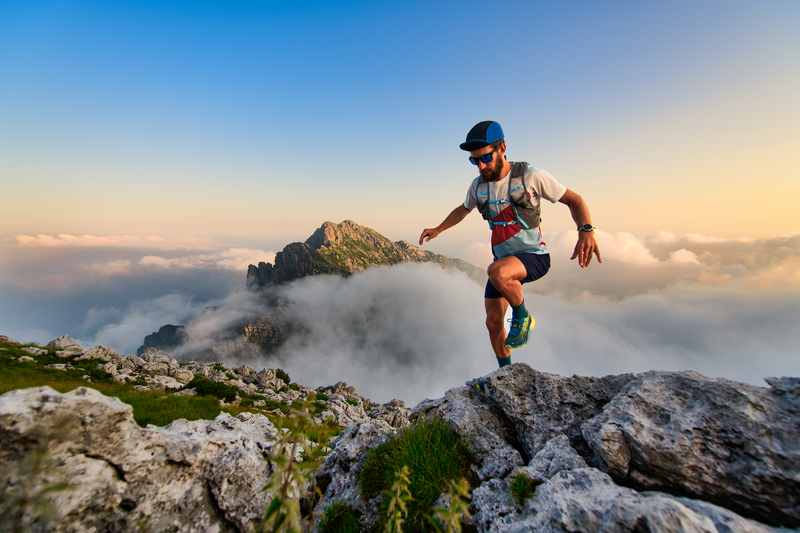
94% of researchers rate our articles as excellent or good
Learn more about the work of our research integrity team to safeguard the quality of each article we publish.
Find out more
REVIEW article
Front. Cell Dev. Biol. , 12 August 2024
Sec. Stem Cell Research
Volume 12 - 2024 | https://doi.org/10.3389/fcell.2024.1432668
Bone marrow stromal cells (BMSCs) play a significant role in bone metabolism as they can differentiate into osteoblasts, bone marrow adipocytes (BMAds), and chondrocytes. BMSCs chronically exposed to nutrient overload undergo adipogenic programming, resulting in bone marrow adipose tissue (BMAT) formation. BMAT is a fat depot transcriptionally, metabolically, and morphologically distinct from peripheral adipose depots. Reactive oxygen species (ROS) are elevated in obesity and serve as important signals directing BMSC fate. ROS produced by the NADPH oxidase (NOX) family of enzymes, such as NOX4, may be responsible for driving BMSC adipogenesis at the expense of osteogenic differentiation. The dual nature of ROS as both cellular signaling mediators and contributors to oxidative stress complicates their effects on bone metabolism. This review discusses the complex interplay between ROS and BMSC differentiation in the context of metabolic bone diseases.Special attention is paid to the role of NOX4-ROS in regulating cellular processes within the bone marrow microenvironment and potential target in metabolic bone diseases.
Bone marrow (BM) is the heterogenous and multicellular tissue located within the medullary cavity of bones. The BM plays an essential role in many physiological and pathological processes, including hematopoiesis, bone remodeling, and even cardiovascular and metabolic diseases (Benova and Tencerova, 2020). Although bone and BM are anatomically connected, they possess some specialized roles (Del Fattore et al., 2010). Bones provide skeletal support and organ protection, store calcium and phosphorus, and regulate various organ systems via the release of bone-derived hormones (“osteokines”), whereas the BM serves as a specialized niche that facilitates the generation of multiple crucial cell types, including red blood cells, white blood cells, and platelets (Del Fattore et al., 2010).
A significant stem cell population within the BM is hematopoietic stem cells (HSCs) (∼0.01%–0.1% of the total number of nuclear cells in BM aspirates) (Pang et al., 2011; Rossi et al., 2011). HSCs are the source of immune cell progenitors and bone resorbing cells (osteoclasts), which serve to negatively remodel bone. Beyond HSCs, multiple other cell types promote BM homeostasis, such as those providing nutrient supply (endothelial cells), innervations (nerve cells), and bone matrix formation (osteoblasts, osteocytes) and their progenitors, known as BM stromal cells (BMSCs) (Peci et al., 2022). BMSCs, though a rare population of cells within the BM (∼0.001%–0.01%) (Bianco et al., 2001), play a crucial role in the BM microenvironment as they can differentiate into osteoblasts, BM adipocytes (BMAds), and chondrocytes (cartrilage forming cells) (Dominici et al., 2006). The ability of BMSCs to differentiate into these different cell types is largely affected by physiological and pathophysiological conditions that result in the activation of different transcriptional programs and secreted factors (Tencerova and Kassem, 2016; Lecka-Czernik et al., 2017).
Within the BM, bone cells and adipocytes exhibit cell-to-cell contact and communication (Lanske and Rosen, 2017), to facilitate bone remodeling, hormonal regulation and nutrient exchange. BMAds collectively form BM adipose tissue (BMAT), a fat depot with unique molecular and physiological properties in comparison to peripheral adipose tissue (AT) (Suchacki and Cawthorn, 2018). During aging, up to 70% of red BM (replete with HSCs) undergoes conversion to yellow BM filled with BMAds (Kricun, 1985). This conversion occurs mainly in distal bones and does so centripetally (from peripheral skeleton to axial skeleton), possibly due to differences in temperature, vascularity, and oxygen tension in distal versus proximal bones (Kricun, 1985). In addition to aging (Farr et al., 2017), other metabolic diseases, such as obesity, diabetes and osteoporosis (Tencerova and Kassem, 2016; Suchacki and Cawthorn, 2018) affect BMSC properties and shift BMSC differentiation towards higher BMAd formation in the BM. Increased BMAT is often associated with reduced bone mineral density (BMD) and higher fracture risk, indicating a potential role of BMAT in the pathogenesis of osteoporosis (Shen et al., 2014; Beekman et al., 2023). Therefore, identifying the signals promoting BMAT accumulation in metabolic diseases could bring insight into the mechanisms affecting cell fate determination.
BMAT accumulation is accompanied with an increased production of reactive oxygen species (ROS), which contributes to a senescent BM microenvironment and increased bone fragility (Tencerova et al., 2019a). Traditionally, ROS were characterized simply as toxic by-products of aerobic metabolism that pathologically contributed to oxidative stress by damaging macromolecules such as lipids, proteins and nucleic acids (Beckman and Ames, 1998). As oxidative stress can accelerate various cellular processes including apoptosis and senescence (Jones, 2006), ROS production has been implicated in many disease processes. However, indiscriminate quenching of ROS can impair cell signaling as these molecules also can act as physiological signaling agents promoting health (Rhee et al., 2000; Finkel, 2011). Due to the two unpaired electrons in its outer orbital, oxygen (O2) is highly susceptible to the formation of ROS such as: O2·−, hydroxyl radical (HO·), H2O2, etc. (Sies, 2020). These ROS are characterized by different half-lives, charges and abilities to cross biological membranes. H2O2 is the most stable ROS as it has a significantly longer half-life and can cross biological membranes, enabling it to actively serve as a signaling molecule (Chen et al., 2008). For instance, H2O2 can directly react with cysteine residues of proteins involved in regulation of cell differentiation (e.g., Phosphatase and tensin homolog (PTEN), Akt2) (Vieceli Dalla Sega et al., 2017; Sies, 2020).
The family of nicotinamide adenine dinucleotide phosphate (NADPH) oxidase (NOX) enzymes is a major source of ROS production within the BM. It has been shown that NOX-ROS play an important role in cell proliferation, differentiation, homing, and senescence (Schroder, 2019). Notably, NOX members are found to be differentially expressed based on cell type, and their activity responds to specific extra- and intracellular signals through generation of ROS, e.g., superoxide (O2·−) or hydrogen peroxide (H2O2) (Brown and Griendling, 2009). Interestingly, NOX4 is unique among the NOX family members as it does not require agonist-stimulated activation to produce ROS (Martyn et al., 2006). A key structural property enabling NOX4 to facilitate essential cellular processes in its third extracystosolic loop (E loop). This loop allows NOX4 to produce H2O2 over O2·− (Takac et al., 2011). Consequenly, NOX4-generated H2O2 may play a significant role in signal transduction (Finkel, 2011).
