- 1Department of Embryology, Institute of Developmental Biology and Biomedical Sciences, Faculty of Biology, University of Warsaw, Warsaw, Poland
- 2Department of Molecular Biology and Genetics, Faculty of Science, University of South Bohemia in České Budějovice, České Budějovice, Czechia
Assisted Reproductive Technologies (ART) have revolutionized infertility treatment and animal breeding, but their success largely depends on selecting high-quality oocytes for fertilization and embryos for transfer. During preimplantation development, embryos undergo complex morphogenetic processes, such as compaction and cavitation, driven by cellular forces dependent on cytoskeletal dynamics and cell-cell interactions. These processes are pivotal in dictating an embryo’s capacity to implant and progress to full-term development. Hence, a comprehensive grasp of the biomechanical attributes characterizing healthy oocytes and embryos is essential for selecting those with higher developmental potential. Various noninvasive techniques have emerged as valuable tools for assessing biomechanical properties without disturbing the oocyte or embryo physiological state, including morphokinetics, analysis of cytoplasmic movement velocity, or quantification of cortical tension and elasticity using microaspiration. By shedding light on the cytoskeletal processes involved in chromosome segregation, cytokinesis, cellular trafficking, and cell adhesion, underlying oogenesis, and embryonic development, this review explores the significance of embryo biomechanics in ART and its potential implications for improving clinical IVF outcomes, offering valuable insights and research directions to enhance oocyte and embryo selection procedures.
1 Introduction
Infertility has been recognized by the World Health Organization (WHO) as a disease and a global public health issue since 2013, following 2 decades of research into its personal and social consequences suffered by those affected (Fidler, 1999; Daar and Merali, 2002; Inhorn et al., 2002; Macaluso et al., 2010; Lemoine and Ravitsky, 2013; Rouchou, 2013; Gimenes et al., 2014; Nik Hazlina et al., 2022). It is estimated that it affects around 10% of couples of reproductive age (Vander Borght and Wyns, 2018), and even 15% of all women (Sadecki et al., 2022).
Assisted Reproductive Technologies (ART), especially in vitro fertilization (IVF), have become one of the most important procedures for treating infertility. Although there have been great advancements in IVF procedures over the years, the live birth rate per ART cycle remains low, especially for patients in advanced maternal age. While women below 35 may be expecting, depending on the reporting institution, about 33%/40%/18% success rate, women over 40 face only about 29%/15%/9% chance to give birth (according to Human Fertilisation & Embryology Authority [HEFA], 2021; Society for Assisted Reproductive Technology [SART], 2022; European Society of Human Reproduction and Embryology [ESHRE], 2023, respectively). Consequently, many couples must undergo several IVF cycles to succeed. This brings additional health risks for women, resulting in their psychological and emotional distress (especially in societies where infertility and ART are stigmatized), and further limits ART accessibility due to economic barriers (Daar and Merali, 2002; Cui, 2010; Macaluso et al., 2010; Rouchou, 2013; Nik Hazlina et al., 2022).
Additionally, IVF is an important method in livestock breeding programs (Silber et al., 2013; Sirard, 2018), and the production of phenotypically valuable livestock (Hansen, 2014), which has been a steadily growing area of commerce (Blondin, 2017; Moore and Hasler, 2017; Sanches et al., 2019; Viana, 2019). IVF is also used as a means of overcoming the significant challenges of managing small, isolated populations in zoos (Herrick, 2019) and the preservation of endangered species (Saragusty et al., 2016; Hildebrandt et al., 2018; Kochan et al., 2019; Lanyon and Burgess, 2019).
IVF is increasingly often accompanied by other procedures, such as in vitro oocyte maturation and cryopreservation of gametes and embryos. Around 25% of female chemotherapy-treated patients, before the age of 30, develop acute ovarian failure or premature menopause (Letourneau et al., 2012) and the risk reaches 40% for women under 40 and more than 80% for women over 40 (Rosenberg and Partridge, 2013). A strategy to preserve fertility in certain groups of female cancer patients is the cryopreservation of ovarian follicles ahead of the oncological treatment and in vitro maturation of oocytes before fertilization after recovery. This approach may also be applied to women with polycystic ovary syndrome, a group of patients with a high risk of ovarian hyperstimulation syndrome (Walls et al., 2015). Both oocyte and sperm cryopreservation are a fertility conservation option for transgender individuals undergoing hormone replacement therapy and genital reconstructive surgery (De Roo et al., 2016) and serve as an efficient banking method of gametes and embryos for infertility patients (Di Santo et al., 2012; Liang and Motan, 2016) and donors (Lindheim and Klock, 2018; Mignini Renzini et al., 2021). In vitro maturation and cryopreservation are also widely used tools in assisted reproduction of domestic and endangered animals (Gandolfi and Brevini, 2010; Hildebrandt et al., 2018; Sjunnesson, 2020).
The efficiency of IVF can be raised by transferring multiple embryos in a single cycle, but it can result in multiple pregnancies and, as a consequence, in serious health complications for mothers and their offspring (Ombelet et al., 2005; Skora and Frankfurter, 2012). Many clinics have therefore introduced elective single embryo transfers (eSET), according to guidelines of good clinical practice. Consequently, scientists and the medical industry are urged to develop novel and reliable methods to select high-quality embryos for transfer. Thus, protocols for noninvasive assessment of embryo competence are a valuable addition to the IVF procedures. Furthermore, the evaluation of oocyte quality serves the purpose of selecting the most suitable oocytes for fertilization. This becomes especially vital when legal restrictions limit the number of eggs that can be fertilized (e.g., six in Poland, unless specific medical conditions or age criteria are met). Reliable evaluation processes allow embryologists to personalize IVF treatments for each patient, including considerations such as the logistics of oocyte cryobanking and helping to manage patient expectations. Equally significant, the outcome of oocyte evaluation can yield supplementary insights that prove valuable in assessing the quality of the resulting embryos.
Plenty of methods for oocyte and embryo selection have been previously proposed (Patrizio et al., 2007; Rienzi et al., 2011; Ajduk and Zernicka-Goetz, 2013; Anagnostopoulou et al., 2022), but their adaptation into a clinical setting has proved challenging, either due to conflicting results or time, personnel, and financial constraints (Ajduk and Zernicka-Goetz, 2013). Current methods used in clinics are primarily based on the assessment of oocyte or embryo morphology, often combined with time-lapse imaging providing extra information on cellular divisions and morphogenetic events occurring during embryo preimplantation development (so called morphokinetics). However, morphology assessment of oocytes is often more informative than predictive (Nikiforov et al., 2022), and in embryos—it allows for excluding low-quality specimens, but not necessarily for indicating those of the highest viability (Gardner and Balaban, 2016). Moreover, this approach is prone to intra- and interobserver bias (Paternot et al., 2009; Bormann et al., 2020). Recently, artificial intelligence algorithms have been explored as a means of enhancing these methods (Zaninovic and Rosenwaks, 2020). However, some scholars point out that this approach still requires proper standardization of methodology (Kragh and Karstoft, 2021). Another technique applied in the evaluation of embryos is preimplantation genetic testing (PGT; Madero et al., 2023). Preimplantation genetic testing for monogenic gene defects (PGT-M) is a well-established method for selecting embryos devoid of disease-related mutations. However, the efficiency and accuracy of preimplantation genetic testing for aneuploidy (PGT-A) and preimplantation genetic testing for structural rearrangements (PGT-SR) remains limited, due to embryonic mosaicism (Popovic et al., 2020; Giuliano et al., 2023). Moreover, all types of preimplantation genetic testing are highly invasive, requiring biopsy of embryonic cells (as a source of genetic material for analysis). Furthermore, PGT-A is controversial due to the lack of unambiguous evidence to support its use (Mastenbroek et al., 2021).
As quality assessment protocols still require improvement, numerous novel methods of quality assessment have been proposed in recent years. One is the metabolic profiling of the embryos, which is achieved by the chemical analysis of spent culture media (Nagy et al., 2009; Zhao et al., 2013; Salmerón et al., 2021). Another technique, fluorescent lifetime imaging microscopy (FLIM) uses the differences in the exponential decay rate of the photon emission of autofluorescent coenzymes NAD(P)H and FAD2+ and allows for quantification of their concentration and thus energy metabolism of the embryo (Ma et al., 2019; Venturas et al., 2022; Venturas et al., 2023). This technique, however, uses UV light to excite autofluorescence, raising concerns about its invasiveness. Finally, it is also possible to analyze the chemical composition of oocytes and embryos using coherent anti-Stokes Raman scattering (CARS; Bogliolo et al., 2013; Davidson et al., 2013; Bradley et al., 2016; Jasensky et al., 2016; Ishigaki et al., 2017; Rusciano et al., 2017; Arena et al., 2021). CARS detects the vibrational spectra of biomolecules, depending on the mass of the atoms constituting the molecule and the strength of their respective bonds (Robert, 2009). Although not particularly efficient in identifying proteins, CARS provides a reliable quantitative analysis of lipids (Evans and Xie, 2008; Zumbusch et al., 2013).
Recently, the application of biomechanical quality assessment in ART has been a growing area of research as well. Biomechanical properties of oocytes and embryos reflect the functionality of key cellular components, including cytoskeleton and intracellular junctions. Therefore, examination of the oocyte/embryo biomechanics may provide novel insights into the quality of those intracellular structures, and, in consequence, improve oocyte/embryo evaluation protocols. Many invasive approaches to the analysis of the biomechanical properties of cells have been proposed. Structure of biomechanically relevant cellular components may be studied with fluorescent probes and confocal microscopy (Kölle et al., 2009). On the other hand, confocal microscopy, together with special nanomechanical chips, can be used to assess the intracellular pressure (Gómez-Martínez et al., 2013). The chip comprises of a mechanical sensor and an optical reference area created by two parallel reflecting membranes, separated by a vacuum gap. Waves can only pass through the reference area when they are in resonance with it. By analyzing the reflected light’s intensity, the system can quantify the pressure-induced membrane deflection. Another interesting method is the implementation of magnetically responsive ferrofluid microdroplets that enable highly precise measurements of mechanical properties, such as viscosity in tissues and embryos (Campàs, 2016; Serwane et al., 2017). The viscosity of tissue affects the movement and deformation of the microdroplets under the influence of the magnetic field, allowing for precise quantitative measurements of mechanical properties. While these approaches have shown promise in research, their application in ART is limited due to concerns about their potential to disrupt natural developmental processes. Noninvasive techniques described in this review (Table 1) represent an alternative, yet promising, avenue for gaining deeper insights into the biomechanical aspects of oocytes and embryos during ART, offering valuable contributions to improving clinical outcomes and reproductive health.
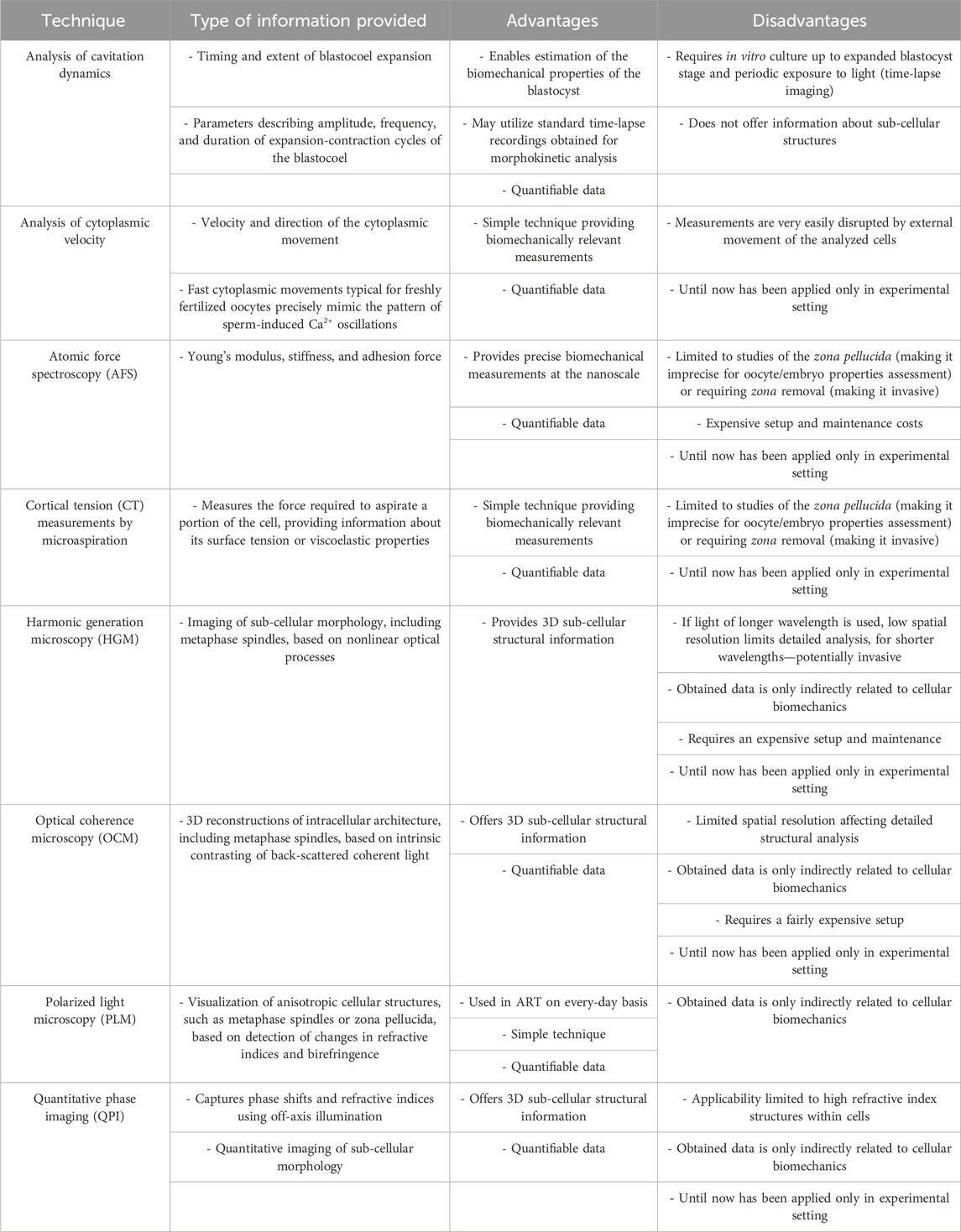
TABLE 1. Advantages and limitations of noninvasive techniques used in assessment of biomechanical properties of oocytes and embryos.
