- Cardiovascular and Metabolic Disorders Research Program, Duke-NUS Medical School, Singapore, Singapore
Metabolic dysfunction-associated steatotic liver disease (MASLD), previously known as non-alcoholic fatty liver disease (NAFLD), is the most common liver disorder worldwide, with an estimated global prevalence of more than 31%. Metabolic dysfunction-associated steatohepatitis (MASH), formerly known as non-alcoholic steatohepatitis (NASH), is a progressive form of MASLD characterized by hepatic steatosis, inflammation, and fibrosis. This review aims to provide a comprehensive analysis of the extrahepatic manifestations of MASH, focusing on chronic diseases related to the cardiovascular, muscular, and renal systems. A systematic review of published studies and literature was conducted to summarize the findings related to the systemic impacts of MASLD and MASH. The review focused on the association of MASLD and MASH with metabolic comorbidities, cardiovascular mortality, sarcopenia, and chronic kidney disease. Mechanistic insights into the concept of lipotoxic inflammatory “spill over” from the MASH-affected liver were also explored. MASLD and MASH are highly associated (50%–80%) with other metabolic comorbidities such as impaired insulin response, type 2 diabetes, dyslipidemia, hypertriglyceridemia, and hypertension. Furthermore, more than 90% of obese patients with type 2 diabetes have MASH. Data suggest that in middle-aged individuals (especially those aged 45–54), MASLD is an independent risk factor for cardiovascular mortality, sarcopenia, and chronic kidney disease. The concept of lipotoxic inflammatory “spill over” from the MASH-affected liver plays a crucial role in mediating the systemic pathological effects observed. Understanding the multifaceted impact of MASH on the heart, muscle, and kidney is crucial for early detection and risk stratification. This knowledge is also timely for implementing comprehensive disease management strategies addressing multi-organ involvement in MASH pathogenesis.
Introduction
Recently, the Delphi process, led by an international consortium of pan-liver associations, has critically evaluated the terminological shortcomings associated with “non-alcoholic fatty liver disease (NAFLD).” As a result, the term has been redefined as “metabolic dysfunction-associated steatotic liver disease (MASLD)” (Rinella et al., 2023). This non-stigmatizing updated terminology, coupled with enhanced diagnostic guidelines, will support both awareness and the accuracy of patient identification, surpassing the limitations of previous nomenclature NAFLD. However, the inflammatory phase of MASLD, steatohepatitis, has been identified as a critical pathophysiological entity, deserving preservation of the term “steatohepatitis” within the clinical context. Consequently, it has been recommended that this condition be reclassified as “metabolic dysfunction-associated steatohepatitis (MASH),” formerly recognized as non-alcoholic steatohepatitis (NASH) (Estes et al., 2018; Fan XZ et al., 2024). MASH represent a spectrum of liver conditions consisting hepatic inflammation and fibrosis associated to metabolic dysfunction with the absence of significant alcohol consumption (Lim et al., 2023; Cusi et al., 2024; Fan XZ et al., 2024). These conditions have emerged as the leading causes of liver-related morbidity and mortality globally (Younossi et al., 2024a; Younossi et al., 2024b). MASLD/MASH is characterized by excessive hepatic fat accumulation, hepatic inflammation, hepatocyte ballooning and fibrosis, which may progress to cirrhosis and hepatocellular carcinoma (HCC) (Sinha et al., 2018; Tripathi et al., 2022).
The history of clinical MASH/NASH studies began about 45 years ago (Adler and Schaffner, 1979; Ludwig et al., 1980), but its recent increase in prevalence poses it as a global pandemic (Pouwels et al., 2022). Several recent studies have quantified the overall global prevalence of MASLD to be 30%–33%. Notably, reports show an increasing rate of MASLD prevalence from 25.3% (1990–2006) to 38.2% (2016–2019), which is a 50.4% increase in the last 30 years (Le et al., 2023). NAFLD/MASLD prevalence increased from 35.42% (2008–2016) to 46.20% (2017–2020) in MENA (Middle East and North Africa) region (Younossi et al., 2024b). Currently, the progression from MASLD to cirrhosis is estimated to be 4%. However, 20% of MASH patients could progress to cirrhosis. Most importantly, recent reports showed a dramatic increase in liver transplant waitlist registration accounted for MASH and associated cirrhosis and HCC (Lim et al., 2023; Younossi et al., 2024c). Epidemiologically, MASH possesses high geographic variability with higher rates encountered in South America and the Middle East, followed by Asia (Younossi et al., 2023). However, a higher MASLD incidence is recorded in the younger (45 years or younger) Asian population, which could be attributed to carbohydrate-rich foods, high central adiposity, and genetic predisposition (Younossi et al., 2023). Africa has recorded a low rate of MASLD (Younossi et al., 2023). Moreover, MASLD and MASH are highly associated (50%–80%) with impaired insulin response, type 2 diabetes, dyslipidaemia, hypertriglyceridemia, hypertension, and more than 90% of obese patients with type 2 diabetes have MASH (Le et al., 2023). Considering its significant association with other metabolic comorbidities, modelling study by Estes et al. (2018), has forecasted the prevalent MASLD cases to increase by 21% from 2015 to 2030, while prevalent MASH cases will increase by 63%. A following study by Estes et al. suggested a potential increase of 6%–20% in the prevalence of MASLD cases across Hong Kong, Singapore, South Korea, and Taiwan between 2019 and 2030. Similarly, they projected a concurrent rise of 20%–35% in prevalent MASH cases during the same period (Estes et al., 2020). Furthermore, their forecast anticipated a substantial surge in MASLD-related mortality, with estimates ranging between 65% and 100% from 2019 to 2030, prompting a significant concern (Estes et al., 2020).
Scientists, thus, predict that MASH could become the top condition for liver transplants soon. Despite its growing prevalence rate, to date, there are no specific therapies approved by the US Food and Drug Administration for MASLD/MASH (Tripathi et al., 2022). Treatment strategies for MASH typically involve lifestyle interventions, such as adherence to Mediterranean diet and increased physical activity (Semmler et al., 2021; Pouwels et al., 2022). However, for patients who do not respond to these interventions, several drugs targeting FXR, PPAR and GLP-1R agonists, focusing on inflammation, ballooning, apoptosis, and fibrosis, are currently in development (Jensen et al., 2018; Loomba et al., 2021; Duan et al., 2022). Bariatric surgery is designated for individuals classified as morbidly obese who have shown inadequate response to lifestyle interventions or weight-loss medications (Nachit et al., 2021; Pouwels et al., 2022). Studies indicated that direct medical expenses for MASH could sum up to $222 billion (excluding indirect and societal costs) (Witkowski et al., 2022).
MASLD and MASH have long been recognized primarily as a liver disease, but recent insights have expanded our understanding to acknowledge its significant systemic implications, especially concerning the cardiovascular, muscular, and renal systems (Nachit et al., 2021; Li et al., 2023; Wegermann et al., 2023). These organs are selected for focused study due to their vital roles in metabolic regulation and their pronounced vulnerability to the metabolic derangements typically associated with MASH, such as insulin resistance (IR), dyslipidemia, and systemic hypertension (Ferrara et al., 2019; Kasper et al., 2021). The pathological processes of MASH, including inflammation and fibrosis, exert profound systemic effects that are particularly observable in these organs (Chakravarthy et al., 2020; Wang et al., 2022). These effects include cardiovascular dysfunction, muscle wasting, and renal impairment, which not only exacerbate the disease burden but also critically influence patient outcomes by contributing significantly to morbidity and mortality (Ferrara et al., 2019; Wang et al., 2022), as illustrated in Figure 1. In contrast, while MASH undoubtedly impacts other tissues, the effects on these non-core systems are often less direct and may not significantly alter disease prognosis or treatment strategies. For instance, the gastrointestinal tract, skin, or adipose tissue (AT) might experience alterations due to metabolic dysfunction; however, these changes may not typically result in immediate life-threatening consequences or require the urgent, targeted interventions demanded by cardiac, muscular, or renal involvement (Alonso-Pena et al., 2023; Zeng et al., 2024). Furthermore, while this review primarily addresses the direct impacts and interactions of liver with heart, kidney and muscle in MASLD/MASH, the roles of other tissues, notably AT, in influencing systemic health outcomes are equally pivotal but beyond the scope of this manuscript. AT, through its endocrine and paracrine functions, significantly affects the heart, muscles, and kidneys, primarily via the secretion of adipokines that modulate inflammation, insulin sensitivity, and lipid metabolism. These interactions are complex and contribute to the multisystem nature of metabolic disorders. For a detailed exploration of the systemic effects of AT and its implications for cardiovascular, musculoskeletal, and renal health in the context of MASLD/MASH, readers are encouraged to consult the following comprehensive reviews (Chow et al., 2022; Gilani et al., 2024; Hafiane, 2024; Jia et al., 2024). Therefore, this manuscript prioritizes the heart, muscles, and kidneys due to their critical interplay with metabolic health and their direct connection to the primary complications associated with MASH, thereby offering clearer targets for therapeutic intervention and management strategies.
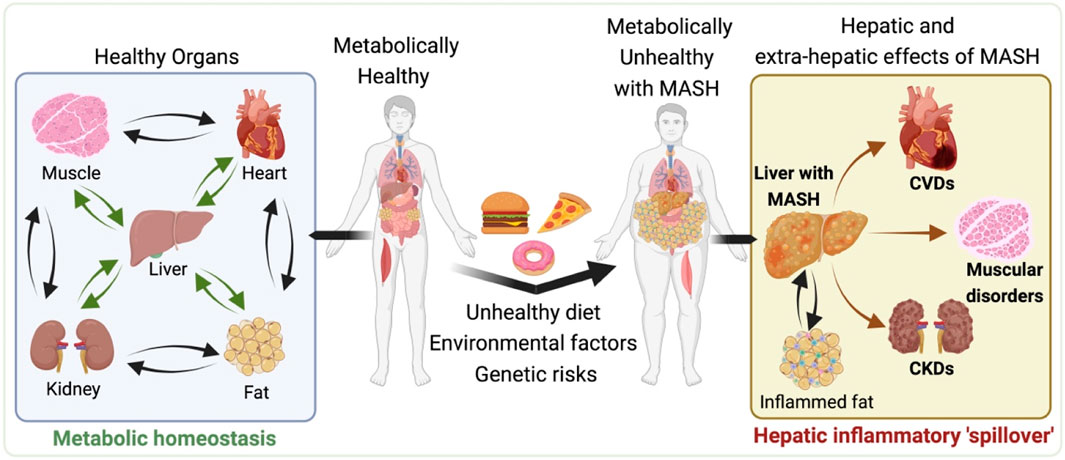
Figure 1. Systemic Impacts of metabolic dysfunction-associated steatotic liver disease (MASLD) and metabolic dysfunction associated steatohepatitis (MASH). Illustration depicts the progression from a metabolically healthy state (left side) to the development of MASLD and MASH and its subsequent systemic effects (right side). The left side shows the interconnectedness of healthy organs, including the heart, liver, muscle, and kidneys, maintaining metabolic balance. However, moving to the right, the impact of an unhealthy diet, environmental factors, and genetic predispositions contribute to the development of MASH, characterized by enlarged liver and visceral fat depot with the accumulated fat and inflammation. The hepatic and extra-hepatic consequences of MASH are evident, as the liver afflicted with MASH causally associated with the increased risk of cardiovascular diseases (CVDs), muscular disorders and chronic kidneys diseases (CKDs). All of which may also adversely impacted by the inflamed visceral fat associated with MASH. This representation highlights the systemic pathological nature of MASH, highlighting the importance of a holistic approach to its management and treatment. The illustration was made on BioRender.com.
Systemic manifestations, as indicated above, are largely attributed to the metabolic dysregulation underlying MASLD/MASH, including obesity, IR, systemic inflammation, and lipid dysmetabolism, which play pivotal roles in the pathogenesis of cardiovascular, muscular and renal complications (Anstee et al., 2020; Venniyoor et al., 2021). The systemic nature of MASLD/MASH and its extrahepatic manifestations necessitates a multidisciplinary approach for its management, integrating the expertise of hepatologists, endocrinologists, cardiologists, nephrologists, and nutritionists. Furthermore, this complexity highlights the importance of ongoing research to unravel the pathophysiological mechanisms linking MASH with its systemic effects, aiming to identify novel therapeutic targets and improve patient outcomes. Thus, the advancements in understanding of MASLD/MASH as a multisystem disease highlights the urgent need for heightened awareness and comprehensive management strategies. This review aims to consolidate present understanding regarding the hepatic and extrahepatic presentations of MASH, which contribute to cardiovascular, muscular, and renal complications, thereby laying the groundwork for further advancements in research and clinical practices.
Liver complications of MASLD/MASH
The hepatic manifestations of MASH involve a complex interplay of metabolic dysfunction and liver pathology. The pathogenesis of MASH is elucidated through the “multiple hit hypothesis,” which supersedes the earlier simplistic “two-hit model” by integrating multiple metabolic, genetic, epigenetic, and environmental factors that collectively drive disease progression. Over the past two decades, research has suggested that the initiation of MASH occurs with the accumulation of excessive intrahepatic fat, exceeding 5% of the total liver weight (Rinella et al., 2023). This accumulation then triggers metabolic disturbances, including alterations in pathways associated with fatty acid oxidation, dysregulated signaling of reactive oxygen species (ROS), mitochondrial dysfunction, compromised proteostasis, and imbalances in gut microbiome (Buzzetti et al., 2016; Machado and Diehl, 2016; Mitten and Baffy, 2022; Rafaqat et al., 2024; Zhong et al., 2024).
The implications of increased free FA accumulation involve augmented β-oxidation rates (referred as “inadequate substrate disposal”) and escalated ROS production within the mitochondrial respiratory chain (Figure 2). This imbalance between ROS generation and antioxidant defence mechanisms instigates oxidative stress, a hallmark of MASH pathogenesis, corroborated by elevated oxidative stress biomarkers in affected individuals. The oxidative milieu activates endoplasmic reticulum (ER) stress facilitating the activation of hepatic immune cells, including hepatocytes, hepatic stellate cells, Kupffer cells, dendritic cells, natural killer cells, T-lymphocytes, and B-lymphocytes, alongside pro-inflammatory signalling pathways. Free FAs exacerbate this inflammatory cascade, fostering the secretion of pro-inflammatory cytokines such as interleukin-6 (IL-6), tumor necrosis factor α (TNFα), and interleukin-1β (IL-1β), thereby perpetuating hepatic inflammation and cellular injury. These molecular derangements compromise the liver’s capacity for storing and exporting free FAs as triglycerides, culminating in hepatocyte lipid overload, steatosis and lipotoxicity. These mechanisms are comprehensively reviewed elsewhere (Buzzetti et al., 2016; Machado and Diehl, 2016; Lebeaupin et al., 2018; Loomba et al., 2021; Powell et al., 2021; Tilg et al., 2021; Llovet et al., 2023; Fan XZ et al., 2024; Habibullah et al., 2024; Jiang et al., 2024; Mahmoudi et al., 2024; Rafaqat et al., 2024; Targher et al., 2024; Verma MKT et al., 2024; Zhang et al., 2024; Zhong et al., 2024).
