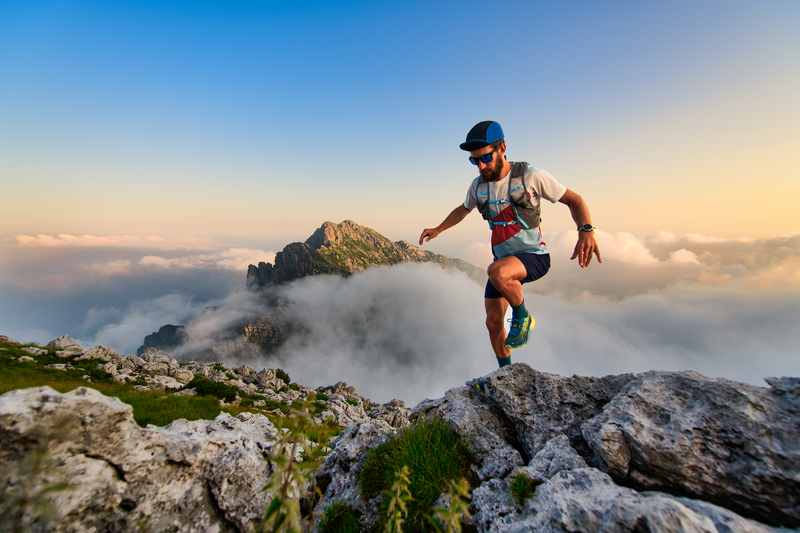
94% of researchers rate our articles as excellent or good
Learn more about the work of our research integrity team to safeguard the quality of each article we publish.
Find out more
REVIEW article
Front. Cell Dev. Biol. , 22 November 2023
Sec. Morphogenesis and Patterning
Volume 11 - 2023 | https://doi.org/10.3389/fcell.2023.1309557
Melanocytes, which originate from the neuroectoderm, are specialized cells responsible for producing pigments and possessing a dendritic morphology. These cells migrate to the epidermis and follicles, contributing to skin and hair pigmentation during embryonic development. The remarkable self-renewal capacity of melanocytes enables them to effectively restore hair and skin pigmentation. The synthesis of melanin to safeguard the skin against damage caused by ultraviolet radiation, as well as the enigmatic immune function of melanocytes, demonstrate their indispensable contributions to maintaining cutaneous homeostasis. The regulation of cutaneous pigmentation involves an intricate network influenced by intrinsic cellular signals within melanocytes and extracellular cues. Therefore, this paper provides a comprehensive review of the role of melanocytes in skin biology. This in-depth analysis could open novel avenues for research aimed at the prevention and treatment of skin disorders.
Melanocytes, referred to as pigment cells, are abundant within the human epidermis, with an approximate of 1,500 cells per square millimetre (Kanitakis, 2002). These pigmented cells are generally located in the dermis or epidermis and play a crucial role in skin pigmentation. They migrate along the dorsolateral pathway to connect the dermomyotome and the overlying ectoderm (Petit and Larue, 2016).
Melanoblasts, the precursors of melanocyte, can generate melanocyte stem cells (MSCs) and melanocytes during mouse embryo development (Cable et al., 1995; Mackenzie et al., 1997; Nishimura et al., 2002). Melanocytes are typically identified by the expression of melanocyte-specific proteins, including dopachrome tautomerase (DCT), tyrosinase-related protein 1 (TYRP1), tyrosinase (TYR), Pmel17/gp100, melanoma antigen recognized by T cells 1 (MART1), and/or microphthalmia-associated transcription factor (MITF) (Belote et al., 2021). However, identifying MSCs is more challenging because of their lack of melanin production. In addition, MSCs do not exhibit the typical protein markers (Nishimura et al., 2002). DCT and/or v-kit Hardy-Zuckerman 4 feline sarcoma viral oncogene homolog, also known as CD117 (C-KIT) may serve as detectable markers for these cells (Sun et al., 2019; Infarinato et al., 2020).
Technological advancements have gradually unveiled the specific functions and locations of melanocytes. Melanocytes and melanin have been detected in different anatomical regions, including the stria vascularis of the cochlea, the substantia nigra, leptomeninges, locus coeruleus within the brain, cardiac tissue, and adipose tissue (Plonka et al., 2009; van Beelen et al., 2020; Hutcheson et al., 2021; Ikeda et al., 2021). Moreover, Schwann cell precursors associated with nerves contribute to melanocytes in extracutaneous locations such as the heart, inner ear, supraorbital regions, and brain (Kaucka et al., 2021). Cells similar to melanocytes in the heart and pulmonary veins trigger atrial arrhythmia, whereas melanocytes with characteristics resembling macrophages residing adjacent to vessels in the inner ear maintain the integrity of the barrier between the intrastrial fluid and blood (Levin et al., 2009; Zhang et al., 2012). Melanocytes have also been identified in the limbal region, where they maintain limbal epithelial stem cells, regulate immune responses, and promote angiostasis (Polisetti et al., 2021).
This review provides a comprehensive summary of the developmental origins of melanocytes in mammals and elucidates how they are maintained by MSCs. We also explored the molecular mechanisms underlying melanocyte migration. This paper includes recent findings from studies that provide insights into the molecular mechanisms that influence skin and hair pigmentation in mammals. Furthermore, this review discusses the immune functions of melanocytes and outlines future research directions in the field of melanocyte biology.
During the initial migration, a sequence of shared transcriptional states is observed during the progression of neural crest cells (NCCs), followed by branching pathways that determine their fate. NCCs express pan-neural crest marker genes, including Sox10, Erbb3, Foxd3, Ets1, Plp1, and Tfap2a (Kastriti et al., 2022). These cells undergo differentiation via characteristic lineage-restriction events involving the simultaneous expression and rivalry of genes that influence alternative destinations. Furthermore, specification of neural and melanocyte cell types may occur through the sequential expression of lineage-specific transcription factors (Soldatov et al., 2019). During migration to the intracranial space, melanocyte-destined cells stall in the migration staging area (MSA), where they express the microphthalmia-associated transcription factor (MITF) (Weston, 1991; Nakayama et al., 1998). Neurogenin 2 (Neurog2), the transcription factor required for sensory neurogenesis, is expressed in NCCs between Embryonic Day (E) 8.5 and E10.5, when they delaminate from the neural tube (Sommer et al., 1996). Neurog2 is transiently expressed in all neural crest derivatives during differentiation. It has two expression peaks: one early after NCC delamination, which has minimal direct regulatory impact; and another late after the commencement of sensory neurogenesis, which can be connected to the corresponding regulatory activity. Neurog2 knockout from E9.5–E15.5 promotes skin melanocyte generation. Thus, Neurog2 is involved in the early repression of melanocyte specification (Soldatov et al., 2019) (Figure 1). In contrast, MITF expression only begins at E10.5, which encourages melanocyte development. There was low expression of Neurog2 at E11.5 within the MSA, whereas MITF was highly expressed in NCCs (Hari et al., 2012). However, the relationship between MITF and Neurog2 remains unclear.
FIGURE 1. Source and development of melanocytes. In mammals, melanoblast lineages are derived from neural crest cells (NCC) through the downregulation of FOXD3 and NEUROG2/or SOX2 in progenitor cells. SOX10 expression continues in the melanoblast lineages, followed by MITF, DCT, and C-KIT expression. After colonizing developing embryonic hair follicles, some melanoblasts differentiate into melanocytes and produce the pigment (melanin) that colors the first hair cycle. Some melanoblasts form melanocyte stem cells (MSCs) in the hair follicle bulge and secondary hair germ cells by downregulating MITF expression. These stem cells replenish mature melanocytes through activation and subsequent proliferation of transit-amplifying cells in the hair cycle. Schwann cell progenitors derived from SOX10-expressing NCC contribute to the population of pigmented cells by downregulating FOXD3 expression. In the skin, NCC like progenitor cells can differentiate into mature melanocytes. This illustration was created using BioRender.com.
Hair follicle (HF) MSCs located in the hair bulge and secondary hair germ (sHG) are the main sources of melanocytes. MSCs are immature, slow-cycling, and self-sustaining cells that can renew melanocytes within hair follicles (Nishimura et al., 2002; Sun et al., 2023). They exhibit specific molecular expression patterns that are positive for DCT and paired box 3 (PAX3) and negative for TYR, TYRP1, MITF, lymphoid enhancer-binding factor-1 (LEF1), SRY-Box transcription factor 10 (SOX10), and MKI67. In contrast, transiently amplified (TA) and terminally differentiated cells express these markers (Osawa et al., 2005). Additionally, C-KIT expression can be used to identify MSCs in hair follicles. A C-KIT-CreER-driven model and immunohistochemistry analyses revealed that approximately 70% of DCT+ MSCs in the bulge/sHG niche of hair follicles also express SOX10 and C-KIT. Furthermore, C-KIT-positive cells were occasionally observed in the dermis, suggesting the potential of C-KIT to target MSCs (Sun et al., 2019; Infarinato et al., 2020) (Figure 1).
Skin melanocytes have a neural crest origin and can be indirectly derived from Schwann cell progenitors (SCPs) after peripheral nerve colonization (Adameyko et al., 2009; Kaucka et al., 2021) (Figure 1). SCPs are NCC-derived cells expressing hub genes, including Serpine2, Itga4, Ednrb, Dlx1, Dlx2, Sox8, Sox10, Erbb3, Foxd3, Ets1, Plp1, and Tfap2 (Kastriti et al., 2022). At approximately E13, SOX10+ MITF+ cells were associated with nerves but were not in direct contact with them in mice. Furthermore, these cells express DCT, a key enzyme involved in melanin-mediated pigment synthesis. The myelin proteolipid protein (PLP)-CreERT2 mouse model was used for fluorescent fate mapping to explore the origin of nerve-associated melanoblasts (Adameyko et al., 2009). This model specifically labels SCPs and Schwann cells (SCs), enabling a detailed examination. A considerable proportion of postnatal skin melanocytes was derived from SCPs in mice. Moreover, SCPs situated along the elongating spinal nerves have been identified as a source of melanocytes. These pigmented cells differentiate later than melanocytes, which originate directly from NCCs. Schwann cell markers PLP1 and fatty acid binding protein 7 (FABP7) were substantially expressed in melanoblasts. However, the PLP-CreERT2 marker is not exclusive to SCPs. Additionally, this marker tags nerves and melanoblasts at various developmental stages in the skin. Thus, researchers used a glial-specific Dhh-Cre/R26R reporter mouse model to specifically label SCPs. However, there was no evidence of melanocytes originating from the SCPs. A recent study using the same Dhh-Cre driver line on a pure FVB/N background, along with a fluorescent Cre reporter, successfully labeled melanocytes, indicating that most skin melanocytes originate from SCPs (Colombo et al., 2012; Hari et al., 2012; Bonnamour et al., 2021).
There are several melanocyte precursor cells in the human interfollicular epidermis. C-KIT-CD90+ cells have neural crest characteristics and can differentiate into multiple lineages (Michalak-Micka et al., 2022). Similarly, dermal stem cells with neural-crest-like characteristics expressed the p75 neurotrophin receptor (NGFRp75), nestin, and octamer-binding transcription factor 4 (OCT4). However, they did not express melanocyte markers and could differentiate into melanocytes in human skin. Additionally, they acquired E-cadherin expression and lost NGFRp75 expression upon contact with epidermal keratinocytes (Li et al., 2010) (Figure 1). Even in the absence of appendages, the tail skin of mice retains a stable number of melanocytes, with a relatively low frequency of amelanotic and actively cycling differentiated melanocytes (Glover et al., 2015). This indicates that non-follicle-associated stem cells may contribute to interfollicular melanocytes.
