- Bay Area Institute of Science, Altos Labs, Redwood City, CA, United States
The Integrated Stress Response (ISR) is an essential homeostatic signaling network that controls the cell’s biosynthetic capacity. Four ISR sensor kinases detect multiple stressors and relay this information to downstream effectors by phosphorylating a common node: the alpha subunit of the eukaryotic initiation factor eIF2. As a result, general protein synthesis is repressed while select transcripts are preferentially translated, thus remodeling the proteome and transcriptome. Mounting evidence supports a view of the ISR as a dynamic signaling network with multiple modulators and feedback regulatory features that vary across cell and tissue types. Here, we discuss updated views on ISR sensor kinase mechanisms, how the subcellular localization of ISR components impacts signaling, and highlight ISR signaling differences across cells and tissues. Finally, we consider crosstalk between the ISR and other signaling pathways as a determinant of cell health.
Introduction
Stress signaling networks endow cells with the means to detect and react to homeostatic deviations. The Integrated Stress Response (ISR) is one such network; it adjusts protein biosynthetic rates and redirects resources to mitigate stress and restore homeostasis [reviewed in Pakos-Zebrucka et al. (2016); Costa-Mattioli and Walter (2020)]. However, if adaptive demands are unmet, the ISR switches to drive cell death, eliminating terminally injured cells for the benefit of the organism. The metazoan ISR has evolved a myriad of variations around this central operative tenet, which remains regulated by conserved building blocks found in all animals. In a nutshell, a battery of ISR sensor kinases transduces information about distinct stress states via phosphorylation of a common node, eIF2, a master regulator of protein synthesis, to terminal effectors that remodel the transcriptome and proteome.
In vertebrates, the ISR is governed by four sensor kinases that detect specific stress inputs: PKR (EIF2AK4), a sensor of double-stranded RNA (dsRNA) with a pivotal role in innate immunity; PERK (EIF2AK3), an ER-transmembrane protein that responds to ER homeostasis deviations; GCN2 (EIF2AK2) which detects amino acid availability and ribosome collisions; and HRI (EIF2AK1), which responds to heme depletion and recently has become recognized as a relay of mitochondrial stress (Donnelly et al., 2013; Pakos-Zebrucka et al., 2016; Costa-Mattioli and Walter, 2020). All these kinases converge on phosphorylating a single serine residue, Ser52 (conventionally referred to as Ser51), in the alpha subunit of the eukaryotic initiation factor 2 (eIF2α), a GTPase essential for translation initiation. The level of phosphorylated eIF2α regulates the availability of the ternary complex (TC), composed of eIF2, GTP, and the initiator methionyl-tRNA.
With initiation factors and the 40S ribosomal subunit, the TC forms the 43S pre-initiation complex (43S-PIC), which scans mRNAs in the 5′-to-3′ direction (Merrick and Pavitt, 2018). Recognition of the start codon by the 43S-PIC, combined with GTP hydrolysis by eIF2, triggers the release of GDP-bound eIF2 and a phosphate ion (Kapp and Lorsch, 2004; Algire et al., 2005; Majumdar and Maitra, 2005). The nucleotide exchange for GTP on eIF2 is catalyzed by eIF2B, eIF2’s dedicated guanine-nucleotide exchange factor (GEF), which enables TC recycling for every new round of translation initiation (Rowlands et al., 1988; Pavitt et al., 1998). Phosphorylated eIF2α acts as a potent inhibitor of eIF2B, thus directly regulating TC availability and global protein synthesis rates during stress encounters (Rowlands et al., 1988; Pavitt et al., 1998; Young and Wek, 2016; Adomavicius et al., 2019; Kashiwagi et al., 2019; Kenner et al., 2019; Schoof et al., 2021; Zyryanova et al., 2021).
As part of the ISR program, certain mRNAs are selectively translated in conditions where translation initiation rates are diminished due to low TC levels. These mRNAs are characterized by regulatory upstream open reading frames (uORFs) in their 5′UTRs and include those encoding the stress-regulated transcription factors ATF4 and CHOP (DDIT3), and GADD34 (PPP1R15A), a regulatory subunit of protein phosphatase 1 (PP1) (reviewed in (Young and Wek, 2016)). PP1-GADD34 dephosphorylates eIF2α, creating a negative feedback loop to terminate ISR signaling (Novoa et al., 2001). An additional non-stress-regulated PP1 regulatory subunit, CReP (PPP1R15B), constitutively dephosphorylates eIF2α to maintain steady-state protein synthesis (Jousse et al., 2003).
Despite its utility, the above-described unadorned view of the ISR remains reductionist and oversimplified. For instance, cellular differentiation implies that physiological ISR inputs, ISR components, and signaling pathways that crosstalk to the ISR, are likely to vary across cell and tissue types, leading to tailored outputs attuned to physiological demands. Indeed, genetic homozygous knock-out mouse models of core ISR components have divergent phenotypes. For example, PKR−/− mice develop normally, while knock-out of its sister kinase PERK leads to strong growth retardation (Table 1). Similarly, patients with ISR mutations can exhibit distinctive clinical manifestations [reviewed in English et al. (2022)]. Mutations that result in ISR activation, for instance, lead to growth impairment in the case of CReP, but leukodystrophy (known as Vanishing White Matter Disease) in the case of eIF2B. Conversely, disorders and conditions of vastly different etiologies present activation of the ISR (Costa-Mattioli and Walter, 2020). Furthermore, core ISR components can vary significantly in RNA and protein levels across tissues, which may lead to different ISR sensitivity (Figure 1; Anisimova et al., 2023). Accordingly, single-cell transcriptomes hint at vastly different ISR sensitivities of different brain cell types (Wong et al., 2019), and cell-type specific ISR signaling differences can be profound amongst cell types sharing ontogeny (Wolzak et al., 2022). Additionally, within the same cell type, emerging evidence suggests ISR regulation through spatial sequestration of components or stoichiometric regulation. We discuss these exciting findings in more detail below.
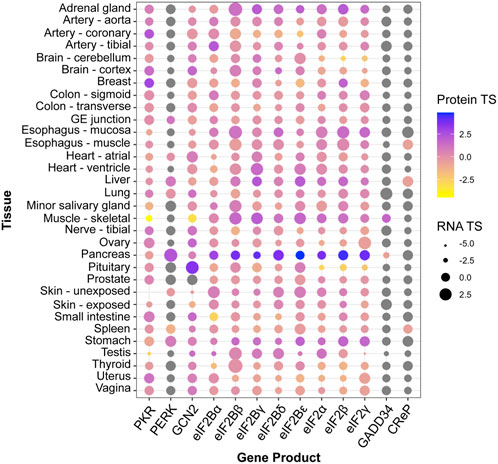
FIGURE 1. Tissue enrichment of core ISR components. Protein and RNA tissue specificity scores (TS) were extracted from the quantitative proteome map of the human body from the GTEx project, and are calculated as standard deviation from the bulk population average across all tissues for each gene product (Jiang et al., 2020). Grey dots: undetected by mass spectrometry. No data was available for ATF4 or HRI (EIF2AK1).
Emerging views on sensor kinase signaling
New sensing mechanisms
In the past decade, the repertoire of stress inputs detected by the ISR sensor kinases—directly, or indirectly through relay proteins—has expanded considerably. For HRI, it is well-established that its kinase domain can directly detect heme to coordinate the translation of globin mRNAs with heme and iron availability (Rafie-Kolpin et al., 2000; Han et al., 2001; Ricketts et al., 2022). However, it can function independently of heme to relay information about mitochondrial stress, ranging from redox imbalances to loss of mitochondrial proteostasis. This task is accomplished via interactions with the mitochondrial membrane protein DELE1, which is stabilized during mitochondrial stress and subsequently activates HRI in the cytosol (Fessler et al., 2020; 2022; Guo et al., 2020). DELE1 itself is also iron- (but not heme)- sensitive, providing another heme-independent path to HRI activation (Sekine et al., 2023). Moreover, HRI can be inhibited by the chaperone HSPB8, whose availability is regulated by specific pattern-recognition receptor “signalosomes” or amyloidogenic aggregates that serve as HSPB8 sinks (Abdel-Nour et al., 2019). These observations underscore the important role of HRI as a multi-stress information hub.
The reversible dissociation of the ER chaperone BiP (GRP78/HSPA5) from PERK during ER stress as a main mechanism controlling PERK activity has been re-evaluated based on biochemical and structural data, supporting a model wherein unfolded peptides serve as PERK-activating ligands (Wang et al., 2018), as occurs for the protein IRE1 (an ER stress sensor for the unfolded protein response) (Gardner and Walter, 2011; Karagöz et al., 2017). The emergent view highlights non-mutually exclusive activation models driven by protein-protein interactions. Other PERK activation mechanisms have surfaced, some of which appear independent of its classically defined lumenal ER stress sensor function. For one, ER-localized STING can transiently activate PERK on the cytosolic leaflet of the ER membrane, linking cGAS signaling and detection of cytosolic DNA to the ISR (Zhang et al., 2022). Similarly, ADP-ribosylation of PERK’s cytosolic domains by the tail-anchored protein PARP16 reportedly activates PERK without stress (Jwa and Chang, 2012). Acute cytosolic calcium depletion can activate PERK independently of its lumenal sensor domain; whether calcium levels directly modulate PERK remains to be determined (van Vliet et al., 2017). Finally, other non-proteinaceous PERK activators include changes in membrane lipid composition, which are thought to be detected through PERK’s transmembrane domain (Volmer et al., 2013).
PKR was initially identified as a sensor of viral dsRNA (Lemaire et al., 2008), but several studies indicate PKR also detects endogenous dsRNAs, expanding its roles beyond innate immunity. Indeed, PKR is activated by structured RNAs, including mitochondrial transcripts that leak upon mitochondrial damage, Alu and LINE1 transcripts, endogenous β-globin and TNF-α mRNAs, and small nuclear RNAs accessible during mitosis (Chu et al., 1998; Kim et al., 2014; Ilan et al., 2017; Namer et al., 2017; Kim et al., 2018). Moreover, a recent study suggests that PKR might sense misfolded IL-24 accumulating in the cytosol of cells with defective proteasomes (Davidson et al., 2022), broadening the repertoire of PKR inputs beyond dsRNA. Whether the IL-24/PKR interaction is direct and which PKR’s domain is implicated in this interaction is still unclear.
The GCN2 arm of the ISR is long-recognized to be engaged during amino acid deprivation (Berlanga et al., 1999; Sood et al., 2000), UV irradiation (Deng et al., 2002) and proteasomal inhibition (Jiang and Wek, 2005), all of which are likely to induce accumulation of uncharged tRNAs, which GCN2 directly recognizes (Wek et al., 1989; Dong et al., 2000; Padyana et al., 2005; Lageix et al., 2014). GCN2 also detects ribosome collisions through its His-tRNA synthetase-like and pseudokinase domains, even in the absence of deacetylated tRNAs, wherein specific ribosomal conformations expose the P-stalk of the ribosome (such as when ribosomes are stalled) to engage GCN2 (Ishimura et al., 2016; Harding et al., 2019; Inglis et al., 2019; Wu et al., 2020). Together, these observations support the notion of ISR kinase sensing polyfunctionality and a broad input repertoire for ISR kinases.
