- 1Models of Cancer Therapies Laboratory, Vall d’Hebron Institute of Oncology (VHIO), Vall d’Hebron Barcelona Hospital Campus, Barcelona, Spain
- 2Department of Biochemistry and Molecular Biology, Universitat Autònoma de Barcelona, Bellaterra, Spain
- 3Institució Catalana de Recerca i Estudis Avançats (ICREA), Barcelona, Spain
- 4Peptomyc S.L., Barcelona, Spain
MYC is a pleiotropic transcription factor involved in multiple cellular processes. While its mechanism of action and targets are not completely elucidated, it has a fundamental role in cellular proliferation, differentiation, metabolism, ribogenesis, and bone and vascular development. Over 4 decades of research and some 10,000 publications linking it to tumorigenesis (by searching PubMed for “MYC oncogene”) have led to MYC becoming a most-wanted target for the treatment of cancer, where many of MYC’s physiological functions become co-opted for tumour initiation and maintenance. In this context, an abundance of reviews describes strategies for potentially targeting MYC in the oncology field. However, its multiple roles in different aspects of cellular biology suggest that it may also play a role in many additional diseases, and other publications are indeed linking MYC to pathologies beyond cancer. Here, we review these physiological functions and the current literature linking MYC to non-oncological diseases. The intense efforts towards developing MYC inhibitors as a cancer therapy will potentially have huge implications for the treatment of other diseases. In addition, with a complementary approach, we discuss some diseases and conditions where MYC appears to play a protective role and hence its increased expression or activation could be therapeutic.
1 Discovery and initial characterisation of MYC
The c-MYC gene encodes for a basic helix-loop-helix protein that acts as a pleiotropic transcription factor. It was discovered more than 40 years ago by the pioneering work to isolate and characterise avian retrovirus MC29, which showed its oncogenic potential, followed by the discovery of c-MYC, its cellular homolog identified from the chicken genome (Duesberg et al., 1977; Sheiness et al., 1978; Hu et al., 1979; Abrams et al., 1982; Vennstrom et al., 1982; Hann et al., 1983; Dang et al., 1989). Later studies discovered two human paralogs with overlapping roles and a more limited tissular expression: MYCN, or N-MYC, identified in Neuroblastoma cells, and MYCL, or L-MYC, found in Lung carcinoma cells, respectively, reviewed in (Massó-Vallés et al., 2020). c-MYC (from now on, MYC) and its paralogs share an N-terminal transactivation domain (TAD), capable of interacting with a plethora of proteins regulating chromatin remodelling, transcription and MYC stability, a central region, and a C-terminus basic helix-loop-helix (bHLH) domain (Beaulieu et al., 2020). The latter initially pointed to MYC as a protein capable of binding DNA, although it was not until 1990 that it was discovered that MYC bound the sequence CACGTG (termed the E-box) (Prendergast and Ziff, 1991). Shortly after, a MYC dimerisation partner was identified: MYC-associated protein X, MAX a bHLH-Zip protein, specifically associated with c-MYC and its paralogs. Using a yeast model, DNA binding and transcriptional transactivation by MYC were found to be both dependent on this heterodimer (Blackwood and Eisenman, 1991; Amati et al., 1992), and a study in Drosophila, revealed that dMyc, dMax and the Max-binding protein dMNT could bind up to ∼15% of the coding regions in the fly genome (Orian et al., 2003).
2 Physiological processes mediated by MYC
In this section we describe how MYC plays a key role in multiple aspects of the biology of cells and tissues. This is also summarised in Figure 1.
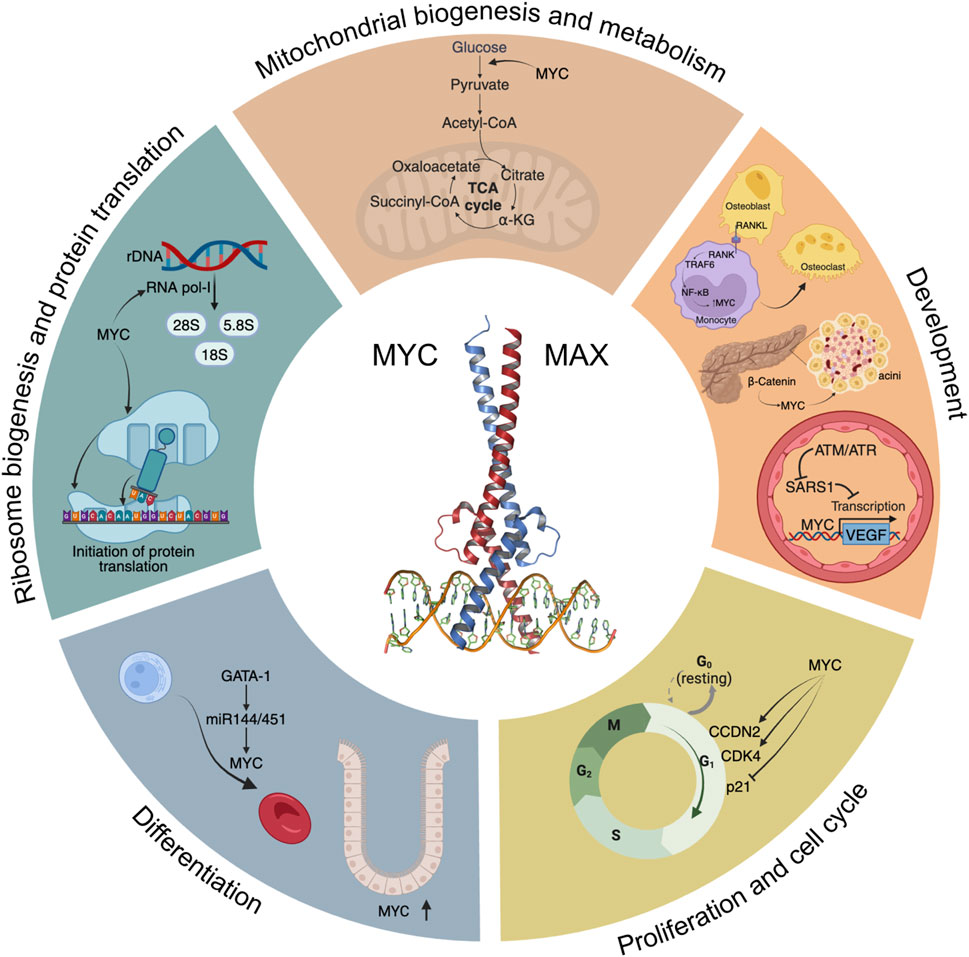
FIGURE 1. A “Hallmarks” style representation of MYC’s physiological functions. Some of the main signalling pathways and processes modulated by MYC are depicted, including glycolysis and mitochondrial biogenesis, development, cell cycle progression, differentiation, ribosome biogenesis and initiation of protein translation.
2.1 Proliferation
MYC’s most established role under physiological conditions is to promote efficient proliferation (Jha et al., 2023). This has been studied in many model systems and organisms from mammalian tissues to flies. Although MYC is virtually undetectable in quiescent cells, upon mitogenic or serum stimulation, MYC levels are induced, and cells enter the G1 phase of the cell cycle through MYC-dependent upregulation and/or activation of key mediators of cell cycle progression, such as CCDN2, CDK4, and the CyclinE2-CDK2 complex, degradation of p27 (Kip1, encoded by CDKN1B), and repression of p21 and p15 (encoded by CDKN1A and CDKN2B, respectively) among others (Pelengaris and Khan, 2003). In contrast, MYC-dependent repression was described to be mediated by MYC-MAX interaction with Miz-1 (Staller et al., 2001). Expression of MYC is necessary, and in some cases sufficient, for inducing cell proliferation. In fact, ectopic expression of MYC locks cells in a continuously proliferating state, even in the absence of mitogens (Evan et al., 1994). This is probably the evolutionary basis for the tight regulation of MYC expression, which is in stark contrast to the ubiquitous expression of its binding partner MAX.
MYC’s key role in proliferation and growth is highly conserved throughout the animal kingdom, with a presence in invertebrates such as Drosophila, where dMYC is the only paralog, whose loss impacts on cellular growth and size. Its overexpression promotes G1/S progression but not cell division, which is dependent on other players (Johnston et al., 1999). Interestingly, expression of dMYC is able to rescue the proliferation defects in mouse embryonic fibroblasts deficient for MYC, although it does not affect cell growth. Thus, MYC and dMYC have similar biological functions, but their outcomes depend on specific cell targets (Trumpp et al., 2001). Given this ancestral conservation, it is curious that MYC itself was lost during the evolution of C.elegans, which, instead, retains orthologous MAX and MLX networks (Yuan et al., 1998; Gallant, 2006; McFerrin and Atchley, 2011).
2.2 Differentiation
A key role for MYC in differentiation has been demonstrated in many tissues. One prominent example is found in the hematopoietic system, where MYC is involved in the expansion of committed progenitors by controlling the balance between self-renewal and differentiation through the modulation of stem cell migration and/or adhesion to the niche. MYC was described as necessary to induce the first differentiation steps in these murine stem cells, whereas in committed progenitors, MYC is required for proliferation and expansion (McFerrin and Atchley, 2011). Additionally, gene expression analyses using Krüppel-like factor 1 (KLF1), a master regulator of adult erythropoiesis (Perkins et al., 2016), and KLF2 knockout mice identified MYC as a central node in a network of genes controlled by both KLF1 and KLF2. Ablation of MYC in primitive proerythroblasts showed that its absence resulted in a block in the normal expansion of erythroid cells (Pang et al., 2012). In addition, the master regulator of haematopoiesis GATA-1 represses MYC transcriptional activity through binding to MYC’s promoter or through activation of miR-144/451, inducing proliferative arrest, thus facilitating erythroid differentiation (Rylski et al., 2003). Conversely, depletion of miR-144/451 blocks erythroid differentiation through de-repression of MYC (Xu et al., 2020). This GATA-1-miR144/451-MYC axis controls normal erythroid differentiation.
Another example of MYC’s role in differentiation can be found in murine embryonic stem cells (mESC), where MYC inhibits the expression of differentiation-specific genes through modulation of a set of miRNAs that attenuate their proliferation (Lin et al., 2009). Its inhibition or deletion strongly curbs transcription, splicing and protein synthesis, leading to a proliferative arrest, reminiscent of embryonic diapause. Remarkably, this arrest is reversible and does not compromise cell pluripotency (Scognamiglio et al., 2016). Additionally, MYC maintains the pluripotent transcriptome by amplifying the transcription of a large set of genes during the transition from mESC to the totipotent two-cell-like state (Fu et al., 2019), and is also important in metabolic and epigenetic regulation of mESCs during mouse embryonic development (Fan and Li, 2023).
In human adipose tissue, MYC was identified as a significant regulator of adipose stem cell differentiation, which is necessary for the maintenance and function of the tissue. MYC is induced by glucocorticoid in the early stages of differentiation and precedes the downregulation of key suppressor genes as well as the induction of functional effectors (Deisenroth et al., 2014).
In crypt development in the small intestine in the mouse, MYC signalling pathways are significantly enriched. Laser capture microdissection followed by functional genomics analysis of epithelial progenitors showed an enrichment, with respect to normal crypt base epithelium, of a series of transcripts encoding for proteins that regulate MYC transcription, protein stability, and transactivation of its target genes (Stappenbeck et al., 2003). Subsequent studies, however, showed that MYC is necessary for normal crypt formation, but does not affect cell proliferation or fate of already formed crypts (Bettess et al., 2005).
A role in differentiation is present in Drosophila too, where dMYC is required for intestinal stem cell maintenance, proliferation, and lineage differentiation during tissue homeostasis (Ren et al., 2013). Also in Drosophila, IGF2BP stabilises MYC mRNA, increasing its protein levels, leading to larger neural stem cells and faster division rates (Samuels et al., 2020). In line with this, GSK3-α and -β differently regulate cortical development through MYC (Ma et al., 2017), while MYC inhibits the differentiation of neural progenitor cells into neurons (Wang et al., 2020).
2.3 Ribosome biogenesis and protein translation
Ribosome biogenesis involves the synthesis and processing of ribosomal RNA (rRNA) proteins, the assembly of ribosomal subunits and their export to the cytoplasm, and it requires the coordinated activities of the three nuclear RNA polymerases (RNA pol I, II and III). Not surprisingly, MYC regulates multiple stages of ribosomal biogenesis through RNA pol I-mediated transcription of rRNA, RNA pol II-dependent transcription of ribosomal protein genes and translation initiation factors, among others (reviewed in van Riggelen et al., 2010). Using Crispr-Cas9-based reverse genetics to dissect the transcriptional networks downstream of MYC in vivo, it was shown that MYC’s ability to drive growth depends on its ability to upregulate ribosome biogenesis (Zielke et al., 2022). Consistent with this, inducible overexpression of MYC stimulates both ribosome biogenesis and protein synthesis (Mori et al., 2021).
Intimately related to ribosome biogenesis, protein translation is a critical process on which cell growth and division depend. It is regulated at different levels, although the key point of control seems to be the initiation of translation, which involves the translation initiation factor eIF4E binding to the 7-methyl guanosine cap at the 5′ end of mRNAs (Schmidt, 2004). Experiments carried out with MYC knockout rat fibroblasts showed that levels of protein translation, a mechanism that is under control of mammalian TOR complex 1 (mTORC1) (Ma and Blenis, 2009), are two-to-three fold higher in MYC wild-type when compared to MYC−/− cells (Mateyak et al., 1997). Microarray analysis of these cells showed that the largest category of MYC-induced genes was involved in protein translation, where an impressive 60% of the genes were upregulated by MYC (Guo et al., 2000). Additionally, a specific role in regulating eIF4E was confirmed after showing its expression correlated with and was regulated by MYC (Rosenwald et al., 1993). Indeed, MYC binds to two canonical E-boxes in the eIF4E promoter and is necessary for its expression. Importantly, inhibition of eIF4E was able to block MYC-induced transformation (Lynch et al., 2004).
The link between MYC and ribosomes is conserved in flies and even in the multicellular eukaryote Nemostella (Brown et al., 2008; Stine et al., 2015). Indeed, in Drosophila, expression of dMYC is necessary and sufficient to control rRNA synthesis and ribosome biogenesis (Grewal et al., 2005) and physiological dMYC targets, whose promoters are enriched in the E-box motif (frequently in the first 100 nucleotides following the transcription start site), play a role in nucleolar function and ribosome biogenesis (Hulf et al., 2005).
2.4 Metabolism and mitochondrial biogenesis
The first evidence of the in vivo induction of glycolysis by MYC was provided using transgenic mouse models where MYC was overexpressed in hepatocytes under the control of phosphoenolpyruvate carboxykinase. Transcriptional analyses of livers from these transgenic mice revealed increased expression of the glycolytic enzymes of glucokinase, PFKFB1, pyruvate-kinase L, and the glucose transporter GLUT2, which resulted in increased glycolysis compared to controls (Hulf et al., 2005). Later on, MYC was shown to induce a collection of glycolytic genes including ALDOA, ENO1, GAPDH, GPI, LDHA, HK2, PFKM, PGK1, PKM, and TPI1 (Kim et al., 2004), confirming the key role of MYC in controlling metabolism.
Importantly, MYC’s effect on metabolism becomes more evident when MYC is absent. Even in the presence of adequate energy-generating substrates, MYC-knockout fibroblasts remain ATP-depleted and respond by activating AMPK, in an attempt to remedy this energy deficit. However, since AMPK activation leads to upregulation of glycolysis and oxidative phosphorylation, both dependent on MYC, the AMPK response fails and the cells, unable to correct the energy production, remain slowly proliferating (Edmunds et al., 2014).
