- Department of Oncology, Shengjing Hospital of China Medical University, Shenyang, Liaoning, China
Immunotherapy has emerged as a promising cancer treatment option in recent years. In immune “hot” tumors, characterized by abundant immune cell infiltration, immunotherapy can improve patients’ prognosis by activating the function of immune cells. By contrast, immune “cold” tumors are often less sensitive to immunotherapy owing to low immunogenicity of tumor cells, an immune inhibitory tumor microenvironment, and a series of immune-escape mechanisms. Immunogenic cell death (ICD) is a promising cellular process to facilitate the transformation of immune “cold” tumors to immune “hot” tumors by eliciting innate and adaptive immune responses through the release of (or exposure to) damage-related molecular patterns. Accumulating evidence suggests that various traditional therapies can induce ICD, including chemotherapy, targeted therapy, radiotherapy, and photodynamic therapy. In this review, we summarize the biological mechanisms and hallmarks of ICD and introduce some newly discovered and technologically innovative inducers that activate the immune system at the molecular level. Furthermore, we also discuss the clinical applications of combing ICD inducers with cancer immunotherapy. This review will provide valuable insights into the future development of ICD-related combination therapeutics and potential management for “cold” tumors.
1 Introduction
Immunotherapy has emerged as a promising treatment option in recent years in certain types of cancers. It is a regimen that treats cancer by regulating the body’s immune system, using immune checkpoint inhibitors (ICIs), monoclonal antibodies, T-cell transfer therapy, and tumor vaccines. The clinical application of immunotherapy has considerably improved the prognosis of multiple types of tumors, in turn improving the prognosis of many patients. However, when it comes to immunosuppressive tumors that are more resistant to immunotherapy, some challenges still urgently need to be addressed. Firstly, patients with “cold” tumor, characterized by an immunosuppressive tumor microenvironment (TME), insufficient T cell infiltration, or a lower immunoscore, gain few benefits from immunotherapy (Vonderheide, 2018; Galon and Bruni, 2019; Zhang and Zhang, 2020).” In addition, malignant cells can evolve several immune-escape mechanisms to prevent being monitored and eliminated (Hanahan and Weinberg, 2011), making immunotherapy more challenging. However, a cellular process discovered in the recent decades called immunogenic cell death (ICD) has highlighted promising tumor management methods (Li C. et al., 2022). In general, ICD remodels the original immunosuppressive TME via the release of various damage associated molecular patterns (DAMPs) and stimulates an immune response to suppress tumor growth, converting the “cold” tumor into a “hot” phenotype. This increases the efficacy of immunotherapy (Wang-Bishop et al., 2020) in patients who had minimal benefited from initial clinical treatment. Importantly, extensive studies have shown that traditional therapies, such as chemotherapy (Fabian et al., 2021; Kodumudi et al., 2010), targeted therapy, radiotherapy (RT) (Huang et al., 2021; Vaes et al., 2021), and photodynamic therapy (PDT) (Yu et al., 2023) can function as ICD inducers to activate the host-reactive immune response (Rodriguez-Ruiz et al., 2020; Zhu et al., 2021), although they were originally acknowledged to directly kill tumor cells (Cherniavsky et al., 2015). Notably, only a limited number of these traditional regimens serve as ICD inducers or induce sufficient ICD (Fu et al., 2021). Thus, investigators need to identify novel inducers (Sen et al., 2023) and explain the rationale behind various combination approaches to promote the efficacy of immunotherapy.
In this review, we briefly summarize the key hallmarks of ICD, discuss their implications for the host immune system, introduce novel regimens for eliciting ICD, and analyze their potential clinical applications.
2 Biology of ICD
2.1 Definition and antitumor effects of ICD
ICD is defined as “a form of regulated cell death that is sufficient to activate an adaptive immune response in immunocompetent hosts” (Galluzzi et al., 2018). Unlike necrosis or apoptosis, ICD is characterized by diverse molecular hallmarks and a subsequent antigen-specific immune response (Medrano et al., 2017). It can improve the maturation of dendritic cells (DCs), attract mature DCs to gather around dying tumor cells (Kobayashi and Choyke, 2019), and stimulate their antigen cross-presenting ability to activate cytotoxic T lymphocytes offering prolonged adaptive immunity (Azizi et al., 2023; Wei et al., 2021). In addition to the increase in cytotoxic T lymphocyte function, their infiltration is also enhanced (Wei et al., 2021). Simultaneously, there is a significant decrease in the proportion of suppressor cells, such as regulatory T lymphocytes (Li et al., 2021).
2.2 Necessary conditions for ICD
In general, the ICD immunogenic properties manifest in two aspects: antigenicity and adjuvanticity (Kroemer et al., 2022). When undergoing cell death, cancer cells must have sufficient antigenicity to trigger an adaptive immune response, resulting in ICD. Tumor neoantigens (TNAs) are a source of antigenicity, arising from accumulated mutations acquired as tumors evolve (Vitale et al., 2019). Additionally, antitumor immune responses can be mediated by tumor-associated antigens (TAAs) (Sprooten et al., 2019) that are not specific to neoplastic cells. The adjuvant aspects of ICD refer to the process by which antigen-presenting cells efficiently present antigens to T cells in the presence of co-stimulatory molecules (Jhunjhunwala et al., 2021). ICD is triggered by endoplasmic reticulum stress (ERS) and/or reactive oxygen species (ROS)-induced stress. It is intricately linked to the molecular biomarkers represented by DAMPs released during cell death, such as calreticulin (CRT), high-mobility group protein box 1 (HMGB1), adenosine triphosphate (ATP), family of heat shock proteins (HSP), cellular nucleic acids such as SAP130, annexin A1 (ANXA1), and immunostimulatory and chemotactic cytokines such as IFN-I, CCL2, CXCL1, and CXCL10 (Apetoh et al., 2007; Sistigu et al., 2014). Different ICD inducers cause cells to release different DAMPs, each of which plays an antitumor role with its unique mechanisms, as elucidated by many previous preclinical and clinical studies (Figure 1). Mechanistically, these inducers can be divided into two major categories based on whether they act directly on the endoplasmic reticulum (ER) (Krysko et al., 2012), Type I ICD inducers (which target is unrelated to the ER, the secondary ERS that it causes tends to be milder) and Type II inducers (which directly act on the ER, and cause relatively potent ERS). When the stress exceeds the adjustable range, it activates inositol-requiring enzyme 1 alpha (IRE1α), and the endonuclease activity at the end of IRE1α specifically splices the mRNA of the transcription factor X-box-Binding Protein 1 (XBP1), which, in turn, activates XBP1 mRNA. Additionally, activated protein kinase RNA-like endoplasmic reticulum kinase (PERK) induces the phosphorylation of eukaryotic initiation factor-2α (eIF2α), which allows for selective translation of activating transcription factor 4 (ATF4) and inhibits the synthesis of general proteins. The production of ERS also activates the membrane protein-activating transcription factor 6 (ATF6), which is transferred to the Golgi apparatus and cleaved into active fragments. Eventually, these three pathways can elicit the transcription of related genes in the nucleus, and ultimately cause translocation of DAMPs and ICD. The related pathways are shown in Figure 2. Of note, most current known inducers, such as chemotherapy and RT, are type I (Sen et al., 2022) and their immune efficacy needs to be enhanced by new biomaterials or combination therapies.
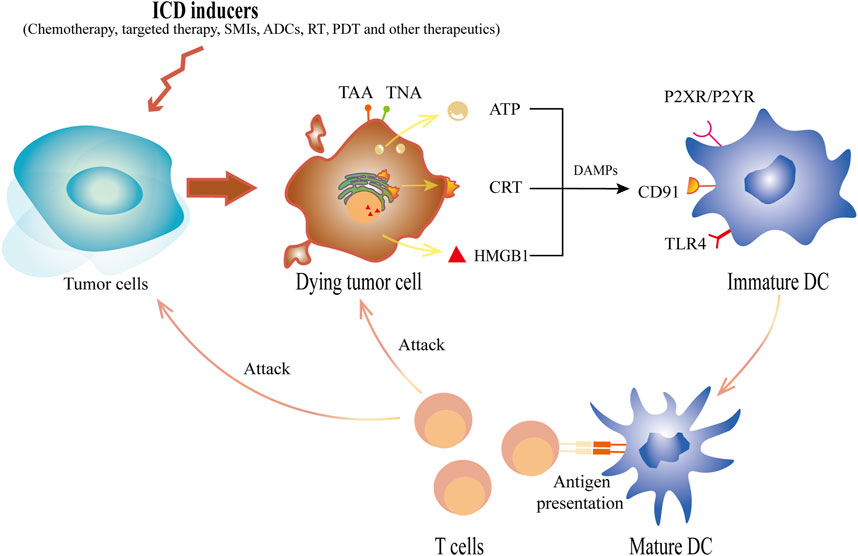
Figure 1. The schematic diagram of ICD inducers acting on tumor cells to induce ICD. ICD inducers, including chemotherapy, targeted therapy, SMIs, ADCs, RT, PDT and other therapeutics, cause tumor cells to die and express tumor antigens, TNAs or TAAs, on the cell surface. Concurrently, DAMPs released by tumor cells undergoing ICD can selectively bind to different specific receptors on DCs. In the early stage of ICD, ATP, one of the major player secreted by neoplastic cells, acts on the purinergic receptor P2XR and P2YR from DCs. Additionally, the translocation of CRT to the surface, which will be recognized by CD91, sends a “eat me” signal. Released in the late stage, HMGB1 binds with TLR-4 and initiates antigen presentation. Ultimately, these DAMPs initiate a cascade of antitumor reactions that lead to the recruitment, maturation and antigen presenting stimulation of DCs, thus eventually causing the polarization of T cells as well as in turn killing dying cells and other tumor cells. ICD, immunogenic cell death; RT, radiotherapy; TNAs, tumor neoantigens; TAAs, tumor-associated antigens; DAMPs, damage associated molecule patterns; DCs, dendritic cells; ATP, adenosine triphosphate; SMIs, small-molecule inhibitors; ADCs, antibody-drug conjugates; PDT, photodynamic therapy; CRT, calreticulin; HMGB1, high-mobility group protein box 1; TLR-4, Toll-like receptor 4.
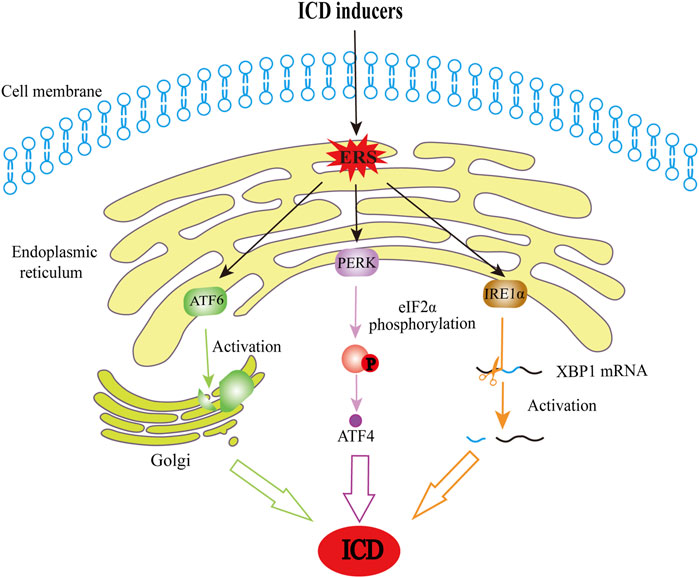
Figure 2. The schematic diagram of ERS-induced cell death. When affected by ICD inducers, cells are exposed to stresses that disturb protein folding and therefore lead to the production of ERS, a process that is also a critical step in ICD induction. In this regard, ERS activates IRE1α, and it plays a role with its endonuclease activity in splicing and activating XBP1 mRNA. PERK activation can induce phosphorylation of eIF2α, and phosphorylated eIF2α allows for selective translation of ATF4. Moreover, ATF6 will move to the Golgi apparatus and be cleaved into active fragments. These pathways constitute the main pathways through which ERS results in ICD. ICD, immunogenic cell death; ERS, endoplasmic reticulum stress; IRE1α, inositol-requiring kinase 1; XBP1, X-box-binding protein 1; PERK, protein kinase R-like ER kinase; eIF2α, eukaryotic initiation factor-2α; ATF4, activating transcription factor 4; ATF6, activating transcription factor 6.
