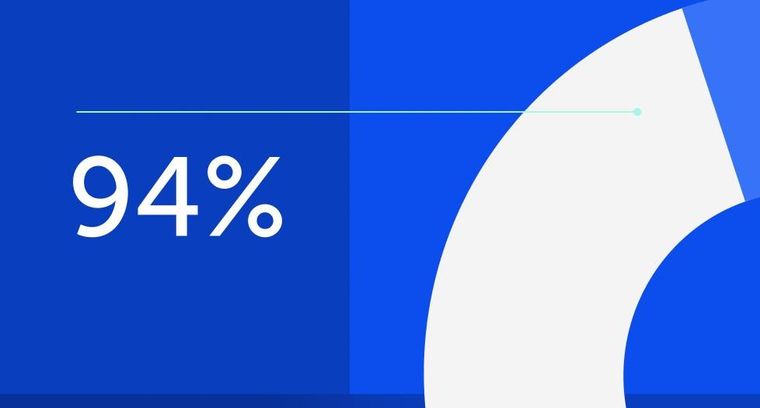
94% of researchers rate our articles as excellent or good
Learn more about the work of our research integrity team to safeguard the quality of each article we publish.
Find out more
ORIGINAL RESEARCH article
Front. Cell Dev. Biol., 11 March 2024
Sec. Molecular and Cellular Reproduction
Volume 12 - 2024 | https://doi.org/10.3389/fcell.2024.1362228
This article is part of the Research TopicProceedings of the 9th International Symposium on the Biology of Vertebrate Sex Determination 2023View all 10 articles
The genetics of sex determination varies across taxa, sometimes even within a species. Major domesticated strains of zebrafish (Danio rerio), including AB and TU, lack a strong genetic sex determining locus, but strains more recently derived from nature, like Nadia (NA), possess a ZZ male/ZW female chromosomal sex-determination system. AB fish pass through a juvenile ovary stage, forming oocytes that survive in fish that become females but die in fish that become males. To understand mechanisms of gonad development in NA zebrafish, we studied histology and single cell transcriptomics in developing ZZ and ZW fish. ZW fish developed oocytes by 22 days post-fertilization (dpf) but ZZ fish directly formed testes, avoiding a juvenile ovary phase. Gonads of some ZW and WW fish, however, developed oocytes that died as the gonad became a testis, mimicking AB fish, suggesting that the gynogenetically derived AB strain is chromosomally WW. Single-cell RNA-seq of 19dpf gonads showed similar cell types in ZZ and ZW fish, including germ cells, precursors of gonadal support cells, steroidogenic cells, interstitial/stromal cells, and immune cells, consistent with a bipotential juvenile gonad. In contrast, scRNA-seq of 30dpf gonads revealed that cells in ZZ gonads had transcriptomes characteristic of testicular Sertoli, Leydig, and germ cells while ZW gonads had granulosa cells, theca cells, and developing oocytes. Hematopoietic and vascular cells were similar in both sex genotypes. These results show that juvenile NA zebrafish initially develop a bipotential gonad; that a factor on the NA W chromosome, or fewer than two Z chromosomes, is essential to initiate oocyte development; and without the W factor, or with two Z doses, NA gonads develop directly into testes without passing through the juvenile ovary stage. Sex determination in AB and TU strains mimics NA ZW and WW zebrafish, suggesting loss of the Z chromosome during domestication. Genetic analysis of the NA strain will facilitate our understanding of the evolution of sex determination mechanisms.
Vertebrates exhibit a wide variety of sex determination mechanisms, including genetic and environmental control factors (Nagahama et al., 2021). Among fishes with genetic sex determination, some species have a single major genetic sex determinant, but others use polygenic sex determination. Unlike mammals and birds, which have evolutionarily rather stable genetic sex determinants (Ioannidis et al., 2021; Terao et al., 2022), different fish lineages can have different major sex determination genes, sometimes even in the same species or genus (Matsuda and Sakaizumi, 2016; Pan et al., 2021; Song et al., 2021). Despite the popularity of zebrafish as a model organism (Bradford et al., 2017) and substantial knowledge about its gonadogenesis (Liew and Orban, 2014; Kossack and Draper, 2019; Aharon and Marlow, 2021), our understanding of the genetic regulation of zebrafish sex determination is insufficient.
Zebrafish gonads have been described as passing through a juvenile ovary stage, with all juveniles developing perinucleolar oocytes by about 20 days post-fertilization (dpf) (Takahashi, 1977; Uchida et al., 2002). In some individuals, these oocytes persist and the fish develops as a female, but in other individuals, oocytes die, the gonad transitions to a testis, and the fish becomes a male (Wang et al., 2007). Previous studies in zebrafish strains domesticated for mutagenesis research (Walker-Durchanek, 1980; Streisinger et al., 1981; Mullins et al., 1994) showed that germ cells, specifically oocytes, are essential for both the establishment and maintenance of female sex because zebrafish that lack germ cells become males (Slanchev et al., 2005), as do mutants that lose oocytes during meiosis (Rodriguez-Mari et al., 2010; Shive et al., 2010; Rodriguez-Mari et al., 2011; Saito et al., 2011; Beer and Draper, 2013; Dranow et al., 2013; Dranow et al., 2016; Ramanagoudr-Bhojappa et al., 2018; Takemoto et al., 2020; Blokhina et al., 2021; Islam et al., 2021). While zebrafish are gonochoristic, mutations that cause progressive oocyte loss in adults but retain some germ cells result in individuals that can first become females and later convert to a male phenotype (Dranow et al., 2013).
Mechanisms that cause some juvenile zebrafish to maintain oocytes and become females are not yet fully known, but oocyte survival and sex ratio are strongly influenced by both genetic and environmental factors. In general, stressful environments masculinize a clutch: an increased fraction of males occurs after hypoxia, high rearing density, high temperature, low nutrition, gamma rays, inbreeding, and exposure to exogenous cortisol (Walker and Streisinger, 1983; Shang et al., 2006; Lawrence et al., 2008; Abozaid et al., 2011; Ribas et al., 2017; Santos et al., 2017; Delomas and Dabrowski, 2018; Valdivieso et al., 2022). Sex phenotype has been mapped to different genetic locations in different zebrafish strains, and sex ratios are consistent in repeated matings of the same individual fish pairs, consistent with polygenic sex determination in laboratory lines (Bradley et al., 2011; Anderson et al., 2012; Howe et al., 2013; Liew and Orban, 2014; Luzio et al., 2015).
Unlike laboratory strains, zebrafish lines not long adapted to laboratory life at the time of investigation, including the Nadia (NA) strain, have a genetic ZZ/ZW sex determination system, with the major Sex-Associated Region on chromosome-4 (sar4) located near the telomere of the right arm of chromosome-4 (Chr4) (as displayed in Ensembl: (ensembl.org/Danio_rerio/Location/Chromosome?r=4) (Tong et al., 2010; Anderson et al., 2012; Wilson et al., 2014), a chromosome that has, in addition to the sex locus, a region that has sex-specific silencing of protein-coding gene transcription in ovaries (Wilson and Postlethwait, 2023). Individual NA strain zebrafish homozygous for Z alleles always develop as males and most ZW individuals become females, but some ZW individuals develop as males in all four strains investigated (Anderson et al., 2012; Wilson et al., 2014). In addition, WW fish in these less domesticated strains tend to become females, although some develop as males. The finding that ZZ fish do not become females suggests that the W allele is necessary but not sufficient for female development, although the hypothesis that Z dosage regulates sex (two Z doses, always male; one or zero Z chromosomes, usually female) is not formally ruled out. Because ZW fish occasionally develop as males, either masculinizing environmental factors or segregating background genetic modifiers can affect zebrafish sex determination. These results raise the question: Do ZZ zebrafish pass through the juvenile ovary stage like all fish in domesticated laboratory strains, or alternatively, do they develop directly into males? Under the hypothesis that the W carries a factor that is necessary, but insufficient, for ovarian development, ZZ fish, lacking the hypothesized W-linked ovary factor, should not form juvenile ovaries with meiotic oocytes.
To test this hypothesis, we investigated gonad development in chromosomally ZW and ZZ zebrafish from the NA strain. Results showed that 1) juvenile NA zebrafish initially develop a bipotential gonad; 2) a factor on the W chromosome, or fewer than two Z chromosomes, is essential to trigger oocyte development; and 3) ZZ zebrafish gonads develop directly into testes. These results are as expected from the hypothesis that the W chromosome contains a factor that is necessary but insufficient for female development or that the Z chromosome contains a dosage-sensitive factor that inhibits female development, with fully penetrant inhibition of ovary development in ZZ fish. Under either hypothesis, standard laboratory strains produce both males and females, as do both ZW and WW NA zebrafish, suggesting loss of the Z chromosome leading to WW populations during domestication.
To confirm the relationship of sex development and sex chromosomes, we crossed NA strain individuals genotyped for sex chromosomes. We evaluated 366 fish that were the offspring of eight different crosses of ZW females x ZZ males (five single pair crosses and three crosses with two males and two females). At sexual maturity, primers specific for Z- and W-chromosomes identified genotypic sex (Wilson et al., 2014), while secondary sexual characteristics distinguished phenotypic sex (for females, a rounded abdomen, and pale ventral and anal fins, and for males, a streamlined body with yellow ventral and anal fins and sex tubercles) (McMillan et al., 2013; Dai et al., 2021b). Offspring included 184 ZZ individuals (50.3%) and 182 ZW fish (49.7%), a 1:1 genotypic sex ratio (p = 0.92, Chi-square test). This result shows first, that in meiosis, Z and W chromosomes segregate as homologous chromosomes, and second, that ZZ and ZW genotypes are equally likely to survive. All 184 ZZ individuals were phenotypic males. Of 182 ZW fish, 128 individuals (70.3%) were phenotypic females and 54 fish (29.7%) were phenotypic males, called neomales (Ginsburger-Vogel, 1972). Families varied widely in the percent of neomales, from 6.7% to 66.7% (Figure 1A). This result would be explained either if sex determination in ZW NA strain zebrafish can be influenced by genetic factors in addition to sar4 or if differences in environmental factors increase the likelihood of male development in NA as in laboratory strains (Liew and Orban, 2014; Valdivieso et al., 2022).
FIGURE 1. Sex ratios and gonad histology in adult ZW and ZZ zebrafish at 5 months post fertilization (mpf). (A) Sex phenotypes in progeny of ZW females crossed to ZZ males. Within each sex genotype, each point represents a different cross. No ZZ individuals became adult females. (B) Sex phenotypes in progeny of ZW females crossed to ZW neomales. No ZZ individuals became adult females. C-G. Cross sections of 5mpf adult gonads. (C) ZZ adult testis. (D) ZW ovary. (E) ZW neomale testis. (F) WW ovary. (G) WW neomale testis. Ovary histology for ZW and WW fish showed no clear difference and testis histology of ZZ, ZW, and WW fish was the same. Abbreviations: o, oocyte; pc, pycnotic cell; sc, spermatocyte; sg, spermatogonium; sp, spermatid; sz, spermatozoa.
To study sex determination in chromosomally WW fish, we made three single pair matings between ZW phenotypic females and ZW neomales, resulting in a total of 158 progeny (Figure 1B). Offspring included 43 (27.2%) ZZ individuals, 74 (46.9%) ZW fish, and 41 (25.9%) WW fish, approximating a 1:2:1 genotypic ratio (p = 0.71, Chi square). All ZZ individuals were phenotypic males (43/43, 100%), while 30 of the 74 (40.5%) ZW fish and 10 of the 41 (25.9%) WW fish developed as neomales. The frequency of sex reversal was again highly variable among crosses, ranging between 5.9% and 74.2% (average 34.4%) for ZW and between 0% and 64.3% (average 23.7%) for WW (Figure 1B). These results show that 1) homozygous W fish have normal viability, and 2) no Z-specific genes are essential to allow a fish to develop testes.
Having shown that some ZW and WW fish can become neomales, we wondered if adult neomale gonads differ from those in ZZ males. Histological sections showed that at 5mpf (months post fertilization), all adult NA ZZ fish examined (n = 4) possessed testes with germ cells organized into cysts containing mitotic spermatogonia, meiotic spermatocytes, and post-meiotic spermatids, with mature spermatozoa present in the testis lumen (Figure 1C). Abdominal sections of adult 5mpf NA ZW fish (n = 4) with female secondary sexual characteristics possessed ovaries with oocytes in various stages of development (Selman et al., 1993), including meiotic perinucleolar oocytes (Stage I), larger oocytes containing cortical alveoli (Stage II), vitellogenic oocytes accumulating yolk (Stage III), and maturing oocytes in the final stages of oogenesis (Stage IV) (Figure 1D). In contrast, in 5mpf genetic ZW neomales (n = 4), testes had morphologies not detectably different from those of ZZ males; notably, 5mpf ZW adult neomales lacked any detectable oocytes (Figure 1E). Gonads in the phenotypically female WW fish (n = 4) were also not detectably different from ovaries in ZW fish (Figure 1F), and gonads in phenotypically neomale WW fish (n = 4) were no different from testes in ZZ fish (Figure 1G). We conclude that NA strain neomales, whether ZW or WW, produce histologically normal testes with all stages of spermatogenesis like ZZ males, and by 5mpf, had no detectable oocytes.
All chromosomally ZZ NA fish had normal testes as adults, but because even phenotypically male adult laboratory zebrafish pass through a juvenile ovary stage (Takahashi, 1977; Uchida et al., 2002; Wang et al., 2007), we wondered whether ZZ zebrafish do too. The hypothesis that a factor on the W chromosome or that fewer than two Z chromosomes is necessary for ovary development predicts that ZZ fish will not pass through the juvenile ovary stage. To test this prediction, we analyzed differences in gonad development in ZZ and ZW NA zebrafish over developmental time in histological sections from two separate NA families at 10, 14, 19, 22, 26, and 30dpf.
Results showed that gonads in ZZ and ZW zebrafish were morphologically indistinguishable in cross-sections of 10, 14, and 19dpf larvae. In five ZW and four ZZ fish examined at 10dpf, bilateral gonads had clusters of germ cells surrounded by somatic cells (Figures 2A, B), as for laboratory strain zebrafish (Takahashi, 1977; Uchida et al., 2002; Rodriguez-Mari et al., 2005; Wang et al., 2007; Dranow et al., 2013; Dranow et al., 2016). Gonads in both ZW and ZZ fish increased in size and in germ cell number at 14dpf and 19dpf, but gonads in ZW and ZZ fish remained morphologically indistinguishable (Figures 2C–F), again as in laboratory strains. We conclude that the morphology of ZW and ZZ gonads appeared to be bipotential up to 19dpf.
FIGURE 2. Developmental trajectory of gonads from ZW and ZZ fish. (A,C,E,G,I,K,M,N) ZW gonads. (B,D,F,H,J,L): ZZ gonads. (A,B) 10dpf. (C,D) 14dpf. (E,F) 19dpf. (G,H) 22dpf. (I,J) 26dpf. (K,L)30dpf. At 19dpf and earlier, ZW and ZZ gonads were histologically similar, but at 22dpf and later, oocytes were developing in ZW, but not ZZ gonads. At 26dpf, some ZZ gonads showed clusters of spermatogonia, and at 30dpf, most ZZ gonads were still immature testes that were organized into cysts containing spermatogonia and spermatocytes. No ZZ gonad studied showed any oocytes at any developmental stage, but instead, showed direct development into testes. (M) At 26dpf, some ZW gonads were mostly ovary with a few pycnotic cells. (N) At 30dpf, some ZW gonads were mostly testes with a few pycnotic cells. Abbreviations: *, gonocytes; g, gonad; I and II, Stage I and Stage II oocytes; in, intestine; li, liver; o, oocyte; pc, pycnotic cell; sc, spermatocyte; sg, spermatogonium.
Between 19 and 22dpf, gonad development in ZW and ZZ sex genotypes began to differ. At 22dpf, gonads in nine of ten ZW individuals examined contained perinucleolar oocytes (Figure 2G), while gonads in the tenth fish examined retained the indifferent morphology of younger fish. In contrast, all ten ZZ individuals at 22dpf still possessed indifferent gonads, failing to advance detectably in developmental stage (Figure 2H). By 26dpf, gonads of all 11 ZW fish studied had perinucleolar oocytes (Figure 2I), but four of these 11 had gonads that also contained pyknotic cells representing dying oocytes (Figure 2M). Similar pyknotic cells appear in juvenile gonads transforming to adult testis in laboratory strain zebrafish and in gonads of mutants experiencing female-to-male sex reversal (Takahashi, 1977; Uchida et al., 2002; Maack and Segner, 2003; Rodriguez-Mari et al., 2010; Rodriguez-Mari et al., 2011; Dranow et al., 2016; Blokhina et al., 2021; Xie et al., 2021). Of nine 26dpf ZZ fish, three contained largely undifferentiated gonads, and the other six had clusters of spermatogonia (Figure 2J), including one with spermatocytes. At 30dpf, 10 of 12 ZW fish examined had a well-developed ovary full of perinucleolar oocytes (Figure 2K). At 30dpf, oocytes had increased in size, but had not yet entered the cortical alveolar stage [Stage II, (Selman et al., 1993)]. Two of these 12 ZW 30dpf fish, however, had gonads that were nearly all testis, including spermatogonia and spermatocytes, but also pyknotic oocyte-like cells and a few remaining oocytes (Figure 2N). We interpret these two fish as ZW individuals that had gone through the juvenile ovary stage, but by 30dpf, were transitioning to neomales.
The developmental trajectory of ZZ males differed from that of ZW neomales. At 30 dpf, gonads in one of 11 ZZ males investigated were still largely undifferentiated; three fish contained gonads with spermatogonia; gonads in four fish were still small but had both spermatogonia and spermatocytes; gonads in two individuals were larger with both spermatogonia and spermatocytes; and gonads in one ZZ male contained spermatids. All three of the ZZ fish with the most mature testes came from Family 1, which was fed paramecia instead of rotifers during development. Perinucleolar oocytes were never observed in any ZZ individual analyzed at any stage. We conclude that gonads in ZZ zebrafish of the NA strain: 1) are slower in developing sex-specific morphologies than ZW fish; 2) do not form immature oocytes; 3) do not pass through a juvenile hermaphrodite stage; and 4) develop directly into testes. This developmental trajectory differs from that of domesticated zebrafish, which all appear to pass through a juvenile hermaphrodite stage. Further, these findings are consistent with the hypothesis that a factor on the W chromosome, or alternatively, fewer than two Z chromosomes, is necessary for oocytes to develop in NA zebrafish gonads.
The histology investigations (Figures 1, 2) showed that at 19dpf, the morphology of ZW and ZZ gonads could not be distinguished, but at 30dpf, gonads in both ZW and ZZ fish showed signs of differentiation. To learn whether gonad transcriptomes of ZW and ZZ fish had become different at 19dpf even though gonad morphologies had not, we performed scRNA-seq on 19dpf and 30dpf gonads dissected from ZW and ZZ animals. From resulting data, we removed red blood cells and contaminating non-gonad cells, and re-clustered remaining cells. We analyzed each time point separately and then merged all four datasets (two ages by two sex genotypes). Analysis of 19dpf gonads resulted in 783 ZW cells and 1270 ZZ cells grouped into 28 clusters (Figures 3A, B, Supplementary Table S1).
FIGURE 3. Cell-type marker genes for 19dpf ZW and ZZ gonads. (A) UMAP plot for 19dpf gonads combining ZW and ZZ cells. (B) Cells originating from ZW (red) or ZZ (blue) gonads, showing all clusters with mixtures of both sex genotypes. (C,D) Germ cells marked by dazl and ddx4 (vasa) were in cluster 19c17. (E,F) Support cells (granulosa and Sertoli) or their precursors marked by gsdf, and lhx9. (G,H) Steriogenic cells (theca, Leydig) or their precursors labeled with inhbaa and robo2 expression. (I,J) Stroma/interstitial cells marked by col1a1a and pcolcea. (K) White blood cells identified by coro1a expression. (L) Vasculature labeled with si:dkeyp-97a10.2. Abbreviations: g, germ cells; si, stroma/interstitial; sd, steroidogenic; su, support; v, vascular; w, white blood cells.
Cell-type marker genes help characterize cluster identities (Qiu et al., 2021) (Supplementary Table S4). Germ cells are marked by a number of genes encoding RNA-binding proteins, including dazl, adad1, ddx4, and dnd1 as well as novel markers including si:ch73-167f10.1 (Fu et al., 2015; Bertho et al., 2021). Expression of these genes identified the 19dpf cluster 17 (19c17) as germ cells (Figures 3C, D, Supplementary Table S4). Support cells, including granulosa cells in ovaries and Sertoli cells in testes, express the fish-specific TGFb-family protein Gsdf (Sawatari et al., 2007; Gautier et al., 2011; Rondeau et al., 2013; Yan et al., 2017; Hsu and Chung, 2021), identifying three clusters (19c10, c15, and c16, Figure 3E). Cluster 19c23 strongly expressed the support cell precursor gene lhx9 (Figure 3F) (Mazaud et al., 2002; Liu et al., 2022). Steroidogenic cells, including theca cells in ovaries and Leydig cells in testes, arise from Nr5a1-expressing precursor cells (Stevant et al., 2019; Yan et al., 2020) and express inhbaa and robo2 Archambeault and Yao (2010), McClelland et al. (2015), Namwanje and Brown (2016), Lu et al. (2020), Zhao et al. (2022); these genes are expressed in 19c1, c15, c16, and c23 (Figures 3G, H, Supplementary Tables S2, S4). Interstitial and stromal cells express the collagen gene col1a1a and the procollagen cleavage enzyme pcolcea (Liu et al., 2022), identifying 19c0, c1, c6, c11, c14, and c18) as stromal/interstitial cells (Figures 3I, J). Leukocytes express coro1a (Song et al., 2004; Xavier et al., 2008), identifying clusters 19c2, c4, c5, c7, c13, c19, c20, c21, c22, c26, c27 (Figure 3K). Vasculature appears early in gonad development in XY mice (Brennan et al., 2002) and in zebrafish (Kossack et al., 2023)and it expresses si:dkeyp-97a10.2, kdr, and lyve1b, identifying 19c12 as blood vessels (Figure 3L; Supplementary Figure S4B; Supplementary Tables S2, S4) (Jackson et al., 2001; Prevo et al., 2001; Covassin et al., 2006b; Bahary et al., 2007; Gomez et al., 2009; Chen et al., 2013).
