- 1Neuroscience Center of Excellence, School of Medicine, L.S.U. Health, New Orleans, LA, United States
- 2Tongji University, Shanghai, China
- 3Biostatistics, Department of Environmental Health, University of Cincinnati College of Medicine, Cincinnati, OH, United States
- 4Tongji Medical College, Huazhong University of Science and Technology, Wuhan, Hubei, China
- 5Tulane University School of Medicine, Center for Stem Cell Research and Regenerative Medicine, New Orleans, LA, United States
- 6Department of Ophthalmology, School of Medicine, L.S.U. Health, New Orleans, LA, United States
Introduction: Stem cells can be used to treat diabetic mellitus and complications. ω3-docosahexaenoic acid (DHA) derived lipid mediators are inflammation-resolving and protective. This study found novel DHA-derived 7S,14R-dihydroxy-4Z,8E,10Z,12E,16Z,19Z-docosahexaenoic acid (7S,14R-diHDHA), a maresin-1 stereoisomer biosynthesized by leukocytes and related enzymes. Moreover, 7S,14R-diHDHA can enhance mesenchymal stem cell (MSC) functions in the amelioration of diabetic mellitus and retinal pericyte loss in diabetic db/db mice.
Methods: MSCs treated with 7S,14R-diHDHA were delivered into db/db mice i.v. every 5 days for 35 days.
Results: Blood glucose levels in diabetic mice were lowered by 7S,14R-diHDHA-treated MSCs compared to control and untreated MSC groups, accompanied by improved glucose tolerance and higher blood insulin levels. 7S,14R-diHDHA-treated MSCs increased insulin+ β-cell ratio and decreased glucogan+ α-cell ratio in islets, as well as reduced macrophages in pancreas. 7S,14R-diHDHA induced MSC functions in promoting MIN6 β-cell viability and insulin secretion. 7S,14R-diHDHA induced MSC paracrine functions by increasing the generation of hepatocyte growth factor and vascular endothelial growth factor. Furthermore, 7S,14R-diHDHA enhanced MSC functions to ameliorate diabetes-caused pericyte loss in diabetic retinopathy by increasing their density in retina in db/db mice.
Discussion: Our findings provide a novel strategy for improving therapy for diabetes and diabetic retinopathy using 7S,14R-diHDHA-primed MSCs.
1 Introduction
Type 2 diabetes, accounting for > 90% of diabetes worldwide, is a major public health problem and places a severe economic burden on healthcare systems. It is characterized by insulin resistance in peripheral tissues. As long as pancreatic β-cells are able to compensate for insulin resistance by enhancing insulin secretion and increasing β-cell mass, euglycemia can be maintained. However, when they are unable to meet the body’s demand for insulin because of genetic defects and/or exogenous insults, diabetes mellitus ensues (Gunton et al., 2005; Florez, 2008; Cerf, 2013; Dludla et al., 2023). Chronic hyperglycemia leads to glucose toxicity and worsening of impaired insulin secretion due to the overworking of pancreatic β-cells, which results in a decreased ability to secrete insulin. Hyperglycemia can also cause oxidative stress, which influences β-cell survival (Tiedge et al., 1997; Eguchi et al., 2021). Reduced β-cell function has been observed in both obese and lean type 2 diabetic humans and in diabetic rodent models (Pick et al., 1998; Butler et al., 2003; Puff et al., 2011; Inaishi and Saisho, 2020; Miranda et al., 2020). Therefore, prevention of the progression of pancreatic β-cell dysfunction in patients with diabetes mellitus should be a key in the long-term management of diabetes mellitus.
Hypoglycemic agents can significantly reduce the development of diabetic complications (1998; Want, 2008; Group et al., 2022); however, many of them cause adverse effects and sometimes result in progressive deterioration in β-cell function (Efanova et al., 1998; Hakobyan et al., 2011; Dalle et al., 2023). Recently, some strategies using antioxidative treatment have been shown to ameliorate diabetic mellitus. An angiotensin II type 1 receptor blocker was shown to decrease oxidative stress markers in β-cells, improve abnormalities of pancreatic islets in diabetes db/db mice (Shao et al., 2006; Chen et al., 2018; Lee et al., 2023). Also, increasing levels of the antioxidant enzyme, glutathione peroxidase-1, specifically in β-cells, has been demonstrated to protect them against the adverse effects of chronic hyperglycemia in db/db mice (Harmon et al., 2009; Robertson, 2023).
Multiple differentiation capacity, paracrine functions, and immunomodulation action make the marrow mesenchymal stem cells (MSCs) a good candidate for prevention or cure of diabetic mellitus and complications (Vija et al., 2009; Bernardi et al., 2012; Miklosz and Chabowski, 2023; Aglan et al., 2024). Transplantation of bone marrow–derived cells increased levels of serum insulin and reduced blood glucose levels in hyperglycemic mice with streptozotocin-induced pancreatic tissue damage, which improved the metabolic state and survival of recipients (Hess et al., 2003; Zhoujun et al., 2023). Studies using rat MSCs (Boumaza et al., 2009; Yousef et al., 2022) or human bone marrow–derived multipotent stromal cells (Lee et al., 2006; Gabr et al., 2013; Carlsson et al., 2023) have shown similar results of enhanced insulin secretion and repair of pancreatic islets after streptozotocin treatment in rodents. Patients with type 2 diabetes received an intrapancreatic autologous bone marrow stem cell infusion; after 1 year, these patients showed improved metabolic control and reduced insulin requirements (Wang et al., 2011; Mathur et al., 2023). However, diabetic hyperglycemia and concomitant oxidative stress damage DNA, proteins, and lipids in various tissues, resulting in dysfunction of cells and enzyme systems. When MSCs are transplanted into diabetes, the inhospitable environment might damage MSC functions (Kornicka et al., 2018; Yin et al., 2021).
Diabetic retinopathy, a secondary microvascular complication of diabetes mellitus, is the leading cause of blindness in the United States among individuals aged 20 to 64 (Bhavsar, 2006; Gange et al., 2021). A persistent increase in blood glucose levels shunts excess glucose into the aldose reductase pathway in certain tissues, which converts sugars into alcohol (e.g., glucose into sorbitol, galactose to dulcitol). Pericytes of retinal capillaries seem to be affected by this increased level of sorbitol. Thus, the loss of function of pericytes results in weakness and eventual vascular outpouching of capillary walls, resulting in microaneurysms (Bhavsar, 2006; Eshaq et al., 2017).
Docosahexaenoic acid (DHA) is an essential omega-3 fatty acid. Resolvin D series, neuroprotectins, and maresins are potent anti-inflammatory lipid mediators (LMs) naturally generated from DHA during inflammation and/or resolution of inflammation (Serhan et al., 2002; Hong et al., 2003; Marcheselli et al., 2003; Antony et al., 2010; Spite and Serhan, 2010; Cortina et al., 2013; Pham and Bazan, 2021). Neuroprotectin D1 and other DHA derived lipid mediators have been reported to protect retinal epithelial cells and neuronal-glial cells from apoptosis induced by oxidative stress (Hong et al., 2003; Mukherjee et al., 2004; Zhao et al., 2011; Hong et al., 2014b), promote neuronal functions and prevent neural degeneration (Bazan, 2006; Asatryan and Bazan, 2017; Pham et al., 2017; Emre et al., 2021; Emre et al., 2022). We identified a DHA-derived LM, 14S,21R-diHDHA, which promotes wound healing (Lu et al., 2010; Tian et al., 2011a), inhibits MSC apoptosis, and enhances MSC functions to ameliorate acute kidney injury (Tian et al., 2012). In 2009, Serhan et al. reported their discovery of maresin 1 (7R,14S-dihydroxy-4Z,8E,10E,12Z,16Z,19Z-DHA and its inflammation-resolving functions (Serhan et al., 2009). Inspired by this discovery, we identified and prepared a novel maresin 1 stereoisomer, 7S,14R-dihydroxy-4Z,8E,10Z,12E,16Z,19Z-DHA (7S,14R-diHDHA) from leukocytes and related enzyme incubations, as we predicted the therapeutic significance that the biofunction of maresin 1 is conserved in this stereoisomer and that it could promote MSC amelioration of multiple diseases and injuries. Maresins promote the beneficial bioactions of stem cells in the treatment of diseases (Cianci et al., 2016; Albuquerque-Souza et al., 2020; Martinez-Fernandez et al., 2021; AlZahrani et al., 2022; Rakian et al., 2022; Sugimoto et al., 2022; Yao et al., 2022; Walker et al., 2024). Maresin 1 regulates insulin signaling in human adipocytes as well as in adipose tissue and muscle of lean and obese mice (Martinez-Fernandez et al., 2021). It inhibits hyperglycemia-induced ferroptosis (Li et al., 2023). DHA preserves visual function by maintaining correct disk morphology in retinal photoreceptor cells (Shindou et al., 2017).
Accordingly, we hypothesized that MSCs can protect pancreas β-cells from the damage of diabetic oxidative stress and ameliorate retinal pericyte loss, and biogenic 7S,14R-diHDHA can induce MSC protective functions. This was further tested to be positive by our studies in vitro and by our experiments using db/db diabetic mouse model in vivo. 7S,14R-diHDHA was formed by the cytochrome P450 enzyme and 5-lipoxygenase (5-LOX) in tandem and by leukocytes. 5-LOX catalyzed the formation of 7S-hydroxyl in DHA and 5-LOX is the major lipoxygenase in leukocytes (el Makhour-Hojeij et al., 1994; Cook-Moreau et al., 2007; Radmark and Samuelsson, 2009; Serhan et al., 2009). 7S,14R-diHDHA induced MSC functions in amelioration of diabetes mellitus in db/db mice by lowering blood sugar and increasing glucose tolerance and blood insulin levels, and decreased the number of macrophages in islets, increasing β-cell viability, density and insulin secretion, and reducing pericyte loss in the retina. 7S,14R-diHDHA can enhance the MSC secretion of trophic growth factors. Overall, pretreatment of MSCs with 7S,14R-diHDHA, or related lipid mediators or structural mimics, may offer a new clinical strategy for improved treatment of diabetic mellitus and retinopathy as well as other diabetic complications.
2 Materials and methods
2.1 Reagents
Racemic ±14-hydroxy-4Z,7Z,10Z,12E,16Z,19Z-DHAs (±14-HDHAs or 14S/R-HDHAs) and 5-lipoxygenase (5-LOX, human recombinant or potato) were supplied by Cayman (Ann Arbor, MI, United States). NaBH4, interleukin (IL)-1β, TNF-α, and Escherichia coli lipopolysaccharide (LPS) were supplied by Sigma-Aldrich (St. Louis, MO, United States).
2.2 Cells
MSCs were isolated from the bone marrow of C57BL/6J mice (8 weeks old, female, Jackson Laboratory), as described in our publications (Tian et al., 2012); more than 95% of isolated MSCs were positive for Sca-1 and CD29 based on flow cytometric analysis (Izadpanah et al., 2006; Tian et al., 2011a; Tian et al., 2012). These MSCs possessed adipogenic and chondrogenic differentiation ability. They were cultured in Dulbecco’s modified Eagle’s medium (DMEM) containing 10% mesenchymal supplement (Stem Cell Technologies, Vancouver, BC, Canada). Cells in passages 7 through 10 were used for the experiments. The Min6 mouse β-cell line (ATCC, Manassas, VA, United States) was cultured in RPMI1640 containing 10% fetal bovine serum (ATCC, Manassas, VA, United States).
2.3 Isolation of human neutrophils, monocytes, and lymphocytes
Human samples were managed following protocols approved by the Institutional Review Boards of our institutes. Established procedures were followed (Serhan and Romano, 1995; Hong et al., 2003). Human peripheral blood supplied by the Blood Center (New Orleans, LA, United States) was from a healthy donor who was unknown to us and who had not been on medication for > 2 weeks prior to donation. We centrifuged the blood in sodium citrate (180 g, 10 min, 23°C); then centrifuged the supernatants (1,100 g, 15 min, 23°C). The plasma (the top layer) was removed. We washed the cell pellets with 7 mM EDTA. Neutrophils and mononuclear cells were separated from blood by a Ficoll-Hypaque gradient. We plated mononuclear cells onto plastic dishes to further separate the cells. The cells adhered to the dishes were monocytes, and nonadhered cells were lymphocytes. Neutrophils, monocytes, and lymphocytes prepared were of > 96% purity. Cell viability was > 95% according to trypan blue exclusion.