A final well-established role for MYC is in the mitochondria biogenesis. Using a combination of in vitro and in vivo MYC-modulating models, a role was shown for MYC in regulating the expression of genes involved in mitochondrial structure, function, and biogenesis. These include TFAM, a key mitochondrial transcriptional factor and mtDNA replication factor. These results point to MYC’s role as a master mitochondrial switch coupling metabolic needs to cell growth and proliferation (Li et al., 2005). In this line, further work suggested that mitochondrial structure, function, and subcellular localisation are regulated over time, responding more rapidly to inactivation of MYC than to its activation. The increased mitochondrial mass induced by MYC was associated with increased organelle turnover, involving both fission and fusion proteins (Graves et al., 2012). Overall, these results reinforce the notion that MYC links cellular energy generation and proliferative needs.
MYC’s role in mitochondrial biogenesis is also conserved in Drosophila, where in the ovary, it stimulates gene expression, including that of many electron transport chain genes required for mtDNA replication and expression (Wang et al., 2019).
2.5 Development
An increasing number of studies point to a role for MYC in the development of multiple tissues and organs, including pancreas, bone, and blood vessels. Given the difference in phenotypes of the tissues, it is perhaps not surprising that the principal targets of MYC in each case are different. In fact, development of tissues is a phenotypic outcome of the physiological processes that MYC helps to control, so that MYC’s regulation of proliferation, differentiation and metabolism results in different phenotypic outcomes depending on the cell-specific gene expression, tissue type and body location. This highlights a reason why the definition of a single critical list of MYC target genes across different tissue contexts has proven impossible.
2.5.1 Pancreas
The expansion of pancreatic acinar cells, the main components of pancreatic parenchyma, is promoted by β-catenin signalling, of which MYC is one key effector (Murtaugh et al., 2005). MYC’s importance in pancreas is stressed by the evidence that pancreatic inactivation of both MYC alleles in a mouse model leads to death after birth. Already at a late embryonic stage, these mice show a severe pancreatic hypoplasia, with poorly branched pancreatic ducts, disruption of exocrine pancreas formation and severe reduction of acini, characterised by reduced MYC target CDK4 expression and proliferation (Nakhai et al., 2008). These results were confirmed in an independent study using a mouse model with a 60%–70% reduction in MYC expression, in which pancreata showed fewer proliferating progenitors at E12.5, leading to significantly reduced pancreatic weight in two-month-old mice. Both arborization of the exocrine tree and acinar development were impaired at birth, but partially recovered at 2 months. Overall, MYC inactivation impairs normal acinar development and maturation, leading to the formation of lipid vacuoles in acinar cells, acquiring an adipocyte phenotype with aging (Bonal et al., 2009; Zhang et al., 2010).
2.5.2 Bone
Bone remodelling results from the balance between two tightly regulated phenomena: osteoclastogenesis and osteogenesis. The osteoclast is a bone-resorbing cell with an origin in the monocyte-macrophage lineage (Yavropoulou and Yovos, 2008). As expected, MYC is involved in bone remodelling and is regulated by different signalling pathways. RANKL, a key cytokine expressed by osteoblasts, mediates osteoclastogenesis through a TRAF6-dependent NF-κB activation. Upon RANKL binding to monocytes, this cascade results in the induction of MYC, which is necessary for osteoclast differentiation, since its inhibition by the MYC dominant negative In373-Myc almost completely inhibits osteoclastogenesis (Battaglino et al., 2002).
On the other hand, inhibition of FOXO1, whose expression decreases upon RANKL-induced osteoclastogenesis, promotes the expression of MYC, while 10058-F4, a small molecular inhibitor of MYC, abrogates the osteoclastogenic effect of FOXO1 inhibition in a dose-dependent manner (Tan et al., 2015). Additionally, RANKL-induced expression of osteoclastogenic marker genes is significantly reduced in MYC-deficient osteoclast progenitors in vitro, but rescued by ectopic expression of MYC (Bae et al., 2017).
Finally, RNA-seq analysis of MYC wild-type and deficient bone marrow cells revealed that MYC regulates mTORC1 signalling. mTORC1 is activated at the early phases after RANKL treatment and suppressed at later phases of osteoclastogenesis, and this biphasic regulation is dependent on MYC. While inhibition of mTORC1 by rapamycin prior to RANKL stimulation almost completely prevented osteoclast formation, osteoclasts showed enhanced resorbing activity when mTORC1 was inhibited three days post-RANKL. In parallel, unfolded protein response (UPR) genes were found downregulated by MYC deficiency. In line with this, the expression of GADD34, a factor that regulates UPR and negatively regulates mTOR signalling, is increased in wild-type cells upon RANKL stimulation but not in MYC-deficient cells, and its deficiency partially restores RANKL-induced mTORC1 inactivation and suppresses osteoclastogenesis. Taken together, these data suggest that mTORC1 is suppressed in osteoclasts through a MYC/GADD34 axis (Bae et al., 2022). This is in stark contrast to protein translation, where MYC and mTORC1 jointly contribute to its regulation.
2.5.3 Vascular development
MYC’s role in vascular development was confirmed by modulation of MYC levels. In fact, c-MYC knockout mice are embryonic lethal and have under-developed vasculature, that can be partially rescued by transgenic VEGF expression (Baudino et al., 2002). On the other hand, overexpression of MYC is also embryonic lethal due to multiple haemorrhagic lesions and defects in the vasculature, with concomitant elevated VEGF levels (Kokai et al., 2009). Thus, MYC and VEGF levels must be precisely controlled during early development. One key control involves Seryl-TRNA synthetase 1 (SARS1), which competes directly with MYC to control VEGF expression levels, and thus enables proper vasculature development (Shi et al., 2014). In hypoxic conditions, SARS1 is phosphorylated by ATM/ATR, and this impairs its DNA binding capacity, allowing MYC to induce VEGF expression (Shi et al., 2020).
3 Physiological MYC functions hijacked by tumour cells
To become fully transformed and tumorigenic, normal cells must overcome several barriers imposed on cell-autonomous programs such as cell cycle progression, DNA replication, evasion of senescence and apoptosis, as well as cell non-autonomous processes such as angiogenesis and immune surveillance. These constitute many of the Hallmarks of Cancer (Hanahan, 2022) and, as MYC may impinge on all these programs, it is a common target for oncogenic activation. Indeed, although its expression is tightly regulated in normal cells, cancer cells are almost unavoidably characterised by deregulated MYC activity. This can be the result of many different processes such as gene amplification, translocation (Figure 2A), epistasis, epigenetic changes, upstream signalling, and increased protein stability (Figure 2B) (Dhanasekaran et al., 2022). Oncogenic MYC promotes tumorigenesis in different yet complementary ways, co-opting many of the physiological processes described above. Its deregulation is associated to uncontrolled proliferation, rewiring of cellular metabolism, increased ribosomal and protein biogenesis and chromosome instability. MYC also affects cell non-autonomous hallmarks including reshaping of the tumour microenvironment, angiogenesis, induction of immunosuppressive cytokine release, and upregulation of immune checkpoint inhibitor proteins (Whitfield and Soucek, 2012; Dhanasekaran et al., 2022).
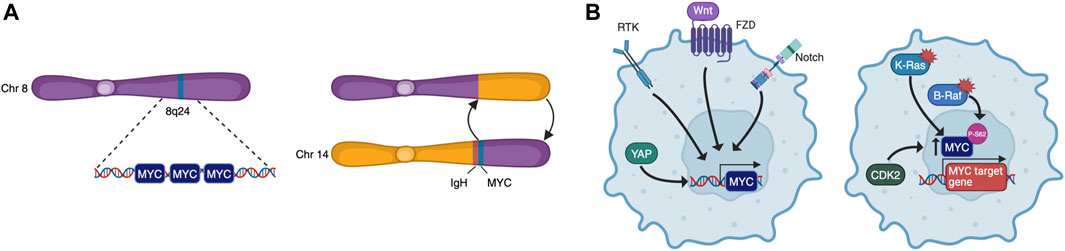
FIGURE 2. MYC is deregulated by multiple mechanisms. These include chromosomal rearrangements that lead to amplification or translocation (A), and upstream signalling that causes increased transcription or protein stabilisation (B).
However, the sole overexpression of MYC is not sufficient for tumorigenesis in most cellular contexts. Indeed, MYC activation usually induces DNA replication and S phase entry without cellular division, hence cells become polyploid, accumulate DNA damage, and undergo proliferative arrest, senescence, or apoptosis depending on the cellular context (Gabay et al., 2014). This is why genetic alterations that circumvent the hurdles imposed by cell cycle checkpoints or apoptosis/senescence usually synergise with MYC overexpression to induce tumorigenesis. This was shown in seminal studies with transgenic mice harbouring tissue-specific inducible forms of MYC. For instance, in MycERTAM mice that express switchable MYC in pancreatic β-cells, MYC activation is sufficient to drive the cells into cell cycle, but unfettered proliferation is constrained by subsequent apoptosis, which quickly results in β-cell loss and diabetes. However, solely by co-expression of the anti-apoptotic protein Bcl-XL, MYC overexpression is then able to drive formation of pancreatic insulinomas (Pelengaris et al., 2002). Similarly, in adult mouse hepatocytes, conditionally expressed MYC leads to polyploidy in the absence of cell division, but concomitant reduction of p53 levels (by crossing with TP53+/− mice) resulted in increased tumorigenesis (Beer et al., 2004).
In this context, MYC expression levels seem an important determinant of the biological outcome. It was reported, for instance, that low levels of deregulated MYC can drive proliferation and oncogenesis by themselves, whereas apoptotic and p53 tumour suppressor pathways are only triggered above a certain MYC threshold (Murphy et al., 2008).
In summary, as observed in cancer but not limited to it, MYC’s role in promoting multiple physiological processes means that its overexpression, deregulation, or insufficiency can lead to an array of human diseases and disorders.
4 MYC’s involvement in diseases and conditions
4.1 Metabolic diseases
Metabolic dysfunction-Associated steatotic liver disease (MASLD), previously known as non-alcoholic fatty liver disease, is strongly associated with obesity and insulin resistance (Browning and Horton, 2004), as well as with increased mortality and cardiovascular disease burden (Kim et al., 2021). It begins with the aberrant accumulation of triglycerides in the liver (steatosis) and can proceed to a Metabolic Dysfunction-Associated Steatohepatitis (MASH), which in turn, can eventually give rise to cirrhosis and liver cancer.
Alb-myctg mice overexpress MYC in hepatocytes, and at 36 weeks, they spontaneously develop metabolic syndrome, characterised by obesity, hypertriglyceridemia, hyper-cholesterolemia, glucose intolerance and insulin resistance. The mouse livers show abnormal accumulation of lipids that leads to compensatory increased ß-oxidation that, in turn, generates oxidative stress. This results in, on the one hand, CD45+, F4/80+ immune infiltration, and on the other, increased hepatocyte apoptosis and compensatory proliferation. Hence, hepatic overexpression of MYC affects metabolism and leads to the development of mild steatohepatitis/fibrosis that progress to liver tumours with long latency. Moreover, MYC overexpression provides a pro-fibrotic tissue environment characterised by moderate but chronic hepatocyte apoptosis, pre-activation of hepatic stellate cells (HSCs) and high basal collagen expression. These HSCs have a high potential to proliferate and to produce extracellular matrix, especially after a second hit. This link between MYC and hepatic fibrosis is reinforced by the fact that MYC mRNA expression was found to be upregulated in patients with liver cirrhosis (Nevzorova et al., 2013).
Alcohol-associated liver disease (ALD) includes a variety of hepatic conditions from steatosis to cirrhosis. In patients with advanced stages of ALD, MYC is strongly upregulated and correlates with the progression of liver fibrosis. In line with this, wild-type mice fed with a Lieber-DeCarli (EtOH) diet showed higher MYC expression at the initial stages of liver injury and MYC remained elevated during the early phase of ALD progression.
MYC overexpression and alcohol consumption were further studied with Alb-myctg mice. Following a 4-week Lieber-DeCarli diet, these mice presented deregulation of multiple disease-related pathways, and an increase in liver mass in the absence of proliferation, accompanied by hepatocyte hypertrophy, enhanced collagen deposition, increased mitochondrial oxygen radicals, and hepatic lipotoxicity. Mitochondrial and ER dysfunction caused metabolic effects involving glucose intolerance. Overall, MYC overexpression in the context of alcohol consumption led to impaired Akt-MDM2-p53 signalling that eventually may trigger ALD progression to fibrosis (Figure 3A) (Nevzorova et al., 2016). To our knowledge, studies to block MYC have not yet been performed in these models.
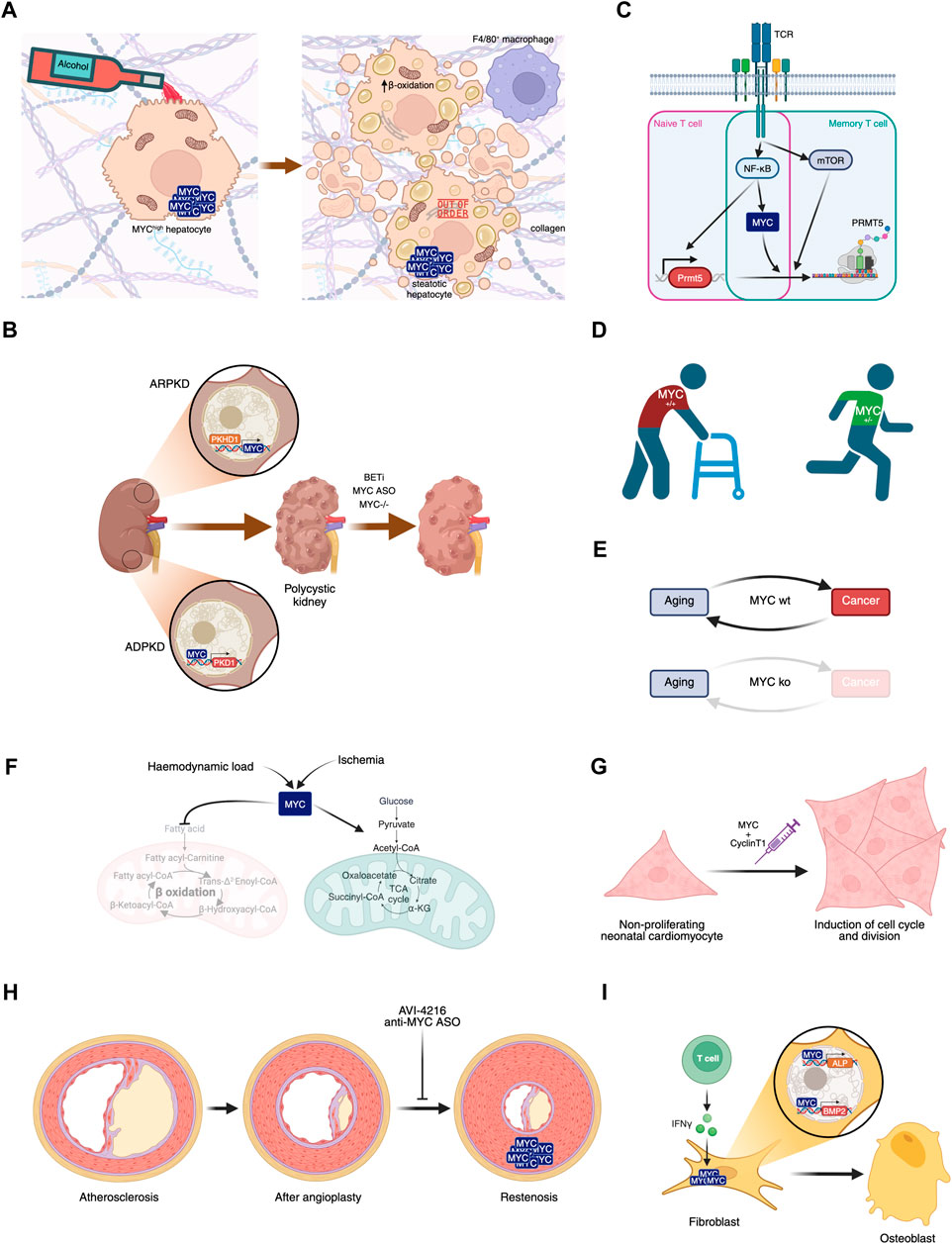
FIGURE 3. Involvement of MYC in diseases. (A) MYC overexpression in hepatocytes, in combination or not with alcohol consumption, leads to liver steatosis. (B) MYC’s role in polycystic kidney disease and its potential inhibition leading to disease amelioration. (C) MYC plays a central role in naive and memory T cell activation in Multiple Sclerosis. (D) MYC haploinsufficiency prevents aged-related phenotypes. (E) MYC knockout after weaning leads to aging in the absence of cancer, disrupting this biunivocal relationship. (F) Haemodynamic load or ischemia lead to MYC-dependent metabolic rewiring in the heart. (G) Transient expression of MYC and Cyclin T1 could have regenerative therapeutic impact in the heart after myocardial infarction. (H) Proliferation of smooth muscle cells leading to restenosis can be prevented by MYC inhibition. (I) IFN-γ-dependent MYC induction of ALP and BMP2 contribute to Ankylosing spondylitis symptoms.