Melanocytes rely on various transcription factors and signaling systems for their development. MITF is vital for melanocyte survival, migration, proliferation, and differentiation. It also promotes the specification of NCCs into melanoblasts and influences their survival by affecting C-KIT expression (Opdecamp et al., 1997; Hou and Pavan, 2008). Melanoblasts derived from NCCs express MITF before the initial expression of DCT. These MITF-positive cells, derived from NCCs, are defined as melanocyte precursors based on the coexpression of markers, such as C-KIT and DCT (Opdecamp et al., 1997; Hou et al., 2000) (Figure 1). MITF and C-KIT signaling are required for tyrosinase expression in melanoblasts and influence gene expression during melanocyte development (Hou et al., 2000). Additionally, MITF comprises various isoforms with distinct 5′ exons and different promoters. However, melanocytes predominantly express the M-isoform (Hershey and Fisher, 2005). MITF contributes to melanocyte differentiation by modulating pigmentation genes, including Tyr, Tyrp1, Dct, Pmel, and Mlan-a. It also contributes to cell survival by regulating anti-apoptotic genes, including Bcl2 and Bcl2a1. MITF further governs the cell cycle and melanocyte metabolism by regulating Cdk2 and Ppargc1a (McGill et al., 2002). It forms protein complexes with the co-factors histone acetyltransferase p300 and cyclic adenosine monophosphate (cAMP) response element-binding (CREB) protein and recruits polybromo and Brahma-related gene 1 (BRG1)-associated factor (PBAF) chromatin remodeling complexes, along with SOX10, transcription factor ap-2 alpha (TFAP2A), and yin yang 1 (YY1), to melanocyte lineage enhancers to regulate pigment gene transcription (Hu et al., 2007; Qi et al., 2013; Laurette et al., 2015).
SRY-box transcription factor 2 (SOX2) and MITF determine the fate of SCP progenitors and melanocytes in the neural crest through cross-regulatory interactions (Figure 1). The direct regulation of MITF by SOX2 occurs through its inhibitory binding to the Mitf promoter during development. The gradual decrease in SOX2 levels facilitates the differentiation of melanocytes from progenitors of both the neural crest and SCP (Adameyko et al., 2012). The winged-helix forkhead transcription factor forkhead box d3 (FOXD3) plays a critical role in NCC specification. FOXD3 acts in a bimodal manner during NCC development, maintaining multipotency and determining cell fates by switching between “permissive” and “repressive” nucleosome and chromatin organization (Lukoseviciute et al., 2018). Distinct lineage tracing of each population indicated that melanocytes derived from NCCs and SCPs were restricted to distinct regions of the body. The epaxial and hypaxial domains contained only melanocytes derived from NCCs and SCPs, respectively. Populations of epaxial and hypaxial melanocytes originate from FOXD3-positive neural cells. However, epaxial melanocytes separate from neural progenitors in the dorsal neural tube and downregulate Foxd3 expression, whereas hypaxial melanocytes lose Foxd3 expression at later stages after separation from the nerve. Furthermore, timely downregulation of FOXD3 is necessary for proper differentiation of melanocyte populations derived from NCCs and SCP (Nitzan et al., 2013). β-catenin promotes the SCP-derived melanocyte specification through MITF repression of FOXD3 (Colombo et al., 2022). Then, FOXD3 interacts with PAX3 to inhibit its binding to Mitf (Thomas and Erickson, 2009). Thus, FOXD3 acts as a switch between SCPs and melanocytes (Figure 1).
Melanocyte specification relies on the correct delivery of signals from the intrinsic and extracellular environments, which must occur in a timely and spatially controlled manner. This process is primarily regulated by molecules such as WNT, stem cell factor (SCF), bone morphogenetic protein (BMP), and endothelin (EDN) 3. Double knockout mice lacking WNT1 and WNT3a exhibit an almost complete loss of melanocytes, indicating an impact on several neural crest (NC) derivatives (Ikeya et al., 1997). Similarly, knockout mice lacking EDN3 or its receptor endothelin receptor b (EDNRB) show a significant loss of melanocytes (Baynash et al., 1994). Combined treatment with BMP4 and EDN3 effectively induces the sequential generation of NC cells and melanocyte precursors from pluripotent stem cells (Mica et al., 2013). In addition, this process involves β-Catenin, a downstream signaling component of WNT. A complete loss of DCT and MITF expression was observed in mutant embryos of β-catenin at E10.5 and E12.5 (Hari et al., 2002). However, the effect of WNT/β-catenin on melanocyte fate decisions is temporary. Activation of β-catenin in NCCs before migration hinders the development of melanocytes, whereas it stimulates melanocyte generation in migrating NCCs (Hari et al., 2002; Hari et al., 2012). However, the initial determination of Schwann cell precursors remains unaltered in the absence of β-catenin. MITF can redirect the transcriptional function of β-catenin towards target MITF-specific promoters rather than the genes regulated by β-catenin/LEF1. Thus, β-catenin/TCF-dependent gene expression is diminished by the overexpression of MITF, whereas that dependent on MITF is augmented by the overexpression of β-catenin (Schepsky et al., 2006). The interplay between WNT and Frizzled receptors (FZD), coupled with their co-receptor low-density lipoprotein receptor-related protein (LRP), stabilizes cytosolic β-catenin. As a result, the transported cytosolic β-catenin enters the nucleus, where it engages and interacts with transcription factors LEF/TCF to assert regulatory dominion over MITF transcription (Steingrimsson et al., 2004; Perugorria et al., 2019).
RNA editing is a crucial process involving the conversion of adenosine into inosine (A to I). The enzyme A-to-I deaminase (ADAR) plays an important role in this process. Conditional NCC ADAR1 knockout leads to global depigmentation, owing to melanocyte apoptosis without immune cell recruitment. The expression of melanocytic markers (SOX10, DCT, and TYR) was downregulated, whereas that of interferon-stimulated genes (ISG signature), including Cxcl10, Isg15, Ifit1, Ifit2, Rsad2, and Mx1, was considerably upregulated in half of the mutants. This was attributed to abnormal interferon production mediated by melanoma differentiation-associated protein 5 (MDA5) at E18.5. In contrast, no disparity was observed between mutants and controls at E16.5, the phase during which melanoblasts commonly initiate their transformation into hair follicles. This indicates that RNA editing plays a crucial role in the survival of melanocytes from E18.5 onwards (Gacem et al., 2020). However, additional studies are required to establish whether alterations occur in the functionality of mutant melanoblasts.
Melanocyte migration is regulated by several signaling pathways and transcription factors. The invasion of the skin by melanoblasts occurs when they enter the dermis at E9.5 during embryonic development. Melanoblasts successfully surpassed the basement membrane (BM) and established their residence in the epidermis at approximately E12.5 and E13.5. The migration of melanoblasts into growing HFs began at E15.5. Melanocytes initially migrate to the upper region of the HF, called the hair bulge, which is the permanent portion and thereafter function as the MSCs population. They continue to migrate as the anagen stage progresses, reaching the most distal part of the HF, known as the hair bulb and thereafter function as melanocytes. Postnatally, the presence of melanocytes in the epidermis of the back skin ceases in mice, and they are exclusively found within HFs, functioning as cells responsible for melanin production (Nishimura et al., 2002; Nishimura et al., 2005; Mort et al., 2015). Imaging and mathematical modeling studies on murine melanoblasts have shown that the migration of melanoblasts in developing dermis at E11.5–E12.5 or epidermis at E13.5–E15.5 do not have directionality (Mort et al., 2016).
Highly mobile melanoblasts move across the dermis and epidermis during embryogenesis. These cells use myosin motors to extend the short and long pseudopodia, enabling navigation through the epidermal keratinocyte layer. The rates of actin assembly and pseudopod extension in melanoblasts are controlled by Rac Family small GTPase 1 (RAC1), a Rho-family small GTPase, with stabilization provided by the actin-bundling protein fascin (Li et al., 2011; Ma et al., 2013). Furthermore, RAC1 activation is facilitated by an integrin-linked kinase (ILK) gene. The deletion of RAC1 and ILK in melanoblasts causes defects in cell migration, cell cycle progression, and cytokinesis (Crawford et al., 2020). Cell division control protein 42 homolog (CDC42) is another crucial regulator of the migration and proliferation of melanoblasts. Its absence results in various impairments in melanoblast migration, including disruption of actin dynamics, contractile activity, and adhesion (Woodham et al., 2017). Melanoblasts migration to the epidermis is accompanied by a change in the surface phenotype from E-cadherinlow P-cadherinhigh to E-cadherinhigh P-cadherinlow, which is reversed when migrating from the epidermis into hair follicles displaying the same patterns as surrounding cells in terms of expression of E- and P-cadherin (Nishimura et al., 1999). Furthermore, the efficiency of melanoblasts migration is related to precise coordination of integrin-mediated cell-extracellular matrix (ECM) adhesion (Haage et al., 2020). However, how different cadherins and integrins are regulated during melanoblasts migration still needs further investigation.
Colonization of the epidermis and HFs by melanocytes is an active process that requires functional C-KIT/SCF signaling. Forced expression of SCF in K14-Scf transgenic mice stimulates the proliferation, differentiation, and movement of melanoblasts during embryogenesis, along with MSCs during hair cycling. Consequently, an increase in the number of epidermal melanocytes and epidermal hyperpigmentation was observed. Additionally, robust SCF expression in the epidermis facilitates the migration of MSCs from the follicle to the epidermis on the dorsal skin of mice, particularly in response to ultraviolet radiation b (UVB) irradiation (Kunisada et al., 1998; Aoki et al., 2009; Yamada et al., 2013). Hair depigmentation was observed in Scfflox/gfp::K14-Cre mice, and upregulating the membrane-bound form of SCF in epidermal keratinocytes did not reverse it (Liao et al., 2017). Moreover, the offspring of mice administered blocking antibodies targeting the C-KIT during pregnancy had a white coat. This further confirmed the critical role of C-KIT/SCF signaling in facilitating melanoblast migration from the dermis to the epidermis and their subsequent localization within the follicles (Nishikawa et al., 1991). Recent studies have shown that SCF exhibits chemokinetic behavior rather than acting as a chemotactic factor (Jordan and Jackson, 2000). The movement of melanocytes into the HF throughout HF development and cycling is controlled by stromal cell-derived factor 1 (SDF-1), also known as C-X-C motif chemokine 12 (CXCL12), and its receptor, C-X-C chemokine receptor 4 (CXCR4). SDF-1 is expressed along the migration route followed by CXCR4-expressing cells in the outer root sheath of HFs. Additionally, CXCR4-positive cells in the HF bulge co-expressed nestin and LEX, which are markers of stem cells, along with SOX10, a marker of NCCs, and DCT, a marker of melanoblasts. During the normal positioning of melanoblasts in the skin at E13.5, the CXCR4 antagonists can lead to melanoblast concentrate in the epidermis without migration into HF. This suggests that SDF-1 promotes the migration of melanoblasts into HF (Figure 2). However, CXCR4 expression decreases considerably in differentiating melanoblasts and is enhanced during the anagen phase of the HF cycle (Belmadani et al., 2009). These findings indicate that external signals play a crucial role in melanoblasts/melanocytes movement. Moreover, it is imperative to adjust receptors associated with their surroundings and signaling alterations (Liao et al., 2017). However, the mechanism by which CXCR4 expression is regulated in melanocytes at different hair follicular stages remains unclear.
FIGURE 2. MSCs maintenance and activation in hair follicle stages. During telogen, niche ESC-derived TGF-β and Notch signaling synergistically maintain MSC stemness. Additionally, ESCs desensitize MSCs to differentiation signaling from SCF and EDN2 by downregulating retinoic acid (RA) levels in the niche and NFIB signaling, respectively. Sympathetic nerves terminate close to hair bulge MSCs and release noradrenaline (NA) to trigger MSCs depletion through ADRB2 receptor. Following the WNT-mediated activation of MSCs by niche ESCs, the BMP, WNT, and END1/2 pathways collaborate to trigger melanocyte proliferation and differentiation during anagen. Upon activation, SCF from the dermal papilla and CXCL12 of the outer hair root sheath direct the migration of MSCs towards the hair bulb. Some activated MSCs revert to a stem-like state upon migration into the WNT-negative bulge. This illustration was created using BioRender.com.