2.4 Biogenesis of novel 7S, 14R-DHA
14R-hydroxy-4Z,7Z,10Z,12E,16Z,19Z-DHA (14R-HDHA) was prepared from ±14-HDHAs as described in our previous publication (Hong et al., 2014a) and the section below. We then incubated the 14R-HDHA (10 µg/incubation) with 5-LOX (3 h, 37°C) to identify the biogenic pathway of the novel maresin-1 stereoisomer, 7S, 14R dihydroxyl DHA, which is expected to be produced by 5-LOX with 14R-hydroxyl preserved. A few grains of NaBH4 powder were added at the end of incubation. For cellular generation of 7S,14R-diHDHA, human leukocytes (6 × 106 neutrophils + 5 × 105 monocytes + 3 × 106 lymphocytes) were incubated (20 min, 37°C) in phosphate-buffered saline (PBS) containing 200 μg 14R-HDHA. This ratio of leukocytes resembles the typical leukocyte profile of healthy adults (Lee et al., 2018; Cheng et al., 2022). The cells were then stimulated (37°C, 30 min) with inflammatory factors (10 ng/mL TNFα, 10 ng/mL IL-1β, and 100 ng/mL LPS) as described in our previous publication, to promote the biosynthesis of lipid mediators (Lu et al., 2010; Tian et al., 2011a; Hong et al., 2014a).
2.5 Analysis and isolation of 7S, 14R-diHDHA and of its precursor 14R-HDHA
14R-HDHA (>98% pure) was isolated from purchased ±14-HDHAs using aqueous reversed-phase chiral liquid chromatography (aR chiral LC) coupled to an ultraviolet photo diode array detector and tandem mass spectrometer (aR chiral LC-UV-MS/MS) as we published previously (Lu et al., 2010; Tian et al., 2011a; Hong et al., 2014a). The incubations were extracted and then analyzed or fractionated for 7S,14R-diHDHA. Briefly, A Chiralpak-IA chiral column (150 mm long x 2.1 mm ID x 5 μm, Chiral Tech, West Chester, PA, United States) was used for the aR chiral-LC separation. The mobile phase flowed at 0.2 mL/min, eluted as B (methanol:H2O:acetic acid = 27:73:0.01) from 0 to 1 min, ramped from B:methanol 40:60 to B:methanol 20:80 by 50 min, ramped to methanol by 55 min, and then flowed as methanol. The instrument used included the system of Survey LC-PDA UV-LTQ linear ion trap MS/MS (Thermo Fisher, Waltham, MA, USA) (Lu et al., 2010; Tian et al., 2011a) and the system of an Agilent 1100 LC system (HPLC-PDA, Agilent Technologies, Santa Clara, CA, United States) and a QTRAP 6500+ quadruple-linear trap MS/MS (Sciex.com, Framingham, MA, United States), with electrospray ionization for both systems (Baravkar et al., 2024). The LC-UV effluents were split at a 1:20 ratio, where 1 portion went into a mass spectrometer that monitored the chromatography of 7S,14R-diHDHA and 20 portions were fraction-collected manually for the aR chiral LC peak of 7S,14R-diHDHA. The collected effluents containing 7S,14R-diHDHA were pooled together and evaporated for solvent removal under vacuum or nitrogen gas blow.
2.6 Protocols for the treatment of cells in vitro
MSCs (2 × 105) were treated with 7S,14R-diHDHA (250 nM) for 12 h. The level of VEGF or HGF in supernatant was analyzed using ELISA kits (RayBiotech, Peachtree Corners, GA, United States, and R&D Systems, United States, respectively). Min6 β-cells were cultured to 60%–80% confluence (1.8 × 105) in lower chamber of a 24-transwell plate (8 µm pore) pretreated with polylycine overnight. MSCs (3 × 104) were cultured in the upper chamber of a 24-transwell pretreated with or without 250 nM 7S,14R-diHDHA for 4 h Min6 β-cells and MSCs were cocultured in RPMI1640 for 8 h, and then treated with 100 or 200 µM H2O2 for 10 h. Cell viability was analyzed using the MTT method. Moreover, Min6 β-cells were treated for 4 h with conditioned medium (CM) of MSCs or 7S,14R-diHDHA-treated MSCs.
2.7 Mice
We used 16-week-old female C57BL/6J mice and diabetic db/db mice (BKS.Cg-m+/+leprdb/J, Strain #:000642) (Jackson Lab, Bar Harbor, ME). Animal protocols were approved by the Institutional Animal Care and Use Committee and Institutional Review Board of Louisiana State University Health Sciences Center, New Orleans, and followed the ARRIVE guidelines (Percie du Sert et al., 2020). The studies were conducted in a blinded fashion.
2.8 Treatment procedures in vivo
MSCs were cultured with or without 250 nM 7S,14R-diHDHA for 4 h and then collected trypsinization (0.25% trypsin/0.53 mM ethylenediaminetetraacetic acid; Invitrogen, Carlsbad, CA, United States), similar to the procedures that we used previously (Tian et al., 2012). MSCs (1 × 106) with or without treatment of 7S,14R-diHDHA were infused into 16-week-old db/db mice via tail vein every 5 days for 35 days. During the intervention period, body weight was measured every week. Nonfasting blood glucose was measured using a OneTouch Ultra glucose meter (LifeScan, Milpitas, CA, United States) every week. The intraperitoneal glucose tolerance test (IPGTT) was conducted as follows. After 5 weeks of treatment, mice were injected intraperitoneally with glucose (2 g/kg body weight) after 8 h of fasting. Blood samples were taken at 0, 60, and 120 min from the tail vein, and blood glucose was measured. Sera were collected at 120 min, and insulin concentrations were measured using ELISA using mouse insulin as a standard.
2.9 Histological study
Mice were perfused with PBS at the end of the treatment. Pancreas and eyes were collected and fixed in 4% paraformaldehyde for 2 h, and cryosections (5 µm) were prepared. Sections were blocked with normal goat serum (10% in PBS) for 30 min at room temperature, and then incubated with the following primary antibodies: rabbit antimouse insulin, rabbit antimouse glucagon, rat antimouse F4/80 (Santa Cruz Biotechnology, Dallas, Texas, United States), and rabbit antimouse α-SMA (Millipore, Burlington, MA, United States). Primary antibodies were followed by incubation with the secondary antibodies: Cy3-labeled goat antirat immunoglobulin G (IgG), Cy5-, or fluorescein isothiocyanate (FITC)-labeled goat antirabbit IgG (Invitrogen, Carlsbad, California, United States). Normal rat IgG and rabbit IgG (Santa Cruz Biotechnology, Dallas, Texas, United States) were used as isotype controls. Nuclei were counterstained with Hochest 33342 (Invitrogen, Carlsbad, California, United States). To assess the α cell ratio, the image analysis software NIH ImageJ was used to calculate the pancreatic islet cell number, the insulin+ and glucagon+ cells in the pancreatic islets to determine the percent β-cell count and α-cell count (relative to the pancreatic islets). The macrophage infiltration in the pancreas was assessed using the number of F4/80+ cells per hpf. To assess retinal pericyte loss, retinal sections were stained with an antibody against α-SMA, which specifically marks pericytes in the capillaries. The number of α-SMA+ cells in the inner nuclear layer of the retinas was counted and normalized against the area of the inner nuclear layer.
2.10 Statistical analysis
All data were analyzed using one-way analysis of variance followed by Fisher’s least significant difference post hoc test and expressed as mean ± standard error of the mean. p < 0.05 was considered statistically significant.
3 Results
3.1 Biogenesis of novel 7S,14R-dihydroxy-4Z,8E,10Z,12E,16Z,19Z-DHA
14R-HDHA forms from DHA by P450 enzymatic conversion, as we observed previously (Hong et al., 2014a). We predicted that 5-LOX or 5-LOX rich leukocytes (el Makhour-Hojeij et al., 1994; Cook-Moreau et al., 2007; Radmark and Samuelsson, 2009; Serhan et al., 2009) convert 14R-HDHA to 7S,14R-dihydroxy-4Z,8E,10Z,12E,16Z,19Z-DHA where the chirality of C-14 is preserved as 14R, and the C-7 takes in the hydroxyl at S-configuration based on the well-established knowledge of 5-LOX (el Makhour-Hojeij et al., 1994; Cook-Moreau et al., 2007; Radmark and Samuelsson, 2009; Serhan et al., 2009). This prediction was tested positive as we observed 7S,14R-diHDHA as a single aR-chiral LC peak at 50.6 min (Figure 1A), with its UV spectral triplet possessing a head at 270 nm and two shoulders at 261 and 281 nm representing its conjugated triene (Figure 1B) and its MS/MS spectrum at m/z 359 [molecular mass M-H]- finger-printing its 7S, 14R and double-bond locations (Figure 1C). The MS/MS ions m/z 359 [M-H]-, 341 [M-H-H2O]-, 323 [M-H-2H2O]-, 315 [M-H-CO2], 297 [M-H-H2O-CO2]-, and 279 [M-H-2H2O-CO2]- were consistent with one carboxyl and a molecular weight (M) of 360 Da. The fragment ions m/z 217, 141, 123 [141-H2O]-, and 113 showed a hydroxyl at the 7 position (C7). The fragment ions m/z 221, 203 [221-H2O]-, and 177 [221-CO2]-, demonstrated another hydroxyl at the 14 position (C14). These data and our previous observation on P450 formation of 14R-HDHA (Hong et al., 2014a) support our prediction that P450 or 5-LOX act together, generating 7S,14R-diHDHA.
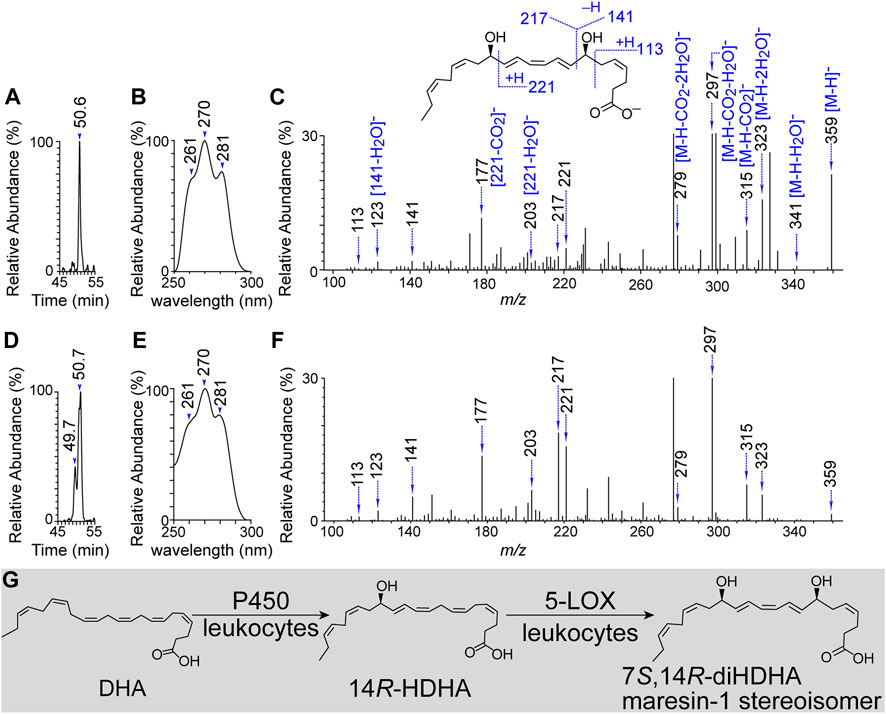
FIGURE 1. Novel 7S,14R-dihydroxy-4Z,8E,10Z,12E,16Z,19Z-DHA produced from 14R-hydroxy DHA by 5-lipoxygenase or activated human leukocytes. (A) Representative aR chiral LC-MS/MS Chromatogram. (B) UV spectrum of the flowing chromatographic peak in (A). (C) MS/MS spectrum and fragment ion interpretation of the chromatographic peak in (A). (D) Representative aR chiral LC-MS/MS Chromatogram. (E) UV spectrum of the flowing chromatographic peak in (D). (F) MS/MS spectrum and fragment ion interpretation of the chromatographic peak in (D). (G) Tentative biogenic pathway for 7S,14R-diHDHAformation. 7S,14R-diHDHA was produced by the incubation of 14R-hydroxy DHA and 5-lipoxygenase (3 h, 37°C) for (A–C). It was also produced by the incubation of human leukocytes (6 × 106 neutrophils + 5 × 105 monocytes + 3 × 106 lymphocytes) in PBS containing 200 μg 14R-HDHA [cultured for 20 min then stimulated by inflammatory factors (10 ng/mL TNFα, 10 ng/mL IL-1β, and 100 ng/mL LPS) for 30 min, 37°C]. The incubation was extracted and analyzed using aR chiral LC-UV-MS/MS as described previously.
