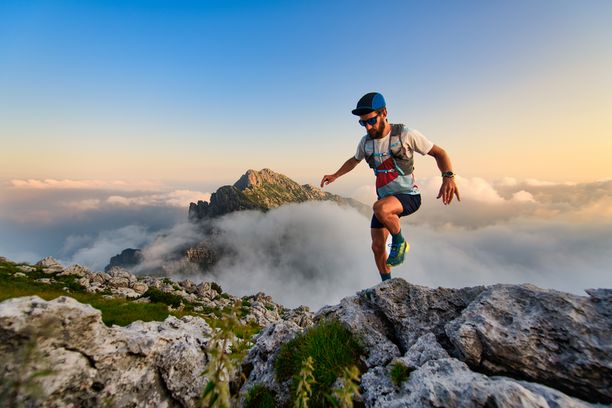
94% of researchers rate our articles as excellent or good
Learn more about the work of our research integrity team to safeguard the quality of each article we publish.
Find out more
MINI REVIEW article
Front. Cell Dev. Biol., 25 June 2024
Sec. Stem Cell Research
Volume 12 - 2024 | https://doi.org/10.3389/fcell.2024.1426395
This article is part of the Research TopicRegulation of Tissue Microenvironment on Stem Cells RegenerationView all 4 articles
Cerebrospinal fluid-contacting neurons (CSF-cNs) represent a distinct group of interneurons characterized by their prominent apical globular protrusions penetrating the spinal cord’s central canal and their basal axons extending towards adjacent cells. Identified nearly a century back, the specific roles and attributes of CSF-cNs have just started to emerge due to the historical lack of definitive markers. Recent findings have confirmed that CSF-cNs expressing PKD2L1 possess attributes of neural stem cells, suggesting a critical function in the regeneration processes following spinal cord injuries. This review aims to elucidate the molecular markers of CSF-cNs as potential neural stem cells during spinal cord development and assess their roles post-spinal cord injury, with an emphasis on their potential therapeutic implications for spinal cord repair.
CSF-cNs, a distinct neuronal class with direct connections to cerebrospinal fluid (CSF), were first depicted by Kolmer and Agduhr (Kolmer, 1921; Agduhr, 1922). Subsequently, Vign et al. investigated the unique morphology of CSF-cNs using electron microscopy and described CSF-cNs in most vertebrate spinal cords (Vigh-Teichmann and VIGH, 1983a). According to the report, dendritic terminals of CSF-cNs extend into the spinal cord’s central canal (Dale et al., 1987a; Vigh and Vigh-Teichmann, 1998). Further research demonstrated that, in some species, the terminals possess multiple microvilli (Vigh et al., 1983; Böhm et al., 2016), or motile cilia (Vigh and Vigh-Teichmann, 1973; Vigh-Teichmann and Vigh, 1983b; Vígh et al., 2004).
Spinal cord injury (SCI) is an intractable and highly disabling condition, lacking effective therapeutic strategy (Tohda and Kuboyama, 2011). Recent advances have positioned neural stem cell (NSC) transplantation as a focal point in spinal cord injury treatment. However, the outcomes of transplantation vary widely (Reekmans et al., 2012; Gao et al., 2013; Huang et al., 2022). There remains a substantial scientific debate concerning the origin of NSCs in the spinal cord of mammals (Teng et al., 2008; Finkel et al., 2021). Recent studies confirmed that CSF-cNs possess NSC properties (Wang et al., 2021a), positioning them as critical for endogenous spinal cord repair.
In this review, we outline the primary biomarkers of CSF-cNs and their pivotal role in spinal cord injury therapeutic application. Cao et al. (2022) have identified CSF-cNs as possessing neural stem cell (NSC) potential, marked by their ability to form neurospheres and express NSC markers, suggesting a role in neural regeneration and repair post-spinal cord injury. Importantly, CSF-cNs exhibit multipotent differentiation capabilities; they can develop into neurons, astrocytes, and oligodendrocytes in vitro. This capacity not only underscores the versatile potential of CSF-cNs in contributing to neural regeneration but also opens new therapeutic avenues for spinal cord injury treatment. The discovery of CSF-cNs as a novel NSC type enriches our understanding of neuroregeneration mechanisms within the spinal cord, and aids in the development of innovative strategies for enhancing functional recovery post-SCI. By elucidating the characteristics and mechanisms of CSF-cNs, this research provides a foundation for improving rehabilitation techniques and treatment outcomes for spinal cord injury patients (Cao et al., 2022).
For decades, research on CSF-cNs was impeded due to the absence of distinct markers. In 2006, Huang et al. (2006) advanced this field by identifying PKD2L1 channels as specific markers for CSF-cNs. Further, transcription factors like GATA2 and GATA3 could help to indentify CSF-cNs (Petracca et al., 2016a; Andrzejczuk et al., 2018). Moreover, the detection of GABA, acid-sensing ion channels (ASICs), and various neuromodulators in CSF-cNs has broadened the understanding of their properties and functions (Dale et al., 1987c).
CSF-cNs in taste buds responsible for sour tastedetection express PKD2L1 (Huang et al., 2006), and this channel is typically involved in the detection of chemical, thermal, and mechanical stimuli (Retailleau and Duprat, 2014; Hussein, 2015). Among the seven subfamilies of TRPs, one well-known channel, PKD2L1, belongs to the transient receptor potential polycystin (TRPP) subfamily, which includes polycystic kidney disease (PKD) proteins (Vígh et al., 2004; Orts-Del’immagine et al., 2012a). Notably, the PKD2L1 channel remains consistent in CSF-cNs in the spinal cord (Djenoune et al., 2014). CSF-cNs selectively express PKD2L1 (Orts-Del'Immagine et al., 2016a; Orts-Del’Immagine et al., 2014a; Orts-Del’Immagine et al., 2017), which is now widely considered a specific marker for CSF-cNs (Wyart et al., 2023). When extracellular or intracellular calcium levels are elevated, or in hypotonic conditions, this channel facilitates large current of CSF-cNs (Orts-Del'Immagine et al., 2016a; Jurčić et al., 2019). PKD2L1 is capable of generating significant depolarizations, acting as a spike generator that can initiate action potentials (Orts-Del'Immagine et al., 2016a; Sternberg et al., 2018a). Additionally, PKD1L2 and PKD2L1 co-expression in CSF-cNs suggests a regulatory interaction (Petracca et al., 2016b; England et al., 2017), moderating neuronal excitation by influencing PKD2L1’s membrane localization (Orts-Del'Immagine et al., 2016b). This elucidates the physiological roles of CSF-cNs, guided by the pivotal discovery of PKD2L1 (Orts-Del'Immagine and Wyart, 2017).
γ-aminobutyric acid (GABA) serves as the primary inhibitory neurotransmitter in the CNS (Roberts, 1974; Watanabe et al., 2002), transitioning from excitatory to inhibitory effects during neuronal maturation (Ben-Ari, 2014). GABA expression in CSF-cNs has been observed across various species (Dale et al., 1987b; Feldblum et al., 1995; Binor and Heathcote, 2001; Yang et al., 2010). Dale et al. described a specific CSF-cN subclass in the spinal cords of frog embryos, located ventrolaterally (Dale et al., 1987c; Dale et al., 1987d). Detailed anatomical studies on CSF-cNs, including their axonal projections, developmental stages, and distribution in amphibians and zebrafish, have employed anti-GABA and glutamic acid decarboxylase (GAD) antibodies for accurate immunolabeling (Bernhardt et al., 1992; Binor and Heathcote, 2001). These neurons are classified as GABAergic through immunohistochemical techniques (Djenoune et al., 2014; Djenoune and Wyart, 2017a). The identification of CSF-cNs primarily depends on their GABAergic properties, proximity to the central canal, and lumen contact (Dale et al., 1987c).
