- 1Department of Pediatrics, University of Minnesota, Minneapolis, MN, United States
- 2Stem Cell Institute, University of Minnesota, Minneapolis, MN, United States
- 3Lillehei Heart Institute, University of Minnesota, Minneapolis, MN, United States
- 4Muscular Dystrophy Center, University of Minnesota, Minneapolis, MN, United States
Producing an adequate number of muscle stem cells (MuSCs) with robust regenerative potential is essential for the successful cell therapy of muscle-wasting disorders. We have recently developed a method to produce skeletal myogenic cells with exceptional engraftability and expandability through an in vivo pluripotent stem cell (PSC) differentiation approach. We have subsequently mapped engraftment and gene expression and found that leukemia inhibitory factor receptor (Lifr) expression is positively correlated with engraftability. We therefore investigated the effect of LIF, the endogenous ligand of LIFR, on cultured MuSCs and examined their engraftment potential. We found that LIF-treated MuSCs exhibited elevated expression of PAX7, formed larger colonies from single cells, and favored the retention of PAX7+ “reserve cells” upon myogenic differentiation. This suggested that LIF promoted the maintenance of cultured MuSCs at a stem cell stage. Moreover, LIF enhanced the engraftment capability of MuSCs that had been expanded in vitro for 12 days by 5-fold and increased the number of MuSCs that repopulated the stem cell pool post-transplantation. These results thereby demonstrated the effectiveness of our in vivo PSC differentiation platform to identify positive regulators of the engraftability of cultured MuSCs.
1 Introduction
Cell therapy is an attractive therapeutic strategy for chronic diseases due to its promise to replace damaged tissues with new healthy donor cells. For Duchenne muscular dystrophy (DMD) which can be caused by any 1 of the more than 2000 different mutations in the DMD gene, cell therapy offers potential benefits regardless of the exact genetic mutation which can vary from patient to patient (Blau and Daley, 2019; Biressi et al., 2020). However, DMD cell therapy has its own unique challenges. A prominent problem is the difficulty of obtaining an ideal donor cell type that is both expandable to vast amounts for clinical use and engraftable to form new fibers after transplantation (Blau and Daley, 2019; Verhaart and Aartsma-Rus, 2019; Biressi et al., 2020). Muscle stem cells (MuSCs), also known as satellite cells, are an endogenous cell population responsible for maintaining the lifetime integrity of skeletal muscles against wear and tear (Günther et al., 2013; von Maltzahn et al., 2013). MuSCs have tremendous regenerative potential in vivo, with a single MuSC capable of regenerating hundreds of fibers (Collins et al., 2005; Sacco et al., 2008). Nevertheless, their clinical applications remain limited due to 2 main reasons. First, MuSCs are scarce and cannot be obtained at a therapeutically meaningful quantity from small muscle biopsies (Roth et al., 2000). An in vitro expansion step is inevitable. Second, MuSCs after in vitro expansion lose their regenerative potential dramatically, such that transplantation of hundreds of thousands of expanded MuSCs can merely give rise to a few hundred fibers (Montarras et al., 2005; Sacco et al., 2008; Xie et al., 2021). There is an urgent need to produce MuSCs that are both expandable and engraftable.
We have recently developed an in vivo pluripotent stem cell (PSC) differentiation method to produce skeletal myogenic cells that are both expandable and engraftable (Chan et al., 2018; Xie et al., 2021; Xie et al., 2023). These skeletal myogenic cells are highly efficient in forming new fibers and reconstituting the MuSC niche upon transplantation, and they can be expanded in vitro for over a month while maintaining high engraftability (Chan et al., 2018; Xie et al., 2021; Xie et al., 2023). Moreover, the development of the skeletal myogenic lineage during in vivo PSC differentiation closely recapitulates embryonic skeletal myogenesis (Pappas et al., 2022). These observations therefore suggest that in vivo PSC differentiation is effective not only as a method to produce engraftable skeletal myogenic cells, but also as a unique platform to study how the engraftability of skeletal myogenic cells is determined. Given the unmet challenge of producing a skeletal myogenic cell type that is both engraftable and expandable, in vivo PSC differentiation offers an invaluable approach to discover factors that promote the engraftability of expanded MuSCs.
In the current study, we have used in vivo PSC differentiation to identify leukemia inhibitory factor (LIF) as a potential regulator of skeletal myogenic engraftment. We subsequently validated our discovery in showing that LIF substantially improved the engraftment potential of expanded MuSCs that have been cultured for 12 days over 3 passages.
2 Materials and methods
2.1 Animals
All procedures involving animals including animal housing, husbandry, and experiments were reviewed and approved by the University of Minnesota Institutional Animal Care and Use Committee with AAALAC accreditation according to protocols (#2201-39776A). Male and female wildtype BL6 (C57BL/6J, Jackson Laboratory, Bar Harbor, ME) and H2B-GFP mice (B6.Cg-Tg (HIST1H2BB/EGFP)1 Pa/J, Jackson Laboratory) at 3–5 months old were used to obtain MuSCs. Transplantation experiments were performed on both male and female 3–5 months old NSG-mdx4cv mice as described previously (Arpke et al., 2013).
2.2 Muscle stem cell isolation
Hindlimb muscles were harvested and chopped into ∼2 mm pieces. The chopped muscle pieces were then incubated in a primary digestion buffer containing Dulbecco’s Minimum Essential Medium/High Glucose (DMEM, HyClone #SH30243.01, Logan, UT), 2 mg/mL Collagenase II (Gibco #17101-015, Gaithersburg, MD), and 1% penicillin/streptomycin (P/S) (Life Technologies #15140-122, Grand Island, NY) on a shaker at 250 rpm, 37 C for 1 h. Primary digestion was then halted by the addition of rinsing buffer consisting of Ham’s/F-10 medium (Caisson Labs #HFL01, Smithfield, UT), 10% horse serum (HyClone #SH30074.03), 1% HEPES buffer solution (Caisson Labs #HOL06), and 1% P/S and then centrifuged at 500 g for 10 min at 4°C. The tissues were then subjected to further enzymatic digestion consisting of rinsing buffer supplemented with 0.1 mg/mL Collagenase II and 0.5 mg/mL Dispase (Gibco #17105-041). This secondary digestion process continued for 30 min on a shaker at 250 rpm, 37°C. The digested tissues were then repeatedly drawn and released into a 10 mL syringe with a 16-gauge needle (4 times) followed by an 18-gauge needle (4 times) to facilitate additional dissociation. The resultant cellular suspension was filtered through a 100 μm cell strainer, spun down, resuspended in rinsing buffer, filtered through a 40 μm cell strainer, and then spun down again. Isolation of muscle stem cells from transplanted tibialis anterior muscles (see below) was performed similarly, except without passing through the 16-gauge needle nor the 100 μm cell strainer.
