- 1Department of Hepatobiliary and Pancreatic Surgery, General Surgery Center, The First Hospital of Jilin University, Changchun, China
- 2Center of Infectious Diseases and Pathogen Biology, Institute of Virology and AIDS Research, Key Laboratory of Organ Regeneration and Transplantation of The Ministry of Education, The First Hospital of Jilin University, Changchun, China
Dendritic cells (DCs) are the most powerful antigen presenting cells (APCs), they are considered one of the key regulatory factors in the liver immune system. There is currently much interest in modulating DC function to improve transplant immune response. In liver transplantation, DCs participate in both the promotion and inhibition of the alloreponse by adopting different phenotypes and function. Thus, in this review, we discussed the origin, maturation, migration and pathological effects of several DC subsets, including the conventional DC (cDC), plasmacytoid DC (pDC) and monocyte-derived DC (Mo-DC) in liver transplantation, and we summarized the roles of these DC subsets in liver transplant rejection and tolerance. In addition, we also outlined the latest progress in DC-based related treatment regimens. Overall, our discussion provides a beneficial resource for better understanding the biology of DCs and their manipulation to improve the immune adaptability of patients in transplant status.
1 Introduction
In the early 1970 s, Ralph Steinman and others discovered a morphologically and functionally distinct, previously uncharacterized population of cells in their experiments. They called the cells “dendritic cell” (DC) due to their “stellate” or “dendritic” morphology (Steinman and Cohn, 1973). In the liver, DCs are preferentially found in the periportal and pericentral space (Rahman and Aloman, 2013; Eckert et al., 2015). They account for about 1% of non-parenchymal cells and are related to innate and adaptive immunity by promoting and regulating T cell immunity, which are considered to be one of the key regulators in liver immune system (Hsu et al., 2007; Thomson et al., 2020; Bošnjak et al., 2022). DCs continuously flow into the liver from the blood, and circulating DCs are recruited to the hepatic sinusoids by hepatic Kupffer cells in a c-type lectin-dependent manner (Kudo et al., 1997; Uwatoku et al., 2001). Liver normally contains more interstitial dendritic cells than other parenchymal organs (Steptoe et al., 2000), which may be the result of the pathogen associated molecular pattern (PAMP) in portal blood.
Liver transplantation is the most effective treatment for various end-stage liver diseases. The liver is an immune preferential organ (Demetris et al., 2016). In addition to patients undergoing transplantation for autoimmune liver diseases (such as primary cholangitis, primary sclerosing cholangitis, and autoimmune hepatitis), clinically more than 30% of patients can get rid of the use of immunosuppressant (IS) and achieve a state of immune tolerance (Clavien et al., 2017). In liver transplantation, DCs participate in both the promotion and inhibition of the alloreponse by adopting different phenotypes and function. Here, we discussed the origin, maturation, migration and pathological effects of several DC subsets, including the conventional DC (cDC), plasmacytoid DC (pDC) and monocyte-derived DC (Mo-DC), and we summarized the roles of these DC subsets in liver transplant rejection and tolerance. In addition, we also outlined the latest progress in DC-based related treatment regimens.
2 The characteristics of DC
2.1 The subgroups of DC
According to the current novel, ontogenetic, and functionally relevant simplified classification system, DCs are classified into cDC, pDC, Mo-DC, and Langerhans cell (LC) (Satpathy et al., 2012). cDC can be further divided into two subsets: conventional DC type 1 (cDC1) and conventional DC type 2 (cDC2). cDC can maintain immune homeostasis, simultaneously rapidly respond to local damage, and initiate and guide innate and adaptive immunity (Cabeza-Cabrerizo et al., 2021). pDC act as both innate antiviral effectors and inducers and regulators of adaptive immunity (Fonteneau et al., 2003), regulating the induction and/or maintenance of tolerance to hematopoietic stem cells (HSCs) or allogeneic organ grafts (Rogers et al., 2013). Mo-DC is considered to be the main inflammatory cell type during infection (Geissmann et al., 2003; Serbina et al., 2003). Monocytes differentiate into Mo-DC during inflammation or infection, which cooperate with cDC to induce T cell-mediated immune responses (Bošnjak et al., 2022). Traditionally, LC has been considered a DC population in the epidermis (Schuler and Steinman, 1985; Romani et al., 1989; Wilson and Villadangos, 2004). Therefore, this article will focus on cDC, pDC, and Mo-DC.
2.2 DC development
Human and mouse DC develop from progenitor cells in the bone marrow and then differentiate into distinct subpopulations that are spread across multiple tissues (Durai and Murphy, 2016; Guilliams et al., 2016). HSCs in bone marrow can give rise to granulocytes, monocytes, and DC progenitor cell (GMDP), which in turn give rise to monocyte and macrophage DC progenitor cell (MDP). They give rise to a common DC progenitor (CDP) and a common monocyte progenitor (cMoP) (Fogg et al., 2006; Hettinger et al., 2013). CDP produces classical/conventional DC precursor cell (pre-cDC) and pDC, both cDC1 and cDC2 are derived from pre-cDC and can be found in blood and bone marrow. cMoP produces monocytes in the bone marrow, then enters the peripheral blood, enters the tissue during inflammation or infection and further differentiates into Mo-DC (Hettinger et al., 2013; Que et al., 2020). The development of DC is depicted in Figure 1.
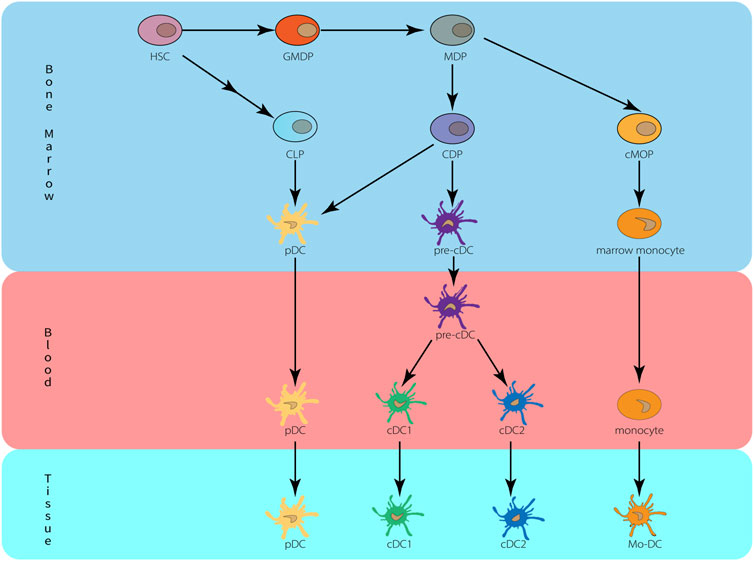
FIGURE 1. Origin and development of dendritic cells. HSC, hematopoietic stem cells; MDP, monocyte-macrophage DC progenitor; CDP, common dendritic cell progenitor; CLP, common lymphoid progenitor; cMoP, common monocyte progenitors; Mo-DC, monocyte-derived DC.
2.3 Markers for DC identification
DC subsets differ in the expression of surface markers and transcription factors (Table 1). Mouse cDC could be divided into two subsets: cDC1 was identified as lineage (Lin)− major histocompatibility complex Class II (MHC-II)+cluster of differentiation (CD)11c+CD103+CD11b−. cDC2 was identified as Lin−MHC-II+CD11c+CD103−CD11b+. Human cDC can be subdivided based on the expression of CD1c+ [also known as blood dendritic cell antigen (BDCA)1] and CD141+ (BDCA3) (Haniffa et al., 2012; Guilliams et al., 2014; Pulendran, 2015). CD141+ cDC differentiate and mature under the influence of INF regulatory factor 8 (IRF8), express XC-chemokine receptor 1 (XCR1)+, C-type lectin domain containing 9A (CLEC9A)+ and B- and T-lymphocyte attenuator 4 (BTLA4)+, secrete interleukin 12 (IL-12) to promote T helper 1 cell (Th1) differentiation, and also cross-present intracellular antigens (Murphy et al., 2016), these functions are similar to those of cDC1 in mice. CD1c+ cDC differentiate and mature under the influence of IRF4, express high levels of CD1b+, CD14+, and signal-regulatory protein alpha (SIRPα)+, and may further differentiate through Krüppel-like factor 4 (KLF4) to promote T helper 2 cell (Th2) differentiation or Notch signaling pathway to promote IL-23, thereby contributing to T helper 17 cell (Th17) differentiation. These functions are similar to those of cDC2 in mice.
The phenotype of mouse pDC was identified as MHC-IIintCD11cintB220+lymphocyte antigen 6 (Ly6)C+ bone marrow stromal antigen (BST)2+Sialic acid-binding immunoglobulin-type lectin (Siglec)-H+ (Colonna et al., 2004). The phenotype of human pDC was identified as human leukocyte antigen–DR isotype (HLA-DR)+CD11c−CD4+ BDCA2+BDCA4+CD123+ (Liu, 2005). Like pDC in mice, human pDC also can secrete type I interferon (IFN-I) and initiate antiviral immunity (Haniffa et al., 2012). The development of pDC depends on FMS-like tyrosine kinase 3 (FLT3) and its ligand FMS-like tyrosine kinase 3 ligand (FLT3L) (Schmid et al., 2010), and macrophage colony-stimulating factor (M-CSF) may also support the generation of pDC (Fancke et al., 2008). Transcription factor 4 (TCF4)(E2-2), IRF8, B-cell lymphoma/leukemia 11A (BCL11A), Zinc finger E-box-binding homeobox 2 (ZEB2), and Spi-B play important roles in the development and maintenance of the pDC phenotype (Reizis, 2019).
The phenotype of mouse Mo-DC was identified as MHC-II+CD11c+CD11b+CD64intLy6Cint C-C chemokine receptor 2 (CCR2)+CD209+ (Schlitzer et al., 2015; Menezes et al., 2016). The phenotype of human Mo-DC was identified as HLA-DR+CD11c+CD14intCD206+CD1c+ (Segura et al., 2013; Tang-Huau and Segura, 2019). Monocyte differentiation into Mo-DC depends on some key regulators, such as PU.1, IRF4, aryl hydrocarbon receptor (AHR), nuclear receptor 4A3 (NR4A3), and nuclear receptor co-repressor 2 (NCOR2) (Lehtonen et al., 2005; Briseño et al., 2016; Menezes et al., 2016; Goudot et al., 2017; Sander et al., 2017; Boulet et al., 2019).
2.4 DC migration and activating T cells
2.4.1 DC migration
A study (Lämmermann et al., 2008) used live cell imaging devices to directly observe the movement of DC sensing and entering afferent lymphatic vessels through wide-field microscopy in a mouse ear skin explant model. DC migration mainly depends on two chemokines: C-C motif ligand 19 (CCL19) and CCL21 (Lämmermann et al., 2008). The chemokine CCL21 is constitutively expressed by lymphatic endothelial cells (LECs) (Saeki et al., 1999; Russo et al., 2016), while its corresponding chemokine receptor CCR7 is induced in mature DC (Sallusto et al., 1998). They are expressed in LECs and lymph node T cell regions and bind to CCR7, which is upregulated on DC after activation (Förster et al., 1999). After approaching the lymphatic vessel, DCs dock with the lymphatic endothelium and enter the lumen. The docking and transport process is mediated by the interaction between lymphatic vessel endothelial hyaluronan receptor 1 (LYVE-1) on the LECs and the hyaluronic acid coating on the surface of the DC bound and organized by CD44 (Johnson et al., 2017; Johnson et al., 2021). Some researchers have used an intravital microscope (IVM) to prove that Rho-associated protein kinase (ROCK) is involved in the process of DC crawling and overall migration in lymph (Nitschké et al., 2012). After entering the initial lymphatic vessels, DCs use lamellipodia to crawl along the lymphatic endothelium and enter downstream by sensing lymphatic flow, and once DCs reach the collecting vessels, they begin to drift freely in lymphocytes (Tal et al., 2011). In vivo studies in mice, two-photon microscopy showed that after mature DC entered the lymph node parenchyma, there was a strong directional migration of DC to the T cell area, which was dependent on the expression of CCR7 (Braun et al., 2011), and possibly depend on the presence of CCL21 (Schumann et al., 2010). LECs, derived from the top of the subcapsular sinus (SCS) of lymph nodes, express atypical chemokine receptor 4 (ACKR4), which is a clearance receptor for CCR7 ligands and a few other chemokines. By scavenging CCR7 ligands in the SCS lumen, CCL21 gradients are actively created and drive DC exit from SCS into the lymph node parenchyma (Ulvmar et al., 2014). Imaging of these compartments revealed that ACKR4-expressing LECs create a chemokine gradient through local clearance of CCL19 and CCL21, which triggers directional DC migration (Ulvmar et al., 2014). Within lymph nodes, DCs process and present exogenous peptides in MHC I and II, and these MHC-peptide complexes are recognized by CD8+ T cells and CD4+ T cells through T cell receptors, respectively (Corse et al., 2011).