Transcription factors are pivotal in the generation of CSF-cNs. Ascl1, a basic helix-loop-helix (bHLH) transcription factor, governs the balance between ependymal cells and CSF-cNs in the spinal cord’s central canal. It is notably expressed in the precursor cells of CSF-cNs, facilitating proper cell population distribution (Di Bella et al., 2019a). Nkx6.1, another transcription factor, promotes the differentiation of motoneurons, with PKD2L1+ CSF-cNs express Nkx6.1 (Orts-Del'Immagine et al., 2014a). Additionally, The co-expression of Nkx6.1 and Pax6 serves as a marker for CSF-cNs (Petracca et al., 2016b). Other significant transcription factors, Nkx2.2 and Foxa2, are involved in embryonic development and differentiation and also mark CSF-cNs (Di Bella et al., 2019b). Although PKD2L1 has traditionally been a primary identifier for CSF-cNs (Djenoune et al., 2014; Djenoune et al., 2017a; Sternberg et al., 2018a), the specific combination of PKD2L1 with GATA2 and GATA3 has been proposed as a more comprehensive marker (Petracca et al., 2016b). These transcription factors have been consistently used in recent studies for identifying CSF-cNs (Di Bella et al., 2019a), which may enhance future research methodologies.
Acid-base homeostasis is essential for organism survival, with ASICs playing a pivotal role as proton-gated excitatory cation channels responsive to pH changes (Boscardin et al., 2016; Soto et al., 2018). ASIC3, the most widely expressed subtype in sensory neurons and nerve terminals, translates mechanical and nociceptive stimuli into electrical signals (Chen and Wong, 2013). Present both peripherally and in the CNS (Deval and Lingueglia, 2015), ASIC3 functions as an electrochemical sensor for somatic and visceral nociception (Leppert et al., 2016). Peripherally, it is essential for managing inflammatory pain and modulating pain signals, including mechano-sensation, at the spinal cord level (Zha, 2013). CSF-cNs, expressing ASIC3, respond to both mechanical stimulation and pH variations (Jalalvand et al., 2016a), with their acidic response and fluid-induced mechanical reactions mediated by ASIC3 channels (Jalalvand et al., 2016b; Jalalvand et al., 2018).
Neuropeptides critically regulate CSF-cNs, maintaining neural function and nervous system health (Smith et al., 2004). CSF-cNs serve as production sites for neuropeptides Urp1 and Urp2 (Quan et al., 2015; Zhang et al., 2018; Gaillard et al., 2023), primarily co-expressed in zebrafish spinal cords (Quan et al., 2015). Additionally, somatostatin 1.1 (sst1.1) is uniquely present in the dorsal CSF-cNs of zebrafish, marking this specific subset (Djenoune et al., 2017b). Neuropeptide C (Nppc), urocortin 3 (Ucn3), and tachykinin 3 (Tac3) also serve as biomarkers for CSF-cNs in zebrafish (Wilson et al., 2017; Prendergast et al., 2023). In contrast, in macaque monkeys, the vasoactive intestinal peptide (VIP) is a distinct marker for CSF-cNs (LaMotte, 1987).
Secretory proteins from CSF-cNs are integral for modulating neuronal activity and enhancing communication (Vígh et al., 2004; Orts-Del’immagine et al., 2012a). Notably, prostate-associated microseminoprotein (Msmp) is recognized as a potential biomarker for CSF-cNs (Prendergast et al., 2023). Additionally, secretogranin-2 (Scg2), important in neurosecretion (Wyart et al., 2023), and the enzyme aromatic L-amino acid decarboxylase (AADC), which synthesizes trace amines, are expressed in these neurons (Wyart et al., 2023). In conclusion, CSF-cNs exhibit distinct features crucial for spinal cord repair, including prominent apical protrusions that facilitate cellular interactions. Cao et al. (2022) highlight the expression of PKD2L1 in CSF-cNs, suggesting their role as neural stem cells aiding neuroregeneration post-SCI and their involvement in maintaining cellular structure, connectivity, and internal regulation.
Monoamines have a substantial impact on the modulating the excitatory and inhibitory of CSF-cNs (Djenoune and Wyart, 2017a). Dopamine, a key monoamine, is essential for the functional regulation of CSF-cNs (Orts-Del’Immagine et al., 2014b). Studies conducted previously have documented the synthesis of dopamine by ventral CSF-cNs in lampreys (Wyart et al., 2023). Additionally, transient expression of serotonin in CSF-cNs of fish (Montgomery et al., 2016; Djenoune et al., 2017b) and birds (Vígh et al., 2004; Orts-Del’immagine et al., 2012a) has been observed, with the biosynthesis mediated by tryptophan hydroxylase 2 (TPH2) (Hirunagi et al., 1992).
Structural proteins such as Myo3b and spin are crucial for maintaining cellular morphology and are specifically expressed in the distal areas of CSF-cNs within the spinal cord (Desban, 2018; Desban et al., 2019). Moreover, flagella-associated protein 57 (FAP57) not only is expressed in CSF-cNs, but also serves as an effective biomarker for identifying CSF-cNs (Wyart et al., 2023). Hence, CSF-cNs are distinctive in their role in spinal cord repair, featuring apical bulbous protrusions that facilitate cellular connections through the central canal. Cao et al. highlight that CSF-cNs express PKD2L1, attributing them with neural stem cell-like properties that aid neuroregeneration post-injury. These neurons also play key roles in sustaining cellular structure, enhancing connectivity, and regulating intracellular activities.
In the mammalian brain, neural stem cells (NSCs) are found in three main regions: the lateral ventricles’ subventricular zone (SVZ), the hippocampus’ subgranular zone (SGZ), and within the spinal cord’s central canal, all supported by growing evidence (Stoeckel ME. et al., 2003; Sabourin et al., 2009; Chaker et al., 2016; Djenoune and Wyart, 2017b). In the spinal cord’s central canal, three distinct cell types can be identified based on morphological characteristics: ciliated ependymal cells with short basal processes that interface with adjacent neural tissue, mono-ciliated ependymal cells, and CSF-cNs (Song and Zhang, 2018; Moreno-Manzano, 2020). Historically, ependymal cells were thought to be NSCs in the spinal cord (Meletis et al., 2008; Marichal et al., 2012; Ortiz-Álvarez et al., 2019; Redmond et al., 2019; Moreno-Manzano, 2020). However, recent single-cell RNA sequencing shows that although they express genes related to cilia, they also exhibit multiple stem cell-associated genes (Ortiz-Alvarez et al., 2019), yet lack typical NSC functions under stimulatory conditions in vivo and in vitro (Shah et al., 2018; Xue et al., 2021).
CSF-cNs, prominently located in the central canal of the spinal cord and other brain regions such as the dorsal raphe nuclei and hypothalamus (Tonelli Gombalova et al., 2020; Yang et al., 2020; Jurcic et al., 2021), begin to develop from the 12th day of embryonic life, peaking around days E14 and E15. Unlike other spinal neurons, CSF-cNs are not restricted to specific neuroepithelial regions and can arise during later neurogenic phases (Kutna et al., 2014).