ISR kinase promiscuity
The term “Integrated Stress Response” was coined two decades ago to describe the convergence of four stress sensor kinases acting on a common substrate, eIF2 (Harding et al., 2003). However, kinases are promiscuous, reflected by their relatively low number compared to all phosphoproteins in the cell (Johnson and Hunter, 2005). This raises the possibility that the ISR kinases phosphorylate more substrates than themselves—all ISR kinases autophosphorylate—and eIF2. Growing evidence supports this view. For example, PKR can phosphorylate the insulin-responsive protein IRS1 (Insulin Receptor Substrate 1), which negatively regulates insulin signaling, linking the ISR to glucose metabolism (Nakamura et al., 2010). GCN2 can phosphorylate and inhibit methionyl-tRNA synthetase (MARS1) in response to UV radiation, amplifying stress signals upon UV damage (Kwon et al., 2011). In vitro work suggests that human GCN2 can phosphorylate yeast eIF2β (Dokládal et al., 2021), although whether eIF2β is a bona fide target in mammalian cells remains confirmed. More recently, GCN2 was reported to phosphorylate PP1α and PP1γ, regulating their activity during mitosis (Stonyte et al., 2023). PERK purportedly phosphorylates the redox homeostasis transcription factor NRF2, leading to its dissociation from its inhibitor KEAP1 and enabling its nuclear translocation (Cullinan et al., 2003). Moreover, recent findings suggest that PERK could phosphorylate the autophagy proteins p62 and ULK1, leading to KEAP1 degradation and orthogonal control of NRF2 in hepatocytes exposed to lipotoxic stress (Lee et al., 2022). Interestingly, PERK has also been shown to regulate phosphatidic acid (PA) levels in an eIF2-dependent manner via the intramembrane PA transporter PRELID1, but also by directly phosphorylating diacylglycerol (DAG) to generate PA, thus impinging on the mTOR/Akt pathway (Bobrovnikova-Marjon et al., 2012; Perea et al., 2023).
Sensor kinase signal modulation
Regulatory signaling modulation can also arise from interactions with ISR kinase regulators, which have been described for each ISR kinase. For example, the RNA binding protein TRBP inhibits PKR through physical interaction (Benkirane et al., 1997) and sequestration of the PKR activator PACT, which in turn controls PKR activity during recalcitrant ER stress (Singh et al., 2009). Similarly, the dsRNA deaminase ADAR1 also modulates PKR in a two-pronged manner: it directly inhibits PKR (Clerzius et al., 2009), but also suppresses endogenous dsRNA levels (Chung et al., 2018). In addition, early work shows PKR inhibition through direct interaction of its kinase domain with p58 (p58IKT/DNAJC3) (Lee et al., 1994; Gale et al., 1996; Polyak et al., 1996; Boriushkin et al., 2016), an interesting finding suggesting polyfunctionality and potential divergent subcellular localization—in the ER lumen and cytosol—of this BiP co-chaperone (Rutkowski et al., 2007; Tao et al., 2010), which can also inhibit PERK (van Huizen et al., 2003). PERK can also be negatively regulated by the Src-containing adaptor Nck1, which protects an inhibitory phosphoresidue in PERK’s cytosolic domain (Yamani et al., 2014). GCN2’s function is stimulated by GCN1, which directly detects ribosome collisions by binding to stalled disomes (Pochopien et al., 2021; Müller et al., 2023). Last, interactions between HRI and positive (DELE1) and negative (HSPA8) regulators can influence HRI signaling, as discussed above (Fessler et al., 2020; Guo et al., 2020).
Newly identified ISR kinases
The complexity of a signaling network can be increased by expanding the repertoire of signal transducers. However, whether additional eIF2 kinases exist remains controversial. Quadruple knockout MEFs lacking PERK, HRI, PKR, and GCN2 (4KO) do not show any signs of eIF2α phosphorylation under acute stresses (Taniuchi et al., 2016), suggesting that the ISR exclusively hinges on its four recognized stress sensor kinases in the initial phase of the response. However, recent evidence suggests that the kinase MARK2, a microtubule-associated kinases (MAPKs) regulator, directly phosphorylates eIF2α in response to chronic proteotoxic stress, even in 4KO cells (Lu et al., 2021). These findings point to the activation of compensatory mechanisms to ensure ISR signaling upon enduring stress. MARK2-mediated phosphorylation of eIF2 requires HSP90 and the isoform δ of protein kinase C (PKCδ), which activate MARK2, making MARK2 a stress information relay. Moreover, the brain-specific kinase FAM69C (DIPK1C) has been shown to directly phosphorylate eIF2α in vitro, and its loss-of-function results in ISR defects in microglia exposed to oxidative stress (Wu et al., 2023). However, the annotated localization of FAM69C’s kinase domain in the ER lumen suggests that the in vivo regulation of eIF2α by FAM69C might be indirect.
Spatial organization of ISR components within cells
The spatial organization and association of molecular components within cells create unique microenvironments that serve as crucibles for biochemical reactions. Likewise, ISR nodes populate specific cellular locales, and ISR kinase activity is regulated by self-association. Several lines of evidence support the notion that active PERK forms high-order oligomers in the plane of the ER membrane (Bertolotti et al., 2000; Carrara et al., 2015; Sundaram et al., 2018; Wang et al., 2018), but the distribution of inactive PERK and PERK oligomers is poorly understood. PERK is also found at ER-mitochondrial contact sites (EMCS) (Verfaillie et al., 2012; Muñoz et al., 2013; van Vliet et al., 2017), and new details about its function and regulation at this specific location have recently been revealed. PERK can act as an adaptor to recruit the lipid-transfer protein E-Syt1 (extended synaptotagmin 1) to EMCS to control mitochondrial respiration; whether this interaction modulates the ISR remains unexplored (Sassano et al., 2023). During ER stress, a covalent interaction between PERK and ERO1α increases the number of EMCS and is essential to improve calcium flux between both organelles (Bassot et al., 2022). Moreover, an inhibitory interaction between PERK and the mitochondrial membrane protein ATAD3 at EMCS allows bypassing ISR translational regulation of transcripts encoding mitochondrial proteins even in the absence of deacetylated tRNAs (Hughes et al., 2022).
The ER provides a platform for the spatial organization and regulation of other ISR components beyond PERK. The constitutive eIF2α phosphatase CReP (PPP1R15B) also associates with the ER-membrane (Kloft et al., 2012), enabling selective protein synthesis of membrane-tethered transcripts despite ISR induction (Kastan et al., 2020). GADD34 (PPP1R15A), the stress-induced counterpart of CReP, can be inserted in the cytoplasmic leaflet of the ER membrane, a process that controls its availability by proteasomal degradation (Brush et al., 2003; Zhou et al., 2011).
In the cytosol, PKR partitions to membrane-less compartments upon detection of dsRNA. These PKR coalescences exclude eIF2α, indicating that they temper enzyme-substrate interactions. Indeed, disruption of PKR clustering enhances ISR signaling, suggesting that PKR utilizes subcellular compartmentalization to limit interactions with eIF2 and buffer ISR signaling (Corbet et al., 2022; Zappa et al., 2022). HRI self-associates into higher-order oligomer assemblies upon activation in vitro (Bauer et al., 2001; Miksanova et al., 2006; Bhavnani et al., 2018). Whether HRI forms coalescences in cells remains to be investigated. Recent structural findings have revealed that DELE1 organizes into an octamer capable of modulating HRI activity, raising the possibility that DELE1 multimers may template HRI multimerization to facilitate autophosphorylation (Yang et al., 2023).
Phosphorylation of eIF2α and the ensuing suppression of translation initiation stimulates the formation of stress granules (SGs); cytosolic, phase-separated, membrane-less compartments containing mRNA and various components of the translational machinery. SGs serve as reservoirs, protecting transcripts from degradation during stress, and act as signaling hubs (Banani et al., 2017; Youn et al., 2019). For instance, the master ISR transcription factor ATF4 is selectively translated in SGs (Mateju et al., 2020), and components of the ER-export machinery are sequestered in SGs to limit secretion during ISR induction, further supporting the roles of SGs as ISR regulators (Zappa et al., 2019). Moreover, in neurons of Alzheimer’s disease brains, both ATF4 protein and mRNA are enriched in axons when compared to the soma, raising the appealing possibility that local synthesis might direct interaction with specific binding partners (Baleriola et al., 2014).
Another example of subcellular compartmentalization in the ISR involves eIF2B. In yeast, eIF2B forms filamentous structures where eIF2 exchanges rapidly within the cytosolic pool (Campbell et al., 2005; Marini et al., 2020; Nüske et al., 2020), and in mammalian cells, eIF2B subunits are found in cytoplasmic foci of varying sizes, even in the absence of stress (Hodgson et al., 2019; Hanson et al., 2023). The dynamic shuttling of eIF2 into eIF2B-containing structures reflects eIF2B activity, suggesting that these foci are enzymatic factories for eIF2 activation. These findings indicate that the reversible formation of condensates and the subcellular localization of ISR components may enable precise control and coordination of the ISR.
Convergence and connectivity: interplay between the ISR and other signaling networks
Crosstalk to other signaling pathways
Stress inputs simultaneously activate multiple intertwined pathways that make up complex signaling networks. The ISR is not exempt from this paradigm, and recent evidence supports the notion that it is deeply interconnected with many signaling pathways. Indeed, the many ways all four arms of the ISR communicate with innate and adaptive immune signaling pathways have been extensively reviewed elsewhere (Martins et al., 2016; Reverendo et al., 2019; Salvagno and Cubillos-Ruiz, 2019; Costa-Mattioli and Walter, 2020; Girardin et al., 2021; Zhao et al., 2023). Specific examples include the modulation of PKR by interferons (Meurs et al., 1990) and the stimulation of NF-kB signaling by PKR (Bonnet et al., 2000; Zamanian-Daryoush et al., 2000); the critical roles of HRI in tuning NOD1 and NOD2—bacterial peptidoglycans sensors that activate NF-κB (Abdel-Nour et al., 2019); and the feed-forward loop resulting from IL-6 induction by ATF4 (Iwasaki et al., 2014).
Beyond immunity, crosstalk can occur through modulatory interactions contingent on subcellular localization. In the ER, unfolded proteins activate PERK, as well as IRE1, and ATF6, two key ER stress sensors. PERK induces the phosphatase RPAP2, which dephosphorylates IRE1 (Chang et al., 2018), establishing negative feedback control to suppress IRE1 pro-survival signals and favor PERK-dependent apoptosis (Chang et al., 2018; Muniozguren et al., 2022). Similarly, ER stress induces BiP downstream of ATF6 and IRE1, thereby exerting negative feedback control of PERK—and IRE1 and ATF6—activities to resolve ER stress (Wang et al., 2000). Interconnectivity also stems from the linkage of ER-associated and cytosolic processes. For example, extrusion of unfolded IL-24 from the lumen of the ER into the cytosol activates PKR (Davidson et al., 2022); and an overwhelmed ubiquitin-proteasome system, as observed in Parkinson’s disease, can activate PERK (Zhang et al., 2010; Hughes and Mallucci, 2019).
The ISR is intimately connected with the cell’s master regulator of anabolic and catabolic processes: the mTOR (mechanistic Target Of Rapamycin) signaling pathway. Amino acid deprivation activates the ISR while suppressing mTORC1 signaling, resulting in exquisite control of protein biosynthetic rates (Liu and Sabatini, 2020). Interestingly, mTORC1 can regulate ATF4 levels in a phospho-eIF2-independent manner by enhancing ATF4 mRNA stability and basal translation through increased mRNA cap-binding protein eIF4E availability (Park et al., 2017; Torrence et al., 2021).
The ISR and mTOR signaling networks are further intertwined through the regulation of autophagy. Deactivation of mTORC1 licenses lysosomal biogenesis and enhances lysosomal function by activating the TFEB family of transcription factors (Settembre et al., 2012). TFEB induces ATF4 and GADD34 mRNA expression, thereby engaging ATF4-driven biosynthetic programs coupled to the negative feedback loop that shuts down ISR signaling (Martina et al., 2016). In this way, during amino acid starvation, the cell ensures the translation of mRNAs encoding autophagy regulators induced by TFEB that require escaping the negative translational control imposed by the ISR (Gambardella et al., 2020). Notably, the ISR gene expression program encompasses autophagy genes, and indeed, eIF2α phosphorylation is required to induce autophagy upon treatment of cells with many pharmacological agents (Humeau et al., 2020). In addition, PERK induces the autophagy regulator ATG12 and stimulates the expression and activation of the autophagosome formation mediator of LC3 (Kouroku et al., 2007); PKR engages autophagy through activation of IKKβ and JNK1, and phosphorylation of IRS1, a known autophagy inducer (Nakamura et al., 2010); and HRI induces BAG3 and HSPB8, key components of chaperone-assisted selective autophagy of cytosolic proteins (Mukherjee et al., 2021). As such, the ISR coordinates catabolism through inhibition of mRNA translation, and by regulation of amino acid recycling through autophagy.