Intestinal MYC is also increased in humans and mice with obesity, likely due to activation of the ß-catenin pathway, of which MYC is a downstream target. In this case, its inhibition has been tested: intestinal-specific MYC disruption protected mice subjected to a high-fat diet against obesity, insulin resistance, hepatic steatosis and fibrosis (Luo et al., 2021). Overall, MYC plays multiple roles in obesity, including the maturation of progenitor cells, fatty acid metabolism and extracellular matrix remodelling. Of note, MYC modulates the inflammatory response, induces insulin resistance, and regulates intestinal dysbiosis (Nevzorova and Cubero, 2023).
Additionally, gerbils fed with a high-fat and high-cholesterol diet showed increased hepatic USP33 expression, whose modulation revealed a signal transduction pathway regulated by both this enzyme and MYC, which controls activation of HSCs, the main cells responsible for liver fibrosis (Ke et al., 2023). In this context, a potential drug treatment of MASH was recently identified: AZD3355, a GABA-B receptor agonist, proved to be anti-fibrotic, anti-inflammatory and hepatoprotective, and interestingly, MYC was identified as the top transcription factor regulated in HSCs treated in vitro with AZD3355 (Bhattacharya et al., 2021). All these data are all in line with a role for MYC in HSC activation and prompt the testing of MYC inhibitors in disease models.
Intriguingly, though, MYC expression in endothelial cells was shown to have a protective effect against diet-induced liver inflammation and fibrosis. In vitro, knockdown of MYC in human umbilical vein endothelial cells (HUVECs) induces cellular senescence accompanied by a proinflammatory senescence associated secretory phenotype (SASP) (Florea et al., 2013). In vivo, loss of endothelial MYC induced a significant increase in proinflammatory molecules CCL7 and osteopontin. Moreover, under a high fat diet, mice with MYC−/− endothelial cells showed transcriptional induction of inflammation-associated pathways characterised by an increase in neutrophil and macrophage infiltration and the secretion of chemo- and cytokines CCL11, CXCL1 and IL-17, all of which have a role in liver inflammation and MASH. Moreover, transcriptional analysis of endothelial cells from MYC knockout mice showed functions associated with liver hyperplasia/hyperproliferation and hepatocellular carcinoma. These findings are in line with scRNA analyses showing that endothelial MYC expression was downregulated in male cirrhotic livers compared to those of healthy individuals (Qi et al., 2022). Whether endothelial MYC knockdown in vivo induces senescence, which is related to inflammation and cancer, was not evaluated.
4.2 Polycystic kidney disease
Polycystic kidney disease (PKD) is a group of genetic disorders characterised by the progressive development of renal cysts. It can be autosomal dominant (ADPKD) or autosomal recessive (ARPKD), and the dominant form affects some 1 in 500–1000 people. A role for MYC in the pathogenesis of PKD was suggested by work using the spontaneous congenital polycystic kidney Cys1cpk/cpk (cpk) mutant mouse that phenocopies human ARPKD. In this model, MYC overexpression was detected in polycystic kidneys, with only a minimal increase in proliferation, and also in collecting duct epithelial cells (Cowley et al., 1987; Harding et al., 1992). Notably, in vivo treatment with a c-MYC antisense oligomer (ASO) decreased cpk mouse kidney weight, improved their renal function and decreased the number of cysts, pointing to a therapeutic effect of MYC inhibition (Figure 3B) (Ricker et al., 2002).
Cystin, encoded by Cys1, is a lipid-microdomain associated protein found in the primary cilium of renal epithelia cells (Yoder et al., 2002) that binds to the MYC promoter and regulates its expression (Wu et al., 2013). While the loss of Cystin’s proper function increases MYC expression, transgenic complementation with Cystin-GFP expression rescues the phenotype with concomitant normalisation of MYC levels (Yang et al., 2021). Also, MYC is overexpressed in kidneys from ARPKD. More in detail, fibrocystin/poliductin protein localise to the nucleus, binds to MYC promoter P1 and activates its expression (Figure 3B) (Harafuji et al., 2023).
Others have reported further links between MYC and PKD. The SBM transgenic mouse model, with an SV40 promoter and beta-globin enhancer that drives MYC overexpression in renal epithelial cells, bears similarities with human ADPKD, which is mainly caused by mutations in the gene PKD1, encoding for polycystin-1 (PC1). These mice show significant upregulation of PC1 and develop PKD with 100% penetrance that leads to fatal renal failure. Examination of the kidneys revealed higher levels of MYC expression in the epithelial lining of cystic and hyperplastic tubules (Trudel et al., 1991).
SBPkd1TAG mice overexpress PKD1 mRNA leading to increased PC1 dosage in renal epithelial cells and exhibit a moderate rate of disease progression that leads to renal failure at five to six months. On the other hand, PC1 dosage-reduced Pkd1-cKO mice develop enlarged cystic kidneys that become very severe by P10 and leads to death due to renal failure. Puzzlingly, in both PC1 dosage-increased and -reduced mice, MYC expression (along with that of β-catenin) was found to be upregulated in renal cells with respect to wild-type mice. Moreover, MYC was found to be enriched in PKD1 promoter regions in adult SBM mouse kidneys, while overexpression of MYC in HEK293 embryonic kidney cells increased the levels of PC1. These data suggest that PKD1 expression is driven at least in part by MYC (Figure 3B) and unveils an inter-regulatory network involving MYC and PC1 that controls cystogenesis (Parrot et al., 2019).
While direct exogenous MYC inhibitors were not applied in these models, either genetic renal-specific ablation of MYC (Parrot et al., 2019), or treatment with inhibitors of BET bromodomain protein 4, an upstream regulator of MYC, reduced disease severity or delayed PKD progression (Zhou et al., 2015). Similarly, loss of MYC suppressed cystogenesis in a Pkd1-KO mouse model (Figure 3B) (Cai et al., 2018).
Combined, these data point to MYC as a causal cystogenic factor and a mediator of ADPKD. Its inhibition is therefore a potential therapy and further testing of inhibitors is highly warranted.
4.3 Multiple sclerosis
Multiple Sclerosis (MS) is an autoimmune disease of the central nervous system (CNS) characterised by the self-reactive T cell-induced demyelination. T cells recognise antigens on myelin basic protein, myelin oligodendrocyte glycoprotein and proteolipid protein, and immunization against these antigens induces the MS-like experimental autoimmune encephalomyelitis disease (EAE) in mice. More than 10 years ago, genome-wide association studies in MS patients identified single nucleotide polymorphisms in the MYC gene (International Multiple Sclerosis Genetics Consortium et al., 2011). In recent years, a series of papers have linked MYC’s transcriptional activity to T cell activation in MS.
First, MYC, together with NF-κB and mTOR, was found to be involved in the activation of memory Th and naïve T cells in EAE through the induction of PRMT5, an arginine methyltransferase that plays a crucial role in inflammatory T cell expansion and EAE disease (Webb et al., 2017; Webb et al., 2019). This constitutes another example of a positive interaction of MYC and mTOR, in contrast to UPR in osteoclastogenesis. Here, MYC’s role was demonstrated using the small molecule inhibitor 10058-F4.
Second, MYC transcriptional activation through phospho-STAT3 and RelA/NF-κB mediates T cell receptor-independent downstream signalling from activated CD28 that leads to inflammatory T cell responses in MS (Figure 3C) (Kunkl et al., 2019).
Finally, bioinformatic analyses of protein-protein interaction networks in MS found common genes and biological pathways for disease susceptibility, among which MYC was found to be a central gene in peripheral blood mononuclear cells from MS patients (Safari-Alighiarloo et al., 2020). A similar study confirmed the role of MYC, along with HNF4α and SP1, as a master regulator of CNS autoimmunity (Colombo et al., 2023). In this case, MYC was inhibited using OTX015, an inhibitor of BET domain proteins that indirectly decreases MYC levels. This inhibitor has been tested in clinical trials (in oncological indications), although it is not specific for MYC only. Further preclinical validation of the role of MYC and the potential of MYC inhibition in MS seems warranted.
4.4 Aging
Many of the biological processes implicated in or associated with aging have also been linked to MYC and its deregulation. These include the so-called hallmarks of aging (López-Otín et al., 2023): genomic instability, epigenetic alterations, stem cell exhaustion, energy production, protein translation, DNA damage, and inflammation. Transgenic mice have been used to explore the impact of systemic MYC level reduction, but so far there are contrasting results. Initially, MYC haploinsufficiency studies showed that MYC+/− mice had significantly extended lifespans with amelioration of aging phenotypes across a variety of pathophysiological processes when compared to wild-type littermates. These included healthier lipid and cholesterol metabolism, less fibrosis and cancer progression, higher metabolic rate and less immunosenescence. The exact mechanism(s) behind this have not yet been established, although they are expected to be multifactorial, through decreased expression of direct MYC targets, or indirectly through other transcription factors and/or miRNAs regulated by MYC. For instance, ribosomal RPL and RPS genes were found to be reduced in MYC haploinsufficient tissues with the concomitant reduction of in vivo translation, which is clearly associated with longer lifespan (Figure 3D) (Hofmann et al., 2015). Additionally, these mice showed decreased systemic levels of IGF1 through MYC-miR122 regulation. Reduced IGF1 has been linked to the development of age-related diseases such as osteoporosis, but female MYC haploinsufficient mice had a decreased incidence of osteoporosis, consistent with the finding that, in bone, IGF1 levels were unaltered (Petrashen et al., 2023).
The current understanding of aging considers it as a multifactorial process in which different signalling pathways converge on autophagy genes to regulate lifespan. WIPI1, and its C. elegans ortholog ATG-18, has been identified as one of the critical autophagic factors involved in extending lifespan (Tóth et al., 2008). In line with this and with MYC’s supposed role in aging, it was found that the ABL-MYC axis represses WIPI1 gene expression. Interfering with this axis promotes autophagy and extends C. elegans lifespan (Sporbeck et al., 2023).
On the other hand and in contrast with the results above, transgenic mice engineered with near-complete elimination of MYC at weaning, named MycKO mice, aged prematurely yet lived longer with decreased cancer incidence. The phenotypic alterations were, as expected, copious and broad: bone marrow hypoplasia, peripheral cytopenia, alopecia, achromotrichia, glucose intolerance and mitochondrial dysfunction. Additionally, colonic epithelial flattening and villous atrophy were found, although there was no effect on body weight (Prochownik and Wang, 2023; Wang et al., 2023).
Hence, according to these latter results, aging appears to be associated with higher cancer incidence only in the presence of MYC (Figure 3E). It remains to be seen what effect chronic administration of MYC inhibitors could have on indicators of aging. Whether such chronic treatment could also be applied as a cancer prevention strategy (and not only to cancer treatment) is not clear beyond preclinical models.
4.5 Cardiac metabolism after pathological stress
Cellular oxygen concentrations are tightly regulated in eukaryotic organisms to maintain proper mitochondrial function and energy production. Mammalian cells adapt to oxygen deprivation by inducing protective mechanisms. For instance, a substantial decrease in protein biosynthesis is among the effects of hypoxic stress on cardiomyocytes, where transcription factor IIIB (TFIIIB) and TFIIIC-dependent RNA polymerase III (pol III) play a key role (Kraggerud et al., 1995; Schramm and Hernandez, 2002). In vitro experiments with neonatal rat myocytes at 1% O2 revealed that HIF-1α induces the dissociation of MYC from TFIIIB, contributing to the decrease in pol III transcription (Ernens, 2006). Other pathological stressors such as haemodynamic load and ischemia divert metabolic pathways away from fatty acid oxidation (FAO) towards glucose metabolism (Stanley et al., 2005). In the adult heart, this metabolic rewiring in the myocardium is mediated by MYC, whose increased levels downregulate genes involved in FAO, while concomitantly upregulating genes mediating glucose oxidation, such as ENO1, PFKM, LDHA and SLC16A1 (Figure 3F). This was associated also with an increase in the number of functional mitochondria and represents MYC-dependent metabolic adaptation towards a better response to ischemic insults (Ahuja et al., 2010).
Cardiac progenitor cells (CPCs), however, become quiescent after ischemic hypoxia, limiting their self-renewal and vasculogenic properties, with the aim of preserving stem cell homeostasis (Guitart et al., 2010). Being a master regulator of the cell cycle and quiescence, it is no surprise that MYC, after in vitro hypoxia (0.5% O2), is downregulated in mouse CPCs isolated from the myocardium, with a concomitant increase in the levels of the CDK inhibitor p21, a MYC target (Bellio et al., 2017).
Neonatal cardiac proliferative potential is lost after a week, coinciding with downregulation of multiple genes involved in cell cycle, including MYC (Walsh et al., 2010; Quaife-Ryan et al., 2017). Ectopic cardiac MYC-dependent transcription and cell cycle progression in the adult heart in vivo depends on the levels of P-TEFb, a protein complex consisting of CDK9 and Cyclin T1. In order to effectively drive cell division in the heart, MYC expression must be accompanied by higher levels of P-TEFb (Bywater et al., 2020). In line with this, transient expression of both MYC and Cyclin T1 by a single intramyocardial dose of a modified RNA coding for both genes was shown to be a potential regenerative therapeutic in the heart after myocardial infarction, inducing cell cycle and division of cardiomyocytes (Figure 3G) (Boikova et al., 2023).
Restoration of reperfusion is the most effective treatment for myocardial infarction. Paradoxically though, reperfusion leads to myocardial ischemia/reperfusion (MI/R) injury, which induces cardiomyocyte apoptosis through increased oxidative stress (Wang et al., 2017). Using an MI/R mouse model, MYC was found to be downregulated, with consequent oxidative stress and cardiomyocyte apoptosis (Wen et al., 2022). Notably, therefore, recovery after ischaemia using these regenerative or protective strategies represents one of the few conditions in which therapy would require MYC expression or activation.
