- Department of Developmental Biology, Erasmus University Medical Center, Rotterdam, Netherlands
Mammalian stem cell-based embryo models have emerged as innovative tools for investigating early embryogenesis in both mice and primates. They not only reduce the need for sacrificing mice but also overcome ethical limitations associated with human embryo research. Furthermore, they provide a platform to address scientific questions that are otherwise challenging to explore in vivo. The usefulness of a stem cell-based embryo model depends on its fidelity in replicating development, efficiency and reproducibility; all essential for addressing biological queries in a quantitative manner, enabling statistical analysis. Achieving such fidelity and efficiency requires robust systems that demand extensive optimization efforts. A profound understanding of pre- and post-implantation development, cellular plasticity, lineage specification, and existing models is imperative for making informed decisions in constructing these models. This review aims to highlight essential differences in embryo development and stem cell biology between mice and humans, assess how these variances influence the formation of partially and fully integrated stem cell models, and identify critical challenges in the field.
Introduction
Headlines featuring “Synthetic Embryo Models” (SEMs) or “Artificial Embryos” are sensational, but can be misleading and potentially cause unnecessary concern among the general public. These terms serve attempts to describe stem cell-based embryo models, which are further categorized into non-integrated and integrated stem cell models (Rossant and Tam, 2021; Hyun et al., 2020). The non-integrated models focus on specific aspects of embryonic development; while the integrated models, such as blastoids and ETX embryoids, simulate the progressive development of the entire mammalian conceptus, including its extra-embryonic tissues. The forthcoming review further classifies integrated stem cell-based embryo models into partially and fully integrated types. Fully integrated models encompass all extra-embryonic lineages, whereas partially integrated models represent only a subset of these extra-embryonic lineages. Integrated stem cell-based embryo models can take shape through an assembly approach, involving the aggregation of various appropriate early lineage-specific stem cells that are known to mutually influence each other’s development. Alternatively, they can also be constructed through an inductive approach, where the formation of the stem cell-based embryo model depends on elaborate cell culture media that will chemically dictate the fate of the used cells. These models are created from biological materials and are thus far from being synthetic or artificial. The primary goal of designing and using stem cell-based embryo models is not to generate human or animal beings from in vitro entities. They rather offer a versatile approach to study early mammalian embryonic development and provide valuable insights into cellular processes and molecular mechanisms, all without the need for real human embryos or sacrificing pregnant lab mice. Their versatility enables researchers to assess specific aspects of mammalian embryonic development, making them effective tools for scientific research and advancements in animal and human reproductive medicine. Furthermore, for drug testing and screening, these models provide a controlled environment enabling the assessment of drug efficacy, in supporting complicated pregnancies, as well as evaluating potential embryo toxicity during pregnancy. In the upcoming sections, this review comprehensively addresses the significant differences in embryo development and stem cell biology between mice and humans, highlighting how these variances influence the production methods of partially and fully integrated stem cell-based embryo models tailored to each species. Moreover, it critically examines recent developments in both human and mouse partially and fully integrated stem cell models, offering insights into the notable challenges encountered within this field. Additionally, the review explores the potential of non-human primate embryos and non-human primate stem cell-based embryo models in advancing knowledge of primate embryogenesis, particularly in contexts where ethical limitations surrounding human embryos and human stem cell-based embryo models restrict research.
Pre- and post-implantation development in mice versus humans
Preimplantation development, spanning fertilization to implantation, is a crucial phase in early mammalian embryogenesis, marked by several key milestones. These key milestones include zygotic genome activation (ZGA), the transition to multicellularity through slow cell division, compaction, polarization, and subsequent blastocyst formation. Within the blastocyst, cells differentiate into the inner cell mass (ICM) and trophectoderm (TE), with further specification of the primitive endoderm (PrE; hypoblast) and epiblast within the ICM. Preimplantation development in mice spans 5 days, whereas in humans it generally takes 6–7 days. While mouse and human preimplantation development appears morphologically similar, differences emerge in cell fate specification, characterized by variations in the expression of lineage specific transcription factors and the activity of signaling pathways. After implantation, mouse and primate embryos clearly exhibit significant morphological and molecular differences. Lab mice have a gestation period of 19–20 days. In contrast, human gestation typically spans approximately 270 days, whereas in cynomolgus and rhesus monkeys this takes around 160 days each (Nakamura et al., 2021) (Figure 1A).
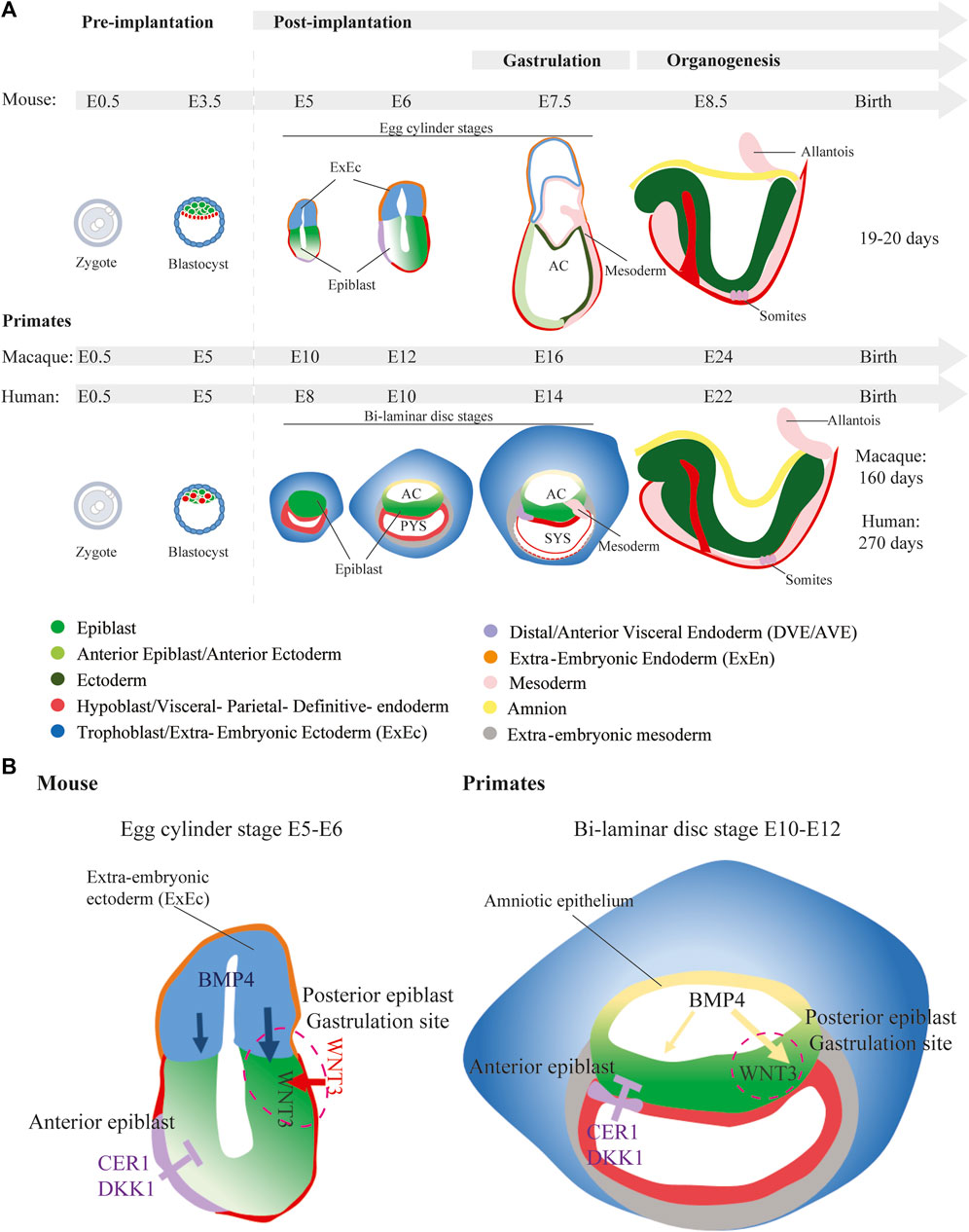
Figure 1. Embryonic development in mice versus primates. (A) Embryonic development of mice and primates. Mice go through egg cylinder stages of development, whereas primates form a bi-laminar disc. E, Embryonic days; AC, Amniotic Coelom; PYS, Primary Yolk Sac; SYC, Secondary Yolk Sac (B) Signaling mechanism inducing gastrulation in mice and in primates. In mice the source of BMP4 is the ExEc, whereas in primates the amnion produces BMP4.
Following implantation, cell proliferation markedly increases in mouse embryos. This is accompanied by epithelialization of both the epiblast and the polar TE, leading to the formation of the characteristic cylindrical, elongated embryo, commonly referred to as the egg cylinder (Bedzhov et al., 2014a). The extra-embryonic ectoderm (ExEc), originating from the polar TE, plays a critical role in BMP4 production (Figure 1B). BMP4 is essential for establishing an anterior-posterior axis and initiating mesoderm formation in a NODAL dependent manner on the posterior side of the epiblast during gastrulation (Winnier et al., 1995; Lawson et al., 1999). After exposure to BMP4, WNT3 is first produced in the posterior visceral endoderm (VE) (Liu et al., 1999) before also appearing in the posterior epiblast (Rivera-Perez and Magnuson, 2005) (Figure 1B). Simultaneously, VE cells located at the anterior side of the egg cylinder are replaced by the anterior VE (AVE) cells which are initially formed at the distal tip of the egg cylinder (Thomas and Beddington, 1996; Thomas et al., 1998). The AVE, marked by transcription factors OTX2, HHEX, HESX1, FOXA2, and LHX1, acts as a protective barrier, preventing adjacent epiblast cells from responding to posteriorizing signals. The AVE does so by producing Wnt, Bmp, and Nodal antagonists like DKK1, CER1, and LEFTY1, thereby inhibiting ectopic primitive streak formation on the anterior side (Acampora et al., 1995; Belo et al., 1997; Thomas et al., 1998; Perea-Gomez et al., 1999; Kimura et al., 2000; Perea-Gomez et al., 2002; Yamamoto et al., 2004; Kimura-Yoshida et al., 2005) (Figure 1B).
In contrast, epiblast and TE cell proliferation in primate embryos, exhibits different morphological characteristics. The TE invades the endometrium, while the epiblast expands to form a flat sheet of cells, resulting in a flattened embryo known as an embryonic disc (Nakamura et al., 2016; Rossant and Tam, 2017; O'Rahilly and Muller, 2010). This early structure consists of two layers: the epiblast and the hypoblast. As development progresses, an amniotic cavity emerges following the separation of the amniotic epithelium from the epiblast layer, while the primary yolk sac is formed by the hypoblast layer. This primate-specific morphogenesis also presents already an extraembryonic mesoderm (ExEM) lineage in the pre-gastrulation embryo whereas in mice the ExEM (including the allantois, and mesoderm component of amnion and yolk sac) develops during gastrulation. However, the origin of the primate ExEM is unclear; it could have an epiblast origin as in mice, but could also be derived from the TE or the hypoblast (reviewed in (Pham et al., 2022; Rossant and Tam, 2022)). Also, in contrast to mice, where the ExEc serves as the signaling source for inducing gastrulation; in primates, BMP4 originates from the amnion (Figure 1B). Staining and profiling of in vivo non-human primate embryos indeed revealed the accumulation of BMP4 in the amnion of pre-gastrulating embryos (Sasaki et al., 2016; Bergmann et al., 2022). Similarly to mice, WNT3/WNT3 is detected in the non-human primate posterior epiblast (Niu et al., 2019; Bergmann et al., 2022). In cynomolgus monkeys, OTX2, along Wnt and Nodal inhibitors DKK1 and CER1, are detected in the AVE (Sasaki et al., 2016; Ma et al., 2019). In the human peri-implantation embryo, and similar to mice, the putative AVE exhibits an accumulation of CER1 and LEFTY1. At the gene expression level, the human AVE also presents an accumulation of LHX1, HHEX and DKK1-transcripts (Mole et al., 2021). In vitro experiments using human pluripotent stem cells (PSCs) have shown that the amnion is the source of BMP4 in primates and that BMP4 induces gastrulation in a WNT-dependent manner (Shao et al., 2017a; Zheng et al., 2019; Yang et al., 2021). It seems therefore that the formation of the primitive steak in mice and primates depends on the same signaling pathways.
These variations in development underscore substantial differences in early post-implantation developmental processes between mice and primates. Thus, making direct assumptions about human embryogenesis is challenging if based solely on the knowledge obtained from mouse development. Ethical restrictions will continue to limit the study of human embryogenesis. Using either non-human primate embryos or primate stem cell-derived embryo models holds the potential to provide valuable insights into the intricacies of human early embryonic development, effectively bridging the gap between mouse and human.
Lineage emergence during preimplantation development
During the preimplantation phase, the TE, the epiblast, and the PrE are the first three lineages to emerge. The TE plays a role in forming the embryonic part of the placenta, while the epiblast gives rise to the embryo proper and the mesoderm of the allantois, amnion, and yolk sac. The PrE is vital for embryo patterning and contributes to the development of the yolk sac.
In mice, the major ZGA at the 2-cell stage (Schultz, 1993) marks the initiation of the expression of genes encoding various lineage specific transcription factors (TFs). The first lineage commitment in mice occurs with the specification of the TE from the outer cells of the morula stage embryo. This commitment is highly dependent on the expression of Cdx2 initiated between the 8- and 16-cell stage (Niwa et al., 2005; Strumpf et al., 2005; Dietrich and Hiiragi, 2007; Ralston and Rossant, 2008; Jedrusik et al., 2010; Ralston et al., 2010) and on the inhibition of the Hippo signaling pathway (Yagi et al., 2007; Nishioka et al., 2008; Nishioka et al., 2009). Hippo-pathway inhibition in the TE cells results in the release of cytoplasmic sequestration of TEAD co-activator YAP1, enabling TE-specific genes like Cdx2 and Gata3 to be transcribed (Yagi et al., 2007; Nishioka et al., 2008). In comparison to mice, the major ZGA in humans occurs at the 4 to 8-cell stage (Braude et al., 1988; Blakeley et al., 2015). Human TE specification seems to be more dependent on GATA3 than on CDX2. GATA3 can be detected in all cells of the morula stage human embryo, but subsequently becomes restricted to the TE (Petropoulos et al., 2016; Gerri et al., 2020), whereas CDX2 only appears well beyond the formation of the human blastocyst (Niakan and Eggan, 2013). Although the TE specifiers TEAD1, YAP and GATA3 predominantly co-localize in polarized outer cells of human 16-cell morulae, their presence is also detected in certain cells of compacting embryos before the establishment of cell polarity (Regin et al., 2023). This observation lends support to the idea that the initiation of TE formation in humans might occur independently of cell polarity. The observation that a genomic deletion of TEAD4 does not affect the GATA3 profile in blastocyst-stage human embryos, unlike CDX2, supports that notion (Stamatiadis et al., 2022). Cell polarity therefore influences the TE lineage formation in humans, but its importance in TE specification appears to be less evident than in mice (Gerri et al., 2020; Zhu et al., 2021).
The second cell fate decision in preimplantation embryos involves the specification of the PrE and the epiblast in ICM cells. The murine ICM is composed of a random mixture of cells in a “salt and pepper” pattern displaying varying levels of GATA6 or NANOG (Chazaud et al., 2006). Ultimately FGF produced by epiblast-biased cells expressing Nanog, support further specification of murine PrE-biased cells (Silva et al., 2009; Messerschmidt and Kemler, 2010; Yamanaka et al., 2010; Frankenberg et al., 2011). Without sufficient FGF signaling, PrE-biased cells revert back to the epiblast fate. This mechanism most likely ensures a perfect balance between the number of PrE and epiblast cells. This same second cell fate decision in human embryos is less well understood, and differs from its murine counterpart as FGF signaling does not seem to be as essential for human PrE development (Kuijk et al., 2012; Roode et al., 2012). Additionally, detection of TFs POU5F1, SOX2 and NANOG generally associated with the pluripotent epiblast, also differs between mouse and human preimplantation embryos. In mouse, SOX2 is the first pluripotency TF to selectively mark the epiblast (Frum and Ralston, 2015), whereas in human this role is reserved to NANOG (Cauffman et al., 2009). Variations can also be observed in the KLF family members expression profiles. As such, while Klf2 expression delineates the mouse epiblast, KLF17 expression performs a similar role in the human epiblast (Blakeley et al., 2015; Lea et al., 2021).