Additional translation modulators significantly influence transcriptional reprogramming during the ISR, and hence may contribute to signaling plasticity across tissues and conditions. The translation factors EIF2D, MCTS1, and DENR were shown to be required for ATF4 translation through their effects on ribosome recycling in both Drosophila (flies) and human cells (Bohlen et al., 2020; Vasudevan et al., 2020; Young et al., 2021). In addition, translation of the ATF4 mRNA is also regulated by RNA methylation (Zhou et al., 2018), an event that is modulated by eIF3D during chronic stress (Mukhopadhyay et al., 2023). These studies underscore the importance of precise translational control during ISR.
Cell-type-specific ISR wiring
Cell identity and the ensuing intrinsic physiological demands require tailored ISR responses, some of which have come to light recently. For example, orthogonal studies in unstressed, wild-type mice have revealed intrinsic ISR signatures in forebrain astrocytes and Bergmann glia in the cerebellum (Wong et al., 2019), and in cholinergic interneurons in the striatum (Helseth et al., 2021), suggesting that these cells rely on the ISR for basic functions. Further evidence suggests distinct ISR outputs in neurons and astrocytes, wherein lack of PERK is compensated by HRI in astrocytes but not in neurons (Wolzak et al., 2022). Moreover, neurons and astrocytes exhibit differences in the localization and composition of eIF2B complexes, and their ISR outcomes vary when subjected to chronic stress and ISR inhibition (Hanson et al., 2023). Inactivating mutations in eIF2B, which are associated with Vanishing White Matter Disease, affect astrocytes and oligodendrocytes prior to neuron dysfunction (Dietrich et al., 2005; Dooves et al., 2016; Wisse et al., 2018; Zhou et al., 2019; Deng et al., 2022). Along this line, ISR modulation can have dramatically different results in neuronal cell types: adjusting the ISR in excitatory and somatostatin-positive inhibitory neurons, but not parvalbumin-positive inhibitory neurons, results in altered long-term memory consolidation (Sharma et al., 2020; Shrestha et al., 2020). Another example highlights the differences in the activation threshold of the ISR in different neural cell types: the GCN2 inhibitor IMPACT is significantly more abundant in hypothalamic neurons than in other cells (Pereira et al., 2005; Bittencourt et al., 2008), which could lead to distinct regulation of the ISR in conditions that activate GCN2.
Beyond the brain, in the mouse and human intestinal epithelium, secretory goblet cells can readily adapt to prolonged ER stress, but absorptive enterocytes are prone to ISR-induced expression of the transcription factor QRICH1, which promotes sustained, unmanageable protein synthesis that leads to cell death (You et al., 2021). Myoblasts and myotubes differ in their ability to mount an ISR during mitochondrial electron transport chain dysfunction due to differences in their metabolic state (Mick et al., 2020). In the testis, increased protein synthesis during spermatogenesis requires an alternative eIF2γ subunit (Eif2s3y in mice, EIF2S3B in humans) (Mazeyrat et al., 2001; Matsubara et al., 2015). All these examples underscore the notion that circuit complexity within the ISR enables cell type-specific adaptations.
Discussion
Specific cellular physiological demands call for distinct ISR adaptations in multicellular organisms. Classic examples include the endocrine pancreas’ reliance on PERK, erythroblasts’ dependence on HRI’s heme-sensing, and the dysregulation of ISR in neuropathology (Kefalas and Larose, 2018; Chen and Zhang, 2019; Bond et al., 2020). Beyond these examples, an emerging constellation of small molecules, lipids, and protein activators of ISR kinases, alternate ISR kinases, additional substrates, and ISR core component availability mediated by expression, stability, enzymatic activity, and spatial sequestration, portrays a complex and intricate network of mechanisms through which cells modulate the ISR with finesse. Speculations abound around this theme, leading us to postulate here less-explored hierarchies of ISR modulation, ranging from metabolite, lipid, and post-translational-modification (PTM)-mediated ISR regulation, to ISR tuning by intercellular communication and ISR molecular memories.
We surmise that many metabolites could affect the ISR, as occurs with fructose-6-phosphate, an enhancer of eIF2B activity (Hao et al., 2021). Moreover, defects in lipid metabolism caused by expression of the 4ε isoform of the lipid transporter APOE activate the ISR (Segev et al., 2013; 2015), suggesting lipid-mediated mechanisms of ISR regulation. Many core components of the ISR are extensively post-translationally modified, implying that PTMs may exert exquisite control to diversify and adjust ISR outputs. For instance, ATF4 is extensively regulated by phosphorylation, methylation, and ubiquitination, which can impact its protein stability and activity (Yang et al., 2004; Frank et al., 2010; Kim et al., 2011; Fan et al., 2014; Yuniati et al., 2016). Similarly, eIF2 and eIF2B can be phosphorylated and acetylated on multiple subunits (Welsh et al., 1998; Wang et al., 2001; Beilsten-Edmands et al., 2015). However, the exact function, regulation, and integration of these PTMs remain poorly understood.
Cell-to-cell communication could endow the ISR with an additional layer of regulation with the potential to impact cellular decisions within cell “ecosystems” and, consequently, the health of tissues and organs. Accumulating evidence suggests adaptive and terminal stress responses propagate signals amongst cell communities to coordinate cell responses across tissues. For example, apoptotic cells secrete proteins and metabolites that allow the transmission of information across spatial scales (Christiansen et al., 2013; Pérez-Garijo et al., 2013; Medina et al., 2020), cells experiencing mitochondrial stress inform neighboring cells via mitokines (Durieux et al., 2011; Berendzen et al., 2016; Shao et al., 2016; Zhang et al., 2018; Lan et al., 2019; Kang et al., 2021), and ER stress signaling in the nervous system induces ER stress responses in peripheral tissues in nematodes and vertebrates (Taylor and Dillin, 2013; Williams et al., 2014; Imanikia et al., 2019; Daniele et al., 2020). These observations suggest that the ISR may engage similar cell non-autonomous mechanisms, especially considering that the ISR induces the expression of chemokines and can trigger apoptosis (Hattori et al., 2003; Iwasaki et al., 2014; Forsström et al., 2019; Sprenkle et al., 2019).
Another aspect that requires further investigation is the mechanistic characterization of an ISR molecular memory that could stand behind cell resilience and the hormetic adaptation of tissues and organs. Hints of such adaptive stress memories have been observed in C. elegans, in which exposure to oxidative stress early in life protects older worms from heat shock (Bazopoulou et al., 2019; Zhang et al., 2021). Closer to the ISR, GADD34 has been suggested to play a central role in cellular adaptation to repetitive stresses (Batjargal et al., 2022; Klein et al., 2022). Whether epigenetic ISR memories exist and how they contribute to heritable hormetic adaptation remains to be defined.
The broad capacity of the ISR to multitask and control cellular decisions supports a model in which the ISR could be envisioned as a central integrator of signaling pathways controlling the homeostatic capacity of the cell. Such a network would allow finely tuned responses tailored to meet the specific demands imposed by the intrinsic physiology of cells and tissues. While much progress has been made to unearth new roles and nuances of the ISR signaling network, it has also made it clear that the ISR will continue to yield surprises for decades to come.
Author contributions
MB: Conceptualization, Writing–original draft, Writing–review and editing. FZ: Conceptualization, Writing–original draft, Writing–review and editing.
Funding
The author(s) declare financial support was received for the research, authorship, and/or publication of this article. This study received funding from Altos Labs Inc. The funder was not involved in the data collection, interpretation, and writing of this article or the decision to submit it for publication.
Acknowledgments
We thank Diego Acosta-Alvear for editing the manuscript and for the insightful discussions. Additionally, we extend our thanks to Peter Walter, Adam Frost, Lucas Reineke, and Carmen Noemí Hernandez-Candía for providing valuable suggestions.
Conflict of interest
Authors MB and FZ were employed by Altos labs.
Publisher’s note
All claims expressed in this article are solely those of the authors and do not necessarily represent those of their affiliated organizations, or those of the publisher, the editors and the reviewers. Any product that may be evaluated in this article, or claim that may be made by its manufacturer, is not guaranteed or endorsed by the publisher.