The role of NOX-ROS across different BM-resident cells in pathophysiological conditions is not well documented. Thus, this review provides an overview of the literature on elucidating the role of NOX-ROS in bone-fat metabolism. As NOX4 is important in BMSC differentiation (Atashi et al., 2015), we will highlight the potential role of NOX4 in the regulation of BMAT expansion and BMSC properties, which may significantly contribute to alterations in bone homeostasis.
The relationship between BMAT and metabolic diseases such as obesity and diabetes has recently attracted increased attention, even though BMAT was first described by anatomists in the late 19th century in histological sections of bone biopsies (Stockman and Greig, 1898). As opposed to the well-studied white, brown, beige and pink AT (Richard et al., 2000), the researchers have only recently begun to characterize the exact functions of BMAT. In the last decade, the BM adiposity literature has rapidly increased, leading to the recent understanding that BMAT acts as a unique fat depot that differs from peripheral AT not only anatomically but also developmentally, functionally, and metabolically (Hardouin et al., 2016; Suchacki and Cawthorn, 2018; Tencerova et al., 2018).
BMAT supplies energy to neighboring BM cells, such as osteoblasts and HSCs (Li et al., 2022; Alekos et al., 2023) during periods of increased energy demand, such as bone remodeling, haematopoiesis or cell proliferation (Shafat et al., 2017; Tabe et al., 2017; Tencerova et al., 2018). Beyond the traditional role of AT as an energy reserve, BMAT can contribute to bone loss through the release of pro-inflammatory and pro-resorptive cytokines and adipokines (e.g., receptor activator of nuclear factor kappa-Β ligand (RANKL), macrophage colony-stimulating factor (M-CSF), dipeptidyl peptidase (DPP4), lipocalin 2 (LCN2), tumor necrosis factor-alpha (TNF-α) and interleukin-6 (IL-6), which negatively regulate bone metabolism) (Herrmann, 2019; Tencerova et al., 2021). BMAT expansion results in compromised osteoblast differentiation, as BMAT originates from BMSCs (Tencerova and Kassem, 2016). This reciprocal relationship is further highlighted by the fact that molecular pathways promoting osteogenesis typically inhibit adipogenesis and vice versa (Li et al., 2013). This may be partially mediated by ROS-induced changes in redox-sensitive microRNAs that inhibit transcription factors such as Runt-related transcription factor 2 (RUNX2), impairing osteogenesis and augmenting adipogenesis in BMSCs through NF-κB signaling (Liao et al., 2013; Wang et al., 2015).
In both osteoporosis and obesity, there is commonly an imbalance in the regulation of osteoblastic and adipogenic BMSC differentiation (Sui et al., 2016). Several studies have shown a negative correlation between BMAT volume and BMD (Yeung et al., 2005; Li et al., 2014), which may underpin elevated risk of bone fractures (Woods et al., 2022; Guimaraes et al., 2023) (Figure 1). In obesity, BMAT resists the development of insulin resistance and inflammation, unlike what is observed in peripheral tissues (Tencerova et al., 2019a). However, continuous recruitment of BMSCs to BMAT as seen in obesity exceeds this protective potential and instead drives progenitor cell exhaustion, reduced osteoblastic recruitment, and ultimately decreased bone formation (Tencerova et al., 2018). Exposure of human BMSCs to sera isolated from overweight individuals increased adipocyte differentiation at the expense of osteogenic differentiation demonstrating that circulating factors are sufficient to skew the BMSC differentiation potential towards adipogenesis (Di Bernardo et al., 2014). In fact, the BM contains BMSCs that are uniquely primed for adipogenesis, which readily proliferate under obesogenic conditions and significantly contribute to BMAT expansion (Tencerova et al., 2019b). Increased ROS levels in obesity are associated with adipogenic BMSCs that demonstrate a shift from glycolysis towards higher oxidative phosphorylation, enhanced insulin signaling, glucose transport, lipid metabolism, and senescence (Guntur et al., 2018; Tencerova et al., 2019b). This hypermetabolic phenotype of BMSCs may represent a mechanism by which obesity contributes to bone fragility (Tencerova et al., 2019a). Thus, one can hypothesize that ROS might mediate the adverse effects of metabolic diseases on bone and BM microenvironment.
Figure 1. Bone homeostasis in normal and pathophysiological conditions. In physiological conditions, bone homeostasis represents a balance between bone formation (formed by OBs) and bone resorption (mediated by OCs). In homeostasis, BMSC differentiation favors OBs over BMAds. OCs, responsible for degrading bone, create lacunae that are subsequently filled with newly synthesized matrices by OBs. However, in pathophysiological conditions such as obesity, diabetes, or aging, this balance is disrupted. BMSC differentiation is shifted towards adipogenesis leading to the accumulation of BMAds within BM through increased PPARγ and insulin signaling. OB-mediated bone formation is diminished, while OC differentiation and activities, as well as subsequent bone resorption, are increased. This imbalance is facilitated directly or indirectly through increased production of RANKL and M-CSF, exacerbating the detrimental effects of oxidative stress on bone health and leading to a higher risk of fracture.
Emerging evidence suggests that elevated ROS in metabolic bone diseases negatively impact bone homeostasis (Table 1). ROS aid in mineralized matrix degradation and influence the behaviour of cells involved in this process (Agidigbi and Kim, 2019). Osteoclasts located on the bone interface generate O2·−and H2O2 which regulates their differentiation and development (Goettsch et al., 2013; Agidigbi and Kim, 2019). Furthermore, ROS released by neighboring cells stimulate osteoclast activity through ERK/NF-κB signaling and increased RANKL production (Ha et al., 2004; Lorenzo, 2017). These signals result in the inhibition of osteoblast lifespan (Deng et al., 2019), differentiation (Bai et al., 2004; Chen et al., 2018), and decreased alkaline phosphatase (ALP) activity (Luo et al., 2020). One of the key signaling pathways influenced by ROS is the Wingless/Int-1 (Wnt) signaling, important for BMSC fate, and homeostasis (Houschyar et al., 2018), which is diminished by excess ROS causing increased BMAd expansion (Atashi et al., 2015). ROS inhibition of Wnt occurs through the oxidation of key signaling molecules, such as β-catenin, which is crucial for Wnt signal transduction (Kajla et al., 2012; Staehlke et al., 2020).Another pathway affected by ROS is the PI3K/Akt pathway, which is vital for cell survival and proliferation (Liu et al., 2021). ROS can inhibit the activity of PTEN, a negative regulator of the PI3K/Akt pathway, leading to increased Akt signaling and altered cell survival and differentiation (Koundouros and Poulogiannis, 2018; Liu et al., 2021). ROS can modulate the MAPK/ERK pathway, which is involved in the regulation of cell growth and differentiation.
The role of ROS in driving BMSC differentiation to BMAT is further confirmed through the use of antioxidants where osteogenic potential was enhanced while adipogenic potential was reduced in mouse and human BMSCs in response to antioxidants such as the flavanol quercetin (Wang et al., 2021), the fullerene-derivative fullerol (Liu et al., 2013), the polyphenol resveratrol (Ali et al., 2020), and the isoflavone formomentin (Gautam et al., 2017). The mechanisms by which ROS quenching reduces BMAT are still being elucidated but have been shown to involve interactions between canonical factors like RUNX2, osterix (OSX), RANKL and osteoprotegerin (OPG), which are crucial in bone remodeling (Wauquier et al., 2009; Ali et al., 2020).