2 Cytoskeletal functions, dynamics, and alternations in oocytes
The developmental capabilities of mammalian embryos are largely determined by the oocyte cargo (Stitzel and Seydoux, 2007; Li et al., 2010). Although fragmentation of DNA or other deterioration of sperm can diminish an embryo’s potency, the oocyte contributes the vast majority of the cytoplasmic contents: cytoskeleton components essential for a multitude of inter- and intracellular processes, mitochondria providing energy to the embryo, lipid droplets supplying metabolic reserves, and maternal mRNA and proteins accumulated during oocyte growth, required as a guiding template before embryonic genome activation. Identifying features of a high-quality oocyte can therefore facilitate ART procedures.
Studies have shown that the biomechanical properties of mammalian oocytes reflect their developmental competence (Ebner et al., 2003; Liu et al., 2010). Whilst these properties stem partially from the zona pellucida’s mechanical characteristics, they predominantly depend on cellular biomechanics. The zona may harden as a result of cortical granule exocytosis at fertilization (the main element of the polyspermy block; Murayama et al., 2006; Shen et al., 2019), but also, as shown in the mouse, during premature cortical granule exocytosis in the in vitro matured and vitrified oocytes (Carroll et al., 1990; Ducibella et al., 1990). Premature zona hardening inhibits in vitro fertilization via gamete co-incubation, as sperm cannot penetrate the zona (Carroll et al., 1990). Conversely, cellular mechanics, i.e., elastic (ability to resist deformation) and plastic (ability to undergo permanent deformation) behavior of cells, reflect their cytoskeletal functionality (Larson et al., 2010; Chaigne et al., 2013; Chaigne et al., 2015; Mackenzie et al., 2016). The cytoskeleton plays a key role in the segregation of chromosomes, cytokinesis, and cellular trafficking, each of which is important for cell cycle progression (Tang, 2012; D’Avino et al., 2015; Prosser and Pelletier, 2017). Microtubules build metaphase spindles, while actin and myosin are required for the spindle positioning and formation of the cytokinetic contraction ring (Schuh and Ellenberg, 2008). Disturbances in the function of these components can result in aneuploidy, which has detrimental effects on future embryonic development, especially when first appearing in meiosis (McCoy et al., 2023). A functioning actomyosin cytoskeleton is also invaluable during so-called cytoplasmic maturation, a process concurrent with the meiotic maturation of oocytes. Research conducted predominantly on mouse oocytes indicates that during cytoplasmic maturation relocation of organelles occurs. Mitochondria move from the perinuclear region towards the cell periphery (Dalton and Carroll, 2013), the Golgi apparatus fragments and shifts to the center of the gamete (Moreno et al., 2002), and the endoplasmic reticulum gathers in the cortical region (Mehlmann et al., 1995; FitzHarris et al., 2007). These changes in organelle distribution depend on intact actin filaments (Dalton and Carroll, 2013). Simultaneously, dynamic changes in the cytoskeleton itself occur. In mouse oocytes, metaphase I spindle migration is supported by an ARP2/3-dependent thickening of the cortical F-actin meshwork, nucleated by formin-2 (Leader et al., 2002; Dumont et al., 2007; Schuh and Ellenberg, 2008) and Spire 1/2 (Pfender et al., 2011). As the cortical F-actin thickens, myosin-II is excluded from the cortex, leading to its softening (Chaigne et al., 2013). These events are regulated by phosphorylated (active) myosin-II regulatory light chain and phosphorylated ezrin-radixin-moesin complex and coordinated temporally by the MOS-MAPK pathway (Larson et al., 2010; Chaigne et al., 2013; Chaigne et al., 2015). Cortical tension (CT) resulting from the force generated by the actomyosin cytoskeleton must be strictly regulated to allow normal spindle migration and positioning: too low or too high CT both lead to spindle anomalies (Chaigne et al., 2013; Chaigne et al., 2015). As shown for human and mouse oocytes, actin filaments may also infiltrate the meiotic spindle and regulate the correct alignment and segregation of the chromosomes (Mogessie and Schuh, 2017).
Interestingly, Larson and others show that there are some discrepancies in cytoskeleton modifications during meiotic maturation between in vivo- and in vitro-matured mouse oocytes (Larson et al., 2010). This could be linked to lower developmental capabilities of in vitro matured human metaphase II oocytes (Jurema and Nogueira, 2006). The cellular cytoskeleton is often damaged during freezing and thawing procedures as well (Hosu et al., 2008; Hendriks et al., 2015). Various cytoskeletal elements can also be affected by postovulatory aging (i.e., the extended period between ovulation and fertilization; reviewed in Miao et al., 2009; Takahashi et al., 2013); in particular, actin distribution and myosin functionality (McGinnis et al., 2015; Mackenzie et al., 2016). Some authors have even suggested that reduced myosin light chain kinase activity in aged mouse oocytes is linked to their susceptibility to parthenogenetic activation, potentially by dysregulation of membrane ion channels (McGinnis et al., 2015; Mackenzie et al., 2016).
Cytoskeletal damage can be caused by reactive oxygen species (ROS; Lord et al., 2013). In somatic cells, proteins damaged by oxidation, such as carbonylated or glycated proteins, accumulate with age and in several pathological states (Stadtman, 1992; Levine, 2002). These proteins are rendered inactive and tend to form large aggregates in the cytoplasm. Similarly, the mammalian germline accumulates these dysfunctional proteins (Hernebring et al., 2006; Haucke et al., 2014), but they are eliminated to some extent during embryo development (Hernebring et al., 2006). Oocytes may carry varying amounts of advanced glycation end (AGE) products and carbonylated or otherwise modified proteins, depending on maternal age and overall female health. Notably, actin is a common target for carbonylation (Aksenov et al., 2001; Soreghan et al., 2003). Mouse oocytes subjected to postovulatory aging or obtained from females of advanced reproductive age feature increased ROS concentrations (Szpila et al., 2019; Czajkowska and Ajduk, 2023). Similarly, vitrification is known to cause oxidative stress in murine, porcine, and human oocytes (reviewed in Tatone et al., 2010). Some studies report that increased ROS levels can be also observed during murine and bovine oocyte in vitro maturation (Morado et al., 2009; Xie et al., 2016). It is feasible that carbonylation/glycation of proteins occurs not only in oocytes obtained from old females but also in oocytes otherwise subjected to oxidative stress (Berlett and Stadtman, 1997), including postovulatory aging and vitrification. It can be speculated that these oocytes’ cytoskeletal dysfunction could be, at least in part, caused by the oxidation of cytoskeletal proteins (Mihalas et al., 2018).
Cytoskeletal functionality is intricately linked to the successful progression of meiosis, making it a pivotal factor in oocyte and early embryo development. As a result, the assessment of cytoskeleton quality in these cells, reflected among others by their biomechanical properties, emerges as a valuable and predictive indicator, offering critical insights into the outcomes of IVF procedures, thus enhancing the understanding and potential success of ART.
3 Quality assessment of oocytes based on cytoskeletal and biomechanical properties
Some biomarkers indicative of the biomechanical properties, e.g., zona pellucida and metaphase spindle, can be observed using polarized light microscopy (PLM; Oldenbourg, 2013; Ajduk and Szkulmowski, 2019). Importantly, zona birefringence also indicates its ability to participate in the acrosome reaction and ability to undergo proper polyspermy block, whereas shape of the metaphase spindle can be associated with the ploidy and the maturation status of the oocyte (Caamaño et al., 2010; Montag et al., 2013; Omidi et al., 2017).
Alas, even PLM does not provide detailed information on inner cell architecture, nor does it have a high in-depth resolution. These limitations might be overcome by harmonic generation microscopy (HGM; Hsieh et al., 2008; Thayil et al., 2011), which obtains contrasts by the sample’s ability to emit photons with half the wavelength of incident light. HGM has been shown to obtain 3D images of microtubules in the spindle (Yu et al., 2014; Sanchez et al., 2019) and myosin heavy-chain B (Mohler et al., 2003). However, HGM is beset by the choice between the imaging depth when using longer excitation wavelengths, which are less harmful to biomaterials, and high spatial resolution when using shorter but more invasive wavelengths (Aghigh et al., 2023). These limitations are shared by optical coherence microscopy (OCM), which can be used for metaphase spindle visualization (Karnowski et al., 2017). In addition, both methods typically require a complex and expensive setup, which might not be readily available in prospective fertility clinics. A simpler solution can perhaps be found in quantitative phase imaging (QPI) techniques, such as holographic microscopy or interferometric phase imaging, which can provide quantitative information about cell morphology based on 3D refractive index distribution (Nguyen et al., 2022). These methods can be used to study cytoskeletal organization and dynamics (Bon et al., 2014), however, to date QPI has mostly been employed to study membranous organelles such as mitochondria, which have a higher refractive index (Sandoz et al., 2019; Salucci et al., 2020).
Cellular mechanics depend on cytoskeletal function, thus probing oocytes or embryos for their biomechanical characteristics can provide insight into their developmental potential. One such method is the analysis of cytoplasmic velocity. Cytoplasmic velocity measurement combines time-lapse imaging with particle image velocimetry (PIV) analysis. PIV is an algorithm frequently used in fluid dynamics that follows the displacement of bright and dark pixel patterns in consecutive images to establish the velocity and direction of the fluid (cytoplasm) movement (Westerweel, 1997; Figure 1). Studies have demonstrated that cytoplasmic velocity monitored at the time of fertilization reflects the capacity of mouse zygotes to correctly complete preimplantation and full-term development (Ajduk et al., 2011). These movements can also be observed in human zygotes (Swann et al., 2012). Fast directional cytoplasmic movements (the so-called “speed-peaks”) correspond to rhythmic actomyosin-mediated spasms, coincident with fertilization-induced Ca2+ oscillations (Ajduk et al., 2011). The mean basal speed represents the average velocity of cytoplasmic movement between speed-peaks, thereby providing information on the functionality of the zygote actomyosin cytoskeleton. For example, a relative decrease in mean basal speed is concurrent with both depolymerization and overstabilization of actin filaments and inhibition of myosin (Ajduk et al., 2011). Moreover, the mean basal speed in freshly fertilized mouse oocytes correlates with the length of the second embryonic cell cycle, the percentage of cells with fragmented nuclei, and the percentage of primitive endoderm cells in the blastocyst (Milewski et al., 2018). The basal velocity of cytoplasmic movement in unfertilized (metaphase II) oocytes is slower than in their fertilized counterparts (Ajduk et al., 2011). Both maternal and postovulatory types of aging are detrimental to the actomyosin cytoskeleton of mammalian, including human, metaphase II oocytes (Pickering et al., 1988; Sun et al., 2012; McGinnis et al., 2015; Mackenzie et al., 2016; Dunkley et al., 2022), which might influence the cytoplasmic dynamics, resulting in lower basal speed in aged oocytes.
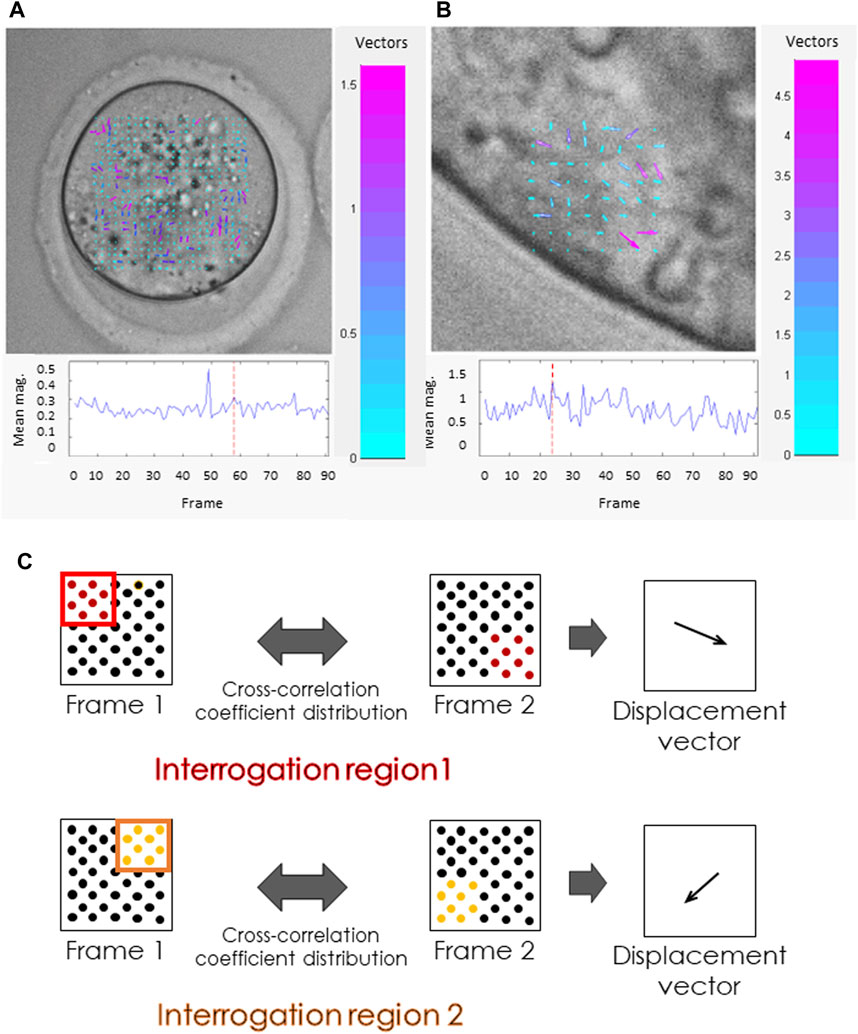
FIGURE 1. Cytoplasmic movement velocity (CMV) assessment by Particle Image Velocimetry (PIV). (A, B) Images from the PIV software used by some of the authors (Ajduk et al., 2011). PIV analysis was conducted for high-resolution time-lapse images of (A) mouse metaphase II oocyte, (B) polar trophectoderm cell in a mouse blastocyst. Both the length and the color of the vectors visible inside the cells reflect the cytoplasmic velocity. Cyan represents the slowest-moving vectors. Magenta represents the fastest-moving vectors. The graphs (below) show the mean cytoplasmic velocity in the analyzed region over time. The direction of the vectors indicates the direction of cytoplasm displacement between frames. (C) Schematic representation of the PIV algorithm. The algorithm divides the images into small interrogation windows, identifying the pattern of pixels in each window, and calculating the displacement of particles between frames. This information is used to generate a map of velocity vectors representing the cytoplasmic flow and to calculate mean cytoplasmic velocity.
