- 1Department of Biomedical Engineering, New Jersey Institute of Technology, Newark, NJ, United States
- 2Chemical and Materials Engineering, New Jersey Institute of Technology, Newark, NJ, United States
- 3Department of Bioengineering, University of Pennsylvania, Philadelphia, PA, United States
- 4Department of Chemical and Biomolecular Engineering, University of Pennsylvania, Philadelphia, PA, United States
- 5Department of Medicine, University of California San Diego, La Jolla, CA, United States
- 6Carisma Therapeutics, Philadelphia, PA, United States
Leukocytes possess the ability to migrate upstream—against the direction of flow—on surfaces of specific chemistry. Upstream migration was first characterized in vitro for T-cells on surfaces comprised of intracellular adhesion molecule-1 (ICAM-1). Upstream migration occurs when the integrin receptor αLβ2 (also known as lymphocyte function-associated antigen-1, or LFA-1) binds to ICAM-1. LFA-1/ICAM-1 interactions are ubiquitous and are widely found in leukocyte trafficking. Upstream migration would be employed after cells come to arrest on the apical surface of the endothelium and might confer an advantage for both trans-endothelial migration and tissue surveillance. It has now been shown that several other motile amoeboid cells which have the responsibility of trafficking from blood vessels into tissues, such as Marginal zone B cells, hematopoietic stem cells, and neutrophils (when macrophage-1 antigen, Mac-1, is blocked), can also migrate upstream on ICAM-1 surfaces. This review will summarize what is known about the basic mechanisms of upstream migration, which cells have displayed this phenomenon, and the possible role of upstream migration in physiology and tissue homeostasis.
Introduction
Leukocytes must traffic in and out of tissues to carry out numerous immune and effector functions. Blood borne cells, carried in a hydrodynamic flow, interact with the vascular endothelium through a multi-step process known as the leukocyte adhesion cascade (Ley et al., 2007). Leukocytes first tether and roll on the apical surface of endothelium, facilitated by selectins. Then integrins on leukocytes are activated through conformational changes and strongly bind to cognate ligands. Selectins and integrins act in synergy to facilitate leukocyte capture (Bhatia et al., 2003; Eniola et al., 2003). Leukocytes will spread and migrate on the apical surface of the endothelium to find junctions for transendothelial migration into tissues. The expression of leukocyte receptors depends on the leukocyte. For example, T-cells bear the integrin αLβ2, also known as lymphocyte function-associated antigen-1 (LFA-1), which binds intracellular adhesion molecule-1(ICAM-1), as well as α4β1, or very late antigen-4 (VLA-4), which binds vascular cell adhesion molecule-1 (VCAM-1) (López-Hoyos et al., 1999). Neutrophils bear two beta-2 integrins—LFA-1 and macrophage-1 antigen (Mac-1, αMβ2), which also binds to ICAM-1 (Heit et al., 2005). These integrin-ligand interactions dictate the dynamics and location of firm adhesion as well as migration of leukocytes in the vascular endothelium.
About a decade ago, Theodoly and colleagues pointed out that the migration of leukocytes on the apical endothelium occurs under a substantial shear flow (Valignat et al., 2013). In post capillary venules, adhesion and migration occur under shear stresses between 4 and 60 dynes/cm2 (Bhatia et al., 2003). Motivated by this observation, as well as the idea that leukocyte activity is modulated by shear stress and that lymphocytes had been observed migrating upstream in the vascular lumen during a murine model of autoimmune encephalitis (Bartholomaus et al., 2009), Theodoly et al. performed in vitro experiments on the migration of several types of leukocytes, including primary (naïve) T-cells, effector (activated) CD4+ T-cells, neutrophils, and a leukemic cell line (HSB2) on ICAM-1 surfaces under flow. Surfaces were prepared with a physisorbed ICAM-1-Fc in combination with the chemokine SDF-1a. Curiously, both naïve and effector T-cells migrated against the direction of flow on ICAM-1 surfaces, whereas neutrophils and HSB2 cells migrated downstream in the direction of flow (Figure 1).
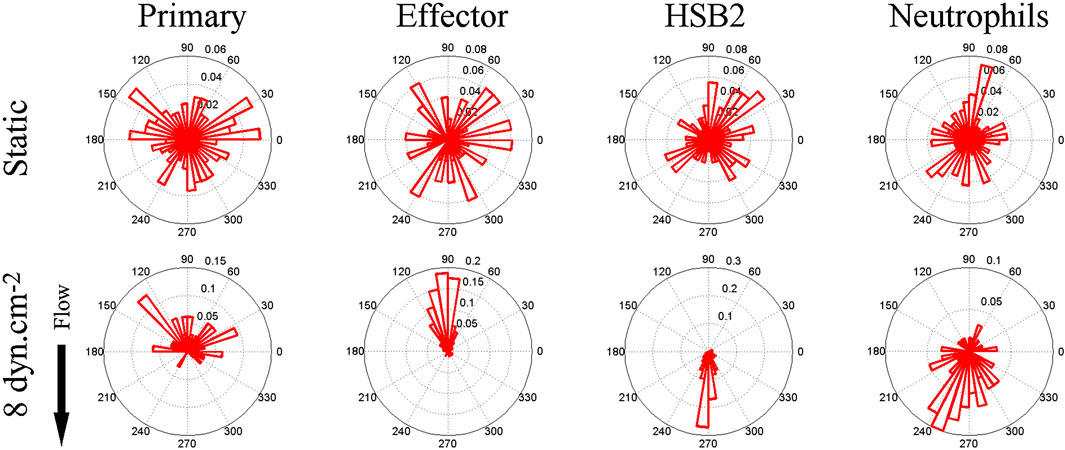
FIGURE 1. Angle histogram for one representative experiment in four different leukocyte cell populations (primary T cell, effector T cells, HSB2 T cells, and neutrophils) in static (upper panels) and flow (lower panels) conditions showing the distribution of θ in ≤40 angle bins. The length of each bin reflects the fraction of cells with a given angle that fall within a group. Under the flow condition, the shear stress was set to a value of 8 dyn cm−2 and the direction of the flow was along the Y-axis from top to bottom, as indicated by the arrow. The numbers of cells analyzed were respectively 192, 234, 148, and 134 in the static condition and 23, 73, 93, and 60 under the flow condition for primary T cells, effector T cells, HSB2 T cells, and neutrophils. Reproduced from Valignat et al., Biophys J, 2013 January 22; 104(2):322-31.
There were several remarkable features of the upstream migration of T-cells. First, in the absence of flow, T-cells migrated randomly, but upon initiation of flow, T-cells rapidly oriented in the direction of flow (within 30 s) (Valignat et al., 2013). Second, effector T-cells oriented more avidly upstream than naïve T-cells (Valignat et al., 2013). Third, T-cells oriented upstream with greater fidelity when the shear stress increased (up to 60 dynes/cm2) (Valignat et al., 2013). And finally, HSB2 cells did not migrate upstream, despite having the same receptor profile as T-cells, and neutrophils also did not migrate upstream (Valignat et al., 2013). At the time, these results suggested that upstream migration is a phenomenon restricted to T-cells, especially effector T-cells. Ultimately, it would be shown that upstream migration is a much more pervasive mode of cell motility.
Essential requirement for LFA-1 for upstream migration
Upstream migration is a fascinating phenomenon in its own right. Valignat and coworkers estimated the applied force on a migrating T-cell to be 0.6 nN at a shear stress of 60 dynes/cm2 (Valignat et al., 2013). Thus, T-cells must both withstand this force and generate a traction that can propel the T-cell against the direction of flow. The question is, what are the molecular origin and structural requirements for upstream migration?