2.3 Novel DAMPs
In addition to commonly known molecular patterns, a new type of immunoactive polypeptide, proTα, known as thymosin, is gradually being confirmed as a new member of the DAMP family and plays a key role in many aspects such as diagnosis, treatment, and prognosis (Birmpilis et al., 2022). Notably, not all molecular markers elicited by dying malignant cells play a role in pathways that inhibit tumor progression. Molecules that inhibit immunity also constitute the inhibitory TME. For example, prostaglandin E2 (PGE2) is an inhibitory DAMP that counteracts the function of immunostimulatory DAMPs (Hangai et al., 2016). Gemcitabine elicits an immunostimulatory DAMP release; however, it also triggers PGE2 release; therefore, a PGE2 blockade is needed to tip the balance between the two types of DAMPs and help induce ICD (Hayashi et al., 2020). Similarly, adenosine (ADO) is an immunosuppressive DAMP (Stultz and Fong, 2021). While, CD39 and CD73 are extracellular nucleotidases that can precisely regulate the content of ATP and ADO in the purinergic pathway of the tumor cell immune microenvironment and maintain the balance between antitumor immunity and immunosuppression (Bao and Xie, 2022).
By detecting DAMPs, researchers can gain a clearer understanding of the mechanisms underlying ICD and determine whether a drug can play an antitumor role by inducing ICD. Detection strategies for different DAMPs were described in previous reviews (Fucikova et al., 2020; Galluzzi et al., 2020). The detection of ICD hallmarks is critical to determine whether a cell death process is immunogenic or typical of apoptosis. For example, transgenic CD8+ T cells and natural killer cells co-cultured with tumor cells cause cytotoxic tumor cell death. The observation of ICD markers demonstrates that cytotoxic cell death is immunogenic (Minute et al., 2020). Additionally, the main components of ICD are of great use in identifying new drugs with immunogenic properties for potential clinical applications. Thus, DAMP signaling may be essential to select patient-specific chemotherapy and tumor-specific therapeutic strategies (Radogna et al., 2019). Notably, not all drugs are qualified inducers although some agents can cause potent intracellular stress and alter the relevant molecular markers (Kroemer et al., 2022; Liu X. et al., 2023).
3 ICD inducers and molecular mechanism
3.1 Chemotherapy
Traditional chemotherapeutic agents with immunogenic effects summarized in the literature include (but are not limited to) anthracyclines (such as doxorubicin (DOX), epirubicin, mitoxantrone, and idarubicin), platinum-based drugs (such as cisplatin and oxaliplatin), anti-microtubule drugs (such as paclitaxel and docetaxel), bortezomib, cyclophosphamide, 5-fluorouracil, and so on (Gmeiner, 2020; Marinello et al., 2018; Shi et al., 2023; Wu and Waxman, 2018; Zhai et al., 2023) (Table 1). Here, we introduce innovations and continuous modifications based on these identified drugs, as well as discoveries of existing agent functions.
3.1.1 Chemotherapy combination complex
The relatively low ICD intensity induced by some of the earliest chemical inducers prompted researchers to compensate for this shortcoming through technological improvements. For example, to enhance the immune regulatory function of platinum-based drugs through structural improvements. Kostrhunova et al. (2023) synthesized a series of platinum (IV) derivatives based on the platinum (II) complex, [PtII56MeSS], which contained diclofenac axial ligands, and compared their outcomes to those of cisplatin. This new platinum-based complex led to platinum-induced ICD and possessed the characteristics of diclofenac, with the presence of diclofenac increasing the selectivity and cytotoxicity of platinum on six human cancer cell line (cervical carcinoma HeLa, breast adenocarcinomas MDA-MB-231 and MCF-7, colon carcinoma HCT-116, ovarian carcinoma A2780 and A2780cisR), and one non-cancerous fibroblast cell line (MRC-5) (Kostrhunova et al., 2023). R,R-1,2 cyclohexane diaminepyrophosphato-platinum (II) (PT-112) is another promising platinum-based compound (Kepp and Kroemer, 2020). Based on platinum (II) and pyrophosphate, PT-112 confers strong and long-lasting protective effects on murine colorectal carcinoma CT26 and MC38 cells making it a suitable inducer (Yamazaki et al., 2020). A phase I trial demonstrated its safety in advanced solid tumors (Karp et al., 2022). Moreover, the antimetabolite agent trifluridine/tipiracil may delete type-2 tumor-associated macrophages (a type of immunosuppressive cell) to modulate the TME in various microsatellite stable colon carcinoma cell lines (CT26, SW620, Caco-2, and Colo-320) (Limagne et al., 2019).
An increasing number of drugs are found to perform their antitumor role through ICD. For example, chromomycin A5 (CA5) can serve as a bona fide ICD inducer, since it enhances the activation of APCs and T-cells in syngeneic mouse models vaccinated with metastatic melanoma B16-F10 cells (Florêncio et al., 2022). Additionally, pemetrexed is a multi-targeting antifolate antagonist established as the first-line chemotherapeutic drug for advanced lung adenocarcinoma, and mesothelioma which increases immune-regulatory genes and induces ICD (Mathew et al., 2018; Sumiyoshi et al., 2021).
3.1.2 Chemotherapy with nanotechnology
The application of nanotechnology has enhanced the strength of original drugs. Researchers organically assembled platinum (II) with novel norcantharidin co-delivery nanoparticles, and the dual synergistic antitumor activities of these nanoparticles were determined using an orthotopic 4T1 murine breast tumor model. These results strongly validated a promising nanotechnology delivering a chemo-immunotherapy paradigm for breast cancer treatment (Xiang et al., 2023). The same was true for other ICD-inducing agents. For example, DOX is a well-established ICD inducer that mediates immune responses through ROS, while ROS produced by DOX can be cleared by the redox functions of intracellular glutathione’s (GSH) (Ponsero et al., 2017). Jeon et al. (2023) encapsulated DOX into the core of a self-immolating polymer. These nanoparticles decomposed in the presence of ROS and formed a structure that strongly reacted with GSH, which effectively solved the problem of DOX-induced ROS-mediated immunogenicity decline caused by the GSH clearance of ROS in breast tumor cells (Jeon et al., 2023). Similarly, Hu et al. (2023) improved the tumor-resisting ability of DOX by depleting GSH in breast tumor cells. The difference is that the outer components of this nanocage can react directly with GSH without the need for other molecules to activate the structural transformation. In another study that combined aluminum hydroxide with DOX and tumor fragments, an improved nanovaccine showed a higher immune response and excellent inhibition of breast tumor growth (Tan et al., 2022). Nanotechnology is frequently used to combine two or more therapies to achieve synergistic effects. Researchers used nanotechnology to assemble DOX with near-infrared dyes to combine chemotherapy and photo-thermal therapy. This combination method significantly prolongs the median survival of breast cancer mouse models when compared to immunotherapy and ICD inducer alone, suggesting that this nanoparticle enhances antitumor immunotherapy (Zhu et al., 2023). Therefore, they provide further nanotechnology application references for combination therapies to improve immunotherapy. Other studies show that nanotechnology delivery systems with chemotherapy drugs may convert local immune tolerance (Zhang J. et al., 2021) and reduce systemic side effects (Moon et al., 2022).
3.2 Targeted therapy and small-molecule inhibitors (SMIs)
In recent years, further understanding of the ICD has stimulated researchers to discover and enhance the ICD-inducing role of targeted therapies. Increasing evidence suggests that many targeted agents exert their antitumor effects by inducing ICD. For example, osimertinib, an SMI known as an epidermal growth factor receptor (EGFR) tyrosine kinase inhibitor (TKI) demonstrates the potential to induce ICD in non-small cell lung cancer (NSCLC) tumor cells through the exposure and release of CRT (Furukawa et al., 2021). Lotsberg et al. (2020) reported that EGFR-TKI erlotinib-resistant cells can exhibit increased autophagic vacuolation and upregulation of the Anexelekto (AXL) receptor tyrosine kinase, whereas bemcentinib (which targets the AXL signaling pathway) can inhibit the transcription of genes associated with autophagy, release DAMPs, and trigger ICD in NSCLC cells. BI2536 is a selective PLK1 inhibitor that induces ICD to promote DC maturation and T-cell infiltration, thereby inducing apoptosis and altering the TME in NSCLC (Zhou et al., 2021). A mitogen-activated protein inhibitor drug, trametinib, could be effective in treating KRAS-mutant lung adenocarcinoma when combined with interleukin-12 by giving rise to ICD through sensitizing cancer cells to ERS and triggering the release of DAMPs (Wang et al., 2020). The EGFR-targeting antibody cetuximab, Bruton’s TKI ibrutinib, anaplastic lymphoma kinase TKI (crizotinib and ceritinib), mesenchymal-epithelial transition factor TKI foretinib, receptor protein TKI lestaurtinib, EGFR-TKI (lapatinib and canertinib), and CDK4/CDK6 inhibitors (abemaciclib and palbociclib) have also been demonstrated to present ICD activity before (Galluzzi et al., 2015; Inoue et al., 2017; Pozzi et al., 2016; Sagiv-Barfi et al., 2015; Schoenwaelder et al., 2021; Xu et al., 2022) (Table 1). Therefore, they have not been described in detail in this review.
There have also been many preclinical studies on SMIs and ICD in recent years. For example, CBL0137 (curaxin) targets histone chaperone FAcilitates Chromatin Transcription (FACT) to mainly interfere with the P53 and nuclear factor-κB (NF-κB) signaling pathways. Diffuse pleural mesothelioma cell lines treated with this SMI had a higher proportion of apoptotic cells and common cell cycle arrest compared to that seen in the control group. Simultaneously, high surface expression of important receptor signals and ICD markers during the long immune response were also observed (Singh et al., 2023). Waad et al. (2023) compared the ability of different SMIs to initiate type I IFN responses against multiple myeloma and identified PR619 (a proteasome inhibitor) as a novel ICD inducer.