All 19dpf clusters contained both ZW and ZZ cells (Figure 3B), agreeing with the histology findings and suggesting that ZW and ZZ gonads were morphologically and transcriptionally similar at 19dpf (Figures 2E, F). Differential expression analysis of 19dpf clusters showed no significantly differentially expressed genes between ZZ and ZW genotypes in nearly all clusters; the most DE genes, mostly mitochondrial genes, appeared in 19c1, suggesting a slight difference in stress levels (Ilicic et al., 2016) in that cluster for ZW and ZZ samples (Supplementary Table S3), and confirming strong similarity of ZZ and ZW cell types at 19dpf.
The histology studies had shown that ZW and ZZ gonads were morphologically different by 30dpf (Figure 2), so we wanted to identify gene expression patterns in 30dpf individuals with different chromosomal sexes by single cell transcriptomics. The 30dpf gonad sample gave 7787 ZW cells and 2843 ZZ cells in 48 clusters (Figure 4A; Supplementary Tables S5, S6). In contrast to 19dpf gonads, 30dpf gonads had several clusters specific for either ZW or ZZ cells (Figure 4B). Germ cells were clearly labeled by dazl and ddx4 in a large group of ZW-specific clusters (30c0-c4, c8, c13, c15, c27, c28, c43) and in c21 (Figures 4C, D), showing substantial proliferation of germ cells in ZW but much less in ZZ gonads. Support cells labeled by gsdf and lhx9 identified mainly 30c24, c40, and c46 (Figures 4E, F). Steroidogenic cells marked by inhbaa and nr5a1b identified 30c11 for both markers, and 30c9, c17, and c22 specific for inhbaa (Figures 4G, H). Interstitial/stroma cells expressed col1a1a in a variety of clusters (30c7, c9, c17, c20, c22, c26, c35, c45) and pcolcea in most of these (Figures 4I, J). Leukocytes marked by coro1a included 14 clusters (Figure 4K). Vascular cell types denoted by si:dkeyp-97a10.2 expression (Gomez et al., 2009) occupied 30c32. We conclude that by 30dpf, ZW and ZZ gonads had diverged considerably in terms of the cell types they contained, consistent with the histology results (Figure 2).
FIGURE 4. Cell-type marker genes for 30dpf ZW and ZZ gonads. (A) UMAP plot for 30dpf gonads combining ZW and ZZ cells. (B) Cells originating from ZW (gold) or ZZ (blue) gonads, showing several clusters with sex-specific genotypes. (C,D). Germ cells marked by dazl and ddx4 (vasa) expression. (E,F). Support cells (granulosa and Sertoli) or their precursors marked by gsdf and lhx9 expression. (G,H). Steriogenic cells (theca, Leydig) or their precursors labeled with inhbaa and nr5a1b expression. (I,J). Stroma/interstitial cells marked by col1a1a and pcolcea expresseion. (K) White blood cells identified by coro1a expression. (L) Vasculature labeled with si:dkeyp-97a10.2 expression. Abbreviations: g, germ cells; oo, oocytes; si, stroma/interstitial; sd, steroidogenic; su, support; v, vascular; w, white blood cells.
Combining all four samples (two ages, each with two sex genotypes) gave 40 clusters (Figure 5A; Supplementary Table S7) with defined marker genes (Supplementary Table S8). Some clusters contained significant proportions of cells from both 19dpf and 30dpf, but many sorted out by age (Figure 5B), suggesting cell type maturation. And, as expected from the 30dpf-only analysis (Figure 5C), some clusters in the combined analysis were mostly ZW cells and others mainly ZZ cells, while many were still mixed. The analysis below provides a more detailed understanding of major cell types (Figure 5D).
FIGURE 5. The combined dataset with two ages, each with two sex genotypes. (A) UMAP displaying clusters. (B) Samples displayed by age (19dpf, red; 30dpf, blue). (C) Samples displayed by sex genotype (ZW, red; ZZ, blue). (D) Inferred cell types.
Germ cell marker genes, including dazl, ddx4, and dnd1 (Olsen et al., 1997; Yoon et al., 1997; Maegawa et al., 1999; Howley and Ho, 2000; Ciruna et al., 2002; Tan et al., 2002; Weidinger et al., 2003; Krovel and Olsen, 2004; Slanchev et al., 2005; Houwing et al., 2008; Saito et al., 2011; Hartung et al., 2014; Hong et al., 2016; Bertho et al., 2021) were detected in the merged 19dpf+30dpf (”1930”) data as expressed in several clusters (1930c0, c1, c3, c5, c15, c16, c17, c24) (Figures 6D–J). Cluster 1930c16 contained 19dpf cells mixed with 30dpf cells, but the large group of dnd1-expressing clusters contained almost exclusively 30dpf ZW cells (Figure 4B). We conclude that the large, several-cluster group of 30dpf ZW cells are developing oocytes and cluster 1930c16 cells are less mature germ cells that are generally similar in ZW and ZZ gonads.
FIGURE 6. Germ cell development in the merged analysis of both ZW and ZZ cells at both 19 and 30dpf. (A) UMAP isolating germ cell clusters. (B) Germ cells marked by age (red, 19dpf; blue, 30dpf). (C) Germ cells marked by sex genotype (red, ZW; blue, ZZ). (D) ddx4(vasa) including all four conditions. (E) ddx4 showing only ZW cells at 19dpf. Insert in lower left of the panel shows ddx4 expression in the ZW germ cell cluster 19c17 in the analysis of 19dpf cells only (see Figure 3). (F) ddx4, only ZW cells at 30dpf. (G) ddx4, only ZZ cells at 19dpf. Insert in lower left of the panel shows ddx4 expression in the ZZ germ cell cluster 19c17 from the analysis of 19dpf cells only. (H) ddx4, only ZZ cells at 30dpf. (I) dnd1 ZW cells only at both 19dpf and 30dpf. (J) dnd1 ZZ cells only at both 19dpf and 30dpf. (K) fkbp6, both ZW and ZZ cells only at 19dpf. (L) fkbp6, both ZW and ZZ cells only at 30dpf. (M) piwil2, ZW and ZZ cells at 19dpf. (N) piwil2, ZW and ZZ cells at 30dpf. (O) bmp15, ZW cells at both 19dpf and 30dpf. (P) bmp15, ZZ cells at both 19dpf and 30dpf. (Q) buc, ZW cells at both 19dpf and 30dpf. (R). buc, ZZ cells at both 19dpf and 30dpf. (S) zp2.2, ZW cells at both 19dpf and 30dpf. (T) zp2.2, ZZ cells at both 19dpf and 30dpf. (U) dmrt1, ZW cells at both 19dpf and 30dpf. (V) dmrt1, ZZ cells at both 19dpf and 30dpf. (W) spo11, ZW cells at both 19dpf and 30dpf. (X) spo11, ZZ cells at both 19dpf and 30dpf. (Y) hormad1, ZW cells at both 19dpf and 30dpf. (Z) hormad1, ZZ cells at both 19dpf and 30dpf. (AA) rad21l1, ZW cells at both 19dpf and 30dpf. (BB) rad21l1, ZZ cells at both 19dpf and 30dpf. (CC) smc1b, ZW cells at both 19dpf and 30dpf. (DD) smc1b, ZZ cells at both 19dpf and 30dpf. (EE) sycp3, ZW cells at both 19dpf and 30dpf. (FF) sycp3 ZZ, cells at both 19dpf and 30dpf.
The Vasa-encoding gene ddx4 was strongly expressed in young germ cells of 1930c16 and less strongly in oocytes (Figure 6D), reflecting the observation that ddx4 expression is higher in early stages of gametogenesis (Wang et al., 2007). Figures 6E–H show each of the four conditions separately in the combined 19 + 30dpf analysis. Results for germ cells (19c17 in the 19dpf-only analysis, Figures 3C, D) are shown in the box at the lower left of the 19dpf panels in Figure 6. The distribution of ddx4-expressing ZZ cells at 19dpf and 30dpf (Figures 6G, H) suggest that the transcriptomes of ZZ germ cells changed little between 19dpf and 30dpf, reflecting the delayed differentiation observed in ZZ gonads in our histological studies. This result also shows that ZZ germ cells did not develop an oocyte-like transcriptome, again consistent with the histology. Expression of dnd1 supports these conclusions (Figures 6I, J).
Oocyte stages recovered are limited by the scRNA-seq protocol, which passes cells through a 40 µm filter. According to recent staging criteria (Bogoch et al., 2022), we would recover only oocytes from the earliest two stages: the symmetry-breaking stage (8–20 μm Selman Stage IA, oogonia and meiotic oocytes from leptotene to pachytene), and early Selman Stage IB, nuclear cleft, 15–50μm, oocytes from pachytene to mid-diplotene (Selman et al., 1993; Bogoch et al., 2022). Comparison of gene expression patterns with other transcriptomic analyses of zebrafish oocytes (Wong et al., 2018; Zhu et al., 2018; Can et al., 2020; Bogoch et al., 2022) are difficult because at least some of the oocyte sizes we examined were not analyzed or were mixed with larger oocytes, and in other studies, oocyte sizes were listed in relative rather than absolute terms, so comparison was not possible (Cabrera-Quio et al., 2021).
Cluster 1930c16 appears to consist of bipotential germ cells. Few genes were expressed at a higher level in 1930c16 at 19dpf than in 30dpf germ cells, but fkbp6 was an exception (Figures 6K, L, samples separated by age, not by sex genotype). Men homozygous for pathogenic variants of FKBP6 show arrested spermatogenesis at the round-spermatid stage and have abnormal piRNA biogenesis like mouse Fkbp6 mutants (Xiol et al., 2012; Wyrwoll et al., 2022). piRNAs interact with Piwi proteins, including Piwil2 in zebrafish, which aids in transposon defense (Houwing et al., 2008). Like fkbp6, piwil2 transcripts accumulated in 1930c16 germ cells at 19dpf and 30dpf (Figures 6M, N). The expression of fkbp6 and piwil2 in 19dpf germ cells shows that these cells represent an early step in the pathway of gametogenesis.
Oocyte-specific genes in NA gonads tended to label strongly the dnd1-expressing germ-cell clusters except for 1930c16. Accumulation of bmp15 and gdf9 transcripts was detected only in 30dpf ZW germ cells, but not in 19dpf ZW germ cells or ZZ germ cells (Figure 6O, P, Supplementary Figure S1A, S1B). The loss of either Bmp15 or Gdf9 function blocks ovarian follicle development in zebrafish and mouse (Dong et al., 1996; Su et al., 2004; Dranow et al., 2016; Chen et al., 2022; Zhai et al., 2022). The 30dpf ZW cells also accumulated transcripts encoding the Balbiani body protein Bucky ball (buc), an early marker of oocyte asymmetry (Marlow and Mullins, 2008; Jamieson-Lucy et al., 2022), but few ZZ cells did, and only at a low level (Figures 6Q, R). The low or undetected expression of bmp15 and gdf9 expression in 1930c16 suggests that these cells were at an earlier stage of differentiation than the large group of germ cell clusters (1930c0, c1, c3, c5, c15, c17, c24). Cells in the large group of 30dpf ZW germ cell clusters also expressed genes encoding zona pellucida egg coat proteins like Zp2.2 (Figures 6S, T). At 19dpf, the germ cell cluster 19c17 had at least one ZW germ cell expressing a zona pellucida gene [zp2.1, zp2.2, zp2.3, zp3, zp3.2, zp3a.1, zp3a.2, zp3b, zp3d.1, zp3d.2, zp3f.1(si:ch211-14a17.7), zpcx] but no ZZ germ cells expressed any zp gene at 19dpf; although this result was not statistically significant for any single gene (Supplementary Table S4), taken as a group, this finding would be expected if ZW germ cells were already beginning to become different from ZZ germ cells and to differentiate as oocytes even before differences were apparent histologically. Other oocyte-specific genes like zar1 (Miao et al., 2017) (Supplementary Figures S1K, S1L) followed the same pattern.
Zebrafish orthologs of presumed fish vitellogenin receptors vldlr and lrp13 (Hiramatsu N et al., 2013; Reading et al., 2014; Mushirobira et al., 2015; Morini et al., 2020) were expressed only in 30dpf ZW oocytes (Supplementary Figures S1M, S1P). Vldlr may be associated with formation of yolk oil droplets and Lrp13 with vitellogenin uptake (Hiramatsu N et al., 2013). Other suggested vitellogenin receptor candidates, like lrp1ab, lrp2a, lrp5 and lrp6, were not expressed in our dataset (Zhai et al., 2022), perhaps because oocytes larger than 40 µm were excluded.
Dmrt1 is essential for normal testis differentiation in mammals and is expressed in both germ cells and Sertoli cells (Raymond et al., 1999; Raymond et al., 2000; Kim et al., 2007; Herpin et al., 2010; Matson et al., 2010; Kopp, 2012). In addition, variants of dmrt1 are major sex determining genes in some amphibia, perhaps some snakes, in birds, and some fish (Nanda et al., 2002; Kobayashi et al., 2004; Smith et al., 2009; Janes et al., 2014; Cui et al., 2017; Mustapha et al., 2018; Ogita et al., 2020; Ioannidis et al., 2021). In NA gonads at 19dpf, dmrt1 was expressed at low levels in both ZW and ZZ germ cells in 1930c16 (Figures 6U, V). At 30dpf, dmrt1 expression continued in 1930c16 germ cells in both ZW and ZZ genotypes, and in addition, appeared in 30dpf ZW germ cells in 1930c17 (Figures 6U, V) along with phospho1 and scg3, which were both also expressed in Sertoli cells like dmrt1 (Supplementary Table S8). Because in situ hybridization in adult TU laboratory strain zebrafish showed that dmrt1 is expressed primarily in Stage IB oocytes (Webster et al., 2017), we conclude that in our NA fish, the dmrt1-expressing ZW germ cells in 1930c17 represent stage 1B oocytes.
These results are consistent with the conclusion that 1) cluster 1930c16 represents largely undifferentiated germ cells that our histology studies (Figure 2) showed were present in 19dpf ZW and ZZ gonads; 2) that some of the 19dpf ZW germ cells in 1930c16 appear to have had already started expressing weakly some oocyte genes; 3) that ZZ germ cells in 1930c16 remained largely unchanged between 19dpf and 30dpf; 4) that most of the large group of 30dpf ZW-specific germ cell clusters represent Stage IA oocytes expressing strongly oocyte-specific genes; and 5) that 1930c17 represents dmrt1-expressing early Stage IB oocytes.
Meiosis gene functions are important for sex determination, as shown by mutations in meiosis genes that produce mostly neomale offspring (Rodriguez-Mari et al., 2010; Shive et al., 2010; Rodriguez-Mari et al., 2011; Saito et al., 2011; Ramanagoudr-Bhojappa et al., 2018; Takemoto et al., 2020; Blokhina et al., 2021; Islam et al., 2021). Meiotic recombination begins with the introduction of double-strand DNA breaks catalyzed by Spo11, Hormad1, and CCDC36 (IHO1) (Keeney, 2008; Shin et al., 2013; Stanzione et al., 2016). In zebrafish, spo11 is necessary for homolog synapsis, for sperm production, and for preventing females from having abnormal offspring (Blokhina et al., 2019). In our samples, spo11 expression appeared in ZW germ cells, but not in any ZZ cells, at both 19dpf and 30dpf (Figures 6W, X), and hormad1 was expressed in 1930c16 germ cells at both ages and both sex genotypes (Figures 6Y, Z). Repair of Spo11-induced double strand breaks occurs by the DNA recombinases Dmc1 and Rad51 (Hunter, 2015) and Fanconi Anemia genes, including fancl and brca2 (Grompe and D'Andrea, 2001). This process involves sister chromatid cohesion, achieved by cohesin components, including rad21l1 in zebrafish, which is required for oogenesis but not spermatogenesis (Blokhina et al., 2021) and in our samples, was expressed at both ages in both sexes in 1930c16 (Figures 6AA, BB). Other meiosis genes, including smc1b (Figures 6C, D, Supplementary Figures S1C–S1F), which is essential for homolog pairing and synapsis (Islam et al., 2021), rec8a, and rec8b, and genes encoding synaptonemal complex proteins Sycp1, Sycp2, and Sycp3 (Figures 6E, F; Supplementary Figures S1G, S1H; Supplementary Table S8) are stronger markers for young germ cells in cluster 1930c16 than for oocytes in clusters 1930c0, c1, c3, c5, c15, c17, c24. The pattern for meiosis genes in general was opposite from the pattern for oocyte-specific genes, like bmp15, buc, and zp2.2, which increased from 1930c16 to the large group of oocyte clusters. How zebrafish regulate the switch from transcribing meiosis genes to maturing oocyte genes is unknown. The expression of meiosis genes in both ZW and ZZ germ cells (except for spo11) deepens the mystery of the mechanism in zebrafish that causes many meiotic gene mutants to tend to develop as males (fancl, brca2, rad21l1, smc1b, sycp1, sycp2 (Rodriguez-Mari et al., 2010; Shive et al., 2010; Rodriguez-Mari et al., 2011; Saito et al., 2011; Ramanagoudr-Bhojappa et al., 2018; Takemoto et al., 2020; Blokhina et al., 2021; Islam et al., 2021), with mlh1 and spo11 being the exceptions (Feitsma et al., 2007; Leal et al., 2008; Blokhina et al., 2019). A hypothesis is that zebrafish females lack a synapsis checkpoint (Imai et al., 2021).
Histone H2ax phosphorylation results in foci at the sites of DNA breaks in meiosis (Hamer et al., 2003; Blokhina et al., 2021; Imai et al., 2021; Islam et al., 2021). Two H2ax histones had a reciprocal pattern of expression in NA gonads: h2ax1(h2afx1) was expressed strongly in nearly all somatic cells in all four samples and in both ZW and ZZ germ cells in 1930c16 but in few oocytes as defined by buc expression (Supplementary Figures S1I, S1J). Reciprocally, h2ax(h2afx) was expressed strongly in 30dpf ZW oocytes but not in somatic cells and weakly in ZZ germ cells (Supplementary Figures S1K, S1L). These results suggest that during oogenesis, histone H2ax replaces the somatic and early germ cell histone H2ax1 in zebrafish oogenesis.
Support cells (granulosa cells in the ovary and Sertoli cells in the testis) control germ cell proliferation, differentiation, and maturation. Support cells in fish express gsdf both in ovaries and in testes and gsdf is required for oocyte maturation (Sawatari et al., 2007; Gautier et al., 2011; Imai et al., 2015; Zhang et al., 2016; Yan et al., 2017; Jiang et al., 2022). Furthermore, a variant of gsdf is the major male sex determining gene in Luzon medaka and sablefish (Myosho et al., 2012; Herpin et al., 2021). In the combined analysis, gsdf was expressed strongly in just three cell clusters: 1930c30, c21, and c8, identifying support cells or their precursors (Figures 7A, B). In 19dpf gonads, gsdf was expressed in 1930c8 and 1930c21 in both ZW and ZZ cells (Figures 7A–C, E) suggesting that these are support cell precursors and, that ZW and ZZ pre-support cells at 19dpf are transcriptionally similar (Figures 7C, E).
FIGURE 7. Support cell gene expression merging both time points and both sex genotypes. (A) gsdf expression across the whole combined dataset. The rectangle marks gsdf-expressing support cells and their precursors, expanded to show cluster assignments in color. (B) Enlargement of rectangle from part A, showing gsdf expression in clusters 1930c30, c21, and c8 combining all four conditions. (C) gsdf expression in 19dpf ZW gonads. (D) gsdf expression in 30dpf ZW cells. (E) gsdf expression in 19dpf ZZ cells. (F) gsdf expression in 30dpf ZZ cells. (G–L) amh expression in samples as described for (B–F). (M–R) foxl2a expression. Box in M around 1930c18 is expanded as inserts in (N–R). (S–X) cyp19a1a expression. Boxed insert in (S) around 1930c18 appears as inserts in (T–X).
At 30dpf, gsdf expression differed substantially in ZW and ZZ gonads. Cluster 1930c30 contained almost exclusively 30dpf ZZ cells (Figure 7F) representing Sertoli cells. In contrast, ZW cells at 30dpf occupied 1930c21 and 1930c8 (Figure 7D), suggesting that these clusters contain granulosa cells in addition to support cell precursors. Both inha, which is necessary for final oocyte maturation and ovulation (Lu et al., 2020), and igf3, which is essential for zebrafish ovarian follicles to develop beyond the primary growth stage (Xie et al., 2021), had virtually the same expression pattern as gsdf (Supplementary Figures S2A–S2F). These results suggest: 1) gsdf-expressing 30dpf ZW cells in 1930c21 represent granulosa cells; 2) gsdf-expressing 30dpf-ZZ cells in 1930c30 represent Sertoli cells; 3) some gsdf-expressing cells of both sex genotypes at 19dpf in 1930c8 and 1930c21 likely represent bipotential precursors of support cells and at 30dpf, the ZW cells represent granulosa cell precursors; 4) substantial differentiation of sex-specific cell types occurs between 19dpf and 30dpf.