Interestingly, the mean basal cytoplasmic speed is also indicative of immature oocyte (so-called GV oocyte) quality (Bui et al., 2017). There are two populations of GV oocytes, which are known to have distinct developmental capabilities: oocytes with surrounded nucleolus (or SN oocytes) that have already transcribed all necessary RNAs and are transcriptionally inactive, and oocytes with non-surrounded nucleolus (or NSN oocytes) that are still transcribing (reviewed in: Tan et al., 2009). These two types of GV oocytes differ in terms of the basal speed at various stages of in vitro maturation (Bui et al., 2017).
Assessment of oocyte quality based on the monitoring of actomyosin cytoskeleton-mediated cell mechanics can also utilize techniques such as micropipette aspiration (Mackenzie et al., 2016; Yanez et al., 2016) and indentation (Liu et al., 2012). Applying negative pressure through a micropipette or positive force through a microlever, results in the deformation of the cell allowing for the calculation of the cell’s physical properties, such as CT or elasticity (Figure 2). CT reflects the biochemical and structural features of the oocyte cortex (Larson et al., 2010; Chaigne et al., 2013; Chaigne et al., 2015; Mackenzie et al., 2016) and zona pellucida (Khalilian et al., 2010; Shen et al., 2019). Studies on mouse oocytes devoid of zona pellucida have shown that CT decreases six-fold during maturation, then increases about 1.6-fold after fertilization (Larson et al., 2010). Also, mature mouse oocytes are polarized, with CT differing 2.5-fold between the stiff cortex over the meiotic spindle (the amicrovillar domain) and the softer, opposing cortex, where the sperm binds (microvillar domain; Larson et al., 2010). The purpose of this asymmetry is unclear. However, in vitro matured oocytes have reduced tension in the amicrovillar domain (Larson et al., 2010). Viscoelastic equilibrium in the cortex is essential to achieve asymmetric cytokinesis (Chaigne et al., 2013; Chaigne et al., 2015). This equilibrium is characteristic of a viable oocyte (Yanez et al., 2016). Too elastic or too plastic cortex results in anomalous spindle migration (Chaigne et al., 2013; Chaigne et al., 2015) and lowered developmental competence, likely due to less effective cortical granule release at fertilization, which could lead to polyspermy (Yanez et al., 2016). CT is also reduced in both maternally (Liu et al., 2012) and postovulatory-aged oocytes (Mackenzie et al., 2016). These differences in viscoelastic properties, hence cytoskeletal properties, offer a valuable tool for quality assessment. Methods for testing oocyte and embryo cytoskeletal properties by CT analysis, however, are relatively time-consuming and labor-intensive. Additionally, most protocols presented to date feature the removal of the zona, which may negatively affect overall embryo development (Fan et al., 2022).
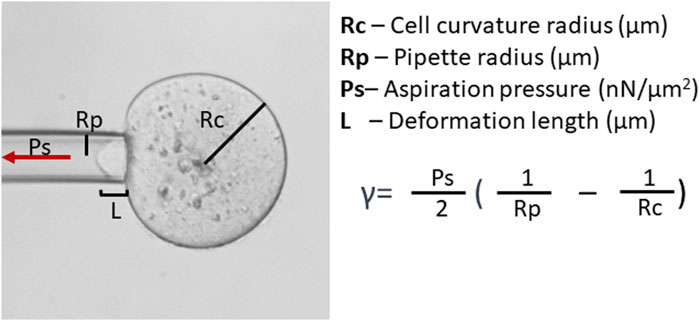
FIGURE 2. Cortical tension (CT, γ) analysis. CT analysis can be conducted by micropipette aspiration. Assessment of CT requires the measurement of cell curvature radius and aspiration pressure (Ps) when the deformation length (L) becomes equal to the micropipette radius (Rp) and utilizes the Young–Laplace equation.
Another method to measure surface forces is atomic force spectroscopy. Here, a sharp tip attached to a cantilever runs over the surface of the sample. The deflection of the cantilever across the sample surface is measured using a laser beam, which is reflected onto a photodetector (Figure 3). The amount of deflection is used to calculate the force exerted on the tip by the sample’s surface (Viljoen et al., 2021). In its noninvasive form, in a similar manner to CT measurements, atomic force spectroscopy is limited to the studies of the zona pellucida (Boccaccio et al., 2012; Andolfi et al., 2016; Battistella et al., 2022).
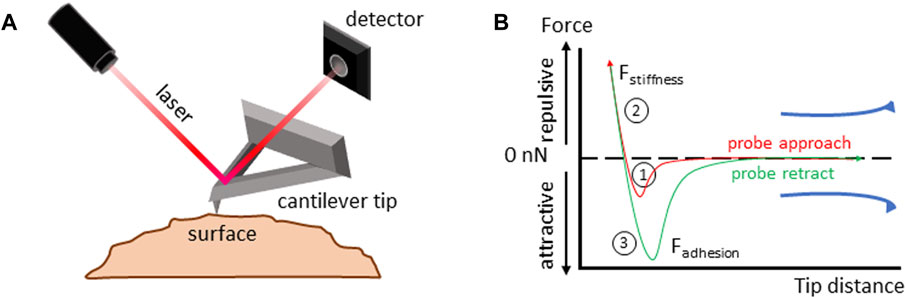
FIGURE 3. Atomic force spectroscopy. (A) Atomic force spectroscopy setup diagram. Details in the main text. (B) Force-distance curve analysis to measure the viscoelastic properties of a material. 1) The cantilever tip approaches the sample until it makes contact, and the force at the interaction between the tip and the sample is measured; 2) the tip is further compressed into the sample, deforming its surface, the forces acting on the tip during compression are recorded, allowing for calculation of the surface stiffness; 3) the tip is retracted from the sample surface. Forces acting on the tip as it moves away from the sample are measured allowing for calculation of surface adhesion.
4 Molecular basis of embryo biomechanics
The success of in vitro fertilization procedures is also contingent on sperm quality and conditions of embryo culture (Chapuis et al., 2017; Colaco and Sakkas, 2018; Consensus Group, 2020). Various molecular mechanisms reflected in changes in biomechanical properties are at play before the blastocyst, the last stage of mammalian preimplantation development, is formed. A blastocyst is built of an inner cell mass (ICM) and an outer trophectoderm (TE) epithelial layer. As the blastocyst expands, ICM cells differentiate into two lineages: the centrally located epiblast (EPI) and the primitive endoderm (PE), also called hypoblast, adjoined to the blastocyst cavity (blastocoel). The TE, on the other hand, differentiates into the polar TE surrounding the ICM and the mural TE surrounding the blastocyst cavity. The ICM that will give rise to the embryo proper (resulting from the EPI) as well as additional extraembryonic membranes (derived from the PE), and the TE will participate in embryo implantation in the uterus and form the future placenta.
Preimplantation development consists of multiple mitotic divisions, which are highly dependent on actin networks (Chaigne et al., 2016). Importantly, zygotic genome activation (ZGA) is connected with maternal protein degradation (Toralova et al., 2020), and considerable changes in cytoskeletal makeup. During embryonic development, the configuration of cells and cell lineages is shaped by the contractile nature of the actomyosin cortex (Murrell et al., 2015; Coravos et al., 2017; Özgüç and Maître, 2020). These cortical contractile forces are essential for the formation of the cleavage furrow during cytokinesis (Fujiwara and Pollard, 1976; Straight et al., 2003; Yamamoto et al., 2021), the movement of cells during migration and maintenance of appropriate cell positioning (Eddy et al., 2000; Tsai et al., 2019), and the withdrawal of cellular blebs (Charras et al., 2006; Taneja and Burnette, 2019). Moreover, changes in actomyosin contractility drive processes such as apical constriction (Martin et al., 2009; Solon et al., 2009) and the restructuring of cell-cell contacts (Bertet et al., 2004; Maître et al., 2012).
Significant biomechanical alterations occur particularly during the compaction of preimplantation embryos, a process that occurs at the 8-cell stage in mice, 16-cell stage in humans, and 32-cell stage in rabbits and cattle (Płusa and Piliszek, 2020). Compaction is accompanied by intra-cellular polarization of blastomeres along the apical-basal axis. In pre-compacted mouse embryos, actomyosin is uniformly distributed in the cellular cortex, whereas during compaction, it accumulates gradually in the apical, contact-free region, and becomes excluded from the cell-cell contact sites (Zhu et al., 2017). Moreover, as the mouse embryo undergoes compaction, the cell adhesion protein, epithelial cadherin (E-cadherin), translocates and becomes phosphorylated at the cell-cell contact sites (Winkel et al., 1990). Additionally, ezrin, a protein responsible for establishing and maintaining microvilli, undergoes phosphorylation and relocates to the contact-free regions of the membranes (Louvet et al., 1996).
Translocation of actomyosin occurring during compaction leads to the progressive increase of the CT on the contact-free interface of the blastomeres. At the same time, junctional E-cadherin keeps actomyosin contractility low at the cell-cell contacts (Maître et al., 2015). As a result, the inner and outer cells of a compacted embryo differ in contractility (Maître et al., 2016). These changes in cellular adhesion and CT are crucial not only for mouse embryo compaction but also for the internalization of the cells that will later form the inner cell mass (ICM; Samarage et al., 2015; Maître et al., 2016).
The biomechanical properties of preimplantation embryos are dynamically regulated by various molecular pathways. The initiation and maintenance of symmetry breaking in a compacting mouse embryo depend on the activity of PLC-PKC signaling (Zhu et al., 2017). On the other hand, formin regulates contractility in preimplantation morphogenesis (Özgüç et al., 2022). The actin nucleator ARP2/3 is critical for blastomere cytokinesis, and its inhibition leads to the failure of blastocyst formation. The RHO-associated coiled-coil-containing protein kinase (ROCK), associated with cell migration and adhesion, vesicular trafficking, and cytoskeletal dynamics, is involved in apicobasal cell polarity proteins maintenance (Marikawa and Alarcon, 2018), and the regulation of angiomotin (AMOT) localization (Mihajlović and Bruce, 2016). AMOT is a scaffolding protein involved in cell-cell junctions. As an activator of the Hippo pathway, it is crucial for the specification of TE and ICM cells in the mouse blastocysts (reviewed in: Mihajlović and Bruce, 2017). Interestingly, recently published data on mouse and human embryos indicate that there is a tight link between actomyosin contractility, lamin-A, a component of the nuclear lamina, and AMOT stability (Skory et al., 2023). Nuclear lamina is linked to the blastomere cortex via an F-actin network. As actomyosin contractility increases during embryo development, lamin-A levels rise as well. However, in cells that underwent internalization in compacted embryos and lost their apical, actomyosin-rich domain, lamin-A becomes downregulated. This leads to the relocalization of actin nucleators from the nucleus to the cytoplasm and an increase in cytoplasmic F-actin. In consequence, AMOT is stabilized and YAP, a key transcription coregulator involved in cell lineage differentiation, undergoes phosphorylation (Hippo pathway activated). Active Hippo pathway directs inner cells towards the ICM fate. By contrast, in outer cells, lamin-A levels are upregulated. This destabilizes AMOT and prevents YAP phosphorylation (Hippo pathway inactive), promoting TE cell fate (Skory et al., 2023).
A critical and final event in preimplantation development is the formation of a blastocyst cavity, in which the radial symmetry of the embryo is broken. In mouse, the apicobasal polarity of outer TE permits the formation of an osmotic gradient that draws water from the external environment via the apical compartment of blastomeres (Eckert et al., 2004; Madan et al., 2007; Moriwaki et al., 2007) into the basal intercellular regions sealed by tight junctions (Zenker et al., 2018). Such fluid accumulation is driven by the secretion of cytoplasmic actin-coated vesicles into the intercellular space (Ryan et al., 2019). Hundreds of microlumens form throughout the mouse embryo between cell-cell contacts by hydraulic fracturing, directed by cadherin reorganization (Dumortier et al., 2019). Some microlumens also display enrichment of the apical marker, phosphorylated ezrin-radixin-moesin complex (Ryan et al., 2019). Microlumens show a swelling phase followed by a siphoning of all the fluid to a single cavity, guided by actomyosin contractions (Dumortier et al., 2019; Schliffka et al., 2023).
Existing data clearly indicate that TE functionality depends on the quality of its cytoskeleton and intracellular junctions that determine the epithelial character of this layer. Additionally, the expression and activity of proteins transporting ions, thus allowing for osmotic gradient formation and consequent cavitation (Bazer et al., 2009; Posfai et al., 2019), also play an important role in TE functioning. These factors are associated with the biomechanical properties of TE cells. It has been shown that inhibition of Na+/K+ pumps or claudins in tight junctions affects the CT of TE cells (Chan et al., 2019). Decreased TE cell tension also coincides with the disassembly of vinculin from tight junctions and disrupts tight junction seal integrity (Chan et al., 2019). Vinculin has been shown to regulate traction force transmission via myosin contractility-dependent adhesion (Dumbauld et al., 2013). Additionally, actin filament remodeling, required to form a sealed TE epithelium, is tension-sensitive (Zenker et al., 2018). Interestingly, it has been shown in mouse embryos that mechanical stretching, typical for TE cells during cavity expansion, facilitates Cdx2 expression (Watanabe et al., 2017). Cdx2 expression is a prerequisite for TE function (although not always for early stages of TE differentiation) in mice, humans, and domestic animals (reviewed in: Piliszek and Madeja, 2018; Posfai et al., 2019). Notably, keratins have been recently proven to be another regulator of TE fate in both mouse and human embryos: they enhance apical polarity and Cdx2 expression in outer cells (Lim et al., 2020). Although keratin knockouts display trophoblast fragility, placental bleeding, and lethality after implantation (Baribault et al., 1993; Hesse et al., 2000; Tamai et al., 2000), depletion of keratins 8 and/or 18 (i.e., variants that are the most abundant in preimplantation embryos; Lu et al., 2005) does not lead to severe phenotypes up to blastocyst stage, either in mice or in cattle (Goossens et al., 2010; Lim et al., 2020). Interestingly, in mouse embryos, keratin 8/18-knockdown cells display a reduced nuclear expression of YAP (required for Cdx2 transcription) and CDX2 itself in TE cells.