Theodoly and coworkers advanced the idea that on ICAM-1 surfaces, T-cells take on a structure that supports upstream migration (Valignat et al., 2014). Roy et al. had observed that T cells on ICAM-1 surfaces have a broad, actin-rich lamellipod, whereas cells on VCAM-1 surfaces have a less pronounced lamellipod and reduced actin polymerization in the leading edge (Roy et al., 2020). 3D scanning microscopy showed that on ICAM-1 surfaces, T-cells have an adherent lamellipod but a detached uropod that vertically extends upward. It was suggested that this uropodial tail acts to passively steer the T-cell, like a “wind vane” (Valignat et al., 2014). This mechanism suggests the effect of receptor-ligand interactions is to maintain cell structure, without the need for internal signaling triggered by flow.
Upstream migration is supported only by engagement of ICAM-1 by LFA-1. Previous work has shown that LFA-1/ICAM-1 forms catch bonds—bonds that dissociate less well when a force is applied (Dembo et al., 1988; Zhang et al., 2002; Wojcikiewicz et al., 2006). It is appealing to think that upon the application of a shear force to the cell, the LFA-1/ICAM-1 bonds in the lamellipod would hold on tighter and pin the lamellipod. However, propulsive migration would also require extension and polymerization of the lamellipodial front, which might be facilitated by outside-in signaling of integrins. β2 integrins are known to signal both ERK and phosphoinositide-3 kinase (PI3K) pathways (Roy et al., 2020), so it seems plausible that LFA-1 could simultaneously act as a catch bond and promote actin polymerization through outside-in signaling. This hypothesis fits with the observation reported by Roy et al that T cells migrating on ICAM-1 coated surfaces had a clear lamellipodial structure with robust actin polymerization. (Roy et al., 2018; Roy et al., 2020). Roy and coworkers had previously shown that an adaptor protein, Crk, was a key intermediate downstream of LFA-1 and facilitated the response of T cells to substrate stiffness, another mechanosensitive response (Roy et al., 2018). In a study led by Janis Burkhardt’s laboratory at the Children’s Hospital of Philadelphia, it was found that Crk as well as the ubiquitin ligase c-Cbl, downstream of LFA-1 but before PI3K (Figure 2A), were critical for promoted upstream migration. Activated murine T-cells from Crk double knock-out (DKO) mice reversed the direction of migration under hydrodynamic flow on ICAM-1 surfaces, without affecting actin polymerization and formation of a lamellipod (Figure 2) (Roy et al., 2020). Complementary experiments involving CRISPR Cas9 editing of T-cells from a Cas9 mouse showed that deletion of c-Cbl also reversed the direction of T-cell migration on ICAM-1 (Roy et al., 2020). Interestingly, PI3K itself—either in response to CRISPR deletion or pharmacological inhibition (Dominguez et al., 2015; Roy et al., 2020) - did not affect upstream migration, which suggests there are other key molecules that are affected by Crk and c-Cbl. Further research is needed to identify other molecular components responsible for upstream migration, perhaps by a large scale CRIPSR screen which has been successfully pursued to identify mediators of motility in HL-60 and T-cells (Belliveau et al., 2022; Johansen et al., 2022).
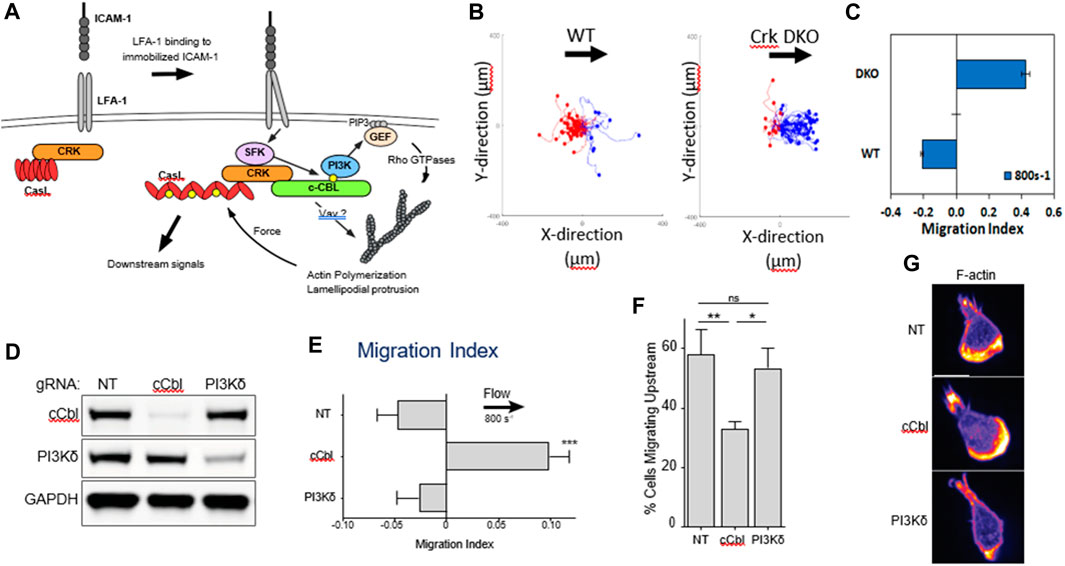
FIGURE 2. (A) Biochemical signals downstream of LFA-1 after engagement with ICAM-1. (B) Flow histograms and (C) Migration Indices of WT and Crk DKO mouse CD4+ T cells migrating on ICAM-1 surface at 800s-1 shear rate. (D) CD4+ T cells from Cas9-expressing mice were transduced with the indicated gRNAs, selected for 3 d in puromycin, and then lysed and immunoblotted for the indicated proteins. NT, non-targeting gRNA control. GAPDH was used as a loading control. (E) Migration index of T cells expressing the indicated gRNAs migrating on ICAM-1 under shear flow (shear rate 800 s−1). (F) Percentage of cells migrating upstream from experiments shown in panel (E) (G) Cells expressing the indicated gRNAs were allowed to migrate on ICAM-1-coated surfaces, fixed, and stained with fluorescent phalloidin. Representative images, Scale bar: 10 μm. Adapted from Roy et al., J Cell Sci, 2020 September 9; 133(17):jcs248328.
Crosstalk among integrins
Two T-cell ligands are expressed on the apical surface of endothelium, ICAM-1 and VCAM-1; these are recognized by the integrins LFA-1 and VLA-4, respectively. Theodoly and colleagues had shown that on ICAM-1 surfaces, T-cell displayed upstream migration only when flow was initiated; otherwise, T-cells moved at random (Valignat et al., 2013). Another study furthered this observation by demonstrating that while murine T cells crawled upstream on ICAM-1, they crawled downstream on ICAM-2 and VCAM-1 surfaces on the Blood Brain Barrier (BBB) Microvasculature. (Steiner et al., 2010). Interestingly, while ICAM-2 did not support upstream migration on murine BBB microvasculature cells, T cells did crawl upstream on both purified ICAM-1 and ICAM-2 FC substrates (Steiner et al., 2010) leading to whether the specific biology of the BBB microvasculature inhibited ICAM-2 mediated upstream migration.
To address the role of VCAM-1 on upstream migration, our laboratory made surfaces by coating protein A/G and mixtures of soluble ICAM-1 and VCAM-1 Fc chimeras to alter the ratio of ICAM-1 to VCAM-1. We found that only a small amount of ICAM-1 (10% of all surface ligands) was necessary to support upstream migration in T-cells (Dominguez et al., 2015). By patterning different densities of adhesive ligands in a gradient, Xuo and coworkers showed that T-cells display a preference in adhesiveness depending on the ligand under static conditions (Luo et al., 2020). T-cells display reverse haptotaxis on ICAM-1, crawling towards lower densities of ICAM-1, and haptotaxis towards higher densities of VCAM-1. While the reverse haptotaxis of T cells on ICAM-1 is an interesting biophysical phenomenon, it may not be physiologically relevant as under shear flow conditions ICAM-1 localizes at higher concentrations upstream of the flow direction on endothelial cells (Piechocka et al., 2021). Coupled with the demonstrated propensity of T-cells to migrate upstream on ICAM-1 surfaces, the propensity of T cells to perform reverse haptotaxis on ICAM-1 may be overridden by the signals generated when exposed to shear flow on the vascular endothelium.