Leukocytes are major blood cells that are essential not only in immunity against infection but also in the protection against or repair of organ degeneration and dysfunction caused by injury, diabetic complication, aging, or other adverse conditions. This is demonstrated by leukocyte production of prohealing resolvins, maresins (Serhan et al., 2009), and/or 14,21-diHDHAs (Lu et al., 2010; Tian et al., 2011b; Hellmann et al., 2012). To further test our first hypothesis, we incubated human leukocytes (monocytes + neutrophils + lymphocytes) in 14R-HDHA containing medium as we conducted previously (Tian et al., 2011a), then activated the cells with factors involved in inflammation and organ degeneration. The incubations were then studied using aR chiral LC-UV-MS/MS. 7S,14R-diHDHA was found to be the peak at the chromatographic retention time (RT) of 50.7 min (Figure 1D) with UV and MS/MS spectra (Figures 1E, F, respectively) matching those of 7S,14R-diHDHA generated by 5-LOX from 14R-HDHA (Figure 1). Of note, a small peak at RT 49.7 min was found to be a 7,14-diHDHA because its UV and MS/MS spectra match those of 7S,14R-diHDHA in Figures 1E, F. This 7,14-diHDHA is generated from added 14R-HDHA as purified leukocytes alone without feeding exogenous substrate did not show detectable 7,14-diHDHA; its 14-hydroxyl should be 14R-hydroxyl because the chirality of its 14-hydroxyl is preserved when undergoing such enzymatic conversion. It had RT 49.7 min, shorter than 50.7 min of 7S,14R-diHDHA, thus, its 7-hydroxyl should be 7R because the aR chiral LC RT is shorter for a fatty acid derived R-hydroxyl compound than for its S-epiomer when other structural features are the same (Figure 1A) on the basis of our extensive studies and publications from others (Schneider et al., 2007; Yin et al., 2007). The 7R-hydroxylation is likely to be catalyzed by P450 enzyme(s) in the leukocytes because P450 can catalyze both R and S hydroxylation. The exact mechanism of this unknown aspect is beyond the scope of this study. In brief, 7S,14R-diHDHA was the major product, and 7R,14R-diHDHA was the minor one when feeding leukocytes with 14R-DHA. These results and our previous finding that P450 converts DHA to 14R-HDHA converged to the biogenic pathway depicted in Figure 1G that human leukocytes produced 7S,14R-diHDHA, while cell-possessed P450 and 5-LOX catalyzed the biosynthesis.
3.2 7S,14R-diHDHA enhanced MSC function to regulate nonfasting blood glucose levels
The db/db mice began to exhibit obesity by 5 weeks of age, and body weight increased rapidly with age (Puff et al., 2011). 16-week old db/db mice weighed twice as much as the control C57BL/6J mice (45.3 ± 1.7 vs. 22.3 ± 0.7 g, p < 0.05, Figure 2A). The weight of non-diabetic control mice increased a little from 16 to 21 weeks old (Figure 2A). In addition, treatment with MSCs or 7S,14R-diHDHA-treated MSCs had no influence on weight (Figure 2A). The nonfasting blood glucose levels in db/db mice were much higher than in normal control C57BL/6J mice (640.0 ± 21.4 vs. 120.7 ± 9.3 mg/dL, p < 0.05, Figure 2B). At the end of intervention, the blood glucose level did not change in MSC infusion groups, but infusion of 7S,14R-diHDHA-condictioned MSCs reduced the blood glucose level compared to the untreated MSC group (Figure 2B). Additionally, infusion of 7S,14R-diHDHA-condictioned MSCs reduced the blood glucose level significantly at day 35 compared to day 0. Blood insulin concentration analysis revealed increased blood insulin levels in the MSC group. Moreover, 7S,14R-diHDHA augmented the MSC effect on insulin secretion (Figure 2C), thus demonstrating that 7S,14R-diHDHA improved MSC amelioration of type 2 diabetic mellitus.
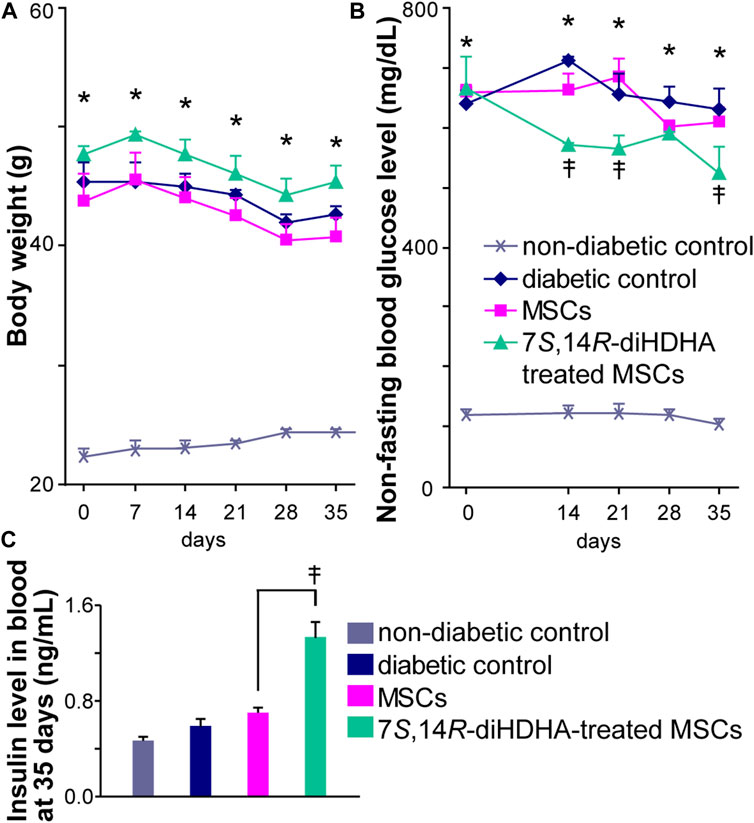
FIGURE 2. 7S,14R-diHDHA enhanced MSCS function to regulate blood glucose levels. C57/BL6J MSCs (1 × 106) with or without treatment of 7S,14R-diHDHA were infused into db/db mice via tail vein every 5 days for 35 days. (A) Body weight. (B) Nonfasting blood glucose levels. (C) Blood insulin levels were measured at the end of treatment (35 days). Data are mean ± SEM, n = 6. *p < 0.05 vs. nondiabetic control, ‡p < 0.05 vs. MSCs alone.
3.3 7S,14R-diHDHA treatment promoted the capacity of MSCs to improve glucose tolerance in db/db mice
IPGTT was performed to determine the effect of the treatments of MSCs and 7S,14R-diHDHA-treated MSCs on glucose tolerance. The blood glucose concentration at 120 min after glucose load was significantly reduced by MSC treatment (1,078.0 ± 23.4 vs. 1,290.0 ± 12.2 mg/dL, p < 0.05, Figure 3). In addition, 7S,14R-diHDHA-treated MSCs were more effective than untreated MSCs in reducing glucose concentration (667.0 ± 66.8 vs. 1,078.0 ± 23.4 mg/dL, p < 0.05, Figure 3). These results suggest that the improvement of glucose tolerance by MSCs and 7S,14R-diHDHA-treated MSCs was at least in part due to improvement of β-cell function.
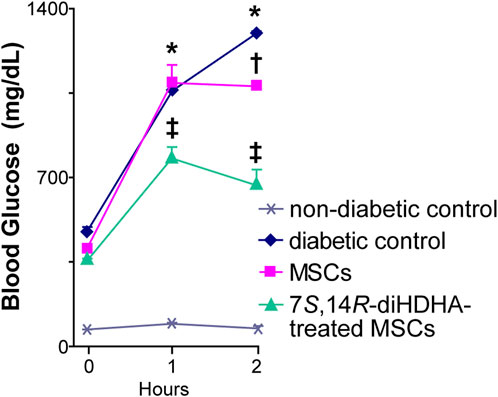
FIGURE 3. 7S,14R-diHDHA enabled MSCS to promote glucose tolerance in db/db mice. C57/BL6J MSCs (1 × 106) with or without treatment of 7S,14R-diHDHA were infused into db/db mice via tail vein every 5 days for 35 days. Mice were then injected with glucose (i.p., 2 g/kg body weight) after 8 h of fasting. Blood samples were taken at 0, 1, and 2 h from the tail vein, and blood glucose was measured. Data are mean ± SEM, n = 6. *p < 0.05 vs. nondiabetic control; †p < 0.05 vs. diabetic control; ‡ p < 0.05 vs. MSCs.
3.4 7S,14R-diHDHA induced MSC function to augment the ratio of β-cells and to reduce the ratio of α-cells in pancreatic islets
Figure 4 shows typical immunostaining patterns of pancreatic islet tissues in the nondiabetic normal C57BL/6J mice, diabetic db/db mice, and diabetic db/db mice infused with MSCs treated with or without 7S,14R-diHDHA. The islet of C57BL/6J mice, β-cells occupied most parts of the islets and existed at the center, whereas α-cells existed peripherally. However, db/db mice generally have hypertrophied pancreatic islets with a mixture of β-cells and α-cells in the islets. In addition, the ratio of β-cells to total islet cells in a pancreatic islet of db/db mice is less than that in C57BL/6J mice. Although MSC and 7S,14R-diHDHA-treated MSC infusions cannot change the distribution of β-and α-cells in the islets of db/db mice, MSC treatment can increase β-cell ratio compared with diabetic control mice (73.3% ± 1.4% vs. 67.8% ± 2.8%, p < 0.05, Figure 4), and 7S,14R-diHDHA can enhance MSC function to augment this ratio (78.2% ± 1.8% vs. 73.3% ± 1.4%, p < 0.05, Figure 4). In addition, 7S,14R-diHDHA can enhance MSC function to reduce α-cell ratio in islets of db/db mice (Figure 4).
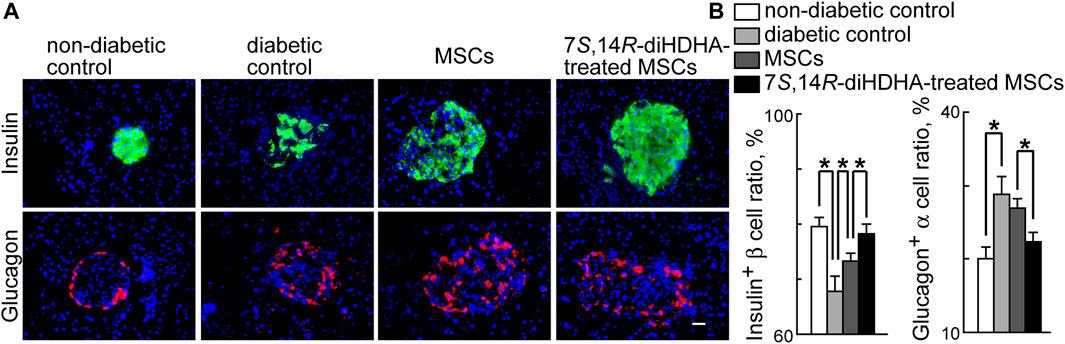
FIGURE 4. 7S,14R-diHDHA-treated MSCs regulated morphological changes in pancreatic islets. C57/BL6J MSCs (1 × 106) with or without treatment of 7S,14R-diHDHA were infused into db/db mice via tail vein every 5 days for 35 days. Pancreas was collected for histological study. (A) Representative pancreatic sections showed insulin+ β-cells (green), glucagon+ α-cells (red) (magnification ×200, scale bar = 50 μm), and nuclei were counterstained with Hochest 33342; (B) Quantification of β-cell ratio and α-cell ratio in islets (n = 60). The results are mean ± SEM. * p < 0.05.
