, F., Haddock, S. H., et al. (2015). Stem cells in Nanomia bijuga (Siphonophora), a colonial animal with localized growth zones. EvoDevo 6, 22–19. doi:10.1186/s13227-015-0018-2
Simeó, C. G., Andree, K. B., and Rotllant, G. (2011). Identification of vasa, a potential marker of primordial germ cells in the spider crab Maja brachydactyla, and its expression during early post-embryonic development. Invertebr. Reproduction and Dev. 55 (2), 91–99. doi:10.1080/07924259.2011.553406
Sköld, H. N., Obst, M., Sköld, M., and Åkesson, B. (2009). “Stem cells in asexual reproduction of marine invertebrates,” in Stem cells in marine organisms (Springer), 105–137.
Sköld, H. N., Stach, T., Bishop, J. D., Herbst, E., and Thorndyke, M. C. (2011). Pattern of cell proliferation during budding in the colonial ascidian Diplosoma listerianum. Biol. Bull. 221 (1), 126–136. doi:10.1086/BBLv221n1p126
Solana, J., Kao, D., Mihaylova, Y., Jaber-Hijazi, F., Malla, S., Wilson, R., et al. (2012). Defining the molecular profile of planarian pluripotent stem cells using a combinatorial RNA-seq, RNA interference and irradiation approach. Genome Biol. 13 (3), R19–R23. doi:10.1186/gb-2012-13-3-r19
Storms, R. W., Trujillo, A. P., Springer, J. B., Shah, L., Colvin, O. M., Ludeman, S. M., et al. (1999). Isolation of primitive human hematopoietic progenitors on the basis of aldehyde dehydrogenase activity. Proc. Natl. Acad. Sci. 96 (16), 9118–9123. doi:10.1073/pnas.96.16.9118
Sun, L., Song, Y., Qu, Y., Yu, X., and Zhang, W. (2007). Purification and in vitro cultivation of archaeocytes (stem cells) of the marine sponge Hymeniacidon perleve (Demospongiae). Cell tissue Res. 328 (1), 223–237. doi:10.1007/s00441-006-0342-x
Sun, X., Li, L., Wu, B., Ge, J., Zheng, Y., Yu, T., et al. (2021). Cell type diversity in scallop adductor muscles revealed by single-cell RNA-Seq. Genomics 113 (6), 3582–3598. doi:10.1016/j.ygeno.2021.08.015
Szabo, R., and Ferrier, D. E. K. (2014). The dynamics of alkaline phosphatase activity during operculum regeneration in the polychaete Pomatoceros lamarckii. Int. J. Dev. Biol. 58 (6-8), 635–642. doi:10.1387/ijdb.140116df
Taichman, R. S., Wang, Z., Shiozawa, Y., Jung, Y., Song, J., Balduino, A., et al. (2010). Prospective identification and skeletal localization of cells capable of multilineage differentiation in vivo. Stem Cells Dev. 19 (10), 1557–1570. doi:10.1089/scd.2009.0445
Takahashi, K., Tanabe, K., Ohnuki, M., Narita, M., Ichisaka, T., Tomoda, K., et al. (2007). Induction of pluripotent stem cells from adult human fibroblasts by defined factors. cell 131 (5), 861–872. doi:10.1016/j.cell.2007.11.019
Takeo, M., Yoshida-Noro, C., and Tochinai, S. (2009). Functional analysis of grimp, a novel gene required for mesodermal cell proliferation at an initial stage of regeneration in Enchytraeus japonensis (Enchytraeidae, Oligochaete). Int. J. Dev. Biol. 54 (1), 151–160. doi:10.1387/ijdb.082790mt