The proposal of the ICD concept has also stimulated investigators to focus on the design of new targeted drugs to induce ICD. For example, researchers applied a CDK12/13 inhibitor (SR-4835) to mice with established 4T1 cells, a syngeneic mouse model of breast cancer, and found that SR-4835 can activate ERS, thus enhancing ICD. They also demonstrated that combining SR-4835 with anti-PD-1 can significantly inhibit tumor growth, providing a potential combination therapy for breast cancer (Li et al., 2020). Hwang et al. (2022) designed a phospholipase D (PLD) inhibitor to induce ICD to regulate TME in colorectal cancer, expecting to offer a promising strategy through this target drug. Moreover, researchers developed a novel fluorinated mitochondria-disrupting helical polypeptide to elicit mitochondrial dysfunction in murine colon adenocarcinoma CT26 cells. This resulted in ERS-mediated ICD, and suggested a synergistic effect with immunotherapy to reduce tumor cells numbers (Jeong et al., 2021). Lu et al. (2021) synthesized an activator (NBS-1MT) with a small molecule inhibitor and photosensitizer and found that it can induce oxidative stress to generate considerable ICD via pyroptosis in breast cancer cells. Although preclinical studies have laid a certain theoretical foundation, the clinical transformation of the combination of targeted therapy and ICIs is still not optimistic (Kroemer et al., 2023).
Taken together, targeted therapy exerts antitumor effects by interfering with various key steps in tumor formation. Although it has been investigated for decades, it still offers novel and innovative methods to improve antitumor efficacy. Identifying the most important targeting sites that can induce ICD is crucial to induce systemic immunity.
3.3 Antibody-drug conjugates (ADCs)
ADCs are new products that evolved during the process of targeted drug development (Hasan et al., 2018). ADCs are efficient drug delivery systems that combine targeted antibodies and cytotoxic antitumor drugs through linker molecules to achieve precise antitumoral effects. Like traditional targeted drugs, ADCs can activate ICD-related stress responses or signaling pathways. Heiser et al. (2023) applied Brentuximab Vedotin (an ADC targeting CD30) to the CD30-expressing lymphomas cell lines L540, HDLM-2, and A20, and tracking CRT provided evidence for the drug’s ICD-inducing properties. Mechanistically, the drug potently disrupts microtubule stability and leads to ERS, thereby activating ICD-related signaling pathways and immune cells (Heiser et al., 2023). PD-1 inhibition was subsequently combined to demonstrate the complementarity of the two approaches (Heiser et al., 2023). Similarly, the immunogenicity-inducing effects of another ADC drug targeting aberrantly expressed oncoproteins GPC2 (D3-GPC2-PBD) were explored. When applied to GPC2-expressing murine neuroblastomas, the drug caused changes in ICD markers such as CRT and HSP70/90, modulated the microenvironment, and improved macrophage phagocytosis, which was enhanced when CD47 was blocked by the combination (Pascual-Pasto et al., 2022). These examples suggest that ADCs are powerful ICD inducers with a promising future in combination therapies.
3.4 Radiotherapy
3.4.1 Limitation of traditional radiotherapy in ICD
RT has been shown to elicit ICD, a typical manifestation of which is abscopal effect (Galassi et al., 2023; Hannon et al., 2023) (Table 1). Abscopal effect refers to an immune-related phenomenon in metastatic tumors, irradiating the orthotopic tumor leads to the inhibitory effect on non-orthotopic tumor cells. However, traditional RT-induced systemic immune responses are rare and insufficient to meet clinical needs (Huang et al., 2021). First, cells undergoing RT can lead to an increased proportion of regulatory T lymphocytes in tumor-infiltrating lymphocytes (Manukian et al., 2021), still making the microenvironment with “cold” characteristics. Second, some small molecules can cause radioresistance. For example, in the pancreatic cancer model, myeloid MyD88 (a downstream TLR expressed by macrophages and other immune cells) disturbs the production of Type I IFN, which is essential for T-cell activation, thus restricting the immune response to RT (Medler et al., 2023). Sun et al. (2022) demonstrated that glioblastoma multiforme cells receiving RT depend on the STAT1-IRF1-CD39 axis to upregulate the expression of CD39 to contribute to radioresistance. In addition to these suppressive factors in the TME, hypoxia and insufficient irradiation absorption are major mechanisms of resistance. Radiation dose and quality are closely related to the effectiveness of the immune response. Therefore, it is essential to determine the modality of RT, the dose regimen, and how to combine other methods to improve the efficacy of RT-induced ICD.
3.4.2 Radiotherapy modalities
At present, heavy iron radiotherapy is an advanced RT method involving carbon ion and proton radiation that was successfully applied to cancer treatment. Compared to traditional photon therapy, heavy iron radiotherapy has stronger ICD potential and clinical application advantages. Carbon iron radiotherapy is an emerging type of ionizing radiation based on 12C6+. In addition to changes in ATP and the phosphorylation level of eIF2α, it leads to higher intranuclear HMGB1 efflux and mRNA level interferon expression in melanoma-bearing mice models compared with X-ray irradiation (Zhou et al., 2022). Proton radiation also presents a stronger tumor-killing effect on multiple human tumor cell lines (such as lung adenocarcinoma, glioma, tongue squamous carcinoma, and nasopharyngeal carcinoma), and has an equal effect to photon rays in inducing CRT exposure (Huang et al., 2019).
3.4.3 Radiation dose fractionated technique
Technologies such as stereotactic body radiotherapy (SBRT) and spatially fractionated radiotherapy (SFRT) have made RT more efficient and can induce more ICD. SBRT has high precision and targets tumors with a very high radiation dose. It shows that tumor tissues in pancreatic ductal adenocarcinoma treated with SBRT have positive outcomes in terms of tumor density and ICD induction, although it does not lead to a decrease in immunosuppressive cells (Mills et al., 2022). To reduce the risk of damage to surrounding healthy tissues, SFRT was also introduced for use in clinical practice. It can deliver relatively high but different radiation doses over different fractions. It is distinguished by its steep dose gradient, which enables irradiation of the central hypoxic area of the tumor at high doses while preserving the function of surrounding tissues to elicit potent ICD (Massaccesi et al., 2020).
3.4.4 Radiotherapy sensitizers
Several potential RT sensitizers have been identified to boost RT-induced ICD. For example, some compounds like Mn-based radiosensitizers 131I-MnO2-BSA mediate Fenton-like reactions to arrest the cell cycle and increase cell sensitivity to RT by regulating the cell cycle. Concurrently, it promotes the decomposition of hydrogen peroxide into O2 in breast and colon cancer model, thereby improving the resistance caused by the hypoxic environment (Zhang et al., 2022). Notably, improving the body’s ability to “correct errors” can also have a surprising effect. ATR inhibitors can inactivate serine/threonine protein kinases related to DNA repair, causing human lung cancer and osteosarcoma cells that fail to repair DNA to undergo mitotic death (Eek et al., 2023). Similarly, ER-associated protein degradation inhibitors can interfere with key proteins in the degradation of misfolded proteins, which is conducive to the amplification of mild ERS induced by RT in esophageal squamous cell carcinoma cell lines (Luo H. et al., 2023). In addition, some sensitizers can improve the efficacy of RT by enhancing key events in the RT-induced ICD process, such as inducing intracellular DNA damage (Zhuang et al., 2023), triggering ferroptosis (Luo et al., 2022), and activating ERS PERK-eIF2α and IRE1α-XBP1 signaling pathways (Xiu et al., 2022; Yang et al., 2020).
3.4.5 Nanotechnology radiotherapy
Nanotechnology has unique advantages when used to improve the efficacy of RT, such as achieving precise RT positioning. In experiments using murine melanoma B16 cells, Song et al. (2022) developed a gadolinium-based, highly efficient, guided irradiation nanoparticle to exacerbate radiation-induced DNA damage, cell cycle arrest, and ICD. In addition to improving immune function, the excellent radiation deposition and tumor penetration ability of nanomaterials were exploited to overcome the problem of low radiation absorption in tumor tissues (Janic et al., 2021; Wang X. et al., 2022). Gold nanoparticles (AuNPs) have promising radiosensitizing effects and can enhance RT-induced ICD in glioblastoma (He C. et al., 2023). Furthermore, the application of nanotechnology can result in an optimal combination of multiple drugs and therapies. Cisplatin enhances radiation-induced abscopal effects in melanoma, colon carcinoma and breast cancer (Luo R. et al., 2023). Cisplatin-based nanoparticles have a greater ability to enhance T cell infiltration and abscopal effects in Lewis lung carcinoma than cisplatin alone (Wang X. et al., 2021). Thus, a synergistic effect was achieved between chemotherapy and RT. Simultaneously, immunotherapy can also be used to generate a stronger antitumor effect (Choi et al., 2020; Song et al., 2022).
3.5 Photodynamic therapy
PDT is a treatment wherein the tumor tissue is dosed with a photosensitizer and locally irradiated with light of an appropriate wavelength to activate the photosensitizer and produce ROS that exert cytotoxic effects. Sun and collaborators demonstrated that the mechanism by which the photosensitizer B5-aminolevulinic acid (5-ALA) exerts antitumoral effects in mouse models of colorectal cancer, is achieved by ICD induction, depending on protein kinase B (AKT) inhibition and effective activation of bone-marrow derived dendritic cells (Sun et al., 2023). Thus, PDT can serve as a bona fide ICD inducer to inhibit tumor progression. Notably, different photosensitizer types, concentrations, and light doses can affect the PDT efficacy (Lin et al., 2022; Rodrigues et al., 2022).
Numerous issues are receiving considerable attention along the process. First, the body has a repair system for the DNA damage caused by ROS, which weakens the immune function of photosensitizers. To solve this problem in breast cancer, researchers have used poly (ADP-ribose) polymerase 1 (PARP1) inhibitors to disrupt the repair function and enhance the antitumor activity of photosensitizers (Li et al., 2023). The second problem that needs to be urgently solved is hypoxia and immunosuppression of the microenvironment caused by tumors, which are not conducive to ROS production. Wang et al. (2023) used nanotechnology to combine photosensitizers, the hypoxia-activated prodrug tirapazamine, and immunosuppressants. Oxygen-boosted PDT was also developed to overcome this limitation (Alzeibak et al., 2021). Moreover, targeting strategies can significantly improve the selectivity and efficacy of photodynamic agents in tumor tissues and have attracted extensive attention. Generally, these strategies can be divided into active and passive targets. Active targeting is associated with the structural characteristics of photodynamic agents that have a high affinity for tumor cells. Zeng et al. (2023) proposed a strategy for fabricating photosensitizers that precisely target the mitochondria, lysosomes, and ER in triple-negative breast cancer (TNBC) 4T1 cell lines to induce pyroptosis and selectively damage localized organelles based on cyanine chromophores and heavy atoms. Li J. et al. (2022) took advantage of the lipophilicity of phosphindole oxide to give full play to the aggregation-induced emission characteristics so that the novel photosensitizer could cross the cell membrane of cervical carcinoma HeLa cell and aggregate in the ER. However, passive targeting often involves the participation of nanomaterials, such as organic micelles (Liang et al., 2023), nano-metal frames (Zhang X. et al., 2021) and composite nanomaterials (Tian et al., 2023; Xiang et al., 2022).
3.6 Other potential inducers
3.6.1 Isolated natural drugs and traditional Chinese medicine
Multiple natural drugs or traditional Chinese medicines can induce ICD and inhibit tumor growth. The application of γ-mangostin to a leukemia mouse model shows that the size and weight of the mouse spleen is significantly reduced after its treatment, while the survival time of the mouse is significantly extended, indicating its benefit in leukemia treatment (Long et al., 2023). Further investigation demonstrates that this effect could be achieved by inducing ICD and activating the cGAS pathway (Long et al., 2023). Lepadin A is another natural compound with ICD inducing activity. In experiments using human melanoma A2058 cells, lepadin A induced higher CRT exposure and translocation, which was closely related to a CD91-dependent pathway that triggered the maturation and activation of DCs (Carbone et al., 2023). Afzelin is a flavonol glycoside found in various plants that can inhibit lung cancer progression by targeting NQO2, thereby activating ERS and inducing ICD (Xia et al., 2023). Therefore, natural drugs may provide benefits to patients with tumors in an ICD-inducing manner.
