- 1Department of Normal, Clinical and Imaging Anatomy, Medical University of Lublin, Lublin, Poland
- 2Students Scientific Association, Department of Normal, Clinical and Imaging Anatomy, Medical University of Lublin, Lublin, Poland
- 3Student Scientific Association, Department of Forensic Medicine, Medical University of Lublin, Lublin, Poland
- 4Department of Animal Physiology, Faculty of Veterinary Medicine, University of Life Sciences in Lublin, Lublin, Poland
- 5Occupational Therapy Laboratory, Chair of Nursing Development, Medical University of Lublin, Lublin, Poland
Stem cell research holds huge promise for regenerative medicine and disease modeling, making the understanding and optimization of stem cell culture a critical aspect of advancing these therapeutic applications. This comprehensive review provides an in-depth overview of stem cell culture, including general information, contemporary techniques, encountered problems, and future perspectives. The article begins by explaining the fundamental characteristics of various stem cell types, elucidating the importance of proper culture conditions in maintaining pluripotency or lineage commitment. A detailed exploration of established culture techniques sheds light on the evolving landscape of stem cell culture methodologies. Common challenges such as genetic stability, heterogeneity, and differentiation efficiency are thoroughly discussed, with insights into cutting-edge strategies and technologies aimed at addressing these hurdles. Moreover, the article delves into the impact of substrate materials, culture media components, and biophysical cues on stem cell behavior, emphasizing the intricate interplay between the microenvironment and cell fate decisions. As stem cell research advances, ethical considerations and regulatory frameworks become increasingly important, prompting a critical examination of these aspects in the context of culture practices. Lastly, the article explores emerging perspectives, including the integration of artificial intelligence and machine learning in optimizing culture conditions, and the potential applications of stem cell-derived products in personalized medicine. This comprehensive overview aims to serve as a valuable resource for researchers and clinicians, fostering a deeper understanding of stem cell culture and its key role in advancing regenerative medicine and biomedical research.
Introduction
In the dynamic field of stem cell research, the evolution of culture methodologies plays a pivotal role in unlocking the vast therapeutic and scientific potential inherent in these remarkable cells. This article provides a comprehensive overview, synthesizing key findings from seminal studies to offer a nuanced exploration of general information, techniques, persistent challenges, and future perspectives in the domain of stem cell culture. The past decade has witnessed groundbreaking discoveries that have reshaped our understanding of stem cells (Chen et al., 2014). The work of Takahashi and Yamanaka (Takahashi and Yamanaka, 2013) introduced induced pluripotent stem cells (iPSCs), a transformative concept that facilitated the reprogramming of somatic cells into a pluripotent state, bypassing ethical concerns associated with embryonic stem cells (Aboul-Soud et al., 2021; Yamanaka, 2020; Karagiannis et al., 2019; Liu et al., 2020). Concurrently, the investigations furthered our understanding of human embryonic stem cells (hESCs), contributing to the establishment of robust culture protocols for these cells (Kobold et al., 2023). Advancements in stem cell culture techniques have been instrumental in harnessing the full potential of these cells for therapeutic applications. Research by Chen et al. (2019) emphasized the importance of three-dimensional (3D) culture systems, demonstrating their superiority in maintaining stem cell pluripotency and enhancing differentiation potential compared to traditional two-dimensional (2D) approaches (Ylostalo, 2020). A comparison of 2D and 3D cultures can be found in Table 1. Additionally, the recent article of Yu et al. (2023) showcased the integration of microfluidic platforms to precisely control the stem cell microenvironment, influencing cellular responses in unprecedented ways. However, the translation of stem cell research into clinical applications is not without its challenges (McKee and Chaudhry, 2017). There is the persistent issue of genetic instability in long-term cultures, necessitating a nuanced approach to mitigate risks associated with genomic alterations (Prieto Gonzalez, 2022). Furthermore, the intrinsic heterogeneity within stem cell populations is a major hurdle, demanding innovative strategies to enhance the homogeneity of cell populations for therapeutic efficacy (Mas-Bargues and Borrás, 2021; Wang et al., 2021a). Looking ahead, the article explores emerging perspectives and potential solutions to current challenges. Recent investigations showcase the integration of artificial intelligence (AI) and machine learning in optimizing stem cell culture conditions, promising data-driven insights for enhanced reproducibility and efficiency (Nosrati and Nosrati, 2023). Additionally, Li et al. (2018) discusses the transformative potential of stem cell-derived products in personalized medicine, providing a glimpse into a future where tailored therapeutic interventions are based on individual patient profiles (Li et al., 2018). This review aims to consolidate the wealth of knowledge offering a comprehensive and up-to-date exploration of stem cell culture to optimize methodologies, propelling the field towards its full potential in biomedical research.
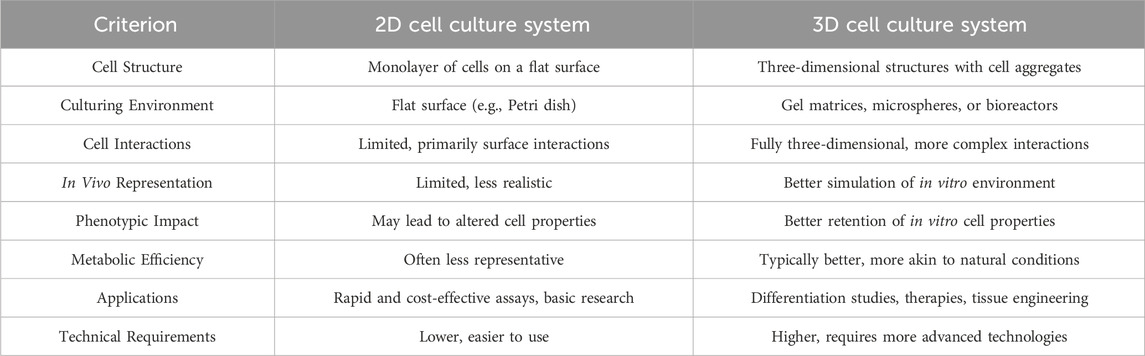
Table 1. Comparison of 2D and 3D cultures (Raitanen et al., 2023; Souza et al., 2018; Ylostalo, 2020; Baharvand et al., 2006).
General information about stem cells
In the context of developmental ontogenesis, stem cells (SCs) can be broadly classified into two principal categories: embryonic stem cells (ESCs), isolated from the inner cell mass of blastocysts, and adult stem cells (ASCs), colloquially referred to as somatic stem cells (SSCs), distributed across various adult tissues (Slack, 2018; Gao et al., 2018). Embryonic stem cells (ESCs), characterized by their self-renewal capacity, embody pluripotency, originating from the inner cell mass (ICM) of developing blastocysts. Pluripotency signifies the singular cell’s capability to engender all cellular lineages throughout both embryonic and adult organismal development. Tissue-specific stem cells orchestrate the dynamic turnover of distinct tissue phenotypes in mammals and other metazoans (Young, 2011; Christopher and Baker, 2018). Their ubiquitous presence spans a diverse array of tissues, including but not limited to bone marrow (BMSCs), adipose tissue (ADSCs), dental pulp (DPSCs), blood (HSCs), amniotic fluid (AFSCs), umbilical cord (UCSCs), and a sundry of other tissues. Despite sharing a vernacular nomenclature, these classifications reveal myriad distinctions (Zeyu et al., 2023; Orticelli et al., 2021).
Segregated according to their differentiative potential, stem cells delineate into totipotent SCs, pluripotent SCs (PSCs), multipotent SCs, and unipotent SCs (Gao et al., 2018). Totipotent cells, represented by the zygote and early blastomeres, possess the exceptional ability to give rise to all cellular lineages, playing a central role in initiating organismal development (Maemura et al., 2021). Conversely, pluripotent cells, found in the inner cell mass of blastocysts, exhibit a broad capacity to generate all cell types, except those specific to the extraembryonic trophoblast lineage. This distinction underscores the critical roles these cell types play in the early stages of life, with totipotent cells guiding the foundational steps of complete organism formation, and pluripotent cells contributing to the differentiation of diverse cell types crucial for embryonic development (Cai et al., 2022; Malik and Wang, 2022; Sobhani et al., 2017). Multipotent stem cells manifest the ability to differentiate into all cell types from a single germ layer, while unipotent stem cells are defined by their competence to differentiate solely into one lineage (Kolios and Moodley, 2013). Oligopotent stem cells, distinguished by their unique capacity for self-renewal, demonstrate the remarkable ability to initiate and sustain two or more distinct lineages within a specific tissue. An exemplary illustration of oligopotent stem cells can be observed in hematopoietic stem cells, known for their versatility in differentiating into both myeloid and lymphoid lineages, thereby contributing to the diverse cellular composition of the blood (Cheng et al., 2020).