2.3 Fluorescence-activated cell sorting (FACS)
Isolated cells were incubated on ice for 1 h with fluorophore-conjugated antibodies for FACS (fluorescence-activated cell sorting). After 1 h, the cells were washed twice and resuspended in FACS buffer (PBS (HyClone #SH30256.01), 0.2% fetal bovine serum (FBS), and 1 μg/mL propidium iodide (PI, Sigma-Aldrich #P4170, St Louis, MO). The addition of PI into FACS Buffer served as a live/dead cell indicator, and only viable cells (PI−) were quantified. Cells were sorted into medium and kept on ice until they were cultured as described below. The antibodies utilized for sorting muscle stem cells (each at concentration of 0.5 μL per million cells) were PE-Cy7 anti-CD31 (BioLegend #102418, RRID: AB_830757, San Diego, CA), PE-Cy7 anti-CD45 (BioLegend #103114, RRID: AB_312979), APC anti-α7-Integrin (AbLab #67-0010-05, Vancouver, Canada), and PE anti-VCAM-1 (BioLegend #105714; RRID: AB_1134164). MuSCs are defined as CD31– CD45– α7-Integrin+ VCAM-1+ (α7-Integrin+ VCAM+). Cell analysis and sorting were executed using BD FACSAriaII instrument (BD Biosciences, San Diego, CA) operated with FACSDiva software (BD Biosciences). A four-way purity precision was employed for bulk sorting, while single-cell precision was implemented when sorting individual cells into 96-well plates for clonal analysis. FACS plots depicting the distribution of cellular populations were generated with FlowJo software (FLOWJO LLC, Ashland, OR).
2.4 In vitro cell expansion and differentiation
FACS-sorted α7-Integrin+ VCAM+ cells were plated in 0.1% gelatin-coated wells and cultured in myogenic expansion medium (Ham’s/F-10, 20% FBS (Sigma-Aldrich #F0926), 10 ng/mL basic FGF (R&D Systems #233- FB/CF, Minneapolis, MN), 1% P/S, 2 mM Glutamax (Life Technologies #35050-061, Paisley, PA), and 0.1 mM β-mercaptoethanol) with or without LIF (1,000 units/mL, Sigma-Aldrich #ESG1107). Cells were passaged once they reached ∼60% confluency. For differentiation experiments, cells at 70%–80% confluency were switched to myogenic differentiation medium (DMEM, 4% horse serum, 2 mM Glutamax, 1 mM sodium pyruvate (Life Technologies #11360070), 1 μg/mL insulin (GeminiBio #800122, West Sacramento, CA), 1 μM dexamethasone (Hello Bio #HB2521, Princeton, NJ), and 1% P/S) for 3 days.
2.5 Clonal analysis
One α7-Integrin+ VCAM+ cell per well was seeded via FACS into 0.1% gelatin-coated 96-well plates in myogenic clonal medium containing DMEM/F12 (Cellgro #15-090-CV, Manassas, VA), 20% FBS, 10% horse serum, 10 ng/mL basic FGF, 1% P/S, 2 mM Glutamax, and 0.5% chick embryo extract (US Biological #C3999, Salem, MA) with or without LIF (1,000 units/mL). Cells were incubated for 7 days undisturbed before analysis.
2.6 Cell transplantation
Two days prior to cell transplantation, the hindlimbs of recipient NSG-mdx4cv mice were exposed to 1,200 cGy X-Ray irradiation. One day prior to transplantation, the recipients’ irradiated tibialis anterior (TA) muscles were injected with 15 μm of cardiotoxin (10 μM, Latoxan #L8102, France) to promote engraftment. For each recipient mouse, 40,000 donor cells were resuspended in PBS (total 10 μL) and injected into the TA muscle using a Hamilton syringe (Hamilton, Reno, NV). Recipients were anesthetized with ketamine (150 mg/kg, Dechra Veterinary Products, NDC: 59399-114-10, Overland Park, KS) and xylazine (10 mg/kg, Bimeda, NDC: 59399-111-50, Cambridge, Canada) intraperitoneally prior to each procedure. Transplanted TA muscles were harvested 4 weeks later for further analysis.
2.7 Sectioning of transplanted TA muscles
Harvested TA muscles were embedded in optimal cutting temperature (OCT) solution (Scigen #4586, Gardena, CA). The cryomold containing the specimens were snap-frozen on 2-methylbutane (Sigma-Aldrich #320404) pre-cooled with liquid nitrogen and stored at −80°C. Cryosections of 10 μm were cut on a Leica CM3050 S cryostat (Leica Microsystems, Buffalo Grove, IL) and collected onto glass slides for immunostaining.
2.8 Immunostaining on cultured cells and muscle sections
For cultured cells, cells were fixed with 4% paraformaldehyde (Sigma-Aldrich #P6148) for 1 h. Cell membranes were permeabilized with 0.3% Triton X-100 (Sigma-Aldrich #X100) followed by blocking with 3% BSA (Thermo Fisher Scientific #BP1605, Waltham, MA). Cells were incubated overnight at 4°C with primary antibodies in 3% BSA. The next day, cells were incubated for 1 h at room temperature with secondary antibodies. Nuclei were stained with 4’, 6-diamidino-2-phenylindole (DAPI) (Life Technologies #D3571) for 10 min. For sections, samples were mounted with Immu-Mount (Thermo Fisher Scientific #9990402). Primary antibodies used were mouse anti-PAX7 (1:10, Developmental Studies Hybridoma Bank (DSHB) #PAX7, RRID: AB_395942, Iowa City, IA), mouse anti-myosin heavy chain (MHC) (1:20, DSHB #MF-20, RRID: AB_427788), mouse anti-MYOD1 (1:100, BD Pharmingen #554130, RRID: AB_395255, Franklin Lakes, NJ), rabbit anti-DYSTROPHIN (1:250, Abcam #ab15277; RRID: AB_301813, Cambridge, United Kingdom), and mouse anti-laminin (1:500, Sigma-Aldrich #L8271; RRID: AB_477162). Secondary antibodies (each 1:1,000) used were: goat anti-mouse IgG1 Alexa Fluor 555 (Life Technologies, #A21127, RRID: AB_2535769), goat anti-mouse IgG2b Alexa Fluor 647 (Life Technologies, #A21242, RRID: AB_ 2535811), goat anti-rabbit Alexa Fluor 555 (Life Technologies, #121429, RRID: AB_2535850), goat anti-mouse Alexa Fluor 647 (Life Technologies, #A21235, RRID: AB_2535804), and goat-anti mouse IgG2b Alexa Fluor 488 (Life Technologies, #121141, RRID: AB_ 2535778).