Cellular plasticity in preimplantation development
In the intricate nomenclature hierarchy of cellular potential, totipotent cells stand at the top. Totipotency can be interpreted as the capacity of a single diploid cell to independently give rise to an entire organism. Alternatively, a more lenient interpretation considers totipotency as the capacity of a cell to differentiate into all types of lineages, in amniotes including all embryonic and extra-embryonic lineages. To delineate these distinctions, the term “totipotency” has been suggested for the former, emphasizing organism-forming ability, while the term “plenipotency” has been proposed for the latter (Condic, 2014). Pluripotent cells, on the other hand, while highly versatile, are confined to producing derivatives specific to the epiblast (for a discussion of used functional assays for pluripotency and their limitations, with mouse and human cells, see (De Los Angeles et al., 2015)). Despite their adaptability, they lack the organizational finesse required to forming an integrated body plan and can generate neither TE nor PrE derivatives. In the strict sense of the word, a fertilized oocyte is classified as totipotent. However, due to ethical considerations, the developmental potential of individual human blastomeres to give rise to viable offspring has never been assessed. In mice, blastomeres of 2-cell stage embryos can be categorized as totipotent because a single blastomere from the 2-cell stage embryo, albeit with a lower potency, is sufficiently competent to produce offspring (Tarkowski, 1959; Tsunoda and McLaren, 1983; Lawitts and Graves, 1988; Papaioannou et al., 1989; Papaioannou and Ebert, 1995; Wang et al., 1997; Sotomaru et al., 1998; Morris et al., 2012b; Casser et al., 2017; Rahbaran et al., 2022). Four-cell stage embryos are generally assumed not be totipotent anymore (Tarkowski and Wroblewska, 1967; Rossant, 1976; Morris et al., 2012b) although at least one report describes offspring from a single blastomere removed from 4-cell stage embryos (Maemura et al., 2021). Whereas a critical aspect of this potency loss is due to an insufficient number of cells forming the epiblast (Rossant, 1976; Morris et al., 2012b), resulting in smaller sized offspring (Tsunoda and McLaren, 1983; Morris et al., 2012b; Casser et al., 2017), blastomeres from 2- and 4-cell stage mouse embryos have also been shown to possess differing developmental potentials (Piotrowska-Nitsche et al., 2005; Torres-Padilla et al., 2007; Morris et al., 2012b). This implies that lineage specification, involving the initial commitment of cells to specific fates starts already at the 2-cell stage. Nevertheless, blastomeres from mouse cleavage stage embryos retain the plasticity to contribute to all lineages and are not yet committed to one specific fate. Even cells within the ICM of early blastocyst-stage mouse embryos (E3) retain plenipotency, capable of producing both the trophectoderm (TE) and the primitive endoderm (PrE) in addition to the epiblast (Handyside, 1978; Hogan and Tilly, 1978; Spindle, 1978; Rossant and Lis, 1979; Suwinska et al., 2008; Wigger et al., 2017). However, TE cells lose the competency to produce the ICM once a blastocyst is formed (Suwinska et al., 2008). In mice, the ICM loses its potency to form the TE after the second cell fate decision, with the specification of the PrE and the pluripotent epiblast (Posfai et al., 2017; Wigger et al., 2017). Blocking this second cell fate decision using small molecules specifically targeting FGF/ERK signaling, preserves the potency of the murine ICM to form TE (Wigger et al., 2017). Plenipotency during human embryonic development is maintained longer than observed in mice. Both the TE and ICM of the human early blastocyst-stage embryo retain the potency to form all lineages, as demonstrated in an embryo dissociation study (De Paepe et al., 2013). Additionally, the human naïve epiblast maintains its potential to form TE and PrE (Guo et al., 2021). Pseudotime analysis of single cell transcriptomics in mouse embryos confirms two sequential lineage determination events, with TE-specific cells emerging at the morula stage, and PrE-specific cells emerging in the ICM of the blastocyst stage embryo (Meistermann et al., 2021). A similar analysis in human embryos also identifies two lineage determination events, but both occur sequentially after the blastocyst is formed (Meistermann et al., 2021), albeit that an earlier study suggested that the first and second lineage segregation occur simultaneously (Petropoulos et al., 2016). It seems, thus, that the morphological appearance of the blastocyst in humans does not fully align with the molecular divergence.
Murine early lineage stem cells
The first murine embryonic stem (ES) cells were isolated in 1981 (Evans and Kaufman, 1981; Martin, 1981). These cells are derived from the epiblast of blastocyst-stage embryos and have the capacity to contribute to all embryonic lineages when injected into murine preimplantation embryos, but these cells rarely contribute to extra-embryonic tissues (Bradley et al., 1984; Beddington and Robertson, 1989). The unrestricted potential to produce all embryonic lineages is the defining trait of pluripotency, a trait which is maintained after implantation of the blastocyst in the uterus up until the epiblast starts gastrulation. Whereas, initially PSCs were derived from the epiblast of preimplantation embryos (Evans and Kaufman, 1981; Martin, 1981), they could also be derived from the post-implantation epiblast (Brons et al., 2007; Tesar et al., 2007). The epiblast-derived PSCs of the pre- and post-implantation embryo were labeled as naïve and primed PSCs (Figure 2A), respectively (Nichols and Smith, 2009). Primed PSCs are also frequently named epiblast stem cells (EpiSC). Naïve ES cells grow as small, compact, domed colonies; while primed ES cells grow as flat epithelialized colonies. Whereas the primed PSCs can contribute to the epiblast when injected into a post-implantation embryo, they are developmentally too advanced to efficiently contribute to offspring when injected into recipient preimplantation embryos (Brons et al., 2007; Tesar et al., 2007; Huang et al., 2012). This makes the generation of chimeric mice using primed PSCs technically challenging.
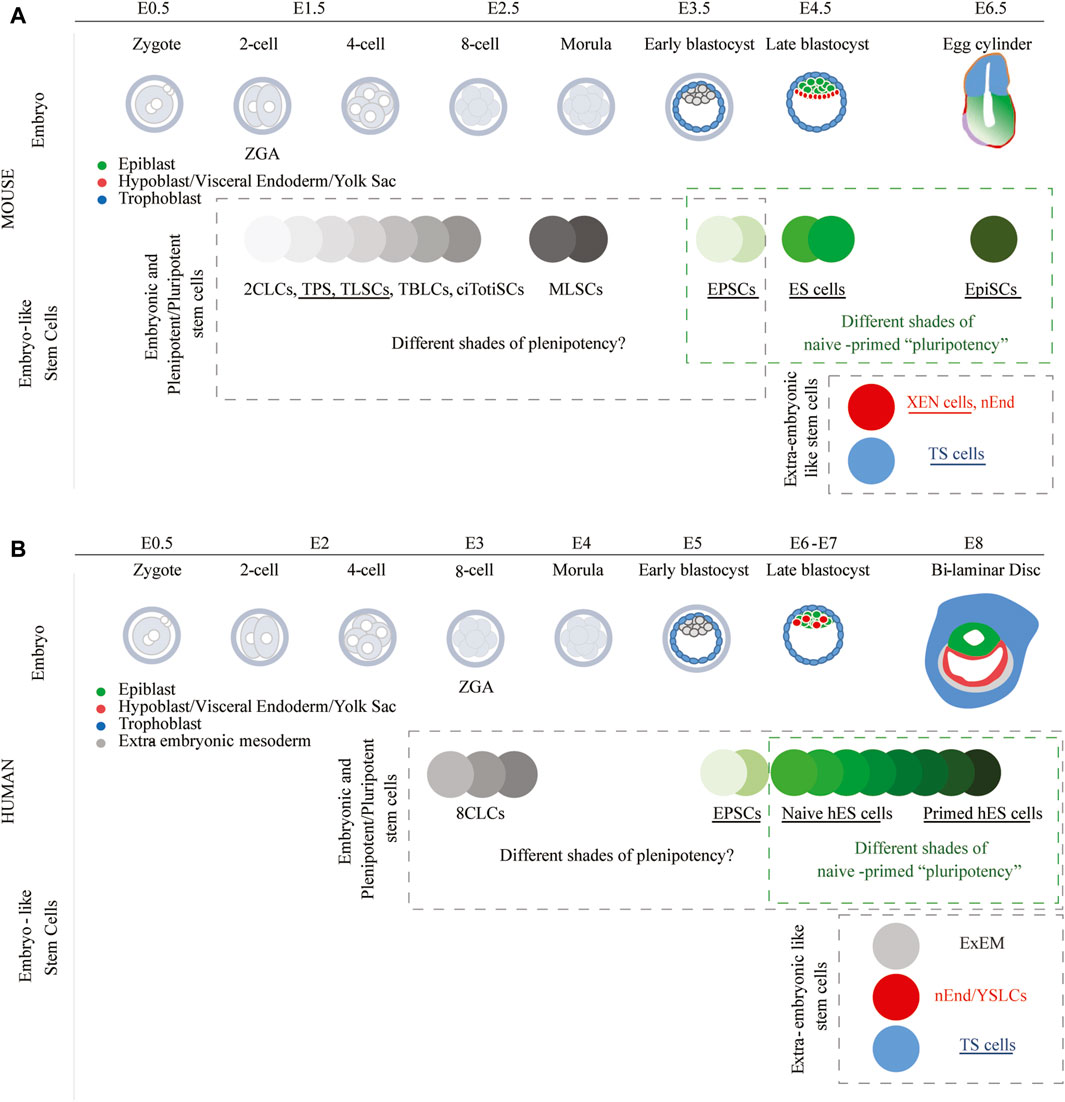
Figure 2. Stem cells resembling early embryonic lineages in mice and humans. Many of these stem cell types have been derived from existing ES cells by modifying culture conditions. Following the implementation of these cell culture conditions, certain studies have also shown that specific cell types could be directly derived from the embryo (underlined stem cell types). (A) Since 2CLCs, TPS cells, TLSCs, TBLCs, ciTotiSCs, MLSCs, and EPSCs can form both embryonic and extra-embryonic murine lineages, these cell types can be classified as plenipotent. On the other hand, EPSCs could also be classified as pluripotent as they have limited potential to form the mouse trophoblast. (B) Whereas naïve and primed human ES cells are classified as pluripotent, they could also, together with 8CLCs and EPSCs, be classified as plenipotent, as all can form the embryonic and extra-embryonic cell lineages.
Furthermore, also mouse trophoblast stem (TS) cells (Tanaka et al., 1998; Kubaczka et al., 2014; Ohinata and Tsukiyama, 2014) and mouse eXtra-embryonic ENdoderm (XEN) stem cells (Kunath et al., 2005; Zhong et al., 2018) have subsequently been derived from the mouse TE and PrE, respectively (Figure 2A). Following injection into preimplantation embryos, TS cells and XEN cells contribute exclusively and respectively to the trophoblast and extra-embryonic endoderm lineages. Whereas ES cells can in adapted culture conditions transdifferentiate to trophoblast (Hayashi et al., 2010) as well as extra-embryonic endoderm (Cho et al., 2012; Niakan et al., 2013; Anderson et al., 2017), the overexpression of lineage-specific transcription factor (TF) (trans)genes greatly facilitates this conversion. As such, induced expression of trophoblast TF (trans)genes (e.g., Cdx2 and Gata3) supports a transformation towards TS cells (Niwa et al., 2000; Niwa et al., 2005; Kuckenberg et al., 2010; Ralston et al., 2010; Cambuli et al., 2014; Wei et al., 2016; Kaiser et al., 2020); whereas the induced expression of PrE TF (trans)genes (e.g., Gata6 and Sox17) supports the formation of mouse XEN cells (Fujikura et al., 2002; Shimoda et al., 2007; Shimosato et al., 2007; Qu et al., 2008; Niakan et al., 2010; McDonald et al., 2014; Wamaitha et al., 2015; Wei et al., 2016). Nevertheless, epigenetic barriers, such as methylation of the Elf5 promoter in ES cells, hamper generally a complete transdifferentiation of ES cells into TS cells (Ng et al., 2008; Hemberger, 2010; Cambuli et al., 2014).
Scientists have therefore been captivated by creating a plenipotent stem cell that could contribute to the embryonic and extra-embryonic tissues. Three types of plenipotent stem cells have been described, i.e., 2-cell like stem cells (2CLCs), expanded/extended potential stem cells (EPSCs) and morula like stem cells (MLSCs). The 2CLCs were discovered as a small (less than 1%) transient population in ES cell cultures (Macfarlan et al., 2012). The 2CLCs present characteristics of blastomeres of the 2-cell stage embryo when major ZGA occurs, and their population size can be increased by modulating culture media and/or overexpression of specific (trans)genes (Macfarlan et al., 2011; Hisada et al., 2012; Macfarlan et al., 2012; Maksakova et al., 2013; Dan et al., 2014; Lu et al., 2014; Hayashi et al., 2016; Choi et al., 2017; Rodriguez-Terrones et al., 2018; De Iaco et al., 2019; Yan et al., 2019; Hu et al., 2020; Rodriguez-Terrones et al., 2020; Huang et al., 2021; Olbrich et al., 2021; Shen et al., 2021; Wang et al., 2021; Xu et al., 2022; Yang et al., 2022; Hu et al., 2023; Meharwade et al., 2023). Cells presenting more or less characteristics of the 2-cell stage embryo have been labeled in the literature as 2-cell-like cells (2CLCs), but also as totipotent blastomere-like cells (TBLCs), totipotent-like stem (TPS) cells, totipotent-like stem cells (TLSCs) and chemically induced totipotent stem cells (ciTotiSCs). These cell types seem to be more potent than ES cells as they can contribute to all extra-embryonic lineages when injected into preimplantation embryos (Macfarlan et al., 2012; Choi et al., 2017; Shen et al., 2021; Wang et al., 2021; Xu et al., 2022; Yang et al., 2022; Meharwade et al., 2023). In this manuscript, the cell types presenting some characteristics of the 2-cell stage are all compiled under 2CLC terminology (Figure 2A). 2CLCs should however not be confounded with EPSCs. Murine EPSCs which can be obtained by modifying culture media composition, have also the potential to form all extra-embryonic lineages (Yang et al., 2017a; Yang et al., 2017b; Yang et al., 2019). A thorough assessment of EPSCs, however, labels EPSCs to be more similar to a blastocyst than a pre-blastocyst stage embryo and their capacity to differentiate into trophoblast has been questioned (Stirparo et al., 2018; Posfai et al., 2021). MLSCs, which would relate to the morula-stage embryo, have recently also been described (Li et al., 2023a). Whereas the authors reported that MLSCs are plenipotent and can form the extra-embryonic lineages, the formation of the trophoblast seemed difficult as observed during the formation of blastoids.
An analytical exploration that highlights the commonalities among embryos at different developmental stages and certain in vitro cell types recognized for their enhanced developmental potential (2CLCs, TBLCs, TPS, and EPSCs) reveals that the analyzed cell types all display a predominant association with morula and pre- and post-implantation epiblast stages (Xu et al., 2022). Are all these alleged murine ‘plenipotent’ cell types fundamentally dissimilar, or are they merely a product of in vitro adaptation to distinct culture conditions or the result of overexpressed (trans)genes? A larger integration of diverse datasets obtained from single-cell RNA sequencing across various stages of preimplantation embryos, including ES cells, EpiSCs, various cell types clustered under 2CLC type in this manuscript, as well as EPSCs and MLSCs, would be extremely welcome to correlate them better with a specific developmental stage (Figure 2A).
Human early lineage stem cells
Human ES (hES) cells were derived over a decade ago, following the establishment of germline-competent mouse ES cell lines (Thomson et al., 1998). Unlike mouse ES cells, which were isolated and maintained in cell culture in their naive state, conventional hES cells resemble the pre-gastrulation epiblast and are thus in the primed pluripotent state (Nakamura et al., 2016). It has been suggested that the naïve ES cells from mice rely on signaling pathways governing murine diapause (Nichols et al., 2001), a reversible state of suspended embryonic development that allows blastocyst-stage embryos to delay implantation until favorable environmental conditions arise. In contrast, human development is continuous, and lacks a natural mechanism like diapause to halt blastocyst development without compromising viability.
The delayed onset of ZGA in humans at the 8-cell stage (Braude et al., 1988; Blakeley et al., 2015), as opposed to the 2-cell stage in mice (Schultz, 1993); is expected to influence lineage specification and modify the signaling pathways governing this process (Rossant and Tam, 2017). Although several culture media have been described to support the culture of naïve human PSCs (Gafni et al., 2013; Takashima et al., 2014; Theunissen et al., 2014; Duggal et al., 2015; Qin et al., 2016; Zimmerlin et al., 2016; Guo et al., 2017; Bredenkamp et al., 2019; Bayerl et al., 2021; Yu et al., 2022; Ai et al., 2023), the precise nature of true naïve pluripotency remains elusive. All human naïve pluripotency cell culture media likely represent different shades of pluripotency between true naïve and primed pluripotency (Figure 2B). To date, 5iLAF (Theunissen et al., 2014), t2iLGö (Takashima et al., 2014; Guo et al., 2017) and PXGL (Bredenkamp et al., 2019) are considered the naive and complex culture systems that produce naive hES cells representing the pre-implantation epiblast (Stirparo et al., 2018). Naive hES cell cultures exhibit considerable heterogeneity, presenting 8-cell-like cells (8CLCs) with features of ZGA (Wang et al., 2018; Mazid et al., 2022; Moya-Jódar et al., 2023; Taubenschmid-Stowers et al., 2022; Yoshihara et al., 2022; Yu et al., 2022) as well as TE- and PrE-like cells (Linneberg-Agerholm et al., 2019; Dong et al., 2020; Moya-Jódar et al., 2023). The human preimplantation epiblast retains the plasticity to form trophoblast and PrE after the blastocyst has been formed (De Paepe et al., 2013; Guo et al., 2021) which is likely associated with the naïve state of the epiblast. Since the human preimplantation epiblast maintains naïve characteristics at least until E7 (Messmer et al., 2019), it could be assumed that this plasticity extends at least until that day. Reflecting this plasticity, naïve hES cells besides having the capacity to form EPSCs (Yang et al., 2017b; Gao et al., 2019; Liu et al., 2021a) can also be easily directed towards the extra-embryonic lineages including TS cells (Xu et al., 2002; Cinkornpumin et al., 2020; Dong et al., 2020; Io et al., 2021; Mischler et al., 2021; Wei et al., 2021; Viukov et al., 2022), hypoblast-like stem cells (Linneberg-Agerholm et al., 2019; Mackinlay et al., 2021) and ExEM (Pham et al., 2022) (Figure 2B).