In summary, ROS play a pivotal role in bone by both promoting osteoclastogenesis and inhibiting osteoblast differentiation in favor of adipogenesis (Figure 2). While the role of ROS in bone remodeling and the impact on osteoclasts and osteoblasts are well-established, there is a critical need for comprehensive research to elucidate the specific ROS-producers and effects of ROS on BMSCs and, thus, BMAT. Unraveling the molecular mechanisms by which ROS are produced and may modulate BMSC fate decisions can provide valuable insights into the complex interplay between oxidative stress and BM homeostasis. This knowledge will not only contribute to a more nuanced understanding of bone physiology but may also unveil potential therapeutic targets for conditions characterized by altered bone homeostasis.
Figure 2. ROS and its effect on bone homeostasis. ROS play a crucial role in modulating signaling pathways within bone cells. They contribute to bone loss by impairing osteoblastogenesis and promoting osteoclastogenesis and adipogenesis. ROS induction of bone resorption occurs either directly by mediating OC function through activation of the mitogen-activated protein kinase (MAPK) signaling pathways such as JNK, p38, and ERK1/2. In addition, they have an important roles in signal transduction that activate cellular responses to many types of stresses, but also control the proliferation, differentiation, and survival of osteoclasts. Indirect activation of OCs is achieved through the upregulation of RANKL expression within OBs. PKA and CREB are central to the cAMP signaling pathway that regulates the production of RANKL. PKA activation leads to CREB phosphorylation, which binds to the RANKL promoter to enhance its transcription. This mechanism underscores the critical role of OBs in this process. On the other hand, osteoblastogenesis, together with bone formation is impaired through inhibiton of Wnt signaling and ALP activity, which are crucial for maintaining bone homeostasis. The activation of PPARγ promotes adipogenic differentiation of BMSCs at the expense of osteoblastogenesis. The presence of BMAds within the BM negatively affects the differentiaton of BMSCs towards OBs by releasing pro-inflammatory and pro- resorptive cytokines and adipokines. Created with BioRender.com
The quenching of high levels of ROS via endogenous or exogenous antioxidants can prevent cell damage and attenuate BMSC apoptosis and loss of viability (Balogh et al., 2016). Yet, as described above, ROS are not only harmful by-products of cell metabolism but also participate in signal transduction and are required for cellular functions such as differentiation (Atashi et al., 2015). Therefore, it is critical to understand the sources and specify locations of ROS and their impacts on BMSC function. NOXs are considered a major source of ROS production within the BM. They are transmembrane proteins that transfer electrons across membranes to O2 using NADPH as an electron donor (Schroder, 2019). Among the NOX family members, research has highlighted the role of NOX2 and NOX4 in BM, demonstrating they contribute to bone loss, marrow adipogenesis, and osteoclastogenesis in mice (Atashi et al., 2015; Rahman et al., 2018; Sun et al., 2021), As osteoclastogenesis is intricately linked to BMSC function, the role of NOX4 in this process is significant. NOX4 is a critical source of ROS in mouse HSCs (Wang et al., 2010), human monocytes and human macrophages (Lee et al., 2010), controlling their function and differentiation (Yang et al., 2004). Notably, it has been reported that NOX4 limits bone mass by promoting osteoclastogenesis in an osteoporotic mouse model (Goettsch et al., 2013) and it is involved in the regulation of osteoprogenitors in bone development (Chen et al., 2022).
During differentiation, the major sources of ROS production include mitochondrial complexes I and III and NOX4 (Mahadev et al., 2001; Furukawa et al., 2004). Interestingly, the relationship between mitochondria and NOX4 seems to be bidirectional. In cancer cells, mitochondrial ATP produced through oxidative phosphorylation limits NOX4 activity by binding to a specific ATP-binding motif in the C-terminal tail of NOX4 (Shanmugasundaram et al., 2017), suggesting that NOX4 serves as an intracellular energy sensor. Indeed, NOX4 is required for mitochondrial biogenesis in the skeletal muscle following conditions of high energy demand like those in exercise (Specht et al., 2021). On the other hand, NOX4 has been shown to repress mitochondrial biogenesis and Complex I activity in fibroblasts (Bernard et al., 2017). As mitochondrial biogenesis increases during BMSC differentiation (Yan et al., 2021), understanding the relationship between NOX4, mitochondrial metabolism and mitochondrial biogenesis in BMSCs may be a fruitful avenue of research.
BMAT and peripheral AT are significantly different tissues (Liu et al., 2011; Miggitsch et al., 2019). However, examining NOX4 and ROS in peripheral AT may reveal crucial insights into NOX4 function and impact on overall metabolic health. In peripheral AT, NOX4 signaling pathways are primarily centered around adipogenesis and metabolic regulation (Den Hartigh et al., 2017). NOX4-ROS production stimulates the differentiation of preadipocytes to mature adipocytes (Schroder et al., 2009). This process involves the activation of adipogenic transcription factors which are essential for adipocyte maturation and lipid accumulation such as peroxisome proliferator-activated receptor gamma (PPARγ) and CCAAT enhancer-binding protein alpha (C/EBPα) (Schroder et al., 2009). In response to hypoxia, Nox4 silencing in adipose-derived stem cells led to reduced proliferation and cell migration, along with decreased phosphorylation of platelet-derived growth factor receptor-β, AKT serine/threonine kinase 1 or Protein kinase B (AKT), and ERK1/2 (Kim et al., 2012). A common stressor to simulate obesogenic condition in vitro is high glucose. With obesity, NOX4 is upregulated in adipocytes (Den Hartigh et al., 2017). Peripheral preadipocytes differentiated from mice lacking adipocyte NOX4 are resistant to high glucose and palmitate-induced inflammation (Den Hartigh et al., 2017). This suggests that NOX4-ROS in AT participates in signaling cascades responsible for the early onset of insulin resistance and the inflammatory response in obesity (Den Hartigh et al., 2017). BMAds were shown to overproduce ROS mediated through the enhanced NOX4 expression, causing increased intracellular ROS levels and downregulating the endogenous antioxidant systems following high glucose treatment (Rharass and Lucas, 2019). Thus, BMAds are sensitive to both glucose and ROS levels, and these together influence their phenotype and functionality.
Comparing the transcriptome of BMAds and peripheral adipocytes demonstrates apparent differences between these two tissues (Suchacki et al., 2020). However, such a comparison may lend insights into the difference in response to ROS and adipogenic priming in obesogenic conditions discussed above. For instance, BMAds demonstrate increased early adipogenic gene expression, and lower late adipogenic genes compared to epididymal adipocytes (Liu et al., 2011). Furthermore, BMAds showed greater expression of pro-inflammatory genes (Liu et al., 2011) and displayed an elevated production of ROS (Miggitsch et al., 2019). These findings suggest that BMAds may be primed to receive maturation cues due to obesogenic stressors compared to peripheral adipocytes due to a comparably decreased antioxidant capacity. Therefore, NOX4-ROS may direct the maturation of BMSCs into BMAds within BM. Together, these data provide evidence that NOX4-ROS are important for activation, differentiation, and the response to metabolic stressors in the peripheral AT, which may also be relevant in the BM. Thus, these findings collectively indicate distinct responses of BMAds and peripheral adipocytes to metabolic stressors (Figure 3).
Figure 3. NOX4-ROS signaling in AT-MSCs and BMSCs. The impact of oxidative stress induced by obesogenic conditions on stem cells varies between adipose tissue-derived AT-MSCs and bone marrow-derived BMSCs. In AT-MSCs, NOX-ROS signaling pathways predominantly affect adipogenesis and metabolic regulation. In obesogenic conditions, the metabolism of AT-MSCs is characterized by compromised insulin response and increased inflammation caused by increased expression of NF-κB, leading to impaired adipogenesis and insulin resistance. On the other hand, BMSCs manifest a distinctive insulin response in obesogenic conditions defined in BM by the absence of inflammation, which leads to different insulin responsiveness and activation of AKT signaling compared to AT. Notably, unlike AT-MSCs, insulin signaling in BMSCs is enhanced in obesity. Obesogenic BMSCs exhibit a molecular phenotype shift towards committed adipocytic progenitors and inhibition of Wnt signaling, a critical factor for OB differentiation. Despite this, increased ROS contribute to an enhanced senescent phenotype in both cell types. Created with BioRender.com.