2.9 Imaging and analysis
Fluorescent imagery was acquired utilizing a Zeiss AxioObserver Z1 inverted microscope paired with an AxioCamMR3 camera (Jena, Germany). Subsequent image processing and quantification procedures were executed with Fiji/ImageJ software (U.S. National Institute of Health, Bethesda, MD). Whole TA images were captured in tile mode and stitched together using ZEN software (Zeiss). Fiber engraftment is defined as the total cross-sectional area of DYSTROPHIN+ fibers over the total cross-sectional area of the whole TA, measured via Muscle2View, a CellProfiler pipeline (Sanz et al., 2019), with adjusted parameters.
2.10 Quantitative RT-PCR
Cells at 70%–80% confluency were harvested for gene expression analysis. Total RNA was extracted using Quick-RNA Miniprep Kit (Zymo Research #R1055, Orange, CA). Genomic DNA removal and reverse transcription (RT) were performed using Verso cDNA Synthesis Kit (Thermo Fisher Scientific #AB1453A). Quantitative reverse transcription polymerase chain reaction (qRT-PCR) was performed in triplicates using TB Premix Ex Taq II (Takara Bio, #RR390A, Japan) in a QuantStudio 6 Flex Real-Time PCR System using QuantStudio Real-Time PCR Software (both Applied Biosystems, Waltham, MA). TaqMan probes used were: Pax7: Mm00834079_m1, Klf5: Mm00456521_m1, Myf5: Mm00435125_m1, Myod1: Mm00440387_m1, Myog: Mm00446194_m1, and Gapdh: Mm99999915_g1 (all Thermo Fisher Scientific). The ΔΔCt method was utilized to calculate gene expression relative to that of the housekeeping gene Gapdh in control samples.
2.11 ATP assay
The ATP assay was performed on LIF-treated and untreated MuSCs using the CellTiter-Glo Luminescent Cell Viability Assay kit (Promega #G7572, Madison, WI) according to the manufacturer’s manual. Luminescence was measured using a Cytation 3 plate reader (BioTek, Winooski, VT).
2.12 Statistical analysis
The RNA-seq dataset in Figure 1 was assessed from GEO: GSE182508. Data are presented as mean ± SEM. Differences between two groups were assessed using Student’s t-test. Differences among three or more groups were assessed by ANOVA with Tukey’s post hoc tests. p values <0.05 were considered significant (*: p < 0.05, **: p < 0.01, and ***: p < 0.001). GraphPad Prism (GraphPad Software, La Jolla, CA) was used to perform statistical analyses.
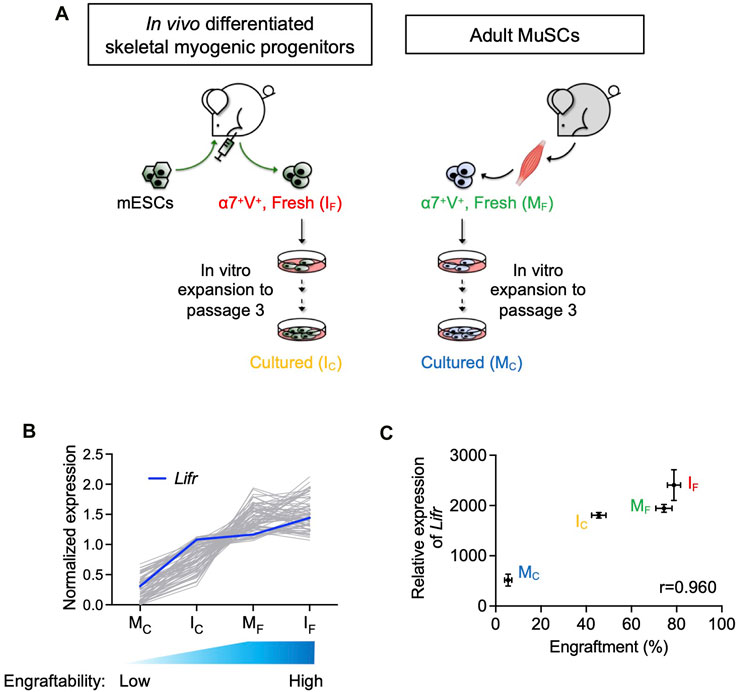
FIGURE 1. Lifr expression was positively correlated with the engraftability of skeletal myogenic cells. (A) Schematic of the 4 skeletal myogenic populations (α7-Integrin+ VCAM+, or α7+V+) used for RNA-seq analysis: freshly isolated (IF) and cultured (IC) in vivo differentiated skeletal myogenic progenitors and freshly isolated (MF) and cultured (MC) adult MuSCs (2 biological replicates from each group) (Xie et al., 2021). (B) Expression of genes with a Pearson correlation coefficient of >0.9 (gray lines). Expression of Lifr is shown in blue. See text for details. (C) Pearson correlation coefficient (r) between engraftment (%) and Lifr gene expression (MRN-normalized read counts) from the 4 cell populations.