The somata of cerebrospinal fluid-contacting neurons (CSF-cNs) are located near the central canal of the spinal cord, a site of neurogenic potential in adult vertebrates capable of endogenous repair (Becker et al., 2018). Electrophysiological studies on CSF-cNs in vitro (Orts-Del'Immagine et al., 2016a; Orts-Del’immagine et al., 2012b; Petracca et al., 2016c; Reali et al., 2011) and in vivo (Sternberg et al., 2018b) have consistently shown elevated membrane resistance (Orts-Del’immagine et al., 2012b; Sternberg et al., 2018b). Immature neurons usually possess a very high membrane resistance. Consequently, spinal CSF-cNs display numerous molecular indicators indicative of neurons development, including reduced NeuN expression and increased levels of doublecortin (DCX) (Ren et al., 2017; Prendergast et al., 2023), homeobox protein NKX6.1, ELAV-like protein 3 (ELAVL3), Ascl1 (Di Bella et al., 2019c) and the polysialylated form of the neural cell adhesion molecule (PSA-NCAM) (Orts-Del'Immagine et al., 2014b; Reali et al., 2011; Stoeckel M-E. et al., 2003; Kútna et al., 2014; Marichal et al., 2009; Russo et al., 2004). These expression patterns indicate a sustained immature state in CSF-cNs, which may enhance their structural flexibility and regenerative capacity following injuries (Bonfanti and Seki, 2021).
Mouse PKD2L1-positive CSF-cNs neurospheres cultivated in vitro exhibit NSC markers expression, proliferative ability, and potential for differentiation into neurons, and oligodendrocytes (Cao et al., 2023). Further in vivo studies indicated that spinal cord injuries or the injection of neurotrophic factors such as bFGF and VEGF into the lateral ventricle can activate and enhance the proliferation of CSF-cNs (Cao et al., 2022). CSF-cNs were also found to express aromatic amino acid decarboxylase (AADC) in response to injury, facilitating monoamines synthesis (Wienecke et al., 2014; Ren et al., 2016; Ren et al., 2017). Remarkably, evidence of recovery following SCI has shown substantial resilience in CSF-cNs, particularly in those concentrated in the lumbar region of the spinal cord in mice, which regained ambulatory abilities through electrical stimulation therapy (Kathe et al., 2022). Additionally, CSF-cNs are abundantly present in the mature primate spinal cord (LaMotte, 1987; Djenoune et al., 2014; Kastner and Wanaverbecq, 2023). These findings collectively highlight the significant NSC potential of CSF-cNs. Gerstmann et al. (2022) posited that cerebrospinal fluid-contacting neurons (CSF-cNs) are integral to mammalian spinal cord motor control, particularly in adaptive control and proprioceptive feedback. These neurons contribute to motor regulation and limb movement fine-tuning by interfacing with the spinal motor circuit and monitoring cerebrospinal fluid dynamics. However, in vivo studies on the stem cell properties of CSF-cNs are still limited, focusing mainly on their potential differentiation capabilities with insufficient exploration of specific signaling pathways and regulatory factors. Additionally, the impact of post-SCI microenvironments, such as inflammatory and immune responses, on CSF-cNs is under-researched. It is essential to conduct comprehensive studies on these aspects to better understand CSF-cNs’ differentiation mechanisms and their stem cell functionality post-SCI, which could advance their clinical applications.
After spinal cord injury in rats, a decline in endogenous neural progenitor cell populations and locomotor function was observed, underscoring the potential role of CSF-cNs in post-injury neurological functions (He et al., 2020). Immunofluorescence studies showed that neurospheres derived from PKD2L1+ CSF-cNs expressed neural stem cell markers such as Sox2, Nestin, and GFAP (Cao et al., 2023), and were capable of differentiating into neurons, oligodendrocytes, and astrocytes in vitro (He et al., 2020). These findings suggest that CSF-cNs are immature neuronal cells with the properties of neural stem cells, able to proliferate and differentiate into various neuronal types (Wang et al., 2021b). Additionally, the significant recovery noted post-injury highlights the robust regeneration capacity of rodent CSF-cNs, which support locomotor recovery and restore ambulatory function via electrical stimulation in the lumbar spinal cord (Kathe et al., 2022). Figure 1A outlines the main biomarkers of CSF-cNs. Spinal cord injury (SCI) involves complex pathological processes, categorized into primary and secondary injuries (Figure 1B). The acute phase is characterized by inflammation, hematoma formation, neuronal death, and blood-spinal cord barrier disruptions (Figure 1C). The subacute phase features fibroblast and microglial infiltration, cyst formation, and axonal dieback (Figure 1D), while the intermediate and chronic phases are marked by astrogliosis, limited remyelination, and restricted axonal regrowth (Figure 1E). This article also examines signaling pathways influencing SCI repair, including PI3K/Akt, Ras, PLC, PTEN/mTOR, Wnt, and TGF-β, which regulate cell survival, inflammation, neurite outgrowth, and axon regeneration (Figure 2A). JAK/STAT pathway’s role in mediating inflammation and supporting neural stem cell functions is also highlighted (Figure 2A). CSF-cNs contribute significantly to SCI repair by differentiating into neurons, astrocytes, and oligodendrocytes, enhancing neural repair and supporting nerve function (Figure 2B). Neurons transmit electrical signals, supported by neurotrophic factors for survival and regeneration. Astrocytes provide metabolic support and regulate inflammation, while oligodendrocytes maintain myelin sheaths, crucial for efficient nerve impulse transmission.
Figure 1. Summarizes the main biomarkers of CSF-cNs and Pathological mechanisms of spinal cord injury. (A) Summarizes the main biomarkers of CSF-cNs. (B) Primary Injury and Secondary Injury of spinal cord injury. (C) Acute phase of spinal cord injury. (D) Subacute phase of spinal cord injury. (E) Intermediate and Chronic phase of spinal cord injury.
Figure 2. The main signaling pathways in spinal cord injury and the main mechanism of CSF-cNs in repair after spinal cord injury. (A) Main signaling pathways in spinal cord injury. (B) Main mechanism of CSF-cNs in repair after spinal cord injury.
The aforementioned findings indicate that CSF-cNs in the spinal cord exhibit NSCs properties. Furthermore, NSCs derived from CSF-cNs in the central canal may serve as a reservoir of neuronal cells, aiding in the replenishment of the damaged region (Wang et al., 2021a). Despite advancements in treatment, SCI often results in significant neurological deficits. The discovery of adult NSCs in mammals, including humans, has enhanced the prospects for treating CNS disorders by promoting self-regeneration (Chaker et al., 2016; Moore, 2016). Conversely, the inherent stem cell properties of CSF-cNs may revolutionize therapeutic approaches for patients with spinal cord injury, suggesting that CSF-cNs play a pivotal role in the treatment of SCI. Future research should focus on the molecular mechanisms of CSF-cNs, particularly factors influencing their neural stem cell characteristics, proliferation, and differentiation. Understanding these mechanisms could identify new targets for developing precise SCI treatment strategies. Exploring the use of CSF-cNs in stem cell therapy might offer avenues for enhancing neural regeneration and functional recovery post-SCI. Additionally, investigations into pharmacological interventions that modulate CSF-cNs’ growth and differentiation could lead to novel therapeutic approaches for SCI. The discovery of regulatory drugs or growth factors may facilitate new drug-based strategies. The potential of CSF-cNs research in clinical therapy includes innovating treatment methods and enhancing the quality of life for SCI patients through personalized, condition-specific therapies that minimize treatment risks and optimize outcomes.