Human extra-embryonic stem cell lines, however, are not as well characterized as their murine counterparts. Human TS cells can be directly derived from embryos (Okae et al., 2018) or transdifferentiated from hES cells (Xu et al., 2002; Cinkornpumin et al., 2020; Dong et al., 2020; Io et al., 2021; Mischler et al., 2021; Wei et al., 2021; Viukov et al., 2022). Human hypoblast-like stem cells, named naïve endoderm (nEnd) and yolk like stem cells (YLSCs), on the other hand, have been exclusively obtained following differentiation of hES cells (Linneberg-Agerholm et al., 2019; Mackinlay et al., 2021). Several studies have suggested that differentiation towards the early extra-embryonic lineages (TE and PrE) is only possible when using naïve hES cells, but impossible when using primed hES cells (Linneberg-Agerholm et al., 2019; Dong et al., 2020; Guo et al., 2021; Io et al., 2021). However, other studies claim that early extra-embryonic lineages can be formed from primed hES cells (Xu et al., 2002; Wei et al., 2021; Viukov et al., 2022). Primed hES cells, representing the post-implantation epiblast, are very responsive to somatic differentiation cues (e.g., BMP4, ACTIVIN) unlike naïve hES cells that do not respond well to somatic differentiation cues and prefer to be re-primed before differentiation (Guo et al., 2017; Liu et al., 2017; Rostovskaya et al., 2019). It is worth considering whether primed hES cells retain the capacity to generate early lineages while also displaying responsiveness in forming somatic cell derivatives, which could potentially result in the overgrowth of the initially formed early lineage cell types. Unlike murine extra-embryonic stem cells, human extra-embryonic stem cells require intricate culture media for maintenance. The true nature of human extra-embryonic stem cell lines is still unclear. Although human TS cells serve as valuable models for investigating trophoblast differentiation, they are generally regarded as having closer associations with post-implantation cytotrophoblasts than with the TE of the blastocyst (Okae et al., 2018; Castel et al., 2020; Mischler et al., 2021). Thus far, no PrE stem cell line has been directly established from human embryos. A better characterization of how the PrE emerges in the human embryo may provide valuable insights on how to generate a hypoblast-like stem cell line directly derived from embryos. Nevertheless, it seems that naïve hES cells are sufficiently plenipotent to form the early lineages emerging during development. Culture procedures for growing naïve hES cells, however, still require further refinement.
The blastoid or pre-implantation integrated stem cell-based embryo model
The blastoid, which mimics the blastocyst stage, has been successfully generated in mouse (Yang et al., 2017b; Rivron et al., 2018; Li et al., 2019; Sozen et al., 2019; Li and Izpisua Belmonte, 2021; Vrij et al., 2022; Xu et al., 2022; Yang et al., 2022; Li et al., 2023a) by assembling one or two types of stem cells in vitro (Figure 3A). While there have been reports of mouse iBlastoids forming spontaneously during the reprogramming of primed to naïve states (Kime et al., 2019), this strategy does not allow robust and high-throughput blastoid production, and their trophectoderm is not well defined. The initial study detailing the formation of murine blastoids used ES cells in combination with TS cells (Rivron et al., 2018). A notable challenge encountered was the underrepresentation of the PrE in these structures as the used ES cells did not naturally form PrE-like cells. To address this issue, the protocol was chemically modified to stimulate ES cell lines to differentiate into PrE, contributing to the formation of blastoids better featuring PrE (Vrij et al., 2022). In alternative approaches, researchers have sought to leverage the increased developmental potential of EPSCs, 2CLCs and MLSCs, to generate blastoids (Yang et al., 2017b; Li et al., 2019; Sozen et al., 2019; Li and Izpisua Belmonte, 2021; Xu et al., 2022; Yang et al., 2022; Li et al., 2023a). Protocols were devised to exclusively use EPSCs (Li et al., 2019; Li and Izpisua Belmonte, 2021) or combine EPSCs with TS cells (Sozen et al., 2019). While blastoids formed using EPSCs morphologically resembled blastocysts and exhibited the correct distribution of lineage markers across all three early lineages of the blastocyst, including the PrE. Detailed single-cell transcriptomic analyses revealed inadequate specification of the TE alongside the presence of undefined intermediate, mesoderm-like populations when EPSCs were part of the aggregation protocol (Posfai et al., 2021). Three additional studies reporting on the formation of stem cells with plenipotent traits, namely, TLSCs (2CLC-like) (Yang et al., 2022), TPS cells (2CLC-like) (Xu et al., 2022) and MLSCs (Li et al., 2023a), demonstrated the competency of these cells to form blastoids. While these blastoids appeared to exhibit the early three lineages (TE, PrE, and epiblast) similar to blastocysts, a comprehensive analysis was not the main focus of these studies. However, an under-representation of TE cells was observed in blastoids generated from TPS cells (Xu et al., 2022) and MLSCs (Li et al., 2023a).
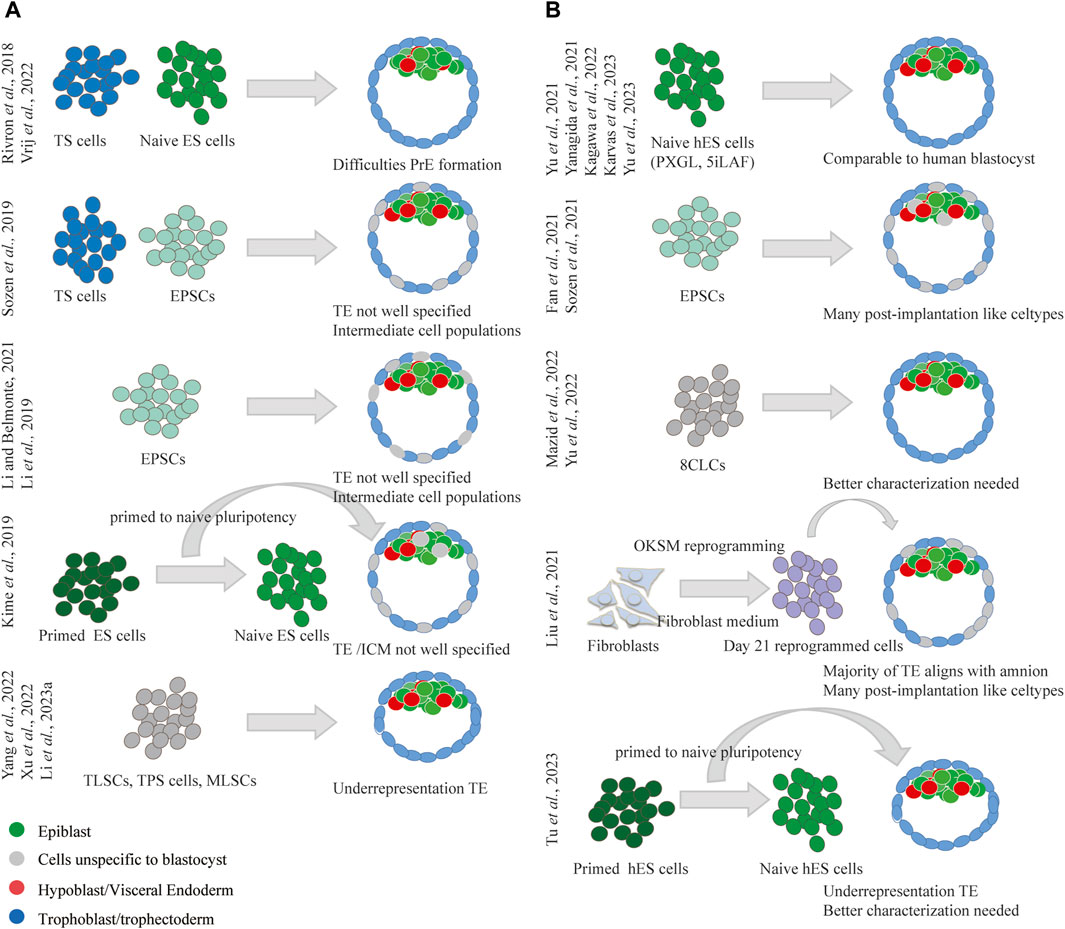
Figure 3. Summary of strategies used for blastoid formation in mouse and human. (A) Diverse strategies have been employed to produce mouse blastoids, all struggle with the formation of the extra-embryonic lineages. (B) Diverse strategies have been employed to produce human blastoids, naïve human ES cells as starting cell type showed the best capacity to produce blastocyst alike blastoids.
Mouse blastoids thus present difficulties in forming extra-embryonic lineages. While ES cells face challenges with PrE formation; EPSCs, 2CLCs, and MLSCs struggle with TE specification. This also partially elucidates the limited capacity of mouse blastoids to develop further in vitro and in vivo. As authentic blastocysts also encounter hurdles in transitioning to post-implantation development in vitro, there is a pressing need for the enhancement of culture platforms supporting the post-implantation development of blastocysts to more accurately assess the developmental potential of blastoids.
Various approaches have been used in the development of human blastoids, employing different cell types as starting materials (Figure 3B). Human blastoids have been successfully generated using hES cells in both the naive (Yanagida et al., 2021; Yu et al., 2021; Kagawa et al., 2022; Karvas et al., 2023; Yu et al., 2023) and primed-to-naive intermediate pluripotency states (Tu et al., 2023), as well as EPSCs (Fan et al., 2021; Sozen et al., 2021) and 8CLCs (Mazid et al., 2022; Yu et al., 2022). Moreover, iBlastoids have been observed to form during the reprogramming of fibroblasts into a pluripotent state (Liu et al., 2021b). Thus far, there have been no reports of human blastoid formation using human TS cells (Okae et al., 2018). This may be explained by the closer similarity of human TS cells to post-implantation cytotrophoblasts rather than TE (Okae et al., 2018; Castel et al., 2020; Mischler et al., 2021). While certain studies have outlined some in vitro post-implantation development following 2D culture, human blastoids typically do not easily transition into this stage. This limitation may be partially attributed to the presence of cell types more related to post-implantation development (ExEM, amnion-like, definitive endoderm, etc. (Pham et al., 2022; Zhao et al., 2024)), particularly evident in iBlastoids (Liu et al., 2021b) and blastoids formed from EPSCs (Fan et al., 2021; Sozen et al., 2021). In general, blastoids derived from naive hES cells appear to align better with the blastocyst (Yanagida et al., 2021; Yu et al., 2021; Kagawa et al., 2022; Karvas et al., 2023; Yu et al., 2023) and present fewer cell types characteristic of advanced developmental stages (Zhao et al., 2024). Blastoids formed from naive hES cells thus have the best capacity to display in vitro post-implantation development, with 3D matrices further augmenting this potential (Karvas et al., 2023; Tu et al., 2023). Blastoids generated from 8CLCs have also been described, but their thorough analysis to assess lineage alignment with human embryonic pre- or post-implantation stages is pending.
Unlike in mice, where the formation of extra-embryonic lineages poses challenges, human blastoids demonstrate the ability to form these lineages using solely hES cells or their derivatives such as EPSCs (Fan et al., 2021; Sozen et al., 2021) and 8CLCs (Mazid et al., 2022; Yu et al., 2022). This distinction is attributed to the plenipotent plasticity of the human stem cells, which facilitates the human blastoid protocol but also introduces unintended or undesired cell types. Further research efforts and refinements of human blastoid generation protocols are essential to overcome current limitations and enhance their accuracy and efficiency as models for both pre- and post-implantation development. While optimizing post-implantation culture protocols can enhance the in vitro development of blastoids, assessing in vivo development of human blastoids will remain ethically restricted (Hyun et al., 2020). To evaluate the implantation potential of blastoids, both 2D and 3D endometrial cell cultures can be used to model the attachment and invasion of blastoids into the endometrium. For instance, blastoids can be seeded onto an open-faced endometrial layer obtained from endometrium organoids cultured in 2D to simulate attachment to the endometrium (Kagawa et al., 2022). Alternatively, recent advancements in 3D endometrial models offer promising alternatives to study not only adhesion but also invasion of blastocysts into the endometrium (Shibata et al., 2024). Furthermore, the recent report of non-human primate blastoid generation (Li et al., 2023b), offers an avenue to evaluate the in vivo implantation and developmental potential of blastoids in non-human primate species.
Optimizing culture conditions for extended embryo culture using real embryos
To develop effective models for studying post-implantation embryogenesis in both mice and humans, prioritizing the optimization of culture conditions first with authentic embryos is essential. However, creating an environment that faithfully mirrors the intricacies of human embryonic development proves more challenging than in mice. In mice, implantation occurs through the mural TE, co-explaining the typical egg cylinder shape. In humans, trophoblast invasion is initiated by the polar TE, leading to the formation of a bi-laminar disc shape (Weberling and Zernicka-Goetz, 2021). In mice, the polar TE independently develops into an extra-embryonic ectoderm, playing an instructive role in epiblast development without direct dependence on the endometrium. Conversely, human epiblast development relies more on interaction with the endometrium. Adding to the complexity is the inherently slower pace of human embryonic development, likely increasing the challenges associated with culture procedures. While in vitro conditions are advancing, they can never replicate the finely tuned environment of the uterine womb perfectly. In fact, the longer the span of in vitro culture, the greater the risk of inducing aberrations in embryonic development (Sunde et al., 2016).
Decades ago, numerous studies documented the in vitro growth of mouse preimplantation embryos, extending up to somite and limb bud stages (Hsu, 1971; Hsu, 1973; Hsu et al., 1974; Pienkowski et al., 1974; Chen and Hsu, 1982; Tachi, 1992). Remarkably, this research was revived nearly 40 years later, initially describing the development of preimplantation embryos up to the egg cylinder stages (Morris et al., 2012a; Bedzhov et al., 2014b; Bedzhov and Zernicka-Goetz, 2014). Subsequent modifications refined the culture procedures, enabling development again up to embryonic day 11 (E11) (Aguilera-Castrejon et al., 2021; Aguilera-Castrejon and Hanna, 2021). However, significant opportunities for further optimization exist. Mouse embryos retrieved at the pre-implantation stage (E3.5-E4.5) rarely progress through in vitro development, and only up to 20% of embryos retrieved at the early post-implantation stage (E5.5) can develop until a stage comparable to E11.5 (Aguilera-Castrejon and Hanna, 2021). The challenge in supporting the development of preimplantation embryos to such advanced stages arises at least partially from the need for these stages to undergo a cell adhesion phase on a 2D surface, disrupting normal embryonic morphogenesis. Consequently, various research groups have invested in developing 3D platforms that would mimic the uterine environment. Recently published studies suggest promising outcomes in these 3D platforms (Gu et al., 2022; Ichikawa et al., 2022; Bondarenko et al., 2023). In one study, it was demonstrated that 11% of retrieved mouse preimplantation embryos developed up to early organogenesis when cultured in a 3D platform (Gu et al., 2022). At E11.5, the placenta is necessary to distribute nutrients to the fetal body and eliminate waste (Zhai et al., 2022). Thus, to extend such in vitro cultures, efforts will have to be undertaken to provide better support of placenta development.
Concurrently, culture conditions conducive to prolonged human embryo development have been established, allowing up to 31% of preimplantation embryos to progress until a stage resembling E14 (Lindenberg et al., 1985; Deglincerti et al., 2016; Shahbazi et al., 2016; Zhou et al., 2019; Xiang et al., 2020). Nevertheless, ethical constraints present considerable challenges to advancing human embryo culture, limiting our understanding of embryonic development beyond gastrulation and impeding the exploration of organogenesis in the human embryo (Warnock, 1985). Given these ethical challenges, the exploration of culture platforms for non-human primate embryos becomes a valuable alternative (Lopata et al., 1995; Ma et al., 2019; Niu et al., 2019; Gong et al., 2023; Zhai et al., 2023). Unlike human embryos, non-human primates offer a more permissive experimental environment, facilitating a more comprehensive exploration of embryonic development, particularly during stages beyond gastrulation and into organogenesis. Using similar culture conditions as those used for extended culture of human preimplantation embryos, 21%–35% of cynomolgus monkey embryos could be cultured until a stage resembling E19-E20 before collapsing, due to technical limitations (Ma et al., 2019; Niu et al., 2019). Remarkably, two recently introduced adapted 3D culture platforms have demonstrated the ability to sustain ex utero growth of 20%–34% of cynomolgus monkey embryos up to E25-like stage, thereby extending ex utero development until early organogenesis (Gong et al., 2023; Zhai et al., 2023). Similar to mice, efforts will have to be directed towards enhancing placental development to support prolonged ex utero development in primates.
Partially and fully integrated stem cell-based embryo models mimicking early mouse post-implantation development
In contrast to blastoids, which rely on complex culture media for formation, the development of integrated post-implantation embryoids primarily depends on the interactive dynamics of employed stem cell lines or formed lineages to guide each other’s development.
