- 1HAND Research Group, School of Medicine and Health Sciences, Mulungushi University, Livingstone, Zambia
- 2Department of Medicine, Vanderbilt University Medical Center, Nashville, TN, United States
- 3Vanderbilt Center for Immunobiology, Nashville, TN, United States
- 4Vanderbilt Institute for Infection, Immunology and Inflammation, Nashville, TN, United States
- 5Vanderbilt Institute for Global Health, Nashville, TN, United States
The endothelial glycocalyx is closely associated with various physiological and pathophysiological events. Significant modification of the endothelial glycocalyx is an early process in the pathogenesis of cardiovascular disease. High dietary salt and HIV infection damages the endothelial glycocalyx causing endothelial dysfunction and increasing the risk for salt-sensitive hypertension and cardiovascular disease. The two factors, HIV infection and dietary salt are critical independent predictors of hypertension and cardiovascular disease and often synergize to exacerbate and accelerate disease pathogenesis. Salt-sensitive hypertension is more common among people living with HIV and is associated with risk for cardiovascular disease, stroke, heart attack and even death. However, the underlying mechanisms linking endothelial glycocalyx damage to dietary salt and HIV infection are lacking. Yet, both HIV infection/treatment and dietary salt are closely linked to endothelial glycocalyx damage and development of salt-sensitive hypertension. Moreover, the majority of individuals globally, consume more salt than is recommended and the burden of HIV especially in sub-Sahara Africa is disproportionately high. In this review, we have discussed the missing link between high salt and endothelial glycocalyx shedding in the pathogenesis of salt-sensitive hypertension. We have further elaborated the role played by HIV infection and treatment in modifying endothelial glycocalyx integrity to contribute to the development of hypertension and cardiovascular disease.
1 Introduction
The endothelial glycocalyx is a carbohydrate-protein-rich layer that lines the surface of the whole vascular endothelium (Reitsma et al., 2007). The endothelial glycocalyx plays a crucial role in vascular homeostasis and has been implicated in the initial development of cardiovascular disease processes (Kim et al., 2017). The endothelial glycocalyx is the main buffer for sodium ions in the vasculature (Oberleithner, 2015; Oberleithner and Wilhelmi, 2015). Degradation or shedding of the endothelial glycocalyx is one of the first steps that is linked to endothelial dysfunction (Li and Bonventre, 2016). A damaged glycocalyx is a pathological feature underlying salt-sensitive hypertension and many forms of cardiovascular disease (Li and Bonventre, 2016).
Chronic high dietary salt is an independent predictor of salt sensitive hypertension, stroke, heart attack and death (Nista et al., 2020). The underlying mechanism behind salt-induced hypertension and cardiovascular disease is related in part to sodium handling in the vascular bed. High salt has been implicated to induce endothelial glycocalyx shedding leading to Na+ and Ca2+ overload, Na+ extravasation into the interstitial space, epithelial cell damage, vascular smooth muscle cell hypertrophy and vascular inflammation (Oberleithner et al., 2007; Oberleithner and Wilhelmi, 2015; Schierke et al., 2017; Bkaily et al., 2018; Weinbaum et al., 2021; Sembajwe et al., 2023). In individuals with salt-sensitive hypertension, endothelial glycocalyx shedding may be more pronounced (Choi et al., 2015; Vinaiphat et al., 2023).
Salt-sensitive hypertension is defined as a significant increase in blood pressure that mirrors an increase in salt intake in persons with hypertension (Elijovich et al., 2016). Salt-sensitive hypertension is a major risk for cardiovascular and cerebrovascular adverse events (Elijovich et al., 2016; Bailey and Dhaun, 2024) and is more common in Black people and among persons living with HIV (PLWH) as reported from a study in Zambia (Elijovich et al., 2016; Masenga et al., 2021). HIV-infection and the use of antiretroviral therapy (ART) increases the risk for hypertension development and cardiovascular disease (Masenga et al., 2019). There is some emerging evidence of a link between HIV infection and damaged endothelial glycocalyx that predisposes PLWH to many cardiovascular diseases and hypertension. However, the use of ART likely ameliorates and restores endothelial glycocalyx integrity (Fragkou et al., 2023). There is little known about the role that HIV and ART plays in the salt-endothelial glycocalyx shedding axis in salt-sensitive hypertension. Unfortunately, there are no studies known to us that provide the link between salt intake, HIV infection and shedding of the endothelial glycocalyx so we will discuss the contribution of salt separate from that of HIV infection with a notion that the two factors synergize to exacerbate hypertension. However, we recently demonstrated that PLWH who are salt sensitive and have hypertension consumed more salt than the HIV negative and had a pressor response to salt that was more pronounced than the HIV negative group (Masenga et al., 2021). We however did not determine endothelial glycocalyx integrity in this cohort, limiting our understanding on the interplay between dietary salt and treated HIV infection on endothelial glycocalyx integrity.
Although the glycocalyx has been implicated in hypertension and cardiovascular disease, the pathophysiological and mechanotransduction mechanisms linking high salt to damaged endothelial glycocalyx in hypertension, let alone PLWH, are not clear. In this review, we have summarized the link between high salt and endothelial glycocalyx shedding and have elaborated in detail, the pathogenesis of salt-sensitive hypertension based on a damaged glycocalyx, and the role played by HIV/ART. We have further given insights on the current therapeutic modalities aimed at restoring the endothelial glycocalyx to prevent the risk for cardiovascular disease development.
2 The composition, structure, and role of endothelial glycocalyx
The endothelial glycocalyx is complex and was difficult to visualize. J.H Luft was likely the first to visualize and describe the endothelial glycocalyx more than 5 decades ago with an electron microscope (Luft, 1966). In the recent years, the endothelial glycocalyx has gained a lot of interest especially as an important feature regulating vascular and blood physiology and an early marker of cardiovascular disease. The endothelial glycocalyx is about 0.5–4.5 μm thick increasing with vascular diameter from capillaries to the carotid arteries (Vink and Duling, 1996; van Haaren et al., 2003; Megens et al., 2007) and covers the surface of endothelial cells, virtually separating them from coming into contact with blood cells, an important physiological characteristic that ensures maintenance of hemodynamic homeostasis, Figure 1A.
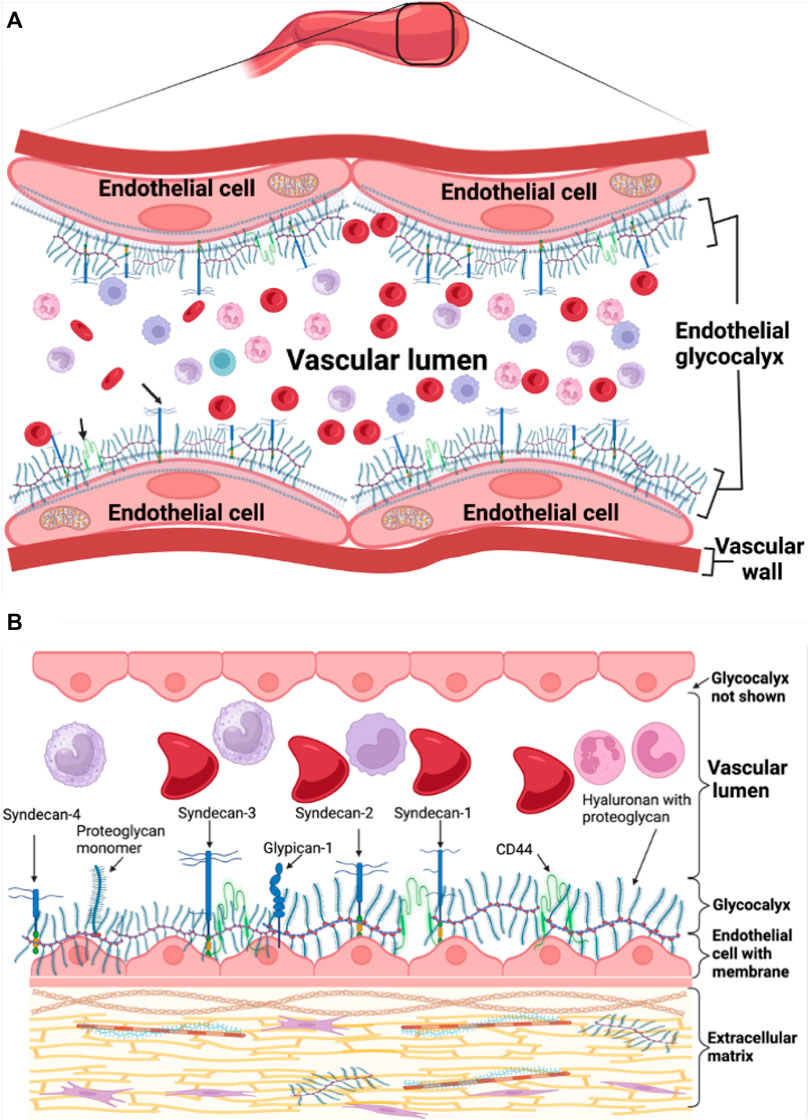
Figure 1. Endothelial glycocalyx schematic representation. (A) Healthy endothelial glycocalyx linked to endothelial cells in the vasculature. (B) Healthy endothelial glycocalyx showing proteoglycan backbone molecules attached to glycosaminoglycan chains.
The endothelial glycocalyx is rich in carbohydrate sugars with mainly proteoglycan and glycoprotein backbone molecules that connect and communicate with vascular endothelial cells (Foote et al., 2022). The endothelial glycocalyx is not fixed but active and soluble allowing for continuous interaction with plasma and blood flowing continuously in the vascular bed hence, constantly modulating its thickness. The endothelial glycocalyx is negatively charged, allowing a red blood cell exclusion zone or region of separation on the luminal side of the endothelium due to the repelling effect between the negatively charged red blood cell membrane and endothelial glycocalyx (Sembajwe et al., 2023). This protects the glycocalyx from shedding or damage. The proteoglycan component of the glycocalyx is the most important backbone molecule attached to glycosaminoglycan chains and is composed of syndecans and glypicans also known as glycosylphosphatidylinositol anchors (Carey, 1997; Fransson et al., 2004; Reitsma et al., 2007) and additional types of secreted soluble proteoglycans like perlecan, versican, mimecan, and biglycan (Iozzo, 1994; Kinsella et al., 2004; Reitsma et al., 2007), Figure 1B. The glycosaminoglycan chains bound to proteoglycans include dermatan and keratan sulfate, heparan and chondroitin sulfate, and hyaluronan. Crucial in playing a role during inflammatory responses are special glycoproteins such as selectins, integrins and immunoglobulin superfamily, within the glycocalyx whose expression is regulated by cytokines and complex signaling pathways (Harjunpää et al., 2019; Qu et al., 2021).
We have previously described the endothelial glycocalyx in detail (Sembajwe et al., 2023), the composition of the endothelial glycocalyx is well elaborated elsewhere (Reitsma et al., 2007; Villalba et al., 2021; Suzuki et al., 2022). We will therefore focus more on its physiological and pathological role in hypertension and HIV later below.
2.1 Physiological importance of the endothelial glycocalyx
The endothelial glycocalyx has several major functions in the vasculature. It regulates vascular permeability (Vink and Duling, 2000), restricts innate cells, red blood cells and other substances from coming into contact with the endothelium (Vink and Duling, 1996), but also facilitates the interaction of white blood cells to adhesion molecules during inflammatory responses, an important aspect of innate immunity. The endothelial glycocalyx also modulates antithrombotic and anticoagulant effects preventing coagulopathies and thrombotic events (Prior et al., 2017; Iba and Levy, 2019).