Hypertension is one of the most common pathologies of the vascular system. It leads to overload, increasing the risk of myocardial infarction, among others. The myocardium of spontaneously hypertensive rats (SHRs) overexpresses MYC and its downstream target CYP2E1, whose overexpression leads to oxidative stress and other pathological processes. Long-term treatment with quercetin, a flavonoid with potential cardiovascular beneficial effects, resulted in a significant reduction of blood pressure with concomitant downregulation of MYC and CYP2E1, significantly improving the prooxidant-antioxidant profile (Maksymchuk et al., 2023). Whether downregulation of MYC alone would reduce blood pressure, CYP2E1 expression, and curb the oxidative stress, still remains to be seen.
4.6 Restenosis
The arterial wall response to pathophysiological stimuli, including atherosclerosis and angioplasty procedures, involves the proliferation of smooth muscle cells (SMC). Indeed, 25%–50% of patients undergoing angioplasty will develop recurrent stenosis, which is essentially a narrowing of the blood vessels, also called restenosis, that consists of the proliferation of medial SMC and their migration to the subintima. Because of this, considerable attention has been paid to the inhibition of SMC proliferation as a way of preventing restenosis. Initial studies involving antisense oligonucleotides (ASOs) targeted SMC PCNA in vitro with significant inhibition of proliferation (Speir and Epstein, 1992). Much later studies focused on the use of a phosphorodiamidate morpholino oligomer (PMO) antisense to the c-MYC translation initiation site, called AVI-4126 (Resten-NG®) (Figure 3H). It was successfully tested in a rabbit balloon injury model (Kipshidze et al., 2002) and porcine restenosis model (Kipshidze et al., 2003; Kipshidze et al., 2002) with promising results: significant reduction of the neointimal area with concomitant MYC inhibition. Although this was further validated in a Phase II trial with positive results (Philipp, 2012), the drug was not developed beyond this point.
4.7 Bone developmental disorders
Septic nonunion (SN) is a bone disorder caused by the failure of fracture healing. It is often caused by local inflammation. Expression of the lncRNA RUNX2-AS1 was detected in SN biopsies, along with proinflammatory cytokines. RUNX2-AS1 negatively regulates RUNX2 expression and its downstream targets, which play an important role in bone differentiation and development. It was found that MYC associates with MAX, p300 and NCOA2 to induce RUNX2-AS1 expression, abrogating the expression of RUNX2 target genes, while LPS-induced inflammation induced the expression of NCOA2 and showed a dose-dependent increased association with MYC-MAX-p300. These results link the inflammatory microenvironment with the downregulation of RUNX2 and its target genes, which impairs bone differentiation and leads to nonunion (Li and Qian, 2022).
Ankylosing spondylitis (AS) is a heritable chronic inflammatory disease that affects the spine and pelvis, ultimately leading to joint ankylosis due to ectopic ossification and disability. Inflammation is an early characteristic of AS and inflammatory cytokines could promote ossification by modulating the osteoblasts (Li et al., 2020). MYC was found to be upregulated in AS ligament samples and in fibroblasts in an in vitro osteogenic model. In this model, two osteogenic genes were found to be dependent on MYC: alkaline phosphatase (ALP) and bone morphogenetic protein 2 (BMP2). Additionally, the inflammatory cytokines IL-23 and IFN-γ upregulate both MYC and ALP in vitro. In AS ligament samples, a higher proportion of IL-23 positive and IFN-γ positive cells were found with respect to osteoarthritis samples (Figure 3I) (Jin et al., 2023). Osteoporosis has also been linked to MYC. Based on bioinformatic analysis, a series of experiments showed that the MYC/ERRα axis regulates mitochondrial respiration in osteoclastogenesis, and their targeting protected mice of oestrogen deficiency-mediated bone loss after ovariectomy, pointing to MYC as a potential therapeutic target for osteoporosis (Bae et al., 2017).
4.8 Potential role of MYC in other diseases and conditions
Various studies link MYC to a range of other disorders, mainly through experiments to determine its expression in model systems or patients. In particular—and not surprisingly—there are strong suggestions of a role in endometriosis (Nothnick et al., 2023), mitochondrial diseases (Purhonen et al., 2023), immune-related, neurodegenerative and other metabolism-related diseases. These are summarised in Table 1 with some of the data hinting at a role for MYC. In general, more work is required to prove a clear link and determine whether MYC is playing a role in disease causation, or even whether modulation of its expression could be preventative.
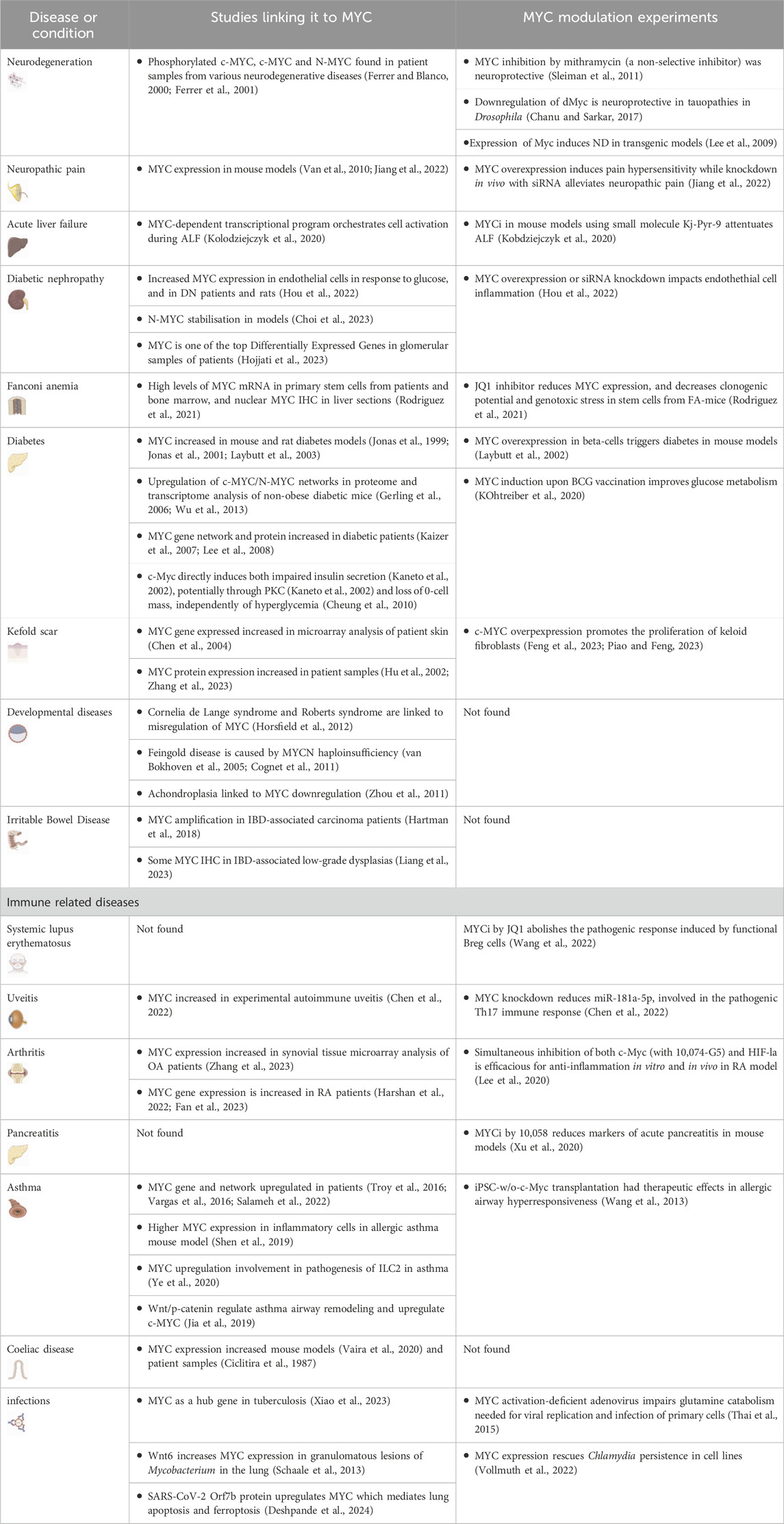
TABLE 1. Additional diseases and conditions in which MYC has been implicated. In these cases, the evidence is more preliminary than for those described in the main text. We have indicated studies showing any links between MYC and the disease, and in particular, any data regarding MYC modulation, either by inhibition or overexpression.
5 Current state of MYC inhibition in the clinical setting
There are a huge number of reviews describing the search for MYC inhibitors and their application to cancer treatment. Here we will only briefly describe some targeting strategies, focusing on those reaching clinical testing, summarised in Figure 4, and refer the reader to a number of other much more in-depth reviews regarding MYC inhibitors, both from our group (Whitfield et al., 2017; Massó-Vallés and Soucek, 2020; Whitfield and Soucek, 2021; Martínez-Martín et al., 2023) and others (Ross et al., 2021; Karadkhelkar et al., 2023; Weber and Hartl, 2023).
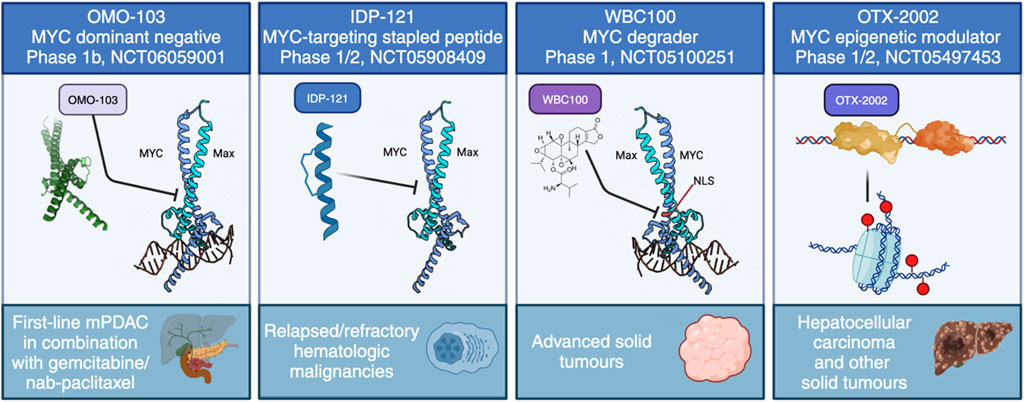
FIGURE 4. The current approaches to directly target MYC in clinical trials. These include four distinct strategies: MYC dominant negative OMO-103, anti-MYC stapled peptide IDP-121, MYC degrader WBC100 and MYC epigenetic modulator OTX-2002.
Strategies employed fall into two main approaches: direct and indirect inhibitors. The latter include a much more expansive set of possibilities, since their target can be anything that interacts with MYC or controls its activity, expression, or localisation. These could also include synthetic lethal targets: here, any protein or signalling pathway that is essential for the survival of MYC-driven tumour cells can be targeted and many such targets are in clinical development. Direct inhibitors, on the other hand, impinge on MYC itself to control the expression or stability of the RNA or protein, or its interaction with DNA or dimeric partners.
Perhaps surprisingly, the earliest MYC inhibitors to be tested in clinical trials were applied to a non-oncological indication (Kipshidze et al., 2002). Antisense oligonucleotides (ASOs) were used for the treatment of heart restenosis (NCT00244647, NCT00248066) (Kipshidze et al., 2007). These showed some positive effects in this coronary disease and were later tested against neoplasms, showing significant tissue accumulation in solid tumours (Devi et al., 2005), but to our knowledge no further development occurred.
Other more recent trials have also been discontinued, including: Quarfloxin (CX-3543) and APTO-253, G-quadruplex stabilisers thought to work by preventing MYC transcription (NCT00780663, NCT02267863); INX-3280, another ASO (Kutryk et al., 2002); and DCR-MYC, an siRNA to prevent MYC translation (NCT02110563, NCT02314052). These were all tested as cancer therapies (reviewed in Whitfield et al., 2017), but none was further pursued.
The first successful Phase I trial has recently concluded using OMO-103, a MYC dominant negative mutant based on the Omomyc mini-protein, delivered intravenously once per week. In line with its extensive preclinical validations, OMO-103 showed a good safety profile and some first hints at efficacy in all-comers solid tumours (Garralda et al., 2024). In addition, biomarkers were identified indicating MYC inhibition. A Phase Ib trial recently started in September 2023 in metastatic pancreatic cancer (NCT06059001).
To our knowledge, there are currently three other ongoing trials with a direct MYC inhibitor: one uses a MYC degrader called WBC100, in MYC-positive advanced solid tumours (NCT05100251), another is an epigenetic controller, OTX-2002, that downregulates MYC and is being tested in hepatocellular carcinoma (NCT05497453), while the third is with IDP-121, a stapled peptide MYC inhibitor being evaluated in patients with relapsed/refractory hematologic malignancies (NCT05908409). In addition, MYC-related indirect approaches have reached clinical trials. For instance, MYC-induced protein translation depends on GSPT1, and a molecular glue degrader of GSPT1 (MRT-2359) is currently being trialled for MYC-driven and other selected solid tumours (NCT05546268). Still, the focus remains firmly on testing in cancer.
As discussed already, any approved inhibitor could potentially be applied to other non-neoplastic conditions.
6 Possibilities to activate or express MYC
As explained in the previous section, the majority of examples of MYC involvement in diseases point to its inhibition as a therapeutic approach. However, MYC activation could be an option to favour regeneration in the heart after myocardial infarction or hypoxia. Further to such repair and regeneration approaches, a recent study highlighted the use of MYC activation by transgenic overexpression to stimulate ex vivo platelet production from induced pluripotent stem cells (Kayama and Eto, 2024). This could eventually provide improved transfusion systems. Additionally, an unexpected indirect approach could benefit Type 1 Diabetes (T1D) patients, in which the administration of BCG vaccine resulted in long-lasting blood sugar control with proper glucose metabolism (Li et al., 2018). A recent study found a gradual MYC mRNA upregulation in monocytes and CD4 T cells from T1D patients. This led to increased transcription of MYC-dependent glucose and glutamine metabolism genes (Kühtreiber et al., 2020).
7 Perspective
While MYC has long been considered an undruggable target, new therapeutic options against it are becoming clinically viable, as demonstrated by the completion of the first successful clinical trial of a direct inhibitor, OMO-103. Most of the trials and recent focus remains in the field of cancer treatment, and indeed the ongoing trials of direct MYC inhibitors are against PDAC, hepatocellular carcinoma, relapsed/refractory hematologic malignancies, and MYC-positive advanced solid tumours. As mentioned in this review, though, MYC’s pleiotropic roles in multiple physiological processes suggest that its modulation could be applied to many other diseases. To date, there is preliminary data pointing to a role in a variety of diseases of different origins and clinical presentations such as neurodegeneration, diseases of the bone, digestive system and related organs, keloid scars, developmental and immune-related diseases (such as asthma, coeliac disease, and others), as well as the aging process. Further pre-clinical testing and even clinical trials seem merited in these cases.
In general, excess or over-active MYC is detrimental, so under physiological circumstances its levels are precisely controlled to keep the multiple downstream processes in check. In most diseases described so far, and as seen in cancer, where deregulation of MYC is frequent, such excessive MYC activity drives various processes that then lead to pathologies due to the unfettered proliferation, changes in differentiation and altered metabolism, among others. There is huge potential, therefore, for using MYC inhibitors that are currently being developed in the cancer field.