In the context of pulmonary biology, ongoing investigations propose a fascinating possibility: bronchoalveolar duct junction cells, identified as oligopotent, may serve as the originators of both bronchiolar epithelium and alveolar epithelium. This intriguing finding underscores the dynamic nature of oligopotent stem cells in orchestrating tissue-specific regeneration and underscores their role in maintaining the intricate cellular architecture of the respiratory system (Ilic and Polak, 2011).
The phenomenon of continuous regeneration serves as an intrinsic process orchestrated by stem cells, playing a pivotal role in maintaining homeostasis within multicellular organisms. Senescent, functionally specialized mature cells within adult organs are perpetually supplanted by newly generated cells (Lee and Hong, 2020). This cellular turnover process is notably heightened within the hematopoietic system, intestinal epithelium, or epidermis, in stark contrast to the more measured pace observed in skeletal muscles, liver, or cardiac tissues (Ratajczak et al., 2012). Tissues characterized by a high rate of cellular turnover, such as blood and gut epithelium, harbor populations of incessantly proliferating adult stem cells and progenitor cells, adept at generating differentiating progeny. Additionally, many tissues maintain populations of stem cells in a quiescent state, either to provide support for cycling progenitors or to serve as a reserve for potential tissue injuries (de Morree and Rando, 2023). A pivotal prerequisite for a stem cell is the demonstration of clonality and the capacity to generate a myriad of functional cells. The ‘self-renewing’ attributes of a stem cell may manifest through symmetrical division, yielding exclusively either stem cells or all progenitor cells, or through asymmetrical division, capable of producing both stem cells and progenitor cells (Sanders et al., 2006).
Sources of stem cells
In the realm of regenerative medicine and cellular therapeutics, stem cells are procured from various biological reservoirs, encompassing four fundamental sources. These encompass (1) embryonic tissues, (2) fetal tissues such as the fetus, placental components (amnion and chorion), amniotic fluid, and umbilical cord structures (Wharton’s jelly and blood), (3) specific niches within the adult organism, notably adipose tissue, bone marrow, skeletal muscle, and blood, and (4) differentiated somatic cells subjected to genetic reprogramming, exemplified by induced pluripotent stem cells (iPSCs) (Figure 1.) (Bacakova et al., 2018; Mushahary et al., 2018; Lovell-Badge et al., 2021). Historically, bone marrow (BM) has stood as the preeminent reservoir of mesenchymal stem cells (MSCs) in the human context. Despite its richness in hematopoietic stem cells, BM harbors only a scant population of MSCs. Moreover, the arduous and anesthesia-requiring BM harvesting procedure has restricted the utilization of BM-derived MSCs (bmMSCs) in both investigative and clinical settings. Presently, MSCs are isolatable from diverse tissue sources, including dental tissues, integumentary structures, salivary glands, limb buds, and menstrual blood (Rodríguez-Fuentes et al., 2021). Adipose tissue (AT) emerges as a salient source of MSCs, characterized by its ubiquity and accessibility, rendering adipose-derived MSCs (adMSCs) pivotal candidates for autologous and allogeneic stem cell-based therapies and tissue engineering (Palumbo et al., 2018). In contradistinction, perinatal tissues, such as the amnion, chorion, and umbilical cord (UC), represent promising repositories of MSCs, given the advantageous attributes conferred by the youthfulness of the donors. While MSC derivation from various perinatal tissues is feasible, UC tissue, specifically Wharton’s jelly, emerges as a superior MSC reservoir when compared to umbilical cord blood (UCB) (Ferrúa et al., 2017; Chu et al., 2020).
The acquisition of stem cells is predicated upon two principal methodologies: enzymatic isolation and explant culturing. Enzymatic isolation procedures are contingent upon the source of MSCs, necessitating tailored techniques, specific media formulations, serum constituents, and cell surface markers (Bacakova et al., 2018; Mushahary et al., 2018). In the explant method, devoid of enzymatic intervention, the original tissue is sectioned into smaller fragments, subsequently arranged in culture dishes or flasks. After this, cells commence migration from the tissue fragments, adhering to the culture surface (Hendijani, 2017). The explant methodology is notable for its proclivity to generate cell populations characterized by reduced heterogeneity, resulting in augmented proliferation rates and enhanced cell viability, as compared to the enzymatic approach (Chu et al., 2020). This inclination is likely attributable to the presence of integral tissue fragments and undissociated extracellular matrix (ECM) during the explant culture process. These components contribute to the establishment of a protective milieu, mitigating the impact of proteolytic and mechanical stressors and thereby creating an environment conducive to the migration of cells (Sajeesh et al., 2020). While enzymatic digestion facilitates the isolation of fibroblast-like (stem) cells and the concomitant liberation of endothelial cells and pericytes, the explant culture paradigm offers additional advantages. These include the release of cytokines and growth factors into the culture medium, an augmented yield of stromal cells, a truncated proliferation timeframe, and the simultaneous expression of surface markers CD73, CD90, and CD105, coupled with the conspicuous absence of CD14, CD31, CD34, and CD45 in all MSC populations (Lu et al., 2021). Quantitative assessments predicated on nucleated cell numbers per unit tissue weight underscore that the explant culture methodology confers a superior yield of stromal cells in comparison to the enzymatic method. This observed advantage may stem from diminished cellular adhesion following exposure to enzymatic treatment and the concomitant loss of cells during the sequential procedures of filtration and washing inherent in the enzymatic methodology (Priya et al., 2014). The amalgamation of these methodologies underscores a nuanced approach to establishing reliable, robust, and standardized MSC isolation protocols, exemplifying the potential of UC and AT as highly relevant and promising tissue sources for MSCs (Mushahary et al., 2018).
Recent improvements in culturing hematopoietic stem cells (HSCs) focus on enhancing their ex vivo expansion and maintaining their functionality over extended periods. Advances include the development of fully defined, albumin-free culture systems that eliminate the variability of traditional supplements like bovine serum albumin. These new methods allow for substantial expansion of HSCs, with fold increases reaching several hundred times over a month. Improved culture conditions also support clonal analysis of HSC heterogeneity and enable transplantation into nonconditioned recipients, offering new potential for research and therapeutic applications in hematopoiesis and immune system studies (Wilkinson et al., 2020).
Current improvements in culturing mesenchymal stromal cells (MSCs) have focused on optimizing growth conditions to enhance their expansion, viability, and therapeutic potential. Key advancements include the use of serum-free or xeno-free media, which eliminates animal-derived components and reduces variability in culture outcomes. Newer bioreactor systems and 3D culture methods have also been developed, enabling more efficient large-scale expansion while preserving MSC functionality. Additionally, refined protocols for maintaining MSC stemness, differentiation potential, and immunomodulatory properties have contributed to their increased use in regenerative medicine and cell-based therapies (Todtenhaupt et al., 2023).
The employment of stem cell culture in therapy and science (cell lines)
The growing interest in culturing stem cells excited many researchers all around the world to better understand the processes occurring in the cultures development and to predict the overall possibilities of these cells’ usage. Throughout recent years, many milestones have been reached in this field, and the use of stem cells cultures continues to grow and pins researchers’ faith on applying it in more treatment tactics and scientific research. Many research works are conducted using rodent stem cells (especially mouse ones), and the outcomes of these works suggest that cultured stem cells can help in the treatment of joints diseases (e.g., rheumatoid arthritis), peritonitis, colitis, and many more (Yen et al., 2023; Lan et al., 2021; Marega et al., 2023; Zaripova et al., 2023).
Therapeutic properties of stem cell cultures can be helpful for various specialists. Dierick et al. (2021) points out that multipotent stem cells (MSC) are currently under consideration as a possible treatment of pulmonary hypertension (Dierick et al., 2021). Jiang and Tuan (2023) in his work describes various types of stem cells used in creating a cartilage-like tissue in human joints, which improves the quality of life of patients affected with arthropathies (Jiang and Tuan, 2023; Giorgino et al., 2023; Hwang et al., 2021). One of the yet-to-overcome challenge remains employing stem cells to repair infarcted cardiac tissue. Cardiovascular diseases remain one of the most common deaths causes all around the world, and the possibility of heart regeneration is very promising (Parrotta et al., 2019; Campostrini et al., 2021; Barreto et al., 2019). The researchers are also examining the usage of cell lines, which are induced into male germ-like cells, in the treatment of male infertility (Malekmohamadi et al., 2019). Chen et al. (2020) in his work presents the idea of forming a healthy liver, using 3D stem cells, which would be transplanted to patients suffering from end-stage liver diseases, such as liver cancer (Chen et al., 2020). Scientists also utilize stem cells in the process of understanding mechanisms that occur in tumors, such as glioblastoma (Nikitin et al., 2023) and more.