In addition, our laboratory did a series of experiments that illustrated that the signals from both integrins are integrated complexly. First, we showed that on surfaces in which the two ligands ICAM-1 and VCAM-1 are mixed 50/50, activated CD4+ T cells retained persistence and continued to migrate in the upstream direction, even when the flow was stopped, which we describe this effect as “migrational memory”. In contrast, T cells possessed no memory of upstream migration on 100% ICAM-1 surfaces when the flow was stopped. These results led our laboratory to do an extensive study of the mechanisms of migrational memory (Kim and Hammer, 2019). Starting with the result that activated CD4+ T-cells on 50/50 ICAM-1/VCAM-1 surfaces migrated upstream for at least 30 min after shear flow of 8 dynes/cm2 was stopped, we used pharmacological inhibitors to show that migrational memory required PI3K, but not the GTPase cdc42 and or the actin branching molecule Arp2/3 (Kim and Hammer, 2019). We showed that while LFA-1 is essential for upstream migration, VLA-4 is essential for the migrational memory. Using a blocking antibody against VLA-4, we also blocked migrational memory in T cells after migrating upstream on VCAM-1/ICAM-1 polyacrylamide gels, independent of surface stiffness and dependent only on the shear flow rate (Kim and Hammer, 2021). On surfaces that lacked VCAM-1, we could promote migrational memory on ICAM-1 surfaces using soluble VCAM-1 to ligate VLA-4 (Kim and Hammer, 2019; Kim and Hammer, 2021). This study implies that the simultaneous activation of LFA-1 and VLA-4 integrins could also propagate actin reorganization to support the upstream migration of T cells. While LFA-1 alone is sufficient to promote upstream migration, we have shown that VLA-4 and LFA-1 together are essential for directed motion of CD4+ T lymphocytes post flow. So, while the upstream direction is determined by LFA-1, the persistence of the upstream direction is maintained by simultaneous VLA-4 activation and consequent PI3K signaling generated from the crosstalk of the two integrins in CD4+ T cells.
Hornung et al. further investigated the crosstalk of LFA-1 and VLA-4 by utilizing pure ICAM-1, VCAM-1, and molar mixtures of the two on the surface to characterize the migration profiles of T cells (Hornung et al., 2020). They reported that LFA-1-ICAM-1 interactions imposed strong adhesion and upstream migration while VLA-4-VCAM-1 interactions led to transient interactions and downstream migration. Furthermore, they demonstrated that cells attached at the front and were detached at the rear (i.e., lamellipods and uropods, respectively) when migrating upstream, while the opposite (front-detached and rear-adhered) was true during downstream migration (Figure 3) (Hornung et al., 2020). Subsequent studies revealed that active VLA-4 was localized towards the rear of the cell while active LFA-1 populated towards the front (Robert et al., 2021).
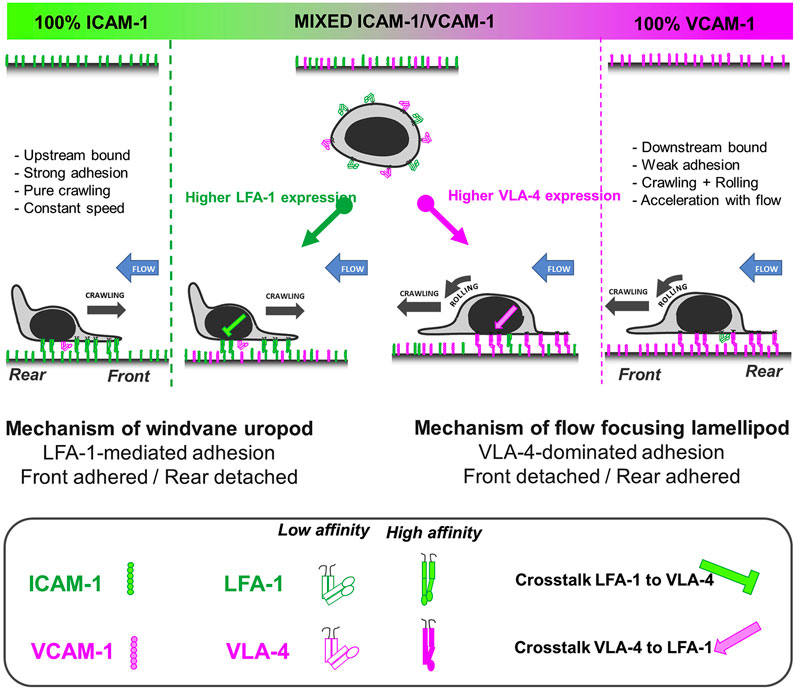
FIGURE 3. A bistable mechanism of cell adhesion spatial regulation explains integrin control of T cell flow mechanotaxis. On pure substrates of ICAM-1 or VCAM-1, the T cell population has homogeneous phenotypes with an opposite orientation on ICAM-1 and VCAM-1. On mixed substrates of ICAM-1 or VCAM-1, T cells distribute in two populations with opposite orientations and characteristics similar to phenotypes on pure substrates. Decisions of orientation on mixed substrates are controlled by the expression level of integrins LFA-1 and VLA-4 via a bistable polarization of cell adhesion; a higher LFA-1 expression leads to a LFA-1-dominated adhesion of cell front (very similar to upstream crawling cells on ICAM-1), whereas a higher expression of VLA-4 leads to adhesion of cell rear and center (very similar to downstream crawling cells on VCAM-1). Inhibiting cross talk of LFA-1 toward VLA-4 reinforces adhesion polarization toward cell front, which favors wind vane mechanism and upstream phenotype. Activating cross talk of VLA-4 toward LFA-1 reinforces the adhesion of cell uropod, which hampers the wind vane mechanism and favors the downstream phenotype. Adapted from Hornung et al., Biophys J, 2020 February 4; 118(3):565-577.
Upstream migration observed in other cell types
The phenomenon of T cells migrating against the direction of shear flow from a fundamental biophysical standpoint is exciting but given the pervasiveness of LFA-1/ICAM-1 interactions, one might question whether other immune cell types might exhibit upstream migration. The following sections summarize the evidence that immune cell types, other than T cells, can migrate upstream against the blood flow, either in vasculature or also in organs and tissues under interstitial flow.
Hematopoietic Stem and Progenitor Cells: Hematopoietic Stem and Progenitor cells (HSPCs) are a heterogeneous mixture of long and short term hematopoietic stem cells (HSCs) and multipotent progenitors (MPPs) of varying degrees of differentiation, defined by the expression of CD34 (Carvalho et al., 2009). Residing in the bone marrow, HSPCs can differentiate into all mature blood cells of both the myeloid and lymphoid origins (Bujko et al., 2019). HSPCs are also found in circulation and able to crawl along the endothelial surface to traffic back to the bone marrow niche (Mazo et al., 1998). The homing and proper trafficking of HSPCs to the bone marrow is considered to be required during HSPC transplantation to restart homeostasis after an ablative chemotherapy (Appelbaum, 2007).
Similar to T cells, HSPCs also express LFA-1 (αLβ2) and VLA-4 (α4β1) in sufficient levels to confer binding to ICAM-1 and VCAM-1 surfaces (Buffone et al., 2018), which implies potential for HSPCs to also migrate upstream. Like T cells, the HSPCs and KG1a cells we tested did not express Mac-1 (αMβ2), which competes with LFA-1 for ICAM-1 binding. To explore the upstream migration of HSPCs, we decided to use KG1a cell line, an immortalized human cell line widely used as a model for CD34+ HSPCs (AbuSamra et al., 2017), to investigate the upstream migration profile in HSPCs. Indeed, KG1a cells migrated upstream on recombinant ICAM-1 surfaces and downstream on VCAM-1 surfaces (Buffone et al., 2018), similar to the of behavior T cells. Further experiments demonstrated that upstream migration required LFA-1-ICAM-1 interactions and was observed on both mixed surfaces of ICAM-1 and VCAM-1, and monolayers of stimulated human umbilical vein endothelial cells (HUVECs) (Buffone et al., 2018). Finally, primary CD34+ HSPCs isolated from bone marrow were confirmed to have similar integrin expression profiles to CD4+ T cells and KG1a cells and exhibited upstream migration on ICAM-1, mixed ICAM-1 + VCAM-1 surfaces, and HUVEC monolayers. This study highlights that the upstream migration is not limited to T cells and can be extended to HSPCs (Buffone et al., 2018). Studying upstream migration in HSPCs may be used to improve the speed of HSPCs homing to the bone marrow and restarting hematopoiesis after transplantation, especially since the T cells have been shown transmigrate twice as fast migrating upstream than downstream (Anderson et al., 2019).