Sertoli cells in fish strongly express amh, dmrt1, and sox9a (Chiang et al., 2001a; Kobayashi et al., 2004; Guo et al., 2005; Rodriguez-Mari et al., 2005; Yan et al., 2005; Lin et al., 2017; Webster et al., 2017). AntiMüllerian Hormone (Amh), secreted by Sertoli cells in mammalian testes, destroys the developing female reproductive tract, and variants of amh or its receptor gene amhr2 are male sex determinants in several species of fish (Morinaga et al., 2007; Hattori et al., 2012; Kamiya et al., 2012; Li et al., 2015; Ieda et al., 2018; Pan et al., 2021). At 19dpf, a few pre-support cells of both ZW and ZZ genotypes weakly expressed amh (Figures 7G–I, K) and dmrt1 (Supplementary Figures S2G–I, S2K). Recall, dmrt1 was also expressed in germ cells, Figures 6U, V). At 30dpf, amh and dmrt1 were still weakly expressed in just a few ZW cells but were strongly expressed in ZZ cells in 1930c30 (Figures 7J–L; Supplementary Figures S2J, S2L). Mammalian Sertoli cells express Sox9, which maintains testis development (Morais da Silva et al., 1996; Qin and Bishop, 2005; Barrionuevo et al., 2006). In our samples, sox9a was expressed strongly in ZZ Sertoli cells (1930c30) and was detected in some 1930c21 cells in ZW gonads and 19dpf ZZ gonads (Supplementary Figures S2M–S2R). These results support the assignment of the 30dpf ZZ-specific cluster 1930c30 as Sertoli cells and 19dpf ZZ cells in 1930c21 as support cell precursors.
Ovarian follicle cells in mammals are multi-layered, with inner granulosa cells that form gap junctions with the oocyte and transport substances, and an outer granulosa cell layer that produces estradiol (Gilchrist et al., 2008). In teleosts, however, ovarian follicle cells form a single-cell epithelium over the oocyte, but it is unclear whether this single layer provides both the transport function through gap junctions and the endocrine function or if that single cell layer consists of two cell types, one that transports substances and one that provides estradiol (Devlin and Nagahama, 2002). The mammalian granulosa gene Foxl2 has two duplicates in zebrafish that arose in the teleost genome duplication (foxl2a, foxl2b) plus a third paralog, lost in mammals, that arose in a genome duplication event before the divergence of teleosts and mammals (foxl2l, alias zgc:194189 or foxl3) (Crespo et al., 2013; Caulier et al., 2015; Bertho et al., 2016; Webster et al., 2017; Yang et al., 2017; Dai et al., 2021a; Liu et al., 2022). In our combined analysis of both ages and both sex genotypes, foxl2a was expressed in two clusters: in the support cell precursors in 1930c21 similar to the pattern of gsdf and amh, and additionally, in 1930c18 (Figure 7M). At 19dpf in 1930c21, expression of foxl2a was similar to that of gsdf, but weaker, and appeared in both ZW and ZZ cells (Figures 7O, Q), consistent with these cells being bipotential support cell precursors. At 30dpf, foxl2a was also expressed in ZW 1930c21, again consistent with these cells representing granulosa cells. A second major foxl2a expression domain appeared in cluster 1930c18 (Figure 7M, box; N-R, insert). Cluster 1930c18 contained few 19dpf cells and only one 30dpf ZZ cell (Figures 7N-R, insert), showing that it was a cell type that began to develop before 19dpf in both ZW and ZZ cells, but by 30dpf, it was ZW-specific.
Expression of foxl2b was like that of foxl2a (Supplementary Figures S2S, S2X). Expression of foxl2l, which lacks a mammalian ortholog, showed scant expression limited to Stage IA and small Stage IB oocytes; other studies show that oocytes at a more mature stage strongly express foxl2l (Kikuchi et al., 2020; Liu et al., 2022).
The foxl2a- and foxl2b-expressing cells in 1930c21 may be performing the transport function of mammalian granulosa cells because the gap junction connexin gene gja11(cx34.5) was specifically expressed in 1930c21 in a pattern like that of gsdf and foxl2a at 19dpf in presumed support cell precursors and at 30dpf in granulosa cells in ZW gonads. Sertoli cells in ZZ gonads also expressed gja11 (Supplementary Figures S2Y, S2DD). In situ hybridization had previously detected gja11 expression in follicle cells surrounding Stage II oocytes (cortical alveolus stage, 140–340 μm (Bogoch et al., 2022), which were not present in our samples), but not in Stage IB oocytes (Liu et al., 2022); our data detected gja11 expression at earlier stages presumably due to increased sensitivity. The oocyte partner of Gja11 could be encoded by gjc4a.1(cx44.2) or gjc4b(cx43.4), which were expressed strongly and rather specifically in 30dpf oocytes (Santos et al., 2007) (Supplementary Figures S2KK, S2LL).
The estrogen-producing function of mammalian granulosa cells might be mediated by a cell type different from the 1930c21 cells that may perform the transport function. Gonadal aromatase, encoded by cyp19a1a, converts testosterone to estradiol or androstenedione to estrone (Chiang et al., 2001b; Chiang et al., 2001c; Tenugu et al., 2021). At 19dpf, a few cyp19a1a-expressing cells appeared with 1930c21 support cell precursors in both ZW and ZZ genotypes (Figures 7S, U, W), while at 30dpf, cyp19a1a was expressed in ZW cells in 1930c18 but not in ZZ cells (Figures 7V, X). The strong expression of gsdf, foxl2a, and gja11 in 1930c21 and foxl2a and cyp19a1a in 1930c18 suggests that the transport function and estrogen-production function of mammalian granulosa cells may be carried out by two different follicle cell types in zebrafish 30dpf ZW ovaries.
We conclude that expression patterns of gsdf, foxl2a, foxl2b, and cyp19a1a suggest that: 1) at 19dpf, support cell precursors were similar in ZW and ZZ gonads; 2) that 30dpf ZW but not ZZ gonads maintained these common support cell precursors; 3) that granulosa cells appeared in the UMAP near support cell precursors but Sertoli cells were distant; and 4) that the aromatase function of 30dpf ovarian follicle cells was provided by a ZW cell type different from the strongly gsdf-expressing 30dpf ZW follicle cell type.
Sex steroids are important for zebrafish gonad development (Menuet et al., 2004; de Waal et al., 2009; Caulier et al., 2015; Luzio et al., 2016; Lu et al., 2017; Zhai et al., 2018), but we know little about steroidogenic cell development in ZW vs. ZZ zebrafish.
Nr5a1 and Star initiate gonadal steroidogenesis (Figure 8A, after (Tenugu et al., 2021). Nr5a1 (Steroidogenic Factor-1, SF1) is a major regulator of genes that encode gonadal steroidogenesis enzymes in theca and Leydig cells and it is one of the earliest markers of gonadal differentiation, turning on in human gonads before SRY (Morohashi et al., 1995; Ikeda et al., 1996; Hanley et al., 1999). Zebrafish has two co-orthologs of Nr5a1 (nr5a1a and nr5a1b) (von Hofsten et al., 2001; Kuo et al., 2005) from the teleost genome duplication (Amores et al., 1998; Postlethwait et al., 1999; Taylor et al., 2003; Jaillon et al., 2004). Loss-of-function mutations in nr5a1a disrupt development strongly in the interrenal and mutations in nr5a1b cause more severe phenotypes in the gonad (Yan et al., 2020). At 19dpf, nr5a1b was expressed in a few support precursor cells (1930c21) and a few 1930c6 cells, while at 30dpf, nr5a1b was strongly expressed in 1930c12 but only weakly in granulosa or Sertoli cells (Figure 8B). We conclude that in 1930c12, the ZW cells are theca cells, and the ZZ cells are Leydig cells. Leydig cells in 1930c12 also specifically expressed insl3, skor2(zgc:153395), and pcdh8 (Supplementary Table S8).
FIGURE 8. Steroidogenesis. (A). An abbreviated pathway of steroidogenesis (after (Tenugu et al., 2021)). (B) nr5a1b expression. The left panel shows expression in the entire dataset. The insert shows cluster demarcation for the most relevant steroidogenic enzymes. Subsequent panels show, at the top 1930c21, the support cell precursor and granulosa cell cluster and at the bottom the clusters corresponding to the insert in the left panel. Displayed from left to right are the enlargements of all four conditions: 19dpf ZW gonads, 30dpf ZW gonads, 19dpf ZZ gonads, and 30dpf ZZ gonads. Cluster numbers are indicated. (C) cyp11a1.2 displayed as explained for nr5a1b. (D) cyp17a1. (E) cyp19a1a. (F). hsd17b1. (G) hsd17b3. (H) cyp11c1. (I) hsd11b2. Abbreviations: L, Leydig cells; th, theca cells.
StAR (steroidogenic acute regulatory protein) gene transcription is turned on by Nr5a1 (Sugawara et al., 1997). In an initial step (Figure 8A), StAR brings cholesterol into the inner mitochondrial membrane (Miller and Auchus, 2011). Zebrafish adult gonads express star (Bauer et al., 2000; Ings and Van Der Kraak, 2006; de Waal et al., 2009), and although NA zebrafish at 19dpf had few star-expressing cells, at 30dpf, star was expressed strongly in theca and Leydig cells (1930c12) and in a few oocytes (Supplementary Figure S3B).
Cyp11a1 in mammals converts cholesterol to pregnenolone (Lai et al., 1998). Zebrafish has tandem duplicates of human CYP11A1 (cyp11a1.1 and cyp11a1.2, previously called cyp11a1 and cyp11a2, respectively) (Hu et al., 2001; Goldstone et al., 2010; Parajes et al., 2013); these genes originated in a duplication event that occurred after the divergence of zebrafish and carp lineages (gene tree ENSGT00940000158575). Disruption of cyp11a1.2 results in androgen loss, and homozygous mutants develop into feminized males with disorganized testes and few spermatozoa (Parajes et al., 2013; Li et al., 2020a; Wang et al., 2022). At 19dpf, cyp11a1.2 showed scant expression, but at 30dpf, it was widely expressed in ZW theca and ZZ Leydig cells and in 1930c6 (Figure 8C). In contrast, expression of the tandem duplicate cyp11a1.1 was not detected at 19dpf (Supplementary Figure S3C) and at 30dpf, it appeared in many ZW oocytes, as expected from its known maternal expression (Hsu et al., 2002; Parajes et al., 2013; Wang et al., 2022), but it was not expressed in ZZ germ cells. The cyp11a1.1 gene was expressed weakly in ZZ Leydig cells but in no theca cells, and weakly in a few 1930c18 cells (Supplementary Figure S3C). We conclude that the expression of cyp11a1.1 and cyp11a1.2 diverged after the tandem duplication event and that cyp11a1.2 maintained the ancestral pattern in theca and Leydig cells but cyp11a1.1 evolved stronger expression in the oocyte germ cell lineage, which does not appear to be an ancestral expression domain because medaka, which lacks the tandem duplication, does not express cyp11a1 in oocytes (Nakamoto et al., 2010). The regulatory mechanism causing different expression patterns for these two tightly linked tandemly duplicated genes is unknown.
Cyp17a1 and Hsd3b act on the product of Cyp11a1, pregnenolone, and convert it to androstenedione (Figure 8A). Knockout of cyp17a1 in AB zebrafish leads to loss of juvenile ovaries and an all-male population with reduced male secondary sex characteristics (Zhai et al., 2018). At 19dpf, cyp17a1 expression was detected in many support cell precursors and in 1930c6, which may be theca/Leydig cell precursors (Figure 8D). At 30dpf, cyp17a1 was expressed weakly in granulosa and Sertoli cells, but at a high level in ZW theca and ZZ Leydig cells, and in many oocytes (Figure 8D). Expression of hsd3b1 was like that of cyp17a1, but at lower levels and in fewer cells (Supplementary Figure S3D). Expression of hsd3b2 (Lin et al., 2015) appeared almost exclusively in oocytes, like cyp11a1.1 (Supplementary Figure S3E).
Cyp19a1 and Hsd17b enzymes carry out the next step, converting androstenediol to estradiol (Miller and Auchus, 2011). In principle, these reactions can occur in either of two ways (Figure 8A): 1) Cyp19a1 can form estrone from which Hsd17b1 forms estradiol (E2); alternatively, 2) Hsd17b3 can form testosterone, which Cyp19a1 converts to estradiol (Figure 8A). Cyp19a1 is important for zebrafish sex development because cyp19a1a mutants develop mostly as males (Dranow et al., 2016; Lau et al., 2016; Yin et al., 2017; Romano et al., 2020; Wu et al., 2020). In 19dpf NA zebrafish, cyp19a1a expression was found in a few support cell precursors (1930c21) in both ZW and ZZ gonads (Figure 8E). At 30dpf, cyp19a1a expression was not detected in any ZZ cells, as expected from its role in estrogen production. Transcripts for cyp19a1a were present in a few ZW theca cells (Figure 8E, 1930c12), but the focus of cyp19a1a expression was in ZW 1930c18 cells (Figure 8E), which also strongly expressed foxl2a and foxl2b but did not strongly express gsdf (Figures 7A, M-R; Supplementary Figure S2S-S2X). Cluster 1930c18 ZW cells at both 19dpf and 30dpf also expressed specifically the transcription factor arxa (Supplementary Figure S3F), whose mammalian ortholog Arx is expressed in, and is necessary for, the maintenance of fetal Leydig progenitors in mouse (Miyabayashi et al., 2013) and the formation of normal genitalia and sex steroid levels in humans (Gupta et al., 2019; Basa et al., 2021). The cyp19a1a cluster 1930c18 also expressed strongly and specifically the G protein-coupled receptors s1pr3a and gpr17, the junctional adhesion molecule jam2b, and the signaling gene wnt11 [wnt11r (Postlethwait et al., 2019)]. Expression of steroidogenic enzymes other than cyp19a1a in 1930c18 was either not detected or detected weakly, except for hsd11b2, which was broadly expressed in nearly all stromal/interstitial cell clusters marked by the collagen gene col1a1a (compare Figure 8I to Figure 9A). These results are consistent with zebrafish having two types of ovarian follicle cells, one strongly and specifically expressing cyp19a1a, arxa, foxl2a, s1pr3a, gpr17, and wnt11 (1930c18), and the other expressing strongly gsdf, foxl2a, lamb2l, inha, and igf3 (1930c21).
FIGURE 9. Stromal/interstitial clusters. (A) Expression of col1a1a defines stromal/interstitial cells (Liu et al., 2022). The left large panel combines cells of all four conditions for the complete dataset and the four smaller panels to the right show an enlargement of the col1a1a-expressing domain for each condition independently. At 19dpf, ZW and ZZ expression patterns are similar but at 30dpf, some clusters are sex-genotype specific. (B) The UMAP is in the left large panel; the middle panel shows the col1a1a-expressing region for samples separated by sex genotype (red, ZW; blue, ZZ); and the right panel shows samples separated by age (red, 19dpf; blue, 30dpf). (C) acta2 expression, with panels as described for (A). (D) panx3 expression in the 30dpf ZZ-specific cluster 1930c32. (E) efn2b expression, indicating likely arterial endothelial cells in 1930c22 boxed in the large panel and expanded in the four condition-specific panels to the right. (F) lyve1a expression, a marker of lymphatics, labeling 1930c22.
Hsd17b, the second estrogen-producing enzyme activity (Figure 8A), is the master sex determinant in Seriola fishes (Purcell et al., 2018; Koyama et al., 2019). Hsd17b activity could be provided in principle by either Hsd17b1 or Hsd17b3 (Mindnich et al., 2004; Mindnich et al., 2005). At 19dpf, hsd17b1 expression appeared in ZZ pre-support cells but not in ZW cells in our samples (Figure 8F). By 30dpf, however, hsd17b1 expression was detected in gsdf-expressing ZW granulosa cells in 1930c21 (Figure 8F) as in adult AB strain female zebrafish (Liu et al., 2022). Expression of hsd17b1, was not detected in the cyp19a1a-expressing 1930c18 ZW cells or in any 30dpf ZZ cells.
In the alternative pathway of estradiol biosynthesis (Figure 8A) and in contrast to hsd17b1, hsd17b3 expression was low at both 19dpf and 30dpf (Figure 8G). We conclude that, as in adult AB strain zebrafish (Liu et al., 2022), hsd17b3 appears to play a smaller role than hsd17b1 in estrogen synthesis in juvenile zebrafish.
These results suggest that three different transcriptomic clusters are important for estrogen production in 30dpf ZW zebrafish (Supplementary Figure S3J-S3L): 1) theca cells (ZW cells in 1930c12) convert pregnenolone to androstenedione using Cyp17a1 (Figure 8D); 2) follicle cells in 1930c18 transform androstenedione to estrone catalyzed by Cyp19a1a (Figure 8E); and 3) a different type of follicle cell in 1930c21 converts estrone to estradiol by Hsd17b1 (Figure 6F). It is possible that the 1930c18 cyp19a1a-expressing cell type and the hsd17b1-expressing cell type are simply different states or stages of the same individual cells. Also, it could be that Hsd17b3 might become more important in older animals or in more mature ovarian follicles than were present in our samples.
Androgens in fish gonads include testosterone and the principal teleost androgen 11-keto-testosterone (11 KT) (Kusakabe et al., 2003; de Waal et al., 2008; Nelson et al., 2013; Tokarz et al., 2015; Tenugu et al., 2021). 11KT is produced by the enzymes Hsd17b3, Cyp11c, and Hsd11b2 (Figure 8A). In 19dpf NA zebrafish, cyp11c1 was expressed only in a few 1930c6 cells in both ZW and ZZ gonads (Figure 8H), consistent with bi-potential gonads. At 30dpf, however, cyp11c was expressed strongly and specifically in ZZ Leydig cells but not in ZW theca cells (Figure 8H). Within 1930c12, ZW cells, but not ZZ cells, expressed the “estrogen genes” cyp19a1a (Figure 7S, Figure 8E) and foxl2a (Figure 7M); reciprocally, ZZ cells, but not ZW cells, expressed the “androgen gene” cyp11c1 (Figure 8H), confirming cell assignments as theca vs. Leydig cells.
Hsd11b2 can follow Cyp11c to produce 11 KT (Figure 8A). The hsd11b2 gene was expressed more broadly than any of the other steroidogenic enzymes discussed here (Figure 8I). At 19dpf, both ZW and ZZ gonads expressed hsd11b2 both in pre-support cells and in 1930c6 cells (Figure 8I), as well as in several stroma/interstitial cell clusters (see Figure 9). At 30dpf, hsd11b2 was expressed in ZW gonads in granulosa cells and cyp19a1a-expressing 1930c18 cells, and in ZZ gonads in Sertoli cells and Leydig cells, as well as in stromal/interstitial cells (Figure 8I). In vitro enzyme assays show that zebrafish Hsd17b3 converts androstenedione to testosterone (Mindnich et al., 2005), but we did not specifically detect hsd17b3 expression in ZZ NA gonads (Figure 8G). Hsd17b1 is unlikely to perform this function because, although hsd17b1 was expressed in pre-support cells in 19dpf ZZ animals, expression was not detected at 30dpf in ZZ gonads (Figure 8F). This result suggests that at 30dpf, neither hsd17b1 nor hsd17b3 is likely to make a significant contribution to 11 KT formation in ZZ zebrafish, although they might at later developmental stages. A different Hsd17b enzyme might provide Hsd17b3 activity in zebrafish testes or our samples may represent a stage too early for significant production of testosterone or 11 KT. Breeding tubercles on male pectoral fins are an assay for androgen activity in zebrafish, but they first appear on males much older than those in our samples (McMillan et al., 2013; Dai et al., 2021b).
Steroid receptor genes were expressed in several steroidogenic cell types. The estrogen receptor gene esr1 was expressed in only a few cells in NA gonads at either 19 or 30dpf, consistent with a weak effect of esr1 mutants on ovary development (Lu et al., 2017). At 19dpf in both ZW and ZZ gonads, esr2a was expressed in pre-support cells and in some interstitial cells, and at 30dpf, in ZW granulosa cells, in cyp19a1a-expressing 1930c18 cells, in a few oocytes, and in some ZZ Leydig cells, as well as in some interstitial/stromal cells in at both ages and sex genotypes (Supplementary Figure S3G). The esr2b gene was expressed like esr2a in 30dpf ZW gonads, but also in 30dpf ZZ Sertoli cells (Supplementary Figure S3H). In addition, unique among the steroid-related gene set examined, esr2b was expressed strongly in19dpf ZW cells in 1930c18 (the cyp19a1a cluster) (Figure 8F). The androgen receptor gene ar was expressed with virtually the same pattern as esr2b (Supplementary Figure S3I). Expression of ar in ZZ cells is in accordance with the expectation that zebrafish ar mutants would mostly develop as females, and ar expression in ZW cells could help explain why even mutant females have reduced fertility (Crowder et al., 2018; Yu et al., 2018).
Interstitial and stromal cells provide structural support, erect barriers, transmit forces, and supply signals, thus offering functions distinct from other gonadal cell types (Kinnear et al., 2020). Many interstitial and stromal cell types construct substantial intercellular matrix and express the collagen gene col1a1a (Liu et al., 2022) (Figure 9A). Sex- and age-specific clusters were prominent among col1a1a-expressing interstitial/stromal cells (Figure 9B). One conspicuous cluster was the 30dpf ZW-restricted cyp19a1a-expressing cluster 1930c18 discussed above, which has strong col1a1a expression (Figure 9A) and low gsdf expression (Figure 7A), further distinguishing the cyp19a1a-expressing granulosa cells in 1930c18 from the hsd17b1-expressing granulosa cells in 1930c21 (Figure 8F). In contrast, clusters 1930c20 and 1930c38 were 30dpf ZZ-specific (Figure 9B). These ZZ-specific clusters expressed many extracellular matrix components including the small leucine-rich proteoglycan gene lumican (Supplementary Figure S3A), nog2, ccn2b(ctgfb), ccn4b(wisp1b), and ogna (Supplementary Table S8). Cluster 1930c32 had far more ZZ cells than ZW cells at 30dpf and no cells at 19dpf, and specifically expressed the channel-forming pannexin gene panx3 (Figure 9D), whose mammalian ortholog is a marker for the epididymis (Turmel et al., 2011), suggesting a role in zebrafish male gonadal ducts.