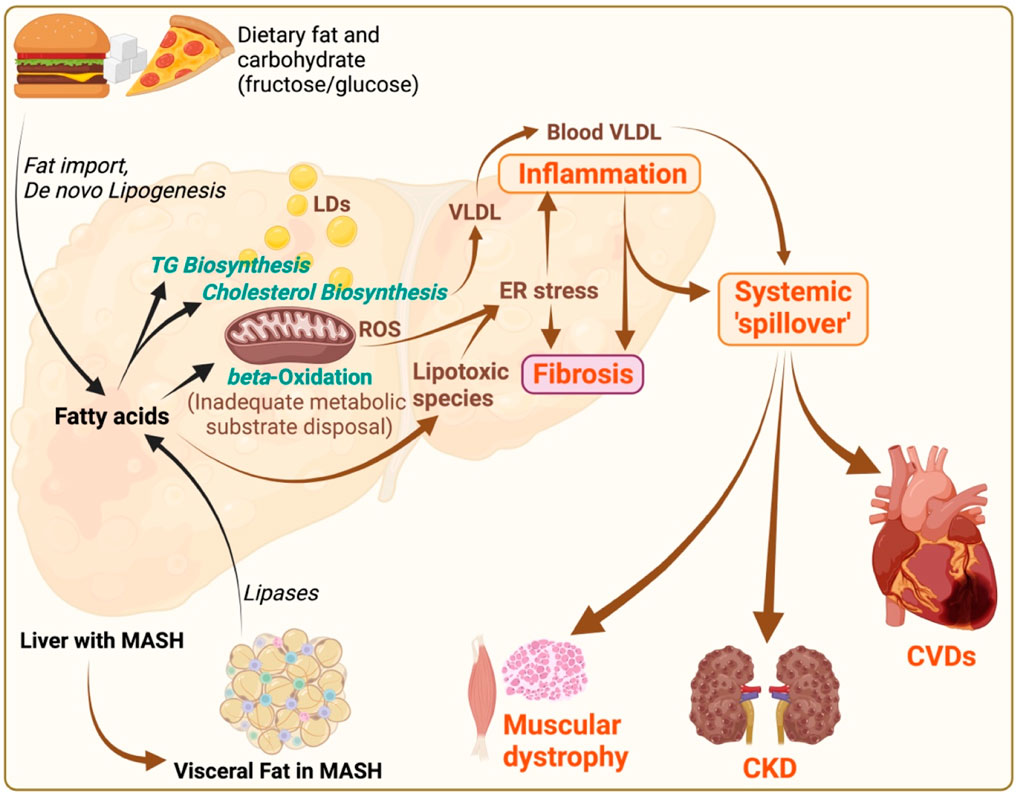
Figure 2. MASLD/MASH pathogenesis and its systemic implications. Illustration depicts the pathophysiological mechanisms of metabolic dysfunction-associated steatotic liver disease (MASLD)/metabolic associated steatohepatitis (MASH), and its systemic consequences. Dietary fats and carbohydrates (fructose in particular) contribute to fat import and de novo lipogenesis respectively within the liver, leading to triglyceride (TG) and cholesterol biosynthesis, and subsequent fatty acid accumulation. Increased flux of free fatty acids to mitochondria causes mitochondrial burnout, leading to inadequate disposal of metabolic substrates due to impaired beta-oxidation. These processes result in; i) increased lipid droplets (LDs) causing steatosis, ii) increased very-low-density lipoprotein (VLDL) production, iii) oxidative stress marked by reactive oxygen species (ROS), and iv) endoplasmic reticulum (ER) stress, promoting lipotoxicity deriving hepatic inflammation and fibrosis. The systemic “spill over” of inflammation from the liver impacts other organs, including the heart, muscles, and kidneys. This “spill over” leads to extrahepatic pathologies such as cardiovascular diseases (CVDs), muscular dystrophy, and chronic kidney disease (CKD). Additionally, visceral fat (with inflammation and fibrosis) in MASH, characterized by increased lipases activity, contributes to the systemic inflammatory responses, thereby exacerbating the cycle of metabolic dysfunction across multiple organ systems. The illustration was made on BioRender.com.
Adding to this hepatic injury, dysfunction of AT/fat deposits during obesity and type 2 diabetes mellitus (T2D) amplifies circulating free FAs and pro-inflammatory cytokines, intensifying the MASH progression and vice versa. This dysregulation perhaps extends beyond hepatic boundaries, affecting systemic metabolic processes such as glucose uptake, glycogen synthesis, glucose and fat oxidation, lipid storage, and lipolysis. Ectopic fat deposition with increased inflammation resulting from MASLD and obesity in non-AT, pose a substantial risk factor for CVDs, highlighting the severe pathogenic impact of MASH beyond the liver (Ferrara et al., 2019; Njoku et al., 2022). Defects in hepatic lipid metabolism not only precipitate intrahepatic lipid accumulation but also promotes lipid storage in non-hepatic tissues, highlighting the liver’s central role in systemic lipid homeostasis. Altered hepatic lipid metabolism under MASH conditions further predisposes individuals to atherogenic dyslipidaemia, characterized by elevated low-density lipoprotein (LDL) cholesterol levels and the ensuing formation of atherosclerotic plaques within arterial walls (Huang et al., 2023; Zhu et al., 2023). Thus, MASH is increasingly recognized not merely as a liver-specific ailment but as a systemic disorder with far-reaching effects on multiple organs. This condition is intricately linked with an elevated risk of a spectrum of severe health outcomes, including both fatal and non-fatal CVDs (64% higher risk), T2D, sarcopenia, and CKDs (Peng et al., 2022; Kawaguchi et al., 2023). The underlying pathophysiology connecting MASH to these systemic complications is multifaceted, involving metabolic dysregulation, inflammation, and altered lipid homeostasis.
The precise mechanisms underlie the causal relationship between MASH and extrahepatic diseases is not fully elucidated. However, it is likely that systemic inflammation and spill over mechanisms play a role in promoting inflammation in other tissues. Central to the systemic nature of MASH is the concept of hepatic inflammatory “spill over,” where pro-inflammatory mediators produced in the liver (Njoku et al., 2022), as a consequence of lipid accumulation and cell injury (lipotoxicity), disseminate through the bloodstream to distant organs (Buzzetti et al., 2016; Loomba et al., 2021; Zhong et al., 2024). This process is facilitated by an array of cytokines, chemokines, and other inflammatory molecules, such as TNF-α, interleukins (e.g., IL-6, IL-1β), and C-reactive protein (CRP), which are elevated in the context of MASH (Buzzetti et al., 2016; Machado and Diehl, 2016; Mitten and Baffy, 2022). These mediators can exacerbate pathological processes in the heart, skeletal muscle, and kidneys, contributing to the multisystem impact of the disease (Hong et al., 2014; Buzzetti et al., 2016; Machado and Diehl, 2016; Bhanji et al., 2017a; Mitten and Baffy, 2022). In this review, we inspect both pre-clinical and clinical evidence to refine our understanding of MASH and its wide-reaching pathological effects on critical organs such as the heart, muscles, and kidneys. A comprehensive, multi-dimensional therapeutic strategy is advocated to improve liver injury while also mitigating adverse cardiovascular and metabolic consequences. This highlights the importance of adopting a holistic/integrated management approach to address the systemic manifestations of MASH.
Cardiovascular complications in MASLD/MASH
MASLD and MASH are increasingly recognized for its systemic implications that exceeds hepatic boundaries. The pathophysiological nexus between MASH and a constellation of extrahepatic conditions, notably CVDs, manifests as a multifaceted challenge in clinical management (Ferrara et al., 2019; Zhu et al., 2023). CVDs are the predominant cause of mortality in patients with MASH, outpacing liver-centric complications and malignancies (Le et al., 2023; Choe et al., 2024). This complex relationship between MASH and cardiovascular morbidity is underscored by shared and interlinked risk factors, including but not limited to IR, T2D, hypertension, dyslipidaemia, metabolic syndrome, and notably, liver fibrosis (Houghton et al., 2019; Ismaiel and Dumitrascu, 2019). Furthermore, the relationship between the likelihood of developing cardiovascular abnormalities and the severity of hepatic fibrosis in MASH is strongly correlated (Yang et al., 2022; Wegermann et al., 2023).
The spectrum of cardiovascular complications associated with MASH is broad, encompassing coronary artery diseases (CAD), atherosclerosis (ASCVD), cardiac remodelling anomalies, hypertrophy, heart failure, and arrhythmias. The precise mechanisms by which MASH contributes to cardiovascular pathology are not fully understood. However, emerging evidence suggests that hepatic fat accumulation serves as a predictor for impaired myocardial metabolism and subsequent cardiac dysfunction (Ferrara et al., 2019; Lim et al., 2019; Njoku et al., 2022; Younossi et al., 2024a; Wang et al., 2024). Furthermore, MASLD is strongly associated with structural and functional cardiac abnormalities, such as left ventricular hypertrophy, enhanced epicardial fat thickness, and various arrhythmogenic manifestations, likely stemming from the liver’s pivotal role in systemic glucose and lipid homeostasis (Ismaiel and Dumitrascu, 2019; Patel and Siddiqui, 2019; Mantovani et al., 2022).
MASH-associated atherosclerotic risk
Independent of traditional cardiovascular risk factors, MASLD/MASH constitutes a significant risk factor for atherosclerotic cardiovascular diseases (ASCVD) (Pais et al., 2019; Arslan and Yenercag, 2020). The onset of MASH is correlated with an elevated production and release of LDL and VLDL particles from the liver during hepatic steatosis. These circulating LDL and VLDL particles in blood then accumulate beneath the arterial lining, leading to the formation of atherosclerotic plaque (Siddiqui et al., 2015; Sinha et al., 2018). Increasingly, research studies have revealed a notable correlation between hepatic steatosis and an augmented vulnerability to subclinical atherosclerosis (Yan et al., 2023; Zhu et al., 2023). Moreover, liver’s exacerbated secretion of pro-inflammatory cytokines, vasoactive substances, and pro-coagulant molecules further scaffolds the cardiovascular disease architecture in MASH patients (Garbuzenko, 2022; Huang et al., 2022).
More commonly, MASH is closely linked to the elevated levels and interactions of various cytokines and chemokines, which orchestrate an inflammatory response that directly impairs endothelial dysfunction during atherosclerosis. Among the key players, Tumor Necrosis Factor-alpha (TNF-α) and Interleukin-6 (IL-6) are central to this process. TNF-α exacerbates endothelial dysfunction by inhibiting nitric oxide (NO) synthesis, a crucial regulator of vascular tone and endothelial health, and by inducing the expression of vascular cell adhesion molecules that promote leukocyte adherence and vascular inflammation (Dimitroglou et al., 2023). IL-6, while possessing both pro- and anti-inflammatory properties, in the context of MASH tends to promote inflammation, contributing to vascular permeability and endothelial activation, thereby facilitating atherosclerosis (Zegeye et al., 2018). Further compounding the endothelial response are Interleukin-1β (IL-1β) and Interleukin-18 (IL-18), which potentiate the inflammatory milieu and are implicated in the upregulation of adhesion molecules and the recruitment of inflammatory cells to endothelial sites, promoting plaque formation and vascular stiffness (Krishnan et al., 2014). Additionally, C-reactive protein (CRP), an acute-phase reactant upregulated in response to IL-6, indirectly influences endothelial function by decreasing NO availability and promoting endothelin-1 production, a potent endothelial activator and vasoconstrictor (Pasceri et al., 2000). Furthermore, monocyte chemoattractant proteins (MCPs), particularly MCP-1, and the C-X-C motif chemokine ligands (CXCLs) like CXCL8 and CXCL12, also play pivotal roles. MCP-1 recruits monocytes to the endothelium, facilitating their transformation into macrophages and foam cells, a key step in atherogenesis (Medrano-Bosch et al., 2023; Su et al., 2023). CXCL8 (IL-8), known for its potent chemotactic abilities, further recruits neutrophils and exacerbates local inflammation, while CXCL12 is involved in stem cell recruitment and tissue repair, highlighting a complex balance of detrimental and potentially reparative mechanisms within the inflamed vasculature (Schober, 2008). Thus, elucidating these molecular interactions offers crucial insights into the pathophysiological mechanisms underlying endothelial dysfunction in MASH. Additionally, quantifying these cytokines may serve as biomarkers for assessing the increased risk of atherosclerotic cardiovascular diseases (ASCVD) associated with MASH.
Moreover, carotid intima-media thickness (CIMT), a non-invasive ultrasound measure of the combined thicknesses of the intimal and medial layers of the carotid artery wall, stands as a validated biomarker for subclinical atherosclerosis (Ismaiel and Dumitrascu, 2019; Ren et al., 2023). A substantial correlation between CIMT and the severity of MASH has been documented, implicating hepatic pathology in MASH as a potential precursor of heightened cardiovascular risk (Ismaiel and Dumitrascu, 2019; Ren et al., 2023). This observed association extends to the severity of histological features within MASLD and MASH, with more pronounced CIMT corresponding to increasingly severe liver pathology (Targher et al., 2006; Nahandi et al., 2014). This implies a potential bidirectional influence, wherein systemic inflammation and metabolic dysregulation inherent to MASH exacerbate vascular pathology and vice versa.
Furthermore, laboratory markers such as gamma-Glutamyltransferase (GGT) and alanine aminotransferase (ALT) have emerged as potential indicators of increased CIMT in MASH patients, highlighting the systemic nature of the disease and its impact on vascular health (Targher et al., 2006). Despite these insights, the pathophysiological mechanisms underpinning the relationship between MASH and ASCVD remains unclear. Factors such as the direct impact of hepatic steatosis on endothelial function, the role of liver-derived proinflammatory cytokines in vascular inflammation, and the contribution of metabolic dysregulation to atherogenesis are areas of ongoing investigation (VanWagner, 2018; Muzurovic et al., 2022). Further, the relationship between MASH and carotid artery disease is becoming more substantiated by a growing body of scientific/clinical evidence (Pais et al., 2019; Huang et al., 2022). Carotid artery disease, characterized by the narrowing or blockage of the carotid arteries which are pivotal for cerebral blood supply (Ratchford and Evans, 2014), has been observed with greater prevalence among individuals diagnosed with MASLD and MASH (Simons et al., 2022; Tang et al., 2022). This association between MASH and carotid artery disease involves various risk factors such as elevated body mass index (BMI), active smoking, elevated levels of LDL, IR, and the presence of metabolic syndrome, contributing to carotid artery disease pathogenesis in MASH (Peng et al., 2020; Katsiki et al., 2021; Zhao J. et al., 2022; Ren et al., 2023). Thus, strategies for accurately evaluating atherosclerosis risk in MASH patients are yet to be established.
MASH-associated coronary microvascular dysfunction
Coronary microvascular dysfunction represents a critical facet of cardiovascular pathology in patients with MASLD and MASH, delineating a spectrum of abnormalities that include impaired endothelial function, reduced coronary artery flow reserve, and compromised collateral vessel formation in response to ischemia (Villanova et al., 2005). Coronary microvascular dysfunction in MASH encompasses a pattern of detrimental effects on the coronary microcirculation, not limited to the endothelial layer but extending to the smooth muscle cells that regulate vascular tone and, consequently, myocardial blood flow and ultimately resulting in myocardial ischemia (Camici and Crea, 2007; Yang et al., 2023). Impaired endothelial function, a hallmark of this dysfunction, results from endothelial cells’ reduced capacity to produce NO, a potent vasodilator, in response to stimuli which required to prevent ischemic injury (Yang et al., 2023). This impairment is closely linked to the systemic inflammatory state and IR inherent to MASH, which also predispose to the formation of coronary plaques that are particularly prone to rupture, further exacerbating the risk of acute coronary events (Del et al., 2021).
Coronary flow reserve (CFR), the ratio of maximal flow to resting flow in the coronary circulation, emerges as a pivotal measure in this context. A reduced CFR signifies an inability to sufficiently increase blood flow to meet myocardial demands during stress, indicating both epicardial stenosis and microvascular myocardial perfusion abnormalities (Villanova et al., 2005; Murthy et al., 2011). Advanced imaging techniques, such as positron emission tomography/computed tomography (PET/CT) scans, enable the quantitative assessment of myocardial perfusion imaging (MPI), offering an integrated view of the heart’s vascular function (Murthy et al., 2011). This approach underscores the systemic impact of MASH on cardiovascular health by providing insights into the compromised myocardial perfusion characteristic of coronary microvascular dysfunction. The presence of both coronary microvascular and diastolic dysfunctions has been associated with an increased risk of developing heart failure with preserved ejection fraction (HFpEF) events (Taqueti et al., 2018).
MASH-associated risk of heart failure with preserved ejection fraction
The complex link between coronary microvascular dysfunction and HFpEF development in MASH patients is of growing interest. HFpEF, featuring heart failure symptoms alongside normal ejection fraction, is increasingly common in those with metabolic syndrome and MASH, primarily due to diastolic dysfunction and altered filling pressures (Yang et al., 2022; Wegermann et al., 2023). This condition constitutes a significant portion of heart failure cases, notably in MASH-related metabolic derangements. Clinical studies reveal pathophysiological pathways connecting MASH to HFpEF, emphasizing systemic inflammation, endothelial dysfunction, and myocardial fibrosis, leading to impaired heart muscle relaxation and elevated filling pressures, hallmarks of diastolic dysfunction. Paulus and Tschöpe (2013) propose an inflammatory HFpEF model, where comorbidities like MASH trigger systemic microvascular endothelial inflammation, resulting in coronary microvascular dysfunction, myocardial stiffness from fibrosis, and cardiomyocyte remodelling. Mohammed et al. (2014) demonstrate significant diastolic dysfunction in metabolic syndrome patients, similar to those with MASH, correlating with metabolic derangement severity, indicating a direct link between metabolic syndrome/MASH and HFpEF.