Marginal Zone B cells: Marginal zone B cells (MZBs), another lymphoid cell type that surveys blood from the circulation as it enters the spleen for antigens (Lopes-Carvalho et al., 2005), were demonstrated to migrate against the direction of shear flow (Tedford et al., 2017). Shear flow in the spleen runs from the follicle, through the marginal zone, and finally into the red pulp. The direction of flow within the spleen requires MZBs to migrate upstream from the marginal zone to deliver antigens to the follicle (Mebius and Kraal, 2005; Cerutti et al., 2013). Splenic endothelial cells express both ICAM-1 and VCAM-1 (Ulyanova et al., 2005), and the shear flow acts as a mechanotransducer to activate both LFA-1 and VLA-4 on the MZBs. MZBs crawl upstream on ICAM-1 and mixed ICAM-1/VCAM-1 surfaces and downstream on VCAM-1 surfaces (Tedford et al., 2017; Tedford et al., 2018). The expression of CXCL13 and Mucosal Vascular Addressin Cellular Adhesion Molecule (MAdCAM-1) in blood vessels retards the upstream migration. Upstream crawling is completely lost when signaling through sphingosine-1-phosphate (S1P) is ablated in knockout mice (Tedford et al., 2017). Interestingly, once the MZBs reach the follicle and become follicular B-cells (FOBs), they lose the ability to crawl upstream (Tedford et al., 2017). These results demonstrate a critical biological function of upstream migration as MZBs must utilize this mode of motility to properly deliver antigen to the follicle.
Neutrophils: While identifying that CD4+ T cells were able to migrate against the direction of shear flow (Valignat et al., 2013), Theodoly and coworkers also interrogated the directional preference of primary human neutrophils. They showed that neutrophils were unable to migrate upstream, even when an antibody against αM chain was present. Other work had shown that neutrophils could migrate perpendicular to the direction of shear flow (Phillipson et al., 2009). We hypothesized that neutrophils exhibit limited upstream migration because neutrophils are of the myeloid lineage that, in addition to LFA-1 and VLA-4, express Mac-1 (αMβ2) (Langereis, 2013), which competes with LFA-1 for ICAM-1 binding (Smith et al., 1989). Indeed, work from our laboratory showed that by varying the amount of ICAM-1 on the surface, both the HL-60 neutrophil-like cell line and primary human neutrophils isolated from whole blood could migrate upstream in ICAM-1 surfaces when Mac-1 function was blocked (Buffone et al., 2019). Blocking Mac-1 allowed both resting and N-Formylmethionine-leucyl-phenylalanine (fMLP)-activated neutrophils to crawl upstream on ICAM-1, mixed ICAM-1/VCAM-1 surfaces, and activated HUVEC monolayers (Figure 4) (Buffone et al., 2019). We believe the difference between our results and the results from the Theodoly laboratory is due to differences in ICAM-1 density; we used lower densities of ICAM-1 which seems to have enhanced the mobility of neutrophils (Valignat et al., 2013; Buffone et al., 2019)
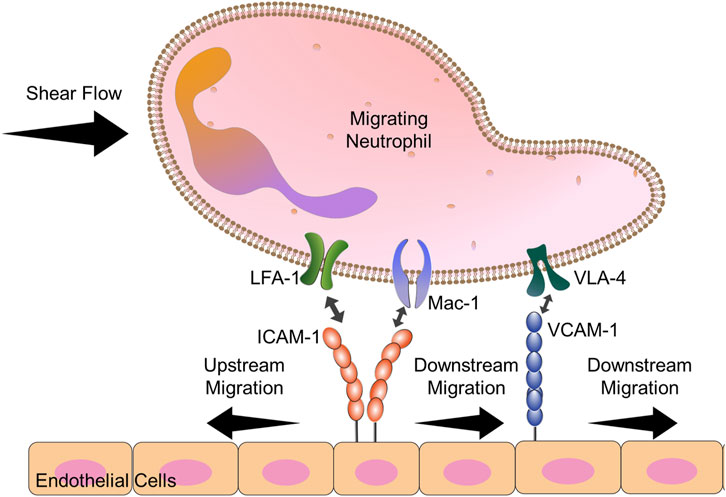
FIGURE 4. Diagram explains the key players in neutrophil upstream migration. VLA-4-VCAM-1 interactions lead always to downstream migration. Neutrophils have 2 cell surface ligands for ICAM-1: LFA-1 and Mac-1. When both Mac-1 and LFA-1 are allowed to engage ICAM-1, downstream migration is seen. Blocking Mac-1 function with monoclonal antibodies allows for LFA-1-ICAM-1 mediated upstream migration.
A recent paper by Dupuy et al. expanded upon the interplay between Mac-1 and LFA-1 in coordinating neutrophil upstream migration (Dupuy et al., 2023). By treating neutrophils with Protein disulfide Isomerase (PDI), Dupuy et al. demonstrated that PDI localizes specifically to Mac-1 at the trailing edge of the cell and selectively cleaves disulfide bonds, leading to Mac-1 release of ICAM-1. Furthermore, while the paper states cleavage of Mac-1 at the rear by PDI led to more downstream migration, this was compared to the bound but uncleaved (oxidized) PDI control. Association of PDI with the αM chain, but not cleavage, lead to significant upstream migration when compared to unbound control, similar to the antibody blocking studies designed by Buffone et al. (Buffone et al., 2019). In both studies (Buffone et al., 2019; Dupuy et al., 2023), globally blocking Mac-1 with oxidized PDI or monoclonal antibodies, lead to over 65% of cells crawling upstream. Dupuy and coworkers’ result indicates that the engagement of PDI alone can promote upstream migration in neutrophils.
Both groups show that neutrophils exhibit upstream migration by blocking Mac-1 and opens possibilities of controlling the ability any immune cell to migrate upstream by expressing or removing Mac-1 function. As to what the difference between LFA-1 and Mac-1, two integrins which compete to bind ICAM-1 and share a common β2 integrin chain, are in terms of distinct, outside-in intracellular signals that confer or disrupt upstream migration is an ongoing area and active area of research in our labs.
Macrophages: While no work to date has demonstrated the ability of monocytes to migrate upstream along the endothelium, recent work has demonstrated that extracellular matrix (ECM) resident macrophages are able to migrate against the direction of interstitial flow (Li et al., 2018), not blood flow like the other immune cells. This expands the phenomenon of upstream migration as this is the first demonstration of interstitial flow in the ECM as a mechanic stimulus (Quaranta, 2000) and with a ligand other than ICAM-1. These macrophages migrate upstream on collagen I surfaces, not vascular ICAM-1 and through β1, not β2 integrins like the other three immune cell types.
Interstitial flow emanating from tumors into the surrounding stroma activates mechanosensitive β1 integrins which activate SRC kinases and polarize macrophages into an M2 phenotype (Li et al., 2018). M2 Macrophages then migrate against the direction of interstitial flow from the ECM into the tumor microenvironment (TME). M2 Macrophage are immunosuppressive, actively play a role in shielding tumors from immunosurveillance, and secrete growth factors which make the tumor more invasive (Li et al., 2018). Furthermore, both MDA-435 melanoma cells and MDA-231 breast cancer cells were more protrusive and migratory when co-cultured with macrophages which had been exposed to interstitial flow (Li et al., 2018). Therefore, upstream migration by interstitial flow may be a critical regulator of escape from immunosurveillance by both polarizing and recruiting M2 macrophages into the tumor microenvironment and tumor progression and metastasis by allowing tumor cells to escape into the vasculature. It should be noted that the mechanism of M2 macrophage movement likely differs compared to other immune cell types, due to its abundant expression of Mac-1, but this remains an open area of investigation as to the direct mechanism.