Immediately prior to implantation, blastocysts hatch from the zona pellucida, exposing the TE, which attaches to the endometrial epithelium of the uterus. Blastocyst attachment initiates a complex cascade of events that lead to the implantation and development of a placenta. Importantly, implantation requires functional TE (Bazer et al., 2009; Aplin and Ruane, 2017; Posfai et al., 2019) and failure in implantation is the main source of reproduction loss in mammals, including humans and cattle (Aplin and Ruane, 2017; D’Occhio et al., 2020).
In summary, it is clear that the biomechanical properties of embryos are highly associated with their developmental potential. By gaining insights into how biomechanical factors influence the formation and quality of embryos, and their subsequent implantation, researchers can set forth noninvasive and robust methods of assessing such properties which could help ART practitioners make more informed decisions when selecting embryos for transfer.
5 Quality assessment based on embryo biomechanical properties
Time-lapse recordings used for classical morphogenetic analysis of preimplantation embryos, if only covering cavitation and blastocysts expansion events, may be applied for the assessment of the biomechanical properties of TE. Mouse blastocysts with inhibited actomyosin contractility, Na+/K+ pumps, or perturbed tight junctions displayed a slower expansion rate (Chan et al., 2019). Moreover, it has been shown in mouse embryos that experimentally reduced cavity size and hydraulic pressure inside the cavity are associated with the increased number of ICM cells and perturbed specification and spatial separation of ICM lineages (EPI and PE; Chan et al., 2019; Ryan et al., 2019). Analysis of the extent of blastocyst expansion has been shown to be a predictor of pregnancy success in human embryos (Du et al., 2016), and expansion kinetics have been related to a human embryo’s ploidy: euploid embryos expanded faster than aneuploid embryos (Huang et al., 2019). The rate of blastocoel re-expansion in frozen-thawed embryos also has been associated with pregnancy likelihood in both humans and domestic animals (Leoni et al., 2008; Yin et al., 2016; Ebner et al., 2017; Lin et al., 2017; Zhao et al., 2019). Interestingly, the rate of human blastocyst re-expansion correlates with the number of TE cells (Iwasawa et al., 2019). This observation has also been confirmed in mouse embryos: smaller blastocyst size is associated with slower blastocoel expansion (Chan et al., 2019).
Blastocyst cavity volume tends to oscillate during the expansion period, undergoing contraction-expansion cycles (Figure 4). This feature seems to be an intrinsic property of the blastocysts in all animals examined, including humans (Niimura, 2003; Huang et al., 2016), and is associated with waves of mitotic divisions in TE and increasing TE cortical tension (Chan et al., 2019). However, the interpretation of these contraction-expansion cycles in terms of embryo quality is ambiguous. It has been reported that human blastocysts that transiently collapsed have less potential to give rise to pregnancy (Marcos et al., 2015; Gazzo et al., 2020; Sciorio et al., 2020) and are characterized by higher aneuploidy odds (Gazzo et al., 2020). Others have claimed that the occurrence of blastocyst collapse is not an independent predictor of reduced live birth rate (Bodri et al., 2016). It has been shown that mouse blastocysts exhibiting stronger contractions of the lumen are less likely to hatch (Shimoda et al., 2016). Moreover, unusually frequent blastocoel contractions in mouse embryos can be caused by aberrant intracellular junctions in TE (Togashi et al., 2015). While the interpretation of contraction-expansion cycles in blastocysts remains inconclusive, monitoring cavitation dynamics might provide useful insights into the quality of blastocyst cytoskeleton as well as the functionality of intracellular junctions and proteins involved in filling blastocoel with fluid. Further research is essential to validate the efficacy of such an approach and its potential for application in ART.
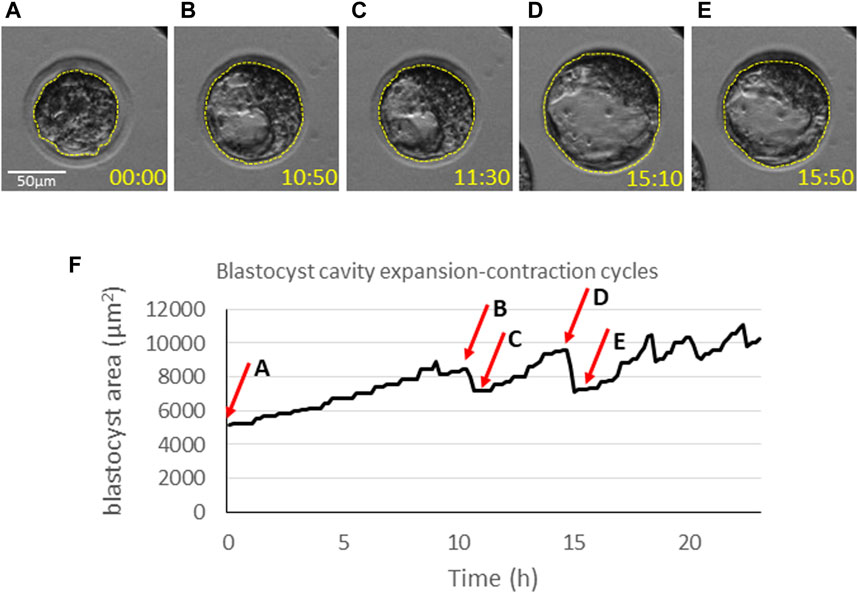
FIGURE 4. Dynamics of blastocyst cavitation. The equatorial area of blastocysts (dashed line) is measured at (A) the onset of cavitation, (B) the time-point of maximum expansion just before the first contraction, (C) the last phase of the first contraction, (D) the time-point of maximum expansion before the next contraction, (E) the last phase of the contraction. Time points (hh:mm) indicate the time of imaging and correspond to the graph below (F). (F) A graph representing oscillations of the blastocyst size over time. The arrows indicate the time points corresponding to the measurements in (A–E).
PIV-based analysis of cytoplasmic movement in blastomeres is yet another method to provide insights into the biomechanical properties of embryos. Cytoplasmic motion reflects the reorganization of cytoskeletal elements that are key for the movement of signaling vesicles, and, as described above, for both compaction and cavitation (Coumailleau et al., 2009; Derivery et al., 2015; Zenker et al., 2017). The reorganization of the actinomyosin cytoskeleton can be observed with PIV analysis (Özgüç et al., 2022), and holds promise as a means to monitor and assess the dynamics of these crucial phases in preimplantation embryo development. Additionally, the analysis of cytoplasmic velocity with the PIV algorithm could be applied in TE cells (Figure 1), where it is associated with the functionality of keratin cytoskeleton, crucial for subsequent implantation: keratin-depleted TE cells have a more mobile (less “rigid”) cytoplasm (Lim et al., 2020).
Keratin 8/18 depletion also increased the apical curvature of TE cells, which is indicative of lower apical tension (Lim et al., 2020). Therefore, microaspiration or indentation methods may help in detecting embryos with keratin defects. Interestingly, in mice, CT of TE cells is associated with the embryo size at least at the early blastocyst stage: smaller embryos (obtained by dissection of the whole embryo in halves or quarters) display higher CT (Chan et al., 2019). Therefore, measuring the CT of cells could provide information on the functionality of the cellular cytoskeleton, intracellular junctions, and ion pumps required for cavitation in TE cells (Chan et al., 2019; Lim et al., 2020).
6 Summary
This review offers a comprehensive overview of the molecular mechanisms that underlie the biomechanical properties of oocytes and embryos, along with the potential noninvasive techniques for assessing those properties. Our intent is to bridge the gap between scientific research and practical applications, providing a background for the suitability of the proposed techniques in the context of ART.
As highlighted here, cytoskeletal proteins play a pivotal role in determining the developmental potential of oocytes and embryos. Cytoskeleton, particularly its actomyosin component, governs key intracellular processes such as cell cycle progression or organelle trafficking, and intercellular processes such as compaction and cavitation. Elucidating biomechanical biomarkers characterizing a high-quality oocyte and properly developing preimplantation embryo with functional TE could provide a novel approach for evaluating quality beyond conventional morphological assessment. We draw attention to promising techniques, such as analysis of cytoplasmic movement or cavitation-related morphokinetic parameters as well as cortical tension and elasticity measurements, which may offer novel insights into oocyte and embryo viability.
The integration of biomechanical assessments into ART could refine currently used procedures. Conventional methods of oocyte and embryo evaluation based solely on morphological criteria have limitations in predicting implantation and pregnancy rates accurately. Biomechanical assessment provides a more comprehensive understanding of oocyte and embryo quality, potentially enhancing the selection of oocytes and embryos with higher developmental potential. While some questions and ambiguities persist, and further research and validation are imperative to establish the reliability and effectiveness of these techniques in a clinical setting, ongoing research in cellular biomechanics holds great potential for enhancing the success rates of fertility treatments.
Author contributions
MF: Project administration, Writing–original draft, Writing–review and editing, Data curation. RC: Writing–review and editing. AW: Writing–review and editing, Data curation. AWB: Writing–review and editing. AA: Supervision, Writing–review and editing, Data curation.
Funding
The author(s) declare financial support was received for the research, authorship, and/or publication of this article. Funding from National Science Centre, Poland: MF was supported by Preludium grant (UMO-2020/37/N/NZ5/02733; awarded to MF) and Opus grant (UMO-2017/27/B/NZ5/00405; awarded to AA); AW was supported by Opus grant (UMO-2020/39/B/NZ5/02962; awarded to AA); AA was supported by both Opus grants. Funding from Czech Science Foundation: RC and AWB were supported by GACR Standard (21-03305S) grant awarded to AWB.
Acknowledgments
We would like to thank Ewa Kosyl for her help with preparing the figure on cavitation dynamics.
Conflict of interest
AA is a co-inventor in the patent “Methods for predicting mammalian embryo viability” (patent no. US 9 410 939 B2) on the application of cytoplasmic movement analysis in evaluation of embryo quality.
The remaining authors declare that the research was conducted in the absence of any commercial or financial relationships that could be construed as a potential conflict of interest.
Publisher’s note
All claims expressed in this article are solely those of the authors and do not necessarily represent those of their affiliated organizations, or those of the publisher, the editors and the reviewers. Any product that may be evaluated in this article, or claim that may be made by its manufacturer, is not guaranteed or endorsed by the publisher.
Abbreviations
ART, assisted reproductive technologies; CT, cortical tension; EPI, epiblast; HGM, harmonic generation microscopy, ICM, inner cell mass; IVF, in vitro fertilization; OCM, optical coherence microscopy; PE, primitive endoderm; PIV, particle image velocimetry; PLM, polarized light microscopy; QPI, quantitative phase imaging; ROS, reactive oxygen species; TE, trophectoderm.