References
Abdel-Nour, M., Carneiro, L. A. M., Downey, J., Tsalikis, J., Outlioua, A., Prescott, D., et al. (2019). The heme-regulated inhibitor is a cytosolic sensor of protein misfolding that controls innate immune signaling. Science 365, eaaw4144. doi:10.1126/science.aaw4144
Abraham, N., Stojdl, D. F., Duncan, P. I., Méthot, N., Ishii, T., Dubé, M., et al. (1999). Characterization of transgenic mice with targeted disruption of the catalytic domain of the double-stranded RNA-dependent protein kinase, PKR. J. Biol. Chem. 274, 5953–5962. doi:10.1074/jbc.274.9.5953
Adomavicius, T., Guaita, M., Zhou, Y., Jennings, M. D., Latif, Z., Roseman, A. M., et al. (2019). The structural basis of translational control by eIF2 phosphorylation. Nat. Commun. 10, 2136. doi:10.1038/s41467-019-10167-3
Algire, M. A., Maag, D., and Lorsch, J. R. (2005). Pi release from eIF2, not GTP hydrolysis, is the step controlled by start-site selection during eukaryotic translation initiation. Mol. Cell 20, 251–262. doi:10.1016/j.molcel.2005.09.008
Anisimova, A. S., Kolyupanova, N. M., Makarova, N. E., Egorov, A. A., Kulakovskiy, I. V., and Dmitriev, S. E. (2023). Human tissues exhibit diverse composition of translation machinery. Int. J. Mol. Sci. 24, 8361. doi:10.3390/ijms24098361
Anthony, T. G., McDaniel, B. J., Byerley, R. L., McGrath, B. C., Cavener, D. R., McNurlan, M. A., et al. (2004). Preservation of liver protein synthesis during dietary leucine deprivation occurs at the expense of skeletal muscle mass in mice deleted for eIF2 kinase GCN2. J. Biol. Chem. 279, 36553–36561. doi:10.1074/jbc.M404559200
Bahnan, W., Boucher, J. C., Gayle, P., Shrestha, N., Rosen, M., Aktas, B., et al. (2018). The eIF2α kinase heme-regulated inhibitor protects the host from infection by regulating intracellular pathogen trafficking. Infect. Immun. 86, e00707–e00717. doi:10.1128/IAI.00707-17
Baleriola, J., Walker, C. A., Jean, Y. Y., Crary, J. F., Troy, C. M., Nagy, P. L., et al. (2014). Axonally synthesized ATF4 transmits a neurodegenerative signal across brain regions. Cell 158, 1159–1172. doi:10.1016/j.cell.2014.07.001
Banani, S. F., Lee, H. O., Hyman, A. A., and Rosen, M. K. (2017). Biomolecular condensates: organizers of cellular biochemistry. Nat. Rev. Mol. Cell Biol. 18, 285–298. doi:10.1038/nrm.2017.7
Bassot, A., Chen, J., Takahashi-Yamashiro, K., Yap, M. C., Gibhardt, C. S., Le, G. N. T., et al. (2022). The endoplasmic reticulum kinase PERK interacts with the oxidoreductase ERO1 to metabolically adapt mitochondria. Cell Rep. 42, 111899. doi:10.1016/j.celrep.2022.111899
Batjargal, T., Zappa, F., Grant, R. J., Piscopio, R. A., Chialastri, A., Dey, S. S., et al. (2022). Optogenetic control of the integrated stress response reveals proportional encoding and the stress memory landscape. Cell Syst. 05 (24), 493309. doi:10.1101/2022.05.24.493309
Bauer, B. N., Rafie-Kolpin, M., Lu, L., Han, A., and Chen, J. J. (2001). Multiple autophosphorylation is essential for the formation of the active and stable homodimer of heme-regulated eIF2alpha kinase. Biochemistry 40, 11543–11551. doi:10.1021/bi010983s
Bazopoulou, D., Knoefler, D., Zheng, Y., Ulrich, K., Oleson, B. J., Xie, L., et al. (2019). Developmental ROS individualizes organismal stress resistance and lifespan. Nature 576, 301–305. doi:10.1038/s41586-019-1814-y
Beilsten-Edmands, V., Gordiyenko, Y., Kung, J. C., Mohammed, S., Schmidt, C., and Robinson, C. V. (2015). eIF2 interactions with initiator tRNA and eIF2B are regulated by post-translational modifications and conformational dynamics. Cell Discov. 1, 15020. doi:10.1038/celldisc.2015.20
Benkirane, M., Neuveut, C., Chun, R. F., Smith, S. M., Samuel, C. E., Gatignol, A., et al. (1997). Oncogenic potential of TAR RNA binding protein TRBP and its regulatory interaction with RNA-dependent protein kinase PKR. EMBO J. 16, 611–624. doi:10.1093/emboj/16.3.611
Berendzen, K. M., Durieux, J., Shao, L.-W., Tian, Y., Kim, H.-E., Wolff, S., et al. (2016). Neuroendocrine coordination of mitochondrial stress signaling and proteostasis. Cell 166, 1553–1563. doi:10.1016/j.cell.2016.08.042
Berlanga, J. J., Santoyo, J., and De Haro, C. (1999). Characterization of a mammalian homolog of the GCN2 eukaryotic initiation factor 2alpha kinase. Eur. J. Biochem. 265, 754–762. doi:10.1046/j.1432-1327.1999.00780.x
Bertolotti, A., Zhang, Y., Hendershot, L. M., Harding, H. P., and Ron, D. (2000). Dynamic interaction of BiP and ER stress transducers in the unfolded-protein response. Nat. Cell Biol. 2, 326–332. doi:10.1038/35014014
Bhavnani, V., Kaviraj, S., Panigrahi, P., Suresh, C. G., Yapara, S., and Pal, J. (2018). Elucidation of molecular mechanism of stability of the heme-regulated eIF2α kinase upon binding of its ligand, hemin in its catalytic kinase domain. J. Biomol. Struct. Dyn. 36, 2845–2861. doi:10.1080/07391102.2017.1368417
Bittencourt, S., Pereira, C. M., Avedissian, M., Delamano, A., Mello, L. E., and Castilho, B. A. (2008). Distribution of the protein IMPACT, an inhibitor of GCN2, in the mouse, rat, and marmoset brain. J. Comp. Neurol. 507, 1811–1830. doi:10.1002/cne.21652
Bobrovnikova-Marjon, E., Pytel, D., Riese, M. J., Vaites, L. P., Singh, N., Koretzky, G. A., et al. (2012). PERK utilizes intrinsic lipid kinase activity to generate phosphatidic acid, mediate Akt activation, and promote adipocyte differentiation. Mol. Cell Biol. 32, 2268–2278. doi:10.1128/MCB.00063-12
Bohlen, J., Harbrecht, L., Blanco, S., Clemm von Hohenberg, K., Fenzl, K., Kramer, G., et al. (2020). DENR promotes translation reinitiation via ribosome recycling to drive expression of oncogenes including ATF4. Nat. Commun. 11, 4676. doi:10.1038/s41467-020-18452-2
Bond, S., Lopez-Lloreda, C., Gannon, P. J., Akay-Espinoza, C., and Jordan-Sciutto, K. L. (2020). The integrated stress response and phosphorylated eukaryotic initiation factor 2α in neurodegeneration. J. Neuropathology Exp. Neurology 79, 123–143. doi:10.1093/jnen/nlz129
Bonnet, M. C., Weil, R., Dam, E., Hovanessian, A. G., and Meurs, E. F. (2000). PKR stimulates NF-kappaB irrespective of its kinase function by interacting with the IkappaB kinase complex. Mol. Cell Biol. 20, 4532–4542. doi:10.1128/mcb.20.13.4532-4542.2000
Boriushkin, E., Wang, J. J., Li, J., Bhatta, M., and Zhang, S. X. (2016). p58(IPK) suppresses NLRP3 inflammasome activation and IL-1β production via inhibition of PKR in macrophages. Sci. Rep. 6, 25013. doi:10.1038/srep25013
Brush, M. H., Weiser, D. C., and Shenolikar, S. (2003). Growth arrest and DNA damage-inducible protein GADD34 targets protein phosphatase 1 alpha to the endoplasmic reticulum and promotes dephosphorylation of the alpha subunit of eukaryotic translation initiation factor 2. Mol. Cell Biol. 23, 1292–1303. doi:10.1128/MCB.23.4.1292-1303.2003
Campbell, S. G., Hoyle, N. P., and Ashe, M. P. (2005). Dynamic cycling of eIF2 through a large eIF2B-containing cytoplasmic body: implications for translation control. J. Cell Biol. 170, 925–934. doi:10.1083/jcb.200503162
Carrara, M., Prischi, F., Nowak, P. R., and Ali, M. M. (2015). Crystal structures reveal transient PERK luminal domain tetramerization in endoplasmic reticulum stress signaling. EMBO J. 34, 1589–1600. doi:10.15252/embj.201489183
Chang, T.-K., Lawrence, D. A., Lu, M., Tan, J., Harnoss, J. M., Marsters, S. A., et al. (2018). Coordination between two branches of the unfolded protein response determines apoptotic cell fate. Mol. Cell 71, 629–636. doi:10.1016/j.molcel.2018.06.038
Chen, J.-J., and Zhang, S. (2019). Heme-regulated eIF2α kinase in erythropoiesis and hemoglobinopathies. Blood 134, 1697–1707. doi:10.1182/blood.2019001915
Christiansen, A. E., Ding, T., Fan, Y., Graves, H. K., Herz, H.-M., Lindblad, J. L., et al. (2013). Non-cell autonomous control of apoptosis by ligand-independent Hedgehog signaling in Drosophila. Cell Death Differ. 20, 302–311. doi:10.1038/cdd.2012.126
Chu, W. M., Ballard, R., Carpick, B. W., Williams, B. R., and Schmid, C. W. (1998). Potential Alu function: regulation of the activity of double-stranded RNA-activated kinase PKR. Mol. Cell Biol. 18, 58–68. doi:10.1128/MCB.18.1.58
Chung, H., Calis, J. J. A., Wu, X., Sun, T., Yu, Y., Sarbanes, S. L., et al. (2018). Human ADAR1 prevents endogenous RNA from triggering translational shutdown. Cell 172, 811–824. doi:10.1016/j.cell.2017.12.038
Clerzius, G., Gélinas, J.-F., Daher, A., Bonnet, M., Meurs, E. F., and Gatignol, A. (2009). ADAR1 interacts with PKR during human immunodeficiency virus infection of lymphocytes and contributes to viral replication. J. Virol. 83, 10119–10128. doi:10.1128/JVI.02457-08
Corbet, G. A., Burke, J. M., Bublitz, G. R., Tay, J. W., and Parker, R. (2022). dsRNA-induced condensation of antiviral proteins modulates PKR activity. Proc. Natl. Acad. Sci. U. S. A. 119, e2204235119. doi:10.1073/pnas.2204235119
Costa-Mattioli, M., Gobert, D., Harding, H., Herdy, B., Azzi, M., Bruno, M., et al. (2005). Translational control of hippocampal synaptic plasticity and memory by the eIF2alpha kinase GCN2. Nature 436, 1166–1173. doi:10.1038/nature03897
Costa-Mattioli, M., and Walter, P. (2020). The integrated stress response: from mechanism to disease. Science 368, eaat5314. doi:10.1126/science.aat5314
Cullinan, S. B., Zhang, D., Hannink, M., Arvisais, E., Kaufman, R. J., and Diehl, J. A. (2003). Nrf2 is a direct PERK substrate and effector of PERK-dependent cell survival. Mol. Cell Biol. 23, 7198–7209. doi:10.1128/MCB.23.20.7198-7209.2003
Daniele, J. R., Higuchi-Sanabria, R., Durieux, J., Monshietehadi, S., Ramachandran, V., Tronnes, S. U., et al. (2020). UPRER promotes lipophagy independent of chaperones to extend life span. Sci. Adv. 6, eaaz1441. eaaz1441. doi:10.1126/sciadv.aaz1441
Davidson, S., Yu, C.-H., Steiner, A., Ebstein, F., Baker, P. J., Jarur-Chamy, V., et al. (2022). Protein kinase R is an innate immune sensor of proteotoxic stress via accumulation of cytoplasmic IL-24. Sci. Immunol. 7, eabi6763. doi:10.1126/sciimmunol.abi6763
Deng, J., Harding, H. P., Raught, B., Gingras, A.-C., Berlanga, J. J., Scheuner, D., et al. (2002). Activation of GCN2 in UV-irradiated cells inhibits translation. Curr. Biol. 12, 1279–1286. doi:10.1016/s0960-9822(02)01037-0