Melanocyte migration is not a one-way process. After wounding or UVB irradiation, MSCs can leave niches and generate epidermal melanocytes in the skin. The migration and differentiation of MSCs into melanocytes in the epidermis occur without proliferation and are dependent on the presence of the melanocortin 1 receptor (MC1R) (Figure 1). Furthermore, the number of MSCs directly migrating to the epidermis of the mouse back skin after wounding is lower in Mc1r mutant mice with nonfunctional MC1R than in littermate controls (Chou et al., 2013). In addition, the transgenic expression of Dkk1, an inhibitor of the WNT pathway, in melanocytes decreased their migration to the epidermis in the dorsal area of mouse wound scars. However, activating WNT signaling by genetically stabilizing β-catenin in melanocytes enhances the production of epidermal melanocytes. Impairing the secretion of WNT ligands, which activate the WNT pathway in basal epidermal keratinocytes, hinders WNT signal activation in epidermal melanocytes. However, the suppression of WNT ligand release by melanocytes does not affect their WNT stimulation, indicating that the paracrine communication of WNT from epidermal keratinocytes plays a vital role in the migration of MSCs towards the epidermis (Sun et al., 2018). In contrast, overexpression of EDN1 within epithelial cells can compensate for the impaired regeneration of epidermal melanocytes triggered by MC1R loss. This compensation occurs through synergistic effects with the WNT pathway, which enhances MSC proliferation and migration. Loss of EDNRB in MSCs compromises their migration to the epidermis after wounding, as demonstrated through EDNRB knockout, specifically in the melanocyte lineage in mice (Takeo et al., 2016). SCP-derived melanoblasts require EDNRB and WNT5a for expansion and EDNRB for migration (Adameyko et al., 2012). These results show that MSCs require both extrinsic and intrinsic cues to drive their migration. These findings further demonstrate that MSC migration is not static and is directed by environmental signals.
HF growth undergoes numerous cycles, including different phases: anagen (growth phase), catagen (regression phase), and telogen (resting phase). These phases are driven by the proliferation and differentiation of epithelial stem cells (ESCs) that express keratin 15 (K15) in the bulge area and sHG (Greco et al., 2009; Zhang et al., 2009). MSC differentiation and melanogenesis are coupled with distinct phases of the hair cycle (Slominski and Paus, 1993). MSCs can produce progeny that undergo rapid proliferation during the early anagen phase. These progeny subsequently mature into melanocytes that synthesize and transmit melanin to differentiating hair cells in the hair bulb, giving the hair a dark color (Infarinato et al., 2020). MSCs and ESCs are activated in coordination at the initiation of the new anagen phase, and synchronized activity continues throughout the hair cycle. Activation of WNT signaling in K15+ ESCs can activate and enhance the proliferation and differentiation of MSCs (Figure 2). This is partially achieved through the secretion of EDN1, a potent mitogen in melanocytes. MSCs express EDNRB, which binds to EDN 1, 2, and 3 to transduce EDN ligand signals at the onset of anagen (Sakurai et al., 1990; Saldana-Caboverde and Kos, 2010; Rabbani et al., 2011; Takeo et al., 2016). Some WNT-activated MSCs can differentiate into an intermediate state and revert to a stem-like state upon migration into a WNT-negative bulge area (Sun et al., 2023) (Figure 2). Moreover, MSCs undergo premature differentiation when the WNT/FZD pathway is activated. However, their differentiation into hair bulb melanocytes is impaired in the absence of β-catenin (Rabbani et al., 2011). Additionally, the coordination of epithelial-MSCs behavior is regulated by the transcription factor Nuclear Factor I B (NFIB), which negatively regulates EDN2 and inhibits MSC differentiation in a C-KIT-dependent manner in ESCs (Chang et al., 2013) (Figure 2). SCF acts as a dermal papillary signal that promotes MSC migration and differentiation. Its overexpression in the hair follicle epithelium increased the proliferative activity of melanocytes (Figure 2). When a C-KIT antibody was injected during the catagen/telogen stage in anagen hair follicles, it only affected the proliferation and differentiation of lineage-committed cells. However, undifferentiated MSCs remained elevated in the hair follicles of Nfibfl/fl-K15-cre mice, suggesting that MSCs respond to SCF differently depending on the stage of development and the environment in which they reside (Botchkareva et al., 2001). During onset of catagen development, the maximal expression of transforming growth factor-β (TGF-β) by bulge outer root sheath was observed (Foitzik et al., 2000). This may lead to the apoptosis of melanocytes in catagen (Sharov et al., 2005; Nishimura et al., 2010).
The bulge must sustain a sufficient number of MSCs for subsequent hair growth cycles to ensure sustainability (Infarinato et al., 2020). Niche ESCs play a crucial role in the telogen phase (Nishimura et al., 2010). TGF-β signaling from ESCs temporarily halts the cell cycle and supports melanocyte immaturity by reducing MITF levels (Figure 2). During the hair cycle, the activation of TGF-β signaling occurs in MSCs as they transition into quiescence state and requires B-cell lymphoma 2 (BCL-2) to antagonize the pro-apoptotic effect of TGF-β. In addition, loss of TGF-β type II receptor (TGFbRII) within the melanocyte lineage leads to the incomplete preservation of MSC immaturity (Nishimura et al., 2010). Collagen XVII (COL17A1), highly expressed in ESCs, maintains TGF-β signaling by mediating anchorage (Nishimura et al., 2005; Nishimura et al., 2010; Tanimura et al., 2011). Moreover, MSCs within their niche rely on BRAF and CRAF for self-maintenance. These two RAF proteins are functionally redundant and compensate for each other. The RAF/extracellular signal-regulated kinase (ERK) pathway regulates the cell cycle, and the absence of RAF proteins in knockout mice affects the entry of MSCs into S phase (Valluet et al., 2012). Furthermore, the Notch signaling pathway determines the fate of MSCs and melanoblasts. In skin organ cultures, the inhibition of Notch signaling led to the apoptosis of MSCs and melanoblasts (Figure 2). Conversely, overexpression of Hes1, specifically in MSCs and melanoblasts, protects melanoblasts from apoptosis by preventing its initiation (Moriyama et al., 2006; Kumano et al., 2008). Although C-KIT signaling plays a vital role in the migration, survival, and differentiation of MSCs, its presence is not required for the self-maintenance of MSCs within their niche. However, ESCs regulate retinoid metabolism, which leads to the downregulation of retinoic acid levels, to desensitize MSCs to differentiation signaling from the C-KIT ligand (Lu et al., 2020) (Figure 2). These results show that signaling from ESCs contributes to the preservation of their regenerative potential by maintaining a self-renewal niche for MSCs.
The transcriptional features of MSCs as they progress through the different stages of their lineage are complex. MSCs and their progeny universally express certain pan-lineage genes such as Sox10. They displayed selective upregulation of gene expression, including that of Pax3, Sbno2, and Bcl2 during the transition from the quiescent to the activated stage, accompanied by high expression of proliferation-related genes (i.e., Mki67 and Ccna2) (Figure 1). In contrast, the transcripts involved in pigment production, such as melanin production enzymes (TYR and TYRP1) and melanosome-related proteins (MART1 and Oculocutaneous albinism type 2 (OCA2)), were elevated during the transition from proliferation to maturity (Figure 1). Mature melanocytes exhibited considerably lower transcript levels of key cell cycle genes than those in the activated stage. Additionally, they were enriched in C-KIT/SCF, WNT signaling (LEF1), and BMP signaling (Infarinato et al., 2020) (Figure 2). However, unlike those in the secondary hair germ (sHG) (CD34− area), MSCs within the bulge (CD34+ area) did not activate WNT signaling at anagen onset. Furthermore, they proliferated even after induction by the WNT ligand (Rabbani et al., 2011). Fluorescence-activated cell sorting recently demonstrated that CD34+ and the CD34- MSCs are functionally distinct. The expression of key melanocyte differentiation genes, including Mitf, Tyr, Tyrp1, Pmel, Pax3, Mc1r, Erbb3, Sox10, Melan-A, and Slc45a2, was elevated in CD34- MSCs. In contrast, CD34+ MSCs displayed a gene expression profile more consistent with a neural crest stem cell, with higher Nr2f2, Nr2f1, Ngfr (p75), Twist1, Twist2, Snai1, Sox9, EdnrA, Gli1, Bmp2, Bmp4, and Bmp7 levels observed (Joshi et al., 2019). These observations suggest that there may be intrinsic heterogeneity among follicular MSCs with differential responsiveness to WNT ligands and SCF. However, additional research is required to fully evaluate this possibility.
Melanocytes mainly produce two types of melanin: eumelanin and pheomelanin. Eumelanin is dark brown and is the primary pigment for protection against ultraviolet radiation (UVR). In contrast, pheomelanin is reddish-yellow and is more susceptible to UVR-induced damage. Melanocytes, which are cells responsible melanin production, generate this pigment within cellular structures known as melanosomes. Melanosomes can be categorized into four stages, ranging from early and unpigmented (stages I–II) to late and pigmented (stages III–IV). These stages can be distinguished based on their morphology. Stage I melanosomes exhibit non-pigmented vacuoles, whereas stage II melanosomes exhibit internal striations. Melanin gradually accumulates on fibrils during stage III, eventually leading to the fully melanized stage IV (Tian et al., 2021). Mature melanosomes are ultimately transferred to epidermal keratinocytes. They then aggregate above the nuclei of keratinocytes that face the sun (Park et al., 2009).
The enzymatic machinery and structural components of melanosomes must be appropriately assembled into newly formed melanosomes. Melanosome formation begins with fiber formation by melanosome structural proteins, such as PMEL17. MART1 binds to this structural protein to facilitate its expression, stability, and trafficking (Tian et al., 2021). The formation of PMEL17 amyloid fibers is affected by pH levels and is most suitable for mildly acidic pH levels (4.5–5.5) (Pfefferkorn et al., 2010). V-ATPase, an H+ pump, is expressed in stage I premelanosomes, creating an acidic environment (Tabata et al., 2008). Melanogenesis begins at stage III, when TYR, TYRPs, ATPase copper transporting alpha (ATP7A), OCA2, SLC45A2, and two pore segment channel 2 (TPC2) are located from the trans-Golgi network to melanosomes. ATP7A functions as a Cu2+ pump, providing Cu2+ as a TYR cofactor. OCA2, SLC45A2, and TPC2 are membrane transport proteins that are essential for converting acidic pH to neutral pH (Le et al., 2020; Wiriyasermkul et al., 2020). Enzymes, such as TYR, TYRP1, and DCT, are required to initiate the oxidation of tyrosine to L-DOPA during melanin production in fully striated melanosomes. Subsequently, L-DOPA is transformed into DOPAquinone, which serves as a precursor of eumelanin and pheomelanin (Raposo and Marks, 2007; Centeno et al., 2023). TYR degradation is regulated by nicotinamide nucleotide transhydrogenase (NNT), an enzyme involved in the mitochondrial redox-regulating pathway, through a ubiquitin-proteasome mechanism (Allouche et al., 2021). A CRISPR-Cas9 genetic screen recently uncovered 135 melanin-promoting genes that had not been previously identified. A notable decrease in melanin production was observed upon the deletion of these genes. The identified melanin-promoting genes play crucial roles in various biological pathways, including transcriptional regulation, RNA processing, and endosomal transport. Depletion of the transcript factor KLF downregulated TYR expression. Furthermore, the endosomal trafficking protein COMM Domain Containing 3 (COMMD3) is required for neutral melanosomal pH maintenance (Bajpai et al., 2023).