Keratinocytes: Recent work was the first to establish that the upstream migration mode of motility is not restricted only to immune cells as a certain sub-population of fish keratinocytes are able to crawl upstream on glass slides (Seveau de Noray et al., 2022). Although the keratinocytes preferentially migrated downstream, a sub-population with a prominent trailing edge were able to crawl upstream, as compared to those with a prominent leading-edge.
The results regarding upstream migration in other cell types are summarized in Table 1.
The physiological role of upstream migration
There are at least three possibilities for the role of upstream migration in physiology. The first, suggested by many, is that upstream migration might be required for immune cells to return to the site of stimulation or insult, a so called “hotspot” (Bartholomaus et al., 2009; Valignat et al., 2013; Grönloh et al., 2023). The second, suggested in a recent seminar by Ulrich H. von Andrian, shows that memory T-cells migrate upstream and this might facilitate the repeated surveillance of a tissue as both effector and memory T cells were observed to repeatedly migrate upstream (Von Andrian, 2022). The third possibility is that upstream migration might facilitate transendothelial migration (TEM), because the mechanobiological forces exerted in upstream migration might be similar to those that are observed in migrating to the right junction for TEM to occur.
To support of the third concept, Anderson, Buffone & Hammer demonstrated upstream migration of T cells on activated human umbilical vein endothelial cells (HUVECs) monolayers (Anderson et al., 2019). T cells were observed to initially migrate upstream after arrest, provided LFA-1 was not blocked; however, the initial population of upstream migrating cells transmigrated (Figure 5; (Anderson et al., 2019)). The fraction of cells that remained on the apical surface of endothelium was greater if LFA-1 was blocked, suggesting LFA-1 facilitated transendothelial migration. Also, if cells were able to migrate upstream, the time to transmigrate was greatly diminished (Figure 5). These results suggest LFA-1 can mediate both transendothelial migration and upstream migration, and that the mechanisms that support upstream migration increase the speed and extent of transendothelial cell migration (Anderson et al., 2019).
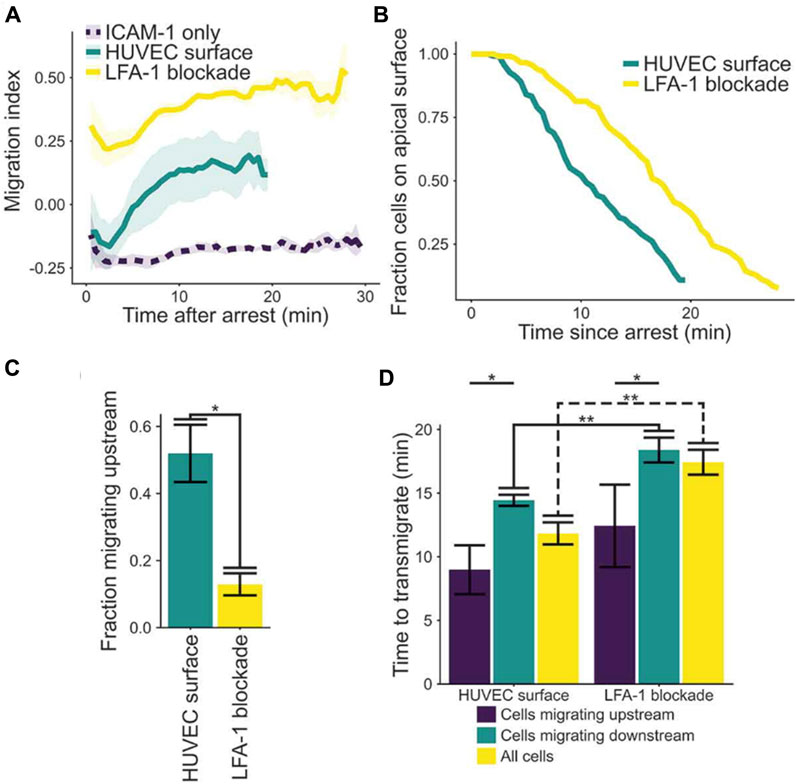
FIGURE 5. (A) Plot of migration index over time of activated CD4+ T cells on activated HUVEC and ICAM-1 surfaces. Upstream migration is indicated by a negative migration index, downstream migration by positive values, and random migration by values near zero. Blockade of LFA-1 prevents upstream migration on stimulated HUVECS, while cells with unblocked LFA-1 initially migrate upstream before reverting to downstream migration. ICAM-1-only recombinant protein surface data is provided for comparison. Data presented mean ± SEM, n = 4 independent experiments. (B) Plot showing the remaining fraction of tracked cells at each time point. Cells on HUVEC monolayers were tracked from initial migration to transmigration or the end of the experiment, whichever is sooner. (C) Comparison of fraction of cells which migrated upstream on HUVEC monolayers with or without LFA-1 blockade. Data presented mean ± SEM, n = 4 independent experiments. (D) Comparison of the time from arrest to transmigration on HUVEC monolayers with or without LFA-1 blockade. Data presented mean ± SEM, n = 4 independent experiments. *p < 0.05, **p < 0.005. Reproduced from Anderson et al., Cell Adh Migr, 2019 December; 13(1):163-168.
Future work
The upstream migration of leukocytes is a fascinating biophysical phenomenon. Cells actively migrate against the direction of flow, which requires harvesting mechanical and chemical machinery. Flow imparts a body force on cells, and cells must overcome that body force to stay adhered, detect the direction of flow, and reorient themselves to move in the opposite direction. Furthermore, what physiological mechanism/process makes it critical that lymphoid cells (T cells, B cells) readily migrate upstream while myeloid cells (neutrophils) do not unless “coaxed” to by antibody blocking is of great interest.
Accurate measurements of the forces generated during upstream migration can lead to a greater understanding of the phenomenon of the whole. Traction mapping through use of either traction force microscopy or multi-post array detectors (mPADs) have been used to generate traction maps previously for a variety of migrating immune cells (Dembo et al., 1996; Smith et al., 2007; Reinhart-King et al., 2008; Jannat et al., 2011; Ricart et al., 2011; Shebanova and Hammer, 2012; Henry et al., 2015; Hind et al., 2015; Hind et al., 2016; Bendell et al., 2017). It has proven difficult to determine traction maps of T-cells (Nordenfelt et al., 2016), as the force they exert is at the lower limit of detection in these assays. But upstream migration is not restricted to T cells, and HL-60 cells, which are often thought to be “neutrophil-like” (Garner et al., 2020), are able to readily migrate upstream on ICAM-1 surfaces where after Mac-1 is blocked (Buffone et al., 2019). Traction forces of neutrophils are readily measurable though bead displacement of embedded marker beads in elastic gels (Smith et al., 2007; Jannat et al., 2011). Interestingly under static conditions, the root mean squared (r.m.s) traction stress for neutrophils is about 50 nN; in chemotaxis, the r.m.s traction stress can be as high as 100 nN (Jannat et al., 2011). Therefore, one would expect traction stresses as high as 50 nN or higher for HL-60 cells or neutrophils that are migrating upstream. Moving forward, neutrophils (both HL-60 and primary neutrophils from whole blood) are viable cells to measure the traction stresses during the upstream migration owing to the ability of their traction forces to be measured (Smith et al., 2007; Jannat et al., 2011), and the ease to which they can be genetically manipulated (Buffone et al., 2013; Mondal et al., 2014; Mondal et al., 2016; Stolfa et al., 2016; Kelkar et al., 2020; Zhu et al., 2021).
An ongoing question is whether immune cells in the vasculature migrate upstream outside of the vasculature. The work of the Kamm lab in macrophage polarization against the direction of interstitial flows (Polacheck et al., 2011; Polacheck et al., 2014) raises the interesting question of whether immune cells also respond to interstitial flow, and migrate in the opposite direction after transmigration, and whether this mechanism can be controlled to promote more efficient cell migration through tissues.