Differential responses between peripheral AT and BMAT may be due to NOX4 expression levels which alter downstream signaling. Transcriptomic profiling revealed that NOX4 is more highly expressed in AT compared to BM (data available from v23.0.proteinatlas.org; https://www.proteinatlas.org/ENSG00000086991-NOX4/tissue#rna_expression). This disparity may suggest regulation beyond the transcriptional level through varying activation and posttranslational modifications of proteins involved in downstream metabolic pathways between the two cell types (Forrester et al., 2018). Further investigation is needed to unravel the distinct role of NOX4 expression in producing the distinct phenotype between these two tissues and to define the unique role of NOX4 in determining the pathophysiology of BM and bone metabolism in obesity.
Obesity is a significant risk factor for insulin resistance, a maladaptive metabolic state characterized by impaired insulin-mediated glucose uptake, changes in insulin secretion and dyslipidemia (Czech, 2017). Importantly, increased NOX4-ROS in AT due to obesity promote the generation of dysregulated metabolism through increased production of adipokines such as plasminogen activator inhibitor 1 (PAI-1), IL-6, and monocyte chemotactic protein-1, and decreases the generation of the insulin-sensitizing factor, adiponectin (Furukawa et al., 2004). Systemic inflammation is another hallmark of obesity linked to poor bone health (Iantomasi et al., 2023). NOX4-ROS contributes to this low-grade inflammation in AT, where inflammation drives obesity-induced impairment of insulin signaling (Den Hartigh et al., 2017). However, mixed findings surround the idea that inflammation caused by obesity disrupts insulin signaling in BMAT. These observations underscore the complexity of how systemic metabolism impacts BMAT homeostasis and expansion (Pham et al., 2020). In obesity, the BM does not demonstrate a clear increase in the inflammatory response compared to the periphery (Tencerova et al., 2018). This suggests the existence of a barrier within the BM, likely due to a distinct microenvironment that significantly influences the the stress response of BM cells (Tencerova et al., 2018; Tencerova et al., 2019a). Indeed, recent animal and clinical studies (Tencerova et al., 2018; Pham et al., 2020) did not observe insulin resistance in obese BMSCs and BMAT, further supporting the hypothesis that significant metabolic and molecular differences exist in the BM compartment versus peripheral tissues. Another study reported that BMAT is capable of insulin-stimulated AKT S473 phosphorylation but lacks AKT T308 phosphorylation (Suchacki et al., 2020). These data suggest a distinct mechanism for lipogenesis in BMAT, possibly less dependent on insulin than in peripheral AT. Thus, these findings offer potential mechanistic insight into the differential responses between BMAds and peripheral adipocytes to metabolic stress (Figure 3). Recent studies using specific NOX4 inhibitors in osteoporotic animal models showed promising results in improvement of bone loss (Woods et al., 2022). Thus, targeting NOX4 in obesity-induced bone fragility may be an interesting target for potential treatment in patients with metabolic bone diseases. However, further studies are needed to better understand the underlying mechanism in the regulation of cellular metabolism and inflammatory responses in BMAT in the context of obesity. While NOX4 generates ROS in both peripheral AT and BMAT, the ultimate impact of NOX4-ROS in metabolic bone diseases appears to depend on the distinct depot and microenvironments within these tissues.
BMAT is heterogeneous: There are different types of BMAT (constitutive vs. regulated), present in different regions of the BM (Li et al., 2018) exhibiting unique properties and responses to ROS. The studies might not account for this heterogeneity, potentially oversimplifying the conclusions.
In vivo models are lacking: The versatility of in vitro models has promoted significant gains in our understanding of the impact of ROS on BMSC differentiation and other instrumental properties of BM cells. However, they also demonstrate the need for in vivo validation due to the widely recognized microenvironmental nuances, the phenotypic heterogeneity and multifaceted roles of BMSCs within the BM niche. Very little in vivo research is available using cell-type-specific genetic modifications or target-specific molecules to elucidate the role of ROS on BMAT in osteoporosis and metabolic diseases.
Sexual dimorphism requires further exploration: Research on females and female-derived cells underrepresent the current knowledge of ROS and BMAT. Due to known sexual dimorphisms in BM adiposity, redox homeostasis, and BMSC properties, further investigation is necessary to understand these differences and their implications (Malorni et al., 2007; Lecka-Czernik et al., 2017; Beekman et al., 2022).
Translation to human physiology: Findings from animal models and in vitro studies may not always translate directly to human physiology. Differences between species and the controlled experimental conditions can limit the applicability of the results to clinical settings.
Taken together, the recent discoveries provide a strong rationale for closer exploration of NOX4-ROS signaling in BMSCs and BMAT, as well as its unique functions when compared to peripheral AT. The BM is rich in progenitors sensitive to cues for adipogenesis in response to stressors such as metabolic diseases and aging, perhaps mediated by NOX4-ROS. Increased evidence in the literature suggests that NOX4-ROS could drive bone fragility in obesity by influencing BMSC senescence, proliferation, and adipocyte differentiation, ultimately promoting the expansion of BMAT (Figure 4). However, the exact signaling pathways and effects of NOX4 in BMAT are still being elucidated. They likely involve interactions with factors like RANKL and OPG, which are crucial in bone remodeling. More mechanistic and clinical studies investigating tissue-specific NOX4-ROS signaling may bring a better understanding of the role of NOX4 in the regulation of bone-fat metabolism and its potential use in the treatment of metabolic bone disease.
Figure 4. Obesity-induced changes in AT and BM microenvironment via NOX4-ROS production. Obesity increases NOX4-ROS production in AT, which affects adipogenesis and AT inflammation. On the other hand, NOX4-ROS in BM microenvironment accelerates BMSC adipogenesis at the expense of osteogenic differentiation. Those changes lead to impaired glucose metabolism and increased bone fragility in obesity. Created with BioRender.com.
MD: Conceptualization, Data curation, Investigation, Methodology, Visualization, Writing–original draft. JB: Conceptualization, Data curation, Investigation, Writing–original draft. SC: Conceptualization, Funding acquisition, Supervision, Writing–review and editing. MT: Conceptualization, Funding acquisition, Supervision, Writing–review and editing.
The author(s) declare that financial support was received for the research, authorship, and/or publication of this article. This study was supported by START UP Research programme by IPHYS, the Czech Science Foundation (GACR 22-12243S; MT), EFSD/NovoNordisk foundation Future leaders award (NNF20SA0066174; MT), National Institute for Research of Metabolic and Cardiovascular Diseases (Program EXCELES, ID Project No. LX22NPO5104)—Funded by the European Union—Next Generation EU; MT), the Ministry of Health of the Czech Republic (NU23-01-00125; MT), the S. J. Ritchey HNFE Fund for Faculty Pilot Studies (SMC) and NIH K01AR073332 (SMC).
The authors declare that the research was conducted in the absence of any commercial or financial relationships that could be construed as a potential conflict of interest.
The author(s) declared that they were an editorial board member of Frontiers, at the time of submission. This had no impact on the peer review process and the final decision.
All claims expressed in this article are solely those of the authors and do not necessarily represent those of their affiliated organizations, or those of the publisher, the editors and the reviewers. Any product that may be evaluated in this article, or claim that may be made by its manufacturer, is not guaranteed or endorsed by the publisher.