Diseases in which MYC activation may instead be desired include those where stimulation of cell proliferation and tissue regeneration is needed, such as after ischaemic damage in the heart, diabetes, and neuronal repair. It has been speculated that in neurodegeneration, MYC activation may be part of a failed neuroprotective response. Thus, extra MYC could help repair and regenerate neurons after cell death or damage. Of note, activation of MYC for such diseases will likely be required locally, in the affected tissues rather than systemic, to avoid the foreseeable massive and deleterious effects that body-wide activated MYC could have.
In summary, if we have learnt something from 40 years of literature about MYC, it is that we still have a lot to discover. Luckily, pharmacological tools for its modulation seem finally viable and hold promise for a better understanding of MYC biology, while also providing the basis for new therapeutics applicable to multiple indications in oncology and beyond.
Author contributions
Resources: LS. Conceptualisation: JW, MZ-F. Writing original draft: MZ-F, JW. Writing–review and editing: JW, MZ-F, LS. Funding acquisition: LS, JW, MZ-F.
Funding
The author(s) declare that financial support was received for the research, authorship, and/or publication of this article. Authors acknowledge funding support from the Spanish Ministry of Science and Innovation (Fondo de Investigación en Salud [FIS] PI19/01277, the State Agency for Research (Agencia Estatal de Investigación) as a Center of Excellence Severo Ochoa (CEX 2020-001024-S/AEI/10.13039/501100011033), the Generalitat de Catalunya (AGAUR 2021/SGR 01509), and the FERO foundation.
Acknowledgments
VHIO would like to acknowledge the Cellex Foundation for providing research facilities and equipment and the CERCA Programme from the Generalitat de Catalunya for their support. All the illustrations used in the figures were created with BioRender.com, some of them modified with the free and open-source raster graphics editor GIMP (GNU Image Manipulation Program, https://www.gimp.org) version 2.10.36. The bottle icon (Figure 3A) is from www.flaticon.com and the crystal structure of MYC and MAX in complex with DNA (Figure 1) was created by Wikipedia user “Mark ‘AbsturZ'” (CC BY-SA 3.0 DEED). Figure layouts were created with Inkscape, free and open-source vector graphics editor (https://inkscape.org/), version 1.3. Images used in the table are from Servier Medical Art (https://smart.servier.com/) and (BioRender.com).
Conflict of interest
All authors are shareholders of Peptomyc SL; LS is CEO and founder of Peptomyc SL.
The remaining authors declare that the research was conducted in the absence of any commercial or financial relationships that could be construed as a potential conflict of interest.
Publisher’s note
All claims expressed in this article are solely those of the authors and do not necessarily represent those of their affiliated organizations, or those of the publisher, the editors and the reviewers. Any product that may be evaluated in this article, or claim that may be made by its manufacturer, is not guaranteed or endorsed by the publisher.
References
Abrams, H. D., Rohrschneider, L. R., and Eisenman, R. N. (1982). Nuclear location of the putative transforming protein of avian myelocytomatosis virus. Cell. 29, 427–439. doi:10.1016/0092-8674(82)90159-3
Ahuja, P., Zhao, P., Angelis, E., Ruan, H., Korge, P., Olson, A., et al. (2010). Myc controls transcriptional regulation of cardiac metabolism and mitochondrial biogenesis in response to pathological stress in mice. J. Clin. Investig. 120, 1494–1505. doi:10.1172/JCI38331
Amati, B., Dalton, S., Brooks, M. W., Littlewood, T. D., Evan, G. I., and Land, H. (1992). Transcriptional activation by the human c-Myc oncoprotein in yeast requires interaction with Max. Nature 359, 423–426. doi:10.1038/359423a0
Bae, S., Lee, M. J., Mun, S. H., Giannopoulou, E. G., Yong-Gonzalez, V., Cross, J. R., et al. (2017). MYC-dependent oxidative metabolism regulates osteoclastogenesis via nuclear receptor ERRα. J. Clin. Investig. 127, 2555–2568. doi:10.1172/JCI89935
Bae, S., Oh, B., Tsai, J., Park, P. S. U., Greenblatt, M. B., Giannopoulou, E. G., et al. (2022). The crosstalk between MYC and mTORC1 during osteoclastogenesis. Front. Cell. Dev. Biol. 10, 920683. doi:10.3389/fcell.2022.920683
Battaglino, R., Kim, D., Fu, J., Vaage, B., Fu, X. Y., and Stashenko, P. (2002). c-myc is required for osteoclast differentiation. J. Bone Min. Res. 17, 763–773. doi:10.1359/jbmr.2002.17.5.763
Baudino, T. A., McKay, C., Pendeville-Samain, H., Nilsson, J. A., Maclean, K. H., White, E. L., et al. (2002). c-Myc is essential for vasculogenesis and angiogenesis during development and tumor progression. Genes. Dev. 16, 2530–2543. doi:10.1101/gad.1024602
Beaulieu, M.-E., Castillo, F., and Soucek, L. (2020). Structural and biophysical insights into the function of the intrinsically disordered myc oncoprotein. Cells 9, 1038. doi:10.3390/cells9041038
Beer, S., Zetterberg, A., Ihrie, R. A., McTaggart, R. A., Yang, Q., Bradon, N., et al. (2004). Developmental context determines latency of MYC-induced tumorigenesis. PLoS Biol. 2, e332. doi:10.1371/journal.pbio.0020332
Bellio, M. A., Pinto, M. T., Florea, V., Barrios, P. A., Taylor, C. N., Brown, A. B., et al. (2017). Hypoxic stress decreases c-myc protein stability in cardiac progenitor cells inducing quiescence and compromising their proliferative and vasculogenic potential. Sci. Rep. 7, 9702. doi:10.1038/s41598-017-09813-x
Bettess, M. D., Dubois, N., Murphy, M. J., Dubey, C., Roger, C., Robine, S., et al. (2005). c-Myc is required for the formation of intestinal crypts but dispensable for homeostasis of the adult intestinal epithelium. Mol. Cell. Biol. 25, 7868–7878. doi:10.1128/MCB.25.17.7868-7878.2005
Bhattacharya, D., Becker, C., Readhead, B., Goossens, N., Novik, J., Fiel, M. I., et al. (2021). Repositioning of a novel GABA-B receptor agonist, AZD3355 (Lesogaberan), for the treatment of non-alcoholic steatohepatitis. Sci. Rep. 11, 20827. doi:10.1038/s41598-021-99008-2
Blackwood, E. M., and Eisenman, R. N. (1991). Max: a helix-loop-helix zipper protein that forms a sequence-specific DNA-binding complex with myc. Science 251, 1211–1217. doi:10.1126/science.2006410
Boikova, A., Quaife-Ryan, G. A., Batho, C. A. P., Lawrence, E., Robinson, H., Ascanelli, C., et al. (2023). A transient modified mRNA encoding Myc and Cyclin T1 induces cardiac regeneration and improves cardiac function after myocardial injury (preprint). Mol. Biol. doi:10.1101/2023.08.02.551469
Bonal, C., Thorel, F., Ait-Lounis, A., Reith, W., Trumpp, A., and Herrera, P. L. (2009). Pancreatic inactivation of c-Myc decreases acinar mass and transdifferentiates acinar cells into adipocytes in mice. Gastroenterology 136, 309–319. doi:10.1053/j.gastro.2008.10.015
Brown, S. J., Cole, M. D., and Erives, A. J. (2008). Evolution of the holozoan ribosome biogenesis regulon. BMC Genomics 9, 442. doi:10.1186/1471-2164-9-442
Browning, J. D., and Horton, J. D. (2004). Molecular mediators of hepatic steatosis and liver injury. J. Clin. Investig. 114, 147–152. doi:10.1172/JCI22422
Bywater, M. J., Burkhart, D. L., Straube, J., Sabò, A., Pendino, V., Hudson, J. E., et al. (2020). Reactivation of Myc transcription in the mouse heart unlocks its proliferative capacity. Nat. Commun. 11, 1827. doi:10.1038/s41467-020-15552-x
Cai, J., Song, X., Wang, W., Watnick, T., Pei, Y., Qian, F., et al. (2018). A RhoA-YAP-c-Myc signaling axis promotes the development of polycystic kidney disease. Genes. Dev. 32, 781–793. doi:10.1101/gad.315127.118
Chanu, S. I., and Sarkar, S. (2017). Targeted downregulation of dMyc suppresses pathogenesis of human neuronal tauopathies in Drosophila by limiting heterochromatin relaxation and tau hyperphosphorylation. Mol. Neurobiol. 54, 2706–2719. doi:10.1007/s12035-016-9858-6
Chen, S., Ma, B., Li, X., Zhang, K., Wei, Y., Du, B., et al. (2022). MYC-mediated silencing of miR-181a-5p promotes pathogenic Th17 responses by modulating AKT3-FOXO3 signaling. iScience 25, 105176. doi:10.1016/j.isci.2022.105176
Chen, W., Fu, X., Ge, S., Sun, X., Zhou, G., Zhao, Z., et al. (2004). Development of gene microarray in screening differently expressed genes in keloid and normal-control skin. Chin. Med. J. Engl. 117, 877–881.
Cheung, L., Zervou, S., Mattsson, G., Abouna, S., Zhou, L., Ifandi, V., et al. (2010). c-Myc directly induces both impaired insulin secretion and loss of β-cell mass, independently of hyperglycemia in vivo. Islets 2, 37–45. doi:10.4161/isl.2.1.10196
Choi, S., Hong, S. P., Bae, J. H., Suh, S. H., Bae, H., Kang, K. P., et al. (2023). Hyperactivation of YAP/TAZ drives alterations in mesangial cells through stabilization of N-myc in diabetic nephropathy. J. Am. Soc. Nephrol. 34, 809–828. doi:10.1681/ASN.0000000000000075
Ciclitira, P. J., Stewart, J., Evan, G., Wight, D. G., and Sikora, K. (1987). Expression of c-myc oncogene in coeliac disease. J. Clin. Pathol. 40, 307–311. doi:10.1136/jcp.40.3.307
Colombo, E., Di Dario, M., Menon, R., Valente, M. M., Bassani, C., Sarno, N., et al. (2023). HNF4α, SP1 and c-myc are master regulators of CNS autoimmunity. J. Autoimmun. 138, 103053. doi:10.1016/j.jaut.2023.103053
Cowley, B. D., Smardo, F. L., Grantham, J. J., and Calvet, J. P. (1987). Elevated c-myc protooncogene expression in autosomal recessive polycystic kidney disease. Proc. Natl. Acad. Sci. U. S. A. 84, 8394–8398. doi:10.1073/pnas.84.23.8394
Dang, C. V., McGuire, M., Buckmire, M., and Lee, W. M. F. (1989). Involvement of the “leucine zipper” region in the oligomerization and transforming activity of human c-myc protein. Nature 337, 664–666. doi:10.1038/337664a0
Deisenroth, C., Black, M. B., Pendse, S., Pluta, L., Witherspoon, S. M., McMullen, P. D., et al. (2014). MYC is an early response regulator of human adipogenesis in adipose stem cells. PLoS One 9, e114133. doi:10.1371/journal.pone.0114133
Deshpande, R., Li, W., Li, T., Fanning, K. V., Clemens, Z., Nyunoya, T., et al. (2024). SARS-CoV-2 accessory protein Orf7b induces lung injury via c-myc mediated apoptosis and ferroptosis. IJMS 25, 1157. doi:10.3390/ijms25021157
Devi, G. R., Beer, T. M., Corless, C. L., Arora, V., Weller, D. L., and Iversen, P. L. (2005). In vivo bioavailability and pharmacokinetics of a c-MYC antisense phosphorodiamidate morpholino oligomer, AVI-4126, in solid tumors. Clin. Cancer Res. 11, 3930–3938. doi:10.1158/1078-0432.CCR-04-2091
Dhanasekaran, R., Deutzmann, A., Mahauad-Fernandez, W. D., Hansen, A. S., Gouw, A. M., and Felsher, D. W. (2022). The MYC oncogene - the grand orchestrator of cancer growth and immune evasion. Nat. Rev. Clin. Oncol. 19, 23–36. doi:10.1038/s41571-021-00549-2
Duesberg, P. H., Bister, K., and Vogt, P. K. (1977). The RNA of avian acute leukemia virus MC29. Proc. Natl. Acad. Sci. U. S. A. 74, 4320–4324. doi:10.1073/pnas.74.10.4320
Edmunds, L. R., Sharma, L., Kang, A., Lu, J., Vockley, J., Basu, S., et al. (2014). c-Myc programs fatty acid metabolism and dictates acetyl-CoA abundance and fate. J. Biol. Chem. 289, 25382–25392. doi:10.1074/jbc.M114.580662
Ernens, I., Goodfellow, S. J., Innes, F., Kenneth, N. S., Derblay, L. E., White, R. J., et al. (2006). Hypoxic stress suppresses RNA polymerase III recruitment and tRNA gene transcription in cardiomyocytes. Nucleic Acids Res. 34, 286–294. doi:10.1093/nar/gkj402
Evan, G., Harrington, E., Fanidi, A., Land, H., Amati, B., and Bennett, M. (1994). Integrated control of cell proliferation and cell death by the c-myc oncogene. Philos. Trans. R. Soc. Lond B Biol. Sci. 345, 269–275. doi:10.1098/rstb.1994.0105
Fan, D.-D., Tan, P.-Y., Jin, L., Qu, Y., and Yu, Q.-H. (2023). Bioinformatic identification and validation of autophagy-related genes in rheumatoid arthritis. Clin. Rheumatol. 42, 741–750. doi:10.1007/s10067-022-06399-2
Fan, W., and Li, X. (2023). The SIRT1-c-Myc axis in regulation of stem cells. Front. Cell. Dev. Biol. 11, 1236968. doi:10.3389/fcell.2023.1236968
Feng, G., Sun, H., and Piao, M. (2023). FBXL6 is dysregulated in keloids and promotes keloid fibroblast growth by inducing c-Myc expression. Int. Wound J. 20, 131–139. doi:10.1111/iwj.13847
Ferrer, I., and Blanco, R. (2000). N-myc and c-myc expression in Alzheimer disease, Huntington disease and Parkinson disease. Brain Res. Mol. Brain Res. 77, 270–276. doi:10.1016/s0169-328x(00)00062-0
Ferrer, I., Blanco, R., Carmona, M., and Puig, B. (2001). Phosphorylated c-MYC expression in Alzheimer disease, Pick’s disease, progressive supranuclear palsy and corticobasal degeneration. Neuropathol. Appl. Neurobiol. 27, 343–351. doi:10.1046/j.1365-2990.2001.00348.x
Florea, V., Bhagavatula, N., Simovic, G., Macedo, F. Y., Fock, R. A., and Rodrigues, C. O. (2013). c-Myc is essential to prevent endothelial pro-inflammatory senescent phenotype. PLoS One 8, e73146. doi:10.1371/journal.pone.0073146
Fu, X., Wu, X., Djekidel, M. N., and Zhang, Y. (2019). Myc and Dnmt1 impede the pluripotent to totipotent state transition in embryonic stem cells. Nat. Cell. Biol. 21, 835–844. doi:10.1038/s41556-019-0343-0
Gabay, M., Li, Y., and Felsher, D. W. (2014). MYC activation is a hallmark of cancer initiation and maintenance. Cold Spring Harb. Perspect. Med. 4, a014241. doi:10.1101/cshperspect.a014241
Gallant, P. (2006). “Myc/max/mad in invertebrates: the evolution of the Max network,” in The myc/max/mad transcription factor network, current topics in microbiology and immunology. Editor R. N. Eisenman (Berlin/Heidelberg: Springer-Verlag), 235–253. doi:10.1007/3-540-32952-8_9
Garralda, E., Beaulieu, M.-E., Moreno, V., Casacuberta-Serra, S., Martínez-Martín, S., Foradada, L., et al. (2024). MYC targeting by OMO-103 in solid tumors: a phase 1 trial. Nat. Med. doi:10.1038/s41591-024-02805-1
Gerling, I. C., Singh, S., Lenchik, N. I., Marshall, D. R., and Wu, J. (2006). New data analysis and mining approaches identify unique proteome and transcriptome markers of susceptibility to autoimmune diabetes. Mol. Cell. Proteomics 5, 293–305. doi:10.1074/mcp.M500197-MCP200
Graves, J. A., Wang, Y., Sims-Lucas, S., Cherok, E., Rothermund, K., Branca, M. F., et al. (2012). Mitochondrial structure, function and dynamics are temporally controlled by c-Myc. PLoS One 7, e37699. doi:10.1371/journal.pone.0037699
Grewal, S. S., Li, L., Orian, A., Eisenman, R. N., and Edgar, B. A. (2005). Myc-dependent regulation of ribosomal RNA synthesis during Drosophila development. Nat. Cell. Biol. 7, 295–302. doi:10.1038/ncb1223
Guitart, A. V., Hammoud, M., Dello Sbarba, P., Ivanovic, Z., and Praloran, V. (2010). Slow-cycling/quiescence balance of hematopoietic stem cells is related to physiological gradient of oxygen. Exp. Hematol. 38, 847–851. doi:10.1016/j.exphem.2010.06.002
Guo, Q. M., Malek, R. L., Kim, S., Chiao, C., He, M., Ruffy, M., et al. (2000). Identification of c-myc responsive genes using rat cDNA microarray. Cancer Res. 60, 5922–5928.