Notably, the emergence of the ICD concept may help researchers recognize the potantial clinical usage of traditional Chinese medicine in oncology therapeutic areas. Researchers (Sha et al., 2022) found that honey-processed Astragalus had increased antitumor immune activity in NSCLC, colon carcinoma and melanoma models compared to that seen with unprocessed Astragalus and the mechanism may be related to the induction of apoptosis and immunogenicity. In addition, the extract of the traditional Chinese medicine Marsdenia tenacissima activates ERS and ICD via AXL suppression to treat NSCLC (Yuan et al., 2023). Trametes robiniophila Murr. (Huaier) stimulates ICD in TNBC cells by promoting CRT exposure and increasing the release of ATP and HMGB1. Furthermore, Huaier may play a role in promoting ICD through the circCLASP1/PKR/eIF2α axis signaling pathway in vitro and in vivo (Li Z. et al., 2022). Self-assembled traditional Chinese nanomedicine using ursolic acid and lentinan can expand the ICD and improve the efficacy of immunotherapy in colorectal cancer (Mao et al., 2022). Licoricidin can affect the ERS of cervical cancer cells and further the release of DAMPs, thus trigger the emergence of ICD (Wu et al., 2023).
3.6.2 Microbiota therapy
It is widely acknowledged that microbiota plays an important role in mediating the efficacy of immunotherapy as it affects systemic immune responses. Investigators suggest that this effect (at least in part) is potentially mediated by ICD (Chen et al., 2020). With the help of nanotechnology, microbiota can be used as efficient carriers of antitumor drugs that can enhance the two-way killing effect of microbiota and drugs. Fusobacterium nucleatum can colonize TNBC and maintain the inhibitory properties of the TME. Based on this biological property, a nanomedicine was developed to precisely target and kill TNBC cells while also killing F. nucleatum, thereby regulating the immune microenvironment, which is conducive to turning “cold” tumors into “hot” tumors (Liu Z. et al., 2023). Moreover, researchers have used the anaerobic properties of Bifidobacterium infantis(B) as a loader for nanoparticles to selectively transport photosensitizers to the hypoxic region of colorectal cancer cells, providing a creative solution to solve the problems of tumor metastasis and drug resistance caused by hypoxia (Dai et al., 2023). Salmonella VNP20009 is a bacterial vector that plays the same role as the microbiota described above. Combining microbiota therapies with radiosensitizers achieves good biosafety while exhibiting an immune-friendly abscopal effect (Duo et al., 2023). The bacterial colonization of tumors also induces adaptive immune responses and exerts antitumor effects (Xie et al., 2023). Nanoparticles composed of Bifidobacteria and DOX, strategically combined bacterial therapy with chemotherapy, significantly inhibited melanoma tumor progression (He T. et al., 2023).
3.6.3 Tumor-treating fields (TTFields)
TTFields are new portable, non-invasive, physical anticancer therapies that interfere with tumor cell mitosis by acting on tubulin using a low-intensity, medium-frequency alternating current electric field. An increasing number of studies have focused on the potential effect of TTFields in eliciting ICD and the synergistic efficacy of TTFields with immunotherapy with the expectation of achieving better tumor control. For example, Voloshin et al. (2020) demonstrated that TTFields effectively potentiated ICD, and elicited a potent adaptive immune response through upregulation of DAMPs, including HMGB1, ATP, and CRT. Moreover, integrating TTFields with anti-PD-1 could enhance antitumoral immunity and achieve better tumor control in the murine colon CT-26 tumor model. Coincidentally, syngeneic NSCLC mouse models demonstrated that the presence of ICD in vivo during TTFields therapy concomitant with immunotherapy could alter the immune microenvironment and increase immune activity (Barsheshet et al., 2022). Moreover, the overall survival of TTFields plus standard therapy (including nivolumab, pembrolizumab, atezolizumab, and docetaxel) was significantly superior to that of standard therapy alone in a randomized phase 3 clinical trial of 276 patients with metastatic NSCLC who had been previously treated with platinum. In this context, TTFields is an alternative treatment for metastatic NSCLC that progresses with platinum-based drugs (Leal et al., 2023). Perhaps, the combination of TTFields and other therapeutic regimens will have broad application prospects in the treatment of many types of tumors.
Overall, these therapeutics mentioned above are still far from sufficient for clinical transformation. Therefore, in vivo experiments and clinical trials are urgently needed to exploit more potential bona fide ICD inducer candidates, combine existing diverse regimens, or design more novel polymers to meet clinical needs.
4 Clinical application
We summarized some of the completed trials published on the ClinicalTrials.gov database in recent years on ICD inducers in combination with immunotherapy from the ClinicalTrials.gov database in recent years, including chemical agents, novel RT, and target therapies (Table 2). Among them, eight studies related to chemotherapy with immunotherapy, seven studies related to radiotherapy with immunotherapy and three studies related to targeted therapy with immunotherapy. Multiple cancer types were included in these trials and a large portion of them focused on TNBC and lung cancer, while others included cervical cancer, uterine cancer, head and neck cancer, melanoma and lymphoma. Additionally, there were two “basket trials” consisting of multiple solid tumors. The role of ICD in clinical application is important but remains difficult to evaluate owing to limited induction protocols, inaccurate monitoring indicators, and limited access to samples (Fabian et al., 2022; Rapoport and Anderson, 2019). Most previous prospective clinical trials have used ICD as an exploratory endpoint and supported the beneficial effects of ICD in the prognosis of some patients with cancer (Fu et al., 2022; Wang Y. et al., 2022). Most of our summarized studies also evaluate ICD as an exploratory endpoint by the measurements of immunological parameters or investigated immune responses. From these studies, the effect of ICD is usually indirectly manifested through DAMPs or immune biomarkers (including, but not limited to, PD-L1 expression levels, T-cell density, and cytokine levels). Some of these trials confirmed the association of ICD with better clinical outcomes. Also, most of the summarized trials focus on combination regimens. For example, a clinical trail (NCT02622074) explored the antitumor activity of immunomodulatory chemotherapy regimens plus pembrolizumab in 60 patients with early-stage TNBC, finding a positive correlation between biomarker levels and clinical outcomes (Schmid et al., 2020). In another phase II randomized controlled trial (NCT03164993), to investigate the efficiency of adding immunotherapy to ICD inducers, researchers combined DOX with cyclophosphamide and atezolizumab to treat 68 patients with TNBC. Flow cytometry data showed that Treg counts reduced after low doses of metronomic cyclophosphamide, thus providing favorable evidence for the hypothesis that the semi-metronomic chemotherapy regimen sensitize patients to immunotherapy through ICD inducing and immunosuppressive cells reducing (Kyte et al., 2020; Røssevold et al., 2022). The trail (NCT02492568) enrolled 92 patients with advanced NSCLC, and the results showed that the objective response rate value was 36% in patients who received SBRT plus pembrolizumab versus 18% in patients who applied pembrolizumab alone. Overall, different combinations of ICD inducers and immunotherapies could potentially benefit patients through the ICD effect. These results emphasize the hopeful prospects and clinical directions in ICD research.
We also assessed clinical studies of ICD inducers in combination with immunotherapy currently underway some of which are presented in Table 3. Among them, eight studies related to chemotherapy with immunotherapy, nine studies related to radiotherapy with immunotherapy and 9 studies related to target therapy with immunotherapy. Unlike the completed studies described above, the ongoing clinical trials are predominately focused on colorectal cancer. Most trials of chemotherapy combined with immunotherapy use oxaliplatin as an interventional method, which is recognized as one of the most effective chemotherapy drugs for colorectal cancer as well as an effective ICD inducer. With respect to induction of ICD by novel radiation therapy, SBRT with ICIs was assessed in four trials, and there were two trials assessed heavy ion radiotherapy with ICIs. In these trials, multiple immunological markers were examined to predict the efficacy of immunotherapy during treatment.
5 Future development and perspectives
It is worth noting that different ICD inducers have their drawbacks. For example, chemotherapy has a risk of severe systemic side effects and drug resistance, while targeted therapy is highly selective. Although RT as a local treatment has relatively few side effects and can confer an abscopal effect on tumor cells at the irradiation site, it leads to an increased proportion of immunosuppressive cells. Thus, it is challenging to regulate its impact on the immune system. PDT has limited penetration and is only suitable for superficial tumors. To some extent, new technologies, such as nanotechnology, compensate for the inherent defects of these ICD inducers by enhancing the stability and efficacy of drugs, improving the precision of action, reducing side effects, and enabling the combination of multiple treatment methods. However, there are still some limitations that must be addressed. First, it is difficult to identify the patients who will benefit the most and those who will not benefit, although researchers have proposed various prognosis models for patient stratification, such as ICD-related gene expression level (Jiang et al., 2023; Wang Y. et al., 2021; Zhang et al., 2023). Based on the biological mechanisms of ICD, we hypothesize that patients with low immunogenic malignancies are less likely to benefit from it. In addition, an increased number of side effects may occur when multiple approaches are used simultaneously to manage tumors or when applied to older or frail individuals. Second, the development of DAMPs has had a disproportionate focus on established markers, and there are few novel markers. Third, most therapeutics do not consider ICD activation as their main mechanism of action, and it is not easy to compare the efficiency of ICD eliciting ability. Finally, limited attention has been paid to the induction protocol, the timing and the doses of the inducer. However, we believe that these current obstacles will be appropriately resolved shortly. With technological advances, the detection and clinical efficacy of ICD could be enhanced to better understand the potential role of ICD induction in combination with immunotherapy. Appropriate combination of regimens with ICD inducers with immunotherapy for different tumors will be developed, and a therapeutic strategy for immunosuppressive tumors will be formulated to better treat distal tumors and maintain memory immunity, allowing wider use of personalized treatment.
6 Conclusion
Although the clinical application of immunotherapy has prolonged the survival of patients with cancer to a considerable extent, the treatment of malignant tumors remains a major problem in medicine today. ICD induction has emerged as an effective enhancement method to address the limited use of immunotherapy for “cold” tumors, and it is expected to further improve patient outcomes. The process is often accompanied by antigenicity, the secretion of DAMPs, and the activation of conduction pathways to achieve alterations in the TME and systemic immune responses. As a feasible therapeutic strategy in antitumor therapy, further elucidating the mechanism of ICD and developing new combination of regimens will benefit an increasing number of patients with cancer.
Author contributions
YH: Writing–original draft. XT: Writing–original draft. JZ: Writing–original draft. ZZ: Conceptualization, Supervision, Validation, Writing–review and editing.
Funding
The author(s) declare that no financial support was received for the research, authorship, and/or publication of this article.
Conflict of interest
The authors declare that the research was conducted in the absence of any commercial or financial relationships that could be construed as a potential conflict of interest.
Publisher’s note
All claims expressed in this article are solely those of the authors and do not necessarily represent those of their affiliated organizations, or those of the publisher, the editors and the reviewers. Any product that may be evaluated in this article, or claim that may be made by its manufacturer, is not guaranteed or endorsed by the publisher.