Apart from creating a physical barrier between the endothelium and contents of the vascular lumen, the endothelial glycocalyx also participates in mechanotransduction (Curry and Adamson, 2012). The endothelial glycocalyx also regulates blood viscosity and hematocrit, modulates shear stress and enhances flow-induced shear forces arising from shear-stress induced nitric oxide (NO) production (Mochizuki et al., 2003). The endothelial glycocalyx is also involved in the production of NO as removal of endothelial glycocalyx components was shown to inhibit the production of NO and impaired vasodilation (Pahakis et al., 2007; Dragovich et al., 2016). The mechanotransduction of the endothelial glycocalyx mediates NO production through Ca2+ entry and activation of endothelial transient receptor potential (TRP) channels (Dragovich et al., 2016). One mechanism for the endothelial glycocalyx mechanotransduction-induced production of NO is that during shear stress, the force applied to the components of the glycocalyx, syndecan and glypican-1, within the membrane caveolae, results in the phosphorylation of endothelial NO synthase (eNOS) by phosphoinositide-3-kinase and protein kinase A, leading to the activation of eNOS and production of NO (Boo et al., 2002). A study by Ebong et al demonstrated that silencing of glypican-1 abrogates eNOS activation and NO production suggesting that shear-induced production of NO was dependent on endothelial glycocalyx components (Ebong et al., 2010). Shear stress also regulates the activation of Ras-Mitogen-Activated Protein Kinases (MAPKs) which enhance cell survival mechanism by activating Krüppel-like factor 2 (KLF2) transcription factor and enhance the expression of syndecans as a protective measure to preserve the endothelial glycocalyx (Voyvodic et al., 2014). These data provide the premise for the shear stress sensing function of the endothelial glycocalyx.
As will be discussed later below, several factors that affect the integrity of the endothelial glycocalyx create a fertile ground for initial pathological processes that can escalate and increase the risk for cardiovascular disease and hypertension.
2.2 Determinants of endothelial glycocalyx integrity
The endothelial glycocalyx is susceptible to various factors that may underlie disease processes. These include but are not limited to chronic pro-atherogenic shear stress, interactive adverse influences from oxidized low density lipoprotein (Ahn et al., 2023), inflammatory cytokines such as tumor necrosis factor-α (TNF-α), interleukin-1 beta (IL-1β) and reactive oxygen species (ROS) that damage the glycocalyx by enhancing the activation of matrix metalloproteinases, heparanase, and hyaluronidase (Rubio-Gayosso et al., 2006; Uchimido et al., 2019). Traditional risk factors for cardiovascular disease such as hypertension, hyperglycemia, smoking, aging, bacterial and viral infections, and dietary salt contribute to the shedding of the endothelial glycocalyx leading to or exacerbating existing disease (Yamaoka-Tojo, 2020b; Patterson et al., 2022). Of all the determinants of the endothelial glycocalyx integrity, high salt intake and HIV infection are important contributors to endothelial glycocalyx shedding and consequently, to the development of hypertension and cardiovascular disease.
3 Sodium-endothelial glycocalyx interaction is the premise for endothelial dysfunction, hypertension, and cardiovascular disease
3.1 Sodium transport from the gut into the systemic circulation
After a salty meal, almost all the dietary salt is absorbed throughout the whole length of the small intestines in the jejunum and ileum under the control of angiotensin II and in the colon via the effects of aldosterone (Ryuzaki et al., 2022). Sodium uptake into the enterocytes across the brush border membrane (transcellular route) is made possible through several transporters such as Sodium/glucose cotransporter 1 (SGLT1), Na+ -dependent excitatory amino acid transporters, Na+ -dependent Ca2+ exchanger (NCX), Na+ -dependent hydrogen exchanger (NHE), Na+ -dependent potassium chloride cotransporter (NKCC), Na+ -dependent chloride cotransporter (NCC), Na+ -dependent magnesium exchanger (NME and Epithelial Sodium Channel (ENaC) (Gagnon and Delpire, 2021). Na+ is pumped out towards the blood stream by Na+/K + ATPase pump on the basolateral membrane (Wright and Loo, 2000).
Owing to the electronegative mucosal surface of the small intestines, Na+ also moves across from the lumen of the gut passively between the enterocytes (paracellular route) through the tight junctions into the interstitium and into portal circulation. In the vascular bed, the endothelial glycocalyx being negatively charged buffers Na+ ions but when in excess, this buffering capacity is reduced resulting in diminished red blood cell-endothelial glycocalyx repelling effect (Oberleithner et al., 2011; Kusche-Vihrog and Oberleithner, 2012). This leads to shedding of the glycocalyx allowing red blood cells to come into contact with endothelial cells, which activates them and stimulates endothelial cells to express adhesion molecules, favoring leukocyte adhesion (Langer and Chavakis, 2009; Jiang et al., 2021). Thus, a damaged glycocalyx promotes leukocyte adhesion and diapedesis as well as platelet recruitment that favors both inflammatory and thrombotic processes (Iba and Levy, 2019; Uchimido et al., 2019). Endothelial glycocalyx damage and endothelial cell activation increases vascular permeability allowing Na+ to extravasate and collect in the interstitial tissue where it induces inflammatory responses and edema (Nijst et al., 2015; Li et al., 2022; Sulyok et al., 2022). When the Na+ in the endothelial glycocalyx accumulates and increases in the luminal surface of the endothelial cells, this increases the activation and activity of the ENaC. ENaC activation leads to more sodium getting pumped into the endothelial cells and this causes stiffness of the cell’s cortex and reduces nitric oxide production especially with subsequent elevation of vascular tone (Kusche-Vihrog et al., 2014). These events contribute to the development of salt-sensitive hypertension (Sulyok et al., 2022), Figure 2.
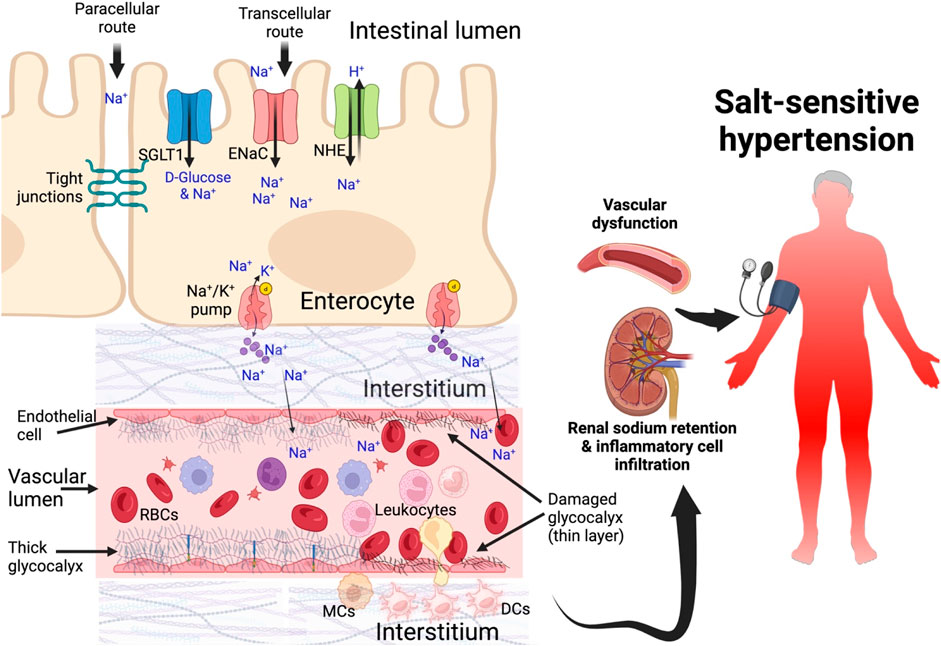
Figure 2. Sodium transport from the gut into blood circulation in salt-sensitive hypertension Sodium is transported from the intestinal lumen through enterocytes via sodium channels and across basolateral membrane into the interstitium and then into systemic circulation. As sodium increases in the interstitial spaces it is transported into the lumen. Moreover, dendritic cells in the interstitial spaces take up sodium and become activated, secreting inflammatory cytokines. Damaged glycocalyx allows sodium to extravasate and contribute to endothelial dysfunction, impair vascular tone, cellular infiltration of kidney and inflammation, sodium retention in the kidney leading to salt-sensitive hypertension. DCs, dendritic cells; SGLT1, sodium glucose transporters; ENaC, epithelial sodium channel; NHE, Na+ -dependent hydrogen exchanger.
3.2 High salt intake impairs endothelial glycocalyx leading to endothelial dysfunction, hypertension, and cardiovascular disease
Vinaiphat et al recently demonstrated that mice feed with high salt diet caused severe shedding and damage to the endothelial glycocalyx and induced hypertension suggesting that dietary salt directly contributes to the shedding of the endothelial glycocalyx, and disrupting the vascular integrity of multiple organs (Vinaiphat et al., 2023). High salt also impaired glycocalyx protein mediators involved in contractility, renal function, mechanotransduction, and coagulation cascades. Vinaiphat and colleagues further demonstrated that high salt promoted arterial wall inflammation due to infiltration by monocytes and macrophages. Monocyte infiltration in the arterial wall was mediated by increased expression of vascular adhesion protein-1 (VCAM-1) induced by high Na+ (Afsar et al., 2018).
In human studies, we have previously demonstrated that high salt intake correlates with poor erythrocyte glycocalyx sensitivity to sodium (eGCSS) (Masenga et al., 2022), a surrogate test for assessing endothelial glycocalyx integrity or damage based on a “salt blood test” (Oberleithner, 2015). In salt -sensitive hypertension, high Na+ reduces the production of NO and induces endothelial secretion of pro-inflammatory cytokines IL-1ß and TNFα (Vinaiphat et al., 2023). Damaged endothelial glycocalyx in the kidney correlates positively with endothelial dysfunction and uraemic toxins (Liew et al., 2021). Conversely, endothelial dysfunction is an independent predictor of atherosclerosis, hypertension and cardiovascular disease (Hadi et al., 2005; Brandes, 2014; Widmer and Lerman, 2014). In a study evaluating the relationship between endothelial glycocalyx damage and acute coronary syndrome, Miranda et al. (2016) found that serum levels of syndecan-1 were elevated in acute coronary syndrome. In another clinical study by Bielecka-Dabrowa et al. (2013), they found serum levels of syndecan-4 correlated negatively with left ventricular ejection fraction (LVEF) but positively with left ventricular systolic and diastolic diameters in patients with dilated cardiomyopathy. In several other studies, damaged endothelial glycocalyx was associated with acute or chronic heart failure and death (Tromp et al., 2014; Neves et al., 2015; Demissei et al., 2016).
Depending on the underlying cause, endothelial glycocalyx damage can either be systemic or localized. For example, in sepsis, bacteria toxins and inflammation can promote systemic glycocalyx thinning that may promote increased vascular permeability leading to systemic edema, hypovolemia and circulatory dysfunction resulting in multiorgan damage (Iba and Levy, 2019; Uchimido et al., 2019). However, there is scarcity of data that reports the extent of glycocalyx damage brought upon by HIV and dietary salt.
Although chronic high salt impairs endothelial glycocalyx, acute salt loading does not appear to affect or impair the endothelial glycocalyx (Vinaiphat et al., 2023). In a recent study we found that individuals with an immediate pressor response to oral salt (IPROS) had better endothelial glycocalyx scores, measured using a salt blood test (Masenga et al., 2022). However, the underlying reasons for this were unknown and beyond the scope of the study.
It takes approximately 5–7 days for a damaged endothelial glycocalyx to be restored in vivo (Potter et al., 2009). Rosemary and colleagues were able to show that plasma is able to restore the endothelial glycocalyx and preserve syndecan-1 after hemorrhagic shock (Kozar et al., 2011). Therapeutic approaches to restoring the endothelial glycocalyx are described in Section 6.
4 HIV infection, antiretroviral therapy and endothelial glycocalyx integrity, premise for endothelial function and cardiovascular disease
4.1 Endothelial glycocalyx integrity in persons with HIV
Currently there is dearth of literature linking endothelial glycocalyx damage and HIV infection. However, there are many studies that discuss endothelial function and the role of HIV in impairing endothelial function. In this section, we will attempt to bridge the gap by proposing mechanisms underlying damage to the endothelial glycocalyx that is mediated by HIV viral proteins and we will also discuss the role of ART on endothelial glycocalyx.
HIV has evolved to use glycocalyx components such as syndecans as attachments receptors allowing interaction (Saphire et al., 2001). The negatively charged endothelial glycocalyx enables binding of HIV viral protein portions that are positively charged and in addition hydrogen bonding and hydrophobic interactions between viral proteins and glycoepitopes ensures tighter binding (Cafaro et al., 2021; Foote et al., 2022; Fragkou et al., 2023).