Contractile cells in 1930c6 specifically expressed the smooth muscle genes acta2 (Figure 9C), csrp1b, cnn1b, tpm2, and mylkb. This strong expression domain appeared in 30dpf gonads and a smaller domain in 19dpf gonads, but about equally in ZW and ZZ cells. In human testes, ACTA2, CSRP1 and other contractile protein genes are expressed in peritubular myoid cells, a layer of flattened contractile cells around seminiferous tubules that help transport spermatozoa and testicular fluid (Maekawa et al., 1996). Peritubular myoid cells also inhabit the interstitial region of fish testes (Schulz and Nóbrega, 2011). ZW cells also expressed this set of smooth muscle cell genes, as do cells in human ovaries (Fan et al., 2019).
Vascular endothelial cells line arterials, venous vessels, and lymphatics (Wolf et al., 2019; Gurung et al., 2022) and enter zebrafish gonads early in gonadogenesis (Kossack et al., 2023). For arterials, Vegf-receptor-2 (kdr, kdrl) activates Notch1 signaling (notch1a, notch1b, notchl, dll4) to provide Ephrin-B2 (efnb2a, efnb2b) at the cell surface (Villa et al., 2001; Covassin et al., 2006b; Masumura et al., 2009; Scheppke et al., 2012; Nakayama et al., 2013). Vegf-receptor, Ephrin-B2, and Notch signaling genes (e.g., kdr, Supplementary Figure S3B) were expressed in 1930c22 at both ages and in both sex genotypes, identifying arterial endothelium. For venous endothelium, smarca4a acts via CouptfII (nr2f2) to place Ephb4 (ephb4a) (Supplementary Figure S3C) and ephb4b) on the cell surface (Pereira et al., 2000; Wang et al., 2002; You et al., 2005; Muto et al., 2011; Davis et al., 2013; Model et al., 2014; Cui et al., 2015). The venous gene set was also expressed in 1930c22, suggesting that gonadal vein endothelial cells also appeared in this cluster. For lymphatics, sox18 turns on Prox1 (prox1a, prox1b) to provide Vegfr3 (flt4) activity and thereby lymphatic identity supported by enriched expression of lyve1a (Figure 7F) and lyve1b (Kaipainen et al., 1995; Jackson et al., 2001; Prevo et al., 2001; Francois et al., 2008; Lee et al., 2009; Aranguren et al., 2013). We conclude that NA gonads at both 19 and 30dpf had all three types of endothelia but no vessel types appeared to be age- or sex-specific.
Several types of white blood cells provide gonads with developmental and homeostatic functions. In mammalian testes, special lymphocytes are essential for normal testis function (Mossadegh-Keller and Sieweke, 2018; Garcia-Alonso et al., 2022). In the mammalian ovary, cytokine-mediated inflammation helps regulate ovulation (Field et al., 2014; Fan et al., 2019) and in domesticated zebrafish, activation of a specific macrophage population propels ovarian failure and masculinization (Bravo et al., 2023). To study leukocytes in developing NA gonads, we identified cells expressing the pan-leukocyte genes coro1a, (Figure 10A), ptprc (cd45), and lcp1 (l-plastin) (Zakrzewska et al., 2010; Torraca et al., 2014) (Supplementary Table S8), all of which marked the same clusters and thus define the leukocytes in our dataset (Figure 10A).
FIGURE 10. Immune cells. (A) coro1a expression identifying leukocytes displayed for all four conditions analyzed together. The remainder of the panels show the boxed region rotated counterclockwise. (B) Cells labeled according to sex genotype (red, ZW; blue, ZZ). (C) Cells labeled according to age (red, 19dpf; blue, 30dpf). (D) ikzf1. (E) rag1. (F) cd4-1. (G) foxp3a. (H) il2rb. (I) igl1c3. (J) spi1a. (K) mpeg1.1. (L) mfap4.1. (M) cxcf3.2. (N) mxf. (O) irf8. (P) mpx. (Q) il13. (R) gata3. (S) itga2b. (T) nitr1m. (U) dicp1.5-6. (V) nkl.4. (W) c3a.4.
None of the leukocyte clusters were exclusively ZW or ZZ (Figure 10B, Supplementary Table S7). Leukocyte cell types, however, partially sorted out by age, with some clusters being almost exclusively 30dpf (1930c2, c13, c19) (Figure 10C). These results suggest that at these stages, NA gonads may not have major sex-specific leukocyte populations and that between 19dpf and 30dpf, new leukocyte populations appear in the gonads.
Lymphocytes and active immunity develop at about 3 weeks post-fertilization in the interval between our sample (Lam et al., 2004). Lymphocytes and natural killer (NK) cell development in mouse and zebrafish require Ikaros (ikzf1), which is expressed early in the lymphocyte lineage (Winandy et al., 1995; Wang et al., 1996; Willett et al., 2001; Schorpp et al., 2006). Both sex genotypes and both ages expressed ikzf1 in several clusters (Figure 10D). In lymphocytes, Rag1 and Rag2 help rearrange immunoglobulin genes and T-cell receptor genes; in NA gonads, rag1 and rag2 were expressed in 1930c27 (Figure 10E) even at 19dpf in both sex genotypes, identifying these cells as lymphoid. A few 1930c27 and adjacent 30dpf cells in 1930c2 expressed T-cell marker genes including cd4-1 (Figure 10F), cd8a, cd8b, zap70, and cd2(si:ch211-132g1) (Blackburn et al., 2014; Moore et al., 2016b; Carmona et al., 2017; Shao et al., 2018). In NA gonads, the regulatory T-cell (Treg) gene foxp3a (Hui et al., 2017) was expressed in both ZW and ZZ gonads at 30dpf (Figure 10G), which contrasts to the testis-restricted expression of foxp3a in adult laboratory strain zebrafish (Li et al., 2020b)); this early foxp3 expression in genetically female cells could explain why gonad function deteriorates in both ovary and testis in foxp3a mutants (Li et al., 2020b). Mixed with T-cells in 1930c2 and extending into 1930c13 were 30dpf cells expressing markers for some NK-like cells including il2rb (Carmona et al., 2017) (Figure 10H), suggesting that in NA zebrafish, as in mammals, gene expression in NK-like cells is similar to that in some T-cells (Bezman et al., 2012).
B-lymphocytes also appeared in both 19dpf and 30dpf gonads of both sexes in 1930c26 marked by both light and heavy immunoglobulin genes including igl1c3 (Figure 10I), igic1s1, igl3v5, and ighv1-4, as well as the B-cell-specific transcription factor gene pax5 (Cobaleda et al., 2007; Moore et al., 2016b). Cluster 1930c26 also expressed cxcr4a, encoding the receptor for the lymphocyte chemoattractant Cxcl12 (Bleul et al., 1996).
Myeloid cells include neutrophils, macrophages, monocytes, mast cells, eosinophils, and basophils (Wattrus and Zon, 2021). Early in myeloid development, zebrafish hematopoietic cells express spi1a and spi1b and these genes are required for macrophage specification (Bukrinsky et al., 2009; Roh-Johnson et al., 2017). NA gonads expressed spi1a in the 30dpf-specific cluster 1930c25 and the mixed-age clusters 1930c7, c9, and c28 (Figure 10J). Clusters 1930c7 and c9 expressed macrophage-specific genes like mpeg1.1 and mfap4.1(mfap4, zgc:77076) (Figures 10K, L) and marco. Macrophages in cluster 1930c7 and c9 were present in both sex genotypes. Macrophage migration during infection and injury in zebrafish depend on Cxcr3, but of the three cxcr3 paralogs in zebrafish, only cxcr3.2 is required (Sommer et al., 2020); for the gonad, this may be because cxcr3.2 is the only one of the three paralogs expressed strongly in most 1930c7 and c9 macrophages (Figure 10M). Functionally different populations of macrophages have been identified in mammalian testes (DeFalco et al., 2015; Garcia-Alonso et al., 2022), but NA gonads did not appear to have macrophage sub-types expressing the relevant orthologs of the mammalian markers. Zebrafish gonads, however, do have multiple macrophage subtypes because 1930c28 represents a primarily 30dpf ZW population of spi1+ mpeg1.1+ cells that uniquely expressed mxf (Figure 10N) and other myxovirus (influenza) resistance paralogs, as well as the inflammation marker tnfa. Cells expressing the macrophage differentiation regulator irf8 are present in 1930c7, c9, and c28 in both NA sex genotypes already by 19dpf (Figure 10O) and so macrophages are likely in place in NA to help remodel ovarian follicles as in laboratory strain zebrafish (Bravo et al., 2023).
Neutrophil-specific markers, including mpx (Figure 10P), lyz, and mmp13a were expressed strongly in 1930c25 in all four age/sex-genotype conditions. Other genes used as neutrophil markers, including nccrp1, cebpa, cpa5, cma1, and prss1 (Tang et al., 2017; Rougeot et al., 2019), were expressed not only in 1930c25, but also strongly in several other clusters, decreasing their utility as neutrophil-specific markers in NA gonads.
Group 2 innate lymphoid cells (ILC2s, nuocytes, Th2 cells) in mammals help provide innate immunity against helminth infection and play a role in allergic airway hyperreactivity (Moro et al., 2010; Neill et al., 2010; Barlow et al., 2012; Klein Wolterink et al., 2013). Key ILC2 genes include IL4, IL5 (zebrafish ortholog: csf2rb), and IL13 (Bottiglione et al., 2020). In zebrafish, il4 and il13 are essential to suppress type-1 immune responses (Bottiglione et al., 2020) and they were expressed specifically in 1930c31 (Figure 10Q). Gata3 is essential for normal expression of IL3 and CSF2R (but not IL4) in ILC2 cells (Zhu et al., 2004), and gata3 was expressed specifically in 1930c31 and c33 (Figure 10R). Genes strongly expressed in 1930c31 and c33 included il11b, csf3a, gata3 and many genes annotated as ‘serine-type endopeptidase activity’ (including si:dkey-78l4.2 and a dozen of its tandem duplicates), which are also strongly expressed in the 5dpf larval thymus (Farnsworth et al., 2019). We hypothesize that the ILC2-like cells in 1930c31 and c33 in zebrafish gonads may be acting to help regulate the extent of inflammation associated with developmental changes as gonads mature.
Thrombocytes appeared in 1930c37, which contained cells of both ages and sex genotypes, according to marker genes itga2b(cd41) (Figure 10S), mpl, gp1bb, zfpm1 and coagulation factor V (f5) (Lin et al., 2005; Khandekar et al., 2012; Tang et al., 2017).
Natural killer (NK)-like cells may be a variant type of cytotoxic innate lymphoid cell. Zebrafish NK-like cells express NK markers like il2rb (Carmona et al., 2017) (Figure 10H). The 1930c2, c13, and c19 cells also expressed novel immune-type receptors (NITRs, e.g., nitr1m, Figure 10T) that have properties like those of mammalian natural killer receptors (NKRs) (Yoder et al., 2010). The nitr gene expression detected in the zebrafish ovary but not as maternal transcripts in oocytes (Yoder et al., 2010), could have been from the NK-like cells described here. Diverse immunoglobulin domain-containing protein (DICP) genes have been suggested to be associated with NK-like cells (Haire et al., 2012; Rodriguez-Nunez et al., 2016; Carmona et al., 2017) and the expression of dicp genes like dicp1.5-6 (Figure 10U) in 1930c2, c13, and c19 supports this notion. The same cell set expresses NK-lysin genes: nkl.4 expression, which is upregulated after viral infection (Pereiro et al., 2015) and increases ten-fold in rag2-deficient zebrafish (Moore et al., 2016a), was expressed specifically in NA gonads in 1930c19 (Figure 10V).
Cluster 1930c11 cells expressed the pan-leucocyte markers coro1a, ptprc (cd45), and lcp1, supporting a white blood cell identity (Figure 10A), but their specific cell type assignment is unclear. 1930c11 cells expressed many genes expressed almost exclusively in the thymus of 5dpf zebrafish (Farnsworth et al., 2019), including c3a.4 (Figure 10W), si:ch73-160i9.2, mfap4.9 (ENSDARG00000095746), si:dkey-203a12.7, and tuno4,2. Cluster 1930c11 also expressed a number of spink2 paralogs (spink2.5, spink2.10, spink2.11) that are expressed only in the zebrafish thymus at 5dpf (Farnsworth et al., 2019), while human SPINK2 is proposed as a marker of hematopoietic stem cells (Calvanese et al., 2022). Nearly all 1930c11 genes were expressed in all four age/genotype conditions. We conclude that 1930c11 may represent an early-stage blood cell in gonads.
Strains of zebrafish long maintained in laboratories pass through a juvenile ovary phase in which all individuals initially form a gonad with oocytes that die in fish that become males but survive in individuals that become females (Takahashi, 1977; Uchida et al., 2002; Maack and Segner, 2003; Wang et al., 2007; Rodriguez-Mari et al., 2010). Those strains have what appears to be a polygenic sex determination mechanism (Bradley et al., 2011; Anderson et al., 2012; Howe et al., 2013; Wilson et al., 2014; Luzio et al., 2015). In contrast, in NA strain zebrafish, which have a ZW female/ZZ male chromosomal sex determination mechanism (Anderson et al., 2012; Wilson et al., 2014), ZZ individuals do not develop juvenile ovaries but instead directly develop testes (Figure 2). Although all NA strain ZW individuals initially form gonads with oocytes, some ZW fish lose these juvenile oocytes as their gonads become a testis, mimicking laboratory strains. Thus, the distinctive feature of the NA strain is the direct development of testis in ZZ fish, bypassing the juvenile ovary phase.
Because gonads in some individuals carrying the W allele initially develop oocytes that undergo apoptosis like gonads in AB and TU strains, and because oocytes do not develop in fish that lack the W chromosome, we suspect that these laboratory strains, which were cleaned of background lethal mutations by either gynogenesis or repeated inbreeding (Walker-Durchanek, 1980; Streisinger et al., 1981; Chakrabarti et al., 1983; Mullins et al., 1994), are likely chromosomally WW.
Direct testis development in ZZ NA fish raises the question of whether they transit a transcriptomic state shared with developing gonads in ZW genetic females or alternatively, if gonads in the two sex genotypes are transcriptionally different even at 19dpf when they are morphologically indistinguishable. Single-cell RNA-seq showed that at 19dpf, all clusters consisted of an intermixture of ZW and ZZ cells, but by 30dpf, sex-genotype-specific clusters had developed. The intermingling of ZW and ZZ cells in 19dpf clusters shows that at this stage, ZW and ZZ gonads in NA fish were not only morphologically similar, but also had not differentiated sex-specific cell types according to gene expression patterns in our experiments.
Despite the general bipotential nature of 19dpf NA ZW and ZZ gonads, germ cells in 19dpf ZW gonads were already expressing low levels of oocyte-specific genes, for example, eggshell (zp) genes, that ZZ germ cells did not express. This result suggests that ovary-specific functions had already begun in ZW, but not ZZ, fish by 19dpf. Whether those differences arise from primary differences in germ cells or subtle differences in support cells, steroidogenic cells, or stromal/interstitial cells is not yet known. The finding that 30dpf ZZ germ cells clustered with 19dpf ZZ germ cells, and that ZZ germ cells did not express spermatogenesis-specific genes, showed that ZZ germ cells generally postponed development until after 30dpf, in contrast to ZW germ cells, many of which showed oocyte-specific expression by 30dpf.
Like germ cells, ZW and ZZ support cells had very similar transcriptomes at 19dpf, but by 30dpf, ZW and ZZ support cells had differentiated into granulosa cells and Sertoli cells, respectively. The differentiated nature of the 30dpf ZZ Sertoli cells vs. the relatively undifferentiated state of the 30dpf ZZ germ cells suggests that in ZZ fish, support cell differentiation preceded germ cell differentiation.
Analysis of steroidogenic cells showed that genes encoding enzymes that catalyze the last two steps in estrogen biosynthesis are expressed in three different cell clusters: theca cells in 1930c12 expressing cyp17a1 can produce androstenedione, which follicle cells in 1930c18 expressing cyp19a1a can convert to estrone, which granulosa cells in 1930c21 expressing hsd17b1 can convert to estradiol. Whether the cyp19a1a-expressing cells and the hsd17b1-expressing cells are different states of the same cells, or alternatively, are totally different cells is unclear. The failure to find significant expression of the testosterone-synthesizing enzyme gene hsd17b3 in 30dpf NA gonads, mimicking findings with 40dpf AB ovaries (Liu et al., 2022), and the lack of sex tubercles at 30dpf (Dai et al., 2021b) raises the question of how or whether gonads at these stages produce testosterone.
Analysis of histological sections supported the notion that, in the four relatively natural zebrafish strains that had a ZW/ZZ sar-4 chromosomal sex determination mechanism (Wilson et al., 2014), the W chromosome has a locus necessary, but not sufficient, for gonads to initiate oocyte development. An alternative hypothesis is that the Z chromosome has a locus that is required in two doses to prevent oocyte development. The location of the genetic sex determinant at the telomere and the enormous number of repetitive elements in the region led to a poor quality genome assembly in this region, which also lacks any of the ‘usual suspects’ for fish sex determination genes (e.g., Sox family, Dmrt1 variants, TGF-beta pathways) (Herpin and Schartl, 2015; Bertho et al., 2016). High quality PacBio genomic sequences from ZZ and WW individuals and detailed genetic analyses promise to lead to the molecular genetic identification of this elusive element.
The NA wild-type strain (NA, ZFIN ID: ZDB-GENO-030115-2), originating from the Nadia region of India, has been raised at the University of Oregon zebrafish facility since 1999, and has been selected for maintenance of the Z chromosome since about 2012. For developmental histology, juveniles of one NA family were raised in embryo medium E2 (Westerfield, 1993) and fed paramecia from 4–10 days post-fertilization (dpf) and other families were raised in E5 and fed rotifers from 4 to 10dpf (Best et al., 2010), but results from both were comparable and were combined. After 10dpf, fish were moved to a circulating fish water system (Westerfield, 1993) and fed Zeigler Adult Zebrafish Diet. Procedures were approved by the University of Oregon IACUC protocol #18-13.
Animals were genotyped for Z and W chromosomes with PCR primers designed from RAD-tag sequences that contained a sex-linked marker from the published NA dataset (Fig. S1), using either the original published primer set (Wilson et al., 2014) or an improved primer set (F: CCGCGTTTATATCCTGGTAA and R: GTTGACCCAACTGGACTCTG), which amplified a 119-nucleotide (nt) fragment from NA genomic DNA. PCR conditions were: 6m 94°C; 45 cycles of 25s 94°C, 25s 61°C, 30s 72°C; followed by 10m at 72°C. The amplicon was digested with the restriction enzyme CviQI according to manufacturer’s instructions (New England Biolabs). The enzyme digested the 119 nt amplicon from the Z allele to produce fragments of 43 and 76 nt, but left the W allele uncut.
Fish were euthanized in ice water and the caudal fin was removed for genotyping. Fish trunks from the gills to the urogenital pore were fixed in Bouin’s fixative (Sigma) for at least 48 h, then washed repeatedly with 70% ethanol. Fixed tissues were embedded in paraffin, sectioned at 7 microns, and stained with hematoxylin and eosin.
Zebrafish were fin-clipped, genotyped for genetic sex, allowed to recover on the water system for a few days, and at 19 and 30 dpf, they were euthanized in ice water and gonads were dissected in chilled phosphate buffered saline (PBS) as described (Wang et al., 2017). Gonads from five fish per genotype were pooled in a well of a 24-well tissue culture plate and dissociated using a protocol adapted from (Covassin et al., 2006a). PBS was replaced with 1.2 mL of a solution containing 0.05% trypsin and 0.02% EDTA. Samples were incubated for 10 min at 28.5°C, pipetting up and down every 2 minutes with a 1000 µl pipette tip to manually break up tissues. Adding 200 µl of a solution containing 30% Fetal Bovine Serum and 6 mM CaCl2 stopped digestion. Cells were transferred to a 1.5 mL microfuge tube and spun for 5 min at 2000 RPM (∼300 RCF) at 4°C. The supernatant was removed, and cells were resuspended in a solution containing 1% fetal bovine serum, 0.8 mM CaCl2, and 1x antibiotic/antimycotic (Sigma #A5955) in L-15 medium. Cells were spun a second time for 5 min at 2,000 RPM at 4°C. The supernatant was removed, and cells were resuspended in the suspension solution, then filtered through a 40-micron strainer (Biologix #15-1040) to generate a suspension of single-cells. After microscopic visualization to check for dissociation, cells were counted with a Bio-RAD TC20 Automated Cell Counter and given to the University of Oregon Genomics and Cell Characterization Core Facility for library construction and sequencing. Libraries were prepared with Chromium v2 chemistry (10x Genomics) targeting 10,000 cells. According to manufacturer’s instructions. Libraries were sequenced on an Illumina NextSeq 500.
Sequences were aligned to the zebrafish genome GRCZ11 using the Lawson zebrafish transcriptome annotation (Lawson et al., 2020) with Cell Ranger v. 6.1.1 (Zheng et al., 2017). Ambient RNA was removed with SoupX v 1.6.2 (Young and Behjati, 2020). For 19dpf individuals, ambient RNA derived from pancreas and erythrocytes, so we used the genes “hbaa1”, “cpa5,” “cpa1,” “cel.2,” “cel.1,” “amy2a,” “hbba1,” “ela2l,” “ela2,” “prss1,” “hbae5,” and “prsS69.2” to estimate the contamination fraction. In 30dpf individuals, ambient RNA was derived from both erythrocytes and fragile oocytes, so we used the following genes to estimate the contamination fraction: “hbaa1,” “hbaa2,” “hbba2,” “hbba1,” “hbae5,” “ddx4,” “dnd1,” “sycp1,” “sycp3,” and “dmc1.” Data were analyzed with Seurat v. 4.2.0 (Butler et al., 2018). Non-gonad clusters, including contaminating pancreatic cells from dissection errors and erythrocytes, were excluded and gonad cells were reclustered. Differential expression analysis was performed on 19dpf clusters comparing ZZ vs. ZW genotypes using the Seurat “FindMarkers” function with the parameters min.pct = 0.05, logfc.threshold = 0.1, test.use = “MAST” (Finak et al., 2015).