Currently, the scientific community is increasingly focusing on the role of CSF-cNs, particularly in their potential for addressing spinal cord pathologies. The intimate association of these neurons with CSF makes intrathecal therapy a viable approach. Despite considerable progress in the characterization of CSF-cNs, highlighted by the identification of the unique marker PKD2L1, many aspects of their neural stem cell-like properties in vitro or in vivo remain poorly understood. For example, of the two distinct CSF-cN populations identified, which one retains neural stem cell properties? In SCI repair, Wyart et al. (2023) considered dorsal CSF-cNs may have closer contact with the CSF, potentially being involved in sensory signal transmission, the restoration of sensory function, and more prominently in posture control and stability. On the other hand, ventral CSF-cNs may be more connected to spinal cord tissue, potentially participating in motor signal transmission, the restoration of motor function, and more prominently in motor control and rapid swimming. These distinct locations and functions may allow CSF-cNs to synergistically contribute to SCI repair, promoting the recovery of neural function and the regeneration of nerve cells. Moreover, the processes by which CSF-cNs act as neural stem cells in spinal cord injury conditions and their role in lesion repair are still to be fully elucidated. Future research should continue to explore the activation and differentiation of CSF-cNs in spinal cord injury scenarios and aim to clarify the specific roles these cells play post-spinal cord injury.
YX: Data curation, Investigation, Resources, Software, Writing–original draft. WP: Investigation, Resources, Software, Writing–original draft. WZ: Data curation, Investigation, Writing–original draft. WS: Data curation, Investigation, Writing–original draft. WY: Writing–original draft. HY: Writing–original draft. YZ: Writing–original draft. QL: Conceptualization, Supervision, Validation, Writing–review and editing. LY: Conceptualization, Funding acquisition, Investigation, Project administration, Supervision, Validation, Writing–review and editing.
The author(s) declare that financial support was received for the research, authorship, and/or publication of this article. This work was supported by grants from the Science and Technology Fund Project of Guizhou Provincial Health Commission (gzwkj 2023-040); National Natural Science Foundation of China (NSFC) Regional Fund Cultivation Programme Project in Affiliated Hospital of Guizhou Medical University (gyfynsfc[2023]-47); Doctoral Research Initiation Fund Project of Affiliated Hospital of Guizhou Medical University (gyfybsky-2-21-46); Growth Programme for Young Scientific and Technological Talents in Ordinary Higher Education Institutions in Guizhou Province (Qianjiaohe KY Zi [2021] 160).
The authors thank the members of the laboratories who contributed to the current and past research on spinal cord injury model.
The authors declare that the research was conducted in the absence of any commercial or financial relationships that could be construed as a potential conflict of interest.
All claims expressed in this article are solely those of the authors and do not necessarily represent those of their affiliated organizations, or those of the publisher, the editors and the reviewers. Any product that may be evaluated in this article, or claim that may be made by its manufacturer, is not guaranteed or endorsed by the publisher.
Agduhr, E. (1922). Über ein zentrales Sinnesorgan (?) bei den Vertebraten. Z. für Anat. Entwicklungsgeschichte 66, 223–360. doi:10.1007/bf02593586
Andrzejczuk, L. A., Banerjee, S., England, S. J., Voufo, C., Kamara, K., and Lewis, K. E. (2018). Tal1, Gata2a, and Gata3 have distinct functions in the development of V2b and cerebrospinal fluid-contacting KA spinal neurons. Front. Neurosci. 12, 170. doi:10.3389/fnins.2018.00170
Becker, C. G., Becker, T., and Hugnot, J.-P. (2018). The spinal ependymal zone as a source of endogenous repair cells across vertebrates. Prog. Neurobiol. 170, 67–80. doi:10.1016/j.pneurobio.2018.04.002
Ben-Ari, Y. (2014). The GABA excitatory/inhibitory developmental sequence: a personal journey. Neuroscience 279, 187–219. doi:10.1016/j.neuroscience.2014.08.001
Bernhardt, R. R., Patel, C. K., Wilson, S. W., and Kuwada, J. Y. (1992). Axonal trajectories and distribution of GABAergic spinal neurons in wildtype and mutant zebrafish lacking floor plate cells. J. Comp. Neurology 326, 263–272. doi:10.1002/cne.903260208
Binor, E., and Heathcote, R. D. (2001). Development of GABA-immunoreactive neuron patterning in the spinal cord. J. Comp. Neurology 438, 1–11. doi:10.1002/cne.1298
Böhm, U. L., Prendergast, A., Djenoune, L., Nunes Figueiredo, S., Gomez, J., Stokes, C., et al. (2016). CSF-contacting neurons regulate locomotion by relaying mechanical stimuli to spinal circuits. Nat. Commun. 7, 10866. doi:10.1038/ncomms10866
Bonfanti, L., and Seki, T. (2021). The PSA-NCAM-positive "immature" neurons: an old discovery providing new vistas on brain structural plasticity. Cells 10, 2542. doi:10.3390/cells10102542
Boscardin, E., Alijevic, O., Hummler, E., Frateschi, S., and Kellenberger, S. (2016). The function and regulation of acid-sensing ion channels (ASICs) and the epithelial Na+ channel (ENaC): IUPHAR Review 19. Br. J. Pharmacol. 173, 2671–2701. doi:10.1111/bph.13533
Cao, L., Huang, M.-Z., Zhang, Q., Luo, Z.-R., Zhang, Y., An, P.-J., et al. (2022). The neural stem cell properties of Pkd2l1+ cerebrospinal fluid-contacting neurons in vivo. Front. Cell. Neurosci. 16, 992520. doi:10.3389/fncel.2022.992520
Cao, L., Zhang, H.-Q., He, Y.-Q., An, P.-J., Yang, L.-L., Tan, W., et al. (2023). Culture of cerebrospinal fluid-contacting neurons from neonatal mouse spinal cord. Cell Tissue Bank. 25, 443–452. doi:10.1007/s10561-023-10098-w
Chaker, Z., Codega, P., and Doetsch, F. (2016). A mosaic world: puzzles revealed by adult neural stem cell heterogeneity. Wiley Interdiscip. Rev. Dev. Biol. 5, 640–658. doi:10.1002/wdev.248
Chen, C. C., and Wong, C. W. (2013). Neurosensory mechanotransduction through acid-sensing ion channels. J. Cell. Mol. Med. 17, 337–349. doi:10.1111/jcmm.12025
Dale, N., Roberts, A., Ottersen, O., and Storm-Mathisen, J. (1987a). The development of a population of spinal cord neurons and their axonal projections revealed by GABA immunocytochemistry in frog embryos. Proc. R. Soc. Lond. Ser. B Biol. Sci. 232, 205–215. doi:10.1098/rspb.1987.0069
Dale, N., Roberts, A., Ottersen, O., and Storm-Mathisen, J. (1987b). The morphology and distribution of ‘Kolmer–Agduhr cells’, a class of cerebrospinal-fluid-contacting neurons revealed in the frog embryo spinal cord by GABA immunocytochemistry. Proc. R. Soc. Lond. Ser. B Biol. Sci. 232, 193–203. doi:10.1098/rspb.1987.0068
Dale, N., Roberts, A., Ottersen, O. P., and Storm-Mathisen, J. (1987c). The development of a population of spinal cord neurons and their axonal projections revealed by GABA immunocytochemistry in frog embryos. Proc. R. Soc. Lond. Ser. B, Biol. Sci. 232, 205–215. doi:10.1098/rspb.1987.0069
Dale, N., Roberts, A., Ottersen, O. P., and Storm-Mathisen, J. (1987d). The morphology and distribution of 'Kolmer-Agduhr cells', a class of cerebrospinal-fluid-contacting neurons revealed in the frog embryo spinal cord by GABA immunocytochemistry. Proc. R. Soc. Lond. Ser. B, Biol. Sci. 232, 193–203. doi:10.1098/rspb.1987.0068
Desban, L. (2018). Molecular determinants of morphology and function of microvilliated sensory cells in zebrafish. Sorbonne université.