3.5 7S,14R-diHDHA enhanced MSC function to decrease the number of macrophages in islets
Massive macrophage infiltration was observed in the islets of db/db mice compared to C57BL/6J mice, which suggests that inflammation may contribute to the dysfunctions of β-cells. In MSC-treated db/db mice, the number of macrophages in islets was reduced compared with nontreated db/db mice (Figure 5). Treatment with 7S,14R-diHDHA improved the anti-inflammatory action of MSCs.
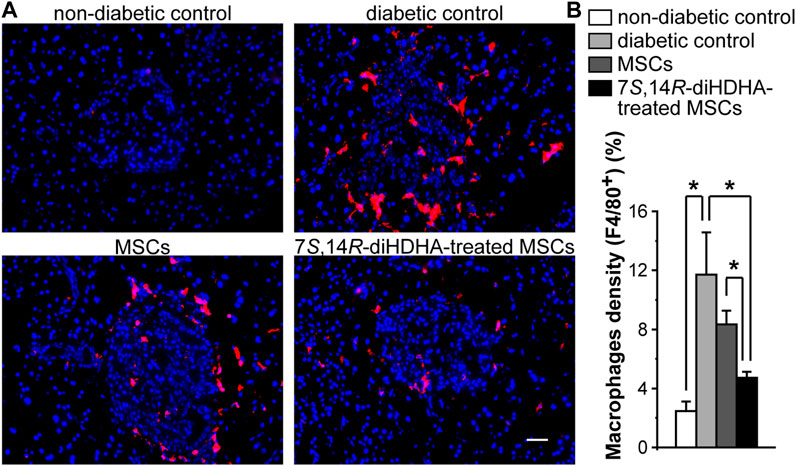
FIGURE 5. 7S,14R-diHDHA enhanced MSC function to reduce macrophage accumulation in islets. C57/BL6J MSCs (1 × 106) with or without treatment of 7S,14R-diHDHA were infused into db/db mice via tail vein every 5 days for 35 days. Pancreas was collected for histological study. (A) Typical images of F4/80+ macrophages (red) in pancreatic cryosections (magnification ×200, scale bar = 50 μm), and nuclei were counterstained with Hochest 33342; (B) Quantification of macrophage density in islets (n = 60). The results are mean ± SEM. *p < 0.05.
3.6 7S,14R-diHDHA augmented MSC function to increase min6 β-cell viability and insulin secretion
H2O2 dose-dependently reduced min6 β-cell viability was measured using the MTT method. When cocultured with MSCs, min6 β-cell viability was significantly increased under H2O2 condition (Figure 6A). Additionally, 7S,14R-diHDHA augmented the MSC effect on increasing min6 β-cell viability (Figure 6A). Furthermore, when min6 β-cells were treated with MSC conditioned medium, min6 β-cells secreted much more insulin (1.3 ± 0.1 vs. 0.9 ± 0.1 ng/mL, p < 0.05; Figure 6B), suggesting that MSCs can generate some trophic factors that trigger insulin release. 7S,14R-diHDHA-treated MSCs further enhanced insulin secretion from Min6 β-cell (1.7 ± 0.1 vs. 1.3 ± 0.1 ng/mL, p < 0.05; Figure 6B), which indicates that 7S,14R-diHDHA can promote MSCs to generate many more trophic factors acting on min6 β-cells to secrete more insulin.
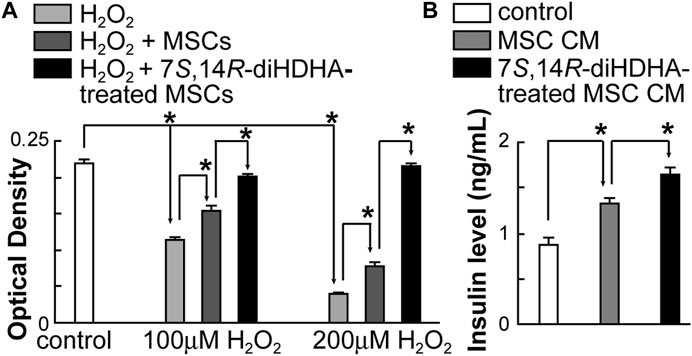
FIGURE 6. 7S,14R-diHDHA augmented MSC function to increase min6 β-cell viability and insulin Secretion. (A) Min6 β-cells were cocultured with MSCs pretreated with or without 7S,14R-diHDHA and challenged with 100 or 200 μM H2O2. (n = 6). (B) Min6 β-cells were treated for 4 h with conditioned medium (CM) of MSCs or 7S,14R-diHDHA-treated MSCs. Insulin levels were analyzed using ELISA (n = 6). The results are mean ± SEM. * p < 0.05.
3.7 7S,14R-diHDHA treatment enhanced MSC secretion of trophic growth factors
MSCs generate an array of cytokines and growth factors (Wu et al., 2007; Shi et al., 2012). Therefore, we postulated that 7S,14R-diHDHA treatment enhanced the beneficial effects of MSCs by augmenting their secretion of trophic factors. We observed that 7S,14R-diHDHA treatment increased the amount of the trophic growth factors VEGF and HGF secreted by MSCs into the cell culture medium (VEGF: 282.5 ± 9.6 vs. 233.0 ± 5.6 pg/mL, p < 0.05; 220.7 ± 13.2 vs. 152.3 ± 22.3 pg/mL, p < 0.05; Figures 7A, B).
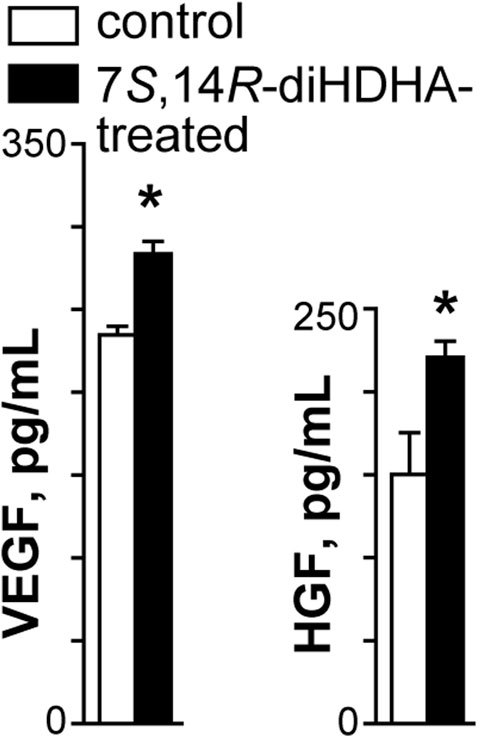
FIGURE 7. 7S,14R-diHDHA increased the production of VEGF and HGF by MSCs. MSCs (2 × 105) were treated with 7S,14R-diHDHA (250 nM) for 12 h. The level of VEGF or HGF in the supernatant was analyzed using ELISA kits. VEGF and HGF secretion from MSCs in vitro were shown (n = 4). The results are mean ± SEM. *p < 0.05 vs. control.
3.8 7S,14R-diHDHA treatment induced MSC function to decrease pericyte loss in the retina
Vasoregression is the primary pathogenic response of the retina to chronic hyperglycemia. Loss of capillary pericytes, followed by the formation of acellular, nonperfused capillaries, is an attempted compensation for retinal hypoxia (Hammes et al., 2011). Controlling mellitus can ameliorate diabetic complications; therefore, we want to know whether 7S,14R-diHDHA-treated MSCs can protect diabetic retinopathy by controlling hyperglycemia. The retinas of db/db mice showed a decrease in pericyte density compared with that of the C57BL/6J mice (Figure 8). However, MSCs can reduce pericyte loss in db/db mice. In addition, 7S,14R-diHDHA-treated MSCs can further increase the pericyte density compared to untreated MSCs (Figure 8), which suggests that 7S,14R-diHDHA enhance MSC paracrine function to ameliorate retinal pericyte loss in diabetes through adjusting hyperglycemia and acting pericyte directly.
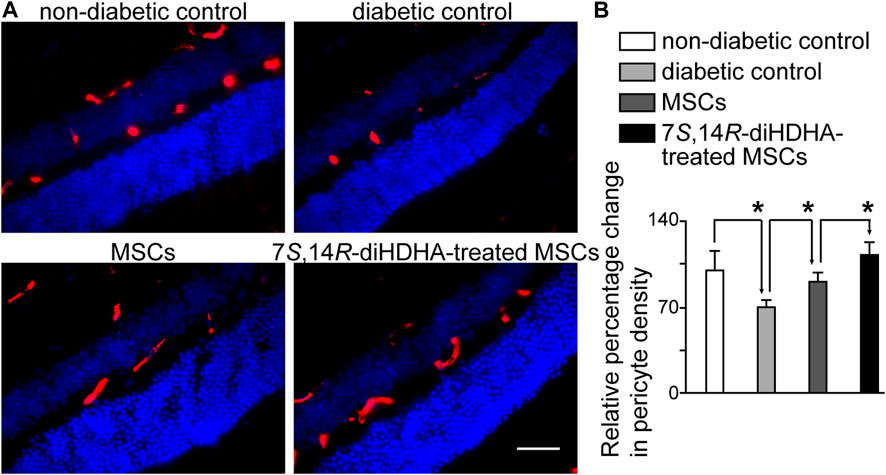
FIGURE 8. 7S,14R-diHDHA treatment induced MSC function to decrease pericyte loss in retina. C57/BL6J MSCs (1 × 106) with or without treatment of 7S,14R-diHDHA were infused into db/db mice via tail vein every 5 days for 35 days. Eyes were collected for histological study. (A) Images of α-SMA+ pericytes (red) in retinal sections (magnification ×400, scale bar = 50 μm), and nuclei were counterstained with Hochest 33342; (B) Quantification of pericyte density in retina (n = 30). The results are mean ± SEM. * p < 0.05.
4 Discussion
4.1 Leukocytes produced 7S,14R-diHDHA while P450 and 5-LOX catalyzed the biosynthesis
Taking our current results and previous findings (Hong et al., 2014a) together revealed that leukocytes and their 5-LOX and P450 can produce a novel 7S,14R-dihydroxy-4Z,8E,10Z,12E,16Z,19Z-DHA or 7S,14R-diHDHA, a maresin-1 stereoisomer from DHA, as depicted in Figure 1G. We previously found that human P450, including 2D6 (CYP2D6), can catalyze the 14R-hydroxylation of DHA, where the contribution of autooxidation is negligible (Hong et al., 2014a). We observed that human leukocytes can convert 14R-HDHA to 7S,14R-diHDHA. Moreover, we observed that human 5-LOX catalyzed the biosynthesis of 7S,14R-diHDHA from 14R-HDHA by generating 7S-hydroxy in 14R-HDHA. Therefore, 5-LOX and P450 of leukocytes are accountable for the biogenesis of 7S,14R-diHDHA since 5-LOX is a major LOX and P450 is readily available in leukocytes (el Makhour-Hojeij et al., 1994; Cook-Moreau et al., 2007; Radmark and Samuelsson, 2009; Serhan et al., 2009). The geometries of double-bonds outside of the region from C7 to C14 and the C14 chirality of 14R-HDHA are conserved after the transformation to 7,14-diHDHA, based on our results and reported analogous data for eicosanoids (Hong et al., 2003; Capdevila et al., 2005). Thus, 7S,14R-diHDHA produced by leukocytes or by sequential catalysis of P450 and 5-LOX is 7S,14R-dihydroxy-4Z,8E,10Z,12E,16Z,19Z-DHA.