3 Results
3.1 Lifr expression was positively correlated with the engraftability of skeletal myogenic cells
We previously reported that in vivo PSC differentiation from both mouse and human PSCs produced skeletal myogenic progenitors that were both engraftable and expandable (Chan et al., 2018; Xie et al., 2021; Pappas et al., 2022; Xie et al., 2023). These in vivo differentiated skeletal myogenic progenitors had excellent muscle regeneration potency upon transplantation and could produce new fibers to a similar extent as adult MuSCs (Chan et al., 2018; Xie et al., 2021). Remarkably, in vivo differentiated skeletal myogenic progenitors could be cultured and expanded in vitro over several passages while still retaining their outstanding engraftability (Xie et al., 2021; Xie et al., 2023). This contrasts with adult MuSCs whose engraftment potential abruptly diminished upon a few days in culture (Montarras et al., 2005; Sacco et al., 2008; Xie et al., 2021). We speculated that factors that regulate engraftment might have their expression level correlating to engraftability. In other words, cell populations with better engraftment would express pro-engraftment factors at higher levels, and vice versa. Therefore, in a previous study, we performed an RNA-seq analysis together with a transplantation assay to evaluate the relationship between gene expression and fiber engraftment on 4 skeletal myogenic populations with various degrees of engraftability: fresh MuSCs (MF), cultured MuSCs (MC), fresh in vivo differentiated skeletal myogenic progenitors (IF), and cultured in vivo differentiated skeletal myogenic progenitors (IC) (Figure 1A) (Xie et al., 2021). We first calculated the Pearson correlation coefficient between gene expression and engraftment and identified genes with a positive correlation of r > 0.9 (Figure 1B). We were particularly interested in factors involved in signaling transduction pathways because their activities would be more readily modulated by commercially available agonists and inhibitors. Using these criteria, we identified Lifr (LIFR): highly expressed in IF and MF (both highly engraftable), moderately expressed in IC (moderately engraftable), and minimally expressed in MC (least engraftable) (Figures 1B,C).
3.2 LIF promoted Pax7 expression in cultured MuSCs
LIFR is a receptor of LIF and the LIF-LIFR pathway has been shown to regulate MuSC biology (Bower et al., 1995; Kami et al., 2000; White et al., 2001; Spangenburg and Booth, 2002). When MuSCs were isolated from the hind limb muscles of adult mice and cultured in vitro for 4 days, the skeletal muscle-specific transcription factor MYOD1 emerged with a concurrent downregulation of the MuSC factor PAX7 (Figures 2A,B). This corresponds to the spontaneous differentiation of MuSCs into a PAX7– MYOD1+ myoblast stage in vitro, where their engraftment potential is severely limited (Olguin and Olwin, 2004; Zammit et al., 2004; Sacco et al., 2008). Interestingly, LIF (1,000 units/mL) upregulated PAX7 while producing minimal effect on MYOD1 (Figures 2A,B). These observations were also supported by gene expression analysis in which MuSCs cultured with LIF exhibited elevated levels of Pax7, while Klf5, Myf5, Myod1 and Myog expression remained unchanged (Figure 2C).
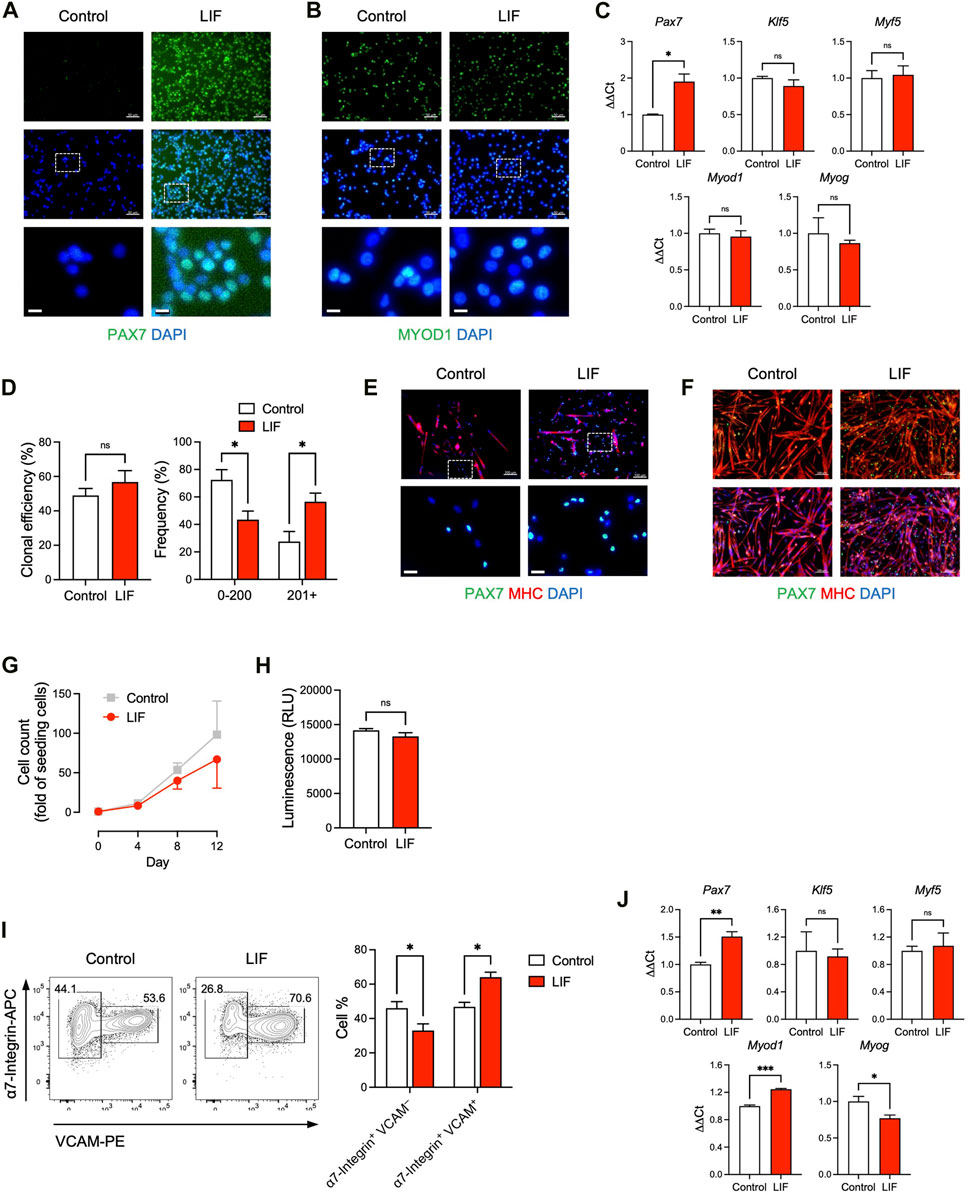
FIGURE 2. LIF promoted the maintenance of cultured MuSCs at a stem cell stage. (A, B) Immunostaining of (A) PAX7 and (B) MYOD1 in MuSCs cultured in vitro with or without LIF for 4 days (scale bar = 50 μm). Magnified images were shown at the bottom (scale bar = 10 μm). (C) qRT-PCR analysis of cultured MuSCs (mean ± SEM, 3 technical replicates, *p < 0.05). (D, E) Clonal analysis of individual MuSCs showing that (D) LIF promoted larger colonies (mean ± SEM, 6 biological replicates, *p < 0.05) and (E) with more PAX7 expression (top: scale bar = 100 μm). Magnified images were shown at the bottom (bottom: scale bar = 20 μm). (F) Upon differentiation, most cultured MuSCs developed into MHC+ multi-nucleated myotubes while some became PAX7+ (“reserve cells”). LIF promoted the “reserve cell” population (scale bar = 100 μm). (G) Growth curves of MuSCs cultured for 12 days over 3 passages with or without LIF. (H) ATP assay of MuSCs cultured with or without LIF (mean ± SEM, 3 biological replicates). (I) FACS analysis of passage 3 MuSCs: (left) typical plots and (right) quantification (mean ± SEM, 3 biological replicates, *p < 0.05). (J) qRT-PCR analysis of passage 3 MuSCs (mean ± SEM, 3 technical replicates, *p < 0.05, ***p < 0.001).