Desban, L., Prendergast, A., Roussel, J., Rosello, M., Geny, D., Wyart, C., et al. (2019). Regulation of the apical extension morphogenesis tunes the mechanosensory response of microvilliated neurons. PLoS Biol. 17, e3000235. doi:10.1371/journal.pbio.3000235
Deval, E., and Lingueglia, E. (2015). Acid-Sensing Ion Channels and nociception in the peripheral and central nervous systems. Neuropharmacology 94, 49–57. doi:10.1016/j.neuropharm.2015.02.009
Di Bella, D. J., Carcagno, A. L., Bartolomeu, M. L., Pardi, M. B., Löhr, H., Siegel, N., et al. (2019a). Ascl1 balances neuronal versus ependymal fate in the spinal cord central canal. Cell Rep. 28, 2264–2274. e2263. doi:10.1016/j.celrep.2019.07.087
Di Bella, D. J., Carcagno, A. L., Bartolomeu, M. L., Pardi, M. B., Löhr, H., Siegel, N., et al. (2019b). Ascl1 balances neuronal versus ependymal fate in the spinal cord central canal. Cell Rep. 28, 2264–2274. e2263. doi:10.1016/j.celrep.2019.07.087
Di Bella, D. J., Carcagno, A. L., Bartolomeu, M. L., Pardi, M. B., Löhr, H., Siegel, N., et al. (2019c). Ascl1 balances neuronal versus ependymal fate in the spinal cord central canal. Cell Rep. 28, 2264–2274. doi:10.1016/j.celrep.2019.07.087
Djenoune, L., Desban, L., Gomez, J., Sternberg, J. R., Prendergast, A., Langui, D., et al. (2017a). The dual developmental origin of spinal cerebrospinal fluid-contacting neurons gives rise to distinct functional subtypes. Sci. Rep. 7, 719. doi:10.1038/s41598-017-00350-1
Djenoune, L., Desban, L., Gomez, J., Sternberg, J. R., Prendergast, A., Langui, D., et al. (2017b). The dual developmental origin of spinal cerebrospinal fluid-contacting neurons gives rise to distinct functional subtypes. Sci. Rep. 7, 719. doi:10.1038/s41598-017-00350-1
Djenoune, L., Khabou, H., Joubert, F., Quan, F. B., Nunes Figueiredo, S., Bodineau, L., et al. (2014). Investigation of spinal cerebrospinal fluid-contacting neurons expressing PKD2L1: evidence for a conserved system from fish to primates. Front. Neuroanat. 8, 26. doi:10.3389/fnana.2014.00026
Djenoune, L., and Wyart, C. (2017a). Light on a sensory interface linking the cerebrospinal fluid to motor circuits in vertebrates. J. Neurogenetics 31, 113–127. doi:10.1080/01677063.2017.1359833
Djenoune, L., and Wyart, C. (2017b). Light on a sensory interface linking the cerebrospinal fluid to motor circuits in vertebrates. J. Neurogenet. 31, 113–127. doi:10.1080/01677063.2017.1359833
England, S. J., Campbell, P. C., Banerjee, S., Swanson, A. J., and Lewis, K. E. (2017). Identification and expression analysis of the complete family of zebrafish pkd genes. Front. Cell Dev. Biol. 5, 5. doi:10.3389/fcell.2017.00005
Feldblum, S., Dumoulin, A., Anoal, M., Sandillon, F., and Privat, A. (1995). Comparative distribution of GAD65 and GAD67 mRNAs and proteins in the rat spinal cord supports a differential regulation of these two glutamate decarboxylases in vivo. J. Neurosci. Res. 42, 742–757. doi:10.1002/jnr.490420603
Finkel, Z., Esteban, F., Rodriguez, B., Fu, T., Ai, X., and Cai, L. (2021). Diversity of adult neural stem and progenitor cells in physiology and disease. Cells 10, 2045. doi:10.3390/cells10082045
Gaillard, A.-L., Mohamad, T., Quan, F. B., De Cian, A., Mosimann, C., Tostivint, H., et al. (2023). Urp1 and Urp2 act redundantly to maintain spine shape in zebrafish larvae. Dev. Biol. 496, 36–51. doi:10.1016/j.ydbio.2023.01.010
Gao, J., Thonhoff, J. R., Dunn, T. J., and Wu, P. (2013). Lipoic acid enhances survival of transplanted neural stem cells by reducing transplantation-associated injury. J. Neurorestoratology 1, 1–12. doi:10.2147/jn.s43745
Gerstmann, K., Jurčić, N., Blasco, E., Kunz, S., de Almeida Sassi, F., Wanaverbecq, N., et al. (2022). The role of intraspinal sensory neurons in the control of quadrupedal locomotion. Curr. Biol. CB 32, 2442–2453.e4. doi:10.1016/j.cub.2022.04.019
He, Y. Q., Shi, X. X., Chen, L., Zhao, W. B., Shan, J., Lin, Z. L., et al. (2020). Cerebrospinal fluid-contacting neurons affect the expression of endogenous neural progenitor cells and the recovery of neural function after spinal cord injury. Int. J. Neurosci. 131, 615–624. doi:10.1080/00207454.2020.1750396
Hirunagi, K., Hasegawa, M., Vigh, B., and Vigh-Teichmann, I. (1992). Immunocytochemical demonstration of serotonin-immunoreactive cerebrospinal fluid-contacting neurons in the paraventricular organ of pigeons and domestic chickens. Prog. Brain Res. 91, 327–330. doi:10.1016/s0079-6123(08)62350-x
Huang, A. L., Chen, X., Hoon, M. A., Chandrashekar, J., Guo, W., Trankner, D., et al. (2006). The cells and logic for mammalian sour taste detection. Nature 442, 934–938. doi:10.1038/nature05084
Huang, H., Sharma, H. S., Saberi, H., Chen, L., Sanberg, P. R., Xue, M., et al. (2022). Spinal cord injury or dysfunction quality of life rating scale (SCIDQLRS)(IANR 2022 version). J. Neurorestoratology 10, 100016. doi:10.1016/j.jnrt.2022.100016
Jalalvand, E., Robertson, B., Tostivint, H., Löw, P., Wallén, P., and Grillner, S. (2018). Cerebrospinal fluid-contacting neurons sense pH changes and motion in the hypothalamus. J. Neurosci. 38, 7713–7724. doi:10.1523/JNEUROSCI.3359-17.2018
Jalalvand, E., Robertson, B., Tostivint, H., Wallén, P., and Grillner, S. (2016b). The spinal cord has an intrinsic system for the control of pH. Curr. Biol. CB 26, 1346–1351. doi:10.1016/j.cub.2016.03.048
Jalalvand, E., Robertson, B., Wallén, P., and Grillner, S. (2016a). Ciliated neurons lining the central canal sense both fluid movement and pH through ASIC3. Nat. Commun. 7, 10002. doi:10.1038/ncomms10002
Jurčić, N., Er-Raoui, G., Airault, C., Trouslard, J., Wanaverbecq, N., and Seddik, R. (2019). GABAB receptors modulate Ca2+ but not G protein-gated inwardly rectifying K+ channels in cerebrospinal-fluid contacting neurones of mouse brainstem. J. Physiology 597, 631–651. doi:10.1113/JP277172
Jurcic, N., Michelle, C., Trouslard, J., Wanaverbecq, N., and Kastner, A. (2021). Evidence for PKD2L1-positive neurons distant from the central canal in the ventromedial spinal cord and medulla of the adult mouse. Eur. J. Neurosci. 54, 4781–4803. doi:10.1111/ejn.15342
Kastner, A., and Wanaverbecq, N. (2023). In Rhesus monkeys, CSF-contacting neurons are the only neurons present in the medullo-spinal peri-ependymal zone. Available at: https://www.biorxiv.org/content/10.1101/2023.03.29.534787v3.