In mouse embryonic development, the ExEc serves as the pivotal signaling center orchestrating germ layer formation. Experimental models, relying on the assembly of TS and ES cells, enable the simulation of early mouse post-implantation development. Initial studies formed self-organized bi-compartmental structures resembling the epiblast and ExEc observed in the egg cylinder, by simple aggregation of ES and TS cell suspensions (Harrison et al., 2017). Those egg cylinder-like aggregates (ETS models) exhibited anterior-posterior embryo polarity and demonstrated the emergence of mesoderm as well as Primordial Germ Cells (PGCs). A similar study, assembling pre-aggregated TS and ES cell aggregates, resulted in the formation of EpiTS embryoids. The authors noted that an egg cylinder displaying anterior-posterior polarity could only be obtained when the ES cell compartment displayed an epithelial-like morphology before the ES and TS cell aggregates were assembled (Girgin et al., 2021). Notably, both studies used Matrigel to induce epithelialization of the epiblast (Bedzhov and Zernicka-Goetz, 2014). In vivo, the basement membrane between the VE and the epiblast plays a crucial role in inducing epiblast cell polarization and lumenogenesis. The murine Embryonic-Trophoblast-eXtra embryonic endoderm (ETX) model circumvents the need for Matrigel by employing real extra-embryonic endoderm cells during aggregation. Mouse ETX models rely solely on the assembly of these three types of stem cells, with minimal interference from culture media. However, achieving optimal results requires precise titration of cell numbers to simulate normal, dose-dependent effects of signaling factors orchestrating embryogenesis and accurately model murine embryonic development. The initial model employing these three lineages for assembly used XEN cells as suppliers for the extra-embryonic endoderm (Sozen et al., 2018). However, this model did not progress beyond early gastrulation. A crucial enhancement for further development involved substituting XEN cells with ES cells expressing PrE-specific TF (trans)genes in an inducible manner (Amadei et al., 2021; Amadei et al., 2022; Lau et al., 2022; Tarazi et al., 2022; Dupont et al., 2023). The limited competence of XEN cells, resembling parietal endoderm (Kunath et al., 2005), likely explains their less favorable performance. Conversely, substituting TS cells with ES cells capable of forming TS cells through induced expression of trophoblast-specific TFs was not beneficial when compared to using authentic TS cells (Lau et al., 2022; Tarazi et al., 2022). Epigenetic barriers between TS and ES cell lineages (Ng et al., 2008; Hemberger, 2010; Cambuli et al., 2014) likely hinder ES cells from easily forming fully developed TS cells. Chimeric experiments have provided ample support regarding the developmental restrictions of ES, TS, and XEN cells (Bradley et al., 1984; Beddington and Robertson, 1989; Tanaka et al., 1998; Kunath et al., 2005; Kubaczka et al., 2014; Ohinata and Tsukiyama, 2014). During the assembly of ETX embryoids, the used stem cells also remain developmentally restricted, contributing exclusively to their lineage of origin (Dupont et al., 2023). This observation is crucial as it enables scientists to explore early lineage interactions using stem cell lines harboring targeted mutations in key developmental genes, thus addressing biological inquiries previously unattainable in in vivo embryos. Currently, the murine post-implantation model recapitulates embryonic development until a stage that strongly resembles E9 (Lau et al., 2022; Tarazi et al., 2022). Given the nuanced nature of developmental outcomes, optimizing the efficiency of ETX embryoid production (Dupont et al., 2023) is still essential to ensure an adequate supply of data points.
Partially and fully integrated stem cell-based embryo models mimicking early human post-implantation development
While extensive knowledge exists regarding mouse embryonic development, ethical constraints limit the study of human embryonic development. Nonetheless, valuable insights have been gained from the post-implantation amniotic sac embryoid (PASE) stem cell model (Shao et al., 2017a; Shao et al., 2017b). This model is a non-integrated stem cell-based embryo model that uses hES cells, and accurately simulates the development of the amniotic epithelium from the epiblast. It shows that the formation of the amniotic epithelium along PGCs, is dependent on BMP signaling (Zheng et al., 2019). The origin of BMP is speculated to be extra-embryonic. Subsequently, the amnion produces BMP4, triggering mesoderm formation in the posterior epiblast in a WNT-dependent manner (Shao et al., 2017a; Shao et al., 2017b; Zheng et al., 2019; Yang et al., 2021). However, there are still unanswered questions about the source of signaling molecules and the manner different lineages interact in these non-integrated stem cell-based embryo models that lack the extra-embryonic cell types. Human stem cell-based embryo models presenting extra-embryonic lineages could offer significant value in addressing these challenges. Six recent studies have presented stem cell-based embryo models featuring a bi-laminar disc structure comprising hypoblast and epiblast cells, while lacking a trophoblast compartment. The key commonality across all six studies is the induced formation of the hypoblast layer, facilitating the development of a bi-laminar disc structure. The models are known as heX embryoid, human extra-embryoid (hEE), peri-gastruloid, human gastruloid, E-assembloid and bilaminoid models (Ai et al., 2023; Hislop et al., 2023; Liu et al., 2023; Okubo et al., 2023; Pedroza et al., 2023; Yuan et al., 2023) (Figure 4). In heX embryoids (Hislop et al., 2023), hypoblast development relied on the continuous induction of GATA6 transcription in primed hES cells. This induction occured while co-culturing hES cells expressing GATA6 with non-induced hES cells on a cell culture dish. Consequently, induced cells encapsulated non-induced ones, leading to the formation of bi-laminar disc structures featuring an amniotic cavity. Notably, some hypoblast cells acquired characteristics similar to the AVE. Prolonged culture under modified conditions triggered the emergence of yolk sac mesoderm and blood progenitors (Hislop et al., 2023). In bilaminoids (Okubo et al., 2023), hypoblast induction relied on induced GATA6 expression in naïve hES cells. Upon aggregation with naïve hES cells, the hES cells expressing GATA6 encapsulated those that did not. Subsequently, the GATA6 non-expressing ES cells gave rise to an amniotic cavity and epithelium, along with AVE-like, mesoderm-like, and PGC-like cells that were observable by day 9. The formation and expansion of the amniotic cavity relied on the addition of IL6 during the first 4 days, whereas the formation of PGCs and most likely also the amnion relied on addition of BMP4 from day 5 onwards (Okubo et al., 2023). The human extra-embryoids (hEEs) model employed hES cells, which underwent spontaneous differentiation toward hypoblast during the aggregation procedure in non-adhesive culture conditions (Pedroza et al., 2023). Human ES cells cultured in RSET (intermediate pluripotency) were chosen due to challenges in forming organized structures with both naïve and primed hES cells. This spontaneous differentiation led to a mixed cell population, with some cells biased toward hypoblast formation while others retained pluripotency. Co-culture with TS cells compromised structural organization, however in their absence organized structures could form. By day 6, the pluripotent cells had formed an amniotic cavity with cells resembling amnion, epiblast, and primitive streak. The hypoblast, predominantly present, encompassed the entire structure, with some cells exhibiting AVE-like characteristics (Pedroza et al., 2023). A fourth paper, outlining the peri-gastruloid model (Liu et al., 2023), used EPSCs as the cell source. During the first 4 days of aggregation in non-adherent culture wells, the cells or aggregates were exposed to culture media promoting hypoblast formation. A portion of the cells acquired the hypoblast fate, leading to their efficient organization into embryoids resembling post-implantation-like human embryos. By day 11, the peri-gastruloids had developed bilaminar discs, amniotic and yolk sac cavities, initiated gastrulation, formed PGCs, and even exhibited features of early organogenesis (Liu et al., 2023). In the fifth paper, primed hES cells were used to form post-implantation-like aggregates known as human gastruloids (Yuan et al., 2023). Upon exposure to culture media supporting nEnd formation (first stage medium), hES cells underwent differentiation into hypoblast-like cells, which subsequently organized into a hypoblast-like cell layer and formed a primary yolk sac. Subsequent exposure to BMP4 and bFGF in the second stage medium facilitated the formation of the amnion. Remarkably, by day 7, the human gastruloids exhibited anterior-posterior polarity, accompanied by the emergence of AVE-like cells, mesoderm, and PGCs (Yuan et al., 2023). Finally, embryo-like assembloids (E-assembloids) (Ai et al., 2023) were derived from naïve hES cells and partially differentiated hES cells, termed Signaling Nest Cells (SNLs), which transiently emerged during trophoblast induction and secrete WNT and BMP ligands. The naïve hES cells were aggregated 1 day prior to the addition of SNLs to the non-adherent wells. While SNLs themselves did not form the trophoblast compartment, their presence facilitated the efficient aggregation of cells into an organized structure resembling a bilaminar embryonic disc. By day 8, the E-assembloids presented an amniotic cavity and a yolk sac, both surrounded by an extra-embryonic cell type. PGC-like cells were detected and although not definitely confirmed, an anterior-posterior axis with cells resembling the AVE and mesoderm was identified. From these models; peri-gastruloids, human gastruloids, and E-assembloids exhibit remarkable similarity to human post-implantation embryos. The reduced dependence on intricate culture media, however, make models like peri-gastruloids of greater utility for studying lineage interactions.
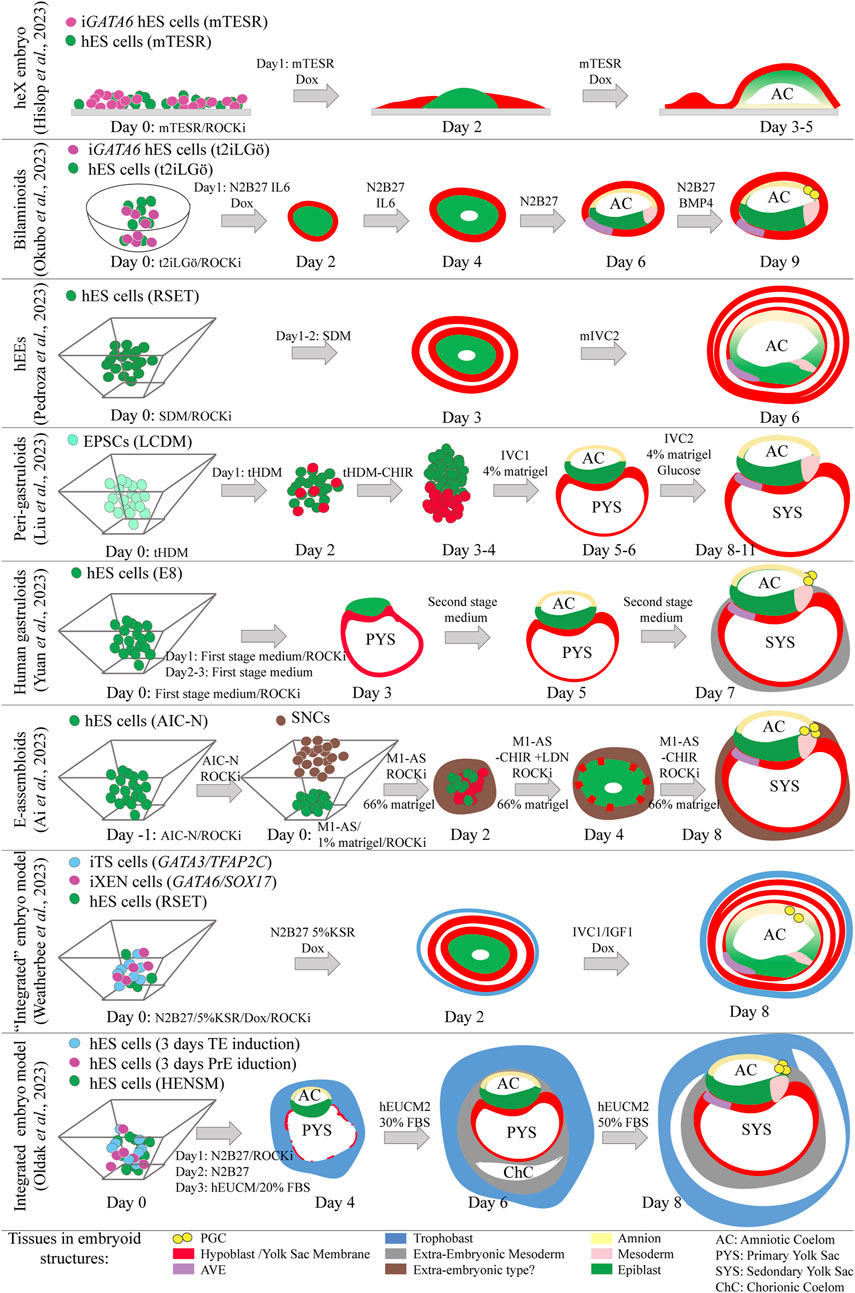
Figure 4. Schematic representation of recent partially and fully integrated stem cell-based embryo models mimicking early human post-implantation development. The upper six models do not display trophectoderm lineages, the bottom two models present epiblast, hypoblast and trophectoderm related lineages. Day 2 and day 4 representations of the E-assembloids are extrapolated from fluorescence images.
Two additional recent studies reported on the development of embryoids capable of incorporating all three early lineages into embryo-like structures (Oldak et al., 2023; Weatherbee et al., 2023) (Figure 4). These models, similar to murine ETX embryoids, involve the assembly of three distinct cell types with the potential to form trophoblast, hypoblast, and epiblast. In one approach, a group used transgene-overexpressing hES cell lines to generate the extra-embryonic lineages (trophoblast and hypoblast) necessary for constructing the human post-implantation model (Weatherbee et al., 2023). This model shares similarities with the human extra-embryoids (hEEs) (Pedroza et al., 2023), albeit that the entire embryoid is surrounded by TE-like cells and PGC-like cells can be detected. However, it may not be classified as fully integrated. In the second approach, researchers successfully employed three lineage-biased stem cell lines to create a structure resembling a human post-implantation embryo (Oldak et al., 2023). They used extra-embryonic stem cell lines partially chemically transdifferentiated from naïve hES cells (Oldak et al., 2023). Morphologically, this study provided compelling evidence, including images demonstrating the similarity of the model to E13-E14 post-implantation human embryos. The efficiency of producing these models remains notably low, likely due to challenges in forming the extra-embryonic lineages.
Enhanced understanding of the embryonic and extra-embryonic early lineages within the human blastocyst is imperative for selecting appropriate cell lines and establishing precise culture conditions in the development of stem cell-based human post-implantation embryo models. Conversely, a diverse array of partially integrated human post-implantation stem cell models has demonstrated remarkable fidelity in modeling post-implantation development beyond gastrulation; using solely either EPSCs, naïve hES cells and even primed hES cells. This significant finding highlights both the plasticity of the human epiblast in efficiently forming the hypoblast and the crucial role of the epiblast in establishing its own signaling center—the amnion. The successful formation of partially integrated post-implantation models modeling embryonic development up to the gastrulation stage suggests that, depending on the research question, integration of the trophectoderm may not be necessary to model and study human early post-implantation development.
Comparative analysis methods of stem cell-based embryo models to natural embryos
To accurately assess the fidelity of stem cell-based embryo models in replicating mouse or primate embryonic development, direct comparisons with natural embryos are indispensable. Since transcription factors are not lineage-specific, accurately localizing a subset of transcription factors within a morphologically similar model is insufficient for evaluating the similarity between the embryo model and the natural embryo. While single-cell RNA sequencing (scRNA-seq) of dissociated embryo models enhances cell type characterization, additional assessments using immunostaining to locate these cell populations within the model are indispensable. To facilitate these comparisons, it is essential to have access to scRNA-seq and immunostaining data from natural embryos. While these libraries exist for mouse pre- and post-implantation embryos (reviewed in (Posfai et al., 2021)), creating similar resources for humans poses ethical challenges. Given these ethical constraints, non-human primate embryos serve as a complement for investigating primate embryonic development. As such, valuable scRNA-seq and immunostaining data have been derived from human (Blakeley et al., 2015; Stirparo et al., 2018; Petropoulos et al., 2016; Yan et al., 2013) and non-human primate (Boroviak et al., 2018; Nakamura et al., 2016) preimplantation embryos, in vitro-cultured human (Zhou et al., 2019; Xiang et al., 2020; Mole et al., 2021; Ai et al., 2023) and non-human (Ma et al., 2019; Niu et al., 2019; Gong et al., 2023; Zhai et al., 2023) embryos into post-implantation stages, human terminated pregnancies (Tyser et al., 2021; Xu et al., 2023) and uterine-retrieved non-human primate embryos (Nakamura et al., 2016; Zhai et al., 2022).
The integration and comparison of data from multiple sources, such as transcriptomic profiles and immunostaining of natural embryos and stem cell-based models, however, is challenging. Transcriptomic data, while informative, may encounter discrepancies between experimental systems due to variations in protocols and employed techniques. Similarly, immunostaining of post-implantation embryos and their corresponding stem-cell based embryo models is complex, as it requires meticulous sample preparation, orientation, and sectioning. It is therefore important to consider technical nuances and potential sources of variability, when comparing natural embryos and stem cell-based embryo models. While dissociation-based approaches struggle to preserve tissue structure, thereby restricting expression analysis within the natural context, spatial transcriptomic technologies aim to overcome this limitation. Whereas some studies have employed spatial transcriptomics technologies to study mouse (Peng et al., 2019; Chen et al., 2022; Sampath Kumar et al., 2023; Srivatsan et al., 2021) and human (Xu et al., 2023; Zeng et al., 2023) embryogenesis, the employed techniques currently still face too many technical and computational challenges (reviewed by (Zhang et al., 2023; Zhou et al., 2023)) to be routinely used for comparative analyses.
Discussion
Partially and fully integrated stem cell-based embryo models offer an innovative tool to study primate and mouse embryonic development. These models not only alleviate the need for sacrificing living pregnant mice but also avoid the use of human embryos for research purposes. Moreover, they offer a framework to explore scientific inquiries that are otherwise impossible or exceedingly challenging to study in vivo. Studying embryonic development using stem cell-based embryo models requires not only faithful replication of embryonic processes but also necessitates achieving a high level of efficiency in replicating these events. Indeed, given the complexity of biological responses, employing large sample sizes is essential for accurate quantitative analysis of results.