It is worth underlining that the increasing knowledge regarding stem cell culture and its application in the modern therapeutic approaches is possible due to discovering new technologies, better understanding the processes taking place in stem cells, and the growing interest in molecular science. Apart from medicine, stem cells also find use in other sciences, such as ecology. Xie et al. (2020) in his work points out that obtaining these cells from various endangered fish species can help preserving them in the environment (Xie et al., 2020).
The methods of culturing stem cells
The process of developing a stem cells culture has been improving and changing for several years. In 2006, the Japanese researchers, Takahashi and Yamanaka (2006), discovered how to turn a multipotent stem cell into a pluripotent one (Takahashi and Yamanaka, 2006). This has been one of the milestones in the stem cells culturing, as it enabled using these cells in human therapy, as the cells obtained in this process are biocompatible with the receiver.
There are several ways to culture stem cells, depending on the source of the cells themselves. In this work, there will be highlighted two methods of culturing stem cells (Figure 2.). Human embryonic stem cells (hESCs), the first type of stem cells, can be obtained from the preimplantation embryos, more precisely-the blastocyst (Zakrzewski et al., 2019). First, the hESCs need to be separated from the blastocyst (which can be done in the early stages of embryo differentiation, as it is safer for the embryo, or in vitro). There are several methods to perform this procedure. The simplest one is the manual separation. The other possibilities consist of using enzymes’ inhibitors, such as checkpoint kinase-1 (CHK1) inhibitor (Ware et al., 2023), trypsin or ethylenediaminetetraacetic acid (EDTA) (Zakrzewski et al., 2019). After the separation is done, the obtained cells need to be placed in a special environment, containing nutrients and growth factors, delivered daily. This mixture consists of such substances as antibiotics, insulin, transferrin, heparin, source of sodium, fibroblast growth factor-4 (FGF-4), and many more (Zhao et al., 2023). The culture will continue to grow, and with adequate factors, can be directed into more specified cells (Zakrzewski et al., 2019).
Moreover, the microenvironment plays a critical role in stem cell behavior, particularly in regulating stem cell fate. Factors such as extracellular matrix (ECM) composition, stiffness, and mechanical forces are key in determining the differentiation pathways of stem cells. For instance, variations in ECM stiffness can direct stem cells toward specific lineages; softer matrices tend to promote neurogenic differentiation, while stiffer matrices support osteogenic differentiation. Mechanical forces like wall shear stress and circumferential strain also significantly influence stem cell fate by modulating cellular signaling pathways and gene expression. These mechanical cues, in combination with the biochemical environment, create a complex microenvironment that ultimately governs stem cell behavior and differentiation (Discher et al., 2005; Engler et al., 2006).
After the differentiation, hESCs become multipotent, meaning that they can only develop into specified germ layer (ectoderm, endoderm, mesoderm). However, the earlier-mentioned discovery of Yamanaka and Takahashi allows to undo this process and acquire a pluripotent cell from a multipotent one. These stem cells are called induced pluripotent stem cells (iPSCs).
To obtain iPSCs, mature cells, such as fibroblasts, epithelial cells, keratinocytes et cetera are drawn from the organism (Zakrzewski et al., 2019). Later, these cells need to be reprogrammed by forcing an expression of oncogenes. To test whether obtained iPSCs have sufficient reproductive properties, a specific test needs to be conducted-the teratoma formation assay (Zakrzewski et al., 2019). In this test, the iPSCs are injected into a rodent, and start to form a teratoma. Further on, the tumor is tested to determine which types of tissues has it formed. The outcome shows how active they obtained iPSCs are. Some scientists also use the teratoma formation to work on the sourced tissues, after collecting the tumor from the rodent (Lee et al., 2020).
It is necessary to mention the efforts from Dr. Raimondi’s lab to develop faithful 3D models to preserve stem cell in a more physiological context (Donnaloja et al., 2020; Steimberg et al., 2020; Rey et al., 2020; Nava et al., 2012; Boeri et al., 2019). These models aim to mimic the natural stem cell niche more accurately than traditional 2D cultures, promoting more realistic cell behavior, differentiation, and self-renewal. Advances include the use of biomimetic scaffolds, hydrogels, and organoids that replicate the physical, chemical, and mechanical properties of the in vivo environment. These 3D systems improve the maintenance of stem cell stemness and functionality, providing more reliable platforms for studying cell biology, disease modeling, and potential therapeutic applications.
There is much yet to be discovered in culturing stem cells. Each year novel methods are discovered, and the therapeutic and research properties of stem cells are amplified.
Problems and most common mistakes in cell culture management
Cell culture is a crucial technique in biological research, and proper management is essential to ensure reliable and reproducible results. Errors can occur in cell culture studies if they are not done correctly. To ensure the repeatability of in vitro research, it is crucial that cell culture investigations be carried out using good cell culture practice (GCCP) (Pamies et al., 2022). The abundance of erroneous and irreproducible results in the scientific literature is specifically caused by common problems in cell culture, such as cross-contamination between different species or within the same species, misidentification of cells, genetic drift, bacterial, fungal, yeast, viral, or chemical contamination, and the lack of strict quality control testing (Nelson-Rees and Flandermeyer, 1976). Rough estimates suggest that 16.1% of studies that were published used cell lines that had problems (Babic et al., 2019). It has been found that adding antibiotics and anti-mycotic to cell culture media helps reduce biological contamination from mycoplasma, yeast, bacterium, and fungus. These drugs usually work by preventing the formation of bacterial initiation complexes between mRNA and the bacterial ribosome (e.g., streptomycin), inhibiting the synthesis of cell walls (e.g., penicillin), or compromising membrane permeability (e.g., amphotericin B). However, prolonged usage of antibiotics may result in the sluggish growth rates of resistant or persistent bacterial pollutants, which may cause subtle alterations in cell behavior and differentiation (Llobet et al., 2015; American Type Culture Collection Standards Development Organization Workgroup ASN-0002, 2010). Therefore, to minimize bacterial contamination in cell culture, researchers should endeavor to maintain rigorous aseptic working conditions and refrain from using antibiotics in cell culture on a permanent basis (Ryu et al., 2017; Varghese et al., 2017). In cell cultures, contamination can come from chemical and biological sources. Indicators of this contamination typically include sluggish cell growth, morphological abnormalities, abrupt pH changes in the media, and higher concentrations of dead or floating cells in the culture. Regular screening for these pollutants in cell cultures is crucial to guarantee consistency in results and prevent potentially harmful consequences.
Different cell types may require specific culture vessel coatings or substrates for optimal attachment and growth. Using inappropriate surfaces can result in poor cell adhesion and viability. Primary cells can be more sensitive than established cell lines. Attention must also be paid to specific requirements, such as shorter passage intervals, specialized media, and unique culture conditions.
In biomedical research, cell culture is essential, but dependability is compromised by problems including contamination and misidentification. Authentication is aided by resources such as International Cell Line Authentication Committee (ICLAC) and required testing. Adopting best practices, training employees, and utilizing modern innovations like patient-derived organoids (PDOs) and patient-derived xenograft (PDXs) are all necessary to ensure safety. Reliance on animals is decreased by the rapid production of patient-derived cell cultures made possible by CRISPR technology. Continuous training of personnel is essential for a dependable cell culture facility.
Decellularized extracellular matrix
Decellularized extracellular matrix (dECM) derived from cells has emerged as a powerful tool in stem cell culture, offering a variety of benefits that enhance the growth, differentiation, and overall functionality of stem cells. The dECM is a scaffold composed of proteins, glycoproteins, and other components that mimic the natural extracellular environment, providing crucial signals and structural support to cultured cells. Here are the key benefits of using cell-derived dECM in stem cell culture (Wang et al., 2021b; Pei, 2017; Kim et al., 2018; Yao et al., 2019; Zhang et al., 2021):
1. Mimicking the Native Microenvironment
• Biochemical Cues: The dECM retains bioactive molecules from the original tissue, which can provide essential biochemical cues that guide stem cell behavior, including proliferation, differentiation, and migration. These cues are difficult to replicate using synthetic materials.
• Structural Support: The natural architecture of dECM offers a physical framework that closely resembles the in vivo environment, supporting proper cell attachment, organization, and growth.