Kathe, C., Skinnider, M. A., Hutson, T. H., Regazzi, N., Gautier, M., Demesmaeker, R., et al. (2022). The neurons that restore walking after paralysis. Nature 611, 540–547. doi:10.1038/s41586-022-05385-7
Kolmer, W. (1921). Das,sagittalorgan,Der wirbeltiere. Z. für Anat. Entwicklungsgeschichte 60, 652–717. doi:10.1007/bf02593657
Kutna, V., Sevc, J., Gombalova, Z., Matiasova, A., and Daxnerova, Z. (2014). Enigmatic cerebrospinal fluid-contacting neurons arise even after the termination of neurogenesis in the rat spinal cord during embryonic development and retain their immature-like characteristics until adulthood. Acta histochem. 116, 278–285. doi:10.1016/j.acthis.2013.08.004
Kútna, V., Ševc, J., Gombalová, Z., Matiašová, A., and Daxnerová, Z. (2014). Enigmatic cerebrospinal fluid-contacting neurons arise even after the termination of neurogenesis in the rat spinal cord during embryonic development and retain their immature-like characteristics until adulthood. Acta Histochem. 116, 278–285. doi:10.1016/j.acthis.2013.08.004
LaMotte, C. C. (1987). Vasoactive intestinal polypeptide cerebrospinal fluid-contacting neurons of the monkey and cat spinal central canal. J. Comp. Neurology 258, 527–541. doi:10.1002/cne.902580405
Leppert, W., Zajaczkowska, R., Wordliczek, J., Dobrogowski, J., Woron, J., and Krzakowski, M. (2016). Pathophysiology and clinical characteristics of pain in most common locations in cancer patients. J. Physiol. Pharmacol. 67, 787–799.
Marichal, N., García, G., Radmilovich, M., Trujillo-Cenóz, O., and Russo, R. E. (2009). Enigmatic central canal contacting cells: immature neurons in "standby mode"? J. Neurosci. Official J. Soc. For Neurosci. 29, 10010–10024. doi:10.1523/JNEUROSCI.6183-08.2009
Marichal, N., García, G., Radmilovich, M., Trujillo-Cenóz, O., and Russo, R. E. (2012). Spatial domains of progenitor-like cells and functional complexity of a stem cell niche in the neonatal rat spinal cord. Stem cells Dayt. Ohio 30, 2020–2031. doi:10.1002/stem.1175
Meletis, K., Barnabé-Heider, F., Carlén, M., Evergren, E., Tomilin, N., Shupliakov, O., et al. (2008). Spinal cord injury reveals multilineage differentiation of ependymal cells. PLOS Biol. 6, e182. doi:10.1371/journal.pbio.0060182
Montgomery, J. E., Wiggin, T. D., Rivera-Perez, L. M., Lillesaar, C., and Masino, M. A. (2016). Intraspinal serotonergic neurons consist of two, temporally distinct populations in developing zebrafish. Dev. Neurobiol. 76, 673–687. doi:10.1002/dneu.22352
Moore, S. A. (2016). The spinal ependymal layer in health and disease. Vet. Pathol. 53, 746–753. doi:10.1177/0300985815618438
Moreno-Manzano, V. (2020). Ependymal cells in the spinal cord as neuronal progenitors. Curr. Opin. Pharmacol. 50, 82–87. doi:10.1016/j.coph.2019.11.008
Ortiz-Álvarez, G., Daclin, M., Shihavuddin, A., Lansade, P., Fortoul, A., Faucourt, M., et al. (2019). Adult neural stem cells and multiciliated ependymal cells share a common lineage regulated by the geminin family members. NEURON 102, 159–172. doi:10.1016/j.neuron.2019.01.051
Ortiz-Alvarez, G., Daclin, M., Shihavuddin, A., Lansade, P., Fortoul, A., Faucourt, M., et al. (2019). Adult neural stem cells and multiciliated ependymal cells share a common lineage regulated by the geminin family members. Neuron 102, 159–172. doi:10.1016/j.neuron.2019.01.051
Orts-Del’Immagine, A., Kastner, A., Tillement, V., Tardivel, C., Trouslard, J., and Wanaverbecq, N. (2014a). Morphology, distribution and phenotype of polycystin kidney disease 2-like 1-positive cerebrospinal fluid contacting neurons in the brainstem of adult mice. PLoS one 9, e87748. doi:10.1371/journal.pone.0087748
Orts-Del'Immagine, A., Kastner, A., Tillement, V., Tardivel, C., Trouslard, J., and Wanaverbecq, N. (2014b). Morphology, distribution and phenotype of polycystin kidney disease 2-like 1-positive cerebrospinal fluid contacting neurons in the brainstem of adult mice. PloS One 9, e87748. doi:10.1371/journal.pone.0087748
Orts-Del’Immagine, A., Seddik, R., Tell, F., Airault, C., Er-Raoui, G., Najimi, M., et al. (2016a). A single polycystic kidney disease 2-like 1 channel opening acts as a spike generator in cerebrospinal fluid-contacting neurons of adult mouse brainstem. Neuropharmacology 101, 549–565. doi:10.1016/j.neuropharm.2015.07.030
Orts-Del’Immagine, A., Seddik, R., Tell, F., Airault, C., Er-Raoui, G., Najimi, M., et al. (2016b). A single polycystic kidney disease 2-like 1 channel opening acts as a spike generator in cerebrospinal fluid-contacting neurons of adult mouse brainstem. Neuropharmacology 101, 549–565. doi:10.1016/j.neuropharm.2015.07.030
Orts-Del’Immagine, A., Trouslard, J., Airault, C., Hugnot, J.-P., Cordier, B., Doan, T., et al. (2017). Postnatal maturation of mouse medullo-spinal cerebrospinal fluid-contacting neurons. Neuroscience 343, 39–54. doi:10.1016/j.neuroscience.2016.11.028
Orts-Del’immagine, A., Wanaverbecq, N., Tardivel, C., Tillement, V., Dallaporta, M., and Trouslard, J. (2012a). Properties of subependymal cerebrospinal fluid contacting neurones in the dorsal vagal complex of the mouse brainstem. J. Physiol. 590, 3719–3741. doi:10.1113/jphysiol.2012.227959
Orts-Del’immagine, A., Wanaverbecq, N., Tardivel, C., Tillement, V., Dallaporta, M., and Trouslard, J. (2012b). Properties of subependymal cerebrospinal fluid contacting neurones in the dorsal vagal complex of the mouse brainstem. J. Physiology 590, 3719–3741. doi:10.1113/jphysiol.2012.227959
Orts-Del’Immagine, A., and Wyart, C. (2017). Cerebrospinal-fluid-contacting neurons. Curr. Biol. 27, R1198–R1200. doi:10.1016/j.cub.2017.09.017
Petracca, Y. L., Sartoretti, M. M., Di Bella, D. J., Marin-Burgin, A., Carcagno, A. L., Schinder, A. F., et al. (2016a). The late and dual origin of cerebrospinal fluid-contacting neurons in the mouse spinal cord. Development 143, 880–891. doi:10.1242/dev.129254