DCs migrate to the liver from the lymphatic or blood circulation. After entering the liver, Kupffer cells selectively capture DCs and transport them to the draining hepatic lymph nodes through the liver sinus-lymph pathway (Matsuno et al., 1996; Kudo et al., 1997; Matsuno and Ezaki, 2000). Blood-derived DCs are attracted by CCL3 on Kupffer cells and extravasate from the endothelial pores of liver sinus into the Disse space (Sato et al., 1998). The DCs then migrate to the portal vein area through the CCR7-CCL21 system and interact with T cells to create portal tract-associated lymphoid tissue (PALT) (Yoneyama et al., 2001). IL-1 receptor antagonist (IL-1RA), derived from hepatocytes, directly activates the IL-1 receptor (IL-1R)/toll-like receptor (TLR) signaling pathway of activated DCs to regulate this migration by inducing the expression of CCR7 (Iizasa et al., 2005).
2.4.2 Activating T cells
DC carrying antigen in lymph node T cell area can be effectively scanned by naive T cells. When naive T cells encounter cognate antigens in MHC-peptide complexes on the surface of DCs, naive T cells are activated and begin to proliferate rapidly (Bošnjak et al., 2022). The priming of T cells generated in lymph nodes occurs in three distinct stages, which can be distinguished by contact time and migration speed (Mempel et al., 2004; Miller et al., 2004). The first stage occurs approximately 8 h after T cells enter the lymph nodes, and the contact time between T cells and DCs are very short, usually no more than 30 min, and this stage is characterized by short contact between T cells and many DCs, mainly around high endothelial blood vessels. Chemokine CCL21 and intercellular adhesion molecule 1 (ICAM-1) played an important role in their brief contact (Bromley and Dustin, 2002; Friedman et al., 2006). The second stage occurs between approximately 8 h and 24 h, and the binding formed by T cells and DCs is stable and lasts for more than 1 h, and this stage is characterized by the formation of mature synaptoid contact areas between T cells and DCs. Adhesion molecules such as CD2 (Binder et al., 2020), lymphocyte function associated 1 (LFA-1) and ICAM-1 (Bromley and Dustin, 2002), as well as cytokines (Mempel et al., 2004) such as CD40L, IL-2 and interferon gamma (IFN-γ) play a role in the formation of mature synaptoid contact areas. The third stage occurs 24 h after the T cells enter the lymph nodes, the T cells separate from the DC, and the T cells rapidly migrate and proliferate, while continuing to make short contact with the DC. Cytokines (Mempel et al., 2004) such as CD40L, IL-2 and IFN-γ play a role in their short contact. The scavenger molecule Clever-1 (also known as Stabilin-1 and FEEL-1) expressed by LECs was shown to affect the migration of DC from the skin to the draining lymph node (dLN), the maturation state of the migrating DC and its ability to induce T-cell proliferation (Tadayon et al., 2021). Moreover, DC contributes to creating a cytokine environment (such as IL-10, IL-12, IL-17, IL-22, IL-23, IFN-I, tumor necrosis factor [TNF]) that drives naive T cells to differentiate into different types of effector T cells (Satpathy et al., 2012).
2.5 DC subpopulations in liver homeostasis
In the liver under homeostasis, hepatic DCs account for approximately 1% of the non-parenchymal hepatic cells and are a diversified population of hepatic APCs linked to innate and adaptive immunity and considered as key modulator of hepatic immune system (Hsu et al., 2007). The conventional hepatic DCs located at the periportal region and central veins, whereas the plasmacytoid hepatic DCs are located within the liver parenchyma (Jomantaite et al., 2004). The cDCs primarily serve as APCs, aiding in the activation and regulation of hepatic immune responses. The pDCs can generate a large amount of IFN-I [IFN-alpha (IFN-α) and IFN-beta (IFN-β)], which are crucial in inhibiting viral replication and promoting antiviral immune responses (Villadangos and Young, 2008; Haniffa et al., 2012). Additionally, pDCs can modulate the functions of other immune cells by producing cytokines and chemical mediators, such as activating NK cells and T cells (Brewitz et al., 2017). What’s more, Mo-DCs play important roles in immune regulation, anti-inflammatory effects, and immune surveillance in the liver homeostasis (León et al., 2007; Mildner et al., 2013; Plantinga et al., 2013). They also contribute to maintaining immune balance and protecting the liver from infection and damage (Boyette et al., 2017; Wolf et al., 2019).
3 cDC in the liver transplantation immune response
3.1 cDC in the liver transplant rejection
Acute cellular rejection (ACR) occurs in up to 30% of patients within the first year after liver transplantation, and the occurrence of ACR is associated with an increased risk of graft failure, graft failure-related death, and all-cause death (Levitsky et al., 2017). ACR is mainly a cellular immune response mediated by T cells after liver transplantation. DCs, as the most important APCs in priming T cells, they present allogeneic antigens to the cognate T-cell receptor (TCR) to activate them, thus initiating cellular immune responses (Yu et al., 2012; Ueta et al., 2021). Under homeostatic conditions, hepatic DCs exhibit an immature/anti-inflammatory phenotype, T cells are barely activated, and are less immunogenic (Bamboat et al., 2009). However, after liver transplantation, DC gradually matured under the stimulation of inflammatory factors such as TNF alpha (TNF-α), IFN-β, IFN-γ, IL-6, and prostaglandin E2 (PGE2). The mature DCs initiate immune responses by inducing T cell proliferation and activation (Reis e Sousa, 2006). Mature DCs are characterized by high expression of MHC-II molecules, costimulatory molecules (such as CD80, CD86 and CD40), chemokine receptors (such as CCR7), adhesion molecules (such as CD62L), and enhanced producing proinflammatory cytokines (such as TNF- α, IL-12) (Penna et al., 2002; Steinman and Banchereau, 2007; Colvin et al., 2008). The production of proinflammatory cytokines due to ischemia-reperfusion injury (IRI) during transplantation is considered to be a key factor involved in the maturation of donor DCs and their migration to recipient secondary lymphoid tissues (Lechler et al., 2001). After transplantation, danger signals mediated by pattern recognition receptors (PRRs) immediately activate DCs, leading to maturation, upregulation of costimulatory molecules, secretion of proinflammatory cytokines, and cytotoxicity (LaRosa et al., 2007).
After liver transplantation, the MHC-peptide complex on donor DC is presented to the host T cells, inducing T cell activation to initiate the immune response, which is a key step leading a donor-direct alloresponse (Hughes et al., 2020; Ronca et al., 2020). It is currently believed that there are three ways of allorecognition (Duneton et al., 2022): the direct pathway is that the donor-derived DC presents the donor MHC-antigen complex to the recipient T cells, the indirect pathway is that the recipient DCs capture donor antigen and present it to the recipient T cells, the semi-direct pathway refers to the presentation of the intact donor MHC I-antigen complex to recipient CD8+T cells in secondary lymphoid organs by “cross-modifying” receptor-derived DCs (it means that recipient DCs have acquired MHC-antigen complexes).
Extracellular vesicles (EVs) with the characteristics of exosomes plays an important role in the semi-direct pathway (Liu et al., 2016; Marino et al., 2016). Exosomes are 70–120 nm EV originated in the endocytic compartment of living cells, which have been shown to transfer proteins and RNAs between cells (Colombo et al., 2014; Robbins and Morelli, 2014). The previous view was that acute graft rejection was related to the migration of immunogenic passenger DCs to recipient lymphoid tissues (Lechler et al., 2001), now the exosomes, as a new mode of intercellular communication, have been shown to participate in IRI and immune regulation early after organ transplantation (Liu et al., 2016; Zheng et al., 2018; Hughes et al., 2020).
3.2 cDC in the liver transplant tolerance
In addition to initiating immune responses, cDC also plays a role in inducing and preserving immune tolerance. Lutz et al. suggested that cDC that induces tolerance is in a semi-mature state, while the induction of T cell responses requires complete DC maturation (Lutz and Schuler, 2002). DCs have been shown to be essential for maintaining central and peripheral tolerance through immune bias, inducing T cell weakness, promoting T cell apoptosis, and inducing regulatory T cells (Tregs) (Baroja-Mazo et al., 2016). Allogeneic organ transplantation can produce high frequency of allogeneic T cells, and the loss of donor reactive T cells is the key to induce transplantation tolerance. DCs can eliminate donor reactive T cells by inhibiting signaling or producing apoptotic factors (Lu et al., 1994; Süss and Shortman, 1996; Lu et al., 1999).
In the liver microenvironment, the promotion of tolerance by cDCs may be associated with hepatic stellate cells, which activate multiple immunosuppressive circuits in cDCs by secreting cytokines and chemokines (such as IL-6, CCL2, and CCL3) and all trans retinoic acid (ATRA) (Bhatt et al., 2014). These cytokines and chemokines activate the signal transducer and activator of transcription 3 (STAT3) signaling pathway in cDCs and promote apoptosis elimination and tolerance induction of effector T cells by upregulating indoleamine 2,3-dioxygenase (IDO) in a programmed death-ligand 1 (PD-L1)-independent manner (Sumpter et al., 2012). IDO inhibits effector T cells and supports Treg cells by decomposing tryptophan, leading to local tryptophan deficiency and secretion of kynurenine (Mellor et al., 2002; Favre et al., 2010; Higashitani et al., 2013). In addition, ATRA can induce arginase 1 (ARG1) and inducible nitric oxide synthase (iNOS) in cDCs, and both arginine catabolism and nitric oxide (NO) secretion can inhibit T cells (Bhatt et al., 2014).
Under homeostatic conditions, mouse and human hepatic DCs are tolerant (Rastellini et al., 1995; Thomson and Lu, 1999; Bamboat et al., 2009). Compared with DCs from extrahepatic tissues, hepatic DCs exhibit an immature phenotype with low expression of MHC-II and costimulatory molecules (Lu et al., 1994), and they exhibit lower endocytic capacity and are poor stimulators of T cells (Lukacs-Kornek and Schuppan, 2013). In addition, hepatic DCs also exert tolerance through the production of anti-inflammatory PGE2, which can upregulate IDO, enhance the secretion of IL-10 and induce the generation of Tregs (Raïch-Regué et al., 2014). Graft infiltrated host DCs expressed high levels of PD-L1, leading to depletion of CD8+ T cells and induction of immune tolerance, suggesting that hepatic DCs have a tolerogenic function after liver transplantation, which was confirmed in a liver transplantation model (Ono et al., 2018). Hepatic cDCs are also less mature in phenotype and function than secondary lymphoid tissues DC. In vivo experiments in mice, cDCs from the liver showed reduced ability to activate allogeneic naive T cells (Pillarisetty et al., 2004; De Creus et al., 2005; Abe et al., 2006). In addition, hepatic cDCs can also induce immune tolerance by affecting the function of T cells. In mouse experiments, hepatic cDCs were injected into allogeneic recipients and found to induce IL-10 secretion of T cells (Khanna et al., 2000). Interestingly, exosomes can also exhibit immunosuppressive effects to affect the immunogenicity of allografts in liver transplantation (Mastoridis et al., 2021). In this study, the cross-dressed sEVs (exosomes) showed the ability to inhibit the proliferation of CD8+ T cells in liver transplantation. In addition, DCs cocultured with exosomes from liver transplant patients can induce lower costimulatory molecule (CD40) expression, and produce less IL-6 and more IL-10, so they have higher ability to induce DC inhibitory phenotype. In a mouse liver transplantation model (Ono et al., 2018), graft infiltrating DCs cross-dressed by donor exosomes expressed high levels of PD-L1 and significantly inhibited the proliferation of donor reactive CD8+ T cells by inducing an exhaustion phenotype. In another rat model of liver transplantation (Ma et al., 2016), exosomes derived from immature DCs have been shown to amplify Tregs and prevent acute rejection.
4 pDC in the liver transplantation immune response
4.1 pDC in the liver transplant rejection
It is well known that pDCs can rapidly secrete large amounts of IFN-I in response to viral infection. In addition to secreting IFN-I, pDCs can express MHC-II molecules and costimulatory molecules CD40, CD80, and CD86, and can present antigens to CD4+ T cells, although not as effective as cDCs (Villadangos and Young, 2008; Reizis et al., 2011). Furthermore, pDCs can also regulate the survival of natural killer (NK) cells, DCs and macrophages through IFN-I, and expand CD4+ T cells and CD8+ T cells (Cervantes-Barragan et al., 2012; Brewitz et al., 2017). pDCs are considered to be a key factor in allograft rejection, but the underlying mechanism is still unclear. Ruben et al. demonstrated that pDC is an effective APC that can coordinate alloimmune responses and may play a key role in inducing chronic rejection in kidney transplantation (Ruben et al., 2018). The role of pDCs in solid organ rejection remain elusive. Experimental transplantation models have demonstrated that pDCs have the ability to present alloantigen (Björck et al., 2005; Ochando et al., 2006; Koyama et al., 2009). Another study showed that there was a large influx of pDCs in the renal tubulointerstitium at the time of rejection compared with the renal biopsy at the time of transplantation (Zuidwijk et al., 2012). In mice liver transplantation, if the donor is injected with FLT3L before transplantation to stimulate the generation of hepatic DCs (Drakes et al., 1997; Kingham et al., 2007), acute allograft rejection will occur in mice receiving liver transplantation without immunosuppression (Steptoe et al., 1997). This may be due to the fact that both cDCs (Steptoe et al., 1997) and pDCs (Kingham et al., 2007) mobilized by FLT3L increased the expression of CD80, CD86 and MHC-II molecules and enhanced the stimulatory ability of allogeneic T cells compared with normal hepatic DCs.