HIV infection and high viremia is associated with damaged endothelial glycocalyx (determined by measuring perfused boundary region) which is only improved after initiating ART (Triantafyllidi et al., 2022; Fragkou et al., 2023). Compared to heathy individuals, PLWH have higher plasma Syndecan-1, a marker of damaged endothelial glycocalyx, and damage to endothelial glycocalyx was associated with markers of kidney dysfunction (Meneses et al., 2017). When PLWH were followed for a longer period (5 years) to determine the link between endothelial glycocalyx damage and kidney dysfunction, Cavalcante et al found that endothelial glycocalyx determined by plasma syndecan-1 levels worsened with chronic use of ART and correlated positively with worsening of creatinine and glomerular filtration rate (GFR) values (Gadelha Cavalcante et al., 2022). These findings have been reproduced in other studies (De Francesco Daher et al., 2016; Yamaoka-Tojo, 2020a; Fragkou et al., 2023) albeit very scarce in the HIV population.
4.2 Endothelial dysfunction in HIV
HIV-1 has limited specific cell types it can infect due to specific signature receptors such as CD4 and the co-receptor C-C chemokine receptor type 5 (CCR5) it can interact with to establish infection (Llorente García and Marsh, 2020; Weichseldorfer et al., 2022). Several cells/organs have been demonstrated to establish HIV-1 infection, we have evidence that HIV-1 can infect endothelial cells of the microvascular system, brain, liver and glomeruli via HIV viral particles without cytolysis (Lafon et al., 1993; Moses et al., 1993; Bagasra et al., 1996). In vitro experiments demonstrated that infected T-cells induce expression of intracellular adhesion molecule-1 and through IFN-γ enhance the adhesion of infected T-cells to endothelial cells activating them while viral replication in infected endothelial cells is enhanced by TNF-α and IL-1, events that all lead to endothelial dysfunction (Conaldi et al., 1995; Dianzani et al., 1996).
Viral particles are believed to play a critical role in shedding endothelial glycocalyx largely attributed to HIV viral proteins such as envelope glycoprotein (gp120), transactivator of transcription (Tat), and Nef which are secreted into- and interact with the endothelial glycocalyx during HIV infection (Anand et al., 2018; Spillings et al., 2022). Viral proteins mostly cause endothelial dysfunction mainly mediated by inflammatory responses. Nef and gp120 can enter into endothelial cells leading to endothelial dysfunction, oxidative stress, increased expression of adhesion molecules and inducing innate immune responses and inflammation (Arese et al., 2001; András et al., 2003; Avraham et al., 2004; Kanmogne et al., 2007).
Endothelial function including distensibility and arterial wall stiffness are mainly determined by flow mediated dilatation (FMD) which directly measures the dilatory effects of NO production by assessing change in brachial artery diameter before and after inducing reactive hyperemia. Determination of the endothelial glycocalyx is not necessarily a measure of endothelial function rather, an early indicator likely to cause endothelial dysfunction. However, serum levels of soluble syndecan-1 were independently associated with FMD suggesting its importance as a surrogate marker of endothelial function (Salmito et al., 2015). Many studies have demonstrated evidence of an impaired endothelial dysfunction measured by FMD among PLWH (Bonnet et al., 2004; Blum et al., 2005; Charakida et al., 2005; Solages et al., 2006). HIV viral load and ART use especially of the class of protease inhibitors, were independent predictors of endothelial function in PLWH (Bonnet et al., 2004; Blum et al., 2005; Charakida et al., 2005; Solages et al., 2006).
5 Mechanisms of endothelial glycocalyx damage, endothelial dysfunction and associated complications
5.1 Glycocalyx and endothelial cell mechanisms of damage
When the glycocalyx is shedding due to damage by infectious agents or other substances, fragments released into circulation serve as damage or danger-associated molecular patterns (DAMPs) and are recognized by pattern recognition receptors (PRR) on innate immune cells, eliciting suboptimal inflammatory response and trauma induced coagulopathy (TIC) within the vasculature (Johansson et al., 2011). High salt is a common cause of endothelial glycocalyx damage that impairs endothelial function. The endothelial glycocalyx participates in the activation of NO via mechanotransduction pathways to induce vasodilatory effects that serve to reduce blood pressure. Therefore, direct damage of the endothelial glycocalyx by salt-induced ROS causes endothelial dysfunction, diminishing vasodilatory function during salt-induced hypertension also seen in pre-eclampsia and increasing the risk for cardiovascular disease development (Bartosch et al., 2017; Schierke et al., 2017; Weissgerber et al., 2019; Stanhewicz et al., 2021). Early during pre-eclampsia, the endothelial glycocalyx has been demonstrated to be diminished, increasing the potential for endothelial activation and vascular injury (Weissgerber et al., 2019). Circulating concentrations of glycocalyx degradation products are higher in pre-eclampsia compared to normotensive women (Carlberg et al., 2022).
HIV viral proteins are able to modify the endothelial cells, disrupting the mechanotransduction pathways and interfering with endothelial cell function to maintain homeostasis (Anand et al., 2018). HIV viral proteins are immunogenic and elicit inflammatory responses which cause damage to the endothelial glycocalyx exposing the endothelial cells. Soluble HIV gp120 has been reported to induce apoptosis in endothelial cells of coronary vessels via the co-receptor CXCR4, induce endothelial permeability leading to endothelial dysfunction and induction of pro-inflammatory cytokines IL-6 and IL-8 in endothelial cells (Fiala et al., 2004; Yang et al., 2009). Gp120 induces apoptosis by activating caspase-3, upregulating Bax and signaling through p38 mitogen-activated protein (MAP) kinase (Ullrich et al., 2000; Khan et al., 2007). Gp120 also enhances endothelial expression of adhesion molecules recruiting inflammatory cells that further disrupt and damage the endothelial glycocalyx. As will be discussed in the next section, HIV viral protein gp120 is known to reduce NO levels leading to abnormal vascular tone regulation and activation of platelets, leading to thrombotic events (Chatterjee and Catravas, 2008; Jiang et al., 2010). The whole mark of HIV viral proteins leading to endothelial glycocalyx damage and endothelial dysfunction is by direct interaction with glycocalyx, endothelial cells and inducing inflammatory responses that further disrupt endothelial function accelerating atherosclerosis and leading to hypertension and cardiovascular disease (Anand et al., 2018).
In summary, the damage to endothelial glycocalyx by both HIV viral proteins and dietary salt as an initial step prior to endothelial dysfunction is mutually exclusive. The presence of HIV undermines vascular integrity so much that high dietary salt intake exacerbates the pathogenesis leading to endothelial dysfunction and cardiovascular disease. Both dietary salt and HIV promote inflammation that contributes to vascular dysfunction and hypertension (Ruggeri Barbaro et al., 2021; Mutengo et al., 2022; Pitzer et al., 2022). However, the mechanisms are obviously different. The whole mark of endothelial glycocalyx damage is that it induces a proinflammatory phenotype and increased leukocyte adhesion and this has been demonstrated in cultured endothelial cells (McDonald et al., 2016).
5.2 Activation of NADPH oxidase, NF-κB pathway and NLRP3 inflammasomes
Excess sodium in the damaged glycocalyx and interstitium increases entry of sodium into the endothelial cells and dendritic cells or monocytes/macrophages, respectively, via ENaC (Barbaro et al., 2017; Mutchler and Kleyman, 2019; Ertuglu and Kirabo, 2022; Nemeth et al., 2022; Pitzer et al., 2022). Na+ is exchanged for Ca2+ by the Na+/Ca2+ exchanger and the increased entry of Ca2+ into the cell activates protein kinase C which phosphorylates NADPH oxidase enzyme increasing its activity (Datla and Griendling, 2010). NADPH oxidase produces reactive oxygen species (ROS) causing oxidant stress and phospholipid peroxidation leading to formation of immunogenic isolevuglandin (IsoLG)-protein adducts (Barbaro et al., 2017). Dendritic cells process IsoLG-protein adducts into major histocompatibility molecules and presents them to naïve CD4+ T-cells, activating them. Activated CD4+ T-cells infiltrate the kidney and secret inflammatory cytokines such as IL-17A, TNF-α, and interferon-gamma (IFN-γ) that damage the vasculature and kidney leading to salt-sensitive hypertension (Dixon et al., 2017; Rucker et al., 2018; Xiao et al., 2018; Ertuglu et al., 2022; Pitzer et al., 2022), Figure 3. Although dendritic cells are referred to here, other cell types such as monocytes, macrophages and lymphocytes are also affected by high salt, contributing to immune activation and inflammation in salt-sensitive hypertension in both human and animal models (Zhang et al., 2015; Ren and Crowley, 2019; Sahinoz et al., 2021; Nemeth et al., 2022).
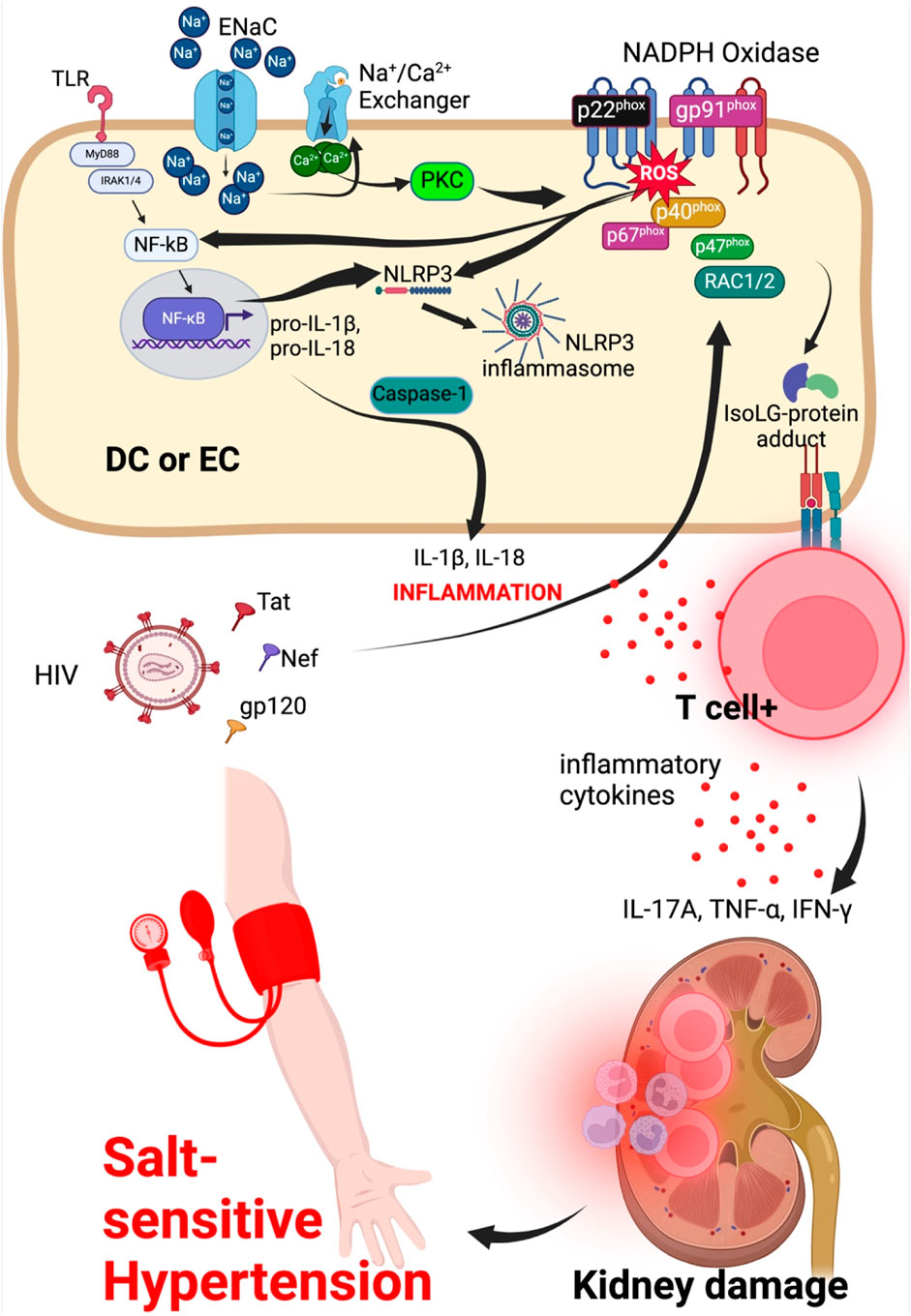
Figure 3. Sodium and HIV induced hypertension signaling mechanisms in dendritic or endothelial cell. Endothelial cells/dendritic cell combined for ease to explain entry of sodium and HIV viral particle effects on endothelial cell to activate intracellular mechanisms contributing to kidney damage and hypertension. ENaC, epithelial sodium channel; NLRP3, NLR family pyrin domain containing 3; NF-κB, Nuclear factor kappa-light-chain-enhancer of activated B-cells; MyD88, Myeloid differentiation primary response 88; TNFR, tumor necrosis factor receptor; TLR, toll-like receptor; IFN-γ, interferon gamma; DC, dendritic cell; EC, endothelial cell.