References
Aghigh, A., Bancelin, S., Rivard, M., Pinsard, M., Ibrahim, H., and Légaré, F. (2023). Second harmonic generation microscopy: a powerful tool for bio-imaging. Biophys. Rev. 15, 43–70. doi:10.1007/S12551-022-01041-6
Ajduk, A., Ilozue, T., Windsor, S., Yu, Y., Seres, K. B., Bomphrey, R. J., et al. (2011). Rhythmic actomyosin-driven contractions induced by sperm entry predict mammalian embryo viability. Nat. Commun. 2, 417–7. doi:10.1038/ncomms1424
Ajduk, A., and Szkulmowski, M. (2019). Light microscopy of mammalian gametes and embryos: methods and applications. Int. J. Dev. Biol. 63, 235–244. doi:10.1387/ijdb.180300aa
Ajduk, A., and Zernicka-Goetz, M. (2013). Quality control of embryo development. Mol. Asp. Med. 34, 903–918. doi:10.1016/j.mam.2013.03.001
Aksenov, M. Y., Aksenova, M. V., Butterfield, D. A., Geddes, J. W., and Markesbery, W. R. (2001). Protein oxidation in the brain in Alzheimer’s disease. Neuroscience 103, 373–383. doi:10.1016/S0306-4522(00)00580-7
Anagnostopoulou, C., Maldonado Rosas, I., Singh, N., Gugnani, N., Chockalingham, A., Singh, K., et al. (2022). Oocyte quality and embryo selection strategies: a review for the embryologists, by the embryologists. Panminerva Med. 64, 171–184. doi:10.23736/S0031-0808.22.04680-8
Andolfi, L., Masiero, E., Giolo, E., Martinelli, M., Luppi, S., Dal Zilio, S., et al. (2016). Investigating the mechanical properties of zona pellucida of whole human oocytes by atomic force spectroscopy. Integr. Biol. 8, 886–893. doi:10.1039/C6IB00044D
Aplin, J. D., and Ruane, P. T. (2017). Embryo-epithelium interactions during implantation at a glance. J. Cell Sci. 130, 15–22. doi:10.1242/jcs.175943
Arena, R., Bisogno, S., Gasior, Ł., Rudnicka, J., Bernhardt, L., Haaf, T., et al. (2021). Lipid droplets in mammalian eggs are utilized during embryonic diapause. Proc. Natl. Acad. Sci. U.S.A. 118, e2018362118. doi:10.1073/pnas.2018362118
Baribault, H., Price, J., Miyai, K., and Oshima, R. G. (1993). Mid-gestational lethality in mice lacking keratin 8. Genes Dev. 7, 1191–1202. doi:10.1101/gad.7.7a.1191
Battistella, A., Andolfi, L., Zanetti, M., Dal Zilio, S., Stebel, M., Ricci, G., et al. (2022). Atomic force spectroscopy-based essay to evaluate oocyte postovulatory aging. Bioeng. Transl. Med. 7, e10294. doi:10.1002/BTM2.10294
Bazer, F. W., Spencer, T. E., Johnson, G. A., Burghardt, R. C., and Wu, G. (2009). Comparative aspects of implantation. Reproduction 138, 195–209. doi:10.1530/REP-09-0158
Berlett, B. S., and Stadtman, E. R. (1997). Protein oxidation in aging, disease, and oxidative stress. J. Biol. Chem. 272, 20313–20316. doi:10.1074/jbc.272.33.20313
Bertet, C., Sulak, L., and Lecuit, T. (2004). Myosin-dependent junction remodelling controls planar cell intercalation and axis elongation. Nature 429, 667–671. doi:10.1038/nature02590
Blondin, P. (2017). Logistics of large scale commercial IVF embryo production. Reprod. Fertil. Dev. 29, 32–36. doi:10.1071/RD16317
Boccaccio, A., Frassanito, M. C., Lamberti, L., Brunelli, R., Maulucci, G., Monaci, M., et al. (2012). Nanoscale characterization of the biomechanical hardening of bovine zona pellucida. J. R. Soc. Interface 9, 2871–2882. doi:10.1098/RSIF.2012.0269
Bodri, D., Sugimoto, T., Yao Serna, J., Kawachiya, S., Kato, R., and Matsumoto, T. (2016). Blastocyst collapse is not an independent predictor of reduced live birth: a time-lapse study. Fertil. Steril. 105, 1476–1483. doi:10.1016/j.fertnstert.2016.02.014
Bogliolo, L., Murrone, O., Di Emidio, G., Piccinini, M., Ariu, F., Ledda, S., et al. (2013). Raman spectroscopy-based approach to detect aging-related oxidative damage in the mouse oocyte. J. Assist. Reprod. Genet. 30, 877–882. doi:10.1007/s10815-013-0046-6
Bon, P., Lécart, S., Fort, E., and Lévêque-Fort, S. (2014). Fast label-free cytoskeletal network imaging in living mammalian cells. Biophys. J. 106, 1588–1595. doi:10.1016/J.BPJ.2014.02.023
Bormann, C. L., Thirumalaraju, P., Kanakasabapathy, M. K., Kandula, H., Souter, I., Dimitriadis, I., et al. (2020). Consistency and objectivity of automated embryo assessments using deep neural networks. Fertil. Steril. 113, 781–787. doi:10.1016/j.fertnstert.2019.12.004
Bradley, J., Pope, I., Masia, F., Sanusi, R., Langbein, W., Swann, K., et al. (2016). Quantitative imaging of lipids in live mouse oocytes and early embryos using CARS microscopy. Development 143, 2238–2247. doi:10.1242/dev.129908
Bui, T. T. H., Belli, M., Fassina, L., Vigone, G., Merico, V., Garagna, S., et al. (2017). Cytoplasmic movement profiles of mouse surrounding nucleolus and not-surrounding nucleolus antral oocytes during meiotic resumption. Mol. Reprod. Dev. 84, 356–362. doi:10.1002/mrd.22788
Caamaño, J. N., Muñoz, M., Diez, C., and Gómez, E. (2010). Polarized light microscopy in mammalian oocytes. Reprod. Domest. Anim. 45, 49–56. doi:10.1111/j.1439-0531.2010.01621.x
Campàs, O. (2016). A toolbox to explore the mechanics of living embryonic tissues. Semin. Cell Dev. Biol. 55, 119–130. doi:10.1016/J.SEMCDB.2016.03.011
Carroll, J., Depypere, H., and Matthews, C. D. (1990). Freeze-thaw-induced changes of the zona pellucida explains decreased rates of fertilization in frozen-thawed mouse oocytes. J. Reprod. Fertil. 90, 547–553. doi:10.1530/jrf.0.0900547
Chaigne, A., Campillo, C., Gov, N. S., Voituriez, R., Azoury, J., Umaña-Diaz, C., et al. (2013). A soft cortex is essential for asymmetric spindle positioning in mouse oocytes. Nat. Cell Biol. 15, 958–966. doi:10.1038/ncb2799
Chaigne, A., Campillo, C., Gov, N. S., Voituriez, R., Sykes, C., Verlhac, M. H., et al. (2015). A narrow window of cortical tension guides asymmetric spindle positioning in the mouse oocyte. Nat. Commun. 6, 6027. doi:10.1038/ncomms7027
Chaigne, A., Campillo, C., Voituriez, R., Gov, N. S., Sykes, C., Verlhac, M. H., et al. (2016). F-actin mechanics control spindle centring in the mouse zygote. Nat. Commun. 7, 10253. doi:10.1038/ncomms10253
Chan, C. J., Costanzo, M., Ruiz-Herrero, T., Mönke, G., Petrie, R. J., Bergert, M., et al. (2019). Hydraulic control of mammalian embryo size and cell fate. Nature 571, 112–116. doi:10.1038/s41586-019-1309-x
Chapuis, A., Gala, A., Ferrières-Hoa, A., Mullet, T., Bringer-Deutsch, S., Vintejoux, E., et al. (2017). Sperm quality and paternal age: effect on blastocyst formation and pregnancy rates. Basic Clin. Androl. 27, 2. doi:10.1186/s12610-016-0045-4
Charras, G. T., Hu, C. K., Coughlin, M., and Mitchison, T. J. (2006). Reassembly of contractile actin cortex in cell blebs. J. Cell Biol. 175, 477–490. doi:10.1083/jcb.200602085
Colaco, S., and Sakkas, D. (2018). Paternal factors contributing to embryo quality. J. Assist. Reprod. Genet. 35, 1953–1968. doi:10.1007/s10815-018-1304-4
Consensus Group, C. (2020). ‘There is only one thing that is truly important in an IVF laboratory: everything’ Cairo Consensus Guidelines on IVF Culture Conditions. Reprod. Biomed. Online 40, 33–60. doi:10.1016/j.rbmo.2019.10.003
Coravos, J. S., Mason, F. M., and Martin, A. C. (2017). Actomyosin pulsing in tissue integrity maintenance during morphogenesis. Trends Cell Biol. 27, 276–283. doi:10.1016/j.tcb.2016.11.008
Coumailleau, F., Fürthauer, M., Knoblich, J. A., and González-Gaitán, M. (2009). Directional Delta and Notch trafficking in Sara endosomes during asymmetric cell division. Nature 458, 1051–1055. doi:10.1038/nature07854
Cui, W. (2010). Mother or nothing: the agony of infertility. Bull. World Health Organ. 88, 881–882. doi:10.2471/blt.10.011210
Czajkowska, K., and Ajduk, A. (2023). Mitochondrial activity and redox status in oocytes from old mice: the interplay between maternal and postovulatory aging. Theriogenology 204, 18–30. doi:10.1016/j.theriogenology.2023.03.022
Daar, A. S., and Merali, Z. (2002). “Infertility and social suffering: the case of ART in developing countries,” in “Medical, ethical and social aspects of assisted reproduction” held at WHO headquarters in Geneva, Switzerland 17–21 september 2001. Editors E. Vayena, P. J. Rowe, and D. P. Griffin (Geneva: World Health Organization), 15–21.
Dalton, C. M., and Carroll, J. (2013). Biased inheritance of mitochondria during asymmetric cell division in the mouse oocyte. J. Cell Sci. 126, 2955–2964. doi:10.1242/jcs.128744
Davidson, B., Murray, A. A., Elfick, A., and Spears, N. (2013). Raman micro-spectroscopy can Be used to investigate the developmental stage of the mouse oocyte. PLoS ONE 8, e67972. doi:10.1371/journal.pone.0067972
D’Avino, P. P., Giansanti, M. G., and Petronczki, M. (2015). Cytokinesis in animal cells. Cold Spring Harb. Perspect. Biol. 7, a015834. doi:10.1101/cshperspect.a015834
Derivery, E., Seum, C., Daeden, A., Loubéry, S., Holtzer, L., Jülicher, F., et al. (2015). Polarized endosome dynamics by spindle asymmetry during asymmetric cell division. Nature 528, 280–285. doi:10.1038/nature16443
De Roo, C., Tilleman, K., Tsjoen, G., and De Sutter, P. (2016). Fertility options in transgender people. Int. Rev. Psychiatry. 28, 112–119. doi:10.3109/09540261.2015.1084275
Di Santo, M., Tarozzi, N., Nadalini, M., and Borini, A. (2012). Human sperm cryopreservation: update on techniques, effect on DNA integrity, and implications for ART. Adv. Urol. 2012, 854837. doi:10.1155/2012/854837
D’Occhio, M. J., Campanile, G., Zicarelli, L., Visintin, J. A., and Baruselli, P. S. (2020). Adhesion molecules in gamete transport, fertilization, early embryonic development, and implantation—role in establishing a pregnancy in cattle: a review. Mol. Reprod. Dev. 87, 206–222. doi:10.1002/mrd.23312
Du, Q. Y., Wang, E. Y., Huang, Y., Guo, X. Y., Xiong, Y. J., Yu, Y. P., et al. (2016). Blastocoele expansion degree predicts live birth after single blastocyst transfer for fresh and vitrified/warmed single blastocyst transfer cycles. Fertil. Steril. 105, 910–919. doi:10.1016/j.fertnstert.2015.12.014
Ducibella, T., Kurasawa, S., Rangarajan, S., Kopf, G. S., and Schultz, R. M. (1990). Precocious loss of cortical granules during mouse oocyte meiotic maturation and correlation with an egg-induced modification of the zona pellucida. Dev. Biol. 137, 46–55. doi:10.1016/0012-1606(90)90006-5
Dumbauld, D. W., Lee, T. T., Singh, A., Scrimgeour, J., Gersbach, C. A., Zamir, E. A., et al. (2013). How vinculin regulates force transmission. Proc. Natl. Acad. Sci. U. S. A. 110, 9788–9793. doi:10.1073/PNAS.1216209110
Dumont, J., Million, K., Sunderland, K., Rassinier, P., Lim, H., Leader, B., et al. (2007). Formin-2 is required for spindle migration and for the late steps of cytokinesis in mouse oocytes. Dev. Biol. 301, 254–265. doi:10.1016/j.ydbio.2006.08.044
Dumortier, J. G., Le Verge-Serandour, M., Tortorelli, A. F., Mielke, A., De Plater, L., Turlier, H., et al. (2019). Hydraulic fracturing and active coarsening position the lumen of the mouse blastocyst. Sci. (80-. ) 365, 465–468. doi:10.1126/science.aaw7709
Dunkley, S., Scheffler, K., and Mogessie, B. (2022). Cytoskeletal form and function in mammalian oocytes and zygotes. Curr. Opin. Cell Biol. 75, 102073. doi:10.1016/J.CEB.2022.02.007
Ebner, T., Moser, M., Sommergruber, M., Puchner, M., Wiesinger, R., and Tews, G. (2003). Developmental competence of oocytes showing increased cytoplasmic viscosity. Hum. Reprod. 18, 1294–1298. doi:10.1093/humrep/deg232
Ebner, T., Oppelt, P., Radler, E., Allerstorfer, C., Habelsberger, A., Mayer, R. B., et al. (2017). Morphokinetics of vitrified and warmed blastocysts predicts implantation potential. J. Assist. Reprod. Genet. 34, 239–244. doi:10.1007/s10815-016-0855-5
Eckert, J. J., McCallum, A., Mears, A., Rumsby, M. G., Cameron, I. T., and Fleming, T. P. (2004). Specific PKC isoforms regulate blastocoel formation during mouse preimplantation development. Dev. Biol. 274, 384–401. doi:10.1016/J.YDBIO.2004.07.027
Eddy, R. J., Pierini, L. M., Matsumura, F., and Maxfield, F. R. (2000). Ca2+-dependent myosin II activation is required for uropod retraction during neutrophil migration. J. Cell Sci. 113, 1287–1298. doi:10.1242/jcs.113.7.1287
European Society of Human Reproduction and Embryology (ESHRE) (2023). ART in Europe, 2019: results generated from European registries by ESHRE. Available from: https://www.eshre.eu.