Deng, J., Zhang, J., Gao, K., Yan, W., Zhou, L., Jiang, Y., et al. (2022). Secretomics alterations and astrocyte dysfunction in human iPSC of leukoencephalopathy with vanishing white matter. Neurochem. Res. 47, 3747–3760. doi:10.1007/s11064-022-03765-z
Dietrich, J., Lacagnina, M., Gass, D., Richfield, E., Mayer-Pröschel, M., Noble, M., et al. (2005). EIF2B5 mutations compromise GFAP+ astrocyte generation in vanishing white matter leukodystrophy. Nat. Med. 11, 277–283. doi:10.1038/nm1195
Dokládal, L., Stumpe, M., Pillet, B., Hu, Z., Garcia Osuna, G. M., Kressler, D., et al. (2021). Global phosphoproteomics pinpoints uncharted Gcn2-mediated mechanisms of translational control. Mol. Cell 81, 1879–1889.e6. doi:10.1016/j.molcel.2021.02.037
Dong, J., Qiu, H., Garcia-Barrio, M., Anderson, J., and Hinnebusch, A. G. (2000). Uncharged tRNA activates GCN2 by displacing the protein kinase moiety from a bipartite tRNA-binding domain. Mol. Cell 6, 269–279. doi:10.1016/s1097-2765(00)00028-9
Donnelly, N., Gorman, A. M., Gupta, S., and Samali, A. (2013). The eIF2α kinases: their structures and functions. Cell. Mol. Life Sci. 70, 3493–3511. doi:10.1007/s00018-012-1252-6
Dooves, S., Bugiani, M., Postma, N. L., Polder, E., Land, N., Horan, S. T., et al. (2016). Astrocytes are central in the pathomechanisms of vanishing white matter. J. Clin. Invest. 126, 1512–1524. doi:10.1172/JCI83908
Durieux, J., Wolff, S., and Dillin, A. (2011). The cell-non-autonomous nature of electron transport chain-mediated longevity. Cell 144, 79–91. doi:10.1016/j.cell.2010.12.016
English, A. M., Green, K. M., and Moon, S. L. (2022). A (dis)integrated stress response: genetic diseases of eIF2α regulators. Wiley Interdiscip. Rev. RNA 13, e1689. doi:10.1002/wrna.1689
Fan, C.-F., Miao, Y., Lin, X.-Y., Zhang, D., and Wang, E.-H. (2014). Expression of a phosphorylated form of ATF4 in lung and non-small cell lung cancer tissues. Tumour Biol. 35, 765–771. doi:10.1007/s13277-013-1104-5
Fessler, E., Eckl, E.-M., Schmitt, S., Mancilla, I. A., Meyer-Bender, M. F., Hanf, M., et al. (2020). A pathway coordinated by DELE1 relays mitochondrial stress to the cytosol. Nature 579, 433–437. doi:10.1038/s41586-020-2076-4
Fessler, E., Krumwiede, L., and Jae, L. T. (2022). DELE1 tracks perturbed protein import and processing in human mitochondria. Nat. Commun. 13, 1853. doi:10.1038/s41467-022-29479-y
Forsström, S., Jackson, C. B., Carroll, C. J., Kuronen, M., Pirinen, E., Pradhan, S., et al. (2019). Fibroblast growth factor 21 drives dynamics of local and systemic stress responses in mitochondrial myopathy with mtDNA deletions. Cell Metab. 30, 1040–1054. doi:10.1016/j.cmet.2019.08.019
Frank, C. L., Ge, X., Xie, Z., Zhou, Y., and Tsai, L.-H. (2010). Control of activating transcription factor 4 (ATF4) persistence by multisite phosphorylation impacts cell cycle progression and neurogenesis. J. Biol. Chem. 285, 33324–33337. doi:10.1074/jbc.M110.140699
Gale, M., Tan, S. L., Wambach, M., and Katze, M. G. (1996). Interaction of the interferon-induced PKR protein kinase with inhibitory proteins P58IPK and vaccinia virus K3L is mediated by unique domains: implications for kinase regulation. Mol. Cell Biol. 16, 4172–4181. doi:10.1128/MCB.16.8.4172
Gambardella, G., Staiano, L., Moretti, M. N., De Cegli, R., Fagnocchi, L., Di Tullio, G., et al. (2020). GADD34 is a modulator of autophagy during starvation. Sci. Adv. 6, eabb0205. doi:10.1126/sciadv.abb0205
Gardner, B. M., and Walter, P. (2011). Unfolded proteins are ire1-activating ligands that directly induce the unfolded protein response. Science 333, 1891–1894. doi:10.1126/science.1209126
Girardin, S. E., Cuziol, C., Philpott, D. J., and Arnoult, D. (2021). The eIF2α kinase HRI in innate immunity, proteostasis, and mitochondrial stress. FEBS J. 288, 3094–3107. doi:10.1111/febs.15553
Guo, X., Aviles, G., Liu, Y., Tian, R., Unger, B. A., Lin, Y.-H. T., et al. (2020). Mitochondrial stress is relayed to the cytosol by an OMA1–DELE1–HRI pathway. Nature 579, 427–432. doi:10.1038/s41586-020-2078-2
Han, A.-P., Yu, C., Lu, L., Fujiwara, Y., Browne, C., Chin, G., et al. (2001). Heme-regulated eIF2alpha kinase (HRI) is required for translational regulation and survival of erythroid precursors in iron deficiency. EMBO J. 20, 6909–6918. doi:10.1093/emboj/20.23.6909
Hanson, F. M., Oliveira, M. I. R. de, Cross, A. K., Allen, K. E., and Campbell, S. G. (2023). eIF2B localisation and its regulation during the integrated stress response is cell type specific. doi:10.1101/2023.01.10.523470
Hao, Q., Heo, J.-M., Nocek, B. P., Hicks, K. G., Stoll, V. S., Remarcik, C., et al. (2021). Sugar phosphate activation of the stress sensor eIF2B. Nat. Commun. 12, 3440. doi:10.1038/s41467-021-23836-z
Harding, H. P., Ordonez, A., Allen, F., Parts, L., Inglis, A. J., Williams, R. L., et al. (2019). The ribosomal P-stalk couples amino acid starvation to GCN2 activation in mammalian cells. eLife 8, e50149. doi:10.7554/eLife.50149
Harding, H. P., Zeng, H., Zhang, Y., Jungries, R., Chung, P., Plesken, H., et al. (2001). Diabetes mellitus and exocrine pancreatic dysfunction in Perk−/− mice reveals a role for translational control in secretory cell survival. Mol. Cell 7, 1153–1163. doi:10.1016/S1097-2765(01)00264-7
Harding, H. P., Zhang, Y., Scheuner, D., Chen, J.-J., Kaufman, R. J., and Ron, D. (2009). Ppp1r15 gene knockout reveals an essential role for translation initiation factor 2 alpha (eIF2alpha) dephosphorylation in mammalian development. PNAS 106, 1832–1837. doi:10.1073/pnas.0809632106
Harding, H. P., Zhang, Y., Zeng, H., Novoa, I., Lu, P. D., Calfon, M., et al. (2003). An integrated stress response regulates amino acid metabolism and resistance to oxidative stress. Mol. Cell 11, 619–633. doi:10.1016/s1097-2765(03)00105-9
Hattori, T., Ohoka, N., Hayashi, H., and Onozaki, K. (2003). C/EBP homologous protein (CHOP) up-regulates IL-6 transcription by trapping negative regulating NF-IL6 isoform. FEBS Lett. 541, 33–39. doi:10.1016/s0014-5793(03)00283-7
Helseth, A. R., Hernandez-Martinez, R., Hall, V. L., Oliver, M. L., Turner, B. D., Caffall, Z. F., et al. (2021). Cholinergic neurons constitutively engage the ISR for dopamine modulation and skill learning in mice. Science 372, eabe1931. doi:10.1126/science.abe1931
Hettmann, T., Barton, K., and Leiden, J. M. (2000). Microphthalmia due to p53-mediated apoptosis of anterior lens epithelial cells in mice lacking the CREB-2 transcription factor. Dev. Biol. 222, 110–123. doi:10.1006/dbio.2000.9699
Hodgson, R. E., Varanda, B. A., Ashe, M. P., Allen, K. E., and Campbell, S. G. (2019). Cellular eIF2B subunit localisation: implications for the integrated stress response and its control by small molecule drugs. Mol. Biol. Cell, mbcE18080538. doi:10.1091/mbc.E18-08-0538
Hughes, D., and Mallucci, G. R. (2019). The unfolded protein response in neurodegenerative disorders - therapeutic modulation of the PERK pathway. FEBS J. 286, 342–355. doi:10.1111/febs.14422
Hughes, D. T., Brar, K. K., Morris, J. L., Subramanian, K., Krishna, S., Gao, F., et al. (2022). PERK-ATAD3A interaction protects mitochondrial proteins synthesis during ER stress. 07.(24), 501280. doi:10.1101/2022.07.24.501280
Humeau, J., Leduc, M., Cerrato, G., Loos, F., Kepp, O., and Kroemer, G. (2020). Phosphorylation of eukaryotic initiation factor-2α (eIF2α) in autophagy. Cell Death Dis. 11, 433–512. doi:10.1038/s41419-020-2642-6
Ilan, L., Osman, F., Namer, L. S., Eliahu, E., Cohen-Chalamish, S., Ben-Asouli, Y., et al. (2017). PKR activation and eIF2α phosphorylation mediate human globin mRNA splicing at spliceosome assembly. Cell Res. 27, 688–704. doi:10.1038/cr.2017.39
Imanikia, S., Sheng, M., Castro, C., Griffin, J. L., and Taylor, R. C. (2019). XBP-1 remodels lipid metabolism to extend longevity. Cell Rep. 28, 581–589. doi:10.1016/j.celrep.2019.06.057
Inglis, A. J., Masson, G. R., Shao, S., Perisic, O., McLaughlin, S. H., Hegde, R. S., et al. (2019). Activation of GCN2 by the ribosomal P-stalk. Proc. Natl. Acad. Sci. U.S.A. 116, 4946–4954. doi:10.1073/pnas.1813352116
Ishimura, R., Nagy, G., Dotu, I., Chuang, J. H., and Ackerman, S. L. (2016). Activation of GCN2 kinase by ribosome stalling links translation elongation with translation initiation. eLife 5, e14295. doi:10.7554/eLife.14295
Iwasaki, Y., Suganami, T., Hachiya, R., Shirakawa, I., Kim-Saijo, M., Tanaka, M., et al. (2014). Activating transcription factor 4 links metabolic stress to interleukin-6 expression in macrophages. Diabetes 63, 152–161. doi:10.2337/db13-0757
Jiang, H.-Y., and Wek, R. C. (2005). GCN2 phosphorylation of eIF2alpha activates NF-kappaB in response to UV irradiation. Biochem. J. 385, 371–380. doi:10.1042/BJ20041164
Jiang, L., Wang, M., Lin, S., Jian, R., Li, X., Chan, J., et al. (2020). A quantitative proteome map of the human body. Cell 183, 269–283.e19. doi:10.1016/j.cell.2020.08.036
Johnson, S. A., and Hunter, T. (2005). Kinomics: methods for deciphering the kinome. Nat. Methods 2, 17–25. doi:10.1038/nmeth731
Jousse, C., Oyadomari, S., Novoa, I., Lu, P., Zhang, Y., Harding, H. P., et al. (2003). Inhibition of a constitutive translation initiation factor 2alpha phosphatase, CReP, promotes survival of stressed cells. J. Cell Biol. 163, 767–775. doi:10.1083/jcb.200308075
Jwa, M., and Chang, P. (2012). PARP16 is a tail-anchored endoplasmic reticulum protein required for the PERK- and IRE1α-mediated unfolded protein response. Nat. Cell Biol. 14, 1223–1230. doi:10.1038/ncb2593
Kang, G. M., Min, S. H., Lee, C. H., Kim, J. Y., Lim, H. S., Choi, M. J., et al. (2021). Mitohormesis in hypothalamic POMC neurons mediates regular exercise-induced high-turnover metabolism. Cell Metab. 33, 334–349.e6. doi:10.1016/j.cmet.2021.01.003
Kapp, L. D., and Lorsch, J. R. (2004). GTP-dependent recognition of the methionine moiety on initiator tRNA by translation factor eIF2. J. Mol. Biol. 335, 923–936. doi:10.1016/j.jmb.2003.11.025
Karagöz, G. E., Acosta-Alvear, D., Nguyen, H. T., Lee, C. P., Chu, F., and Walter, P. (2017). An unfolded protein-induced conformational switch activates mammalian IRE1. eLife 6, e30700. doi:10.7554/eLife.30700