HOMAGE (Heart “Omics” in AGEing) research elucidated biomarkers’ predictive value for HFpEF, including in those with MASH. Elevated NT-proBNP and galectin-3 levels, indicative of myocardial stress and fibrosis, forecasted HFpEF development in metabolic disorder patients (Jacobs et al., 2014). Advanced imaging like cardiac magnetic resonance imaging (CMRI) revealed increased left ventricular mass, myocardial fibrosis, and impaired strain as HFpEF predictors in MASH patients (Wegermann et al., 2023). These findings underscore the intertwined coronary microvascular and diastolic dysfunctions’ role in MASH-related HFpEF pathogenesis, advocating for holistic cardiovascular assessment and management. Early interventions addressing cardiovascular risks in MASH may potentially curb HFpEF progression, emphasizing integrated care strategies for metabolic dysfunction’s hepatic and cardiac dimensions.
MASH-associated cardiac structural and functional alterations
MASH is linked to cardiac structural and functional changes, extending its negative impact beyond hepatic dysfunction to significant cardiovascular implications. Ballestri et al. showed increased left ventricular mass and impaired diastolic function in MASLD patients (Ballestri et al., 2014), while van der Meer et al. found myocardial triglyceride accumulation associated with reduced left ventricular (LV) diastolic function in metabolic syndrome patients (van der Meer et al., 2008a; van der Meer et al., 2008b). Moreover, studies have reported changes such as increased diastolic posterior-wall thickness, LV mass, relative wall thickness and left atrial volume, (Chang et al., 2019; Ismaiel and Dumitrascu, 2019). Additionally, variations in ejection fraction, tissue Doppler imaging results, and the E/A ratio have been observed, indicating significant cardiac involvement in patients with MASLD (Petta et al., 2015; Lee et al., 2018). Magnetic resonance imaging (MRI) has further substantiated these findings, revealing noteworthy alterations in cardiac structure and function in individuals with MASLD, irrespective of the presence of clinically evident cardiac disease (Hallsworth et al., 2013). These findings highlight metabolic dysregulation’s direct effect on cardiac tissue, even in MASLD patients without major risk factors (Chang et al., 2019). Moreover, increased epicardial fat thickness has been linked to cardiac dysfunction via pro-inflammatory cytokine production (Petta et al., 2015). Further, the association between MASLD and a higher prevalence of atrial fibrillation highlights the potential for inflammatory milieu of MASH to foster electrical remodelling of the atria (Mantovani et al., 2019; Mantovani et al., 2022). Epidemiological studies also have drawn attention to the subtle yet persistent elevations in serum liver enzymes among MASLD patients, positioning these markers as predictive of the development of heart failure (Wannamethee et al., 2012; Wang et al., 2013). This correlation highlights the broader systemic consequences of liver pathology on cardiovascular health.
Compelling evidence links MASLD/MASH to a wide range of cardiovascular complications, identifying it as a critical risk factor for ASCVD, CADs, and alterations in LV function and structure. MASLD/MASH patients are notably at risk for carotid artery obstructions and exhibit significant changes in cardiac geometry and function, which, combined with impaired endothelial function and coronary plaque formation, significantly heighten cardiovascular event risks, including heart failure. The pathogenesis involves increased release of pro-inflammatory cytokines and LDL/VLDL from the liver, alongside risk factors like IR and dyslipidaemia. Cardiac assessments, particularly CFR measurements, are vital for identifying cardiovascular risks in MASLD/MASH patients. Despite well-established connections between MASH and cardiovascular disease, further research is crucial for understanding these complex interactions and improving management strategies, emphasizing the need for integrated cardiac and liver disease care to mitigate cardiovascular risks and enhance patient outcomes.
Muscular complication in MASLD/MASH
Muscular tissue constitutes approximately 40% of total body weight and accounts for 50%–75% of all body proteins (Frontera and Ochala, 2015). It plays a pivotal role in metabolic processes, particularly in insulin-dependent glucose metabolism and fatty acid oxidation (Holecek, 2021). Functioning as an active endocrine entity, skeletal muscle influences inflammation regulation through the secretion of signalling molecules known as myokines (Hoffmann and Weigert, 2017). The integrity of muscle mass is crucial for maintaining muscle power, strength, and endurance, which collectively determine overall muscle performance. Hepatic steatosis disrupts the liver-muscle axis, initiating a detrimental cycle where liver disease impairs muscle protein synthesis and exacerbates metabolic imbalances, further fuelling MASLD and systemic inflammation (Bhanji et al., 2017a). This cycle is compounded by anabolic resistance in individuals with liver cirrhosis, a condition that diminishes skeletal muscle’s capacity to synthesize protein in response to nutrient intake, often leading to sarcopenia (Roman et al., 2014). Mechanistically, MASLD/MASH are associated with significant hormonal changes that impact muscle protein synthesis and degradation. Insulin-like growth factor-1 (IGF-1) levels are notably reduced in MASLD/MASH patients. IGF-1, produced mainly by the liver, plays a crucial role in muscle protein synthesis through the activation of the PI3K/Akt signaling pathway, which promotes muscle growth and inhibits protein degradation (Yoshida and Delafontaine, 2020). Conversely, myostatin, a negative regulator of muscle growth, is often elevated in MASLD/MASH (Meng et al., 2016). Myostatin inhibits muscle differentiation and protein synthesis by activating the SMAD2/3 signaling pathway, leading to muscle atrophy (Rodriguez et al., 2014). Increased myostatin levels are correlated with higher muscle protein degradation and reduced muscle mass in these patients (Yarasheski et al., 2002).
Additionally, alterations in adiponectin and leptin levels are observed in MASLD/MASH patients (Valenzuela-Vallejo et al., 2023; Venkatesh et al., 2024). Adiponectin, typically reduced in these conditions, has anti-inflammatory and insulin-sensitizing effects that support muscle protein synthesis (Yadav et al., 2013). Low adiponectin levels exacerbate muscle insulin resistance and protein breakdown. In contrast, leptin levels are often elevated and, while leptin promotes muscle protein synthesis in physiological conditions, chronic hyperleptinemia in MASLD/MASH can lead to leptin resistance, impairing its beneficial effects on muscle metabolism (Yadav et al., 2013; Polyzos et al., 2015). The hormonal imbalances in MASLD/MASH disrupt muscle protein synthesis and degradation, leading to sarcopenia and muscle wasting, highlighting the extensive impact on skeletal muscle metabolism and structural integrity.
Sarcopenia in MASLD/MASH
Sarcopenia, characterized by the loss of muscle mass and function, emerges as a prevalent complication within MASLD, affecting up to 60% of patients with end-stage liver disease (ESLD) (Bhanji et al., 2017a; Bhanji et al., 2017b). This condition, beyond being a mere consequence of aging, is increasingly recognized as a progressive disease linked with higher risks of obesity, T2D, osteoporosis, CVDs, and cancer (Purnamasari et al., 2022; Yu et al., 2022; Damluji et al., 2023). The loss of muscle mass and strength in sarcopenia critically challenges physical performance and poses significant health risks, including increased disability, frailty, and mortality (Santilli et al., 2014; Lai et al., 2021). Sarcopenia shares pathophysiological pathways with MASLD, including metabolic dysfunction, hormonal imbalances, altered gut microbiome, and systemic inflammation (Lee et al., 2015; Petta et al., 2017; Wijarnpreecha et al., 2019). This multifaceted interaction contributes to a cycle where liver disease exacerbates muscle protein breakdown and inhibits synthesis, leading to decreased muscle mass and the onset of sarcopenia (Meyer et al., 2020). Factors such as gluconeogenesis, oxidative stress, mitochondrial dysfunction, and anabolic resistance play critical roles in this process, further complicated by the systemic effects of IR, adiposopathy, and hyperammonaemia (Walker, 2014; Holecek, 2021).
IR, a hallmark of MASH, disrupts normal insulin signalling, contributing to muscle loss, while adiposopathy promotes inflammatory pathways that aggravate sarcopenia through mechanisms like TNFα activation and myostatin release, inhibiting muscle protein synthesis (Merz and Thurmond, 2020; Sethi and Hotamisligil, 2021). Furthermore, NF-κB exacerbates sarcopenia by facilitating dyslipidaemia, while the accumulation of AT within skeletal muscles, referred to as myosteatosis, emerges as an additional outcome of adiposopathy (Gumucio et al., 2019; Joo and Kim, 2023). This condition notably impairs muscle strength and functionality, positioning myosteatosis as a critical indicator for both MASH and sarcopenia. MASH contributes to a reduction in muscle mass coupled with an escalation in fat accumulation, a phenomenon known as sarcopenic obesity (Gumucio et al., 2019; Nachit et al., 2021). In populations with chronic MASLD, statistics reveal that 43% exhibit sarcopenia, 26% are affected by sarcopenic obesity, and 52% display myosteatosis (Montano-Loza et al., 2016; Kang and Yoon, 2023). Additionally, vitamin D deficiency and low testosterone levels have been implicated in the pathology of sarcopenia within the MASLD context, suggesting potential therapeutic targets (Dhindsa et al., 2018; Zhang et al., 2022). Despite growing awareness, gaps in understanding the precise mechanisms and effective management strategies for sarcopenia in MASLD patients persist, necessitating further research to elucidate the contributions of MASH to sarcopenia and develop comprehensive treatment approaches.
MASLD-associated inflammatory myopathies
Emerging evidence also points to the association of MASH with inflammatory myopathies, muscle disorders marked by skeletal muscle inflammation (Lundberg et al., 2021). Although traditionally linked to autoimmune conditions like dermatomyositis and polymyositis, MASH-related systemic inflammation has been implicated in muscle abnormalities’ development and progression (Bian et al., 2013; Chaudhry et al., 2019). Immune cell infiltration into skeletal muscles, accompanied by pro-inflammatory cytokine release, creates a local inflammatory milieu conducive to muscle damage, associated with MASH-related inflammatory “spill over” (Pillon et al., 2013). Inflammatory myopathies in MASLD may involve autoimmune responses, with autoantibodies and immune complexes contributing to muscle inflammation and damage (Lian et al., 2022). This process can lead to muscle fibre degeneration, manifesting as muscle weakness, fatigue, and impaired function, and complicating muscle repair and regeneration due to persistent inflammation (Mounier et al., 2013). Shared pathophysiological pathways between MASH and inflammatory myopathies involve chronic inflammation, immune dysregulation, and oxidative stress, contributing to muscle damage and inflammation. While the association between MASH and inflammatory myopathies is recognized, further research is warranted to comprehend underlying mechanisms and clinical implications. Identifying and addressing muscle inflammation in MASH may have therapeutic benefits by reducing systemic inflammation and immune dysregulation, potentially improving muscle function and overall disease outcomes.
Renal complications in MASLD/MASH
Renal complications, particularly chronic kidney disease (CKD), are integral to the systemic impact of MASLD/MASH, underscoring the complex interplay of metabolic disorders (Byrne and Targher, 2020; Cao et al., 2021; Umbro et al., 2021). CKD, characterized by progressive renal function loss, involves waste product accumulation and disruptions in fluid and electrolyte balances, often evidenced by biomarkers indicating kidney damage or reduced glomerular filtration rate (GFR) (Byrne, 2013; Byrne and Targher, 2020). In the United States, the prevalence of individuals necessitating renal replacement therapy exceeds 400,000, with projections indicating a surge to 2.2 million by 2030, highlighting the escalating burden of kidney disease (Marcuccilli and Chonchol, 2016). Emerging clinical research has identified MASLD/MASH as significant independent predictors for both the onset and progression of CKD, suggesting a profound link between hepatic steatosis and renal dysfunction (Byrne, 2013; Byrne and Targher, 2020). These studies elucidate that the severity of liver disease, characterized by fat accumulation and inflammation in the liver, correlates with the risk of developing kidney complications, positioning MASLD/MASH within the broader constellation of metabolic syndrome-related conditions that adversely affect renal health.
The pathophysiological bridges between MASLD/MASH and CKD are multifaceted, encompassing impaired antioxidant defences, persistent low-grade systemic inflammation, activation of the renin-angiotensin system, and aberrant lipid metabolism (Byrne and Targher, 2020; Bilson et al., 2023; Li et al., 2023). These factors collectively contribute to a milieu conducive to renal injury. Specifically, the role of pro-inflammatory, pro-fibrogenic, and anti-fibrinolytic mediators such as fetuin-A, fibroblast growth factor (FGF)-21, TNF-α, transforming growth factor (TGF)-β, and plasminogen activator inhibitor-1 (PAI-1), has been implicated in promoting kidney damage through mechanisms that include the exacerbation of inflammation and fibrosis within the renal tissue (Musso et al., 2014; Byrne and Targher, 2020). Additionally, liver-derived metabolites, including uremic toxins, play a significant role in the pathogenesis and progression of CKD. Uremic toxins such as indoxyl sulfate (IS), p-cresyl sulfate (PCS), and trimethylamine N-oxide (TMAO) are primarily generated in the liver through the metabolism of dietary components by gut microbiota, followed by hepatic processing (Zhen et al., 2023). These metabolites are recognized for their nephrotoxic effects and their contribution to CKD progression (Castillo-Rodriguez et al., 2018). Indoxyl sulfate, a protein-bound uremic toxin derived from the metabolism of tryptophan, is one of the most studied nephrotoxic metabolites. Once produced in the liver, IS poorly eliminated by the kidneys in CKD patients, leading to its accumulation. Elevated IS levels induce oxidative stress and inflammation in renal tubular cells, promoting fibrosis and accelerating the decline in renal function (Barreto et al., 2009). Moreover, IS was also found to be increased in MASLD patients (Hotamisligil, 2006; Ribeiro et al., 2023). Mechanistically, IS activates the aryl hydrocarbon receptor (AhR) pathway and induces the expression of TGF-β and pro-inflammatory cytokines, thereby exacerbating renal injury (Delgado-Marin et al., 2024). Similarly, PCS, a metabolite of tyrosine, has been implicated in CKD progression through mechanisms akin to those of IS. PCS induces endothelial dysfunction and increases vascular permeability, contributing to renal damage and cardiovascular complications commonly seen in CKD patients (Meijers et al., 2010). Additionally, PCS has been shown to inhibit the proliferation and repair of renal epithelial cells, further impairing renal function (Han et al., 2015).
TMAO, another liver-derived metabolite, originates from the hepatic oxidation of trimethylamine, a product of gut microbial metabolism of choline, phosphatidylcholine, and carnitine. Elevated TMAO levels are associated with adverse renal outcomes, including glomerular sclerosis and interstitial fibrosis. TMAO enhances renal oxidative stress and inflammatory responses, contributing to the progression of CKD (Tang et al., 2015). Moreover, TMAO has been linked to the upregulation of pro-fibrotic and pro-inflammatory genes, thereby exacerbating renal fibrosis and dysfunction (Zhang et al., 2020). These liver-derived metabolites, by inducing oxidative stress, inflammation, and fibrosis, significantly contribute to the nephrotoxic milieu in CKD.
Despite the accumulating evidence of a link between MASH and CKD, establishing a definitive causal relationship remains challenging. The complexity of metabolic syndrome, with its array of cardiovascular, hepatic, and renal manifestations, necessitates a holistic understanding of these interconnected systems. Further research, employing longitudinal studies and advanced biomolecular techniques, is required to dissect the intricate mechanisms by which MASLD/MASH contributes to the development and progression of CKD. Such insights will be crucial for devising targeted therapeutic interventions aimed at mitigating renal complications in patients with metabolic liver disease, thereby addressing an important component of the morbidity and mortality associated with this condition.
Pathophysiological links between MASLD/MASH and extrahepatic complications
The pathophysiological interrelation between MAFLD or MASH and its extraneous manifestations, notably CVDs, CKD and muscular anomalies, constitutes a multidimensional domain marked by inflammation and oxidative stress, perturbations in gut microbiota composition, and dyslipidaemia. Each component intricately contributes to this complex network of pathogenesis.