Evans, C. L., and Xie, X. S. (2008). Coherent anti-Stokes Raman scattering microscopy: chemical imaging for biology and medicine. Annu. Rev. Anal. Chem. 1, 883–909. doi:10.1146/annurev.anchem.1.031207.112754
Fan, W., Huang, T., Wu, T., Bai, H., Kawahara, M., and Takahashi, M. (2022). Zona pellucida removal by acid Tyrode’s solution affects pre- and post-implantation development and gene expression in mouse embryos†. Biol. Reprod. 107, 1228–1241. doi:10.1093/biolre/ioac155
Fidler, A. T., and Bernstein, J. (1999). Infertility: from a personal to a public health problem. Public Health Rep. 14, 494–511. doi:10.1093/phr/114.6.494
FitzHarris, G., Marangos, P., and Carroll, J. (2007). Changes in endoplasmic reticulum structure during mouse oocyte maturation are controlled by the cytoskeleton and cytoplasmic dynein. Dev. Biol. 305, 133–144. doi:10.1016/j.ydbio.2007.02.006
Fujiwara, K., and Pollard, T. D. (1976). Fluorescent antibody localization of myosin in the cytoplasm, cleavage furrow, and mitotic spindle of human cells. J. Cell Biol. 71, 848–875. doi:10.1083/jcb.71.3.848
Gandolfi, F., and Brevini, T. A. L. (2010). RFD Award Lecture 2009. in vitro maturation of farm animal oocytes: a useful tool for investigating the mechanisms leading to full-term development. Reprod. Fertil. Dev. 22, 495–507. doi:10.1071/RD09151
Gardner, D. K., and Balaban, B. (2016). Assessment of human embryo development using morphological criteria in an era of time-lapse, algorithms and ‘OMICS’: is looking good still important? Mol. Hum. Rep. 22, 704–718. doi:10.1093/molehr/gaw057
Gazzo, E., Peña, F., Valdéz, F., Chung, A., Velit, M., Ascenzo, M., et al. (2020). Blastocyst contractions are strongly related with aneuploidy, lower implantation rates, and slow-cleaving embryos: a time lapse study. J. Bras. Reprod. Assist. 24, 77–81. doi:10.5935/1518-0557.20190053
Gimenes, F., Souza, R. P., Bento, J. C., Teixeira, J. J. V., Maria-Engler, S. S., Bonini, M. G., et al. (2014). Male infertility: a public health issue caused by sexually transmitted pathogens. Nat. Rev. Urol. 11, 672–687. doi:10.1038/nrurol.2014.285
Giuliano, R., Maione, A., Vallefuoco, A., Sorrentino, U., and Zuccarello, D. (2023). Preimplantation genetic testing for genetic diseases: limits and review of current literature. Genes 14, 2095. doi:10.3390/genes14112095
Gómez-Martínez, R., Hernández-Pinto, A. M., Duch, M., Vázquez, P., Zinoviev, K., De La Rosa, E. J., et al. (2013). Silicon chips detect intracellular pressure changes in living cells. Nat. Nanotechnol. 8, 517–521. doi:10.1038/nnano.2013.118
Goossens, K., Tesfaye, D., Rings, F., Schellander, K., Hölker, M., Van Poucke, M., et al. (2010). Suppression of keratin 18 gene expression in bovine blastocysts by RNA interference. Reprod. Fertil. Dev. 22, 395–404. doi:10.1071/RD09080
Hansen, P. J. (2014). Current and future assisted reproductive technologies for mammalian farm animals. Adv. Exp. Med. Biol. 752, 1–22. doi:10.1007/978-1-4614-8887-3_1
Haucke, E., Santos, A. N., Simm, A., Henning, C., Glomb, M. A., Gürke, J., et al. (2014). Accumulation of advanced glycation end products in the rabbit blastocyst under maternal diabetes. Reproduction 148, 169–178. doi:10.1530/REP-14-0149
Hendriks, W. K., Roelen, B. A. J., Colenbrander, B., and Stout, T. A. E. (2015). Cellular damage suffered by equine embryos after exposure to cryoprotectants or cryopreservation by slow-freezing or vitrification. Equine Vet. J. 47, 701–707. doi:10.1111/evj.12341
Hernebring, M., Brolén, G., Aguilaniu, H., Semb, H., and Nyström, T. (2006). Elimination of damaged proteins during differentiation of embryonic stem cells. Proc. Natl. Acad. Sci. U. S. A. 103, 7700–7705. doi:10.1073/pnas.0510944103
Herrick, J. R. (2019). Assisted reproductive technologies for endangered species conservation: developing sophisticated protocols with limited access to animals with unique reproductive mechanisms. Biol. Reprod. 100, 1158–1170. doi:10.1093/biolre/ioz025
Hesse, M., Franz, T., Tamai, Y., Taketo, M. M., and Magin, T. M. (2000). Targeted deletion of keratins 18 and 19 leads to trophoblast fragility and early embryonic lethality. EMBO J. 19, 5060–5070. doi:10.1093/emboj/19.19.5060
Hildebrandt, T. B., Hermes, R., Colleoni, S., Diecke, S., Holtze, S., Renfree, M. B., et al. (2018). Embryos and embryonic stem cells from the white rhinoceros. Nat. Commun. 9, 2589. doi:10.1038/s41467-018-04959-2
Hosu, B. G., Mullen, S. F., Critser, J. K., and Forgacs, G. (2008). Reversible disassembly of the actin cytoskeleton improves the survival rate and developmental competence of cryopreserved mouse oocytes. PLoS One 3, e2787, e2787. doi:10.1371/journal.pone.0002787
Hsieh, C.-S., Chen, S.-U., Lee, Y.-W., Yang, Y.-S., and Sun, C.-K. (2008). Higher harmonic generation microscopy of in vitro cultured mammal oocytes and embryos. Opt. Express 16, 11574–11588. doi:10.1364/oe.16.011574
Huang, T. T., Huang, D. H., Ahn, H. J., Arnett, C., and Huang, C. T. (2019). Early blastocyst expansion in euploid and aneuploid human embryos: evidence for a non-invasive and quantitative marker for embryo selection. Reprod. Biomed. Online 39, 27–39. doi:10.1016/j.rbmo.2019.01.010
Huang, T. T. F., Chinn, K., Kosasa, T., Ahn, H. J., and Kessel, B. (2016). Morphokinetics of human blastocyst expansion in vitro. Reprod. Biomed. Online 33, 659–667. doi:10.1016/j.rbmo.2016.08.020
Human Fertilisation and Embryology Authority (HEFA) (2021). HFEA Fertility treatment 2019: trends and figures. Available from: https://www.hfea.gov.uk.
Inhorn, M. C., van Balen, F., Sandelowski, M., de Lacey, S., Thompson, C. M., Greil, A. L., et al. (2002). Infertility around the globe: new thinking on childlessness, gender, and reproductive technologies., infertility around the globe. Berkeley: University of California Press. doi:10.1525/9780520927810
Ishigaki, M., Hashimoto, K., Sato, H., and Ozaki, Y. (2017). Non-destructive monitoring of mouse embryo development and its qualitative evaluation at the molecular level using Raman spectroscopy. Sci. Rep. 7, 43942. doi:10.1038/srep43942
Iwasawa, T., Takahashi, K., Goto, M., Anzai, M., Shirasawa, H., Sato, W., et al. (2019). Human frozen-thawed blastocyst morphokinetics observed using time-lapse cinematography reflects the number of trophectoderm cells. PLoS One 14, e0210992. doi:10.1371/journal.pone.0210992
Jasensky, J., Boughton, A. P., Khmaladze, A., Ding, J., Zhang, C., Swain, J. E., et al. (2016). Live-cell quantification and comparison of mammalian oocyte cytosolic lipid content between species, during development, and in relation to body composition using nonlinear vibrational microscopy. Analyst 141, 4694–4706. doi:10.1039/c6an00629a
Jurema, M. W., and Nogueira, D. (2006). In vitro maturation of human oocytes for assisted reproduction. Fertil. Steril. 86, 1277–1291. doi:10.1016/j.fertnstert.2006.02.126
Karnowski, K., Ajduk, A., Wieloch, B., Tamborski, S., Krawiec, K., Wojtkowski, M., et al. (2017). Optical coherence microscopy as a novel, non-invasive method for the 4D live imaging of early mammalian embryos. Sci. Rep. 7, 4165. doi:10.1038/s41598-017-04220-8
Khalilian, M., Navidbakhsh, M., Valojerdi, M. R., Chizari, M., and Yazdi, P. E. (2010). Estimating Young’s modulus of zona pellucida by micropipette aspiration in combination with theoretical models of ovum. J. R. Soc. Interface 7, 687–694. doi:10.1098/rsif.2009.0380
Kochan, J., Niżański, W., Moreira, N., Cubas, Z. S., Nowak, A., Prochowska, S., et al. (2019). ARTs in wild felid conservation programmes in Poland and in the world. J. Vet. Res. 63, 457–464. doi:10.2478/jvetres-2019-0043
Kölle, S., Reese, S., and Kummer, W. (2009). New aspects of gamete transport, fertilization, and embryonic development in the oviduct gained by means of live cell imaging. Theriogenology 73, 786–795. doi:10.1016/j.theriogenology.2009.11.002
Kragh, M. F., and Karstoft, H. (2021). Embryo selection with artificial intelligence: how to evaluate and compare methods? J. Assist. Reprod. Genet. 38, 1675–1689. doi:10.1007/s10815-021-02254-6
Lanyon, J. M., and Burgess, E. A. (2019). “Reproductive science methods for wild, fully-marine mammals: current approaches and future applications,” in Advances in experimental medicine and Biology (New York LLC: Springer), 363–411. doi:10.1007/978-3-030-23633-5_13
Larson, S. M., Lee, H. J., Hung, P. H., Matthews, L. M., Robinson, D. N., and Evans, J. P. (2010). Cortical mechanics and meiosis II completion in mammalian oocytes are mediated by myosin-II and Ezrin-Radixin-Moesin (ERM) proteins. Mol. Biol. Cell 21, 3182–3192. doi:10.1091/mbc.E10-01-0066
Leader, B., Lim, H., Carabatsos, M. J., Harrington, A., Ecsedy, J., Pellman, D., et al. (2002). Formin-2, polyploidy, hypofertility and positioning of the meiotic spindle in mouse oocytes. Nat. Cell Biol. 4, 921–928. doi:10.1038/ncb880
Lemoine, M. E., and Ravitsky, V. (2013). Toward a public health approach to infertility: the ethical dimensions of infertility prevention. Public Health Ethics 6, 287–301. doi:10.1093/phe/pht026
Leoni, G. G., Berlinguer, F., Succu, S., Bebbere, D., Mossa, F., Madeddu, M., et al. (2008). A new selection criterion to assess good quality ovine blastocysts after vitrification and to predict their transfer into recipients. Mol. Reprod. Dev. 75, 373–382. doi:10.1002/mrd.20754
Letourneau, J. M., Ebbel, E. E., Katz, P. P., Oktay, K. H., McCulloch, C. E., Ai, W. Z., et al. (2012). Acute ovarian failure underestimates age-specific reproductive impairment for young women undergoing chemotherapy for cancer. Cancer 118, 1933–1939. doi:10.1002/cncr.26403
Levine, R. L. (2002). Carbonyl modified proteins in cellular regulation, aging, and disease. Free Radic. Biol. Med. 32, 790–796. doi:10.1016/S0891-5849(02)00765-7
Li, L., Zheng, P., and Dean, J. (2010). Maternal control of early mouse development. Development 137, 859–870. doi:10.1242/dev.039487
Liang, T., and Motan, T. (2016). Mature oocyte cryopreservation for fertility preservation. Adv. Exp. Med. Biol. 951, 155–161. doi:10.1007/978-3-319-45457-3_13
Lim, H. Y. G., Alvarez, Y. D., Gasnier, M., Wang, Y., Tetlak, P., Bissiere, S., et al. (2020). Keratins are asymmetrically inherited fate determinants in the mammalian embryo. Nature 585, 404–409. doi:10.1038/s41586-020-2647-4
Lin, R., Feng, G., Shu, J., Zhang, B., Zhou, H., Gan, X., et al. (2017). Blastocoele re-expansion time in vitrified–warmed cycles is a strong predictor of clinical pregnancy outcome. J. Obstet. Gynaecol. Res. 43, 689–695. doi:10.1111/jog.13257
Lindheim, S. R., and Klock, S. C. (2018). Oocyte donation: lessons from the past, directions for the future. Fertil. Steril. 110, 979–980. doi:10.1016/j.fertnstert.2018.09.019
Liu, X., Fernandes, R., Jurisicova, A., Casper, R., Sun, Yu, Liu, X., et al. (2010). In situ mechanical characterization of mouse oocytes using a cell holding device. Lab. Chip 10, 2154–2161. doi:10.1039/C004706F
Liu, X., Shi, J., Zong, Z., Wan, K. T., and Sun, Y. (2012). Elastic and viscoelastic characterization of mouse oocytes using micropipette indentation. Ann. Biomed. Eng. 40, 2122–2130. doi:10.1007/s10439-012-0595-3
Lord, T., Aitken, J. R., John Aitken, R., and Aitken, J. R. (2013). Oxidative stress and ageing of the post-ovulatory oocyte. Reproduction 146, 217–227. doi:10.1530/rep-13-0111
Louvet, S., Aghion, J., Santa-Maria, A., Mangeat, P., and Maro, B. (1996). Ezrin becomes restricted to outer cells following asymmetrical division in the preimplantation mouse embryo. Dev. Biol. 177, 568–579. doi:10.1006/dbio.1996.0186
Lu, H., Hesse, M., Peters, B., and Magin, T. M. (2005). Type II keratins precede type I keratins during early embryonic development. Eur. J. Cell Biol. 84, 709–718. doi:10.1016/j.ejcb.2005.04.001
Ma, N., Mochel, N. R., Pham, P. D., Yoo, T. Y., Cho, K. W. Y., and Digman, M. A. (2019). Label-free assessment of pre-implantation embryo quality by the Fluorescence Lifetime Imaging Microscopy (FLIM)-phasor approach. Sci. Rep. 9, 13206. doi:10.1038/s41598-019-48107-2
Macaluso, M., Wright-Schnapp, T. J., Chandra, A., Johnson, R., Satterwhite, C. L., Pulver, A., et al. (2010). A public health focus on infertility prevention, detection, and management. Fertil. Steril. 93, 16.e1–10. doi:10.1016/j.fertnstert.2008.09.046
Mackenzie, A. C. L., Kyle, D. D., McGinnis, L. A., Lee, H. J., Aldana, N., Robinson, D. N., et al. (2016). Cortical mechanics and myosin-II abnormalities associated with post-ovulatory aging: implications for functional defects in aged eggs. Mol. Hum. Reprod. 22, 397–409. doi:10.1093/molehr/gaw019
Madan, P., Rose, K., and Watson, A. J. (2007). Na/K-ATPase beta1 subunit expression is required for blastocyst formation and normal assembly of trophectoderm tight junction-associated proteins. J. Biol. Chem. 282, 12127–12134. doi:10.1074/JBC.M700696200
Madero, J. I., Manotas, M. C., García-Acero, M., López Cáceres, A., and López Jaimes, C. (2023). Preimplantation genetic testing in assisted reproduction. Minerva Obstet. Gynecol. 75, 260–272. doi:10.23736/S2724-606X.21.04805-3
Maître, J. L., Berthoumieux, H., Krens, S. F. G., Salbreux, G., Jülicher, F., Paluch, E., et al. (2012). Adhesion functions in cell sorting by mechanically coupling the cortices of adhering cells. Science 338, 253–256. doi:10.1126/science.1225399
Maître, J. L., Niwayama, R., Turlier, H., Nedelec, F., and Hiiragi, T. (2015). Pulsatile cell-autonomous contractility drives compaction in the mouse embryo. Nat. Cell Biol. 17, 849–855. doi:10.1038/ncb3185
Maître, J. L., Turlier, H., Illukkumbura, R., Eismann, B., Niwayama, R., Nédélec, F., et al. (2016). Asymmetric division of contractile domains couples cell positioning and fate specification. Nature 536, 344–348. doi:10.1038/nature18958
Marcos, J., Pérez-Albalá, S., Mifsud, A., Molla, M., Landeras, J., and Meseguer, M. (2015). Collapse of blastocysts is strongly related to lower implantation success: a time-lapse study. Hum. Reprod. 30, 2501–2508. doi:10.1093/humrep/dev216