Our manuscript “Direct Male Development in Chromosomally ZZ Zebrafish” by Wilson, Bazel, and Postlethwait is submitted to Frontiers in Cell and Developmental Biology for the special volume entitled: Proceedings of the ninth International Symposium on the Biology of Vertebrate Sex Determination 2023. Our work uses histology and single-cell transcriptomics to probe the mechanisms of gonadal development in a zebrafish strain that maintains the chromosomal sex determination system found in the wild rather than in domesticated laboratory strains that lost the natural mechanism. Results show that gonads in chromosomally ZZ individuals develop directly into testes and avoid the juvenile ovary state found in gonads of all individuals in domesticated strains. Our scRNA-seq experiments revealed that gene expression in gonads at the histologically indifferent stage are highly similar in ZZ (all male) and ZW (mostly female) individuals. Eleven days later, however, when testes and ovaries are distinct morphologically, many transcriptional cell types are ZZ- or ZW-specific. This is the first work to identify genes that distinguish gonadal cell types at these early developmental states and the first to study gonad development in a zebrafish strain with the wild genetic sex determination system. This work is in the journal scope because it investigates “molecular and cellular reproduction” and “morphogenesis and patterning.”
The datasets presented in this study can be found in online repositories. The names of the repository/repositories and accession number(s) can be found in the article/Supplementary Material.
The animal study was approved by the Institutional Animal Care and Use Committee, University of Oregon. The study was conducted in accordance with the local legislation and institutional requirements.
CW: Conceptualization, Formal Analysis, Investigation, Writing–original draft, Writing–review and editing, Data curation, Software. PB: Conceptualization, Data curation, Formal Analysis, Investigation, Software, Writing–review and editing. JP: Conceptualization, Formal Analysis, Investigation, Writing–review and editing, Funding acquisition, Project administration, Supervision, Writing–original draft.
The author(s) declare financial support was received for the research, authorship, and/or publication of this article. This work was funded by National Institutes of Health grant R35 GM139635.
This work benefited from access to the University of Oregon high performance computing cluster, Talapas, and the Zebrafish Information Network [(ZFIN) (RRID:SCR_002560)] and ENSEMBL Ensembl (RRID:SCR_002344) databases (Bradford et al., 2022; Martin et al., 2023).
We thank Ruth Bremiller (deceased), who published her first scientific paper in 1951 (White and Allen, 1951) and her 42d paper 71 years later in 2022 (Petersen et al., 2022), and Poh Kheng Loi for help with histology, and the University of Oregon’s Genomics and Cell Characterization Core Facility (GC3F) for library preparation and sequencing.
The authors declare that the research was conducted in the absence of any commercial or financial relationships that could be construed as a potential conflict of interest.
All claims expressed in this article are solely those of the authors and do not necessarily represent those of their affiliated organizations, or those of the publisher, the editors and the reviewers. Any product that may be evaluated in this article, or claim that may be made by its manufacturer, is not guaranteed or endorsed by the publisher.
The Supplementary Material for this article can be found online at: https://www.frontiersin.org/articles/10.3389/fcell.2024.1362228/full#supplementary-material
SUPPLEMENTARY TABLE S1 | Cell counts per genotype per cluster for the 19dpf single-cell dataset.
SUPPLEMENTARY TABLE S2 | Cell type marker genes for 19dpf ZW and ZZ gonads.
SUPPLEMENTARY TABLE S3 | Gene expression markers for 19dpf clusters. We required genes to be expressed in a minimum of 10% of cells and a minimum log fold change of 0.25.
SUPPLEMENTARY TABLE S4 | Differential expression analysis comparing ZZ cells to ZW cells for each cluster at 19dpf.
SUPPLEMENTARY TABLE S5 | Cell counts per genotype per cluster for the 30dpf single-cell dataset.
SUPPLEMENTARY TABLE S6 | Gene expression markers for 30dpf clusters. We required genes to be expressed in a minimum of 10% of cells and a minimum log fold change of 0.25.
SUPPLEMENTARY TABLE S7 | Cell counts per age per genotype per cluster for the combined 19dpf and 30dpf single-cell dataset.
SUPPLEMENTARY TABLE S8 | Gene expression markers for combined 19dpf and 30dpf clusters. We required genes to be expressed in a minimum of 10% of cells and a minimum log fold change of 0.25.
SUPPLEMENTARY METHOD S1 | R scripts utilizing Seurat to analyze 19dpf single-cell data.
SUPPLEMENTARY METHOD S2 | R scripts utilizing Seurat to analyze 30dpf data.
SUPPLEMENTARY METHOD S3 | R scripts utilizing Seurat to analyze combined single-cell data.
SUPPLEMENTARY METHOD S4 | R scripts utilizing the SoupX package to remove ambient RNA.
Abozaid, H., Wessels, S., and Horstgen-Schwark, G. (2011). Effect of rearing temperatures during embryonic development on the phenotypic sex in zebrafish (Danio rerio). Sex. Dev. 5, 259–265. doi:10.1159/000330120
Aharon, D., and Marlow, F. L. (2021). Sexual determination in zebrafish. Cell Mol. Life Sci. 79, 8. doi:10.1007/s00018-021-04066-4
Amores, A., Force, A., Yan, Y. L., Joly, L., Amemiya, C., Fritz, A., et al. (1998). Zebrafish hox clusters and vertebrate genome evolution. Science 282, 1711–1714. doi:10.1126/science.282.5394.1711
Anderson, J. L., Rodriguez Mari, A., Braasch, I., Amores, A., Hohenlohe, P., Batzel, P., et al. (2012). Multiple sex-associated regions and a putative sex chromosome in zebrafish revealed by RAD mapping and population genomics. PLoS One 7, e40701. doi:10.1371/journal.pone.0040701
Aranguren, X. L., Beerens, M., Coppiello, G., Wiese, C., Vandersmissen, I., Lo Nigro, A., et al. (2013). COUP-TFII orchestrates venous and lymphatic endothelial identity by homo- or hetero-dimerisation with PROX1. J. Cell Sci. 126, 1164–1175. doi:10.1242/jcs.116293
Archambeault, D. R., and Yao, H. H. (2010). Activin A, a product of fetal Leydig cells, is a unique paracrine regulator of Sertoli cell proliferation and fetal testis cord expansion. Proc. Natl. Acad. Sci. U. S. A. 107, 10526–10531. doi:10.1073/pnas.1000318107
Bahary, N., Goishi, K., Stuckenholz, C., Weber, G., Leblanc, J., Schafer, C. A., et al. (2007). Duplicate VegfA genes and orthologues of the KDR receptor tyrosine kinase family mediate vascular development in the zebrafish. Blood 110, 3627–3636. doi:10.1182/blood-2006-04-016378
Barlow, J. L., Bellosi, A., Hardman, C. S., Drynan, L. F., Wong, S. H., Cruickshank, J. P., et al. (2012). Innate IL-13-producing nuocytes arise during allergic lung inflammation and contribute to airways hyperreactivity. J. Allergy Clin. Immunol. 129, 191–198. doi:10.1016/j.jaci.2011.09.041
Barrionuevo, F., Bagheri-Fam, S., Klattig, J., Kist, R., Taketo, M. M., Englert, C., et al. (2006). Homozygous inactivation of Sox9 causes complete XY sex reversal in mice. Biol. Reprod. 74, 195–201. doi:10.1095/biolreprod.105.045930
Basa, M., Vukovic, R., Sarajlija, A., Milenkovic, T., Djordjevic, M., Vucetic, B., et al. (2021). Ambiguous genitalia and lissencephaly in A 46,XY neonate with a novel variant of aristaless gene. Acta Endocrinol. (Buchar) 17, 402–405. doi:10.4183/aeb.2021.402
Bauer, M. P., Bridgham, J. T., Langenau, D. M., Johnson, A. L., and Goetz, F. W. (2000). Conservation of steroidogenic acute regulatory (StAR) protein structure and expression in vertebrates. Mol. Cell Endocrinol. 168, 119–125. doi:10.1016/s0303-7207(00)00316-6
Beer, R. L., and Draper, B. W. (2013). nanos3 maintains germline stem cells and expression of the conserved germline stem cell gene nanos2 in the zebrafish ovary. Dev. Biol. 374, 308–318. doi:10.1016/j.ydbio.2012.12.003
Bertho, S., Clapp, M., Banisch, T. U., Bandemer, J., Raz, E., and Marlow, F. L. (2021). Zebrafish dazl regulates cystogenesis and germline stem cell specification during the primordial germ cell to germline stem cell transition. Development 148, dev187773. doi:10.1242/dev.187773
Bertho, S., Pasquier, J., Pan, Q., Le Trionnaire, G., Bobe, J., Postlethwait, J. H., et al. (2016). Foxl2 and its relatives are evolutionary conserved players in gonadal sex differentiation. Sex. Dev. 10, 111–129. doi:10.1159/000447611
Best, J., Adatto, I., Cockington, J., James, A., and Lawrence, C. (2010). A novel method for rearing first-feeding larval zebrafish: polyculture with Type L saltwater rotifers (Brachionus plicatilis). Zebrafish 7, 289–295. doi:10.1089/zeb.2010.0667
Bezman, N. A., Kim, C. C., Sun, J. C., Min-Oo, G., Hendricks, D. W., Kamimura, Y., et al. (2012). Molecular definition of the identity and activation of natural killer cells. Nat. Immunol. 13, 1000–1009. doi:10.1038/ni.2395
Blackburn, J. S., Liu, S., Wilder, J. L., Dobrinski, K. P., Lobbardi, R., Moore, F. E., et al. (2014). Clonal evolution enhances leukemia-propagating cell frequency in T cell acute lymphoblastic leukemia through Akt/mTORC1 pathway activation. Cancer Cell 25, 366–378. doi:10.1016/j.ccr.2014.01.032
Bleul, C. C., Fuhlbrigge, R. C., Casasnovas, J. M., Aiuti, A., and Springer, T. A. (1996). A highly efficacious lymphocyte chemoattractant, stromal cell-derived factor 1 (SDF-1). J. Exp. Med. 184, 1101–1109. doi:10.1084/jem.184.3.1101
Blokhina, Y. P., Frees, M. A., Nguyen, A., Sharifi, M., Chu, D. B., Bispo, K., et al. (2021). Rad21l1 cohesin subunit is dispensable for spermatogenesis but not oogenesis in zebrafish. PLoS Genet. 17, e1009127. doi:10.1371/journal.pgen.1009127
Blokhina, Y. P., Nguyen, A. D., Draper, B. W., and Burgess, S. M. (2019). The telomere bouquet is a hub where meiotic double-strand breaks, synapsis, and stable homolog juxtaposition are coordinated in the zebrafish, Danio rerio. PLoS Genet. 15, e1007730. doi:10.1371/journal.pgen.1007730
Bogoch, Y., Jamieson-Lucy, A., Vejnar, C. E., Levy, K., Giraldez, A. J., Mullins, M. C., et al. (2022). Stage specific transcriptomic analysis and database for zebrafish oogenesis. Front. Cell Dev. Biol. 10, 826892. doi:10.3389/fcell.2022.826892
Bottiglione, F., Dee, C. T., Lea, R., Zeef, L. A. H., Badrock, A. P., Wane, M., et al. (2020). Zebrafish IL-4-like cytokines and IL-10 suppress inflammation but only IL-10 is essential for gill homeostasis. J. Immunol. 205, 994–1008. doi:10.4049/jimmunol.2000372
Bradford, Y. M., Toro, S., Ramachandran, S., Ruzicka, L., Howe, D. G., Eagle, A., et al. (2017). Zebrafish models of human disease: gaining insight into human disease at ZFIN. ILAR J. 58, 4–16. doi:10.1093/ilar/ilw040
Bradford, Y. M., Van Slyke, C. E., Ruzicka, L., Singer, A., Eagle, A., Fashena, D., et al. (2022). Zebrafish information network, the knowledgebase for Danio rerio research. Genetics 220, iyac016. doi:10.1093/genetics/iyac016
Bradley, K. M., Breyer, J. P., Melville, D. B., Broman, K. W., Knapik, E. W., and Smith, J. R. (2011). An SNP-based linkage map for zebrafish reveals sex determination loci. G3 (Bethesda) 1, 3–9. doi:10.1534/g3.111.000190
Bravo, P., Liu, Y., Draper, B. W., and Marlow, F. L. (2023). Macrophage activation drives ovarian failure and masculinization. bioRxiv, 2023.01.03.522645. doi:10.1101/2023.01.03.522645
Brennan, J., Karl, J., and Capel, B. (2002). Divergent vascular mechanisms downstream of Sry establish the arterial system in the XY gonad. Dev. Biol. 244, 418–428. doi:10.1006/dbio.2002.0578
Bukrinsky, A., Griffin, K. J., Zhao, Y., Lin, S., and Banerjee, U. (2009). Essential role of spi-1-like (spi-1l) in zebrafish myeloid cell differentiation. Blood 113, 2038–2046. doi:10.1182/blood-2008-06-162495
Butler, A., Hoffman, P., Smibert, P., Papalexi, E., and Satija, R. (2018). Integrating single-cell transcriptomic data across different conditions, technologies, and species. Nat. Biotechnol. 36, 411–420. doi:10.1038/nbt.4096
Cabrera-Quio, L. E., Schleiffer, A., Mechtler, K., and Pauli, A. (2021). Zebrafish Ski7 tunes RNA levels during the oocyte-to-embryo transition. PLoS Genet. 17, e1009390. doi:10.1371/journal.pgen.1009390
Calvanese, V., Capellera-Garcia, S., Ma, F., Fares, I., Liebscher, S., Ng, E. S., et al. (2022). Mapping human haematopoietic stem cells from haemogenic endothelium to birth. Nature 604, 534–540. doi:10.1038/s41586-022-04571-x
Can, H., Chanumolu, S. K., Gonzalez-Munoz, E., Prukudom, S., Otu, H. H., and Cibelli, J. B. (2020). Comparative analysis of single-cell transcriptomics in human and Zebrafish oocytes. BMC Genomics 21, 471. doi:10.1186/s12864-020-06860-z
Carmona, S. J., Teichmann, S. A., Ferreira, L., Macaulay, I. C., Stubbington, M. J., Cvejic, A., et al. (2017). Single-cell transcriptome analysis of fish immune cells provides insight into the evolution of vertebrate immune cell types. Genome Res. 27, 451–461. doi:10.1101/gr.207704.116
Caulier, M., Brion, F., Chadili, E., Turies, C., Piccini, B., Porcher, J. M., et al. (2015). Localization of steroidogenic enzymes and Foxl2a in the gonads of mature zebrafish (Danio rerio). Comp. Biochem. Physiol. A Mol. Integr. Physiol. 188, 96–106. doi:10.1016/j.cbpa.2015.06.016
Chakrabarti, S., Streisinger, G., Singer, F., and Walker, C. (1983). Frequency of gamma-ray induced specific locus and recessive lethal mutations in mature germ cells of the zebrafish, Brachydanio rerio. Genetics 103, 109–123. doi:10.1093/genetics/103.1.109
Chen, W., Tseng, X., Lin, G., Schreiner, A., Chen, H., Voigt, M. M., et al. (2013). The ortholog of LYVE-1 is required for thoracic duct formation in zebrafish. CellBio 2, 228–247. doi:10.4236/cellbio.2013.24026
Chen, W., Zhai, Y., Zhu, B., Wu, K., Fan, Y., Zhou, X., et al. (2022). Loss of growth differentiation factor 9 causes an arrest of early folliculogenesis in zebrafish – a novel insight into its action mechanism. BioRxiv. doi:10.1101/2022.07.01.498398
Chiang, E. F., Pai, C. I., Wyatt, M., Yan, Y. L., Postlethwait, J., and Chung, B. (2001a). Two sox9 genes on duplicated zebrafish chromosomes: expression of similar transcription activators in distinct sites. Dev. Biol. 231, 149–163. doi:10.1006/dbio.2000.0129
Chiang, E. F., Yan, Y. L., Guiguen, Y., Postlethwait, J., and Chung, B. (2001b). Two Cyp19 (P450 aromatase) genes on duplicated zebrafish chromosomes are expressed in ovary or brain. Mol. Biol. Evol. 18, 542–550. doi:10.1093/oxfordjournals.molbev.a003833
Chiang, E. F., Yan, Y. L., Tong, S. K., Hsiao, P. H., Guiguen, Y., Postlethwait, J., et al. (2001c). Characterization of duplicated zebrafish cyp19 genes. J. Exp. Zool. 290, 709–714. doi:10.1002/jez.1121
Ciruna, B., Weidinger, G., Knaut, H., Thisse, B., Thisse, C., Raz, E., et al. (2002). Production of maternal-zygotic mutant zebrafish by germ-line replacement. Proc. Natl. Acad. Sci. U. S. A. 99, 14919–14924. doi:10.1073/pnas.222459999
Cobaleda, C., Schebesta, A., Delogu, A., and Busslinger, M. (2007). Pax5: the guardian of B cell identity and function. Nat. Immunol. 8, 463–470. doi:10.1038/ni1454
Covassin, L. D., Villefranc, J. A., Kacergis, M. C., Weinstein, B. M., and Lawson, N. D. (2006b). Distinct genetic interactions between multiple Vegf receptors are required for development of different blood vessel types in zebrafish. Proc. Natl. Acad. Sci. U. S. A. 103, 6554–6559. doi:10.1073/pnas.0506886103
Covassin, L., Amigo, J. D., Suzuki, K., Teplyuk, V., Straubhaar, J., and Lawson, N. D. (2006a). Global analysis of hematopoietic and vascular endothelial gene expression by tissue specific microarray profiling in zebrafish. Dev. Biol. 299, 551–562. doi:10.1016/j.ydbio.2006.08.020
Crespo, B., Lan-Chow-Wing, O., Rocha, A., Zanuy, S., and Gomez, A. (2013). foxl2 and foxl3 are two ancient paralogs that remain fully functional in teleosts. Gen. Comp. Endocrinol. 194, 81–93. doi:10.1016/j.ygcen.2013.08.016
Crowder, C. M., Lassiter, C. S., and Gorelick, D. A. (2018). Nuclear androgen receptor regulates testes organization and oocyte maturation in zebrafish. Endocrinology 159, 980–993. doi:10.1210/en.2017-00617
Cui, X., Lu, Y. W., Lee, V., Kim, D., Dorsey, T., Wang, Q., et al. (2015). Venous endothelial marker COUP-tfii regulates the distinct pathologic potentials of adult arteries and veins. Sci. Rep. 5, 16193. doi:10.1038/srep16193
Cui, Z., Liu, Y., Wang, W., Wang, Q., Zhang, N., Lin, F., et al. (2017). Genome editing reveals dmrt1 as an essential male sex-determining gene in Chinese tongue sole (Cynoglossus semilaevis). Sci. Rep. 7, 42213. doi:10.1038/srep42213
Dai, S., Qi, S., Wei, X., Liu, X., Li, Y., Zhou, X., et al. (2021a). Germline sexual fate is determined by the antagonistic action of dmrt1 and foxl3/foxl2 in tilapia. Development 148, dev199380. doi:10.1242/dev.199380
Dai, X., Pu, D., Wang, L., Cheng, X., Liu, X., Yin, Z., et al. (2021b). Emergence of breeding tubercles and puberty onset in male zebrafish: evidence for a dependence on body growth. J. Fish. Biol. 99, 1071–1078. doi:10.1111/jfb.14811
Davis, R. B., Curtis, C. D., and Griffin, C. T. (2013). BRG1 promotes COUP-TFII expression and venous specification during embryonic vascular development. Development 140, 1272–1281. doi:10.1242/dev.087379
Defalco, T., Potter, S. J., Williams, A. V., Waller, B., Kan, M. J., and Capel, B. (2015). Macrophages contribute to the spermatogonial niche in the adult testis. Cell Rep. 12, 1107–1119. doi:10.1016/j.celrep.2015.07.015
Delomas, T. A., and Dabrowski, K. (2018). Effects of homozygosity on sex determination in zebrafish Danio rerio. J. Fish. Biol. 93, 1178–1187. doi:10.1111/jfb.13836
Devlin, R. H., and Nagahama, Y. (2002). Sex determination and sex differentiation in fish: an overview of genetic, physiological, and environmental influences. Aquaculture 208, 191–364. doi:10.1016/s0044-8486(02)00057-1
De Waal, P. P., Leal, M. C., Garcia-Lopez, A., Liarte, S., De Jonge, H., Hinfray, N., et al. (2009). Oestrogen-induced androgen insufficiency results in a reduction of proliferation and differentiation of spermatogonia in the zebrafish testis. J. Endocrinol. 202, 287–297. doi:10.1677/JOE-09-0050
De Waal, P. P., Wang, D. S., Nijenhuis, W. A., Schulz, R. W., and Bogerd, J. (2008). Functional characterization and expression analysis of the androgen receptor in zebrafish (Danio rerio) testis. Reproduction 136, 225–234. doi:10.1530/REP-08-0055
Dong, J., Albertini, D. F., Nishimori, K., Kumar, T. R., Lu, N., and Matzuk, M. M. (1996). Growth differentiation factor-9 is required during early ovarian folliculogenesis. Nature 383, 531–535. doi:10.1038/383531a0
Dranow, D. B., Hu, K., Bird, A. M., Lawry, S. T., Adams, M. T., Sanchez, A., et al. (2016). Bmp15 is an oocyte-produced signal required for maintenance of the adult female sexual phenotype in zebrafish. PLoS Genet. 12, e1006323. doi:10.1371/journal.pgen.1006323
Dranow, D. B., Tucker, R. P., and Draper, B. W. (2013). Germ cells are required to maintain a stable sexual phenotype in adult zebrafish. Dev. Biol. 376, 43–50. doi:10.1016/j.ydbio.2013.01.016
Fan, X., Bialecka, M., Moustakas, I., Lam, E., Torrens-Juaneda, V., Borggreven, N. V., et al. (2019). Single-cell reconstruction of follicular remodeling in the human adult ovary. Nat. Commun. 10, 3164. doi:10.1038/s41467-019-11036-9
Farnsworth, D. R., Saunders, L. M., and Miller, A. C. (2019). A single-cell transcriptome atlas for zebrafish development. Dev. Biol. 459, 100–108. doi:10.1016/j.ydbio.2019.11.008
Feitsma, H., Leal, M. C., Moens, P. B., Cuppen, E., and Schulz, R. W. (2007). Mlh1 deficiency in zebrafish results in male sterility and aneuploid as well as triploid progeny in females. Genetics 175, 1561–1569. doi:10.1534/genetics.106.068171
Field, S. L., Dasgupta, T., Cummings, M., and Orsi, N. M. (2014). Cytokines in ovarian folliculogenesis, oocyte maturation and luteinisation. Mol. Reprod. Dev. 81, 284–314. doi:10.1002/mrd.22285
Finak, G., Mcdavid, A., Yajima, M., Deng, J., Gersuk, V., Shalek, A. K., et al. (2015). MAST: a flexible statistical framework for assessing transcriptional changes and characterizing heterogeneity in single-cell RNA sequencing data. Genome Biol. 16, 278. doi:10.1186/s13059-015-0844-5
Francois, M., Caprini, A., Hosking, B., Orsenigo, F., Wilhelm, D., Browne, C., et al. (2008). Sox18 induces development of the lymphatic vasculature in mice. Nature 456, 643–647. doi:10.1038/nature07391
Fu, X. F., Cheng, S. F., Wang, L. Q., Yin, S., De Felici, M., and Shen, W. (2015). DAZ family proteins, key players for germ cell development. Int. J. Biol. Sci. 11, 1226–1235. doi:10.7150/ijbs.11536
Garcia-Alonso, L., Lorenzi, V., Mazzeo, C. I., Alves-Lopes, J. P., Roberts, K., Sancho-Serra, C., et al. (2022). Single-cell roadmap of human gonadal development. Nature 607, 540–547. doi:10.1038/s41586-022-04918-4
Gautier, A., Sohm, F., Joly, J. S., Le Gac, F., and Lareyre, J. J. (2011). The proximal promoter region of the zebrafish gsdf gene is sufficient to mimic the spatio-temporal expression pattern of the endogenous gene in Sertoli and granulosa cells. Biol. Reprod. 85, 1240–1251. doi:10.1095/biolreprod.111.091892
Gilchrist, R. B., Lane, M., and Thompson, J. G. (2008). Oocyte-secreted factors: regulators of cumulus cell function and oocyte quality. Hum. Reprod. Update 14, 159–177. doi:10.1093/humupd/dmm040
Ginsburger-Vogel, T. (1972). Inversion desfemelles d'Orchestiagammarella Pallqs (Crustacés Amphipodes Talitridaé) en néo-mâles fonctionnelspar greffe de glandes androgènes avant la mue de première différenciation externe du sexe. C R. Acad. Hebd. Seances Acad. Sci. D. 274, 3606–3609.