ROS also activate the nuclear factor kappa-light-chain-enhancer of activated B (NF-κB) which translocate into the nucleus to induce transcription of pro-inflammatory cytokines IL-1β and IL-18 (Pitzer et al., 2022). NF-κB also activates NLR Family Pyrin Domain Containing 3 (NLRP3) inflammasome which acts through caspase-1 component to cleave pro-inflammatory cytokines IL-1β and IL-18 into active IL-1β and IL-18. Activation of NF-κB and NLRP3 inflammasome is associated with production of tumor necrosis factor-alpha (TNF-α), IL-1β, and IL-6 inflammatory cytokines leading to vascular damage, endothelial dysfunction resulting in hypertension and cardiovascular disease (Ertuglu et al., 2022; Pitzer et al., 2022; Masenga et al., 2023). The NF-κB pathway is also activated by shear stress of the endothelial glycocalyx via activation of Rho family GTPases which in turn activates and induces the transcriptional activity of NF-κB (Li et al., 2005).
In HIV infection, damaged endothelial glycocalyx increases interaction between HIV viral proteins and endothelial cells. The viral proteins gp120, Tat and Nef impair ROS regulation. Tat induces activation of small GTPase Rac1/2 which phosphorylates a subunit of the dissociated NADPH oxidase, p47phox causing it to translocate to the membrane and merge with additional subunits p67phox, p40phox, gp91 phox and p22 phox, activating the NADPH oxidase and increasing its activity. NADPH oxidase is made up of two membrane subunits (p22phox and gp91phox) and four cytosolic subunits (p40phox, p47phox, p67phox and Rac1/2 (Rueckschloss et al., 2003; Belambri et al., 2018; Margaritis, 2019), Figure 3. Downstream signal transduction pathways that are activated owing to the activity of the NADPH oxidase include the Jun N-terminal kinase (JNK), extracellular signal-regulated kinase (ERK) and NF-kB pathways, promoting inflammatory responses by mechanisms already explained. Compared to dietary salt (sodium to be specific) which reduces NO generation via ENaC-protein kinase C-NADPH oxidase activation to produce ROS that directly inhibit NO generation, HIV viral protein gp120, Tat and Nef downregulate eNOS mRNA transcription and translation leading to reduced levels of the enzyme and consequently reducing the production of NO required to maintain vascular tone during hypertension (Margaritis, 2019).
5.3 Oxidative stress and enzymes directly damage the glycocalyx by fragmentation of its components
Oxidative stress is a key feature of endothelial dysfunction and results from an imbalance between enzymes that generate ROS (NADPH oxidase and eNOS) and antioxidant scavengers to counteract and destroy ROS in the vasculature (Münzel et al., 2017). Normally, when vascular tone increases, NO production is increased via conversion of L-arginine to L-citrulline by enzymatic activity of endothelial nitric oxide synthase, this leads to vasodilation of blood vessels, ameliorating high blood pressure (Loperena and Harrison, 2017; Ray et al., 2023). However, the high production of ROS resulting from increased NADPH oxidase activation (a result of increased Na+ entry into endothelial cells via ENaC) causes ROS to react directly and inhibit nitric oxide production. This impairs vasodilatory function, a characteristic feature of endothelial dysfunction in salt -sensitive hypertension (Datla and Griendling, 2010; Patik et al., 2021; Ruggeri Barbaro et al., 2021; Ertuglu et al., 2022).
ROS-induced production by inflammatory cells and endothelial cells directly damage and modify the endothelial glycocalyx increasing leukocyte adhesion and inflammatory responses (Rubio-Gayosso et al., 2006). Moreover, circulating fragments of damaged glycocalyx are immunogenic, thus, exacerbating the immune response and contribute to salt-sensitive hypertension (van Golen et al., 2012). The endothelial glycocalyx is very fragile to enzymatic (hyaluronidase, metalloproteinases, and heparinase) and ROS degradation. Metalloproteinases are secreted by endothelial cells, innate immune cells and smooth muscle cells when stimulated by inflammatory cytokines, shear stress, ROS and hypoxia (Hobeika et al., 2007; Castro et al., 2008; Iba and Levy, 2019). Metalloproteinases mainly degrade extracellular domains of syndecans and chondroitin sulfate (Dreyfuss et al., 2009; Sieve et al., 2018; Bogner-Flatz et al., 2019) while heparinase, which is secreted by endothelial cells, inflammatory cells and platelets cleaves the inner chains of heparan sulfate under the stimulation of ROS, hyperglycemia, advanced glycosylation products and inflammatory cytokines (Ishai-Michaeli et al., 1990; Chen et al., 2004; Han et al., 2005; Kramer et al., 2006; An et al., 2011; Masola et al., 2018).
5.4 Renal dysfunction and endothelial glycocalyx
In the kidney, ROS damage the glycocalyx contributing to kidney disease and hypertension (Singh et al., 2013). Oxidative stress in salt-sensitive hypertension is exaggerated as demonstrated by levels of urine F2-isoprostanes during low and high salt diets (Beltowski et al., 2004; Al-Solaiman et al., 2009). In the kidney, damaged endothelial glycocalyx induced by ROS and inflammatory cytokines increases production of endothelin-1 from activated endothelial cells contributing to vasoconstriction, inflammation and fibrosis (Rossi et al., 1999; Clozel and Salloukh, 2005; Neuhofer and Pittrow, 2006).
6 Diagnostic and therapeutic approaches to vascular glycocalyx
6.1 In vitro diagnostic visualization techniques
It is very difficult to develop direct visualization techniques to study the glycocalyx because its highly vulnerable to reagents. However, through the decades since 1966, several protocols have been developed to aid in visualizing the glycocalyx via transmission electron microscopy (TEM) using various reagents such as ruthenium red, Alcian blue 8GX, and fluorocarbon-based oxygen carrying fixatives (Luft, 1966; Rostgaard and Qvortrup, 1997; Rostgaard and Qvortrup, 2002; van den Berg et al., 2003). Apart from TEM, other visualization techniques that are more or have similar robustness can be employed such as confocal laser scanning microscopy (CLSM) (Barker et al., 2004) and two-photon laser scanning microscopy (Megens et al., 2007). TEM cannot be used in vivo.
Several direct approaches have been employed using proteins that bind specific glycocalyx components. Examples include use of lectins to bind components of glycosaminoglycan chains (Florian et al., 2003; Barker et al., 2004; Mulivor and Lipowsky, 2004) and use of antibodies that bind to specific glycocalyx components such as syndecan, hyaluronan and heparan sulfate (Florian et al., 2003; Mulivor and Lipowsky, 2004). The use of antibodies are particularly important to target specific components of the glycocalyx. Puerta-Guardo and colleagues used antibodies in Immunofluorescence staining to study endothelial glycocalyx using human pulmonary, dermal, and umbilical vein microvascular endothelial cells (Puerta-Guardo et al., 2016). The antibodies used in studying the glycocalyx included anti-human cathepsin L, anti-human heparanase 1, anti-human CD138 for syndecan-1, anti-heparan sulfate proteoglycan two for perlecan among others. For imaging modalities, they used inverted fluorescence microscopy. Several other studies have used antibodies to visualize the endothelial glycocalyx (McDonald et al., 2016; Puerta-Guardo et al., 2016; Butler et al., 2019; Wang et al., 2019).
Several in vitro techniques have been described elsewhere (Haymet et al., 2021) and will therefore not be repeated here.
6.2 In vivo experimental visualization techniques
In 1996, Vink and colleagues were able to indirectly visualize the endothelial glycocalyx using intravital microscopy in muscle capillaries of hamster cremaster (Vink and Duling, 1996). They labeled plasma with a fluorescent dextran so that the plasma exclusion zone represented the glycocalyx area. This method has been used by many researchers onwards using both hamsters (Henry and Duling, 1999; 2000; Vink and Duling, 2000) and mice (Constantinescu et al., 2003; Rubio-Gayosso et al., 2006) but is an indirect technique of visualizing the glycocalyx.
Two-photon laser scanning microscopy can be used for both ex vivo and in vivo visualization of the glycocalyx with good resolution (Megens et al., 2007).
More recently, the sublingual microcirculation with its glycocalyx has been visualized using the glycoCheck system using a camera and dedicated software with moderate reproducibility (Eickhoff et al., 2020; Bol et al., 2022). The glycoCheck system measures the perfused boundary region to determine glycocalyx thickness, and is also able to determine red blood cell filling percentage, and microvascular vessel density (Eickhoff et al., 2020; Bol et al., 2022).
Surrogate tests for assessing endothelial glycocalyx integrity are available. Determination of plasma levels of Syndecan-1 can be used as a marker of endothelial glycocalyx. Syndecan-1 could also be used as a marker of renal dysfunction in PLWH where it correlates positively with serum levels of creatinine and urea (Meneses et al., 2017). Syndecans are more important and clinically relevant to determine as they are the main components of the endothelila glycocalyx correlating with human health and disease.
6.3 Current therapeutic approaches
Several experimental and non-experimental approaches to restore endothelial glycocalyx have been described. Use of spironolactone has been demonstrated to reduce endothelial glycocalyx deterioration and restore endothelial function via mineralocorticoid receptor antagonism (Schierke et al., 2017). In animal models, use of hydrocortisone not only protected rats against ischaemia-reperfusion injury but prevented damage and degradation of the endothelial glycocalyx (Chappell et al., 2007; Gao et al., 2015). Human studies to modulate endothelial glycocalyx remain scarce. However, several therapies are being repurposed to induce endothelial glycocalyx regeneration. Examples of drug therapies include artersil, endocalyx, rosuvastatin, sulodexide, hydrocortisone, metformin, etanercept, antithrombin, poloxamer, albumin, fresh-frozen plasma, hydroxyethyl starch, and heparin, these have discussed in detail by Banerjee et al. (2021) and Masola et al. (2021).
In PLWH, use of an integrase strand transfer inhibitor such as dolutegravir, raltegravir or elvitegravir/cobicistat is associated with restoration of endothelial glycocalyx integrity (Fragkou et al., 2023).
7 Future perspectives
More studies are required to bridge the gap and link between high salt and HIV in promoting endothelial glycocalyx degradation, endothelial dysfunction and hypertension. There are currently no studies known to us that have investigated the synergistic role contributed by dietary salt and HIV in the study of the endothelial glycocalyx.
8 Conclusion
High salt intake damages the endothelial glycocalyx inducing salt sensitive hypertension. Moreover, it is also well established that PLWH have a damaged endothelial glycocalyx due to HIV viral proteins and use of ART which is associated with subsequent endothelial dysfunction and development of cardiovascular disease. Although salt sensitive hypertension is more prevalent in PLWH, the role of dietary salt and HIV-related factors in contributing to endothelial glycocalyx damage are limited.
Author contributions
SM: Conceptualization, Data curation, Formal Analysis, Funding acquisition, Investigation, Methodology, Validation, Visualization, Writing–original draft, Writing–review and editing. SL: Validation, Writing–original draft, Writing–review and editing. AK: Conceptualization, Funding acquisition, Methodology, Supervision, Validation, Writing–original draft, Writing–review and editing.
Funding
The author(s) declare that financial support was received for the research, authorship, and/or publication of this article. This work was supported by the Fogarty International Center and National Institute of Diabetes and Digestive and Kidney Diseases of the National Institutes of Health grants R01HL147818 and R01HL144941 (AK) and 2D43TW009744 (SM), R21TW012635 (AK and SM) and the American Heart Association Award Number 24IVPHA1297559 https://doi.org/10.58275/AHA.24IVPHA1297559.pc.gr.193866 (AK and SM).
Conflict of interest
The authors declare that the research was conducted in the absence of any commercial or financial relationships that could be construed as a potential conflict of interest.