Inflammation and fibrosis
Metabolic inflammation in MASH is marked by a systemic, low-grade inflammatory response triggered by factors like high-fat diets. This systemic inflammation is facilitated by the infiltration of inflammatory cells such as macrophages and lymphocytes into the liver, releasing pro-inflammatory cytokines that contribute to liver damage (Buzzetti et al., 2016; Bhanji et al., 2017a; Boesch et al., 2023). Kupffer cells, the liver’s resident macrophages, are central to orchestrating liver inflammation (Jensen et al., 2018; Cusi et al., 2024). The crosstalk between hepatocytes and immune cells, mediated by inflammasomes, plays a crucial role in this process (Fontes-Cal et al., 2021; Duan et al., 2022). Inflammation leads to fibrosis, an abnormal wound healing response characterized by the deposition of extracellular matrix proteins and formation of scar tissue, potentially progressing to cirrhosis (Iwakiri and Trebicka, 2021; Zhao X. et al., 2022). Cirrhosis increases resistance in hepatic vasculature, leading to portal hypertension, collateral vessel formation, and increased cardiac workload, potentially resulting in heart failure (Gonzalez et al., 2020; Iwakiri and Trebicka, 2021; Umbro et al., 2021).
Insulin resistance
A key feature of MASLD and MASH, IR disrupts glucose homeostasis, promoting fat accumulation in the liver and progressing from simple steatosis to MASH and potentially to cirrhosis and liver failure (Llovet et al., 2023; Cusi et al., 2024). IR fosters inflammation and oxidative stress, contributing to liver cell injury and disease progression (Loomba et al., 2021; Muzurovic et al., 2022; Njoku et al., 2022). The complex mechanisms underlying IRinvolve genetic factors, obesity, inflammation, lipotoxicity, mitochondrial dysfunction, and hormonal imbalances (Powell et al., 2021; Salah et al., 2021; Tripathi et al., 2022; Wong et al., 2023).
Gut microbiota
Dysbiosis, or the imbalance of gut microbiota, has been increasingly recognized for its role in MASH and CVD (Brody, 2020; Violi et al., 2023). Increased gut permeability, or “leaky gut,” allows toxins to reach the liver, exacerbating inflammation and damage (Brody, 2020; Song and Zhang, 2022; Violi et al., 2023). Endotoxins from gut bacteria, such as lipopolysaccharides (LPS), stimulate inflammation, contributing to liver injury (Solanki et al., 2023; Violi et al., 2023). Alterations in gut microbiota also affect bile acid metabolism, influencing liver inflammation and metabolism (Xiang et al., 2022). Therapeutic modulation of gut microbiota through probiotics, prebiotics, dietary changes, short-chain fatty acids (SCFAs) or faecal microbiota transplantation has shown promise in improving MASH outcomes (Philips et al., 2020; Nogal et al., 2021; Xiang et al., 2022).
Dyslipidaemia
Characterized by abnormal levels of blood lipids, dyslipidaemia is a crucial risk factor for CVD, ASCVD, CAD and CKD (Linton et al., 2000; Stasi et al., 2022). High LDL cholesterol and triglycerides contribute to atherosclerosis, while low HDL cholesterol is linked to increased cardiovascular risk (Linton et al., 2000). Dyslipidaemia not only contributes to MASH development but also exacerbates the condition, necessitating comprehensive management to mitigate cardiovascular and liver damage risks (Mendez-Sanchez et al., 2020).
Genetics
Genetic predispositions play a significant role in the susceptibility to MASLD and its progression (Younossi et al., 2023). Variants in genes related to lipid metabolism (e.g., PNPLA3, TM6SF2), inflammation, oxidative stress, and fibrosis have been implicated in increasing the risk of developing MASLD (Anstee et al., 2020; Sveinbjornsson et al., 2022; Xie et al., 2023). These genetic factors affect liver fat processing and storage, lipid metabolism, and the inflammatory response, underscoring the genetic complexity of MASLD and its systemic manifestations.
Hepatokines
Unlike liver-derived metabolites, hepatokines are the molecular transducers with hormone-like activities (Seo et al., 2021). These are central to the inter-organ communication that regulates metabolic homeostasis (Stefan et al., 2023). These molecules are implicated in a variety of physiological processes including glucose and lipid metabolism, inflammation, and energy homeostasis (Jensen-Cody and Potthoff, 2021; Khan et al., 2022; Stefan et al., 2023). As metabolic regulators, hepatokines provide a mechanistic link between liver function and metabolic disorders such as diabetes, obesity, CVDs, CKD, and sarcopenia. Some previous reports have reviewed these hepatokines excellently (de Oliveira Dos Santos et al., 2021; Jensen-Cody and Potthoff, 2021; Khan et al., 2022; Berezin et al., 2023; Stefan et al., 2023). Few of the important hepatokines are summarized in Table 1 with their target organs, and their physiological roles. Mechanistically, hepatokines exert their effects through autocrine, paracrine, or endocrine mechanisms, influencing not only the liver itself but also distant organs. These effects can be either beneficial, such as improving insulin sensitivity, or detrimental, such as promoting IRand inflammation depending on the pathological state of the organism. For instance, fibroblast growth factor 21 (FGF21), which is a stellar hepatokine among others, modulates glucose and lipid metabolism in cardiac and muscle tissues (Jimenez et al., 2018; Zhang et al., 2019). The extensive influence of hepatokines on multiple organ systems highlights their potential as targets for therapeutic intervention in metabolic diseases. Understanding the specific roles of hepatokines not only aids in translating the pathophysiology of metabolic disorders but also opens new ways for treatment, such as the use of recombinant FGF21 or its analogs for CVDs, CKD and muscle disorders during MASH (Sunaga et al., 2019). Future research focusing on the interaction between hepatokines and their receptors may provide novel insights into their mechanistic pathways and therapeutic potential.
The association between MASH and its extrahepatic manifestations, especially CVDs and CKD, is reinforced by complex pathophysiological mechanisms. While inflammation, fibrosis, IR, dysbiosis, dyslipidaemia, and genetic factors each contribute to the disease process, their interplay exacerbates the systemic impact of MASH. Understanding these mechanisms is crucial for developing targeted therapeutic strategies to manage MASLD and its broad spectrum of complications. Further research is needed to elucidate these complex interactions fully and identify effective interventions for patients with MASH.
Multidisciplinary and innovative therapeutic strategies for managing MASH and associated extrahepatic manifestations
The management of MASH and its associated systemic/extrahepatic manifestations, including CVDs, sarcopenia, and CKDs, demands a holistic and multidisciplinary approach (Figure 3). Collaboration among hepatologists, endocrinologists, dietitians, and exercise specialists are essential to address the multifaceted nature of the disease comprehensively. Tailoring treatment plans to individual patient profiles, considering disease severity and comorbidities, is paramount for optimizing health outcomes. Lifestyle interventions, focusing on diet, exercise, and weight management, serve as the cornerstone of MASH treatment (Machado, 2021; Semmler et al., 2021; Viveiros, 2021), aiming to mitigate metabolic derangements, reduce hepatic fat accumulation, enhance insulin sensitivity, and alleviate inflammation and oxidative stress. For example, caloric restriction and adherence to a Mediterranean-style diet have shown promise in diminishing hepatic fat and improving liver health, while aerobic and resistance training exercises enhance insulin sensitivity and support weight loss (Hallsworth et al., 2011; Anania et al., 2018; Ristic-Medic et al., 2020). Addressing micronutrient imbalances, such as deficiencies in vitamins B12, folate, and potentially beneficial supplements like vitamin E and omega-3 fatty acids, may offer therapeutic benefits by modulating hyperhomocysteinemia, inflammation, oxidative stress, and liver fibrosis (Calder, 2010; Koplay et al., 2011; Ryan et al., 2013; Mahamid et al., 2018; Musa-Veloso et al., 2018; Zaric et al., 2019; Levy et al., 2021; Tripathi et al., 2022).
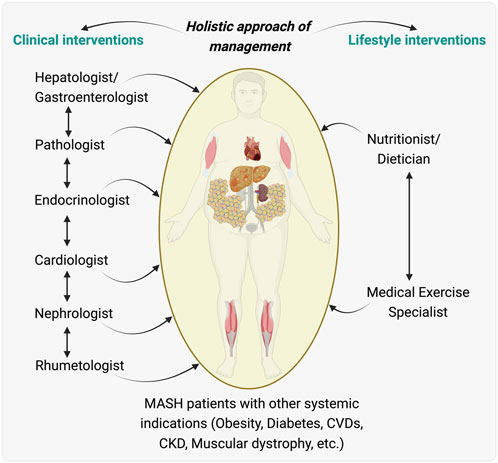
Figure 3. An Integrated holistic care model for managing patients with metabolic associated steatohepatitis (MASH) and other MASH-associated effects. Illustration proposed an integrated care model for patients with MASH who also have systemic indications such as obesity, diabetes, cardiovascular diseases (CVDs), chronic kidney disease (CKD), and muscular dystrophy. Clinical interventions proposed are outlined, showing a flow of care from a hepatologist/gastroenterologist to a team including a pathologist, endocrinologist, cardiologist, nephrologist, and rheumatologist, indicating the need for collaborative medical management across different organ systems affected during/by MASH. Lifestyle interventions are highlighted, with a nutritionist/dietitian and a medical exercise specialist playing key roles in managing the patient’s nutrition and physical activity, essential for the overall treatment strategy. This model highlights the importance of a multidisciplinary integrative team working in concert to provide comprehensive care that addresses the multifaceted aspects of MASH and its related systemic diseases. The illustration was made on BioRender.com.
Emerging therapeutic targets and pharmacological interventions represent significant areas of active research. The exploration of thyromimetics, which mimic thyroid hormone actions to regulate metabolism, demonstrates potential in ameliorating MASH-related liver damage, inflammation, and fibrosis, as evidenced by clinical trials with agents like resmetirom (Sinha et al., 2020). Similarly, agents targeting metabolic pathways, such as GLP-1 receptor agonists and PPARα agonists, are under investigation for their capacity to correct metabolic abnormalities integral to MASH pathophysiology (Zhu et al., 2021; Zhang et al., 2023). Moreover, the complex interplay between MASH and gut microbiota suggests that modulating the gut microbiome through probiotics, prebiotics, or faecal microbiota transplantation could offer a novel avenue for treatment, addressing dysbiosis and its contributions to liver inflammation and damage (Xiang et al., 2022; Solanki et al., 2023).
Given MASH’s association with metabolic disturbances, combination therapies targeting various aspects of the disease process, ranging from inflammation and fibrosis to metabolic dysfunctions, are likely to emerge as a promising strategy. The synergy between pharmacological agents with complementary mechanisms of action could enhance treatment efficacy, potentially offering a more comprehensive approach to managing MASH and its complications. However, the path to optimizing MASH management extends beyond current therapeutic modalities. Future research directions include the identification of specific molecular and genetic markers to guide personalized treatment strategies, the utilization of non-invasive imaging techniques for liver assessment, and the exploration of novel therapeutic targets. Such advancements are anticipated to refine therapeutic interventions, improve prognostication, and ultimately enhance the quality of life for patients with MASH.
Conclusion
The management of MASH necessitates a comprehensive, personalized strategy that encompasses lifestyle interventions, pharmacological measures, and emerging therapeutic agents to address both hepatic and systemic manifestations. Central to this approach is the recognition of MASH as a systemic disorder with significant impacts on cardiovascular health, skeletal muscle function, and renal integrity. The interconnections between MASH and its extrahepatic effects underline the importance of a multidisciplinary care model, emphasizing early detection, precise risk assessment, and tailored treatment plans that mitigate the progression of associated conditions. Key lifestyle modifications such as dietary adjustments, increased physical activity, and weight management form the cornerstone of MASH management, targeting causal metabolic dysfunctions including IR and dyslipidaemia. Additionally, addressing chronic inflammation and oxidative stress through targeted pharmacological interventions is critical for ameliorating the broader health impacts of MASH. As research advances, the potential for novel therapies targeting specific pathophysiological pathways offers hope for more effective and individualized treatments, aiming to improve overall patient outcomes and reduce the burden of complex MASLD and MASH. Future research directions should concentrate on unveiling new therapeutic targets, adopting precision medicine strategies, and understanding the long-term effects of MASH on organ health to optimize care for affected individuals.
Author contributions
RS: Writing–original draft, Writing–review and editing. SS: Writing–original draft, Writing–review and editing. PG: Writing–original draft, Writing–review and editing. JB: Writing–original draft, Writing–review and editing. MT: Writing–original draft, Writing–review and editing, Conceptualization, Funding acquisition. BS: Conceptualization, Funding acquisition, Writing–original draft, Writing–review and editing, Resources, Supervision.
Funding
The author(s) declare that financial support was received for the research, authorship, and/or publication of this article. Ministry of Health (MOH), and National Medical Research Council (NMRC), Singapore, grant number NMRC/OFYIRG/0002/2016 and MOH-000319 (MOH-OFIRG19may-0002), Duke/Duke-NUS Research Collaboration Pilot Project Award (Duke/Duke-NUS/RECA(Pilot)/2022/0060), and KBrFA (Duke-NUS-KBrFA/2023/0075) to BKS and NMRC/OFYIRG/077/2018 to MT.
Acknowledgments
The authors like to acknowledge that the research is funded by the Ministry of Health (MOH), and National Medical Research Council (NMRC), Singapore, grant number NMRC/OFYIRG/0002/2016 and MOH-000319 (MOH-OFIRG19may-0002), Duke/Duke-NUS Research Collaboration Pilot Project Award (Duke/Duke-NUS/RECA(Pilot)/2022/0060), and KBrFA (Duke-NUS-KBrFA/2023/0075) to BKS and NMRC/OFYIRG/077/2018 to MT.
Conflict of interest
The authors declare that the research was conducted in the absence of any commercial or financial relationships that could be construed as a potential conflict of interest.
The author(s) declared that they were an editorial board member of Frontiers, at the time of submission. This had no impact on the peer review process and the final decision.
Publisher’s note
All claims expressed in this article are solely those of the authors and do not necessarily represent those of their affiliated organizations, or those of the publisher, the editors and the reviewers. Any product that may be evaluated in this article, or claim that may be made by its manufacturer, is not guaranteed or endorsed by the publisher.
Abbreviations
AT, Adipose tissue; ALT, Alanine aminotransferase; ASCVD, Atherosclerotic cardiovascular diseases; BMI, Body mass index; CRP, C-reactive protein; CMRI, Cardiac magnetic resonance imaging; CVDs, Cardiovascular diseases; CIMT, Carotid intima-media thickness; CKDs, Chronic kidney diseases; CT, Computed tomography; CAD, Coronary artery diseases; CFR, Coronary flow reserve; ESLD, End-stage liver disease; ER, Endoplasmic reticulum; FXR, Farnesoid X Receptor; FA, Fatty acids; HFpEF, Heart failure with preserved ejection fraction; IR, Insulin resistance; LV, Left ventricular; LDs, Lipid droplets; MASH, Metabolic dysfunction associated steatohepatitis; NASH, Non-alcoholic steatohepatitis; NF-κB, Nuclear factor kappa-light-chain-enhancer of activated B cells; PPAR, Peroxisome proliferator-activated receptor; PAI-1, Plasminogen activator inhibitor-1; SCFAs, Short-chain fatty acids; TGF-β, Transforming growth factor; TNFα, Tumor necrosis factor α; T2D, Type 2 diabetes mellitus; VLDL, Very-low-density lipoprotein.