Kashiwagi, K., Yokoyama, T., Nishimoto, M., Takahashi, M., Sakamoto, A., Yonemochi, M., et al. (2019). Structural basis for eIF2B inhibition in integrated stress response. Science 364, 495–499. doi:10.1126/science.aaw4104
Kastan, J. P., Dobrikova, E. Y., Bryant, J. D., and Gromeier, M. (2020). CReP mediates selective translation initiation at the endoplasmic reticulum. Sci. Adv. 6, eaba0745. doi:10.1126/sciadv.aba0745
Kefalas, G., and Larose, L. (2018). PERK leads a hub dictating pancreatic β cell homoeostasis. Biol. Cell 110, 27–32. doi:10.1111/boc.201700059
Kenner, L. R., Anand, A. A., Nguyen, H. C., Myasnikov, A. G., Klose, C. J., McGeever, L. A., et al. (2019). eIF2B-catalyzed nucleotide exchange and phosphoregulation by the integrated stress response. Science 364, 491–495. doi:10.1126/science.aaw2922
Kim, W., Bennett, E. J., Huttlin, E. L., Guo, A., Li, J., Possemato, A., et al. (2011). Systematic and quantitative assessment of the ubiquitin-modified proteome. Mol. Cell 44, 325–340. doi:10.1016/j.molcel.2011.08.025
Kim, Y., Lee, J. H., Park, J.-E., Cho, J., Yi, H., and Kim, V. N. (2014). PKR is activated by cellular dsRNAs during mitosis and acts as a mitotic regulator. Genes Dev. 28, 1310–1322. doi:10.1101/gad.242644.114
Kim, Y., Park, J., Kim, S., Kim, M., Kang, M.-G., Kwak, C., et al. (2018). PKR senses nuclear and mitochondrial signals by interacting with endogenous double-stranded RNAs. Mol. Cell 71, 1051–1063. doi:10.1016/j.molcel.2018.07.029
Klein, P., Kallenberger, S. M., Roth, H., Roth, K., Ly-Hartig, T. B. N., Magg, V., et al. (2022). Temporal control of the integrated stress response by a stochastic molecular switch. Sci. Adv. 8, eabk2022. eabk2022. doi:10.1126/sciadv.abk2022
Kloft, N., Neukirch, C., von Hoven, G., Bobkiewicz, W., Weis, S., Boller, K., et al. (2012). A subunit of eukaryotic translation initiation factor 2α-phosphatase (CreP/PPP1R15B) regulates membrane traffic. J. Biol. Chem. 287, 35299–35317. doi:10.1074/jbc.M112.379883
Kojima, E., Takeuchi, A., Haneda, M., Yagi, A., Hasegawa, T., Yamaki, K., et al. (2003). The function of GADD34 is a recovery from a shutoff of protein synthesis induced by ER stress: elucidation by GADD34-deficient mice. FASEB J. 17, 1573–1575. doi:10.1096/fj.02-1184fje
Kouroku, Y., Fujita, E., Tanida, I., Ueno, T., Isoai, A., Kumagai, H., et al. (2007). ER stress (PERK/eIF2alpha phosphorylation) mediates the polyglutamine-induced LC3 conversion, an essential step for autophagy formation. Cell Death Differ. 14, 230–239. doi:10.1038/sj.cdd.4401984
Kwon, N. H., Kang, T., Lee, J. Y., Kim, H. H., Kim, H. R., Hong, J., et al. (2011). Dual role of methionyl-tRNA synthetase in the regulation of translation and tumor suppressor activity of aminoacyl-tRNA synthetase-interacting multifunctional protein-3. Proc. Natl. Acad. Sci. U. S. A. 108, 19635–19640. doi:10.1073/pnas.1103922108
Lageix, S., Rothenburg, S., Dever, T. E., and Hinnebusch, A. G. (2014). Enhanced interaction between pseudokinase and kinase domains in Gcn2 stimulates eIF2α phosphorylation in starved cells. PLoS Genet. 10, e1004326. doi:10.1371/journal.pgen.1004326
Lan, J., Rollins, J. A., Zang, X., Wu, D., Zou, L., Wang, Z., et al. (2019). Translational regulation of non-autonomous mitochondrial stress response promotes longevity. Cell Rep. 28, 1050–1062. doi:10.1016/j.celrep.2019.06.078
Lange, P. S., Chavez, J. C., Pinto, J. T., Coppola, G., Sun, C.-W., Townes, T. M., et al. (2008). ATF4 is an oxidative stress–inducible, prodeath transcription factor in neurons in vitro and in vivo. J. Exp. Med. 205, 1227–1242. doi:10.1084/jem.20071460
Lee, D. H., Park, J. S., Lee, Y. S., and Bae, S. H. (2022). PERK prevents hepatic lipotoxicity by activating the p62-ULK1 axis-mediated noncanonical KEAP1-Nrf2 pathway. Redox Biol. 50, 102235. doi:10.1016/j.redox.2022.102235
Lee, T. G., Tang, N., Thompson, S., Miller, J., and Katze, M. G. (1994). The 58,000-dalton cellular inhibitor of the interferon-induced double-stranded RNA-activated protein kinase (PKR) is a member of the tetratricopeptide repeat family of proteins. Mol. Cell Biol. 14, 2331–2342. doi:10.1128/mcb.14.4.2331
Lemaire, P. A., Anderson, E., Lary, J., and Cole, J. L. (2008). Mechanism of PKR activation by dsRNA. J. Mol. Biol. 381, 351–360. doi:10.1016/j.jmb.2008.05.056
Liu, G. Y., and Sabatini, D. M. (2020). mTOR at the nexus of nutrition, growth, ageing and disease. Nat. Rev. Mol. Cell Biol. 21, 183–203. doi:10.1038/s41580-019-0199-y
Lu, Y.-N., Kavianpour, S., Zhang, T., Zhang, X., Nguyen, D., Thombre, R., et al. (2021). MARK2 phosphorylates eIF2α in response to proteotoxic stress. PLOS Biol. 19, e3001096. doi:10.1371/journal.pbio.3001096
Majumdar, R., and Maitra, U. (2005). Regulation of GTP hydrolysis prior to ribosomal AUG selection during eukaryotic translation initiation. EMBO J. 24, 3737–3746. doi:10.1038/sj.emboj.7600844
Marini, G., Nüske, E., Leng, W., Alberti, S., and Pigino, G. (2020). Reorganization of budding yeast cytoplasm upon energy depletion. Mol. Biol. Cell 31, 1232–1245. doi:10.1091/mbc.E20-02-0125
Martina, J. A., Diab, H. I., Brady, O. A., and Puertollano, R. (2016). TFEB and TFE3 are novel components of the integrated stress response. EMBO J. 35, 479–495. doi:10.15252/embj.201593428
Martins, A. S., Alves, I., Helguero, L., Domingues, M. R., and Neves, B. M. (2016). The unfolded protein response in homeostasis and modulation of mammalian immune cells. Int. Rev. Immunol. 35, 457–476. doi:10.3109/08830185.2015.1110151
Masuoka, H. C., and Townes, T. M. (2002). Targeted disruption of the activating transcription factor 4 gene results in severe fetal anemia in mice. Blood 99, 736–745. doi:10.1182/blood.v99.3.736
Mateju, D., Eichenberger, B., Voigt, F., Eglinger, J., Roth, G., and Chao, J. A. (2020). Single-Molecule imaging reveals translation of mRNAs localized to stress granules. Cell 183, 1801–1812.e13. doi:10.1016/j.cell.2020.11.010
Matsubara, Y., Kato, T., Kashimada, K., Tanaka, H., Zhi, Z., Ichinose, S., et al. (2015). TALEN-mediated gene disruption on Y chromosome reveals critical role of EIF2S3Y in mouse spermatogenesis. Stem Cells Dev. 24, 1164–1170. doi:10.1089/scd.2014.0466
Mazeyrat, S., Saut, N., Grigoriev, V., Mahadevaiah, S. K., Ojarikre, O. A., Rattigan, A., et al. (2001). A Y-encoded subunit of the translation initiation factor Eif2 is essential for mouse spermatogenesis. Nat. Genet. 29, 49–53. doi:10.1038/ng717
McEwen, E., Kedersha, N., Song, B., Scheuner, D., Gilks, N., Han, A., et al. (2005). Heme-regulated inhibitor kinase-mediated phosphorylation of eukaryotic translation initiation factor 2 inhibits translation, induces stress granule formation, and mediates survival upon arsenite exposure. J. Biol. Chem. 280, 16925–16933. doi:10.1074/jbc.M412882200
Medina, C. B., Mehrotra, P., Arandjelovic, S., Perry, J. S. A., Guo, Y., Morioka, S., et al. (2020). Metabolites released from apoptotic cells act as tissue messengers. Nature 580, 130–135. doi:10.1038/s41586-020-2121-3
Merrick, W. C., and Pavitt, G. D. (2018). Protein synthesis initiation in eukaryotic cells. Cold Spring Harb. Perspect. Biol. 10, a033092. doi:10.1101/cshperspect.a033092
Meurs, E., Chong, K., Galabru, J., Thomas, N. S., Kerr, I. M., Williams, B. R., et al. (1990). Molecular cloning and characterization of the human double-stranded RNA-activated protein kinase induced by interferon. Cell 62, 379–390. doi:10.1016/0092-8674(90)90374-n
Mick, E., Titov, D. V., Skinner, O. S., Sharma, R., Jourdain, A. A., and Mootha, V. K. (2020). Distinct mitochondrial defects trigger the integrated stress response depending on the metabolic state of the cell. Elife 9, e49178. doi:10.7554/eLife.49178
Miksanova, M., Igarashi, J., Minami, M., Sagami, I., Yamauchi, S., Kurokawa, H., et al. (2006). Characterization of heme-regulated eIF2alpha kinase: roles of the N-terminal domain in the oligomeric state, heme binding, catalysis, and inhibition. Biochemistry 45, 9894–9905. doi:10.1021/bi060556k
Mukherjee, T., Ramaglia, V., Abdel-Nour, M., Bianchi, A. A., Tsalikis, J., Chau, H. N., et al. (2021). The eIF2α kinase HRI triggers the autophagic clearance of cytosolic protein aggregates. J. Biol. Chem. 296, 100050. doi:10.1074/jbc.RA120.014415
Mukhopadhyay, S., Amodeo, M. E., and Lee, A. S. Y. (2023). eIF3d controls the persistent integrated stress response. Mol. Cell (23), 3303–3313.e6. –3. doi:10.1016/j.molcel.2023.08.008
Müller, M. B. D., Kasturi, P., Jayaraj, G. G., and Hartl, F. U. (2023). Mechanisms of readthrough mitigation reveal principles of GCN1-mediated translational quality control. Cell (23), 3227–3244.e20. –1. doi:10.1016/j.cell.2023.05.035
Muniozguren, N. L., Zappa, F., and Acosta-Alvear, D. (2022). The integrated stress response induces a common cell-autonomous death receptor 5-dependent apoptosis switch. 498696. doi:10.1101/2022.07.04.498696
Muñoz, J. P., Ivanova, S., Sánchez-Wandelmer, J., Martínez-Cristóbal, P., Noguera, E., Sancho, A., et al. (2013). Mfn2 modulates the UPR and mitochondrial function via repression of PERK. EMBO J. 32, 2348–2361. doi:10.1038/emboj.2013.168
Nakamura, T., Furuhashi, M., Li, P., Cao, H., Tuncman, G., Sonenberg, N., et al. (2010). Double-stranded RNA-dependent protein kinase links pathogen sensing with stress and metabolic homeostasis. Cell 140, 338–348. doi:10.1016/j.cell.2010.01.001
Namer, L. S., Osman, F., Banai, Y., Masquida, B., Jung, R., and Kaempfer, R. (2017). An ancient pseudoknot in TNF-α pre-mRNA activates PKR, inducing eIF2α phosphorylation that potently enhances splicing. Cell Rep. 20, 188–200. doi:10.1016/j.celrep.2017.06.035
Nishio, N., Hasegawa, T., Tatsuno, I., Isaka, M., and Isobe, K.-I. (2017). Female GADD34 mice develop age-related inflammation and hepatocellular carcinoma. Geriatr. Gerontol. Int. 17, 2593–2601. doi:10.1111/ggi.13080
Nishio, N., and Isobe, K. (2015). GADD34-deficient mice develop obesity, nonalcoholic fatty liver disease, hepatic carcinoma and insulin resistance. Sci. Rep. 5, 13519. doi:10.1038/srep13519
Nishio, N., Ito, S., and Isobe, K. (2014). Loss of GADD34 induces early age-dependent deviation to the myeloid lineage. Immunol. Cell Biol. 92, 170–180. doi:10.1038/icb.2013.78