Agidigbi, T. S., and Kim, C. (2019). Reactive oxygen species in osteoclast differentiation and possible pharmaceutical targets of ROS-mediated osteoclast diseases. Int. J. Mol. Sci. 20, 3576. doi:10.3390/ijms20143576
Alekos, N. S., Kushwaha, P., Kim, S. P., Li, Z., Abood, A., Dirckx, N., et al. (2023). Mitochondrial β-oxidation of adipose-derived fatty acids by osteoblasts fuels parathyroid hormone-induced bone formation. JCI Insight 8, e165604. doi:10.1172/jci.insight.165604
Ali, D., Chen, L., Kowal, J. M., Okla, M., Manikandan, M., AlShehri, M., et al. (2020). Resveratrol inhibits adipocyte differentiation and cellular senescence of human bone marrow stromal stem cells. Bone 133, 115252. doi:10.1016/j.bone.2020.115252
Atashi, F., Modarressi, A., and Pepper, M. S. (2015). The role of reactive oxygen species in mesenchymal stem cell adipogenic and osteogenic differentiation: a review. Stem Cells Dev. 24, 1150–1163. doi:10.1089/scd.2014.0484
Bai, X. C., Lu, D., Bai, J., Zheng, H., Ke, Z. Y., Li, X. M., et al. (2004). Oxidative stress inhibits osteoblastic differentiation of bone cells by ERK and NF-kappaB. Biochem. Biophys. Res. Commun. 314, 197–207. doi:10.1016/j.bbrc.2003.12.073
Balogh, E., Tolnai, E., Nagy, B., Nagy, B., Balla, G., Balla, J., et al. (2016). Iron overload inhibits osteogenic commitment and differentiation of mesenchymal stem cells via the induction of ferritin. Biochim. Biophys. Acta 1862, 1640–1649. doi:10.1016/j.bbadis.2016.06.003
Beckman, K. B., and Ames, B. N. (1998). The free radical theory of aging matures. Physiol. Rev. 78, 547–581. doi:10.1152/physrev.1998.78.2.547
Beekman, K. M., Duque, G., Corsi, A., Tencerova, M., Bisschop, P. H., and Paccou, J. (2023). Osteoporosis and bone marrow adipose tissue. Curr. Osteoporos. Rep. 21, 45–55. doi:10.1007/s11914-022-00768-1
Beekman, K. M., Regenboog, M., Nederveen, A. J., Bravenboer, N., den Heijer, M., Bisschop, P. H., et al. (2022). Gender- and age-associated differences in bone marrow adipose tissue and bone marrow fat unsaturation throughout the skeleton, quantified using chemical shift encoding-based water-fat MRI. Front. Endocrinol. (Lausanne) 13, 815835. doi:10.3389/fendo.2022.815835
Benova, A., and Tencerova, M. (2020). Obesity-induced changes in bone marrow homeostasis. Front. Endocrinol. (Lausanne) 11, 294. doi:10.3389/fendo.2020.00294
Bernard, K., Logsdon, N. J., Miguel, V., Benavides, G. A., Zhang, J., Carter, A. B., et al. (2017). NADPH oxidase 4 (Nox4) suppresses mitochondrial biogenesis and bioenergetics in lung fibroblasts via a nuclear factor erythroid-derived 2-like 2 (Nrf2)-dependent pathway. J. Biol. Chem. 292, 3029–3038. doi:10.1074/jbc.m116.752261
Bianco, P., Riminucci, M., Gronthos, S., and Robey, P. G. (2001). Bone marrow stromal stem cells: nature, biology, and potential applications. Stem Cells 19, 180–192. doi:10.1634/stemcells.19-3-180
Brown, D. I., and Griendling, K. K. (2009). Nox proteins in signal transduction. Free Radic. Biol. Med. 47, 1239–1253. doi:10.1016/j.freeradbiomed.2009.07.023
Chen, J. R., Lazarenko, O. P., Blackburn, M. L., Chen, J. F., Randolph, C. E., Zabaleta, J., et al. (2022). Nox4 expression in osteo-progenitors controls bone development in mice during early life. Commun. Biol. 5, 583. doi:10.1038/s42003-022-03544-0
Chen, K., Kirber, M. T., Xiao, H., Yang, Y., and Keaney, J. F. (2008). Regulation of ROS signal transduction by NADPH oxidase 4 localization. J. Cell Biol. 181, 1129–1139. doi:10.1083/jcb.200709049
Chen, X., Wang, Z., Duan, N., Zhu, G., Schwarz, E. M., and Xie, C. (2018). Osteoblast-osteoclast interactions. Connect. Tissue Res. 59, 99–107. doi:10.1080/03008207.2017.1290085
Czech, M. P. (2017). Insulin action and resistance in obesity and type 2 diabetes. Nat. Med. 23, 804–814. doi:10.1038/nm.4350
Darden, A. G., Ries, W. L., Wolf, W. C., Rodriguiz, R. M., and Key, L. L. (1996). Osteoclastic superoxide production and bone resorption: stimulation and inhibition by modulators of NADPH oxidase. J. Bone Min. Res. 11, 671–675. doi:10.1002/jbmr.5650110515
Del Fattore, A., Capannolo, M., and Rucci, N. (2010). Bone and bone marrow: the same organ. Arch. Biochem. Biophys. 503, 28–34. doi:10.1016/j.abb.2010.07.020
Deng, S., Dai, G., Chen, S., Nie, Z., Zhou, J., Fang, H., et al. (2019). Dexamethasone induces osteoblast apoptosis through ROS-PI3K/AKT/GSK3β signaling pathway. Biomed. Pharmacother. 110, 602–608. doi:10.1016/j.biopha.2018.11.103
Den Hartigh, L. J., Omer, M., Goodspeed, L., Wang, S., Wietecha, T., O'Brien, K. D., et al. (2017). Adipocyte-specific deficiency of NADPH oxidase 4 delays the onset of insulin resistance and attenuates adipose tissue inflammation in obesity. Arterioscler. Thromb. Vasc. Biol. 37, 466–475. doi:10.1161/ATVBAHA.116.308749
Di Bernardo, G., Messina, G., Capasso, S., Del Gaudio, S., Cipollaro, M., Peluso, G., et al. (2014). Sera of overweight people promote in vitro adipocyte differentiation of bone marrow stromal cells. Stem Cell Res. Ther. 5, 4. doi:10.1186/scrt393
Dominici, M., Le Blanc, K., Mueller, I., Slaper-Cortenbach, I., Marini, F., Krause, D., et al. (2006). Minimal criteria for defining multipotent mesenchymal stromal cells. The International Society for Cellular Therapy position statement. Cytotherapy 8, 315–317. doi:10.1080/14653240600855905
Farr, J. N., Xu, M., Weivoda, M. M., Monroe, D. G., Fraser, D. G., Onken, J. L., et al. (2017). Targeting cellular senescence prevents age-related bone loss in mice. Nat. Med. 23, 1072–1079. doi:10.1038/nm.4385
Finkel, T. (2011). Signal transduction by reactive oxygen species. J. Cell Biol. 194, 7–15. doi:10.1083/jcb.201102095
Forrester, S. J., Kikuchi, D. S., Hernandes, M. S., Xu, Q., and Griendling, K. K. (2018). Reactive oxygen species in metabolic and inflammatory signaling. Circ. Res. 122, 877–902. doi:10.1161/circresaha.117.311401
Furukawa, S., Fujita, T., Shimabukuro, M., Iwaki, M., Yamada, Y., Nakajima, Y., et al. (2004). Increased oxidative stress in obesity and its impact on metabolic syndrome. J. Clin. Invest 114, 1752–1761. doi:10.1172/jci21625
Gautam, J., Khedgikar, V., Kushwaha, P., Choudhary, D., Nagar, G. K., Dev, K., et al. (2017). Formononetin, an isoflavone, activates AMP-activated protein kinase/β-catenin signalling to inhibit adipogenesis and rescues C57BL/6 mice from high-fat diet-induced obesity and bone loss. Br. J. Nutr. 117, 645–661. doi:10.1017/S0007114517000149