Tan, E. S., Izumi, R., Takeuchi, Y., Isomura, N., and Takemura, A. (2020). Molecular approaches underlying the oogenic cycle of the scleractinian coral, Acropora tenuis. Sci. Rep. 10 (1), 9914–9916. doi:10.1038/s41598-020-66020-x
Taupin, P. (2007). BrdU immunohistochemistry for studying adult neurogenesis: paradigms, pitfalls, limitations, and validation. Brain Res. Rev. 53 (1), 198–214. doi:10.1016/j.brainresrev.2006.08.002
Thomson, T., and Lin, H. (2009). The biogenesis and function of PIWI proteins and piRNAs: progress and prospect. Annu. Rev. Cell Dev. 25, 355–376. doi:10.1146/annurev.cellbio.24.110707.175327
Traut, W., Szczepanowski, M., Vítková, M., Opitz, C., Marec, F., and Zrzavý, J. (2007). The telomere repeat motif of basal Metazoa. Chromosome Res. 15, 371–382. doi:10.1007/s10577-007-1132-3
Vanni, V., Salonna, M., Gasparini, F., Martini, M., Anselmi, C., Gissi, C., et al. (2022). Yamanaka factors in the budding tunicate Botryllus schlosseri show a shared spatio-temporal expression pattern in chordates. Front. Cell Dev. Biol. 10, 782722. doi:10.3389/fcell.2022.782722
Varghese, M., Olstorn, H., Berg-Johnsen, J., Moe, M. C., Murrell, W., and Langmoen, I. A. (2009). Isolation of human multipotent neural progenitors from adult filum terminale. Stem cells Dev. 18 (4), 603–613. doi:10.1089/scd.2008.0144
Vasiliou, V., Thompson, D. C., Smith, C., Fujita, M., and Chen, Y. (2013). Aldehyde dehydrogenases: from eye crystallins to metabolic disease and cancer stem cells. Chemico-biological Interact. 202 (1-3), 2–10. doi:10.1016/j.cbi.2012.10.026
Vassalli, G. (2019). Aldehyde dehydrogenases: not just markers, but functional regulators of stem cells. Stem cells Int. 2019, 3904645. doi:10.1155/2019/3904645
Vickers, N. J. (2017). Animal communication: when i’m calling you, will you answer too? Curr. Biol. 27 (14), R713–R715. doi:10.1016/j.cub.2017.05.064
Wang, G., and Reinke, V. (2008). A C. elegans Piwi, PRG-1, regulates 21U-RNAs during spermatogenesis. Curr. Biol. 18 (12), 861–867. doi:10.1016/j.cub.2008.05.009
Wang, M., Xia, J., Jawad, M., Wei, W., Gui, L., Liang, X., et al. (2022). Transcriptome sequencing analysis of sex-related genes and miRNAs in the gonads of Mytilus coruscus. Front. Mar. Sci. 9. doi:10.3389/fmars.2022.1013857
Wang, Y., Wang, B., Shao, X., Liu, M., Jiang, K., Wang, M., et al. (2020). A comparative transcriptomic analysis in late embryogenesis of the red claw crayfish Cherax quadricarinatus. Mol. Genet. Genomics 295, 299–311. doi:10.1007/s00438-019-01621-4
Wang, Y., Zayas, R. M., Guo, T., and Newmark, P. A. (2007). Nanos function is essential for development and regeneration of planarian germ cells. Proc. Natl. Acad. Sci. 104 (14), 5901–5906. doi:10.1073/pnas.0609708104
Wang, Z., Gao, J., Song, H., Wu, X., Sun, Y., Qi, J., et al. (2014). Sexually dimorphic expression of vasa isoforms in the tongue sole (Cynoglossus semilaevis). PloS one 9 (3), e93380. doi:10.1371/journal.pone.0093380
Watanabe, H., Mättner, R., and Holstein, T. W. (2009). “Immortality and the base of multicellular life: lessons from cnidarian stem cells,” in Seminars in cell and developmental biology (Elsevier).