2. Enhanced Stem Cell Differentiation
• Tissue-Specific Differentiation: dECM derived from specific tissues can induce lineage-specific differentiation of stem cells. For instance, cardiac dECM can promote cardiac lineage differentiation, while bone dECM can enhance osteogenic differentiation.
• Regulation of Stemness: The presence of specific ECM components can maintain the balance between stem cell self-renewal and differentiation, depending on the culture conditions and the type of dECM used.
3. Improved Cell Viability and Proliferation
• Reduced Apoptosis: The bioactive environment provided by dECM reduces cell stress and apoptosis, leading to higher cell viability during culture.
• Proliferative Support: The natural composition of dECM can stimulate cell proliferation more effectively than traditional culture substrates, allowing for the expansion of stem cell populations.
4. Immunomodulatory Effects
• Immune Compatibility: dECM derived from autologous or allogeneic cells can reduce the immune response in regenerative medicine applications, which is critical for the survival and integration of transplanted stem cells.
• Anti-inflammatory Properties: Some dECM components possess inherent anti-inflammatory properties, which can help in maintaining a conducive environment for stem cell growth and function.
5. Customization and Versatility
• Source Flexibility: dECM can be derived from various tissues, enabling customization for specific stem cell applications, such as neural, cardiac, or musculoskeletal tissues.
• Combinatorial Approaches: dECM can be combined with growth factors, cytokines, or other bioactive molecules to further tailor the microenvironment for specific stem cell needs.
6. Facilitating 3D Culture Systems
• 3D Scaffold Formation: dECM can be used to create three-dimensional (3D) culture systems that more accurately mimic the in vivo conditions, which is crucial for studying cell behavior in a more realistic context.
• Tissue Engineering: The use of dECM in 3D culture systems facilitates the development of complex tissue constructs, supporting the growth of stem cells in a manner that promotes tissue regeneration and repair.
7. Reduction in Use of Animal-Derived Products
• Xeno-Free Cultures: dECM provides a viable alternative to animal-derived matrices, such as Matrigel, in creating xeno-free culture environments, which is especially important for clinical and translational applications.
8. Potential for High-Throughput Screening
• Scalability: dECM can be processed and applied in high-throughput screening platforms, allowing for the testing of various conditions and compounds on stem cells in a controlled and reproducible manner.
In summary, cell-derived dECM offers a multitude of benefits for stem cell culture, including the provision of a native-like microenvironment, enhanced differentiation and proliferation, and the ability to customize the scaffold for specific applications. These advantages make dECM a valuable tool in advancing stem cell research, regenerative medicine, and tissue engineering.
Discussion
The article’s examination of stem cell cultivation techniques is more than just a summary of recent developments; it's a story that spans decades of cellular biology’s evolutionary landscape and captures decades of scientific research, invention, and hope. Stem cells represent the unfathomable complexities of nature and provide insight into the regeneration potential hidden in all living things. They are frequently hailed as the cornerstone of regenerative medicine (Daley, 2012; Engle and Puppala, 2013).
The essay takes readers on a tour through the history of stem cell research, starting with the seminal findings of Takahashi and Yamanaka, whose ground-breaking work revealed the revolutionary idea of induced pluripotent stem cells (iPSCs). Because iPSCs can be derived from somatic cells and yet have the pluripotency of embryonic stem cells, they have not only changed the ethical landscape of stem cell research but have also made cellular reprogramming more accessible to the public. This has opened new avenues for personalized medicine and disease modeling (Nonaka et al., 2004).
During the acknowledgment of these paradigm-shifting discoveries, the piece also elucidates the complex interplay between scientific advancement and inherent challenges. Stem cell cultivation, with its intricate dynamics, presents significant obstacles for researchers. A primary concern is the genetic instability observed in long-term cultures, which necessitates a meticulous balance between preservation and expansion. Monitoring genetic stability is crucial to ensure that stem cells maintain their intended characteristics and avoid accumulating deleterious mutations. Due to the inherent heterogeneity within stem cell populations—comparable to a painter’s palette—novel methodologies are required to achieve uniformity. Such homogeneity is essential for effective therapeutic applications, as consistent genetic profiles are vital for reliable and successful treatments (Ben-David, 2015; Briu et al., 2021; Turinetto et al., 2017).
The essay lays out a path towards the horizon of possibility in response to these difficulties, where cutting-edge innovations like machine learning and artificial intelligence (AI) serve as sentinels, pointing the way forward. AI integration offers a symbiotic evolution where human creativity and data-driven insights combine to create previously unimaginable levels of efficiency and reproducibility. This is in addition to optimization (Altyar et al., 2023; Nosrati et al., 2021).
Furthermore, the conversation touches on a wide range of scientific topics, going beyond the boundaries of medicinal applications. Like the crucibles of alchemists, stem cell cultures are at the crossroads of several fields, ranging from ecological conservation to pulmonary biology. In addition to providing a window into the secrets of disease pathology, they hold the key to restoring the delicate balance of ecosystems, conserving endangered species, and opening the door to nature’s resilience (Ponti et al., 2005; Todaro et al., 2007; Eramo et al., 2008; Fischer et al., 2015).
However, amidst the grandeur of scientific endeavor, the article grounds itself in the sobering reality of laboratory practice. It underscores the paramount importance of meticulous cell culture management, where the pursuit of reliability and reproducibility stands as a sentinel against the specter of contamination and misidentification. Strategies ranging from stringent aseptic technique. The essay, however, firmly roots itself in the grim realities of laboratory work, even amid the magnificence of scientific achievement. It emphasizes how crucial it is to handle cell cultures meticulously, with the goal of reproducibility and dependability acting as a guard against contamination and misidentification. Techniques varying from strict aseptic methods to prudent use of antibiotics serve as the barrier against the incursion of biological whims, guaranteeing that the foundation of scientific investigation is integrity (Smith et al., 2014; Luizon et al., 2016).
Finally, the paper becomes more than just a discussion; it becomes a symphony that speaks to the goals, victories, and struggles that the scientific community shares. It is evidence of the tenacious spirit of inquiry, the unrelenting search for truth, and the unshakable faith in the revolutionary potential of stem cell research to show the way towards a more promising and health-conscious future for everybody.
Conclusion
The paper explores advancements in stem cell research, covering methods, challenges, and perspectives. It discusses breakthroughs like induced pluripotent stem cells (iPSCs) and hurdles such as genetic instability. It also highlights the potential of stem cell-derived products in personalized therapies. The section on stem cell origins includes perinatal tissues and induced pluripotent stem cells (iPSCs), detailing acquisition methods and their benefits. It emphasizes the importance of technology and molecular research in improving stem cell culture. The article discusses techniques for generating iPSCs and human embryonic stem cells (hESCs) and addresses common issues in cell culture management.
- iPSCs and hESCs present promising avenues for research and therapy;
- Genetic instability poses a significant challenge in stem cell applications;
- Stem cell-derived products hold immense potential for personalized medicine;
- Perinatal tissues and iPSCs offer accessible sources for stem cell research;
- Technological advancements and molecular insights are crucial for enhancing stem cell culture;
- Training, best practices, and cutting-edge technology are imperative for progress in biomedical research.
Overall, the paper underscores the critical role of stem cell culture in advancing medicine and emphasizes the importance of addressing key challenges and leveraging emerging opportunities in the field.
Author contributions
AG: Conceptualization, Investigation, Resources, Visualization, Writing–original draft, Writing–review and editing. MT: Conceptualization, Investigation, Project administration, Visualization, Writing–original draft, Writing–review and editing. BB: Conceptualization, Investigation, Visualization, Writing–original draft, Writing–review and editing. AB: Investigation, Resources, Visualization, Writing–original draft, Writing–review and editing. SS: Funding acquisition, Writing–review and editing. RM: Formal Analysis, Investigation, Project administration, Supervision, Visualization, Writing–original draft, Writing–review and editing.
Funding
The author(s) declare that no financial support was received for the research, authorship, and/or publication of this article. This research received no external funding.
Conflict of interest
The authors declare that the research was conducted in the absence of any commercial or financial relationships that could be construed as a potential conflict of interest.
Publisher’s note
All claims expressed in this article are solely those of the authors and do not necessarily represent those of their affiliated organizations, or those of the publisher, the editors and the reviewers. Any product that may be evaluated in this article, or claim that may be made by its manufacturer, is not guaranteed or endorsed by the publisher.