References
Alzeibak, R., Mishchenko, T. A., Shilyagina, N. Y., Balalaeva, I. V., Vedunova, M. V., and Krysko, D. V. (2021). Targeting immunogenic cancer cell death by photodynamic therapy: past, present and future. J. Immunother. Cancer 9 (1), e001926. doi:10.1136/jitc-2020-001926
Apetoh, L., Ghiringhelli, F., Tesniere, A., Obeid, M., Ortiz, C., Criollo, A., et al. (2007). Toll-like receptor 4-dependent contribution of the immune system to anticancer chemotherapy and radiotherapy. Nat. Med. 13 (9), 1050–1059. doi:10.1038/nm1622
Azizi, M., Salehi-Mazandarani, S., Nikpour, P., Andalib, A., and Rezaei, M. (2023). The role of unfolded protein response-associated mirnas in immunogenic cell death amplification: a literature review and bioinformatics analysis. Life Sci. 314, 121341. doi:10.1016/j.lfs.2022.121341
Bao, X., and Xie, L. (2022). Targeting purinergic pathway to enhance radiotherapy-induced immunogenic cancer cell death. J. Exp. Clin. Cancer Res. 41 (1), 222. doi:10.1186/s13046-022-02430-1
Barsheshet, Y., Voloshin, T., Brant, B., Cohen, G., Koren, L., Blatt, R., et al. (2022). Tumor treating fields (ttfields) concomitant with immune checkpoint inhibitors are therapeutically effective in non-small cell lung cancer (nsclc) in vivo model. Int. J. Mol. Sci. 23 (22), 14073. doi:10.3390/ijms232214073
Birmpilis, A. I., Paschalis, A., Mourkakis, A., Christodoulou, P., Kostopoulos, I. V., Antimissari, E., et al. (2022). Immunogenic cell death, damps and prothymosin α as a putative anticancer immune response biomarker. Cells 11 (9), 1415. doi:10.3390/cells11091415
Carbone, D., Gallo, C., Nuzzo, G., Barra, G., Dell'Isola, M., Affuso, M., et al. (2023). Marine natural product lepadin a as a novel inducer of immunogenic cell death via cd91-dependent pathway. Nat. Product. Bioprospecting. 13 (1), 34. doi:10.1007/s13659-023-00401-3
Chen, W., Wang, S., Wu, Y., Shen, X., Guo, Z., Li, Q., et al. (2020). Immunogenic cell death: a link between gut microbiota and anticancer effects. Microb. Pathog. 141, 103983. doi:10.1016/j.micpath.2020.103983
Cherniavsky, L. M., Karlish, S. J., and Garty, H. (2015). Cardiac glycosides induced toxicity in human cells expressing α1-α2-or α3-isoforms of na-k-atpase. Am. J. Physiol.-Cell Physiol. 309 (2), C126–C135. doi:10.1152/ajpcell.00089.2015
Choi, B., Choi, H., Yu, B., and Kim, D. H. (2020). Synergistic local combination of radiation and anti-programmed death ligand 1 immunotherapy using radiation-responsive splintery metallic nanocarriers. ACS Nano 14 (10), 13115–13126. doi:10.1021/acsnano.0c04701
Dai, J., Li, J., Zhang, Y., Wen, Q., Lu, Y., Fan, Y., et al. (2023). Gm-csf augmented the photothermal immunotherapeutic outcome of self-driving gold nanoparticles against a mouse ct-26 colon tumor model. Biomater. Res. 27 (1), 105. doi:10.1186/s40824-023-00430-6
Duo, Y., Chen, Z., Li, Z., Li, X., Yao, Y., Xu, T., et al. (2023). Combination of bacterial-targeted delivery of gold-based aiegen radiosensitizer for fluorescence-image-guided enhanced radio-immunotherapy against advanced cancer. Bioact. Mat. 30, 200–213. doi:10.1016/j.bioactmat.2023.05.010
Eek, M. A., Hauge, S., Kongsrud, K., and Syljuåsen, R. G. (2023). Immunogenic cell death after combined treatment with radiation and atr inhibitors is dually regulated by apoptotic caspases. Front. Immunol. 14, 1138920. doi:10.3389/fimmu.2023.1138920
Fabian, K. P., Kowalczyk, J. T., Reynolds, S. T., and Hodge, J. W. (2022). Dying of stress: chemotherapy, radiotherapy, and small-molecule inhibitors in immunogenic cell death and immunogenic modulation. Cells 11 (23), 3826. doi:10.3390/cells11233826
Fabian, K. P., Wolfson, B., and Hodge, J. W. (2021). From immunogenic cell death to immunogenic modulation: select chemotherapy regimens induce a spectrum of immune-enhancing activities in the tumor microenvironment. Front. Oncol. 11, 728018. doi:10.3389/fonc.2021.728018
Florêncio, K., Edson, E. A., Fernandes, K., Luiz, J., Pinto, F., Pessoa, O., et al. (2022). Chromomycin a(5) induces bona fide immunogenic cell death in melanoma. Front. Immunol. 13, 941757. doi:10.3389/fimmu.2022.941757
Fu, J., Zhang, W., and Jiang, T. (2022). Immunogenic cell death mediation patterns reveal novel paradigm for characterizing the immune microenvironment and immunotherapeutic responses in bladder cancer. Front. Genet. 13, 1035484. doi:10.3389/fgene.2022.1035484
Fu, L., Zhou, X., and He, C. (2021). Polymeric nanosystems for immunogenic cell death-based cancer immunotherapy. Macromol. Biosci. 21 (7), e2100075. doi:10.1002/mabi.202100075
Fucikova, J., Kepp, O., Kasikova, L., Petroni, G., Yamazaki, T., Liu, P., et al. (2020). Detection of immunogenic cell death and its relevance for cancer therapy. Cell Death Dis. 11 (11), 1013. doi:10.1038/s41419-020-03221-2
Furukawa, R., Inoue, H., Yoneshima, Y., Tsutsumi, H., Iwama, E., Ikematsu, Y., et al. (2021). Cytotoxic chemotherapeutic agents and the egfr-tki osimertinib induce calreticulin exposure in non-small cell lung cancer. Lung Cancer 155, 144–150. doi:10.1016/j.lungcan.2021.03.018
Galassi, C., Klapp, V., Yamazaki, T., and Galluzzi, L. (2023). Molecular determinants of immunogenic cell death elicited by radiation therapy. Immunol. Rev. 321, 20–32. doi:10.1111/imr.13271
Galluzzi, L., Buqué, A., Kepp, O., Zitvogel, L., and Kroemer, G. (2015). Immunological effects of conventional chemotherapy and targeted anticancer agents. Cancer Cell 28 (6), 690–714. doi:10.1016/j.ccell.2015.10.012
Galluzzi, L., Vitale, I., Aaronson, S. A., Abrams, J. M., Adam, D., Agostinis, P., et al. (2018). Molecular mechanisms of cell death: recommendations of the nomenclature committee on cell death 2018. Cell Death Differ. 25 (3), 486–541. doi:10.1038/s41418-017-0012-4
Galluzzi, L., Vitale, I., Warren, S., Adjemian, S., Agostinis, P., Martinez, A. B., et al. (2020). Consensus guidelines for the definition, detection and interpretation of immunogenic cell death. J. Immunother. Cancer 8 (1), e000337. doi:10.1136/jitc-2019-000337
Galon, J., and Bruni, D. (2019). Approaches to treat immune hot, altered and cold tumours with combination immunotherapies. Nat. Rev. Drug Discov. 18 (3), 197–218. doi:10.1038/s41573-018-0007-y
Gmeiner, W. H. (2020). Fluoropyrimidine modulation of the anti-tumor immune response-prospects for improved colorectal cancer treatment. Cancers 12 (6), 1641. doi:10.3390/cancers12061641
Hanahan, D., and Weinberg, R. A. (2011). Hallmarks of cancer: the next generation. Cell 144 (5), 646–674. doi:10.1016/j.cell.2011.02.013
Hangai, S., Ao, T., Kimura, Y., Matsuki, K., Kawamura, T., Negishi, H., et al. (2016). Pge2 induced in and released by dying cells functions as an inhibitory damp. Proc. Natl. Acad. Sci. 113 (14), 3844–3849. doi:10.1073/pnas.1602023113
Hannon, G., Lesch, M. L., and Gerber, S. A. (2023). Harnessing the immunological effects of radiation to improve immunotherapies in cancer. Int. J. Mol. Sci. 24 (8), 7359. doi:10.3390/ijms24087359
Hasan, M., Alam, S., and Poddar, S. K. (2018). Antibody-drug conjugates: a review on the epitome of targeted anti-cancer therapy. Curr. Clin. Pharmacol. 13 (4), 236–251. doi:10.2174/1574884712666180802095521
Hayashi, K., Nikolos, F., Lee, Y. C., Jain, A., Tsouko, E., Gao, H., et al. (2020). Tipping the immunostimulatory and inhibitory damp balance to harness immunogenic cell death. Nat. Commun. 11 (1), 6299. doi:10.1038/s41467-020-19970-9
He, C., Ding, H., Li, L., Chen, J., Mo, X., Ding, Y., et al. (2023a). Gold nanoparticles enhance the ability of radiotherapy to induce immunogenic cell death in glioblastoma. Int. J. Nanomed. 18, 5701–5712. doi:10.2147/IJN.S419712
He, T., Wang, L., Gou, S., Lu, L., Liu, G., Wang, K., et al. (2023b). Enhanced immunogenic cell death and antigen presentation via engineered bifidobacterium bifidum to boost chemo-immunotherapy. ACS Nano 17 (11), 9953–9971. doi:10.1021/acsnano.2c11474
Heiser, R. A., Cao, A. T., Zeng, W., Ulrich, M., Younan, P., Anderson, M. E., et al. (2023). Brentuximab vedotin-driven microtubule disruption results in endoplasmic reticulum stress leading to immunogenic cell death and antitumor immunity. Mol. Cancer Ther. 23, 68–83. doi:10.1158/1535-7163.MCT-23-0118
Hu, J., Liang, M., Ye, M., Xu, J., Liu, H., Zhang, X., et al. (2023). Reduction-triggered polycyclodextrin supramolecular nanocage induces immunogenic cell death for improved chemotherapy. Carbohydr. Polym. 301 (Pt B), 120365. doi:10.1016/j.carbpol.2022.120365
Huang, Y., Dong, Y., Zhao, J., Zhang, L., Kong, L., and Lu, J. J. (2019). Comparison of the effects of photon, proton and carbon-ion radiation on the ecto-calreticulin exposure in various tumor cell lines. Ann. Transl. Med. 7 (20), 542. doi:10.21037/atm.2019.09.128
Huang, Z., Wang, Y., Yao, D., Wu, J., Hu, Y., and Yuan, A. (2021). Nanoscale coordination polymers induce immunogenic cell death by amplifying radiation therapy mediated oxidative stress. Nat. Commun. 12 (1), 145. doi:10.1038/s41467-020-20243-8
Hwang, W. C., Song, D., Lee, H., Oh, C., Lim, S. H., Bae, H. J., et al. (2022). Inhibition of phospholipase d1 induces immunogenic cell death and potentiates cancer immunotherapy in colorectal cancer. Exp. Mol. Med. 54 (9), 1563–1576. doi:10.1038/s12276-022-00853-6