References
Adler, M., and Schaffner, F. (1979). Fatty liver hepatitis and cirrhosis in obese patients. Am. J. Med. 67, 811–816. doi:10.1016/0002-9343(79)90740-x
Alonso-Pena, M., Del Barrio, M., Peleteiro-Vigil, A., Jimenez-Gonzalez, C., Santos-Laso, A., Arias-Loste, M. T., et al. (2023). Innovative therapeutic approaches in non-alcoholic fatty liver disease: when knowing your patient is key. Int. J. Mol. Sci. 24, 10718. doi:10.3390/ijms241310718
Anania, C., Perla, F. M., Olivero, F., Pacifico, L., and Chiesa, C. (2018). Mediterranean diet and nonalcoholic fatty liver disease. World J. Gastroenterol. 24, 2083–2094. doi:10.3748/wjg.v24.i19.2083
Anstee, Q. M., Darlay, R., Cockell, S., Meroni, M., Govaere, O., Tiniakos, D., et al. (2020). Genome-wide association study of non-alcoholic fatty liver and steatohepatitis in a histologically characterised cohort(☆). J. Hepatol. 73, 505–515. doi:10.1016/j.jhep.2020.04.003
Arslan, U., and Yenercag, M. (2020). Relationship between non-alcoholic fatty liver disease and coronary heart disease. World J. Clin. Cases. 8, 4688–4699. doi:10.12998/wjcc.v8.i20.4688
Ballestri, S., Lonardo, A., Bonapace, S., Byrne, C. D., Loria, P., and Targher, G. (2014). Risk of cardiovascular, cardiac and arrhythmic complications in patients with non-alcoholic fatty liver disease. World J. Gastroenterol. 20, 1724–1745. doi:10.3748/wjg.v20.i7.1724
Barreto, F. C., Barreto, D. V., Liabeuf, S., Meert, N., Glorieux, G., Temmar, M., et al. (2009). Serum indoxyl sulfate is associated with vascular disease and mortality in chronic kidney disease patients. Clin. J. Am. Soc. Nephrol. 4, 1551–1558. doi:10.2215/CJN.03980609
Berezin, A. A., Obradovic, Z., Berezina, T. A., Boxhammer, E., and Lichtenauer, M. (2023). Cardiac hepatopathy: new perspectives on old problems through a prism of endogenous metabolic regulations by hepatokines. Antioxidants (Basel) 12, 516. doi:10.3390/antiox12020516
Bhanji, R. A., Carey, E. J., Yang, L., and Watt, K. D. (2017b). The long winding road to transplant: how sarcopenia and debility impact morbidity and mortality on the waitlist. Clin. Gastroenterol. Hepatol. 15, 1492–1497. doi:10.1016/j.cgh.2017.04.004
Bhanji, R. A., Narayanan, P., Allen, A. M., Malhi, H., and Watt, K. D. (2017a). Sarcopenia in hiding: the risk and consequence of underestimating muscle dysfunction in nonalcoholic steatohepatitis. Hepatology 66, 2055–2065. doi:10.1002/hep.29420
Bian, Z., Peng, Y., You, Z., Wang, Q., Miao, Q., Liu, Y., et al. (2013). CCN1 expression in hepatocytes contributes to macrophage infiltration in nonalcoholic fatty liver disease in mice. J. Lipid. Res. 54, 44–54. doi:10.1194/jlr.M026013
Bilson, J., Mantovani, A., Byrne, C. D., and Targher, G. (2023). Steatotic liver disease, MASLD and risk of chronic kidney disease. Diabetes. Metab. 50, 101506. doi:10.1016/j.diabet.2023.101506
Boesch, M., Lindhorst, A., Feio-Azevedo, R., Brescia, P., Silvestri, A., Lannoo, M., et al. (2023). Adipose tissue macrophage dysfunction is associated with a breach of vascular integrity in NASH. J. Hepatol. 80, 397–408. doi:10.1016/j.jhep.2023.10.039
Buzzetti, E., Pinzani, M., and Tsochatzis, E. A. (2016). The multiple-hit pathogenesis of non-alcoholic fatty liver disease (NAFLD). Metabolism 65, 1038–1048. doi:10.1016/j.metabol.2015.12.012
Byrne, C. D. (2013). Ectopic fat, insulin resistance and non-alcoholic fatty liver disease. Proc. Nutr. Soc. 72, 412–419. doi:10.1017/S0029665113001249
Byrne, C. D., and Targher, G. (2020). NAFLD as a driver of chronic kidney disease. J. Hepatol. 72, 785–801. doi:10.1016/j.jhep.2020.01.013
Calder, P. C. (2010). Omega-3 fatty acids and inflammatory processes. Nutrients 2, 355–374. doi:10.3390/nu2030355
Camici, P. G., and Crea, F. (2007). Coronary microvascular dysfunction. N. Engl. J. Med. 356, 830–840. doi:10.1056/NEJMra061889
Cao, Y., Deng, Y., Wang, J., Zhao, H., Zhang, J., and Xie, W. (2021). The association between NAFLD and risk of chronic kidney disease: a cross-sectional study. Ther. Adv. Chronic. Dis. 12, 20406223211048649. doi:10.1177/20406223211048649
Castillo-Rodriguez, E., Fernandez-Prado, R., Esteras, R., Perez-Gomez, M. V., Gracia-Iguacel, C., Fernandez-Fernandez, B., et al. (2018). Impact of altered intestinal microbiota on chronic kidney disease progression. Toxins (Basel) 10, 300. doi:10.3390/toxins10070300
Chakravarthy, M. V., Siddiqui, M. S., Forsgren, M. F., and Sanyal, A. J. (2020). Harnessing muscle-liver crosstalk to treat nonalcoholic steatohepatitis. Front. Endocrinol. (Lausanne) 11, 592373. doi:10.3389/fendo.2020.592373
Chang, W., Wang, Y., Sun, L., Yu, D., and Li, G. (2019). Evaluation of left atrial function in type 2 diabetes mellitus patients with nonalcoholic fatty liver disease by two-dimensional speckle tracking echocardiography. Echocardiography 36, 1290–1297. doi:10.1111/echo.14400
Chaudhry, S., Emond, J., and Griesemer, A. (2019). Immune cell trafficking to the liver. Transplantation 103, 1323–1337. doi:10.1097/TP.0000000000002690
Choe, H. J., Moon, J. H., Kim, W., Koo, B. K., and Cho, N. H. (2024). Steatotic liver disease predicts cardiovascular disease and advanced liver fibrosis: a community-dwelling cohort study with 20-year follow-up. Metabolism 153, 155800. doi:10.1016/j.metabol.2024.155800
Chow, L. S., Gerszten, R. E., Taylor, J. M., Pedersen, B. K., van Praag, H., Trappe, S., et al. (2022). Exerkines in health, resilience and disease. Nat. Rev. Endocrinol. 18, 273–289. doi:10.1038/s41574-022-00641-2
Cusi, K., Younossi, Z., and Roden, M. (2024). From NAFLD to MASLD: promise and pitfalls of a new definition †. Hepatology 79, E13–E15. doi:10.1097/HEP.0000000000000706
Damluji, A. A., Alfaraidhy, M., AlHajri, N., Rohant, N. N., Kumar, M., Al Malouf, C., et al. (2023). Sarcopenia and cardiovascular diseases. Circulation 147, 1534–1553. doi:10.1161/CIRCULATIONAHA.123.064071
Deacon, C. F. (2011). Dipeptidyl peptidase-4 inhibitors in the treatment of type 2 diabetes: a comparative review. Diabetes. Obes. Metab. 13, 7–18. doi:10.1111/j.1463-1326.2010.01306.x
Del, B. M. G., Montone, R. A., Camilli, M., Carbone, S., Narula, J., Lavie, C. J., et al. (2021). Coronary microvascular dysfunction across the spectrum of cardiovascular diseases: JACC state-of-the-art review. J. Am. Coll. Cardiol. 78, 1352–1371. doi:10.1016/j.jacc.2021.07.042
Delgado-Marin, M., Sanchez-Esteban, S., Cook-Calvete, A., Jorquera-Ortega, S., Zaragoza, C., and Saura, M. (2024). Indoxyl sulfate-induced valve endothelial cell endothelial-to-mesenchymal transition and calcification in an integrin-linked kinase-dependent manner. Cells 13, 481. doi:10.3390/cells13060481
de Oliveira Dos Santos, A. R., de Oliveira Zanuso, B., Miola, V. F. B., Barbalho, S. M., Santos Bueno, P. C., Flato, U. A. P., et al. (2021). Adipokines, myokines, and hepatokines: crosstalk and metabolic repercussions. Int. J. Mol. Sci. 22, 2639. doi:10.3390/ijms22052639
Dhindsa, S., Ghanim, H., Batra, M., and Dandona, P. (2018). Hypogonadotropic hypogonadism in men with diabesity. Diabetes .Care. 41, 1516–1525. doi:10.2337/dc17-2510
Dimitroglou, Y., Aggeli, C., Theofilis, P., Tsioufis, P., Oikonomou, E., Chasikidis, C., et al. (2023). Novel anti-inflammatory therapies in coronary artery disease and acute coronary syndromes. Life (Basel) 13, 1669. doi:10.3390/life13081669
Duan, Y., Pan, X., Luo, J., Xiao, X., Li, J., Bestman, P. L., et al. (2022). Association of inflammatory cytokines with non-alcoholic fatty liver disease. Front. Immunol. 13, 880298. doi:10.3389/fimmu.2022.880298
Ebert, T., Kralisch, S., Hoffmann, A., Bachmann, A., Lössner, U., Kratzsch, J., et al. (2014). Circulating angiopoietin-like protein 8 is independently associated with fasting plasma glucose and type 2 diabetes mellitus. J. Clin. Endocrinol. Metab. 99, E2510–E2517. doi:10.1210/jc.2013-4349
Estes, C., Chan, H. L. Y., Chien, R. N., Chuang, W. L., Fung, J., Goh, G. B. B., et al. (2020). Modelling NAFLD disease burden in four Asian regions-2019-2030. Aliment. Pharmacol. Ther. 51, 801–811. doi:10.1111/apt.15673
Estes, C., Razavi, H., Loomba, R., Younossi, Z., and Sanyal, A. J. (2018). Modeling the epidemic of nonalcoholic fatty liver disease demonstrates an exponential increase in burden of disease. Hepatol. 67, 123–133. doi:10.1002/hep.29466
Fan Xz, B., Shi, Y., Liu, L., and Zhao, J. (2024). Systemic metabolic Abnormalities: key drivers of complications and mortality in MASLD. J. Hepatol. 80, e246–e248. doi:10.1016/j.jhep.2024.02.001
Ferrara, D., Montecucco, F., Dallegri, F., and Carbone, F. (2019). Impact of different ectopic fat depots on cardiovascular and metabolic diseases. J. Cell. Physiol. 234, 21630–21641. doi:10.1002/jcp.28821
Fontes-Cal, T. C. M., Mattos, R. T., Medeiros, N. I., Pinto, B. F., Belchior-Bezerra, M., Roque-Souza, B., et al. (2021). Crosstalk between plasma cytokines, inflammation, and liver damage as a new strategy to monitoring NAFLD progression. Front. Immunol. 12, 708959. doi:10.3389/fimmu.2021.708959
Frontera, W. R., and Ochala, J. (2015). Skeletal muscle: a brief review of structure and function. Calcif. Tissue. Int. 96, 183–195. doi:10.1007/s00223-014-9915-y
Ganz, T., and Nemeth, E. (2012). Hepcidin and iron homeostasis. Biochim. Biophys. Acta. 1823, 1434–1443. doi:10.1016/j.bbamcr.2012.01.014
Garbuzenko, D. V. (2022). Pathophysiological mechanisms of cardiovascular disorders in non-alcoholic fatty liver disease. Gastroenterol. Hepatol. Bed. Bench. 15, 194–203. doi:10.22037/ghfbb.v15i3.2549
Gilani, A., Stoll, L., Homan, E. A., and Lo, J. C. (2024). Adipose signals regulating distal organ health and disease. Diabetes 73, 169–177. doi:10.2337/dbi23-0005
Gonzalez, A., Huerta-Salgado, C., Orozco-Aguilar, J., Aguirre, F., Tacchi, F., Simon, F., et al. (2020). Role of oxidative stress in hepatic and extrahepatic dysfunctions during nonalcoholic fatty liver disease (NAFLD). Oxid. Med. Cell. Longev. 2020, 1617805. doi:10.1155/2020/1617805
Graham, T. E., Yang, Q., Bluher, M., Hammarstedt, A., Ciaraldi, T. P., Henry, R. R., et al. (2006). Retinol-binding protein 4 and insulin resistance in lean, obese, and diabetic subjects. N. Engl. J. Med. 354, 2552–2563. doi:10.1056/NEJMoa054862
Gumucio, J. P., Qasawa, A. H., Ferrara, P. J., Malik, A. N., Funai, K., McDonagh, B., et al. (2019). Reduced mitochondrial lipid oxidation leads to fat accumulation in myosteatosis. FASEB J. 33, 7863–7881. doi:10.1096/fj.201802457RR
Habibullah, M., Jemmieh, K., Ouda, A., Haider, M. Z., Malki, M. I., and Elzouki, A. N. (2024). Metabolic-associated fatty liver disease: a selective review of pathogenesis, diagnostic approaches, and therapeutic strategies. Front. Med. (Lausanne) 11, 1291501. doi:10.3389/fmed.2024.1291501
Hafiane, A. (2024). Adiponectin-mediated regulation of the adiponectin cascade in cardiovascular disease: updates. Biochem. Biophys. Res. Commun. 694, 149406. doi:10.1016/j.bbrc.2023.149406
Hallsworth, K., Fattakhova, G., Hollingsworth, K. G., Thoma, C., Moore, S., Taylor, R., et al. (2011). Resistance exercise reduces liver fat and its mediators in non-alcoholic fatty liver disease independent of weight loss. Gut 60, 1278–1283. doi:10.1136/gut.2011.242073
Hallsworth, K., Hollingsworth, K. G., Thoma, C., Jakovljevic, D., MacGowan, G. A., Anstee, Q. M., et al. (2013). Cardiac structure and function are altered in adults with non-alcoholic fatty liver disease. J. Hepatol. 58, 757–762. doi:10.1016/j.jhep.2012.11.015
Han, H., Zhu, J., Zhu, Z., Ni, J., Du, R., Dai, Y., et al. (2015). p-Cresyl sulfate aggravates cardiac dysfunction associated with chronic kidney disease by enhancing apoptosis of cardiomyocytes. J. Am. Heart. Assoc. 4, e001852. doi:10.1161/JAHA.115.001852
Hoffmann, C., and Weigert, C. (2017). Skeletal muscle as an endocrine organ: the role of myokines in exercise adaptations. Cold. Spring. Harb. Perspect. Med. 7, a029793. doi:10.1101/cshperspect.a029793
Holecek, M. (2021). The role of skeletal muscle in the pathogenesis of altered concentrations of branched-chain amino acids (valine, leucine, and isoleucine) in liver cirrhosis, diabetes, and other diseases. Physiol. Res. 70, 293–305. doi:10.33549/physiolres.934648
Hong, H. C., Hwang, S. Y., Choi, H. Y., Yoo, H. J., Seo, J. A., Kim, S. G., et al. (2014). Relationship between sarcopenia and nonalcoholic fatty liver disease: the Korean Sarcopenic Obesity Study. Hepatol. 59, 1772–1778. doi:10.1002/hep.26716
Hotamisligil, G. S. (2006). Inflammation and metabolic disorders. Nature 444, 860–867. doi:10.1038/nature05485
Houghton, D., Zalewski, P., Hallsworth, K., Cassidy, S., Thoma, C., Avery, L., et al. (2019). The degree of hepatic steatosis associates with impaired cardiac and autonomic function. J. Hepatol. 70, 1203–1213. doi:10.1016/j.jhep.2019.01.035
Huang, D. Q., Downes, M., Evans, R. M., Witztum, J. L., Glass, C. K., and Loomba, R. (2022). Shared mechanisms between cardiovascular disease and NAFLD. Semin. Liver. Dis. 42, 455–464. doi:10.1055/a-1930-6658
Huang, Y. C., Huang, J. C., Chien, H. H., Lin, C. I., Cheng, H. Y., et al. (2023). Performance of nonalcoholic fatty liver fibrosis score in estimating atherosclerotic cardiovascular disease risk. Nutr. Metab. Cardiovasc. Dis. 33, 2479–2487. doi:10.1016/j.numecd.2023.08.005
Ismaiel, A., and Dumitrascu, D. L. (2019). Cardiovascular risk in fatty liver disease: the liver-heart axis-literature review. Front. Med. (Lausanne) 6, 202. doi:10.3389/fmed.2019.00202