4.2 pDC in the liver transplant tolerance
Like cDCs, pDCs exhibit dual functions of immunogenicity and tolerance based on receptor connectivity and activation status. pDCs act as both innate antiviral immune effectors and inducers and regulators of adaptive immunity (Fonteneau et al., 2003), including hepatic T cell responses (Swiecki and Colonna, 2010). They drive the development of natural Tregs (Martín-Gayo et al., 2010), induce and maintain antigen-specific Tregs (Moseman et al., 2004; Palomares et al., 2012). Compared with lymphoid tissue pDCs, donor derived hepatic pDCs express high levels of DNAX-activating protein of 12 kDa (DAP12), triggering receptor expressed on myeloid cells 2 (TREM2) and a high proportion of T cell coinhibitory molecule PD-L1: costimulatory molecule CD80/86, which can attenuate graft infiltrating T effector cell responses, enhance forkhead box P3 (FOXP3)+Treg, and promote spontaneous tolerance of liver allograft in mice (Nakano et al., 2021). Compared with spleen-derived pDCs, mouse liver-derived pDCs exhibit an immature phenotype and lower levels of IL-12p70 secretion, and therefore, they exhibit reduced ability to present antigens or activate T cells (Kingham et al., 2007; Tokita et al., 2008). In addition, compared with spleen pDCs, liver pDCs secrete lower levels of IFN-I after cytosine phosphate guanosine (CpG) stimulation (Castellaneta et al., 2009), which can be explained by their relatively high expression of nucleotide-binding oligomerization domain-containing protein 2 (NOD2), a member of the nucleotide-binding oligomerization domain-like receptor (NLR) family. NOD2 can inhibit DC maturation and its ability to induce the proliferation of allogeneic T cells by inhibiting TLR signaling (Manicassamy and Pulendran, 2011). NOD2 can also interfere with TLR4 and TLR9 signaling pathways in pDCs through its ligands, but not in cDCs, resulting in decreased secretion of pro-inflammatory cytokines (IL-6, IL-12p70, TNF-α, and IFN-γ) (Castellaneta et al., 2009). What’s more, NOD2 ligation upregulated the coinhibitory PD-L1 expression on pDCs, resulting in its reduced ability to stimulate T cell proliferation (Castellaneta et al., 2009). pDCs promote tolerance in vivo by inducing energy deficiency or deletion of circulating T cells (Thomson and Knolle, 2010). Studies have shown that pDCs can induce the expression of IL-10 in Tregs, as well as release IFN-I (IFN-α/β) and IDO to attenuate the activation of allogeneic T cells (Cella et al., 1999; Munn et al., 2004; Ito et al., 2007). IDO has been shown to skew the development of naive CD4+ T cells toward the Treg lineage (Fallarino et al., 2006), which relies on cell-cell contact mediated mechanisms (Sharma et al., 2007). The expression of highly inducible co-stimulator ligand (ICOS-L) endows mature pDC with the ability to suppress effector T cells and increase IL-10-producing Treg cells (Campana et al., 2015).
5 Mo-DC in the liver transplantation immune response
Monocytes can be divided into two main types in mice and humans (Auffray et al., 2007; Yona et al., 2013; Guilliams et al., 2018): classical or inflammatory monocytes (Ly6ChiCX3CR1int in mice and CD14hiCD16- in human), nonclassical or patrolling monocytes (Ly6ClowCX3CR1hi in mice and CD14lowCD16+ in human). At steady state, classical monocytes are stored in the bone marrow and other extramedullary sites, such as the spleen (Wolf et al., 2019). They can maintain the stability of tissue-resident macrophages in multiple organs, as well as the conversion to nonclassical monocytes (Guilliams et al., 2018). During infection and inflammation, classical or inflammatory monocytes can immediately deploy to infected or injured tissues to control infection, limit inflammatory damage and initiate tissue repair by producing macrophages or Mo-DCs (Boyette et al., 2017; Wolf et al., 2019). Nonclassical or patrolling monocytes are characterized by patrolling the circulation, clearing cellular debris and repairing the endothelium in homeostasis (Hanna et al., 2011; Carlin et al., 2013).
Most of our knowledge about Mo-DC comes from infection models. Under inflammatory conditions, classical monocyte-derived DC can become a large population that complements the range of steady-state DCs (Serbina et al., 2003; Le Borgne et al., 2006; León et al., 2007). Mo-DCs upregulate CD11c and MHC-II, but generally retain expression of monocyte markers such as Ly6C, Ly6B, CD64 (León et al., 2007; Rosas et al., 2010; Plantinga et al., 2013). They mediate effector functions through the production of Th1 cytokines TNF-α and IL-12, as well as direct cytotoxicity through NO production (Serbina et al., 2003; León et al., 2007; Goldszmid et al., 2012; Plantinga et al., 2013). In addition, Mo-DCs have phagocytic activity and the ability to process antigens, participating in the initiation of naive T cells or the reactivation of antigen experienced T cells (Mildner et al., 2013). Furthermore, it has been found in some tumor studies that the presence of Mo-DC is associated with the activation of CD8+ T cells and treatment success in some tumors (Rich et al., 2012; Kuhn et al., 2013; Kuhn et al., 2015). Mo-DC based vaccination also plays a role in various malignant tumors (Frankenberger et al., 2005; Javed et al., 2016; Van den Bergh et al., 2017).
Unlike classical monocytes, which play a major role in the site of inflammation (Serbina et al., 2003), nonclassical monocytes play a major role in sterile inflammation (for instance, in atherosclerotic plaques (Tacke et al., 2007)). In organ transplantation, the synergistic effect of allogeneic non-self and sterile inflammation may trigger the differentiation of nonclassical monocytes into DCs (Zhuang et al., 2016), nonclassical monocyte-derived DCs may also arise in the context of transplanted organs and may be involved in inducing allograft rejection (Chow et al., 2016). In a study of heart and kidney transplantation in mice, it was found that donor DCs would be rapidly replaced by recipient DCs after transplantation. Recipient nonclassical monocyte derived DCs played an important role in rejection by promoting the proliferation and survival of effector T cells in the graft (Zhuang et al., 2016). In another experimental study in mice, Mo-DCs was rapidly recruited after experimental heart and kidney transplantation, allogeneic grafts elicited persistent differentiation of monocytes to mature DCs that express IL-12, stimulate T cell proliferation and IFN-γ production, and precipitate graft rejection (Oberbarnscheidt et al., 2014). Similarly, Zecher et al. injected allogeneic splenocytes into the ear pinnae of recombination activating gene (RAG)−/− mice, resulting in significantly greater skin swelling and infiltration by host bone marrow cells compared with injection of syngeneic splenocytes. This response is mediated by Gr-1 (Ly6C)int monocytes, suggesting that they are able to discriminate between self and nonself tissue to initiate effector responses (Zecher et al., 2009). In another study, it was also observed that intravenous injection of allogeneic splenocytes in mice could stimulate the rapid (within 1 day) accumulation of splenic Mo-DCs more than syngeneic splenocytes or nothing injection. These findings suggest that (Chow et al., 2016) Mo-DCs have a potentially important role in responding to allogeneic stimuli, such as those occurring in the context of organ transplantation. In addition, in mouse pancreas transplantation, Mo-DCs can impair the early graft function after islet allograft transplantation, and the graft function of recipients with Mo-DC removed is improved as early as the first day after transplantation, which strongly suggests that Mo-DCs can participate in the early injury of islet allografts (Chow et al., 2017). In an experimental study of bone marrow transplantation (Asiedu et al., 2002), non-human primate (NHP) mature Mo-DCs can be genetically engineered as an alloantigen specific cellular immunosuppressant, which has the potential to promote the induction of allograft tolerance in vivo. What’s more, In a recent study (Lee et al., 2021), due to the relatively simple culture process and more efficient Treg expansion capacity of stimulated mature Mo-DCs, they may be a viable alternative for producing alloreactive Tregs for clinical use. But until recently, experimental research on Mo-DC in liver transplantation was extremely rare. In a study of human liver transplantation (Shariat et al., 2014), researchers found that compared with healthy individuals, the gene expression of IL-12 and TLR2 secreted by Mo-DCs in liver transplant patients were significantly increased. Signaling through TLR2 can induce DCs to mature in a myeloid differentiation primary response 88 (MyD88) dependent or independent manner, ultimately leading to the release of proinflammatory cytokines (Chinen and Buckley, 2010). In addition, the increase of TLR2 and TLR4 levels can also be used for the early prediction of acute rejection after liver transplantation (Deng et al., 2007). IL-12 can induce the development and inflammatory process of Th1 cells in transplanted patients (Goriely and Goldman, 2008), which may contribute to the occurrence of liver transplantation rejection. Overall, although the role of Mo-DC in infection and inflammation has been increasingly understood, their special role in organ transplantation, especially in the case of liver transplantation, still needs more research to explore.
6 The latest progress of DC based treatment
The use of donor or host-derived tolerogenic dendritic cell (Tol-DC) or in situ targeted DC for cell therapy to promote its tolerance is an emerging method to reduce the use of systemic IS in transplant patients and promote donor-specific tolerance (Ochando and Braza, 2017; Marín et al., 2018). Tol-DC refers to some DCs that can inhibit immune responses, including immature DC (imDC), regulatory DC (DCreg), maturation resistant or alternatively activated DC (Morelli and Thomson, 2007). Tol-DCs are characterized by low expression of MHC-II and co-stimulatory molecules CD80/CD86 and CD40, high expression of anti-inflammatory cytokines such as IL-10 and transforming growth factor beta (TGF-β), and low expression of pro-inflammatory cytokines such as IL-12p70 (Maldonado and von Andrian, 2010; Marín et al., 2018). In addition, Tol-DCs also have low antigen-presenting function and participate in immune tolerance by inducing reactive T cell weakness and apoptosis (Choo et al., 2017; Spiering et al., 2019). Tol-DC has been extensively studied in preclinical models and is very effective in limiting autoimmune diseases (Hilkens et al., 2010) or allograft rejection in transplantation (Ochando et al., 2020; Chen et al., 2023).
Extensive studies in rodent and NHP models have shown that adoptively transferred regulatory immune cells can promote transplant tolerance. DCreg is an important candidate for adoptive cell therapy in organ transplantation (Moreau et al., 2017; Thomson et al., 2019), which can suppress T cell responses by inducing T cell weakness or apoptosis (Lu et al., 1997; Lu and Thomson, 2002; Zahorchak et al., 2018). What’s more, DCregs also have the potential to retain, expand or induce Treg (Morelli and Thomson, 2007; Huang et al., 2010; Raker et al., 2015). DCregs were generated in granulocyte-macrophage colony-stimulating factor (GM-CSF) and IL-4 from rodent and human bone marrow precursor cells or human circulating blood monocytes in vitro (Zahorchak et al., 2023). In the culture of DCreg, one or more agents, cytokines or growth factors or genetic engineering (Bonham et al., 2002) methods are added to inhibit DC maturation and enhance its tolerance (Hackstein and Thomson, 2004). These agents include immunomodulatory drugs [such as vitamin D3 (VitD3), corticosteroids, rapamycin, cyclosporine, tacrolimus, and aspirin], cytokines or growth factors [such as hepatocyte growth factor (HGF), vasoactive intestinal peptide (VIP), thymic stromal lymphopoietin, PGE2, IL-10, and TGF- β] (Raker et al., 2015; Thomson et al., 2019). However, there is still no consensus on the best protocol for generating clinical-grade human DCreg (Navarro-Barriuso et al., 2018; Thomson et al., 2019). DCreg infusion improves the incidence of safe withdrawal of IS and operational tolerance in human liver transplantation (Thomson et al., 2019). The safety, feasibility and potential efficacy of autologous DCreg infusion has been confirmed in the treatment of various diseases, including type-1 diabetes, rheumatoid arthritis, Crohn’s colitis, multiple sclerosis (Giannoukakis et al., 2011; Jauregui-Amezaga et al., 2015; Bell et al., 2017; Zubizarreta et al., 2019). Studies have described the rationale for the first human trial of donor-derived DCreg injected before living donor liver transplantation (LDLT) in combination with triple IS (steroid hormones, mycophenolate mofetil and tacrolimus) to promote operational tolerance (Zahorchak et al., 2018). In another study (Macedo et al., 2021), infusion of donor monocyte-derived DCregs in the first 7 days of LDLT can induce changes in host APC, memory CD8+T cell and Treg, which may be beneficial to regulate immune reactivity during transplantation, thereby promoting operational tolerance. In the latest study of manufacturing GMP grade DCreg for 27 liver transplant recipients (Zahorchak et al., 2023), under GMP conditions, circulating monocytes can easily produce high-purity DCreg that meets a series of quality standards to attain the target cell number injected into potential organ transplant recipients (Figure 2). Two clinical trials of DCreg in liver transplantation (NCT03164265 and NCT04208919) are ongoing to evaluate the safety and efficacy of donor derived DCreg in LDLT through a single infusion. The registered clinical trials targeting DC in transplantation are shown in Table 2.