Human and mouse stem cell-based embryo models, whether partially or fully integrated, present distinct challenges. Firstly, developmental differences between mice and primates impact the approach to construct these models. For example, in mice, where the ExEc acts as a pivotal signaling hub directing gastrulation, the inclusion of a trophoblast compartment becomes imperative when modeling post-implantation development. In contrast, in humans, where the epiblast-derived amnion governs gastrulation, the inclusion of a trophoblast compartment in early post-implantation stem cell models is not as important. Variations in potency of existing stem cell lines in humans and mice also contribute to differences observed between mouse and human integrated stem cell models. Human stem cell lines, such as 8CLCs, EPSCs, naïve- and primed-pluripotent stem cells, demonstrate plenipotent characteristics and have been used as the primary cell source for forming human integrated stem cell-based pre- and post-implantation embryo models. In contrast, the development of these mouse models depends on the incorporation of multiple lineage-restricted stem cell populations. This results in two discernable strategies, the ‘inductive’ and ‘assembly’ approaches. While the inductive strategy uses complex culture media to direct lineage development, the assembly approach leverages the self-organizing potential of different stem cell lineages. Both strategies have their advantages and disadvantages. Assembly methods, such as those seen in models like ETX, require precise titration of cell numbers from various stem cell lines to accurately model the normal, dose-dependent effects of signaling factors during early embryogenesis. Meanwhile, the use of lineage-restricted stem cells, facilitates genetic perturbation studies in specific cell lineages. On the other hand, approaches based on ‘induction’ using plenipotent stem cells, as seen in human integrated post-implantation models, do not require as rigorous a titration of cell numbers but may not be as suitable for lineage-specific genetic perturbation studies, considering the uncertain developmental fate of a plenipotent cell. A deeper understanding of human early lineage specification may, in the future, aid in the development of integrated human embryo models constructed from lineage-restricted stem cell lines.
In addition to addressing biological challenges, it is imperative to consider technical and ethical limitations when developing models for studying post-implantation embryogenesis in mice and humans. Optimizing in vitro culture conditions with authentic embryos is important for refining the culture procedure and establishing a framework for comparing the models. However, due to the ethical limitations surrounding human embryo research, these 3D culture procedures may be more easily optimized using non-human primates. Additionally, non-human primates provide an opportunity to explore culture procedures beyond gastrulation and into organogenesis. The development of culture platforms that support placental development will most likely be pivotal to support development even further. Due to ethical restrictions that human integrated stem cell-based embryo models face (Hyun et al., 2020), the development of non-human primate integrated embryo models will also be instrumental. These non-human primate models offer an avenue to explore the developmental potential of these models beyond gastrulation and into organogenesis, both in vivo and during in vitro culture.
In conclusion, mammalian integrated stem cell-based embryo models have become instrumental tools for studying early embryogenesis in both mice and primates. The differences in embryonic development and stem cell biology between humans and mice have shaped and will continue to shape strategies used to construct these models.
Author contributions
CD: Writing–original draft, Writing–review and editing, Conceptualization, Investigation.
Funding
The author(s) declare that financial support was received for the research, authorship, and/or publication of this article. This work has become possible through a ZonMW-PSIDER grant (10250022120002) awarded to Joost Gribnau.
Acknowledgments
I would like to express my sincere gratitude to Danny Huylebroeck and Sarra Merzouk for generously dedicating their time and expertise to read and provide valuable feedback on this manuscript.
Conflict of interest
The author declares that the research was conducted in the absence of any commercial or financial relationships that could be construed as a potential conflict of interest.
Publisher’s note
All claims expressed in this article are solely those of the authors and do not necessarily represent those of their affiliated organizations, or those of the publisher, the editors and the reviewers. Any product that may be evaluated in this article, or claim that may be made by its manufacturer, is not guaranteed or endorsed by the publisher.
References
Acampora, D., Mazan, S., Lallemand, Y., Avantaggiato, V., Maury, M., Simeone, A., et al. (1995). Forebrain and midbrain regions are deleted in Otx2-/- mutants due to a defective anterior neuroectoderm specification during gastrulation. Development 121, 3279–3290. doi:10.1242/dev.121.10.3279
Aguilera-Castrejon, A., and Hanna, J. H. (2021). Ex utero culture of mouse embryos from pregastrulation to advanced organogenesis. J. Vis. Exp. doi:10.3791/63160
Aguilera-Castrejon, A., Oldak, B., Shani, T., Ghanem, N., Itzkovich, C., Slomovich, S., et al. (2021). Ex utero mouse embryogenesis from pre-gastrulation to late organogenesis. Nature 593, 119–124. doi:10.1038/s41586-021-03416-3
Ai, Z., Niu, B., Yin, Y., Xiang, L., Shi, G., Duan, K., et al. (2023). Dissecting peri-implantation development using cultured human embryos and embryo-like assembloids. Cell Res. 33, 661–678. doi:10.1038/s41422-023-00846-8
Amadei, G., Handford, C. E., Qiu, C., De Jonghe, J., Greenfeld, H., Tran, M., et al. (2022). Embryo model completes gastrulation to neurulation and organogenesis. Nature 610, 143–153. doi:10.1038/s41586-022-05246-3
Amadei, G., Lau, K. Y. C., De Jonghe, J., Gantner, C. W., Sozen, B., Chan, C., et al. (2021). Inducible stem-cell-derived embryos capture mouse morphogenetic events in vitro. Dev. Cell 56, 366–382 e9. doi:10.1016/j.devcel.2020.12.004
Anderson, K. G. V., Hamilton, W. B., Roske, F. V., Azad, A., Knudsen, T. E., Canham, M. A., et al. (2017). Insulin fine-tunes self-renewal pathways governing naive pluripotency and extra-embryonic endoderm. Nat. Cell Biol. 19, 1164–1177. doi:10.1038/ncb3617
Bayerl, J., Ayyash, M., Shani, T., Manor, Y. S., Gafni, O., Massarwa, R., et al. (2021). Principles of signaling pathway modulation for enhancing human naive pluripotency induction. Cell Stem Cell 28, 1549–1565 e12. doi:10.1016/j.stem.2021.04.001
Beddington, R. S., and Robertson, E. J. (1989). An assessment of the developmental potential of embryonic stem cells in the midgestation mouse embryo. Development 105, 733–737. doi:10.1242/dev.105.4.733
Bedzhov, I., Graham, S. J., Leung, C. Y., and Zernicka-Goetz, M. (2014a). Developmental plasticity, cell fate specification and morphogenesis in the early mouse embryo. Philos. Trans. R. Soc. Lond. B Biol. Sci. 369, 20130538. doi:10.1098/rstb.2013.0538
Bedzhov, I., Leung, C. Y., Bialecka, M., and Zernicka-Goetz, M. (2014b). In vitro culture of mouse blastocysts beyond the implantation stages. Nat. Protoc. 9, 2732–2739. doi:10.1038/nprot.2014.186
Bedzhov, I., and Zernicka-Goetz, M. (2014). Self-organizing properties of mouse pluripotent cells initiate morphogenesis upon implantation. Cell 156, 1032–1044. doi:10.1016/j.cell.2014.01.023
Belo, J. A., Bouwmeester, T., Leyns, L., Kertesz, N., Gallo, M., Follettie, M., et al. (1997). Cerberus-like is a secreted factor with neutralizing activity expressed in the anterior primitive endoderm of the mouse gastrula. Mech. Dev. 68, 45–57. doi:10.1016/s0925-4773(97)00125-1
Bergmann, S., Penfold, C. A., Slatery, E., Siriwardena, D., Drummer, C., Clark, S., et al. (2022). Spatial profiling of early primate gastrulation in utero. Nature 609, 136–143. doi:10.1038/s41586-022-04953-1
Blakeley, P., Fogarty, N. M., Del Valle, I., Wamaitha, S. E., Hu, T. X., Elder, K., et al. (2015). Defining the three cell lineages of the human blastocyst by single-cell RNA-seq. Development 142, 3151–3165. doi:10.1242/dev.123547
Bondarenko, V., Nikolaev, M., Kromm, D., Belousov, R., Wolny, A., Blotenburg, M., et al. (2023). Embryo-uterine interaction coordinates mouse embryogenesis during implantation. EMBO J. 42, e113280. doi:10.15252/embj.2022113280
Boroviak, T., Stirparo, G. G., Dietmann, S., Hernando-Herraez, I., Mohammed, H., Reik, W., et al. (2018). Single cell transcriptome analysis of human, marmoset and mouse embryos reveals common and divergent features of preimplantation development. Development 145.
Bradley, A., Evans, M., Kaufman, M. H., and Robertson, E. (1984). Formation of germ-line chimaeras from embryo-derived teratocarcinoma cell lines. Nature 309, 255–256. doi:10.1038/309255a0
Braude, P., Bolton, V., and Moore, S. (1988). Human gene expression first occurs between the four- and eight-cell stages of preimplantation development. Nature 332, 459–461. doi:10.1038/332459a0
Bredenkamp, N., Stirparo, G. G., Nichols, J., Smith, A., and Guo, G. (2019). The cell-surface marker sushi containing domain 2 facilitates establishment of human naive pluripotent stem cells. Stem Cell Rep. 12, 1212–1222. doi:10.1016/j.stemcr.2019.03.014
Brons, I. G., Smithers, L. E., Trotter, M. W., Rugg-Gunn, P., Sun, B., Chuva De Sousa Lopes, S. M., et al. (2007). Derivation of pluripotent epiblast stem cells from mammalian embryos. Nature 448, 191–195. doi:10.1038/nature05950
Cambuli, F., Murray, A., Dean, W., Dudzinska, D., Krueger, F., Andrews, S., et al. (2014). Epigenetic memory of the first cell fate decision prevents complete ES cell reprogramming into trophoblast. Nat. Commun. 5, 5538. doi:10.1038/ncomms6538
Casser, E., Israel, S., Witten, A., Schulte, K., Schlatt, S., Nordhoff, V., et al. (2017). Totipotency segregates between the sister blastomeres of two-cell stage mouse embryos. Sci. Rep. 7, 8299. doi:10.1038/s41598-017-08266-6
Castel, G., Meistermann, D., Bretin, B., Firmin, J., Blin, J., Loubersac, S., et al. (2020). Induction of human trophoblast stem cells from somatic cells and pluripotent stem cells. Cell Rep. 33, 108419. doi:10.1016/j.celrep.2020.108419
Cauffman, G., De Rycke, M., Sermon, K., Liebaers, I., and Van De Velde, H. (2009). Markers that define stemness in ESC are unable to identify the totipotent cells in human preimplantation embryos. Hum. Reprod. 24, 63–70. doi:10.1093/humrep/den351
Chazaud, C., Yamanaka, Y., Pawson, T., and Rossant, J. (2006). Early lineage segregation between epiblast and primitive endoderm in mouse blastocysts through the Grb2-MAPK pathway. Dev. Cell 10, 615–624. doi:10.1016/j.devcel.2006.02.020
Chen, L. T., and Hsu, Y. C. (1982). Development of mouse embryos in vitro: preimplantation to the limb bud stage. Science 218, 66–68. doi:10.1126/science.7123220
Chen, A., Liao, S., Cheng, M., Ma, K., Wu, L., Lai, Y., et al. (2022). Spatiotemporal transcriptomic atlas of mouse organogenesis using DNA nanoball-patterned arrays. Cell 185, 1777–1792. doi:10.1016/j.cell.2022.04.003
Cho, L. T., Wamaitha, S. E., Tsai, I. J., Artus, J., Sherwood, R. I., Pedersen, R. A., et al. (2012). Conversion from mouse embryonic to extra-embryonic endoderm stem cells reveals distinct differentiation capacities of pluripotent stem cell states. Development 139, 2866–2877. doi:10.1242/dev.078519
Choi, Y. J., Lin, C. P., Risso, D., Chen, S., Kim, T. A., Tan, M. H., et al. (2017). Deficiency of microRNA miR-34a expands cell fate potential in pluripotent stem cells. Science 355, eaag1927. doi:10.1126/science.aag1927
Cinkornpumin, J. K., Kwon, S. Y., Guo, Y., Hossain, I., Sirois, J., Russett, C. S., et al. (2020). Naive human embryonic stem cells can give rise to cells with a trophoblast-like transcriptome and methylome. Stem Cell Rep. 15, 198–213. doi:10.1016/j.stemcr.2020.06.003
Condic, M. L. (2014). Totipotency: what it is and what it is not. Stem Cells Dev. 23, 796–812. doi:10.1089/scd.2013.0364
Dan, J., Liu, Y., Liu, N., Chiourea, M., Okuka, M., Wu, T., et al. (2014). Rif1 maintains telomere length homeostasis of ESCs by mediating heterochromatin silencing. Dev. Cell 29, 7–19. doi:10.1016/j.devcel.2014.03.004
Deglincerti, A., Croft, G. F., Pietila, L. N., Zernicka-Goetz, M., Siggia, E. D., and Brivanlou, A. H. (2016). Self-organization of the in vitro attached human embryo. Nature 533, 251–254. doi:10.1038/nature17948
De Iaco, A., Coudray, A., Duc, J., and Trono, D. (2019). DPPA2 and DPPA4 are necessary to establish a 2C-like state in mouse embryonic stem cells. EMBO Rep. 20, e47382. doi:10.15252/embr.201847382
De Los Angeles, A., Ferrari, F., Xi, R., Fujiwara, Y., Benvenisty, N., Deng, H., et al. (2015). Hallmarks of pluripotency. Nature 525, 469–478. doi:10.1038/nature15515
De Paepe, C., Cauffman, G., Verloes, A., Sterckx, J., Devroey, P., Tournaye, H., et al. (2013). Human trophectoderm cells are not yet committed. Hum. Reprod. 28, 740–749. doi:10.1093/humrep/des432
Dietrich, J. E., and Hiiragi, T. (2007). Stochastic patterning in the mouse pre-implantation embryo. Development 134, 4219–4231. doi:10.1242/dev.003798
Dong, C., Beltcheva, M., Gontarz, P., Zhang, B., Popli, P., Fischer, L. A., et al. (2020). Derivation of trophoblast stem cells from naive human pluripotent stem cells. Elife 9, e52504. doi:10.7554/eLife.52504
Duggal, G., Warrier, S., Ghimire, S., Broekaert, D., Van Der Jeught, M., Lierman, S., et al. (2015). Alternative routes to induce naive pluripotency in human embryonic stem cells. Stem Cells 33, 2686–2698. doi:10.1002/stem.2071
Dupont, C., Schaffers, O. J. M., Tan, B. F., Merzouk, S., Bindels, E. M., Zwijsen, A., et al. (2023). Efficient generation of ETX embryoids that recapitulate the entire window of murine egg cylinder development. Sci. Adv. 9, eadd2913. doi:10.1126/sciadv.add2913
Evans, M. J., and Kaufman, M. H. (1981). Establishment in culture of pluripotential cells from mouse embryos. Nature 292, 154–156. doi:10.1038/292154a0
Fan, Y., Min, Z., Alsolami, S., Ma, Z., Zhang, E., Chen, W., et al. (2021). Generation of human blastocyst-like structures from pluripotent stem cells. Cell Discov. 7, 81. doi:10.1038/s41421-021-00316-8
Frankenberg, S., Gerbe, F., Bessonnard, S., Belville, C., Pouchin, P., Bardot, O., et al. (2011). Primitive endoderm differentiates via a three-step mechanism involving Nanog and RTK signaling. Dev. Cell 21, 1005–1013. doi:10.1016/j.devcel.2011.10.019
Frum, T., and Ralston, A. (2015). Cell signaling and transcription factors regulating cell fate during formation of the mouse blastocyst. Trends Genet. 31, 402–410. doi:10.1016/j.tig.2015.04.002
Fujikura, J., Yamato, E., Yonemura, S., Hosoda, K., Masui, S., Nakao, K., et al. (2002). Differentiation of embryonic stem cells is induced by GATA factors. Genes Dev. 16, 784–789. doi:10.1101/gad.968802