Further investigation of the physiological role of upstream migration is needed. Since most data describing this phenomenon has been done in in-vitro assays (Valignat et al., 2013; Dominguez et al., 2015; Tedford et al., 2017; Buffone et al., 2018; Anderson et al., 2019; Buffone et al., 2019; Roy et al., 2020), more careful studies into the specific context in which cells migrate upstream in-vivo is needed. Some studies have shown a random migration of immune cells in-vivo (Phillipson et al., 2006; Phillipson et al., 2009; Snelgrove et al., 2019; Kibaek et al., 2021) while others have shown prominent upstream migration in-vivo (Bartholomaus et al., 2009; Steiner et al., 2010). The next step of truly understanding the importance of upstream migration will be in which physiologically relevant situations it occurs. Such an in-vivo test would involve an animal disease model for immune cell surveillance and clearance, in which one can compare the physiological response of cells which are able to migrate upstream (wild type T cells) and those in which upstream migration is disabled (edited T cells). The work of Roy et al. points to candidate molecules that can be depleted to disable upstream migration (Roy et al., 2020), but wider screens may be necessary to identify specific molecules that enable upstream migration without affecting other physiological responses. Such experiments would also be aided by in vivo fluorescent tracking of the differential response of immune cells in a disease model (John et al., 2011).
Author contributions
AB: Conceptualization, Formal Analysis, Funding acquisition, Investigation, Project administration, Supervision, Writing–original draft, Writing–review and editing. DH: Conceptualization, Data curation, Formal Analysis, Funding acquisition, Investigation, Methodology, Resources, Software, Supervision, Writing–original draft, Writing–review and editing. SK: Writing–original draft, Writing–review and editing. NA: Writing–original draft, Writing–review and editing. AM: Writing–original draft, Writing–review and editing. D-HL: Writing–original draft, Writing–review and editing. SG: Writing–original draft, Writing–review and editing.
Funding
The author(s) declare financial support was received for the research, authorship, and/or publication of this article. This work was funded by NIH R01 GM143357 to DH and AB.
Conflict of interest
NA is a current employee of Carisma Therapeutics (Philadelphia, PA).
The remaining authors declare that the research was conducted in the absence of any commercial or financial relationships that could be construed as a potential conflict of interest.
Publisher’s note
All claims expressed in this article are solely those of the authors and do not necessarily represent those of their affiliated organizations, or those of the publisher, the editors and the reviewers. Any product that may be evaluated in this article, or claim that may be made by its manufacturer, is not guaranteed or endorsed by the publisher.
References
AbuSamra, D. B., Aleisa, F. A., Al-Amoodi, A. S., Jalal Ahmed, H. M., Chin, C. J., Abuelela, A. F., et al. (2017). Not just a marker: CD34 on human hematopoietic stem/progenitor cells dominates vascular selectin binding along with CD44. Blood Adv. 1 (27), 2799–2816. doi:10.1182/bloodadvances.2017004317
Anderson, N. R., Buffone, A., and Hammer, D. A. (2019). T lymphocytes migrate upstream after completing the leukocyte adhesion cascade. Cell Adhesion Migr. 13 (1), 163–168. doi:10.1080/19336918.2019.1587269
Appelbaum, F. R. (2007). Hematopoietic-cell transplantation at 50. N. Engl. J. Med. 357 (15), 1472–1475. doi:10.1056/NEJMp078166
Bartholomaus, I., Kawakami, N., Odoardi, F., Schläger, C., Miljkovic, D., Ellwart, J. W., et al. (2009). Effector T cell interactions with meningeal vascular structures in nascent autoimmune CNS lesions. Nature 462 (7269), 94–98. doi:10.1038/nature08478
Belliveau, N. M., Footer, M. J., Akdogan, E., van Loon, A. P., Collins, S. R., and Theriot, J. A. (2022). Cell migration CRISPRi screens in human neutrophils reveal regulators of context-dependent migration and differentiation state. bioRxiv, 2022. doi:10.1101/2022.12.16.520717
Bendell, A. C., Williamson, E. K., Chen, C. S., Burkhardt, J. K., and Hammer, D. A. (2017). The Arp2/3 complex binding protein HS1 is required for efficient dendritic cell random migration and force generation. Integr. Biol. quantitative Biosci. Nano macro 9 (8), 695–708. doi:10.1039/c7ib00070g
Bhatia, S. K., King, M. R., and Hammer, D. A. (2003). The state diagram for cell adhesion mediated by two receptors. Biophys. J. 84 (4), 2671–2690. doi:10.1016/S0006-3495(03)75073-5
Buffone, A., Anderson, N. R., and Hammer, D. A. (2019). Human neutrophils will crawl upstream on ICAM-1 if mac-1 is blocked. Biophysical J. 117 (8), 1393–1404. doi:10.1016/j.bpj.2019.08.044
Buffone, A., Anderson, N. R., and Hammer, D. A. (2018). Migration against the direction of flow is LFA-1-dependent in human hematopoietic stem and progenitor cells. J. Cell Sci. 131 (1), jcs205575. doi:10.1242/jcs.205575
Buffone, A., Mondal, N., Gupta, R., McHugh, K. P., Lau, J. T. Y., and Neelamegham, S. (2013). Silencing α1,3-fucosyltransferases in human leukocytes reveals a role for FUT9 enzyme during E-selectin-mediated cell adhesion. J. Biol. Chem. 288 (3), 1620–1633. doi:10.1074/jbc.M112.400929
Bujko, K., Kucia, M., Ratajczak, J., and Ratajczak, M. Z. (2019). Hematopoietic stem and progenitor cells (HSPCs). Adv. Exp. Med. Biol. 1201, 49–77. doi:10.1007/978-3-030-31206-0_3
Carvalho, J. M., Souza, M. K. d., Buccheri, V., Rubens, C. V., Kerbauy, J., and Oliveira, J. S. R. d. (2009). CD34-positive cells and their subpopulations characterized by flow cytometry analyses on the bone marrow of healthy allogenic donors. Sao Paulo Med. J. 127, 12–18. doi:10.1590/s1516-31802009000100004
Cerutti, A., Cols, M., and Puga, I. (2013). Marginal zone B cells: virtues of innate-like antibody-producing lymphocytes. Nat. Rev. Immunol. 13 (2), 118–132. doi:10.1038/nri3383
Dembo, M., Oliver, T., Ishihara, A., and Jacobson, K. (1996). Imaging the traction stresses exerted by locomoting cells with the elastic substratum method. Biophysical J. 70 (4), 2008–2022. doi:10.1016/S0006-3495(96)79767-9
Dembo, M., Torney, D. C., Saxman, K., and Hammer, D. (1988). The reaction-limited kinetics of membrane-to-surface adhesion and detachment. Proc. R. Soc. Lond. B 234, 55–83. doi:10.1098/rspb.1988.0038
Dominguez, G. A., Anderson, N. R., and Hammer, D. A. (2015). The direction of migration of T-lymphocytes under flow depends upon which adhesion receptors are engaged. Integr. Biol. quantitative Biosci. Nano macro 7 (3), 345–355. doi:10.1039/c4ib00201f