Goettsch, C., Babelova, A., Trummer, O., Erben, R. G., Rauner, M., Rammelt, S., et al. (2013). NADPH oxidase 4 limits bone mass by promoting osteoclastogenesis. J. Clin. Invest 123, 4731–4738. doi:10.1172/JCI67603
Guimaraes, G. C., Coelho, J. B. C., Silva, J. G. O., de Sant'Ana, A. C. C., de Sa, C. A. C., Moreno, J. M., et al. (2023). Obesity, diabetes and risk of bone fragility: how BMAT behavior is affected by metabolic disturbances and its influence on bone health. Osteoporos. Int. 35, 575–588. doi:10.1007/s00198-023-06991-5
Guntur, A. R., Gerencser, A. A., Le, P. T., DeMambro, V. E., Bornstein, S. A., Mookerjee, S. A., et al. (2018). Osteoblast-like mc3t3-E1 cells prefer glycolysis for ATP production but adipocyte-like 3T3-L1 cells prefer oxidative phosphorylation. J. Bone Min. Res. 33, 1052–1065. doi:10.1002/jbmr.3390
Ha, H., Kwak, H. B., Lee, S. W., Jin, H. M., Kim, H. M., Kim, H. H., et al. (2004). Reactive oxygen species mediate RANK signaling in osteoclasts. Exp. Cell Res. 301, 119–127. doi:10.1016/j.yexcr.2004.07.035
Halade, G. V., El Jamali, A., Williams, P. J., Fajardo, R. J., and Fernandes, G. (2011). Obesity-mediated inflammatory microenvironment stimulates osteoclastogenesis and bone loss in mice. Exp. Gerontol. 46, 43–52. doi:10.1016/j.exger.2010.09.014
Han, J., Park, D., Park, J. Y., and Han, S. (2022). Inhibition of NADPH oxidases prevents the development of osteoarthritis. Antioxidants (Basel) 11, 2346. doi:10.3390/antiox11122346
Hardouin, P., Rharass, T., and Lucas, S. (2016). Bone marrow adipose tissue: to Be or not to Be a typical adipose tissue? Front. Endocrinol. (Lausanne) 7, 85. doi:10.3389/fendo.2016.00085
Herrmann, M. (2019). Marrow fat-secreted factors as biomarkers for osteoporosis. Curr. Osteoporos. Rep. 17, 429–437. doi:10.1007/s11914-019-00550-w
Houschyar, K. S., Tapking, C., Borrelli, M. R., Popp, D., Duscher, D., Maan, Z. N., et al. (2018). Wnt pathway in bone repair and regeneration - what do we know so far. Front. Cell Dev. Biol. 6, 170. doi:10.3389/fcell.2018.00170
Iantomasi, T., Romagnoli, C., Palmini, G., Donati, S., Falsetti, I., Miglietta, F., et al. (2023). Oxidative stress and inflammation in osteoporosis: molecular mechanisms involved and the relationship with microRNAs. Int. J. Mol. Sci. 24, 3772. doi:10.3390/ijms24043772
Jones, D. P. (2006). Redefining oxidative stress. Antioxid. Redox Signal 8, 1865–1879. doi:10.1089/ars.2006.8.1865
Joo, J. H., Huh, J. E., Lee, J. H., Park, D. R., Lee, Y., Lee, S. G., et al. (2016). A novel pyrazole derivative protects from ovariectomy-induced osteoporosis through the inhibition of NADPH oxidase. Sci. Rep. 6, 22389. doi:10.1038/srep22389
Kajla, S., Mondol, A. S., Nagasawa, A., Zhang, Y., Kato, M., Matsuno, K., et al. (2012). A crucial role for Nox 1 in redox-dependent regulation of Wnt-β-catenin signaling. FASEB J. 26, 2049–2059. doi:10.1096/fj.11-196360
Kim, J. H., Song, S. Y., Park, S. G., Song, S. U., Xia, Y., and Sung, J. H. (2012). Primary involvement of NADPH oxidase 4 in hypoxia-induced generation of reactive oxygen species in adipose-derived stem cells. Stem Cells Dev. 21, 2212–2221. doi:10.1089/scd.2011.0561
Kim, M. J., Kim, H. J., Hong, Y. H., Lee, C. K., Kim, Y. W., Shon, O. J., et al. (2015). Age-related NADPH oxidase (arNOX) activity correlated with cartilage degradation and bony changes in age-related osteoarthritis. J. Korean Med. Sci. 30, 1246–1252. doi:10.3346/jkms.2015.30.9.1246
Koundouros, N., and Poulogiannis, G. (2018). Phosphoinositide 3-kinase/akt signaling and redox metabolism in cancer. Front. Oncol. 8, 160. doi:10.3389/fonc.2018.00160
Kricun, M. E. (1985). Red-yellow marrow conversion: its effect on the location of some solitary bone lesions. Skelet. Radiol. 14, 10–19. doi:10.1007/BF00361188
Lanske, B., and Rosen, C. (2017). Bone marrow adipose tissue: the first 40 years. J. Bone Min. Res. 32, 1153–1156. doi:10.1002/jbmr.3140
Lecka-Czernik, B., Stechschulte, L. A., Czernik, P. J., Sherman, S. B., Huang, S., and Krings, A. (2017). Marrow adipose tissue: skeletal location, sexual dimorphism, and response to sex steroid deficiency. Front. Endocrinol. (Lausanne) 8, 188. doi:10.3389/fendo.2017.00188
Lee, C. F., Qiao, M., Schroder, K., Zhao, Q., and Asmis, R. (2010). Nox4 is a novel inducible source of reactive oxygen species in monocytes and macrophages and mediates oxidized low density lipoprotein-induced macrophage death. Circ. Res. 106, 1489–1497. doi:10.1161/CIRCRESAHA.109.215392
Li, D., Zhang, R., Zhu, W., Xue, Y., Zhang, Y., Huang, Q., et al. (2013). S100A16 inhibits osteogenesis but stimulates adipogenesis. Mol. Biol. Rep. 40, 3465–3473. doi:10.1007/s11033-012-2413-2
Li, G. W., Xu, Z., Chen, Q. W., Tian, Y. N., Wang, X. Y., Zhou, L., et al. (2014). Quantitative evaluation of vertebral marrow adipose tissue in postmenopausal female using MRI chemical shift-based water-fat separation. Clin. Radiol. 69, 254–262. doi:10.1016/j.crad.2013.10.005
Li, Z., Bowers, E., Zhu, J., Yu, H., Hardij, J., Bagchi, D. P., et al. (2022). Lipolysis of bone marrow adipocytes is required to fuel bone and the marrow niche during energy deficits. Elife 11, e78496. doi:10.7554/eLife.78496
Li, Z., Hardij, J., Bagchi, D. P., Scheller, E. L., and MacDougald, O. A. (2018). Development, regulation, metabolism and function of bone marrow adipose tissues. Bone 110, 134–140. doi:10.1016/j.bone.2018.01.008
Liao, L., Yang, X., Su, X., Hu, C., Zhu, X., Yang, N., et al. (2013). Redundant miR-3077-5p and miR-705 mediate the shift of mesenchymal stem cell lineage commitment to adipocyte in osteoporosis bone marrow. Cell Death Dis. 4, e600. doi:10.1038/cddis.2013.130
Liu, L. F., Shen, W. J., Ueno, M., Patel, S., and Kraemer, F. B. (2011). Characterization of age-related gene expression profiling in bone marrow and epididymal adipocytes. BMC Genomics 12, 212. doi:10.1186/1471-2164-12-212
Liu, Q., Jin, L., Shen, F. H., Balian, G., and Li, X. J. (2013). Fullerol nanoparticles suppress inflammatory response and adipogenesis of vertebral bone marrow stromal cells--a potential novel treatment for intervertebral disc degeneration. Spine J. 13, 1571–1580. doi:10.1016/j.spinee.2013.04.004