Novoa, I., Zeng, H., Harding, H. P., and Ron, D. (2001). Feedback inhibition of the unfolded protein response by GADD34-mediated dephosphorylation of eIF2alpha. J. Cell Biol. 153, 1011–1022. doi:10.1083/jcb.153.5.1011
Nüske, E., Marini, G., Richter, D., Leng, W., Bogdanova, A., Franzmann, T. M., et al. (2020). Filament formation by the translation factor eIF2B regulates protein synthesis in starved cells. Biol. Open 9, bio046391. doi:10.1242/bio.046391
Padyana, A. K., Qiu, H., Roll-Mecak, A., Hinnebusch, A. G., and Burley, S. K. (2005). Structural basis for autoinhibition and mutational activation of eukaryotic initiation factor 2alpha protein kinase GCN2. J. Biol. Chem. 280, 29289–29299. doi:10.1074/jbc.M504096200
Pakos-Zebrucka, K., Koryga, I., Mnich, K., Ljujic, M., Samali, A., and Gorman, A. M. (2016). The integrated stress response. EMBO Rep. 17, 1374–1395. doi:10.15252/embr.201642195
Park, Y., Reyna-Neyra, A., Philippe, L., and Thoreen, C. C. (2017). mTORC1 balances cellular amino acid supply with demand for protein synthesis through post-transcriptional control of ATF4. Cell Rep. 19, 1083–1090. doi:10.1016/j.celrep.2017.04.042
Pavitt, G. D., Ramaiah, K. V., Kimball, S. R., and Hinnebusch, A. G. (1998). eIF2 independently binds two distinct eIF2B subcomplexes that catalyze and regulate guanine-nucleotide exchange. Genes Dev. 12, 514–526. doi:10.1101/gad.12.4.514
Perea, V., Cole, C., Lebeau, J., Dolina, V., Baron, K. R., Madhavan, A., et al. (2023). PERK signaling promotes mitochondrial elongation by remodeling membrane phosphatidic acid. EMBO J. 42, e113908. doi:10.15252/embj.2023113908
Pereira, C. M., Sattlegger, E., Jiang, H.-Y., Longo, B. M., Jaqueta, C. B., Hinnebusch, A. G., et al. (2005). IMPACT, a protein preferentially expressed in the mouse brain, binds GCN1 and inhibits GCN2 activation. J. Biol. Chem. 280, 28316–28323. doi:10.1074/jbc.M408571200
Pérez-Garijo, A., Fuchs, Y., and Steller, H. (2013). Apoptotic cells can induce non-autonomous apoptosis through the TNF pathway. Elife 2, e01004. doi:10.7554/eLife.01004
Pochopien, A. A., Beckert, B., Kasvandik, S., Berninghausen, O., Beckmann, R., Tenson, T., et al. (2021). Structure of Gcn1 bound to stalled and colliding 80S ribosomes. Proc. Natl. Acad. Sci. 118, e2022756118. doi:10.1073/pnas.2022756118
Polyak, S. J., Tang, N., Wambach, M., Barber, G. N., and Katze, M. G. (1996). The P58 cellular inhibitor complexes with the interferon-induced, double-stranded RNA-dependent protein kinase, PKR, to regulate its autophosphorylation and activity. J. Biol. Chem. 271, 1702–1707. doi:10.1074/jbc.271.3.1702
Rafie-Kolpin, M., Chefalo, P. J., Hussain, Z., Hahn, J., Uma, S., Matts, R. L., et al. (2000). Two heme-binding domains of heme-regulated eukaryotic initiation factor-2alpha kinase. N terminus and kinase insertion. J. Biol. Chem. 275, 5171–5178. doi:10.1074/jbc.275.7.5171
Reverendo, M., Mendes, A., Argüello, R. J., Gatti, E., and Pierre, P. (2019). At the crossway of ER-stress and proinflammatory responses. FEBS J. 286, 297–310. doi:10.1111/febs.14391
Ricketts, M. D., Emptage, R. P., Blobel, G. A., and Marmorstein, R. (2022). The heme-regulated inhibitor kinase requires dimerization for heme-sensing activity. J. Biol. Chem. 298, 102451. doi:10.1016/j.jbc.2022.102451
Rowlands, A. G., Panniers, R., and Henshaw, E. C. (1988). The catalytic mechanism of guanine nucleotide exchange factor action and competitive inhibition by phosphorylated eukaryotic initiation factor 2. J. Biol. Chem. 263, 5526–5533. doi:10.1016/s0021-9258(18)60596-4
Rutkowski, D. T., Kang, S.-W., Goodman, A. G., Garrison, J. L., Taunton, J., Katze, M. G., et al. (2007). The role of p58IPK in protecting the stressed endoplasmic reticulum. Mol. Biol. Cell 18, 3681–3691. doi:10.1091/mbc.e07-03-0272
Salvagno, C., and Cubillos-Ruiz, J. R. (2019). “Chapter Three - the impact of endoplasmic reticulum stress responses in dendritic cell immunobiology,” in International review of cell and molecular Biology immunobiology of dendritic cells Part B. Editors C. Lhuillier, and L. Galluzzi (Academic Press), 153–176. doi:10.1016/bs.ircmb.2019.08.004
Sassano, M. L., van Vliet, A. R., Vervoort, E., Van Eygen, S., Van den Haute, C., Pavie, B., et al. (2023). PERK recruits E-Syt1 at ER-mitochondria contacts for mitochondrial lipid transport and respiration. J. Cell Biol. 222, e202206008. doi:10.1083/jcb.202206008
Schoof, M., Boone, M., Wang, L., Lawrence, R., Frost, A., and Walter, P. (2021). eIF2B conformation and assembly state regulate the integrated stress response. eLife 10, e65703. doi:10.7554/eLife.65703
Segev, Y., Barrera, I., Ounallah-Saad, H., Wibrand, K., Sporild, I., Livne, A., et al. (2015). PKR inhibition rescues memory deficit and ATF4 overexpression in ApoE ε4 human replacement mice. J. Neurosci. 35, 12986–12993. doi:10.1523/JNEUROSCI.5241-14.2015
Segev, Y., Michaelson, D. M., and Rosenblum, K. (2013). ApoE ε4 is associated with eIF2α phosphorylation and impaired learning in young mice. Neurobiol. Aging 34, 863–872. doi:10.1016/j.neurobiolaging.2012.06.020
Sekine, Y., Houston, R., Eckl, E.-M., Fessler, E., Narendra, D. P., Jae, L. T., et al. (2023). A mitochondrial iron-responsive pathway regulated by DELE1. Mol. Cell 83, 2059–2076.e6. doi:10.1016/j.molcel.2023.05.031
Settembre, C., Zoncu, R., Medina, D. L., Vetrini, F., Erdin, S., Erdin, S., et al. (2012). A lysosome-to-nucleus signalling mechanism senses and regulates the lysosome via mTOR and TFEB. EMBO J. 31, 1095–1108. doi:10.1038/emboj.2012.32
Shao, L.-W., Niu, R., and Liu, Y. (2016). Neuropeptide signals cell non-autonomous mitochondrial unfolded protein response. Cell Res. 26, 1182–1196. doi:10.1038/cr.2016.118
Sharma, V., Sood, R., Khlaifia, A., Eslamizade, M. J., Hung, T.-Y., Lou, D., et al. (2020). eIF2α controls memory consolidation via excitatory and somatostatin neurons. Nature 586, 412–416. doi:10.1038/s41586-020-2805-8
Shrestha, P., Ayata, P., Herrero-Vidal, P., Longo, F., Gastone, A., LeDoux, J. E., et al. (2020). Cell-type-specific drug-inducible protein synthesis inhibition demonstrates that memory consolidation requires rapid neuronal translation. Nat. Neurosci. 23, 281–292. doi:10.1038/s41593-019-0568-z
Singh, M., Fowlkes, V., Handy, I., Patel, C. V., and Patel, R. C. (2009). Essential role of PACT-mediated PKR activation in tunicamycin-induced apoptosis. J. Mol. Biol. 385, 457–468. doi:10.1016/j.jmb.2008.10.068
Sood, R., Porter, A. C., Olsen, D. A., Cavener, D. R., and Wek, R. C. (2000). A mammalian homologue of GCN2 protein kinase important for translational control by phosphorylation of eukaryotic initiation factor-2alpha. Genetics 154, 787–801. doi:10.1093/genetics/154.2.787
Sprenkle, N. T., Lahiri, A., Simpkins, J. W., and Meares, G. P. (2019). Endoplasmic reticulum stress is transmissible in vitro between cells of the central nervous system. J. Neurochem. 148, 516–530. doi:10.1111/jnc.14642
Stonyte, V., Mastrangelopoulou, M., Timmer, R., Lindbergsengen, L., Vietri, M., Campsteijn, C., et al. (2023). The GCN2/eIF2αK stress kinase regulates PP1 to ensure mitotic fidelity. EMBO Rep. 24, e56100. doi:10.15252/embr.202256100
Sundaram, A., Appathurai, S., Plumb, R., and Mariappan, M. (2018). Dynamic changes in complexes of IRE1α, PERK, and ATF6α during endoplasmic reticulum stress. Mol. Biol. Cell 29, 1376–1388. doi:10.1091/mbc.E17-10-0594
Tanaka, T., Tsujimura, T., Takeda, K., Sugihara, A., Maekawa, A., Terada, N., et al. (1998). Targeted disruption of ATF4 discloses its essential role in the formation of eye lens fibres. Genes cells. 3, 801–810. doi:10.1046/j.1365-2443.1998.00230.x
Taniuchi, S., Miyake, M., Tsugawa, K., Oyadomari, M., and Oyadomari, S. (2016). Integrated stress response of vertebrates is regulated by four eIF2α kinases. Sci. Rep. 6, 32886. doi:10.1038/srep32886
Tao, J., Petrova, K., Ron, D., and Sha, B. (2010). Crystal structure of P58(IPK) TPR fragment reveals the mechanism for its molecular chaperone activity in UPR. J. Mol. Biol. 397, 1307–1315. doi:10.1016/j.jmb.2010.02.028
Taylor, R. C., and Dillin, A. (2013). XBP-1 is a cell-nonautonomous regulator of stress resistance and longevity. Cell 153, 1435–1447. doi:10.1016/j.cell.2013.05.042
Torrence, M. E., MacArthur, M. R., Hosios, A. M., Valvezan, A. J., Asara, J. M., Mitchell, J. R., et al. (2021). The mTORC1-mediated activation of ATF4 promotes protein and glutathione synthesis downstream of growth signals. eLife 10, e63326. doi:10.7554/eLife.63326
van Huizen, R., Martindale, J. L., Gorospe, M., and Holbrook, N. J. (2003). P58IPK, a novel endoplasmic reticulum stress-inducible protein and potential negative regulator of eIF2alpha signaling. J. Biol. Chem. 278, 15558–15564. doi:10.1074/jbc.M212074200
van Vliet, A. R., Giordano, F., Gerlo, S., Segura, I., Van Eygen, S., Molenberghs, G., et al. (2017). The ER stress sensor PERK coordinates ER-plasma membrane contact site formation through interaction with filamin-A and F-actin remodeling. Mol. Cell 65, 885–899. doi:10.1016/j.molcel.2017.01.020
Vasudevan, D., Neuman, S. D., Yang, A., Lough, L., Brown, B., Bashirullah, A., et al. (2020). Translational induction of ATF4 during integrated stress response requires noncanonical initiation factors eIF2D and DENR. Nat. Commun. 11, 4677. doi:10.1038/s41467-020-18453-1
Verfaillie, T., Rubio, N., Garg, A. D., Bultynck, G., Rizzuto, R., Decuypere, J.-P., et al. (2012). PERK is required at the ER-mitochondrial contact sites to convey apoptosis after ROS-based ER stress. Cell Death Differ. 19, 1880–1891. doi:10.1038/cdd.2012.74
Volmer, R., van der Ploeg, K., and Ron, D. (2013). Membrane lipid saturation activates endoplasmic reticulum unfolded protein response transducers through their transmembrane domains. Proc. Natl. Acad. Sci. U.S.A. 110, 4628–4633. doi:10.1073/pnas.1217611110
Wang, P., Li, J., Tao, J., and Sha, B. (2018). The luminal domain of the ER stress sensor protein PERK binds misfolded proteins and thereby triggers PERK oligomerization. J. Biol. Chem. 293, 4110–4121. doi:10.1074/jbc.RA117.001294