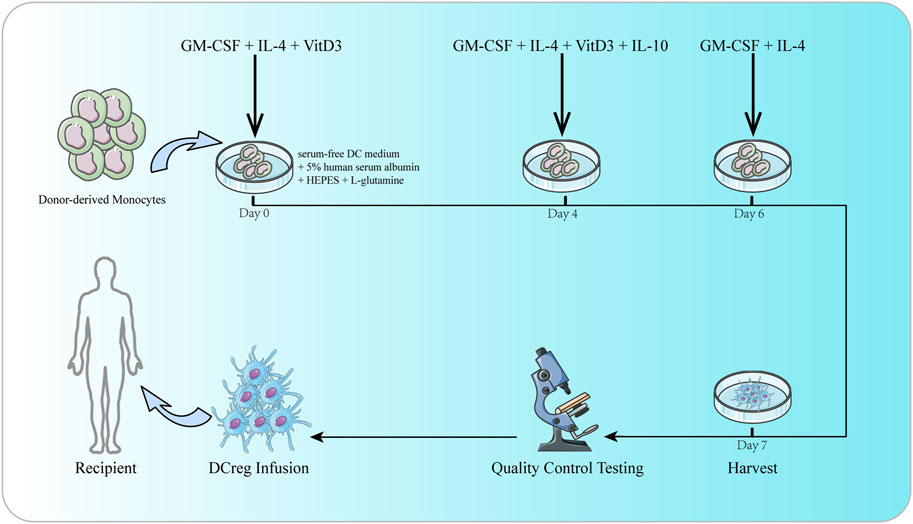
FIGURE 2. The culture of DCreg. Donor-derived monocytes are resuspended in DC culture media consisting of serum-free DC medium, 5% human serum albumin, HEPES, and L-glutamine. GM-CSF, IL-4 and VitD3 are added on days 0 and 4. IL-10 is also added on day 4. On day 6, culture media is replenished with DC media supplemented with GM-CSF and IL-4. DCreg products are harvested on day 7. Before infusion, DCreg products are subjected to rigorous quality control testing to ensure purity, sterility, yield and viability.
What’s more, a recent study have used the optimization of physical and chemical properties of nanoparticles to design DC targeted nanomedicines for immune tolerance in organ transplantation and autoimmune diseases (Cifuentes-Rius et al., 2021). Targeting specific receptors on DC using nanomedicines not only helps to enhance absorption, but also has the ability of antigen cross presentation, thereby promoting peripheral tolerance. Despite its great potential, this method is still in its infancy, and its persistence, safety, and complexity related to the immune system and multiple mechanisms may limit the development of this method.
7 Conclusion and future prospects
In this article, we described the subgroups, development, phenotype, migration and function of DC, and the roles of these DC subsets, including cDC, pDC, and Mo-DC in liver transplant rejection and tolerance. During rejection, cDC plays an important role in the initiation of immune rejection by presenting donor antigens. pDC, as a weaker APC, cannot be overlooked as they also enhance the stimulation ability of allogeneic T cells by expressing MHC-II molecules and costimulatory molecules. Now, increasingly evidence suggested the Mo-DC is the major mediator to activate alloreactive T cell in the graft, but their role in liver transplantation is not fully revealed. In the future, more effort is needed to explore the role of Mo-DC in alloresponse in the liver allograft.
By adopting an immature phenotype, both cDC and pDC can promote T cell apoptosis and generate Tregs to promote immune tolerance. Adoptive transfer of the DCreg or Tol-DC exhibiting immature phenotype induces tolerance, but the best clinical grade DCreg or Tol-DC production scheme is still needed.
In conclusion, these discussions provide useful resources for better understanding the biology of DC and improving the immune adaptability of transplant patients by manipulating DC.
Author contributions
XD: Writing–original draft. ML: Writing–original draft, Writing–review and editing. CH: Writing–review and editing. GL: Writing–review and editing.
Funding
The author(s) declare financial support was received for the research, authorship, and/or publication of this article. Financial support was provided by the National Natural Science Foundation of China (U20A20360 and 81901627), Science and Technology Department of Jilin Province (YDZJ202201ZYTS678).
Acknowledgments
We thank the financial support by the National Natural Science Foundation of China (U20A20360 and 81901627), Science and Technology Department of Jilin Province (YDZJ202201ZYTS678).
Conflict of interest
The authors declare that the research was conducted in the absence of any commercial or financial relationships that could be construed as a potential conflict of interest.
Publisher’s note
All claims expressed in this article are solely those of the authors and do not necessarily represent those of their affiliated organizations, or those of the publisher, the editors and the reviewers. Any product that may be evaluated in this article, or claim that may be made by its manufacturer, is not guaranteed or endorsed by the publisher.
Supplementary material
The Supplementary Material for this article can be found online at: https://www.frontiersin.org/articles/10.3389/fcell.2023.1277743/full#supplementary-material
References
Abe, M., Tokita, D., Raimondi, G., and Thomson, A. W. (2006). Endotoxin modulates the capacity of CpG-activated liver myeloid DC to direct Th1-type responses. Eur. J. Immunol. 36 (9), 2483–2493. doi:10.1002/eji.200535767
Anderson, D. A., Murphy, K. M., and Briseño, C. G. (2018). Development, Diversity, and Function of Dendritic Cells in Mouse and Human. Cold Spring Harb. Perspect. Biol. 10 (11), a028613. doi:10.1101/cshperspect.a028613
Asiedu, C., Dong, S. S., Pereboev, A., Wang, W., Navarro, J., Curiel, D. T., et al. (2002). Rhesus monocyte-derived dendritic cells modified to over-express TGF-beta1 exhibit potent veto activity. Transplantation 74 (5), 629–637. doi:10.1097/00007890-200209150-00008
Auffray, C., Fogg, D., Garfa, M., Elain, G., Join-Lambert, O., Kayal, S., et al. (2007). Monitoring of blood vessels and tissues by a population of monocytes with patrolling behavior. Sci. (New York, N.Y.) 317 (5838), 666–670. doi:10.1126/science.1142883
Bachem, A., Güttler, S., Hartung, E., Ebstein, F., Schaefer, M., Tannert, A., et al. (2010). Superior antigen cross-presentation and XCR1 expression define human CD11c+CD141+ cells as homologues of mouse CD8+ dendritic cells. J. Exp. Med. 207 (6), 1273–1281. doi:10.1084/jem.20100348
Bamboat, Z. M., Stableford, J. A., Plitas, G., Burt, B. M., Nguyen, H. M., Welles, A. P., et al. (2009). Human liver dendritic cells promote T cell hyporesponsiveness. J. Immunol. 182 (4), 1901–1911. doi:10.4049/jimmunol.0803404
Baroja-Mazo, A., Revilla-Nuin, B., Parrilla, P., Martínez-Alarcón, L., Ramírez, P., and Pons, J. A. (2016). Tolerance in liver transplantation: biomarkers and clinical relevance. World J. Gastroenterology 22 (34), 7676–7691. doi:10.3748/wjg.v22.i34.7676
Bell, G. M., Anderson, A. E., Diboll, J., Reece, R., Eltherington, O., Harry, R. A., et al. (2017). Autologous tolerogenic dendritic cells for rheumatoid and inflammatory arthritis. Ann. Rheumatic Dis. 76 (1), 227–234. doi:10.1136/annrheumdis-2015-208456
Bhatt, S., Qin, J., Bennett, C., Qian, S., Fung, J. J., Hamilton, T. A., et al. (2014). All-trans retinoic acid induces arginase-1 and inducible nitric oxide synthase-producing dendritic cells with T cell inhibitory function. J. Immunol. 192 (11), 5098–5108. doi:10.4049/jimmunol.1303073
Binder, C., Cvetkovski, F., Sellberg, F., Berg, S., Paternina Visbal, H., Sachs, D. H., et al. (2020). CD2 Immunobiology. Front. Immunol. 11, 1090. doi:10.3389/fimmu.2020.01090
Björck, P., Coates, P. T. H., Wang, Z., Duncan, F. J., and Thomson, A. W. (2005). Promotion of long-term heart allograft survival by combination of mobilized donor plasmacytoid dendritic cells and anti-CD154 monoclonal antibody. J. Heart Lung Transplant. 24 (8), 1118–1120. doi:10.1016/j.healun.2004.10.007
Bonham, C. A., Peng, L., Liang, X., Chen, Z., Wang, L., Ma, L., et al. (2002). Marked prolongation of cardiac allograft survival by dendritic cells genetically engineered with NF-kappa B oligodeoxyribonucleotide decoys and adenoviral vectors encoding CTLA4-Ig. J. Immunol. 169 (6), 3382–3391. doi:10.4049/jimmunol.169.6.3382
Bošnjak, B., Do, K. T. H., Förster, R., and Hammerschmidt, S. I. (2022). Imaging dendritic cell functions. Immunol. Rev. 306 (1), 137–163. doi:10.1111/imr.13050
Boulet, S., Daudelin, J.-F., Odagiu, L., Pelletier, A.-N., Yun, T. J., Lesage, S., et al. (2019). The orphan nuclear receptor NR4A3 controls the differentiation of monocyte-derived dendritic cells following microbial stimulation. Proc. Natl. Acad. Sci. U. S. A. 116 (30), 15150–15159. doi:10.1073/pnas.1821296116
Boyette, L. B., Macedo, C., Hadi, K., Elinoff, B. D., Walters, J. T., Ramaswami, B., et al. (2017). Phenotype, function, and differentiation potential of human monocyte subsets. PloS One 12 (4), e0176460. doi:10.1371/journal.pone.0176460
Braun, A., Worbs, T., Moschovakis, G. L., Halle, S., Hoffmann, K., Bölter, J., et al. (2011). Afferent lymph-derived T cells and DCs use different chemokine receptor CCR7-dependent routes for entry into the lymph node and intranodal migration. Nat. Immunol. 12 (9), 879–887. doi:10.1038/ni.2085
Brewitz, A., Eickhoff, S., Dähling, S., Quast, T., Bedoui, S., Kroczek, R. A., et al. (2017). CD8+ T Cells Orchestrate pDC-XCR1+ Dendritic Cell Spatial and Functional Cooperativity to Optimize Priming. Immunity 46 (2), 205–219. doi:10.1016/j.immuni.2017.01.003
Briseño, C. G., Haldar, M., Kretzer, N. M., Wu, X., Theisen, D. J., Kc, W., et al. (2016). Distinct Transcriptional Programs Control Cross-Priming in Classical and Monocyte-Derived Dendritic Cells. Cell Rep. 15 (11), 2462–2474. doi:10.1016/j.celrep.2016.05.025
Bromley, S. K., and Dustin, M. L. (2002). Stimulation of naïve T-cell adhesion and immunological synapse formation by chemokine-dependent and -independent mechanisms. Immunology 106 (3), 289–298. doi:10.1046/j.1365-2567.2002.01441.x
Cabeza-Cabrerizo, M., Cardoso, A., Minutti, C. M., Pereira da Costa, M., and Reis e Sousa, C. (2021). Dendritic Cells Revisited. Annu. Rev. Immunol. 39, 131–166. doi:10.1146/annurev-immunol-061020-053707
Campana, S., De Pasquale, C., Carrega, P., Ferlazzo, G., and Bonaccorsi, I. (2015). Cross-dressing: an alternative mechanism for antigen presentation. Immunol. Lett. 168 (2), 349–354. doi:10.1016/j.imlet.2015.11.002
Carlin, L. M., Stamatiades, E. G., Auffray, C., Hanna, R. N., Glover, L., Vizcay-Barrena, G., et al. (2013). Nr4a1-dependent Ly6C(low) monocytes monitor endothelial cells and orchestrate their disposal. Cell 153 (2), 362–375. doi:10.1016/j.cell.2013.03.010
Castellaneta, A., Sumpter, T. L., Chen, L., Tokita, D., and Thomson, A. W. (2009). NOD2 ligation subverts IFN-alpha production by liver plasmacytoid dendritic cells and inhibits their T cell allostimulatory activity via B7-H1 up-regulation. J. Immunol. 183 (11), 6922–6932. doi:10.4049/jimmunol.0900582
Cella, M., Jarrossay, D., Facchetti, F., Alebardi, O., Nakajima, H., Lanzavecchia, A., et al. (1999). Plasmacytoid monocytes migrate to inflamed lymph nodes and produce large amounts of type I interferon. Nat. Med. 5 (8), 919–923. doi:10.1038/11360
Cervantes-Barragan, L., Lewis, K. L., Firner, S., Thiel, V., Hugues, S., Reith, W., et al. (2012). Plasmacytoid dendritic cells control T-cell response to chronic viral infection. Proc. Natl. Acad. Sci. U. S. A. 109 (8), 3012–3017. doi:10.1073/pnas.1117359109
Chen, J., Cao, Y., Jia, O., Wang, X., Luo, Y., Cheuk, Y. C., et al. (2023). Monomethyl fumarate prevents alloimmune rejection in mouse heart transplantation by inducing tolerogenic dendritic cells. Acta Biochimica Biophysica Sinica 55 (5), 866–877. doi:10.3724/abbs.2023088