Liu, Y., Shi, C., He, Z., Zhu, F., Wang, M., He, R., et al. (2021). Inhibition of PI3K/AKT signaling via ROS regulation is involved in Rhein-induced apoptosis and enhancement of oxaliplatin sensitivity in pancreatic cancer cells. Int. J. Biol. Sci. 17, 589–602. doi:10.7150/ijbs.49514
Lorenzo, J. (2017). The many ways of osteoclast activation. J. Clin. Invest 127, 2530–2532. doi:10.1172/JCI94606
Luo, M. L., Jiao, Y., Gong, W. P., Li, Y., Niu, L. N., Tay, F. R., et al. (2020). Macrophages enhance mesenchymal stem cell osteogenesis via down-regulation of reactive oxygen species. J. Dent. 94, 103297. doi:10.1016/j.jdent.2020.103297
Mahadev, K., Wu, X., Zilbering, A., Zhu, L., Lawrence, J. T., and Goldstein, B. J. (2001). Hydrogen peroxide generated during cellular insulin stimulation is integral to activation of the distal insulin signaling cascade in 3T3-L1 adipocytes. J. Biol. Chem. 276, 48662–48669. doi:10.1074/jbc.M105061200
Malorni, W., Campesi, I., Straface, E., Vella, S., and Franconi, F. (2007). Redox features of the cell: a gender perspective. Antioxid. Redox Signal 9, 1779–1801. doi:10.1089/ars.2007.1596
Martyn, K. D., Frederick, L. M., von Loehneysen, K., Dinauer, M. C., and Knaus, U. G. (2006). Functional analysis of Nox4 reveals unique characteristics compared to other NADPH oxidases. Cell Signal 18, 69–82. doi:10.1016/j.cellsig.2005.03.023
Miggitsch, C., Meryk, A., Naismith, E., Pangrazzi, L., Ejaz, A., Jenewein, B., et al. (2019). Human bone marrow adipocytes display distinct immune regulatory properties. EBioMedicine 46, 387–398. doi:10.1016/j.ebiom.2019.07.023
Pang, W. W., Price, E. A., Sahoo, D., Beerman, I., Maloney, W. J., Rossi, D. J., et al. (2011). Human bone marrow hematopoietic stem cells are increased in frequency and myeloid-biased with age. Proc. Natl. Acad. Sci. U. S. A. 108, 20012–20017. doi:10.1073/pnas.1116110108
Peci, F., Dekker, L., Pagliaro, A., van Boxtel, R., Nierkens, S., and Belderbos, M. (2022). The cellular composition and function of the bone marrow niche after allogeneic hematopoietic cell transplantation. Bone Marrow Transpl. 57, 1357–1364. doi:10.1038/s41409-022-01728-0
Pham, T. T., Ivaska, K. K., Hannukainen, J. C., Virtanen, K. A., Lidell, M. E., Enerback, S., et al. (2020). Human bone marrow adipose tissue is a metabolically active and insulin-sensitive distinct fat depot. J. Clin. Endocrinol. Metab. 105, 2300–2310. doi:10.1210/clinem/dgaa216
Rahman, M. M., El Jamali, A., Halade, G. V., Ouhtit, A., Abou-Saleh, H., and Pintus, G. (2018). Nox2 activity is required in obesity-mediated alteration of bone remodeling. Oxid. Med. Cell Longev. 2018, 6054361. doi:10.1155/2018/6054361
Rharass, T., and Lucas, S. (2019). High glucose level impairs human mature bone marrow adipocyte function through increased ROS production. Front. Endocrinol. (Lausanne) 10, 607. doi:10.3389/fendo.2019.00607
Rhee, S. G., Bae, Y. S., Lee, S. R., and Kwon, J. (2000). Hydrogen peroxide: a key messenger that modulates protein phosphorylation through cysteine oxidation. Sci. STKE 2000, pe1. doi:10.1126/stke.2000.53.pe1
Richard, A. J., White, U., Elks, C. M., and Stephens, J. M. (2000). Adipose tissue: physiology to metabolic dysfunction. Editors K. R. Feingold, B. Anawalt, M. R. Blackman, A. Boyce, G. Chrousos, E. Corpaset al. (South Dartmouth (MA): Endotext).
Rossi, L., Challen, G. A., Sirin, O., Lin, K. K., and Goodell, M. A. (2011). Hematopoietic stem cell characterization and isolation. Methods Mol. Biol. 750, 47–59. doi:10.1007/978-1-61779-145-1_3
Schroder, K. (2019). NADPH oxidases in bone homeostasis and osteoporosis. Free Radic. Biol. Med. 132, 67–72. doi:10.1016/j.freeradbiomed.2018.08.036
Schroder, K., Wandzioch, K., Helmcke, I., and Brandes, R. P. (2009). Nox4 acts as a switch between differentiation and proliferation in preadipocytes. Arterioscler. Thromb. Vasc. Biol. 29, 239–245. doi:10.1161/ATVBAHA.108.174219
Shafat, M. S., Oellerich, T., Mohr, S., Robinson, S. D., Edwards, D. R., Marlein, C. R., et al. (2017). Leukemic blasts program bone marrow adipocytes to generate a protumoral microenvironment. Blood 129, 1320–1332. doi:10.1182/blood-2016-08-734798
Shanmugasundaram, K., Nayak, B. K., Friedrichs, W. E., Kaushik, D., Rodriguez, R., and Block, K. (2017). NOX4 functions as a mitochondrial energetic sensor coupling cancer metabolic reprogramming to drug resistance. Nat. Commun. 8, 997. doi:10.1038/s41467-017-01106-1
Shen, W., Velasquez, G., Chen, J., Jin, Y., Heymsfield, S. B., Gallagher, D., et al. (2014). Comparison of the relationship between bone marrow adipose tissue and volumetric bone mineral density in children and adults. J. Clin. Densitom. 17, 163–169. doi:10.1016/j.jocd.2013.02.009
Sies, H. (2020). Oxidative stress: concept and some practical aspects. Antioxidants (Basel) 9, 852. doi:10.3390/antiox9090852
Specht, K. S., Kant, S., Addington, A. K., McMillan, R. P., Hulver, M. W., Learnard, H., et al. (2021). Nox4 mediates skeletal muscle metabolic responses to exercise. Mol. Metab. 45, 101160. doi:10.1016/j.molmet.2020.101160
Staehlke, S., Haack, F., Waldner, A. C., Koczan, D., Moerke, C., Mueller, P., et al. (2020). ROS dependent wnt/β-catenin pathway and its regulation on defined micro-pillars-A combined in vitro and in silico study. Cells 9, 1784. doi:10.3390/cells9081784
Stockman, R., and Greig, E. D. W. (1898). The action of arsenic on the bone-marrow and blood. J. Physiol. 23 (1898), 376–382. doi:10.1113/jphysiol.1898.sp000734
Suchacki, K. J., and Cawthorn, W. P. (2018). Molecular interaction of bone marrow adipose tissue with energy metabolism. Curr. Mol. Biol. Rep. 4, 41–49. doi:10.1007/s40610-018-0096-8
Suchacki, K. J., Tavares, A. A. S., Mattiucci, D., Scheller, E. L., Papanastasiou, G., Gray, C., et al. (2020). Bone marrow adipose tissue is a unique adipose subtype with distinct roles in glucose homeostasis. Nat. Commun. 11, 3097. doi:10.1038/s41467-020-16878-2
Sui, B., Hu, C., Liao, L., Chen, Y., Zhang, X., Fu, X., et al. (2016). Mesenchymal progenitors in osteopenias of diverse pathologies: differential characteristics in the common shift from osteoblastogenesis to adipogenesis. Sci. Rep. 6, 30186. doi:10.1038/srep30186
Sun, J., Chen, W., Li, S., Yang, S., Zhang, Y., Hu, X., et al. (2021). Nox4 promotes RANKL-induced autophagy and osteoclastogenesis via activating ROS/PERK/eIF-2α/ATF4 pathway. Front. Pharmacol. 12, 751845. doi:10.3389/fphar.2021.751845