Goldstone, J. V., Mcarthur, A. G., Kubota, A., Zanette, J., Parente, T., Jonsson, M. E., et al. (2010). Identification and developmental expression of the full complement of Cytochrome P450 genes in Zebrafish. BMC Genomics 11, 643. doi:10.1186/1471-2164-11-643
Gomez, G. A., Veldman, M. B., Zhao, Y., Burgess, S., and Lin, S. (2009). Discovery and characterization of novel vascular and hematopoietic genes downstream of etsrp in zebrafish. PLoS One 4, e4994. doi:10.1371/journal.pone.0004994
Grompe, M., and D'Andrea, A. (2001). Fanconi anemia and DNA repair. Hum. Mol. Genet. 10, 2253–2259. doi:10.1093/hmg/10.20.2253
Guo, Y., Cheng, H., Huang, X., Gao, S., Yu, H., and Zhou, R. (2005). Gene structure, multiple alternative splicing, and expression in gonads of zebrafish Dmrt1. Biochem. Biophys. Res. Commun. 330, 950–957. doi:10.1016/j.bbrc.2005.03.066
Gupta, B., Ramteke, P., Paul, V. K., Kumar, T., and Das, P. (2019). Ambiguous genitalia associated with an extremely rare syndrome: a case report of xlag syndrome and review of the literature. Turk Patoloji Derg. 35, 162–165. doi:10.5146/tjpath.2017.01391
Gurung, S., Restrepo, N. K., Chestnut, B., Klimkaite, L., and Sumanas, S. (2022). Single-cell transcriptomic analysis of vascular endothelial cells in zebrafish embryos. Sci. Rep. 12, 13065. doi:10.1038/s41598-022-17127-w
Haire, R. N., Cannon, J. P., O'Driscoll, M. L., Ostrov, D. A., Mueller, M. G., Turner, P. M., et al. (2012). Genomic and functional characterization of the diverse immunoglobulin domain-containing protein (DICP) family. Genomics 99, 282–291. doi:10.1016/j.ygeno.2012.02.004
Hamer, G., Roepers-Gajadien, H. L., Van Duyn-Goedhart, A., Gademan, I. S., Kal, H. B., Van Buul, P. P., et al. (2003). DNA double-strand breaks and gamma-H2AX signaling in the testis. Biol. Reprod. 68, 628–634. doi:10.1095/biolreprod.102.008672
Hanley, N. A., Ball, S. G., Clement-Jones, M., Hagan, D. M., Strachan, T., Lindsay, S., et al. (1999). Expression of steroidogenic factor 1 and Wilms' tumour 1 during early human gonadal development and sex determination. Mech. Dev. 87, 175–180. doi:10.1016/s0925-4773(99)00123-9
Hartung, O., Forbes, M. M., and Marlow, F. L. (2014). Zebrafish vasa is required for germ-cell differentiation and maintenance. Mol. Reprod. Dev. 81, 946–961. doi:10.1002/mrd.22414
Hattori, R. S., Murai, Y., Oura, M., Masuda, S., Majhi, S. K., Sakamoto, T., et al. (2012). A Y-linked anti-Mullerian hormone duplication takes over a critical role in sex determination. Proc. Natl. Acad. Sci. U. S. A. 109, 2955–2959. doi:10.1073/pnas.1018392109
Herpin, A., Braasch, I., Kraeussling, M., Schmidt, C., Thoma, E. C., Nakamura, S., et al. (2010). Transcriptional rewiring of the sex determining dmrt1 gene duplicate by transposable elements. PLoS Genet. 6, e1000844. doi:10.1371/journal.pgen.1000844
Herpin, A., and Schartl, M. (2015). Plasticity of gene-regulatory networks controlling sex determination: of masters, slaves, usual suspects, newcomers, and usurpators. EMBO Rep. 16, 1260–1274. doi:10.15252/embr.201540667
Herpin, A., Schartl, M., Depince, A., Guiguen, Y., Bobe, J., Hua-Van, A., et al. (2021). Allelic diversification after transposable element exaptation promoted gsdf as the master sex determining gene of sablefish. Genome Res. 31, 1366–1380. doi:10.1101/gr.274266.120
Hiramatsu, N., Luo, W., Reading, B. J., Sullivan, C. V., Mizuta, H., Ryu, Y. W., et al. (2013). Multiple ovarian lipoprotein receptors in teleosts. Fish Physiology Biochem. 39, 29–32. doi:10.1007/s10695-012-9612-6
Hong, N., Li, M., Yuan, Y., Wang, T., Yi, M., Xu, H., et al. (2016). Dnd is a critical specifier of primordial germ cells in the medaka fish. Stem Cell Rep. 6, 411–421. doi:10.1016/j.stemcr.2016.01.002
Houwing, S., Berezikov, E., and Ketting, R. F. (2008). Zili is required for germ cell differentiation and meiosis in zebrafish. EMBO J. 27, 2702–2711. doi:10.1038/emboj.2008.204
Howe, K., Clark, M. D., Torroja, C. F., Torrance, J., Berthelot, C., Muffato, M., et al. (2013). The zebrafish reference genome sequence and its relationship to the human genome. Nature 496, 498–503. doi:10.1038/nature12111
Howley, C., and Ho, R. K. (2000). mRNA localization patterns in zebrafish oocytes. Mech. Dev. 92, 305–309. doi:10.1016/s0925-4773(00)00247-1
Hsu, C. W., and Chung, B. C. (2021). Evolution, expression, and function of gonadal somatic cell-derived factor. Front. Cell Dev. Biol. 9, 684352. doi:10.3389/fcell.2021.684352
Hsu, H. J., Hsiao, P., Kuo, M. W., and Chung, B. C. (2002). Expression of zebrafish cyp11a1 as a maternal transcript and in yolk syncytial layer. Gene Expr. Patterns 2, 219–222. doi:10.1016/s1567-133x(02)00059-5
Hu, M. C., Chiang, E. F., Tong, S. K., Lai, W., Hsu, N. C., Wang, L. C., et al. (2001). Regulation of steroidogenesis in transgenic mice and zebrafish. Mol. Cell Endocrinol. 171, 9–14. doi:10.1016/s0303-7207(00)00385-3
Hui, S. P., Sheng, D. Z., Sugimoto, K., Gonzalez-Rajal, A., Nakagawa, S., Hesselson, D., et al. (2017). Zebrafish regulatory T cells mediate organ-specific regenerative programs. Dev. Cell 43, 659–672. doi:10.1016/j.devcel.2017.11.010
Hunter, N. (2015). Meiotic recombination: the essence of heredity. Cold Spring Harb. Perspect. Biol. 7, a016618. doi:10.1101/cshperspect.a016618
Ieda, R., Hosoya, S., Tajima, S., Atsumi, K., Kamiya, T., Nozawa, A., et al. (2018). Identification of the sex-determining locus in grass puffer (Takifugu niphobles) provides evidence for sex-chromosome turnover in a subset of Takifugu species. PLoS One 13, e0190635. doi:10.1371/journal.pone.0190635
Ikeda, Y., Swain, A., Weber, T. J., Hentges, K. E., Zanaria, E., Lalli, E., et al. (1996). Steroidogenic factor 1 and Dax-1 colocalize in multiple cell lineages: potential links in endocrine development. Mol. Endocrinol. 10, 1261–1272. doi:10.1210/mend.10.10.9121493
Ilicic, T., Kim, J. K., Kolodziejczyk, A. A., Bagger, F. O., Mccarthy, D. J., Marioni, J. C., et al. (2016). Classification of low quality cells from single-cell RNA-seq data. Genome Biol. 17, 29. doi:10.1186/s13059-016-0888-1
Imai, T., Saino, K., and Matsuda, M. (2015). Mutation of Gonadal soma-derived factor induces medaka XY gonads to undergo ovarian development. Biochem. Biophys. Res. Commun. 467, 109–114. doi:10.1016/j.bbrc.2015.09.112
Imai, Y., Olaya, I., Sakai, N., and Burgess, S. M. (2021). Meiotic chromosome dynamics in zebrafish. Front. Cell Dev. Biol. 9, 757445. doi:10.3389/fcell.2021.757445
Ings, J. S., and Van Der Kraak, G. J. (2006). Characterization of the mRNA expression of StAR and steroidogenic enzymes in zebrafish ovarian follicles. Mol. Reprod. Dev. 73, 943–954. doi:10.1002/mrd.20490
Ioannidis, J., Taylor, G., Zhao, D., Liu, L., Idoko-Akoh, A., Gong, D., et al. (2021). Primary sex determination in birds depends on DMRT1 dosage, but gonadal sex does not determine adult secondary sex characteristics. Proc. Natl. Acad. Sci. U. S. A. 118, e2020909118. doi:10.1073/pnas.2020909118
Islam, K. N., Modi, M. M., and Siegfried, K. R. (2021). The zebrafish meiotic cohesin complex protein Smc1b is required for key events in meiotic prophase I. Front. Cell Dev. Biol. 9, 714245. doi:10.3389/fcell.2021.714245
Jackson, D. G., Prevo, R., Clasper, S., and Banerji, S. (2001). LYVE-1, the lymphatic system and tumor lymphangiogenesis. Trends Immunol. 22, 317–321. doi:10.1016/s1471-4906(01)01936-6
Jaillon, O., Aury, J. M., Brunet, F., Petit, J. L., Stange-Thomann, N., Mauceli, E., et al. (2004). Genome duplication in the teleost fish Tetraodon nigroviridis reveals the early vertebrate proto-karyotype. Nature 431, 946–957. doi:10.1038/nature03025
Jamieson-Lucy, A. H., Kobayashi, M., James Aykit, Y., Elkouby, Y. M., Escobar-Aguirre, M., Vejnar, C. E., et al. (2022). A proteomics approach identifies novel resident zebrafish Balbiani body proteins Cirbpa and Cirbpb. Dev. Biol. 484, 1–11. doi:10.1016/j.ydbio.2022.01.006
Janes, D. E., Organ, C. L., Stiglec, R., O'Meally, D., Sarre, S. D., Georges, A., et al. (2014). Molecular evolution of Dmrt1 accompanies change of sex-determining mechanisms in reptilia. Biol. Lett. 10, 20140809. doi:10.1098/rsbl.2014.0809
Jiang, D. N., Peng, Y. X., Liu, X. Y., Mustapha, U. F., Huang, Y. Q., Shi, H. J., et al. (2022). Homozygous mutation of gsdf causes infertility in female nile Tilapia (Oreochromis niloticus). Front. Endocrinol. (Lausanne) 13, 813320. doi:10.3389/fendo.2022.813320
Kaipainen, A., Korhonen, J., Mustonen, T., Van Hinsbergh, V. W., Fang, G. H., Dumont, D., et al. (1995). Expression of the fms-like tyrosine kinase 4 gene becomes restricted to lymphatic endothelium during development. Proc. Natl. Acad. Sci. U. S. A. 92, 3566–3570. doi:10.1073/pnas.92.8.3566
Kamiya, T., Kai, W., Tasumi, S., Oka, A., Matsunaga, T., Mizuno, N., et al. (2012). A trans-species missense SNP in Amhr2 is associated with sex determination in the tiger pufferfish, Takifugu rubripes (fugu). PLoS Genet. 8, e1002798. doi:10.1371/journal.pgen.1002798
Keeney, S. (2008). Spo11 and the formation of DNA double-strand breaks in meiosis. Genome Dyn. Stab. 2, 81–123. doi:10.1007/7050_2007_026
Khandekar, G., Kim, S., and Jagadeeswaran, P. (2012). Zebrafish thrombocytes: functions and origins. Adv. Hematol. 2012, 857058. doi:10.1155/2012/857058
Kikuchi, M., Nishimura, T., Ishishita, S., Matsuda, Y., and Tanaka, M. (2020). foxl3, a sexual switch in germ cells, initiates two independent molecular pathways for commitment to oogenesis in medaka. Proc. Natl. Acad. Sci. U. S. A. 117, 12174–12181. doi:10.1073/pnas.1918556117
Kim, S., Bardwell, V. J., and Zarkower, D. (2007). Cell type-autonomous and non-autonomous requirements for Dmrt1 in postnatal testis differentiation. Dev. Biol. 307, 314–327. doi:10.1016/j.ydbio.2007.04.046
Kinnear, H. M., Tomaszewski, C. E., Chang, F. L., Moravek, M. B., Xu, M., Padmanabhan, V., et al. (2020). The ovarian stroma as a new frontier. Reproduction 160, R25–R39. doi:10.1530/REP-19-0501
Klein Wolterink, R. G., Serafini, N., Van Nimwegen, M., Vosshenrich, C. A., De Bruijn, M. J., Fonseca Pereira, D., et al. (2013). Essential, dose-dependent role for the transcription factor Gata3 in the development of IL-5+ and IL-13+ type 2 innate lymphoid cells. Proc. Natl. Acad. Sci. U. S. A. 110, 10240–10245. doi:10.1073/pnas.1217158110
Kobayashi, T., Matsuda, M., Kajiura-Kobayashi, H., Suzuki, A., Saito, N., Nakamoto, M., et al. (2004). Two DM domain genes, DMY and DMRT1, involved in testicular differentiation and development in the medaka, Oryzias latipes. Dev. Dyn. 231, 518–526. doi:10.1002/dvdy.20158
Kopp, A. (2012). Dmrt genes in the development and evolution of sexual dimorphism. Trends Genet. 28, 175–184. doi:10.1016/j.tig.2012.02.002
Kossack, M. E., and Draper, B. W. (2019). Genetic regulation of sex determination and maintenance in zebrafish (Danio rerio). Curr. Top. Dev. Biol. 134, 119–149. doi:10.1016/bs.ctdb.2019.02.004
Kossack, M. E., Tian, L., Bowie, K., and Plavicki, J. S. (2023). More than germ cells: vascular development in the early zebrafish 1 (Danio rerio) gonad. bioRxiv, 2023.01.18.524593. doi:10.1101/2023.01.18.524593
Koyama, T., Nakamoto, M., Morishima, K., Yamashita, R., Yamashita, T., Sasaki, K., et al. (2019). A SNP in a steroidogenic enzyme is associated with phenotypic sex in Seriola fishes. Curr. Biol. 29, 1901–1909. doi:10.1016/j.cub.2019.04.069
Krovel, A. V., and Olsen, L. C. (2004). Sexual dimorphic expression pattern of a splice variant of zebrafish vasa during gonadal development. Dev. Biol. 271, 190–197. doi:10.1016/j.ydbio.2004.04.004
Kuo, M. W., Postlethwait, J., Lee, W. C., Lou, S. W., Chan, W. K., and Chung, B. C. (2005). Gene duplication, gene loss and evolution of expression domains in the vertebrate nuclear receptor NR5A (Ftz-F1) family. Biochem. J. 389, 19–26. doi:10.1042/BJ20050005
Kusakabe, M., Nakamura, I., and Young, G. (2003). 11beta-hydroxysteroid dehydrogenase complementary deoxyribonucleic acid in rainbow trout: cloning, sites of expression, and seasonal changes in gonads. Endocrinology 144, 2534–2545. doi:10.1210/en.2002-220446
Lai, W. W., Hsiao, P. H., Guiguen, Y., and Chung, B. C. (1998). Cloning of zebrafish cDNA for 3beta-hydroxysteroid dehydrogenase and P450scc. Endocr. Res. 24, 927–931. doi:10.3109/07435809809032708
Lam, S. H., Chua, H. L., Gong, Z., Lam, T. J., and Sin, Y. M. (2004). Development and maturation of the immune system in zebrafish, Danio rerio: a gene expression profiling, in situ hybridization and immunological study. Dev. Comp. Immunol. 28, 9–28. doi:10.1016/s0145-305x(03)00103-4
Lau, E. S., Zhang, Z., Qin, M., and Ge, W. (2016). Knockout of zebrafish ovarian aromatase gene (cyp19a1a) by TALEN and CRISPR/Cas9 leads to all-male offspring due to failed ovarian differentiation. Sci. Rep. 6, 37357. doi:10.1038/srep37357
Lawrence, C., Ebersole, J. P., and Kesseli, R. V. (2008). Rapid growth and out-crossing promote female development in zebrafish (Danio rerio). Environ. Biol. Fishes 81, 239–246. doi:10.1007/s10641-007-9195-8
Lawson, N. D., Li, R., Shin, M., Grosse, A., Yukselen, O., Stone, O. A., et al. (2020). An improved zebrafish transcriptome annotation for sensitive and comprehensive detection of cell type-specific genes. Elife 9, e55792. doi:10.7554/eLife.55792
Leal, M. C., Feitsma, H., Cuppen, E., Franca, L. R., and Schulz, R. W. (2008). Completion of meiosis in male zebrafish (Danio rerio) despite lack of DNA mismatch repair gene mlh1. Cell Tissue Res. 332, 133–139. doi:10.1007/s00441-007-0550-z
Lee, S., Kang, J., Yoo, J., Ganesan, S. K., Cook, S. C., Aguilar, B., et al. (2009). Prox1 physically and functionally interacts with COUP-TFII to specify lymphatic endothelial cell fate. Blood 113, 1856–1859. doi:10.1182/blood-2008-03-145789
Liew, W. C., and Orban, L. (2014). Zebrafish sex: a complicated affair. Brief. Funct. Genomics 13, 172–187. doi:10.1093/bfgp/elt041
Li, M., Sun, Y., Zhao, J., Shi, H., Zeng, S., Ye, K., et al. (2015). A tandem duplicate of anti-mullerian hormone with a missense SNP on the Y chromosome is essential for male sex determination in nile Tilapia, Oreochromis niloticus. PLoS Genet. 11, e1005678. doi:10.1371/journal.pgen.1005678
Lin, H. F., Traver, D., Zhu, H., Dooley, K., Paw, B. H., Zon, L. I., et al. (2005). Analysis of thrombocyte development in CD41-GFP transgenic zebrafish. Blood 106, 3803–3810. doi:10.1182/blood-2005-01-0179
Lin, J. C., Hu, S., Ho, P. H., Hsu, H. J., Postlethwait, J. H., and Chung, B. C. (2015). Two zebrafish hsd3b genes are distinct in function, expression, and evolution. Endocrinology 156, 2854–2862. doi:10.1210/en.2014-1584
Li, N., Oakes, J. A., Storbeck, K. H., Cunliffe, V. T., and Krone, N. P. (2020a). The P450 side-chain cleavage enzyme Cyp11a2 facilitates steroidogenesis in zebrafish. J. Endocrinol. 244, 309–321. doi:10.1530/JOE-19-0384
Lin, Q., Mei, J., Li, Z., Zhang, X., Zhou, L., and Gui, J. F. (2017). Distinct and cooperative roles of amh and dmrt1 in self-renewal and differentiation of male germ cells in zebrafish. Genetics 207, 1007–1022. doi:10.1534/genetics.117.300274
Liu, Y., Kossack, M. E., Mcfaul, M. E., Christensen, L. N., Siebert, S., Wyatt, S. R., et al. (2022). Single-cell transcriptome reveals insights into the development and function of the zebrafish ovary. Elife 11, e76014. doi:10.7554/eLife.76014
Li, X., Zhang, F., Wu, N., Ye, D., Wang, Y., Zhang, X., et al. (2020b). A critical role of foxp3a-positive regulatory T cells in maintaining immune homeostasis in zebrafish testis development. J. Genet. Genomics 47, 547–561. doi:10.1016/j.jgg.2020.07.006
Lu, H., Cui, Y., Jiang, L., and Ge, W. (2017). Functional analysis of nuclear estrogen receptors in zebrafish reproduction by genome editing approach. Endocrinology 158, 2292–2308. doi:10.1210/en.2017-00215
Lu, H., Zhao, C., Zhu, B., Zhang, Z., and Ge, W. (2020). Loss of inhibin advances follicle activation and female puberty onset but blocks oocyte maturation in zebrafish. Endocrinology 161, bqaa184. doi:10.1210/endocr/bqaa184
Luzio, A., Coimbra, A. M., Benito, C., Fontainhas-Fernandes, A. A., and Matos, M. (2015). Screening and identification of potential sex-associated sequences in Danio rerio. Mol. Reprod. Dev. 82, 756–764. doi:10.1002/mrd.22508
Luzio, A., Matos, M., Santos, D., Fontainhas-Fernandes, A. A., Monteiro, S. M., and Coimbra, A. M. (2016). Disruption of apoptosis pathways involved in zebrafish gonad differentiation by 17α-ethinylestradiol and fadrozole exposures. Aquat. Toxicol. 177, 269–284. doi:10.1016/j.aquatox.2016.05.029
Maack, G., and Segner, H. (2003). Morphological development of the gonads in zebrafish. J. Fish Biol. 62, 895–906. doi:10.1046/j.1095-8649.2003.00074.x
Maegawa, S., Yasuda, K., and Inoue, K. (1999). Maternal mRNA localization of zebrafish DAZ-like gene. Mech. Dev. 81, 223–226. doi:10.1016/s0925-4773(98)00242-1