We further evaluated how LIF influenced the colony-forming ability of individual MuSCs. Single α7-Integrin+ VCAM+ MuSCs were FACS-sorted and cultured for 7 days under conditions that supported both cell self-renewal and cell differentiation, i.e., not biasing towards self-renewal or differentiation (Yablonka-Reuveni, 2004; Day et al., 2010; Ippolito et al., 2012; Xie et al., 2021). Although LIF had minimal impact on the capability of single MuSCs to form myogenic colonies per se, LIF-treated MuSCs tended to develop into larger colonies (Figures 2D,E).
Under pro-differentiation conditions, isolated MuSCs gradually developed into MHC+ multi-nucleated myotubes (Figure 2F). Nevertheless, a small population of MuSCs did not differentiate but remained PAX7+, known as “reserve cells” (Olguin and Olwin, 2004; Day et al., 2010). Interestingly, we observed an abundance of PAX7+ “reserve cells” in LIF-treated MuSC undergoing differentiation (Figure 2F). The higher level of PAX7 in MuSCs treated with LIF under both maintenance and differentiation conditions suggested that LIF might promote cultured MuSCs to remain at the stem cell stage, and thereby making them more likely to be engraftable.
The above experiments were performed on MuSCs cultured for a relatively short period of time (e.g., 4 days) and without passaging. We next evaluated the effect of LIF on MuSCs that have been cultured for 12 days over 3 passages. We did not observe a significant difference in cell growth between control and LIF-treated MuSCs, although the latter tended to grow slower (Figure 2G). We also found minimal effects of LIF on MuSC growth in an ATP assay that determined the number of viable cells based on ATP quantification (Figure 2H). Interestingly, LIF promoted the α7-Integrin+ VCAM+ population (markers of MuSCs) in day 12/passage 3 MuSCs at the expense of the α7-Integrin+ VCAM–population (markers of myoblasts) (Figure 2I). Moreover, similarly to the effect on the unpassaged MuSCs, LIF upregulated Pax7 gene expression in passage 3 MuSCs (Figure 2J, top left panel). In addition, LIF-treated passage 3 MuSCs exhibited higher levels of Myod1 with lower levels of Myog than untreated controls (Figure 2J, bottom panels). The levels of Klf5 and Myf5 remained unchanged (Figure 2J, top middle and right panels). Altogether, these findings suggested that LIF promoted the maintenance of cultured MuSCs at a stem cell stage.
3.3 LIF enhanced the engraftability of cultured MuSCs
The above results encouraged us to evaluate whether LIF could improve the engraftment potential of cultured MuSCs in a transplantation assay (Figure 3A). We first obtained MuSCs from the hind limb muscles of wildtype BL6 mice by FACS using established markers: CD31– CD45– (i.e., non-endothelial and non-hematopoietic, respectively) α7-Integrin+ VCAM+. We subsequently cultured isolated α7-Integrin+ VCAM+ cells in myogenic medium with or without LIF (1,000 units/mL) for 12 days over 3 passages. Untreated and LIF-treated passage 3 MuSC cultures were then transplanted at 40,000 cells/muscle into the TA muscles of NSG-mdx4Cv mice, a DMD model we have previously used to evaluate cell transplantation success (Arpke et al., 2013; Chan et al., 2018; Xie et al., 2021; Xie and Chan, 2023; Xie et al., 2023). Four weeks later, the transplanted muscles were harvested for fiber engraftment evaluation, as defined by donor-derived DYSTROPHIN+ fibers (recipient mice lack dystrophin in their muscles). As expected, untreated cultures engrafted poorly (3.5% ± 0.8%, n = 8). Remarkably, LIF treatment significantly increased the ability of passage 3 MuSCs to develop into DYSTROPHIN+ fibers upon transplantation (18.2% ± 3.2%, n = 8, p < 0.001) (Figures 3B,C).