Gafni, O., Weinberger, L., Mansour, A. A., Manor, Y. S., Chomsky, E., Ben-Yosef, D., et al. (2013). Derivation of novel human ground state naive pluripotent stem cells. Nature 504, 282–286. doi:10.1038/nature12745
Gao, X., Nowak-Imialek, M., Chen, X., Chen, D., Herrmann, D., Ruan, D., et al. (2019). Establishment of porcine and human expanded potential stem cells. Nat. Cell Biol. 21, 687–699. doi:10.1038/s41556-019-0333-2
Gerri, C., Mccarthy, A., Alanis-Lobato, G., Demtschenko, A., Bruneau, A., Loubersac, S., et al. (2020). Initiation of a conserved trophectoderm program in human, cow and mouse embryos. Nature 587, 443–447. doi:10.1038/s41586-020-2759-x
Girgin, M. U., Broguiere, N., Hoehnel, S., Brandenberg, N., Mercier, B., Arias, A. M., et al. (2021). Bioengineered embryoids mimic post-implantation development in vitro. Nat. Commun. 12, 5140. doi:10.1038/s41467-021-25237-8
Gong, Y., Bai, B., Sun, N., Ci, B., Shao, H., Zhang, T., et al. (2023). Ex utero monkey embryogenesis from blastocyst to early organogenesis. Cell 186, 2092–2110 e23. doi:10.1016/j.cell.2023.04.020
Gu, Z., Guo, J., Zhai, J., Feng, G., Wang, X., Gao, Z., et al. (2022). A uterus-inspired niche drives blastocyst development to the early organogenesis. Adv. Sci. (Weinh) 9, e2202282. doi:10.1002/advs.202202282
Guo, G., Stirparo, G. G., Strawbridge, S. E., Spindlow, D., Yang, J., Clarke, J., et al. (2021). Human naive epiblast cells possess unrestricted lineage potential. Cell Stem Cell 28, 1040–1056 e6. doi:10.1016/j.stem.2021.02.025
Guo, G., Von Meyenn, F., Rostovskaya, M., Clarke, J., Dietmann, S., Baker, D., et al. (2017). Epigenetic resetting of human pluripotency. Development 144, 2748–2763. doi:10.1242/dev.146811
Handyside, A. H. (1978). Time of commitment of inside cells isolated from preimplantation mouse embryos. J. Embryol. Exp. Morphol. 45, 37–53. doi:10.1242/dev.45.1.37
Harrison, S. E., Sozen, B., Christodoulou, N., Kyprianou, C., and Zernicka-Goetz, M. (2017). Assembly of embryonic and extraembryonic stem cells to mimic embryogenesis in vitro. Science 356, eaal1810. doi:10.1126/science.aal1810
Hayashi, M., Maehara, K., Harada, A., Semba, Y., Kudo, K., Takahashi, H., et al. (2016). Chd5 regulates MuERV-L/MERVL expression in mouse embryonic stem cells via H3K27me3 modification and histone H3.1/H3.2. J. Cell Biochem. 117, 780–792. doi:10.1002/jcb.25368
Hayashi, Y., Furue, M. K., Tanaka, S., Hirose, M., Wakisaka, N., Danno, H., et al. (2010). BMP4 induction of trophoblast from mouse embryonic stem cells in defined culture conditions on laminin. Vitro Cell Dev. Biol. Anim. 46, 416–430. doi:10.1007/s11626-009-9266-6
Hemberger, M. (2010). Genetic-epigenetic intersection in trophoblast differentiation: implications for extraembryonic tissue function. Epigenetics 5, 24–29. doi:10.4161/epi.5.1.10589
Hisada, K., Sanchez, C., Endo, T. A., Endoh, M., Roman-Trufero, M., Sharif, J., et al. (2012). RYBP represses endogenous retroviruses and preimplantation- and germ line-specific genes in mouse embryonic stem cells. Mol. Cell Biol. 32, 1139–1149. doi:10.1128/MCB.06441-11
Hislop, J., Song, Q., Keshavarz, F. K., Alavi, A., Schoenberger, R., Legraw, R., et al. (2023). Modeling post-implantation human development to yolk sac blood emergence. Nature. doi:10.1038/s41586-023-06914-8
Hogan, B., and Tilly, R. (1978). In vitro development of inner cell masses isolated immunosurgically from mouse blastocysts: II. Inner cell masses from 3·5- to 4·0-day p.c. blastocysts. J. Embryol. Exp. Morphol. 45, 107–121. doi:10.1242/dev.45.1.107
Hsu, Y. C. (1971). Post-blastocyst differentiation in vitro. Nature 231, 100–102. doi:10.1038/231100a0
Hsu, Y. C. (1973). Differentiation in vitro of mouse embryos to the stage of early somite. Dev. Biol. 33, 403–411. doi:10.1016/0012-1606(73)90145-0
Hsu, Y. C., Baskar, J., Stevens, L. C., and Rash, J. E. (1974). Development in vitro of mouse embryos from the two-cell egg stage to the early somite stage. J. Embryol. Exp. Morphol. 31, 235–245. doi:10.1242/dev.31.1.235
Hu, Y., Yang, Y., Tan, P., Zhang, Y., Han, M., Yu, J., et al. (2023). Induction of mouse totipotent stem cells by a defined chemical cocktail. Nature 617, 792–797. doi:10.1038/s41586-022-04967-9
Hu, Z., Tan, D. E. K., Chia, G., Tan, H., Leong, H. F., Chen, B. J., et al. (2020). Maternal factor NELFA drives a 2C-like state in mouse embryonic stem cells. Nat. Cell Biol. 22, 175–186. doi:10.1038/s41556-019-0453-8
Huang, Y., Osorno, R., Tsakiridis, A., and Wilson, V. (2012). In vivo differentiation potential of epiblast stem cells revealed by chimeric embryo formation. Cell Rep. 2, 1571–1578. doi:10.1016/j.celrep.2012.10.022
Huang, Z., Yu, J., Cui, W., Johnson, B. K., Kim, K., and Pfeifer, G. P. (2021). The chromosomal protein SMCHD1 regulates DNA methylation and the 2c-like state of embryonic stem cells by antagonizing TET proteins. Sci. Adv. 7, eabb9149. doi:10.1126/sciadv.abb9149
Hyun, I., Munsie, M., Pera, M. F., Rivron, N. C., and Rossant, J. (2020). Toward guidelines for research on human embryo models formed from stem cells. Stem Cell Rep. 14, 169–174. doi:10.1016/j.stemcr.2019.12.008
Ichikawa, T., Zhang, H. T., Panavaite, L., Erzberger, A., Fabreges, D., Snajder, R., et al. (2022). An ex vivo system to study cellular dynamics underlying mouse peri-implantation development. Dev. Cell 57, 373–386 e9. doi:10.1016/j.devcel.2021.12.023
Io, S., Kabata, M., Iemura, Y., Semi, K., Morone, N., Minagawa, A., et al. (2021). Capturing human trophoblast development with naive pluripotent stem cells in vitro. Cell Stem Cell 28, 1023–1039 e13. doi:10.1016/j.stem.2021.03.013
Jedrusik, A., Bruce, A. W., Tan, M. H., Leong, D. E., Skamagki, M., Yao, M., et al. (2010). Maternally and zygotically provided Cdx2 have novel and critical roles for early development of the mouse embryo. Dev. Biol. 344, 66–78. doi:10.1016/j.ydbio.2010.04.017
Kagawa, H., Javali, A., Khoei, H. H., Sommer, T. M., Sestini, G., Novatchkova, M., et al. (2022). Human blastoids model blastocyst development and implantation. Nature 601, 600–605. doi:10.1038/s41586-021-04267-8
Kaiser, F., Kubaczka, C., Graf, M., Langer, N., Langkabel, J., Arevalo, L., et al. (2020). Choice of factors and medium impinge on success of ESC to TSC conversion. Placenta 90, 128–137. doi:10.1016/j.placenta.2019.12.017
Karvas, R. M., Zemke, J. E., Ali, S. S., Upton, E., Sane, E., Fischer, L. A., et al. (2023). 3D-cultured blastoids model human embryogenesis from pre-implantation to early gastrulation stages. Cell Stem Cell 30, 1148–1165 e7. doi:10.1016/j.stem.2023.08.005
Kime, C., Kiyonari, H., Ohtsuka, S., Kohbayashi, E., Asahi, M., Yamanaka, S., et al. (2019). Induced 2C expression and implantation-competent blastocyst-like cysts from primed pluripotent stem cells. Stem Cell Rep. 13, 485–498. doi:10.1016/j.stemcr.2019.07.011
Kimura, C., Yoshinaga, K., Tian, E., Suzuki, M., Aizawa, S., and Matsuo, I. (2000). Visceral endoderm mediates forebrain development by suppressing posteriorizing signals. Dev. Biol. 225, 304–321. doi:10.1006/dbio.2000.9835
Kimura-Yoshida, C., Nakano, H., Okamura, D., Nakao, K., Yonemura, S., Belo, J. A., et al. (2005). Canonical Wnt signaling and its antagonist regulate anterior-posterior axis polarization by guiding cell migration in mouse visceral endoderm. Dev. Cell 9, 639–650. doi:10.1016/j.devcel.2005.09.011
Kubaczka, C., Senner, C., Arauzo-Bravo, M. J., Sharma, N., Kuckenberg, P., Becker, A., et al. (2014). Derivation and maintenance of murine trophoblast stem cells under defined conditions. Stem Cell Rep. 2, 232–242. doi:10.1016/j.stemcr.2013.12.013
Kuckenberg, P., Buhl, S., Woynecki, T., Van Furden, B., Tolkunova, E., Seiffe, F., et al. (2010). The transcription factor TCFAP2C/AP-2gamma cooperates with CDX2 to maintain trophectoderm formation. Mol. Cell Biol. 30, 3310–3320. doi:10.1128/MCB.01215-09
Kuijk, E. W., Van Tol, L. T., Van De Velde, H., Wubbolts, R., Welling, M., Geijsen, N., et al. (2012). The roles of FGF and MAP kinase signaling in the segregation of the epiblast and hypoblast cell lineages in bovine and human embryos. Development 139, 871–882. doi:10.1242/dev.071688
Kunath, T., Arnaud, D., Uy, G. D., Okamoto, I., Chureau, C., Yamanaka, Y., et al. (2005). Imprinted X-inactivation in extra-embryonic endoderm cell lines from mouse blastocysts. Development 132, 1649–1661. doi:10.1242/dev.01715
Lau, K. Y. C., Rubinstein, H., Gantner, C. W., Hadas, R., Amadei, G., Stelzer, Y., et al. (2022). Mouse embryo model derived exclusively from embryonic stem cells undergoes neurulation and heart development. Cell Stem Cell 29, 1445–1458 e8. doi:10.1016/j.stem.2022.08.013
Lawitts, J. A., and Graves, C. N. (1988). Viability of mouse half-embryos in vitro and in vivo. Gamete Res. 20, 421–430. doi:10.1002/mrd.1120200404
Lawson, K. A., Dunn, N. R., Roelen, B. A., Zeinstra, L. M., Davis, A. M., Wright, C. V., et al. (1999). Bmp4 is required for the generation of primordial germ cells in the mouse embryo. Genes Dev. 13, 424–436. doi:10.1101/gad.13.4.424
Lea, R. A., Mccarthy, A., Boeing, S., Fallesen, T., Elder, K., Snell, P., et al. (2021). KLF17 promotes human naive pluripotency but is not required for its establishment. Development 148, dev199378. doi:10.1242/dev.199378
Li, H., Chang, L., Wu, J., Huang, J., Guan, W., Bates, L. E., et al. (2023a). In vitro generation of mouse morula-like cells. Dev. Cell 58, 2510–2527 e7. doi:10.1016/j.devcel.2023.09.013
Li, J., Zhu, Q., Cao, J., Liu, Y., Lu, Y., Sun, Y., et al. (2023b). Cynomolgus monkey embryo model captures gastrulation and early pregnancy. Cell Stem Cell 30, 362–377 e7. doi:10.1016/j.stem.2023.03.009
Li, R., and Izpisua Belmonte, J. C. (2021). Protocol for the generation of blastocyst-like structures from mouse extended pluripotent stem cells. Star. Protoc. 2, 100745. doi:10.1016/j.xpro.2021.100745
Li, R., Zhong, C., Yu, Y., Liu, H., Sakurai, M., Yu, L., et al. (2019). Generation of blastocyst-like structures from mouse embryonic and adult cell cultures. Cell 179, 687–702. doi:10.1016/j.cell.2019.09.029
Lindenberg, S., Nielsen, M. H., and Lenz, S. (1985). In vitro studies of human blastocyst implantation. Ann. N. Y. Acad. Sci. 442, 368–374. doi:10.1111/j.1749-6632.1985.tb37541.x
Linneberg-Agerholm, M., Wong, Y. F., Romero Herrera, J. A., Monteiro, R. S., Anderson, K. G. V., and Brickman, J. M. (2019). Naive human pluripotent stem cells respond to Wnt, Nodal and LIF signalling to produce expandable naive extra-embryonic endoderm. Development 146, dev180620. doi:10.1242/dev.180620
Liu, B., Chen, S., Xu, Y., Lyu, Y., Wang, J., Du, Y., et al. (2021a). Chemically defined and xeno-free culture condition for human extended pluripotent stem cells. Nat. Commun. 12, 3017. doi:10.1038/s41467-021-23320-8
Liu, L., Oura, S., Markham, Z., Hamilton, J. N., Skory, R. M., Li, L., et al. (2023). Modeling post-implantation stages of human development into early organogenesis with stem-cell-derived peri-gastruloids. Cell 186, 3776–3792 e16. doi:10.1016/j.cell.2023.07.018
Liu, P., Wakamiya, M., Shea, M. J., Albrecht, U., Behringer, R. R., and Bradley, A. (1999). Requirement for Wnt3 in vertebrate axis formation. Nat. Genet. 22, 361–365. doi:10.1038/11932
Liu, X., Nefzger, C. M., Rossello, F. J., Chen, J., Knaupp, A. S., Firas, J., et al. (2017). Comprehensive characterization of distinct states of human naive pluripotency generated by reprogramming. Nat. Methods 14, 1055–1062. doi:10.1038/nmeth.4436
Liu, X., Tan, J. P., Schroder, J., Aberkane, A., Ouyang, J. F., Mohenska, M., et al. (2021b). Modelling human blastocysts by reprogramming fibroblasts into iBlastoids. Nature 591, 627–632. doi:10.1038/s41586-021-03372-y
Lopata, A., Kohlman, D. J., Bowes, L. G., and Watkins, W. B. (1995). Culture of marmoset blastocysts on matrigel: a model of differentiation during the implantation period. Anat. Rec. 241, 469–486. doi:10.1002/ar.1092410405
Lu, F., Liu, Y., Jiang, L., Yamaguchi, S., and Zhang, Y. (2014). Role of Tet proteins in enhancer activity and telomere elongation. Genes Dev. 28, 2103–2119. doi:10.1101/gad.248005.114
Ma, H., Zhai, J., Wan, H., Jiang, X., Wang, X., Wang, L., et al. (2019). In vitro culture of cynomolgus monkey embryos beyond early gastrulation. Science 366, eaax7890. doi:10.1126/science.aax7890
Macfarlan, T. S., Gifford, W. D., Agarwal, S., Driscoll, S., Lettieri, K., Wang, J., et al. (2011). Endogenous retroviruses and neighboring genes are coordinately repressed by LSD1/KDM1A. Genes Dev. 25, 594–607. doi:10.1101/gad.2008511
Macfarlan, T. S., Gifford, W. D., Driscoll, S., Lettieri, K., Rowe, H. M., Bonanomi, D., et al. (2012). Embryonic stem cell potency fluctuates with endogenous retrovirus activity. Nature 487, 57–63. doi:10.1038/nature11244
Mackinlay, K. M., Weatherbee, B. A., Souza Rosa, V., Handford, C. E., Hudson, G., Coorens, T., et al. (2021). An in vitro stem cell model of human epiblast and yolk sac interaction. Elife 10, e63930. doi:10.7554/eLife.63930
Maemura, M., Taketsuru, H., Nakajima, Y., Shao, R., Kakihara, A., Nogami, J., et al. (2021). Totipotency of mouse zygotes extends to single blastomeres of embryos at the four-cell stage. Sci. Rep. 11, 11167. doi:10.1038/s41598-021-90653-1
Maksakova, I. A., Thompson, P. J., Goyal, P., Jones, S. J., Singh, P. B., Karimi, M. M., et al. (2013). Distinct roles of KAP1, HP1 and G9a/GLP in silencing of the two-cell-specific retrotransposon MERVL in mouse ES cells. Epigenetics Chromatin 6, 15. doi:10.1186/1756-8935-6-15
Martin, G. R. (1981). Isolation of a pluripotent cell line from early mouse embryos cultured in medium conditioned by teratocarcinoma stem cells. Proc. Natl. Acad. Sci. U. S. A. 78, 7634–7638. doi:10.1073/pnas.78.12.7634
Mazid, M. A., Ward, C., Luo, Z., Liu, C., Li, Y., Lai, Y., et al. (2022). Rolling back human pluripotent stem cells to an eight-cell embryo-like stage. Nature 605, 315–324. doi:10.1038/s41586-022-04625-0
Mcdonald, A. C., Biechele, S., Rossant, J., and Stanford, W. L. (2014). Sox17-mediated XEN cell conversion identifies dynamic networks controlling cell-fate decisions in embryo-derived stem cells. Cell Rep. 9, 780–793. doi:10.1016/j.celrep.2014.09.026
Meharwade, T., Joumier, L., Parisotto, M., Huynh, V., Lummertz Da Rocha, E., and Malleshaiah, M. (2023). Cross-activation of FGF, NODAL, and WNT pathways constrains BMP-signaling-mediated induction of the totipotent state in mouse embryonic stem cells. Cell Rep. 42, 112438. doi:10.1016/j.celrep.2023.112438
Meistermann, D., Bruneau, A., Loubersac, S., Reignier, A., Firmin, J., Francois-Campion, V., et al. (2021). Integrated pseudotime analysis of human pre-implantation embryo single-cell transcriptomes reveals the dynamics of lineage specification. Cell Stem Cell 28, 1625–1640 e6. doi:10.1016/j.stem.2021.04.027
Messerschmidt, D. M., and Kemler, R. (2010). Nanog is required for primitive endoderm formation through a non-cell autonomous mechanism. Dev. Biol. 344, 129–137. doi:10.1016/j.ydbio.2010.04.020