4.2 Harnessing mesenchymal stem cells by 7S,14R-diHDHA to ameliorate diabetic mellitus and retinal pericyte loss
The etiologies for type 1 and type 2 diabetes differ. Type 1 diabetes is a chronic autoimmune disease hallmarked by immune-mediated destruction of the pancreatic β-cells. T lymphocytes are aberrantly activated by the antigen-presenting cells in the pancreatic draining lymph nodes, and the activated T lymphocytes then circulate, target, invade the islets and destroyed the β-cells (Mathis et al., 2001; Pugliese, 2017). However, type 2 diabetes, hallmarked by underlying insulin resistance, is also characterized by defects in glucose-responsive insulin secretion in addition to an eventual decline in β-cell function (Rhodes, 2005; Dludla et al., 2023), and oxidative stress caused by hyperglycemia is considered to be the main reason leading β-cell apoptosis (Cerf, 2013; Inaishi and Saisho, 2020; Miranda et al., 2020; Eguchi et al., 2021; Dinic et al., 2022; Dludla et al., 2023).
MSCs have multiple differentiation and paracrine functions (Miklosz and Chabowski, 2023). It has been confirmed that MSC transplantation into STZ-induced type 1 diabetic mouse and NOD models can enhance islet regeneration, lower blood sugar, and increase blood insulin levels (Jurewicz et al., 2010; Gabr et al., 2013; Yousef et al., 2022; Carlsson et al., 2023). Although MSCs can be recruited to the pancreas, less than 3% of cells migrate to the pancreas (Lee et al., 2006; Preda et al., 2021) confirmed the direct differentiation capacity of MSCs is not the main mechanism, which suggests that the paracrine function may confer tissue-repairing function to MSCs. MSCs can generate many bioactive factors, such as TGF-α, HGF, IDO, PGE2, which play important roles in modulating immuno-cell functions, such as inhibiting T cell proliferation, dendritic cell activation, and inflammatory cytokine generation (Soleymaninejadian et al., 2012; Jiang and Xu, 2020). On the basis of generation immunomodulating factors, MSCs inhibit the onset of type 1 diabetes and reverse β-cell functions (Vija et al., 2009; Jayasinghe et al., 2022). However, type 2 diabetes is different from type 1 diabetes, and immunomodulating functions may not be the reason for MSCs repairing damaged β-cells in type 2 diabetes. Increased leukocyte infiltration is associated with the onset of type 2 diabetes (Ford, 2002). Depletion of macrophages by clodronate in liposomes reduced pancreatic invasion of macrophages and destruction of islet β-cells (Inokuchi et al., 2009; Chan et al., 2019). Our results revealed that MSCs can inhibit macrophage infiltration into islets. The increased oxidative stress observed in islets of diabetic states seems to be one of the main factors of deteriorated β-cell function. We have found that MSCs can inhibit macrophage generation of the inflammatory cytokines TNF-α and ROS (Tian et al., 2012), which could implicate the potential anti-inflammatory functions of MSC protecting β-cell functions in type 2 diabetes. This implication could be confirmed by the levels of inflammation markers, such as TNFα, IF1, and IF6, which is an important direction that we need to pursue in the future.
The islet capillary network is about five times denser than the capillary network of exocrine tissue. The enriched vascularity meets the requirement for the quick secretion of insulin from β-cell in response to blood glucose levels (Konstantinova and Lammert, 2004). In diabetic db/db mice, the number of endothelial cells was reduced compared with normal nondiabetic mice. VEGF is one of the most important angiogenic cytokines (Li et al., 2003). We have confirmed that MSCs can generate VEGF and promote angiogenesis in wounds, which promotes wound healing in db/db mice (Tian et al., 2011a). MSCs promote vascularity in islets of db/db mice may also through generating VEGF.
DHA is a typical ω-3 long-chain poly-unsaturated fatty acid, it can be transformed enzymatically into potent inflammation-resolving lipid mediators, such as Resolvin D series (Spite and Serhan, 2010), neuroprotectin/protectins (Hong et al., 2003; Mukherjee et al., 2004; Zhao et al., 2011; Hong et al., 2014b), maresins (Serhan et al., 2009; Martinez-Fernandez et al., 2021), maresin-likes (Hong et al., 2014a), and 7S,14R-diHDHA. We also found a DHA-derived lipid mediators 14S,21R-diHDHA, which can enhance MSC functions to promote wound healing and repair acute kidney injury (Tian et al., 2011a; Tian et al., 2012). 7S,14R-diHDHA is a new lipid mediator that has a potential anti-inflammatory function. In this study, we observed that 7S,14R-diHDHA can promote MSC paracrine function to generate VEGF and HGF. HGF has anti-inflammatory properties; it can increase IL-10 levels and reduce IL-1β levels in pancreatitis or diabetes (Warzecha et al., 2004; Oliveira et al., 2018; Greer et al., 2022). These data suggest that 7S,14R-diHDHA promotes MSC functions to ameliorate diabetic mellitus mainly by enhancing MSC paracrine functions. These results are consistent with reports that maresins promote the beneficial bioactions of stem cells in the treatment of diseases (Martinez-Fernandez et al., 2021). Maresin 1 regulates insulin signaling in human adipocytes as well as in adipose tissue and muscle of lean and obese mice (Martinez-Fernandez et al., 2021). Maresin 1 inhibits hyperglycemia-induced ferroptosis (Li et al., 2023).
Diabetic retinopathy is a clinically well-defined, sight-threatening, chronic microvascular complication that eventually affects virtually all patients with diabetes. Diabetic retinopathy is characterized by gradually progressive alterations in the retinal microvasculature, which start with the loss of the two cellular components of retinal capillaries: the pericyte, a vessel support cell, and the endothelial cell, and pericytes disappear before endothelial cells start to vanish (Hammes et al., 2004; Eshaq et al., 2017; Gange et al., 2021). db/db mice develop hyperglycemia starting at 8-week age as a result of excessive food consumption (Kodama et al., 1994; Dalboge et al., 2013). They show early signs of diabetic retinopathy, such as thickening of capillary basement membrane at 22 weeks (Clements et al., 1998) and loss of pericytes in retinas at 26 weeks, followed by endothelial cell loss at 34 weeks (Midena et al., 1989). In our study, pericyte loss occurred as early as 22 weeks of age in db/db mice. MSCs can inhibit pericyte loss. Moreover, 7S,14R-diHDHA-treated MSCs further markedly increased pericyte density compared with untreated MSCs. This suggests that 7S,14R-diHDHA enhances MSC paracrine function to ameliorate retinal pericyte loss in diabetes by adjusting for hyperglycemia and/or acting pericyte directly. This enhancement is likely to occur through MSC secretion of VEGF, as 7S,14R-diHDHA can significantly promote MSC production of VEGF (Figure 7), and VEGF is thought to be an important cytokine inducing vasoregeneration in diabetic retinopathy (Hammes et al., 2011). When MSCs were infused into diabetic mice, MSCs have an anti-inflammatory function, reducing oxidative stress. Under this condition, VEGF or HGF generated from MSCs may trigger the PI3K/AKT signaling pathway and maintain retinal vascular integrity (Abid et al., 2004; Yun, 2021), while 7S,14R-diHDHA treatment can enhance MSC function via this pathway. The mechanism for the amelioration of diabetic damage of retinal pericytes by 7S,14R-diHDHA-treated MSCs is also likely attributable to their amelioration of diabetes, especially diabetic hyperglycemia.
4.3 Future directions
The scope of this pilot study provides multiple opportunities for future research. 5-LOX could convert DHA to 7S-hydroxyl DHA first, followed by P450 14R-hydroxylation in sequential enzymatic catalysis to generate 7S,14R-diHDHA from DHA. However, the exact P450 enzymes involved in 14R-hydroxylation are unknown. The appearance of 7S,14R-diHDHA in the blood, pancreas, and other organs in vivo in animals and humans under healthy and diabetic conditions is likely to be transient, which needs to be studied systematically at different time points. We will study the actions of 7S,14R-diHDHA alone. Future studies should also evaluate the effect of our treatments on the other aspects of diabetes and on diabetic retinopathy, as well as conduct assessments, including cell cycle analysis, characterization of biomarkers, interactions with immune cells, and morphological evaluations of mouse and human MSCs with and without 7S,14R-diHDHA treatment. Our future study will be performed in vitro to determine if the MSCs with and without the treatment by this novel lipid mediator can affect immune cells, including lymphocyte inhibition assay, migration and cytokine production of lymphocytes, monocytes, neutrophils, eosinophils, and basophils. We will compare how mouse MSCs from fat tissue and bone marrow react to this novel lipid mediator to delineate systematically if one source is better than the other or similar as this is another important milestone to translate our work to clinical application. We observed that only when MSCs were treated with 7S,14R-diHDHA could increase insulin level and reduce blood sugar level. This may be due to the low dose of MSCs that we used. In the future, we will apply high doses of MSCs to treat diabetes. In addition, type 2 diabetes is insulin resistance. The level of blood sugar is not only related to the level of insulin, but also related to the regulatory functions of the liver and other organs. 7S,14R-diHDHA-treated MSCs may not only promote the secretion of insulin, but also have the functions of improving insulin resistance, improving the regulatory functions of the liver and other organs, and reducing the level of blood sugar in diabetes. We will further detect the function of the liver and other organs in regulating blood sugar.
5 Conclusion
We identified a novel DHA-derived 7S,14R-dihydroxy-4Z,8E,10Z,12E,16Z,19Z-DHA (7S,14R-diHDHA), a maresin-1 stereoisomer, biosynthesized by leukocytes and related P450 and 5-LOX enzymes (el Makhour-Hojeij et al., 1994; Cook-Moreau et al., 2007; Radmark and Samuelsson, 2009). 7S,14R-diHDHA promoted MSC paracrine functions in db/db mice in vivo to ameliorate diabetic mellitus through improving β-cell function, lowering blood sugar, improving glucose tolerance, and increasing blood insulin levels. It augmented the proportion of β-cells and reduced that of α-cells to total islet pancreatic cells, and decreased the number of macrophages in islets in db/db mice in vivo. Also, the lipid mediator enhanced MSC function to increase min6 β-cell viability and insulin and MSC secretion of trophic growth factors. Furthermore, 7S,14R-diHDHA treatment induced MSC function to decrease pericyte loss in the retinas of diabetic db/db mice in vivo. Taken together, pretreatment of MSCs with 14S,21R-diHDHA or related LMs or structural mimics may offer a new clinical strategy for improved treatment of diabetic mellitus and diabetic complications.
Data availability statement
The original contributions presented in the study are included in the article/supplementary material, further inquiries can be directed to the corresponding authors.
Ethics statement
The studies involving humans were approved by Institutional Review Boards of Louisiana State University, Health, New Orleans, LA, United States. The studies were conducted in accordance with the local legislation and institutional requirements. The human samples used in this study were acquired from Blood Center (New Orleans, LA, United States). Written informed consent for participation was not required from the participants or the participants’ legal guardians/next of kin in accordance with the national legislation and institutional requirements. The animal study was approved by Institutional Animal Care and Use Committee and Institutional Review Board of Louisiana State University Health Sciences Center, New Orleans, United States. The study was conducted in accordance with the local legislation and institutional requirements.
Author contributions
YL: Conceptualization, Data curation, Formal Analysis, Investigation, Methodology, Validation, Visualization, Writing–original draft, Writing–review and editing. HT: Conceptualization, Data curation, Formal Analysis, Investigation, Methodology, Validation, Visualization, Writing–original draft, Writing–review and editing. HP: Formal Analysis, Investigation, Methodology, Validation, Writing–original draft, Writing–review and editing. QW: Data curation, Formal Analysis, Investigation, Methodology, Validation, Writing–original draft, Writing–review and editing. BB: Investigation, Methodology, Resources, Validation, Writing–original draft, Writing–review and editing. NB: Conceptualization, Funding acquisition, Methodology, Resources, Validation, Writing–original draft, Writing–review and editing. SH: Conceptualization, Data curation, Formal Analysis, Funding acquisition, Investigation, Methodology, Project administration, Resources, Software, Supervision, Validation, Visualization, Writing–original draft, Writing–review and editing.