The skin is the primary barrier to the external environment and relies on melanin for photoprotection (Singh et al., 2017). Melanin transfer from melanocytes to keratinocytes is influenced by calcium flux regulation. UVR also elevates intra-melanocyte Ca2+ levels by activating calcium release-activated calcium Modulator 1 (ORAI1) ion channels that are calcium selective. This leads to upregulation of the expression of filopodia-associated proteins, including E-Cadherin, CDC42, vasodilator-stimulated phosphoprotein (VASP), and β-catenin (Figure 3). The inhibition of E-cadherin expression and knockdown of transient receptor potential cation channel subfamily M member 1 (TRPM1) reduces the transfer of melanosomes from melanocytes to keratinocytes following exposure to ultraviolet radiation a (UVA) or UVB radiation (Hu et al., 2017; Singh et al., 2017). EDN and acetylcholine from keratinocytes trigger localized dendritic Ca2+ transients within melanocyte dendrites in vitro (Belote and Simon, 2020) (Figure 3). The coordination of melanocyte-keratinocyte communication and contact by caveolae is also crucial for melanosome transfer to keratinocytes. UVR and keratinocyte-released factors lead to the preferential location of caveolae at the melanocyte-keratinocyte interface (Figure 3). Cav1/caveolae downregulation induces pigment production in melanocytes by increasing cAMP production, whereas upregulation favors changes in cell morphology and promotes contact with keratinocytes, both of which lead to melanin transfer and skin pigmentation. These findings demonstrate that melanocytes effectively respond to external signals from keratinocytes by utilizing the signaling and mechanistic mechanisms of the caveolae (Domingues et al., 2020).
FIGURE 3. Major signaling pathways involved in skin pigmentation and melanin transfer. Keratinocytes regulate melanogenesis through various paracrine signaling pathways that converge at the CRTC3/CREB/MITF axis. Signaling from neighboring keratinocyte-secreted factors, including acetylcholine (ACh), can trigger localized Ca2+ transients that initiate melanin transfer within melanocyte dendrites. PGE2 from keratinocytes stimulate melanocyte filopodia formation. Melanocytes: Keratinocyte-contact-dependent melanin transfer is mediated by E-cadherin and Cav1/caveolae, whereas melanin secreted by melanocytes is phagocytosed by keratinocytes in a PAR-2 dependent manner. This illustration was created using BioRender.com.
Pigmentation occurs when melanin is synthesized within lysosome-like structures known as melanosomes by melanocytes and then delivered to keratinocytes (Raposo and Marks, 2007). There are four possible models of melanosome transfer from melanocytes to keratinocytes mainly based on in vitro experiments (Wu and Hammer, 2014; Tadokoro and Takahashi, 2017). In the cytophagocytosis model, keratinocytes can phagocytize entire constituents of melanocytes (e.g., dendrites or filopodia) as a means of internalizing melanin. In the fusion model, melanosomes move along filopodia and form a channel into the keratinocyte. It is believed that filopodia fuse with the keratinocyte membrane during melanosome transfer. In the vesicle transfer model, membrane vesicles containing either individual or groups of melanosomes are released from the melanocyte and then captured and phagocytized by keratinocytes. In the exocytosis model, melanin units without their melanosomal membranes (termed melanocores) are released into the extracellular space between the melanocyte and keratinocyte. Subsequently, the melanocores are internalized by keratinocytes through phagocytosis, which relies on the activation of protease-activated receptor-2 (PAR-2) (Wu and Hammer, 2014; Tadokoro and Takahashi, 2017; Moreiras et al., 2022). Following the transfer of melanin to keratinocytes, the cytoplasmic intermediate protein chains of dynein (Dync1i1) link the entire motor complex and the p150Glued (DCTN1) subunit of the dynactin complex, which regulates the localization of perinuclear melanin (Byers et al., 2003; Byers et al., 2007). The calcium-dependent G protein-coupled and Akt signaling pathways upregulate the expression of DCTN1 in keratinocytes after exposure to UVA radiation. This process is facilitated by opsin3 (OPN3) (Lan et al., 2023). Melanocyte uptake induces melanin accumulation inside keratinocytes within hybrid endocytic compartments that exhibit low acidity and limited degradation capacity. These distinct endosomes enable melanin to persist in keratinocytes for an extended duration by evading lysosomal degradation (Correia et al., 2018).
Skin pigmentation, a crucial defense process against UV light, involves key cellular players in the epidermis, namely, melanocytes and keratinocytes. The close interconnections between these cellular types play a vital role in the regulation of skin pigmentation. Although the regulation of melanin biosynthesis involves various signaling pathways and factors, its pivotal role in UVB-induced MITF expression and melanogenesis can be attributed to cAMP and CREB (Yoo et al., 2021). UVR induces melanocyte-stimulating hormone (α-MSH) secretion in keratinocytes in a p53-dependent manner (Cui et al., 2007). The binding of α-MSH to receptor MC1R on melanocytes triggers the signaling pathway, which begins with Gαs activation of adenylate cyclase (Figure 3). This increases cAMP levels and phosphorylation of CREB transcription factor family members through PKA signaling (Cui et al., 2007). CREB transcriptionally activates MITF, which induces TYRP1, DCT, and TYR, subsequently driving melanosome maturation and increasing eumelanin production (Iozumi et al., 1993; Levy et al., 2006; Paterson et al., 2015; Tian et al., 2021) (Figure 3). The elevation in cAMP levels also significantly decreases the levels of CLEC12B, a C-type lectin receptor that inhibits CREB protein degradation (Sormani et al., 2022). Additionally, cAMP-regulated transcription co-activators 1–3 (CRTC1-3) are sensors and key regulators of melanogenesis that act as effectors of cAMP signaling (Yoo et al., 2021). Salt-inducible kinases (SIKs) initially retain CRTCs in the cytoplasm through phosphorylation at the 14-3-3 binding sites. Elevated cAMP levels promote protein kinase A (PKA)-mediated phosphorylation, which inhibits SIKs. Then, nuclear migration occurs following CRTC dephosphorylation, leading to recruitment to the binding sites of CREB. This recruitment is essential for activating CREB complex-mediated transcription, which is necessary for complete activation of CREB-mediated transcription (Altarejos and Montminy, 2011). In contrast to the nonspecific stimulation of all CRTCs caused by high cAMP levels, CRTC3 is synergistically activated by ERK1/2 and cAMP during low cAMP signaling in melanocytes. Melanocyte mutants with CRTC3 exhibit flawed maturation of melanosomes, owing to the reduced expression of OCA2, an essential pigment regulator (Ostojic et al., 2021) (Figure 3).
Paracrine signals from keratinocytes modulate melanogenesis. Basic fibroblast radiation factor (bFGF) expression is upregulated in keratinocytes and promotes melanocyte proliferation and melanin synthesis (Halaban et al., 1988). It binds to its receptor on melanocytes to enhance PAX3 expression (Dong et al., 2012). UV irradiation also upregulates the expression of keratinocyte-derived nerve growth factor (NGF), which is chemotactic in melanocytes and induces their dendricity (Yaar et al., 1991). NGF helps preserve UV-induced depletion of cutaneous melanocytes by increasing the expression of the anti-apoptotic BCL-2 protein (Stefanato et al., 2003). Hepatocyte growth factor (HGF), mainly produced by keratinocytes and fibroblasts, is the exclusive ligand of cellular mesenchymal epithelial transition (c-MET) factor, a membrane-bound receptor with kinase activity (Upadhyay et al., 2021). HGF/c-MET signaling activates mitogen-activated protein kinases (MAPKs) and phosphoinositide 3-kinases (PI3K)/protein kinase B (AKT) signaling to modulate CREB activity. It further affects melanocyte proliferation, motility, and survival. UV-induced secretion of interleukin (IL)-1α by keratinocytes also upregulates HGF synthesis by fibroblasts (Mildner et al., 2007; Czyz, 2018). After UVB radiation, adenosine 50-triphosphate (ATP) release increases in keratinocytes. Thus, ATP can act as an extracellular signaling molecule by activating cell surface P2X7 receptors to promote melanin production in melanocytes. Although ATP increases intracellular Ca2+, p-CREB, and MITF levels in melanocytes, an inhibitor of protein kinase C (PKC) abrogates this effect. This suggests that the Ca2+ influx/PKC/p-CREB/MITF axis is involved in ATP-induced melanogenesis (Khakh and North, 2012; Lee et al., 2019). Filopodial-associated E-Cadherin, VASP, CDC42, and β-catenin, whose expression was upregulated by UVR/UVA and Ca2+ in melanocytes, are required for melanin transfer (Singh et al., 2017) (Figure 3). Exosomes, soluble factors released by keratinocytes, carry membrane proteins and cytosolic components modulated by UVB. In addition, they could play a role in the regulation of melanogenesis (Lo Cicero et al., 2015). Low-dose UVB irradiation induces the expression of the gene Col2a1, encoding ECM. Collagen type II alpha 1 (COL2A1) promotes melanogenesis through activation of the MAPK pathway (Li et al., 2021).
Inflammatory responses are also involved in UVB-induced melanocyte proliferation and skin pigmentation. UVB radiation stimulates the release of the C-C chemokine receptor type 2 (CCR2) ligand C-C Motif Chemokine Ligand (CCL) 8 by melanocytes, which attracts CCR2+ macrophages to the skin. The recruited macrophages produce Interferon (IFN), which promotes melanocyte proliferation and migration (Zaidi et al., 2011) (Figure 4). However, Ly6clowMHCIIhi macrophages primarily promote melanocyte reactivation, which is partly IL-17 dependent and CCR2 independent (Handoko et al., 2013). UV exposure increases keratinocyte synthesis of IL-1α, IL-6, and tumor necrosis factor (TNF)-α (Kock et al., 1990; Chung et al., 1996), which also inhibit melanocyte proliferation and melanogenesis (Swope et al., 1991). UVR induces the release of prostaglandin E(2) [PGE(2)] by keratinocytes, thereby stimulating filopodia formation in melanocytes. PGE(2) also stimulates EP4 receptor signaling in melanocytes, resulting in increased tyrosinase activity and proliferation (Starner et al., 2010) (Figure 3).
FIGURE 4. Melanocytes as sentinel immune cells in the skin. Melanocytes sense and respond to pathogens through intracellular or extracellular pattern recognition receptors, such as RIG-I, MDA5, and TLRs. These receptors activate melanocytes. Then, innate immune molecules (e.g., chemokines and cytokines) are produced to alert professional immune cells (e.g., macrophages) in a concerted effort to thwart off pathogens. Furthermore, melanocytes have a pathogen phagocytosis ability and present antigens to T cells. This illustration was created using BioRender.com.
An earlier study found that intraepidermal free nerve endings (IEFNs) were in contact with melanocytes. The ultrastructural features of the chemical synapses can be observed in these contacts. IEFNs show enlarged endings that form boutons closely adjacent to melanocyte cell bodies, resulting in membrane-to-membrane appositions. Furthermore, both neurites and melanocytes exhibited membrane thickening in proximity to vesicles that aggregated in nerve fibers, indicating possible efferent communication from IEFNs to melanocytes. Additionally, certain fibers complete their course by being enveloped within melanocyte cytoplasmic invaginations (Hara et al., 1996). A higher occurrence of contact between sensory neuron terminals and melanocytes was observed within the area of senile lentigo (SL) than in the encompassing region. Repulsive guidance molecule B (RGMB) secreted by neurons promotes melanogenesis, and the survival of melanocytes is higher in senile lentigo (SL) than in the healthy surrounding skin (Chow et al., 2022). Similarly, sympathetic nerves terminate close to hair bulge MSCs and release noradrenaline to trigger MSC depletion through adrenoceptor beta 2 (ADRB2) receptor (Zhang et al., 2020) (Figure 2). In addition, stimulation with the calcitonin gene-related peptide (CGRP) induced melanocyte proliferation and increased intracellular cAMP accumulation (Toyoda et al., 1999). However, the prevalence of CGRP-expressing IEFNs in the central depigmented region, which lacks melanocytes, exceeds that in unaffected vitiligo skin (Lazarova et al., 2000). These results suggest that the cutaneous nervous system disrupts melanocyte regulation under pathological skin conditions.