Petracca, Y. L., Sartoretti, M. M., Di Bella, D. J., Marin-Burgin, A., Carcagno, A. L., Schinder, A. F., et al. (2016b). The late and dual origin of cerebrospinal fluid-contacting neurons in the mouse spinal cord. Development 143, 880–891. doi:10.1242/dev.129254
Petracca, Y. L., Sartoretti, M. M., Di Bella, D. J., Marin-Burgin, A., Carcagno, A. L., Schinder, A. F., et al. (2016c). The late and dual origin of cerebrospinal fluid-contacting neurons in the mouse spinal cord. Dev. Camb. Engl. 143, 880–891. doi:10.1242/dev.129254
Prendergast, A. E., Jim, K. K., Marnas, H., Desban, L., Quan, F. B., Djenoune, L., et al. (2023). CSF-contacting neurons respond to Streptococcus pneumoniae and promote host survival during central nervous system infection. Curr. Biol. CB 33, 940–956.e10. doi:10.1016/j.cub.2023.01.039
Quan, F. B., Dubessy, C., Galant, S., Kenigfest, N. B., Djenoune, L., Leprince, J., et al. (2015). Comparative distribution and in vitro activities of the urotensin II-related peptides URP1 and URP2 in zebrafish: evidence for their colocalization in spinal cerebrospinal fluid-contacting neurons. PLoS One 10, e0119290. doi:10.1371/journal.pone.0119290
Reali, C., Fernández, A., Radmilovich, M., Trujillo-Cenóz, O., and Russo, R. E. (2011). GABAergic signalling in a neurogenic niche of the turtle spinal cord. J. Physiology 589, 5633–5647. doi:10.1113/jphysiol.2011.214312
Redmond, S. A., Figueres-Oñate, M., Obernier, K., Nascimento, M. A., Parraguez, J. I., López-Mascaraque, L., et al. (2019). Development of ependymal and postnatal neural stem cells and their origin from a common embryonic progenitor. Cell Rep. 27, 429–441. doi:10.1016/j.celrep.2019.01.088
Reekmans, K., Praet, J., Daans, J., Reumers, V., Pauwels, P., Van der Linden, A., et al. (2012). Current challenges for the advancement of neural stem cell biology and transplantation research. Stem Cell Rev. Rep. 8, 262–278. doi:10.1007/s12015-011-9266-2
Ren, L.-Q., Chen, M., Hultborn, H., Guo, S., Zhang, Y., and Zhang, M. (2017). Heterogenic distribution of aromatic L-amino acid decarboxylase neurons in the rat spinal cord. Front. Integr. Neurosci. 11, 31. doi:10.3389/fnint.2017.00031
Ren, L.-Q., Wienecke, J., Hultborn, H., and Zhang, M. (2016). Production of dopamine by aromatic l-amino acid decarboxylase cells after spinal cord injury. J. Neurotrauma 33, 1150–1160. doi:10.1089/neu.2015.4037
Retailleau, K., and Duprat, F. (2014). Polycystins and partners: proposed role in mechanosensitivity. J. physiology 592, 2453–2471. doi:10.1113/jphysiol.2014.271346
Roberts, E. (1974). Gamma-aminobutyric acid and nervous system function--a perspective. Biochem. Pharmacol. 23, 2637–2649. doi:10.1016/0006-2952(74)90033-1
Russo, R. E., Fernández, A., Reali, C., Radmilovich, M., and Trujillo-Cenóz, O. (2004). Functional and molecular clues reveal precursor-like cells and immature neurones in the turtle spinal cord. J. Physiology 560, 831–838. doi:10.1113/jphysiol.2004.072405
Sabourin, J. C., Ackema, K. B., Ohayon, D., Guichet, P. O., Perrin, F. E., Garces, A., et al. (2009). A mesenchymal-like ZEB1(+) niche harbors dorsal radial glial fibrillary acidic protein-positive stem cells in the spinal cord. Stem Cells 27, 2722–2733. doi:10.1002/stem.226
Shah, P. T., Stratton, J. A., Stykel, M. G., Abbasi, S., Sharma, S., Mayr, K. A., et al. (2018). Single-cell transcriptomics and fate mapping of ependymal cells reveals an absence of neural stem cell function. Cell 173, 1045–1057. doi:10.1016/j.cell.2018.03.063
Smith, D. E., Johanson, C. E., and Keep, R. F. (2004). Peptide and peptide analog transport systems at the blood–CSF barrier. Adv. drug Deliv. Rev. 56, 1765–1791. doi:10.1016/j.addr.2004.07.008
Song, S.-Y., and Zhang, L.-C. (2018). The establishment of a CSF-contacting nucleus “knockout” model animal. Front. Neuroanat. 12, 22. doi:10.3389/fnana.2018.00022
Soto, E., Ortega-Ramírez, A., and Vega, R. (2018). Protons as messengers of intercellular communication in the nervous system. Front. Cell. Neurosci. 12, 342. doi:10.3389/fncel.2018.00342
Sternberg, J. R., Prendergast, A. E., Brosse, L., Cantaut-Belarif, Y., Thouvenin, O., Orts-Del’Immagine, A., et al. (2018a). Pkd2l1 is required for mechanoception in cerebrospinal fluid-contacting neurons and maintenance of spine curvature. Nat. Commun. 9, 3804. doi:10.1038/s41467-018-06225-x
Sternberg, J. R., Prendergast, A. E., Brosse, L., Cantaut-Belarif, Y., Thouvenin, O., Orts-Del'Immagine, A., et al. (2018b). Pkd2l1 is required for mechanoception in cerebrospinal fluid-contacting neurons and maintenance of spine curvature. Nat. Commun. 9, 3804. doi:10.1038/s41467-018-06225-x
Stoeckel, M. E., Uhl-Bronner, S., Hugel, S., Veinante, P., Klein, M. J., Mutterer, J., et al. (2003a). Cerebrospinal fluid-contacting neurons in the rat spinal cord, a gamma-aminobutyric acidergic system expressing the P2X2 subunit of purinergic receptors, PSA-NCAM, and GAP-43 immunoreactivities: light and electron microscopic study. J. Comp. Neurol. 457, 159–174. doi:10.1002/cne.10565
Stoeckel, M.-E., Uhl-Bronner, S., Hugel, S., Veinante, P., Klein, M.-J., Mutterer, J., et al. (2003b). Cerebrospinal fluid-contacting neurons in the rat spinal cord, a gamma-aminobutyric acidergic system expressing the P2X2 subunit of purinergic receptors, PSA-NCAM, and GAP-43 immunoreactivities: light and electron microscopic study. J. Comp. Neurology 457, 159–174. doi:10.1002/cne.10565
Teng, Y. D., Santos, F. N., Black, P. M., Konya, D., Park, K. I., Sidman, R. L., et al. (2008). “Neural stem cells,” in Principles of regenerative medicine Editors A. Atala, J. Thomson, R. Lanza, and R. M. Nerem 300–317.