Maekawa, M., Kamimura, K., and Nagano, T. (1996). Peritubular myoid cells in the testis: their structure and function. Arch. Histol. Cytol. 59, 1–13. doi:10.1679/aohc.59.1
Marlow, F. L., and Mullins, M. C. (2008). Bucky ball functions in Balbiani body assembly and animal-vegetal polarity in the oocyte and follicle cell layer in zebrafish. Dev. Biol. 321, 40–50. doi:10.1016/j.ydbio.2008.05.557
Martin, F. J., Amode, M. R., Aneja, A., Austine-Orimoloye, O., Azov, A. G., Barnes, I., et al. (2023). Ensembl 2023. Nucleic Acids Res. 51, D933–D941. doi:10.1093/nar/gkac958
Masumura, T., Yamamoto, K., Shimizu, N., Obi, S., and Ando, J. (2009). Shear stress increases expression of the arterial endothelial marker ephrinB2 in murine ES cells via the VEGF-Notch signaling pathways. Arterioscler. Thromb. Vasc. Biol. 29, 2125–2131. doi:10.1161/ATVBAHA.109.193185
Matson, C. K., Murphy, M. W., Griswold, M. D., Yoshida, S., Bardwell, V. J., and Zarkower, D. (2010). The mammalian doublesex homolog DMRT1 is a transcriptional gatekeeper that controls the mitosis versus meiosis decision in male germ cells. Dev. Cell 19, 612–624. doi:10.1016/j.devcel.2010.09.010
Matsuda, M., and Sakaizumi, M. (2016). Evolution of the sex-determining gene in the teleostean genus Oryzias. Gen. Comp. Endocrinol. 239, 80–88. doi:10.1016/j.ygcen.2015.10.004
Mazaud, S., Oreal, E., Guigon, C. J., Carre-Eusebe, D., and Magre, S. (2002). Lhx9 expression during gonadal morphogenesis as related to the state of cell differentiation. Gene Expr. Patterns 2, 373–377. doi:10.1016/s1567-133x(02)00050-9
Mcclelland, K. S., Bell, K., Larney, C., Harley, V. R., Sinclair, A. H., Oshlack, A., et al. (2015). Purification and transcriptomic analysis of mouse fetal Leydig cells reveals candidate genes for specification of gonadal steroidogenic cells. Biol. Reprod. 92, 145. doi:10.1095/biolreprod.115.128918
Mcmillan, S. C., Xu, Z. T., Zhang, J., Teh, C., Korzh, V., Trudeau, V. L., et al. (2013). Regeneration of breeding tubercles on zebrafish pectoral fins requires androgens and two waves of revascularization. Development 140, 4323–4334. doi:10.1242/dev.095992
Menuet, A., Le Page, Y., Torres, O., Kern, L., Kah, O., and Pakdel, F. (2004). Analysis of the estrogen regulation of the zebrafish estrogen receptor (ER) reveals distinct effects of ERalpha, ERbeta1 and ERbeta2. J. Mol. Endocrinol. 32, 975–986. doi:10.1677/jme.0.0320975
Miao, L., Yuan, Y., Cheng, F., Fang, J., Zhou, F., Ma, W., et al. (2017). Translation repression by maternal RNA binding protein Zar1 is essential for early oogenesis in zebrafish. Development 144, 128–138. doi:10.1242/dev.144642
Miller, W. L., and Auchus, R. J. (2011). The molecular biology, biochemistry, and physiology of human steroidogenesis and its disorders. Endocr. Rev. 32, 81–151. doi:10.1210/er.2010-0013
Mindnich, R., Deluca, D., and Adamski, J. (2004). Identification and characterization of 17 beta-hydroxysteroid dehydrogenases in the zebrafish, Danio rerio. Mol. Cell Endocrinol. 215, 19–30. doi:10.1016/j.mce.2003.11.010
Mindnich, R., Haller, F., Halbach, F., Moeller, G., Hrabe De Angelis, M., and Adamski, J. (2005). Androgen metabolism via 17beta-hydroxysteroid dehydrogenase type 3 in mammalian and non-mammalian vertebrates: comparison of the human and the zebrafish enzyme. J. Mol. Endocrinol. 35, 305–316. doi:10.1677/jme.1.01853
Miyabayashi, K., Katoh-Fukui, Y., Ogawa, H., Baba, T., Shima, Y., Sugiyama, N., et al. (2013). Aristaless related homeobox gene, Arx, is implicated in mouse fetal Leydig cell differentiation possibly through expressing in the progenitor cells. PLoS One 8, e68050. doi:10.1371/journal.pone.0068050
Model, L. S., Hall, M. R., Wong, D. J., Muto, A., Kondo, Y., Ziegler, K. R., et al. (2014). Arterial shear stress reduces eph-b4 expression in adult human veins. Yale J. Biol. Med. 87, 359–371.
Moore, F. E., Garcia, E. G., Lobbardi, R., Jain, E., Tang, Q., Moore, J. C., et al. (2016a). Single-cell transcriptional analysis of normal, aberrant, and malignant hematopoiesis in zebrafish. J. Exp. Med. 213, 979–992. doi:10.1084/jem.20152013
Moore, J. C., Mulligan, T. S., Yordan, N. T., Castranova, D., Pham, V. N., Tang, Q., et al. (2016b). T cell immune deficiency in zap70 mutant zebrafish. Mol. Cell Biol. 36, 2868–2876. doi:10.1128/MCB.00281-16
Morais Da Silva, S., Hacker, A., Harley, V., Goodfellow, P., Swain, A., and Lovell-Badge, R. (1996). Sox9 expression during gonadal development implies a conserved role for the gene in testis differentiation in mammals and birds. Nat. Genet. 14, 62–68. doi:10.1038/ng0996-62
Morinaga, C., Saito, D., Nakamura, S., Sasaki, T., Asakawa, S., Shimizu, N., et al. (2007). The hotei mutation of medaka in the anti-Mullerian hormone receptor causes the dysregulation of germ cell and sexual development. Proc. Natl. Acad. Sci. U. S. A. 104, 9691–9696. doi:10.1073/pnas.0611379104
Morini, M., Lafont, A. G., Maugars, G., Baloche, S., Dufour, S., Asturiano, J. F., et al. (2020). Identification and stable expression of vitellogenin receptor through vitellogenesis in the European eel. Animal 14, 1213–1222. doi:10.1017/S1751731119003355
Morohashi, K., Hatano, O., Nomura, M., Takayama, K., Hara, M., Yoshii, H., et al. (1995). Function and distribution of a steroidogenic cell-specific transcription factor, Ad4BP. J. Steroid Biochem. Mol. Biol. 53, 81–88. doi:10.1016/0960-0760(95)00041-w
Moro, K., Yamada, T., Tanabe, M., Takeuchi, T., Ikawa, T., Kawamoto, H., et al. (2010). Innate production of T(H)2 cytokines by adipose tissue-associated c-Kit(+)Sca-1(+) lymphoid cells. Nature 463, 540–544. doi:10.1038/nature08636
Mossadegh-Keller, N., and Sieweke, M. H. (2018). Testicular macrophages: guardians of fertility. Cell Immunol. 330, 120–125. doi:10.1016/j.cellimm.2018.03.009
Mullins, M. C., Hammerschmidt, M., Haffter, P., and Nusslein-Volhard, C. (1994). Large-scale mutagenesis in the zebrafish: in search of genes controlling development in a vertebrate. Curr. Biol. 4, 189–202. doi:10.1016/s0960-9822(00)00048-8
Mushirobira, Y., Mizuta, H., Luo, W., Todo, T., Hara, A., Reading, B. J., et al. (2015). Molecular cloning and partial characterization of a low-density lipoprotein receptor-related protein 13 (Lrp13) involved in vitellogenin uptake in the cutthroat trout (Oncorhynchus clarki). Mol. Reprod. Dev. 82, 986–1000. doi:10.1002/mrd.22579
Mustapha, U. F., Jiang, D.-N., Liang, Z.-H., Gu, H.-T., Yang, W., Chen, H.-P., et al. (2018). Male-specific Dmrt1 is a candidate sex determination gene in spotted scat (Scatophagus argus). Aquaculture 495, 351–358. doi:10.1016/j.aquaculture.2018.06.009
Muto, A., Yi, T., Harrison, K. D., Davalos, A., Fancher, T. T., Ziegler, K. R., et al. (2011). Eph-B4 prevents venous adaptive remodeling in the adult arterial environment. J. Exp. Med. 208, 561–575. doi:10.1084/jem.20101854
Myosho, T., Otake, H., Masuyama, H., Matsuda, M., Kuroki, Y., Fujiyama, A., et al. (2012). Tracing the emergence of a novel sex-determining gene in medaka, Oryzias luzonensis. Genetics 191, 163–170. doi:10.1534/genetics.111.137497
Nagahama, Y., Chakraborty, T., Paul-Prasanth, B., Ohta, K., and Nakamura, M. (2021). Sex determination, gonadal sex differentiation, and plasticity in vertebrate species. Physiol. Rev. 101, 1237–1308. doi:10.1152/physrev.00044.2019
Nakamoto, M., Fukasawa, M., Orii, S., Shimamori, K., Maeda, T., Suzuki, A., et al. (2010). Cloning and expression of medaka cholesterol side chain cleavage cytochrome P450 during gonadal development. Dev. Growth Differ. 52, 385–395. doi:10.1111/j.1440-169X.2010.01178.x
Nakayama, A., Nakayama, M., Turner, C. J., Hoing, S., Lepore, J. J., and Adams, R. H. (2013). Ephrin-B2 controls PDGFRβ internalization and signaling. Genes Dev. 27, 2576–2589. doi:10.1101/gad.224089.113
Namwanje, M., and Brown, C. W. (2016). Activins and inhibins: roles in development, physiology, and disease. Cold Spring Harb. Perspect. Biol. 8, a021881. doi:10.1101/cshperspect.a021881
Nanda, I., Kondo, M., Hornung, U., Asakawa, S., Winkler, C., Shimizu, A., et al. (2002). A duplicated copy of DMRT1 in the sex-determining region of the Y chromosome of the medaka, Oryzias latipes. Proc. Natl. Acad. Sci. U. S. A. 99, 11778–11783. doi:10.1073/pnas.182314699
Neill, D. R., Wong, S. H., Bellosi, A., Flynn, R. J., Daly, M., Langford, T. K., et al. (2010). Nuocytes represent a new innate effector leukocyte that mediates type-2 immunity. Nature 464, 1367–1370. doi:10.1038/nature08900
Nelson, D. R., Goldstone, J. V., and Stegeman, J. J. (2013). The cytochrome P450 genesis locus: the origin and evolution of animal cytochrome P450s. Philos. Trans. R. Soc. Lond B Biol. Sci. 368, 20120474. doi:10.1098/rstb.2012.0474
Ogita, Y., Mawaribuchi, S., Nakasako, K., Tamura, K., Matsuda, M., Katsumura, T., et al. (2020). Parallel evolution of two dmrt1-derived genes, dmy and dm-W, for vertebrate sex determination. iScience 23, 100757. doi:10.1016/j.isci.2019.100757
Olsen, L. C., Aasland, R., and Fjose, A. (1997). A vasa-like gene in zebrafish identifies putative primordial germ cells. Mech. Dev. 66, 95–105. doi:10.1016/s0925-4773(97)00099-3
Pan, Q., Feron, R., Jouanno, E., Darras, H., Herpin, A., Koop, B., et al. (2021). The rise and fall of the ancient northern pike master sex determining gene. Elife 10, e62858. doi:10.7554/eLife.62858
Parajes, S., Griffin, A., Taylor, A. E., Rose, I. T., Miguel-Escalada, I., Hadzhiev, Y., et al. (2013). Redefining the initiation and maintenance of zebrafish interrenal steroidogenesis by characterizing the key enzyme cyp11a2. Endocrinology 154, 2702–2711. doi:10.1210/en.2013-1145
Pereira, F. A., Tsai, M. J., and Tsai, S. Y. (2000). COUP-TF orphan nuclear receptors in development and differentiation. Cell Mol. Life Sci. 57, 1388–1398. doi:10.1007/PL00000624
Pereiro, P., Varela, M., Diaz-Rosales, P., Romero, A., Dios, S., Figueras, A., et al. (2015). Zebrafish Nk-lysins: first insights about their cellular and functional diversification. Dev. Comp. Immunol. 51, 148–159. doi:10.1016/j.dci.2015.03.009
Petersen, A. M., Small, C. M., Yan, Y. L., Wilson, C., Batzel, P., Bremiller, R. A., et al. (2022). Evolution and developmental expression of the sodium-iodide symporter (NIS, slc5a5) gene family: implications for perchlorate toxicology. Evol. Appl. 15, 1079–1098. doi:10.1111/eva.13424
Postlethwait, J. H., Navajas Acedo, J., and Piotrowski, T. (2019). Evolutionary origin and nomenclature of vertebrate wnt11-family genes. Zebrafish 16, 469–476. doi:10.1089/zeb.2019.1760
Postlethwait, J., Amores, A., Force, A., and Yan, Y. L. (1999). The zebrafish genome. Methods Cell Biol. 60, 149–163.
Prevo, R., Banerji, S., Ferguson, D. J., Clasper, S., and Jackson, D. G. (2001). Mouse LYVE-1 is an endocytic receptor for hyaluronan in lymphatic endothelium. J. Biol. Chem. 276, 19420–19430. doi:10.1074/jbc.M011004200
Purcell, C. M., Seetharam, A. S., Snodgrass, O., Ortega-Garcia, S., Hyde, J. R., and Severin, A. J. (2018). Insights into teleost sex determination from the Seriola dorsalis genome assembly. BMC Genomics 19, 31. doi:10.1186/s12864-017-4403-1
Qin, Y., and Bishop, C. E. (2005). Sox9 is sufficient for functional testis development producing fertile male mice in the absence of Sry. Hum. Mol. Genet. 14, 1221–1229. doi:10.1093/hmg/ddi133
Qiu, Y., Wang, J., Lei, J., and Roeder, K. (2021). Identification of cell-type-specific marker genes from co-expression patterns in tissue samples. Bioinformatics 37, 3228–3234. doi:10.1093/bioinformatics/btab257
Ramanagoudr-Bhojappa, R., Carrington, B., Ramaswami, M., Bishop, K., Robbins, G. M., Jones, M., et al. (2018). Multiplexed CRISPR/Cas9-mediated knockout of 19 Fanconi anemia pathway genes in zebrafish revealed their roles in growth, sexual development and fertility. PLoS Genet. 14, e1007821. doi:10.1371/journal.pgen.1007821
Raymond, C. S., Kettlewell, J. R., Hirsch, B., Bardwell, V. J., and Zarkower, D. (1999). Expression of Dmrt1 in the genital ridge of mouse and chicken embryos suggests a role in vertebrate sexual development. Dev. Biol. 215, 208–220. doi:10.1006/dbio.1999.9461
Raymond, C. S., Murphy, M. W., O'Sullivan, M. G., Bardwell, V. J., and Zarkower, D. (2000). Dmrt1, a gene related to worm and fly sexual regulators, is required for mammalian testis differentiation. Genes Dev. 14, 2587–2595. doi:10.1101/gad.834100
Reading, B. J., Hiramatsu, N., Schilling, J., Molloy, K. T., Glassbrook, N., Mizuta, H., et al. (2014). Lrp13 is a novel vertebrate lipoprotein receptor that binds vitellogenins in teleost fishes. J. Lipid Res. 55, 2287–2295. doi:10.1194/jlr.M050286
Ribas, L., Valdivieso, A., Diaz, N., and Piferrer, F. (2017). Appropriate rearing density in domesticated zebrafish to avoid masculinization: links with the stress response. J. Exp. Biol. 220, 1056–1064. doi:10.1242/jeb.144980
Rodriguez-Mari, A., Canestro, C., Bremiller, R. A., Nguyen-Johnson, A., Asakawa, K., Kawakami, K., et al. (2010). Sex reversal in zebrafish fancl mutants is caused by Tp53-mediated germ cell apoptosis. PLoS Genet. 6, e1001034. doi:10.1371/journal.pgen.1001034
Rodriguez-Mari, A., Wilson, C., Titus, T. A., Canestro, C., Bremiller, R. A., Yan, Y. L., et al. (2011). Roles of brca2 (fancd1) in oocyte nuclear architecture, gametogenesis, gonad tumors, and genome stability in zebrafish. PLoS Genet. 7, e1001357. doi:10.1371/journal.pgen.1001357
Rodriguez-Mari, A., Yan, Y. L., Bremiller, R. A., Wilson, C., Canestro, C., and Postlethwait, J. H. (2005). Characterization and expression pattern of zebrafish Anti-Mullerian hormone (Amh) relative to sox9a, sox9b, and cyp19a1a, during gonad development. Gene Expr. Patterns 5, 655–667. doi:10.1016/j.modgep.2005.02.008
Rodriguez-Nunez, I., Wcisel, D. J., Litman, R. T., Litman, G. W., and Yoder, J. A. (2016). The identification of additional zebrafish DICP genes reveals haplotype variation and linkage to MHC class I genes. Immunogenetics 68, 295–312. doi:10.1007/s00251-016-0901-6
Roh-Johnson, M., Shah, A. N., Stonick, J. A., Poudel, K. R., Kargl, J., Yang, G. H., et al. (2017). Macrophage-Dependent cytoplasmic transfer during melanoma invasion in vivo. Dev. Cell 43, 549–562. doi:10.1016/j.devcel.2017.11.003
Romano, S., Kaufman, O. H., and Marlow, F. L. (2020). Loss of dmrt1 restores zebrafish female fates in the absence of cyp19a1a but not rbpms2a/b. Development 147, dev190942. doi:10.1242/dev.190942
Rondeau, E. B., Messmer, A. M., Sanderson, D. S., Jantzen, S. G., Von Schalburg, K. R., Minkley, D. R., et al. (2013). Genomics of sablefish (Anoplopoma fimbria): expressed genes, mitochondrial phylogeny, linkage map and identification of a putative sex gene. BMC Genomics 14, 452. doi:10.1186/1471-2164-14-452
Rougeot, J., Torraca, V., Zakrzewska, A., Kanwal, Z., Jansen, H. J., Sommer, F., et al. (2019). RNAseq profiling of leukocyte populations in zebrafish larvae reveals a cxcl11 chemokine gene as a marker of macrophage polarization during mycobacterial infection. Front. Immunol. 10, 832. doi:10.3389/fimmu.2019.00832
Saito, K., Siegfried, K. R., Nusslein-Volhard, C., and Sakai, N. (2011). Isolation and cytogenetic characterization of zebrafish meiotic prophase I mutants. Dev. Dyn. 240, 1779–1792. doi:10.1002/dvdy.22661
Santos, E. M., Workman, V. L., Paull, G. C., Filby, A. L., Van Look, K. J., Kille, P., et al. (2007). Molecular basis of sex and reproductive status in breeding zebrafish. Physiol. Genomics 30, 111–122. doi:10.1152/physiolgenomics.00284.2006
Santos, D., Luzio, A., and Coimbra, A. M. (2017). Zebrafish sex differentiation and gonad development: a review on the impact of environmental factors. Aquat. Toxicol. 191, 141–163. doi:10.1016/j.aquatox.2017.08.005
Sawatari, E., Shikina, S., Takeuchi, T., and Yoshizaki, G. (2007). A novel transforming growth factor-beta superfamily member expressed in gonadal somatic cells enhances primordial germ cell and spermatogonial proliferation in rainbow trout (Oncorhynchus mykiss). Dev. Biol. 301, 266–275. doi:10.1016/j.ydbio.2006.10.001
Scheppke, L., Murphy, E. A., Zarpellon, A., Hofmann, J. J., Merkulova, A., Shields, D. J., et al. (2012). Notch promotes vascular maturation by inducing integrin-mediated smooth muscle cell adhesion to the endothelial basement membrane. Blood 119, 2149–2158. doi:10.1182/blood-2011-04-348706
Schorpp, M., Bialecki, M., Diekhoff, D., Walderich, B., Odenthal, J., Maischein, H. M., et al. (2006). Conserved functions of Ikaros in vertebrate lymphocyte development: genetic evidence for distinct larval and adult phases of T cell development and two lineages of B cells in zebrafish. J. Immunol. 177, 2463–2476. doi:10.4049/jimmunol.177.4.2463
Schulz, R. W., and Nóbrega, R. H. (2011). “The reproductive organs and processes | anatomy and histology of fish testis,” in Encyclopedia of fish physiology. From genome to environment. Editor A. P. Farrell (San Diego: Academic Press).