References
Aboul-Soud, M. A. M., Alzahrani, A. J., and Mahmoud, A. (2021). Induced pluripotent stem cells (iPSCs)-roles in regenerative therapies, disease modelling and drug screening. Cells 10 (9), 2319. doi:10.3390/cells10092319
Altyar, A. E., El-Sayed, A., Abdeen, A., Piscopo, M., Mousa, S. A., Najda, A., et al. (2023). Future regenerative medicine developments and their therapeutic applications. Biomed. & Pharmacother. 158, 114131. doi:10.1016/j.biopha.2022.114131
American Type Culture Collection Standards Development Organization Workgroup ASN-0002 (2010). Cell line misidentification: the beginning of the end. Nat. Rev. Cancer 10 (6), 441–448. doi:10.1038/nrc2852
Avar, M., Heinzer, D., Steinke, N., Dogancay, B., Moos, R., Lugan, S., et al. (2020). Prion infection, transmission, and cytopathology modeled in a low-biohazard human cell line. Life Sci. Alliance 3 (8), e202000814. doi:10.26508/lsa.202000814
Babic, Z., Capes-Davis, A., Martone, M. E., Bairoch, A., Ozyurt, B., Gillespie, T. H., et al. (2019). Incidences of problematic cell lines are lower in papers that use RRIDs to identify cell lines. Elife e41676 8, e41676. doi:10.7554/eLife.41676
Bacakova, L., Zarubova, J., Travnickova, M., Musilkova, J., Pajorova, J., Slepicka, P., et al. (2018). Stem cells: their source, potency and use in regenerative therapies with focus on adipose-derived stem cells – a review. Biotechnol. Adv. 36 (4), 1111–1126. doi:10.1016/j.biotechadv.2018.03.011
Baharvand, H., Hashemi, S. M., Kazemi Ashtiani, S., and Farrokhi, A. (2006). Differentiation of human embryonic stem cells into hepatocytes in 2D and 3D culture systems in vitro. Int. J. Dev. Biol. 50 (7), 645–652. doi:10.1387/ijdb.052072hb
Barreto, S., Hamel, L., Schiatti, T., Yang, Y., and George, V. (2019). Cardiac progenitor cells from stem cells: learning from genetics and biomaterials. Cells 8 (12), 1536. doi:10.3390/cells8121536
Bartfeld, S., Bayram, T., van de Wetering, M., Huch, M., Begthel, H., Kujala, P., et al. (2015). In vitro expansion of human gastric epithelial stem cells and their responses to bacterial infection. Gastroenterology 148 (1), 126–136. doi:10.1053/j.gastro.2014.09.042
Ben-David, U. (2015). Genomic instability, driver genes and cell selection: projections from cancer to stem cells. BBA Gene Regul. Mech. 1849 (4), 427–435. doi:10.1016/j.bbagrm.2014.08.005
Boeri, L., Albani, D., Raimondi, M. T., and Jacchetti, E. (2019). Mechanical regulation of nucleocytoplasmic translocation in mesenchymal stem cells: characterization and methods for investigation. Biophys. Rev. 11 (5), 817–831. doi:10.1007/s12551-019-00594-3
Borojevic, R., Monteiro, A. N., Vinhas, S. A., Domont, G. B., Mourao, P. A., Emonard, H., et al. (1985). Establishment of a continuous cell line from fibrotic schistosomal granulomas in mice livers. Vitro Cell Dev. Biol. 21 (7), 382–390. doi:10.1007/BF02623469
Briu, L. M., Maric, C., and Cadoret, J. C. (2021). Replication stress, genomic instability, and replication timing: a complex relationship. Int. J. Mol. Sci. 22 (9), 4764. doi:10.3390/ijms22094764
Cai, J., Chen, H., Xie, S., Hu, Z., and Bai, Y. (2022). Research progress of totipotent stem cells. Stem Cells Dev. 31 (13-14), 335–345. doi:10.1089/scd.2022.0061
Campostrini, G., Windt, L. M., van Meer, B. J., Bellin, M., and Mummery, C. L. (2021). Cardiac tissues from stem cells: new routes to maturation and cardiac regeneration. Circ. Res. 128 (6), 775–801. doi:10.1161/CIRCRESAHA.121.318183
Capes-Davis, A., Theodosopoulos, G., Atkin, I., Dexler, H. G., Kohara, A., MacLeod, R. A. F., et al. (2010). Check your cultures! A list of cross-contaminated or misidentified cell lines. Int. J. Cancer 127 (1), 1–8. doi:10.1002/ijc.25242
Chen, F., Wang, H., and Xiao, J. (2020). Regulated differentiation of stem cells into an artificial 3D liver as a transplantable source. Clin. Mol. Hepatol. 26 (2), 163–179. doi:10.3350/cmh.2019.0022n
Chen, K. G., Mallon, B. S., McKay, R. D., and Robey, P. G. (2014). Human pluripotent stem cell culture: considerations for maintenance, expansion, and therapeutics. Cell Stem Cell 14 (1), 13–26. doi:10.1016/j.stem.2013.12.005
Chen, P., Zheng, L., Wang, Y., Tao, M., Xie, Z., Xia, C., et al. (2019). Desktop-stereolithography 3D printing of a radially oriented extracellular matrix/mesenchymal stem cell exosome bioink for osteochondral defect regeneration. Theranostics 9 (9), 2439–2459. doi:10.7150/thno.31017
Cheng, H., Zheng, Z., and Cheng, T. (2020). New paradigms on hematopoietic stem cell differentiation. Protein Cell 11 (1), 34–44. doi:10.1007/s13238-019-0633-0
Chou, M. L., Bailey, A., Avory, T., Tanimoto, J., and Burnouf, T. (2015). Removal of transmissible spongiform encephalopathy prion from large volumes of cell culture media supplemented with fetal bovine serum by using hollow fiber anion-exchange membrane chromatography. PLoS One 10 (4), e0122300. doi:10.1371/journal.pone.0122300
Christopher, L., and Baker, M. F. P. (2018). Capturing totipotent stem cells. Cell Stem Cell 22 (1), 25–34. doi:10.1016/j.stem.2017.12.011
Chu, D. T., Phuong, T. N. T., Tien, N. L. B., Tran, D. K., Thanh, V. V., Quang, T. L., et al. (2020). An update on the progress of isolation, culture, storage, and clinical application of human bone marrow mesenchymal stem/stromal cells. Int. J. Mol. Sci. 21 (3), 708. doi:10.3390/ijms21030708
Daley, G. Q. (2012). The promise and perils of stem cell therapeutics. Cell Stem Cell 10 (6), 740–749. doi:10.1016/j.stem.2012.05.010
de Morree, A., and Rando, T. A. (2023). Regulation of adult stem cell quiescence and its functions in the maintenance of tissue integrity. Nat. Rev. Mol. Cell Biol. 24 (5), 334–354. doi:10.1038/s41580-022-00568-6
Dierick, F., Solinc, J., Bignard, J., Soubrier, F., and Nadaud, S. (2021). Progenitor/stem cells in vascular remodeling during pulmonary arterial hypertension. Cells 10 (6), 1338. doi:10.3390/cells10061338
Discher, D. E., Janmey, P., and Wang, Y. L. (2005). Tissue cells feel and respond to the stiffness of their substrate. Science 310 (5751), 1139–1143. doi:10.1126/science.1116995
Donnaloja, F., Jacchetti, E., Soncini, M., and Raimondi, M. T. (2020). Natural and synthetic polymers for bone scaffolds optimization. Polym. (Basel) 12 (4), 905. doi:10.3390/polym12040905
Drexler, H., and Uphoff, C. C. (2002). Mycoplasma contamination of cell cultures: incidence, sources, effects, detection, elimination, prevention. Cytotechnology 39 (2), 75–90. doi:10.1023/A:1022913015916
Engle, S. J., and Puppala, D. (2013). Integrating human pluripotent stem cells into drug development. Cell Stem Cell 12 (6), 669–677. doi:10.1016/j.stem.2013.05.011
Engler, A. J., Sen, S., Sweeney, H. L., and Discher, D. E. (2006). Matrix elasticity directs stem cell lineage specification. Cell. 126 (4), 677–689. doi:10.1016/j.cell.2006.06.044
Eramo, A., Lotti, F., Sette, G., Pilozzi, E., Biffoni, M., Di Virgilio, A., et al. (2008). Identification and expansion of the tumorigenic lung cancer stem cell population. Cell Death Differ. 15 (3), 504–514. doi:10.1038/sj.cdd.4402283