Funding
The author(s) declare financial support was received for the research, authorship, and/or publication of this article. This research was supported by LSU Health-New Orleans research enhancement fund (SH), by United States National Institute of Health grants to SH(3R01DK087800, 1R21AG068756, 1R21AG066119, and 1R01GM136874), and to NGB (5R01EY005121), by EENT Foundation (NB).
Conflict of interest
The authors declare that the research was conducted in the absence of any commercial or financial relationships that could be construed as a potential conflict of interest.
Publisher’s note
All claims expressed in this article are solely those of the authors and do not necessarily represent those of their affiliated organizations, or those of the publisher, the editors and the reviewers. Any product that may be evaluated in this article, or claim that may be made by its manufacturer, is not guaranteed or endorsed by the publisher.
References
Abid, M. R., Guo, S., Minami, T., Spokes, K. C., Ueki, K., Skurk, C., et al. (2004). Vascular endothelial growth factor activates PI3K/Akt/forkhead signaling in endothelial cells. Arterioscler. Thromb. Vasc. Biol. 24 (2), 294–300. doi:10.1161/01.ATV.0000110502.10593.06
Aglan, H. A., Kotob, S. E., Mahmoud, N. S., Kishta, M. S., and Ahmed, H. H. (2024). Bone marrow stem cell-derived β-cells: new issue for diabetes cell therapy. Tissue Cell 86, 102280. doi:10.1016/j.tice.2023.102280
Albuquerque-Souza, E., Schulte, F., Chen, T., Hardt, M., Hasturk, H., Van Dyke, T. E., et al. (2020). Maresin-1 and resolvin E1 promote regenerative properties of periodontal ligament stem cells under inflammatory conditions. Front. Immunol. 11, 585530. doi:10.3389/fimmu.2020.585530
AlZahrani, S., Shinwari, Z., Gaafar, A., Alaiya, A., and Al-Kahtani, A. (2022). Anti-inflammatory effect of specialized proresolving lipid mediators on mesenchymal stem cells: an in vitro study. Cells 12 (1), 122. doi:10.3390/cells12010122
Antony, R., Lukiw, W. J., and Bazan, N. G. (2010). Neuroprotectin D1 induces dephosphorylation of Bcl-xL in a PP2A-dependent manner during oxidative stress and promotes retinal pigment epithelial cell survival. J. Biol. Chem. 285 (24), 18301–18308. doi:10.1074/jbc.M109.095232
Asatryan, A., and Bazan, N. G. (2017). Molecular mechanisms of signaling via the docosanoid neuroprotectin D1 for cellular homeostasis and neuroprotection. J. Biol. Chem. 292 (30), 12390–12397. doi:10.1074/jbc.R117.783076
Baravkar, S. B., Lu, L., Masoud, A. R., Zhao, Q., He, J., and Hong, S. (2024). Development of a novel covalently bonded conjugate of caprylic acid tripeptide (Isoleucine–Leucine–Aspartic acid) for wound-compatible and injectable hydrogel to accelerate healing. biomolecules 14 (1), 94. doi:10.3390/biom14010094
Bazan, N. G. (2006). Cell survival matters: docosahexaenoic acid signaling, neuroprotection and photoreceptors. Trends Neurosci. 29 (5), 263–271. doi:10.1016/j.tins.2006.03.005
Bernardi, S., Severini, G. M., Zauli, G., and Secchiero, P. (2012). Cell-based therapies for diabetic complications. Exp. Diabetes Res. 2012, 872504. doi:10.1155/2012/872504
Bhavsar, A. R. (2006). Diabetic retinopathy: the latest in current management. Retina 26 (6), S71–S79. doi:10.1097/01.iae.0000236466.23640.c9
Boumaza, I., Srinivasan, S., Witt, W. T., Feghali-Bostwick, C., Dai, Y., Garcia-Ocana, A., et al. (2009). Autologous bone marrow-derived rat mesenchymal stem cells promote PDX-1 and insulin expression in the islets, alter T cell cytokine pattern and preserve regulatory T cells in the periphery and induce sustained normoglycemia. J. Autoimmun. 32 (1), 33–42. doi:10.1016/j.jaut.2008.10.004
Butler, A. E., Janson, J., Bonner-Weir, S., Ritzel, R., Rizza, R. A., and Butler, P. C. (2003). Beta-cell deficit and increased beta-cell apoptosis in humans with type 2 diabetes. Diabetes 52 (1), 102–110. doi:10.2337/diabetes.52.1.102
Capdevila, J. H., Holla, V. R., and Faick, J. R. (2005). “Cytochrome P450 and the metabolism and bioactivation of arachidonic acid and eicosanoids,” in Cytochrome P450: structure, Mechanism, and Biochemistry, 3e. Editor R. O. d.M. Paul (New York: Kluwer Academic/Plenum Publishers).
Carlsson, P. O., Espes, D., Sisay, S., Davies, L. C., Smith, C. I. E., and Svahn, M. G. (2023). Umbilical cord-derived mesenchymal stromal cells preserve endogenous insulin production in type 1 diabetes: a Phase I/II randomised double-blind placebo-controlled trial. Diabetologia 66 (8), 1431–1441. doi:10.1007/s00125-023-05934-3
Cerf, M. E. (2013). Beta cell dysfunction and insulin resistance. Front. Endocrinol. (Lausanne) 4, 37. doi:10.3389/fendo.2013.00037
Chan, J. Y., Lee, K., Maxwell, E. L., Liang, C., and Laybutt, D. R. (2019). Macrophage alterations in islets of obese mice linked to beta cell disruption in diabetes. Diabetologia 62 (6), 993–999. doi:10.1007/s00125-019-4844-y
Chen, H., Zhou, W., Ruan, Y., Yang, L., Xu, N., Chen, R., et al. (2018). Reversal of angiotensin ll-induced β-cell dedifferentiation via inhibition of NF-κb signaling. Mol. Med. 24 (1), 43. doi:10.1186/s10020-018-0044-3
Cheng, Y., Wang, Y., Wang, X., Jiang, Z., Zhu, L., and Fang, S. (2022). Neutrophil-to-Lymphocyte ratio, platelet-to-lymphocyte ratio, and monocyte-to-lymphocyte ratio in depression: an updated systematic review and meta-analysis. Front. Psychiatry 13, 893097. doi:10.3389/fpsyt.2022.893097
Cianci, E., Recchiuti, A., Trubiani, O., Diomede, F., Marchisio, M., Miscia, S., et al. (2016). Human periodontal stem cells release specialized proresolving mediators and carry immunomodulatory and prohealing properties regulated by lipoxins. Stem Cells Transl. Med. 5 (1), 20–32. doi:10.5966/sctm.2015-0163
Clements, R. S., Robison, W. G., and Cohen, M. P. (1998). Anti-glycated albumin therapy ameliorates early retinal microvascular pathology in db/db mice. J. Diabetes Complicat. 12 (1), 28–33. doi:10.1016/s1056-8727(97)00051-2
Cook-Moreau, J. M., El-Makhour Hojeij, Y., Barriere, G., Rabinovitch-Chable, H. C., Faucher, K. S., Sturtz, F. G., et al. (2007). Expression of 5-lipoxygenase (5-LOX) in T lymphocytes. Immunology 122 (2), 157–166. doi:10.1111/j.1365-2567.2007.02621.x
Cortina, M. S., He, J., Russ, T., Bazan, N. G., and Bazan, H. E. (2013). Neuroprotectin D1 restores corneal nerve integrity and function after damage from experimental surgery. Invest. Ophthalmol. Vis. Sci. 54 (6), 4109–4116. doi:10.1167/iovs.13-12075
Dalboge, L. S., Almholt, D. L., Neerup, T. S., Vassiliadis, E., Vrang, N., Pedersen, L., et al. (2013). Characterisation of age-dependent beta cell dynamics in the male db/db mice. PLoS One 8 (12), e82813. doi:10.1371/journal.pone.0082813
Dalle, S., Abderrahmani, A., and Renard, E. (2023). Pharmacological inhibitors of β-cell dysfunction and death as therapeutics for diabetes. Front. Endocrinol. (Lausanne) 14, 1076343. doi:10.3389/fendo.2023.1076343
Dinic, S., Arambasic Jovanovic, J., Uskokovic, A., Mihailovic, M., Grdovic, N., Tolic, A., et al. (2022). Oxidative stress-mediated beta cell death and dysfunction as a target for diabetes management. Front. Endocrinol. (Lausanne) 13, 1006376. doi:10.3389/fendo.2022.1006376
Dludla, P. V., Mabhida, S. E., Ziqubu, K., Nkambule, B. B., Mazibuko-Mbeje, S. E., Hanser, S., et al. (2023). Pancreatic β-cell dysfunction in type 2 diabetes: implications of inflammation and oxidative stress. World J. Diabetes 14 (3), 130–146. doi:10.4239/wjd.v14.i3.130
Efanova, I. B., Zaitsev, S. V., Zhivotovsky, B., Kohler, M., Efendic, S., Orrenius, S., et al. (1998). Glucose and tolbutamide induce apoptosis in pancreatic beta-cells. A process dependent on intracellular Ca2+ concentration. J. Biol. Chem. 273 (50), 33501–33507. doi:10.1074/jbc.273.50.33501
Eguchi, N., Vaziri, N. D., Dafoe, D. C., and Ichii, H. (2021). The role of oxidative stress in pancreatic β cell dysfunction in diabetes. Int. J. Mol. Sci. 22 (4), 1509. doi:10.3390/ijms22041509
el Makhour-Hojeij, Y., Baclet, M. C., Chable-Rabinovitch, H., Beneytout, J. L., and Cook, J. (1994). Expression of 5-lipoxygenase in lymphoblastoid B and T cells. Prostaglandins 48 (1), 21–29. doi:10.1016/0090-6980(94)90093-0
Emre, C., Arroyo-Garcia, L. E., Do, K. V., Jun, B., Ohshima, M., Alcalde, S. G., et al. (2022). Intranasal delivery of pro-resolving lipid mediators rescues memory and gamma oscillation impairment in App(NL-G-F/NL-G-F) mice. Commun. Biol. 5 (1), 245. doi:10.1038/s42003-022-03169-3
Emre, C., Do, K. V., Jun, B., Hjorth, E., Alcalde, S. G., Kautzmann, M. I., et al. (2021). Age-related changes in brain phospholipids and bioactive lipids in the APP knock-in mouse model of Alzheimer's disease. Acta Neuropathol. Commun. 9 (1), 116. doi:10.1186/s40478-021-01216-4
Eshaq, R. S., Aldalati, A. M. Z., Alexander, J. S., and Harris, N. R. (2017). Diabetic retinopathy: breaking the barrier. Pathophysiology 24 (4), 229–241. doi:10.1016/j.pathophys.2017.07.001
Florez, J. C. (2008). Newly identified loci highlight beta cell dysfunction as a key cause of type 2 diabetes: where are the insulin resistance genes? Diabetologia 51 (7), 1100–1110. doi:10.1007/s00125-008-1025-9
Ford, E. S. (2002). Leukocyte count, erythrocyte sedimentation rate, and diabetes incidence in a national sample of US adults. Am. J. Epidemiol. 155 (1), 57–64. doi:10.1093/aje/155.1.57
Gabr, M. M., Zakaria, M. M., Refaie, A. F., Ismail, A. M., Abou-El-Mahasen, M. A., Ashamallah, S. A., et al. (2013). Insulin-producing cells from adult human bone marrow mesenchymal stem cells control streptozotocin-induced diabetes in nude mice. Cell Transpl. 22 (1), 133–145. doi:10.3727/096368912X647162
Gange, W. S., Lopez, J., Xu, B. Y., Lung, K., Seabury, S. A., and Toy, B. C. (2021). Incidence of proliferative diabetic retinopathy and other neovascular sequelae at 5 Years following diagnosis of type 2 diabetes. Diabetes Care 44 (11), 2518–2526. doi:10.2337/dc21-0228