Inoue, Y., Hazama, S., Suzuki, N., Tokumitsu, Y., Kanekiyo, S., Tomochika, S., et al. (2017). Cetuximab strongly enhances immune cell infiltration into liver metastatic sites in colorectal cancer. Cancer Sci. 108 (3), 455–460. doi:10.1111/cas.13162
Janic, B., Brown, S. L., Neff, R., Liu, F., Mao, G., Chen, Y., et al. (2021). Therapeutic enhancement of radiation and immunomodulation by gold nanoparticles in triple negative breast cancer. Cancer Biol. Ther. 22 (2), 124–135. doi:10.1080/15384047.2020.1861923
Jeon, J., Yoon, B., Dey, A., Song, S. H., Li, Y., Joo, H., et al. (2023). Self-immolative polymer-based immunogenic cell death inducer for regulation of redox homeostasis. Biomaterials 295, 122064. doi:10.1016/j.biomaterials.2023.122064
Jeong, S. D., Jung, B. K., Ahn, H. M., Lee, D., Ha, J., Noh, I., et al. (2021). Immunogenic cell death inducing fluorinated mitochondria-disrupting helical polypeptide synergizes with pd-l1 immune checkpoint blockade. Adv. Sci. 8 (7), 2001308. doi:10.1002/advs.202001308
Jhunjhunwala, S., Hammer, C., and Delamarre, L. (2021). Antigen presentation in cancer: insights into tumour immunogenicity and immune evasion. Nat. Rev. Cancer. 21 (5), 298–312. doi:10.1038/s41568-021-00339-z
Jiang, S., Dong, Y., Wang, J., Zhang, X., Liu, W., Wei, Y., et al. (2023). Identification of immunogenic cell death-related signature on prognosis and immunotherapy in kidney renal clear cell carcinoma. Front. Immunol. 14, 1207061. doi:10.3389/fimmu.2023.1207061
Karp, D. D., Camidge, D. R., Infante, J. R., Ames, T. D., Price, M. R., Jimeno, J., et al. (2022). Phase i study of pt-112, a novel pyrophosphate-platinum immunogenic cell death inducer, in advanced solid tumours. EClinicalMedicine 49, 101430. doi:10.1016/j.eclinm.2022.101430
Kepp, O., and Kroemer, G. (2020). A novel platinum-based chemotherapeutic inducing immunogenic cell death. OncoImmunology 9 (1), 1729022. doi:10.1080/2162402X.2020.1729022
Kobayashi, H., and Choyke, P. L. (2019). Near-infrared photoimmunotherapy of cancer. Accounts Chem. Res. 52 (8), 2332–2339. doi:10.1021/acs.accounts.9b00273
Kodumudi, K. N., Woan, K., Gilvary, D. L., Sahakian, E., Wei, S., and Djeu, J. Y. (2010). A novel chemoimmunomodulating property of docetaxel: suppression of myeloid-derived suppressor cells in tumor bearers. Clin. Cancer Res. 16 (18), 4583–4594. doi:10.1158/1078-0432.CCR-10-0733
Kostrhunova, H., McGhie, B. S., Markova, L., Novakova, O., Kasparkova, J., Aldrich-Wright, J. R., et al. (2023). Platinum(iv) derivatives of [pt(1s,2s-diaminocyclohexane)(5,6-dimethyl-1,10-phenanthroline)] with diclofenac ligands in the axial positions: a new class of potent multi-action agents exhibiting selectivity to cancer cells. J. Med. Chem. 66 (12), 7894–7908. doi:10.1021/acs.jmedchem.3c00269
Kroemer, G., Chan, T. A., Eggermont, A., and Galluzzi, L. (2023). Immunosurveillance in clinical cancer management. CA-Cancer J. Clin. 74, 187–202. doi:10.3322/caac.21818
Kroemer, G., Galassi, C., Zitvogel, L., and Galluzzi, L. (2022). Immunogenic cell stress and death. Nat. Immunol. 23 (4), 487–500. doi:10.1038/s41590-022-01132-2
Krysko, D. V., Garg, A. D., Kaczmarek, A., Krysko, O., Agostinis, P., and Vandenabeele, P. (2012). Immunogenic cell death and damps in cancer therapy. Nat. Rev. Cancer. 12 (12), 860–875. doi:10.1038/nrc3380
Kyte, J. A., Røssevold, A., Falk, R. S., and Naume, B. (2020). Alice: a randomized placebo-controlled phase ii study evaluating atezolizumab combined with immunogenic chemotherapy in patients with metastatic triple-negative breast cancer. J. Transl. Med. 18 (1), 252. doi:10.1186/s12967-020-02424-7
Leal, T., Kotecha, R., Ramlau, R., Zhang, L., Milanowski, J., Cobo, M., et al. (2023). Tumor treating fields therapy with standard systemic therapy versus standard systemic therapy alone in metastatic non-small-cell lung cancer following progression on or after platinum-based therapy (lunar): a randomised, open-label, pivotal phase 3 study. Lancet Oncol. 24 (9), 1002–1017. doi:10.1016/S1470-2045(23)00344-3
Li, C., Wang, X., Chen, T., Li, W., Zhou, X., Wang, L., et al. (2022a). Huaier induces immunogenic cell death via circclasp1/pkr/eif2α signaling pathway in triple negative breast cancer. Front. Cell. Dev. Biol. 10, 913824. doi:10.3389/fcell.2022.913824
Li, C., Zhang, Y., Yan, S., Zhang, G., Wei, W., Qi, Z., et al. (2021). Alternol triggers immunogenic cell death via reactive oxygen species generation. OncoImmunology 10 (1), 1952539. doi:10.1080/2162402X.2021.1952539
Li, J., Dai, J., Zhuang, Z., Meng, Z., Hu, J. J., Lou, X., et al. (2022b). Combining pd-l1 blockade with immunogenic cell death induced by aie photosensitizer to improve antitumor immunity. Biomaterials 291, 121899. doi:10.1016/j.biomaterials.2022.121899
Li, P., Du, Y., Qiu, J., Li, D., Li, G., and Shan, G. (2023). Nuclear parp1-targeted photosensitizer as a dual-mode dna-damaging agent and immune activator for tumor ablation. Adv. Healthc. Mat. 12, e2301517. doi:10.1002/adhm.202301517
Li, Y., Zhang, H., Li, Q., Zou, P., Huang, X., Wu, C., et al. (2020). Cdk12/13 inhibition induces immunogenic cell death and enhances anti-pd-1 anticancer activity in breast cancer. Cancer Lett. 495, 12–21. doi:10.1016/j.canlet.2020.09.011
Li, Z., Lai, X., Fu, S., Ren, L., Cai, H., Zhang, H., et al. (2022c). Immunogenic cell death activates the tumor immune microenvironment to boost the immunotherapy efficiency. Adv. Sci. 9 (22), e2201734. doi:10.1002/advs.202201734
Liang, Y., Wang, P. Y., Liu, Z. Y., Sun, H. F., Wang, Q., Sun, G. B., et al. (2023). Dual stimuli-responsive micelles for imaging-guided mitochondrion-targeted photothermal/photodynamic/chemo combination therapy-induced immunogenic cell death. Int. J. Nanomed. 18, 4381–4402. doi:10.2147/IJN.S410047
Limagne, E., Thibaudin, M., Nuttin, L., Spill, A., Derangère, V., Fumet, J. D., et al. (2019). Trifluridine/tipiracil plus oxaliplatin improves pd-1 blockade in colorectal cancer by inducing immunogenic cell death and depleting macrophages. Cancer Immunol. Res. 7 (12), 1958–1969. doi:10.1158/2326-6066.CIR-19-0228
Lin, X., Mao, D., and Bai, R. (2022). Comparison of three commercial photosensitizers for efficiency of inducing immunogenic cell death in anti-tumor immunotherapy. Nan Fang. Yi Ke Da Xue Xue Bao 42 (12), 1791–1798. doi:10.12122/j.issn.1673-4254.2022.12.06
Liu, X., Sun, M., Pu, F., Ren, J., and Qu, X. (2023a). Transforming intratumor bacteria into immunopotentiators to reverse cold tumors for enhanced immuno-chemodynamic therapy of triple-negative breast cancer. J. Am. Chem. Soc. 145, 26296–26307. doi:10.1021/jacs.3c09472
Liu, Z., Xu, X., Liu, K., Zhang, J., Ding, D., and Fu, R. (2023b). Immunogenic cell death in hematological malignancy therapy. Adv. Sci. 10 (13), e2207475. doi:10.1002/advs.202207475
Long, Z. J., Wang, J. D., Qiu, S. X., Zhang, Y., Wu, S. J., Lei, X. X., et al. (2023). Dietary γ-mangostin triggers immunogenic cell death and activates cgas signaling in acute myeloid leukemia. Pharmacol. Res. 197, 106973. doi:10.1016/j.phrs.2023.106973
Lotsberg, M. L., Wnuk-Lipinska, K., Terry, S., Tan, T. Z., Lu, N., Trachsel-Moncho, L., et al. (2020). Axl targeting abrogates autophagic flux and induces immunogenic cell death in drug-resistant cancer cells. J. Thorac. Oncol. 15 (6), 973–999. doi:10.1016/j.jtho.2020.01.015
Lu, Y., Xu, F., Wang, Y., Shi, C., Sha, Y., He, G., et al. (2021). Cancer immunogenic cell death via photo-pyroptosis with light-sensitive indoleamine 2,3-dioxygenase inhibitor conjugate. Biomaterials 278, 121167. doi:10.1016/j.biomaterials.2021.121167
Luo, H., Sun, Y., Wang, L., Liu, H., Zhao, R., Song, M., et al. (2023a). Targeting endoplasmic reticulum associated degradation pathway combined with radiotherapy enhances the immunogenicity of esophageal cancer cells. Cancer Biol. Ther. 24 (1), 2166763. doi:10.1080/15384047.2023.2166763
Luo, H., Wang, X., Song, S., Wang, Y., Dan, Q., and Ge, H. (2022). Targeting stearoyl-coa desaturase enhances radiation induced ferroptosis and immunogenic cell death in esophageal squamous cell carcinoma. OncoImmunology 11 (1), 2101769. doi:10.1080/2162402X.2022.2101769
Luo, R., Onyshchenko, K., Wang, L., Gaedicke, S., Grosu, A. L., Firat, E., et al. (2023b). Necroptosis-dependent immunogenicity of cisplatin: implications for enhancing the radiation-induced abscopal effect. Clin. Cancer Res. 29 (3), 667–683. doi:10.1158/1078-0432.CCR-22-1591
Manukian, G., Kivolowitz, C., DeAngelis, T., Shastri, A. A., Savage, J. E., Camphausen, K., et al. (2021). Caloric restriction impairs regulatory t cells within the tumor microenvironment after radiation and primes effector t cells. Int. J. Radiat. Oncol. Biol. Phys. 110 (5), 1341–1349. doi:10.1016/j.ijrobp.2021.02.029
Mao, Q., Min, J., Zeng, R., Liu, H., Li, H., Zhang, C., et al. (2022). Self-assembled traditional Chinese nanomedicine modulating tumor immunosuppressive microenvironment for colorectal cancer immunotherapy. Theranostics 12 (14), 6088–6105. doi:10.7150/thno.72509
Marinello, J., Delcuratolo, M., and Capranico, G. (2018). Anthracyclines as topoisomerase ii poisons: from early studies to new perspectives. Int. J. Mol. Sci. 19 (11), 3480. doi:10.3390/ijms19113480
Massaccesi, M., Boldrini, L., Piras, A., Stimato, G., Quaranta, F., Azario, L., et al. (2020). Spatially fractionated radiotherapy (sfrt) targeting the hypoxic tumor segment for the intentional induction of non-targeted effects: an in silico study to exploit a new treatment paradigm. Tech. Innov. Patient Support Radiat. Oncol. 14, 11–14. doi:10.1016/j.tipsro.2020.02.003