Publisher’s note
All claims expressed in this article are solely those of the authors and do not necessarily represent those of their affiliated organizations, or those of the publisher, the editors and the reviewers. Any product that may be evaluated in this article, or claim that may be made by its manufacturer, is not guaranteed or endorsed by the publisher.
Author disclaimer
The content is solely the responsibility of the authors and does not represent the official views of the National Institutes of Health and the American Heart Association.
References
Afsar, B., Kuwabara, M., Ortiz, A., Yerlikaya, A., Siriopol, D., Covic, A., et al. (2018). Salt intake and immunity. Hypertension 72, 19–23. doi:10.1161/HYPERTENSIONAHA.118.11128
Ahn, S. J., Le Master, E., Granados, S. T., and Levitan, I. (2023). “Chapter One - impairment of endothelial glycocalyx in atherosclerosis and obesity,” in Current topics in membranes. Editors I. S. Fancher, and A. Z. Chignalia (Academic Press), 1–19. doi:10.1016/bs.ctm.2023.02.001
Al-Solaiman, Y., Jesri, A., Zhao, Y., Morrow, J. D., and Egan, B. M. (2009). Low-sodium DASH reduces oxidative stress and improves vascular function in salt-sensitive humans. J. Hum. Hypertens. 23, 826–835. doi:10.1038/jhh.2009.32
An, X.-F., Zhou, L., Jiang, P.-J., Yan, M., Huang, Y.-J., Zhang, S.-N., et al. (2011). Advanced glycation end-products induce heparanase expression in endothelial cells by the receptor for advanced glycation end products and through activation of the FOXO4 transcription factor. Mol. Cell Biochem. 354, 47–55. doi:10.1007/s11010-011-0804-7
Anand, A. R., Rachel, G., and Parthasarathy, D. (2018). HIV proteins and endothelial dysfunction: implications in cardiovascular disease. Front. Cardiovasc Med. 5, 185. doi:10.3389/fcvm.2018.00185
András, I. E., Pu, H., Deli, M. A., Nath, A., Hennig, B., and Toborek, M. (2003). HIV-1 Tat protein alters tight junction protein expression and distribution in cultured brain endothelial cells. J. Neurosci. Res. 74, 255–265. doi:10.1002/jnr.10762
Arese, M., Ferrandi, C., Primo, L., Camussi, G., and Bussolino, F. (2001). HIV-1 tat protein stimulates in vivo vascular permeability and lymphomononuclear cell recruitment. J. Immunol. 166, 1380–1388. doi:10.4049/jimmunol.166.2.1380
Avraham, H. K., Jiang, S., Lee, T.-H., Prakash, O., and Avraham, S. (2004). HIV-1 Tat-mediated effects on focal adhesion assembly and permeability in brain microvascular endothelial cells. J. Immunol. 173, 6228–6233. doi:10.4049/jimmunol.173.10.6228
Bagasra, O., Lavi, E., Bobroski, L., Khalili, K., Pestaner, J. P., Tawadros, R., et al. (1996). Cellular reservoirs of HIV-1 in the central nervous system of infected individuals: identification by the combination of: in situ: polymerase chain reaction and immunohistochemistry. AIDS 10, 573–585. doi:10.1097/00002030-199606000-00002
Bailey, M. A., and Dhaun, N. (2024). Salt sensitivity: causes, consequences, and recent advances. Hypertension 81, 476–489. doi:10.1161/HYPERTENSIONAHA.123.17959
Banerjee, S., Mwangi, J. G., Stanley, T. K., Mitra, R., and Ebong, E. E. (2021). Regeneration and assessment of the endothelial glycocalyx to address cardiovascular disease. Ind. Eng. Chem. Res. 60, 17328–17347. doi:10.1021/acs.iecr.1c03074
Barbaro, N. R., Foss, J. D., Kryshtal, D. O., Tsyba, N., Kumaresan, S., Xiao, L., et al. (2017). Dendritic cell amiloride-sensitive channels mediate sodium-induced inflammation and hypertension. Cell Rep. 21, 1009–1020. doi:10.1016/j.celrep.2017.10.002
Barker, A. L., Konopatskaya, O., Neal, C. R., Macpherson, J. V., Whatmore, J. L., Winlove, C. P., et al. (2004). Observation and characterisation of the glycocalyx of viable human endothelial cells using confocal laser scanning microscopy. Phys. Chem. Chem. Phys. 6, 1006–1011. doi:10.1039/B312189E
Bartosch, A. M. W., Mathews, R., and Tarbell, J. M. (2017). Endothelial glycocalyx-mediated nitric oxide production in response to selective AFM pulling. Biophysical J. 113, 101–108. doi:10.1016/j.bpj.2017.05.033
Belambri, S. A., Rolas, L., Raad, H., Hurtado-Nedelec, M., Dang, P. M.-C., and El-Benna, J. (2018). NADPH oxidase activation in neutrophils: role of the phosphorylation of its subunits. Eur. J. Clin. Invest. 48 (Suppl. 2), e12951. doi:10.1111/eci.12951
Beltowski, J., Wójcicka, G., Marciniak, A., and Jamroz, A. (2004). Oxidative stress, nitric oxide production, and renal sodium handling in leptin-induced hypertension. Life Sci. 74, 2987–3000. doi:10.1016/j.lfs.2003.10.029
Bielecka-Dabrowa, A., von Haehling, S., Aronow, W. S., Ahmed, M. I., Rysz, J., and Banach, M. (2013). Heart failure biomarkers in patients with dilated cardiomyopathy. Int. J. Cardiol. 168, 2404–2410. doi:10.1016/j.ijcard.2013.01.157
Bkaily, G., Simon, Y., Menkovic, I., Bkaily, C., and Jacques, D. (2018). High salt-induced hypertrophy of human vascular smooth muscle cells associated with a decrease in glycocalyx. J. Mol. Cell. Cardiol. 125, 1–5. doi:10.1016/j.yjmcc.2018.10.006
Blum, A., Hadas, V., Burke, M., Yust, I., and Kessler, A. (2005). Viral load of the human immunodeficiency virus could be an independent risk factor for endothelial dysfunction. Clin. Cardiol. 28, 149–153. doi:10.1002/clc.4960280311
Bogner-Flatz, V., Braunstein, M., Ocker, L. E., Kusmenkov, T., Tschoep, J., Ney, L., et al. (2019). On-the-Scene hyaluronan and syndecan-1 serum concentrations and outcome after cardiac arrest and resuscitation. Mediat. Inflamm. 2019, e8071619. doi:10.1155/2019/8071619
Bol, M. E., Broddin, B. E. K., Delhaas, T., Sels, J. E. M., and van de Poll, M. C. G. (2022). Variability of microcirculatory measurements in healthy volunteers. Sci. Rep. 12, 19887. doi:10.1038/s41598-022-22947-x
Bonnet, D., Aggoun, Y., Szezepanski, I., Bellal, N., and Blanche, S. (2004). Arterial stiffness and endothelial dysfunction in HIV-infected children. AIDS 18, 1037–1041. doi:10.1097/00002030-200404300-00012
Boo, Y. C., Hwang, J., Sykes, M., Michell, B. J., Kemp, B. E., Lum, H., et al. (2002). Shear stress stimulates phosphorylation of eNOS at Ser635 by a protein kinase A-dependent mechanism. Am. J. Physiology-Heart Circulatory Physiology 283, H1819–H1828. doi:10.1152/ajpheart.00214.2002
Brandes, R. P. (2014). Endothelial dysfunction and hypertension. Hypertension 64, 924–928. doi:10.1161/HYPERTENSIONAHA.114.03575
Butler, M. J., Ramnath, R., Kadoya, H., Desposito, D., Riquier-Brison, A., Ferguson, J. K., et al. (2019). Aldosterone induces albuminuria via matrix metalloproteinase-dependent damage of the endothelial glycocalyx. Kidney Int. 95, 94–107. doi:10.1016/j.kint.2018.08.024
Cafaro, A., Barillari, G., Moretti, S., Palladino, C., Tripiciano, A., Falchi, M., et al. (2021). HIV-1 tat protein enters dysfunctional endothelial cells via integrins and renders them permissive to virus replication. Int. J. Mol. Sci. 22, 317. doi:10.3390/ijms22010317
Carey, D. J. (1997). Syndecans: multifunctional cell-surface co-receptors. Biochem. J. 327, 1–16. doi:10.1042/bj3270001
Carlberg, N., Cluver, C., Hesse, C., Thörn, S.-E., Gandley, R., Damén, T., et al. (2022). Circulating concentrations of glycocalyx degradation products in preeclampsia. Front. Physiol. 13, 1022770. doi:10.3389/fphys.2022.1022770
Castro, M. M., Rizzi, E., Figueiredo-Lopes, L., Fernandes, K., Bendhack, L. M., Pitol, D. L., et al. (2008). Metalloproteinase inhibition ameliorates hypertension and prevents vascular dysfunction and remodeling in renovascular hypertensive rats. Atherosclerosis 198, 320–331. doi:10.1016/j.atherosclerosis.2007.10.011
Chappell, D., Jacob, M., Hofmann-Kiefer, K., Bruegger, D., Rehm, M., Conzen, P., et al. (2007). Hydrocortisone preserves the vascular barrier by protecting the endothelial glycocalyx. Anesthesiology 107, 776–784. doi:10.1097/01.anes.0000286984.39328.96
Charakida, M., Donald, A. E., Green, H., Storry, C., Clapson, M., Caslake, M., et al. (2005). Early structural and functional changes of the vasculature in HIV-infected children: impact of disease and antiretroviral therapy. Circulation 112, 103–109. doi:10.1161/CIRCULATIONAHA.104.517144
Chatterjee, A., and Catravas, J. D. (2008). Endothelial nitric oxide (NO) and its pathophysiologic regulation. Vasc. Pharmacol. 49, 134–140. doi:10.1016/j.vph.2008.06.008
Chen, G., Wang, D., Vikramadithyan, R., Yagyu, H., Saxena, U., Pillarisetti, S., et al. (2004). Inflammatory cytokines and fatty acids regulate endothelial cell heparanase expression. Biochemistry 43, 4971–4977. doi:10.1021/bi0356552
Choi, H. Y., Park, H. C., and Ha, S. K. (2015). Salt sensitivity and hypertension: a paradigm shift from kidney malfunction to vascular endothelial dysfunction. Electrolyte Blood Press 13, 7–16. doi:10.5049/EBP.2015.13.1.7
Clozel, M., and Salloukh, H. (2005). Role of endothelin in fibrosis and anti-fibrotic potential of bosentan. Ann. Med. 37, 2–12. doi:10.1080/07853890410018925
Conaldi, P. G., Serra, C., Dolei, A., Basolo, F., Falcone, V., Mariani, G., et al. (1995). Productive HIV-1 infection of human vascular endothelial cells requires cell proliferation and is stimulated by combined treatment with interleukin-1 beta plus tumor necrosis factor-alpha. J. Med. Virology 47, 355–363. doi:10.1002/jmv.1890470411
Constantinescu, A. A., Vink, H., and Spaan, J. A. E. (2003). Endothelial cell glycocalyx modulates immobilization of leukocytes at the endothelial surface. Arterioscler. Thromb. Vasc. Biol. 23, 1541–1547. doi:10.1161/01.ATV.0000085630.24353.3D
Curry, F. E., and Adamson, R. H. (2012). Endothelial glycocalyx: permeability barrier and mechanosensor. Ann. Biomed. Eng. 40, 828–839. doi:10.1007/s10439-011-0429-8
Datla, S. R., and Griendling, K. K. (2010). Reactive oxygen species, NADPH oxidases, and hypertension. Hypertension 56, 325–330. doi:10.1161/HYPERTENSIONAHA.109.142422
De Francesco Daher, E. D., Gadelha Cavalcante, M., Cavalcante Meneses, G., Costa Martins, A. M., Braga Libório, A., and Bezerra da Silva Junior, G. (2016). Sp205endothelial glycocalyx damage and renal dysfunction in hiv patients receiving combined antiretroviral therapy. Nephrol. Dial. Transplant. 31, i154–i155. doi:10.1093/ndt/gfw162.24
Demissei, B. G., Valente, M. A. E., Cleland, J. G., O’Connor, C. M., Metra, M., Ponikowski, P., et al. (2016). Optimizing clinical use of biomarkers in high-risk acute heart failure patients. Eur. J. Heart Fail 18, 269–280. doi:10.1002/ejhf.443