Marikawa, Y., and Alarcon, V. B. (2018). RHOA activity in expanding blastocysts is essential to regulate HIPPO-YAP signaling and to maintain the trophectoderm-specific gene expression program in a ROCK/actin filament-independent manner. Mol. Hum. Reprod. 25, 43–60. doi:10.1093/molehr/gay048
Martin, A. C., Kaschube, M., and Wieschaus, E. F. (2009). Pulsed contractions of an actin-myosin network drive apical constriction. Nature 457, 495–499. doi:10.1038/nature07522
Mastenbroek, S., de Wert, G., and Adashi, E. Y. (2021). The imperative of responsible innovation in reproductive medicine. N. Engl. J. Med. 385, 2096–2100. doi:10.1056/NEJMsb2101718
McCoy, R. C., Summers, M. C., McCollin, A., Ottolini, C. S., Ahuja, K., and Handyside, A. H. (2023). Meiotic and mitotic aneuploidies drive arrest of in vitro fertilized human preimplantation embryos. Genome Med. 15, 77. doi:10.1186/S13073-023-01231-1
McGinnis, L. A., Lee, H. J., Robinson, D. N., and Evans, J. P. (2015). MAPK3/1 (ERK1/2) and myosin light chain kinase in mammalian eggs affect myosin-II function and regulate the metaphase II state in a calcium- and zinc-dependent manner. Biol. Reprod. 92, 146. doi:10.1095/biolreprod.114.127027
Mehlmann, L. M., Terasaki, M., Jaffe, L. A., and Kline, D. (1995). Reorganization of the endoplasmic reticulum during meiotic maturation of the mouse oocyte. Dev. Biol. 170, 607–615. doi:10.1006/dbio.1995.1240
Miao, Y. L., Kikuchi, K., Sun, Q. Y., and Schatten, H. (2009). Oocyte aging: cellular and molecular changes, developmental potential and reversal possibility. Hum. Reprod. Update. 15, 573–585. doi:10.1093/humupd/dmp014
Mignini Renzini, M., Dal Canto, M., Guglielmo, M. C., Garcia, D., De Ponti, E., La Marca, A., et al. (2021). Sperm donation: an alternative to improve post-ICSI live birth rates in advanced maternal age patients. Hum. Reprod. 36, 2148–2156. doi:10.1093/humrep/deab148
Mihajlović, A. I., and Bruce, A. W. (2016). Rho-associated protein kinase regulates subcellular localisation of Angiomotin and Hippo-signalling during preimplantation mouse embryo development. Reprod. Biomed. Online 33, 381–390. doi:10.1016/j.rbmo.2016.06.028
Mihajlović, A. I., and Bruce, A. W. (2017). The first cell-fate decision of mouse preimplantation embryo development: integrating cell position and polarity. Open Biol. 7, 170210. doi:10.1098/rsob.170210
Mihalas, B. P., Bromfield, E. G., Sutherland, J. M., De Iuliis, G. N., McLaughlin, E. A., John Aitken, R., et al. (2018). Oxidative damage in naturally aged mouse oocytes is exacerbated by dysregulation of proteasomal activity. J. Biol. Chem. 293, 18944–18964. doi:10.1074/jbc.RA118.005751
Milewski, R., Szpila, M., and Ajduk, A. (2018). Dynamics of cytoplasm and cleavage divisions correlates with preimplantation embryo development. Reproduction 155, 1–14. doi:10.1530/REP-17-0230
Mogessie, B., and Schuh, M. (2017). Actin protects mammalian eggs against chromosome segregation errors. Science 357, 1647. doi:10.1126/science.aal1647
Mohler, W., Millard, A. C., and Campagnola, P. J. (2003). Second harmonic generation imaging of endogenous structural proteins. Methods 29, 97–109. doi:10.1016/S1046-2023(02)00292-X
Montag, M., Toth, B., and Strowitzki, T. (2013). New approaches to embryo selection. Reprod. Biomed. Online 27, 539–546. doi:10.1016/j.rbmo.2013.05.013
Moore, S. G., and Hasler, J. F. (2017). A 100-Year Review: reproductive technologies in dairy science. J. Dairy Sci. 100, 10314–10331. doi:10.3168/JDS.2017-13138
Morado, S. A., Cetica, P. D., Beconi, M. T., and Dalvit, G. C. (2009). Reactive oxygen species in bovine oocyte maturation in vitro. Reprod. Fertil. Dev. 21, 608–614. doi:10.1071/RD08198
Moreno, R. D., Schatten, G., and Ramalho-Santos, J. (2002). Golgi apparatus dynamics during mouse oocyte in vitro maturation: effect of the membrane trafficking inhibitor brefeldin A. Biol. Reprod. 66, 1259–1266. doi:10.1095/biolreprod66.5.1259
Moriwaki, K., Tsukita, S., and Furuse, M. (2007). Tight junctions containing claudin 4 and 6 are essential for blastocyst formation in preimplantation mouse embryos. Dev. Biol. 312, 509–522. doi:10.1016/J.YDBIO.2007.09.049
Murayama, Y., Mizuno, J., Kamakura, H., Fueta, Y., Nakamura, H., Akaishi, K., et al. (2006). Mouse zona pellucida dynamically changes its elasticity during oocyte maturation, fertilization, and early embryo development. Hum. Cell 19, 119–125. doi:10.1111/j.1749-0774.2006.00019.x
Murrell, M., Oakes, P. W., Lenz, M., and Gardel, M. L. (2015). Forcing cells into shape: the mechanics of actomyosin contractility. Nat. Rev. Mol. Cell Biol. 16, 486–498. doi:10.1038/nrm4012
Nagy, Z. P., Jones-Colon, S., Roos, P., Botros, L., Greco, E., Dasig, J., et al. (2009). Metabolomic assessment of oocyte viability. Reprod. Biomed. Online 18, 219–225. doi:10.1016/s1472-6483(10)60259-3
Nguyen, T. L., Pradeep, S., Judson-Torres, R. L., Reed, J., Teitell, M. A., and Zangle, T. A. (2022). Quantitative phase imaging: recent advances and expanding potential in biomedicine. ACS Nano 16, 11516–11544. doi:10.1021/ACSNANO.1C11507
Niimura, S. (2003). Time-lapse videomicrographic analyses of contractions in mouse blastocysts. J. Reprod. Dev. 49, 413–423. doi:10.1262/jrd.49.413
Nik Hazlina, N. H., Norhayati, M. N., Shaiful Bahari, I., and Nik Muhammad Arif, N. A. (2022). Worldwide prevalence, risk factors and psychological impact of infertility among women: a systematic review and meta-analysis. BMJ Open 12, e057132. doi:10.1136/bmjopen-2021-057132
Nikiforov, D., Grøndahl, M. L., Hreinsson, J., and Andersen, C. Y. (2022). Human oocyte morphology and outcomes of infertility treatment: a systematic review. Reprod. Sci. 29, 2768–2785. doi:10.1007/s43032-021-00723-y
Oldenbourg, R. (2013). Polarized light microscopy: principles and practice. Cold Spring Harb. Protoc. 2013, 078600. doi:10.1101/pdb.top078600
Ombelet, W., De Sutter, P., Van der Elst, J., and Martens, G. (2005). Multiple gestation and infertility treatment: registration, reflection and reaction - the Belgian project. Hum. Reprod. Update 11, 3–14. doi:10.1093/humupd/dmh048
Omidi, M., Faramarzi, A., Agharahimi, A., and Khalili, M. A. (2017). Noninvasive imaging systems for gametes and embryo selection in IVF programs: a review. J. Microsc. 267, 253–264. doi:10.1111/jmi.12573
Özgüç, Ö., de Plater, L., Kapoor, V., Tortorelli, A. F., Clark, A. G., and Maître, J. L. (2022). Cortical softening elicits zygotic contractility during mouse preimplantation development. PLoS Biol. 20, e3001593. doi:10.1371/journal.pbio.3001593
Özgüç, Ö., and Maître, J. L. (2020). Multiscale morphogenesis of the mouse blastocyst by actomyosin contractility. Curr. Opin. Cell Biol. 66, 123–129. doi:10.1016/j.ceb.2020.05.002
Paternot, G., Devroe, J., Debrock, S., D’Hooghe, T. M., and Spiessens, C. (2009). Intra- and inter-observer analysis in the morphological assessment of early-stage embryos. Reprod. Biol. Endocrinol. 7, 105. doi:10.1186/1477-7827-7-105
Patrizio, P., Fragouli, E., Bianchi, V., Borini, A., and Wells, D. (2007). Molecular methods for selection of the ideal oocyte. Reprod. Biomed. Online 15, 346–353. doi:10.1016/S1472-6483(10)60349-5
Pfender, S., Kuznetsov, V., Pleiser, S., Kerkhoff, E., and Schuh, M. (2011). Spire-type actin nucleators cooperate with formin-2 to drive asymmetric oocyte division. Curr. Biol. 21, 955–960. doi:10.1016/j.cub.2011.04.029
Pickering, S. J., Johnson, M. H., Braude, P. R., and Houliston, E. (1988). Cytoskeletal organization in fresh, aged and spontaneously activated human oocytes. Hum. Reprod. 3, 978–989. doi:10.1093/oxfordjournals.humrep.a136828
Piliszek, A., and Madeja, Z. E. (2018). “Pre-implantation development of domestic animals,” in Current topics in developmental Biology (United States: Academic Press), 267–294. doi:10.1016/bs.ctdb.2017.11.005
Płusa, B., and Piliszek, A. (2020). Common principles of early mammalian embryo self-organisation. Development 147, 183079. doi:10.1242/DEV.183079
Popovic, M., Dhaenens, L., Boel, A., Menten, B., and Heindryckx, B. (2020). Chromosomal mosaicism in human blastocysts: the ultimate diagnostic dilemma. Hum. Reprod. Update 26, 313–334. doi:10.1093/humupd/dmz050
Posfai, E., Rovic, I., and Jurisicova, A. (2019). The mammalian embryo’s first agenda: making trophectoderm. Int. J. Dev. Biol. 63, 157–170. doi:10.1387/ijdb.180404ep
Prosser, S. L., and Pelletier, L. (2017). Mitotic spindle assembly in animal cells: a fine balancing act. Nat. Rev. Mol. Cell Biol. 18, 187–201. doi:10.1038/nrm.2016.162
Rienzi, L., Vajta, G., and Ubaldi, F. (2011). Predictive value of oocyte morphology in human IVF: a systematic review of the literature. Hum. Reprod. Update. 17, 34–45. doi:10.1093/humupd/dmq029
Robert, B. (2009). Resonance Raman spectroscopy. Photosynth. Res. 101, 147–155. doi:10.1007/s11120-009-9440-4
Rosenberg, S. M., and Partridge, A. H. (2013). Premature menopause in young breast cancer: effects on quality of life and treatment interventions. J. Thorac. Dis. 5 Suppl 1, S55–S61. doi:10.3978/j.issn.2072-1439.2013.06.20
Rouchou, B. (2013). Consequences of infertility in developing countries. Perspect. Public Health 133, 174–179. doi:10.1177/1757913912472415
Rusciano, G., De Canditiis, C., Zito, G., Rubessa, M., Roca, M. S., Carotenuto, R., et al. (2017). Raman-microscopy investigation of vitrification-induced structural damages in mature bovine oocytes. PLoS ONE 12, e0177677. doi:10.1371/journal.pone.0177677
Ryan, A. Q., Chan, C. J., Graner, F., and Hiiragi, T. (2019). Lumen expansion facilitates epiblast-primitive endoderm fate specification during mouse blastocyst formation. Dev. Cell 51, 684–697. doi:10.1016/j.devcel.2019.10.011
Sadecki, E., Weaver, A., Zhao, Y., Stewart, E. A., and Ainsworth, A. J. (2022). Fertility trends and comparisons in a historical cohort of US women with primary infertility. Reprod. Health 19, 13. doi:10.1186/s12978-021-01313-6
Salmerón, A. M., Abreu, A. C., Vilches-Ferrón, M., and Fernández, I. (2021). Solution NMR in human embryo culture media as an option for assessment of embryo implantation potential. NMR Biomed. 34, e4536. doi:10.1002/nbm.4536
Salucci, S., Battistelli, M., Burattini, S., Sbrana, F., and Falcieri, E. (2020). Holotomographic microscopy: a new approach to detect apoptotic cell features. Microsc. Res. Tech. 83, 1464–1470. doi:10.1002/JEMT.23539
Samarage, C. R., White, M. D., Álvarez, Y. D., Fierro-González, J. C., Henon, Y., Jesudason, E. C., et al. (2015). Cortical tension allocates the first inner cells of the mammalian embryo. Dev. Cell 34, 435–447. doi:10.1016/j.devcel.2015.07.004
Sanches, B. V., Zangirolamo, A. F., and Seneda, M. M. (2019). Intensive use of IVF by large-scale dairy programs. Anim. Reprod. 16, 394–401. doi:10.21451/1984-3143-AR2019-0058
Sanchez, T., Venturas, M., Aghvami, S. A., Yang, X., Fraden, S., Sakkas, D., et al. (2019). Combined noninvasive metabolic and spindle imaging as potential tools for embryo and oocyte assessment. Hum. Reprod. 34, 2349–2361. doi:10.1093/humrep/dez210
Sandoz, P. A., Tremblay, C., Gisou van der Goot, F., and Frechin, M. (2019). Image-based analysis of living mammalian cells using label-free 3D refractive index maps reveals new organelle dynamics and dry mass flux. PLoS Biol. 17, e3000553. doi:10.1371/JOURNAL.PBIO.3000553
Saragusty, J., Diecke, S., Drukker, M., Durrant, B., Friedrich Ben-Nun, I., Galli, C., et al. (2016). Rewinding the process of mammalian extinction. Zoo. Biol. 35, 280–292. doi:10.1002/zoo.21284
Schliffka, M. F., Dumortier, J. G., Pelzer, D., Mukherjee, A., and Maître, J. L. (2023). Inverse blebs operate as hydraulic pumps during mouse blastocyst formation. bioRxiv, 2023.05.03.539105. doi:10.1101/2023.05.03.539105
Schuh, M., and Ellenberg, J. (2008). A new model for asymmetric spindle positioning in mouse oocytes. Curr. Biol. 18, 1986–1992. doi:10.1016/j.cub.2008.11.022
Sciorio, R., Thong, K. J., and Pickering, S. J. (2020). Spontaneous blastocyst collapse as an embryo marker of low pregnancy outcome: a time-lapse study. J. Bras. Reprod. Assist. 24, 34–40. doi:10.5935/1518-0557.20190044
Serwane, F., Mongera, A., Rowghanian, P., Kealhofer, D. A., Lucio, A. A., Hockenbery, Z. M., et al. (2017). In vivo quantification of spatially varying mechanical properties in developing tissues. Nat. Methods 14, 181–186. doi:10.1038/nmeth.4101
Shen, T., Benet, E., Sridhar, S. L., Abadie, J., Piat, E., and Vernerey, F. J. (2019). Separating the contributions of zona pellucida and cytoplasm in the viscoelastic response of human oocytes. Acta Biomater. 85, 253–262. doi:10.1016/j.actbio.2018.12.034
Shimoda, Y., Kumagai, J., Anzai, M., Kabashima, K., Togashi, K., Miura, Y., et al. (2016). Time-lapse monitoring reveals that vitrification increases the frequency of contraction during the pre-hatching stage in mouse embryos. J. Reprod. Dev. 62, 187–193. doi:10.1262/JRD.2015-150
Silber, S. J., Barbey, N., Lenahan, K., and Silber, D. Z. (2013). Applying clinically proven human techniques for contraception and fertility to endangered species and zoo animals: a review. J. Zoo. Wildl. Med. 44, 111–S122. doi:10.1638/1042-7260-44.4S.S111
Sirard, M. A. (2018). 40 years of bovine IVF in the new genomic selection context. Reproduction 156, R1. doi:10.1530/REP-18-0008
Sjunnesson, Y. (2020). In vitro fertilisation in domestic mammals—a brief overview. J. Med. Sci. 125, 68–76. doi:10.1080/03009734.2019.1697911
Skora, D., and Frankfurter, D. (2012). Adverse perinatal events associated with ART. Semin. Reprod. Med. 30, 84–91. doi:10.1055/s-0032-1307416
Skory, R. M., Moverley, A. A., Ardestani, G., Alvarez, Y., Domingo-Muelas, A., Pomp, O., et al. (2023). The nuclear lamina couples mechanical forces to cell fate in the preimplantation embryo via actin organization. Nat. Commun. 14, 3101–3114. doi:10.1038/s41467-023-38770-5
Society for Assisted Reproductive Technology (SART) (2022). Final national summary report for 2020. Available from: https://sartcorsonline.com.