Messmer, T., Von Meyenn, F., Savino, A., Santos, F., Mohammed, H., Lun, A. T. L., et al. (2019). Transcriptional heterogeneity in naive and primed human pluripotent stem cells at single-cell resolution. Cell Rep. 26, 815–824. doi:10.1016/j.celrep.2018.12.099
Mischler, A., Karakis, V., Mahinthakumar, J., Carberry, C. K., San Miguel, A., Rager, J. E., et al. (2021). Two distinct trophectoderm lineage stem cells from human pluripotent stem cells. J. Biol. Chem. 296, 100386. doi:10.1016/j.jbc.2021.100386
Mole, M. A., Coorens, T. H. H., Shahbazi, M. N., Weberling, A., Weatherbee, B. a. T., Gantner, C. W., et al. (2021). A single cell characterisation of human embryogenesis identifies pluripotency transitions and putative anterior hypoblast centre. Nat. Commun. 12, 3679. doi:10.1038/s41467-021-23758-w
Morris, S. A., Grewal, S., Barrios, F., Patankar, S. N., Strauss, B., Buttery, L., et al. (2012a). Dynamics of anterior-posterior axis formation in the developing mouse embryo. Nat. Commun. 3, 673. doi:10.1038/ncomms1671
Morris, S. A., Guo, Y., and Zernicka-Goetz, M. (2012b). Developmental plasticity is bound by pluripotency and the Fgf and Wnt signaling pathways. Cell Rep. 2, 756–765. doi:10.1016/j.celrep.2012.08.029
Moya-Jódar, M., Ullate-Agote, A., Barlabé, P., Rodríguez-Madoz, J. R., Abizanda, G., Barreda, C., et al. (2023). Revealing cell populations catching the early stages of human embryo development in naive pluripotent stem cell cultures. Stem Cell Reports 18, 64–80. doi:10.1016/j.stemcr.2022.11.015
Nakamura, T., Fujiwara, K., Saitou, M., and Tsukiyama, T. (2021). Non-human primates as a model for human development. Stem Cell Rep. 16, 1093–1103. doi:10.1016/j.stemcr.2021.03.021
Nakamura, T., Okamoto, I., Sasaki, K., Yabuta, Y., Iwatani, C., Tsuchiya, H., et al. (2016). A developmental coordinate of pluripotency among mice, monkeys and humans. Nature 537, 57–62. doi:10.1038/nature19096
Ng, R. K., Dean, W., Dawson, C., Lucifero, D., Madeja, Z., Reik, W., et al. (2008). Epigenetic restriction of embryonic cell lineage fate by methylation of Elf5. Nat. Cell Biol. 10, 1280–1290. doi:10.1038/ncb1786
Niakan, K. K., and Eggan, K. (2013). Analysis of human embryos from zygote to blastocyst reveals distinct gene expression patterns relative to the mouse. Dev. Biol. 375, 54–64. doi:10.1016/j.ydbio.2012.12.008
Niakan, K. K., Ji, H., Maehr, R., Vokes, S. A., Rodolfa, K. T., Sherwood, R. I., et al. (2010). Sox17 promotes differentiation in mouse embryonic stem cells by directly regulating extraembryonic gene expression and indirectly antagonizing self-renewal. Genes Dev. 24, 312–326. doi:10.1101/gad.1833510
Niakan, K. K., Schrode, N., Cho, L. T., and Hadjantonakis, A. K. (2013). Derivation of extraembryonic endoderm stem (XEN) cells from mouse embryos and embryonic stem cells. Nat. Protoc. 8, 1028–1041. doi:10.1038/nprot.2013.049
Nichols, J., Chambers, I., Taga, T., and Smith, A. (2001). Physiological rationale for responsiveness of mouse embryonic stem cells to gp130 cytokines. Development 128, 2333–2339. doi:10.1242/dev.128.12.2333
Nichols, J., and Smith, A. (2009). Naive and primed pluripotent states. Cell Stem Cell 4, 487–492. doi:10.1016/j.stem.2009.05.015
Nishioka, N., Inoue, K., Adachi, K., Kiyonari, H., Ota, M., Ralston, A., et al. (2009). The Hippo signaling pathway components Lats and Yap pattern Tead4 activity to distinguish mouse trophectoderm from inner cell mass. Dev. Cell 16, 398–410. doi:10.1016/j.devcel.2009.02.003
Nishioka, N., Yamamoto, S., Kiyonari, H., Sato, H., Sawada, A., Ota, M., et al. (2008). Tead4 is required for specification of trophectoderm in pre-implantation mouse embryos. Mech. Dev. 125, 270–283. doi:10.1016/j.mod.2007.11.002
Niu, Y., Sun, N., Li, C., Lei, Y., Huang, Z., Wu, J., et al. (2019). Dissecting primate early post-implantation development using long-term in vitro embryo culture. Science 366, eaaw5754. doi:10.1126/science.aaw5754
Niwa, H., Miyazaki, J., and Smith, A. G. (2000). Quantitative expression of Oct-3/4 defines differentiation, dedifferentiation or self-renewal of ES cells. Nat. Genet. 24, 372–376. doi:10.1038/74199
Niwa, H., Toyooka, Y., Shimosato, D., Strumpf, D., Takahashi, K., Yagi, R., et al. (2005). Interaction between Oct3/4 and Cdx2 determines trophectoderm differentiation. Cell 123, 917–929. doi:10.1016/j.cell.2005.08.040
Ohinata, Y., and Tsukiyama, T. (2014). Establishment of trophoblast stem cells under defined culture conditions in mice. PLoS One 9, e107308. doi:10.1371/journal.pone.0107308
Okae, H., Toh, H., Sato, T., Hiura, H., Takahashi, S., Shirane, K., et al. (2018). Derivation of human trophoblast stem cells. Cell Stem Cell 22, 50–63. doi:10.1016/j.stem.2017.11.004
Okubo, T., Rivron, N., Kabata, M., Masaki, H., Kishimoto, K., Semi, K., et al. (2023). Hypoblast from human pluripotent stem cells regulates epiblast development. Nature 626, 357–366. doi:10.1038/s41586-023-06871-2
Olbrich, T., Vega-Sendino, M., Tillo, D., Wu, W., Zolnerowich, N., Pavani, R., et al. (2021). CTCF is a barrier for 2C-like reprogramming. Nat. Commun. 12, 4856. doi:10.1038/s41467-021-25072-x
Oldak, B., Wildschutz, E., Bondarenko, V., Comar, M. Y., Zhao, C., Aguilera-Castrejon, A., et al. (2023). Complete human day 14 post-implantation embryo models from naive ES cells. Nature 622, 562–573. doi:10.1038/s41586-023-06604-5
O'rahilly, R., and Muller, F. (2010). Developmental stages in human embryos: revised and new measurements. Cells Tissues Organs 192, 73–84. doi:10.1159/000289817
Papaioannou, V. E., and Ebert, K. M. (1995). Mouse half embryos: viability and allocation of cells in the blastocyst. Dev. Dyn. 203, 393–398. doi:10.1002/aja.1002030402
Papaioannou, V. E., Mkandawire, J., and Biggers, J. D. (1989). Development and phenotypic variability of genetically identical half mouse embryos. Development 106, 817–827. doi:10.1242/dev.106.4.817
Pedroza, M., Gassaloglu, S. I., Dias, N., Zhong, L., Hou, T. J., Kretzmer, H., et al. (2023). Self-patterning of human stem cells into post-implantation lineages. Nature 622, 574–583. doi:10.1038/s41586-023-06354-4
Peng, G., Suo, S., Cui, G., Yu, F., Wang, R., Chen, J., et al. (2019). Molecular architecture of lineage allocation and tissue organization in early mouse embryo. Nature 572, 528–532.
Perea-Gomez, A., Shawlot, W., Sasaki, H., Behringer, R. R., and Ang, S. (1999). HNF3beta and Lim1 interact in the visceral endoderm to regulate primitive streak formation and anterior-posterior polarity in the mouse embryo. Development 126, 4499–4511. doi:10.1242/dev.126.20.4499
Perea-Gomez, A., Vella, F. D., Shawlot, W., Oulad-Abdelghani, M., Chazaud, C., Meno, C., et al. (2002). Nodal antagonists in the anterior visceral endoderm prevent the formation of multiple primitive streaks. Dev. Cell 3, 745–756. doi:10.1016/s1534-5807(02)00321-0
Petropoulos, S., Edsgard, D., Reinius, B., Deng, Q., Panula, S. P., Codeluppi, S., et al. (2016). Single-cell RNA-seq reveals lineage and X chromosome dynamics in human preimplantation embryos. Cell 165, 1012–1026. doi:10.1016/j.cell.2016.03.023
Pham, T. X. A., Panda, A., Kagawa, H., To, S. K., Ertekin, C., Georgolopoulos, G., et al. (2022). Modeling human extraembryonic mesoderm cells using naive pluripotent stem cells. Cell Stem Cell 29, 1346–1365 e10. doi:10.1016/j.stem.2022.08.001
Pienkowski, M., Solter, D., and Koprowski, H. (1974). Early mouse embryos: growth and differentiation in vitro. Exp. Cell Res. 85, 424–428. doi:10.1016/0014-4827(74)90145-1
Piotrowska-Nitsche, K., Perea-Gomez, A., Haraguchi, S., and Zernicka-Goetz, M. (2005). Four-cell stage mouse blastomeres have different developmental properties. Development 132, 479–490. doi:10.1242/dev.01602
Posfai, E., Petropoulos, S., De Barros, F. R. O., Schell, J. P., Jurisica, I., Sandberg, R., et al. (2017). Position- and Hippo signaling-dependent plasticity during lineage segregation in the early mouse embryo. Elife 6, e22906. doi:10.7554/eLife.22906
Posfai, E., Schell, J. P., Janiszewski, A., Rovic, I., Murray, A., Bradshaw, B., et al. (2021). Evaluating totipotency using criteria of increasing stringency. Nat. Cell Biol. 23, 49–60. doi:10.1038/s41556-020-00609-2
Qin, H., Hejna, M., Liu, Y., Percharde, M., Wossidlo, M., Blouin, L., et al. (2016). YAP induces human naive pluripotency. Cell Rep. 14, 2301–2312. doi:10.1016/j.celrep.2016.02.036
Qu, X. B., Pan, J., Zhang, C., and Huang, S. Y. (2008). Sox17 facilitates the differentiation of mouse embryonic stem cells into primitive and definitive endoderm in vitro. Dev. Growth Differ. 50, 585–593. doi:10.1111/j.1440-169x.2008.01056.x
Rahbaran, M., Tayefeh, A. R., and Heidari, F. (2022). The effects of embryo splitting on Cdx2, Sox2, Oct4, and Nanog gene expression in mouse blastocysts. Iran. J. Vet. Res. 23, 331–336. doi:10.22099/IJVR.2022.42487.6172
Ralston, A., Cox, B. J., Nishioka, N., Sasaki, H., Chea, E., Rugg-Gunn, P., et al. (2010). Gata3 regulates trophoblast development downstream of Tead4 and in parallel to Cdx2. Development 137, 395–403. doi:10.1242/dev.038828
Ralston, A., and Rossant, J. (2008). Cdx2 acts downstream of cell polarization to cell-autonomously promote trophectoderm fate in the early mouse embryo. Dev. Biol. 313, 614–629. doi:10.1016/j.ydbio.2007.10.054
Regin, M., Essahib, W., Demtschenko, A., Dewandre, D., David, L., Gerri, C., et al. (2023). Lineage segregation in human pre-implantation embryos is specified by YAP1 and TEAD1. Lineage 38, 1484–1498. doi:10.1093/humrep/dead107
Rivera-Perez, J. A., and Magnuson, T. (2005). Primitive streak formation in mice is preceded by localized activation of Brachyury and Wnt3. Dev. Biol. 288, 363–371. doi:10.1016/j.ydbio.2005.09.012
Rivron, N. C., Frias-Aldeguer, J., Vrij, E. J., Boisset, J. C., Korving, J., Vivie, J., et al. (2018). Blastocyst-like structures generated solely from stem cells. Nature 557, 106–111. doi:10.1038/s41586-018-0051-0
Rodriguez-Terrones, D., Gaume, X., Ishiuchi, T., Weiss, A., Kopp, A., Kruse, K., et al. (2018). A molecular roadmap for the emergence of early-embryonic-like cells in culture. Nat. Genet. 50, 106–119. doi:10.1038/s41588-017-0016-5
Rodriguez-Terrones, D., Hartleben, G., Gaume, X., Eid, A., Guthmann, M., Iturbide, A., et al. (2020). A distinct metabolic state arises during the emergence of 2-cell-like cells. EMBO Rep. 21, e48354. doi:10.15252/embr.201948354
Roode, M., Blair, K., Snell, P., Elder, K., Marchant, S., Smith, A., et al. (2012). Human hypoblast formation is not dependent on FGF signalling. Dev. Biol. 361, 358–363. doi:10.1016/j.ydbio.2011.10.030
Rossant, J. (1976). Postimplantation development of blastomeres isolated from 4- and 8-cell mouse eggs. J. Embryol. Exp. Morphol. 36, 283–290. doi:10.1242/dev.36.2.283
Rossant, J., and Lis, W. T. (1979). Potential of isolated mouse inner cell masses to form trophectoderm derivatives in vivo. Dev. Biol. 70, 255–261. doi:10.1016/0012-1606(79)90022-8
Rossant, J., and Tam, P. P. L. (2017). New insights into early human development: lessons for stem cell derivation and differentiation. Cell Stem Cell 20, 18–28. doi:10.1016/j.stem.2016.12.004
Rossant, J., and Tam, P. P. L. (2021). Opportunities and challenges with stem cell-based embryo models. Stem Cell Reports 16, 1031–1038.
Rossant, J., and Tam, P. P. L. (2022). Early human embryonic development: blastocyst formation to gastrulation. Dev. Cell 57, 152–165. doi:10.1016/j.devcel.2021.12.022
Rostovskaya, M., Stirparo, G. G., and Smith, A. (2019). Capacitation of human naive pluripotent stem cells for multi-lineage differentiation. Development 146, dev172916. doi:10.1242/dev.172916
Sampath Kumar, A., Tian, L., Bolondi, A., Hernandez, A. A., Stickels, R., Kretzmer, H., et al. (2023). Spatiotemporal transcriptomic maps of whole mouse embryos at the onset of organogenesis. Nat. Genet. 55, 1176–1185.
Sasaki, K., Nakamura, T., Okamoto, I., Yabuta, Y., Iwatani, C., Tsuchiya, H., et al. (2016). The germ cell fate of cynomolgus monkeys is specified in the nascent amnion. Dev. Cell 39, 169–185. doi:10.1016/j.devcel.2016.09.007
Schultz, R. M. (1993). Regulation of zygotic gene activation in the mouse. Bioessays 15, 531–538. doi:10.1002/bies.950150806
Shahbazi, M. N., Jedrusik, A., Vuoristo, S., Recher, G., Hupalowska, A., Bolton, V., et al. (2016). Self-organization of the human embryo in the absence of maternal tissues. Nat. Cell Biol. 18, 700–708. doi:10.1038/ncb3347
Shao, Y., Taniguchi, K., Gurdziel, K., Townshend, R. F., Xue, X., Yong, K. M. A., et al. (2017a). Self-organized amniogenesis by human pluripotent stem cells in a biomimetic implantation-like niche. Nat. Mater 16, 419–425. doi:10.1038/nmat4829
Shao, Y., Taniguchi, K., Townshend, R. F., Miki, T., Gumucio, D. L., and Fu, J. (2017b). A pluripotent stem cell-based model for post-implantation human amniotic sac development. Nat. Commun. 8, 208. doi:10.1038/s41467-017-00236-w
Shen, H., Yang, M., Li, S., Zhang, J., Peng, B., Wang, C., et al. (2021). Mouse totipotent stem cells captured and maintained through spliceosomal repression. Cell 184, 2843–2859 e20. doi:10.1016/j.cell.2021.04.020
Shibata, S., Endo, S., Nagai, L. a. E., E, H. K., Oike, A., Kobayashi, N., et al. (2024). Modeling embryo-endometrial interface recapitulating human embryo implantation. Sci. Adv. 10, eadi4819. doi:10.1126/sciadv.adi4819
Shimoda, M., Kanai-Azuma, M., Hara, K., Miyazaki, S., Kanai, Y., Monden, M., et al. (2007). Sox17 plays a substantial role in late-stage differentiation of the extraembryonic endoderm in vitro. J. Cell Sci. 120, 3859–3869. doi:10.1242/jcs.007856
Shimosato, D., Shiki, M., and Niwa, H. (2007). Extra-embryonic endoderm cells derived from ES cells induced by GATA factors acquire the character of XEN cells. BMC Dev. Biol. 7, 80. doi:10.1186/1471-213X-7-80
Silva, J., Nichols, J., Theunissen, T. W., Guo, G., Van Oosten, A. L., Barrandon, O., et al. (2009). Nanog is the gateway to the pluripotent ground state. Cell 138, 722–737. doi:10.1016/j.cell.2009.07.039
Sotomaru, Y., Kato, Y., and Tsunoda, Y. (1998). Production of monozygotic twins after freezing and thawing of bisected mouse embryos. Cryobiology 37, 139–145. doi:10.1006/cryo.1998.2111
Sozen, B., Amadei, G., Cox, A., Wang, R., Na, E., Czukiewska, S., et al. (2018). Self-assembly of embryonic and two extra-embryonic stem cell types into gastrulating embryo-like structures. Nat. Cell Biol. 20, 979–989. doi:10.1038/s41556-018-0147-7
Sozen, B., Cox, A. L., De Jonghe, J., Bao, M., Hollfelder, F., Glover, D. M., et al. (2019). Self-organization of mouse stem cells into an extended potential blastoid. Dev. Cell 51, 698–712. doi:10.1016/j.devcel.2019.11.014
Sozen, B., Jorgensen, V., Weatherbee, B. a. T., Chen, S., Zhu, M., and Zernicka-Goetz, M. (2021). Reconstructing aspects of human embryogenesis with pluripotent stem cells. Nat. Commun. 12, 5550. doi:10.1038/s41467-021-25853-4
Spindle, A. I. (1978). Trophoblast regeneration by inner cell masses isolated from cultured mouse embryos. J. Exp. Zool. 203, 483–489. doi:10.1002/jez.1402030315
Srivatsan, S. R., Regier, M. C., Barkan, E., Franks, J. M., Packer, J. S., Grosjean, P., et al. (2021). Embryo-scale, single-cell spatial transcriptomics. Science 373, 111–117.