Dianzani, F., Scheglovitova, O., Gentile, M., Scanio, V., Barresi, C., Ficociello, B., et al. (1996). Interferon gamma stimulates cell-mediated transmission of HIV type 1 from abortively infected endothelial cells. AIDS Res. Hum. Retroviruses 12, 621–627. doi:10.1089/aid.1996.12.621
Dixon, K. B., Davies, S. S., and Kirabo, A. (2017). Dendritic cells and isolevuglandins in immunity, inflammation, and hypertension. Am. J. Physiology-Heart Circulatory Physiology 312, H368–H374. doi:10.1152/ajpheart.00603.2016
Dragovich, M. A., Chester, D., Fu, B. M., Wu, C., Xu, Y., Goligorsky, M. S., et al. (2016). Mechanotransduction of the endothelial glycocalyx mediates nitric oxide production through activation of TRP channels. Am. J. Physiology-Cell Physiology 311, C846–C853. doi:10.1152/ajpcell.00288.2015
Dreyfuss, J. L., Regatieri, C. V., Jarrouge, T. R., Cavalheiro, R. P., Sampaio, L. O., and Nader, H. B. (2009). Heparan sulfate proteoglycans: structure, protein interactions and cell signaling. An. Acad. Bras. Ciênc. 81, 409–429. doi:10.1590/S0001-37652009000300007
Ebong, E. E., Spray, D. C., and Tarbell, J. M. (2010). “The endothelial glycocalyx: its structure and role in eNOS mechano-activation,” in Proceedings of the 2010 IEEE 36th annual northeast bioengineering conference (NEBEC), 1–2. doi:10.1109/NEBC.2010.5458171
Eickhoff, M. K., Winther, S. A., Hansen, T. W., Diaz, L. J., Persson, F., Rossing, P., et al. (2020). Assessment of the sublingual microcirculation with the GlycoCheck system: reproducibility and examination conditions. PLoS One 15, e0243737. doi:10.1371/journal.pone.0243737
Elijovich, F., Weinberger, M. H., Anderson, C. A. M., Appel, L. J., Bursztyn, M., Cook, N. R., et al. (2016). Salt sensitivity of blood pressure: a scientific statement from the American heart association. Hypertension 68, e7–e46. doi:10.1161/HYP.0000000000000047
Ertuglu, L. A., and Kirabo, A. (2022). Dendritic cell epithelial Sodium Channel in inflammation, salt-sensitive hypertension, and kidney damage. Kidney 3, 1620–1629. doi:10.34067/KID.0001272022
Ertuglu, L. A., Mutchler, A. P., Yu, J., and Kirabo, A. (2022). Inflammation and oxidative stress in salt sensitive hypertension; the role of the NLRP3 inflammasome. Front. Physiology 13, 1096296. doi:10.3389/fphys.2022.1096296
Fiala, M., Polik, W., Qiao, J.-H., Lossinsky, A. S., Alce, T., Tran, K., et al. (2004). HIV-1 induces cardiomyopathy by cardiomyocyte invasion and gp120, tat, and cytokine apoptotic signaling. Cardiovasc Toxicol. 4, 97–107. doi:10.1385/CT:4:2:097
Florian, J. A., Kosky, J. R., Ainslie, K., Pang, Z., Dull, R. O., and Tarbell, J. M. (2003). Heparan sulfate proteoglycan is a mechanosensor on endothelial cells. Circ. Res. 93, e136–e142. doi:10.1161/01.RES.0000101744.47866.D5
Foote, C. A., Soares, R. N., Ramirez-Perez, F. I., Ghiarone, T., Aroor, A., Manrique-Acevedo, C., et al. (2022). Endothelial glycocalyx. Compr. Physiol. 12, 3781–3811. doi:10.1002/cphy.c210029
Fragkou, P. C., Ikonomidis, I., Benas, D., Kavatha, D., Moschopoulos, C. D., Protopapas, K., et al. (2023). Endothelial glycocalyx integrity in treatment-naïve people living with HIV before and one year after antiretroviral treatment initiation. Viruses 15, 1505. doi:10.3390/v15071505
Fransson, L.-A., Belting, M., Cheng, F., Jönsson, M., Mani, K., and Sandgren, S. (2004). Novel aspects of glypican glycobiology. Cell Mol. Life Sci. 61, 1016–1024. doi:10.1007/s00018-004-3445-0
Gadelha Cavalcante, M., Cavalcante Meneses, G., Bezerra, G., Maria Costa Martins, A., Gondim Lima Oliveira, M., Gabriel Monteiro Santos, S., et al. (2022). MO410: early endothelial glycocalyx injury damage associated with further kidney dysfunction in hiv patients: five years follow-up. Nephrol. Dial. Transplant. 37, gfac070024. doi:10.1093/ndt/gfac070.024
Gagnon, K. B., and Delpire, E. (2021). Sodium transporters in human health and disease. Front. Physiology 11, 588664. doi:10.3389/fphys.2020.588664
Gao, S.-L., Zhang, Y., Zhang, S.-Y., Liang, Z.-Y., Yu, W.-Q., and Liang, T.-B. (2015). The hydrocortisone protection of glycocalyx on the intestinal capillary endothelium during severe acute pancreatitis. Shock 43, 512–517. doi:10.1097/SHK.0000000000000326
Hadi, H. A., Carr, C. S., and Al Suwaidi, J. (2005). Endothelial dysfunction: cardiovascular risk factors, therapy, and outcome. Vasc. Health Risk Manag. 1, 183–198.
Han, J., Mandal, A. K., and Hiebert, L. M. (2005). Endothelial cell injury by high glucose and heparanase is prevented by insulin, heparin and basic fibroblast growth factor. Cardiovasc. Diabetol. 4, 12. doi:10.1186/1475-2840-4-12
Harjunpää, H., Llort Asens, M., Guenther, C., and Fagerholm, S. C. (2019). Cell adhesion molecules and their roles and regulation in the immune and tumor microenvironment. Front. Immunol. 10, 1078. doi:10.3389/fimmu.2019.01078
Haymet, A. B., Bartnikowski, N., Wood, E. S., Vallely, M. P., McBride, A., Yacoub, S., et al. (2021). Studying the endothelial glycocalyx in vitro: what is missing? Front. Cardiovasc Med. 8, 647086. doi:10.3389/fcvm.2021.647086
Henry, C. B., and Duling, B. R. (1999). Permeation of the luminal capillary glycocalyx is determined by hyaluronan. Am. J. Physiol. 277, H508–H514. doi:10.1152/ajpheart.1999.277.2.H508
Henry, C. B., and Duling, B. R. (2000). TNF-alpha increases entry of macromolecules into luminal endothelial cell glycocalyx. Am. J. Physiol. Heart Circ. Physiol. 279, H2815–H2823. doi:10.1152/ajpheart.2000.279.6.H2815
Hobeika, M. J., Thompson, R. W., Muhs, B. E., Brooks, P. C., and Gagne, P. J. (2007). Matrix metalloproteinases in peripheral vascular disease. J. Vasc. Surg. 45, 849–857. doi:10.1016/j.jvs.2006.09.066
Iba, T., and Levy, J. H. (2019). Derangement of the endothelial glycocalyx in sepsis. J. Thrombosis Haemostasis 17, 283–294. doi:10.1111/jth.14371
Iozzo, R. V. (1994). Perlecan: a gem of a proteoglycan. Matrix Biol. 14, 203–208. doi:10.1016/0945-053x(94)90183-x
Ishai-Michaeli, R., Eldor, A., and Vlodavsky, I. (1990). Heparanase activity expressed by platelets, neutrophils, and lymphoma cells releases active fibroblast growth factor from extracellular matrix. Cell Regul. 1, 833–842. doi:10.1091/mbc.1.11.833
Jiang, J., Fu, W., Wang, X., Lin, P. H., Yao, Q., and Chen, C. (2010). HIV gp120 induces endothelial dysfunction in tumour necrosis factor-alpha-activated porcine and human endothelial cells. Cardiovasc. Res. 87, 366–374. doi:10.1093/cvr/cvq013
Jiang, X. Z., Goligorsky, M. S., and Luo, K. H. (2021). Cross talk between endothelial and red blood cell glycocalyces via near-field flow. Biophysical J. 120, 3180–3191. doi:10.1016/j.bpj.2021.06.002
Johansson, P. I., Sørensen, A. M., Perner, A., Welling, K. L., Wanscher, M., Larsen, C. F., et al. (2011). Disseminated intravascular coagulation or acute coagulopathy of trauma shock early after trauma? An observational study. Crit. Care 15, R272. doi:10.1186/cc10553
Kanmogne, G. D., Schall, K., Leibhart, J., Knipe, B., Gendelman, H. E., and Persidsky, Y. (2007). HIV-1 gp120 compromises blood-brain barrier integrity and enhances monocyte migration across blood-brain barrier: implication for viral neuropathogenesis. J. Cereb. Blood Flow. Metab. 27, 123–134. doi:10.1038/sj.jcbfm.9600330
Khan, N. A., Di Cello, F., Stins, M., and Kim, K. S. (2007). Gp120-mediated cytotoxicity of human brain microvascular endothelial cells is dependent on p38 mitogen-activated protein kinase activation. J. NeuroVirology 13, 242–251. doi:10.1080/13550280701286531
Kim, Y.-H., Nijst, P., Kiefer, K., and Wilson Tang, W. H. (2017). Endothelial glycocalyx as biomarker for cardiovascular diseases: mechanistic and clinical implications. Curr. Heart Fail Rep. 14, 117–126. doi:10.1007/s11897-017-0320-5
Kinsella, M. G., Bressler, S. L., and Wight, T. N. (2004). The regulated synthesis of versican, decorin, and biglycan: extracellular matrix proteoglycans that influence cellular phenotype. Crit. Rev. Eukaryot. Gene Expr. 14, 203–234. doi:10.1615/critreveukaryotgeneexpr.v14.i3.40
Kozar, R. A., Peng, Z., Zhang, R., Holcomb, J. B., Pati, S., Park, P., et al. (2011). Plasma restoration of endothelial glycocalyx in a rodent model of hemorrhagic shock. Anesth. Analgesia 112, 1289–1295. doi:10.1213/ANE.0b013e318210385c
Kramer, A., van den Hoven, M., Rops, A., Wijnhoven, T., van den Heuvel, L., Lensen, J., et al. (2006). Induction of glomerular heparanase expression in rats with adriamycin nephropathy is regulated by reactive oxygen species and the renin-angiotensin system. J. Am. Soc. Nephrol. 17, 2513–2520. doi:10.1681/ASN.2006020184
Kusche-Vihrog, K., Jeggle, P., and Oberleithner, H. (2014). The role of ENaC in vascular endothelium. Pflugers Arch. - Eur. J. Physiol. 466, 851–859. doi:10.1007/s00424-013-1356-3
Kusche-Vihrog, K., and Oberleithner, H. (2012). An emerging concept of vascular salt sensitivity. F1000 Biol. Rep. 4, 20. doi:10.3410/B4-20
Lafon, M. E., Gendrault, J. L., Royer, C., Jaeck, D., Kirn, A., and Steffan, A. M. (1993). Human endothelial cells isolated from the hepatic sinusoids and the umbilical vein display a different permissiveness for HIV1. Res. Virology 144, 99–104. doi:10.1016/S0923-2516(06)80018-X
Langer, H. F., and Chavakis, T. (2009). Leukocyte – endothelial interactions in inflammation. J. Cell. Mol. Med. 13, 1211–1220. doi:10.1111/j.1582-4934.2009.00811.x
Li, L., and Bonventre, J. V. (2016). Endothelial glycocalyx: not just a sugar coat. Am. J. Respir. Crit. Care Med. 194, 390–393. doi:10.1164/rccm.201603-0624ED
Li, X., Alu, A., Wei, Y., Wei, X., and Luo, M. (2022). The modulatory effect of high salt on immune cells and related diseases. Cell Prolif. 55, e13250. doi:10.1111/cpr.13250
Li, Y.-S. J., Haga, J. H., and Chien, S. (2005). Molecular basis of the effects of shear stress on vascular endothelial cells. J. Biomechanics 38, 1949–1971. doi:10.1016/j.jbiomech.2004.09.030
Liew, H., Roberts, M. A., Pope, A., and McMahon, L. P. (2021). Endothelial glycocalyx damage in kidney disease correlates with uraemic toxins and endothelial dysfunction. BMC Nephrol. 22, 21. doi:10.1186/s12882-020-02219-4
Llorente García, I., and Marsh, M. (2020). A biophysical perspective on receptor-mediated virus entry with a focus on HIV. Biochim. Biophys. Acta Biomembr. 1862, 183158. doi:10.1016/j.bbamem.2019.183158
Loperena, R., and Harrison, D. G. (2017). Oxidative stress and hypertensive diseases. Med. Clin. North Am. 101, 169–193. doi:10.1016/j.mcna.2016.08.004
Luft, J. H. (1966). Fine structures of capillary and endocapillary layer as revealed by ruthenium red. Fed. Proc. 25, 1773–1783.