Mathew, M., Enzler, T., Shu, C. A., and Rizvi, N. A. (2018). Combining chemotherapy with pd-1 blockade in nsclc. Pharmacol. Ther. 186, 130–137. doi:10.1016/j.pharmthera.2018.01.003
Medler, T. R., Blair, T. C., Alice, A. F., Dowdell, A. K., Piening, B. D., Crittenden, M. R., et al. (2023). Myeloid myd88 restricts cd8(+) t cell response to radiation therapy in pancreatic cancer. Sci. Rep. 13 (1), 8634. doi:10.1038/s41598-023-35834-w
Medrano, R., Hunger, A., Catani, J., and Strauss, B. E. (2017). Uncovering the immunotherapeutic cycle initiated by p19Arf and interferon-β gene transfer to cancer cells: an inducer of immunogenic cell death. OncoImmunology 6 (7), e1329072. doi:10.1080/2162402X.2017.1329072
Mills, B. N., Qiu, H., Drage, M. G., Chen, C., Mathew, J. S., Garrett-Larsen, J., et al. (2022). Modulation of the human pancreatic ductal adenocarcinoma immune microenvironment by stereotactic body radiotherapy. Clin. Cancer Res. 28 (1), 150–162. doi:10.1158/1078-0432.CCR-21-2495
Minute, L., Teijeira, A., Sanchez-Paulete, A. R., Ochoa, M. C., Alvarez, M., Otano, I., et al. (2020). Cellular cytotoxicity is a form of immunogenic cell death. J. Immunother. Cancer 8 (1), e000325. doi:10.1136/jitc-2019-000325
Moon, Y., Shim, M. K., Choi, J., Yang, S., Kim, J., Yun, W. S., et al. (2022). Anti-pd-l1 peptide-conjugated prodrug nanoparticles for targeted cancer immunotherapy combining pd-l1 blockade with immunogenic cell death. Theranostics 12 (5), 1999–2014. doi:10.7150/thno.69119
Pascual-Pasto, G., McIntyre, B., Shraim, R., Buongervino, S. N., Erbe, A. K., Zhelev, D. V., et al. (2022). Gpc2 antibody-drug conjugate reprograms the neuroblastoma immune milieu to enhance macrophage-driven therapies. J. Immunother. Cancer 10 (12), e004704. doi:10.1136/jitc-2022-004704
Ponsero, A. J., Igbaria, A., Darch, M. A., Miled, S., Outten, C. E., Winther, J. R., et al. (2017). Endoplasmic reticulum transport of glutathione by sec61 is regulated by ero1 and bip. Mol. Cell. 67 (6), 962–973. doi:10.1016/j.molcel.2017.08.012
Pozzi, C., Cuomo, A., Spadoni, I., Magni, E., Silvola, A., Conte, A., et al. (2016). The egfr-specific antibody cetuximab combined with chemotherapy triggers immunogenic cell death. Nat. Med. 22 (6), 624–631. doi:10.1038/nm.4078
Radogna, F., Dicato, M., and Diederich, M. (2019). Natural modulators of the hallmarks of immunogenic cell death. Biochem. Pharmacol. 162, 55–70. doi:10.1016/j.bcp.2018.12.016
Rapoport, B. L., and Anderson, R. (2019). Realizing the clinical potential of immunogenic cell death in cancer chemotherapy and radiotherapy. Int. J. Mol. Sci. 20 (4), 959. doi:10.3390/ijms20040959
Rodrigues, M. C., de Sousa, J. W., Mundim, T., Vale, C., de Oliveira, J. V., Ganassin, R., et al. (2022). Induction of immunogenic cell death by photodynamic therapy mediated by aluminum-phthalocyanine in nanoemulsion. Pharmaceutics 14 (1), 196. doi:10.3390/pharmaceutics14010196
Rodriguez-Ruiz, M. E., Vitale, I., Harrington, K. J., Melero, I., and Galluzzi, L. (2020). Immunological impact of cell death signaling driven by radiation on the tumor microenvironment. Nat. Immunol. 21 (2), 120–134. doi:10.1038/s41590-019-0561-4
Røssevold, A. H., Andresen, N. K., Bjerre, C. A., Gilje, B., Jakobsen, E. H., Raj, S. X., et al. (2022). Atezolizumab plus anthracycline-based chemotherapy in metastatic triple-negative breast cancer: the randomized, double-blind phase 2b alice trial. Nat. Med. 28 (12), 2573–2583. doi:10.1038/s41591-022-02126-1
Sagiv-Barfi, I., Kohrt, H. E., Burckhardt, L., Czerwinski, D. K., and Levy, R. (2015). Ibrutinib enhances the antitumor immune response induced by intratumoral injection of a tlr9 ligand in mouse lymphoma. Blood 125 (13), 2079–2086. doi:10.1182/blood-2014-08-593137
Schmid, P., Salgado, R., Park, Y. H., Muñoz-Couselo, E., Kim, S. B., Sohn, J., et al. (2020). Pembrolizumab plus chemotherapy as neoadjuvant treatment of high-risk, early-stage triple-negative breast cancer: results from the phase 1b open-label, multicohort keynote-173 study. Ann. Oncol. 31 (5), 569–581. doi:10.1016/j.annonc.2020.01.072
Schoenwaelder, N., Salewski, I., Engel, N., Krause, M., Schneider, B., Müller, M., et al. (2021). The individual effects of cyclin-dependent kinase inhibitors on head and neck cancer cells-a systematic analysis. Cancers 13 (10), 2396. doi:10.3390/cancers13102396
Sen, S., Karoscik, K., Maier, E., and Arambula, J. F. (2023). Immunogenic cell death-inducing metal complexes: from the benchtop to the clinic. Curr. Opin. Chem. Biol. 73, 102277. doi:10.1016/j.cbpa.2023.102277
Sen, S., Won, M., Levine, M. S., Noh, Y., Sedgwick, A. C., Kim, J. S., et al. (2022). Metal-based anticancer agents as immunogenic cell death inducers: the past, present, and future. Chem. Soc. Rev. 51 (4), 1212–1233. doi:10.1039/d1cs00417d
Sha, X., Xu, X., Liao, S., Chen, H., and Rui, W. (2022). Evidence of immunogenic cancer cell death induced by honey-processed astragalus polysaccharides in vitro and in vivo. Exp. Cell Res. 410 (1), 112948. doi:10.1016/j.yexcr.2021.112948
Shi, F., Huang, X., Hong, Z., Lu, N., Huang, X., Liu, L., et al. (2023). Improvement strategy for immune checkpoint blockade: a focus on the combination with immunogenic cell death inducers. Cancer Lett. 562, 216167. doi:10.1016/j.canlet.2023.216167
Singh, A., Pruett, N., Dixit, S., Gara, S. K., Wang, H., Pahwa, R., et al. (2023). Targeting facilitates chromatin transcription complex inhibits pleural mesothelioma and enhances immunotherapy. J. Exp. Clin. Cancer Res. 42 (1), 304. doi:10.1186/s13046-023-02889-6
Sistigu, A., Yamazaki, T., Vacchelli, E., Chaba, K., Enot, D. P., Adam, J., et al. (2014). Cancer cell-autonomous contribution of type i interferon signaling to the efficacy of chemotherapy. Nat. Med. 20 (11), 1301–1309. doi:10.1038/nm.3708
Song, H., Sun, H., He, N., Xu, C., Wang, Y., Du, L., et al. (2022). Gadolinium-based ultra-small nanoparticles augment radiotherapy-induced t-cell response to synergize with checkpoint blockade immunotherapy. Nanoscale 14 (31), 11429–11442. doi:10.1039/d2nr02620a
Sprooten, J., Ceusters, J., Coosemans, A., Agostinis, P., De Vleeschouwer, S., Zitvogel, L., et al. (2019). Trial watch: dendritic cell vaccination for cancer immunotherapy. OncoImmunology 8 (11), e1638212. doi:10.1080/2162402X.2019.1638212
Stultz, J., and Fong, L. (2021). How to turn up the heat on the cold immune microenvironment of metastatic prostate cancer. Prostate Cancer Prostatic Dis. 24 (3), 697–717. doi:10.1038/s41391-021-00340-5
Sumiyoshi, I., Okabe, T., Togo, S., Takagi, H., Motomura, H., Ochi, Y., et al. (2021). High lymphocyte population-related predictive factors for a long-term response in non-small cell lung cancer patients treated with pemetrexed: a retrospective observational study. J. Transl. Med. 19 (1), 92. doi:10.1186/s12967-021-02761-1
Sun, T., Li, Y., Yang, Y., Liu, B., Cao, Y., and Yang, W. (2022). Enhanced radiation-induced immunogenic cell death activates chimeric antigen receptor t cells by targeting cd39 against glioblastoma. Cell Death Dis. 13 (10), 875. doi:10.1038/s41419-022-05319-1
Sun, Z., Zhao, M., Wang, W., Hong, L., Wu, Z., Luo, G., et al. (2023). 5-ala mediated photodynamic therapy with combined treatment improves anti-tumor efficacy of immunotherapy through boosting immunogenic cell death. Cancer Lett. 554, 216032. doi:10.1016/j.canlet.2022.216032
Tan, J., Ding, B., Zheng, P., Chen, H., Ma, P., and Lin, J. (2022). Hollow aluminum hydroxide modified silica nanoadjuvants with amplified immunotherapy effects through immunogenic cell death induction and antigen release. Small 18 (34), e2202462. doi:10.1002/smll.202202462
Tian, J., Wang, J., Xu, H., Zou, B., Chen, W., Liu, Y., et al. (2023). Nanoscale metal-organic framework delivers rapamycin to induce tissue immunogenic cell death and potentiates cancer immunotherapy. Nanomedicine 50, 102678. doi:10.1016/j.nano.2023.102678
Vaes, R., Hendriks, L., Vooijs, M., and De Ruysscher, D. (2021). Biomarkers of radiotherapy-induced immunogenic cell death. Cells 10 (4), 930. doi:10.3390/cells10040930
Vitale, I., Sistigu, A., Manic, G., Rudqvist, N. P., Trajanoski, Z., and Galluzzi, L. (2019). Mutational and antigenic landscape in tumor progression and cancer immunotherapy. Trends Cell Biol. 29 (5), 396–416. doi:10.1016/j.tcb.2019.01.003
Voloshin, T., Kaynan, N., Davidi, S., Porat, Y., Shteingauz, A., Schneiderman, R. S., et al. (2020). Tumor-treating fields (ttfields) induce immunogenic cell death resulting in enhanced antitumor efficacy when combined with anti-pd-1 therapy. Cancer Immunol. Immunother. 69 (7), 1191–1204. doi:10.1007/s00262-020-02534-7
Vonderheide, R. H. (2018). The immune revolution: a case for priming, not checkpoint. Cancer Cell 33 (4), 563–569. doi:10.1016/j.ccell.2018.03.008
Waad, S. Z., Brioli, A., Al-Abdulla, R., Çetin, G., Schütt, J., Murua, E. H., et al. (2023). Immunogenic cell death triggered by impaired deubiquitination in multiple myeloma relies on dysregulated type i interferon signaling. Front. Immunol. 14, 982720. doi:10.3389/fimmu.2023.982720