Dupuy, A., Aponte-Santamaría, C., Yeheskel, A., Hortle, E., Oehlers, S. H., Gräter, F., et al. (2023). Mechano-redox control of mac-1 de-adhesion by PDI promotes directional movement under flow. Circulation Res. 132 (9), e151–e168. doi:10.1161/CIRCRESAHA.122.321926
Eniola, A. O., Willcox, J., and Hammer, D. A. (2003). Interplay between rolling and firm adhesion elucidated with a cell-free system engineered with two distinct receptor-ligand pairs. Biophysical J. 85, 2720–2731. doi:10.1016/S0006-3495(03)74695-5
Garner, R. M., Skariah, G., Hadjitheodorou, A., Belliveau, N. M., Savinov, A., Footer, M. J., et al. (2020). Neutrophil-like HL-60 cells expressing only GFP-tagged beta-actin exhibit nearly normal motility. Cytoskeleton 77 (5-6), 181–196. doi:10.1002/cm.21603
Grönloh, M. L. B., Tebbens, M. E., Kotsi, M., Arts, J. J. G., and van Buul, J. D. (2023). ICAM-2 regulates diapedesis hotspots by allowing neutrophil crawling against the direction of flow. Vasc. Biol., VB-23–0005. doi:10.1530/VB-23-0005
Heit, B., Colarusso, P., and Kubes, P. (2005). Fundamentally different roles for LFA-1, Mac-1 and alpha4-integrin in neutrophil chemotaxis. J. Cell Sci. 118 (22), 5205–5220. doi:10.1242/jcs.02632
Henry, S. J., Chen, C. S., Crocker, J. C., and Hammer, D. A. (2015). Protrusive and contractile forces of spreading human neutrophils. Biophysical J. 109 (4), 699–709. doi:10.1016/j.bpj.2015.05.041
Hind, L. E., Dembo, M., and Hammer, D. A. (2015). Macrophage motility is driven by frontal-towing with a force magnitude dependent on substrate stiffness. Integr. Biol. 7 (4), 447–453. doi:10.1039/c4ib00260a
Hind, L. E., Lurier, E. B., Dembo, M., Spiller, K. L., and Hammer, D. A. (2016). Effect of M1-M2 polarization on the motility and traction stresses of primary human macrophages. Cell Mol. Bioeng. 9 (3), 455–465. doi:10.1007/s12195-016-0435-x
Hornung, A., Sbarrato, T., Garcia-Seyda, N., Aoun, L., Luo, X., Biarnes-Pelicot, M., et al. (2020). A bistable mechanism mediated by integrins controls mechanotaxis of leukocytes. Biophys. J. 118 (3), 565–577. doi:10.1016/j.bpj.2019.12.013
Jannat, R. A., Dembo, M., and Hammer, D. A. (2011). Traction forces of neutrophils migrating on compliant substrates. Biophysical J. 101 (3), 575–584. doi:10.1016/j.bpj.2011.05.040
Johansen, K. H., Golec, D. P., Huang, B., Park, C., Thomsen, J. H., Preite, S., et al. (2022). A CRISPR screen targeting PI3K effectors identifies RASA3 as a negative regulator of LFA-1-mediated adhesion in T cells. Sci. Signal 15 (743), eabl9169. doi:10.1126/scisignal.abl9169
John, B., Ricart, B., Tait Wojno, E. D., Harris, T. H., Randall, L. M., Christian, D. A., et al. (2011). Analysis of behavior and trafficking of dendritic cells within the brain during Toxoplasmic encephalitis. PLoS Pathog. 7 (9), e1002246. doi:10.1371/journal.ppat.1002246
Kelkar, A., Zhu, Y., Groth, T., Stolfa, G., Stablewski, A. B., Singhi, N., et al. (2020). Doxycycline-dependent self-inactivation of CRISPR-cas9 to temporally regulate on- and off-target editing. Mol. Ther. 28 (1), 29–41. doi:10.1016/j.ymthe.2019.09.006
Kibaek, C., Moon, J., Lee, S. Y., Song, E., Back, J. H., Song, J. H., et al. (2021). Stepwise transmigration of T- and B cells through a perivascular channel in high endothelial venules. Life Sci. Alliance 4 (8), e202101086. doi:10.26508/lsa.202101086
Kim, S. H. J., and Hammer, D. A. (2021). Integrin cross-talk modulates stiffness-independent motility of CD4+ T lymphocytes. Mol. Biol. Cell 32 (18), 1749–1757. doi:10.1091/mbc.E21-03-0131
Kim, S. H. J., and Hammer, D. A. (2019). Integrin crosstalk allows CD4+T lymphocytes to continue migrating in the upstream direction after flow. Integr. Biol. 11 (10), 384–393. doi:10.1093/intbio/zyz034
Langereis, J. D. (2013). Neutrophil integrin affinity regulation in adhesion, migration, and bacterial clearance. Cell adhesion Migr. 7 (6), 476–481. doi:10.4161/cam.27293
Ley, K., Laudanna, C., Cybulsky, M. I., and Nourshargh, S. (2007). Getting to the site of inflammation: the leukocyte adhesion cascade updated. Nat. Rev. Immunol. 7 (9), 678–689. doi:10.1038/nri2156
Li, R., Serrano, J. C., Xing, H., Lee, T. A., Azizgolshani, H., Zaman, M., et al. (2018). Interstitial flow promotes macrophage polarization toward an M2 phenotype. Mol. Biol. Cell 29 (16), 1927–1940. doi:10.1091/mbc.E18-03-0164
Lopes-Carvalho, T., Foote, J., and Kearney, J. F. (2005). Marginal zone B cells in lymphocyte activation and regulation. Curr. Opin. Immunol. 17 (3), 244–250. doi:10.1016/j.coi.2005.04.009
López-Hoyos, M., Revilladagger, C., Conde, C., Del Campo, E. G., González, A., and Merino, J. (1999). Different roles for LFA-1 and VLA-4 integrins in T-B-cell interactions in vivo. Immunology 97 (3), 438–446. doi:10.1046/j.1365-2567.1999.00794.x
Luo, X., Seveau de Noray, V., Aoun, L., Biarnes-Pelicot, M., Strale, P. O., Studer, V., et al. (2020). Lymphocytes perform reverse adhesive haptotaxis mediated by LFA-1 integrins. J. Cell Sci. 133 (16), jcs242883. doi:10.1242/jcs.242883
Mazo, I. B., Gutierrez-Ramos, J. C., Frenette, P. S., Hynes, R. O., Wagner, D. D., and von Andrian, U. H. (1998). Hematopoietic progenitor cell rolling in bone marrow microvessels: parallel contributions by endothelial selectins and vascular cell adhesion molecule 1. J. Exp. Med. 188 (3), 465–474. doi:10.1084/jem.188.3.465
Mebius, R. E., and Kraal, G. (2005). Structure and function of the spleen. Nat. Rev. Immunol. 5 (8), 606–616. doi:10.1038/nri1669
Mondal, N., Buffone, A., Stolfa, G., Antonopoulos, A., Lau, J. T. Y., Haslam, S. M., et al. (2014). ST3Gal-4 is the primary sialyltransferase regulating the synthesis of E-P-and L-selectin ligands on human myeloid leukocytes. Blood 125 (4), 687–696. doi:10.1182/blood-2014-07-588590
Mondal, N., Stolfa, G., Antonopoulos, A., Zhu, Y., Wang, S. S., Buffone, A., et al. (2016). Glycosphingolipids on human myeloid cells stabilize E-selectin–dependent rolling in the multistep leukocyte adhesion cascade. Arteriosclerosis, Thrombosis, Vasc. Biol. 36 (4), 718–727. doi:10.1161/ATVBAHA.115.306748
Nordenfelt, P., Elliott, H. L., and Springer, T. A. (2016). Coordinated integrin activation by actin-dependent force during T-cell migration. Nat. Commun. 7 (1), 13119. doi:10.1038/ncomms13119
Phillipson, M., Heit, B., Colarusso, P., Liu, L., Ballantyne, C. M., and Kubes, P. (2006). Intraluminal crawling of neutrophils to emigration sites: a molecularly distinct process from adhesion in the recruitment cascade. J. Exp. Med. 203 (12), 2569–2575. doi:10.1084/jem.20060925
Phillipson, M., Heit, B., Parsons, S. A., Petri, B., Mullaly, S. C., Colarusso, P., et al. (2009). Vav1 is essential for mechanotactic crawling and migration of neutrophils out of the inflamed microvasculature. J. Immunol. 182 (11), 6870–6878. doi:10.4049/jimmunol.0803414