Hanahan, D. (2022). Hallmarks of cancer: new dimensions. Cancer Discov. 12, 31–46. doi:10.1158/2159-8290.CD-21-1059
Hann, S. R., Abrams, H. D., Rohrschneider, L. R., and Eisenman, R. N. (1983). Proteins encoded by v-myc and c-myc oncogenes: identification and localization in acute leukemia virus transformants and bursal lymphoma cell lines. Cell. 34, 789–798. doi:10.1016/0092-8674(83)90535-4
Harafuji, N., Yang, C., Wu, M., Thiruvengadam, G., Gordish-Dressman, H., Thompson, R. G., et al. (2023). Differential regulation of MYC expression by PKHD1/Pkhd1 in human and mouse kidneys: phenotypic implications for recessive polycystic kidney disease. Front. Cell. Dev. Biol. 11, 1270980. doi:10.3389/fcell.2023.1270980
Harding, M. A., Gattone, V. H., Grantham, J. J., and Calvet, J. P. (1992). Localization of overexpressed c-myc mRNA in polycystic kidneys of the cpk mouse. Kidney Int. 41, 317–325. doi:10.1038/ki.1992.44
Harshan, S., Dey, P., and Raghunathan, S. (2022). Altered transcriptional regulation of glycolysis in circulating CD8+ T cells of rheumatoid arthritis patients. Genes. (Basel) 13, 1216. doi:10.3390/genes13071216
Hartman, D. J., Binion, D. G., Regueiro, M. D., Miller, C., Herbst, C., and Pai, R. K. (2018). Distinct histopathologic and molecular alterations in inflammatory bowel disease-associated intestinal adenocarcinoma: c-MYC amplification is common and associated with mucinous/signet ring cell differentiation. Inflamm. Bowel Dis. 24, 1780–1790. doi:10.1093/ibd/izy057
Hofmann, J. W., Zhao, X., De Cecco, M., Peterson, A. L., Pagliaroli, L., Manivannan, J., et al. (2015). Reduced expression of MYC increases longevity and enhances healthspan. Cell. 160, 477–488. doi:10.1016/j.cell.2014.12.016
Hojjati, F., Roointan, A., Gholaminejad, A., Eshraghi, Y., and Gheisari, Y. (2023). Identification of key genes and biological regulatory mechanisms in diabetic nephropathy: meta-analysis of gene expression datasets. Nefrol. Engl. Ed. 43, 575–586. doi:10.1016/j.nefroe.2022.06.006
Horsfield, J. A., Print, C. G., and Mönnich, M. (2012). Diverse developmental disorders from the one ring: distinct molecular pathways underlie the cohesinopathies. Front. Genet. 3, 171. doi:10.3389/fgene.2012.00171
Hou, W., Lu, L., Li, X., Sun, M., Zhu, M., and Miao, C. (2022). c-Myc participates in high glucose-mediated endothelial inflammation via upregulation of IRAK1 expression in diabetic nephropathy. Cell. Signal 92, 110263. doi:10.1016/j.cellsig.2022.110263
Hu, S. S., Lai, M. M., and Vogt, P. K. (1979). Genome of avian myelocytomatosis virus MC29: analysis by heteroduplex mapping. Proc. Natl. Acad. Sci. U. S. A. 76, 1265–1268. doi:10.1073/pnas.76.3.1265
Hu, Z., Lou, L., and Luo, S. (2002). Experimental study of the expression of c-myc, c-fos and proto-oncogenes on hypertrophic and scars. Zhonghua Zheng Xing Wai Ke Za Zhi 18, 165–167.
Hulf, T., Bellosta, P., Furrer, M., Steiger, D., Svensson, D., Barbour, A., et al. (2005). Whole-genome analysis reveals a strong positional bias of conserved dMyc-dependent E-boxes. Mol. Cell. Biol. 25, 3401–3410. doi:10.1128/MCB.25.9.3401-3410.2005
International Multiple Sclerosis Genetics Consortium Sawcer, S., Hellenthal, G., Pirinen, M., Spencer, C. C. A., Patsopoulos, N. A., Moutsianas, L., et al. (2011). Genetic risk and a primary role for cell-mediated immune mechanisms in multiple sclerosis. Nature 476, 214–219. doi:10.1038/nature10251
Jha, R. K., Kouzine, F., and Levens, D. (2023). MYC function and regulation in physiological perspective. Front. Cell. Dev. Biol. 11, 1268275. doi:10.3389/fcell.2023.1268275
Jia, X.-X., Zhu, T.-T., Huang, Y., Zeng, X.-X., Zhang, H., and Zhang, W.-X. (2019). Wnt/β-catenin signaling pathway regulates asthma airway remodeling by influencing the expression of c-Myc and cyclin D1 via the p38 MAPK-dependent pathway. Exp. Ther. Med. 18, 3431–3438. doi:10.3892/etm.2019.7991
Jiang, B.-C., Ding, T.-Y., Guo, C.-Y., Bai, X.-H., Cao, D.-L., Wu, X.-B., et al. (2022). NFAT1 orchestrates spinal microglial transcription and promotes microglial proliferation via c-MYC contributing to nerve injury-induced neuropathic pain. Adv. Sci. (Weinh) 9, e2201300. doi:10.1002/advs.202201300
Jin, Q., Liu, Y., Zhang, Z., Wen, X., Chen, Z., Tian, H., et al. (2023). MYC promotes fibroblast osteogenesis by regulating ALP and BMP2 to participate in ectopic ossification of ankylosing spondylitis. Arthritis Res. Ther. 25, 28. doi:10.1186/s13075-023-03011-z
Johnston, L. A., Prober, D. A., Edgar, B. A., Eisenman, R. N., and Gallant, P. (1999). Drosophila myc regulates cellular growth during development. Cell. 98, 779–790. doi:10.1016/S0092-8674(00)81512-3
Jonas, J. C., Laybutt, D. R., Steil, G. M., Trivedi, N., Pertusa, J. G., Van de Casteele, M., et al. (2001). High glucose stimulates early response gene c-Myc expression in rat pancreatic beta cells. J. Biol. Chem. 276, 35375–35381. doi:10.1074/jbc.M105020200
Jonas, J. C., Sharma, A., Hasenkamp, W., Ilkova, H., Patanè, G., Laybutt, R., et al. (1999). Chronic hyperglycemia triggers loss of pancreatic beta cell differentiation in an animal model of diabetes. J. Biol. Chem. 274, 14112–14121. doi:10.1074/jbc.274.20.14112
Jones, R. M., Branda, J., Johnston, K. A., Polymenis, M., Gadd, M., Rustgi, A., et al. (1996). An essential E box in the promoter of the gene encoding the mRNA cap-binding protein (eukaryotic initiation factor 4E) is a target for activation by c-myc. Mol. Cell. Biol. 16, 4754–4764. doi:10.1128/MCB.16.9.4754
Kaizer, E. C., Glaser, C. L., Chaussabel, D., Banchereau, J., Pascual, V., and White, P. C. (2007). Gene expression in peripheral blood mononuclear cells from children with diabetes. J. Clin. Endocrinol. Metab. 92, 3705–3711. doi:10.1210/jc.2007-0979
Kaneto, H., Sharma, A., Suzuma, K., Laybutt, D. R., Xu, G., Bonner-Weir, S., et al. (2002a). Induction of c-Myc expression suppresses insulin gene transcription by inhibiting NeuroD/BETA2-mediated transcriptional activation. J. Biol. Chem. 277, 12998–13006. doi:10.1074/jbc.M111148200
Kaneto, H., Suzuma, K., Sharma, A., Bonner-Weir, S., King, G. L., and Weir, G. C. (2002b). Involvement of protein kinase C beta 2 in c-myc induction by high glucose in pancreatic beta-cells. J. Biol. Chem. 277, 3680–3685. doi:10.1074/jbc.M109647200
Karadkhelkar, N. M., Lin, M., Eubanks, L. M., and Janda, K. D. (2023). Demystifying the druggability of the MYC family of oncogenes. J. Am. Chem. Soc. 145, 3259–3269. doi:10.1021/jacs.2c12732
Kayama, A., and Eto, K. (2024). Mass production of iPSC-derived platelets toward the clinical application. Regen. Ther. 25, 213–219. doi:10.1016/j.reth.2023.12.009
Ke, X., Hu, H., Peng, Q., Ying, H., and Chu, X. (2023). USP33 promotes nonalcoholic fatty acid disease-associated fibrosis in gerbils via the c-myc signaling. Biochem. Biophys. Res. Commun. 669, 68–76. doi:10.1016/j.bbrc.2023.05.100
Kim, D., Konyn, P., Sandhu, K. K., Dennis, B. B., Cheung, A. C., and Ahmed, A. (2021). Metabolic dysfunction-associated fatty liver disease is associated with increased all-cause mortality in the United States. J. Hepatol. 75, 1284–1291. doi:10.1016/j.jhep.2021.07.035
Kim, J., Zeller, K. I., Wang, Y., Jegga, A. G., Aronow, B. J., O’Donnell, K. A., et al. (2004). Evaluation of myc E-box phylogenetic footprints in glycolytic genes by chromatin immunoprecipitation assays. Mol. Cell. Biol. 24, 5923–5936. doi:10.1128/MCB.24.13.5923-5936.2004
Kipshidze, N., Iversen, P., Keane, E., Stein, D., Chawla, P., Skrinska, V., et al. (2002). Complete vascular healing and sustained suppression of neointimal thickening after local delivery of advanced c-myc antisense at six months follow-up in a rabbit balloon injury model. Cardiovasc. Radiat. Med. 3, 26–30. doi:10.1016/S1522-1865(02)00149-X
Kipshidze, N., Iversen, P., Overlie, P., Dunlap, T., Titus, B., Lee, D., et al. (2007). First human experience with local delivery of novel antisense AVI-4126 with Infiltrator catheter in de novo native and restenotic coronary arteries: 6-month clinical and angiographic follow-up from AVAIL study. Cardiovasc. Revascularization Med. 8, 230–235. doi:10.1016/j.carrev.2007.04.002
Kipshidze, N. N., Kim, H.-S., Iversen, P., Yazdi, H. A., Bhargava, B., New, G., et al. (2002). Intramural coronary delivery of advanced antisense oligonucleotides reduces neointimal formation in the porcine stent restenosis model. J. Am. Coll. Cardiol. 39, 1686–1691. doi:10.1016/S0735-1097(02)01830-2
Kipshidze, N. N., Porter, T. R., Dangas, G., Yazdi, H., Tio, F., Xie, F., et al. (2003). Systemic targeted delivery of antisense with perflourobutane gas microbubble carrier reduced neointimal formation in the porcine coronary restenosis model. Cardiovasc. Radiat. Med. 4, 152–159. doi:10.1016/S1522-1865(03)00184-7
Kokai, E., Voss, F., Fleischer, F., Kempe, S., Marinkovic, D., Wolburg, H., et al. (2009). Myc regulates embryonic vascular permeability and remodeling. Circulation Res. 104, 1151–1159. doi:10.1161/CIRCRESAHA.108.191460
Kolodziejczyk, A. A., Federici, S., Zmora, N., Mohapatra, G., Dori-Bachash, M., Hornstein, S., et al. (2020). Acute liver failure is regulated by MYC- and microbiome-dependent programs. Nat. Med. 26, 1899–1911. doi:10.1038/s41591-020-1102-2
Kraggerud, S. M., Sandvik, J. A., and Pettersen, E. O. (1995). Regulation of protein synthesis in human cells exposed to extreme hypoxia. Anticancer Res. 15, 683–686.