Margaritis, M. (2019). Endothelial dysfunction in HIV infection: experimental and clinical evidence on the role of oxidative stress. Ann. Res. Hosp. 3, 7. doi:10.21037/arh.2019.02.01
Masenga, S. K., Hamooya, B. M., Nzala, S., Kwenda, G., Heimburger, D. C., Mutale, W., et al. (2019). Patho-immune mechanisms of hypertension in HIV: a systematic and thematic review. Curr. Hypertens. Rep. 21, 56. doi:10.1007/s11906-019-0956-5
Masenga, S. K., Kabwe, L. S., Chakulya, M., and Kirabo, A. (2023). Mechanisms of oxidative stress in metabolic syndrome. Int. J. Mol. Sci. 24, 7898. doi:10.3390/ijms24097898
Masenga, S. K., Kirabo, A., Hamooya, B. M., Nzala, S., Kwenda, G., Heimburger, D. C., et al. (2021). HIV-positive demonstrate more salt sensitivity and nocturnal non-dipping blood pressure than HIV-negative individuals. Clin. Hypertens. 27, 2. doi:10.1186/s40885-020-00160-0
Masenga, S. K., Pilic, L., Malumani, M., and Hamooya, B. M. (2022). Erythrocyte sodium buffering capacity status correlates with self-reported salt intake in a population from Livingstone, Zambia. PLOS ONE 17, e0264650. doi:10.1371/journal.pone.0264650
Masola, V., Bellin, G., Gambaro, G., and Onisto, M. (2018). Heparanase: a multitasking protein involved in extracellular matrix (ecm) remodeling and intracellular events. Cells 7, 236. doi:10.3390/cells7120236
Masola, V., Zaza, G., Arduini, A., Onisto, M., and Gambaro, G. (2021). Endothelial glycocalyx as a regulator of fibrotic processes. Int. J. Mol. Sci. 22, 2996. doi:10.3390/ijms22062996
McDonald, K. K., Cooper, S., Danielzak, L., and Leask, R. L. (2016). Glycocalyx degradation induces a proinflammatory phenotype and increased leukocyte adhesion in cultured endothelial cells under flow. PLoS One 11, e0167576. doi:10.1371/journal.pone.0167576
Megens, R. T. A., Reitsma, S., Schiffers, P. H. M., Hilgers, R. H. P., De Mey, J. G. R., Slaaf, D. W., et al. (2007). Two-photon microscopy of vital murine elastic and muscular arteries. Combined structural and functional imaging with subcellular resolution. J. Vasc. Res. 44, 87–98. doi:10.1159/000098259
Meneses, G. C., Cavalcante, M. G., da Silva Junior, G. B., Martins, A. M. C., Neto, R. da J. P., Libório, A. B., et al. (2017). Endothelial glycocalyx damage and renal dysfunction in HIV patients receiving combined antiretroviral therapy. AIDS Res. Hum. Retroviruses 33, 703–710. doi:10.1089/AID.2016.0284
Miranda, C. H., de Carvalho Borges, M., Schmidt, A., Marin-Neto, J. A., and Pazin-Filho, A. (2016). Evaluation of the endothelial glycocalyx damage in patients with acute coronary syndrome. Atherosclerosis 247, 184–188. doi:10.1016/j.atherosclerosis.2016.02.023
Mochizuki, S., Vink, H., Hiramatsu, O., Kajita, T., Shigeto, F., Spaan, J. A. E., et al. (2003). Role of hyaluronic acid glycosaminoglycans in shear-induced endothelium-derived nitric oxide release. Am. J. Physiol. Heart Circ. Physiol. 285, H722–H726. doi:10.1152/ajpheart.00691.2002
Moses, A. V., Bloom, F. E., Pauza, C. D., and Nelson, J. A. (1993). Human immunodeficiency virus infection of human brain capillary endothelial cells occurs via a CD4/galactosylceramide-independent mechanism. Proc. Natl. Acad. Sci. 90, 10474–10478. doi:10.1073/pnas.90.22.10474
Mulivor, A. W., and Lipowsky, H. H. (2004). Inflammation- and ischemia-induced shedding of venular glycocalyx. Am. J. Physiol. Heart Circ. Physiol. 286, H1672–H1680. doi:10.1152/ajpheart.00832.2003
Münzel, T., Camici, G. G., Maack, C., Bonetti, N. R., Fuster, V., and Kovacic, J. C. (2017). Impact of oxidative stress on the heart and vasculature Part 2 of a 3-Part Series. J. Am. Coll. Cardiol. 70, 212–229. doi:10.1016/j.jacc.2017.05.035
Mutchler, S. M., and Kleyman, T. R. (2019). New insights regarding epithelial Na+ channel regulation and its role in the kidney, immune system and vasculature. Curr. Opin. Nephrol. Hypertens. 28, 113–119. doi:10.1097/MNH.0000000000000479
Mutengo, K. H., Masenga, S. K., Mwesigwa, N., Patel, K. P., and Kirabo, A. (2022). Hypertension and human immunodeficiency virus: a paradigm for epithelial sodium channels? Front. Cardiovasc. Med. 9, 968184. doi:10.3389/fcvm.2022.968184
Nemeth, Z., Hildebrandt, E., Parsa, N., Fleming, A. B., Wasson, R., Pittman, K., et al. (2022). Epithelial sodium channels in macrophage migration and polarization: role of proinflammatory cytokines TNFα and IFNγ. Am. J. Physiol. Regul. Integr. Comp. Physiol. 323, R763–R775. doi:10.1152/ajpregu.00207.2022
Neuhofer, W., and Pittrow, D. (2006). Role of endothelin and endothelin receptor antagonists in renal disease. Eur. J. Clin. Investigation 36, 78–88. doi:10.1111/j.1365-2362.2006.01689.x
Neves, F. M. de O., Meneses, G. C., Sousa, N. E. A., Menezes, R. R. P. P. B. de, Parahyba, M. C., Martins, A. M. C., et al. (2015). Syndecan-1 in acute decompensated heart failure--association with renal function and mortality. Circ. J. 79, 1511–1519. doi:10.1253/circj.CJ-14-1195
Nijst, P., Verbrugge, F. H., Grieten, L., Dupont, M., Steels, P., Tang, W. H. W., et al. (2015). The pathophysiological role of interstitial sodium in heart failure. J. Am. Coll. Cardiol. 65, 378–388. doi:10.1016/j.jacc.2014.11.025
Nista, F., Gatto, F., Albertelli, M., and Musso, N. (2020). Sodium intake and target organ damage in hypertension—an update about the role of a real villain. Int. J. Environ. Res. Public Health 17, 2811. doi:10.3390/ijerph17082811
Oberleithner, H. (2015). Sodium selective erythrocyte glycocalyx and salt sensitivity in man. Pflugers Arch. - Eur. J. Physiol. 467, 1319–1325. doi:10.1007/s00424-014-1577-0
Oberleithner, H., Peters, W., Kusche-Vihrog, K., Korte, S., Schillers, H., Kliche, K., et al. (2011). Salt overload damages the glycocalyx sodium barrier of vascular endothelium. Pflugers Arch. - Eur. J. Physiol. 462, 519–528. doi:10.1007/s00424-011-0999-1
Oberleithner, H., Riethmüller, C., Schillers, H., MacGregor, G. A., de Wardener, H. E., and Hausberg, M. (2007). Plasma sodium stiffens vascular endothelium and reduces nitric oxide release. Proc. Natl. Acad. Sci. 104, 16281–16286. doi:10.1073/pnas.0707791104
Oberleithner, H., and Wilhelmi, M. (2015). Vascular glycocalyx sodium store - determinant of salt sensitivity? Blood Purif. 39, 7–10. doi:10.1159/000368922
Pahakis, M. Y., Kosky, J. R., Dull, R. O., and Tarbell, J. M. (2007). The role of endothelial glycocalyx components in mechanotransduction of fluid shear stress. Biochem. Biophysical Res. Commun. 355, 228–233. doi:10.1016/j.bbrc.2007.01.137
Patik, J. C., Lennon, S. L., Farquhar, W. B., and Edwards, D. G. (2021). Mechanisms of dietary sodium-induced impairments in endothelial function and potential countermeasures. Nutrients 13, 270. doi:10.3390/nu13010270
Patterson, E. K., Cepinskas, G., and Fraser, D. D. (2022). Endothelial glycocalyx degradation in critical illness and injury. Front. Med. (Lausanne) 9, 898592. doi:10.3389/fmed.2022.898592
Pitzer, A., Elijovich, F., Laffer, C. L., Ertuglu, L. A., Sahinoz, M., Saleem, M., et al. (2022). DC ENaC-dependent inflammasome activation contributes to salt-sensitive hypertension. Circulation Res. 131, 328–344. doi:10.1161/CIRCRESAHA.122.320818
Potter, D. R., Jiang, J., and Damiano, E. R. (2009). The recovery time course of the endothelial cell glycocalyx in vivo and its implications in vitro. Circulation Res. 104, 1318–1325. doi:10.1161/CIRCRESAHA.108.191585
Prior, S. M., Cohen, M. J., Conroy, A. S., Nelson, M. F., Kornblith, L. Z., Howard, B. M., et al. (2017). Correlation between factor (F)XIa, FIXa and tissue factor and trauma severity. J. Trauma Acute Care Surg. 82, 1073–1079. doi:10.1097/TA.0000000000001449
Puerta-Guardo, H., Glasner, D. R., and Harris, E. (2016). Dengue virus NS1 disrupts the endothelial glycocalyx, leading to hyperpermeability. PLoS Pathog. 12, e1005738. doi:10.1371/journal.ppat.1005738
Qu, J., Cheng, Y., Wu, W., Yuan, L., and Liu, X. (2021). Glycocalyx impairment in vascular disease: focus on inflammation. Front. Cell Dev. Biol. 9, 730621. doi:10.3389/fcell.2021.730621
Ray, A., Ch. Maharana, K., Meenakshi, S., and Singh, S. (2023). Endothelial dysfunction and its relation in different disorders: recent update. Health Sci. Rev. 7, 100084. doi:10.1016/j.hsr.2023.100084
Reitsma, S., Slaaf, D. W., Vink, H., van Zandvoort, M. A. M. J., and oude Egbrink, M. G. A. (2007). The endothelial glycocalyx: composition, functions, and visualization. Pflugers Arch. 454, 345–359. doi:10.1007/s00424-007-0212-8
Ren, J., and Crowley, S. D. (2019). Role of T-cell activation in salt-sensitive hypertension. Am. J. Physiol. Heart Circ. Physiol. 316, H1345–H1353. doi:10.1152/ajpheart.00096.2019
Rossi, G. P., Sacchetto, A., Cesari, M., and Pessina, A. C. (1999). Interactions between endothelin-1 and the renin–angiotensin–aldosterone system. Cardiovasc. Res. 43, 300–307. doi:10.1016/S0008-6363(99)00110-8
Rostgaard, J., and Qvortrup, K. (1997). Electron microscopic demonstrations of filamentous molecular sieve plugs in capillary fenestrae. Microvasc. Res. 53, 1–13. doi:10.1006/mvre.1996.1987
Rostgaard, J., and Qvortrup, K. (2002). Sieve plugs in fenestrae of glomerular capillaries--site of the filtration barrier? Cells Tissues Organs 170, 132–138. doi:10.1159/000046186
Rubio-Gayosso, I., Platts, S. H., and Duling, B. R. (2006). Reactive oxygen species mediate modification of glycocalyx during ischemia-reperfusion injury. Am. J. Physiol. Heart Circ. Physiol. 290, H2247–H2256. doi:10.1152/ajpheart.00796.2005
Rucker, A. J., Rudemiller, N. P., and Crowley, S. D. (2018). Salt, hypertension, and immunity. Annu. Rev. Physiology 80, 283–307. doi:10.1146/annurev-physiol-021317-121134