Wei, Z., Angerer, R. C., and Angerer, L. M. (2011). Direct development of neurons within foregut endoderm of sea urchin embryos. Proc. Natl. Acad. Sci. 108 (22), 9143–9147. doi:10.1073/pnas.1018513108
Weigert, A., Helm, C., Hausen, H., Zakrzewski, A.-C., and Bleidorn, C. (2013). Expression pattern of Piwi-like genes in adult Myzostoma cirriferum (Annelida). Dev. genes Evol. 223, 329–334. doi:10.1007/s00427-013-0444-x
Wlodkowic, D., Skommer, J., Akagi, J., Fujimura, Y., and Takeda, K. (2013). Multiparameter analysis of apoptosis using lab-on-a-chip flow cytometry. Curr. Protoc. Cytom. 66 (1), 9.42.1–9.42.15. doi:10.1002/0471142956.cy0942s66
Wlodkowic, D., Skommer, J., and Darzynkiewicz, Z. (2011). “Rapid quantification of cell viability and apoptosis in B-cell lymphoma cultures using cyanine SYTO probes,” in Mammalian cell viability (Springer), 81–89.
Wu, F., Wu, Q., Li, D., Zhang, Y., Wang, R., Liu, Y., et al. (2018). Oct4 regulates DNA methyltransferase 1 transcription by direct binding of the regulatory element. Cell. and Mol. Biol. Lett. 23, 39–13. doi:10.1186/s11658-018-0104-2
Xia, X., Guan, C., Chen, J., Qiu, M., Qi, J., Wei, M., et al. (2020). Molecular characterization of AwSox2 from bivalve Anodonta woodiana: elucidating its player in the immune response. Innate Immun. 26 (5), 381–397. doi:10.1177/1753425919897823
Xu, C.-M., and Sun, S.-C. (2020). Expression of piwi genes during the regeneration of lineus sanguineus (nemertea, pilidiophora, heteronemertea). Genes 11 (12), 1484. doi:10.3390/genes11121484
Xu, Y., Zhang, J.-a., Xu, Y., Guo, S.-l., Wang, S., Wu, D., et al. (2015). Antiphotoaging effect of conditioned medium of dedifferentiated adipocytes on skin in vivo and in vitro: a mechanistic study. Stem Cells Dev. 24 (9), 1096–1111. doi:10.1089/scd.2014.0321
Yakovlev, K. V., Battulin, N. R., Serov, O. L., and Odintsova, N. A. (2010). Isolation of oogonia from ovaries of the sea urchin Strongylocentrotus nudus. Cell Tissue Res. 342 (3), 479–490. doi:10.1007/s00441-010-1074-5
Yoshida-Noro, C., and Tochinai, S. (2010). Stem cell system in asexual and sexual reproduction of Enchytraeus japonensis (Oligochaeta, Annelida). Dev. growth and Differ. 52 (1), 43–55. doi:10.1111/j.1440-169X.2009.01149.x
Yoshizaki, G., Sakatani, S., Tominaga, H., and Takeuchi, T. (2000). Cloning and characterization of a vasa-like gene in rainbow trout and its expression in the germ cell lineage. Mol. Reproduction Dev. Incorporating Gamete Res. 55 (4), 364–371. doi:10.1002/(SICI)1098-2795(200004)55:4<364::AID-MRD2>3.0.CO;2-8
Yu, S., Qiao, X., Song, X., Yang, Y., Zhang, D., Sun, W., et al. (2022). The proliferating cell nuclear antigen (PCNA) is a potential proliferative marker in oyster Crassostrea gigas. Fish and Shellfish Immunol. 122, 306–315. doi:10.1016/j.fsi.2022.02.018
Yuh, C.-H., Bolouri, H., and Davidson, E. H. (1998). Genomic cis-regulatory logic: experimental and computational analysis of a sea urchin gene. Science 279 (5358), 1896–1902. doi:10.1126/science.279.5358.1896
Zare, A., Afshar, A., Khoradmehr, A., Baghban, N., Mohebbi, G., Barmak, A., et al. (2023). Chemical compositions and experimental and computational modeling of the anticancer effects of cnidocyte venoms of jellyfish cassiopea andromeda and catostylus mosaicus on human adenocarcinoma A549 cells. Mar. Drugs 21 (3), 168. doi:10.3390/md21030168
Zattara, E. E., and Bely, A. E. (2013). Investment choices in post-embryonic development: quantifying interactions among growth, regeneration, and asexual reproduction in the annelid Pristina leidyi. J. Exp. Zoology Part B Mol. Dev. Evol. 320 (8), 471–488. doi:10.1002/jez.b.22523
Zavalnaya, E., Shamshurina, E., and Eliseikina, M. (2020). The Immunocytochemical Identification of PIWI-positive cells during the recovery of a coelomocyte population after evisceration in the holothurian Eupentacta fraudatrix (Djakonov et Baranova, 1958) (Holothuroidea: Dendrochirota). Russ. J. Mar. Biol. 46, 97–104. doi:10.1134/s106307402002011x
Zelada González, Y. F. (2005). Germline development in Platynereis dumerilii and its connection to embryonic patterning.