Iwakiri, Y., and Trebicka, J. (2021). Portal hypertension in cirrhosis: pathophysiological mechanisms and therapy. JHEP Rep. 3, 100316. doi:10.1016/j.jhepr.2021.100316
Jacobs, L., Thijs, L., Jin, Y., Zannad, F., Mebazaa, A., Rouet, P., et al. (2014). Heart 'omics' in AGEing (HOMAGE): design, research objectives and characteristics of the common database. J. Biomed. Res. 28, 349–359. doi:10.7555/JBR.28.20140045
Jensen, T., Abdelmalek, M. F., Sullivan, S., Nadeau, K. J., Green, M., Roncal, C., et al. (2018). Fructose and sugar: a major mediator of non-alcoholic fatty liver disease. J. Hepatol. 68, 1063–1075. doi:10.1016/j.jhep.2018.01.019
Jensen-Cody, S. O., and Potthoff, M. J. (2021). Hepatokines and metabolism: deciphering communication from the liver. Mol. Metab. 44, 101138. doi:10.1016/j.molmet.2020.101138
Jia, D., Zhang, H., Liu, T., and Wang, R. (2024). Exercise alleviates aging of adipose tissue through adipokine regulation. Metabolites 14, 135. doi:10.3390/metabo14030135
Jiang, Y., Wu, L., Zhu, X., Bian, H., Gao, X., and Xia, M. (2024). Advances in management of metabolic dysfunction-associated steatotic liver disease: from mechanisms to therapeutics. Lipids. Health. Dis. 23, 95. doi:10.1186/s12944-024-02092-2
Jimenez, V., Jambrina, C., Casana, E., Sacristan, V., Muñoz, S., Darriba, S., et al. (2018). FGF21 gene therapy as treatment for obesity and insulin resistance. EMBO Mol. Med. 10, e8791. doi:10.15252/emmm.201708791
Joo, S. K., and Kim, W. (2023). Interaction between sarcopenia and nonalcoholic fatty liver disease. Clin. Mol. Hepatol. 29, S68–S78. doi:10.3350/cmh.2022.0358
Kang, S. H., and Yoon, E. L. (2023). Sarcopenic obesity, the possible culprit for nonalcoholic fatty liver disease or fibrosis. Gut. Liver. 17, 8–9. doi:10.5009/gnl220543
Kasper, P., Martin, A., Lang, S., Kütting, F., Goeser, T., Demir, M., et al. (2021). NAFLD and cardiovascular diseases: a clinical review. Clin. Res. Cardiol. 110, 921–937. doi:10.1007/s00392-020-01709-7
Katsiki, N., Dimitriadis, G. D., and Mikhailidis, D. P. (2021). Serum uric acid and diabetes: from pathophysiology to cardiovascular disease. Curr. Pharm. Des. 27, 1941–1951. doi:10.2174/1381612827666210104124320
Kawaguchi, T., Takahashi, H., and Gerber, L. H. (2023). Clinics in liver disease: update on nonalcoholic steatohepatitis: sarcopenia and nonalcoholic fatty liver disease. Clin. Liver. Dis. 27, 275–286. doi:10.1016/j.cld.2023.01.005
Khan, M. S., Lee, C., and Kim, S. G. (2022). Non-alcoholic fatty liver disease and liver secretome. Arch. Pharm. Res. 45, 938–963. doi:10.1007/s12272-022-01419-w
Kir, S., Beddow, S. A., Samuel, V. T., Miller, P., Previs, S. F., Suino-Powell, K., et al. (2011). FGF19 as a postprandial, insulin-independent activator of hepatic protein and glycogen synthesis. Science 331, 1621–1624. doi:10.1126/science.1198363
Koplay, M., Gulcan, E., and Ozkan, F. (2011). Association between serum vitamin B12 levels and the degree of steatosis in patients with nonalcoholic fatty liver disease. J. Investig. Med. 59, 1137–1140. doi:10.2310/JIM.0b013e31822a29f5
Krishnan, S. M., Sobey, C. G., Latz, E., Mansell, A., and Drummond, G. R. (2014). IL-1beta and IL-18: inflammatory markers or mediators of hypertension? Br. J. Pharmacol. 171, 5589–5602. doi:10.1111/bph.12876
Kumar, K. G., Trevaskis, J. L., Lam, D. D., Sutton, G. M., Koza, R. A., Chouljenko, V. N., et al. (2008). Identification of adropin as a secreted factor linking dietary macronutrient intake with energy homeostasis and lipid metabolism. Cell. Metab. 8, 468–481. doi:10.1016/j.cmet.2008.10.011
Lai, J. C., Tandon, P., Bernal, W., Tapper, E. B., Ekong, U., Dasarathy, S., et al. (2021). Malnutrition, frailty, and sarcopenia in patients with cirrhosis: 2021 practice guidance by the American association for the study of liver diseases. Hepatol. 74, 1611–1644. doi:10.1002/hep.32049
Le, M. H., Le, D. M., Baez, T. C., Wu, Y., Ito, T., Lee, E. Y., et al. (2023). Global incidence of non-alcoholic fatty liver disease: a systematic review and meta-analysis of 63 studies and 1,201,807 persons. J. Hepatol. 79, 287–295. doi:10.1016/j.jhep.2023.03.040
Lebeaupin, C., Vallee, D., Hazari, Y., Hetz, C., Chevet, E., and Bailly-Maitre, B. (2018). Endoplasmic reticulum stress signalling and the pathogenesis of non-alcoholic fatty liver disease. J. Hepatol. 69, 927–947. doi:10.1016/j.jhep.2018.06.008
Lee, S. J., and McPherron, A. C. (1999). Myostatin and the control of skeletal muscle mass. Curr. Opin. Genet. Dev. 9, 604–607. doi:10.1016/s0959-437x(99)00004-0
Lee, Y. H., Jung, K. S., Kim, S. U., Yoon, H. J., Yun, Y. J., Lee, B. W., et al. (2015). Sarcopaenia is associated with NAFLD independently of obesity and insulin resistance: nationwide surveys (KNHANES 2008-2011). J. Hepatol. 63, 486–493. doi:10.1016/j.jhep.2015.02.051
Lee, Y. H., Kim, K. J., Yoo, M. E., Kim, G., Yoon, H. J., Jo, K., et al. (2018). Association of non-alcoholic steatohepatitis with subclinical myocardial dysfunction in non-cirrhotic patients. J. Hepatol. 68, 764–772. doi:10.1016/j.jhep.2017.11.023
Levy, J., Rodriguez-Gueant, R. M., Oussalah, A., Jeannesson, E., Wahl, D., Ziuly, S., et al. (2021). Cardiovascular manifestations of intermediate and major hyperhomocysteinemia due to vitamin B12 and folate deficiency and/or inherited disorders of one-carbon metabolism: a 3.5-year retrospective cross-sectional study of consecutive patients. Am. J. Clin. Nutr. 113, 1157–1167. doi:10.1093/ajcn/nqaa432
Li, M. D. (2011). Leptin and beyond: an odyssey to the central control of body weight. Yale J. Biol. Med. 84, 1–7.
Li, X., Bhattacharya, D., Yuan, Y., Wei, C., Zhong, F., Ding, F., et al. (2023). Chronic kidney disease in a murine model of non-alcoholic steatohepatitis (NASH). Kidney. Int. 105, 540–561. doi:10.1016/j.kint.2023.12.009
Lian, D., Chen, M. M., Wu, H., Deng, S., and Hu, X. (2022). The role of oxidative stress in skeletal muscle myogenesis and muscle disease. Antioxidants (Basel) 11, 755. doi:10.3390/antiox11040755
Lim, S., Taskinen, M. R., and Boren, J. (2019). Crosstalk between nonalcoholic fatty liver disease and cardiometabolic syndrome. Obes. Rev. 20, 599–611. doi:10.1111/obr.12820
Lim, W. H., Ng, C. H., Tan, D., Tseng, M., Xiao, J., Yong, J. N., et al. (2023). Natural history of NASH cirrhosis in liver transplant waitlist registrants. J. Hepatol. 79, 1015–1024. doi:10.1016/j.jhep.2023.05.034
Linton, M. F., Yancey, P. G., Davies, S. S., et al. (2000). Endotext. in The role of lipids and lipoproteins in atherosclerosis. Editors K. R. Feingold, B. Anawalt, and M. R. Blackman (South Dartmouth (MA): Endotext).
Liu, J., Xu, Y., Hu, Y., and Wang, G. (2015). The role of fibroblast growth factor 21 in the pathogenesis of non-alcoholic fatty liver disease and implications for therapy. Metabolism 64, 380–390. doi:10.1016/j.metabol.2014.11.009
Llovet, J. M., Willoughby, C. E., Singal, A. G., Greten, T. F., Heikenwälder, M., El-Serag, H. B., et al. (2023). Nonalcoholic steatohepatitis-related hepatocellular carcinoma: pathogenesis and treatment. Nat. Rev. Gastroenterol. Hepatol. 20, 487–503. doi:10.1038/s41575-023-00754-7
Loomba, R., Friedman, S. L., and Shulman, G. I. (2021). Mechanisms and disease consequences of nonalcoholic fatty liver disease. Cell 184, 2537–2564. doi:10.1016/j.cell.2021.04.015
Ludwig, J., Viggiano, T. R., McGill, D. B., and Oh, B. J. (1980). Nonalcoholic steatohepatitis: mayo Clinic experiences with a hitherto unnamed disease. Mayo Clin. Proc. 55, 434–438.
Lundberg, I. E., Fujimoto, M., Vencovsky, J., Aggarwal, R., Holmqvist, M., Christopher-Stine, L., et al. (2021). Idiopathic inflammatory myopathies. Nat. Rev. Dis. Prim. 7, 86. doi:10.1038/s41572-021-00321-x
Machado, M. V. (2021). Aerobic exercise in the management of metabolic dysfunction associated fatty liver disease. Diabetes Metab. Syndr. Obes. 14, 3627–3645. doi:10.2147/DMSO.S304357
Machado, M. V., and Diehl, A. M. (2016). Pathogenesis of nonalcoholic steatohepatitis. Gastroenterol. 150, 1769–1777. doi:10.1053/j.gastro.2016.02.066
Mahamid, M., Mahroum, N., Bragazzi, N. L., Shalaata, K., Yavne, Y., Adawi, M., et al. (2018). Folate and B12 levels correlate with histological severity in NASH patients. Nutrients 10, 440. doi:10.3390/nu10040440
Mahmoudi, S. K., Tarzemani, S., Aghajanzadeh, T., Kasravi, M., Hatami, B., Zali, M. R., et al. (2024). Exploring the role of genetic variations in NAFLD: implications for disease pathogenesis and precision medicine approaches. Eur. J. Med. Res. 29, 190. doi:10.1186/s40001-024-01708-8
Mantovani, A., Byrne, C. D., Benfari, G., Bonapace, S., Simon, T. G., and Targher, G. (2022). Risk of heart failure in patients with nonalcoholic fatty liver disease: JACC review topic of the week. J. Am. Coll. Cardiol. 79, 180–191. doi:10.1016/j.jacc.2021.11.007
Mantovani, A., Dauriz, M., Sandri, D., Bonapace, S., Zoppini, G., Tilg, H., et al. (2019). Association between non-alcoholic fatty liver disease and risk of atrial fibrillation in adult individuals: an updated meta-analysis. Liver. Int. 39, 758–769. doi:10.1111/liv.14044
Marcuccilli, M., and Chonchol, M. (2016). NAFLD and chronic kidney disease. Int. J. Mol. Sci. 17, 562. doi:10.3390/ijms17040562
Medrano-Bosch, M., Simon-Codina, B., Jimenez, W., Edelman, E. R., and Melgar-Lesmes, P. (2023). Monocyte-endothelial cell interactions in vascular and tissue remodeling. Front. Immunol. 14, 1196033. doi:10.3389/fimmu.2023.1196033
Meijers, B. K., Claes, K., Bammens, B., de Loor, H., Viaene, L., Verbeke, K., et al. (2010). p-Cresol and cardiovascular risk in mild-to-moderate kidney disease. Clin. J. Am. Soc. Nephrol. 5, 1182–1189. doi:10.2215/CJN.07971109
Mendez-Sanchez, N., Cerda-Reyes, E., Higuera-de-la-Tijera, F., Salas-García, A. K., Cabrera-Palma, S., Cabrera-Álvarez, G., et al. (2020). Dyslipidemia as a risk factor for liver fibrosis progression in a multicentric population with non-alcoholic steatohepatitis. F1000Res 9, 56. doi:10.12688/f1000research.21918.1
Meng, G., Wu, H., Fang, L., Yu, F., Zhang, Q., et al. (2016). Relationship between grip strength and newly diagnosed nonalcoholic fatty liver disease in a large-scale adult population. Sci. Rep. 6, 33255. doi:10.1038/srep33255
Merz, K. E., and Thurmond, D. C. (2020). Role of skeletal muscle in insulin resistance and glucose uptake. Compr. Physiol. 10, 785–809. doi:10.1002/cphy.c190029
Meyer, F., Bannert, K., Wiese, M., Esau, S., Sautter, L. F., Ehlers, L., et al. (2020). Molecular mechanism contributing to malnutrition and sarcopenia in patients with liver cirrhosis. Int. J. Mol. Sci. 21, 5357. doi:10.3390/ijms21155357
Misu, H., Takamura, T., Takayama, H., Hayashi, H., Matsuzawa-Nagata, N., Kurita, S., et al. (2010). A liver-derived secretory protein, selenoprotein P, causes insulin resistance. Cell Metab. 12, 483–495. doi:10.1016/j.cmet.2010.09.015
Mitten, E. K., and Baffy, G. (2022). Mechanotransduction in the pathogenesis of non-alcoholic fatty liver disease. J. Hepatol. 77, 1642–1656. doi:10.1016/j.jhep.2022.08.028
Mohammed, S. F., Hussain, I., AbouEzzeddine, O. F., Takahama, H., Kwon, S. H., et al. (2014). Right ventricular function in heart failure with preserved ejection fraction: a community-based study. Circulation 130, 2310–2320. doi:10.1161/CIRCULATIONAHA.113.008461
Montano-Loza, A. J., Angulo, P., Meza-Junco, J., Prado, C. M. M., Sawyer, M. B., Beaumont, C., et al. (2016). Sarcopenic obesity and myosteatosis are associated with higher mortality in patients with cirrhosis. J. Cachexia. Sarc. Mus. 7, 126–135. doi:10.1002/jcsm.12039
Mounier, R., Theret, M., Arnold, L., Cuvellier, S., Bultot, L., Göransson, O., et al. (2013). AMPKα1 regulates macrophage skewing at the time of resolution of inflammation during skeletal muscle regeneration. Cell. Metab. 18, 251–264. doi:10.1016/j.cmet.2013.06.017
Murthy, V. L., Naya, M., Foster, C. R., Hainer, J., Gaber, M., Di Carli, G., et al. (2011). Improved cardiac risk assessment with noninvasive measures of coronary flow reserve. Circulation 124, 2215–2224. doi:10.1161/CIRCULATIONAHA.111.050427
Musa-Veloso, K., Venditti, C., Lee, H. Y., Darch, M., Floyd, S., West, S., et al. (2018). Systematic review and meta-analysis of controlled intervention studies on the effectiveness of long-chain omega-3 fatty acids in patients with nonalcoholic fatty liver disease. Nutr. Rev. 76, 581–602. doi:10.1093/nutrit/nuy022
Musso, G., Gambino, R., Tabibian, J. H., Ekstedt, M., Kechagias, S., Hamaguchi, M., et al. (2014). Association of non-alcoholic fatty liver disease with chronic kidney disease: a systematic review and meta-analysis. PLoS. Med. 11, e1001680. doi:10.1371/journal.pmed.1001680
Muzurovic, E., Peng, C. C., Belanger, M. J., Sanoudou, D., Mikhailidis, D. P., and Mantzoros, C. S. (2022). Nonalcoholic fatty liver disease and cardiovascular disease: a review of shared cardiometabolic risk factors. Hypertension 79, 1319–1326. doi:10.1161/HYPERTENSIONAHA.122.17982
Nachit, M., Kwanten, W. J., Thissen, J. P., Op De Beeck, B., Van Gaal, L., Vonghia, L., et al. (2021). Muscle fat content is strongly associated with NASH: a longitudinal study in patients with morbid obesity. J. Hepatol. 75, 292–301. doi:10.1016/j.jhep.2021.02.037
Nahandi, M. Z., Khoshbaten, M., Ramazanzadeh, E., Abbaszadeh, L., Javadrashid, R., Shirazi, K. M., et al. (2014). Effect of non-alcoholic fatty liver disease on carotid artery intima-media thickness as a risk factor for atherosclerosis. Gastroenterol. Hepatol. Bed. Bench. 7, 55–62.