Chinen, J., and Buckley, R. H. (2010). Transplantation immunology: solid organ and bone marrow. J. Allergy Clin. Immunol. 125 (2), S324–S335. doi:10.1016/j.jaci.2009.11.014
Choo, E. H., Lee, J.-H., Park, E.-H., Park, H. E., Jung, N.-C., Kim, T.-H., et al. (2017). Infarcted Myocardium-Primed Dendritic Cells Improve Remodeling and Cardiac Function After Myocardial Infarction by Modulating the Regulatory T Cell and Macrophage Polarization. Circulation 135 (15), 1444–1457. doi:10.1161/CIRCULATIONAHA.116.023106
Chow, K. V., Carrington, E. M., Zhan, Y., Lew, A. M., and Sutherland, R. M. (2017). Monocyte-Derived Dendritic Cells Impair Early Graft Function Following Allogeneic Islet Transplantation. Cell Transplant. 26 (2), 319–326. doi:10.3727/096368916X693482
Chow, K. V., Delconte, R. B., Huntington, N. D., Tarlinton, D. M., Sutherland, R. M., Zhan, Y., et al. (2016). Innate Allorecognition Results in Rapid Accumulation of Monocyte-Derived Dendritic Cells. J. Immunol. 197 (5), 2000–2008. doi:10.4049/jimmunol.1600181
Cifuentes-Rius, A., Desai, A., Yuen, D., Johnston, A. P. R., and Voelcker, N. H. (2021). Inducing immune tolerance with dendritic cell-targeting nanomedicines. Nat. Nanotechnol. 16 (1), 37–46. doi:10.1038/s41565-020-00810-2
Cisse, B., Caton, M. L., Lehner, M., Maeda, T., Scheu, S., Locksley, R., et al. (2008). Transcription factor E2-2 is an essential and specific regulator of plasmacytoid dendritic cell development. Cell 135 (1), 37–48. doi:10.1016/j.cell.2008.09.016
Clavien, P.-A., Muller, X., de Oliveira, M. L., Dutkowski, P., and Sanchez-Fueyo, A. (2017). Can immunosuppression be stopped after liver transplantation? Lancet. Gastroenterology Hepatology 2 (7), 531–537. doi:10.1016/S2468-1253(16)30208-4
Colombo, M., Raposo, G., and Théry, C. (2014). Biogenesis, secretion, and intercellular interactions of exosomes and other extracellular vesicles. Annu. Rev. Cell Dev. Biol. 30, 255–289. doi:10.1146/annurev-cellbio-101512-122326
Colonna, M., Trinchieri, G., and Liu, Y.-J. (2004). Plasmacytoid dendritic cells in immunity. Nat. Immunol. 5 (12), 1219–1226. doi:10.1038/ni1141
Colvin, B. L., Matta, B. M., and Thomson, A. W. (2008). Dendritic cells and chemokine-directed migration in transplantation: where are we headed? Clin. Laboratory Med. 28 (3), 375–384. doi:10.1016/j.cll.2008.07.003
Corse, E., Gottschalk, R. A., and Allison, J. P. (2011). Strength of TCR-peptide/MHC interactions and in vivo T cell responses. J. Immunol. 186 (9), 5039–5045. doi:10.4049/jimmunol.1003650
Crozat, K., Guiton, R., Contreras, V., Feuillet, V., Dutertre, C.-A., Ventre, E., et al. (2010). The XC chemokine receptor 1 is a conserved selective marker of mammalian cells homologous to mouse CD8alpha+ dendritic cells. J. Exp. Med. 207 (6), 1283–1292. doi:10.1084/jem.20100223
De Creus, A., Abe, M., Lau, A. H., Hackstein, H., Raimondi, G., and Thomson, A. W. (2005). Low TLR4 expression by liver dendritic cells correlates with reduced capacity to activate allogeneic T cells in response to endotoxin. J. Immunol. 174 (4), 2037–2045. doi:10.4049/jimmunol.174.4.2037
Demetris, A. J., Bellamy, C. O. C., Gandhi, C. R., Prost, S., Nakanuma, Y., and Stolz, D. B. (2016). Functional Immune Anatomy of the Liver-As an Allograft. Am. J. Transplant. 16 (6), 1653–1680. doi:10.1111/ajt.13749
Deng, J. F., Geng, L., Qian, Y. G., Li, H., Wang, Y., Xie, H. Y., et al. (2007). The role of toll-like receptors 2 and 4 in acute allograft rejection after liver transplantation. Transplant. Proc. 39 (10), 3222–3224. doi:10.1016/j.transproceed.2007.02.102
Drakes, M. L., Lu, L., Subbotin, V. M., and Thomson, A. W. (1997). In vivo administration of flt3 ligand markedly stimulates generation of dendritic cell progenitors from mouse liver. J. Immunol. 159 (9), 4268–4278. doi:10.4049/jimmunol.159.9.4268
Duneton, C., Winterberg, P. D., and Ford, M. L. (2022). Activation and regulation of alloreactive T cell immunity in solid organ transplantation. Nat. Rev. Nephrol. 18 (10), 663–676. doi:10.1038/s41581-022-00600-0
Durai, V., and Murphy, K. M. (2016). Functions of Murine Dendritic Cells. Immunity 45 (4), 719–736. doi:10.1016/j.immuni.2016.10.010
Eckert, C., Klein, N., Kornek, M., and Lukacs-Kornek, V. (2015). The complex myeloid network of the liver with diverse functional capacity at steady state and in inflammation. Front. Immunol. 6, 179. doi:10.3389/fimmu.2015.00179
Edelson, B. T., Kc, W., Juang, R., Kohyama, M., Benoit, L. A., Klekotka, P. A., et al. (2010). Peripheral CD103+ dendritic cells form a unified subset developmentally related to CD8alpha+ conventional dendritic cells. J. Exp. Med. 207 (4), 823–836. doi:10.1084/jem.20091627
Fallarino, F., Grohmann, U., You, S., McGrath, B. C., Cavener, D. R., Vacca, C., et al. (2006). The combined effects of tryptophan starvation and tryptophan catabolites down-regulate T cell receptor zeta-chain and induce a regulatory phenotype in naive T cells. J. Immunol. 176 (11), 6752–6761. doi:10.4049/jimmunol.176.11.6752
Fancke, B., Suter, M., Hochrein, H., and O'Keeffe, M. (2008). M-CSF: a novel plasmacytoid and conventional dendritic cell poietin. Blood 111 (1), 150–159. doi:10.1182/blood-2007-05-089292
Favre, D., Mold, J., Hunt, P. W., Kanwar, B., Loke, P. n., Seu, L., et al. (2010). Tryptophan catabolism by indoleamine 2,3-dioxygenase 1 alters the balance of TH17 to regulatory T cells in HIV disease. Sci. Transl. Med. 2 (32), 32ra36. doi:10.1126/scitranslmed.3000632
Fogg, D. K., Sibon, C., Miled, C., Jung, S., Aucouturier, P., Littman, D. R., et al. (2006). A clonogenic bone marrow progenitor specific for macrophages and dendritic cells. Sci. (New York, N.Y.) 311 (5757), 83–87. doi:10.1126/science.1117729
Fonteneau, J.-F., Gilliet, M., Larsson, M., Dasilva, I., Münz, C., Liu, Y.-J., et al. (2003). Activation of influenza virus-specific CD4+ and CD8+ T cells: a new role for plasmacytoid dendritic cells in adaptive immunity. Blood 101 (9), 3520–3526. doi:10.1182/blood-2002-10-3063
Förster, R., Schubel, A., Breitfeld, D., Kremmer, E., Renner-Müller, I., Wolf, E., et al. (1999). CCR7 coordinates the primary immune response by establishing functional microenvironments in secondary lymphoid organs. Cell 99 (1), 23–33. doi:10.1016/s0092-8674(00)80059-8
Frankenberger, B., Regn, S., Geiger, C., Noessner, E., Falk, C. S., Pohla, H., et al. (2005). Cell-based vaccines for renal cell carcinoma: genetically-engineered tumor cells and monocyte-derived dendritic cells. World J. Urology 23 (3), 166–174. doi:10.1007/s00345-005-0505-5
Friedman, R. S., Jacobelli, J., and Krummel, M. F. (2006). Surface-bound chemokines capture and prime T cells for synapse formation. Nat. Immunol. 7 (10), 1101–1108. doi:10.1038/ni1384
Geissmann, F., Jung, S., and Littman, D. R. (2003). Blood monocytes consist of two principal subsets with distinct migratory properties. Immunity 19 (1), 71–82. doi:10.1016/s1074-7613(03)00174-2
Giannoukakis, N., Phillips, B., Finegold, D., Harnaha, J., and Trucco, M. (2011). Phase I (safety) study of autologous tolerogenic dendritic cells in type 1 diabetic patients. Diabetes Care 34 (9), 2026–2032. doi:10.2337/dc11-0472
Goldszmid, R. S., Caspar, P., Rivollier, A., White, S., Dzutsev, A., Hieny, S., et al. (2012). NK cell-derived interferon-γ orchestrates cellular dynamics and the differentiation of monocytes into dendritic cells at the site of infection. Immunity 36 (6), 1047–1059. doi:10.1016/j.immuni.2012.03.026
Goriely, S., and Goldman, M. (2008). Interleukin-12 family members and the balance between rejection and tolerance. Curr. Opin. Organ Transplant. 13 (1), 4–9. doi:10.1097/MOT.0b013e3282f406c4
Goudot, C., Coillard, A., Villani, A.-C., Gueguen, P., Cros, A., Sarkizova, S., et al. (2017). Aryl Hydrocarbon Receptor Controls Monocyte Differentiation into Dendritic Cells versus Macrophages. Immunity 47 (3), 582–596. doi:10.1016/j.immuni.2017.08.016
Grajkowska, L. T., Ceribelli, M., Lau, C. M., Warren, M. E., Tiniakou, I., Nakandakari Higa, S., et al. (2017). Isoform-Specific Expression and Feedback Regulation of E Protein TCF4 Control Dendritic Cell Lineage Specification. Immunity 46 (1), 65–77. doi:10.1016/j.immuni.2016.11.006
Guilliams, M., Dutertre, C.-A., Scott, C. L., McGovern, N., Sichien, D., Chakarov, S., et al. (2016). Unsupervised High-Dimensional Analysis Aligns Dendritic Cells across Tissues and Species. Immunity 45 (3), 669–684. doi:10.1016/j.immuni.2016.08.015
Guilliams, M., Ginhoux, F., Jakubzick, C., Naik, S. H., Onai, N., Schraml, B. U., et al. (2014). Dendritic cells, monocytes and macrophages: a unified nomenclature based on ontogeny. Nat. Rev. Immunol. 14 (8), 571–578. doi:10.1038/nri3712
Guilliams, M., Mildner, A., and Yona, S. (2018). Developmental and Functional Heterogeneity of Monocytes. Immunity 49 (4), 595–613. doi:10.1016/j.immuni.2018.10.005
Hackstein, H., and Thomson, A. W. (2004). Dendritic cells: emerging pharmacological targets of immunosuppressive drugs. Nat. Rev. Immunol. 4 (1), 24–34. doi:10.1038/nri1256
Haniffa, M., Shin, A., Bigley, V., McGovern, N., Teo, P., See, P., et al. (2012). Human tissues contain CD141hi cross-presenting dendritic cells with functional homology to mouse CD103+ nonlymphoid dendritic cells. Immunity 37 (1), 60–73. doi:10.1016/j.immuni.2012.04.012
Hanna, R. N., Carlin, L. M., Hubbeling, H. G., Nackiewicz, D., Green, A. M., Punt, J. A., et al. (2011). The transcription factor NR4A1 (Nur77) controls bone marrow differentiation and the survival of Ly6C- monocytes. Nat. Immunol. 12 (8), 778–785. doi:10.1038/ni.2063
Hettinger, J., Richards, D. M., Hansson, J., Barra, M. M., Joschko, A.-C., Krijgsveld, J., et al. (2013). Origin of monocytes and macrophages in a committed progenitor. Nat. Immunol. 14 (8), 821–830. doi:10.1038/ni.2638
Higashitani, K., Kanto, T., Kuroda, S., Yoshio, S., Matsubara, T., Kakita, N., et al. (2013). Association of enhanced activity of indoleamine 2,3-dioxygenase in dendritic cells with the induction of regulatory T cells in chronic hepatitis C infection. J. Gastroenterology 48 (5), 660–670. doi:10.1007/s00535-012-0667-z
Hilkens, C. M. U., Isaacs, J. D., and Thomson, A. W. (2010). Development of dendritic cell-based immunotherapy for autoimmunity. Int. Rev. Immunol. 29 (2), 156–183. doi:10.3109/08830180903281193
Hsu, W., Shu, S.-A., Gershwin, E., and Lian, Z.-X. (2007). The current immune function of hepatic dendritic cells. Cell. Mol. Immunol. 4 (5), 321–328.