Zhan, M. (2008). Genomic studies to explore self-renewal and differentiation properties of embryonic stem cells. Front. Biosci. 13 (13), 276–283. doi:10.2741/2678
Zhang, Y., Wang, J., Lv, M., Gao, H., Meng, L., Yunga, A., et al. (2021). Diversity, function and evolution of marine invertebrate genomes. bioRxiv: 2021.2010. 2031.465852.
Zheng, M., Zueva, O., and Hinman, V. F. (2022). Regeneration of the larval sea star nervous system by wounding induced respecification to the Sox2 lineage. eLife 11, e72983. doi:10.7554/eLife.72983
Zhou, L., Sheng, D., Wang, D., Ma, W., Deng, Q., Deng, L., et al. (2019). Identification of cancer-type specific expression patterns for active aldehyde dehydrogenase (ALDH) isoforms in ALDEFLUOR assay. Cell Biol. Toxicol. 35 (2), 161–177. doi:10.1007/s10565-018-9444-y
Zhou, Q., Shao, M., Qin, Z., Kyoung, H. K., and Zhang, Z. (2010). Cloning, characterization, and expression analysis of the DEAD-box family genes, Fc-vasa and Fc-PL10a, in Chinese shrimp (Fenneropenaeus chinensis). Chin. J. Oceanol. Limnol. 28 (1), 37–45. doi:10.1007/s00343-010-9231-y
Zielke, S., and Bodnar, A. (2010). Telomeres and telomerase activity in scleractinian corals and Symbiodinium spp. Biol. Bull. 218 (2), 113–121. doi:10.1086/BBLv218n2p113
Keywords: invertebrates, stem cells, marker, marine, regeneration, reproduction
Citation: Mohajer F, Khoradmehr A, Riazalhosseini B, Zendehboudi T, Nabipour I and Baghban N (2024) In vitro detection of marine invertebrate stem cells: utilizing molecular and cellular biology techniques and exploring markers. Front. Cell Dev. Biol. 12:1440091. doi: 10.3389/fcell.2024.1440091
Received: 28 May 2024; Accepted: 07 August 2024;
Published: 22 August 2024.
Edited by:
Prasad S. Koka, Biomedical Research Institute of Southern California, United StatesReviewed by:
Olga Zueva, Marine Biological Laboratory (MBL), United StatesBirgitta Sundell-Ranby, Wayne State University, United States
Copyright © 2024 Mohajer, Khoradmehr, Riazalhosseini, Zendehboudi, Nabipour and Baghban. This is an open-access article distributed under the terms of the Creative Commons Attribution License (CC BY). The use, distribution or reproduction in other forums is permitted, provided the original author(s) and the copyright owner(s) are credited and that the original publication in this journal is cited, in accordance with accepted academic practice. No use, distribution or reproduction is permitted which does not comply with these terms.
*Correspondence: Neda Baghban, bmVkYS5iYWdoYmFuQGdtYWlsLmNvbQ==
†These authors share first authorship