Ferrúa, C. P., Centeno, E. G. Z., Rosa, L. C. D., Amaral, C. C. D., Severo, R. F., Sarkis-Onofre, R., et al. (2017). How has dental pulp stem cells isolation been conducted? A scoping review. Braz Oral Res. 31, e87. doi:10.1590/1807-3107BOR-2017.vol31.0087
Fischer, U., Backes, C., Raslan, A., Keller, A., Meier, C., and Meese, E. (2015). Gene amplification during differentiation of mammalian neural stem cells in vitro and in vivo. Oncotarget 6 (9), 7023–7039. doi:10.18632/oncotarget.3248
Gao, L., Xu, W., Li, T., Chen, J., Shao, A., Yan, F., et al. (2018). Stem cell therapy: a promising therapeutic method for intracerebral hemorrhage. Cell Transpl. 27 (12), 1809–1824. doi:10.1177/0963689718773363
Geraghty, R., Capes-Davis, A., Davis, J., Downward, J., Freshney, R. I., Knezevic, I., et al. (2014). Guidelines for the use of cell lines in biomedical research. Br. J. Cancer 111 (6), 1021–1046. doi:10.1038/bjc.2014.166
Giorgino, R., Albano, D., Fusco, S., Peretti, G. M., Mangiavini, L., and Messina, C. (2023). Knee osteoarthritis: epidemiology, pathogenesis, and mesenchymal stem cells: what else is new? An update. Int. J. Mol. Sci. 24 (7), 6405. doi:10.3390/ijms24076405
Gombold, J., Karakasidis, S., Niksa, P., Podczasy, J., Neumann, K., Richardson, J., et al. (2014). Systematic evaluation of in vitro and in vivo adventitious virus assays for the detection of viral contamination of cell banks and biological products. Vaccine 32 (24), 2916–2926. doi:10.1016/j.vaccine.2014.02.021
Grzelak, A., Rychlik, B., and Bartosz, G. (2001). Light-dependent generation of reactive oxygen species in cell culture media. Free Radic. Biol. Med. 30 (12), 1418–1425. doi:10.1016/s0891-5849(01)00545-7
Hendijani, F. (2017). Explant culture: an advantageous method for isolation of mesenchymal stem cells from human tissues. Cell Prolif. 50, e12334. doi:10.1111/cpr.12334
Herbert, W. J., Parratt, D., Van Meirvenne, N., and Lennox, B. (1980). An accidental laboratory infection with trypanosomes of a defined stock. II. Studies on the serological response of the patient and the identity of the infecting organism. J. Infect. 2 (2), 113–124. doi:10.1016/s0163-4453(80)91109-3
Herwaldt, B. L. (2001). Laboratory-acquired parasitic infections from accidental exposures. Clin. Microbiol. Rev. 14 (4), 659–688. doi:10.1128/CMR.14.3.659-688.2001
Huang, Y., Liu, Y., Zheng, C., and Shen, C. (2017). Investigation of cross-contamination and misidentification of 278 widely used tumor cell lines. PloS One 12 (1), e0170384. doi:10.1371/journal.pone.0170384
Hwang, J. J., Rim, Y. A., Nam, Y., and Ju, J. H. (2021). Recent developments in clinical applications of mesenchymal stem cells in the treatment of rheumatoid arthritis and osteoarthritis. Front. Immunol. 12, 631291. doi:10.3389/fimmu.2021.631291
Ilic, D., and Polak, J. M. (2011). Stem cells in regenerative medicine: introduction. Br. Med. Bull. 98, 117–126. doi:10.1093/bmb/ldr012
Into, T., Kiura, K., Yasuda, M., Kataoka, H., Inoue, N., Hasebe, A., et al. (2004). Stimulation of human Toll-like receptor (TLR) 2 and TLR6 with membrane lipoproteins of Mycoplasma fermentans induces apoptotic cell death after NF-kappa B activation. Cell Microbiol. 6 (2), 187–199. doi:10.1046/j.1462-5822.2003.00356.x
Jiang, Y., and Tuan, R. S. (2023). Bioactivity of human adult stem cells and functional relevance of stem cell-derived extracellular matrix in chondrogenesis. Stem Cell Res. Ther. 14 (1), 160. doi:10.1186/s13287-023-03392-7
Karagiannis, P., Takahashi, K., Saito, M., Yoshida, Y., Okita, K., Watanabe, A., et al. (2019). Induced pluripotent stem cells and their use in human models of disease and development. Physiol. Rev. 99 (1), 79–114. doi:10.1152/physrev.00039.2017
Kim, Y. S., Majid, M., Melchiorri, A. J., and Mikos, A. G. (2018). Applications of decellularized extracellular matrix in bone and cartilage tissue engineering. Bioeng. & Transl. Med. 4 (1), 83–95. doi:10.1002/btm2.10110
Kobold, S., Bultjer, N., Stacey, G., Mueller, S. C., Kurtz, A., and Mah, N. (2023). History and current status of clinical studies using human pluripotent stem cells. Stem Cell Rep. 18 (8), 1592–1598. doi:10.1016/j.stemcr.2023.03.005
Kolios, G., and Moodley, Y. (2013). Introduction to stem cells and regenerative medicine. Respiration 85 (1), 3–10. doi:10.1159/000345615
Lan, T., Luo, M., and Wei, X. (2021). Mesenchymal stem/stromal cells in cancer therapy. J. Hematol. Oncol. 14 (1), 195. doi:10.1186/s13045-021-01208-w
Lee, J. Y., and Hong, S. H. (2020). Hematopoietic stem cells and their roles in tissue regeneration. Int. J. Stem Cells 13 (1), 1–12. doi:10.15283/ijsc19127
Lee, W. J., Lee, J. E., Hong, Y. J., Yoon, S. H., Song, H., Park, C., et al. (2020). Generation of brain organoids from mouse ESCs via teratoma formation. Stem Cell Res. 49, 102100. doi:10.1016/j.scr.2020.102100
Li, Y. R., Dunn, Z. S., Yu, Y., Li, M., Wang, P., and Yang, L. (2018). Advancing cell-based cancer immunotherapy through stem cell engineering. Cell Stem Cell 30 (5), 592–610. doi:10.1016/j.stem.2023.02.009
Liu, G., David, B. T., Trawczynski, M., and Fessler, R. G. (2020). Advances in pluripotent stem cells: history, mechanisms, technologies, and applications. Stem Cell Rev. Rep. 16 (1), 3–32. doi:10.1007/s12015-019-09935-x
Llobet, L., Montoya, J., Lopez-Gallardo, E., and Ruiz-Pesini, E. (2015). Side effects of culture media antibiotics on cell differentiation. Tissue Eng. Part C. Methods 21 (11), 1143–1147. doi:10.1089/ten.TEC.2015.0062
Lovell-Badge, R., Anthony, E., Barker, R. A., Bubela, T., Brivanlou, A. H., Carpenter, M., et al. (2021). ISSCR guidelines for stem cell research and clinical translation: the 2021 update. Stem Cell Rep. 16 (6), 1398–1408. doi:10.1016/j.stemcr.2021.05.012
Lu, V., Tennyson, M., Zhang, J., and Khan, W. (2021). Mesenchymal stem cell-derived extracellular vesicles in tendon and ligament aepair: a systematic review of in vivo studies. Cells 10 (10), 2553. doi:10.3390/cells10102553
Luizon, M. R., Eckalbar, W. L., Wang, Y., Jones, S. L., Smith, P. R., Laurance, M., et al. (2016). Genomic characterization of metformin hepatic response. PLoS Genet. 12 (11), e1006449. doi:10.1371/journal.pgen.1006449
Maemura, M., Taketsuru, H., Nakajima, Y., Shao, R., Kakihara, A., Nogami, J., et al. (2021). Totipotency of mouse zygotes extends to single blastomeres of embryos at the four-cell stage. Sci. Rep. 11 (1), 11167. doi:10.1038/s41598-021-90653-1
Malekmohamadi, N., Abdanipour, A., Ghorbanlou, M., Shokri, S., Shirazi, R., Dimitriadis, E., et al. (2019). Differentiation of bone marrow derived mesenchymal stem cells into male germ-like cells in co-culture with testicular cells. Endocr. Regul. 53 (2), 93–99. doi:10.2478/enr-2019-0011
Malik, V., and Wang, J. (2022). Pursuing totipotency: authentic totipotent stem cells in culture. Trends Genet. 38 (7), 632–636. doi:10.1016/j.tig.2022.03.012
Marega, M., El-Merhie, N., Gökyildirim, M. Y., Orth, V., Bellusci, S., and Chao, C. M. (2023). Stem/progenitor cells and related therapy in bronchopulmonary dysplasia. Int. J. Mol. Sci. 24 (13), 11229. doi:10.3390/ijms241311229