Wang, D., Cong, J., Fu, B., Zheng, X., Sun, R., Tian, Z., et al. (2020). Immunogenic chemotherapy effectively inhibits kras-driven lung cancer. Cancer Lett. 492, 31–43. doi:10.1016/j.canlet.2020.07.043
Wang, M., He, M., Zhang, M., Xue, S., Xu, T., Zhao, Y., et al. (2023). Controllable hypoxia-activated chemotherapy as a dual enhancer for synergistic cancer photodynamic immunotherapy. Biomaterials 301, 122257. doi:10.1016/j.biomaterials.2023.122257
Wang, X., Huang, H., Liu, X., Li, J., Wang, L., Li, L., et al. (2022a). Immunogenic cell death-related classifications in breast cancer identify precise immunotherapy biomarkers and enable prognostic stratification. Front. Genet. 13, 1052720. doi:10.3389/fgene.2022.1052720
Wang, X., Wu, S., Liu, F., Ki, D., Wang, X., Pan, D., et al. (2021a). An immunogenic cell death-related classification predicts prognosis and response to immunotherapy in head and neck squamous cell carcinoma. Front. Immunol. 12, 781466. doi:10.3389/fimmu.2021.781466
Wang, Y., Chen, J., Duan, R., Gu, R., Wang, W., Wu, J., et al. (2022b). High-z-sensitized radiotherapy synergizes with the intervention of the pentose phosphate pathway for in situ tumor vaccination. Adv. Mat. 34 (13), e2109726. doi:10.1002/adma.202109726
Wang, Y., Shen, N., Wang, Y., Li, M., Zhang, W., Fan, L., et al. (2021b). Cisplatin nanoparticles boost abscopal effect of radiation plus anti-pd1 therapy. Biomater. Sci. 9 (8), 3019–3027. doi:10.1039/d1bm00112d
Wang-Bishop, L., Wehbe, M., Shae, D., James, J., Hacker, B. C., Garland, K., et al. (2020). Potent sting activation stimulates immunogenic cell death to enhance antitumor immunity in neuroblastoma. J. Immunother. Cancer 8 (1), e000282. doi:10.1136/jitc-2019-000282
Wei, B., Pan, J., Yuan, R., Shao, B., Wang, Y., Guo, X., et al. (2021). Polarization of tumor-associated macrophages by nanoparticle-loaded escherichia coli combined with immunogenic cell death for cancer immunotherapy. Nano Lett. 21 (10), 4231–4240. doi:10.1021/acs.nanolett.1c00209
Wu, J., and Waxman, D. J. (2018). Immunogenic chemotherapy: dose and schedule dependence and combination with immunotherapy. Cancer Lett. 419, 210–221. doi:10.1016/j.canlet.2018.01.050
Wu, P. J., Chiou, H. L., Hsieh, Y. H., Lin, C. L., Lee, H. L., Liu, I. C., et al. (2023). Induction of immunogenic cell death effect of licoricidin in cervical cancer cells by enhancing endoplasmic reticulum stress-mediated high mobility group box 1 expression. Environ. Toxicol. 38 (7), 1641–1650. doi:10.1002/tox.23793
Xia, L., Xu, X., Li, M., Zhang, X., and Cao, F. (2023). Afzelin induces immunogenic cell death against lung cancer by targeting nqo2. BMC Complement. Med. Ther. 23 (1), 381. doi:10.1186/s12906-023-04221-3
Xiang, J., Liu, K., Xu, H., Zhao, Z., Piao, Y., Shao, S., et al. (2023). Dual synergistic tumor-specific polymeric nanoparticles for efficient chemo-immunotherapy. Adv. Sci. 10 (29), e2301216. doi:10.1002/advs.202301216
Xiang, Q., Yang, C., Luo, Y., Liu, F., Zheng, J., Liu, W., et al. (2022). Near-infrared ii nanoadjuvant-mediated chemodynamic, photodynamic, and photothermal therapy combines immunogenic cell death with pd-l1 blockade to enhance antitumor immunity. Small 18 (13), e2107809. doi:10.1002/smll.202107809
Xie, B., Zhao, H., Ding, Y. F., Wang, Z., Gao, C., Li, S., et al. (2023). Supramolecularly engineered conjugate of bacteria and cell membrane-coated magnetic nanoparticles for enhanced ferroptosis and immunotherapy of tumors. Adv. Sci. 10, e2304407. doi:10.1002/advs.202304407
Xiu, Z., Sun, T., Yang, Y., He, Y., Yang, S., Xue, X., et al. (2022). Curcumin enhanced ionizing radiation-induced immunogenic cell death in glioma cells through endoplasmic reticulum stress signaling pathways. Oxidative Med. Cell. Longev. 2022, 5424411. doi:10.1155/2022/5424411
Xu, J., Xiong, Y., Xu, Z., Xing, H., Zhou, L., and Zhang, X. (2022). From targeted therapy to a novel way: immunogenic cell death in lung cancer. Front. Med. 9, 1102550. doi:10.3389/fmed.2022.1102550
Yamazaki, T., Buqué, A., Ames, T. D., and Galluzzi, L. (2020). Pt-112 induces immunogenic cell death and synergizes with immune checkpoint blockers in mouse tumor models. OncoImmunology 9 (1), 1721810. doi:10.1080/2162402X.2020.1721810
Yang, W., Xiu, Z., He, Y., Huang, W., Li, Y., and Sun, T. (2020). Bip inhibition in glioma stem cells promotes radiation-induced immunogenic cell death. Cell Death Dis. 11 (9), 786. doi:10.1038/s41419-020-03000-z
Yu, Q., Li, X., Wang, J., Guo, L., Huang, L., Gao, W., et al. (2023). Recent advances in reprogramming strategy of tumor microenvironment for rejuvenating photosensitizers-mediated photodynamic therapy. Small 20, e2305708. doi:10.1002/smll.202305708
Yuan, Y., Guo, Y., Guo, Z. W., Hao, H. F., Jiao, Y. N., Deng, X. X., et al. (2023). Marsdenia tenacissima extract induces endoplasmic reticulum stress-associated immunogenic cell death in non-small cell lung cancer cells through targeting axl. J. Ethnopharmacol. 314, 116620. doi:10.1016/j.jep.2023.116620
Zeng, S., Chen, C., Zhang, L., Liu, X., Qian, M., Cui, H., et al. (2023). Activation of pyroptosis by specific organelle-targeting photodynamic therapy to amplify immunogenic cell death for anti-tumor immunotherapy. Bioact. Mat. 25, 580–593. doi:10.1016/j.bioactmat.2022.07.016
Zhai, J., Gu, X., Liu, Y., Hu, Y., Jiang, Y., and Zhang, Z. (2023). Chemotherapeutic and targeted drugs-induced immunogenic cell death in cancer models and antitumor therapy: an update review. Front. Pharmacol. 14, 1152934. doi:10.3389/fphar.2023.1152934
Zhang, J., Chen, C., Li, A., Jing, W., Sun, P., Huang, X., et al. (2021a). Immunostimulant hydrogel for the inhibition of malignant glioma relapse post-resection. Nat. Nanotechnol. 16 (5), 538–548. doi:10.1038/s41565-020-00843-7
Zhang, J., Yang, M., Fan, X., Zhu, M., Yin, Y., Li, H., et al. (2022). Biomimetic radiosensitizers unlock radiogenetics for local interstitial radiotherapy to activate systematic immune responses and resist tumor metastasis. J. Nanobiotechnol. 20 (1), 103. doi:10.1186/s12951-022-01324-w
Zhang, P., Zhang, H., Tang, J., Ren, Q., Zhang, J., Chi, H., et al. (2023). The integrated single-cell analysis developed an immunogenic cell death signature to predict lung adenocarcinoma prognosis and immunotherapy. Aging (Albany NY) 15 (19), 10305–10329. doi:10.18632/aging.205077
Zhang, X., Lu, Y., Jia, D., Qiu, W., Ma, X., Zhang, X., et al. (2021b). Acidic microenvironment responsive polymeric mof-based nanoparticles induce immunogenic cell death for combined cancer therapy. J. Nanobiotechnol. 19 (1), 455. doi:10.1186/s12951-021-01217-4
Zhang, Y., and Zhang, Z. (2020). The history and advances in cancer immunotherapy: understanding the characteristics of tumor-infiltrating immune cells and their therapeutic implications. Cell. Mol. Immunol. 17 (8), 807–821. doi:10.1038/s41423-020-0488-6
Zhou, H., Tu, C., Yang, P., Li, J., Kepp, O., Li, H., et al. (2022). Carbon ion radiotherapy triggers immunogenic cell death and sensitizes melanoma to anti-pd-1 therapy in mice. OncoImmunology 11 (1), 2057892. doi:10.1080/2162402X.2022.2057892
Zhou, J., Yang, Q., Lu, L., Tuo, Z., Shou, Z., and Cheng, J. (2021). Plk1 inhibition induces immunogenic cell death and enhances immunity against nsclc. Int. J. Med. Sci. 18 (15), 3516–3525. doi:10.7150/ijms.60135
Zhu, H., Yang, K., Yao, H., Chen, X., Yan, S., He, Y., et al. (2023). Multifunctional nanoplatform-mediated chemo-photothermal therapy combines immunogenic cell death with checkpoint blockade to combat triple-negative breast cancer and distant metastasis. Int. J. Nanomed. 18, 3109–3124. doi:10.2147/IJN.S408855
Zhu, M., Yang, M., Zhang, J., Yin, Y., Fan, X., Zhang, Y., et al. (2021). Immunogenic cell death induction by ionizing radiation. Front. Immunol. 12, 705361. doi:10.3389/fimmu.2021.705361
Zhuang, Y., Wang, Y., Liu, C., Li, S., Du, S., and Li, G. (2023). Yes-associated protein 1 inhibition induces immunogenic cell death and synergizes with radiation and pd-1 blockade. Int. J. Radiat. Oncol. Biol. Phys. 116 (4), 894–905. doi:10.1016/j.ijrobp.2022.12.045
Glossary
Keywords: immunogenic cell death (ICD), tumor microenvironment (TME), cancer immunotherapy, immunosuppressive tumors, inducers, clinical application
Citation: Han Y, Tian X, Zhai J and Zhang Z (2024) Clinical application of immunogenic cell death inducers in cancer immunotherapy: turning cold tumors hot. Front. Cell Dev. Biol. 12:1363121. doi: 10.3389/fcell.2024.1363121
Received: 29 December 2023; Accepted: 23 April 2024;
Published: 07 May 2024.
Edited by:
Giulia Petroni, University of Florence, ItalyReviewed by:
Karthik Dhatchinamoorthy, University of Massachusetts Medical School, United StatesMartina Musella, Catholic University of the Sacred Heart, Rome, Italy
Copyright © 2024 Han, Tian, Zhai and Zhang. This is an open-access article distributed under the terms of the Creative Commons Attribution License (CC BY). The use, distribution or reproduction in other forums is permitted, provided the original author(s) and the copyright owner(s) are credited and that the original publication in this journal is cited, in accordance with accepted academic practice. No use, distribution or reproduction is permitted which does not comply with these terms.
*Correspondence: Zhenyong Zhang, enp5enotZG9jQDE2My5jb20=
†These authors have contributed equally to this work