Melanocytes are not only responsible for melanin production. They also actively contribute to the functioning of the cutaneous immune system. The large surface area, dendritic structure, and strategic location of the superficial layers of melanocytes provide the structural foundation and potential to serve as skin sentinel cells (Plonka et al., 2009). Human melanocytes express functional toll-like receptors (TLRs), including TLRs 2, 3, 4, 6, 7, and 9. In vitro stimulation of TLR2 and 4 by Poly I:C and Lipopolysaccharide (LPS) induced IL-8 and IL-6 production in melanocytes, respectively. Furthermore, TLRs 2, 3, 4, 6, and 9 stimulation upregulated the expression of chemokines involved in leukocyte recruitment (CCL2, CCL3, and CCL5), which was not the case with TLR7 stimulation (Yu et al., 2009; Yu et al., 2021). Melanocytes also express other pattern recognition receptors (PRRs), including retinoic acid-inducible gene (RIG)-I-like receptors (RLRs) and MDA5, to detect and respond to pathogens. These receptors will initiate signaling pathways that promote the expression of type-I interferons (IFN-α/β) (Besch et al., 2009; Wang et al., 2015). Melanocytes have phagocytic abilities and most express MHC class I molecules. They also express MHC class II molecules through IFN-γ stimulation in vitro (Le Poole et al., 1993; Hedley et al., 1998). IFN-γ stimulation promotes CD40 expression by melanocytes. It further promotes the expression of the co-stimulating molecule intercellular adhesion molecule 1 (ICAM-1) following CD40 ligation, indicating the melanocyte ability to bind antigens and activate T cells (Lu et al., 2002; Polisetti et al., 2021). Melanocytes can also produce IL-3, IL-6, and TNF-α to trigger the maturation of plasmacytoid dendritic cells (pDCs) (Halasi et al., 2023). However, their immunological capacity remains largely unexplored, and most empirical observations have been derived from in vitro studies. Therefore, further investigations are required to establish a holistic understanding of the immunological function of melanocytes (Koike and Yamasaki, 2020) (Figure 4).
This review elucidates the function, migration, and regulation of melanocytes in the skin. The contributions of melanocytes to cutaneous homeostasis, as well as the reliance of their migration on environmental signals have been elucidated in existing literature. Furthermore, the mechanistic mechanisms of the caveolae have been linked to melanocyte responses to external signals. Although technological advances have gradually revealed the function and regulation of melanocytes over the years, there remain many unknown aspects of their biology, owing to the complexity of their regulatory mechanism. Whether melanocytes can be derived from nerve cells, have nerve-like functions, can regulate keratinocyte function in addition to delivering melanin to keratinocytes, or have “autonomy” warrants further investigation. Furthermore, the mechanisms underlying the regulation of melanocytes by nerves in the skin remain unclear. Similarly, the mechanisms underlying the balance of different signaling pathways to enable melanocyte migration and function warrant further study. Therefore, a comprehensive understanding of melanocyte biology requires new research modalities. The role of melanocytes in the skin will become more prominent with further research, providing new perspectives on the pathogenesis and treatment of skin diseases.
Y-ZC: Conceptualization, Formal Analysis, Resources, Writing–original draft. X-YM: Conceptualization, Funding acquisition, Writing–review and editing.
The authors declare financial support was received for the research, authorship, and/or publication of this article. This study was supported by grants from the National Natural Science Foundation of China (Nos 82230104, 82203905, and 81930089).
The authors wish to thank Prof. Yong Yang, Prof. Qing-Sheng Mi for providing kind advice on this project.
The authors declare that the research was conducted in the absence of any commercial or financial relationships that could be construed as a potential conflict of interest.
All claims expressed in this article are solely those of the authors and do not necessarily represent those of their affiliated organizations, or those of the publisher, the editors and the reviewers. Any product that may be evaluated in this article, or claim that may be made by its manufacturer, is not guaranteed or endorsed by the publisher.
Adameyko, I., Lallemend, F., Aquino, J. B., Pereira, J. A., Topilko, P., Muller, T., et al. (2009). Schwann cell precursors from nerve innervation are a cellular origin of melanocytes in skin. Cell 139 (2), 366–379. doi:10.1016/j.cell.2009.07.049
Adameyko, I., Lallemend, F., Furlan, A., Zinin, N., Aranda, S., Kitambi, S. S., et al. (2012). Sox2 and Mitf cross-regulatory interactions consolidate progenitor and melanocyte lineages in the cranial neural crest. Development 139 (2), 397–410. doi:10.1242/dev.065581
Allouche, J., Rachmin, I., Adhikari, K., Pardo, L. M., Lee, J. H., McConnell, A. M., et al. (2021). Nnt mediates redox-dependent pigmentation via a uvb- and mitf-independent mechanism. Cell 184 (16), 4268–4283.e20. doi:10.1016/j.cell.2021.06.022
Altarejos, J. Y., and Montminy, M. (2011). Creb and the crtc Co-activators: sensors for hormonal and metabolic signals. Nat. Rev. Mol. Cell Biol. 12 (3), 141–151. doi:10.1038/nrm3072
Aoki, H., Yamada, Y., Hara, A., and Kunisada, T. (2009). Two distinct types of mouse melanocyte: differential signaling requirement for the maintenance of non-cutaneous and dermal versus epidermal melanocytes. Development 136 (15), 2511–2521. doi:10.1242/dev.037168
Bajpai, V. K., Swigut, T., Mohammed, J., Naqvi, S., Arreola, M., Tycko, J., et al. (2023). A genome-wide genetic screen uncovers determinants of human pigmentation. Science 381 (6658), eade6289. doi:10.1126/science.ade6289
Baynash, A. G., Hosoda, K., Giaid, A., Richardson, J. A., Emoto, N., Hammer, R. E., et al. (1994). Interaction of endothelin-3 with endothelin-B receptor is essential for development of epidermal melanocytes and enteric neurons. Cell 79 (7), 1277–1285. doi:10.1016/0092-8674(94)90018-3
Belmadani, A., Jung, H., Ren, D., and Miller, R. J. (2009). The chemokine sdf-1/cxcl12 regulates the migration of melanocyte progenitors in mouse hair follicles. Differentiation 77 (4), 395–411. doi:10.1016/j.diff.2008.10.015
Belote, R. L., Le, D., Maynard, A., Lang, U. E., Sinclair, A., Lohman, B. K., et al. (2021). Human melanocyte development and melanoma dedifferentiation at single-cell resolution. Nat. Cell Biol. 23 (9), 1035–1047. doi:10.1038/s41556-021-00740-8
Belote, R. L., and Simon, S. M. (2020). Ca2+ transients in melanocyte dendrites and dendritic spine-like structures evoked by cell-to-cell signaling. J. Cell Biol. 219 (1), e201902014. doi:10.1083/jcb.201902014
Besch, R., Poeck, H., Hohenauer, T., Senft, D., Hacker, G., Berking, C., et al. (2009). Proapoptotic signaling induced by rig-I and mda-5 results in type I interferon-independent apoptosis in human melanoma cells. J. Clin. Invest. 119 (8), 2399–2411. doi:10.1172/JCI37155
Bonnamour, G., Soret, R., and Pilon, N. (2021). Dhh-expressing Schwann cell precursors contribute to skin and cochlear melanocytes, but not to vestibular melanocytes. Pigment. Cell Melanoma Res. 34 (3), 648–654. doi:10.1111/pcmr.12938
Botchkareva, N. V., Khlgatian, M., Longley, B. J., Botchkarev, V. A., and Gilchrest, B. A. (2001). Scf/C-Kit signaling is required for cyclic regeneration of the hair pigmentation unit. FASEB J. 15 (3), 645–658. doi:10.1096/fj.00-0368com
Byers, H. R., Dykstra, S. G., and Boissel, S. J. (2007). Requirement of dynactin P150(glued) subunit for the functional integrity of the keratinocyte microparasol. J. Invest. Dermatol 127 (7), 1736–1744. doi:10.1038/sj.jid.5700760
Byers, H. R., Maheshwary, S., Amodeo, D. M., and Dykstra, S. G. (2003). Role of cytoplasmic dynein in perinuclear aggregation of phagocytosed melanosomes and supranuclear melanin cap formation in human keratinocytes. J. Invest. Dermatol 121 (4), 813–820. doi:10.1046/j.1523-1747.2003.12481.x
Cable, J., Jackson, I. J., and Steel, K. P. (1995). Mutations at the W locus affect survival of neural crest-derived melanocytes in the mouse. Mech. Dev. 50 (2-3), 139–150. doi:10.1016/0925-4773(94)00331-g
Centeno, P. P., Pavet, V., and Marais, R. (2023). The journey from melanocytes to melanoma. Nat. Rev. Cancer 23 (6), 372–390. doi:10.1038/s41568-023-00565-7
Chang, C. Y., Pasolli, H. A., Giannopoulou, E. G., Guasch, G., Gronostajski, R. M., Elemento, O., et al. (2013). Nfib is a governor of epithelial-melanocyte stem cell behaviour in a shared niche. Nature 495 (7439), 98–102. doi:10.1038/nature11847
Chou, W. C., Takeo, M., Rabbani, P., Hu, H., Lee, W., Chung, Y. R., et al. (2013). Direct migration of follicular melanocyte stem cells to the epidermis after wounding or uvb irradiation is dependent on Mc1r signaling. Nat. Med. 19 (7), 924–929. doi:10.1038/nm.3194
Chow, S. Y. A., Nakayama, K., Osaki, T., Sugiyama, M., Yamada, M., Takeuchi, H., et al. (2022). Human sensory neurons modulate melanocytes through secretion of rgmb. Cell Rep. 40 (12), 111366. doi:10.1016/j.celrep.2022.111366
Chung, J. H., Youn, S. H., Koh, W. S., Eun, H. C., Cho, K. H., Park, K. C., et al. (1996). Ultraviolet B irradiation-enhanced interleukin (Il)-6 production and mrna expression are mediated by il-1 alpha in cultured human keratinocytes. J. Invest. Dermatol 106 (4), 715–720. doi:10.1111/1523-1747.ep12345608
Colombo, S., Champeval, D., Rambow, F., and Larue, L. (2012). Transcriptomic analysis of mouse embryonic skin cells reveals previously unreported genes expressed in melanoblasts. J. Invest. Dermatol 132 (1), 170–178. doi:10.1038/jid.2011.252
Colombo, S., Petit, V., Wagner, R. Y., Champeval, D., Yajima, I., Gesbert, F., et al. (2022). Stabilization of beta-catenin promotes melanocyte specification at the expense of the Schwann cell lineage. Development 149 (2), dev194407. doi:10.1242/dev.194407
Correia, M. S., Moreiras, H., Pereira, F. J. C., Neto, M. V., Festas, T. C., Tarafder, A. K., et al. (2018). Melanin transferred to keratinocytes resides in nondegradative endocytic compartments. J. Invest. Dermatol 138 (3), 637–646. doi:10.1016/j.jid.2017.09.042