Mas-Bargues, C., and Borrás, C. (2021). Importance of stem cell culture conditions for their derived extracellular vesicles therapeutic effect. Free Radic. Biol. Med. 168, 16–24. doi:10.1016/j.freeradbiomed.2021.03.028
McKee, C., and Chaudhry, G. R. (2017). Advances, and challenges in stem cell culture. Colloids Surf. B Biointerfaces 159, 62–77. doi:10.1016/j.colsurfb.2017.07.051
Meten, O. W. (2002). Virus contaminations of cell cultures–a biotechnological view. Cytotechnology 39, 91–116. doi:10.1023/A:1022969101804
Mushahary, D., Spittler, A., Kasper, C., Weber, V., and Charwat, V. (2018). Isolation, cultivation, and characterization of human mesenchymal stem cells. Cytometry 93, 19–31. doi:10.1002/cyto.a.23242
Nava, M. M., Raimondi, M. T., and Pietrabissa, R. (2012). Controlling self-renewal and differentiation of stem cells via mechanical cues. J. Biomed. Biotechnol. 2012, 797410. doi:10.1155/2012/797410
Nelson-Rees, W. A., and Flandermeyer, R. R. (1976). HeLa cultures defined. Science 191 (4222), 96–98. doi:10.1126/science.1246601
Nikitin, P. V., Musina, G. R., Polozov, V. N., Goreiko, D. N., Krasnovsky, V. M., Werkenbark, L., et al. (2023). Development of glioblastoma from stem cells to a full-fledged tumor. Turk Patoloji Derg. 39 (2), 117–132. doi:10.5146/tjpath.2020.01582
Nims, R. W., and Price, P. J. (2017). Best practices for detecting and mitigating the risk of cell culture contaminants. Vitro Cell. & Dev. Biology-Animal 53 (10), 872–879. doi:10.1007/s11626-017-0203-9
Nonaka, M., Yoshikawa, M., Nishimura, F., Yokota, H., Kimura, H., Hirabayashi, H., et al. (2004). Intraventricular transplantation of embryonic stem cell-derived neural stem cells in intracerebral hemorrhage rats. Neurol. Res. 26 (3), 265–272. doi:10.1179/016164104225014049
Nosrati, H., Khouy, R. A., Nosrati, A., Khodaei, M., Banitalebi-Dehkordi, M., Ashrafi-Dehkordi, K., et al. (2021). Nanocomposite scaffolds for accelerating chronic wound healing by enhancing angiogenesis. J. Nanobiotechnology 19 (1), 1. doi:10.1186/s12951-020-00755-7
Nosrati, H., and Nosrati, M. (2023). Artificial intelligence in regenerative medicine: applications and implications. Biomimetics 8 (5), 442. doi:10.3390/biomimetics8050442
Orticelli, V., Papait, A., Vertua, E., Bonassi Signoroni, P., Romele, P., Di Pietro, L., et al. (2021). Human amniotic mesenchymal stromal cells support the ex vivo expansion of cord blood hematopoietic stem cells. Stem Cells Transl. Med. 10 (11), 1516–1529. doi:10.1002/sctm.21-0130
Otto, W. R., and Green, A. M. (2020). Fungal infections in children with haematologic malignancies and stem cell transplant recipients. Br. J. Hematol. 189 (4), 607–624. doi:10.1111/bjh.16452
Palumbo, P., Lombardi, F., Siragusa, G., Cifone, M. G., Cinque, B., and Giuliani, M. (2018). Methods of isolation, characterization, and expansion of human adipose-derived stem cells (ASCs): an overview. Int. J. Mol. Sci. 19 (7), 1897. doi:10.3390/ijms19071897
Pamies, D., Leist, M., Coecke, S., Bowe, G., Allen, D. G., Gstraunthaler, G., et al. (2022). Guidance document on good cell and tissue culture practice 2.0 (GCCP 2.0). Altern. Animal Exp. 39 (1), 30–70. doi:10.14573/altex.2111011
Parrotta, E. I., Scalise, S., Scaramuzzino, L., and Cuda, G. (2019). Stem cells: the game changers of human cardiac disease modelling and regenerative medicine. Int. J. Mol. Sci. 20 (22), 5760. doi:10.3390/ijms20225760
Pei, M. (2017). Environmental preconditioning rejuvenates adult stem cells' proliferation and chondrogenic potential. Biomaterials 117, 10–23. doi:10.1016/j.biomaterials.2016.11.049
Ponti, D., Costa, A., Zaffaroni, N., Pratesi, G., Petrangolini, G., Coradini, D., et al. (2005). Isolation and in vitro propagation of tumorigenic breast cancer cells with stem/progenitor cell properties. Cancer Res. 65 (13), 5506–5511. doi:10.1158/0008-5472.CAN-05-0626
Prieto Gonzalez, E. A. (2022). “A multilevel approach to the causes of genetic instability in stem cells,” in Handbook of stem cell therapy. Springer, 1–55. doi:10.1007/978-981-16-6016-0_26-1
Priya, N., Sarcar, S., Majumdar, A. S., and SundarRaj, S. (2014). Explant culture: a simple, reproducible, efficient, and economic technique for isolation of mesenchymal stromal cells from human adipose tissue and lipoaspirate. J. Tissue Eng. Regen. Med. 8, 706–716. doi:10.1002/term.1569
Puerta-Alcalde, P., and Garcia-Vidal, C. (2021). Changing epidemiology of invasive fungal disease in allogeneic hematopoietic stem cell transplantation. J. Fungi 7 (10), 848. doi:10.3390/jof7100848
Raitanen, J., Barta, B., Hacker, M., Georg, D., Balber, T., and Mitterhauser, M. (2023). Comparison of radiation response between 2D and 3D cell culture models of different human cancer cell lines. Cells 12 (3), 360. doi:10.3390/cells12030360
Ratajczak, M. Z., Zuba-Surma, E., Kucia, M., Poniewierska, A., Suszynska, M., and Ratajczak, J. (2012). Pluripotent and multipotent stem cells in adult tissues. Adv. Med. Sci. 57 (1), 1–17. doi:10.2478/v10039-012-0020-z
Rey, F., Barzaghini, B., Nardini, A., Bordoni, M., Zuccotti, G. V., Cereda, C., et al. (2020). Advances in tissue engineering and innovative fabrication techniques for 3-D-structures: translational applications in neurodegenerative diseases. Cells 9 (7), 1636. doi:10.3390/cells9071636
Rodríguez-Fuentes, D. E., Fernández-Garza, L. E., Samia-Meza, J. A., Barrera-Barrera, S. A., Caplan, A. I., and Barrera-Saldaña, H. A. (2021). Mesenchymal stem cells current clinical applications: a systematic review. Arch. Med. Res. 52 (1), 93–101. doi:10.1016/j.arcmed.2020.08.006
Rottem, S. (2003). Interaction of mycoplasmas with host cells. Physiol. Rev. 83 (2), 417–432. doi:10.1152/physrev.00030.2002
Ryu, A. H., Eckalbar, W. L., Kreimer, A., Yosef, N., and Ahituv, N. (2017). Use antibiotics in cell culture with caution: genome-wide identification of antibiotic-induced changes in gene expression and regulation. Sci. Rep. 7 (1), 7533. doi:10.1038/s41598-017-07757-w
Sajeesh, S., Broekelman, T., Mecham, R. P., and Ramamurthi, A. (2020). Stem cell derived extracellular vesicles for vascular elastic matrix regenerative repair. Acta Biomater. 113, 267–278. doi:10.1016/j.actbio.2020.07.002
Sanders, R. C., Slayton, W. B., Cogle, C. R., Fisher, R. C., and Scott, E. W. (2006). Stem cell research. Pediatr. Respir. Rev. 7 (2), 135–140. doi:10.1016/j.prrv.2006.03.009
Schroder, S. K., Schuler, H. M., Petersen, K. V., Tesauro, C., Knudsen, B. R., Pedersen, F. S., et al. (2022). Genetic and molecular characterization of the immortalized murine hepatic stellate cell line GRX. Cells 11 (9), 1504. doi:10.3390/cells11091504
Slack, J. (2018). What is a stem cell? Wiley Interdiscip. Rev. Dev. Biol. 7 (5), e323. doi:10.1002/wdev.323
Smith, R. P., Eckalbar, W. L., Morrissey, K. M., Luizon, M. R., Hoffman, T. J., Sun, X., et al. (2014). Genome-wide discovery of drug-dependent human liver regulatory elements. PloS Genet. 10 (10), e1004648. doi:10.1371/journal.pgen.1004648
Sobhani, A., Khanlarkhani, N., Baazm, M., Mohammadzadeh, F., Najafi, A., Mehdinejadiani, S., et al. (2017). Multipotent stem cell and current application. Acta Med. Iran. 55 (1), 6–23.