Kühtreiber, W. M., Takahashi, H., Keefe, R. C., Song, Y., Tran, L., Luck, T. G., et al. (2020). BCG vaccinations upregulate myc, a central switch for improved glucose metabolism in diabetes. iScience 23, 101085. doi:10.1016/j.isci.2020.101085
Kunkl, M., Sambucci, M., Ruggieri, S., Amormino, C., Tortorella, C., Gasperini, C., et al. (2019). CD28 autonomous signaling up-regulates C-myc expression and promotes glycolysis enabling inflammatory T cell responses in multiple sclerosis. Cells 8, 575. doi:10.3390/cells8060575
Kutryk, M. J. B., Foley, D. P., Van Den Brand, M., Hamburger, J. N., Van Der Giessen, W. J., deFeyter, P. J., et al. (2002). Local intracoronary administration of antisense oligonucleotide against c-myc for the prevention of in-stent restenosis: results of the randomized investigation by the Thoraxcenter of antisense DNA using local delivery and IVUS after coronary stenting (ITALICS) trial. J. Am. Coll. Cardiol. 39, 281–287. doi:10.1016/S0735-1097(01)01741-7
Laybutt, D. R., Glandt, M., Xu, G., Ahn, Y. B., Trivedi, N., Bonner-Weir, S., et al. (2003). Critical reduction in beta-cell mass results in two distinct outcomes over time. Adaptation with impaired glucose tolerance or decompensated diabetes. J. Biol. Chem. 278, 2997–3005. doi:10.1074/jbc.M210581200
Laybutt, D. R., Weir, G. C., Kaneto, H., Lebet, J., Palmiter, R. D., Sharma, A., et al. (2002). Overexpression of c-Myc in beta-cells of transgenic mice causes proliferation and apoptosis, downregulation of insulin gene expression, and diabetes. Diabetes 51, 1793–1804. doi:10.2337/diabetes.51.6.1793
Lee, H., Casadesus, G., Nunomura, A., Zhu, X., Castellani, R. J., Richardson, S. L., et al. (2009). The neuronal expression of MYC causes a neurodegenerative phenotype in a novel transgenic mouse. Am. J. Pathol. 174, 891–897. doi:10.2353/ajpath.2009.080583
Lee, S.-H., Demeterco, C., Geron, I., Abrahamsson, A., Levine, F., and Itkin-Ansari, P. (2008). Islet specific Wnt activation in human type II diabetes. Exp. Diabetes Res. 2008, 728763. doi:10.1155/2008/728763
Lee, Y.-Z., Guo, H.-C., Zhao, G.-H., Yang, C.-W., Chang, H.-Y., Yang, R.-B., et al. (2020). Tylophorine-based compounds are therapeutic in rheumatoid arthritis by targeting the caprin-1 ribonucleoprotein complex and inhibiting expression of associated c-Myc and HIF-1α. Pharmacol. Res. 152, 104581. doi:10.1016/j.phrs.2019.104581
Li, C., and Qian, Y.-H. (2022). Inflammation-dependent activation of NCOA2 associates with p300 and c-MYC/Max heterodimer to transactivate RUNX2-AS1 and mediate RUNX2 downstream bone differentiation genes in the pathology of septic nonunion. Cytokine 158, 155992. doi:10.1016/j.cyto.2022.155992
Li, F., Wang, Y., Zeller, K. I., Potter, J. J., Wonsey, D. R., O’Donnell, K. A., et al. (2005). Myc stimulates nuclearly encoded mitochondrial genes and mitochondrial biogenesis. Mol. Cell. Biol. 25, 6225–6234. doi:10.1128/MCB.25.14.6225-6234.2005
Li, P., Liu, Q., Luo, H., Liang, K., Han, Y., Roland, K. L., et al. (2018). Bi-valent polysaccharides of Vi capsular and O9 O-antigen in attenuated Salmonella Typhimurium induce strong immune responses against these two antigens. npj Vaccines 3, 1. doi:10.1038/s41541-017-0041-5
Li, X., Chen, S., Hu, Z., Chen, D., Wang, J., Li, Z., et al. (2020). Aberrant upregulation of CaSR promotes pathological new bone formation in ankylosing spondylitis. EMBO Mol. Med. 12, e12109. doi:10.15252/emmm.202012109
Liang, Y., Hao, Y., Xiong, Y., Zhong, M., and Jain, D. (2023). MYC overexpression in inflammatory bowel disease-associated conventional dysplasia and association of subsequent low-grade dysplasia in follow-up biopsies. Pathol. Res. Pract. 248, 154642. doi:10.1016/j.prp.2023.154642
Lin, C.-H., Jackson, A. L., Guo, J., Linsley, P. S., and Eisenman, R. N. (2009). Myc-regulated microRNAs attenuate embryonic stem cell differentiation. EMBO J. 28, 3157–3170. doi:10.1038/emboj.2009.254
López-Otín, C., Blasco, M. A., Partridge, L., Serrano, M., and Kroemer, G. (2023). Hallmarks of aging: an expanding universe. Cell. 186, 243–278. doi:10.1016/j.cell.2022.11.001
Luo, Y., Yang, S., Wu, X., Takahashi, S., Sun, L., Cai, J., et al. (2021). Intestinal MYC modulates obesity-related metabolic dysfunction. Nat. Metab. 3, 923–939. doi:10.1038/s42255-021-00421-8
Lynch, M., Fitzgerald, C., Johnston, K. A., Wang, S., and Schmidt, E. V. (2004). Activated eIF4E-binding protein slows G1 progression and blocks transformation by c-myc without inhibiting cell growth. J. Biol. Chem. 279, 3327–3339. doi:10.1074/jbc.M310872200
Ma, X. M., and Blenis, J. (2009). Molecular mechanisms of mTOR-mediated translational control. Nat. Rev. Mol. Cell. Biol. 10, 307–318. doi:10.1038/nrm2672
Ma, Y.-X., Wang, X.-L., Chen, J.-Q., Li, B., Hur, E.-M., and Saijilafu, null (2017). Differential roles of glycogen synthase kinase 3 subtypes alpha and beta in cortical development. Front. Mol. Neurosci. 10, 391. doi:10.3389/fnmol.2017.00391
Maksymchuk, O., Shysh, A., and Kotliarova, A. (2023). Quercetin inhibits the expression of MYC and CYP2E1 and reduces oxidative stress in the myocardium of spontaneously hypertensive rats. Acta Biochim. Pol. 70, 199–204. doi:10.18388/abp.2020_6517
Martínez-Martín, S., Beaulieu, M.-E., and Soucek, L. (2023). Targeting MYC-driven lymphoma: lessons learned and future directions. Cancer Drug Resist 6, 205–222. doi:10.20517/cdr.2022.127
Massó-Vallés, D., Beaulieu, M.-E., and Soucek, L. (2020). MYC, MYCL, and MYCN as therapeutic targets in lung cancer. Expert Opin. Ther. Targets 24, 101–114. doi:10.1080/14728222.2020.1723548
Massó-Vallés, D., and Soucek, L. (2020). Blocking myc to treat cancer: reflecting on two decades of Omomyc. Cells 9, 883. doi:10.3390/cells9040883
Mateyak, M. K., Obaya, A. J., Adachi, S., and Sedivy, J. M. (1997). Phenotypes of c-Myc-deficient rat fibroblasts isolated by targeted homologous recombination. Cell. Growth Differ. 8, 1039–1048.
McFerrin, L. G., and Atchley, W. R. (2011). Evolution of the Max and mlx networks in animals. Genome Biol. Evol. 3, 915–937. doi:10.1093/gbe/evr082
Mori, T., Ato, S., Knudsen, J. R., Henriquez-Olguin, C., Li, Z., Wakabayashi, K., et al. (2021). c-Myc overexpression increases ribosome biogenesis and protein synthesis independent of mTORC1 activation in mouse skeletal muscle. Am. J. Physiol. Endocrinol. Metab. 321, E551–E559. doi:10.1152/ajpendo.00164.2021
Murphy, D. J., Junttila, M. R., Pouyet, L., Karnezis, A., Shchors, K., Bui, D. A., et al. (2008). Distinct thresholds govern myc’s biological output in vivo. Cancer Cell. 14, 447–457. doi:10.1016/j.ccr.2008.10.018
Murtaugh, L. C., Law, A. C., Dor, Y., and Melton, D. A. (2005). Beta-catenin is essential for pancreatic acinar but not islet development. Development 132, 4663–4674. doi:10.1242/dev.02063
Nakhai, H., Siveke, J. T., Mendoza-Torres, L., and Schmid, R. M. (2008). Conditional inactivation of Myc impairs development of the exocrine pancreas. Development 135, 3191–3196. doi:10.1242/dev.017137
Nevzorova, Y. A., and Cubero, F. J. (2023). Obesity under the moonlight of c-MYC. Front. Cell. Dev. Biol. 11, 1293218. doi:10.3389/fcell.2023.1293218
Nevzorova, Y. A., Cubero, F. J., Hu, W., Hao, F., Haas, U., Ramadori, P., et al. (2016). Enhanced expression of c-myc in hepatocytes promotes initiation and progression of alcoholic liver disease. J. Hepatol. 64, 628–640. doi:10.1016/j.jhep.2015.11.005
Nevzorova, Y. A., Hu, W., Cubero, F. J., Haas, U., Freimuth, J., Tacke, F., et al. (2013). Overexpression of c-myc in hepatocytes promotes activation of hepatic stellate cells and facilitates the onset of liver fibrosis. Biochim. Biophys. Acta 1832, 1765–1775. doi:10.1016/j.bbadis.2013.06.001
Nothnick, W. B., Arachchige, S. P., Minchella, P., Stephens, E. B., and Graham, A. (2023). Targeting c-MYC: a potential non-hormonal therapeutic approach for endometriosis treatment. Front. Cell. Dev. Biol. 11, 1225055. doi:10.3389/fcell.2023.1225055
Orian, A., Van Steensel, B., Delrow, J., Bussemaker, H. J., Li, L., Sawado, T., et al. (2003). Genomic binding by the Drosophila Myc, Max, Mad/Mnt transcription factor network. Genes. Dev. 17, 1101–1114. doi:10.1101/gad.1066903
Pang, C. J., Lemsaddek, W., Alhashem, Y. N., Bondzi, C., Redmond, L. C., Ah-Son, N., et al. (2012). Kruppel-like factor 1 (KLF1), KLF2, and Myc control a regulatory network essential for embryonic erythropoiesis. Mol. Cell. Biol. 32, 2628–2644. doi:10.1128/MCB.00104-12
Parrot, C., Kurbegovic, A., Yao, G., Couillard, M., Côté, O., and Trudel, M. (2019). c-Myc is a regulator of the PKD1 gene and PC1-induced pathogenesis. Hum. Mol. Genet. 28, 751–763. doi:10.1093/hmg/ddy379
Pelengaris, S., and Khan, M. (2003). The many faces of c-MYC. Arch. Biochem. Biophys. 416, 129–136. doi:10.1016/s0003-9861(03)00294-7
Pelengaris, S., Khan, M., and Evan, G. I. (2002). Suppression of Myc-induced apoptosis in beta cells exposes multiple oncogenic properties of Myc and triggers carcinogenic progression. Cell. 109, 321–334. doi:10.1016/s0092-8674(02)00738-9
Perkins, A., Xu, X., Higgs, D. R., Patrinos, G. P., Arnaud, L., Bieker, J. J., et al. (2016). Krüppeling erythropoiesis: an unexpected broad spectrum of human red blood cell disorders due to KLF1 variants. Blood 127, 1856–1862. doi:10.1182/blood-2016-01-694331
Petrashen, A. P., Verdesca, A. D., Kreiling, J. A., and Sedivy, J. M. (2023). Regulation of the somatotropic axis by MYC-mediated miRNA repression. Front. Cell. Dev. Biol. 11, 1269860. doi:10.3389/fcell.2023.1269860
Philipp, S. (2012). The appraisal-trial: evaluating RESTEN-MPTM in patients with bare metal stent de novo native coronary artery lesions. J. Clin. Exp. Cardiol. 03. doi:10.4172/2155-9880.1000218
Piao, M., and Feng, G. (2023). The deubiquitinating enzyme USP37 promotes keloid fibroblasts proliferation and collagen production by regulating the c-Myc expression. Int. Wound J. 20, 1517–1524. doi:10.1111/iwj.14006
Prendergast, G. C., and Ziff, E. B. (1991). Methylation-sensitive sequence-specific DNA binding by the c-Myc basic region. Science 251, 186–189. doi:10.1126/science.1987636
Prochownik, E. V., and Wang, H. (2023). Lessons in aging from Myc knockout mouse models. Front. Cell. Dev. Biol. 11, 1244321. doi:10.3389/fcell.2023.1244321
Purhonen, J., Klefström, J., and Kallijärvi, J. (2023). MYC—an emerging player in mitochondrial diseases. Front. Cell. Dev. Biol. 11, 1257651. doi:10.3389/fcell.2023.1257651
Qi, Y., Qadir, M. M. F., Hastreiter, A. A., Fock, R. A., Machi, J. F., Morales, A. A., et al. (2022). Endothelial c-Myc knockout enhances diet-induced liver inflammation and fibrosis. FASEB J. 36, e22077. doi:10.1096/fj.202101086R
Quaife-Ryan, G. A., Sim, C. B., Ziemann, M., Kaspi, A., Rafehi, H., Ramialison, M., et al. (2017). Multicellular transcriptional analysis of mammalian heart regeneration. Circulation 136, 1123–1139. doi:10.1161/CIRCULATIONAHA.117.028252
Ren, F., Shi, Q., Chen, Y., Jiang, A., Ip, Y. T., Jiang, H., et al. (2013). Drosophila Myc integrates multiple signaling pathways to regulate intestinal stem cell proliferation during midgut regeneration. Cell. Res. 23, 1133–1146. doi:10.1038/cr.2013.101
Ricker, J. L., Mata, J. E., Iversen, P. L., and Gattone, V. H. (2002). c-myc antisense oligonucleotide treatment ameliorates murine ARPKD. Kidney Int. 61, S125–S131. doi:10.1046/j.1523-1755.2002.0610s1125.x
Rodríguez, A., Zhang, K., Färkkilä, A., Filiatrault, J., Yang, C., Velázquez, M., et al. (2021). MYC promotes bone marrow stem cell dysfunction in fanconi anemia. Cell. Stem Cell. 28, 33–47.e8. doi:10.1016/j.stem.2020.09.004
Rosenwald, I. B., Rhoads, D. B., Callanan, L. D., Isselbacher, K. J., and Schmidt, E. V. (1993). Increased expression of eukaryotic translation initiation factors eIF-4E and eIF-2 alpha in response to growth induction by c-myc. Proc. Natl. Acad. Sci. U. S. A. 90, 6175–6178. doi:10.1073/pnas.90.13.6175
Ross, J., Miron, C. E., Plescia, J., Laplante, P., McBride, K., Moitessier, N., et al. (2021). Targeting MYC: from understanding its biology to drug discovery. Eur. J. Med. Chem. 213, 113137. doi:10.1016/j.ejmech.2020.113137
Rylski, M., Welch, J. J., Chen, Y.-Y., Letting, D. L., Diehl, J. A., Chodosh, L. A., et al. (2003). GATA-1-mediated proliferation arrest during erythroid maturation. Mol. Cell. Biol. 23, 5031–5042. doi:10.1128/MCB.23.14.5031-5042.2003
Safari-Alighiarloo, N., Taghizadeh, M., Mohammad Tabatabaei, S., Namaki, S., and Rezaei-Tavirani, M. (2020). Identification of common key genes and pathways between type 1 diabetes and multiple sclerosis using transcriptome and interactome analysis. Endocrine 68, 81–92. doi:10.1007/s12020-019-02181-8
Salameh, L., Bhamidimarri, P. M., Saheb Sharif-Askari, N., Dairi, Y., Hammoudeh, S. M., Mahdami, A., et al. (2022). In silico bioinformatics followed by molecular validation using archival FFPE tissue biopsies identifies a panel of transcripts associated with severe asthma and lung cancer. Cancers (Basel) 14, 1663. doi:10.3390/cancers14071663
Samuels, T. J., Järvelin, A. I., Ish-Horowicz, D., and Davis, I. (2020). Imp/IGF2BP levels modulate individual neural stem cell growth and division through myc mRNA stability. Elife 9, e51529. doi:10.7554/eLife.51529
Schaale, K., Brandenburg, J., Kispert, A., Leitges, M., Ehlers, S., and Reiling, N. (2013). Wnt6 is expressed in granulomatous lesions of Mycobacterium tuberculosis-infected mice and is involved in macrophage differentiation and proliferation. J. Immunol. 191, 5182–5195. doi:10.4049/jimmunol.1201819