Huang, H., Dawicki, W., Zhang, X., Town, J., and Gordon, J. R. (2010). Tolerogenic dendritic cells induce CD4+CD25hiFoxp3+ regulatory T cell differentiation from CD4+CD25-/loFoxp3-effector T cells. J. Immunol. 185 (9), 5003–5010. doi:10.4049/jimmunol.0903446
Hughes, A. D., Zhao, D., Dai, H., Abou-Daya, K. I., Tieu, R., Rammal, R., et al. (2020). Cross-dressed dendritic cells sustain effector T cell responses in islet and kidney allografts. J. Clin. Investigation 130 (1), 287–294. doi:10.1172/JCI125773
Iizasa, H., Yoneyama, H., Mukaida, N., Katakoka, Y., Naito, M., Yoshida, N., et al. (2005). Exacerbation of granuloma formation in IL-1 receptor antagonist-deficient mice with impaired dendritic cell maturation associated with Th2 cytokine production. J. Immunol. 174 (6), 3273–3280. doi:10.4049/jimmunol.174.6.3273
Ito, T., Yang, M., Wang, Y.-H., Lande, R., Gregorio, J., Perng, O. A., et al. (2007). Plasmacytoid dendritic cells prime IL-10-producing T regulatory cells by inducible costimulator ligand. J. Exp. Med. 204 (1), 105–115. doi:10.1084/jem.20061660
Jauregui-Amezaga, A., Cabezón, R., Ramírez-Morros, A., España, C., Rimola, J., Bru, C., et al. (2015). Intraperitoneal Administration of Autologous Tolerogenic Dendritic Cells for Refractory Crohn's Disease: A Phase I Study. J. Crohn's Colitis 9 (12), 1071–1078. doi:10.1093/ecco-jcc/jjv144
Javed, A., Sato, S., and Sato, T. (2016). Autologous melanoma cell vaccine using monocyte-derived dendritic cells (NBS20/eltrapuldencel-T). Future Oncol. Lond. Engl. 12 (6), 751–762. doi:10.2217/fon.16.13
Johnson, L. A., Banerji, S., Lagerholm, B. C., and Jackson, D. G. (2021). Dendritic cell entry to lymphatic capillaries is orchestrated by CD44 and the hyaluronan glycocalyx. Life Sci. Alliance 4 (5), e202000908. doi:10.26508/lsa.202000908
Johnson, L. A., Banerji, S., Lawrance, W., Gileadi, U., Prota, G., Holder, K. A., et al. (2017). Dendritic cells enter lymph vessels by hyaluronan-mediated docking to the endothelial receptor LYVE-1. Nat. Immunol. 18 (7), 762–770. doi:10.1038/ni.3750
Jomantaite, I., Dikopoulos, N., Kröger, A., Leithäuser, F., Hauser, H., Schirmbeck, R., et al. (2004). Hepatic dendritic cell subsets in the mouse. Eur. J. Immunol. 34 (2), 355–365. doi:10.1002/eji.200324336
Khanna, A., Morelli, A. E., Zhong, C., Takayama, T., Lu, L., and Thomson, A. W. (2000). Effects of liver-derived dendritic cell progenitors on Th1- and Th2-like cytokine responses in vitro and in vivo. J. Immunol. 164 (3), 1346–1354. doi:10.4049/jimmunol.164.3.1346
Kingham, T. P., Chaudhry, U. I., Plitas, G., Katz, S. C., Raab, J., and DeMatteo, R. P. (2007). Murine liver plasmacytoid dendritic cells become potent immunostimulatory cells after Flt-3 ligand expansion. Hepatol. Baltim. Md 45 (2), 445–454. doi:10.1002/hep.21457
Koyama, M., Hashimoto, D., Aoyama, K., Matsuoka, K.-i., Karube, K., Niiro, H., et al. (2009). Plasmacytoid dendritic cells prime alloreactive T cells to mediate graft-versus-host disease as antigen-presenting cells. Blood 113 (9), 2088–2095. doi:10.1182/blood-2008-07-168609
Kudo, S., Matsuno, K., Ezaki, T., and Ogawa, M. (1997). A novel migration pathway for rat dendritic cells from the blood: hepatic sinusoids-lymph translocation. J. Exp. Med. 185 (4), 777–784. doi:10.1084/jem.185.4.777
Kuhn, S., Hyde, E. J., Yang, J., Rich, F. J., Harper, J. L., Kirman, J. R., et al. (2013). Increased numbers of monocyte-derived dendritic cells during successful tumor immunotherapy with immune-activating agents. J. Immunol. 191 (4), 1984–1992. doi:10.4049/jimmunol.1301135
Kuhn, S., Yang, J., and Ronchese, F. (2015). Monocyte-Derived Dendritic Cells Are Essential for CD8(+) T Cell Activation and Antitumor Responses After Local Immunotherapy. Front. Immunol. 6, 584. doi:10.3389/fimmu.2015.00584
Lämmermann, T., Bader, B. L., Monkley, S. J., Worbs, T., Wedlich-Söldner, R., Hirsch, K., et al. (2008). Rapid leukocyte migration by integrin-independent flowing and squeezing. Nature 453 (7191), 51–55. doi:10.1038/nature06887
LaRosa, D. F., Rahman, A. H., and Turka, L. A. (2007). The innate immune system in allograft rejection and tolerance. J. Immunol. 178 (12), 7503–7509. doi:10.4049/jimmunol.178.12.7503
Le Borgne, M., Etchart, N., Goubier, A., Lira, S. A., Sirard, J. C., van Rooijen, N., et al. (2006). Dendritic cells rapidly recruited into epithelial tissues via CCR6/CCL20 are responsible for CD8+ T cell crosspriming in vivo. Immunity 24 (2), 191–201. doi:10.1016/j.immuni.2006.01.005
Lechler, R., Ng, W. F., and Steinman, R. M. (2001). Dendritic cells in transplantation--friend or foe? Immunity 14 (4), 357–368. doi:10.1016/s1074-7613(01)00116-9
Lee, L. M., Zhang, H., Lee, K., Liang, H., Merleev, A., Vincenti, F., et al. (2021). A Comparison of Ex Vivo Expanded Human Regulatory T Cells Using Allogeneic Stimulated B Cells or Monocyte-Derived Dendritic Cells. Front. Immunol. 12, 679675. doi:10.3389/fimmu.2021.679675
Lehtonen, A., Veckman, V., Nikula, T., Lahesmaa, R., Kinnunen, L., Matikainen, S., et al. (2005). Differential expression of IFN regulatory factor 4 gene in human monocyte-derived dendritic cells and macrophages. J. Immunol. 175 (10), 6570–6579. doi:10.4049/jimmunol.175.10.6570
León, B., López-Bravo, M., and Ardavín, C. (2007). Monocyte-derived dendritic cells formed at the infection site control the induction of protective T helper 1 responses against Leishmania. Immunity 26 (4), 519–531. doi:10.1016/j.immuni.2007.01.017
Levitsky, J., Goldberg, D., Smith, A. R., Mansfield, S. A., Gillespie, B. W., Merion, R. M., et al. (2017). Acute Rejection Increases Risk of Graft Failure and Death in Recent Liver Transplant Recipients. Clin. Gastroenterology Hepatology 15 (4), 584–593. doi:10.1016/j.cgh.2016.07.035
Lewis, K. L., Caton, M. L., Bogunovic, M., Greter, M., Grajkowska, L. T., Ng, D., et al. (2011). Notch2 receptor signaling controls functional differentiation of dendritic cells in the spleen and intestine. Immunity 35 (5), 780–791. doi:10.1016/j.immuni.2011.08.013
Liu, Q., Rojas-Canales, D. M., Divito, S. J., Shufesky, W. J., Stolz, D. B., Erdos, G., et al. (2016). Donor dendritic cell-derived exosomes promote allograft-targeting immune response. J. Clin. Investigation 126 (8), 2805–2820. doi:10.1172/JCI84577
Liu, Y.-J. (2005). IPC: professional type 1 interferon-producing cells and plasmacytoid dendritic cell precursors. Annu. Rev. Immunol. 23, 275–306. doi:10.1146/annurev.immunol.23.021704.115633
Lu, L., Li, W., Zhong, C., Qian, S., Fung, J. J., Thomson, A. W., et al. (1999). Increased apoptosis of immunoreactive host cells and augmented donor leukocyte chimerism, not sustained inhibition of B7 molecule expression are associated with prolonged cardiac allograft survival in mice preconditioned with immature donor dendritic cells plus anti-CD40L mAb. Transplantation 68 (6), 747–757. doi:10.1097/00007890-199909270-00006
Lu, L., Qian, S., Hershberger, P. A., Rudert, W. A., Lynch, D. H., and Thomson, A. W. (1997). Fas ligand (CD95L) and B7 expression on dendritic cells provide counter-regulatory signals for T cell survival and proliferation. J. Immunol. 158 (12), 5676–5684. doi:10.4049/jimmunol.158.12.5676
Lu, L., and Thomson, A. W. (2002). Manipulation of dendritic cells for tolerance induction in transplantation and autoimmune disease. Transplantation 73 (1), S19–S22. doi:10.1097/00007890-200201151-00008
Lu, L., Woo, J., Rao, A. S., Li, Y., Watkins, S. C., Qian, S., et al. (1994). Propagation of dendritic cell progenitors from normal mouse liver using granulocyte/macrophage colony-stimulating factor and their maturational development in the presence of type-1 collagen. J. Exp. Med. 179 (6), 1823–1834. doi:10.1084/jem.179.6.1823
Lukacs-Kornek, V., and Schuppan, D. (2013). Dendritic cells in liver injury and fibrosis: shortcomings and promises. J. Hepatology 59 (5), 1124–1126. doi:10.1016/j.jhep.2013.05.033
Lutz, M. B., and Schuler, G. (2002). Immature, semi-mature and fully mature dendritic cells: which signals induce tolerance or immunity? Trends Immunol. 23 (9), 445–449. doi:10.1016/s1471-4906(02)02281-0
Ma, B., Yang, J.-Y., Song, W.-J., Ding, R., Zhang, Z.-C., Ji, H.-C., et al. (2016). Combining Exosomes Derived from Immature DCs with Donor Antigen-Specific Treg Cells Induces Tolerance in a Rat Liver Allograft Model. Sci. Rep. 6, 32971. doi:10.1038/srep32971
Macedo, C., Tran, L. M., Zahorchak, A. F., Dai, H., Gu, X., Ravichandran, R., et al. (2021). Donor-derived regulatory dendritic cell infusion results in host cell cross-dressing and T cell subset changes in prospective living donor liver transplant recipients. Am. J. Transplant. 21 (7), 2372–2386. doi:10.1111/ajt.16393
Maldonado, R. A., and von Andrian, U. H. (2010). How tolerogenic dendritic cells induce regulatory T cells. Adv. Immunol. 108, 111–165. doi:10.1016/B978-0-12-380995-7.00004-5
Manicassamy, S., and Pulendran, B. (2011). Dendritic cell control of tolerogenic responses. Immunol. Rev. 241 (1), 206–227. doi:10.1111/j.1600-065X.2011.01015.x
Marín, E., Cuturi, M. C., and Moreau, A. (2018). Tolerogenic Dendritic Cells in Solid Organ Transplantation: where Do We Stand? Front. Immunol. 9, 274. doi:10.3389/fimmu.2018.00274
Marino, J., Babiker-Mohamed, M. H., Crosby-Bertorini, P., Paster, J. T., LeGuern, C., Germana, S., et al. (2016). Donor exosomes rather than passenger leukocytes initiate alloreactive T cell responses after transplantation. Sci. Immunol. 1 (1), aaf8759. doi:10.1126/sciimmunol.aaf8759
Martín-Gayo, E., Sierra-Filardi, E., Corbí, A. L., and Toribio, M. L. (2010). Plasmacytoid dendritic cells resident in human thymus drive natural Treg cell development. Blood 115 (26), 5366–5375. doi:10.1182/blood-2009-10-248260
Mastoridis, S., Londoño, M.-C., Kurt, A., Kodela, E., Crespo, E., Mason, J., et al. (2021). Impact of donor extracellular vesicle release on recipient cell "cross-dressing" following clinical liver and kidney transplantation. Am. J. Transplant. 21 (7), 2387–2398. doi:10.1111/ajt.16123
Matsuno, K., and Ezaki, T. (2000). Dendritic cell dynamics in the liver and hepatic lymph. Int. Rev. Cytol. 197, 83–136. doi:10.1016/s0074-7696(00)97003-7
Matsuno, K., Ezaki, T., Kudo, S., and Uehara, Y. (1996). A life stage of particle-laden rat dendritic cells in vivo: their terminal division, active phagocytosis, and translocation from the liver to the draining lymph. J. Exp. Med. 183 (4), 1865–1878. doi:10.1084/jem.183.4.1865
Mellor, A. L., Keskin, D. B., Johnson, T., Chandler, P., and Munn, D. H. (2002). Cells expressing indoleamine 2,3-dioxygenase inhibit T cell responses. J. Immunol. 168 (8), 3771–3776. doi:10.4049/jimmunol.168.8.3771
Mempel, T. R., Henrickson, S. E., and Von Andrian, U. H. (2004). T-cell priming by dendritic cells in lymph nodes occurs in three distinct phases. Nature 427 (6970), 154–159. doi:10.1038/nature02238
Menezes, S., Melandri, D., Anselmi, G., Perchet, T., Loschko, J., Dubrot, J., et al. (2016). The Heterogeneity of Ly6Chi Monocytes Controls Their Differentiation into iNOS+ Macrophages or Monocyte-Derived Dendritic Cells. Immunity 45 (6), 1205–1218. doi:10.1016/j.immuni.2016.12.001
Merad, M., Sathe, P., Helft, J., Miller, J., and Mortha, A. (2013). The dendritic cell lineage: ontogeny and function of dendritic cells and their subsets in the steady state and the inflamed setting. Annu. Rev. Immunol. 31, 563–604. doi:10.1146/annurev-immunol-020711-074950
Mildner, A., Yona, S., and Jung, S. (2013). A close encounter of the third kind: monocyte-derived cells. Adv. Immunol. 120, 69–103. doi:10.1016/B978-0-12-417028-5.00003-X