Njoku, D. B., Schilling, J. D., and Finck, B. N. (2022). Mechanisms of nonalcoholic steatohepatitis-associated cardiomyopathy: key roles for liver-heart crosstalk. Curr. Opin. Lipidol. 33, 295–299. doi:10.1097/MOL.0000000000000845
Nogal, A., Valdes, A. M., and Menni, C. (2021). The role of short-chain fatty acids in the interplay between gut microbiota and diet in cardio-metabolic health. Gut. Microbes. 13, 1–24. doi:10.1080/19490976.2021.1897212
Pais, R., Redheuil, A., Cluzel, P., Ratziu, V., and Giral, P. (2019). Relationship among fatty liver, specific and multiple-site atherosclerosis, and 10-year framingham score. Hepatol. 69, 1453–1463. doi:10.1002/hep.30223
Pasceri, V., Willerson, J. T., and Yeh, E. T. (2000). Direct proinflammatory effect of C-reactive protein on human endothelial cells. Circulation 102, 2165–2168. doi:10.1161/01.cir.102.18.2165
Patel, S. S., and Siddiqui, M. S. (2019). The interplay between nonalcoholic fatty liver disease and atherosclerotic heart disease. Hepatol. 69, 1372–1374. doi:10.1002/hep.30410
Paulus, W. J., and Tschope, C. (2013). A novel paradigm for heart failure with preserved ejection fraction: comorbidities drive myocardial dysfunction and remodeling through coronary microvascular endothelial inflammation. J. Am. Coll. Cardiol. 62, 263–271. doi:10.1016/j.jacc.2013.02.092
Peng, C., Stewart, A. G., Woodman, O. L., Ritchie, R. H., and Qin, C. X. (2020). Non-alcoholic steatohepatitis: a review of its mechanism, models and medical treatments. Front. Pharmacol. 11, 603926. doi:10.3389/fphar.2020.603926
Peng, H., Wang, S., Wang, M., Ye, Y., Xue, E., Chen, X., et al. (2022). Nonalcoholic fatty liver disease and cardiovascular diseases: a Mendelian randomization study. Metabolism 133, 155220. doi:10.1016/j.metabol.2022.155220
Perdomo, C. M., Gomez-Ambrosi, J., Becerril, S., Valentí, V., Moncada, R., Fernández-Sáez, E. M., et al. (2021). Role of ANGPTL8 in NAFLD improvement after bariatric surgery in experimental and human obesity. Int. J. Mol. Sci. 22, 12945. doi:10.3390/ijms222312945
Peter, A., Kovarova, M., Staiger, H., Machann, J., Schick, F., Königsrainer, A., et al. (2018). The hepatokines fetuin-A and fetuin-B are upregulated in the state of hepatic steatosis and may differently impact on glucose homeostasis in humans. Am. J. Physiol. Endocrinol. Metab. 314, E266–E273. doi:10.1152/ajpendo.00262.2017
Petta, S., Argano, C., Colomba, D., Cammà, C., Di Marco, V., Cabibi, D., et al. (2015). Epicardial fat, cardiac geometry and cardiac function in patients with non-alcoholic fatty liver disease: association with the severity of liver disease. J. Hepatol. 62, 928–933. doi:10.1016/j.jhep.2014.11.030
Petta, S., Ciminnisi, S., Di Marco, V., Cabibi, D., Cammà, C., Licata, A., et al. (2017). Sarcopenia is associated with severe liver fibrosis in patients with non-alcoholic fatty liver disease. Aliment. Pharmacol. Ther. 45, 510–518. doi:10.1111/apt.13889
Philips, C. A., Augustine, P., Yerol, P. K., Ramesh, G. N., Ahamed, R., Rajesh, S., et al. (2020). Modulating the intestinal microbiota: therapeutic opportunities in liver disease. J. Clin. Transl. Hepatol. 8, 87–99. doi:10.14218/JCTH.2019.00035
Pillon, N. J., Bilan, P. J., Fink, L. N., and Klip, A. (2013). Cross-talk between skeletal muscle and immune cells: muscle-derived mediators and metabolic implications. Am. J. Physiol. Endocrinol. Metab. 304, E453–E465. doi:10.1152/ajpendo.00553.2012
Plomgaard, P., Hansen, J. S., Townsend, L. K., Gudiksen, A., Secher, N. H., Clemmesen, J. O., et al. (2022). GDF15 is an exercise-induced hepatokine regulated by glucagon and insulin in humans. Front. Endocrinol. (Lausanne) 13, 1037948. doi:10.3389/fendo.2022.1037948
Polyzos, S. A., Kountouras, J., and Mantzoros, C. S. (2015). Leptin in nonalcoholic fatty liver disease: a narrative review. Metabolism 64, 60–78. doi:10.1016/j.metabol.2014.10.012
Pouwels, S., Sakran, N., Graham, Y., Leal, A., Pintar, T., Yang, W., et al. (2022). Non-alcoholic fatty liver disease (NAFLD): a review of pathophysiology, clinical management and effects of weight loss. BMC Endocr. Disord. 22, 63. doi:10.1186/s12902-022-00980-1
Powell, E. E., Wong, V. W., and Rinella, M. (2021). Non-alcoholic fatty liver disease. Lancet 397, 2212–2224. doi:10.1016/S0140-6736(20)32511-3
Purnamasari, D., Tetrasiwi, E. N., Kartiko, G. J., Astrella, C., Husam, K., and Laksmi, P. W. (2022). Sarcopenia and chronic complications of type 2 diabetes mellitus. Rev. Diabet. Stud. 18, 157–165. doi:10.1900/RDS.2022.18.157
Rafaqat, S., Gluscevic, S., Mercantepe, F., and Klisic, A. (2024). Interleukins: pathogenesis in non-alcoholic fatty liver disease. Metabolites 14, 153. doi:10.3390/metabo14030153
Raghow, R. (2013). Betatrophin: a liver-derived hormone for the pancreatic β-cell proliferation. World J. Diabetes. 4, 234–237. doi:10.4239/wjd.v4.i6.234
Ratchford, E. V., and Evans, N. S. (2014). Carotid artery disease. Vasc. Med. 19, 512–515. doi:10.1177/1358863X14557722
Ren, Z., Simons, P., Wesselius, A., Stehouwer, C. D. A., and Brouwers, M. C. G. J. (2023). Relationship between NAFLD and coronary artery disease: a Mendelian randomization study. Hepatol. 77, 230–238. doi:10.1002/hep.32534
Ribeiro, A., Liu, F., Srebrzynski, M., Rother, S., Adamowicz, K., Wadowska, M., et al. (2023). Uremic toxin indoxyl sulfate promotes macrophage-associated low-grade inflammation and epithelial cell senescence. Int. J. Mol. Sci. 24, 8031. doi:10.3390/ijms24098031
Rinella, M. E., Lazarus, J. V., Ratziu, V., Francque, S. M., Sanyal, A. J., Kanwal, F., et al. (2023). A multisociety Delphi consensus statement on new fatty liver disease nomenclature. J. Hepatol. 79, 1542–1556. doi:10.1016/j.jhep.2023.06.003
Ristic-Medic, D., Kovacic, M., Takic, M., Arsic, A., Petrovic, S., Paunovic, M., et al. (2020). Calorie-restricted mediterranean and low-fat diets affect fatty acid status in individuals with nonalcoholic fatty liver disease. Nutrients 13, 15. doi:10.3390/nu13010015
Rodriguez, J., Vernus, B., Chelh, I., Cassar-Malek, I., Gabillard, J. C., Hadj Sassi, A., et al. (2014). Myostatin and the skeletal muscle atrophy and hypertrophy signaling pathways. Cell Mol. Life. Sci. 71, 4361–4371. doi:10.1007/s00018-014-1689-x
Roman, E., Torrades, M. T., Nadal, M. J., Cárdenas, G., Nieto, J. C., Vidal, S., et al. (2014). Randomized pilot study: effects of an exercise programme and leucine supplementation in patients with cirrhosis. Dig. Dis. Sci. 59, 1966–1975. doi:10.1007/s10620-014-3086-6
Ryan, M. C., Itsiopoulos, C., Thodis, T., Ward, G., Trost, N., Hofferberth, S., et al. (2013). The Mediterranean diet improves hepatic steatosis and insulin sensitivity in individuals with non-alcoholic fatty liver disease. J. Hepatol. 59, 138–143. doi:10.1016/j.jhep.2013.02.012
Salah, H. M., Pandey, A., Soloveva, A., Abdelmalek, M. F., Diehl, A. M., Moylan, C. A., et al. (2021). Relationship of nonalcoholic fatty liver disease and heart failure with preserved ejection fraction. JACC Basic Transl. Sci. 6, 918–932. doi:10.1016/j.jacbts.2021.07.010
Santilli, V., Bernetti, A., Mangone, M., and Paoloni, M. (2014). Clinical definition of sarcopenia. Clin. Cases Min. Bone. Metab. 11, 177–180.
Santulli, G. (2014). Angiopoietin-like proteins: a comprehensive look. Front. Endocrinol. (Lausanne) 5, 4. doi:10.3389/fendo.2014.00004
Scheller, J., Chalaris, A., Schmidt-Arras, D., and Rose-John, S. (2011). The pro- and anti-inflammatory properties of the cytokine interleukin-6. Biochim. Biophys. Acta. 1813, 878–888. doi:10.1016/j.bbamcr.2011.01.034
Schober, A. (2008). Chemokines in vascular dysfunction and remodeling. Arterioscler. Thromb. Vasc. Biol. 28, 1950–1959. doi:10.1161/ATVBAHA.107.161224
Semmler, G., Datz, C., Reiberger, T., and Trauner, M. (2021). Diet and exercise in NAFLD/NASH: beyond the obvious. Liver. Int. 41, 2249–2268. doi:10.1111/liv.15024
Seo, D. Y., Park, S. H., Marquez, J., Kwak, H. B., Kim, T. N., Bae, J. H., et al. (2021). Hepatokines as a molecular transducer of exercise. J. Clin. Med. 10, 385. doi:10.3390/jcm10030385
Sethi, J. K., and Hotamisligil, G. S. (2021). Metabolic Messengers: tumour necrosis factor. Nat. Metab. 3, 1302–1312. doi:10.1038/s42255-021-00470-z
Shimada, T., Kakitani, M., Yamazaki, Y., Hasegawa, H., Takeuchi, Y., Fujita, T., et al. (2004). Targeted ablation of Fgf23 demonstrates an essential physiological role of FGF23 in phosphate and vitamin D metabolism. J. Clin. Invest. 113, 561–568. doi:10.1172/JCI19081
Siddiqui, M. S., Fuchs, M., Idowu, M. O., Luketic, V. A., Boyett, S., Sargeant, C., et al. (2015). Severity of nonalcoholic fatty liver disease and progression to cirrhosis are associated with atherogenic lipoprotein profile. Clin. Gastroenterol. Hepatol. 13, 1000–1008. doi:10.1016/j.cgh.2014.10.008
Simons, P., Valkenburg, O., Stehouwer, C. D. A., and Brouwers, M. C. G. J. (2022). Association between de novo lipogenesis susceptibility genes and coronary artery disease. Nutr. Metab. Cardiovasc. Dis. 32, 2883–2889. doi:10.1016/j.numecd.2022.09.003
Sinha, R. A., Bruinstroop, E., Singh, B. K., and Yen, P. M. (2020). Thyroid hormones and thyromimetics: a new approach to nonalcoholic steatohepatitis? Hepatol. 72, 770–771. doi:10.1002/hep.31204
Sinha, R. A., Singh, B. K., and Yen, P. M. (2018). Direct effects of thyroid hormones on hepatic lipid metabolism. Nat. Rev. Endocrinol. 14, 259–269. doi:10.1038/nrendo.2018.10
Solanki, R., Karande, A., and Ranganathan, P. (2023). Emerging role of gut microbiota dysbiosis in neuroinflammation and neurodegeneration. Front. Neurol. 14, 1149618. doi:10.3389/fneur.2023.1149618
Song, Q., and Zhang, X. (2022). The role of gut-liver Axis in gut microbiome dysbiosis associated NAFLD and NAFLD-HCC. Biomedicines 10, 524. doi:10.3390/biomedicines10030524
Stasi, A., Cosola, C., Caggiano, G., Cimmarusti, M. T., Palieri, R., Acquaviva, P. M., et al. (2022). Obesity-related chronic kidney disease: principal mechanisms and new approaches in nutritional management. Front. Nutr. 9, 925619. doi:10.3389/fnut.2022.925619
Stefan, N., Schick, F., Birkenfeld, A. L., Häring, H. U., and White, M. F. (2023). The role of hepatokines in NAFLD. Cell. Metab. 35, 236–252. doi:10.1016/j.cmet.2023.01.006
Su, C., Lu, Y., Wang, Z., Guo, J., Hou, Y., Wang, X., et al. (2023). Atherosclerosis: the involvement of immunity, cytokines and cells in pathogenesis, and potential novel therapeutics. Aging. Dis. 14, 1214–1242. doi:10.14336/AD.2022.1208
Sunaga, H., Koitabashi, N., Iso, T., Matsui, H., Obokata, M., Kawakami, R., et al. (2019). Activation of cardiac AMPK-FGF21 feed-forward loop in acute myocardial infarction: role of adrenergic overdrive and lipolysis byproducts. Sci. Rep. 9, 11841. doi:10.1038/s41598-019-48356-1
Sveinbjornsson, G., Ulfarsson, M. O., Thorolfsdottir, R. B., Jonsson, B. A., Einarsson, E., Gunnlaugsson, G., et al. (2022). Multiomics study of nonalcoholic fatty liver disease. Nat. Genet. 54, 1652–1663. doi:10.1038/s41588-022-01199-5
Tang, A. S. P., Chan, K. E., Quek, J., Xiao, J., Tay, P., Teng, M., et al. (2022). Non-alcoholic fatty liver disease increases risk of carotid atherosclerosis and ischemic stroke: an updated meta-analysis with 135,602 individuals. Clin. Mol. Hepatol. 28, 483–496. doi:10.3350/cmh.2021.0406
Tang, W. H., Wang, Z., Kennedy, D. J., Wu, Y., Buffa, J. A., Agatisa-Boyle, B., et al. (2015). Gut microbiota-dependent trimethylamine N-oxide (TMAO) pathway contributes to both development of renal insufficiency and mortality risk in chronic kidney disease. Circ. Res. 116, 448–455. doi:10.1161/CIRCRESAHA.116.305360
Taqueti, V. R., Solomon, S. D., Shah, A. M., Desai, A. S., Groarke, J. D., Osborne, M. T., et al. (2018). Coronary microvascular dysfunction and future risk of heart failure with preserved ejection fraction. Eur. Heart J. 39, 840–849. doi:10.1093/eurheartj/ehx721
Targher, G., Bertolini, L., Padovani, R., Rodella, S., Zoppini, G., Zenari, L., et al. (2006). Relations between carotid artery wall thickness and liver histology in subjects with nonalcoholic fatty liver disease. Diabetes Care 29, 1325–1330. doi:10.2337/dc06-0135
Targher, G., Byrne, C. D., and Tilg, H. (2024). MASLD: a systemic metabolic disorder with cardiovascular and malignant complications. Gut 73, 691–702. doi:10.1136/gutjnl-2023-330595
Tilg, H., Adolph, T. E., Dudek, M., and Knolle, P. (2021). Non-alcoholic fatty liver disease: the interplay between metabolism, microbes and immunity. Nat. Metab. 3, 1596–1607. doi:10.1038/s42255-021-00501-9
Tripathi, M., Singh, B. K., Zhou, J., Tikno, K., Widjaja, A., Sandireddy, R., et al. (2022). Vitamin B(12) and folate decrease inflammation and fibrosis in NASH by preventing syntaxin 17 homocysteinylation. J. Hepatol. 77, 1246–1255. doi:10.1016/j.jhep.2022.06.033
Umbro, I., Baratta, F., Angelico, F., and Del Ben, M. (2021). Nonalcoholic fatty liver disease and the kidney: a review. Biomedicines 9, 1370. doi:10.3390/biomedicines9101370
Valenzuela-Vallejo, L., Chrysafi, P., Kouvari, M., Guatibonza-Garcia, V., Mylonakis, S. C., Katsarou, A., et al. (2023). Circulating hormones in biopsy-proven steatotic liver disease and steatohepatitis: a Multicenter Observational Study. Metabolism 148, 155694. doi:10.1016/j.metabol.2023.155694
van der Meer, R. W., Hammer, S., Lamb, H. J., Frölich, M., Diamant, M., Rijzewijk, L. J., et al. (2008a). Effects of short-term high-fat, high-energy diet on hepatic and myocardial triglyceride content in healthy men. J. Clin. Endocrinol. Metab. 93, 2702–2708. doi:10.1210/jc.2007-2524
van der Meer, R. W., Rijzewijk, L. J., Diamant, M., Hammer, S., Schär, M., Bax, J. J., et al. (2008b). The ageing male heart: myocardial triglyceride content as independent predictor of diastolic function. Eur. Heart J. 29, 1516–1522. doi:10.1093/eurheartj/ehn207
VanWagner, L. B. (2018). New insights into NAFLD and subclinical coronary atherosclerosis. J. Hepatol. 68, 890–892. doi:10.1016/j.jhep.2018.01.023
Venkatesh, S. K., Idilman, I. S., Li, J., and Yin, M. (2024). Shrinking fat, healing liver: unlocking the metabolic dysfunction associated steatohepatitis puzzle. Hepatobiliary Surg. Nutr. 13, 132–135. doi:10.21037/hbsn-23-569
Venniyoor, A., Al Farsi, A. A., and Al, B. B. (2021). The troubling link between non-alcoholic fatty liver disease (NAFLD) and extrahepatic cancers (EHC). Cureus 13, e17320. doi:10.7759/cureus.17320
Verma Mkt, M., and Singh, B. K. (2024). “Dietary determinants of metabolic syndrome: focus on the obesity and metabolic dysfunction-associated steatotic liver disease (MASLD),” in Metabolic syndrome - lifestyle and biological risk factors intechopen. Editors H. C. G. Monyeki,, and P. Modjadji (London, United Kingdom: IntechOpen), 1–22.