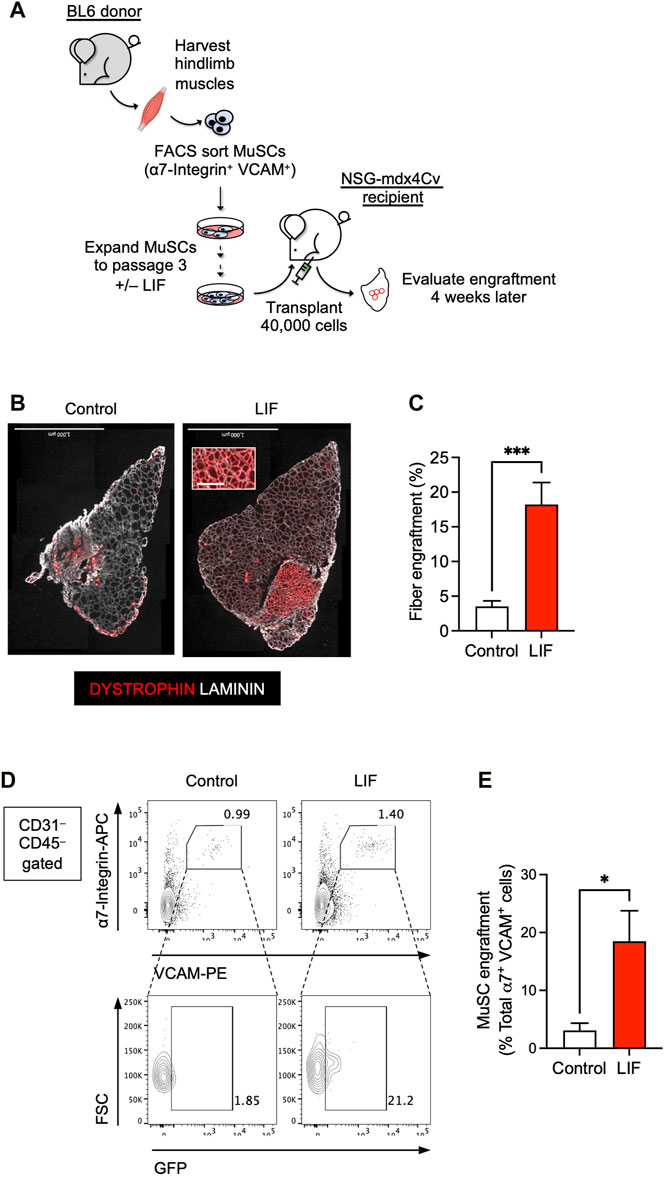
FIGURE 3. LIF enhanced the potential of cultured MuSCs to develop into muscle fibers and repopulate the endogenous MuSC niche upon transplantation. (A) Schematic of the evaluation of the effect of LIF treatment on the engraftability of cultured MuSCs. (B) LIF-treated passage 3 MuSCs engrafted and formed new DYSTROPHIN+ fibers. Total fibers (donor-derived and recipient) are indicated as LAMININ+ (scale bar = 1,000 μm). (C) Quantification of (B) (mean ± SEM, 8 biological replicates, ***p < 0.001). (D) FACS analysis showing LIF-treated passage 3 MuSCs engrafted and repopulated the MuSC compartment. (E) Quantification of (D) (mean ± SEM, 3 biological replicates, *p < 0.05).
We next evaluated whether LIF-treated MuSC cultures could repopulate the endogenous MuSC pool. For this experiment, we used H2B-GFP BL6 mice as donors to obtain α7-Integrin+ VCAM+ MuSCs. These mice constitutively express GFP in their nuclei and thereby allow distinction between donor (GFP+) and recipient (GFP–) cells upon transplantation. FACS-sorted GFP+ MuSCs were subsequently cultured with or without LIF (1,000 units/mL) for 12 days over 3 passages for transplantation. Four weeks after transplantation, we observed a significant contribution of donor-derived cells in the MuSC compartment (GFP+ α7-Integrin+ VCAM+) (Figures 3D,E). Therefore, these results confirmed the role of LIF-LIFR signaling in regulating the engraftment of cultured MuSCs to develop into muscle fibers and repopulate the endogenous MuSC niche.
4 Discussion
A major obstacle in developing cell therapy to treat muscular dystrophies is poor engraftment outcome of donor cells. Whereas endogenous muscle stem cells have tremendous regenerative potency when they are transplanted right away after isolation, their engraftability abruptly diminish once they are expanded in vitro (Montarras et al., 2005; Sacco et al., 2008; Xie et al., 2021). Despite advancements such as p38 modulation and extracellular matrix modification, muscle stem cells are unable to engraft robustly beyond 7 days in culture (Gilbert et al., 2010; Parker et al., 2012; Charville et al., 2015; Quarta et al., 2016). We need a new strategy to discover factors that can improve the engraftment potential of cultured muscle stem cells.
We recently demonstrated that skeletal myogenic progenitors from PSC in vivo differentiation had remarkable regenerative potency, and their engraftment potential was on par with bona fide endogenous muscle stem cells (Chan et al., 2018; Xie et al., 2023). Importantly, these skeletal myogenic progenitors remain engraftable even after prolonged in vitro culture (Xie et al., 2021). We have successfully used this in vivo PSC differentiation method for both mouse and human ESC lines with different genetic backgrounds and mouse and human iPSC lines derived from different tissues. The PSC lines we have successfully used include: mouse ESCs (E14 ESCs with a 129P2/Ola background, PRX-B6N #1 ESCs with a C57BL/6N background), mouse iPSCs (Pax7-ZsGreen iPSCs derived from tail-tip fibroblasts), human ESCs (H1 ESCs, H9 ESCs), and human iPSCs (6B4 iPSCs derived from adult conjunctival cells, PCBC16iPS iPSCs derived from neonatal dermal fibroblasts) (Chan et al., 2018; Xie et al., 2021; Pappas et al., 2022; Xie et al., 2023). The superior engraftability and expandability of in vivo differentiated skeletal myogenic progenitors make them a novel platform for identifying factors that regulate engraftment. In this regard, we have identified LIF-LIFR signaling as a potential engraftment-regulatory pathway. The expression of LIFR was positively correlated to engraftability: highest expression in the highly engraftable cells, modest expression in the moderately engraftable cells, and minimal expression in the less engraftable cells. We subsequently showed that LIF, an endogenous ligand for LIFR, upregulated the muscle stem cell factor PAX7 in cultured muscle stem cells and increased the number of PAX7+ “reserve cells” in differentiated cultures. More importantly, LIF improved the engraftment potential of muscle stem cells that had been cultured and expanded in vitro for 12 days by 5-fold. This is particularly interesting because prior research primarily examined the role of LIF signaling in regulating MuSC functions during highly regenerative conditions such as injured muscles in vivo, i.e., LIF activation further accelerated the already proficient regenerative capacity of endogenous MuSCs (Tham et al., 1997; Kami and Senba, 1998; Austin et al., 2000; Kami et al., 2000; White et al., 2001). In contrast, our current study focused on cultured/expanded MuSCs that have already lost their engraftment potential. Our work thus supplemented previous findings.