Crawford, M., Leclerc, V., Barr, K., and Dagnino, L. (2020). Essential role for integrin-linked kinase in melanoblast colonization of the skin. J. Invest. Dermatol 140 (2), 425–434. doi:10.1016/j.jid.2019.07.681
Cui, R., Widlund, H. R., Feige, E., Lin, J. Y., Wilensky, D. L., Igras, V. E., et al. (2007). Central role of P53 in the suntan response and pathologic hyperpigmentation. Cell 128 (5), 853–864. doi:10.1016/j.cell.2006.12.045
Czyz, M. (2018). Hgf/C-Met signaling in melanocytes and melanoma. Int. J. Mol. Sci. 19 (12), 3844. doi:10.3390/ijms19123844
Domingues, L., Hurbain, I., Gilles-Marsens, F., Sires-Campos, J., Andre, N., Dewulf, M., et al. (2020). Coupling of melanocyte signaling and mechanics by caveolae is required for human skin pigmentation. Nat. Commun. 11 (1), 2988. doi:10.1038/s41467-020-16738-z
Dong, L., Li, Y., Cao, J., Liu, F., Pier, E., Chen, J., et al. (2012). Fgf2 regulates melanocytes viability through the stat3-transactivated Pax3 transcription. Cell Death Differ. 19 (4), 616–622. doi:10.1038/cdd.2011.132
Foitzik, K., Lindner, G., Mueller-Roever, S., Maurer, M., Botchkareva, N., Botchkarev, V., et al. (2000). Control of murine hair follicle regression (catagen) by tgf-beta1 in vivo. FASEB J. 14 (5), 752–760. doi:10.1096/fasebj.14.5.752
Gacem, N., Kavo, A., Zerad, L., Richard, L., Mathis, S., Kapur, R. P., et al. (2020). Adar1 mediated regulation of neural crest derived melanocytes and Schwann cell development. Nat. Commun. 11 (1), 198. doi:10.1038/s41467-019-14090-5
Glover, J. D., Knolle, S., Wells, K. L., Liu, D., Jackson, I. J., Mort, R. L., et al. (2015). Maintenance of distinct melanocyte populations in the interfollicular epidermis. Pigment. Cell Melanoma Res. 28 (4), 476–480. doi:10.1111/pcmr.12375
Greco, V., Chen, T., Rendl, M., Schober, M., Pasolli, H. A., Stokes, N., et al. (2009). A two-step mechanism for stem cell activation during hair regeneration. Cell Stem Cell 4 (2), 155–169. doi:10.1016/j.stem.2008.12.009
Haage, A., Wagner, K., Deng, W., Venkatesh, B., Mitchell, C., Goodwin, K., et al. (2020). Precise coordination of cell-ecm adhesion is essential for efficient melanoblast migration during development. Development 147 (14), dev184234. doi:10.1242/dev.184234
Halaban, R., Langdon, R., Birchall, N., Cuono, C., Baird, A., Scott, G., et al. (1988). Basic fibroblast growth factor from human keratinocytes is a natural mitogen for melanocytes. J. Cell Biol. 107 (4), 1611–1619. doi:10.1083/jcb.107.4.1611
Halasi, M., Talmon, A., Tal, Y., Yosipovitch, G., and Adini, I. (2023). Dark pigmentation and related low fmod expression increase il-3 and facilitateplasmacytoid dendritic cell maturation. Clin. Immunol. 251, 109638. doi:10.1016/j.clim.2023.109638
Handoko, H. Y., Rodero, M. P., Boyle, G. M., Ferguson, B., Engwerda, C., Hill, G., et al. (2013). Uvb-induced melanocyte proliferation in neonatal mice driven by ccr2-independent recruitment of ly6c(Low)Mhcii(hi) macrophages. J. Invest. Dermatol 133 (7), 1803–1812. doi:10.1038/jid.2013.9
Hara, M., Toyoda, M., Yaar, M., Bhawan, J., Avila, E. M., Penner, I. R., et al. (1996). Innervation of melanocytes in human skin. J. Exp. Med. 184 (4), 1385–1395. doi:10.1084/jem.184.4.1385
Hari, L., Brault, V., Kleber, M., Lee, H. Y., Ille, F., Leimeroth, R., et al. (2002). Lineage-specific requirements of beta-catenin in neural crest development. J. Cell Biol. 159 (5), 867–880. doi:10.1083/jcb.200209039
Hari, L., Miescher, I., Shakhova, O., Suter, U., Chin, L., Taketo, M., et al. (2012). Temporal control of neural crest lineage generation by Wnt/β-catenin signaling. Development 139 (12), 2107–2117. doi:10.1242/dev.073064
Hedley, S. J., Metcalfe, R., Gawkrodger, D. J., Weetman, A. P., and Mac Neil, S. (1998). Vitiligo melanocytes in long-term culture show normal constitutive and cytokine-induced expression of intercellular adhesion molecule-1 and major histocompatibility complex class I and class ii molecules. Br. J. Dermatol 139 (6), 965–973. doi:10.1046/j.1365-2133.1998.02550.x
Hershey, C. L., and Fisher, D. E. (2005). Genomic analysis of the microphthalmia locus and identification of the mitf-J/mitf-J isoform. Gene 347 (1), 73–82. doi:10.1016/j.gene.2004.12.002
Hou, L., Panthier, J. J., and Arnheiter, H. (2000). Signaling and transcriptional regulation in the neural crest-derived melanocyte lineage: interactions between kit and Mitf. Development 127 (24), 5379–5389. doi:10.1242/dev.127.24.5379
Hou, L., and Pavan, W. J. (2008). Transcriptional and signaling regulation in neural crest stem cell-derived melanocyte development: do all roads lead to Mitf? Cell Res. 18 (12), 1163–1176. doi:10.1038/cr.2008.303
Hu, Q. M., Yi, W. J., Su, M. Y., Jiang, S., Xu, S. Z., and Lei, T. C. (2017). Induction of retinal-dependent calcium influx in human melanocytes by uva or uvb radiation contributes to the stimulation of melanosome transfer. Cell Prolif. 50 (6), e12372. doi:10.1111/cpr.12372
Hu, R., Sharma, S. M., Bronisz, A., Srinivasan, R., Sankar, U., and Ostrowski, M. C. (2007). Eos, MITF, and PU.1 recruit corepressors to osteoclast-specific genes in committed myeloid progenitors. Mol. Cell Biol. 27 (11), 4018–4027. doi:10.1128/MCB.01839-06
Hutcheson, J. D., Schlotter, F., Creager, M. D., Li, X., Pham, T., Vyas, P., et al. (2021). Elastogenesis correlates with pigment production in murine aortic valve leaflets. Front. Cardiovasc Med. 8, 678401. doi:10.3389/fcvm.2021.678401
Ikeda, Y., Wada, A., Hasegawa, T., Yokota, M., Koike, M., and Ikeda, S. (2021). Melanocyte progenitor cells reside in human subcutaneous adipose tissue. PLoS One 16 (8), e0256622. doi:10.1371/journal.pone.0256622
Ikeya, M., Lee, S. M., Johnson, J. E., McMahon, A. P., and Takada, S. (1997). Wnt signalling required for expansion of neural crest and cns progenitors. Nature 389 (6654), 966–970. doi:10.1038/40146
Infarinato, N. R., Stewart, K. S., Yang, Y., Gomez, N. C., Pasolli, H. A., Hidalgo, L., et al. (2020). Bmp signaling: at the gate between activated melanocyte stem cells and differentiation. Genes Dev. 34 (23-24), 1713–1734. doi:10.1101/gad.340281.120
Iozumi, K., Hoganson, G. E., Pennella, R., Everett, M. A., and Fuller, B. B. (1993). Role of tyrosinase as the determinant of pigmentation in cultured human melanocytes. J. Invest. Dermatol 100 (6), 806–811. doi:10.1111/1523-1747.ep12476630
Jordan, S. A., and Jackson, I. J. (2000). Mgf (kit ligand) is a chemokinetic factor for melanoblast migration into hair follicles. Dev. Biol. 225 (2), 424–436. doi:10.1006/dbio.2000.9856
Joshi, S. S., Tandukar, B., Pan, L., Huang, J. M., Livak, F., Smith, B. J., et al. (2019). Cd34 defines melanocyte stem cell subpopulations with distinct regenerative properties. PLoS Genet. 15 (4), e1008034. doi:10.1371/journal.pgen.1008034
Kanitakis, J. (2002). Anatomy, histology and immunohistochemistry of normal human skin. Eur. J. Dermatol 12 (4), 390–399; quiz 400-401.
Kastriti, M. E., Faure, L., Von Ahsen, D., Bouderlique, T. G., Bostrom, J., Solovieva, T., et al. (2022). Schwann cell precursors represent a neural crest-like state with biased multipotency. EMBO J. 41 (17), e108780. doi:10.15252/embj.2021108780
Kaucka, M., Szarowska, B., Kavkova, M., Kastriti, M. E., Kameneva, P., Schmidt, I., et al. (2021). Nerve-associated Schwann cell precursors contribute extracutaneous melanocytes to the heart, inner ear, supraorbital locations and brain meninges. Cell Mol. Life Sci. 78 (16), 6033–6049. doi:10.1007/s00018-021-03885-9
Khakh, B. S., and North, R. A. (2012). Neuromodulation by extracellular atp and P2x receptors in the cns. Neuron 76 (1), 51–69. doi:10.1016/j.neuron.2012.09.024
Kock, A., Schwarz, T., Kirnbauer, R., Urbanski, A., Perry, P., Ansel, J. C., et al. (1990). Human keratinocytes are a source for tumor necrosis factor alpha: evidence for synthesis and release upon stimulation with endotoxin or ultraviolet light. J. Exp. Med. 172 (6), 1609–1614. doi:10.1084/jem.172.6.1609
Koike, S., and Yamasaki, K. (2020). Melanogenesis connection with innate immunity and toll-like receptors. Int. J. Mol. Sci. 21 (24), 9769. doi:10.3390/ijms21249769
Kumano, K., Masuda, S., Sata, M., Saito, T., Lee, S. Y., Sakata-Yanagimoto, M., et al. (2008). Both Notch1 and Notch2 contribute to the regulation of melanocyte homeostasis. Pigment. Cell Melanoma Res. 21 (1), 70–78. doi:10.1111/j.1755-148X.2007.00423.x
Kunisada, T., Yoshida, H., Yamazaki, H., Miyamoto, A., Hemmi, H., Nishimura, E., et al. (1998). Transgene expression of steel factor in the basal layer of epidermis promotes survival, proliferation, differentiation and migration of melanocyte precursors. Development 125 (15), 2915–2923. doi:10.1242/dev.125.15.2915
Lan, Y., Zeng, W., Wang, Y., Dong, X., Shen, X., Gu, Y., et al. (2023). Opsin 3 mediates uva-induced keratinocyte supranuclear melanin cap formation. Commun. Biol. 6 (1), 238. doi:10.1038/s42003-023-04621-8
Laurette, P., Strub, T., Koludrovic, D., Keime, C., Le Gras, S., Seberg, H., et al. (2015). Transcription factor Mitf and remodeller Brg1 define chromatin organisation at regulatory elements in melanoma cells. Elife 4, e06857. doi:10.7554/eLife.06857
Lazarova, R., Hristakieva, E., Lazarov, N., and Shani, J. (2000). Vitiligo-related neuropeptides in nerve fibers of the skin. Arch. Physiol. Biochem. 108 (3), 262–267. doi:10.1076/1381345520000710831zft262
Le, L., Escobar, I. E., Ho, T., Lefkovith, A. J., Latteri, E., Haltaufderhyde, K. D., et al. (2020). Slc45a2 protein stability and regulation of melanosome ph determine melanocyte pigmentation. Mol. Biol. Cell 31 (24), 2687–2702. doi:10.1091/mbc.E20-03-0200
Lee, E. J., Kim, J. Y., Ahn, Y., Lee, B. M., Heo, Y., Hwang, S., et al. (2019). Critical role of atp-P2x7 Axis in uv-induced melanogenesis. J. Invest. Dermatol 139 (7), 1554–1563. doi:10.1016/j.jid.2019.02.031
Le Poole, I. C., van den Wijngaard, R. M., Westerhof, W., Verkruisen, R. P., Dutrieux, R. P., Dingemans, K. P., et al. (1993). Phagocytosis by normal human melanocytes in vitro. Exp. Cell Res. 205 (2), 388–395. doi:10.1006/excr.1993.1102