Schmidt, E. V. (2004). The role of c-myc in regulation of translation initiation. Oncogene 23, 3217–3221. doi:10.1038/sj.onc.1207548
Schramm, L., and Hernandez, N. (2002). Recruitment of RNA polymerase III to its target promoters. Genes. Dev. 16, 2593–2620. doi:10.1101/gad.1018902
Scognamiglio, R., Cabezas-Wallscheid, N., Thier, M. C., Altamura, S., Reyes, A., Prendergast, Á. M., et al. (2016). Myc depletion induces a pluripotent dormant state mimicking diapause. Cell. 164, 668–680. doi:10.1016/j.cell.2015.12.033
Sheiness, D., Fanshier, L., and Bishop, J. M. (1978). Identification of nucleotide sequences which may encode the oncogenic capacity of avian retrovirus MC29. J. Virol. 28, 600–610. doi:10.1128/JVI.28.2.600-610.1978
Shen, J., Zhao, J., Ye, Q.-Y., and Gu, X.-D. (2019). Interference of miR-943-3p with secreted frizzled-related proteins4 (SFRP4) in an asthma mouse model. Cell. Tissue Res. 378, 67–80. doi:10.1007/s00441-019-03026-6
Shi, Y., Liu, Z., Zhang, Q., Vallee, I., Mo, Z., Kishi, S., et al. (2020). Phosphorylation of seryl-tRNA synthetase by ATM/ATR is essential for hypoxia-induced angiogenesis. PLoS Biol. 18, e3000991. doi:10.1371/journal.pbio.3000991
Shi, Y., Xu, X., Zhang, Q., Fu, G., Mo, Z., Wang, G. S., et al. (2014). tRNA synthetase counteracts c-Myc to develop functional vasculature. eLife 3, e02349. doi:10.7554/eLife.02349
Sleiman, S. F., Langley, B. C., Basso, M., Berlin, J., Xia, L., Payappilly, J. B., et al. (2011). Mithramycin is a gene-selective Sp1 inhibitor that identifies a biological intersection between cancer and neurodegeneration. J. Neurosci. 31, 6858–6870. doi:10.1523/JNEUROSCI.0710-11.2011
Speir, E., and Epstein, S. E. (1992). Inhibition of smooth muscle cell proliferation by an antisense oligodeoxynucleotide targeting the messenger RNA encoding proliferating cell nuclear antigen. Circulation 86, 538–547. doi:10.1161/01.CIR.86.2.538
Sporbeck, K., Haas, M. L., Pastor-Maldonado, C. J., Schüssele, D. S., Hunter, C., Takacs, Z., et al. (2023). The ABL-MYC axis controls WIPI1-enhanced autophagy in lifespan extension. Commun. Biol. 6, 872. doi:10.1038/s42003-023-05236-9
Staller, P., Peukert, K., Kiermaier, A., Seoane, J., Lukas, J., Karsunky, H., et al. (2001). Repression of p15INK4b expression by Myc through association with Miz-1. Nat. Cell. Biol. 3, 392–399. doi:10.1038/35070076
Stanley, W. C., Recchia, F. A., and Lopaschuk, G. D. (2005). Myocardial substrate metabolism in the normal and failing heart. Physiol. Rev. 85, 1093–1129. doi:10.1152/physrev.00006.2004
Stappenbeck, T. S., Mills, J. C., and Gordon, J. I. (2003). Molecular features of adult mouse small intestinal epithelial progenitors. Proc. Natl. Acad. Sci. U. S. A. 100, 1004–1009. doi:10.1073/pnas.242735899
Stine, Z. E., Walton, Z. E., Altman, B. J., Hsieh, A. L., and Dang, C. V. (2015). MYC, metabolism, and cancer. Cancer Discov. 5, 1024–1039. doi:10.1158/2159-8290.CD-15-0507
Tan, P., Guan, H., Xie, L., Mi, B., Fang, Z., Li, J., et al. (2015). FOXO1 inhibits osteoclastogenesis partially by antagnozing MYC. Sci. Rep. 5, 16835. doi:10.1038/srep16835
Thai, M., Thaker, S. K., Feng, J., Du, Y., Hu, H., Ting Wu, T., et al. (2015). MYC-induced reprogramming of glutamine catabolism supports optimal virus replication. Nat. Commun. 6, 8873. doi:10.1038/ncomms9873
Tóth, M. L., Sigmond, T., Borsos, É., Barna, J., Erdélyi, P., Takács-Vellai, K., et al. (2008). Longevity pathways converge on autophagy genes to regulate life span in Caenorhabditis elegans. Autophagy 4, 330–338. doi:10.4161/auto.5618
Troy, N. M., Hollams, E. M., Holt, P. G., and Bosco, A. (2016). Differential gene network analysis for the identification of asthma-associated therapeutic targets in allergen-specific T-helper memory responses. BMC Med. Genomics 9, 9. doi:10.1186/s12920-016-0171-z
Trudel, M., D’Agati, V., and Costantini, F. (1991). C-myc as an inducer of polycystic kidney disease in transgenic mice. Kidney Int. 39, 665–671. doi:10.1038/ki.1991.80
Trumpp, A., Refaeli, Y., Oskarsson, T., Gasser, S., Murphy, M., Martin, G. R., et al. (2001). c-Myc regulates mammalian body size by controlling cell number but not cell size. Nature 414, 768–773. doi:10.1038/414768a
Vaira, V., Gaudioso, G., Laginestra, M. A., Terrasi, A., Agostinelli, C., Bosari, S., et al. (2020). Deregulation of miRNAs-cMYC circuits is a key event in refractory celiac disease type-2 lymphomagenesis. Clin. Sci. (Lond) 134, 1151–1166. doi:10.1042/CS20200032
Valera, A., Pujol, A., Gregori, X., Riu, E., Visa, J., and Bosch, F. (1995). Evidence from transgenic mice that myc regulates hepatic glycolysis. FASEB J. 9, 1067–1078. doi:10.1096/fasebj.9.11.7649406
van Riggelen, J., Yetil, A., and Felsher, D. W. (2010). MYC as a regulator of ribosome biogenesis and protein synthesis. Nat. Rev. Cancer 10, 301–309. doi:10.1038/nrc2819
Vargas, J. E., Porto, B. N., Puga, R., Stein, R. T., and Pitrez, P. M. (2016). Identifying a biomarker network for corticosteroid resistance in asthma from bronchoalveolar lavage samples. Mol. Biol. Rep. 43, 697–710. doi:10.1007/s11033-016-4007-x
Vennstrom, B., Sheiness, D., Zabielski, J., and Bishop, J. M. (1982). Isolation and characterization of c-myc, a cellular homolog of the oncogene (v-myc) of avian myelocytomatosis virus strain 29. J. Virol. 42, 773–779. doi:10.1128/JVI.42.3.773-779.1982
Vollmuth, N., Schlicker, L., Guo, Y., Hovhannisyan, P., Janaki-Raman, S., Kurmasheva, N., et al. (2022). c-Myc plays a key role in IFN-γ-induced persistence of Chlamydia trachomatis. Elife 11, e76721. doi:10.7554/eLife.76721
Walsh, S., Pontén, A., Fleischmann, B. K., and Jovinge, S. (2010). Cardiomyocyte cell cycle control and growth estimation in vivo—an analysis based on cardiomyocyte nuclei. Cardiovasc. Res. 86, 365–373. doi:10.1093/cvr/cvq005
Wang, C.-Y., Chiou, G.-Y., Chien, Y., Wu, C.-C., Wu, T.-C., Lo, W.-T., et al. (2013). Induced pluripotent stem cells without c-Myc reduce airway responsiveness and allergic reaction in sensitized mice. Transplantation 96, 958–965. doi:10.1097/TP.0b013e3182a53ef7
Wang, H., Lu, J., Stevens, T., Roberts, A., Mandel, J., Avula, R., et al. (2023). Premature aging and reduced cancer incidence associated with near-complete body-wide Myc inactivation. Cell. Rep. 42, 112830. doi:10.1016/j.celrep.2023.112830
Wang, X.-L., Ma, Y.-X., Xu, R.-J., Ma, J.-J., Zhang, H.-C., Qi, S.-B., et al. (2020). c-Myc controls the fate of neural progenitor cells during cerebral cortex development. J. Cell. Physiol. 235, 4011–4021. doi:10.1002/jcp.29297
Wang, X.-Y., Wei, Y., Hu, B., Liao, Y., Wang, X., Wan, W.-H., et al. (2022). c-Myc-driven glycolysis polarizes functional regulatory B cells that trigger pathogenic inflammatory responses. Signal Transduct. Target Ther. 7, 105. doi:10.1038/s41392-022-00948-6
Wang, Y., Jin, L., Song, Y., Zhang, M., Shan, D., Liu, Y., et al. (2017). β-arrestin 2 mediates cardiac ischemia-reperfusion injury via inhibiting GPCR-independent cell survival signalling. Cardiovasc. Res. 113, 1615–1626. doi:10.1093/cvr/cvx147
Wang, Z.-H., Liu, Y., Chaitankar, V., Pirooznia, M., and Xu, H. (2019). Electron transport chain biogenesis activated by a JNK-insulin-Myc relay primes mitochondrial inheritance in Drosophila. Elife 8, e49309. doi:10.7554/eLife.49309
Webb, L. M., Amici, S. A., Jablonski, K. A., Savardekar, H., Panfil, A. R., Li, L., et al. (2017). PRMT5-Selective inhibitors suppress inflammatory T cell responses and experimental autoimmune encephalomyelitis. J. Immunol. 198, 1439–1451. doi:10.4049/jimmunol.1601702
Webb, L. M., Narvaez Miranda, J., Amici, S. A., Sengupta, S., Nagy, G., and Guerau-de-Arellano, M. (2019). NF-κB/mTOR/MYC Axis drives PRMT5 protein induction after T cell activation via transcriptional and non-transcriptional mechanisms. Front. Immunol. 10, 524. doi:10.3389/fimmu.2019.00524
Weber, L. I., and Hartl, M. (2023). Strategies to target the cancer driver MYC in tumor cells. Front. Oncol. 13, 1142111. doi:10.3389/fonc.2023.1142111
Wen, C., Lan, M., Tan, X., Wang, X., Zheng, Z., Lv, M., et al. (2022). GSK3β exacerbates myocardial ischemia/reperfusion injury by inhibiting myc. Oxidative Med. Cell. Longev. 2022, 1–23. doi:10.1155/2022/2588891
Whitfield, J. R., Beaulieu, M.-E., and Soucek, L. (2017). Strategies to inhibit myc and their clinical applicability. Front. Cell. Dev. Biol. 5, 10. doi:10.3389/fcell.2017.00010
Whitfield, J. R., and Soucek, L. (2012). Tumor microenvironment: becoming sick of Myc. Cell. Mol. Life Sci. 69, 931–934. doi:10.1007/s00018-011-0860-x
Whitfield, J. R., and Soucek, L. (2021). The long journey to bring a Myc inhibitor to the clinic. J. Cell. Biol. 220, e202103090. doi:10.1083/jcb.202103090
Wilson, A., Murphy, M. J., Oskarsson, T., Kaloulis, K., Bettess, M. D., Oser, G. M., et al. (2004). c-Myc controls the balance between hematopoietic stem cell self-renewal and differentiation. Genes. Dev. 18, 2747–2763. doi:10.1101/gad.313104
Wu, M., Yang, C., Tao, B., Bu, S., and Guay-Woodford, L. M. (2013). The ciliary protein cystin forms a regulatory complex with necdin to modulate Myc expression. PLoS One 8, e83062. doi:10.1371/journal.pone.0083062
Xiao, S., Zhou, T., Pan, J., Ma, X., Shi, G., Jiang, B., et al. (2023). Identifying autophagy-related genes as potential targets for immunotherapy in tuberculosis. Int. Immunopharmacol. 118, 109956. doi:10.1016/j.intimp.2023.109956
Xu, D., Xie, R., Xu, Z., Zhao, Z., Ding, M., Chen, W., et al. (2020). mTOR-Myc axis drives acinar-to-dendritic cell transition and the CD4+ T cell immune response in acute pancreatitis. Cell. Death Dis. 11, 416. doi:10.1038/s41419-020-2517-x
Xu, L., Wu, F., Yang, L., Wang, F., Zhang, T., Deng, X., et al. (2020). miR-144/451 inhibits c-Myc to promote erythroid differentiation. FASEB J. 34, 13194–13210. doi:10.1096/fj.202000941R
Yan, X.-T., Xu, Y., Cheng, X.-L., He, X.-H., Wang, Y., Zheng, W.-Z., et al. (2019). SP1, MYC, CTNNB1, CREB1, JUN genes as potential therapy targets for neuropathic pain of brain. J. Cell. Physiol. 234, 6688–6695. doi:10.1002/jcp.27413
Yang, C., Harafuji, N., O’Connor, A. K., Kesterson, R. A., Watts, J. A., Majmundar, A. J., et al. (2021). Cystin genetic variants cause autosomal recessive polycystic kidney disease associated with altered Myc expression. Sci. Rep. 11, 18274. doi:10.1038/s41598-021-97046-4
Yavropoulou, M. P., and Yovos, J. G. (2008). Osteoclastogenesis--current knowledge and future perspectives. J. Musculoskelet. Neuronal Interact. 8, 204–216.
Ye, L., Pan, J., Liang, M., Pasha, M. A., Shen, X., D’Souza, S. S., et al. (2020). A critical role for c-Myc in group 2 innate lymphoid cell activation. Allergy 75, 841–852. doi:10.1111/all.14149
Yoder, B. K., Hou, X., and Guay-Woodford, L. M. (2002). The polycystic kidney disease proteins, polycystin-1, polycystin-2, polaris, and cystin, are co-localized in renal cilia. J. Am. Soc. Nephrol. 13, 2508–2516. doi:10.1097/01.asn.0000029587.47950.25
Yuan, J., Tirabassi, R. S., Bush, A. B., and Cole, M. D. (1998). The C. elegans MDL-1 and MXL-1 proteins can functionally substitute for vertebrate MAD and MAX. Oncogene 17, 1109–1118. doi:10.1038/sj.onc.1202036
Zhang, P., Metukuri, M. R., Bindom, S. M., Prochownik, E. V., O’Doherty, R. M., and Scott, D. K. (2010). c-Myc is required for the chrebp-dependent activation of glucose-responsive genes. Mol. Endocrinol. 24 (6), 1274–1286. doi:10.1210/me.2009-0437
Zhang, M.-Z., Dong, X.-H., Zhang, W.-C., Li, M., Si, L.-B., Liu, Y.-F., et al. (2023). A comparison of proliferation levels in normal skin, physiological scar and keloid tissue. J. Plast. Surg. Hand Surg. 57, 122–128. doi:10.1080/2000656X.2021.2017294
Zhang, Q., Sun, C., Liu, X., Zhu, C., Ma, C., and Feng, R. (2023). Mechanism of immune infiltration in synovial tissue of osteoarthritis: a gene expression-based study. J. Orthop. Surg. Res. 18, 58. doi:10.1186/s13018-023-03541-x
Zhou, X., Fan, L. X., Peters, D. J. M., Trudel, M., Bradner, J. E., and Li, X. (2015). Therapeutic targeting of BET bromodomain protein, Brd4, delays cyst growth in ADPKD. Hum. Mol. Genet. 24, 3982–3993. doi:10.1093/hmg/ddv136
Zhou, Z.-Q., Shung, C.-Y., Ota, S., Akiyama, H., Keene, D. R., and Hurlin, P. J. (2011). Sequential and coordinated actions of c-Myc and N-Myc control appendicular skeletal development. PLoS One 6, e18795. doi:10.1371/journal.pone.0018795
Keywords: MYC, targeting, therapy, non-oncological diseases, transcription factor
Citation: Zacarías-Fluck MF, Soucek L and Whitfield JR (2024) MYC: there is more to it than cancer. Front. Cell Dev. Biol. 12:1342872. doi: 10.3389/fcell.2024.1342872
Received: 22 November 2023; Accepted: 20 February 2024;
Published: 06 March 2024.
Edited by:
Alice Turdo, University of Palermo, ItalyReviewed by:
Paola Bellosta, University of Trento, ItalyBrian James Altman, University of Rochester Medical Center, United States
Copyright © 2024 Zacarías-Fluck, Soucek and Whitfield. This is an open-access article distributed under the terms of the Creative Commons Attribution License (CC BY). The use, distribution or reproduction in other forums is permitted, provided the original author(s) and the copyright owner(s) are credited and that the original publication in this journal is cited, in accordance with accepted academic practice. No use, distribution or reproduction is permitted which does not comply with these terms.
*Correspondence: Laura Soucek, bHNvdWNla0B2aGlvLm5ldA==