Despite numerous efforts, the molecular mechanisms that regulate whether a given transplanted muscle cell population is engraftable remains unclear. In the current study, we have demonstrated the feasibility of our in vivo differentiation platform for identifying novel factors that regulate engraftability. Specifically, we have successfully used our platform to discover the beneficial role of LIF-LIFR signaling in improving the ability of cultured muscle stem cells to form new fibers in transplantation assays. Future investigations might reveal additional candidates for further promoting the engraftability of donor muscle cells.
Data availability statement
The datasets presented in this study can be found in online repositories. The names of the repository/repositories and accession number(s) can be found in the article/Supplementary material.
Ethics statement
The animal study was approved by University of Minnesota Institutional Animal Care and Use Committee. The study was conducted in accordance with the local legislation and institutional requirements.
Author contributions
NX: Formal Analysis, Investigation, Methodology, Visualization, Writing–original draft, Writing–review and editing. KR: Formal Analysis, Investigation, Visualization, Writing–original draft, Writing–review and editing. TS: Investigation, Writing–original draft. Sunny SC: Conceptualization, Data curation, Formal Analysis, Funding acquisition, Investigation, Methodology, Project administration, Resources, Software, Supervision, Validation, Visualization, Writing–original draft, Writing–review and editing.
Funding
The author(s) declare financial support was received for the research, authorship, and/or publication of this article. The study was supported by Regenerative Medicine Minnesota Discovery Science Grant (RMM 102516 001 and RMM 092319 DS 003), Greg Marzolf Jr. Foundation Research Grant, and University of Minnesota startup and Children’s Discovery–Winefest funds.
Acknowledgments
We thank the van Berlo lab for their assistance in immunostaining. The monoclonal antibody to PAX7, embryonic MHC, neonatal MHC, MHC, MHC-I and MHC-IIa were obtained from the Developmental Studies Hybridoma Bank developed under the auspices of the NICHD and maintained by the University of Iowa.
Conflict of interest
The authors declare that the research was conducted in the absence of any commercial or financial relationships that could be construed as a potential conflict of interest.
Publisher’s note
All claims expressed in this article are solely those of the authors and do not necessarily represent those of their affiliated organizations, or those of the publisher, the editors and the reviewers. Any product that may be evaluated in this article, or claim that may be made by its manufacturer, is not guaranteed or endorsed by the publisher.
References
Arpke, R. W., Darabi, R., Mader, T. L., Zhang, Y., Toyama, A., Lonetree, C. L., et al. (2013). A new immuno-dystrophin-deficient model, the NSG-mdx(4Cv) mouse, provides evidence for functional improvement following allogeneic satellite cell transplantation. Stem Cells 31, 1611–1620. doi:10.1002/stem.1402
Austin, L., Bower, J. J., Bennett, T. M., Lynch, G. S., Kapsa, R., White, J. D., et al. (2000). Leukemia inhibitory factor ameliorates muscle fiber degeneration in the mdx mouse. Muscle & nerve 23, 1700–1705. doi:10.1002/1097-4598(200011)23:11<1700::aid-mus5>3.0.co;2-w
Biressi, S., Filareto, A., and Rando, T. A. (2020). Stem cell therapy for muscular dystrophies. J. Clin. Investig. 130, 5652–5664. doi:10.1172/JCI142031
Blau, H. M., and Daley, G. Q. (2019). Stem cells in the treatment of disease. N. Engl. J. Med. 380, 1748–1760. doi:10.1056/NEJMra1716145
Bower, J., Vakakis, N., Nicola, N. A., and Austin, L. (1995). Specific binding of leukemia inhibitory factor to murine myoblasts in culture. J. Cell. Physiol. 164, 93–98. doi:10.1002/jcp.1041640112
Chan, S. S., Arpke, R. W., Filareto, A., Xie, N., Pappas, M. P., Penaloza, J. S., et al. (2018). Skeletal muscle stem cells from PSC-derived teratomas have functional regenerative capacity. Cell Stem Cell 23, 74–85. doi:10.1016/j.stem.2018.06.010
Charville, G. W., Cheung, T. H., Yoo, B., Santos, P. J., Lee, G. K., Shrager, J. B., et al. (2015). Ex vivo expansion and in vivo self-renewal of human muscle stem cells. Stem Cell Rep. 5, 621–632. doi:10.1016/j.stemcr.2015.08.004
Collins, C. A., Olsen, I., Zammit, P. S., Heslop, L., Petrie, A., Partridge, T. A., et al. (2005). Stem cell function, self-renewal, and behavioral heterogeneity of cells from the adult muscle satellite cell niche. Cell 122, 289–301. doi:10.1016/j.cell.2005.05.010
Day, K., Shefer, G., Shearer, A., and Yablonka-Reuveni, Z. (2010). The depletion of skeletal muscle satellite cells with age is concomitant with reduced capacity of single progenitors to produce reserve progeny. Dev. Biol. 340, 330–343. doi:10.1016/j.ydbio.2010.01.006
Gilbert, P. M., Havenstrite, K. L., Magnusson, K. E., Sacco, A., Leonardi, N. A., Kraft, P., et al. (2010). Substrate elasticity regulates skeletal muscle stem cell self-renewal in culture. Science 329, 1078–1081. doi:10.1126/science.1191035
Günther, S., Kim, J., Kostin, S., Lepper, C., Fan, C. M., and Braun, T. (2013). Myf5-positive satellite cells contribute to Pax7-dependent long-term maintenance of adult muscle stem cells. Cell Stem Cell 13, 590–601. doi:10.1016/j.stem.2013.07.016
Ippolito, J., Arpke, R. W., Haider, K. T., Zhang, J., and Kyba, M. (2012). Satellite cell heterogeneity revealed by G-Tool, an open algorithm to quantify myogenesis through colony-forming assays. Skelet. Muscle 2, 13. doi:10.1186/2044-5040-2-13