Stamatiadis, P., Cosemans, G., Boel, A., Menten, B., De Sutter, P., Stoop, D., et al. (2022). TEAD4 regulates trophectoderm differentiation upstream of CDX2 in a GATA3-independent manner in the human preimplantation embryo. Hum. Reprod. 37, 1760–1773. doi:10.1093/humrep/deac138
Stirparo, G. G., Boroviak, T., Guo, G., Nichols, J., Smith, A., and Bertone, P. (2018). Integrated analysis of single-cell embryo data yields a unified transcriptome signature for the human pre-implantation epiblast. Development 145, dev158501. doi:10.1242/dev.158501
Strumpf, D., Mao, C. A., Yamanaka, Y., Ralston, A., Chawengsaksophak, K., Beck, F., et al. (2005). Cdx2 is required for correct cell fate specification and differentiation of trophectoderm in the mouse blastocyst. Development 132, 2093–2102. doi:10.1242/dev.01801
Sunde, A., Brison, D., Dumoulin, J., Harper, J., Lundin, K., Magli, M. C., et al. (2016). Time to take human embryo culture seriously. Hum. Reprod. 31, 2174–2182. doi:10.1093/humrep/dew157
Suwinska, A., Czolowska, R., Ozdzenski, W., and Tarkowski, A. K. (2008). Blastomeres of the mouse embryo lose totipotency after the fifth cleavage division: expression of Cdx2 and Oct4 and developmental potential of inner and outer blastomeres of 16- and 32-cell embryos. Dev. Biol. 322, 133–144. doi:10.1016/j.ydbio.2008.07.019
Tachi, C. (1992). Partial characterization of macromolecular components in fetal bovine serum required for development of mouse blastocysts cultured in vitro: (blastocysts/culture/in vitro/fetal bovine serum/mouse). Dev. Growth Differ. 34, 69–77. doi:10.1111/j.1440-169X.1992.00069.x
Takashima, Y., Guo, G., Loos, R., Nichols, J., Ficz, G., Krueger, F., et al. (2014). Resetting transcription factor control circuitry toward ground-state pluripotency in human. Cell 158, 1254–1269. doi:10.1016/j.cell.2014.08.029
Tanaka, S., Kunath, T., Hadjantonakis, A. K., Nagy, A., and Rossant, J. (1998). Promotion of trophoblast stem cell proliferation by FGF4. Science 282, 2072–2075. doi:10.1126/science.282.5396.2072
Tarazi, S., Aguilera-Castrejon, A., Joubran, C., Ghanem, N., Ashouokhi, S., Roncato, F., et al. (2022). Post-gastrulation synthetic embryos generated ex utero from mouse naive ESCs. Cell 185, 3290–3306 e25. doi:10.1016/j.cell.2022.07.028
Tarkowski, A. K. (1959). Experiments on the development of isolated blastomers of mouse eggs. Nature 184, 1286–1287. doi:10.1038/1841286a0
Tarkowski, A. K., and Wroblewska, J. (1967). Development of blastomeres of mouse eggs isolated at the 4- and 8-cell stage. J. Embryol. Exp. Morphol. 18, 155–180. doi:10.1242/dev.18.1.155
Taubenschmid-Stowers, J., Rostovskaya, M., Santos, F., Ljung, S., Argelaguet, R., Krueger, F., et al. (2022). 8C-like cells capture the human zygotic genome activation program in vitro. Cell Stem Cell 29, 449–459 e6. doi:10.1016/j.stem.2022.01.014
Tesar, P. J., Chenoweth, J. G., Brook, F. A., Davies, T. J., Evans, E. P., Mack, D. L., et al. (2007). New cell lines from mouse epiblast share defining features with human embryonic stem cells. Nature 448, 196–199. doi:10.1038/nature05972
Theunissen, T. W., Powell, B. E., Wang, H., Mitalipova, M., Faddah, D. A., Reddy, J., et al. (2014). Systematic identification of culture conditions for induction and maintenance of naive human pluripotency. Cell Stem Cell 15, 471–487. doi:10.1016/j.stem.2014.07.002
Thomas, P., and Beddington, R. (1996). Anterior primitive endoderm may be responsible for patterning the anterior neural plate in the mouse embryo. Curr. Biol. 6, 1487–1496. doi:10.1016/s0960-9822(96)00753-1
Thomas, P. Q., Brown, A., and Beddington, R. S. (1998). Hex: a homeobox gene revealing peri-implantation asymmetry in the mouse embryo and an early transient marker of endothelial cell precursors. Development 125, 85–94. doi:10.1242/dev.125.1.85
Thomson, J. A., Itskovitz-Eldor, J., Shapiro, S. S., Waknitz, M. A., Swiergiel, J. J., Marshall, V. S., et al. (1998). Embryonic stem cell lines derived from human blastocysts. Science 282, 1145–1147. doi:10.1126/science.282.5391.1145
Torres-Padilla, M. E., Parfitt, D. E., Kouzarides, T., and Zernicka-Goetz, M. (2007). Histone arginine methylation regulates pluripotency in the early mouse embryo. Nature 445, 214–218. doi:10.1038/nature05458
Tsunoda, Y., and Mclaren, A. (1983). Effect of various procedures on the viability of mouse embryos containing half the normal number of blastomeres. J. Reprod. Fertil. 69, 315–322. doi:10.1530/jrf.0.0690315
Tu, Z., Bi, Y., Zhu, X., Liu, W., Hu, J., Wu, L., et al. (2023). Modeling human pregastrulation development by 3D culture of blastoids generated from primed-to-naive transitioning intermediates. Protein Cell 14, 337–349. doi:10.1093/procel/pwac041
Tyser, R. C. V., Mahammadov, E., Nakanoh, S., Vallier, L., Scialdone, A., Srinivas, S., et al. (2021). Single-cell transcriptomic characterization of a gastrulating human embryo. Nature 600, 285–289.
Viukov, S., Shani, T., Bayerl, J., Aguilera-Castrejon, A., Oldak, B., Sheban, D., et al. (2022). Human primed and naive PSCs are both able to differentiate into trophoblast stem cells. Stem Cell Rep. 17, 2484–2500. doi:10.1016/j.stemcr.2022.09.008
Vrij, E. J., Scholte Op Reimer, Y. S., Roa Fuentes, L., Misteli Guerreiro, I., Holzmann, V., Frias Aldeguer, J., et al. (2022). A pendulum of induction between the epiblast and extra-embryonic endoderm supports post-implantation progression. Development 149, dev192310. doi:10.1242/dev.192310
Wamaitha, S. E., Del Valle, I., Cho, L. T., Wei, Y., Fogarty, N. M., Blakeley, P., et al. (2015). Gata6 potently initiates reprograming of pluripotent and differentiated cells to extraembryonic endoderm stem cells. Genes Dev. 29, 1239–1255. doi:10.1101/gad.257071.114
Wang, M., Kato, Y., and Tsunoda, Y. (1997). Effects of several factors on the monozygotic twin production in the mouse. J. Reproduction Dev. 43, 91–95. doi:10.1262/jrd.43.91
Wang, Y., Na, Q., Li, X., Tee, W. W., Wu, B., and Bao, S. (2021). Retinoic acid induces NELFA-mediated 2C-like state of mouse embryonic stem cells associates with epigenetic modifications and metabolic processes in chemically defined media. Cell Prolif. 54, e13049. doi:10.1111/cpr.13049
Wang, Y., Zhao, C., Hou, Z., Yang, Y., Bi, Y., Wang, H., et al. (2018). Unique molecular events during reprogramming of human somatic cells to induced pluripotent stem cells (iPSCs) at naive state. Elife 7, e29518. doi:10.7554/eLife.29518
Warnock, M. (1985). Moral thinking and government policy: the warnock committee on human embryology. Milbank Mem. Fund. Q. Health Soc. 63, 504–522. doi:10.2307/3349845
Weatherbee, B. a. T., Gantner, C. W., Iwamoto-Stohl, L. K., Daza, R. M., Hamazaki, N., Shendure, J., et al. (2023). Pluripotent stem cell-derived model of the post-implantation human embryo. Nature 622, 584–593. doi:10.1038/s41586-023-06368-y
Weberling, A., and Zernicka-Goetz, M. (2021). Trophectoderm mechanics direct epiblast shape upon embryo implantation. Cell Rep. 34, 108655. doi:10.1016/j.celrep.2020.108655
Wei, S., Zou, Q., Lai, S., Zhang, Q., Li, L., Yan, Q., et al. (2016). Conversion of embryonic stem cells into extraembryonic lineages by CRISPR-mediated activators. Sci. Rep. 6, 19648. doi:10.1038/srep19648
Wei, Y., Wang, T., Ma, L., Zhang, Y., Zhao, Y., Lye, K., et al. (2021). Efficient derivation of human trophoblast stem cells from primed pluripotent stem cells. Sci. Adv. 7, eabf4416. doi:10.1126/sciadv.abf4416
Wigger, M., Kisielewska, K., Filimonow, K., Plusa, B., Maleszewski, M., and Suwinska, A. (2017). Plasticity of the inner cell mass in mouse blastocyst is restricted by the activity of FGF/MAPK pathway. Sci. Rep. 7, 15136. doi:10.1038/s41598-017-15427-0
Winnier, G., Blessing, M., Labosky, P. A., and Hogan, B. L. (1995). Bone morphogenetic protein-4 is required for mesoderm formation and patterning in the mouse. Genes Dev. 9, 2105–2116. doi:10.1101/gad.9.17.2105
Xiang, L., Yin, Y., Zheng, Y., Ma, Y., Li, Y., Zhao, Z., et al. (2020). A developmental landscape of 3D-cultured human pre-gastrulation embryos. Nature 577, 537–542. doi:10.1038/s41586-019-1875-y
Xu, R. H., Chen, X., Li, D. S., Li, R., Addicks, G. C., Glennon, C., et al. (2002). BMP4 initiates human embryonic stem cell differentiation to trophoblast. Nat. Biotechnol. 20, 1261–1264. doi:10.1038/nbt761
Xu, Y., Zhang, T., Zhou, Q., Hu, M., Qi, Y., Xue, Y., et al. (2023). A single-cell transcriptome atlas profiles early organogenesis in human embryos. Nat Cell Biol. 25, 604–615.
Xu, Y., Zhao, J., Ren, Y., Wang, X., Lyu, Y., Xie, B., et al. (2022). Derivation of totipotent-like stem cells with blastocyst-like structure forming potential. Cell Res. 32, 513–529. doi:10.1038/s41422-022-00668-0
Yagi, R., Kohn, M. J., Karavanova, I., Kaneko, K. J., Vullhorst, D., Depamphilis, M. L., et al. (2007). Transcription factor TEAD4 specifies the trophectoderm lineage at the beginning of mammalian development. Development 134, 3827–3836. doi:10.1242/dev.010223
Yamamoto, M., Saijoh, Y., Perea-Gomez, A., Shawlot, W., Behringer, R. R., Ang, S. L., et al. (2004). Nodal antagonists regulate formation of the anteroposterior axis of the mouse embryo. Nature 428, 387–392. doi:10.1038/nature02418
Yamanaka, Y., Lanner, F., and Rossant, J. (2010). FGF signal-dependent segregation of primitive endoderm and epiblast in the mouse blastocyst. Development 137, 715–724. doi:10.1242/dev.043471
Yan, L., Yang, M., Guo, H., Yang, L., Wu, J., Li, R., et al. (2013). Single-cell RNA-Seq profiling of human preimplantation embryos and embryonic stem cells. Nat. Struct. Mol. Biol. 20, 1131–1139.
Yan, Y. L., Zhang, C., Hao, J., Wang, X. L., Ming, J., Mi, L., et al. (2019). DPPA2/4 and SUMO E3 ligase PIAS4 opposingly regulate zygotic transcriptional program. PLoS Biol. 17, e3000324. doi:10.1371/journal.pbio.3000324
Yanagida, A., Spindlow, D., Nichols, J., Dattani, A., Smith, A., and Guo, G. (2021). Naive stem cell blastocyst model captures human embryo lineage segregation. Cell Stem Cell 28, 1016–1022 e4. doi:10.1016/j.stem.2021.04.031
Yang, J., Ryan, D. J., Lan, G., Zou, X., and Liu, P. (2019). In vitro establishment of expanded-potential stem cells from mouse pre-implantation embryos or embryonic stem cells. Nat. Protoc. 14, 350–378. doi:10.1038/s41596-018-0096-4
Yang, J., Ryan, D. J., Wang, W., Tsang, J. C., Lan, G., Masaki, H., et al. (2017a). Establishment of mouse expanded potential stem cells. Nature 550, 393–397. doi:10.1038/nature24052
Yang, M., Yu, H., Yu, X., Liang, S., Hu, Y., Luo, Y., et al. (2022). Chemical-induced chromatin remodeling reprograms mouse ESCs to totipotent-like stem cells. Cell Stem Cell 29, 400–418 e13. doi:10.1016/j.stem.2022.01.010
Yang, R., Goedel, A., Kang, Y., Si, C., Chu, C., Zheng, Y., et al. (2021). Amnion signals are essential for mesoderm formation in primates. Nat. Commun. 12, 5126. doi:10.1038/s41467-021-25186-2
Yang, Y., Liu, B., Xu, J., Wang, J., Wu, J., Shi, C., et al. (2017b). Derivation of pluripotent stem cells with in vivo embryonic and extraembryonic potency. Cell 169, 243–257. doi:10.1016/j.cell.2017.02.005
Yoshihara, M., Kirjanov, I., Nykanen, S., Sokka, J., Weltner, J., Lundin, K., et al. (2022). Transient DUX4 expression in human embryonic stem cells induces blastomere-like expression program that is marked by SLC34A2. Stem Cell Rep. 17, 1743–1756. doi:10.1016/j.stemcr.2022.06.002
Yu, L., Logsdon, D., Pinzon-Arteaga, C. A., Duan, J., Ezashi, T., Wei, Y., et al. (2023). Large-scale production of human blastoids amenable to modeling blastocyst development and maternal-fetal cross talk. Cell Stem Cell 30, 1246–1261 e9. doi:10.1016/j.stem.2023.08.002
Yu, L., Wei, Y., Duan, J., Schmitz, D. A., Sakurai, M., Wang, L., et al. (2021). Blastocyst-like structures generated from human pluripotent stem cells. Nature 591, 620–626. doi:10.1038/s41586-021-03356-y
Yu, X., Liang, S., Chen, M., Yu, H., Li, R., Qu, Y., et al. (2022). Recapitulating early human development with 8C-like cells. Cell Rep. 39, 110994. doi:10.1016/j.celrep.2022.110994
Yuan, G., Wang, J., Liu, Z., Chen, M., Zhu, P., Zhang, H., et al. (2023). Establishment of a novel non-integrated human pluripotent stem cell-based gastruloid model. bioRxiv. doi:10.1101/2023.06.28.546720
Zeng, B., Liu, Z., Lu, Y., Zhong, S., Qin, S., Huang, L., et al. (2023). The single-cell and spatial transcriptional landscape of human gastrulation and early brain development. Cell Stem Cell 30, 851–866.
Zhai, J., Xiao, Z., Wang, Y., and Wang, H. (2022). Human embryonic development: from peri-implantation to gastrulation. Trends Cell Biol. 32, 18–29. doi:10.1016/j.tcb.2021.07.008
Zhai, J., Xu, Y., Wan, H., Yan, R., Guo, J., Skory, R., et al. (2023). Neurulation of the cynomolgus monkey embryo achieved from 3D blastocyst culture. Cell 186, 2078–2091 e18. doi:10.1016/j.cell.2023.04.019
Zhang, X., Cao, Q., Rajachandran, S., Grow, E. J., Evans, M., Chen, H., et al. (2023). Dissecting mammalian reproduction with spatial transcriptomics. Hum. Reprod. Update 29, 794–810.
Zhao, C., Reyes, A. P., Schell, J. P., Weltner, J., Ortega, N. M., Zheng, Y., et al. (2024). A comprehensive human embryogenesis reference tool using single-cell RNA-sequencing data. bioRxiv. doi:10.1101/2021.05.07.442980
Zheng, Y., Xue, X., Shao, Y., Wang, S., Esfahani, S. N., Li, Z., et al. (2019). Controlled modelling of human epiblast and amnion development using stem cells. Nature 573, 421–425. doi:10.1038/s41586-019-1535-2
Zhong, Y., Choi, T., Kim, M., Jung, K. H., Chai, Y. G., and Binas, B. (2018). Isolation of primitive mouse extraembryonic endoderm (pXEN) stem cell lines. Stem Cell Res. 30, 100–112. doi:10.1016/j.scr.2018.05.008
Zhou, F., Wang, R., Yuan, P., Ren, Y., Mao, Y., Li, R., et al. (2019). Reconstituting the transcriptome and DNA methylome landscapes of human implantation. Nature 572, 660–664. doi:10.1038/s41586-019-1500-0
Zhou, R., Yang, G., Zhang, Y., and Wang, Y. (2023). Spatial transcriptomics in development and disease. Mol. Biomed. 4, 32
Zhu, M., Shahbazi, M., Martin, A., Zhang, C., Sozen, B., Borsos, M., et al. (2021). Human embryo polarization requires PLC signaling to mediate trophectoderm specification. Elife 10, e65068. doi:10.7554/eLife.65068
Keywords: synthetic embryo models, stem cell-based embryo models, mouse embryogenesis, human embryogenesis, embryonic development, developmental biology, stem cells
Citation: Dupont C (2024) A comprehensive review: synergizing stem cell and embryonic development knowledge in mouse and human integrated stem cell-based embryo models. Front. Cell Dev. Biol. 12:1386739. doi: 10.3389/fcell.2024.1386739
Received: 16 February 2024; Accepted: 05 April 2024;
Published: 22 April 2024.
Edited by:
Michael B. Morris, The University of Sydney, AustraliaReviewed by:
Jiawei Sun, Massachusetts General Hospital and Harvard Medical School, United StatesGuang Hu, National Institute of Environmental Health Sciences (NIH), United States
Copyright © 2024 Dupont. This is an open-access article distributed under the terms of the Creative Commons Attribution License (CC BY). The use, distribution or reproduction in other forums is permitted, provided the original author(s) and the copyright owner(s) are credited and that the original publication in this journal is cited, in accordance with accepted academic practice. No use, distribution or reproduction is permitted which does not comply with these terms.
*Correspondence: Cathérine Dupont, Yy5kdXBvbnRAZXJhc211c21jLm5s