Solon, J., Kaya-Çopur, A., Colombelli, J., and Brunner, D. (2009). Pulsed forces timed by a ratchet-like mechanism drive directed tissue movement during dorsal closure. Cell 137, 1331–1342. doi:10.1016/j.cell.2009.03.050
Soreghan, B. A., Yang, F., Thomas, S. N., Hsu, J., and Yang, A. J. (2003). High-throughput proteomic-based identification of oxidatively induced protein carbonylation in mouse brain. Pharm. Res. 20, 1713–1720. doi:10.1023/B:PHAM.0000003366.25263.78
Stadtman, E. R. (1992). Protein oxidation and aging. Science 257, 1220–1224. doi:10.1126/science.1355616
Stitzel, M. L., and Seydoux, G. (2007). Regulation of the oocyte-to-zygote transition. Science 316, 407–408. doi:10.1126/science.1138236
Straight, A. F., Cheung, A., Limouze, J., Chen, I., Westwood, N. J., Sellers, J. R., et al. (2003). Dissecting temporal and spatial control of cytokinesis with a myosin II inhibitor. Science 299, 1743–1747. doi:10.1126/science.1081412
Sun, S. C., Gao, W. W., Xu, Y. N., Jin, Y. X., Wang, Q. L., Yin, X. J., et al. (2012). Degradation of actin nucleators affects cortical polarity of aged mouse oocytes. Fertil. Steril. 97, 984–990. doi:10.1016/j.fertnstert.2012.01.101
Swann, K., Windsor, S., Campbell, K., Elgmati, K., Nomikos, M., Zernicka-Goetz, M., et al. (2012). Phospholipase C-ζ-induced Ca 2+ oscillations cause coincident cytoplasmic movements in human oocytes that failed to fertilize after intracytoplasmic sperm injection. Fertil. Steril. 97, 742–747. doi:10.1016/j.fertnstert.2011.12.013
Szpila, M., Walewska, A., Sabat-Pośpiech, D., Strączyńska, P., Ishikawa, T., Milewski, R., et al. (2019). Postovulatory ageing modifies sperm-induced Ca2+ oscillations in mouse oocytes through a conditions-dependent, multi-pathway mechanism. Sci. Rep. 9, 11859. doi:10.1038/s41598-019-48281-3
Takahashi, T., Igarashi, H., Amita, M., Hara, S., Matsuo, K., and Kurachi, H. (2013). Molecular mechanism of poor embryo development in postovulatory aged oocytes: mini review. J. Obstet. Gynaecol. Res. 39, 1431–1439. doi:10.1111/jog.12111
Tamai, Y., Ishikawa, T. O., Bösl, M. R., Mori, M., Nozaki, M., Baribault, H., et al. (2000). Cytokeratins 8 and 19 in the mouse placental development. J. Cell Biol. 151, 563–572. doi:10.1083/jcb.151.3.563
Tan, J.-H. J. H., Wang, H. L. H.-L., Sun, X. S. X.-S., Liu, Y., Sui, H. S. H.-S., and Zhang, J. (2009). Chromatin configurations in the germinal vesicle of mammalian oocytes. Mol. Hum. Reprod. 15, 1–9. doi:10.1093/molehr/gan069
Taneja, N., and Burnette, D. T. (2019). Myosin IIA drives membrane bleb retraction. Mol. Biol. Cell 30, 1051–1059. doi:10.1091/mbc.E18-11-0752
Tang, B. L. (2012). Membrane trafficking components in cytokinesis. Cell. Physiol. biochem. 30, 1097–1108. doi:10.1159/000343301
Tatone, C., Di Emidio, G., Vento, M., Ciriminna, R., and Artini, P. G. (2010). Cryopreservation and oxidative stress in reproductive cells. Gynecol. Endocrinol. 26, 563–567. doi:10.3109/09513591003686395
Thayil, A., Jesacher, A., Wilson, T., Booth, M., Watanabe, T., and Srinivas, S. (2011). Long-term imaging of mouse embryos using adaptive harmonic generation microscopy. J. Biomed. Opt. 16, 046018. doi:10.1117/1.3569614
Togashi, K., Kumagai, J., Sato, E., Shirasawa, H., Shimoda, Y., Makino, K., et al. (2015). Dysfunction in gap junction intercellular communication induces aberrant behavior of the inner cell mass and frequent collapses of expanded blastocysts in mouse embryos. J. Assist. Reprod. Genet. 32, 969–976. doi:10.1007/s10815-015-0479-1
Toralova, T., Kinterova, V., Chmelikova, E., and Kanka, J. (2020). The neglected part of early embryonic development: maternal protein degradation. Cell. Mol. Life Sci. 77, 3177–3194. doi:10.1007/s00018-020-03482-2
Tsai, T. Y. C., Collins, S. R., Chan, C. K., Hadjitheodorou, A., Lam, P. Y., Lou, S. S., et al. (2019). Efficient front-rear coupling in neutrophil chemotaxis by dynamic myosin II localization. Dev. Cell 49, 189–205. doi:10.1016/j.devcel.2019.03.025
Vander Borght, M., and Wyns, C. (2018). Fertility and infertility: definition and epidemiology. Clin. Biochem. 62, 2–10. doi:10.1016/j.clinbiochem.2018.03.012
Venturas, M., Shah, J. S., Yang, X., Sanchez, T. H., Conway, W., Sakkas, D., et al. (2022). Metabolic state of human blastocysts measured by fluorescence lifetime imaging microscopy. Hum. Reprod. 37, 411–427. doi:10.1093/humrep/deab283
Venturas, M., Yang, X., Sakkas, D., and Needleman, D. (2023). Noninvasive metabolic profiling of cumulus cells, oocytes, and embryos via fluorescence lifetime imaging microscopy: a mini-review. Hum. Reprod. 38, 799–810. doi:10.1093/humrep/dead063
Viana, J. H. (2019). 2018 Statistics of embryo production and transfer in domestic farm animals Embryo industry on a new level: over one million embryos produced in vitro. Embryo Technol. Newsl. 36, 8–25.
Viljoen, A., Mathelié-Guinlet, M., Ray, A., Strohmeyer, N., Oh, Y. J., Hinterdorfer, P., et al. (2021). Force spectroscopy of single cells using atomic force microscopy. Nat. Rev. Methods Prim. 11 (1), 63–24. doi:10.1038/s43586-021-00062-x
Walls, M. L., Hunter, T., Ryan, J. P., Keelan, J. A., Nathan, E., and Hart, R. J. (2015). In vitro maturation as an alternative to standard in vitro fertilization for patients diagnosed with polycystic ovaries: a comparative analysis of fresh, frozen and cumulative cycle outcomes. Hum. Reprod. 30, 88–96. doi:10.1093/humrep/deu248
Watanabe, Y., Miyasaka, K. Y., Kubo, A., Kida, Y. S., Nakagawa, O., Hirate, Y., et al. (2017). Notch and Hippo signaling converge on Strawberry Notch 1 (Sbno1) to synergistically activate Cdx2 during specification of the trophectoderm. Sci. Rep. 7, 46135–46217. doi:10.1038/srep46135
Westerweel, J. (1997). Fundamentals of digital particle image velocimetry. Meas. Sci. Technol. 8, 1379–1392. doi:10.1088/0957-0233/8/12/002
Winkel, G. K., Ferguson, J. E., Takeichi, M., and Nuccitelli, R. (1990). Activation of protein kinase C triggers premature compaction in the four-cell stage mouse embryo. Dev. Biol. 138, 1–15. doi:10.1016/0012-1606(90)90171-E
Xie, H.-L. L., Wang, Y.-B. B., Jiao, G.-Z. Z., Kong, D.-L. L., Li, Q., Li, H., et al. (2016). Effects of glucose metabolism during in vitro maturation on cytoplasmic maturation of mouse oocytes. Sci. Rep. 6, 20764. doi:10.1038/srep20764
Yamamoto, K., Miura, H., Ishida, M., Mii, Y., Kinoshita, N., Takada, S., et al. (2021). Optogenetic relaxation of actomyosin contractility uncovers mechanistic roles of cortical tension during cytokinesis. Nat. Commun. 12, 7145. doi:10.1038/s41467-021-27458-3
Yanez, L. Z., Han, J., Behr, B. B., Pera, R. A. R., and Camarillo, D. B. (2016). Human oocyte developmental potential is predicted by mechanical properties within hours after fertilization. Nat. Commun. 7, 10809–10812. doi:10.1038/ncomms10809
Yin, H., Jiang, H., He, R., Wang, C., Zhu, J., and Li, Y. (2016). The effects of blastocyst morphological score and blastocoele re-expansion speed after warming on pregnancy outcomes. Clin. Exp. Reprod. Med. 43, 31–37. doi:10.5653/cerm.2016.43.1.31
Yu, C. H., Langowitz, N., Wu, H. Y., Farhadifar, R., Brugues, J., Yoo, T. Y., et al. (2014). Measuring microtubule polarity in spindles with second-harmonic generation. Biophys. J. 106, 1578–1587. doi:10.1016/j.bpj.2014.03.009
Zaninovic, N., and Rosenwaks, Z. (2020). Artificial intelligence in human in vitro fertilization and embryology. Fert. Steril. 114, 914–920. doi:10.1016/j.fertnstert.2020.09.157
Zenker, J., White, M. D., Gasnier, M., Alvarez, Y. D., Lim, H. Y. G., Bissiere, S., et al. (2018). Expanding actin rings zipper the mouse embryo for blastocyst formation. Cell 173, 776–791. doi:10.1016/j.cell.2018.02.035
Zenker, J., White, M. D., Templin, R. M., Parton, R. G., Thorn-Seshold, O., Bissiere, S., et al. (2017). A microtubule-organizing center directing intracellular transport in the early mouse embryo. Science 357, 925–928. doi:10.1126/science.aam9335
Zhao, J., Yan, Y., Huang, X., Sun, L., and Li, Y. (2019). Blastocoele expansion: an important parameter for predicting clinical success pregnancy after frozen-warmed blastocysts transfer. Reprod. Biol. Endocrinol. 17, 15–18. doi:10.1186/s12958-019-0454-2
Zhao, Q., Yin, T., Peng, J., Zou, Y., Yang, J., Shen, A., et al. (2013). Noninvasive metabolomic profiling of human embryo culture media using a simple spectroscopy adjunct to morphology for embryo assessment in in vitro fertilization (IVF). Int. J. Mol. Sci. 14, 6556–6570. doi:10.3390/ijms14046556
Zhu, M., Leung, C. Y., Shahbazi, M. N., and Zernicka-Goetz, M. (2017). Actomyosin polarisation through PLC-PKC triggers symmetry breaking of the mouse embryo. Nat. Commun. 8, 921–1016. doi:10.1038/s41467-017-00977-8
Keywords: oocyte, embryo, mouse, preimplantation development, biomechanics, cytoskeleton, quality assessment, assisted reproductive technologies
Citation: Fluks M, Collier R, Walewska A, Bruce AW and Ajduk A (2024) How great thou ART: biomechanical properties of oocytes and embryos as indicators of quality in assisted reproductive technologies. Front. Cell Dev. Biol. 12:1342905. doi: 10.3389/fcell.2024.1342905
Received: 22 November 2023; Accepted: 01 February 2024;
Published: 15 February 2024.
Edited by:
Ahmed Balboula, University of Missouri, United StatesReviewed by:
Zuzana Holubcová, Masaryk University, CzechiaAhmed Gad, Colorado State University, United States
Copyright © 2024 Fluks, Collier, Walewska, Bruce and Ajduk. This is an open-access article distributed under the terms of the Creative Commons Attribution License (CC BY). The use, distribution or reproduction in other forums is permitted, provided the original author(s) and the copyright owner(s) are credited and that the original publication in this journal is cited, in accordance with accepted academic practice. No use, distribution or reproduction is permitted which does not comply with these terms.
*Correspondence: Monika Fluks, bS5mbHVrc0B1dy5lZHUucGw=, bWZsdWtzQGpjdS5jeg==