Villanova, N., Moscatiello, S., Ramilli, S., Bugianesi, E., Magalotti, D., Vanni, E., et al. (2005). Endothelial dysfunction and cardiovascular risk profile in nonalcoholic fatty liver disease. Hepatol. 42, 473–480. doi:10.1002/hep.20781
Violi, F., Cammisotto, V., Bartimoccia, S., Pignatelli, P., Carnevale, R., and Nocella, C. (2023). Gut-derived low-grade endotoxaemia, atherothrombosis and cardiovascular disease. Nat. Rev. Cardiol. 20, 24–37. doi:10.1038/s41569-022-00737-2
Viveiros, K. (2021). The role of life style modifications in comprehensive non-alcoholic fatty liver disease treatment. Clin. Liver. Dis. Hob. 17, 11–14. doi:10.1002/cld.1007
Walker, V. (2014). Ammonia metabolism and hyperammonemic disorders. Adv. Clin. Chem. 67, 73–150. doi:10.1016/bs.acc.2014.09.002
Wang, S. W., Hsieh, T. H., Cheng, Y. M., Wang, C. C., and Kao, J. H. (2024). Liver and atherosclerotic risks of patients with cryptogenic steatotic liver disease. Hepatol. Int. 18, 943–951. doi:10.1007/s12072-023-10624-8
Wang, T. Y., Wang, R. F., Bu, Z. Y., Targher, G., Byrne, C. D., Sun, D. Q., et al. (2022). Association of metabolic dysfunction-associated fatty liver disease with kidney disease. Nat. Rev. Nephrol. 18, 259–268. doi:10.1038/s41581-021-00519-y
Wang, Y., Tuomilehto, J., Jousilahti, P., Salomaa, V., Li, B., Antikainen, R., et al. (2013). Serum gamma-glutamyltransferase and the risk of heart failure in men and women in Finland. Heart 99, 163–167. doi:10.1136/heartjnl-2012-302972
Wannamethee, S. G., Whincup, P. H., Shaper, A. G., Lennon, L., and Sattar, N. (2012). Γ-glutamyltransferase, hepatic enzymes, and risk of incident heart failure in older men. Arterioscler. Thromb. Vasc. Biol. 32, 830–835. doi:10.1161/ATVBAHA.111.240457
Wegermann, K., Fudim, M., Henao, R., Howe, C. F., McGarrah, R., Guy, C., et al. (2023). Serum metabolites are associated with HFpEF in biopsy-proven nonalcoholic fatty liver disease. J. Am. Heart Assoc. 12, e029873. doi:10.1161/JAHA.123.029873
Wijarnpreecha, K., Kim, D., Raymond, P., Scribani, M., and Ahmed, A. (2019). Associations between sarcopenia and nonalcoholic fatty liver disease and advanced fibrosis in the USA. Eur. J. Gastroenterol. Hepatol. 31, 1121–1128. doi:10.1097/MEG.0000000000001397
Witkowski, M., Moreno, S. I., Fernandes, J., Johansen, P., Augusto, M., and Nair, S. (2022). The economic burden of non-alcoholic steatohepatitis: a systematic review. Pharmacoeconomics 40, 751–776. doi:10.1007/s40273-022-01140-y
Wong, V. W., Ekstedt, M., Wong, G. L., and Hagström, H. (2023). Changing epidemiology, global trends and implications for outcomes of NAFLD. J. Hepatol. 79, 842–852. doi:10.1016/j.jhep.2023.04.036
Xiang, H., Sun, D., Liu, X., She, Z. G., and Chen, Y. (2022). The role of the intestinal microbiota in nonalcoholic steatohepatitis. Front. Endocrinol. (Lausanne) 13, 812610. doi:10.3389/fendo.2022.812610
Xie, J., Huang, H., Liu, Z., Yu, C., Xu, L., et al. (2023). The associations between modifiable risk factors and nonalcoholic fatty liver disease: a comprehensive Mendelian randomization study. Hepatol. 77, 949–964. doi:10.1002/hep.32728
Xie, T., and Leung, P. S. (2017). Fibroblast growth factor 21: a regulator of metabolic disease and health span. Am. J. Physiol. Endocrinol. Metab. 313, E292–E302. doi:10.1152/ajpendo.00101.2017
Yadav, A., Kataria, M. A., Saini, V., et al. (2013). Role of leptin and adiponectin in insulin resistance. Clin. Chim. Acta. 417, 80–84. doi:10.1016/j.cca.2012.12.007
Yan, F., Nie, G., Zhou, N., Zhang, M., and Peng, W. (2023). Association of fat-to-muscle ratio with non-alcoholic fatty liver disease: a single-centre retrospective study. BMJ Open 13, e072489. doi:10.1136/bmjopen-2023-072489
Yang, Z., Liu, Y., Li, Z., Feng, S., Lin, S., Ge, Z., et al. (2023). Coronary microvascular dysfunction and cardiovascular disease: pathogenesis, associations and treatment strategies. Biomed. Pharmacother. 164, 115011. doi:10.1016/j.biopha.2023.115011
Yang, Z., Tian, R., Zhang, X. J., Cai, J., and She, Z. G. (2022). Effects of treatment of non-alcoholic fatty liver disease on heart failure with preserved ejection fraction. Front. Cardiovasc. Med. 9, 1120085. doi:10.3389/fcvm.2022.1120085
Yarasheski, K. E., Bhasin, S., Sinha-Hikim, I., Pak-Loduca, J., and Gonzalez-Cadavid, N. F. (2002). Serum myostatin-immunoreactive protein is increased in 60-92 year old women and men with muscle wasting. J. Nutr. Health. Aging. 6, 343–348.
Yoshida, T., and Delafontaine, P. (2020). Mechanisms of IGF-1-mediated regulation of skeletal muscle hypertrophy and atrophy. Cells 9, 1970. doi:10.3390/cells9091970
Younossi, Z. M., Golabi, P., Paik, J., Owrangi, S., Yilmaz, Y., El-Kassas, M., et al. (2024b). Prevalence of metabolic dysfunction-associated steatotic liver disease in the Middle East and North Africa. Liver. Int. 44, 1061–1070. doi:10.1111/liv.15852
Younossi, Z. M., Golabi, P., Paik, J. M., Henry, A., Van Dongen, C., and Henry, L. (2023). The global epidemiology of nonalcoholic fatty liver disease (NAFLD) and nonalcoholic steatohepatitis (NASH): a systematic review. Hepatol. 77, 1335–1347. doi:10.1097/HEP.0000000000000004
Younossi, Z. M., Paik, J. M., Stepanova, M., Ong, J., Alqahtani, S., and Henry, L. (2024a). Clinical profiles and mortality rates are similar for metabolic dysfunction-associated steatotic liver disease and non-alcoholic fatty liver disease. J. Hepatol. 80, 694–701. doi:10.1016/j.jhep.2024.01.014
Younossi, Z. M., Stepanova, M., Al Shabeeb, R., Eberly, K. E., Shah, D., Nguyen, V., et al. (2024c). The changing epidemiology of adult liver transplantation in the United States in 2013-2022: the dominance of metabolic dysfunction-associated steatotic liver disease and alcohol-associated liver disease. Hepatol. Commun. 8, e0352. doi:10.1097/HC9.0000000000000352
Yu, X., Sun, S., Zhang, S., Hao, Q., Zhu, B., Teng, Y., et al. (2022). A pooled analysis of the association between sarcopenia and osteoporosis. Med. Baltim. 101, e31692. doi:10.1097/MD.0000000000031692
Zaric, B. L., Obradovic, M., Bajic, V., Haidara, M. A., Jovanovic, M., and Isenovic, E. R. (2019). Homocysteine and hyperhomocysteinaemia. Curr. Med. Chem. 26, 2948–2961. doi:10.2174/0929867325666180313105949
Zegeye, M. M., Lindkvist, M., Falker, K., Kumawat, A. K., Paramel, G., Grenegård, M., et al. (2018). Activation of the JAK/STAT3 and PI3K/AKT pathways are crucial for IL-6 trans-signaling-mediated pro-inflammatory response in human vascular endothelial cells. Cell. Commun. Signal. 16, 55. doi:10.1186/s12964-018-0268-4
Zeng, J., Fan, J. G., and Francque, S. M. (2024). Therapeutic management of metabolic dysfunction associated steatotic liver disease. United Eur. Gastroenterol. J. 12, 177–186. doi:10.1002/ueg2.12525
Zhang, J., Zhou, J., He, Z., and Li, H. (2024). Bacteroides and NAFLD: pathophysiology and therapy. Front. Microbiol. 15, 1288856. doi:10.3389/fmicb.2024.1288856
Zhang, X., Li, Y., Yang, P., Liu, X., Lu, L., Chen, Y., et al. (2020). Trimethylamine-N-Oxide promotes vascular calcification through activation of NLRP3 (Nucleotide-Binding domain, leucine-rich-containing family, pyrin domain-containing-3) inflammasome and NF-κB (nuclear factor κB) signals. Arterioscler. Thromb. Vasc. Biol. 40, 751–765. doi:10.1161/ATVBAHA.119.313414
Zhang, X., Yang, L., Xu, X., Tang, F., Yi, P., Qiu, B., et al. (2019). A review of fibroblast growth factor 21 in diabetic cardiomyopathy. Heart Fail Rev. 24, 1005–1017. doi:10.1007/s10741-019-09809-x
Zhang, Z., Chen, C., Wang, Y., Chen, Y., Lu, Y., et al. (2022). The associations of total testosterone with probable nonalcoholic steatohepatitis and nonalcoholic fatty liver disease fibrotic progression in men with type 2 diabetes: a cross-sectional study. Eur. J. Med. Res. 27, 307. doi:10.1186/s40001-022-00958-8
Zhang, Z. Y., Yan, Q., Wu, W. H., Zhao, Y., Zhang, H., and Li, J. (2023). PPAR-alpha/gamma agonists, glucagon-like peptide-1 receptor agonists and metformin for non-alcoholic fatty liver disease: a network meta-analysis. J. Int. Med. Res. 51, 3000605231177191. doi:10.1177/03000605231177191
Zhao, J., Fan, H., Wang, T., Yu, B., Mao, S., Wang, X., et al. (2022a). TyG index is positively associated with risk of CHD and coronary atherosclerosis severity among NAFLD patients. Cardiovasc. Diabetol. 21, 123. doi:10.1186/s12933-022-01548-y
Zhao, X., Chen, J., Sun, H., Zhang, Y., and Zou, D. (2022b). New insights into fibrosis from the ECM degradation perspective: the macrophage-MMP-ECM interaction. Cell Biosci. 12, 117. doi:10.1186/s13578-022-00856-w
Zhen, J., Zhou, Z., He, M., Han, H. X., Lv, E. H., Wen, P. B., et al. (2023). The gut microbial metabolite trimethylamine N-oxide and cardiovascular diseases. Front. Endocrinol. (Lausanne) 14, 1085041. doi:10.3389/fendo.2023.1085041
Zhong, H., Dong, J., Zhu, L., Mao, J., Zhao, Y., et al. (2024). Non-alcoholic fatty liver disease: pathogenesis and models. Am. J. Transl. Res. 16, 387–399. doi:10.62347/KMSA5983
Zhu, B., Wu, H., Li, K. S., Eisa-Beygi, S., Singh, B., Bielenberg, D. R., et al. (2023). Two sides of the same coin: non-alcoholic fatty liver disease and atherosclerosis. Vasc. Pharmacol. 154, 107249. doi:10.1016/j.vph.2023.107249
Zhu, Y., Xu, J., Zhang, D., Mu, X., Shi, Y., Chen, S., et al. (2021). Efficacy and safety of GLP-1 receptor agonists in patients with type 2 diabetes mellitus and non-alcoholic fatty liver disease: a systematic review and meta-analysis. Front. Endocrinol. (Lausanne) 12, 769069. doi:10.3389/fendo.2021.769069
Keywords: Adipose tissue (AT), metabolic dysfunction-associated steatotic liver disease (MASLD)/non-alcoholic fatty liver disease (NAFLD), metabolic dysfunction-associated steatohepatitis (MASH)/non-alcoholic steatohepatitis (NASH), cardiovascular diseases (CVDs), sarcopenia, chronic kidney diseases (CKDs), heart, muscle
Citation: Sandireddy R, Sakthivel S, Gupta P, Behari J, Tripathi M and Singh BK (2024) Systemic impacts of metabolic dysfunction-associated steatotic liver disease (MASLD) and metabolic dysfunction-associated steatohepatitis (MASH) on heart, muscle, and kidney related diseases. Front. Cell Dev. Biol. 12:1433857. doi: 10.3389/fcell.2024.1433857
Received: 16 May 2024; Accepted: 01 July 2024;
Published: 16 July 2024.
Edited by:
Joy Jiang, University of California, Davis, United StatesReviewed by:
Sangam Rajak, Sanjay Gandhi Post Graduate Institute of Medical Sciences (SGPGI), IndiaTsutomu Matsubara, Osaka Metropolitan University, Japan
Copyright © 2024 Sandireddy, Sakthivel, Gupta, Behari, Tripathi and Singh. This is an open-access article distributed under the terms of the Creative Commons Attribution License (CC BY). The use, distribution or reproduction in other forums is permitted, provided the original author(s) and the copyright owner(s) are credited and that the original publication in this journal is cited, in accordance with accepted academic practice. No use, distribution or reproduction is permitted which does not comply with these terms.
*Correspondence: Brijesh Kumar Singh, c2luZ2hicmlqZXNoa0BkdWtlLW51cy5lZHUuc2c=, c2luZ2hicmlqZXNoa0Bob3RtYWlsLmNvbQ==; Madhulika Tripathi, bWFkaHVsaWthLnRyaXBhdGhpQGR1a2UtbnVzLmVkdS5zZw==, bWFkaHVsaWthX3RyaXBhdGhpQHlhaG9vLmNvbQ==