Tabe, Y., Yamamoto, S., Saitoh, K., Sekihara, K., Monma, N., Ikeo, K., et al. (2017). Bone marrow adipocytes facilitate fatty acid oxidation activating AMPK and a transcriptional network supporting survival of acute monocytic leukemia cells. Cancer Res. 77, 1453–1464. doi:10.1158/0008-5472.can-16-1645
Takac, I., Schroder, K., Zhang, L., Lardy, B., Anilkumar, N., Lambeth, J. D., et al. (2011). The E-loop is involved in hydrogen peroxide formation by the NADPH oxidase Nox4. J. Biol. Chem. 286, 13304–13313. doi:10.1074/jbc.M110.192138
Tencerova, M., Ferencakova, M., and Kassem, M. (2021). Bone marrow adipose tissue: role in bone remodeling and energy metabolism. Best. Pract. Res. Clin. Endocrinol. Metab. 35, 101545. doi:10.1016/j.beem.2021.101545
Tencerova, M., Figeac, F., Ditzel, N., Taipaleenmaki, H., Nielsen, T. K., and Kassem, M. (2018). High-fat diet-induced obesity promotes expansion of bone marrow adipose tissue and impairs skeletal stem cell functions in mice. J. Bone Min. Res. 33, 1154–1165. doi:10.1002/jbmr.3408
Tencerova, M., Frost, M., Figeac, F., Nielsen, T. K., Ali, D., Lauterlein, J. L., et al. (2019a). Obesity-associated hypermetabolism and accelerated senescence of bone marrow stromal stem cells suggest a potential mechanism for bone fragility. Cell Rep. 27, 2050–2062 e6. doi:10.1016/j.celrep.2019.04.066
Tencerova, M., and Kassem, M. (2016). The bone marrow-derived stromal cells: commitment and regulation of adipogenesis. Front. Endocrinol. (Lausanne) 7, 127. doi:10.3389/fendo.2016.00127
Tencerova, M., Rendina-Ruedy, E., Neess, D., Faergeman, N., Figeac, F., Ali, D., et al. (2019b). Metabolic programming determines the lineage-differentiation fate of murine bone marrow stromal progenitor cells. Bone Res. 7, 35. doi:10.1038/s41413-019-0076-5
van Dalen, S. C. M., Kruisbergen, N. N. L., Walgreen, B., Helsen, M. M. A., Sloetjes, A. W., Cremers, N. A. J., et al. (2018). The role of NOX2-derived reactive oxygen species in collagenase-induced osteoarthritis. Osteoarthr. Cartil. 26, 1722–1732. doi:10.1016/j.joca.2018.08.014
Vieceli Dalla Sega, F., Prata, C., Zambonin, L., Angeloni, C., Rizzo, B., Hrelia, S., et al. (2017). Intracellular cysteine oxidation is modulated by aquaporin-8-mediated hydrogen peroxide channeling in leukaemia cells. Biofactors 43, 232–242. doi:10.1002/biof.1340
Wang, N., Wang, L., Yang, J., Wang, Z., and Cheng, L. (2021). Quercetin promotes osteogenic differentiation and antioxidant responses of mouse bone mesenchymal stem cells through activation of the AMPK/SIRT1 signaling pathway. Phytother. Res. 35, 2639–2650. doi:10.1002/ptr.7010
Wang, Y., Liu, L., Pazhanisamy, S. K., Li, H., Meng, A., and Zhou, D. (2010). Total body irradiation causes residual bone marrow injury by induction of persistent oxidative stress in murine hematopoietic stem cells. Free Radic. Biol. Med. 48, 348–356. doi:10.1016/j.freeradbiomed.2009.11.005
Wang, Y., Zou, X., Guo, Y., Wang, L., Liu, Y., Zeng, Q., et al. (2015). Mechanical strain affects some microrna profiles in pre-oeteoblasts. Cell Mol. Biol. Lett. 20, 586–596. doi:10.1515/cmble-2015-0034
Wauquier, F., Leotoing, L., Coxam, V., Guicheux, J., and Wittrant, Y. (2009). Oxidative stress in bone remodelling and disease. Trends Mol. Med. 15, 468–477. doi:10.1016/j.molmed.2009.08.004
Woods, G. N., Ewing, S. K., Schafer, A. L., Gudnason, V., Sigurdsson, S., Lang, T., et al. (2022). Saturated and unsaturated bone marrow lipids have distinct effects on bone density and fracture risk in older adults. J. Bone Min. Res. 37, 700–710. doi:10.1002/jbmr.4504
Wu, Z., Hou, Q., Chen, T., Jiang, X., Wang, L., Xu, J., et al. (2022). ROS-reactive PMS/PC drug delivery system improves new bone formation under diabetic conditions by promoting angiogenesis-osteogenesis coupling via down-regulating NOX2-ROS signalling axis. Biomaterials 291, 121900. doi:10.1016/j.biomaterials.2022.121900
Yan, W., Diao, S., and Fan, Z. (2021). The role and mechanism of mitochondrial functions and energy metabolism in the function regulation of the mesenchymal stem cells. Stem Cell Res. Ther. 12, 140. doi:10.1186/s13287-021-02194-z
Yang, S., Zhang, Y., Ries, W., and Key, L. (2004). Expression of Nox4 in osteoclasts. J. Cell Biochem. 92, 238–248. doi:10.1002/jcb.20048
Yeung, D. K., Griffith, J. F., Antonio, G. E., Lee, F. K., Woo, J., and Leung, P. C. (2005). Osteoporosis is associated with increased marrow fat content and decreased marrow fat unsaturation: a proton MR spectroscopy study. J. Magn. Reson Imaging 22, 279–285. doi:10.1002/jmri.20367
Keywords: bone marrow stromal cells, bone marrow adipose tissue, ROS, NADPH oxidase, bone fragility, obesity, senescence
Citation: Dzubanova M, Bond JM, Craige SM and Tencerova M (2024) NOX4-reactive oxygen species axis: critical regulators of bone health and metabolism. Front. Cell Dev. Biol. 12:1432668. doi: 10.3389/fcell.2024.1432668
Received: 14 May 2024; Accepted: 29 July 2024;
Published: 12 August 2024.
Edited by:
Ming Li, Osaka University, JapanReviewed by:
Kshitiz Raj Shrestha, Independent Researcher, Kathmandu, NepalCopyright © 2024 Dzubanova, Bond, Craige and Tencerova. This is an open-access article distributed under the terms of the Creative Commons Attribution License (CC BY). The use, distribution or reproduction in other forums is permitted, provided the original author(s) and the copyright owner(s) are credited and that the original publication in this journal is cited, in accordance with accepted academic practice. No use, distribution or reproduction is permitted which does not comply with these terms.
*Correspondence: Michaela Tencerova, bWljaGFlbGEudGVuY2Vyb3ZhQGZndS5jYXMuY3o=
†These authors have contributed equally to this work and share first authorship
‡These authors have contributed equally to this work and share last authorship
Disclaimer: All claims expressed in this article are solely those of the authors and do not necessarily represent those of their affiliated organizations, or those of the publisher, the editors and the reviewers. Any product that may be evaluated in this article or claim that may be made by its manufacturer is not guaranteed or endorsed by the publisher.
Research integrity at Frontiers
Learn more about the work of our research integrity team to safeguard the quality of each article we publish.