Piechocka, I. K., Keary, S., Sosa-Costa, A., Lau, L., Mohan, N., Stanisavljevic, J., et al. (2021). Shear forces induce ICAM-1 nanoclustering on endothelial cells that impact on T-cell migration. Biophysical J. 120 (13), 2644–2656. doi:10.1016/j.bpj.2021.05.016
Polacheck, W. J., Charest, J. L., and Kamm, R. D. (2011). Interstitial flow influences direction of tumor cell migration through competing mechanisms. Proc. Natl. Acad. Sci. 108 (27), 11115–11120. doi:10.1073/pnas.1103581108
Polacheck, W. J., German, A. E., Mammoto, A., Ingber, D. E., and Kamm, R. D. (2014). Mechanotransduction of fluid stresses governs 3D cell migration. Proc. Natl. Acad. Sci. 111 (7), 2447–2452. doi:10.1073/pnas.1316848111
Quaranta, V. (2000). Cell migration through extracellular matrix: membrane-type metalloproteinases make the way. J. Cell Biol. 149 (6), 1167–1170. doi:10.1083/jcb.149.6.1167
Reinhart-King, C. A., Dembo, M., and Hammer, D. A. (2008). Cell-cell mechanical communication through compliant substrates. Biophys. J. 95 (12), 6044–6051. doi:10.1529/biophysj.107.127662
Ricart, B. G., Yang, M. T., Hunter, C. A., Chen, C. S., and Hammer, D. A. (2011). Measuring traction forces of motile dendritic cells on micropost arrays. Biophysical J. 101 (11), 2620–2628. doi:10.1016/j.bpj.2011.09.022
Robert, P., Biarnes-Pelicot, M., Garcia-Seyda, N., Hatoum, P., Touchard, D., Brustlein, S., et al. (2021). Functional mapping of adhesiveness on live cells reveals how guidance phenotypes can emerge from complex spatiotemporal integrin regulation. Front. Bioeng. Biotechnol. 9, 625366. doi:10.3389/fbioe.2021.625366
Roy, N. H., Kim, S. H. J., Buffone, A., Blumenthal, D., Huang, B., Agarwal, S., et al. (2020). LFA-1 signals to promote actin polymerization and upstream migration in T cells. J. Cell Sci. 133 (17), jcs248328. doi:10.1242/jcs.248328
Roy, N. H., MacKay, J. L., Robertson, T. F., Hammer, D. A., and Burkhardt, J. K. (2018). Crk adaptor proteins mediate actin-dependent T cell migration and mechanosensing induced by the integrin LFA-1. Sci. Signal. 11 (560), 16. doi:10.1126/scisignal.aat3178
Seveau de Noray, V., Manca, F., Mainil, I., Remson, A., Biarnes-Pelicot, M., Gabriele, S., et al. (2022). Keratocytes migrate against flow with a roly-poly-like mechanism. Proc. Natl. Acad. Sci. 119 (48), e2210379119. doi:10.1073/pnas.2210379119
Shebanova, O., and Hammer, D. A. (2012). Biochemical and mechanical extracellular matrix properties dictate mammary epithelial cell motility and assembly. Biotechnol. J. 7 (3), 397–408. doi:10.1002/biot.201100188
Smith, C. W., Marlin, S. D., Rothlein, R., Toman, C., and Anderson, D. C. (1989). Cooperative interactions of LFA-1 and Mac-1 with intercellular adhesion molecule-1 in facilitating adherence and transendothelial migration of human neutrophils in vitro. J. Clin. Invest. 83 (6), 2008–2017. doi:10.1172/JCI114111
Smith, L. A., Aranda-Espinoza, H., Haun, J. B., Dembo, M., and Hammer, D. A. (2007). Neutrophil traction stresses are concentrated in the uropod during migration. Biophys. J. 92 (7), L58–L60. doi:10.1529/biophysj.106.102822
Snelgrove, S. L., Abeynaike, L. D., Thevalingam, S., Deane, J. A., and Hickey, M. J. (2019). Regulatory T cell transmigration and intravascular migration undergo mechanistically distinct regulation at different phases of the inflammatory response. J. Immunol. 203 (11), 2850–2861. doi:10.4049/jimmunol.1900447
Steiner, O., Coisne, C., Cecchelli, R., Boscacci, R., Deutsch, U., Engelhardt, B., et al. (2010). Differential roles for endothelial ICAM-1, ICAM-2, and VCAM-1 in shear-resistant T cell arrest, polarization, and directed crawling on blood–brain barrier endothelium. J. Immunol. 185 (8), 4846–4855. doi:10.4049/jimmunol.0903732
Stolfa, G., Mondal, N., Zhu, Y., Yu, X., Buffone, A., and Neelamegham, S. (2016). Using CRISPR-Cas9 to quantify the contributions of O-glycans, N-glycans and Glycosphingolipids to human leukocyte-endothelium adhesion. Sci. Rep. 6, 30392. doi:10.1038/srep30392
Tedford, K., Steiner, M., Koshutin, S., Richter, K., Tech, L., Eggers, Y., et al. (2017). The opposing forces of shear flow and sphingosine-1-phosphate control marginal zone B cell shuttling. Nat. Commun. 8 (1), 2261. doi:10.1038/s41467-017-02482-4
Tedford, K., Tech, L., Steiner, M., Korthals, M., and Fischer, K. D. (2018). Analysis of shear flow-induced migration of murine marginal zone B cells in vitro. JoVE (141), e58759. doi:10.3791/58759
Ulyanova, T., Scott, L. M., Priestley, G. V., Jiang, Y., Nakamoto, B., Koni, P. A., et al. (2005). VCAM-1 expression in adult hematopoietic and nonhematopoietic cells is controlled by tissue-inductive signals and reflects their developmental origin. Blood 106 (1), 86–94. doi:10.1182/blood-2004-09-3417
Valignat, M.-P., Theodoly, O., Gucciardi, A., Hogg, N., and Lellouch, A. C. (2013). T lymphocytes orient against the direction of fluid flow during LFA-1-mediated migration. Biophysical J. 104 (2), 322–331. doi:10.1016/j.bpj.2012.12.007
Valignat, M. P., Nègre, P., Cadra, S., Lellouch, A. C., Gallet, F., Hénon, S., et al. (2014). Lymphocytes can self-steer passively with wind vane uropods. Nat. Commun. 5, 5213. doi:10.1038/ncomms6213
Wojcikiewicz, E. P., Abdulreda, M. H., Zhang, X., and Moy, V. T. (2006). Force spectroscopy of LFA-1 and its ligands, ICAM-1 and ICAM-2. Biomacromolecules 7 (11), 3188–3195. doi:10.1021/bm060559c
Zhang, X., Wojcikiewicz, E., and Moy, V. (2002). Force spectroscopy of the leukocyte function-associated antigen-1/intercellular adhesion molecule-1 interaction. Biophysical J. 83, 2270–2279. doi:10.1016/S0006-3495(02)73987-8
Keywords: T-cells, leukocytes, inflammation, LFA-1, ICAM-1, hematopoietic stem cells, migration
Citation: Buffone A Jr, Hammer DA, Kim SHJ, Anderson NR, Mochida A, Lee D-H and Guin S (2023) Not all (cells) who wander are lost: Upstream migration as a pervasive mode of amoeboid cell motility. Front. Cell Dev. Biol. 11:1291201. doi: 10.3389/fcell.2023.1291201
Received: 08 September 2023; Accepted: 06 October 2023;
Published: 02 November 2023.
Edited by:
Arie Horowitz, Université de Rouen, FranceReviewed by:
Valentin P. Yakubenko, East Tennessee State University, United StatesKihong Lim, University of Rochester, United States
Copyright © 2023 Buffone, Hammer, Kim, Anderson, Mochida, Lee and Guin. This is an open-access article distributed under the terms of the Creative Commons Attribution License (CC BY). The use, distribution or reproduction in other forums is permitted, provided the original author(s) and the copyright owner(s) are credited and that the original publication in this journal is cited, in accordance with accepted academic practice. No use, distribution or reproduction is permitted which does not comply with these terms.
*Correspondence: Alexander Buffone Jr, YWIyNTc0QG5qaXQuZWR1; Daniel A. Hammer, aGFtbWVyQHNlYXMudXBlbm4uZWR1