Levin, M. D., Lu, M. M., Petrenko, N. B., Hawkins, B. J., Gupta, T. H., Lang, D., et al. (2009). Melanocyte-like cells in the heart and pulmonary veins contribute to atrial arrhythmia triggers. J. Clin. Invest. 119 (11), 3420–3436. doi:10.1172/JCI39109
Levy, C., Khaled, M., and Fisher, D. E. (2006). Mitf: master regulator of melanocyte development and melanoma oncogene. Trends Mol. Med. 12 (9), 406–414. doi:10.1016/j.molmed.2006.07.008
Li, A., Ma, Y., Yu, X., Mort, R. L., Lindsay, C. R., Stevenson, D., et al. (2011). Rac1 drives melanoblast organization during mouse development by orchestrating pseudopod- driven motility and cell-cycle progression. Dev. Cell 21 (4), 722–734. doi:10.1016/j.devcel.2011.07.008
Li, L., Fukunaga-Kalabis, M., Yu, H., Xu, X., Kong, J., Lee, J. T., et al. (2010). Human dermal stem cells differentiate into functional epidermal melanocytes. J. Cell Sci. 123 (6), 853–860. doi:10.1242/jcs.061598
Li, M. Y., Flora, P., Pu, H., Bar, C., Silva, J., Cohen, I., et al. (2021). Uv-induced reduction in polycomb repression promotes epidermal pigmentation. Dev. Cell 56 (18), 2547–2561.e8. doi:10.1016/j.devcel.2021.08.006
Liao, C. P., Booker, R. C., Morrison, S. J., and Le, L. Q. (2017). Identification of hair shaft progenitors that create a niche for hair pigmentation. Genes Dev. 31 (8), 744–756. doi:10.1101/gad.298703.117
Lo Cicero, A., Delevoye, C., Gilles-Marsens, F., Loew, D., Dingli, F., Guere, C., et al. (2015). Exosomes released by keratinocytes modulate melanocyte pigmentation. Nat. Commun. 6, 7506. doi:10.1038/ncomms8506
Lu, Y., Zhu, W. Y., Tan, C., Yu, G. H., and Gu, J. X. (2002). Melanocytes are potential immunocompetent cells: evidence from recognition of immunological characteristics of cultured human melanocytes. Pigment. Cell Res. 15 (6), 454–460. doi:10.1034/j.1600-0749.2002.02065.x
Lu, Z., Xie, Y., Huang, H., Jiang, K., Zhou, B., Wang, F., et al. (2020). Hair follicle stem cells regulate retinoid metabolism to maintain the self-renewal niche for melanocyte stem cells. Elife 9, e52712. doi:10.7554/eLife.52712
Lukoseviciute, M., Gavriouchkina, D., Williams, R. M., Hochgreb-Hagele, T., Senanayake, U., Chong-Morrison, V., et al. (2018). From pioneer to repressor: bimodal Foxd3 activity dynamically remodels neural crest regulatory landscape in vivo. Dev. Cell 47 (5), 608–628. doi:10.1016/j.devcel.2018.11.009
Ma, Y., Li, A., Faller, W. J., Libertini, S., Fiorito, F., Gillespie, D. A., et al. (2013). Fascin 1 is transiently expressed in mouse melanoblasts during development and promotes migration and proliferation. Development 140 (10), 2203–2211. doi:10.1242/dev.089789
Mackenzie, M. A., Jordan, S. A., Budd, P. S., and Jackson, I. J. (1997). Activation of the receptor tyrosine kinase kit is required for the proliferation of melanoblasts in the mouse embryo. Dev. Biol. 192 (1), 99–107. doi:10.1006/dbio.1997.8738
McGill, G. G., Horstmann, M., Widlund, H. R., Du, J., Motyckova, G., Nishimura, E. K., et al. (2002). Bcl2 regulation by the melanocyte master regulator Mitf modulates lineage survival and melanoma cell viability. Cell 109 (6), 707–718. doi:10.1016/s0092-8674(02)00762-6
Mica, Y., Lee, G., Chambers, S. M., Tomishima, M. J., and Studer, L. (2013). Modeling neural crest induction, melanocyte specification, and disease-related pigmentation defects in hescs and patient-specific ipscs. Cell Rep. 3 (4), 1140–1152. doi:10.1016/j.celrep.2013.03.025
Michalak-Micka, K., Buchler, V. L., Zapiorkowska-Blumer, N., Biedermann, T., and Klar, A. S. (2022). Characterization of a melanocyte progenitor population in human interfollicular epidermis. Cell Rep. 38 (9), 110419. doi:10.1016/j.celrep.2022.110419
Mildner, M., Mlitz, V., Gruber, F., Wojta, J., and Tschachler, E. (2007). Hepatocyte growth factor establishes autocrine and paracrine feedback loops for the protection of skin cells after uv irradiation. J. Invest. Dermatol 127 (11), 2637–2644. doi:10.1038/sj.jid.5700938
Moreiras, H., Bento-Lopes, L., Neto, M. V., Escrevente, C., Cabaco, L. C., Hall, M. J., et al. (2022). Melanocore uptake by keratinocytes occurs through phagocytosis and involves protease-activated receptor-2 internalization. Traffic 23 (6), 331–345. doi:10.1111/tra.12843
Moriyama, M., Osawa, M., Mak, S. S., Ohtsuka, T., Yamamoto, N., Han, H., et al. (2006). Notch signaling via Hes1 transcription factor maintains survival of melanoblasts and melanocyte stem cells. J. Cell Biol. 173 (3), 333–339. doi:10.1083/jcb.200509084
Mort, R. L., Jackson, I. J., and Patton, E. E. (2015). The melanocyte lineage in development and disease. Development 142 (7), 1387. doi:10.1242/dev.123729
Mort, R. L., Ross, R. J. H., Hainey, K. J., Harrison, O. J., Keighren, M. A., Landini, G., et al. (2016). Reconciling diverse mammalian pigmentation patterns with a fundamental mathematical model. Nat. Commun. 7, 10288. doi:10.1038/ncomms10288
Nakayama, A., Nguyen, M. T., Chen, C. C., Opdecamp, K., Hodgkinson, C. A., and Arnheiter, H. (1998). Mutations in microphthalmia, the mouse homolog of the human deafness gene Mitf, affect neuroepithelial and neural crest-derived melanocytes differently. Mech. Dev. 70 (1-2), 155–166. doi:10.1016/s0925-4773(97)00188-3
Nishikawa, S., Kusakabe, M., Yoshinaga, K., Ogawa, M., Hayashi, S., Kunisada, T., et al. (1991). In utero manipulation of coat color formation by a monoclonal anti-C-kit antibody: two distinct waves of C-Kit-Dependency during melanocyte development. EMBO J. 10 (8), 2111–2118. doi:10.1002/j.1460-2075.1991.tb07744.x
Nishimura, E. K., Granter, S. R., and Fisher, D. E. (2005). Mechanisms of hair graying: incomplete melanocyte stem cell maintenance in the niche. Science 307 (5710), 720–724. doi:10.1126/science.1099593
Nishimura, E. K., Jordan, S. A., Oshima, H., Yoshida, H., Osawa, M., Moriyama, M., et al. (2002). Dominant role of the niche in melanocyte stem-cell fate determination. Nature 416 (6883), 854–860. doi:10.1038/416854a
Nishimura, E. K., Suzuki, M., Igras, V., Du, J., Lonning, S., Miyachi, Y., et al. (2010). Key roles for transforming growth factor beta in melanocyte stem cell maintenance. Cell Stem Cell 6 (2), 130–140. doi:10.1016/j.stem.2009.12.010
Nishimura, E. K., Yoshida, H., Kunisada, T., and Nishikawa, S. I. (1999). Regulation of E- and P-cadherin expression correlated with melanocyte migration and diversification. Dev. Biol. 215 (2), 155–166. doi:10.1006/dbio.1999.9478
Nitzan, E., Pfaltzgraff, E. R., Labosky, P. A., and Kalcheim, C. (2013). Neural crest and Schwann cell progenitor-derived melanocytes are two spatially segregated populations similarly regulated by Foxd3. Proc. Natl. Acad. Sci. U. S. A. 110 (31), 12709–12714. doi:10.1073/pnas.1306287110
Opdecamp, K., Nakayama, A., Nguyen, M. T., Hodgkinson, C. A., Pavan, W. J., and Arnheiter, H. (1997). Melanocyte development in vivo and in neural crest cell cultures: crucial dependence on the Mitf basic-helix-loop-helix-zipper transcription factor. Development 124 (12), 2377–2386. doi:10.1242/dev.124.12.2377
Osawa, M., Egawa, G., Mak, S. S., Moriyama, M., Freter, R., Yonetani, S., et al. (2005). Molecular characterization of melanocyte stem cells in their niche. Development 132 (24), 5589–5599. doi:10.1242/dev.02161
Ostojic, J., Yoon, Y. S., Sonntag, T., Nguyen, B., Vaughan, J. M., Shokhirev, M., et al. (2021). Transcriptional Co-activator regulates melanocyte differentiation and oncogenesis by integrating camp and mapk/erk pathways. Cell Rep. 35 (7), 109136. doi:10.1016/j.celrep.2021.109136
Park, H. Y., Kosmadaki, M., Yaar, M., and Gilchrest, B. A. (2009). Cellular mechanisms regulating human melanogenesis. Cell Mol. Life Sci. 66 (9), 1493–1506. doi:10.1007/s00018-009-8703-8
Paterson, E. K., Fielder, T. J., MacGregor, G. R., Ito, S., Wakamatsu, K., Gillen, D. L., et al. (2015). Tyrosinase depletion prevents the maturation of melanosomes in the mouse hair follicle. PLoS One 10 (11), e0143702. doi:10.1371/journal.pone.0143702
Perugorria, M. J., Olaizola, P., Labiano, I., Esparza-Baquer, A., Marzioni, M., Marin, J. J. G., et al. (2019). Wnt-beta-catenin signalling in liver development, health and disease. Nat. Rev. Gastroenterol. Hepatol. 16 (2), 121–136. doi:10.1038/s41575-018-0075-9
Petit, V., and Larue, L. (2016). Any route for melanoblasts to colonize the skin. Exp. Dermatol 25 (9), 669–673. doi:10.1111/exd.13061
Pfefferkorn, C. M., McGlinchey, R. P., and Lee, J. C. (2010). Effects of ph on aggregation kinetics of the repeat domain of a functional amyloid, Pmel17. Proc. Natl. Acad. Sci. U. S. A. 107 (50), 21447–21452. doi:10.1073/pnas.1006424107
Plonka, P. M., Passeron, T., Brenner, M., Tobin, D. J., Shibahara, S., Thomas, A., et al. (2009). What are melanocytes really doing all day long. Exp. Dermatol 18 (9), 799–819. doi:10.1111/j.1600-0625.2009.00912.x
Polisetti, N., Giessl, A., Zenkel, M., Heger, L., Dudziak, D., Naschberger, E., et al. (2021). Melanocytes as emerging key players in niche regulation of limbal epithelial stem cells. Ocul. Surf. 22, 172–189. doi:10.1016/j.jtos.2021.08.006
Qi, X., Hong, J., Chaves, L., Zhuang, Y., Chen, Y., Wang, D., et al. (2013). Antagonistic regulation by the transcription factors C/EBPα and MITF specifies basophil and mast cell fates. Immunity 39 (1), 97–110. doi:10.1016/j.immuni.2013.06.012
Rabbani, P., Takeo, M., Chou, W., Myung, P., Bosenberg, M., Chin, L., et al. (2011). Coordinated activation of wnt in epithelial and melanocyte stem cells initiates pigmented hair regeneration. Cell 145 (6), 941–955. doi:10.1016/j.cell.2011.05.004
Raposo, G., and Marks, M. S. (2007). Melanosomes--Dark organelles enlighten endosomal membrane transport. Nat. Rev. Mol. Cell Biol. 8 (10), 786–797. doi:10.1038/nrm2258
Sakurai, T., Yanagisawa, M., Takuwa, Y., Miyazaki, H., Kimura, S., Goto, K., et al. (1990). Cloning of a cdna encoding a non-isopeptide-selective subtype of the endothelin receptor. Nature 348 (6303), 732–735. doi:10.1038/348732a0
Saldana-Caboverde, A., and Kos, L. (2010). Roles of endothelin signaling in melanocyte development and melanoma. Pigment. Cell Melanoma Res. 23 (2), 160–170. doi:10.1111/j.1755-148X.2010.00678.x