Selman, K., Wallace, R. A., Sarka, A., and Qi, X. (1993). Stages of oocyte development in the zebrafish, Brachydanio rerio. J. Morphol. 218, 203–224. doi:10.1002/jmor.1052180209
Shang, E. H., Yu, R. M., and Wu, R. S. (2006). Hypoxia affects sex differentiation and development, leading to a male-dominated population in zebrafish (Danio rerio). Environ. Sci. Technol. 40, 3118–3122. doi:10.1021/es0522579
Shao, T., Shi, W., Zheng, J. Y., Xu, X. X., Lin, A. F., Xiang, L. X., et al. (2018). Costimulatory function of Cd58/Cd2 interaction in adaptive humoral immunity in a zebrafish model. Front. Immunol. 9, 1204. doi:10.3389/fimmu.2018.01204
Shin, Y. H., Mcguire, M. M., and Rajkovic, A. (2013). Mouse HORMAD1 is a meiosis i checkpoint protein that modulates DNA double-strand break repair during female meiosis. Biol. Reprod. 89, 29. doi:10.1095/biolreprod.112.106773
Shive, H. R., West, R. R., Embree, L. J., Azuma, M., Sood, R., Liu, P., et al. (2010). brca2 in zebrafish ovarian development, spermatogenesis, and tumorigenesis. Proc. Natl. Acad. Sci. U. S. A. 107, 19350–19355. doi:10.1073/pnas.1011630107
Slanchev, K., Stebler, J., De La Cueva-Mendez, G., and Raz, E. (2005). Development without germ cells: the role of the germ line in zebrafish sex differentiation. Proc. Natl. Acad. Sci. U. S. A. 102, 4074–4079. doi:10.1073/pnas.0407475102
Smith, C. A., Roeszler, K. N., Ohnesorg, T., Cummins, D. M., Farlie, P. G., Doran, T. J., et al. (2009). The avian Z-linked gene DMRT1 is required for male sex determination in the chicken. Nature 461, 267–271. doi:10.1038/nature08298
Sommer, F., Torraca, V., Kamel, S. M., Lombardi, A., and Meijer, A. H. (2020). Frontline Science: antagonism between regular and atypical Cxcr3 receptors regulates macrophage migration during infection and injury in zebrafish. J. Leukoc. Biol. 107, 185–203. doi:10.1002/JLB.2HI0119-006R
Song, H. D., Sun, X. J., Deng, M., Zhang, G. W., Zhou, Y., Wu, X. Y., et al. (2004). Hematopoietic gene expression profile in zebrafish kidney marrow. Proc. Natl. Acad. Sci. U. S. A. 101, 16240–16245. doi:10.1073/pnas.0407241101
Song, W., Xie, Y., Sun, M., Li, X., Fitzpatrick, C. K., Vaux, F., et al. (2021). A duplicated amh is the master sex-determining gene for Sebastes rockfish in the Northwest Pacific. Open Biol. 11, 210063. doi:10.1098/rsob.210063
Stanzione, M., Baumann, M., Papanikos, F., Dereli, I., Lange, J., Ramlal, A., et al. (2016). Meiotic DNA break formation requires the unsynapsed chromosome axis-binding protein IHO1 (CCDC36) in mice. Nat. Cell Biol. 18, 1208–1220. doi:10.1038/ncb3417
Stevant, I., Kuhne, F., Greenfield, A., Chaboissier, M. C., Dermitzakis, E. T., and Nef, S. (2019). Dissecting cell lineage specification and sex fate determination in gonadal somatic cells using single-cell transcriptomics. Cell Rep. 26, 3272–3283. doi:10.1016/j.celrep.2019.02.069
Streisinger, G., Walker, C., Dower, N., Knauber, D., and Singer, F. (1981). Production of clones of homozygous diploid zebra fish (Brachydanio rerio). Nature 291, 293–296. doi:10.1038/291293a0
Su, Y. Q., Wu, X., O'Brien, M. J., Pendola, F. L., Denegre, J. N., Matzuk, M. M., et al. (2004). Synergistic roles of BMP15 and GDF9 in the development and function of the oocyte-cumulus cell complex in mice: genetic evidence for an oocyte-granulosa cell regulatory loop. Dev. Biol. 276, 64–73. doi:10.1016/j.ydbio.2004.08.020
Sugawara, T., Kiriakidou, M., Mcallister, J. M., Holt, J. A., Arakane, F., and Strauss, J. F. (1997). Regulation of expression of the steroidogenic acute regulatory protein (StAR) gene: a central role for steroidogenic factor 1. Steroids 62, 5–9. doi:10.1016/s0039-128x(96)00152-3
Takahashi, H. (1977). Juvenile hermaphroditism in the zebrafish, Brachydanio rerio. Bull. Fac. Fish. Hokkaido Univ. 28, 57–65.
Takemoto, K., Imai, Y., Saito, K., Kawasaki, T., Carlton, P. M., Ishiguro, K. I., et al. (2020). Sycp2 is essential for synaptonemal complex assembly, early meiotic recombination and homologous pairing in zebrafish spermatocytes. PLoS Genet. 16, e1008640. doi:10.1371/journal.pgen.1008640
Tan, C. H., Lee, T. C., Weeraratne, S. D., Korzh, V., Lim, T. M., and Gong, Z. (2002). ziwi, the zebrafish homologue of the Drosophila piwi: co-localization with vasa at the embryonic genital ridge and gonad-specific expression in the adults. Gene Expr. Patterns 2, 257–260. doi:10.1016/s1567-133x(02)00052-2
Tang, Q., Iyer, S., Lobbardi, R., Moore, J. C., Chen, H., Lareau, C., et al. (2017). Dissecting hematopoietic and renal cell heterogeneity in adult zebrafish at single-cell resolution using RNA sequencing. J. Exp. Med. 214, 2875–2887. doi:10.1084/jem.20170976
Taylor, J. S., Braasch, I., Frickey, T., Meyer, A., and Van De Peer, Y. (2003). Genome duplication, a trait shared by 22000 species of ray-finned fish. Genome Res. 13, 382–390. doi:10.1101/gr.640303
Tenugu, S., Pranoty, A., Mamta, S., and Senthilkumaran, B. (2021). Development and organisation of gonadal steroidogenesis in bony fishes - a review. Aquac. Fish. 6, 223–246. doi:10.1016/j.aaf.2020.09.004
Terao, M., Ogawa, Y., Takada, S., Kajitani, R., Okuno, M., Mochimaru, Y., et al. (2022). Turnover of mammal sex chromosomes in the Sry-deficient Amami spiny rat is due to male-specific upregulation of Sox9. Proc. Natl. Acad. Sci. U. S. A. 119, e2211574119. doi:10.1073/pnas.2211574119
Tokarz, J., Moller, G., Hrabe De Angelis, M., and Adamski, J. (2015). Steroids in teleost fishes: a functional point of view. Steroids 103, 123–144. doi:10.1016/j.steroids.2015.06.011
Tong, S. K., Hsu, H. J., and Chung, B. C. (2010). Zebrafish monosex population reveals female dominance in sex determination and earliest events of gonad differentiation. Dev. Biol. 344, 849–856. doi:10.1016/j.ydbio.2010.05.515
Torraca, V., Masud, S., Spaink, H. P., and Meijer, A. H. (2014). Macrophage-pathogen interactions in infectious diseases: new therapeutic insights from the zebrafish host model. Dis. Model Mech. 7, 785–797. doi:10.1242/dmm.015594
Turmel, P., Dufresne, J., Hermo, L., Smith, C. E., Penuela, S., Laird, D. W., et al. (2011). Characterization of pannexin1 and pannexin3 and their regulation by androgens in the male reproductive tract of the adult rat. Mol. Reprod. Dev. 78, 124–138. doi:10.1002/mrd.21280
Uchida, D., Yamashita, M., Kitano, T., and Iguchi, T. (2002). Oocyte apoptosis during the transition from ovary-like tissue to testes during sex differentiation of juvenile zebrafish. J. Exp. Biol. 205, 711–718. doi:10.1242/jeb.205.6.711
Valdivieso, A., Wilson, C. A., Amores, A., Da Silva Rodrigues, M., Nobrega, R. H., Ribas, L., et al. (2022). Environmentally-induced sex reversal in fish with chromosomal vs. polygenic sex determination Environ. Res. 213, 113549. doi:10.1016/j.envres.2022.113549
Villa, N., Walker, L., Lindsell, C. E., Gasson, J., Iruela-Arispe, M. L., and Weinmaster, G. (2001). Vascular expression of Notch pathway receptors and ligands is restricted to arterial vessels. Mech. Dev. 108, 161–164. doi:10.1016/s0925-4773(01)00469-5
Von Hofsten, J., Jones, I., Karlsson, J., and Olsson, P. E. (2001). Developmental expression patterns of FTZ-F1 homologues in zebrafish (Danio rerio). Gen. Comp. Endocrinol. 121, 146–155. doi:10.1006/gcen.2000.7582
Walker, C., and Streisinger, G. (1983). Induction of mutations by gamma-rays in pregonial germ cells of zebrafish embryos. Genetics 103, 125–136. doi:10.1093/genetics/103.1.125
Walker-Durchanek, R. C. (1980). Induction of germ line mutations by irradiation of zebrafish embryos. Masters, University of Oregon, Eugene OR USA.
Wang, J. H., Nichogiannopoulou, A., Wu, L., Sun, L., Sharpe, A. H., Bigby, M., et al. (1996). Selective defects in the development of the fetal and adult lymphoid system in mice with an Ikaros null mutation. Immunity 5, 537–549. doi:10.1016/s1074-7613(00)80269-1
Wang, X. G., Bartfai, R., Sleptsova-Freidrich, I., and Orban, L. (2007). The timing and extent of ‘juvenile ovary’ phase are highly variable during zebrafish testis differentiation. J. Fish Biol. 70, 33–44. doi:10.1111/j.1095-8649.2007.01363.x
Wang, X., Chen, S., Zhang, W., Ren, Y., Zhang, Q., and Peng, G. (2017). Dissection of larval zebrafish gonadal tissue. J. Vis. Exp., 55294. doi:10.3791/55294
Wang, Y., Ye, D., Zhang, F., Zhang, R., Zhu, J., Wang, H., et al. (2022). Cyp11a2 is essential for oocyte development and spermatogonial stem cell differentiation in zebrafish. Endocrinology 163, bqab258. doi:10.1210/endocr/bqab258
Wang, Z., Miura, N., Bonelli, A., Mole, P., Carlesso, N., Olson, D. P., et al. (2002). Receptor tyrosine kinase, EphB4 (HTK), accelerates differentiation of select human hematopoietic cells. Blood 99, 2740–2747. doi:10.1182/blood.v99.8.2740
Wattrus, S. J., and Zon, L. I. (2021). Blood in the water: recent uses of zebrafish to study myeloid biology. Curr. Opin. Hematol. 28, 43–49. doi:10.1097/MOH.0000000000000627
Webster, K. A., Schach, U., Ordaz, A., Steinfeld, J. S., Draper, B. W., and Siegfried, K. R. (2017). Dmrt1 is necessary for male sexual development in zebrafish. Dev. Biol. 422, 33–46. doi:10.1016/j.ydbio.2016.12.008
Weidinger, G., Stebler, J., Slanchev, K., Dumstrei, K., Wise, C., Lovell-Badge, R., et al. (2003). Dead end, a novel vertebrate germ plasm component, is required for zebrafish primordial germ cell migration and survival. Curr. Biol. 13, 1429–1434. doi:10.1016/s0960-9822(03)00537-2
Westerfield, M. (1993). The zebrafish book: a guide for the laboratory use of zebrafish (Brachydanio rerio). Eugene, OR: M. Westerfield.
White, R. T., and Allen, R. A. (1951). An improved clinical microtome for sectioning frozen tissue. Stain Technol. 26, 137–138. doi:10.3109/10520295109113195
Willett, C. E., Kawasaki, H., Amemiya, C. T., Lin, S., and Steiner, L. A. (2001). Ikaros expression as a marker for lymphoid progenitors during zebrafish development. Dev. Dyn. 222, 694–698. doi:10.1002/dvdy.1223
Wilson, C. A., High, S. K., Mccluskey, B. M., Amores, A., Yan, Y. L., Titus, T. A., et al. (2014). Wild sex in zebrafish: loss of the natural sex determinant in domesticated strains. Genetics 198, 1291–1308. doi:10.1534/genetics.114.169284
Wilson, C. A., and Postlethwait, J. H. (2023). A maternal-to-zygotic-transition gene block on the zebrafish sex chromosome. bioRxiv, 2023.12.06.570431. doi:10.1101/2023.12.06.570431
Winandy, S., Wu, P., and Georgopoulos, K. (1995). A dominant mutation in the Ikaros gene leads to rapid development of leukemia and lymphoma. Cell 83, 289–299. doi:10.1016/0092-8674(95)90170-1
Wolf, K., Hu, H., Isaji, T., and Dardik, A. (2019). Molecular identity of arteries, veins, and lymphatics. J. Vasc. Surg. 69, 253–262. doi:10.1016/j.jvs.2018.06.195
Wong, Q. W., Sun, M. A., Lau, S. W., Parsania, C., Zhou, S., Zhong, S., et al. (2018). Identification and characterization of a specific 13-miRNA expression signature during follicle activation in the zebrafish ovary. Biol. Reprod. 98, 42–53. doi:10.1093/biolre/iox160
Wu, K., Song, W., Zhang, Z., and Ge, W. (2020). Disruption of dmrt1 rescues the all-male phenotype of the cyp19a1a mutant in zebrafish - a novel insight into the roles of aromatase/estrogens in gonadal differentiation and early folliculogenesis. Development 147, dev182758. doi:10.1242/dev.182758
Wyrwoll, M. J., Gaasbeek, C. M., Golubickaite, I., Stakaitis, R., Oud, M. S., Nagirnaja, L., et al. (2022). The piRNA-pathway factor FKBP6 is essential for spermatogenesis but dispensable for control of meiotic LINE-1 expression in humans. Am. J. Hum. Genet. 109, 1850–1866. doi:10.1016/j.ajhg.2022.09.002
Xavier, C. P., Eichinger, L., Fernandez, M. P., Morgan, R. O., and Clemen, C. S. (2008). Evolutionary and functional diversity of coronin proteins. Subcell. Biochem. 48, 98–109. doi:10.1007/978-0-387-09595-0_9
Xie, Y., Huang, D., Chu, L., Liu, Y., Sun, X., Li, J., et al. (2021). Igf3 is essential for ovary differentiation in zebrafish†. Biol. Reprod. 104, 589–601. doi:10.1093/biolre/ioaa218
Xiol, J., Cora, E., Koglgruber, R., Chuma, S., Subramanian, S., Hosokawa, M., et al. (2012). A role for Fkbp6 and the chaperone machinery in piRNA amplification and transposon silencing. Mol. Cell 47, 970–979. doi:10.1016/j.molcel.2012.07.019
Yan, Y. L., Desvignes, T., Bremiller, R., Wilson, C., Dillon, D., High, S., et al. (2017). Gonadal soma controls ovarian follicle proliferation through Gsdf in zebrafish. Dev. Dyn. 246, 925–945. doi:10.1002/dvdy.24579
Yan, Y.-L., Titus, T., Desvignes, T., Bremiller, R., Batzel, P., Sydes, J., et al. (2020). A fish with no sex: gonadal and adrenal functions partition between zebrafish NR5A1 co-orthologs. GENETICS/2020/303676.
Yan, Y. L., Willoughby, J., Liu, D., Crump, J. G., Wilson, C., Miller, C. T., et al. (2005). A pair of Sox: distinct and overlapping functions of zebrafish sox9 co-orthologs in craniofacial and pectoral fin development. Development 132, 1069–1083. doi:10.1242/dev.01674
Yang, Y. J., Wang, Y., Li, Z., Zhou, L., and Gui, J. F. (2017). Sequential, divergent, and cooperative requirements of Foxl2a and Foxl2b in ovary development and maintenance of zebrafish. Genetics 205, 1551–1572. doi:10.1534/genetics.116.199133
Yin, Y., Tang, H., Liu, Y., Chen, Y., Li, G., Liu, X., et al. (2017). Targeted disruption of aromatase reveals dual functions of cyp19a1a during sex differentiation in zebrafish. Endocrinology 158, 3030–3041. doi:10.1210/en.2016-1865
Yoder, J. A., Turner, P. M., Wright, P. D., Wittamer, V., Bertrand, J. Y., Traver, D., et al. (2010). Developmental and tissue-specific expression of NITRs. Immunogenetics 62, 117–122. doi:10.1007/s00251-009-0416-5
Yoon, C., Kawakami, K., and Hopkins, N. (1997). Zebrafish vasa homologue RNA is localized to the cleavage planes of 2- and 4-cell-stage embryos and is expressed in the primordial germ cells. Development 124, 3157–3165. doi:10.1242/dev.124.16.3157
You, L. R., Lin, F. J., Lee, C. T., Demayo, F. J., Tsai, M. J., and Tsai, S. Y. (2005). Suppression of Notch signalling by the COUP-TFII transcription factor regulates vein identity. Nature 435, 98–104. doi:10.1038/nature03511
Young, M. D., and Behjati, S. (2020). SoupX removes ambient RNA contamination from droplet-based single-cell RNA sequencing data. Gigascience 9, giaa151. doi:10.1093/gigascience/giaa151
Yu, G., Zhang, D., Liu, W., Wang, J., Liu, X., Zhou, C., et al. (2018). Zebrafish androgen receptor is required for spermatogenesis and maintenance of ovarian function. Oncotarget 9, 24320–24334. doi:10.18632/oncotarget.24407
Zakrzewska, A., Cui, C., Stockhammer, O. W., Benard, E. L., Spaink, H. P., and Meijer, A. H. (2010). Macrophage-specific gene functions in Spi1-directed innate immunity. Blood 116, e1–e11. doi:10.1182/blood-2010-01-262873
Zhai, G., Shu, T., Xia, Y., Lu, Y., Shang, G., Jin, X., et al. (2018). Characterization of sexual trait development in cyp17a1-deficient zebrafish. Endocrinology 159, 3549–3562. doi:10.1210/en.2018-00551
Zhai, Y., Zhao, C., Geng, R., Wu, K., Yuan, M., Ai, N., et al. (2022). Phenotypical rescue of Bmp15 deficiency by mutation of inhibin a (inha) provides novel clues to how Bmp15 controls zebrafish folliculogenesis. bioRxiv. preprint. doi:10.1101/2022.12.30.522301
Zhang, X., Guan, G., Li, M., Zhu, F., Liu, Q., Naruse, K., et al. (2016). Autosomal gsdf acts as a male sex initiator in the fish medaka. Sci. Rep. 6, 19738. doi:10.1038/srep19738
Zhao, C., Zhai, Y., Geng, R., Wu, K., Song, W., Ai, N., et al. (2022). Genetic analysis of activin/inhibin β subunits in zebrafish development and reproduction. PLoS Genet. 18, e1010523. doi:10.1371/journal.pgen.1010523
Zheng, G. X., Terry, J. M., Belgrader, P., Ryvkin, P., Bent, Z. W., Wilson, R., et al. (2017). Massively parallel digital transcriptional profiling of single cells. Nat. Commun. 8, 14049. doi:10.1038/ncomms14049
Zhu, B., Pardeshi, L., Chen, Y., and Ge, W. (2018). Transcriptomic analysis for differentially expressed genes in ovarian follicle activation in the zebrafish. Front. Endocrinol. (Lausanne) 9, 593. doi:10.3389/fendo.2018.00593
Keywords: sex determination, gonad development, scRNA-seq, juvenile ovary, bipotential gonad, single cell transcriptomics, interstitial/stroma cells, hematopoietic cells
Citation: Wilson CA, Batzel P and Postlethwait JH (2024) Direct male development in chromosomally ZZ zebrafish. Front. Cell Dev. Biol. 12:1362228. doi: 10.3389/fcell.2024.1362228
Received: 27 December 2023; Accepted: 20 February 2024;
Published: 11 March 2024.
Edited by:
Talia L. Hatkevich, Duke University, United StatesReviewed by:
Yong Zhu, East Carolina University, United StatesCopyright © 2024 Wilson, Batzel and Postlethwait. This is an open-access article distributed under the terms of the Creative Commons Attribution License (CC BY). The use, distribution or reproduction in other forums is permitted, provided the original author(s) and the copyright owner(s) are credited and that the original publication in this journal is cited, in accordance with accepted academic practice. No use, distribution or reproduction is permitted which does not comply with these terms.
*Correspondence: John H. Postlethwait, anBvc3RsZUB1b3JlZ29uLmVkdQ==
Disclaimer: All claims expressed in this article are solely those of the authors and do not necessarily represent those of their affiliated organizations, or those of the publisher, the editors and the reviewers. Any product that may be evaluated in this article or claim that may be made by its manufacturer is not guaranteed or endorsed by the publisher.
Research integrity at Frontiers
Learn more about the work of our research integrity team to safeguard the quality of each article we publish.