Tohda, C., and Kuboyama, T. (2011). Current and future therapeutic strategies for functional repair of spinal cord injury. Pharmacol. Ther. 132, 57–71. doi:10.1016/j.pharmthera.2011.05.006
Tonelli Gombalova, Z., Kosuth, J., Alexovic Matiasova, A., Zrubakova, J., Zezula, I., Giallongo, T., et al. (2020). Majority of cerebrospinal fluid-contacting neurons in the spinal cord of C57Bl/6N mice is present in ectopic position unlike in other studied experimental mice strains and mammalian species. J. Comp. Neurol. 528, 2523–2550. doi:10.1002/cne.24909
Vígh, B., Manzano e Silva, M. J., Frank, C. L., Vincze, C., Czirok, S. J., Szabó, A., et al. (2004). The system of cerebrospinal fluid-contacting neurons. Its supposed role in the nonsynaptic signal transmission of the brain. Histology Histopathol. 19, 607–628. doi:10.14670/HH-19.607
Vigh, B., and Vigh-Teichmann, I. (1973). Comparative ultrastructure of the cerebrospinal fluid-contacting neurons. Int. Rev. Cytol. 35, 189–251. doi:10.1016/s0074-7696(08)60355-1
Vigh, B., and Vigh-Teichmann, I. (1998). Actual problems of the cerebrospinal fluid-contacting neurons. Microsc. Res. Tech. 41, 57–83. doi:10.1002/(SICI)1097-0029(19980401)41:1<57::AID-JEMT6>3.0.CO;2-R
Vigh, B., Vigh-Teichmann, I., Röhlich, P., and Oksche, A. (1983). Cerebrospinal fluid-contacting neurons, sensory pinealocytes and Landolt's clubs of the retina as revealed by means of an electron-microscopic immunoreaction against opsin. Cell Tissue Res. 233, 539–548. doi:10.1007/BF00212223
Vigh-Teichmann, I., and Vigh, B. (1983a). The system of cerebrospinal fluid-contacting neurons. Arch. Histol. Jpn. = Nihon Soshikigaku Kiroku 46, 427–468. doi:10.1679/aohc.46.427
Vigh-Teichmann, I., and Vigh, B. (1983b). The system of cerebrospinal fluid-contacting neurons. Arch. Histol. Jpn. 46, 427–468. doi:10.1679/aohc.46.427
Wang, S., He, Y., Zhang, H., Chen, L., Cao, L., Yang, L., et al. (2021a). The neural stem cell properties of PKD2L1+ cerebrospinal fluid-contacting neurons in vitro. Front. Cell. Neurosci. 15, 630882. doi:10.3389/fncel.2021.630882
Wang, S., He, Y., Zhang, H., Chen, L., Cao, L., Yang, L., et al. (2021b). The neural stem cell properties of PKD2L1(+) cerebrospinal fluid-contacting neurons in vitro. Front. Cell Neurosci. 15, 630882. doi:10.3389/fncel.2021.630882
Watanabe, M., Maemura, K., Kanbara, K., Tamayama, T., and Hayasaki, H. (2002). GABA and GABA receptors in the central nervous system and other organs. Int. Rev. Cytol. 213, 1–47. doi:10.1016/s0074-7696(02)13011-7
Wienecke, J., Ren, L.-Q., Hultborn, H., Chen, M., Møller, M., Zhang, Y., et al. (2014). Spinal cord injury enables aromatic L-amino acid decarboxylase cells to synthesize monoamines. J. Neurosci. Official J. Soc. For Neurosci. 34, 11984–12000. doi:10.1523/JNEUROSCI.3838-13.2014
Wilson, M. O., McNeill, B. A., Barrell, G. K., Prickett, T. C. R., and Espiner, E. A. (2017). Dexamethasone increases production of C-type natriuretic peptide in the sheep brain. J. Endocrinol. 235, 15–25. doi:10.1530/JOE-17-0148
Wyart, C., Carbo-Tano, M., Cantaut-Belarif, Y., Orts-Del'Immagine, A., and Böhm, U. L. (2023). Cerebrospinal fluid-contacting neurons: multimodal cells with diverse roles in the CNS. Nat. Rev. Neurosci. 24, 540–556. doi:10.1038/s41583-023-00723-8
Xue, X., Shu, M., Xiao, Z., Zhao, Y., Li, X., Zhang, H., et al. (2021). Lineage tracing reveals the origin of Nestin-positive cells are heterogeneous and rarely from ependymal cells after spinal cord injury. Sci. China Life Sci. 65, 757–769. doi:10.1007/s11427-020-1901-4
Yang, L., Rastegar, S., and Strähle, U. (2010). Regulatory interactions specifying Kolmer-Agduhr interneurons. Development 137, 2713–2722. doi:10.1242/dev.048470
Yang, L., Wang, F., and Strahle, U. (2020). The genetic programs specifying kolmer-agduhr interneurons. Front. Neurosci. 14, 577879. doi:10.3389/fnins.2020.577879
Zha, X.-m. (2013). Acid-sensing ion channels: trafficking and synaptic function. Mol. Brain 6, 1. doi:10.1186/1756-6606-6-1
Keywords: neural stem cells, neural regeneration, CSF-cNs, spinal cord injury, repair, PKD2L1
Citation: Xiong Y, Pi W, Zhao W, Shi W, Yan W, Yang H, Zhou Y, Li Q and Yang L (2024) Roles of cerebrospinal fluid-contacting neurons as potential neural stem cells in the repair and regeneration of spinal cord injuries. Front. Cell Dev. Biol. 12:1426395. doi: 10.3389/fcell.2024.1426395
Received: 01 May 2024; Accepted: 28 May 2024;
Published: 25 June 2024.
Edited by:
Chenyu Sun, AMITA Health, United StatesReviewed by:
Xiaolei Li, University of Pennsylvania, United StatesCopyright © 2024 Xiong, Pi, Zhao, Shi, Yan, Yang, Zhou, Li and Yang. This is an open-access article distributed under the terms of the Creative Commons Attribution License (CC BY). The use, distribution or reproduction in other forums is permitted, provided the original author(s) and the copyright owner(s) are credited and that the original publication in this journal is cited, in accordance with accepted academic practice. No use, distribution or reproduction is permitted which does not comply with these terms.
*Correspondence: Qing Li, bGlxMTY4QGdtYy5lZHUuY24=; Leiluo Yang, eWFuZ2xlaWx1bzIwMjNAMTYzLmNvbQ==
†These authors have contributed equally to this work
Disclaimer: All claims expressed in this article are solely those of the authors and do not necessarily represent those of their affiliated organizations, or those of the publisher, the editors and the reviewers. Any product that may be evaluated in this article or claim that may be made by its manufacturer is not guaranteed or endorsed by the publisher.
Research integrity at Frontiers
Learn more about the work of our research integrity team to safeguard the quality of each article we publish.