Miller, M. J., Safrina, O., Parker, I., and Cahalan, M. D. (2004). Imaging the single cell dynamics of CD4+ T cell activation by dendritic cells in lymph nodes. J. Exp. Med. 200 (7), 847–856. doi:10.1084/jem.20041236
Moreau, A., Alliot-Licht, B., Cuturi, M.-C., and Blancho, G. (2017). Tolerogenic dendritic cell therapy in organ transplantation. Transpl. Int. Official J. Eur. Soc. For Organ Transplant. 30 (8), 754–764. doi:10.1111/tri.12889
Morelli, A. E., and Thomson, A. W. (2007). Tolerogenic dendritic cells and the quest for transplant tolerance. Nat. Rev. Immunol. 7 (8), 610–621. doi:10.1038/nri2132
Moseman, E. A., Liang, X., Dawson, A. J., Panoskaltsis-Mortari, A., Krieg, A. M., Liu, Y.-J., et al. (2004). Human plasmacytoid dendritic cells activated by CpG oligodeoxynucleotides induce the generation of CD4+CD25+ regulatory T cells. J. Immunol. 173 (7), 4433–4442. doi:10.4049/jimmunol.173.7.4433
Munn, D. H., Sharma, M. D., Hou, D., Baban, B., Lee, J. R., Antonia, S. J., et al. (2004). Expression of indoleamine 2,3-dioxygenase by plasmacytoid dendritic cells in tumor-draining lymph nodes. J. Clin. Investigation 114 (2), 280–290. doi:10.1172/JCI21583
Murphy, T. L., Grajales-Reyes, G. E., Wu, X., Tussiwand, R., Briseño, C. G., Iwata, A., et al. (2016). Transcriptional Control of Dendritic Cell Development. Annu. Rev. Immunol. 34, 93–119. doi:10.1146/annurev-immunol-032713-120204
Nakano, R., Yoshida, O., Kimura, S., Nakao, T., Yokota, S., Ono, Y., et al. (2021). Donor plasmacytoid dendritic cells modulate effector and regulatory T cell responses in mouse spontaneous liver transplant tolerance. Am. J. Transplant. 21 (6), 2040–2055. doi:10.1111/ajt.16412
Navarro-Barriuso, J., Mansilla, M. J., and Martínez-Cáceres, E. M. (2018). Searching for the Transcriptomic Signature of Immune Tolerance Induction-Biomarkers of Safety and Functionality for Tolerogenic Dendritic Cells and Regulatory Macrophages. Front. Immunol. 9, 2062. doi:10.3389/fimmu.2018.02062
Nitschké, M., Aebischer, D., Abadier, M., Haener, S., Lucic, M., Vigl, B., et al. (2012). Differential requirement for ROCK in dendritic cell migration within lymphatic capillaries in steady-state and inflammation. Blood 120 (11), 2249–2258. doi:10.1182/blood-2012-03-417923
Oberbarnscheidt, M. H., Zeng, Q., Li, Q., Dai, H., Williams, A. L., Shlomchik, W. D., et al. (2014). Non-self recognition by monocytes initiates allograft rejection. J. Clin. Investigation 124 (8), 3579–3589. doi:10.1172/JCI74370
Ochando, J., and Braza, M. S. (2017). Nanoparticle-Based Modulation and Monitoring of Antigen-Presenting Cells in Organ Transplantation. Front. Immunol. 8, 1888. doi:10.3389/fimmu.2017.01888
Ochando, J. C., Homma, C., Yang, Y., Hidalgo, A., Garin, A., Tacke, F., et al. (2006). Alloantigen-presenting plasmacytoid dendritic cells mediate tolerance to vascularized grafts. Nat. Immunol. 7 (6), 652–662. doi:10.1038/ni1333
Ochando, J., Ordikhani, F., Jordan, S., Boros, P., and Thomson, A. W. (2020). Tolerogenic dendritic cells in organ transplantation. Transpl. Int. Official J. Eur. Soc. For Organ Transplant. 33 (2), 113–127. doi:10.1111/tri.13504
Ono, Y., Perez-Gutierrez, A., Nakao, T., Dai, H., Camirand, G., Yoshida, O., et al. (2018). Graft-infiltrating PD-L1hi cross-dressed dendritic cells regulate antidonor T cell responses in mouse liver transplant tolerance. Hepatol. Baltim. Md 67 (4), 1499–1515. doi:10.1002/hep.29529
Palomares, O., Rückert, B., Jartti, T., Kücüksezer, U. C., Puhakka, T., Gomez, E., et al. (2012). Induction and maintenance of allergen-specific FOXP3+ Treg cells in human tonsils as potential first-line organs of oral tolerance. J. Allergy Clin. Immunol. 129 (2), 510–520. doi:10.1016/j.jaci.2011.09.031
Penna, G., Vulcano, M., Sozzani, S., and Adorini, L. (2002). Differential migration behavior and chemokine production by myeloid and plasmacytoid dendritic cells. Hum. Immunol. 63 (12), 1164–1171. doi:10.1016/s0198-8859(02)00755-3
Pillarisetty, V. G., Shah, A. B., Miller, G., Bleier, J. I., and DeMatteo, R. P. (2004). Liver dendritic cells are less immunogenic than spleen dendritic cells because of differences in subtype composition. J. Immunol. 172 (2), 1009–1017. doi:10.4049/jimmunol.172.2.1009
Plantinga, M., Guilliams, M., Vanheerswynghels, M., Deswarte, K., Branco-Madeira, F., Toussaint, W., et al. (2013). Conventional and monocyte-derived CD11b(+) dendritic cells initiate and maintain T helper 2 cell-mediated immunity to house dust mite allergen. Immunity 38 (2), 322–335. doi:10.1016/j.immuni.2012.10.016
Poulin, L. F., Reyal, Y., Uronen-Hansson, H., Schraml, B. U., Sancho, D., Murphy, K. M., et al. (2012). DNGR-1 is a specific and universal marker of mouse and human Batf3-dependent dendritic cells in lymphoid and nonlymphoid tissues. Blood 119 (25), 6052–6062. doi:10.1182/blood-2012-01-406967
Pulendran, B. (2015). The varieties of immunological experience: of pathogens, stress, and dendritic cells. Annu. Rev. Immunol. 33, 563–606. doi:10.1146/annurev-immunol-020711-075049
Que, W., Guo, W.-Z., and Li, X.-K. (2020). Manipulation of Regulatory Dendritic Cells for Induction Transplantation Tolerance. Front. Immunol. 11, 582658. doi:10.3389/fimmu.2020.582658
Rahman, A. H., and Aloman, C. (2013). Dendritic cells and liver fibrosis. Biochimica Biophysica Acta 1832 (7), 998–1004. doi:10.1016/j.bbadis.2013.01.005
Raïch-Regué, D., Glancy, M., and Thomson, A. W. (2014). Regulatory dendritic cell therapy: from rodents to clinical application. Immunol. Lett. 161 (2), 216–221. doi:10.1016/j.imlet.2013.11.016
Raker, V. K., Domogalla, M. P., and Steinbrink, K. (2015). Tolerogenic Dendritic Cells for Regulatory T Cell Induction in Man. Front. Immunol. 6, 569. doi:10.3389/fimmu.2015.00569
Rastellini, C., Lu, L., Ricordi, C., Starzl, T. E., Rao, A. S., and Thomson, A. W. (1995). Granulocyte/macrophage colony-stimulating factor-stimulated hepatic dendritic cell progenitors prolong pancreatic islet allograft survival. Transplantation 60 (11), 1366–1370.
Reis e Sousa, C. (2006). Dendritic cells in a mature age. Nat. Rev. Immunol. 6 (6), 476–483. doi:10.1038/nri1845
Reizis, B., Bunin, A., Ghosh, H. S., Lewis, K. L., and Sisirak, V. (2011). Plasmacytoid dendritic cells: recent progress and open questions. Annu. Rev. Immunol. 29, 163–183. doi:10.1146/annurev-immunol-031210-101345
Reizis, B. (2019). Plasmacytoid Dendritic Cells: development, Regulation, and Function. Immunity 50 (1), 37–50. doi:10.1016/j.immuni.2018.12.027
Rich, F. J., Kuhn, S., Hyde, E. J., Harper, J. L., Ronchese, F., and Kirman, J. R. (2012). Induction of T cell responses and recruitment of an inflammatory dendritic cell subset following tumor immunotherapy with Mycobacterium smegmatis. Cancer Immunol. Immunother. CII 61 (12), 2333–2342. doi:10.1007/s00262-012-1291-8
Robbins, P. D., and Morelli, A. E. (2014). Regulation of immune responses by extracellular vesicles. Nat. Rev. Immunol. 14 (3), 195–208. doi:10.1038/nri3622
Rogers, N. M., Isenberg, J. S., and Thomson, A. W. (2013). Plasmacytoid dendritic cells: no longer an enigma and now key to transplant tolerance? Am. J. Transplant. 13 (5), 1125–1133. doi:10.1111/ajt.12229
Romani, N., Koide, S., Crowley, M., Witmer-Pack, M., Livingstone, A. M., Fathman, C. G., et al. (1989). Presentation of exogenous protein antigens by dendritic cells to T cell clones. Intact protein is presented best by immature, epidermal Langerhans cells. J. Exp. Med. 169 (3), 1169–1178. doi:10.1084/jem.169.3.1169
Ronca, V., Wootton, G., Milani, C., and Cain, O. (2020). The Immunological Basis of Liver Allograft Rejection. Front. Immunol. 11, 2155. doi:10.3389/fimmu.2020.02155
Rosas, M., Thomas, B., Stacey, M., Gordon, S., and Taylor, P. R. (2010). The myeloid 7/4-antigen defines recently generated inflammatory macrophages and is synonymous with Ly-6B. J. Leukoc. Biol. 88 (1), 169–180. doi:10.1189/jlb.0809548
Ruben, J. M., García-Romo, G. S., Breman, E., van der Kooij, S., Redeker, A., Arens, R., et al. (2018). Human plasmacytoid dendritic cells acquire phagocytic capacity by TLR9 ligation in the presence of soluble factors produced by renal epithelial cells. Kidney Int. 93 (2), 355–364. doi:10.1016/j.kint.2017.08.006
Russo, E., Teijeira, A., Vaahtomeri, K., Willrodt, A.-H., Bloch, J. S., Nitschké, M., et al. (2016). Intralymphatic CCL21 Promotes Tissue Egress of Dendritic Cells through Afferent Lymphatic Vessels. Cell Rep. 14 (7), 1723–1734. doi:10.1016/j.celrep.2016.01.048
Saeki, H., Moore, A. M., Brown, M. J., and Hwang, S. T. (1999). Cutting edge: secondary lymphoid-tissue chemokine (SLC) and CC chemokine receptor 7 (CCR7) participate in the emigration pathway of mature dendritic cells from the skin to regional lymph nodes. J. Immunol. 162 (5), 2472–2475. doi:10.4049/jimmunol.162.5.2472
Sallusto, F., Schaerli, P., Loetscher, P., Schaniel, C., Lenig, D., Mackay, C. R., et al. (1998). Rapid and coordinated switch in chemokine receptor expression during dendritic cell maturation. Eur. J. Immunol. 28 (9), 2760–2769. doi:10.1002/(SICI)1521-4141(199809)28:09<2760::AID-IMMU2760>3.0.CO;2-N
Sander, J., Schmidt, S. V., Cirovic, B., McGovern, N., Papantonopoulou, O., Hardt, A.-L., et al. (2017). Cellular Differentiation of Human Monocytes Is Regulated by Time-Dependent Interleukin-4 Signaling and the Transcriptional Regulator NCOR2. Immunity 47 (6), 1051–1066. doi:10.1016/j.immuni.2017.11.024
Sato, T., Yamamoto, H., Sasaki, C., and Wake, K. (1998). Maturation of rat dendritic cells during intrahepatic translocation evaluated using monoclonal antibodies and electron microscopy. Cell Tissue Res. 294 (3), 503–514. doi:10.1007/s004410051201
Satpathy, A. T., Briseño, C. G., Lee, J. S., Ng, D., Manieri, N. A., Kc, W., et al. (2013). Notch2-dependent classical dendritic cells orchestrate intestinal immunity to attaching-and-effacing bacterial pathogens. Nat. Immunol. 14 (9), 937–948. doi:10.1038/ni.2679
Satpathy, A. T., Wu, X., Albring, J. C., and Murphy, K. M. (2012). Re(de)fining the dendritic cell lineage. Nat. Immunol. 13 (12), 1145–1154. doi:10.1038/ni.2467
Schlitzer, A., McGovern, N., and Ginhoux, F. (2015). Dendritic cells and monocyte-derived cells: two complementary and integrated functional systems. Seminars Cell & Dev. Biol. 41, 9–22. doi:10.1016/j.semcdb.2015.03.011
Schmid, M. A., Kingston, D., Boddupalli, S., and Manz, M. G. (2010). Instructive cytokine signals in dendritic cell lineage commitment. Immunol. Rev. 234 (1), 32–44. doi:10.1111/j.0105-2896.2009.00877.x
Schuler, G., and Steinman, R. M. (1985). Murine epidermal Langerhans cells mature into potent immunostimulatory dendritic cells in vitro. J. Exp. Med. 161 (3), 526–546. doi:10.1084/jem.161.3.526
Schumann, K., Lämmermann, T., Bruckner, M., Legler, D. F., Polleux, J., Spatz, J. P., et al. (2010). Immobilized chemokine fields and soluble chemokine gradients cooperatively shape migration patterns of dendritic cells. Immunity 32 (5), 703–713. doi:10.1016/j.immuni.2010.04.017
Segura, E., Touzot, M., Bohineust, A., Cappuccio, A., Chiocchia, G., Hosmalin, A., et al. (2013). Human inflammatory dendritic cells induce Th17 cell differentiation. Immunity 38 (2), 336–348. doi:10.1016/j.immuni.2012.10.018
Serbina, N. V., Salazar-Mather, T. P., Biron, C. A., Kuziel, W. A., and Pamer, E. G. (2003). TNF/iNOS-producing dendritic cells mediate innate immune defense against bacterial infection. Immunity 19 (1), 59–70. doi:10.1016/s1074-7613(03)00171-7
Shariat, A., Karimi, M. H., Mokhtariazad, T., Moazzeni, S. M., Geramizadeh, B., MalekHosseini, S. A., et al. (2014). Maturation state and function of monocyte derived dendritic cells in liver transplant recipients. Iran. J. Immunol. 11 (3), 153–165.