Greer, P. J., Lee, P. J., Paragomi, P., Stello, K., Phillips, A., Hart, P., et al. (2022). Severe acute pancreatitis exhibits distinct cytokine signatures and trajectories in humans: a prospective observational study. Am. J. Physiol. Gastrointest. Liver Physiol. 323 (5), G428–G438. doi:10.1152/ajpgi.00100.2022
Group, G. S. R., Nathan, D. M., Lachin, J. M., Balasubramanyam, A., Burch, H. B., Buse, J. B., et al. (2022). Glycemia reduction in type 2 diabetes - glycemic outcomes. N. Engl. J. Med. 387 (12), 1063–1074. doi:10.1056/NEJMoa2200433
Gunton, J. E., Kulkarni, R. N., Yim, S., Okada, T., Hawthorne, W. J., Tseng, Y. H., et al. (2005). Loss of ARNT/HIF1beta mediates altered gene expression and pancreatic-islet dysfunction in human type 2 diabetes. Cell 122 (3), 337–349. doi:10.1016/j.cell.2005.05.027
Hakobyan, L., Haaijer-Ruskamp, F. M., de Zeeuw, D., Dobre, D., and Denig, P. (2011). Comparing adverse event rates of oral blood glucose-lowering drugs reported by patients and healthcare providers: a post-hoc analysis of observational studies published between 1999 and 2011. Drug Saf. 34 (12), 1191–1202. doi:10.2165/11593810-000000000-00000
Hammes, H. P., Feng, Y., Pfister, F., and Brownlee, M. (2011). Diabetic retinopathy: targeting vasoregression. Diabetes 60 (1), 9–16. doi:10.2337/db10-0454
Hammes, H. P., Lin, J., Wagner, P., Feng, Y., Vom Hagen, F., Krzizok, T., et al. (2004). Angiopoietin-2 causes pericyte dropout in the normal retina: evidence for involvement in diabetic retinopathy. Diabetes 53 (4), 1104–1110. doi:10.2337/diabetes.53.4.1104
Harmon, J. S., Bogdani, M., Parazzoli, S. D., Mak, S. S., Oseid, E. A., Berghmans, M., et al. (2009). beta-Cell-specific overexpression of glutathione peroxidase preserves intranuclear MafA and reverses diabetes in db/db mice. Endocrinology 150 (11), 4855–4862. doi:10.1210/en.2009-0708
Hellmann, J., Tang, Y., and Spite, M. (2012). Proresolving lipid mediators and diabetic wound healing. Curr. Opin. Endocrinol. Diabetes Obes. 19 (2), 104–108. doi:10.1097/MED.0b013e3283514e00
Hess, D., Li, L., Martin, M., Sakano, S., Hill, D., Strutt, B., et al. (2003). Bone marrow-derived stem cells initiate pancreatic regeneration. Nat. Biotechnol. 21 (7), 763–770. doi:10.1038/nbt841
Hong, S., Gronert, K., Devchand, P. R., Moussignac, R. L., and Serhan, C. N. (2003). Novel docosatrienes and 17S-resolvins generated from docosahexaenoic acid in murine brain, human blood, and glial cells. Autacoids in anti-inflammation. J. Biol. Chem. 278 (17), 14677–14687. doi:10.1074/jbc.M300218200
Hong, S., Lu, Y., Tian, H., Alapure, B. V., Wang, Q., Bunnell, B. A., et al. (2014a). Maresin-like lipid mediators are produced by leukocytes and platelets and rescue reparative function of diabetes-impaired macrophages. Chem. Biol. 21 (10), 1318–1329. doi:10.1016/j.chembiol.2014.06.010
Hong, S., Tian, H., Lu, Y., Laborde, J. M., Muhale, F. A., Wang, Q., et al. (2014b). Neuroprotectin/protectin D1: endogenous biosynthesis and actions on diabetic macrophages in promoting wound healing and innervation impaired by diabetes. Am. J. Physiol. Cell Physiol. 307 (11), C1058–C1067. doi:10.1152/ajpcell.00270.2014
Inaishi, J., and Saisho, Y. (2020). Beta-cell mass in obesity and type 2 diabetes, and its relation to pancreas fat: a mini-review. Nutrients 12 (12), 3846. doi:10.3390/nu12123846
Inokuchi, C., Ueda, H., Hamaguchi, T., Miyagawa, J., Shinohara, M., Okamura, H., et al. (2009). Role of macrophages in the development of pancreatic islet injury in spontaneously diabetic torii rats. Exp. Anim. 58 (4), 383–394. doi:10.1538/expanim.58.383
Intensive blood-glucose control (1998). Intensive blood-glucose control with sulphonylureas or insulin compared with conventional treatment and risk of complications in patients with type 2 diabetes (UKPDS 33). UK Prospective Diabetes Study (UKPDS) Group. Lancet 352 (9131), 837–853. doi:10.1016/S0140-6736(98)07019-6
Izadpanah, R., Trygg, C., Patel, B., Kriedt, C., Dufour, J., Gimble, J. M., et al. (2006). Biologic properties of mesenchymal stem cells derived from bone marrow and adipose tissue. J. Cell Biochem. 99 (5), 1285–1297. doi:10.1002/jcb.20904
Jayasinghe, M., Prathiraja, O., Perera, P. B., Jena, R., Silva, M. S., Weerawarna, P. S. H., et al. (2022). The role of mesenchymal stem cells in the treatment of type 1 diabetes. Cureus 14 (7), e27337. doi:10.7759/cureus.27337
Jiang, W., and Xu, J. (2020). Immune modulation by mesenchymal stem cells. Cell Prolif. 53 (1), e12712. doi:10.1111/cpr.12712
Jurewicz, M., Yang, S., Augello, A., Godwin, J. G., Moore, R. F., Azzi, J., et al. (2010). Congenic mesenchymal stem cell therapy reverses hyperglycemia in experimental type 1 diabetes. Diabetes 59 (12), 3139–3147. doi:10.2337/db10-0542
Kodama, H., Fujita, M., and Yamaguchi, I. (1994). Development of hyperglycaemia and insulin resistance in conscious genetically diabetic (C57BL/KsJ-db/db) mice. Diabetologia 37 (8), 739–744. doi:10.1007/BF00404329
Konstantinova, I., and Lammert, E. (2004). Microvascular development: learning from pancreatic islets. Bioessays 26 (10), 1069–1075. doi:10.1002/bies.20105
Kornicka, K., Houston, J., and Marycz, K. (2018). Dysfunction of mesenchymal stem cells isolated from metabolic syndrome and type 2 diabetic patients as result of oxidative stress and autophagy may limit their potential therapeutic use. Stem Cell Rev. Rep. 14 (3), 337–345. doi:10.1007/s12015-018-9809-x
Lee, J. S., Kim, N. Y., Na, S. H., Youn, Y. H., and Shin, C. S. (2018). Reference values of neutrophil-lymphocyte ratio, lymphocyte-monocyte ratio, platelet-lymphocyte ratio, and mean platelet volume in healthy adults in South Korea. Med. Baltim. 97 (26), e11138. doi:10.1097/MD.0000000000011138
Lee, J. W., Gu, H. O., Jung, Y., Jung, Y., Seo, S. Y., Hong, J. H., et al. (2023). Candesartan, an angiotensin-II receptor blocker, ameliorates insulin resistance and hepatosteatosis by reducing intracellular calcium overload and lipid accumulation. Exp. Mol. Med. 55 (5), 910–925. doi:10.1038/s12276-023-00982-6
Lee, R. H., Seo, M. J., Reger, R. L., Spees, J. L., Pulin, A. A., Olson, S. D., et al. (2006). Multipotent stromal cells from human marrow home to and promote repair of pancreatic islets and renal glomeruli in diabetic NOD/scid mice. Proc. Natl. Acad. Sci. U. S. A. 103 (46), 17438–17443. doi:10.1073/pnas.0608249103
Li, J., Zhang, Y. P., and Kirsner, R. S. (2003). Angiogenesis in wound repair: angiogenic growth factors and the extracellular matrix. Microsc. Res. Tech. 60 (1), 107–114. doi:10.1002/jemt.10249
Li, Y., Liu, J., Ma, X., and Bai, X. (2023). Maresin-1 inhibits high glucose induced ferroptosis in ARPE-19 cells by activating the Nrf2/HO-1/GPX4 pathway. BMC Ophthalmol. 23 (1), 368. doi:10.1186/s12886-023-03115-9
Lu, Y., Tian, H., and Hong, S. (2010). Novel 14,21-dihydroxy-docosahexaenoic acids: structures, formation pathways, and enhancement of wound healing. J. Lipid Res. 51 (5), 923–932. doi:10.1194/jlr.M000059
Marcheselli, V. L., Hong, S., Lukiw, W. J., Tian, X. H., Gronert, K., Musto, A., et al. (2003). Novel docosanoids inhibit brain ischemia-reperfusion-mediated leukocyte infiltration and pro-inflammatory gene expression. J. Biol. Chem. 278 (44), 43807–43817. doi:10.1074/jbc.M305841200
Martinez-Fernandez, L., Gonzalez-Muniesa, P., Sainz, N., Escote, X., Martinez, J. A., Arbones-Mainar, J. M., et al. (2021). Maresin 1 regulates insulin signaling in human adipocytes as well as in adipose tissue and muscle of lean and obese mice. J. Physiol. Biochem. 77 (1), 167–173. doi:10.1007/s13105-020-00775-9
Mathis, D., Vence, L., and Benoist, C. (2001). beta-Cell death during progression to diabetes. Nature 414 (6865), 792–798. doi:10.1038/414792a
Mathur, A., Taurin, S., and Alshammary, S. (2023). The safety and efficacy of mesenchymal stem cells in the treatment of type 2 diabetes- A literature review. Diabetes Metab. Syndr. Obes. 16, 769–777. doi:10.2147/DMSO.S392161
Midena, E., Segato, T., Radin, S., di Giorgio, G., Meneghini, F., Piermarocchi, S., et al. (1989). Studies on the retina of the diabetic db/db mouse. I. Endothelial cell-pericyte ratio. Ophthalmic Res. 21 (2), 106–111. doi:10.1159/000266787
Miklosz, A., and Chabowski, A. (2023). Adipose-derived mesenchymal stem cells therapy as a new treatment option for diabetes mellitus. J. Clin. Endocrinol. Metab. 108 (8), 1889–1897. doi:10.1210/clinem/dgad142
Miranda, M. A., Carson, C., St Pierre, C. L., Macias-Velasco, J. F., Hughes, J. W., Kunzmann, M., et al. (2020). Spontaneous restoration of functional β-cell mass in obese SM/J mice. Physiol. Rep. 8 (20), e14573. doi:10.14814/phy2.14573
Mukherjee, P. K., Marcheselli, V. L., Serhan, C. N., and Bazan, N. G. (2004). Neuroprotectin D1: a docosahexaenoic acid-derived docosatriene protects human retinal pigment epithelial cells from oxidative stress. Proc. Natl. Acad. Sci. U. S. A. 101 (22), 8491–8496. doi:10.1073/pnas.0402531101
Oliveira, A. G., Araujo, T. G., Carvalho, B. M., Rocha, G. Z., Santos, A., and Saad, M. J. A. (2018). The role of hepatocyte growth factor (HGF) in insulin resistance and diabetes. Front. Endocrinol. (Lausanne) 9, 503. doi:10.3389/fendo.2018.00503
Percie du Sert, N., Hurst, V., Ahluwalia, A., Alam, S., Avey, M. T., Baker, M., et al. (2020). The ARRIVE guidelines 2.0: updated guidelines for reporting animal research. J. Cereb. Blood Flow. Metab. 40 (9), 1769–1777. doi:10.1177/0271678X20943823
Pham, T. L., and Bazan, H. E. P. (2021). Docosanoid signaling modulates corneal nerve regeneration: effect on tear secretion, wound healing, and neuropathic pain. J. Lipid Res. 62, 100033. doi:10.1194/jlr.TR120000954
Pham, T. L., He, J., Kakazu, A. H., Jun, B., Bazan, N. G., and Bazan, H. E. P. (2017). Defining a mechanistic link between pigment epithelium-derived factor, docosahexaenoic acid, and corneal nerve regeneration. J. Biol. Chem. 292 (45), 18486–18499. doi:10.1074/jbc.M117.801472