Souza, A. G., Silva, I. B. B., Campos-Fernandez, E., Barcelos, L. S., Souza, J. B., Marangoni, K., et al. (2018). Comparative assay of 2D and 3D cell culture models: proliferation, gene expression and anticancer drug response. Curr. Pharm. Des. 24 (15), 1689–1694. doi:10.2174/1381612824666180404152304
Steimberg, N., Bertero, A., Chiono, V., Dell'Era, P., Di Angelantonio, S., Hartung, T., et al. (2020). iPS, organoids and 3D models as advanced tools for in vitro toxicology. ALTEX 37 (1), 136–140. doi:10.14573/altex.1911071
Takahashi, K., and Yamanaka, S. (2006). Induction of pluripotent stem cells from mouse embryonic and adult fibroblast cultures by defined factors. Cell 126 (4), 663–676. doi:10.1016/j.cell.2006.07.024
Takahashi, K., and Yamanaka, S. (2013). Induced pluripotent stem cells in medicine and biology. Development 140 (12), 2457–2461. doi:10.1242/dev.092551
Taur, Y., Jenq, R. R., Parales, M. A., Littmann, E. R., Morjaria, S., Ling, L., et al. (2014). The effects of intestinal tract bacterial diversity on mortality following allogenic hematopoietic steam cell transplantation. Blood 124 (7), 1174–1182. doi:10.1182/blood-2014-02-554725
Todaro, M., Alea, M. P., Di Stefano, A. D., Cammareri, P., Vermeulen, L., Iovino, F., et al. (2007). Colon cancer stem cells dictate tumor growth and resist cell death by production of interleukin 4. Cell Stem Cell 1 (4), 389–402. doi:10.1016/j.stem.2007.08.001
Todtenhaupt, P., Franken, L. A., Groene, S. G., van Hoolwerff, M., van der Meeren, L. E., van Klink, J. M. M., et al. (2023). A robust and standardized method to isolate and expand mesenchymal stromal cells from human umbilical cord. Cytotherapy 25 (10), 1057–1068. doi:10.1016/j.jcyt.2023.07.004
Turinetto, V., Orlando, L., and Giachino, C. (2017). Induced pluripotent stem cells: advances in the quest for genetic stability during reprogramming process. Int. J. Mol. Sci. 18 (9), 1952. doi:10.3390/ijms18091952
Varghese, D. S., Paeween, S., Ardah, M. T., Starling Emerald, B., and Ansari, S. A. (2017). Effects of aminoglycoside antibiotics on human embryonic stem cell viability during differentiation in vitro. Stem Cells Int. 2017, 2451927. doi:10.1155/2017/2451927
Wang, Y., Hu, G., Hill, R. C., Dzieciatkowska, M., Hansen, K. C., Zhang, X. B., et al. (2021a). Matrix reverses immortalization-mediated stem cell fate determination. Biomaterials 265, 120387. doi:10.1016/j.biomaterials.2020.120387
Wang, Y. H., Tao, Y. C., Wu, D. B., Wang, M. L., Tang, H., and Chen, E. Q. (2021b). Cell heterogeneity, rather than the cell storage solution, affects the behavior of mesenchymal stem cells in vitro and in vivo. Stem Cell Res. Ther. 12, 391. doi:10.1186/s13287-021-02450-2
Ware, C. B., Jonlin, E. C., Anderson, D. J., Cavanaugh, C., Hesson, J., Sidhu, S., et al. (2023). Derivation of naïve human embryonic stem cells using a CHK1 inhibitor. Stem Cell Rev. Rep. 19 (8), 2980–2990. doi:10.1007/s12015-023-10613-2
Weiskirchen, R. (2022). Established liver cell lines: are you sure to have the right ones? Livers 2 (3), 171–177. doi:10.3390/livers2030015
Wilkinson, A. C., Ishida, R., Nakauchi, H., and Yamazaki, S. (2020). Long-term ex vivo expansion of mouse hematopoietic stem cells. Nat. Protoc. 15 (2), 628–648. doi:10.1038/s41596-019-0263-2
Xie, X., Nóbrega, R., and Pšenička, M. (2020). Spermatogonial stem cells in fish: characterization, isolation, enrichment, and recent advances of in vitro culture systems. Biomolecules 10 (4), 644. doi:10.3390/biom10040644
Yamanaka, S. (2020). Pluripotent stem cell-based cell therapy-promise and challenges. Cell Stem Cell 27 (4), 523–531. doi:10.1016/j.stem.2020.09.014
Yao, Q., Zheng, Y. W., Lan, Q. H., Kou, L., Xu, H. L., and Zhao, Y. Z. (2019). Recent development and biomedical applications of decellularized extracellular matrix biomaterials. Mater. Sci. & Eng. C, Mater. Biol. Appl. 104, 109942. doi:10.1016/j.msec.2019.109942
Yen, B. L., Hsieh, C. C., Hsu, P. J., Chang, C. C., Wang, L. T., and Yen, M. L. (2023). Three-dimensional spheroid culture of human mesenchymal stem cells: offering therapeutic advantages and in vitro glimpses of the in vivo state. Stem Cells Transl. Med. 12 (5), 235–244. doi:10.1093/stcltm/szad011
Young, R. A. (2011). Control of the embryonic stem cell state. Cell 144 (6), 940–954. doi:10.1016/j.cell.2011.01.032
Yu, N., Zhang, F., Tang, X., Liu, Y., Zhang, J., Yang, B., et al. (2023). Hierarchical hydrogel microarrays fabricated based on a microfluidic printing platform for high-throughput screening of stem cell lineage specification. Acta Biomater. 161, 144–153. doi:10.1016/j.actbio.2023.02.036
Zakrzewski, W., Dobrzyński, M., Szymonowicz, M., and Rybak, Z. (2019). Stem cells: past, present, and future. Stem Cell Res. Ther. 10 (1), 68. doi:10.1186/s13287-019-1165-5
Zaripova, L. N., Midgley, A., Christmas, S. E., Beresford, M. W., Pain, C., Baildam, E. M., et al. (2023). Mesenchymal stem cells in the pathogenesis and therapy of autoimmune and autoinflammatory diseases. Int. J. Mol. Sci. 24 (22), 16040. doi:10.3390/ijms242216040
Zeyu, T., Tao, Y., Jun, L., Ting, W., and Akon, H. (2023). Introduction to stem cells. Prog. Mol. Biol. Transl. Sci. 199, 3–32. doi:10.1016/bs.pmbts.2023.02.012
Zhang, X., Chen, X., Hong, H., Hu, R., Liu, J., and Liu, C. (2021). Decellularized extracellular matrix scaffolds: recent trends and emerging strategies in tissue engineering. Bioact. Mater. 10, 15–31. doi:10.1016/j.bioactmat.2021.09.014
Zhao, Y., Wang, Q., Zhang, W., Jia, Q., Gao, Q., and Shuai, L. (2023). Protocol to generate induced trophoblast stem cells from embryonic stem cells in mice. Star. Protoc. 4 (1), 102092. doi:10.1016/j.xpro.2023.102092
Keywords: stem, cells, culture, methods, pluripotency, perspectives
Citation: Górska A, Trubalski M, Borowski B, Brachet A, Szymańczyk S and Markiewicz R (2024) Navigating stem cell culture: insights, techniques, challenges, and prospects. Front. Cell Dev. Biol. 12:1435461. doi: 10.3389/fcell.2024.1435461
Received: 03 June 2024; Accepted: 09 October 2024;
Published: 11 November 2024.
Edited by:
Ming Pei, West Virginia University, United StatesReviewed by:
Sandeep P. Dumbali, Moffitt Cancer Center, United StatesAnastasia Conti, San Raffaele Telethon Institute for Gene Therapy (SR-Tiget), Italy
Copyright © 2024 Górska, Trubalski, Borowski, Brachet, Szymańczyk and Markiewicz. This is an open-access article distributed under the terms of the Creative Commons Attribution License (CC BY). The use, distribution or reproduction in other forums is permitted, provided the original author(s) and the copyright owner(s) are credited and that the original publication in this journal is cited, in accordance with accepted academic practice. No use, distribution or reproduction is permitted which does not comply with these terms.
*Correspondence: Renata Markiewicz, cmVuYXRhbWFya2lld2ljekB1bWx1Yi5wbA==; Sylwia Szymańczyk, c3lsd2lhLnN6eW1hbmN6eWtAdXAubHVibGluLnBs