Wang, X., Paulin, F. E. M., Campbell, L. E., Gomez, E., O’Brien, K., Morrice, N., et al. (2001). Eukaryotic initiation factor 2B: identification of multiple phosphorylation sites in the ε-subunit and their functions in vivo. EMBO J. 20, 4349–4359. doi:10.1093/emboj/20.16.4349
Wang, Y., Shen, J., Arenzana, N., Tirasophon, W., Kaufman, R. J., and Prywes, R. (2000). Activation of ATF6 and an ATF6 DNA binding site by the endoplasmic reticulum stress response. J. Biol. Chem. 275, 27013–27020. doi:10.1074/jbc.M003322200
Wei, J., Sheng, X., Feng, D., McGrath, B., and Cavener, D. R. (2008). PERK is essential for neonatal skeletal development to regulate osteoblast proliferation and differentiation. J. Cell Physiol. 217, 693–707. doi:10.1002/jcp.21543
Wek, R. C., Jackson, B. M., and Hinnebusch, A. G. (1989). Juxtaposition of domains homologous to protein kinases and histidyl-tRNA synthetases in GCN2 protein suggests a mechanism for coupling GCN4 expression to amino acid availability. Proc. Natl. Acad. Sci. U. S. A. 86, 4579–4583. doi:10.1073/pnas.86.12.4579
Welsh, G. I., Miller, C. M., Loughlin, A. J., Price, N. T., and Proud, C. G. (1998). Regulation of eukaryotic initiation factor eIF2B: glycogen synthase kinase-3 phosphorylates a conserved serine which undergoes dephosphorylation in response to insulin. FEBS Lett. 421, 125–130. doi:10.1016/s0014-5793(97)01548-2
Williams, K. W., Liu, T., Kong, X., Fukuda, M., Deng, Y., Berglund, E. D., et al. (2014). Xbp1s in Pomc neurons connects ER stress with energy balance and glucose homeostasis. Cell Metab. 20, 471–482. doi:10.1016/j.cmet.2014.06.002
Wisse, L. E., Ter Braak, T. J., van de Beek, M.-C., van Berkel, C. G. M., Wortel, J., Heine, V. M., et al. (2018). Adult mouse eIF2Bε Arg191His astrocytes display a normal integrated stress response in vitro. Sci. Rep. 8, 3773. doi:10.1038/s41598-018-21885-x
Wolzak, K., Nölle, A., Farina, M., Abbink, T. E., van der Knaap, M. S., Verhage, M., et al. (2022). Neuron-specific translational control shift ensures proteostatic resilience during ER stress. EMBO J. 41, e110501. doi:10.15252/embj.2021110501
Wong, Y. L., LeBon, L., Basso, A. M., Kohlhaas, K. L., Nikkel, A. L., Robb, H. M., et al. (2019). eIF2B activator prevents neurological defects caused by a chronic integrated stress response. Elife 8, e42940. doi:10.7554/eLife.42940
Wu, C. C.-C., Peterson, A., Zinshteyn, B., Regot, S., and Green, R. (2020). Ribosome collisions trigger general stress responses to regulate cell fate. Cell 182, 404–416. doi:10.1016/j.cell.2020.06.006
Wu, Z., Mei, F., Gan, Y., Liu, A., Hu, J., Jin, Y., et al. (2023). FAM69C functions as a kinase for eIF2α and promotes stress granule assembly. EMBO Rep. 24, e55641. doi:10.15252/embr.202255641
Yamani, L., Latreille, M., and Larose, L. (2014). Interaction of Nck1 and PERK phosphorylated at Y⁵⁶¹ negatively modulates PERK activity and PERK regulation of pancreatic β-cell proinsulin content. MBoC 25, 702–711. doi:10.1091/mbc.e13-09-0511
Yang, J., Baron, K. R., Pride, D. E., Schneemann, A., Guo, X., Chen, W., et al. (2023). DELE1 oligomerization promotes integrated stress response activation. Nat. Struct. Mol. Biol. 30, 1295–1302. doi:10.1038/s41594-023-01061-0
Yang, X., Matsuda, K., Bialek, P., Jacquot, S., Masuoka, H. C., Schinke, T., et al. (2004). ATF4 is a substrate of RSK2 and an essential regulator of osteoblast biology; implication for Coffin-Lowry Syndrome. Cell 117, 387–398. doi:10.1016/s0092-8674(04)00344-7
Yang, Y. L., Reis, L. F., Pavlovic, J., Aguzzi, A., Schäfer, R., Kumar, A., et al. (1995). Deficient signaling in mice devoid of double-stranded RNA-dependent protein kinase. EMBO J. 14, 6095–6106. doi:10.1002/j.1460-2075.1995.tb00300.x
Yoshizawa, T., Hinoi, E., Jung, D. Y., Kajimura, D., Ferron, M., Seo, J., et al. (2009). The transcription factor ATF4 regulates glucose metabolism in mice through its expression in osteoblasts. J. Clin. Invest. 119, 2807–2817. doi:10.1172/JCI39366
You, K., Wang, L., Chou, C.-H., Liu, K., Nakata, T., Jaiswal, A., et al. (2021). QRICH1 dictates the outcome of ER stress through transcriptional control of proteostasis. Science 371, eabb6896. doi:10.1126/science.abb6896
Youn, J.-Y., Dyakov, B. J. A., Zhang, J., Knight, J. D. R., Vernon, R. M., Forman-Kay, J. D., et al. (2019). Properties of stress granule and P-body proteomes. Mol. Cell 76, 286–294. doi:10.1016/j.molcel.2019.09.014
Young, D. J., Meydan, S., and Guydosh, N. R. (2021). 40S ribosome profiling reveals distinct roles for Tma20/Tma22 (MCT-1/DENR) and Tma64 (eIF2D) in 40S subunit recycling. Nat. Commun. 12, 2976. doi:10.1038/s41467-021-23223-8
Young, S. K., and Wek, R. C. (2016). Upstream open reading frames differentially regulate gene-specific translation in the integrated stress response. J. Biol. Chem. 291, 16927–16935. doi:10.1074/jbc.R116.733899
Yu, X., Xu, X., Dong, W., Yang, C., Luo, Y., He, Y., et al. (2022). DDIT3/CHOP mediates the inhibitory effect of ER stress on chondrocyte differentiation by AMPKα-SIRT1 pathway. Biochimica Biophysica Acta (BBA) - Mol. Cell Res. 1869, 119265. doi:10.1016/j.bbamcr.2022.119265
Yuniati, L., van der Meer, L. T., Tijchon, E., van Ingen Schenau, D., van Emst, L., Levers, M., et al. (2016). Tumor suppressor BTG1 promotes PRMT1-mediated ATF4 function in response to cellular stress. Oncotarget 7, 3128–3143. doi:10.18632/oncotarget.6519
Zamanian-Daryoush, M., Mogensen, T. H., DiDonato, J. A., and Williams, B. R. (2000). NF-kappaB activation by double-stranded-RNA-activated protein kinase (PKR) is mediated through NF-kappaB-inducing kinase and IkappaB kinase. Mol. Cell Biol. 20, 1278–1290. doi:10.1128/MCB.20.4.1278-1290.2000
Zappa, F., Muniozguren, N. L., Wilson, M. Z., Costello, M. S., Ponce-Rojas, J. C., and Acosta-Alvear, D. (2022). Signaling by the integrated stress response kinase PKR is fine-tuned by dynamic clustering. J. Cell Biol. 221, e202111100. doi:10.1083/jcb.202111100
Zappa, F., Wilson, C., Di Tullio, G., Santoro, M., Pucci, P., Monti, M., et al. (2019). The TRAPP complex mediates secretion arrest induced by stress granule assembly. EMBO J. 38, e101704. doi:10.15252/embj.2019101704
Zhang, D., Liu, Y., Zhu, Y., Zhang, Q., Guan, H., Liu, S., et al. (2022). A non-canonical cGAS–STING–PERK pathway facilitates the translational program critical for senescence and organ fibrosis. Nat. Cell Biol. 24, 766–782. doi:10.1038/s41556-022-00894-z
Zhang, L., Ebenezer, P. J., Dasuri, K., Bruce-Keller, A. J., Fernandez-Kim, S. O., Liu, Y., et al. (2010). Activation of PERK kinase in neural cells by proteasome inhibitor treatment. J. Neurochem. 112, 238–245. doi:10.1111/j.1471-4159.2009.06448.x
Zhang, P., McGrath, B., Li, S., Frank, A., Zambito, F., Reinert, J., et al. (2002b). The PERK eukaryotic initiation factor 2 alpha kinase is required for the development of the skeletal system, postnatal growth, and the function and viability of the pancreas. Mol. Cell Biol. 22, 3864–3874. doi:10.1128/MCB.22.11.3864-3874.2002
Zhang, P., McGrath, B. C., Reinert, J., Olsen, D. S., Lei, L., Gill, S., et al. (2002a). The GCN2 eIF2alpha kinase is required for adaptation to amino acid deprivation in mice. Mol. Cell. Biol. 22, 6681–6688. doi:10.1128/mcb.22.19.6681-6688.2002
Zhang, Q., Wang, Z., Zhang, W., Wen, Q., Li, X., Zhou, J., et al. (2021). The memory of neuronal mitochondrial stress is inherited transgenerationally via elevated mitochondrial DNA levels. Nat. Cell Biol. 23, 870–880. doi:10.1038/s41556-021-00724-8
Zhang, Q., Wu, X., Chen, P., Liu, L., Xin, N., Tian, Y., et al. (2018). The mitochondrial unfolded protein response is mediated cell-non-autonomously by retromer-dependent wnt signaling. Cell 174, 870–883. doi:10.1016/j.cell.2018.06.029
Zhao, C., Guo, H., Hou, Y., Lei, T., Wei, D., and Zhao, Y. (2023). Multiple roles of the stress sensor GCN2 in immune cells. Int. J. Mol. Sci. 24, 4285. doi:10.3390/ijms24054285
Zhou, J., Wan, J., Shu, X. E., Mao, Y., Liu, X.-M., Yuan, X., et al. (2018). N6-Methyladenosine guides mRNA alternative translation during integrated stress response. Mol. Cell. 69, 636–647. doi:10.1016/j.molcel.2018.01.019
Zhou, L., Li, P., Chen, N., Dai, L.-F., Gao, K., Liu, Y.-N., et al. (2019). Modeling vanishing white matter disease with patient-derived induced pluripotent stem cells reveals astrocytic dysfunction. CNS Neurosci. Ther. 25, 759–771. doi:10.1111/cns.13107
Zhou, W., Brush, M. H., Choy, M. S., and Shenolikar, S. (2011). Association with endoplasmic reticulum promotes proteasomal degradation of GADD34 protein. J. Biol. Chem. 286, 21687–21696. doi:10.1074/jbc.M110.212787
Zhu, P. J., Huang, W., Kalikulov, D., Yoo, J. W., Placzek, A. N., Stoica, L., et al. (2011). Suppression of PKR promotes network excitability and enhanced cognition by interferon-γ-mediated disinhibition. Cell 147, 1384–1396. doi:10.1016/j.cell.2011.11.029
Zinszner, H., Kuroda, M., Wang, X., Batchvarova, N., Lightfoot, R. T., Remotti, H., et al. (1998). CHOP is implicated in programmed cell death in response to impaired function of the endoplasmic reticulum. Genes Dev. 12, 982–995. doi:10.1101/gad.12.7.982
Keywords: integrated stress response, signaling network, homeostasis, signal heterogeneity, subcellular compartmentalization, sensor kinase, signal crosstalk
Citation: Boone M and Zappa F (2023) Signaling plasticity in the integrated stress response. Front. Cell Dev. Biol. 11:1271141. doi: 10.3389/fcell.2023.1271141
Received: 01 August 2023; Accepted: 29 November 2023;
Published: 07 December 2023.
Edited by:
Paolo Grumati, Telethon Institute of Genetics and Medicine (TIGEM), ItalyReviewed by:
Deepika Vasudevan, University of Pittsburgh, United StatesCopyright © 2023 Boone and Zappa. This is an open-access article distributed under the terms of the Creative Commons Attribution License (CC BY). The use, distribution or reproduction in other forums is permitted, provided the original author(s) and the copyright owner(s) are credited and that the original publication in this journal is cited, in accordance with accepted academic practice. No use, distribution or reproduction is permitted which does not comply with these terms.
*Correspondence: Francesca Zappa, ZnphcHBhQGFsdG9zbGFicy5jb20=; Morgane Boone, bWJvb25lQGFsdG9zbGFicy5jb20=
†These authors have contributed equally to this work