Pick, A., Clark, J., Kubstrup, C., Levisetti, M., Pugh, W., Bonner-Weir, S., et al. (1998). Role of apoptosis in failure of beta-cell mass compensation for insulin resistance and beta-cell defects in the male Zucker diabetic fatty rat. Diabetes 47 (3), 358–364. doi:10.2337/diabetes.47.3.358
Preda, M. B., Neculachi, C. A., Fenyo, I. M., Vacaru, A. M., Publik, M. A., Simionescu, M., et al. (2021). Short lifespan of syngeneic transplanted MSC is a consequence of in vivo apoptosis and immune cell recruitment in mice. Cell Death Dis. 12 (6), 566. doi:10.1038/s41419-021-03839-w
Puff, R., Dames, P., Weise, M., Goke, B., Seissler, J., Parhofer, K. G., et al. (2011). Reduced proliferation and a high apoptotic frequency of pancreatic beta cells contribute to genetically-determined diabetes susceptibility of db/db BKS mice. Horm. Metab. Res. 43 (5), 306–311. doi:10.1055/s-0031-1271817
Pugliese, A. (2017). Autoreactive T cells in type 1 diabetes. J. Clin. Invest. 127 (8), 2881–2891. doi:10.1172/JCI94549
Radmark, O., and Samuelsson, B. (2009). 5-Lipoxygenase: mechanisms of regulation. J. Lipid Res. 50, S40–S45. doi:10.1194/jlr.R800062-JLR200
Rakian, A., Rakian, R., Shay, A. E., Serhan, C. N., and Van Dyke, T. E. (2022). Periodontal stem cells synthesize maresin conjugate in tissue regeneration 3. J. Dent. Res. 101 (10), 1205–1213. doi:10.1177/00220345221090879
Rhodes, C. J. (2005). Type 2 diabetes-a matter of beta-cell life and death? Science 307 (5708), 380–384. doi:10.1126/science.1104345
Robertson, R. P. (2023). Nrf2 and antioxidant response in animal models of type 2 diabetes. Int. J. Mol. Sci. 24 (4), 3082. doi:10.3390/ijms24043082
Schneider, C., Yu, Z., Boeglin, W. E., Zheng, Y., and Brash, A. R. (2007). Enantiomeric separation of hydroxy and hydroperoxy eicosanoids by chiral column chromatography. Methods Enzymol. 433, 145–157. doi:10.1016/S0076-6879(07)33008-5
Serhan, C. N., Hong, S., Gronert, K., Colgan, S. P., Devchand, P. R., Mirick, G., et al. (2002). Resolvins: a family of bioactive products of omega-3 fatty acid transformation circuits initiated by aspirin treatment that counter proinflammation signals. J. Exp. Med. 196 (8), 1025–1037. doi:10.1084/jem.20020760
Serhan, C. N., and Romano, M. (1995). Lipoxin biosynthesis and actions: role of the human platelet LX-synthase. J. Lipid Mediat Cell Signal 12 (2-3), 293–306. doi:10.1016/0929-7855(95)00035-o
Serhan, C. N., Yang, R., Martinod, K., Kasuga, K., Pillai, P. S., Porter, T. F., et al. (2009). Maresins: novel macrophage mediators with potent antiinflammatory and proresolving actions. J. Exp. Med. 206 (1), 15–23. doi:10.1084/jem.20081880
Shao, J., Iwashita, N., Ikeda, F., Ogihara, T., Uchida, T., Shimizu, T., et al. (2006). Beneficial effects of candesartan, an angiotensin II type 1 receptor blocker, on beta-cell function and morphology in db/db mice. Biochem. Biophys. Res. Commun. 344 (4), 1224–1233. doi:10.1016/j.bbrc.2006.04.011
Shi, Y., Su, J., Roberts, A. I., Shou, P., Rabson, A. B., and Ren, G. (2012). How mesenchymal stem cells interact with tissue immune responses. Trends Immunol. 33 (3), 136–143. doi:10.1016/j.it.2011.11.004
Shindou, H., Koso, H., Sasaki, J., Nakanishi, H., Sagara, H., Nakagawa, K. M., et al. (2017). Docosahexaenoic acid preserves visual function by maintaining correct disc morphology in retinal photoreceptor cells. J. Biol. Chem. 292 (29), 12054–12064. doi:10.1074/jbc.M117.790568
Soleymaninejadian, E., Pramanik, K., and Samadian, E. (2012). Immunomodulatory properties of mesenchymal stem cells: cytokines and factors. Am. J. Reprod. Immunol. 67 (1), 1–8. doi:10.1111/j.1600-0897.2011.01069.x
Spite, M., and Serhan, C. N. (2010). Novel lipid mediators promote resolution of acute inflammation: impact of aspirin and statins. Circ. Res. 107 (10), 1170–1184. doi:10.1161/CIRCRESAHA.110.223883
Sugimoto, S., Mena, H. A., Sansbury, B. E., Kobayashi, S., Tsuji, T., Wang, C. H., et al. (2022). Brown adipose tissue-derived MaR2 contributes to cold-induced resolution of inflammation. Nat. Metab. 4 (6), 775–790. doi:10.1038/s42255-022-00590-0
Tian, H., Lu, Y., Shah, S. P., and Hong, S. (2011a). 14S,21R-Dihydroxydocosahexaenoic acid remedies impaired healing and mesenchymal stem cell functions in diabetic wounds. J. Biol. Chem. 286 (6), 4443–4453. doi:10.1074/jbc.M110.100388
Tian, H., Lu, Y., Shah, S. P., and Hong, S. (2011b). Autacoid 14S,21R-dihydroxy-docosahexaenoic acid counteracts diabetic impairment of macrophage prohealing functions. Am. J. Pathol. 179 (4), 1780–1791. doi:10.1016/j.ajpath.2011.06.026
Tian, H., Lu, Y., Shah, S. P., Wang, Q., and Hong, S. (2012). 14S,21R-dihydroxy-docosahexaenoic acid treatment enhances mesenchymal stem cell amelioration of renal ischemia/reperfusion injury. Stem Cells Dev. 21 (7), 1187–1199. doi:10.1089/scd.2011.0220
Tiedge, M., Lortz, S., Drinkgern, J., and Lenzen, S. (1997). Relation between antioxidant enzyme gene expression and antioxidative defense status of insulin-producing cells. Diabetes 46 (11), 1733–1742. doi:10.2337/diab.46.11.1733
Vija, L., Farge, D., Gautier, J. F., Vexiau, P., Dumitrache, C., Bourgarit, A., et al. (2009). Mesenchymal stem cells: stem cell therapy perspectives for type 1 diabetes. Diabetes Metab. 35 (2), 85–93. doi:10.1016/j.diabet.2008.10.003
Walker, M. E., Kodani, S. D., Mena, H. A., Tseng, Y. H., Cypess, A. M., and Spite, M. (2024). Brown adipose tissue activation in humans increases plasma levels of lipid mediators. J. Clin. Endocrinol. Metab., dgae016. doi:10.1210/clinem/dgae016
Wang, L., Zhao, S., Mao, H., Zhou, L., Wang, Z. J., and Wang, H. X. (2011). Autologous bone marrow stem cell transplantation for the treatment of type 2 diabetes mellitus. Chin. Med. J. Engl. 124 (22), 3622–3628. doi:10.3760/cma.j.issn.0366-6999.2011.22.005
Want, L. L. (2008). Optimizing treatment success with an amylin analogue. Diabetes Educ. 34 (1), 11S–17S. doi:10.1177/0145721707313940
Warzecha, Z., Dembinski, A., Ceranowicz, P., Konturek, S., Tomaszewska, R., Stachura, J., et al. (2004). Inhibition of cyclooxygenase-2 reduces the protective effect of hepatocyte growth factor in experimental pancreatitis. Eur. J. Pharmacol. 486 (1), 107–119. doi:10.1016/j.ejphar.2003.12.015
Wu, Y., Chen, L., Scott, P. G., and Tredget, E. E. (2007). Mesenchymal stem cells enhance wound healing through differentiation and angiogenesis. Stem Cells 25 (10), 2648–2659. doi:10.1634/stemcells.2007-0226
Yao, D., Zou, Y., and Lv, Y. (2022). Maresin 1 enhances osteogenic potential of mesenchymal stem cells by modulating macrophage peroxisome proliferator-activated receptor-gamma-mediated inflammation resolution. Biomater. Adv. 141, 213116. doi:10.1016/j.bioadv.2022.213116
Yin, H., Gao, L., Tai, H. H., Murphey, L. J., Porter, N. A., and Morrow, J. D. (2007). Urinary prostaglandin F2alpha is generated from the isoprostane pathway and not the cyclooxygenase in humans. J. Biol. Chem. 282 (1), 329–336. doi:10.1074/jbc.M608975200
Yin, M., Zhang, Y., Yu, H., and Li, X. (2021). Role of hyperglycemia in the senescence of mesenchymal stem cells. Front. Cell Dev. Biol. 9, 665412. doi:10.3389/fcell.2021.665412
Yousef, H. N., Sakr, S. M., and Sabry, S. A. (2022). Mesenchymal stem cells ameliorate hyperglycemia in type I diabetic developing male rats. Stem Cells Int. 2022, 7556278. doi:10.1155/2022/7556278
Yun, J. H. (2021). Hepatocyte growth factor prevents pericyte loss in diabetic retinopathy. Microvasc. Res. 133, 104103. doi:10.1016/j.mvr.2020.104103
Zhao, Y., Calon, F., Julien, C., Winkler, J. W., Petasis, N. A., Lukiw, W. J., et al. (2011). Docosahexaenoic acid-derived neuroprotectin D1 induces neuronal survival via secretase- and PPARγ-mediated mechanisms in Alzheimer's disease models. PLoS One 6 (1), e15816. doi:10.1371/journal.pone.0015816
Keywords: diabetic mellitus and complications, mesenchymal stem cell, 7S,14R-diHDHA, blood glucose level, β-cell and α-cell, insulin, pancreas and islets, retinopathy
Citation: Lu Y, Tian H, Peng H, Wang Q, Bunnell BA, Bazan NG and Hong S (2024) Novel lipid mediator 7S,14R-docosahexaenoic acid: biogenesis and harnessing mesenchymal stem cells to ameliorate diabetic mellitus and retinal pericyte loss. Front. Cell Dev. Biol. 12:1380059. doi: 10.3389/fcell.2024.1380059
Received: 31 January 2024; Accepted: 29 February 2024;
Published: 12 March 2024.
Edited by:
Bin Jiang, Shenzhen Second People’s Hospital, ChinaReviewed by:
Li Yan, University of Maryland, College Park, United StatesYuyao Tian, Massachusetts General Hospital and Harvard Medical School, United States
Chenxiang Luo, Michigan State University, United States
Copyright © 2024 Lu, Tian, Peng, Wang, Bunnell, Bazan and Hong. This is an open-access article distributed under the terms of the Creative Commons Attribution License (CC BY). The use, distribution or reproduction in other forums is permitted, provided the original author(s) and the copyright owner(s) are credited and that the original publication in this journal is cited, in accordance with accepted academic practice. No use, distribution or reproduction is permitted which does not comply with these terms.
*Correspondence: Song Hong, c2hvbmdAbHN1aHNjLmVkdQ==; Haibin Tian, dGlhbmhiQHRvbmdqaS5lZHUuY24=
†Present addresses: Haibin Tian, Department of Ophthalmology of Tongji Hospital, Laboratory of Clinical and Visual Sciences of Tongji Eye Institute, School of Medicine, Tongji University, Shanghai, China; Department of Physiology and Pharmacology; TUSM, Shanghai, China
Quansheng Wang, Department of Integrated Chinese and Western Medicine Union Hospital, Tongji Medical College, Huazhong University of Science and Technology, Wuhan, Hubei, China
Bruce A. Bunnell, Department of Microbiology, Immunology and Genetics at the University of North Texas Health Science Center in Fort Worth, Fort Worth, TX, United States
‡These authors have contributed equally to this work and share first authorship