Sharma, M. D., Baban, B., Chandler, P., Hou, D.-Y., Singh, N., Yagita, H., et al. (2007). Plasmacytoid dendritic cells from mouse tumor-draining lymph nodes directly activate mature Tregs via indoleamine 2,3-dioxygenase. J. Clin. Investigation 117 (9), 2570–2582. doi:10.1172/JCI31911
Spiering, R., Jansen, M. A. A., Wood, M. J., Fath, A. A., Eltherington, O., Anderson, A. E., et al. (2019). Targeting of tolerogenic dendritic cells to heat-shock proteins in inflammatory arthritis. J. Transl. Med. 17 (1), 375. doi:10.1186/s12967-019-2128-4
Steinman, R. M., and Banchereau, J. (2007). Taking dendritic cells into medicine. Nature 449 (7161), 419–426. doi:10.1038/nature06175
Steinman, R. M., and Cohn, Z. A. (1973). Identification of a novel cell type in peripheral lymphoid organs of mice. I. Morphology, quantitation, tissue distribution. J. Exp. Med. 137 (5), 1142–1162. doi:10.1084/jem.137.5.1142
Steptoe, R. J., Fu, F., Li, W., Drakes, M. L., Lu, L., Demetris, A. J., et al. (1997). Augmentation of dendritic cells in murine organ donors by Flt3 ligand alters the balance between transplant tolerance and immunity. J. Immunol. 159 (11), 5483–5491. doi:10.4049/jimmunol.159.11.5483
Steptoe, R. J., Patel, R. K., Subbotin, V. M., and Thomson, A. W. (2000). Comparative analysis of dendritic cell density and total number in commonly transplanted organs: morphometric estimation in normal mice. Transpl. Immunol. 8 (1), 49–56. doi:10.1016/s0966-3274(00)00010-1
Sumpter, T. L., Dangi, A., Matta, B. M., Huang, C., Stolz, D. B., Vodovotz, Y., et al. (2012). Hepatic stellate cells undermine the allostimulatory function of liver myeloid dendritic cells via STAT3-dependent induction of IDO. J. Immunol. 189 (8), 3848–3858. doi:10.4049/jimmunol.1200819
Süss, G., and Shortman, K. (1996). A subclass of dendritic cells kills CD4 T cells via Fas/Fas-ligand-induced apoptosis. J. Exp. Med. 183 (4), 1789–1796. doi:10.1084/jem.183.4.1789
Swiecki, M., and Colonna, M. (2010). Unraveling the functions of plasmacytoid dendritic cells during viral infections, autoimmunity, and tolerance. Immunol. Rev. 234 (1), 142–162. doi:10.1111/j.0105-2896.2009.00881.x
Tacke, F., Alvarez, D., Kaplan, T. J., Jakubzick, C., Spanbroek, R., Llodra, J., et al. (2007). Monocyte subsets differentially employ CCR2, CCR5, and CX3CR1 to accumulate within atherosclerotic plaques. J. Clin. Investigation 117 (1), 185–194. doi:10.1172/JCI28549
Tadayon, S., Dunkel, J., Takeda, A., Eichin, D., Virtakoivu, R., Elima, K., et al. (2021). Lymphatic Endothelial Cell Activation and Dendritic Cell Transmigration Is Modified by Genetic Deletion of Clever-1. Front. Immunol. 12, 602122. doi:10.3389/fimmu.2021.602122
Tal, O., Lim, H. Y., Gurevich, I., Milo, I., Shipony, Z., Ng, L. G., et al. (2011). DC mobilization from the skin requires docking to immobilized CCL21 on lymphatic endothelium and intralymphatic crawling. J. Exp. Med. 208 (10), 2141–2153. doi:10.1084/jem.20102392
Tang-Huau, T.-L., and Segura, E. (2019). Human in vivo-differentiated monocyte-derived dendritic cells. Seminars Cell & Dev. Biol. 86, 44–49. doi:10.1016/j.semcdb.2018.02.018
Thomson, A. W., and Knolle, P. A. (2010). Antigen-presenting cell function in the tolerogenic liver environment. Nat. Rev. Immunol. 10 (11), 753–766. doi:10.1038/nri2858
Thomson, A. W., and Lu, L. (1999). Are dendritic cells the key to liver transplant tolerance? Immunol. Today 20 (1), 27–32. doi:10.1016/s0167-5699(98)01378-4
Thomson, A. W., Metes, D. M., Ezzelarab, M. B., and Raïch-Regué, D. (2019). Regulatory dendritic cells for human organ transplantation. Transplant. Rev. Orl. Fla 33 (3), 130–136. doi:10.1016/j.trre.2019.05.001
Thomson, A. W., Vionnet, J., and Sanchez-Fueyo, A. (2020). Understanding, predicting and achieving liver transplant tolerance: from bench to bedside. Nat. Rev. Gastroenterol. Hepatol. 17 (12), 719–739. doi:10.1038/s41575-020-0334-4
Tokita, D., Sumpter, T. L., Raimondi, G., Zahorchak, A. F., Wang, Z., Nakao, A., et al. (2008). Poor allostimulatory function of liver plasmacytoid DC is associated with pro-apoptotic activity, dependent on regulatory T cells. J. Hepatology 49 (6), 1008–1018. doi:10.1016/j.jhep.2008.07.028
Ueta, H., Xu, X.-D., Yu, B., Kitazawa, Y., Yu, E., Hara, Y., et al. (2021). Suppression of liver transplant rejection by anti-donor MHC antibodies via depletion of donor immunogenic dendritic cells. Int. Immunol. 33 (5), 261–272. doi:10.1093/intimm/dxaa076
Ulvmar, M. H., Werth, K., Braun, A., Kelay, P., Hub, E., Eller, K., et al. (2014). The atypical chemokine receptor CCRL1 shapes functional CCL21 gradients in lymph nodes. Nat. Immunol. 15 (7), 623–630. doi:10.1038/ni.2889
Uwatoku, R., Suematsu, M., Ezaki, T., Saiki, T., Tsuiji, M., Irimura, T., et al. (2001). Kupffer cell-mediated recruitment of rat dendritic cells to the liver: roles of N-acetylgalactosamine-specific sugar receptors. Gastroenterology 121 (6), 1460–1472. doi:10.1053/gast.2001.29594
Van den Bergh, J. M. J., Smits, E. L. J. M., Berneman, Z. N., Hutten, T. J. A., De Reu, H., Van Tendeloo, V. F. I., et al. (2017). Monocyte-Derived Dendritic Cells with Silenced PD-1 Ligands and Transpresenting Interleukin-15 Stimulate Strong Tumor-Reactive T-cell Expansion. Cancer Immunol. Res. 5 (8), 710–715. doi:10.1158/2326-6066.CIR-16-0336
Villadangos, J. A., and Young, L. (2008). Antigen-presentation properties of plasmacytoid dendritic cells. Immunity 29 (3), 352–361. doi:10.1016/j.immuni.2008.09.002
Wilson, N. S., and Villadangos, J. A. (2004). Lymphoid organ dendritic cells: beyond the Langerhans cells paradigm. Immunol. Cell Biol. 82 (1), 91–98. doi:10.1111/j.1440-1711.2004.01216.x
Wolf, A. A., Yáñez, A., Barman, P. K., and Goodridge, H. S. (2019). The Ontogeny of Monocyte Subsets. Front. Immunol. 10, 1642. doi:10.3389/fimmu.2019.01642
Yin, X., Chen, S., and Eisenbarth, S. C. (2021). Dendritic Cell Regulation of T Helper Cells. Annu. Rev. Immunol. 39, 759–790. doi:10.1146/annurev-immunol-101819-025146
Yona, S., Kim, K.-W., Wolf, Y., Mildner, A., Varol, D., Breker, M., et al. (2013). Fate mapping reveals origins and dynamics of monocytes and tissue macrophages under homeostasis. Immunity 38 (1), 79–91. doi:10.1016/j.immuni.2012.12.001
Yoneyama, H., Matsuno, K., Zhang, Y., Murai, M., Itakura, M., Ishikawa, S., et al. (2001). Regulation by chemokines of circulating dendritic cell precursors, and the formation of portal tract-associated lymphoid tissue, in a granulomatous liver disease. J. Exp. Med. 193 (1), 35–49. doi:10.1084/jem.193.1.35
Yu, B., Ueta, H., Kitazawa, Y., Tanaka, T., Adachi, K., Kimura, H., et al. (2012). Two immunogenic passenger dendritic cell subsets in the rat liver have distinct trafficking patterns and radiosensitivities. Hepatol. Baltim. Md 56 (4), 1532–1545. doi:10.1002/hep.25795
Zahorchak, A. F., DeRiggi, M. L., Muzzio, J. L., Sutherland, V., Humar, A., Lakkis, F. G., et al. (2023). Manufacturing and validation of Good Manufacturing Practice-compliant regulatory dendritic cells for infusion into organ transplant recipients. Cytotherapy 25 (4), 432–441. doi:10.1016/j.jcyt.2022.11.005
Zahorchak, A. F., Macedo, C., Hamm, D. E., Butterfield, L. H., Metes, D. M., and Thomson, A. W. (2018). High PD-L1/CD86 MFI ratio and IL-10 secretion characterize human regulatory dendritic cells generated for clinical testing in organ transplantation. Cell. Immunol. 323, 9–18. doi:10.1016/j.cellimm.2017.08.008
Zecher, D., van Rooijen, N., Rothstein, D. M., Shlomchik, W. D., and Lakkis, F. G. (2009). An innate response to allogeneic nonself mediated by monocytes. J. Immunol. 183 (12), 7810–7816. doi:10.4049/jimmunol.0902194
Zheng, L., Li, Z., Ling, W., Zhu, D., Feng, Z., and Kong, L. (2018). Exosomes Derived from Dendritic Cells Attenuate Liver Injury by Modulating the Balance of Treg and Th17 Cells After Ischemia Reperfusion. Cell. Physiology Biochem. 46 (2), 740–756. doi:10.1159/000488733
Zhuang, Q., Liu, Q., Divito, S. J., Zeng, Q., Yatim, K. M., Hughes, A. D., et al. (2016). Graft-infiltrating host dendritic cells play a key role in organ transplant rejection. Nat. Commun. 7, 12623. doi:10.1038/ncomms12623
Zubizarreta, I., Flórez-Grau, G., Vila, G., Cabezón, R., España, C., Andorra, M., et al. (2019). Immune tolerance in multiple sclerosis and neuromyelitis optica with peptide-loaded tolerogenic dendritic cells in a phase 1b trial. Proc. Natl. Acad. Sci. U. S. A. 116 (17), 8463–8470. doi:10.1073/pnas.1820039116
Zuidwijk, K., de Fijter, J. W., Mallat, M. J. K., Eikmans, M., van Groningen, M. C., Goemaere, N. N., et al. (2012). Increased influx of myeloid dendritic cells during acute rejection is associated with interstitial fibrosis and tubular atrophy and predicts poor outcome. Kidney Int. 81 (1), 64–75. doi:10.1038/ki.2011.289
Keywords: liver transplantation, dendritic cells, immune rejection, immune tolerance, DC based treatment
Citation: Du X, Li M, Huan C and Lv G (2023) Dendritic cells in liver transplantation immune response. Front. Cell Dev. Biol. 11:1277743. doi: 10.3389/fcell.2023.1277743
Received: 15 August 2023; Accepted: 27 September 2023;
Published: 13 October 2023.
Edited by:
Antonios Chatzigeorgiou, National and Kapodistrian University of Athens, GreeceReviewed by:
Scott Philip Davies, University of Birmingham, United KingdomAhmed Uosef, Houston Methodist Hospital, United States
Copyright © 2023 Du, Li, Huan and Lv. This is an open-access article distributed under the terms of the Creative Commons Attribution License (CC BY). The use, distribution or reproduction in other forums is permitted, provided the original author(s) and the copyright owner(s) are credited and that the original publication in this journal is cited, in accordance with accepted academic practice. No use, distribution or reproduction is permitted which does not comply with these terms.
*Correspondence: Guoyue Lv, bHZneUBqbHUuZWR1LmNu; Chen Huan, bG1zeHNtbEBqbHUuZWR1LmNu
†These authors have contributed equally to this work