Schepsky, A., Bruser, K., Gunnarsson, G. J., Goodall, J., Hallsson, J. H., Goding, C. R., et al. (2006). The microphthalmia-associated transcription factor Mitf interacts with beta-catenin to determine target gene expression. Mol. Cell Biol. 26 (23), 8914–8927. doi:10.1128/MCB.02299-05
Sharov, A., Tobin, D. J., Sharova, T. Y., Atoyan, R., and Botchkarev, V. A. (2005). Changes in different melanocyte populations during hair follicle involution (catagen). J. Invest. Dermatol 125 (6), 1259–1267. doi:10.1111/j.0022-202X.2005.23959.x
Singh, S. K., Baker, R., Sikkink, S. K., Nizard, C., Schnebert, S., Kurfurst, R., et al. (2017). E-cadherin mediates ultraviolet radiation- and calcium-induced melanin transfer in human skin cells. Exp. Dermatol 26 (11), 1125–1133. doi:10.1111/exd.13395
Slominski, A., and Paus, R. (1993). Melanogenesis is coupled to murine anagen: toward new concepts for the role of melanocytes and the regulation of melanogenesis in hair growth. J. Invest. Dermatol 101 (1), 90S–97S. doi:10.1111/1523-1747.ep12362991
Soldatov, R., Kaucka, M., Kastriti, M. E., Petersen, J., Chontorotzea, T., Englmaier, L., et al. (2019). Spatiotemporal structure of cell fate decisions in murine neural crest. Science 364 (6444), eaas9536. doi:10.1126/science.aas9536
Sommer, L., Ma, Q., and Anderson, D. J. (1996). Neurogenins, a novel family of atonal-related bhlh transcription factors, are putative mammalian neuronal determination genes that reveal progenitor cell heterogeneity in the developing cns and pns. Mol. Cell Neurosci. 8 (4), 221–241. doi:10.1006/mcne.1996.0060
Sormani, L., Montaudie, H., Blot, L., Heim, M., Cardot Leccia, N., Mhaidly, R., et al. (2022). Clec12b is a melanocytic gene regulating the color of the skin. J. Invest. Dermatol 142 (7), 1858–1868.e8. doi:10.1016/j.jid.2021.08.450
Starner, R. J., McClelland, L., Abdel-Malek, Z., Fricke, A., and Scott, G. (2010). Pge(2) is a uvr-inducible autocrine factor for human melanocytes that stimulates tyrosinase activation. Exp. Dermatol 19 (7), 682–684. doi:10.1111/j.1600-0625.2010.01074.x
Stefanato, C. M., Yaar, M., Bhawan, J., Phillips, T. J., Kosmadaki, M. G., Botchkarev, V., et al. (2003). Modulations of nerve growth factor and bcl-2 in ultraviolet-irradiated human epidermis. J. Cutan. Pathol. 30 (6), 351–357. doi:10.1034/j.1600-0560.2003.00065.x
Steingrimsson, E., Copeland, N. G., and Jenkins, N. A. (2004). Melanocytes and the microphthalmia transcription factor network. Annu. Rev. Genet. 38, 365–411. doi:10.1146/annurev.genet.38.072902.092717
Sun, Q., Lee, W., Hu, H., Ogawa, T., De Leon, S., Katehis, I., et al. (2023). Dedifferentiation maintains melanocyte stem cells in a dynamic niche. Nature 616 (7958), 774–782. doi:10.1038/s41586-023-05960-6
Sun, Q., Lee, W., Mohri, Y., Takeo, M., Lim, C. H., Xu, X., et al. (2019). A novel mouse model demonstrates that oncogenic melanocyte stem cells engender melanoma resembling human disease. Nat. Commun. 10 (1), 5023. doi:10.1038/s41467-019-12733-1
Sun, Q., Rabbani, P., Takeo, M., Lee, S. H., Lim, C. H., Noel, E. S., et al. (2018). Dissecting wnt signaling for melanocyte regulation during wound healing. J. Invest. Dermatol 138 (7), 1591–1600. doi:10.1016/j.jid.2018.01.030
Swope, V. B., Abdel-Malek, Z., Kassem, L. M., and Nordlund, J. J. (1991). Interleukins 1 alpha and 6 and tumor necrosis factor-alpha are paracrine inhibitors of human melanocyte proliferation and melanogenesis. J. Invest. Dermatol 96 (2), 180–185. doi:10.1111/1523-1747.ep12460991
Tabata, H., Kawamura, N., Sun-Wada, G. H., and Wada, Y. (2008). Vacuolar-type H(+)-Atpase with the A3 isoform is the proton pump on premature melanosomes. Cell Tissue Res. 332 (3), 447–460. doi:10.1007/s00441-008-0597-5
Tadokoro, R., and Takahashi, Y. (2017). Intercellular transfer of organelles during body pigmentation. Curr. Opin. Genet. Dev. 45, 132–138. doi:10.1016/j.gde.2017.05.001
Takeo, M., Lee, W., Rabbani, P., Sun, Q., Hu, H., Lim, C. H., et al. (2016). Ednrb governs regenerative response of melanocyte stem cells by crosstalk with wnt signaling. Cell Rep. 15 (6), 1291–1302. doi:10.1016/j.celrep.2016.04.006
Tanimura, S., Tadokoro, Y., Inomata, K., Binh, N. T., Nishie, W., Yamazaki, S., et al. (2011). Hair follicle stem cells provide a functional niche for melanocyte stem cells. Cell Stem Cell 8 (2), 177–187. doi:10.1016/j.stem.2010.11.029
Thomas, A. J., and Erickson, C. A. (2009). Foxd3 regulates the lineage switch between neural crest-derived glial cells and pigment cells by repressing Mitf through a non-canonical mechanism. Development 136 (11), 1849–1858. doi:10.1242/dev.031989
Tian, X., Cui, Z., Liu, S., Zhou, J., and Cui, R. (2021). Melanosome transport and regulation in development and disease. Pharmacol. Ther. 219, 107707. doi:10.1016/j.pharmthera.2020.107707
Toyoda, M., Luo, Y., Makino, T., Matsui, C., and Morohashi, M. (1999). Calcitonin gene-related peptide upregulates melanogenesis and enhances melanocyte dendricity via induction of keratinocyte-derived melanotrophic factors. J. Investig. Dermatol Symp. Proc. 4 (2), 116–125. doi:10.1038/sj.jidsp.5640194
Upadhyay, P. R., Ho, T., and Abdel-Malek, Z. A. (2021). Participation of keratinocyte- and fibroblast-derived factors in melanocyte homeostasis, the response to uv, and pigmentary disorders. Pigment. Cell Melanoma Res. 34 (4), 762–776. doi:10.1111/pcmr.12985
Valluet, A., Druillennec, S., Barbotin, C., Dorard, C., Monsoro-Burq, A. H., Larcher, M., et al. (2012). B-raf and C-raf are required for melanocyte stem cell self-maintenance. Cell Rep. 2 (4), 774–780. doi:10.1016/j.celrep.2012.08.020
van Beelen, E. S. A., van der Valk, W. H., de Groot, J., Hensen, E. F., Locher, H., and van Benthem, P. P. G. (2020). Migration and fate of vestibular melanocytes during the development of the human inner ear. Dev. Neurobiol. 80 (11-12), 411–432. doi:10.1002/dneu.22786
Wang, S., Liu, D., Ning, W., and Xu, A. (2015). Cytosolic dsdna triggers apoptosis and pro-inflammatory cytokine production in normal human melanocytes. Exp. Dermatol 24 (4), 298–300. doi:10.1111/exd.12621
Weston, J. A. (1991). Sequential segregation and fate of developmentally restricted intermediate cell populations in the neural crest lineage. Curr. Top. Dev. Biol. 25, 133–153. doi:10.1016/s0070-2153(08)60414-7
Wiriyasermkul, P., Moriyama, S., and Nagamori, S. (2020). Membrane transport proteins in melanosomes: regulation of ions for pigmentation. Biochim. Biophys. Acta Biomembr. 1862 (12), 183318. doi:10.1016/j.bbamem.2020.183318
Woodham, E. F., Paul, N. R., Tyrrell, B., Spence, H. J., Swaminathan, K., Scribner, M. R., et al. (2017). Coordination by Cdc42 of actin, contractility, and adhesion for melanoblast movement in mouse skin. Curr. Biol. 27 (5), 624–637. doi:10.1016/j.cub.2017.01.033
Wu, X., and Hammer, J. A. (2014). Melanosome transfer: it is best to give and receive. Curr. Opin. Cell Biol. 29, 1–7. doi:10.1016/j.ceb.2014.02.003
Yaar, M., Grossman, K., Eller, M., and Gilchrest, B. A. (1991). Evidence for nerve growth factor-mediated paracrine effects in human epidermis. J. Cell Biol. 115 (3), 821–828. doi:10.1083/jcb.115.3.821
Yamada, T., Hasegawa, S., Inoue, Y., Date, Y., Yamamoto, N., Mizutani, H., et al. (2013). Wnt/β-catenin and kit signaling sequentially regulate melanocyte stem cell differentiation in UVB-induced epidermal pigmentation. J. Invest. Dermatol 133 (12), 2753–2762. doi:10.1038/jid.2013.235
Yoo, H., Lee, H. R., Kim, K. H., Kim, M. A., Bang, S., Kang, Y. H., et al. (2021). Crtc3, a sensor and key regulator for melanogenesis, as a tunable therapeutic target for pigmentary disorders. Theranostics 11 (20), 9918–9936. doi:10.7150/thno.66378
Yu, H., Lin, X., Cen, J., Cheng, H., and Seifert, O. (2021). Increased expression of toll-like receptor 7 and 9 in vitiligo melanocytes: a pilot study. Clin. Exp. Dermatol 46 (1), 89–95. doi:10.1111/ced.14374
Yu, N., Zhang, S., Zuo, F., Kang, K., Guan, M., and Xiang, L. (2009). Cultured human melanocytes express functional toll-like receptors 2-4, 7 and 9. J. Dermatol Sci. 56 (2), 113–120. doi:10.1016/j.jdermsci.2009.08.003
Zaidi, M. R., Davis, S., Noonan, F. P., Graff-Cherry, C., Hawley, T. S., Walker, R. L., et al. (2011). Interferon-gamma links ultraviolet radiation to melanomagenesis in mice. Nature 469 (7331), 548–553. doi:10.1038/nature09666
Zhang, B., Ma, S., Rachmin, I., He, M., Baral, P., Choi, S., et al. (2020). Hyperactivation of sympathetic nerves drives depletion of melanocyte stem cells. Nature 577 (7792), 676–681. doi:10.1038/s41586-020-1935-3
Zhang, W., Dai, M., Fridberger, A., Hassan, A., Degagne, J., Neng, L., et al. (2012). Perivascular-resident macrophage-like melanocytes in the inner ear are essential for the integrity of the intrastrial fluid-blood barrier. Proc. Natl. Acad. Sci. U. S. A. 109 (26), 10388–10393. doi:10.1073/pnas.1205210109
Keywords: melanocytes, pigmentation, melanin synthesis, melanocyte stem cells, melanoblasts, neural crest cells, Wnt signaling, epidermal keratinocytes
Citation: Cui Y-Z and Man X-Y (2023) Biology of melanocytes in mammals. Front. Cell Dev. Biol. 11:1309557. doi: 10.3389/fcell.2023.1309557
Received: 11 October 2023; Accepted: 10 November 2023;
Published: 22 November 2023.
Edited by:
Yoshiko Takahashi, Kyoto University, JapanCopyright © 2023 Cui and Man. This is an open-access article distributed under the terms of the Creative Commons Attribution License (CC BY). The use, distribution or reproduction in other forums is permitted, provided the original author(s) and the copyright owner(s) are credited and that the original publication in this journal is cited, in accordance with accepted academic practice. No use, distribution or reproduction is permitted which does not comply with these terms.
*Correspondence: Xiao-Yong Man, bWFueHlAemp1LmVkdS5jbg==
Disclaimer: All claims expressed in this article are solely those of the authors and do not necessarily represent those of their affiliated organizations, or those of the publisher, the editors and the reviewers. Any product that may be evaluated in this article or claim that may be made by its manufacturer is not guaranteed or endorsed by the publisher.
Research integrity at Frontiers
Learn more about the work of our research integrity team to safeguard the quality of each article we publish.