Rueckschloss, U., Duerrschmidt, N., and Morawietz, H. (2003). NADPH oxidase in endothelial cells: impact on atherosclerosis. Antioxid. Redox Signal 5, 171–180. doi:10.1089/152308603764816532
Ruggeri Barbaro, N., Van Beusecum, J., Xiao, L., do Carmo, L., Pitzer, A., Loperena, R., et al. (2021). Sodium activates human monocytes via the NADPH oxidase and isolevuglandin formation. Cardiovasc. Res. 117, 1358–1371. doi:10.1093/cvr/cvaa207
Ryuzaki, M., Miyashita, K., Sato, M., Inoue, H., Fujii, K., Hagiwara, A., et al. (2022). Activation of the intestinal tissue renin-angiotensin system by transient sodium loading in salt-sensitive rats. J. Hypertens. 40, 33–45. doi:10.1097/HJH.0000000000002974
Sahinoz, M., Elijovich, F., Laffer, C., Pitzer, A., Ikizler, T., and Kirabo, A. (2021). Reduction in monocyte isolevuglandins associated with high interstitial sodium mirrors salt-sensitivity of blood pressure in patients with essential hypertension. FASEB J. 35. doi:10.1096/fasebj.2021.35.S1.02022
Salmito, F. T. S., de Oliveira Neves, F. M., Meneses, G. C., de Almeida Leitão, R., Martins, A. M. C., and Libório, A. B. (2015). Glycocalyx injury in adults with nephrotic syndrome: Association with endothelial function. Clin. Chim. Acta 447, 55–58. doi:10.1016/j.cca.2015.05.013
Saphire, A. C., Bobardt, M. D., Zhang, Z., David, G., and Gallay, P. A. (2001). Syndecans serve as attachment receptors for human immunodeficiency virus type 1 on macrophages. J. Virol. 75, 9187–9200. doi:10.1128/JVI.75.19.9187-9200.2001
Schierke, F., Wyrwoll, M. J., Wisdorf, M., Niedzielski, L., Maase, M., Ruck, T., et al. (2017). Nanomechanics of the endothelial glycocalyx contribute to Na+-induced vascular inflammation. Sci. Rep. 7, 46476. doi:10.1038/srep46476
Sembajwe, L. F., Ssekandi, A. M., Namaganda, A., Muwonge, H., Kasolo, J. N., Kalyesubula, R., et al. (2023). Glycocalyx–sodium interaction in vascular endothelium. Nutrients 15, 2873. doi:10.3390/nu15132873
Sieve, I., Münster-Kühnel, A. K., and Hilfiker-Kleiner, D. (2018). Regulation and function of endothelial glycocalyx layer in vascular diseases. Vasc. Pharmacol. 100, 26–33. doi:10.1016/j.vph.2017.09.002
Singh, A., Ramnath, R. D., Foster, R. R., Wylie, E. C., Fridén, V., Dasgupta, I., et al. (2013). Reactive oxygen species modulate the barrier function of the human glomerular endothelial glycocalyx. PLOS ONE 8, e55852. doi:10.1371/journal.pone.0055852
Solages, A., Vita, J. A., Thornton, D. J., Murray, J., Heeren, T., Craven, D. E., et al. (2006). Endothelial function in HIV-infected persons. Clin. Infect. Dis. 42, 1325–1332. doi:10.1086/503261
Spillings, B. L., Day, C. J., Garcia-Minambres, A., Aggarwal, A., Condon, N. D., Haselhorst, T., et al. (2022). Host glycocalyx captures HIV proximal to the cell surface via oligomannose-GlcNAc glycan-glycan interactions to support viral entry. Cell Rep. 38, 110296. doi:10.1016/j.celrep.2022.110296
Stanhewicz, A. E., Nuckols, V. R., and Pierce, G. L. (2021). Maternal microvascular dysfunction during preeclamptic pregnancy. Clin. Sci. 135, 1083–1101. doi:10.1042/CS20200894
Sulyok, E., Farkas, B., Nagy, B., Várnagy, Á., Kovács, K., and Bódis, J. (2022). Tissue sodium accumulation: pathophysiology and clinical implications. Antioxidants 11, 750. doi:10.3390/antiox11040750
Suzuki, A., Tomita, H., and Okada, H. (2022). Form follows function: the endothelial glycocalyx. Transl. Res. 247, 158–167. doi:10.1016/j.trsl.2022.03.014
Triantafyllidi, H., Fragkou, P., Birmpa, D., Benas, D., Thymis, I., Kostelli, G., et al. (2022). Endothelial glycocalyx improvement after six months and one-year of antiretroviral therapy in treatment naive patients with human immunodeficiency virus. Eur. Heart J. 43, ehac5442605. doi:10.1093/eurheartj/ehac544.2605
Tromp, J., van der Pol, A., Klip, IJ. T., de Boer, R. A., Jaarsma, T., van Gilst, W. H., et al. (2014). Fibrosis marker syndecan-1 and outcome in patients with heart failure with reduced and preserved ejection fraction. Circ. Heart Fail 7, 457–462. doi:10.1161/CIRCHEARTFAILURE.113.000846
Uchimido, R., Schmidt, E. P., and Shapiro, N. I. (2019). The glycocalyx: a novel diagnostic and therapeutic target in sepsis. Crit. Care 23, 16. doi:10.1186/s13054-018-2292-6
Ullrich, C. K., Groopman, J. E., and Ganju, R. K. (2000). HIV-1 gp120-and gp160-induced apoptosis in cultured endothelial cells is mediated by caspases. Blood 96, 1438–1442. doi:10.1182/blood.v96.4.1438
van den Berg, B. M., Vink, H., and Spaan, J. A. E. (2003). The endothelial glycocalyx protects against myocardial edema. Circ. Res. 92, 592–594. doi:10.1161/01.RES.0000065917.53950.75
van Golen, R. F., van Gulik, T. M., and Heger, M. (2012). Mechanistic overview of reactive species-induced degradation of the endothelial glycocalyx during hepatic ischemia/reperfusion injury. Free Radic. Biol. Med. 52, 1382–1402. doi:10.1016/j.freeradbiomed.2012.01.013
van Haaren, P. M. A., VanBavel, E., Vink, H., and Spaan, J. A. E. (2003). Localization of the permeability barrier to solutes in isolated arteries by confocal microscopy. Am. J. Physiol. Heart Circ. Physiol. 285, H2848–H2856. doi:10.1152/ajpheart.00117.2003
Villalba, N., Baby, S., and Yuan, S. Y. (2021). The endothelial glycocalyx as a double-edged sword in microvascular homeostasis and pathogenesis. Front. Cell Dev. Biol. 9, 711003. doi:10.3389/fcell.2021.711003
Vinaiphat, A., Pazhanchamy, K., JebaMercy, G., Ngan, S. C., Leow, M. K.-S., Ho, H. H., et al. (2023). Endothelial damage arising from high salt hypertension is elucidated by vascular bed systematic profiling. Arteriosclerosis, Thrombosis, Vasc. Biol. 43, 427–442. doi:10.1161/ATVBAHA.122.318439
Vink, H., and Duling, B. R. (1996). Identification of distinct luminal domains for macromolecules, erythrocytes, and leukocytes within mammalian capillaries. Circ. Res. 79, 581–589. doi:10.1161/01.res.79.3.581
Vink, H., and Duling, B. R. (2000). Capillary endothelial surface layer selectively reduces plasma solute distribution volume. Am. J. Physiol. Heart Circ. Physiol. 278, H285–H289. doi:10.1152/ajpheart.2000.278.1.H285
Voyvodic, P. L., Min, D., Liu, R., Williams, E., Chitalia, V., Dunn, A. K., et al. (2014). Loss of syndecan-1 induces a pro-inflammatory phenotype in endothelial cells with a dysregulated response to atheroprotective flow. J. Biol. Chem. 289, 9547–9559. doi:10.1074/jbc.M113.541573
Wang, G.-H., Ma, K.-L., Zhang, Y., Hu, Z.-B., Liu, L., Lu, J., et al. (2019). Platelet microparticles contribute to aortic vascular endothelial injury in diabetes via the mTORC1 pathway. Acta Pharmacol. Sin. 40, 468–476. doi:10.1038/s41401-018-0186-4
Weichseldorfer, M., Tagaya, Y., Reitz, M., DeVico, A. L., and Latinovic, O. S. (2022). Identifying CCR5 coreceptor populations permissive for HIV-1 entry and productive infection: implications for in vivo studies. J. Transl. Med. 20, 39. doi:10.1186/s12967-022-03243-8
Weinbaum, S., Cancel, L. M., Fu, B. M., and Tarbell, J. M. (2021). The glycocalyx and its role in vascular physiology and vascular related diseases. Cardiovasc Eng. Tech. 12, 37–71. doi:10.1007/s13239-020-00485-9
Weissgerber, T. L., Garcia-Valencia, O., Milic, N. M., Codsi, E., Cubro, H., Nath, M. C., et al. (2019). Early onset preeclampsia is associated with glycocalyx degradation and reduced microvascular perfusion. J. Am. Heart Assoc. 8, e010647. doi:10.1161/JAHA.118.010647
Widmer, R. J., and Lerman, A. (2014). Endothelial dysfunction and cardiovascular disease. Glob. Cardiol. Sci. Pract. 2014, 291–308. doi:10.5339/gcsp.2014.43
Wright, E. M., and Loo, D. D. (2000). Coupling between Na+, sugar, and water transport across the intestine. Ann. N. Y. Acad. Sci. 915, 54–66. doi:10.1111/j.1749-6632.2000.tb05223.x
Xiao, L., Patrick, D. M., Aden, L. A., and Kirabo, A. (2018). Mechanisms of isolevuglandin-protein adduct formation in inflammation and hypertension. Prostagl. Other Lipid Mediat. 139, 48–53. doi:10.1016/j.prostaglandins.2018.09.008
Yamaoka-Tojo, M. (2020a). Endothelial glycocalyx damage as a systemic inflammatory microvascular endotheliopathy in COVID-19. Biomed. J. 43, 399–413. doi:10.1016/j.bj.2020.08.007
Yamaoka-Tojo, M. (2020b). Vascular endothelial glycocalyx as a mechanism of vascular endothelial dysfunction and atherosclerosis. World J. Cardiovasc. Dis. 10, 731–749. doi:10.4236/wjcd.2020.1010070
Yang, B., Akhter, S., Chaudhuri, A., and Kanmogne, G. D. (2009). HIV-1 gp120 induces cytokine expression, leukocyte adhesion, and transmigration across the blood–brain barrier: modulatory effects of STAT1 signaling. Microvasc. Res. 77, 212–219. doi:10.1016/j.mvr.2008.11.003
Keywords: salt, sodium, hypertension, salt-sensitive hypertension, HIV, glycocalyx, endothelial glycocalyx
Citation: Masenga SK, Liweleya S and Kirabo A (2024) High salt intake and HIV infection on endothelial glycocalyx shedding in salt-sensitive hypertension. Front. Cell Dev. Biol. 12:1395885. doi: 10.3389/fcell.2024.1395885
Received: 04 March 2024; Accepted: 28 June 2024;
Published: 16 July 2024.
Edited by:
Ye Zeng, Sichuan University, ChinaReviewed by:
Solomon Arko Mensah, Worcester Polytechnic Institute, United StatesDominik Skiba, University of Glasgow, United Kingdom
Copyright © 2024 Masenga, Liweleya and Kirabo. This is an open-access article distributed under the terms of the Creative Commons Attribution License (CC BY). The use, distribution or reproduction in other forums is permitted, provided the original author(s) and the copyright owner(s) are credited and that the original publication in this journal is cited, in accordance with accepted academic practice. No use, distribution or reproduction is permitted which does not comply with these terms.
*Correspondence: Sepiso K. Masenga, c2VwaXNvbWFzZW5nYUBnbWFpbC5jb20=; Annet Kirabo, YW5uZXQua2lyYWJvQHZ1bWMub3Jn