Kami, K., Morikawa, Y., Sekimoto, M., and Senba, E. (2000). Gene expression of receptors for IL-6, LIF, and CNTF in regenerating skeletal muscles. J. Histochem. Cytochem. 48, 1203–1213. doi:10.1177/002215540004800904
Kami, K., and Senba, E. (1998). Localization of leukemia inhibitory factor and interleukin-6 messenger ribonucleic acids in regenerating rat skeletal muscle. Muscle & nerve 21, 819–822. doi:10.1002/(sici)1097-4598(199806)21:6<819::aid-mus20>3.0.co;2-m
Montarras, D., Morgan, J., Collins, C., Relaix, F., Zaffran, S., Cumano, A., et al. (2005). Direct isolation of satellite cells for skeletal muscle regeneration. Science 309, 2064–2067. doi:10.1126/science.1114758
Olguin, H. C., and Olwin, B. B. (2004). Pax-7 up-regulation inhibits myogenesis and cell cycle progression in satellite cells: a potential mechanism for self-renewal. Dev. Biol. 275, 375–388. doi:10.1016/j.ydbio.2004.08.015
Pappas, M. P., Xie, N., Penaloza, J. S., and Chan, S. S. K. (2022). Defining the skeletal myogenic lineage in human pluripotent stem cell-derived teratomas. Cells 11, 1589. doi:10.3390/cells11091589
Parker, M. H., Loretz, C., Tyler, A. E., Duddy, W. J., Hall, J. K., Olwin, B. B., et al. (2012). Activation of Notch signaling during ex vivo expansion maintains donor muscle cell engraftment. Stem Cells 30, 2212–2220. doi:10.1002/stem.1181
Quarta, M., Brett, J. O., DiMarco, R., De Morree, A., Boutet, S. C., Chacon, R., et al. (2016). An artificial niche preserves the quiescence of muscle stem cells and enhances their therapeutic efficacy. Nat. Biotechnol. 34, 752–759. doi:10.1038/nbt.3576
Roth, S. M., Martel, G. F., Ivey, F. M., Lemmer, J. T., Metter, E. J., Hurley, B. F., et al. (2000). Skeletal muscle satellite cell populations in healthy young and older men and women. Anat. Rec. 260, 351–358. doi:10.1002/1097-0185(200012)260:4<350::AID-AR30>3.0.CO;2-6
Sacco, A., Doyonnas, R., Kraft, P., Vitorovic, S., and Blau, H. M. (2008). Self-renewal and expansion of single transplanted muscle stem cells. Nature 456, 502–506. doi:10.1038/nature07384
Sanz, G., Martínez-Aranda, L. M., Tesch, P. A., Fernandez-Gonzalo, R., and Lundberg, T. R. (2019). Muscle2View, a CellProfiler pipeline for detection of the capillary-to-muscle fiber interface and high-content quantification of fiber type-specific histology. J. Appl. Physiol. 127, 1698–1709. doi:10.1152/japplphysiol.00257.2019
Spangenburg, E. E., and Booth, F. W. (2002). Multiple signaling pathways mediate LIF-induced skeletal muscle satellite cell proliferation. Am. J. Physiol. 283, C204–C211. doi:10.1152/ajpcell.00574.2001
Tham, S., Dowsing, B., Finkelstein, D., Donato, R., Cheema, S. S., Bartlett, P. F., et al. (1997). Leukemia inhibitory factor enhances the regeneration of transected rat sciatic nerve and the function of reinnervated muscle. J. Neurosci. Res. 47, 208–215. doi:10.1002/(sici)1097-4547(19970115)47:2<208::aid-jnr9>3.0.co;2-j
Verhaart, I. E. C., and Aartsma-Rus, A. (2019). Therapeutic developments for Duchenne muscular dystrophy. Nat. Rev. Neurol. 15, 373–386. doi:10.1038/s41582-019-0203-3
von Maltzahn, J., Jones, A. E., Parks, R. J., and Rudnicki, M. A. (2013). Pax7 is critical for the normal function of satellite cells in adult skeletal muscle. Proc. Natl. Acad. Sci. U.S.A. 110, 16474–16479. doi:10.1073/pnas.1307680110
White, J. D., Bower, J. J., Kurek, J. B., and Austin, L. (2001). Leukemia inhibitory factor enhances regeneration in skeletal muscles after myoblast transplantation. Muscle & nerve 24, 695–697. doi:10.1002/mus.1057
Xie, N., and Chan, S. S. K. (2023). Producing engraftable skeletal myogenic progenitors from pluripotent stem cells via teratoma formation. Methods Mol. Biol. 2640, 175–189. doi:10.1007/978-1-0716-3036-5_13
Xie, N., Chu, S. N., Azzag, K., Schultz, C. B., Peifer, L. N., Kyba, M., et al. (2021). In vitro expanded skeletal myogenic progenitors from pluripotent stem cell-derived teratomas have high engraftment capacity. Stem Cell Rep. 16, 2900–2912. doi:10.1016/j.stemcr.2021.10.014
Xie, N., Chu, S. N., Schultz, C. B., and Chan, S. S. K. (2023). Efficient muscle regeneration by human PSC-derived CD82+ ERBB3+ NGFR+ skeletal myogenic progenitors. Cells 12, 362. doi:10.3390/cells12030362
Yablonka-Reuveni, Z. (2004). Isolation and characterization of myogenic stem cells from adult skeletal muscle. Sci. Rep. 9, 571–580. doi:10.1038/s41598-019-40042-6
Keywords: pluripotent stem cells, myogenic differentiation, muscle stem cells, transplantation, cell therapy, muscular dystrophy
Citation: Xie N, Robinson K, Sundquist T and Chan SSK (2024) In vivo PSC differentiation as a platform to identify factors for improving the engraftability of cultured muscle stem cells. Front. Cell Dev. Biol. 12:1362671. doi: 10.3389/fcell.2024.1362671
Received: 28 December 2023; Accepted: 06 February 2024;
Published: 15 February 2024.
Edited by:
Hidetoshi Sakurai, Kyoto University, JapanReviewed by:
Md Shaifur Rahman, Atomic Energy Research Establishment, BangladeshYuyao Tian, Harvard Medical School, United States
Copyright © 2024 Xie, Robinson, Sundquist and Chan. This is an open-access article distributed under the terms of the Creative Commons Attribution License (CC BY). The use, distribution or reproduction in other forums is permitted, provided the original author(s) and the copyright owner(s) are credited and that the original publication in this journal is cited, in accordance with accepted academic practice. No use, distribution or reproduction is permitted which does not comply with these terms.
*Correspondence: Sunny S. K. Chan, c3NjaGFuQHVtbi5lZHU=